- Biochemistry and Molecular Biology Dept., University of Texas Medical Branch, Galveston, TX, United States
The mechanisms that maintain genome stability are critical for preventing tumor progression. In the past decades, many strategies were developed for cancer treatment to disrupt the DNA repair machinery or alter repair pathway selection. Evidence indicates that alterations in nuclear phosphoinositide lipids occur rapidly in response to genotoxic stresses. This implies that nuclear phosphoinositides are an upstream element involved in DNA damage signaling. Phosphoinositides constitute a new signaling interface for DNA repair pathway selection and hence a new opportunity for developing cancer treatment strategies. However, our understanding of the underlying mechanisms by which nuclear phosphoinositides regulate DNA damage repair, and particularly the dynamics of those processes, is rather limited. This is partly because there are a limited number of techniques that can monitor changes in the location and/or abundance of nuclear phosphoinositide lipids in real time and in live cells. This review summarizes our current knowledge regarding the roles of nuclear phosphoinositides in DNA damage response with an emphasis on the dynamics of these processes. Based upon recent findings, there is a novel model for p53’s role with nuclear phosphoinositides in DNA damage response that provides new targets for synthetic lethality of tumors.
Introduction
Nuclear Localization of Phosphoinositides and Their Metabolism
The existence of phospholipids in the nucleus was demonstrated in 1965 and 1977 (Rose and Frenster, 1965; Manzoli et al., 1977). A significant change in our understanding of nuclear phosphoinositides occurred in 1983 when Smith and Wells showed early evidence that the phosphoinositide kinases that generate Phosphatidylinositol (4,5)-bisphosphate were present in highly purified nuclear envelopes (Smith and Wells, 1983; Smith and Wells, 1984). 32P-Labelling of phosphatidic acid (PA), PI(4)P and PI(4,5)P2 occurred when intact rat liver nuclei were isolated and incubated with [γ32P]-ATP. It indicated that phosphoinositides can be synthesized in the nucleus in the absence of cytosolic phosphoinositide kinases. Later, adventurous studies by Irvine and coworkers lead to the discovery of the generation of phosphoinositides in the nucleus (Cocco et al., 1987; Divecha et al., 1991). The discovery of nuclear phospholipid signaling has revolutionized our view of the processes regulated by phospholipids. Interestingly, a substantial pool of nuclear phosphoinositides does not appear to be associated with the nuclear membrane (Payrastre et al., 1992). These observations are helping to unravel another facet of phospholipid signaling in cells.
To date, all the polyphosphoinositides, except PI(3,5)P2, have been detected in the nucleus using various approaches (Jacobsen et al., 2019). Further evidence that nuclear phosphoinositides constitute a different pool than those in the cytoplasm comes from the acyl compositions of nuclear PI(4,5)P2 (34:1) and (36:1), which is different from that of PI(4,5)P2 in the plasma membrane (38:4) (Ogiso et al., 2010). This finding further strengthens the hypothesis that nuclear phosphoinositides are generated through routes different from those in the plasma membrane.
Intracellular phosphoinositide localization in human cells has been investigated through imaging approaches using purified pleckstrin homology (PH) domains and antibodies (Boronenkov et al., 1998; Osborne et al., 2001; Kalasova et al., 2016). While phosphoinositides are not subject to formaldehyde fixation, an earlier study investigated the subcellular phosphoinositide distribution using ultrathin cryo-sections (Watt et al., 2002). The labelling procedure was carried out close to 0°C which overcame the issue that phospholipids could not be fully immobilized through aldehyde fixation. While the highest proportion of labelling of PI(4,5)P2 was over the plasma membrane (≈40% of total), the labelling in the nucleus was substantial (17–21% of total) and was particularly concentrated on electron-dense patches of heterochromatin (Watt et al., 2002). There is a tendency for immunolabelling to under-report the amounts of PI(4,5)P2 since it likely detects only freely exposed PI(4,5)P2 and not necessarily PI(4,5)P2 already bound to proteins. These and other findings from cellular fractionation and radioisotope labeling do not answer how nuclear phosphoinositides regulate nuclear function and in what form they exist in the nucleus (Divecha et al., 1993; Irvine, 2003; Barlow et al., 2010).
The nuclear phosphoinositide level is not kept constant. Accumulating evidence suggests that it fluctuates throughout the cell cycle (Clarke et al., 2001), plus levels respond to growth factor treatment (Divecha et al., 1991; Martelli et al., 1991; Martelli et al., 2000), cellular stressors (Jones et al., 2006), DNA damage (Jones et al., 2006; Li et al., 2012; Wickramasinghe et al., 2013; Wang et al., 2017; Choi et al., 2019), and differentiation (Cocco et al., 1987; Divecha et al., 1995; Neri et al., 2002). In vivo changes in phosphoinositides are also observed in liver nuclei in response to partial hepatectomy (Banfić et al., 1993; Neri et al., 1997). In this review, we will focus on the roles of phosphoinositides in the DNA damage response and summarize their responses at multiple time scales.
Interactome Studies and General Functions
In an analysis of phosphoinositide-interacting proteins, the largest portion of these proteins identified were concentrated in the nucleus (Jungmichel et al., 2014). In total, 405 proteins were identified through a whole-cell stable isotope labeling with amino acids in cell culture (SILAC) proteome analysis that interacted with either PI(4)P, PI(4,5)P2 or PI(3,4,5)P3. A significant enrichment (p < 7.0 × 10−7) of phosphoinositide interactors was found in the nucleus in comparison to the total interactors among intracellular proteins (Jungmichel et al., 2014). Nearly 40% of them were either located in the nucleus or shuttled between nucleus and cytosol. In contrast, the density of cytosolic interactors was significantly lower compared to the intracellular distribution of overall proteins (p < 5.5 × 10−3). Lewis et al. characterized nuclear PI(4,5)P2 interactome and revealed that 168 out of 349 nuclear PI(4,5)P2-binding proteins isolated by neomycin extraction contained at least one phosphoinositide-binding domain, including plant homeodomain (PHD), PH or K/R-rich motifs (Lewis et al., 2011). It should be noted that enrichment of small nuclear ribonucleoproteins (snRNPs) are frequently found in such interactome studies and they typically colocalize with PI(4,5)P2 at nuclear speckles. A recent study from the same author(s) characterized the nuclear PI(3,4,5)P3 interactome that was composed of 179 proteins and identified new interaction partners associated with the nucleolus. A few DNA damage repair proteins including PARP1 were also identified (Mazloumi Gavgani et al., 2021). In an independent study, synthetic probes featuring PI(3,4,5)P3 headgroups with photoaffinity tags were used for covalently labeling PI(3,4,5)P3-binding proteins. An additional alkyne group in the probes allowed for conjugation with biotin that was used for further protein purification. This study revealed 265 PI(3,4,5)P3-interacting proteins. (Rowland et al., 2011). However, these two PI(3,4,5)P3 interactome studies shared few consensus proteins. Such a discrepancy could partially be explained by the difference in their approaches. In the work by Rowland et al., the synthetic probes were fed to the cells and UV crosslinked to capture any transient binding. This approach did not rely on protein-membrane interactions and could capture isolated protein-lipid complexes. In contrast, Gavgani et al. used phosphoinositide-coated beads for protein enrichment. The phosphoinositide lipids were in a membranous form, and might not have been able to capture freely diffusing protein-lipid complexes because of the lipid configuration. Another possible explanation is, however, the bifunctionally modified lipids failed to mimic natural phosphoinositides in their interactions with nuclear proteins. Although consensus on the nuclear phosphoinositide interactome has remained elusive, recent findings indicate that many phosphoinositide interactors largely reside in the nucleus.
Nuclear phosphoinositides potentially regulate nuclear functions by altering protein-protein or protein-DNA interactions (Viiri et al., 2012). Compared to the known physiological functions of phosphoinositides in the plasma membrane, very little is known about the role of phosphoinositide-protein interactions in the nucleus. Nuclear PI(4,5)P2 is central to nuclear phosphoinositide signaling not only because it is a second messenger but also because it interacts with a broad spectrum of nuclear proteins. The vast majority of nuclear PI(4,5)P2-interacting proteins contained lysine/arginine-rich patches with the following motif, K/R-(Xn=3–7)-K-X-K/R-K/R, while a smaller subset of proteins contain known phosphoinositide-binding modules such as PH or PHD modules (Lewis et al., 2011). An analysis of over-represented biological processes for the PI(4,5)P2-interacting proteins, compared to all proteins annotated to the nucleus compartment, points to roles of nuclear PI(4,5)P2-interacting proteins in mRNA transcription regulation, mRNA splicing and protein folding (Lewis et al., 2011). So far, nuclear phosphoinositides have been shown to regulate many nuclear functions including transcription (Yu et al., 1998; Ulicna et al., 2018), splicing (Boronenkov et al., 1998; Osborne et al., 2001), export (York et al., 1999; Wickramasinghe et al., 2013) of mRNAs, as well as responses to genotoxic stress (Jones et al., 2013; Wang et al., 2017; Choi et al., 2019). Many facets of nuclear phosphoinositide functions have been widely reviewed (Fiume et al., 2012; Shah et al., 2013; Castano et al., 2019; Fiume et al., 2019; Jacobsen et al., 2019; Chen et al., 2020). In the following section, we will go through a brief summary of phosphoinositide-protein interactions and their functions in nucleus.
Nuclear PI(4,5)P2 appears to be involved in several important nuclear functions. First, nuclear phosphoinositides may regulate nuclear protein functions by altering protein-chromatin interactions (reviewed elsewhere (Hamann and Blind, 2018)). For example, PI(4,5)P2 interacts with histone H1 and disrupts its ability to suppress basal transcription by RNA polymerase in vitro (Yu et al., 1998). The interaction of PI(4,5)P2 with histone H1 is abolished upon Protein Kinase C (PKC)-mediated phosphorylation of H1. In addition, PI(4,5)P2 promotes chromatin association of the SWI/SNF-like chromatin remodeling complex BAF (Brahma-related gene association factor) upon lymphocyte activation (Zhao et al., 1998). BAF complex contains β-actin, BAF53 (an actin-related protein), and the ATPase subunit, BRG1. PI(4,5)P2 binding to the purified BAF complex subunit BRG1 stabilizes the complex by increasing its interaction with nuclear matrix and allows the complex to bind actin filaments (Rando et al., 2002). PI(4,5)P2 also interacts directly with the DNA Topoisomerase IIα (TopoIIα) and modulates TopoIIα decatenation activity in vitro (Lewis et al., 2011). Examples of nuclear PI(4,5)P2 mediated protein interaction with DNA are also found with the repression of transcription by WT1–BASP1, which requires the myristoylation of BASP1 and the PI(4,5)P2-dependent recruitment of HDAC1 (Toska et al., 2014). PI(4,5)P2 appears to be critical for forming protein-protein contacts between BASP1 and HDAC1. Both histone deacetylases (Gozani et al., 2003; Watson et al., 2012; Millard et al., 2013; Watson et al., 2016) and ATP-dependent chromatin remodeling activities (Zhao et al., 1998; Shen et al., 2003; Steger et al., 2003) require phosphoinositides or inositol phosphates for their activities.
