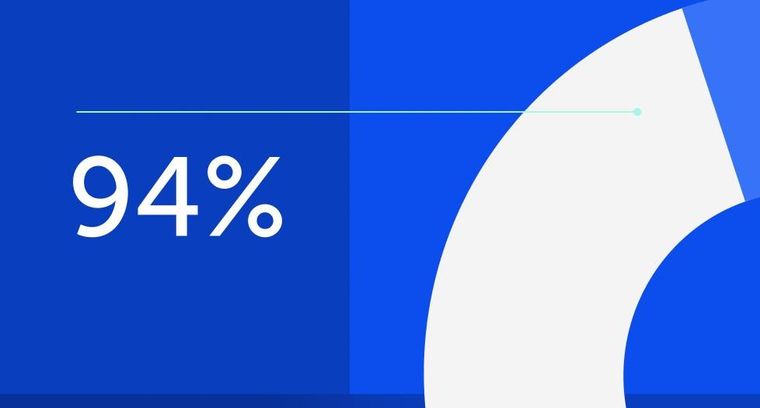
94% of researchers rate our articles as excellent or good
Learn more about the work of our research integrity team to safeguard the quality of each article we publish.
Find out more
MINI REVIEW article
Front. Cell Dev. Biol., 27 May 2022
Sec. Cell Adhesion and Migration
Volume 10 - 2022 | https://doi.org/10.3389/fcell.2022.910736
This article is part of the Research TopicIntegrin Adhesion Receptors in Health and DiseaseView all 16 articles
Dictyostelium amoebae adhere to extracellular material using similar mechanisms to metazoan cells. Notably, the cellular anchorage loci in Amoebozoa and Metazoa are both arranged in the form of discrete spots and incorporate a similar repertoire of intracellular proteins assembled into multicomponent complexes located on the inner side of the plasma membrane. Surprisingly, however, Dictyostelium lacks integrins, the canonical transmembrane heterodimeric receptors that dominantly mediate adhesion of cells to the extracellular matrix in multicellular animals. In this review article, we summarize the current knowledge about the cell-substratum adhesion in Dictyostelium, present an inventory of the involved proteins, and draw parallels with the situation in animal cells. The emerging picture indicates that, while retaining the basic molecular architecture common to their animal relatives, the adhesion complexes in free-living amoeboid cells have evolved to enable less specific interactions with diverse materials encountered in their natural habitat in the deciduous forest soil. Dissection of molecular mechanisms that underlay short lifetime of the cell-substratum attachments and high turnover rate of the adhesion complexes in Dictyostelium should provide insight into a similarly modified adhesion phenotype that accompanies the mesenchymal-amoeboid transition in tumor metastasis.
The mid-1980s, when integrins were being unraveled as the receptors that mediate cellular adhesion to extracellular matrix in mammals and other animals (Hynes, 1987, 2004), also witnessed prominent activity in the characterization of the adhesion of unicellular amoeba Dictyostelium discoideum to external surfaces (Gingell and Vince, 1982; Owens et al., 1987, 1988). Dictyostelium as model organism was on the forefront of the homotypic cell-cell adhesion research (Beug et al., 1973), but its use as a model for the cell-substratum adhesion was hampered by the lack of a well-defined extracellular matrix. The early work was therefore focused on the influence of the physico-chemical characteristics of various glass coatings on cell adhesion. Briefly, it turned out that Dictyostelium cells adhered strongly to hydrophobic and positively charged surfaces and weaker to hydrophilic surfaces (Gingell and Vince, 1982; Owens et al., 1988). Of special importance was the introduction of antiadhesive coatings based on entropic repulsion of long polyethylene glycol chains, a concept that has been frequently used ever since to abrogate adhesion of cells to underlying surfaces (Owens et al., 1987; Amiji and Park, 1992). This early body of work by Gingell and co-workers is also significant because of the extensive use of reflection interference contrast microscopy (RICM) and the introduction of internal reflection aqueous fluorescence microscopy for quantitative assessment of adhesion zones (Gingell and Vince, 1982; Todd et al., 1988).
In the 1990s, it was discovered that aggregation-competent cells can migrate with surprisingly little interaction with the substratum, forming discrete attachment spots with a footprint of less than 10% of the cell surface (Wessels et al., 1994; Weber et al., 1995). These attachment zones visible in RICM must, however, be distinguished from much smaller punctuate structures at the bottom cell membrane such as the actin-rich podosomes (Fukui and Inoué, 1997) and the ventral adhesion foci (Patel et al., 2008) (Table 1). A major step forward in the molecular characterization of the adhesion apparatus arrived with the cloning of a Dictyostelium talin homologue, talin A (Kreitmeier et al., 1995), which proved to be vital for cell-substratum and EDTA-sensitive cell-cell adhesion (Niewöhner et al., 1997). It was soon discovered that talin A and other proteins such as myosin VII and paxillin B localized to the ventral adhesion foci, the transient punctuate structures at the cell membrane closely apposed to the underlying surface (Kreitmeier et al., 1995; Bukharova et al., 2005; Patel et al., 2008). Their composition, dot-like appearance and immobility relative to the substratum resembled the focal adhesions well-known from fibroblasts and other mammalian cells in culture, which are, however, invariably coupled to transmembrane heterodimeric receptors integrins (Legerstee and Houtsmuller, 2021). Although putative adhesion receptors in Dictyostelium were subsequently identified, only SibA showed a limited relatedness to integrin beta subunit, primarily at its C-terminus (Cornillon et al., 2006).
TABLE 1. Reported observations and composition of punctuate structures at the cell-substratum interface in Dictyostelium cells in chronological order. Abbreviations: IF—immunofluorescence; DIC—differential interference contrast; TEM—transmission electron microscopy; GFP—green fluorescent protein; TIRF—total internal reflection of fluorescence; RICM—reflection interference contrast microscopy; TalA—talin A; TalB—talin B; MyoIB—myosin IB; ABP120—actin binding protein; Arp3—actin-related protein three; PaxB—paxillin B; RasGEF—Ras guanine nucleotide-exchange factor; RapGAP1—Rap GTPase-activating protein one; VinA—vinculin A; CtxI—cortexillin I.
A hallmark of the metastatic spread of tumor cells, the mesenchymal-amoeboid transition, is accompanied by a profound loss of cell adhesion to the extracellular matrix and the disappearance of canonical focal adhesions (Liu et al., 2015). Amoeboid mode of migration in Dictyostelium amoeba presents a good model for the migration of metastatic cells in humans, especially since the two organisms share the basic biophysical and regulatory mechanisms responsible for the actin-driven locomotion (Artemenko et al., 2014; Filić et al., 2021). It may seem surprising that much more is known about the structure, assembly, regulation and function of the focal adhesion complexes in mammals than about their apparently much simpler counterparts in Dictyostelium. However, the Dictyostelium punctuate adhesion contacts are small, fragile and short-lived, and as such still elude more comprehensive characterization. In the following, we provide an inventory of proteins involved in the cell-substratum adhesion in Dictyostelium (Table 2).
TABLE 2. Knock-out phenotypes and relevant interactors of core proteins involved in the regulation of the cell-substratum adhesion in Dictyostelium. The list does not include a number of proteins that were found to influence the adhesion but were not characterized in detail. The phenotype of knock-out strains was described only in the context of the cell-substratum adhesion. The interactions were mostly identified using pull-down and co-immunoprecipitation assays. Additionaly, some binding partners were detected by GDI (guanine nucleotide dissociation inhibitor) and GAP (GTPase-activating protein) assays, RapA activation assay and yeast-two-hybrid assay. Abbreviations: HL5—HL5 nutrient medium; PB—phosphate buffer; MFA—microfluidic assay; CtxI—cortexillin I; TalA—talin A; TalB—talin B; MyoVII—myosin VII; PldB—phospholipase D. An asterisk (*) indicates conflicting reports.
