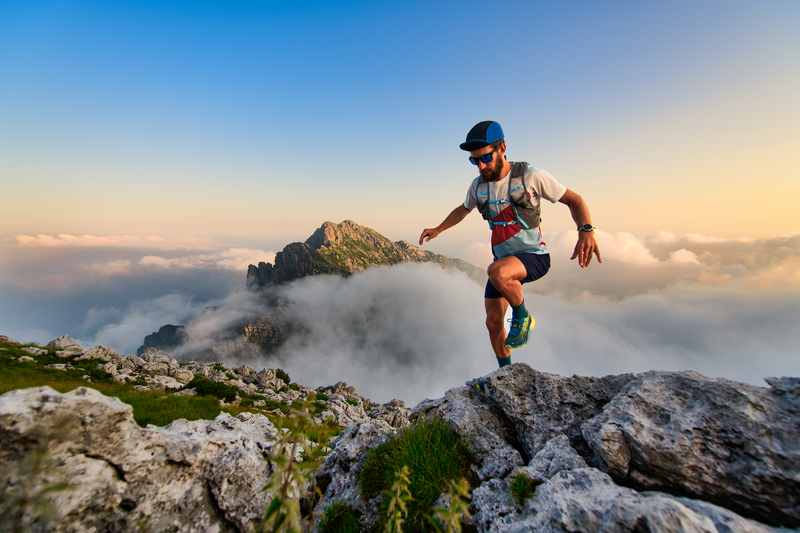
94% of researchers rate our articles as excellent or good
Learn more about the work of our research integrity team to safeguard the quality of each article we publish.
Find out more
REVIEW article
Front. Cell Dev. Biol. , 30 September 2022
Sec. Stem Cell Research
Volume 10 - 2022 | https://doi.org/10.3389/fcell.2022.908790
Cardiomyopathy is a major cause of heart failure, leading to systolic and diastolic dysfunction and promoting adverse cardiac remodeling. Macrophages, as key immune cells of the heart, play a crucial role in inflammation and fibrosis. Moreover, exogenous and cardiac resident macrophages are functionally and phenotypically different during cardiac injury. Although experimental evidence has shown that macrophage-targeted therapy is promising in cardiomyopathy, clinical translation remains challenging. In this article, the molecular mechanism of macrophages in cardiomyopathy has been discussed in detail based on existing literature. The issues and considerations of clinical treatment strategies for myocardial fibrosis has also been analyzed.
Although great progress has been made in preventing and treating cardiovascular diseases, it still has the highest morbidity and mortality worldwide (Roth et al., 2020). The development of cardiovascular disease to the end-stage leads to heart failure, one of the main causes of cardiovascular death (Crespo-Leiro et al., 2018; Roth et al., 2020). Cardiomyopathy is the major cause of heart failure. Cardiomyopathy is defined as a heterogeneous myocardial disease with inappropriate hypertrophy or dilation of the ventricle (Brieler et al., 2017). Besides, the definition and classification of cardiomyopathy has considerably changed, including primary and secondary categories (McKenna et al., 2017). The categories are subdivided into varied phenotypes including dilated, hypertrophic, and restrictive patterns. Heart transplantation is the only effective treatment for heart failure (Truby and Rogers, 2020). Therefore, it is important to understand the pathological development of cardiomyopathy.
The heart comprises various cells, including cardiomyocytes, smooth muscle cells, fibroblasts, endothelial cells, and various immune cells (Frangogiannis, 2019; Peet et al., 2020). Monocytes are a key component of immune cells. They can produce various inflammatory cytokines such as IL-1, TNFα and IL-6, by exposing endogenous damage-associated molecular patterns (DAMPs) after cell injury. Chemokines can recruit monocytes from the blood and spleen to the heart and activate their separation into macrophages (Honold and Nahrendorf, 2018). Macrophages have in recent years become a key research area in cardiovascular diseases. Macrophages are central regulators of the immune system. They can activate and proliferate lymphocytes to generate innate and adaptive immune responses for defense, inflammation, and tissue recovery (Varol et al., 2015; Wynn and Vannella, 2016; Shapouri-Moghaddam et al., 2018).
Macrophages perform different functions during myocardial damage. In the myocardial infarction/ischemia reperfusion injury, pro-inflammatory macrophages play a dominant role in the early stages, repair macrophages play an important role as the disease progresses. In other cardiomyopathy, macrophages exhibit different functional phenotypes under different stimuli. Macrophages, as primary immune cells, can regulate repair and healing after myocardial injury. Therefore, they are potential target immune cell for heart failure prevention and therapeutic modalities (Sager et al., 2016; Dick et al., 2019; Zhao et al., 2019). In this paper, we focused on the main function of macrophages in cardiomyopathy.
Macrophages are key elements of innate and adaptive immune responses. Macrophages regulate inflammation and homeostasis during organ development (Wynn et al., 2013). For mant years, quite a few people were sure that all human macrophages were differentiated from blood monocytes after penetrating blood vessels (van Furth and Cohn, 1968), however, Epelman et al. refute this hypothesis (Epelman et al., 2014a). Currently, the yolk sac, fetal liver and bone marrow hematopoietic cells are the main sources of tissue-macrophages (Hogg et al., 2020). In fact, each organ has the unique macrophages combination. In addition, monocyte-derived macrophages release proinflammatory factors to increase the inflammatory response and tissue-macrophages assist in host defense and tissue remodeling. Macrophages have a highly plastic phenotype dependent on the activation (in vitro) or the microenvironment (in vivo) under appropriate environmental stimuli (Zhang et al., 2021). Notably, macrophages act as a pro-inflammatory or an anti-inflammatory subtype to protect the body from injury.
Macrophage exposure to excessive stimuli may make macrophages have different functions and cell surface markers. Macrophages are classified into M1 and M2 based on the stimulation type, surface molecule expression, function, and secretion profile (Figure 1). Proinflammatory M1 macrophages have anti-pathogen activity. M1a macrophages are stimulated by IFNγ, while M1b macrophages are stimulated by LPS (Mukhopadhyay et al., 2004; Martinez et al., 2008). Anti-inflammatory M2 macrophages promote tissue repair. M2a macrophages with a predominantly anti-inflammatory phenotype are polarized by IL-4 and IL-13 and secrete IL-10 and IL-1 receptor antagonists to suppress the inflammatory responses (Lee et al., 2001; Sierra-Filardi et al., 2010; Jetten et al., 2014). The M2b macrophages with pro- and anti-inflammatory phenotypes produce IL-1β, IL-6, TNFα, and IL-10 vi LPS stimulation (Mosser, 2003). M2c macrophages activated by IL-10 can secrete transforming growth factor 1 (TGFβ1) and glucocorticoids (Mosser and Edwards, 2008). M2d macrophages are activated by adenosine A2A receptor (A2AR) agonists and toll-like receptor (TLR) agonists (Ferrante et al., 2013). M2f cells macrophages are stimulated by macrophage apoptotic clearance (MAC) (O'Rourke et al., 2019). In summary, the polarization of macrophages is closely related to changes in glycolysis (M1-like) and oxidative phosphorylation (M2-like).