In the case of PI(5)P, its binding to the PHD domain in ING2 protein promotes its association with chromatin (Gozani et al., 2003), whereas it inhibits chromatin-binding of another PHD-containing protein, ATX1 (Alvarez-Venegas et al., 2006; Ndamukong et al., 2010). PI(5)P also displaces the DNA from the zinc finger structure in SAP30 family members (Viiri et al., 2009). As an example, PI(3,4,5)P3 binds to the helix-turn-helix (HTH) structures in the homeobox (HOX) domains of HOXA5, HOXB6, HOXC6, and HOXD4. The competitive binding of phosphoinositides with target DNA sequences potentially modulates the transcription activities of these proteins (Bidlingmaier et al., 2011). They support the idea that the regulation of many nuclear proteins is mediated by their binding to phosphoinositides. Later, Divecha and Fischle et al. report two examples showing how PI(5)P could potentially regulate nuclear functions. In the first example, UHRF1 is shown to be allosterically regulated by PI(5)P for its interaction with unmodified histone H3 versus H3K9me3 by controlling their access to the PHD and tandem tudor domains (TTD), respectively (Gelato et al., 2014). In the second example, the basal transcriptional complex protein TAF3 is shown to directly bind to PI(5)P and transduce changes in nuclear phosphoinositides into differential transcriptional output that affects myoblast differentiation. The lipid kinase PIPKIIβ, phosphoinositides, and TAF3 form a conserved nuclear signaling pathway that selectively regulates transcription (Stijf-Bultsma et al., 2015).
Particularly interesting are the roles that nuclear phosphoinositides play in RNA function. PI(4,5)P2 interacts with the mRNA export protein, ALY, and thereby regulates selective mRNA export (Wickramasinghe et al., 2013). It also regulates RNA splicing and polyadenylation by interacting with RNA splicing complexes (Boronenkov et al., 1998; Bidlingmaier et al., 2011) and a non-canonical poly(A) polymerase, Star-PAP, also known as Tut1 (Mellman et al., 2008). Star-PAP modulates 3′-mRNA maturation and is strongly activated by nuclear PI(4,5)P2 (Mellman et al., 2008; Li et al., 2013; Li et al., 2017). Star-PAP interacts with PIPKIα through its C-terminus and colocalizes with PIPKIα at nuclear speckles (Mellman et al., 2008). In vitro, Star-PAP activity is dramatically stimulated by PI(4,5)P2, indicating that it is also a downstream target for nuclear PI(4,5)P2 signaling. These data indicate that the Star-PAP complex acts as a hub for nuclear PI(4,5)P2 signaling to control the response to oxidative stress by regulating the expression of PTEN (Li et al., 2017) and BIK, which is an important switch in the mitochondrial apoptosis pathway (Li et al., 2012).
Connections Between Nuclear Phosphoinositides and DNA Damage Responses
The first connection that linked nuclear phosphoinositides to DNA damage response came from changes in the level of nuclear phosphoinositides following DNA damage induction. An early study reported increased synthesis of nuclear phosphoinositides after DNA damage. They used [γ32P]-ATP incorporation into PI(4,5)P2 that was detected using thin layer chromotagraphy (TLC) of isolated nuclei and found that nuclear PI(4,5)P2 level doubled within the first hour after damage by ionizing radiation (IR), but slowly returned to normal 18 h later in murine erythroleukemia cells (Rana et al., 1994). It was also noted that the nuclear PI(4,5)P2 accumulation preceded the marked increase in DNA synthesis after irradiation. This finding indicated that there is involvement of nuclear inositol lipids in the cascade of the early events leading to the regulation of DNA repair in the nucleus. (Rana et al., 1994). The mass of nuclear PI(5)P also increased two to four fold within 30 min following increased genotoxic stress induced either by UVC (254 nm), H2O2 or etoposide, a topoisomerase inhibitor, as determined by a radioactive mass assay. Interestingly, nuclear PI(5)P level remained unchanged after being exposed to γ-irradiation (from a Caesium-137 source) for up to 30 min (Jones et al., 2006). Our work showed that nuclear PI(4,5)P2 levels increased by about 80% within minutes and lasted for an hour following UVC irradiation, which preceded the increase of γH2AX, as revealed by immunofluorescence staining (Wang et al., 2017). This finding was in accordance with an earlier finding that tert-Butylhydroquinone (tBHQ), an oxidative stress inducer that also caused DNA damage, specifically promoted the generation of PtdIns(4,5)P2 in the nuclear speckles (Mellman et al., 2008). More recently, the same group also reported an increase in the nuclear PI(4,5)P2 level through type I PI4P-5K activation with cisplatin-induced DNA damage 24 h after treatment (Choi et al., 2019). Together, these observations strengthened a potential role of nuclear phosphoinositides in response to genotoxic and oxidative stress. However, it was also noted that H2O2 treatment triggered a 5-fold increase of PI(5)P at a whole cell level, which was accompanied by a global increase of PI(4)P by 70% (Chen et al., 2009; Jones et al., 2013) and a global decrease of PI(4,5)P2 by 35–50% (Halstead et al., 2006; Chen et al., 2009). The decrease of overall PI(4,5)P2 was possibly due to spleen tyrosine kinase (Syk)-mediated phosphorylation and deactivation of PIP5Kβ (Chen et al., 2009). It was not clear how nuclear PI(4,5)P2 levels varied in these studies. However, H2O2 likely triggered a broader oxidative stress response that included but was not limited to DNA damage. Whether nuclear PtdIns(4,5)P2 levels respond differently to different DNA damage induction mechanisms remains to be investigated.
Another connection between nuclear phosphoinositide and DNA damage response came from proteomic interactome studies. A nuclear PI(4,5)P2 interactome study was carried out using SILAC. In this proteome analysis, neomycin was used to release PI(4,5)P2-binding proteins in the nuclear fraction that were then pulled down using PI(4,5)P2-coated beads (Lewis et al., 2011; Rowland et al., 2011). This approach led to the identification of 168 nuclear proteins harboring lysine/arginine-rich patches or phosphoinositide-binding domains. Similar studies were performed recently using PI(3,4,5)P3-coated beads (Mazloumi Gavgani et al., 2021). This led to an identification of a small set of DNA damage repair proteins; including proteins from various repair pathways. For example, the non-homologous end-joining (NHEJ) proteins XRCC5 (Ku80), DNA-PKcs (PRKDC), NBN (NBS1), and potentially RECQL were present. Further, this included the homologous recombination (HR) proteins NBN and potentially RECQL, the single strand break repair (SSBR) proteins PARP1 and LIG3, the base excision repair (BER) protein MPG, and the translesion synthesis (TLS) protein RECQL. More generally, nuclear PI(3,4,5)P3 might have broadly impacted DNA repair processes, even if it was not directly required for the repair. The discovery of PARP1 as a PI(3,4,5)P3-interacting protein was exciting, since PARP1 was considered as the first responder to DNA damage. It mediated poly-ADP ribosylation (PARylation) of DNA repair proteins which promoted the recruitment of many repair proteins to DNA damage sites. PARP-mediated PARylation also mediated the rapid recruitment of p53 to DNA damage sites, which directly impacted repair pathway selection (Wang et al., 2022). A proteomic study that used anti-PI(4)P antibody for immunoprecipitation also led to the discovery of PI(4)P-interacting DNA repair proteins, which included homologous recombination protein, RPA3, and the translesion synthesis proteins, MCM4 and PCNA (Fáberová et al., 2020). Interestingly, immunostaining demonstrated different nuclear localizations of PI(4,5)P2 and PI(4)P during interphase and mitosis. Additionally, RPA3 was found to only interact with PI(4)P but not PI(4,5)P2. This indicated that there were functional differences between the two in the nucleus (Fáberová et al., 2020).
Further investigation of cells under genotoxic conditions might lead to discovery of more PI(3,4,5)P3-interacting proteins that are involved in the DNA damage repair, since many repair proteins go through post-translational modification following damage induction. A study identifies DNA polymerase kappa (Polκ/POLS) as a phosphoinositide-binding protein by using a yeast surface displayed cDNA library exon microarray to probe for phosphoinositide-protein interactions (Bidlingmaier et al., 2011). Polκ is a Y-family DNA polymerase involved in TLS, which bypasses DNA lesions during replication. It is therefore possible that nuclear phosphoinositides might play a role in TLS. Nuclear lipids potentially also regulate nuclear functions by inducing intranuclear phase separations, which is reviewed elsewhere (Sztacho et al., 2019). Whether nuclear phosphoinositides actually regulate pathways that involve the above-mentioned DNA repair proteins requires further investigation. A schematic summary of nuclear phosphoinositide metabolism along with several key nuclear phosphoinositide-metabolizing enzymes that respond to DNA damage is shown in Figure 1. How these enzymes are regulated in response to DNA damage will be discussed in later sections.