After the identification of a crucial role played by a Dictyostelium talin orthologue in cell-substratum adhesion (Niewöhner et al., 1997), it appeared plausible that this process relied on a variant of integrin-mediated adhesive complexes (IACs), similar to those found in Metazoa (Kang et al., 2021). A quest to identify candidate adhesion receptors began by a screen of randomly generated mutants for defects in phagocytosis (Cornillon et al., 2000). The screen exposed Phg1A, a protein belonging to the transmembrane nine family (TM9) with the typical large extracellular domain and nine hydrophobic C-terminal transmembrane domains (Froquet et al., 2008), soon followed by the discovery of related TM9 proteins Phg1B and Phg1C (Benghezal et al., 2003). Characterization of single and double knock-outs of Phg1A and Phg1B revealed a severe defect in adhesion of phg1A- cells to hydrophilic particles and suggested a synergistic effects of the two proteins in controlling the adhesion (Cornillon et al., 2000; Benghezal et al., 2003). Concomitantly to TM9 proteins, yet another protein with nine putative transmembrane domains, SadA, was shown to be important for adhesion in Dictyostelium. sadA- cells were unable to attach to plastic dishes and to spread, and their phagocytosis was strongly impaired (Fey et al., 2002; Froquet et al., 2012). Since SadA contains three conserved extracellular EGF-like repeats that are also present in integrins and tenascins, it was suggested that it represents a genuine adhesion receptor (Fey et al., 2002). It was later shown that the cytoplasmic tail of SadA interacts with the talin A/myosin VII complex (Tuxworth et al., 2005; Cornillon et al., 2006), and with cortexillin I (Kowal and Chisholm, 2011), indicating a link to the actin cytoskeleton.
The most likely candidate for the main adhesion receptor in Dictyostelium, however, was identified in another random mutagenesis screen and named SibA (similar to integrin beta A) (Cornillon et al., 2006). As indicated by its name, SibA is a type I transmembrane protein with features similar to metazoan integrin β-chains, e.g. an extracellular Von Willebrandt A domain and a single glycine-rich transmembrane domain, but also with significant structural differences in comparison to integrin β (Cornillon et al., 2006). There are four close homologs of SibA (SibB to SibE) in Dictyostelium and all of them bind talin A via their cytosolic domain (Cornillon et al., 2006). Only SibA and SibC are expressed abundantly in vegetative cells and their individual genetic inactivation caused a partial loss of adhesion to various substrata and particles, but generation of a double sibA-/sibC- knockout strain failed, suggesting possible lethality (Cornillon et al., 2008). Taken together, the present state of knowledge about the transmembrane proteins involved in the regulation of adhesion to external surfaces suggests that Sib proteins are the primary receptors, whereas SadA and Phg1 proteins play an auxiliary and regulatory role. The expression level, stability and targeting of SibA to the cell surface are influenced by Phg1A and SadA (Froquet et al., 2012). Compared to wild-type, SibA, SadA and Phg1 deficient cells exhibit comparable defects in phagocytosis and the adhesion to underlying surfaces (Fey et al., 2002; Benghezal et al., 2003; Froquet et al., 2012; Tarantola et al., 2014).
The phosphorylation of functional motifs in the cytoplasmic integrin tails leads to conformational changes that enable the recruitment of downstream proteins such as 14-3-3, talin, and kindlin (Gahmberg and Grönholm, 2022). Most integrin β-tails contain conserved NPXY motifs that belong to a recognition sequence for phosphotyrosine-binding (PTB) domains (Calderwood et al., 2003). Besides PTBs, Src homology 2 (SH2) domains also bind phosphorylated tyrosine and are important in integrin signaling (Gahmberg and Grönholm, 2022). Instead by canonical tyrosine kinases, tyrosine phosphorylation in Dictyostelium is mediated by tyrosine kinase-like (TLK) proteins (Goldberg et al., 2006). While some TLKs are known to phosphorylate tyrosines exclusively, e.g. ZakA and Dpyk2-4, the others are dual-specificity kinases, e.g. SplA and Shk1 (Goldberg et al., 2006). The prime adhesion receptor in Dictyostelium, SibA, contains two NPXY motifs (Cornillon et al., 2006). It was shown that the membrane-proximal NPXY motif is essential for binding of SibA to talin A similar to situation in metazoa, thus suggesting that SibA might be regulated in a manner analogous to integrins (Cornillon et al., 2006; Anthis et al., 2009).
FERM (the four-point-one, ezrin, radixin and moesin) domain proteins encompass, together with paxillin and vinculin, the core members of IAC in metazoans (Calderwood et al., 2013), and seven have been identified in Dictyostelium: talin A and B, myosin VII, myosin G, and FrmA-C (Patel et al., 2008; Breshears et al., 2010). The importance for the cell-substratum adhesion of the first characterized member, talin A, lived up to expectations: talA- cells showed varying degrees of weakened adhesion to multiple substrata (Niewöhner et al., 1997; Simson et al., 1998; Gebbie et al., 2004; Tarantola et al., 2014), and defective uptake of various particles (Niewöhner et al., 1997; Gebbie et al., 2004). Talin A is the first protein to show up in the nascent ventral adhesion foci and at the distal ends of attached filopodia (Kreitmeier et al., 1995; Patel et al., 2008), but is also present at the trailing regions of locomoting cells (Hibi et al., 2004; Tuxworth et al., 2005; Tsujioka et al., 2012). Cells lacking another talin paralog in Dictyostelium, talin B, show only slightly impaired adhesion to the substratum, but when both talins are inactivated, the double KO cells are unable to attach at all when cultivated in the HL5 nutrient medium (Tsujioka et al., 2008; Plak et al., 2016). Paxillin B is a close homolog of mammalian paxillin, contains four highly conserved LIM domains and four paxillin LD domains, and is recruited to the tips of filopodia and the ventral adhesion foci sequentially after talin A (Bukharova et al., 2005; Patel et al., 2008; Nagasaki et al., 2009). Cells lacking paxillin B are defective in adhesion to substrata, but, interestingly, its overexpression was also reported to impair adhesion (Bukharova et al., 2005; Duran et al., 2009; Nagasaki et al., 2009). A class VII myosin is also enriched in the ventral adhesion foci and the filopodia tips (Tuxworth et al., 2001; Petersen et al., 2016), whereas the myoVII- cells show reduced attachment areas and diminished binding to particles (Tuxworth et al., 2001; Gebbie et al., 2004). Dictyostelium vinculins A and B possess regions with binding sites for α-actinin, talin, paxillin and actin, similar to human vinculin (Nagasaki et al., 2009; Huber and O’Day, 2012). Vinculin A appears to localize to the ventral adhesion foci, but its importance for the cell-substratum adhesion in general has not been investigated, apart from its requirement for cytokinesis in cells devoid of a functional myosin II (Nagasaki et al., 2009).