FIGURE 1. Functions and phenotype of macrophages. Macrophages are classified into M1 and M2 based on the stimulation type and function. M1 macrophages have pro-inflammatory activity, M2 macrophages have anti-inflammatory and tissue repair activity. These macrophages are divided into subtypes based on the stimulation. Proinflammatory M1 macrophages are divided into M1a macrophages stimulated by IFNγ and M1b macrophages stimulated by LPS. Anti-inflammatory M1 macrophages are divided into M2a macrophages stimulated by IL-4 and IL-13, M2b macrophages stimulated by LPS, M2c macrophages stimulated by IL-10, M2d macrophages stimulated by A2AR and TLR agonists and M2f macrophages stimulated by macrophage apoptotic clearance. M2a macrophages can suppress the inflammatory responses. M2b macrophages have pro- and anti-inflammatory activity. M2c macrophages can secrete TGFβ1 and glucocorticoids.
Although the origin of tissue macrophages has been controversial over the past years, tissue macrophages are currently a hot research area. Resident tissue macrophages in most adult organs develop from fetal monocytes and can be locally sustained throughout the life cycle (Sawai et al., 2016; Bajpai et al., 2018). Resident cardiac macrophages have an essential role in homeostasis, cardiac function, and tissue repair. The four populations in normal mouse hearts have varying levels of Ly6C, CD11c, CCR2, and the major histocompatibility complex (MHCII) (Epelman et al., 2014a). Single-cell RNA sequencing of cardiac macrophages used an unbiased approach to confirm the presence of three subpopulations. Cardiac macrophages were of M2 type during the stabilization period, accompanied by high expression of Ly6C, and had a repair function. Interestingly, cardiac macrophages for CCR2 are located in the myocardial wall. They are essential for the reconstruction of the original coronary by secreting pro-angiogenic signals. CCR2-macrophages develop in the heart during the embryonic. They are maintained under homeostatic conditions independently of peripheral blood mononuclear cell infiltration, and promote electrical conduction within the atrioventricular node function (Bajpai et al., 2019).
Myocarditis is an inflammatory process affecting the muscle tissue (myocardium) of the heart (Cooper, 2009). Autopsy studies in the general population have shown that myocarditis is the main cause of sudden death and an early cause of cardiomyopathy (Ammirati et al., 2020). Viral infection is a major cause of myocarditis. It also activates the immune system to cause myocardial inflammation, necrosis, the release of DAMPs, and ventricular dysfunction by directly killing virus-infected cardiomyocytes and increasing the production of inflammatory cytokines. As a result, it affects the function of cardiomyocytes, eventually leading to the occurrence of dilated cardiomyopathy (Luo et al., 2010; Pollack et al., 2015). The incidence of viral myocarditis is closely associated with various viral infections, such as enteroviruses, influenza viruses, and cytomegalovirus. The pathogenesis of viral myocarditis has three stages: acute viremia stage, subacute infiltration stage, and chronic stage. Heart transplantation is the main treatment method for myocarditis clinically.
Monocytes and macrophages are the major constituents of inflammatory cell infiltration in human myocarditis and may play an immunomodulatory role. Cardiac injury caused by myocarditis leads to early Ly6Chi inflammatory macrophage recruitment (Barin et al., 2012). Macrophages affect the cardiac immune microenvironment by secreting proinflammatory cytokines, including TNF-α, IL-1β, IL-6, and chemokine CCL2. Macrophages can also modulate the strength, bias, and persistence of subsequent adaptive immune responses. B cells can aggravate myocardial injury by secreting cytokines and promoting monocyte recruitment and CCR2 macrophage infiltration (Bajpai et al., 2019; Heinrichs et al., 2021). M2 macrophages inhibit inflammatory responses and promote cardiac fibrotic healing. Therefore, it is a hallmark of the transition from acute myocarditis to chronic pathological remodeling, with macrophage replacement by profibrotic myofibroblasts.
Coronavirus is a class of enveloped, positive, single-stranded, and highly diverse RNA viruses. SARS coronavirus 2 that causes COVID-19 is a novel β-coronavirus with high sequence homology to the early SARS coronavirus that caused the SARS outbreak (Zhou et al., 2021). The new coronavirus causes many and very serious sequelae, especially in the cardiovascular aspect. This indicates that troponin-I, a biomarker of myocardial damage, is elevated after myocardial injury in COVID-19 patients (Nishiga et al., 2020; Zaninotto et al., 2020). Moreover, COVID-19 patients have other cardiovascular complications, such as acute myocardial infarction, myocarditis, and heart failure. Myocarditis develops longer (up to 10–15 days) after the onset of symptoms in COVID-19 patients. A 32 adaptive T-cell-mediated immunity may play a key role in the development of myocardial inflammation after 2 weeks of COVID-19 onset. Notably, an increase in the proinflammatory CCR6+ and Th17 in CD4+ T cells, the main inflammatory mediator of myocarditis, has been reported in severe cases (Golovkin et al., 2021).
SARS-CoV2 can replicate in cardiomyocytes derived by human-induced pluripotent stem cell (hiPSC) and induce cytotoxic effects to eliminating cardiomyocyte beating (Sharma et al., 2020; Lee et al., 2021). Another study showed that SARS-CoV2 utilizes the ACE2 receptors for internalization via the transmembrane protease serine 2 protease (Hatmal et al., 2020). Virus-induced downregulation of ACE2 may attenuate the function, reduce anti-inflammatory effect, and elevate the effect of AngII in susceptible patients (Perez-Bermejo et al., 2020). Yang et al. have found macrophages mediates myocardial injury caused by SARS-CoV2. Macrophages can increase TNFα and IL-6 production and active JAK/STAT pathway to exacerbate oxidative stress (Yang et al., 2021). Meanwhie, Husam Jum’ah et al. have demonstrated the density of macrophages is positively correlated with myocardial apoptosis (Jum’ah et al., 2022). However, the infectivity of cardiac resident macrophages is unknown.
Current researches have demonstrated that immune cells play a crucial role in the formation and prognosis of cardiomyopathy. Cardiomyopathy is segmented into primary cardiomyopathy and secondary cardiomyopathy (Burke et al., 2016; Brieler et al., 2017). Primary cardiomyopathy includes dilated cardiomyopathy, hypertrophic cardiomyopathy, restrictive cardiomyopathy, and indeterminate cardiomyopathy while secondary cardiomyopathy involves cardiomyopathy caused by systemic disease. This article focuses on the role of macrophages in three types of cardiomyopathy: ischemic cardiomyopathy, dilated cardiomyopathy, and diabetic cardiomyopathy.