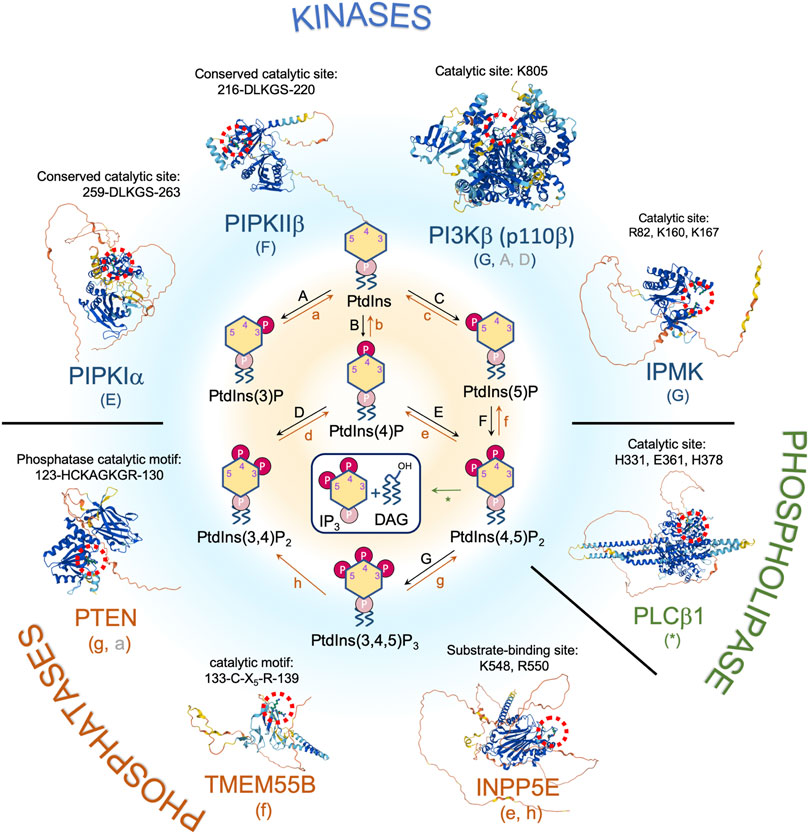
FIGURE 1. Nuclear phosphoinositide metabolism and involved enzymes with implications in DNA damage response and/or tumor suppression. Representative proteins/isoforms are shown as full length structures predicted by AlphaFold. Studies indicate that PIPKIα, PIPKIIβ, and PTEN might exist as dimers. Whether dimerization is required for their kinase activities and in response to DNA damage requires further investigation (Heinrich et al., 2015; Hu et al., 2015; Droubi et al., 2016). Red dotted circle labels the catalytic site of each protein. The letters in the parentheses under each enzyme correspond to the pathways indicated in the center panel. Letters in gray represent a minor pathway. Note that PI(3,5)P2 is not detected in nucleus.
Methodology
The general methodology for studying nuclear lipids is nicely summarized in a recent review (Moriel-Carretero, 2021). Here we briefly go through common techniques that are available for studying the roles of nuclear lipids in DNA damage repair. The methods can be put into two categories; the first measures the level changes and/or intranuclear localization of the nuclear phosphoinositides and the second involves antibody-based techniques. The methods in the first category are well developed. Radioisotope labeling using isolated nuclei detects newly phosphorylated phosphoinositides. To measure the mass level of individual nuclear phosphoinositide lipids requires nuclear isolation and lipid extraction using Bligh-Dyer or Folch methods followed by de-acylation before liquid chromatography analysis (Jones et al., 2013). However, this method can be strongly affected by the quality of samples, since residual ER membrane might remain attached to the nuclear envelope, which results in contamination from the cytosolic membranous organelles. Additionally, isolation procedures can result in the loss of nuclear phosphoinositides that form complexes with free-diffusing nuclear proteins such as nuclear receptors, NR5A1 (SF1) and NR5A2 (LRH1). (NR5A1 is also known as steroidogenic factor 1 (SF1), but SF1 now more commonly refers to splicing factor 1). In the second case of antibody-based methods including immunostaining, immunogold labeling for electron microscopy, and recently the popular proximity ligation assay (PLA), staining of phosphoinositides relies on the fixation of their binding proteins since lipids are not subject to formaldehyde fixation. Thus, staining might not reveal the genuine intranuclear distribution of phosphoinositides but rather gives indirect information.
Another set of methods involves the investigation of nuclear phosphoinositide-protein interactions. While PLA is also used for this purpose, the throughput is very limited. Instead, mass spec-based proteomic studies provide a more global picture of the phosphoinositide interactomes. In this case, neomycin extraction of nuclear fractions is used for phosphoinositide-binding protein enrichment. The sample is then further purified by affinity-based purification with phosphoinositide lipid-coated beads. Depending on the phosphoinositide on the beads, the binding specificities of nuclear proteins can be investigated. This approach is suitable for proteins that form strong and stable interactions with the phosphoinositide lipids in a bilayer form. For transient protein-lipid interactions, chemically modified bifunctional phosphoinositide lipids are used (Haberkant et al., 2013). Such lipids carry a photoactivable group that allows UV-induced crosslinking between the lipids and protein, and an alkyne group that allows for Click reaction for affinity column purification. These so called “clickable” lipids are commercially available. The main drawback of this system is that any chemical modification either at the head group or acyl chain of the phospholipids might lead to a dramatic change in their physical chemical properties, as the modifications are often comparable in size to a lipid head group.
Most methods do not allow us to probe for the early dynamics of nuclear phosphoinositides in the DNA damage response. Alternatively, genetically encoded phosphoinositide-sensing probes with fluorescent tags allow for visualizing phosphoinositides in living cells. This is made possible with the significant contributions from the Hammond and Balla laboratories [reviewed elsewhere (Hammond and Balla, 2015)]. Further, nuclear localization signal (NLS)-tagged phosphoinositide-binding domains fused with fluorescent proteins provide rapid sensing of lipid distributions in the nucleus. However, the major limitation comes from the high background noise of the lipid-free fluorescent proteins in the nucleoplasm. SV40-derived NLS-tag commonly leads to artefactual accumulation of target proteins at nucleoli (Kitamura et al., 2015). As a result, only significant changes in the localization of the phosphoinositides in the nuclear matrix become detectable (Kalasova et al., 2016; Wang et al., 2017). Much better results can be obtained with strong focused perturbations such as laser microirradiation to generate localized DNA damage. With that system, the changes in the local concentrations of the phosphoinositides and the recruitment of DNA repair proteins can be detected in live-cell imaging. The overexpressed lipid-binding domains also serve as sequestering agents. The expression of these probes mimics the depletion of nuclear phosphoinositides and allows for the investigation of the role of individual lipid species. While certain kinases and phosphatases such as PTEN can utilize both proteins and lipids as substrates (Bassi et al., 2013), this approach is advantageous because it helps to see the dual roles of such signaling proteins without perturbing their expression levels.
Involvement of Nuclear Phosphoinositides in DNA Damage Signaling
In the next section, we will discuss studies using nuclear phosphoinositide binding domains to probe for roles of nuclear phosphoinositides in DNA damage signaling. The changes in the local enrichment of DNA repair protein provide clues about how phosphoinositides are involved at different stages of DNA damage response.
Nuclear Phosphoinositide Sequestering Alters DNA Damage Signaling
Accumulating evidence indicates that increases in nuclear phosphoinositides are part of the DNA damage response, or in a broader term, stress response. The elevation of nuclear phosphoinositide influences gene regulation in many studies, which leads to stabilization (Choi et al., 2019) and activation of p53 (Gozani et al., 2003) or the expression of the pro-apoptotic protein, Bcl2-interacting killer (BIK) (Li et al., 2012). The information regarding how phosphoinositides affect DNA damage repair per se is rather limited. Our previous work focused on how nuclear phosphoinositide sequestering affected repair protein recruitment. Instead of depleting relevant phosphoinositide kinases and phosphatases, we used NLS-tagged phosphoinositide-binding domains and laser microirradiation to interrogate the role of individual nuclear phosphoinositides in DNA damage repair (Wang et al., 2017). The key finding was that the sequestering of nuclear PI(4)P, PI(4,5)P2 or PI(3,4,5)P3 suppressed ATR recruitment to different degrees. Sequestration of nuclear PI(3,4,5)P3 was the most effective, followed by PI(4,5)P2 and then PI(4)P. Among the three major PI3K-like kinases: ATR, ATM and DNA-PKcs that served as DNA damage signal transducers, only the recruitment and activation of ATR was significantly suppressed by sequestration of phosphoinositides. The local accumulation of ATR together with its interacting protein, ATRIP, was suppressed by more than 80%. Activation of ATR, as indicated by the phosphorylation of Chk1, was also significantly suppressed (Figure 2). Since all experiments were performed 6–12 h post transfection, we expected that the cellular protein expression profile was not significantly altered by the NLS-tagged PH or P4M domains. Interestingly, the recruitment of ATR was typically mediated by the binding between ATRIP and RPA in a canonical pathway. However, in this study, the recruitment of RPA remained unaffected and only the recruitments of ATRIP and ATR were suppressed. This indicated that nuclear phosphoinositide sequestration directly affected protein-protein interactions in the DNA damage repair network.
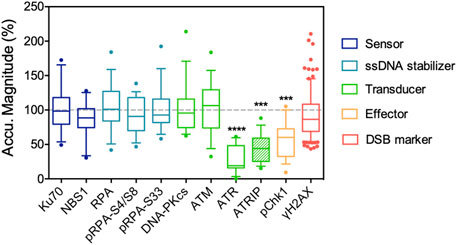
FIGURE 2. Nuclear PI(4,5)P2 sequestering by NLS-tagged PLCδPH domain suppresses the recruitment of ATR/ATRIP complex following UVA laser microirradiation, and the accumulation of its downstream effector, phospho-S345 Chk1. The recruitment of ATM, DNA-PKcs and other DNA repair proteins listed here are not affected. The data is adapted with permission from (Wang et al., 2017).
Nuclear Phosphoinositides Mediate DNA Damage Response at Multiple Time Scales
In this section, we would like to summarize the current findings regarding the roles of phosphoinositides in DNA damage at different time scales. Many studies have probed the roles of phosphoinositides in stress responses including DNA damage; however, those studies cover a wide range of time scales. To get a larger view of what happens with phosphoinositide levels and the relevant enzymes in the nucleus, we dissect this process into three stages that are discussed separately (Figure 3).