Similar localizations and mutant phenotypes indicated that talin A, paxillin B and myosin VII belong to the same complex, probably related to metazoan IACs. Indeed, it was shown that talin A is stabilized against degradation via its interaction with the myosin VII tail (Gebbie et al., 2004; Tuxworth et al., 2005; Galdeen et al., 2007). Although the two proteins do not depend on each other for localization (Tuxworth et al., 2005), formation of the complex prolongs the residence of myosin VII on the plasma membrane (Galdeen et al., 2007). FrmA is required for the proper cell-substratum adhesion by promoting the turnover of the ventral adhesion structures (Patel et al., 2008). In frmA− cells, the ventral adhesion foci containing paxillin B localize aberrantly around the circumference of the cell-substratum contact area and the persistence of these foci increases greatly, which is probably responsible for an increased adhesion of the mutant cells to the substratum (Patel et al., 2008). On the other hand, frmB− cells have a significantly reduced adhesion (Kim et al., 2017). Multiple evidence about functional analogies and interactions between the FERM and the transmembrane proteins strengthens the notion that they assemble into transient structures of the IAC type. For instance, the talin A/myosin VII complex interacts with the conserved cytosolic domain of Sib family proteins (Tuxworth et al., 2005; Cornillon et al., 2006). Also, adhesion defects of talA- and myoVII- cells are more pronounced on hydrophilic substrata, similar to those of phg1- and phg2- cells, suggesting an involvement in the same process (Gebbie et al., 2004). Small GTPase Rap1 is considered to be one of the key regulators of cell adhesion in Metazoa via its direct and indirect interactions with talin (Calderwood et al., 2013; Zhang et al., 2014; Zhu et al., 2017). In Dictyostelium too, RapA interacts directly with the RA domain of talin B, and regulates talin B signaling by local allosteric activation rather than by its recruitment (Plak et al., 2016). Since the deletion of RapA is likely lethal (Kang et al., 2002; Jeon et al., 2007b), data about the role of RapA in cell adhesion are based on overexpressor strains and genetic deletion of its regulators. Overexpression of active RapA in wild-type cells strongly increases adhesion (Rebstein et al., 1993; Jeon et al., 2007b, 2021), in single talA- or talB- the effect is modest, while in double mutants there is no effect, suggesting that RapA regulates cell-substratum adhesion also via an indirect activation of Talin A (Plak et al., 2016), similar to the activation of Talin1 by RIAM proteins in mammalian cells (Lagarrigue et al., 2016). Consistent with these results, cells lacking the RapGEF GbpD are more loosely attached to the substratum compared to wild-type cells, while activation of RapA by GbpD leads to a stronger attachment (Bosgraaf et al., 2005; Kortholt et al., 2006). Further corroboration of a positive role played by RapA in promoting cell-substratum adhesion comes from the studies of RapGAP proteins. So, the lack of RapGAP1, as well as of RapGAPB, leads to an increase in cell attachment, whereas RapGAP1-overexpressing cells are weakly attached to the substratum (Jeon et al., 2007a; Parkinson et al., 2009).
Attachment of cells to external surfaces, especially during locomotion, depends also on other processes within the actin cytoskeleton in addition to adhesion. A classic example is the myosin II-driven contractility that supports detachment of the rear end of migrating cells (Jay et al., 1995). It has thus been proposed that RapA, in addition to its talin-mediated role, negatively controls myosin II assembly through the activation of the serine/threonine kinase Phg2 specifically at the leading edges of migrating and dividing cells (Kortholt et al., 2006; Jeon et al., 2007a). Indeed, phg2- cells are strongly impaired in cell-substratum adhesion and form exceedingly large assemblies of F-actin at the ventral cell surface, suggesting an additional actin depolymerization activity of Phg2 (Gebbie et al., 2004), possibly related to the established role of mammalian serine/threonine kinases in the regulation of integrin-mediated adhesion (Bachmann et al., 2019). Another evidence that the spatial balance in actin polymerization influences cell adhesion comes from the cells lacking a functional SCAR/WAVE complex, which show reduced cell–substratum interactions in migration, although they still possess actin pseudopods (Veltman et al., 2012). This finding thus indicates a specific role of SCAR/WAVE in regulating the strength of the cellular traction stresses (Bastounis et al., 2011). Cells lacking a component of the SCAR/WAVE complex NapA have an even more pronounced adhesion defect, suggesting additional involvement of NapA in a SCAR/WAVE-independent pathway (Ibarra et al., 2006).
A number of other proteins were connected to the regulation of the cell-substratum adhesion in Dictyostelium, but the mechanisms of their action have not been clarified. For example, RapC is a close homolog of RapA with antagonistic functions in cell adhesion and migration, since rapC- cells show an increased substratum adhesion (Park et al., 2018a; Kim et al., 2021). Interestingly, mammalian Rap2 was found to promote integrin-dependent adhesion similar to Rap1 (McLeod et al., 2004). Increased cell-substratum adhesion has also been detected in Dictyostelium cells lacking AmpA and Sma (Kelsey and Blumberg, 2013), SepA (Müller-Taubenberger et al., 2009), coronin 7 (Shina et al., 2010b), dynamin B (Rai et al., 2011), copine A (Buccilli et al., 2019), KrsB (Artemenko et al., 2012), SpdA (Dias et al., 2016), LrrkA (Bodinier et al., 2021), and in mutants identified in a screen for increased cell attachment, e.g. PTEN, HtmA, AraA, DspA, and AbnC (Lampert et al., 2017). In mammals, coronin 1 is important for integrin β2 translocation to the platelet surface (Riley et al., 2020), dynamin 2 was shown to control Rap1 activation via FAK/Pyk2 and RapGEF leading to integrin clustering in T lymphocytes (Eppler et al., 2017), whereas PTEN reduces tyrosine phosphorylation by FAK and thereby negatively regulates the formation of focal adhesions and spreading in fibroblasts (Tamura et al., 1998). Conversely, diminished adhesion of Dictyostelium cells has been reported after the knock-out of genes coding for Gp130 (Chia et al., 2005), Ate1 (Batsios et al., 2019), Cbp7 (Park et al., 2018b), ForH (Schirenbeck et al., 2005), Ino1 (Frej et al., 2016), LrrA (Liu et al., 2005), and SecG (Shina et al., 2010a). Cytohesin 1, a mammalian homolog of SecG, was shown to bind β2 integrin chain in T lymphocytes (Kolanus et al., 1996). Mutant Dictyostelium cells devoid of IBARa have been described as lacking the dynamic spreading behavior characteristic for wild-type cells (Linkner et al., 2014).
Among over 200 human adhesome proteins, fewer than 50 are found in Dictyostelium (Zaidel-Bar, 2009). Although the adhesive properties of Dictyostelium cells are remarkably similar to those of animals, most Dictyostelium adhesion molecules have little sequence similarity to animal proteins (Abedin and King, 2010). However, over the past decade, integrins and other components of the IAC (or adhesome) were identified outside of Metazoa, leading to the suggestion that the IAC and associated proteins have a more ancient evolutionary origin than previously anticipated (Abedin and King, 2010; Sebé-Pedrós et al., 2010; Kang et al., 2021), similar to proteins involved in other cell adhesion systems (Harwood and Coates, 2004; Murray and Zaidel-Bar, 2014). Based on the sequenced genomes of Dictyostelium discoideum and Acanthamoeba castellanii, it was thought until recently that integrins α and β were not represented in Amoebozoa (Cavalier-Smith, 2017). However, a recent examination of 113 genomes and transcriptomes identified integrin α homologs in 23 and integrin β homologs in 19 amoebozoan taxa (Kang et al., 2021). Peculiarly, no evidence of integrins was found in the few lineages of Amoebozoa that aggregate to form tissue-like assemblies, such as Dictyostelida, although they produce an elaborate ECM during multicellular development (Huber and O’Day, 2017). It is probable that this is due to a secondary loss of integrins and other IAC components in majority of amoebozoan taxa, similar to the situation in the closest relative of animals, Choanoflagellata, which also lack integrins. One should also not completely dismiss a tantalizing possibility that some IAC components, including integrins, were lost during axenic selection of common laboratory strains such as the AX4 whose genome was sequenced (Eichinger et al., 2005; Bloomfield et al., 2008). Since integrin repeats were identified in two phyla of Asgard archaea (Liu et al., 2021), it is highly likely that integrins were present in the common ancestor of Amoebozoa and Metazoa. Very little is known about the function of amoebozoan integrins, but they are probably involved in the adhesion to external surfaces as in unicellular holozoans (Custodio et al., 1995; Parra-Acero et al., 2020). Interestingly, many amoebozoan species contain either integrins α or β, and yet possess all the signaling components of the IAC (Kang et al., 2021). It would therefore be interesting to examine the functional consequences of a possible integrin homodimerization in these organisms.