Ischemic cardiomyopathy (ICM), a special type of an advanced stage of coronary heart disease, is a long-term myocardial ischemia caused by coronary atherosclerosis. It results in diffuse myocardial fibrosis and a disease-like clinical syndrome. With the increasing incidence of coronary heart disease, the harm caused by ICM to human health is becoming more and more serious. Myocardial infarction and myocardial defect-reperfusion injury are major causes of ICM. Ischemic damage occurs in the areas oxygenated by blocked arteries in the heart. Thrombolysis and percutaneous coronary intervention are usually used clinically to restore coronary blood flow. However, ischemia/reperfusion injury is still a common complication (Fan et al., 2019). This article focuses on the interaction between IR and macrophages.
Peripheral monocyte mobilization and infiltration in cardiovascular disease is primarily considered a maladaptive response associated with adverse outcomes including infarct dilation, left ventricular systolic dysfunction, and formation of atherosclerotic plaques (Bajpai et al., 2019). Several evidences have shown that the immune response has a central role in IR injury, characterized by the recruitment and activation of the innate and adaptive immune cells (Fan et al., 2019; Wei et al., 2019). For example, peripheral blood CD14+ monocyte abundance is associated with larger infarct size and worsening of left ventricular systolic function in myocardial infarction (Kaczorowski et al., 2009).
CCR2+ macrophages increase MyD88-dependent CCL2 production in mice, thus promoting monocyte recruitment. In contrast, CCR2-resident macrophages impair monocyte recruitment (Li et al., 2016). Expression profiling has shown that CCR2+ macrophages are required for neutrophil extravasation into the myocardium (Li et al., 2016). Furthermore, studies have shown that the expression of type I interferon-stimulated genes in resident CCR2-macrophages after myocardial injury is significantly different compared with recruited CCR2+ macrophages, suggesting that CCR2-resident macrophages are responsible for the production of myocardial infarction during myocardial infarction (Calcagno et al., 2020). The Ly6Chi population associated with the M1 pro-inflammatory macrophage phenotype is recruited through increased CCL2/CCR2 chemokine/monocyte receptor interaction and endothelial adhesion molecule expression in the early stage of MI inflammation (Duncan et al., 2020). The late Ly6Clow population associated with the M2 ″healing” phenotype infiltrated the myocardium between days 4 and 7 post-MI(54). These Ly6Clow macrophages are reparative and non-inflammatory and can promote myofibroblast accumulation, angiogenesis, and collagen deposition. Inhibition of monocyte recruitment by blocking the CCL2 and CCR2 signaling pathways reduces excessive inflammation, myocardial infarction and atherosclerosis is protective in a sclerosis-like mouse model (Tsou et al., 2007).
Dilated cardiomyopathy is a primary heart muscle disease with an unknown cause. The disease is characterized by left or right ventricle or bilateral ventricular enlargement and ventricular systolic hypofunction, with or without congestive heart failure (Japp et al., 2016). The disease is caused by various factors, including genetic factors, viral infections, and autoimmune phenomena. Heart transplantation is the only treatment for the disease. However, it is also difficult to find the heart. The adverse reactions, such as postoperative anti-rejection reactions and decreased renal function, also affect patient survival. Therefore, understanding the pathophysiological process of dilated cardiomyopathy is necessary to explore new treatment methods and control its occurrence and development in the early stage. This article focused on the relationship between doxorubicin-mediated dilated cardiomyopathy and macrophages.
Doxorubicin-induced cardiomyopathy (DIC) is characterized by decreased contractility, rhythmic relaxation, and adverse cardiac remodeling (Chatterjee et al., 2010). Cardiomyocyte apoptosis, fibrosis, hypertrophy, and cell vacuolization are the most classic physiological and pathological changes of cardiomyopathy caused by adriamycin (Lipshultz et al., 1991; Zhao et al., 2018). Doxorubicin causes cardiomyocyte death through DNA damage and production of reactive oxygen species (ROS), which trigger mitochondrial dysfunction (Enomoto et al., 2021). Cardiomyocytes contain several mitochondria, thus providing metabolic energy and mechanical demands for the heart to eliminate dysfunctional mitochondria, thus maintaining a healthy state of cardiomyocytes (Garbern and Lee, 2021). Depletion of cardiac macrophages affects mitochondrial elimination, accumulation of abnormal mitochondria in myocardial tissue, activation of inflammasomes, metabolism, and ventricular dysfunction (Nicolas-Avila et al., 2020).
Macrophages in non-ischemic cardiomyopathy respond to injury-related molecular patterns and chemokine signaling, then differentiate into defined phenotypes guided by local cytokine signaling. Resident cardiac macrophages are associated with adaptive cardiac response to stress overload (Epelman et al., 2014b). Recent studies have suggested that DIC may be involved in M1 macrophage infiltration, induction of inflammation, and pathogenesis. For instance, Qi Chen suggested that peripheral pro-inflammatory monocyte-derived cardiac macrophages predominate during DIC. However, cardiac macrophages eventually mobilize and self-renew in response to doxorubicin and reduce adverse cardiac remodeling (Zhang et al., 2020).
Although a good beginning has been made in the study of macrophages in the DIC, there are still some important problems to be solved. This includes how to adjust the immune strategy to respond to the activation of recruited macrophages and the survival of cardiac resident macrophages.
Diabetic cardiomyopathy occurs in diabetic patients. It leads to extensive focal necrosis of the myocardium, eventually causing heart failure, arrhythmia, and cardiogenic shock (Dillmann, 2019). Its pathogenesis is mainly manifested as chronic hyperglycemia, insulin resistance, and chronic inflammation. Higher white blood cell counts can predict the risk of cardiovascular disease in diabetic patients, suggesting that these cells play a key role in the worsening of diabetes-related cardiovascular disease (Zhang et al., 2019).
Diabetic patients have increased immune cell infiltration and monocyte activity (Morano et al., 2007; Wu et al., 2020). The heart is primarily dependent on glycolysis during decompensated heart failure. Its central failure accounts for the activation of HIF-1α, which induces glycolysis and transcription of pro-inflammatory genes (Holscher et al., 2012). Macrophages can lead to protein glycation and advanced glycation end products due to excess glucose levels, activating the NF-κB pathway and the production of inflammatory cytokines, leading to microvascular and macrovascular complications (Volz et al., 2010; Bezold et al., 2019). Moreover, blood glucose level elevation increases superoxide production, which may directly lead to cell damage (Iannantuoni et al., 2020).