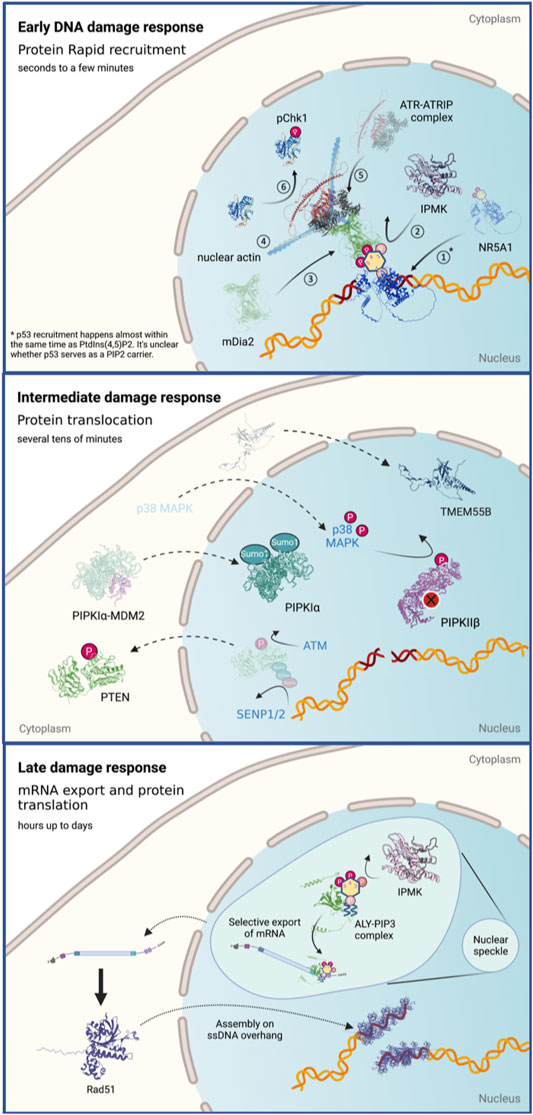
FIGURE 3. The roles of nuclear phosphoinositides and the regulation of their kinases and phosphatases in response to DNA damage at different time scales. Figures created with BioRender.com.
Early Damage Responses
We define the early DNA damage response as events that happen within the first 1–3 min following damage induction. The damage response at this stage mainly involves intranuclear redistribution and local accumulation of the nuclear factors at damage sites.
Our knowledge of the roles of nuclear phosphoinositides at this time scale is rather limited because there are few relevant assays. UVA laser microirradiation at 355 nm and localization of GFP-tagged phosphoinositide-binding domains document accumulation of phosphoinositides at damage sites. Phosphoinositide-binding domains rapidly accumulate at DNA damage sites within seconds and the accumulation of phosphoinositide drives the recruitment and activation of ATR. Both PI(4,5)P2-binding PLCδ-PH and PI(3,4,5)P3-binding Btk-PH domains are rapidly recruited to damage sites with similar dynamics. The magnitude of Btk-PH domain accumulation decreases after wortmannin inhibition or IPMK depletion, suggesting the involvement of PI3K activity in this process. Since IPMK doesn’t accumulate at damage sites, IPMK might help to maintain the PI(4,5)P2/PI(3,4,5)P3 ratio and nuclear phosphoinositide homeostasis. The phosphoinositide accumulation is driven, at least partially, by a nuclear receptor NR5A1, which is a known substrate of IPMK and a PI(4,5)P2/PI(3,4,5)P3 carrier through its acyl chain-binding domain. It remains unclear how phosphoinositides are loaded into NR5A1. Intriguingly, SUMOylation of NR5A1 drives NR5A1 to localize at nuclear speckles (Chen et al., 2004). Hence, NR5A1 SUMOylation provides a plausible mechanism by which NR5A1 can be loaded with PI(4,5)P2 via direct uptake or by the action of phospholipid transport proteins (PLTPs) at nuclear speckles (Davis et al., 2015). Whether inhibition of NR5A1 by SUMOylation alters DNA repair requires further investigation. However, it should be noted that the distribution of NR5A1 in human body is highly uneven. It is concentrated only in a handful of tissues which includes endocrinal tissues, bone marrow, lymphoid tissues and reproductive organs. Therefore, whether NR5A1-medaited early DNA damage signaling serves as a general mechanism in all tissues remains a question. While this study does not exclude the involvement of other phosphoinositide carriers, the involvement of other phosphoinositide carriers remains to be explored. Interestingly, the recruitment dynamics of phosphoinositides are almost identical to those of recruitment of p53. It is however not clear whether p53 serves as a PI(4,5)P2 carrier during the early DNA damage signaling events. Both RNAi depletions of IPMK or NR5A1 suppress ATR activation, which supports the proposed pathway.
To address how nuclear phosphoinositide accumulation drove ATR recruitment, we investigated the involvement of nuclear actin, whose assembly was induced by DNA damage and was critical for DNA damage clearance (Belin et al., 2015). Evidence of the involvement of nuclear actin assembly in ATR recruitment came from Latrunculin A (Lat A) inhibition. Actin disassembly by Lat A suppressed both ATR recruitment and Chk1 phosphorylation. While effects of Lat A on disrupted nuclear 3D architecture cannot be excluded, our observation is consistent with earlier findings that introduction of nuclear-targeted mutant actin that cannot polymerize, or the depolymerization of endogenous actin filaments by the addition of cytochalasin D (Cyto D) or Lat A, promotes chromatin association of DNA damage repair proteins from both the NHEJ and the HR pathways following ionizing irradiation. It also leads to prolonged retention of Ku80 at sites of damage in live cells (Andrin et al., 2012). Depletion of IPO9, a nuclear actin import factor, leads to delayed removal of 53BP1 and γH2AX foci (Belin et al., 2015). It’s also in line with the observation that the overexpression of cofilin, an actin-depolymerizing factor, alters nuclear actin dynamics and sensitize cells to radiation induced DNA damage (Lee et al., 2005). While the involvement of nuclear actin in the DNA damage response has drawn increasing attention in recent years, the identification of a nucleation polymerization factor (e.g., N-WASP, Formins or Arp2/3) remains controversial [see (Caridi et al., 2019) for a related review]. We found that nuclear phosphoinositide accumulation was followed by the accumulation of nuclear formins and nuclear actin, thereby completing the signaling axis (Wang et al., 2017).
The rapid local accumulation of PI(3,4,5)P3 at damage sites was also reported in an independent study. In a study by Kumar et al., class IA PI3Kβ (p110β) and PI(3,4,5)P3 both accumulated at DNA damage sites after either UV or IR irradiation (Kumar et al., 2010). Depletion of p110β lead to inhibition of ATM and ATR repair pathways as indicated by the suppressed phosphorylation of downstream effectors. p110β was the PI3K isoform that predominantly resided in the nucleus. Further investigation revealed that purified p110β interacted with Rad50 (an MRN complex component), Rad17 (an ATR effector), and Rad9B. Endogenous p110β interacted with both Rad50 and Nbs1 in intact nuclei upon UV or IR treatment. The recruitment of Nbs1 to damage sites following laser microirradiation depended on the expression, but to a lesser degree on the kinase activity of p110β. This indicated that p110β governed Nbs1 recruitment through a protein-protein interaction that was independent of its kinase activity. Finally, p110β deletion was shown to induce genomic instability, which strengthened its role in the DNA damage response. Twenty percent of untreated mouse embryonic fibroblasts (MEF) derived from p110β−/− mice had spontaneous DNA lesions in a comet assay, but not MEF cells derived from WT or kinase-dead p110β mice. It was therefore concluded that p110β expression was required for a DDR process, in which its kinase activity was not required. These results indicated that class I PI3Kβ was a critical factor for genomic integrity.
Interestingly, the recruitment dynamics of p110β and nuclear PI(3,4,5)P3, as determined using a BtkPH-EGFP construct, were around 50 s and 25 s, respectively, after UVA laser microirradiation (365 nm) (Kumar et al., 2010). The fact that the halftime of p110β recruitment was longer than that of BtkPH indicated that other enzymes with PI3K activity such as IPMK were involved in this process. This supported the hypothesis that p110β′s role in DDR was essentially kinase-independent, although its kinase activity facilitated the repair process. Additionally, the recruitment dynamics of BtkPH, and therefore PI(3,4,5)P3, from this study were significantly slower than those in our study (Wang et al., 2017). It should be noted that comparing results from different laser microirradiation studies is usually very difficult due to the lack of standardized parameters (e.g., laser wavelength, pulse width, repetition rate, power delivered at the back focal plane of the objective etc.). Comparisons of recruitment dynamics of different DNA repair components are largely limited to results from the same system.
Intermediate Damage Responses
The intermediate DNA damage response is defined as the events that happen within tens of minutes of the damage. At this time scale, the changes in phosphoinositide metabolism, and therefore their levels in the nucleus, mainly involve the posttranslational modification and translocation of phosphoinositide kinases and phosphatases.
In the following section, we examine how the levels of individual phosphoinositide species change, followed by how its regulation is altered in response to DNA damage. Starting with PI(5)P, the level of nuclear PI(5)P increased by 4–6 fold within 20–40 min following H2O2 treatment. This increase correlated with a small but significant decrease in PI5P-4K activity through p38 MAPK-mediated phosphorylation of PIPKIIβ at Ser326 and Thr322 (Jones et al., 2013). The nuclear translocation of p38 MAPK was driven by DNA damage induced activation and conformational change after exposure to ionizing irradiation or UV (Wood et al., 2009). p38 MAPK was activated through phosphorylation at Thr180/Tyr182 by upstream MAPKKs (Raingeaud et al., 1995). The phosphorylation of PIPKIIβ by nuclear p38 suppressed PIPKIIβ kinase activity by about 40% (Jones et al., 2013). As the change in PIPKIIβ activity was relatively minor, the significant increase in PI(5)P levels likely also involved the nuclear import of TMEM55B, also known as PIP4P1, which is a type I PI(4,5)P2 4-phosphatase. TMEM55B predominately localized in the cytosol of cells in a resting state (Ungewickell et al., 2005). The nuclear fraction of TMEM55B increased from 15 to 40% within 4 h in cells exposed to etoposide. The same phenomenon has been observed in both the endogenous and overexpression systems. Since the total amount of TMEM55B appeared unchanged, an increase in nuclear TMEM55B likely resulted from the redistribution of this enzyme (Zou et al., 2007). The resulting increase of PI(5)P created a positive feedback loop by driving p38-dependent activation of a nuclear ubiquitination ligase complex, SPOP-Cul3, which promoted the degradation of PIPKIIβ (Bunce et al., 2008). In summary, the nuclear PI(5)P increase in response to DNA damage was jointly regulated by the inactivation and down-regulation of PIPKIIβ as well as the nuclear import of TMEM55B.