It would be of immediate interest to invest more work into the characterization of Dictyostelium adhesome/IAC. One obvious strategy would be to use the powerful tools of proteomics that were utilized to establish a consensus core adhesome of 60 proteins in mammals (Horton et al., 2015). A complementary, ultrastructural approach should be used to obtain a three-dimensional reconstruction of ventral focal adhesion complexes using cryo-electron tomography (Patla et al., 2010). It has been proposed that the focal adhesions mediate the cell attachment by suppressing the repulsive thermal undulations of the cell plasma membrane (Zidovska and Sackmann, 2006; Huang et al., 2012; Fenz et al., 2017). The use of discrete, punctuate adhesive contacts between the cell and its substratum appears to be a universal strategy to accomplish a contact between the two surfaces with a minimal investment of multiprotein assemblies. The emerging Dictyostelium adhesome and a relative ease in manipulating the adhesive conditions in this organism might provide a fruitful independent testing ground of this concept (Loomis et al., 2012).
LM and IW contributed to the conception of the article, searched the literature, wrote the manuscript, prepared the tables, and read and approved the submitted version.
The work of doctoral student LM has been supported by the “Young researchers’ career development project—training of doctoral students” of the Croatian Science Foundation funded by the European Union from the European Social Fund.
The authors declare that the research was conducted in the absence of any commercial or financial relationships that could be construed as a potential conflict of interest.
All claims expressed in this article are solely those of the authors and do not necessarily represent those of their affiliated organizations, or those of the publisher, the editors and the reviewers. Any product that may be evaluated in this article, or claim that may be made by its manufacturer, is not guaranteed or endorsed by the publisher.
We apologize to all colleagues whose work could not be cited owing to space limitations.
Abedin, M., and King, N. (2010). Diverse Evolutionary Paths to Cell Adhesion. Trends Cell Biol. 20, 734–742. doi:10.1016/j.tcb.2010.08.002
Amiji, M., and Park, K. (1992). Prevention of Protein Adsorption and Platelet Adhesion on Surfaces by PEO/PPO/PEO Triblock Copolymers. Biomaterials 13, 682–692. doi:10.1016/0142-9612(92)90128-B
Anthis, N. J., Haling, J. R., Oxley, C. L., Memo, M., Wegener, K. L., Lim, C. J., et al. (2009). β Integrin Tyrosine Phosphorylation Is a Conserved Mechanism for Regulating Talin-Induced Integrin Activation. J. Biol. Chem. 284, 36700–36710. doi:10.1074/jbc.M109.061275
Artemenko, Y., Batsios, P., Borleis, J., Gagnon, Z., Lee, J., Rohlfs, M., et al. (2012). Tumor Suppressor Hippo/MST1 Kinase Mediates Chemotaxis by Regulating Spreading and Adhesion. Proc. Natl. Acad. Sci. U.S.A. 109, 13632–13637. doi:10.1073/pnas.1211304109
Artemenko, Y., Lampert, T. J., and Devreotes, P. N. (2014). Moving towards a Paradigm: Common Mechanisms of Chemotactic Signaling in Dictyostelium and Mammalian Leukocytes. Cell Mol. Life Sci. 71, 3711–3747. doi:10.1007/s00018-014-1638-8
Bachmann, M., Kukkurainen, S., Hytönen, V. P., and Wehrle-Haller, B. (2019). Cell Adhesion by Integrins. Physiol. Rev. 99, 1655–1699. doi:10.1152/physrev.00036.2018
Bastounis, E., Meili, R., Alonso-Latorre, B., del Álamo, J. C., Lasheras, J. C., and Firtel, R. A. (2011). The SCAR/WAVE Complex Is Necessary for Proper Regulation of Traction Stresses during Amoeboid Motility. Mol. Biol. Cell 22, 3995–4003. doi:10.1091/mbc.e11-03-0278
Batsios, P., Ishikawa-Ankerhold, H. C., Roth, H., Schleicher, M., Wong, C. C. L., and Müller-Taubenberger, A. (2019). Ate1-mediated Posttranslational Arginylation Affects Substrate Adhesion and Cell Migration in Dictyostelium discoideum. Mol. Biol. Cell 30, 453–466. doi:10.1091/mbc.E18-02-0132
Benghezal, M., Cornillon, S., Gebbie, L., Alibaud, L., Brückert, F., Letourneur, F., et al. (2003). Synergistic Control of Cellular Adhesion by Transmembrane 9 Proteins. Mol. Biol. Cell 14, 2890–2899. doi:10.1091/mbc.e02-11-0724
Beug, H., Katz, F. E., and Gerisch, G. (1973). Dynamics of Antigenic Membrane Sites Relating to Cell Aggregation in Dictyostelium discoideum. J. Cell Biol. 56, 647–658. doi:10.1083/JCB.56.3.647
Bloomfield, G., Tanaka, Y., Skelton, J., Ivens, A., and Kay, R. R. (2008). Widespread Duplications in the Genomes of Laboratory Stocks of Dictyostelium discoideum. Genome Biol. 9, R75. doi:10.1186/gb-2008-9-4-r75
Bodinier, R., Sabra, A., Leiba, J., Marchetti, A., Lamrabet, O., Ayadi, I., et al. (2021). Role of LrrkA in the Control of Phagocytosis and Cell Motility in Dictyostelium discoideum. Front. Cell Dev. Biol. 9, 1–11. doi:10.3389/fcell.2021.629200
Bosgraaf, L., Waijer, A., Engel, R., Visser, A. J. W. G., Wessels, D., Soll, D., et al. (2005). RasGEF-containing Proteins GbpC and GbpD Have Differential Effects on Cell Polarity and Chemotaxis in Dictyostelium. J. Cell Sci. 118, 1899–1910. doi:10.1242/jcs.02317
Breshears, L. M., Wessels, D., Soll, D. R., and Titus, M. A. (2010). An Unconventional Myosin Required for Cell Polarization and Chemotaxis. Proc. Natl. Acad. Sci. U.S.A. 107, 6918–6923. doi:10.1073/pnas.0909796107
Bretschneider, T., Diez, S., Anderson, K., Heuser, J., Clarke, M., Müller-Taubenberger, A., et al. (2004). Dynamic Actin Patterns and Arp2/3 Assembly at the Substrate-Attached Surface of Motile Cells. Curr. Biol. 14, 1–10. doi:10.1016/j.cub.2003.12.005
Buccilli, M. J., Ilacqua, A. N., Han, M., Banas, A. A., Wight, E. M., Mao, H., et al. (2019). Copine A Interacts with Actin Filaments and Plays a Role in Chemotaxis and Adhesion. Cells 8, 758. doi:10.3390/cells8070758
Bukahrova, T., Weijer, G., Bosgraaf, L., Dormann, D., van Haastert, P. J., and Weijer, C. J. (2005). Paxillin Is Required for Cell-Substrate Adhesion, Cell Sorting and Slug Migration during Dictyostelium Development. J. Cell Sci. 118, 4295–4310. doi:10.1242/jcs.02557