Myocardial injury causes myocardial fibrosis. Myocardial injury makes fibroblasts overactive, and thus their secreted extracellular matrix is excessively deposited in the cardiac interstitium and around blood vessels. As a result, it leads to myocardial wall thickening, contraction, relaxation and dysfunction, thus reducing cardiac function (Fan et al., 2012). Fibroblasts are key in the homeostasis of the extracellular matrix under physiological conditions. The extracellular matrix provides a structural scaffold for cardiomyocytes. The extracellular matrix also wraps the cardiomyocytes and stabilizes the mechanical force and electrical conduction between the cardiomyocytes. Way to ensure the structural and functional integrity of the heart (Camelliti et al., 2005; Porter and Turner, 2009; Souders et al., 2009). Pathophysiological stimuli, such as inflammation and pressure overload, can also cause myocardial injury. For instance, the massive death of myocardial cells triggers a strong inflammatory response. As a result, fibroblasts are rapidly activated and transformed into fibroblasts, which secrete several extracellular cells (Ivey et al., 2018). Matrix protein forms a collagen-based scar to replace cardiomyocytes to prevent rupture of the heart, thus protecting heart function. However, prolonged or excessive fibrosis can lead to reduced cardiac compliance, left ventricular systolic and diastolic dysfunction, and eventually congestive heart failure.
Macrophages play a key role in cardiac remodeling. Myocardial injury recruits several subsets of peripheral blood monocytes/macrophages differentiated from the bone marrow or spleen, including pro- and anti-inflammatory cells. These cells secrete pro- or anti-inflammatory factors, pro-angiogenic factors, pro-fibrotic repair factors, and promote the repair response of the heart by phagocytosing and removing necrotic or apoptotic cardiomyocytes (Aurora et al., 2014; Lavine et al., 2014; Hulsmans et al., 2018). The degree of inflammation is significantly associated with the severity of heart failure. Pro-inflammatory macrophages mainly secrete a large number of pro-inflammatory cytokines and chemokines such as IL-6, IL-1β, in the early stage of myocardial infarction. As a result, anti-inflammatory/reparative macrophages dominate by secreting high levels of anti-inflammatory and angiogenic factors, such as vascular endothelial growth factor (VEGF), fibroblast growth factor 2 (FGF2), and transforming growth factor-beta (TGF-β), promoting cardiac repair and maintain cardiac structural integrity by inducing myofibroblast activation, collagen deposition, and development of new blood vessels (Nahrendorf et al., 2007; Gombozhapova et al., 2017; Mouton et al., 2018). Recent studies have shown that macrophages can suppress excessive cardiac fibrosis by secreting oncostatin-m (OSM) through hypoxic signaling (Abe et al., 2019). Activation of the YAP/TAZ pathway enhances macrophage-mediated fibrosis and tissue remodeling in cardiomyocytes (Mia et al., 2020). These evidences further demonstrate the role of macrophage-mediated fibrosis after myocardial injury.
Besides recruited macrophages, resident macrophages in the heart also play a key role in the fibrosis process after myocardial injury. Studies have shown that cardiac resident CCR2-macrophages can promote cardiac recovery by promoting the proliferation of cardiomyocytes and angiogenesis (Lavine et al., 2014). However, they are replaced by recruited pro-inflammatory macrophages after myocardial injury and lack repair activity. Recent studies have demonstrated that cardiac resident CCR2-macrophages stimulate angiogenesis while inhibiting fibrosis during cardiac pressure overload (Revelo et al., 2021). Similarly, miRNA21 in macrophages can promote fibroblast activation in a heart failure model via paracrine, leading to myocardial fibrosis (Verma et al., 2017). However, the specific molecular mechanism of the inhibitory effect of cardiac resident CCR2-macrophages on fibrosis requires further research.
The phenotypic switch of resident-macrophages and monocyte-derived macrophages plays a key role in the development and progression in both ischemic and non-ischemic cardiomyopathy (Figure 2). M1-like macrophages exacerbate the inflammation to lead to HF, M2-like macrophages suppress the inflammation. Notably, M2-like macrophages slowly increased. In addition, M1-like and M2-like macrophages rely on the glycolytic and oxidative phosphorylation. Both types of macrophages interact with cardiac other cells such as fibroblasts cells to control disease progression. Thus, regulation of phenotypic transformation of macrophages could be a potential therapeutic target for cardiomyopathy.
FIGURE 2. Functions and phenotype of macrophages during infection and tissue repair. When cardiomyocytes undergo injury, the heart recruit peripheral monocyte to differentiate M1 macrophages. M1 macrophages will activate CCL2 and NF-κB and promote TNF-α, IL-1β, and IL-6 to inhibit cardiac repair and aggravate cardiomyocytes necrosis. As time goes on, M1 macrophages can transform M2 macrophages through CB2 receptor axis. M2 macrophages can inhibit TNF-α, IL-1β, and IL-6 to promote cardiac repair. Meanwhile, M2 macrophages can promote angiogenesis and fibroblast formation. Cardiac resident-macrophages have the same effect as M2 macrophages.
Duerr et al. demonstrated that the endocannabinoid CB2 receptor axis protects the ischemic heart by modulating macrophage polarization (M1 to M2), reducing the inflammation and adverse cardiac remodeling after myocardial ischemia-reperfusion (Duerr et al., 2014). Qi Chen et al. also demonstrated that the SR-A1-c-Myc axis may be a promising target for the treatment of DIC by enhancing cardiac repair macrophage proliferation (Zhang et al., 2020). Macrophage migration inhibitory factor is a crucial cardioprotective factor against DIC by promoting autophagolysosome formation. GHSR deficiency aggravates inflammasome in macrophages. It also aggravated ISO-induced myocardial fibrosis and the degree of heart failure, suggesting that GHSR is a potential target for intervention in myocardial fibrosis (Wang et al., 2020). Therefore, controlling the transformation of macrophage phenotype can treat the myocardial injury.
Significant advances have been made in immune system concerning cardiovascular disease in recent years, especially in maintaining cardiac function. The role of macrophages, as a key immune cell, in cardiomyopathy has been extensively studied. Scientists have found that macrophages play a crucial role in myocardial inflammation and fibrosis, and thus may become a new therapeutic target for cardiomyopathy. However, both the recruitment of peripheral macrophages and the response of cardiac resident macrophages depend on the source of the myocardial damage signal. Immune cells activate fibroblasts to maintain impaired heart function. However, sustained cardiac injury continuously promotes extracellular matrix production, leading to decreased cardiac function and heart failure. Although phenotypic heterogeneity and plasticity of macrophages have been reported in cardiac disease for a decade, the role of M2 macrophages in cardiomyopathy is not fully understood. Therefore, a better understanding of the phenotype, function of macrophages in the cardiomyopathy damage and repair may enhance the development of new therapeutic approaches.
GC, HJ, and YY searched the relative papers and writed this manuscript. ZT and WC helped to revise the manuscript. FH and XC gave their professional suggestions and supervisd and edited this paper.
The current study was supported by the National Natural Science Foundation of China [No. 81870193].
We would like to thank the authors from the Department of Thoracic and Cardiovascular Surgery at Nanjing First Hospital and Shanghai Pulmonary Hospital for their contributions to this study.
The authors declare that the research was conducted in the absence of any commercial or financial relationships that could be construed as a potential conflict of interest.
All claims expressed in this article are solely those of the authors and do not necessarily represent those of their affiliated organizations, or those of the publisher, the editors and the reviewers. Any product that may be evaluated in this article, or claim that may be made by its manufacturer, is not guaranteed or endorsed by the publisher.