It should be noted that the type of DNA damage also plays a role in the damage response dynamics. Ionizing radiation and UV share an advantage that there is clear time zero in the sequence of events. However, ionizing radiation produces various types of DNA damage, which include base damage, apurinic/apyrimidinic (AP) sites, DNA single-strand breaks (SSBs), DNA double-strand breaks (DSBs), and DNA–protein crosslinks (DPCs) (Nakano et al., 2022). The same complexity is also seen with UV-induced damage, but the types of damage strongly depend on the wavelength. UVA induces both UV-specific DNA adducts (such as cyclobutane pyrimidine dimers (CPDs) and pyrimidine-pyrimidone (6–4) photoproducts) and oxidative damage in a 1:1 ratio, while the latter includes a wide spectrum of DNA products (Cooke et al., 2003). UVC is 10,000 times more effective than UVA and 100 times more effective than UVB in DNA adduct formation. The DNA adduct:oxidative damage ratio reaches 100:1 in UVC-induced damage in vitro (Kuluncsics et al., 1999). Therefore, UVC is commonly used if UV-specific damage is to be studied. By contrast, DSBs induced by etoposide slowly increase and reach a maximal level after 40 min, but H2AX phosphorylation continues to increase and reaches its maximum only after 160 min (Muslimović et al., 2009). Additionally, etoposide causes both SSBs and DSBs with DSBs being a minor product (∼3%). Alternatively, several chemical agents are considered radiomimetic drugs in that they imitate the effect of ionizing radiation and break the DNA directly. Radiomimetic drugs include bleomycin, phleomycin and neocarzinostatin, which attack DNA directly and create DSBs within the first few minutes (Vítor et al., 2020). To better assess the dynamics of DSB repair, chemicals like neocarzinostatin are recommended, as its excess will be quickly hydrolyzed in the medium. Different types of damage effectors have different kinetics.
Secondly, we considered the regulation of PI(4,5)P2 at an intermediate time scale. While it was clear that nuclear PI(4,5)P2 played a role in the DNA damage response, how nuclear PI(4,5)P2 level was regulated upon DNA damage remained elusive. The level of nuclear PI(4,5)P2 increased by about 80% shortly after being exposed to UVC (within minutes) and colocalized with DNA damage sites (γH2AX foci) (Wang et al., 2017). The finding was later confirmed by a study in which elevation of nuclear PI(4,5)P2 was also observed in cells treated with cisplatin for 24 h. Additionally, PIPKIα, PI(4,5)P2, and p53 strongly colocalized with γH2AX foci upon cisplatin treatment (Choi et al., 2019). The dynamic aspect of this process has not been investigated in detail. Recent evidence indicated that the regulation of PIPKIα activity was mediated by complex formation and was context dependent. PIPKIα was activated in different complexes. Two scenarios of upregulated PIPKIα activity were described in response to DNA damage. One was mediated by complex formation with p53 and small heat shock proteins (sHSP) (Choi et al., 2019). DNA damage induced dissociation of PIPKIα from MDM2 in the cytosol and promoted nuclear translocation of PIPKIα. The association of PIPKIα and p53 in the nucleus mediated p53-PI(4,5)P2 complex formation, which promoted the recruitment of sHSP thereby stabilizing p53 following cisplatin treatment (Choi et al., 2019). How PI(4,5)P2 interacted with p53 will be further discussed in a later section. Another PIPKIα activation mechanism in response to DNA damage was reported by the same group in a different context. In this other scenario, DNA damage induced by etoposide treatment for 4 h triggered a nuclear translocation of PKCδ, which formed a complex with PIPKIα and Star-PAP. PI(4,5)P2 produced by PIPKIα activated PKCδ. Activated PKCδ in turn activated Star-PAP for the 3’ end processing of the mRNA (Li et al., 2012). This still begs the question of how PI(4,5)P2 level is regulated by damage.
Another aspect of nuclear PI(4,5)P2 signaling is the regulation of nuclear 5′-phosphatases, which include SHIP1&2 and INPP5E. SHIP1 is present in nucleoli (Ehm et al., 2015). Phosphorylated SHIP2 (pSer132) colocalizes with PI(4,5)P2 in nuclear speckles (Déléris et al., 2003), which implies PI(4,5)P2 phosphatase activity is present (Taylor et al., 2000; Elong Edimo et al., 2011). Conversely, a substrate analysis of SHIP2 using the recombinant SHIP2 catalytic domain in vitro showed the following preference: Ins(1, 2, 3, 4, 5)P5 > Ins(1, 3, 4, 5)P4 > PI(3,4,5)P3 ≈ PI(3,5)P2 ≈ Ins(1, 4, 5, 6)P4 > PI(4,5)P2 (non-detectable) (Chi et al., 2004). Therefore, whether SHIP2 is the major nuclear 5′-phosphatase remains debatable and whether it responds to genotoxic stress needs to be investigated. The type IV 5′-phosphatase, INPP5E, on the other hand, shows a stronger connection with DNA damage repair and preserving genome stability. It utilizes PI(3,4,5)P3 as its primary substrate, and has also been shown to dephosphorylate PI(4,5)P2 and PI(3,5)P2. INPP5E localizes to centrosomes, chromosomes, and kinetochores in early mitosis and shuttles to the midzone spindle at mitotic exit. It supports genomic stability through regulation of mitosis (Sierra Potchanant et al., 2017). Knockdown of INPP5E causes chromosomal instability in primary human fibroblasts. Micronucleus assays reveal increased frequencies of both mitotic errors and unrepaired double-strand DNA breaks in INPP5E-deficient cells (Sierra Potchanant et al., 2017). Additionally, INPP5E is phosphorylated at ATM/ATR recognition sites following exposure to ionizing radiation (Matsuoka et al., 2007), but how ATR-mediated phosphorylation regulates INPP5E activity is not yet understood in depth. Thus, the roles of the nuclear 5′-phosphatases in the DNA damage response are not well understood.
The last issue in the intermediate timescale is the regulation of PI(3,4,5)P3. In addition to the early recruitment to DNA damage sites of p110β, which coincides with local enrichment of PI(3,4,5)P3 (Kumar et al., 2010), the 3′-phosphatase PTEN also plays a role in mediating DNA damage response. The roles of PTEN in the DNA damage response involve both phosphatase-dependent and phosphatase-independent functions. Further, the PTEN story is complicated by the fact that PTEN possesses both lipid and protein phosphatase activities. Although it’s getting clear that nuclear PTEN is important for efficient DNA repair, chromosome stability, and cell cycle progression (reviewed elsewhere (Milella et al., 2015)), an increasing body of evidence suggests that nuclear PTEN mediates DNA damage response largely through a lipid phosphatase-independent activity (Bassi et al., 2013; Ma et al., 2019). Although PTEN has been shown to act on PI(3,4,5)P3-NR5A1 complexes in cell studies (Blind et al., 2012), an earlier report indicates that there is an independent pool of nuclear PI(3,4,5)P3 that is insensitive to PTEN (Lindsay et al., 2006). These findings argue that PTEN is more than a lipid phosphatase in the DNA damage response.
The phosphatase-independent functions of PTEN in the DNA damage response often involve post-translational modification and nuclear translocation of PTEN. Despite the absence of a classic nuclear localization signal, a few nuclear translocation mechanisms of PTEN have been proposed. These include simple diffusion, active shuttling, cytoplasmic localization signal-dependent export and post-translational modification-dependent import (Planchon et al., 2008). It has been shown that SUMOylation (SUMO, small ubiquitin-like modifier) of PTEN at Lys254 by UBC9 drives its nuclear localization in HEK293 cells (Bassi et al., 2013). Ionizing radiation causes a gradual reduction in the amounts of SUMO-PTEN, and therefore depletion of nuclear PTEN beginning 1 h after IR exposure with the steady-state amounts returning 8 h later. Other forms of genotoxic stress, such as treatment with cisplatin or doxorubicin, also cause depletion of SUMO-PTEN, in conjunction with the appearance of DNA damage elicited by these agents. ATM-mediated phosphorylation of PTEN at Thr398 promotes the deSUMOylation of PTEN, which is mediated by SENP1/2 (Bassi et al., 2013; Bawa-Khalfe et al., 2017), and therefore facilitates the nuclear export of PTEN in response to IR. Cells lacking nuclear PTEN are hypersensitive to DNA damage, whereas PTEN-deficient cells are susceptible to killing by a combination of genotoxic stress and a small-molecule PI3K inhibitor both in vitro and in vivo (Bassi et al., 2013). Importantly, a PTEN mutant lacking the lipid phosphatase activity demonstrates similar DNA damage clearance dynamics as the wildtype, while the mutant lacking both lipid and protein phosphatase activities demonstrates decreased HR efficiency, similar to the PTEN-depleted cells (Bassi et al., 2013). In another example, ionizing radiation leads to FGFR2-mediated tyrosine phosphorylation of nuclear PTEN at Y240, which happens independently of the SUMOylation of PTEN at K254 (Ma et al., 2019). Inhibition of Y240 phosphorylation using FGFR inhibitors sensitizes tumors to ionizing radiation. pY240-PTEN binds to chromatin through interaction with Ki-67 and promotes HR-mediated DSB repair by facilitating RAD51 filament formation, which requires neither protein nor lipid phosphatase activity of PTEN (Ma et al., 2019).
Some earlier studies that reveal the role of PTEN in DNA damage repair didn’t look into the dependence of its phosphatase activity and are summarized here. In addition to the role of PTEN in homologous recombination, PTEN also positively regulates global genome NER (GG-NER) by promoting XPC transcription in keratinocytes (Ming et al., 2011; Ming and He, 2012). Inhibition of PTEN impairs GG-NER capacity through suppressing the expression of XPC. The PTEN/AKT/p38 axis seems critical for regulating XPC levels and therefore GG-NER capacities (Ming et al., 2011). These studies demonstrate that cells deficient in nuclear PTEN have defective DNA repair, consistent with the genomic instability phenotype in PTEN-depleted cells (Shen et al., 2007). Altogether, although the roles of nuclear PTEN in DNA damage repair are largely phosphatase-independent, PTEN has clinical value in DNA damage-based cancer treatment through synthetic lethality with FGFR (which governs the phosphorylation of PTEN at Y240) and/or PI3K inhibitors (Bassi et al., 2013).