Calderwood, D. A., Campbell, I. D., and Critchley, D. R. (2013). Talins and Kindlins: Partners in Integrin-Mediated Adhesion. Nat. Rev. Mol. Cell Biol. 14, 503–517. doi:10.1038/nrm3624
Calderwood, D. A., Fujioka, Y., De Pereda, J. M., García-Alvarez, B., Nakamoto, T., Margolis, B., et al. (2003). Integrin β Cytoplasmic Domain Interactions with Phosphotyrosine-Binding Domains: A Structural Prototype for Diversity in Integrin Signaling. Proc. Natl. Acad. Sci. U.S.A. 100, 2272–2277. doi:10.1073/pnas.262791999
Cavalier-Smith, T. (2017). Origin of Animal Multicellularity: Precursors, Causes, Consequences-The Choanoflagellate/sponge Transition, Neurogenesis and the Cambrian Explosion. Phil. Trans. R. Soc. B 372, 20150476. doi:10.1098/rstb.2015.0476
Chia, C. P., Gomathinayagam, S., Schmaltz, R. J., and Smoyer, L. K. (2005). Glycoprotein Gp130 of Dictyostelium discoideum Influences Macropinocytosis and Adhesion. Mol. Biol. Cell 16, 2681–2693. doi:10.1091/mbc.e04-06-0483
Cornillon, S., Froquet, R., and Cosson, P. (2008). Involvement of Sib Proteins in the Regulation of Cellular Adhesion in Dictyostelium discoideum. Eukaryot. Cell 7, 1600–1605. doi:10.1128/EC.00155-08
Cornillon, S., Gebbie, L., Benghezal, M., Nair, P., Keller, S., Wehrle‐Haller, B., et al. (2006). An Adhesion Molecule in Free‐Living Dictyostelium Amoebae with Integrin β Features. EMBO Rep. 7, 617–621. doi:10.1038/sj.embor.7400701
Cornillon, S., Pech, E., Benghezal, M., Ravanel, K., Gaynor, E., Letourneur, F., et al. (2000). Phg1p Is a Nine-Transmembrane Protein Superfamily Member Involved in Dictyostelium Adhesion and Phagocytosis. J. Biol. Chem. 275, 34287–34292. doi:10.1074/jbc.M006725200
Custodio, M. R., Imsiecke, G., Borojevic, R., Rinkevich, B., Rogerson, A., and Müller, W. E. G. (1995). Evolution of Cell Adhesion Systems: Evidence for Arg-Gly-Asp-Mediated Adhesion in the Protozoan Neoparamoeba Aestuarina. J. Eukaryot. Microbiol. 42, 721–724. doi:10.1111/j.1550-7408.1995.tb01623.x
Dias, M., Brochetta, C., Marchetti, A., Bodinier, R., Brückert, F., and Cosson, P. (2016). Role of SpdA in Cell Spreading and Phagocytosis in Dictyostelium. PLoS One 11, e0160376–17. doi:10.1371/journal.pone.0160376
Duran, M. B., Rahman, A., Colten, M., and Brazill, D. (2009). Dictyostelium discoideum Paxillin Regulates Actin-Based Processes. Protist 160, 221–232. doi:10.1016/j.protis.2008.09.005
Eichinger, L., Pachebat, J. A., Glöckner, G., Rajandream, M. A., Sucgang, R., Berriman, M., et al. (2005). The Genome of the Social Amoeba Dictyostelium discoideum. Nature 435, 43–57. doi:10.1038/nature03481
Eppler, F. J., Quast, T., and Kolanus, W. (2017). Dynamin2 Controls Rap1 Activation and Integrin Clustering in Human T Lymphocyte Adhesion. PLoS One 12, e0172443–30. doi:10.1371/journal.pone.0172443
Fenz, S. F., Bihr, T., Schmidt, D., Merkel, R., Seifert, U., Sengupta, K., et al. (2017). Membrane Fluctuations Mediate Lateral Interaction between Cadherin Bonds. Nat. Phys. 13, 906–913. doi:10.1038/nphys4138
Fey, P., Stephens, S., Titus, M. A., and Chisholm, R. L. (2002). SadA, a Novel Adhesion Receptor in Dictyostelium. J. Cell Biol. 159, 1109–1119. doi:10.1083/jcb.200206067
Filić, V., Mijanović, L., Putar, D., Talajić, A., Ćetković, H., and Weber, I. (2021). Regulation of the Actin Cytoskeleton via Rho Gtpase Signalling in Dictyostelium and Mammalian Cells: A Parallel Slalom. Cells 10, 1592. doi:10.3390/cells10071592
Frej, A. D., Clark, J., Le Roy, C. I., Lilla, S., Thomason, P. A., Otto, G. P., et al. (2016). The Inositol-3-Phosphate Synthase Biosynthetic Enzyme Has Distinct Catalytic and Metabolic Roles. Mol. Cell Biol. 36, 1464–1479. doi:10.1128/mcb.00039-16
Froquet, R., Cherix, N., Birke, R., Benghezal, M., Cameroni, E., Letourneur, F., et al. (2008). Control of Cellular Physiology by TM9 Proteins in Yeast and Dictyostelium. J. Biol. Chem. 283, 6764–6772. doi:10.1074/jbc.M704484200
Froquet, R., le Coadic, M., Perrin, J., Cherix, N., Cornillon, S., and Cosson, P. (2012). TM9/Phg1 and SadA Proteins Control Surface Expression and Stability of SibA Adhesion Molecules in Dictyostelium. Mol. Biol. Cell 23, 679–686. doi:10.1091/mbc.E11-04-0338
Fukui, Y., De Hostos, E., Yumura, S., Kitanishi-Yumura, T., and Inoué, S. (1999). Architectural Dynamics of F-Actin in Eupodia Suggests Their Role in Invasive Locomotion in Dictyostelium. Exp. Cell Res. 249, 33–45. doi:10.1006/excr.1999.4445
Fukui, Y., and Inoué, S. (1997). Amoeboid Movement Anchored by Eupodia, New Actin-Rich Knobby Feet in Dictyostelium. Cell Motil. Cytoskelet. 36, 339–354. doi:10.1002/(SICI)1097-0169
Gahmberg, C. G., and Grönholm, M. (2022). How Integrin Phosphorylations Regulate Cell Adhesion and Signaling. Trends Biochem. Sci. 47, 265–278. doi:10.1016/j.tibs.2021.11.003
Galdeen, S. A., Stephens, S., Thomas, D. D., and Titus, M. A. (2007). Talin Influences the Dynamics of the Myosin VII-Membrane Interaction. Mol. Biol. Cell 18, 4074–4084. doi:10.1091/mbc.e06-07-0586
Gebbie, L., Benghezal, M., Cornillon, S., Froquet, R., Cherix, N., Malbouyres, M., et al. (2004). Phg2, a Kinase Involved in Adhesion and Focal Site Modeling in Dictyostelium. Mol. Biol. Cell 15, 3915–3925. doi:10.1091/mbc.E03–12–0908
Gingell, D., and Vince, S. (1982). Substratum Wettability and Charge Influence the Spreading of Dictyostelium Amoebae and the Formation of Ultrathin Cytoplasmic Lamellae. J. Cell Sci. 54, 255–285. doi:10.1242/jcs.54.1.255
Goldberg, J. M., Manning, G., Liu, A., Fey, P., Pilcher, K. E., Xu, Y., et al. (2006). The Dictyostelium Kinome-Analysis of the Protein Kinases from a Simple Model Organism. PLoS Genet. 2, e38. doi:10.1371/journal.pgen.0020038
Harwood, A., and Coates, J. C. (2004). A Prehistory of Cell Adhesion. Curr. Opin. Cell Biol. 16, 470–476. doi:10.1016/j.ceb.2004.07.011