Abe, H., Takeda, N., Isagawa, T., Semba, H., Nishimura, S., Morioka, M. S., et al. (2019). Macrophage hypoxia signaling regulates cardiac fibrosis via Oncostatin M. Nat. Commun. 10 (1), 2824. doi:10.1038/s41467-019-10859-w
Ammirati, E., Frigerio, M., Adler, E. D., Basso, C., Birnie, D. H., Brambatti, M., et al. (2020). Management of acute myocarditis and chronic inflammatory cardiomyopathy: An expert consensus document. Circ. Heart Fail. 13 (11), e007405. doi:10.1161/CIRCHEARTFAILURE.120.007405
Aurora, A. B., Porrello, E. R., Tan, W., Mahmoud, A. I., Hill, J. A., Bassel-Duby, R., et al. (2014). Macrophages are required for neonatal heart regeneration. J. Clin. Invest. 124 (3), 1382–1392. doi:10.1172/JCI72181
Bajpai, G., Bredemeyer, A., Li, W., Zaitsev, K., Koenig, A. L., Lokshina, I., et al. (2019). Tissue resident CCR2- and CCR2+ cardiac macrophages differentially orchestrate monocyte recruitment and fate specification following myocardial injury. Circ. Res. 124 (2), 263–278. doi:10.1161/CIRCRESAHA.118.314028
Bajpai, G., Schneider, C., Wong, N., Bredemeyer, A., Hulsmans, M., Nahrendorf, M., et al. (2018). The human heart contains distinct macrophage subsets with divergent origins and functions. Nat. Med. 24 (8), 1234–1245. doi:10.1038/s41591-018-0059-x
Barin, J. G., Baldeviano, G. C., Talor, M. V., Wu, L., Ong, S., Quader, F., et al. (2012). Macrophages participate in IL-17-mediated inflammation. Eur. J. Immunol. 42 (3), 726–736. doi:10.1002/eji.201141737
Bezold, V., Rosenstock, P., Scheffler, J., Geyer, H., Horstkorte, R., and Bork, K. (2019). Glycation of macrophages induces expression of pro-inflammatory cytokines and reduces phagocytic efficiency. Aging (Albany NY) 11 (14), 5258–5275. doi:10.18632/aging.102123
Brieler, J., Breeden, M. A., and Tucker, J. (2017). Cardiomyopathy: An overview. Am. Fam. Physician 96 (10), 640–646.
Burke, M. A., Cook, S. A., Seidman, J. G., and Seidman, C. E. (2016). Clinical and mechanistic insights into the genetics of cardiomyopathy. J. Am. Coll. Cardiol. 68 (25), 2871–2886. doi:10.1016/j.jacc.2016.08.079
Calcagno, D. M., Ng, R. P., Toomu, A., Zhang, C., Huang, K., Aguirre, A. D., et al. (2020). The myeloid type I interferon response to myocardial infarction begins in bone marrow and is regulated by Nrf2-activated macrophages. Sci. Immunol. 5 (51), eaaz1974. doi:10.1126/sciimmunol.aaz1974
Camelliti, P., Borg, T. K., and Kohl, P. (2005). Structural and functional characterisation of cardiac fibroblasts. Cardiovasc. Res. 65 (1), 40–51. doi:10.1016/j.cardiores.2004.08.020
Chatterjee, K., Zhang, J., Honbo, N., and Karliner, J. S. (2010). Doxorubicin cardiomyopathy. Cardiology 115 (2), 155–162. doi:10.1159/000265166
Crespo-Leiro, M. G., Metra, M., Lund, L. H., Milicic, D., Costanzo, M. R., Filippatos, G., et al. (2018). Advanced heart failure: A position statement of the heart failure association of the European society of cardiology. Eur. J. Heart Fail. 20 (11), 1505–1535. doi:10.1002/ejhf.1236
Dick, S. A., Macklin, J. A., Nejat, S., Momen, A., Clemente-Casares, X., Althagafi, M. G., et al. (2019). Self-renewing resident cardiac macrophages limit adverse remodeling following myocardial infarction. Nat. Immunol. 20 (1), 29–39. doi:10.1038/s41590-018-0272-2
Dillmann, W. H. (2019). Diabetic cardiomyopathy. Circ. Res. 124 (8), 1160–1162. doi:10.1161/CIRCRESAHA.118.314665
Duerr, G. D., Heinemann, J. C., Suchan, G., Kolobara, E., Wenzel, D., Geisen, C., et al. (2014). The endocannabinoid-CB2 receptor axis protects the ischemic heart at the early stage of cardiomyopathy. Basic Res. Cardiol. 109 (4), 425. doi:10.1007/s00395-014-0425-x
Duncan, S. E., Gao, S., Sarhene, M., Coffie, J. W., Linhua, D., Bao, X., et al. (2020). Macrophage activities in myocardial infarction and heart failure. Cardiol. Res. Pract. 2020, 4375127. doi:10.1155/2020/4375127
Enomoto, H., Mittal, N., Inomata, T., Arimura, T., Izumi, T., Kimura, A., et al. (2021). Dilated cardiomyopathy-linked heat shock protein family D member 1 mutations cause up-regulation of reactive oxygen species and autophagy through mitochondrial dysfunction. Cardiovasc. Res. 117 (4), 1118–1131. doi:10.1093/cvr/cvaa158
Epelman, S., Lavine, K. J., Beaudin, A. E., Sojka, D. K., Carrero, J. A., Calderon, B., et al. (2014). Embryonic and adult-derived resident cardiac macrophages are maintained through distinct mechanisms at steady state and during inflammation. Immunity 40 (1), 91–104. doi:10.1016/j.immuni.2013.11.019
Epelman, S., Lavine, K. J., and Randolph, G. J. (2014). Origin and functions of tissue macrophages. Immunity 41 (1), 21–35. doi:10.1016/j.immuni.2014.06.013
Fan, D., Takawale, A., Lee, J., and Kassiri, Z. (2012). Cardiac fibroblasts, fibrosis and extracellular matrix remodeling in heart disease. Fibrogenes. Tissue Repair 5 (1), 15. doi:10.1186/1755-1536-5-15
Fan, Q., Tao, R., Zhang, H., Xie, H., Lu, L., Wang, T., et al. (2019). Dectin-1 contributes to myocardial ischemia/reperfusion injury by regulating macrophage polarization and neutrophil infiltration. Circulation 139 (5), 663–678. doi:10.1161/CIRCULATIONAHA.118.036044