Late Damage Responses
We define the late damage responses as the events that happen from several hours to even days following damage induction. They include the two PIPKIα activation mechanisms mentioned in the previous section that are mediated by complex formation with PLCδ and p53, respectively. The former involves the nuclear import of PLCδ, which leads to the activation of Star-PAP and elevation of BIK mRNA and protein level within 4 h after etoposide treatment. The latter involves p53 stabilization and transactivation 24 h following cisplatin treatment.
The last example of nuclear phosphoinositides in the DNA damage response at longer time scales involves PI(3,4,5)P3-mediated selective mRNA export (Wickramasinghe et al., 2013). mRNA export from the nucleus to the cytoplasm is essential for the translation of DNA damage response proteins. mRNA export is coupled to prior steps including RNA transcription and splicing by the TREX (transcription-export) complex, which is recruited by the RNA splicing machinery. TREX components including the RNA-binding adaptor protein ALY colocalize with splicing factors in nuclear speckle domains (Masuda et al., 2005). Further studies reveal that ALY functions to release spliced mRNA from nuclear speckles for export into the cytoplasm (Dias et al., 2010). Interestingly, the mRNA export activity, as well as nuclear speckle domain residence of ALY, can be regulated via binding to the second messengers PI(4,5)P2 and PI(3,4,5)P3, suggesting that nuclear phosphoinositide signaling plays a role in nuclear mRNA export (Okada et al., 2008).
In this last example, Wickramasinghe et al. demonstrate that IPMK has a transcript-selective function in nuclear mRNA export in human cells, and show that its activity is necessary for the expression of certain proteins involved in HR, including RAD51, but not other related repair factors involved in NHEJ. This finding is consistent with a genetic screen in which IPMK depletion has a significant effect on HR but not NHEJ (Certo et al., 2011). In more detail, IPMK depletion or catalytic inactivation followed DNA damage by 24 h regardless of damage induction mechanism including methyl methanesulfonate, carboplatin or ionizing irradiation leads to inhibition of the nuclear export of the polyA+ mRNAs that encode essential HR factors such as RAD51, CHK1, or FANCD2, decreasing protein abundance. In contrast, several genes involved in NHEJ are unaffected. The downregulation of RAD51, which is a DNA repair protein essential for homologous recombination (HR) that stabilizes ssDNA at DNA lesions, provokes sensitivity to genotoxic lesions repaired by HR, and causes structural chromosome aberrations typical of defective HR. Further characterization reveals that recognition of a sequence motif in the untranslated region of RAD51 transcripts by the mRNA export factor ALY requires PI(3,4,5)P3, which is a product of IPMK. Exogenous PI(3,4,5)P3 restores ALY recognition in IPMK-depleted cell extracts, indicating a mechanism underlying transcript selection. It is therefore suggested that IPMK plays a role in a transcript-selective mRNA export pathway in response to DNA damage by regulating nuclear phosphoinositide turnover that helps to preserve genome integrity (Wickramasinghe et al., 2013).
The Link Between Nuclear Phosphoinositide and p53 in Response to Genotoxic Stress
Genotoxic Stress Perturbs Nuclear Phosphoinositide Homeostasis
In this section, we provide a PI(4,5)P2-centric view to show how the nuclear phosphoinositides are differentially regulated in a resting state (Figure 4A) vs under genotoxic stress (Figure 4B). We mainly focus on enzymes that have shown nuclear localization and altered activity in response to DNA damage. For example, nuclear PLCβ1 is involved in regulating nuclear PI(4,5)P2 fluctuations throughout the cell cycle and differentiation (Cocco et al., 2006; Ratti et al., 2017). Its dysregulation is implied in the progression from myelodisplastic syndrome to acute myeloid leukemia (Martelli et al., 2005; Fiume et al., 2010; Ratti et al., 2021). However, its regulation in response to DNA damage and/or interaction with DNA repair proteins is minimal (Piazzi et al., 2013) and is therefore neglected.
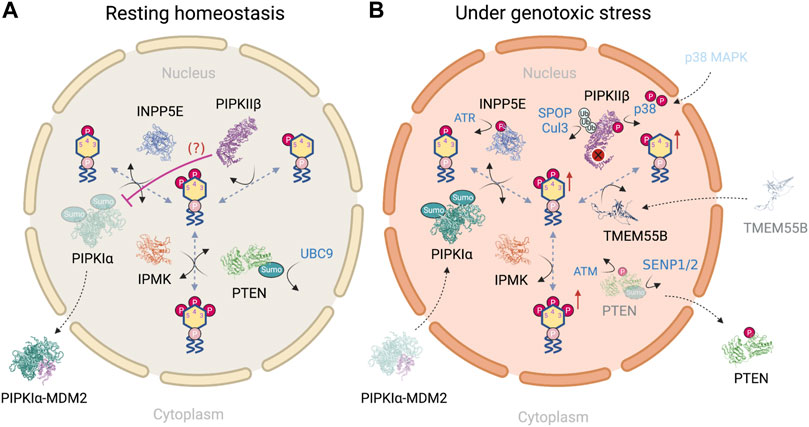
FIGURE 4. (A) PI(4,5)P2-centric view of nuclear phosphoinositide homeostasis in a resting state and (B) how the regulatory steps are altered in response to genotoxic stress. Figures created with BioRender.com.
Although both PIPKIα and PIPKIIβ pathways lead to the synthesis of nuclear PI(4,5)P2, it is suggested that the pool of nuclear PI(4,5)P2 is mainly maintained by PIPKIα through the phosphorylation of PI(4)P. This conclusion is based on the following findings: first, the amount of PI(4)P is at least 20-fold higher than that of PI(5)P in nucleus (Keune et al., 2011); second [γ32P] phosphate labeling using isolated rat liver nuclei demonstrates that the relative labeling ratio for PI(4,5)P2 at the 5′- vs. 4′-OH position is approximately 1.8, suggesting that PIPKIs are the major kinases for PI(4,5)P2 synthesis within the nucleus (Vann et al., 1997); third, this experiment has been performed in the presence of PI4K inhibitors, wortmannin and adenosine, which inhibit PI4KIII and PI4KII, respectively (Balla and Balla, 2006). The ratio of radiolabeling of the 5′- to the 4′-OH of PI(4,5)P2 in the presence of wortmannin increases up to 10:1 while adenosine has no effect. These simple and elegant studies indicate that at least 90% of nuclear PI(4,5)P2 is derived from the PIP5K pathway (Keune et al., 2011), and indicates that PIPKIIβ regulates nuclear PI(5)P level, as will be discussed later.
As mentioned earlier, nuclear PI(4,5)P2 level is increased upon genotoxic stress (Wang et al., 2017; Choi et al., 2019). It’s regulation has largely been attributed to the catalytic activity of PIPKIα. Although PIPKIγi4 is another PIPKI and the only PIPKIγ subfamily member that resides in the nucleus (Schill et al., 2009), it is omitted in this picture as its regulation under DNA damage remains to be characterized. This raises the question of how PIPKIα is differentially regulated during the resting state and under genotoxic stress. Four pathways that regulate PIPKIα have been described. The first pathway is associated with the retinoblastoma protein (pRB), that is highly mutated in human tumors. pRB is a nuclear scaffold protein for lipid kinases such as PIPKIs and DAG kinases (Divecha et al., 2002; Los et al., 2007). pRB binds to PIPKI through its pocket domain, which is often mutated in human tumors, and highly activates PIPKIα in a large T antigen-regulated manner (Divecha et al., 2002). However, how pRB regulates PIPKIα upon DNA damage induction remains unclear. pRB modulates transcription of proteins that control cell cycle progression, differentiation, and is recruited to DNA damage sites in response to DNA damage (Vélez-Cruz et al., 2016). The deregulation of pRB in tumors implicates the pRB-PIPKIα complex in directing DNA damage signaling at sites of damage and/or at a transcriptional level. In the second pathway, DNA damage also triggers the nuclear translocation of protein kinase C delta (PKCδ), which is required for the initiation of apoptosis (Devries et al., 2002). PKCδ directly interacts with PIPKIα in the nucleus. PI(4,5)P2, presumably produced locally by PIPKIα, stimulates PKCδ kinase activity toward Star-PAP (Li et al., 2012). As a result, PKCδ does not directly regulate PIPKIα but serves as a downstream effector of PIPKIα through nuclear PI(4,5)P2 in regulating protein expression. Third, DNA damage-induced by etoposide for 24 h promotes polySUMOylation of PIPKIα at K33, K244 and K490 (Chakrabarti et al., 2015) as well as the nuclear translocation of PIPKIα (Chakrabarti et al., 2013). Similar nuclear translocation of PIPKIα in response to genotoxic stress has been shown with cisplatin treatment (Choi et al., 2019). Lastly, it has been suggested PIPKIα complexes with MDM2 in the cytosol in a resting state. Such an interaction is disrupted upon damage induction, which is accompanied by the nuclear translocation of PIPKIα (Choi et al., 2019). In contrast, the association between PIPKIα and wildtype p53 is not detected in unstressed cells, but is dramatically increased by DNA-damaging agents (cisplatin and etoposide) and oxidative stress (tBHQ) (Choi et al., 2019). Together, these findings indicate that nuclear PI(4,5)P2 level is mainly regulated by two mechanisms: one is the nuclear level of PIPKIα, that is governed by the post-translational modification of PIPKIα; the other factor is the availability of downstream PIP2 effectors in close proximity.
Recently, an additional mechanism that regulates PIPKIα activity has been reported. The loss of PIP4Ks, which includes PIPKIIα, β, γ, in vitro results in an unexpected increase in PI(4,5)P2 and a concomitant increase in insulin-stimulated production of PI(3,4,5)P3. A rescue using either wild-type or kinase-dead mutants of the PIP4Ks restores cellular PI(4,5)P2 levels and insulin stimulation of the PI3K pathway. It therefore suggests a catalytic-independent role of PIP4Ks in regulating PI(4,5)P2 levels (Wang et al., 2019). These effects are explained by an increase in PIP5K activity upon the deletion of PIP4Ks, which normally suppress PIP5K activity through a direct binding mediated by the N-terminal motif of PIP4K. Therefore, PIP4Ks act as negative regulators of PIP5Ks. Although this finding is based on studies in a different context than the DNA damage response and is not limited to phosphoinositides in the nucleus, the regulation mechanism might be related. On the other hand, although type I PI(4,5)P2 5-phosphatase, INPP5E, potentially plays a role in maintaining genome stability (Sierra Potchanant et al., 2017) and is an enzymatic substrate of ATR upon ATR activation (Matsuoka et al., 2007), it is not clear how ATR-mediated phosphorylation affects INPP5E activity and therefore nuclear PI(4,5)P2 level.