Hibi, M., Nagasaki, A., Takahashi, M., Yamagishi, A., and Uyeda, T. Q. P. (2004). Dictyostelium discoideum Talin A Is Crucial for Myosin II-independent and Adhesion-dependent Cytokinesis. J. Muscle Res. Cell Motil. 25, 127–140. doi:10.1023/B:JURE.0000035842.71415.f3
Horton, E. R., Byron, A., Askari, J. A., Ng, D. H. J., Millon-Frémillon, A., Robertson, J., et al. (20152015). Definition of a Consensus Integrin Adhesome and its Dynamics during Adhesion Complex Assembly and Disassembly. Nat. Cell Biol. 17, 1577–1587. doi:10.1038/ncb3257
Huang, J., Peng, X., Xiong, C., and Fang, J. (2012). Influence of Substrate Rigidity on Primary Nucleation of Cell Adhesion: A Thermal Fluctuation Model. J. Colloid Interface Sci. 366, 200–208. doi:10.1016/j.jcis.2011.09.046
Huber, R. J., and O'Day, D. H. (2012). EGF-like Peptide-Enhanced Cell Movement in Dictyostelium Is Mediated by Protein Kinases and the Activity of Several Cytoskeletal Proteins. Cell Signal. 24, 1770–1780. doi:10.1016/j.cellsig.2012.05.004
Huber, R. J., and O'Day, D. H. (2017). Extracellular Matrix Dynamics and Functions in the Social Amoeba Dictyostelium: A Critical Review. Biochimica Biophysica Acta (BBA) - General Subj. 1861, 2971–2980. doi:10.1016/j.bbagen.2016.09.026
Hynes, R. (1987). Integrins: A Family of Cell Surface Receptors. Cell 48, 549–554. doi:10.1016/0092-8674(87)90233-9
Hynes, R. O. (2004). The Emergence of Integrins: a Personal and Historical Perspective. Matrix Biol. 23, 333–340. doi:10.1016/J.MATBIO.2004.08.001
Ibarra, N., Blagg, S. L., Vazquez, F., and Insall, R. H. (2006). Nap1 Regulates Dictyostelium Cell Motility and Adhesion through SCAR-dependent and -Independent Pathways. Curr. Biol. 16, 717–722. doi:10.1016/j.cub.2006.02.068
Iwadate, Y., and Yumura, S. (2008). Actin-based Propulsive Forces and Myosin-II-Based Contractile Forces in migrating Dictyostelium Cells. J. Cell. Sci. 121, 1314–1324. doi:10.1242/jcs.021576
Jay, P. Y., Pham, P. A., Wong, S. A., and Elson, E. L. (1995). A Mechanical Function of Myosin II in Cell Motility. J. Cell. Sci. 108, 387–393. doi:10.1242/JCS.108.1.387
Jeon, J., Kim, D., and Jeon, T. J. (2021). Opposite Functions of RapA and RapC in Cell Adhesion and Migration in Dictyostelium. Animal Cells Syst. 25, 203–210. doi:10.1080/19768354.2021.1947372
Jeon, T. J., Lee, D.-J., Lee, S., Weeks, G., and Firtel, R. A. (2007a). Regulation of Rap1 Activity by RapGAP1 Controls Cell Adhesion at the Front of Chemotaxing Cells. J. Cell. Biol. 179, 833–843. doi:10.1083/jcb.200705068
Jeon, T. J., Lee, D.-J., Merlot, S., Weeks, G., and Firtel, R. A. (2007b). Rap1 Controls Cell Adhesion and Cell Motility through the Regulation of Myosin II. J. Cell. Biol. 176, 1021–1033. doi:10.1083/jcb.200607072
Kamprad, N., Witt, H., Schröder, M., Kreis, C. T., Bäumchen, O., Janshoff, A., et al. (2018). Adhesion Strategies of Dictyostelium discoideum- a Force Spectroscopy Study. Nanoscale 10, 22504–22519. doi:10.1039/c8nr07107a
Kang, R., Kae, H., Ip, H., Spiegelman, G. B., and Weeks, G. (2002). Evidence for a Role for the Dictyostelium Rap1 in Cell Viability and the Response to Osmotic Stress. J. Cell. Sci. 115, 3675–3682. doi:10.1242/jcs.00039
Kang, S., Tice, A. K., Stairs, C. W., Jones, R. E., Lahr, D. J. G., and Brown, M. W. (2021). The Integrin-Mediated Adhesive Complex in the Ancestor of Animals, Fungi, and Amoebae. Curr. Biol. 31, 3073–3085. e3. doi:10.1016/J.CUB.2021.04.076
Kelsey, J. S., and Blumberg, D. D. (2013). A SAP Domain-Containing Protein Shuttles between the Nucleus and Cell Membranes and Plays a Role in Adhesion and Migration in D. discoideum. Biol. Open 2, 396–406. doi:10.1242/bio.20133889
Kim, D., Kim, W., and Jeon, T. J. (2021). Reversible Function of RapA with the C-Terminus of RapC in Dictyostelium. J. Microbiol. 59, 848–853. doi:10.1007/s12275-021-1400-5
Kim, H., Lee, M.-R., and Jeon, T. J. (2017). Loss of FrmB Results in Increased Size of Developmental Structures during the Multicellular Development of Dictyostelium Cells. J. Microbiol. 55, 730–736. doi:10.1007/s12275-017-7221-x
Kolanus, W., Nagel, W., Schiller, B., Zeitlmann, L., Godar, S., Stockinger, H., et al. (1996). αLβ2 Integrin/LFA-1 Binding to ICAM-1 Induced by Cytohesin-1, a Cytoplasmic Regulatory Molecule. Cell. 86, 233–242. doi:10.1016/S0092-8674(00)80095-1
Kortholt, A., Bolourani, P., Rehmann, H., Keizer-Gunnink, I., Weeks, G., Wittinghofer, A., et al. (2010). A Rap/Phosphatidylinositol 3-Kinase Pathway Controls Pseudopod Formation. Mol. Biol. Cell 21, 936–945. doi:10.1091/mbc.e09-03-0177
Kortholt, A., Rehmann, H., Kae, H., Bosgraaf, L., Keizer-Gunnink, I., Weeks, G., et al. (2006). Characterization of the GbpD-Activated Rap1 Pathway Regulating Adhesion and Cell Polarity in Dictyostelium discoideum. J. Biol. Chem. 281, 23367–23376. doi:10.1074/jbc.M600804200
Kowal, A. S., and Chisholm, R. L. (2011). Uncovering a Role for the Tail of the Dictyostelium discoideum Sada Protein in Cell-Substrate Adhesion. Eukaryot. Cell. 10, 662–671. doi:10.1128/EC.00221-10
Kreitmeier, M., Gerisch, G., Heizer, C., and Müller-Taubenberger, A. (1995). A Talin Homologue of Dictyostelium Rapidly Assembles at the Leading Edge of Cells in Response to Chemoattractant. J. Cell. Biol. 129, 179–188. doi:10.1083/jcb.129.1.179
Lagarrigue, F., Kim, C., and Ginsberg, M. H. (2016). The Rap1-RIAM-Talin axis of Integrin Activation and Blood Cell Function. Blood 128, 479–487. doi:10.1182/blood-2015-12-638700
Lampert, T. J., Kamprad, N., Edwards, M., Borleis, J., Watson, A. J., Tarantola, M., et al. (2017). Shear Force-Based Genetic Screen Reveals Negative Regulators of Cell Adhesion and Protrusive Activity. Proc. Natl. Acad. Sci. U.S.A. 114, E7727–E7736. doi:10.1073/pnas.1616600114
Legerstee, K., and Houtsmuller, A. (2021). A Layered View on Focal Adhesions. Biology 10, 1189. doi:10.3390/biology10111189
Linkner, J., Witte, G., Zhao, H., Junemann, A., Nordholz, B., Runge-Wollmann, P., et al. (2014). The Inverse BAR-Domain Protein IBARa Drives Membrane Remodelling to Control Osmoregulation, Phagocytosis and Cytokinesis. J. Cell. Sci. 127, 1279–1292. doi:10.1242/jcs.140756