Ferrante, C. J., Pinhal-Enfield, G., Elson, G., Cronstein, B. N., Hasko, G., Outram, S., et al. (2013). The adenosine-dependent angiogenic switch of macrophages to an M2-like phenotype is independent of interleukin-4 receptor alpha (IL-4Rα) signaling. Inflammation 36 (4), 921–931. doi:10.1007/s10753-013-9621-3
Frangogiannis, N. G. (2019). Cardiac fibrosis: Cell biological mechanisms, molecular pathways and therapeutic opportunities. Mol. Asp. Med. 65, 70–99. doi:10.1016/j.mam.2018.07.001
Garbern, J. C., and Lee, R. T. (2021). Mitochondria and metabolic transitions in cardiomyocytes: Lessons from development for stem cell-derived cardiomyocytes. Stem Cell Res. Ther. 12 (1), 177. doi:10.1186/s13287-021-02252-6
Golovkin, A., Kalinina, O., Bezrukikh, V., Aquino, A., Zaikova, E., Karonova, T., et al. (2021). Imbalanced immune response of T-cell and B-cell subsets in patients with moderate and severe COVID-19. Viruses 13 (10), 1966. doi:10.3390/v13101966
Gombozhapova, A., Rogovskaya, Y., Shurupov, V., Rebenkova, M., Kzhyshkowska, J., Popov, S. V., et al. (2017). Macrophage activation and polarization in post-infarction cardiac remodeling. J. Biomed. Sci. 24 (1), 13. doi:10.1186/s12929-017-0322-3
Hatmal, M. M., Alshaer, W., Al-Hatamleh, M. A. I., Hatmal, M., Smadi, O., Taha, M. O., et al. (2020). Comprehensive structural and molecular comparison of spike proteins of SARS-CoV-2, SARS-CoV and MERS-CoV, and their interactions with ACE2. Cells 9 (12), E2638. doi:10.3390/cells9122638
Heinrichs, M., Ashour, D., Siegel, J., Buchner, L., Wedekind, G., Heinze, K. G., et al. (2021). The healing myocardium mobilizes a distinct B-cell subset through a CXCL13-CXCR5-dependent mechanism. Cardiovasc. Res. 117 (13), 2664–2676. doi:10.1093/cvr/cvab181
Hogg, C., Horne, A. W., and Greaves, E. (2020). Endometriosis-associated macrophages: Origin, phenotype, and function. Front. Endocrinol. 11, 7. doi:10.3389/fendo.2020.00007
Holscher, M., Schafer, K., Krull, S., Farhat, K., Hesse, A., Silter, M., et al. (2012). Unfavourable consequences of chronic cardiac HIF-1α stabilization. Cardiovasc. Res. 94 (1), 77–86. doi:10.1093/cvr/cvs014
Honold, L., and Nahrendorf, M. (2018). Resident and monocyte-derived macrophages in cardiovascular disease. Circ. Res. 122 (1), 113–127. doi:10.1161/CIRCRESAHA.117.311071
Hulsmans, M., Sager, H. B., Roh, J. D., Valero-Munoz, M., Houstis, N. E., Iwamoto, Y., et al. (2018). Cardiac macrophages promote diastolic dysfunction. J. Exp. Med. 215 (2), 423–440. doi:10.1084/jem.20171274
Iannantuoni, F., MdM., A., Abad-Jimenez, Z., Canet, F., Diaz-Pozo, P., Lopez-Domenech, S., et al. (2020). Mitochondrial alterations and enhanced human leukocyte/endothelial cell interactions in type 1 diabetes. J. Clin. Med. 9 (7), E2155. doi:10.3390/jcm9072155
Ivey, M. J., Kuwabara, J. T., Pai, J. T., Moore, R. E., Sun, Z., and Tallquist, M. D. (2018). Resident fibroblast expansion during cardiac growth and remodeling. J. Mol. Cell. Cardiol. 114, 161–174. doi:10.1016/j.yjmcc.2017.11.012
Japp, A. G., Gulati, A., Cook, S. A., Cowie, M. R., and Prasad, S. K. (2016). The diagnosis and evaluation of dilated cardiomyopathy. J. Am. Coll. Cardiol. 67 (25), 2996–3010. doi:10.1016/j.jacc.2016.03.590
Jetten, N., Verbruggen, S., Gijbels, M. J., Post, M. J., De Winther, M. P., and Donners, M. M. (2014). Anti-inflammatory M2, but not pro-inflammatory M1 macrophages promote angiogenesis in vivo. Angiogenesis 17 (1), 109–118. doi:10.1007/s10456-013-9381-6
Jum'ah, H., Kundrapu, S., Jabri, A., Kondapaneni, M., Tomashefski, J. F., and Loeffler, A. G. (2022). Cardiac macrophage density in covid-19 infection: Relationship to myocyte necrosis and acute lung injury. Cardiovasc. Pathol. 60, 107447. doi:10.1016/j.carpath.2022.107447
Kaczorowski, D. J., Nakao, A., Vallabhaneni, R., Mollen, K. P., Sugimoto, R., Kohmoto, J., et al. (2009). Mechanisms of Toll-like receptor 4 (TLR4)-mediated inflammation after cold ischemia/reperfusion in the heart. Transplantation 87 (10), 1455–1463. doi:10.1097/TP.0b013e3181a36e5e
Lavine, K. J., Epelman, S., Uchida, K., Weber, K. J., Nichols, C. G., Schilling, J. D., et al. (2014). Distinct macrophage lineages contribute to disparate patterns of cardiac recovery and remodeling in the neonatal and adult heart. Proc. Natl. Acad. Sci. U. S. A. 111 (45), 16029–16034. doi:10.1073/pnas.1406508111
Lee, C. G., Homer, R. J., Zhu, Z., Lanone, S., Wang, X., Koteliansky, V., et al. (2001). Interleukin-13 induces tissue fibrosis by selectively stimulating and activating transforming growth factor beta(1). J. Exp. Med. 194 (6), 809–821. doi:10.1084/jem.194.6.809
Lee, C. Y., Huang, C. H., Rastegari, E., Rengganaten, V., Liu, P. C., Tsai, P. H., et al. (2021). Tumor necrosis factor-alpha exacerbates viral entry in SARS-CoV2-infected iPSC-derived cardiomyocytes. Int. J. Mol. Sci. 22 (18), 9869. doi:10.3390/ijms22189869
Li, W., Hsiao, H. M., Higashikubo, R., Saunders, B. T., Bharat, A., Goldstein, D. R., et al. (2016). Heart-resident CCR2(+) macrophages promote neutrophil extravasation through TLR9/MyD88/CXCL5 signaling. JCI Insight 1 (12), 87315. doi:10.1172/jci.insight.87315
Lipshultz, S. E., Colan, S. D., Gelber, R. D., Perez-Atayde, A. R., Sallan, S. E., and Sanders, S. P. (1991). Late cardiac effects of doxorubicin therapy for acute lymphoblastic leukemia in childhood. N. Engl. J. Med. 324 (12), 808–815. doi:10.1056/NEJM199103213241205