The last key lipid species is PI(3,4,5)P3, which has been shown to be upregulated in response to DNA damage. Nuclear PI(3,4,5)P3 is regulated by both IPMK and class I PI3Kβ (p110β) and potentially PTEN. IPMK demonstrates greater substrate specificity than class I PI3Ks since IPMK phosphorylates only PI(4,5)P2 (Resnick et al., 2005). The phosphorylation of IPMK at Ser284 by protein kinase CK2 decreases the nuclear localization of IPMK (Meyer et al., 2012). Interestingly, CK2 accumulates at DNA damage sites and is involved in a wide spectrum of DNA repair mechanisms, such as NHEJ (Olsen et al., 2012), HR (Yata et al., 2012), mismatch repair (MMR) (Weßbecher et al., 2018) and nucleotide excision repair (NER) (Shah et al., 2018). It’s not clear if CK2 regulates DNA repair processes through nuclear phosphoinositide-mediated DNA damage signaling. IPMK is almost exclusively nuclear and is unaffected by wortmannin or other PI3K selective inhibitors in vitro (Resnick et al., 2005). The same group has shown that inhibition of p110 PI3-kinases by wortmannin prevents IPMK phosphorylation and activation (Maag et al., 2011). Although IPMK and classical PI3K phosphorylate PI(4,5)P2 in vitro in lipid mixtures or in membranes (Resnick et al., 2005), the NR5A1-PI(4,5)P2 complex is a substrate of IPMK, but not classical PI3K(102). By contrast, while PTEN is strongly involved in the DNA damage response, its lipid phosphatase-dependent role in this process remains elusive. PTEN is maintained in a resting state after the SUMOylation of PTEN at Lys254 by Ubc9, which drives its nuclear localization. Further, ATM-mediated phosphorylation of PTEN at Thr398 promotes the deSUMOylation by SENP1/2 and therefore the nuclear export of PTEN as part of the DNA damage response (Bassi et al., 2013). It potentially explains the mechanism by which nuclear PI(3,4,5)P3 is upregulated in response to DNA damage, assuming the kinase activity of IPMK is not significantly altered during this process.
Roles of Different Lipid Species and Their Interaction With p53
In the previous section, genotoxic stress leads to increased levels of PI(5)P, PI(4,5)P2 and PI(3,4,5)P3 in the nucleus. Accumulating evidence suggests that these changes affect p53 activities, which include a variety of responses following DNA damage, such as cell cycle arrest, DNA repair, senescence and apoptosis. Here we summarize related findings and discuss how nuclear phosphoinositide signaling impacts regulation of p53 function.
Our previous studies showed that increased PI(4,5)P2 speckles induced by UVC damage strongly co-localized with γH2AX foci (Wang et al., 2017). The upregulation of nuclear PI(4,5)P2 due to cisplatin-induced genotoxic stress was also observed in a different study and was linked to a complex formation with p53 (Choi et al., 2019). The close contact between PIPKIα, PI(4,5)P2 and p53 (within 40 nm apart) has been confirmed using a proximity ligation assay and all were found to strongly co-localize with γH2AX foci. The association between PIPKIα and MDM2 was disrupted upon cisplatin treatment and it was accompanied by enhanced complex formation between PIPKIα and wildtype p53 in the nucleus, which was otherwise undetected in a resting state. Interestingly, PIPKIα formed stable complexes with mutant p53s even in a resting state. In the case of mutant p53s, PIPKIα binding was required for the stabilization of mutant p53s at a high expression level. PIPKIα interacted with p53 through the p53 oligomerization domain (OD) and CTD. The production of PI(4,5)P2 in the close vicinity of p53 promoted the association of Hsp27 and/or HspB1 but attenuated the association of PIPKIα with p53 (Figure 5A). Both PIPKIα depletion and PIPKIα inhibitor, ISA-2011B, impaired the association of Hsp27 with p53. By contrast, mutant p53 stabilization depends on its constant complex formation with PIPKIα and Hsp70 or Hsp90 chaperone proteins. Hsp70 and Hsp90 were postulated to block access of E3 ligase to mutant p53. However, Hsp90 is mostly cytosolic and Hsp70-p53 binding does not require p53-PI(4,5)P2 for complex formation. Additionally, the binding of PI(4,5)P2 prevents p53 from interacting with MDM2, which is another mechanism to stabilize p53. The binding of PI(4,5)P2 to p53 is mapped to the C-terminal polybasic stretch of p53, which indicates electrostatic interactions play a major role when interacting with phosphoinositide lipids in nucleus (Mazloumi Gavgani et al., 2021). Surprisingly, the PI(4,5)P2-p53 interaction was very stable and was resistant to denaturation during SDS-polyacrylamide gel electrophoresis (SDS-PAGE) (Choi et al., 2019). Altogether, nuclear PI(4,5)P2 mediated p53 stabilization offers a mechanism different from the canonical Hsp70-Hsp90-MDM2 pathway and its dysregulation has direct implications in tumorigenicity.
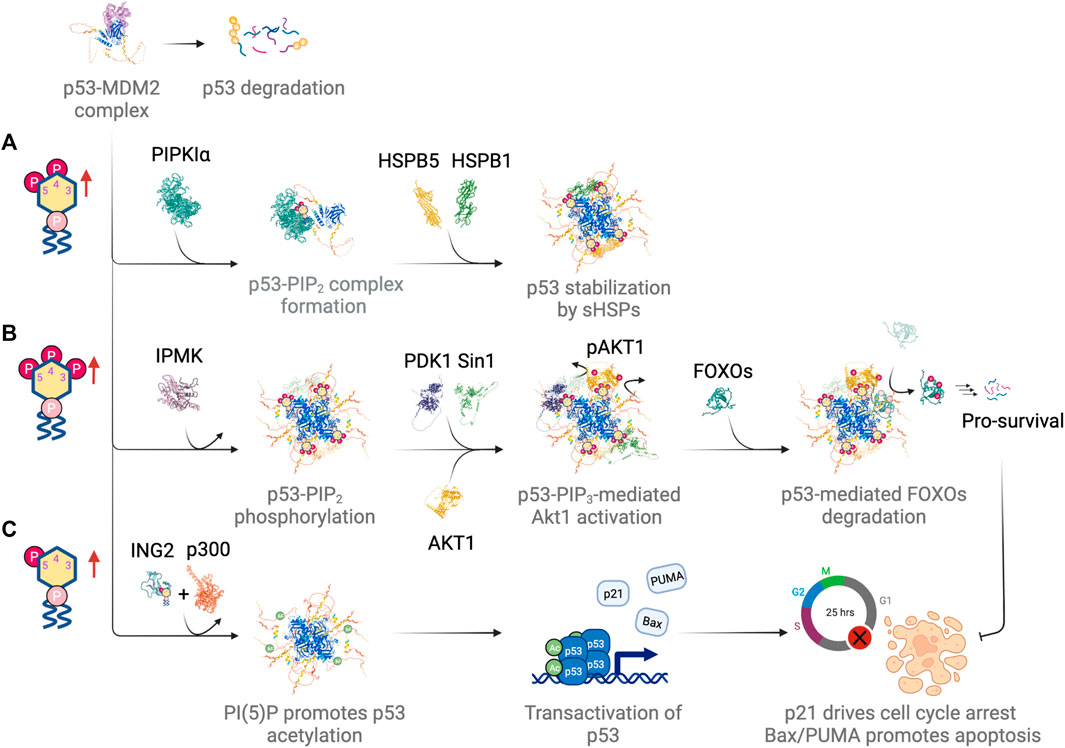
FIGURE 5. Differential regulation of p53 by different nuclear phosphoinositide species in response to DNA damage. (A) PI(4,5)P2 drives complex formation with p53 and promotes p53 stabilization by recruiting small heat shock proteins (sHSPs). (B) IPMK phosphorylates p53-PI(4,5)P2 complex, which serves as a signaling hub for Akt activation and subsequent phosphorylation and degradation of FOXOs. (C) PI(5)P promotes chromatin association of ING2. It drives p300-mediated acetylation and transactivation of p53. Figures created with BioRender.com.
Multiple roles have been suggested for PI(3,4,5)P3-mediated regulation of p53. In one case, the p53-PI(3,4,5)P3 complex served as a signaling hub for Akt signaling (Chen et al., 2021a). Activation of the nuclear Akt pathway involved the kinase activity of IPMK, which turned the p53-PI(4,5)P2 complex into p53-PI(3,4,5)P3. The complex then recruited Akt as well as the PI(3,4,5)P3-binding kinases PDK1 and Sin1. The latter was a subunit of the mTORC2 complex. Both PDK1-dependent phosphorylation of Akt at Thr308 and Sin1-dependent phosphorylation of Akt at Ser473 activated the nuclear Akt pathway, which has been shown to be required for p53 stabilization (Boehme et al., 2008). This led to the phosphorylation of the forkhead box O (FOXO) protein family, which included FOXO1, FOXO3, FOXO4 and FOXO6. p53-mediated phosphorylation of FOXO proteins by Akt promoted the degradation of FOXOs, which was a pro-survival signal and inhibited DNA damage-induced apoptosis (Figure 5B). FOXOs directly interacted with p53 (Wang et al., 2008) and p53 promoted FOXOs degradation through MDM2-mediated ubiquitination (Fu et al., 2009).
Surprisingly, other than mediating p53-dependent Akt activation, IPMK also functions as a transcriptional coactivator of p53 (Xu et al., 2013) (reviewed elsewhere (Kim et al., 2017)). IPMK overexpression increases transcriptional activity of p53 as reflected in mRNA levels for canonical p53 targets (e.g., PUMA, Bax, and p21) (Xu et al., 2013; Xu and Snyder, 2013). The increase in p53 transcriptional activity following etoposide treatment also depends on IPMK. IPMK binds to p53 directly and stimulates its acetylation by binding to p300, an acetyltransferase. IPMK depletion disrupts the p53-p300 interaction, which leads to a decreased acetylation of both p53 and histones, and thereby reduces the transcription of p53 target genes and p53-mediated apoptosis. Interestingly, IPMK-dependent p53 acetylation is independent of IPMK kinase activity, since overexpression of the kinase deficient mutant of IPMK (K129A-S235A) in U2OS causes a similar increase in p53-IPMK interaction upon etoposide treatment as with wildtype IPMK.