Liu, C.-I., Cheng, T.-L., Chen, S.-Z., Huang, Y.-C., and Chang, W.-T. (2005). LrrA, a Novel Leucine-Rich Repeat Protein Involved in Cytoskeleton Remodeling, Is Required for Multicellular Morphogenesis in Dictyostelium discoideum. Dev. Biol. 285, 238–251. doi:10.1016/j.ydbio.2005.05.045
Liu, Y.-J., Le Berre, M., Lautenschlaeger, F., Maiuri, P., Callan-Jones, A., Heuzé, M., et al. (2015). Confinement and Low Adhesion Induce Fast Amoeboid Migration of Slow Mesenchymal Cells. Cell 160, 659–672. doi:10.1016/j.cell.2015.01.007
Liu, Y., Makarova, K. S., Huang, W.-C., Wolf, Y. I., Nikolskaya, A. N., Zhang, X., et al. (2021). Expanded Diversity of Asgard Archaea and Their Relationships with Eukaryotes. Nature 593, 553–557. doi:10.1038/s41586-021-03494-3
Loomis, W. F., Fuller, D., Gutierrez, E., Groisman, A., and Rappel, W.-J. (2012). Innate Non-specific Cell Substratum Adhesion. PLoS One 7, e42033. doi:10.1371/journal.pone.0042033
McLeod, S. J., Shum, A. J., Lee, R. L., Takei, F., and Gold, M. R. (2004). The Rap GTPases Regulate Integrin-Mediated Adhesion, Cell Spreading, Actin Polymerization, and Pyk2 Tyrosine Phosphorylation in B Lymphocytes. J. Biol. Chem. 279, 12009–12019. doi:10.1074/jbc.M313098200
Müller-Taubenberger, A., Ishikawa-Ankerhold, H. C., Kastner, P. M., Burghardt, E., and Gerisch, G. (2009). The STE Group Kinase SepA Controls Cleavage Furrow Formation in Dictyostelium. Cell Motil. Cytoskelet. 66, 929–939. doi:10.1002/cm.20386
Murray, P. S., and Zaidel-Bar, R. (2014). Pre-metazoan Origins and Evolution of the Cadherin Adhesome. Biol. Open 3, 1183–1195. doi:10.1242/BIO.20149761
Nagasaki, A., Kanada, M., and Uyeda, T. Q. (2009). Cell Adhesion Molecules Regulate Contractile Ring-independent Cytokinesis in Dictyostelium discoideum. Cell Res. 19, 236–246. doi:10.1038/cr.2008.318
Niewöhner, J., Weber, I., Maniak, M., Müller-Taubenberger, A., and Gerisch, G. (1997). Talin-Null Cells of Dictyostelium Are Strongly Defective in Adhesion to Particle and Substrate Surfaces and Slightly Impaired in Cytokinesis. J. Cell Biol. 138, 349–361.
Owens, N. F., Gingell, D., and Rutter, P. R. (1987). Inhibition of Cell Adhesion by a Synthetic Polymer Adsorbed to Glass Shown under Defined Hydrodynamic Stress. J. Cell Sci. 87, 667–675. doi:10.1242/jcs.87.5.667
Owens, N. F., Gingell, D., and Trommler, A. (1988). Cell Adhesion to Hydroxyl Groups of a Monolayer Film. J. Cell Sci. 91 (Pt 2), 269–279. doi:10.1242/jcs.91.2.269
Park, B., Shin, D. Y., and Jeon, T. J. (2018b). CBP7 Interferes with the Multicellular Development of Dictyostelium Cells by Inhibiting Chemoattractant-Mediated Cell Aggregation. Mol. Cells 41, 103–109. doi:10.14348/molcells.2018.2170
Park, B., Kim, H., and Jeon, T. J. (2018a). Loss of RapC Causes Defects in Cytokinesis, Cell Migration, and Multicellular Development of Dictyostelium. Biochem. Biophysical Res. Commun. 499, 783–789. doi:10.1016/j.bbrc.2018.03.223
Parkinson, K., Bolourani, P., Traynor, D., Aldren, N. L., Kay, R. R., Weeks, G., et al. (2009). Regulation of Rap1 Activity Is Required for Differential Adhesion, Cell-type Patterning and Morphogenesis in Dictyostelium. J. Cell. Sci. 122, 335–344. doi:10.1242/jcs.036822
Parra-Acero, H., Harcet, M., Sánchez-Pons, N., Casacuberta, E., Brown, N. H., Dudin, O., et al. (2020). Integrin-Mediated Adhesion in the Unicellular Holozoan Capsaspora owczarzaki. Curr. Biol. 30, 4270–4275. doi:10.1016/j.cub.2020.08.015
Patel, H., König, I., Tsujioka, M., Frame, M. C., Anderson, K. I., and Brunton, V. G. (2008). The Multi-FERM-Domain-Containing Protein FrmA Is Required for Turnover of Paxillin-Adhesion Sites during Cell Migration of Dictyostelium. J. Cell Sci. 121, 1159–1164. doi:10.1242/jcs.021725
Patla, I., Volberg, T., Elad, N., Hirschfeld-Warneken, V., Grashoff, C., Fässler, R., et al. (2010). Dissecting the Molecular Architecture of Integrin Adhesion Sites by Cryo-Electron Tomography. Nat. Cell. Biol. 12 (12), 909–915. doi:10.1038/ncb2095
Petersen, K. J., Goodson, H. V., Arthur, A. L., Luxton, G. W. G., Houdusse, A., Titus, M. A., et al. (2016). MyTH4-FERM Myosins Have an Ancient and Conserved Role in Filopod Formation. Proc. Natl. Acad. Sci. U.S.A. 113, E8059–E8068. doi:10.1073/pnas.1615392113
Plak, K., Pots, H., Van Haastert, P. J. M., and Kortholt, A. (2016). Direct Interaction between TalinB and Rap1 Is Necessary for Adhesion of Dictyostelium Cells. BMC Cell Biol. 17, 1–8. doi:10.1186/s12860-015-0078-0
Pribic, J., Garcia, R., Kong, M., and Brazill, D. (2011). Paxillin and Phospholipase D Interact to Regulate Actin-Based Processes in dictyostelium Discoideum. Eukaryot. Cell 10, 977–984. doi:10.1128/EC.00282-10
Rai, A., Nöthe, H., Tzvetkov, N., Korenbaum, E., and Manstein, D. J. (2011). Dictyostelium Dynamin B Modulates Cytoskeletal Structures and Membranous Organelles. Cell Mol. Life Sci. 68, 2751–2767. doi:10.1007/s00018-010-0590-5
Rebstein, P. J., Weeks, G., and Spiegelman, G. B. (1993). Altered Morphology of Vegetative Amoebae Induced by Increased Expression of the Dictyostelium discoideum Ras-Related Gene rap 1. Dev. Genet. 14, 347–355. doi:10.1002/dvg.1020140504
Riley, D. R. J., Khalil, J. S., Pieters, J., Naseem, K. M., and Rivero, F. (2020). Coronin 1 Is Required for Integrin β2 Translocation in Platelets. Int. J. Mol. Sci. 21, 356–420. doi:10.3390/ijms21010356
Schirenbeck, A., Bretschneider, T., Arasada, R., Schleicher, M., and Faix, J. (2005). The Diaphanous-Related Formin dDia2 Is Required for the Formation and Maintenance of Filopodia. Nat. Cell Biol. 7, 619–625. doi:10.1038/ncb1266
Sebé-Pedrós, A., Roger, A. J., Lang, F. B., King, N., and Ruiz-Trillo, I. (2010). Ancient Origin of the Integrin-Mediated Adhesion and Signaling Machinery. Proc. Natl. Acad. Sci. U.S.A. 107, 10142–10147. doi:10.1073/pnas.1002257107