Luo, H., Wong, J., and Wong, B. (2010). Protein degradation systems in viral myocarditis leading to dilated cardiomyopathy. Cardiovasc. Res. 85 (2), 347–356. doi:10.1093/cvr/cvp225
Martinez, F. O., Sica, A., Mantovani, A., and Locati, M. (2008). Macrophage activation and polarization. Front. Biosci. 13, 453–461. doi:10.2741/2692
McKenna, W. J., Maron, B. J., and Thiene, G. (2017). Classification, epidemiology, and global burden of cardiomyopathies. Circ. Res. 121 (7), 722–730. doi:10.1161/CIRCRESAHA.117.309711
Mia, M. M., Cibi, D. M., Abdul Ghani, S. A. B., Song, W., Tee, N., Ghosh, S., et al. (2020). YAP/TAZ deficiency reprograms macrophage phenotype and improves infarct healing and cardiac function after myocardial infarction. PLoS Biol. 18 (12), e3000941. doi:10.1371/journal.pbio.3000941
Morano, S., Gatti, A., Mandosi, E., Tiberti, C., Fallarino, M., Cipriani, R., et al. (2007). Circulating monocyte oxidative activity is increased in patients with type 2 diabetes and erectile dysfunction. J. Urol. 177 (2), 655–659. doi:10.1016/j.juro.2006.09.046
Mosser, D. M., and Edwards, J. P. (2008). Exploring the full spectrum of macrophage activation. Nat. Rev. Immunol. 8 (12), 958–969. doi:10.1038/nri2448
Mosser, D. M. (2003). The many faces of macrophage activation. J. Leukoc. Biol. 73 (2), 209–212. doi:10.1189/jlb.0602325
Mouton, A. J., DeLeon-Pennell, K. Y., Rivera Gonzalez, O. J., Flynn, E. R., Freeman, T. C., Saucerman, J. J., et al. (2018). Mapping macrophage polarization over the myocardial infarction time continuum. Basic Res. Cardiol. 113 (4), 26. doi:10.1007/s00395-018-0686-x
Mukhopadhyay, S., Peiser, L., and Gordon, S. (2004). Activation of murine macrophages by Neisseria meningitidis and IFN-gamma in vitro: Distinct roles of class A scavenger and toll-like pattern recognition receptors in selective modulation of surface phenotype. J. Leukoc. Biol. 76 (3), 577–584. doi:10.1189/jlb.0104014
Nahrendorf, M., Swirski, F. K., Aikawa, E., Stangenberg, L., Wurdinger, T., Figueiredo, J. L., et al. (2007). The healing myocardium sequentially mobilizes two monocyte subsets with divergent and complementary functions. J. Exp. Med. 204 (12), 3037–3047. doi:10.1084/jem.20070885
Nicolas-Avila, J. A., Lechuga-Vieco, A. V., Esteban-Martinez, L., Sanchez-Diaz, M., Diaz-Garcia, E., Santiago, D. J., et al. (2020). A network of macrophages supports mitochondrial homeostasis in the heart. Cell 183 (1), 94–109. doi:10.1016/j.cell.2020.08.031
Nishiga, M., Wang, D. W., Han, Y., Lewis, D. B., and Wu, J. C. (2020). COVID-19 and cardiovascular disease: From basic mechanisms to clinical perspectives. Nat. Rev. Cardiol. 17 (9), 543–558. doi:10.1038/s41569-020-0413-9
O'Rourke, S. A., Dunne, A., and Monaghan, M. G. (2019). The role of macrophages in the infarcted myocardium: Orchestrators of ECM remodeling. Front. Cardiovasc. Med. 6, 101. doi:10.3389/fcvm.2019.00101
Peet, C., Ivetic, A., Bromage, D. I., and Shah, A. M. (2020). Cardiac monocytes and macrophages after myocardial infarction. Cardiovasc. Res. 116 (6), 1101–1112. doi:10.1093/cvr/cvz336
Perez-Bermejo, J. A., Kang, S., Rockwood, S. J., Simoneau, C. R., Joy, D. A., Ramadoss, G. N., et al. (2020). SARS-CoV-2 infection of human iPSC-derived cardiac cells predicts novel cytopathic features in hearts of COVID-19 patients. New York: bioRxiv.
Pollack, A., Kontorovich, A. R., Fuster, V., and Dec, G. W. (2015). Viral myocarditis--diagnosis, treatment options, and current controversies. Nat. Rev. Cardiol. 12 (11), 670–680. doi:10.1038/nrcardio.2015.108
Porter, K. E., and Turner, N. A. (2009). Cardiac fibroblasts: At the heart of myocardial remodeling. Pharmacol. Ther. 123 (2), 255–278. doi:10.1016/j.pharmthera.2009.05.002
Revelo, X. S., Parthiban, P., Chen, C., Barrow, F., Fredrickson, G., Wang, H., et al. (2021). Cardiac resident macrophages prevent fibrosis and stimulate angiogenesis. Circ. Res. 129 (12), 1086–1101. doi:10.1161/CIRCRESAHA.121.319737
Roth, G. A., Mensah, G. A., Johnson, C. O., Addolorato, G., Ammirati, E., Baddour, L. M., et al. (2020). Global burden of cardiovascular diseases and risk factors, 1990-2019: Update from the GBD 2019 study. J. Am. Coll. Cardiol. 76 (25), 2982–3021. doi:10.1016/j.jacc.2020.11.010
Sager, H. B., Hulsmans, M., Lavine, K. J., Moreira, M. B., Heidt, T., Courties, G., et al. (2016). Proliferation and recruitment contribute to myocardial macrophage expansion in chronic heart failure. Circ. Res. 119 (7), 853–864. doi:10.1161/CIRCRESAHA.116.309001
Sawai, C. M., Babovic, S., Upadhaya, S., Knapp, D., Lavin, Y., Lau, C. M., et al. (2016). Hematopoietic stem cells are the major source of multilineage hematopoiesis in adult animals. Immunity 45 (3), 597–609. doi:10.1016/j.immuni.2016.08.007
Shapouri-Moghaddam, A., Mohammadian, S., Vazini, H., Taghadosi, M., Esmaeili, S. A., Mardani, F., et al. (2018). Macrophage plasticity, polarization, and function in health and disease. J. Cell. Physiol. 233 (9), 6425–6440. doi:10.1002/jcp.26429
Sharma, A., Garcia, G., Arumugaswami, V., and Svendsen, C. N. (2020). Human iPSC-derived cardiomyocytes are susceptible to SARS-CoV-2 infection. New York: bioRxiv.
Sierra-Filardi, E., Vega, M. A., Sanchez-Mateos, P., Corbi, A. L., and Puig-Kroger, A. (2010). Heme Oxygenase-1 expression in M-CSF-polarized M2 macrophages contributes to LPS-induced IL-10 release. Immunobiology 215 (9-10), 788–795. doi:10.1016/j.imbio.2010.05.020
Souders, C. A., Bowers, S. L., and Baudino, T. A. (2009). Cardiac fibroblast: The renaissance cell. Circ. Res. 105 (12), 1164–1176. doi:10.1161/CIRCRESAHA.109.209809
Truby, L. K., and Rogers, J. G. (2020). Advanced heart failure: Epidemiology, diagnosis, and therapeutic approaches. JACC. Heart Fail. 8 (7), 523–536. doi:10.1016/j.jchf.2020.01.014
Tsou, C. L., Peters, W., Si, Y., Slaymaker, S., Aslanian, A. M., Weisberg, S. P., et al. (2007). Critical roles for CCR2 and MCP-3 in monocyte mobilization from bone marrow and recruitment to inflammatory sites. J. Clin. Invest. 117 (4), 902–909. doi:10.1172/JCI29919
van Furth, R., and Cohn, Z. A. (1968). The origin and kinetics of mononuclear phagocytes. J. Exp. Med. 128 (3), 415–435. doi:10.1084/jem.128.3.415
Varol, C., Mildner, A., and Jung, S. (2015). Macrophages: Development and tissue specialization. Annu. Rev. Immunol. 33, 643–675. doi:10.1146/annurev-immunol-032414-112220
Verma, S. K., Garikipati, V. N. S., Krishnamurthy, P., Schumacher, S. M., Grisanti, L. A., Cimini, M., et al. (2017). Interleukin-10 inhibits bone marrow fibroblast progenitor cell-mediated cardiac fibrosis in pressure-overloaded myocardium. Circulation 136 (10), 940–953. doi:10.1161/CIRCULATIONAHA.117.027889
Volz, H. C., Seidel, C., Laohachewin, D., Kaya, Z., Muller, O. J., Pleger, S. T., et al. (2010). HMGB1: The missing link between diabetes mellitus and heart failure. Basic Res. Cardiol. 105 (6), 805–820. doi:10.1007/s00395-010-0114-3
Wang, M., Qian, L., Li, J., Ming, H., Fang, L., Li, Y., et al. (2020). GHSR deficiency exacerbates cardiac fibrosis: Role in macrophage inflammasome activation and myofibroblast differentiation. Cardiovasc. Res. 116 (13), 2091–2102. doi:10.1093/cvr/cvz318
Wei, Z., Qiao, S., Zhao, J., Liu, Y., Li, Q., Wei, Z., et al. (2019). miRNA-181a over-expression in mesenchymal stem cell-derived exosomes influenced inflammatory response after myocardial ischemia-reperfusion injury. Life Sci. 232, 116632. doi:10.1016/j.lfs.2019.116632
Wu, W. Y., Zhou, X. J., Sun, P. P., Yu, X. J., Wang, S. X., Qu, L., et al. (2020). Interstitial eosinophilic infiltration in diabetic nephropathy is indicative of poor prognosis, with no therapy benefit from steroid. J. Diabetes 12 (12), 881–894. doi:10.1111/1753-0407.13077
Wynn, T. A., Chawla, A., and Pollard, J. W. (2013). Macrophage biology in development, homeostasis and disease. Nature 496 (7446), 445–455. doi:10.1038/nature12034
Wynn, T. A., and Vannella, K. M. (2016). Macrophages in tissue repair, regeneration, and fibrosis. Immunity 44 (3), 450–462. doi:10.1016/j.immuni.2016.02.015
Yang, L., Han, Y., Jaffre, F., Nilsson-Payant, B. E., Bram, Y., Wang, P., et al. (2021). An immuno-cardiac model for macrophage-mediated inflammation in COVID-19 hearts. Circ. Res. 129 (1), 33–46. doi:10.1161/CIRCRESAHA.121.319060
Zaninotto, M., Mion, M. M., Padoan, A., Babuin, L., and Plebani, M. (2020). Cardiac troponin I in SARS-CoV-2-patients: The additional prognostic value of serial monitoring. Clin. Chim. Acta. 511, 75–80. doi:10.1016/j.cca.2020.09.036
Zhang, F., Zhao, Q., Jiang, Y., Liu, N., Liu, Q., Shi, F. D., et al. (2019). Augmented brain infiltration and activation of leukocytes after cerebral ischemia in type 2 diabetic mice. Front. Immunol. 10, 2392. doi:10.3389/fimmu.2019.02392
Zhang, H., Xu, A., Sun, X., Yang, Y., Zhang, L., Bai, H., et al. (2020). Self-maintenance of cardiac resident reparative macrophages attenuates doxorubicin-induced cardiomyopathy through the SR-A1-c-myc Axis. Circ. Res. 127 (5), 610–627. doi:10.1161/CIRCRESAHA.119.316428
Zhang, Z., Tang, J., Cui, X., Qin, B., Zhang, J., Zhang, L., et al. (2021). New insights and novel therapeutic potentials for macrophages in myocardial infarction. Inflammation 44 (5), 1696–1712. doi:10.1007/s10753-021-01467-2
Zhao, J., Li, X., Hu, J., Chen, F., Qiao, S., Sun, X., et al. (2019). Mesenchymal stromal cell-derived exosomes attenuate myocardial ischaemia-reperfusion injury through miR-182-regulated macrophage polarization. Cardiovasc. Res. 115 (7), 1205–1216. doi:10.1093/cvr/cvz040
Zhao, J., Lv, T., Quan, J., Zhao, W., Song, J., Li, Z., et al. (2018). Identification of target genes in cardiomyopathy with fibrosis and cardiac remodeling. J. Biomed. Sci. 25 (1), 63. doi:10.1186/s12929-018-0459-8
Keywords: macrophage, heart failure, cardiomyopathy, phenotype, resident macrophages
Citation: Chen G, Jiang H, Yao Y, Tao Z, Chen W, Huang F and Chen X (2022) Macrophage, a potential targeted therapeutic immune cell for cardiomyopathy. Front. Cell Dev. Biol. 10:908790. doi: 10.3389/fcell.2022.908790
Received: 31 March 2022; Accepted: 15 September 2022;
Published: 30 September 2022.
Edited by:
Laura Iop, University of Padua, ItalyReviewed by:
Ienglam Lei, University of Michigan, United StatesCopyright © 2022 Chen, Jiang, Yao, Tao, Chen, Huang and Chen. This is an open-access article distributed under the terms of the Creative Commons Attribution License (CC BY). The use, distribution or reproduction in other forums is permitted, provided the original author(s) and the copyright owner(s) are credited and that the original publication in this journal is cited, in accordance with accepted academic practice. No use, distribution or reproduction is permitted which does not comply with these terms.
*Correspondence: Fuhua Huang, aHVhbmdmdWh1YUBzaW5hLmNu; Xin Chen, c3RldmVjeEBuam11LmVkdS5jbg==
Disclaimer: All claims expressed in this article are solely those of the authors and do not necessarily represent those of their affiliated organizations, or those of the publisher, the editors and the reviewers. Any product that may be evaluated in this article or claim that may be made by its manufacturer is not guaranteed or endorsed by the publisher.
Research integrity at Frontiers
Learn more about the work of our research integrity team to safeguard the quality of each article we publish.