Shina, M. C., Müller, R., Blau-Wasser, R., Glöckner, G., Schleicher, M., Eichinger, L., et al. (2010a). A Cytohesin Homolog in Dictyostelium Amoebae. PLoS One 5, e9378. doi:10.1371/journal.pone.0009378
Shina, M. C., Ünal, C., Eichinger, L., Müller-Taubenberger, A., Schleicher, M., Steinert, M., et al. (2010b). A Coronin7 Homolog with Functions in Actin-Driven Processes. J. Biol. Chem. 285, 9249–9261. doi:10.1074/jbc.M109.083725
Simson, R., Wallraff, E., Faix, J., Niewöhner, J., Gerisch, G., and Sackmann, E. (1998). Membrane Bending Modulus and Adhesion Energy of Wild-type and Mutant Cells of Dictyostelium Lacking Talin or Cortexillins. Biophysical J. 74, 514–522. doi:10.1016/S0006-3495(98)77808-7
Tamura, M., Gu, J., Matsumoto, K., Aota, S.-i., Parsons, R., and Yamada, K. M. (1998). Inhibition of Cell Migration, Spreading, and Focal Adhesions by Tumor Suppressor PTEN. Science 280, 1614–1617. doi:10.1126/science.280.5369.1614
Tarantola, M., Bae, A., Fuller, D., Bodenschatz, E., Rappel, W.-J., and Loomis, W. F. (2014). Cell Substratum Adhesion during Early Development of Dictyostelium discoideum. PLoS One 9, e106574–7. doi:10.1371/journal.pone.0106574
Titus, M. A. (1999). A Class VII Unconventional Myosin Is Required for Phagocytosis. Curr. Biol. 9, 1297–1303. doi:10.1016/S0960-9822(00)80051-2
Todd, I., Mellor, J. S., and Gingell, D. (1988). Mapping Cell-Glass Contacts of Dictyostelium Amoebae by Total Internal Reflection Aqueous Fluorescence Overcomes a Basic Ambiguity of Interference Reflection Microscopy. J. Cell Sci. 89, 107–114. doi:10.1242/jcs.89.1.107
Tsujioka, M., Yoshida, K., and Inouye, K. (2004). Talin B Is Required for Force Transmission in Morphogenesis of Dictyostelium. EMBO J. 23, 2216–2225. doi:10.1038/sj.emboj.7600238
Tsujioka, M., Yoshida, K., Nagasaki, A., Yonemura, S., Müller Taubenberger, A., and Uyeda, T. Q. P. (2008). Overlapping Functions of the Two Talin Homologues in Dictyostelium. Eukaryot. Cell 7, 906–916. doi:10.1128/EC.00464-07
Tsujioka, M., Yumura, S., Inouye, K., Patel, H., Ueda, M., and Yonemura, S. (2012). Talin Couples the Actomyosin Cortex to the Plasma Membrane during Rear Retraction and Cytokinesis. Proc. Natl. Acad. Sci. U.S.A. 109, 12992–12997. doi:10.1073/pnas.1208296109
Tuxworth, R. I., Stephens, S., Ryan, Z. C., and Titus, M. A. (2005). Identification of a Myosin VII-Talin Complex. J. Biol. Chem. 280, 26557–26564. doi:10.1074/jbc.M503699200
Tuxworth, R. I., Weber, I., Wessels, D., Addicks, G. C., Soll, D. R., Gerisch, G., et al. (2001). A Role for Myosin VII in Dynamic Cell Adhesion. Curr. Biol. 11, 318–329. doi:10.1016/S0960-9822(01)00097-5
Uchida, K. S. K., and Yumura, S. (2004). Dynamics of Novel Feet of Dictyostelium Cells during Migration. J. Cell Sci. 117, 1443–1455. doi:10.1242/jcs.01015
Veltman, D. M., King, J. S., Machesky, L. M., and Insall, R. H. (2012). SCAR Knockouts in Dictyostelium: WASP Assumes SCAR's Position and Upstream Regulators in Pseudopods. J. Cell Biol. 198, 501–508. doi:10.1083/jcb.201205058
Weber, I., Wallraff, E., Albrecht, R., and Gerisch, G. (1995). Motility and Substratum Adhesion of Dictyostelium Wild-type and Cytoskeletal Mutant Cells: A Study by RICM/bright-field Double-View Image Analysis. J. Cell Sci. 108, 1519–1530. doi:10.1242/jcs.108.4.1519
Wessels, D., Vawter-Hugart, H., Murray, J., and Soll, D. R. (1994). Three-dimensional Dynamics of Pseudopod Formation and the Regulation of Turning during the Motility Cycle of Dictyostelium. Cell Motil. Cytoskelet. 27, 1–12. doi:10.1002/CM.970270102
Yumura, S., and Kitanishi-Yumura, T. (1990). Fluorescence-mediated Visualization of Actin and Myosin Filaments in the Contractile Membrane-Cytoskeleton Complex of Dictyostelium discoideum. Cell Struct. Funct. 15, 355–364. doi:10.1247/csf.15.355
Zaidel-Bar, R. (2009). Evolution of Complexity in the Integrin Adhesome. J. Cell Biol. 186, 317–321. doi:10.1083/JCB.200811067
Zhang, H., Chang, Y.-C., Brennan, M. L., and Wu, J. (2014). The Structure of RAP1 in Complex with RIAM Reveals Specificity Determinants and Recruitment Mechanism. Jpn. J. Clin. Oncol. 6, 128–139. doi:10.1093/jmcb/mjt044
Zhu, L., Yang, J., Bromberger, T., Holly, A., Lu, F., Liu, H., et al. (2017). Structure of Rap1b Bound to Talin Reveals a Pathway for Triggering Integrin Activation. Nat. Commun. 8, 1–11. doi:10.1038/s41467-017-01822-8
Keywords: cell-substratum adhesion, adhesion receptors, cell migration, actin cytoskeleton, amoebozoa, integrins, mesenchymal-amoeboid transition
Citation: Mijanović L and Weber I (2022) Adhesion of Dictyostelium Amoebae to Surfaces: A Brief History of Attachments. Front. Cell Dev. Biol. 10:910736. doi: 10.3389/fcell.2022.910736
Received: 01 April 2022; Accepted: 13 May 2022;
Published: 27 May 2022.
Edited by:
Vassiliki Kostourou, Alexander Fleming Biomedical Sciences Research Center, GreeceReviewed by:
Dongmyung Oh, University of Texas Medical Branch at Galveston, United StatesCopyright © 2022 Mijanović and Weber. This is an open-access article distributed under the terms of the Creative Commons Attribution License (CC BY). The use, distribution or reproduction in other forums is permitted, provided the original author(s) and the copyright owner(s) are credited and that the original publication in this journal is cited, in accordance with accepted academic practice. No use, distribution or reproduction is permitted which does not comply with these terms.
*Correspondence: Igor Weber, aXdlYmVyQGlyYi5ocg==
Disclaimer: All claims expressed in this article are solely those of the authors and do not necessarily represent those of their affiliated organizations, or those of the publisher, the editors and the reviewers. Any product that may be evaluated in this article or claim that may be made by its manufacturer is not guaranteed or endorsed by the publisher.
Research integrity at Frontiers
Learn more about the work of our research integrity team to safeguard the quality of each article we publish.