PTEN also contributes to p53 regulation in both a phosphatase-dependent and phosphatase-independent manner (Freeman et al., 2003; Trotman and Pandolfi, 2003). While p53 is known to drive PTEN expression following DNA damage such as γ irradiation (Stambolic et al., 2001), the effect of PTEN on p53-mediated transcription is also known. PTEN, but not its phosphatase-dead mutant, is able to produce a moderate increase in p53-mediated transcription in the presence of MDM2, indicating that PTEN can indirectly protect p53 from MDM2-mediated degradation (Mayo et al., 2002). Intriguingly, a molecular interaction between PTEN and p53 is mapped to the C-terminal of p53, where PI(4,5)P2 binds (Choi et al., 2019), using GST-pulldown of p53 partial deletion mutants (Freeman et al., 2003). The association of p53, on the other hand, is mapped to the C2 domain of PTEN, which is a Ca2+-dependent membrane-targeting module (Lee et al., 1999; Georgescu et al., 2000; Yasui et al., 2014). However, phosphatase-dead mutants of PTEN can also protect p53 from MDM2-independent degradation. Addition of a phosphatase-dead PTEN mutant or the PTEN C2 domain also results in the elevation of p53 level, consistent with a role for PTEN in p53 stabilization through direct interaction (Freeman et al., 2003). PTEN also complexes with p300 in the nucleus and plays a role in maintenance of high p53 acetylation in response to DNA damage (Li et al., 2006). Intriguingly, p53 acetylation not only promotes PTEN-p53 interaction but also promotes p53 tetramerization. Importantly, PTEN is unable to induce the transactivation of the acetylation-deficient mutant of p53 (p53-5 KR), which has all five acetylation sites, Lys370, 372, 373, 381, and 382, mutated. This indicates that p53 acetylation is required for activation of p53 by PTEN and subsequent maintenance of high p53 acetylation (Li et al., 2006). Interestingly, these sites are essentially the same sites for p53-PI(4,5)P2 interaction (Choi et al., 2019).
Finally, we consider the consequences of nuclear PI(5)P upregulation in p53 function (Figure 5C). As mentioned previously, the upregulation of nuclear PI(5)P leads to the binding of ING2 (inhibitor of growth family member 2) to PI(5)P and thereby promotes ING2 association with chromatin upon DNA damage (Gozani et al., 2003; Zou et al., 2007). ING2 is a component of a chromatin-regulatory complex that represses a specific subset of genes in response to DNA damage (Bua et al., 2013). ING2-mediated gene repression is through the interaction between ING2 and H3K4me3, which stabilizes ING2-SIN3A-HDAC1 complexes at target gene promoters and promotes histone deacetylation (Shi et al., 2006; Smith et al., 2010). ING2 contains a PHD finger domain that coordinates with zinc and binds to PI(5)P (Gozani et al., 2003). Possibly, the generation of PI(5)P at specific target sites on chromatin recruits and activates ING2 (Gozani et al., 2003). The loss of ING2 binding to PI(5)P reduces p53-mediated apoptosis in response to etoposide or H2O2 (Gozani et al., 2003). This is consistent with studies that show ING2 upregulation and increases in nuclear localization of Type I PI(4,5)P2 4-phosphatase, TMEM55B, the main PI(5)P generating enzyme after DNA damage (Nagashima et al., 2001; Zou et al., 2007; Bua et al., 2013). Interestingly, similar to IPMK, ING2 also complexes with the histone acetyltransferase p300. It enhances the interaction between p53 and p300 as well as being a cofactor for p300-mediated p53 acetylation (Nagashima et al., 2001; Pedeux et al., 2005). Furthermore, overexpression of ING2 induces senescence in young fibroblasts in a p53-dependent manner. The level of ING2 expression directly modulates the onset of replicative senescence (Pedeux et al., 2005). Taken together, these studies show that PI(5)P plays a key role in localizing ING2 to chromatin and activating it in response to genotoxic and oxidative stress.
Nuclear Phosphoinositides in Genome Stability and Tumor Suppression
A p53-Centric View of DNA Damage Repair Pathway Selection
Next, we focus on new findings that provide a different view of p53’s role in DNA damage repair, which involves repair pathway choice. In a commonly accepted model, the downstream transactivation of p53 is considered its major role in response to DNA damage. p53 helps maintain genome stability by promoting the expression of proteins involved in cell cycle arrest, DNA repair, apoptosis and senescence. However, the roles of p53 as a tumor suppressor cannot be fully explained by the transcriptional activity of p53. In this last section, we provide a p53-centric view and focus on an upstream and transcription-independent function of p53 in directing DNA repair pathway selection that strongly correlates with its tumor suppressive activity.
p53 was known to direct repair pathway choice (Sengupta and Harris, 2005). This feature was highlighted in recent years due to advances in genomic editing using CRISPR-Cas9. Two independent studies both pointed out that wildtype p53 inhibited Cas9-mediated genomic editing by suppressing homologous recombination (Haapaniemi et al., 2018; Ihry et al., 2018). By contrast, p53 depletion increased the rate of homologous recombination and inhibited the DNA damage response. This finding led to a dilemma when creating genomic-edited cells for therapeutic purposes because successfully edited cells might have inherited defects in p53 function and were therefore more likely to be tumorigenic.
Repair pathway selection is an early event in the DNA damage response. It likely relies on transcription-independent functions, rather than transactivation of p53. Several studies focus on the transcription-independent functions of p53 in DNA repair (Williams and Schumacher, 2016; Ho et al., 2019). One proposed mechanism by which p53 suppresses homologous recombination (HR) is through p53-mediated sequestering of Rad51 (Linke et al., 2003). In this model, direct binding of p53 to Rad51 through its C-terminal domain prevents Rad51 polymerization on ssDNA and hence HR. Our recent work provides an alternative mechanism (Wang et al., 2022). We show that PARP-dependent modification of p53 enables rapid recruitment of p53 to damage sites within a few seconds of laser microirradiation-induced DNA damage (Figures 6A–D). Rapid recruitment of p53 requires both its DNA-binding domain (DBD) and C-terminal domain (CTD) and is uncorrelated with its transcriptional activity. The rapid recruitment of p53 promotes the subsequent recruitment of upstream repair factors including 53BP1, whose recruitment promotes the NHEJ pathway (Escribano ‐ Diaz and Durocher, 2013; Gupta et al., 2014; Swift et al., 2021), and DDB1, whose recruitment initiates nucleotide excision repair (NER) (Figures 6C,E). The clearance efficiency of UV-specific damage by the NER pathway strongly depends on the early recruitment of p53. This is evident when comparing the repair efficiencies of recruitment-competent (P47S, R175P and the double QS mutant) vs recruitment-deficient (R175H, R278Q, ΔCTD) mutants of p53 (Figure 6F). Our model helps explain how tumor-derived p53 mutants promote, rather than suppress HR (Bertrand et al., 1997; Saintigny et al., 1999; Saintigny and Lopez, 2002; Linke et al., 2003), although the CTD of tumor-derived p53 mutants remain intact and are capable of interacting with Rad51. It also provides an explanation regarding how certain transcriptionally-deficient p53 mutants retain tumor suppressive functions.
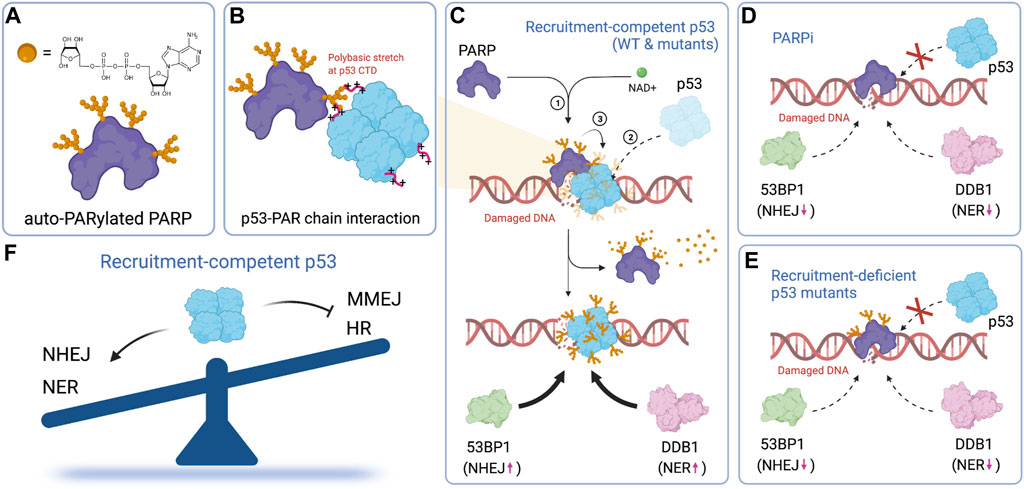
FIGURE 6. PARP-dependent rapid recruitment of p53 directs repair pathway choice. (A) Chemical structure of ADP-ribose and the illustration of auto-PARylated PARP. (B) p53 interacts with auto-PARylated PARP through its C-terminal polybasic stretch. (C) Auto-PARylated PARP drives the accumulation of p53, which promotes the recruitment of 53BP1 and DDB1 and therefore NHEJ and NER, respectively. (D) The recruitment of p53, as well as the subsequent recruitment of 53BP1 and DDB1, is suppressed by a PARP inhibitor. (E) Mutations that impair p53 rapid recruitment also suppress recruitment of 53BP1 and DDB1. (F) Early recruitment of p53 tips the balance of repair pathway selection in favor of the NHEJ and NER repair pathway in a transcription-independent fashion. Figures created with BioRender.com.
Roles of Nuclear Phosphoinositide in p53-Mediated Tumor Suppression
Because p53 mutation is involved in almost 50% of cancers, nuclear phosphoinositide-mediated regulation of p53 potentially provides a novel entry for developing therapeutic strategies. Moreover, p53 governs not only tumor suppression, but also many aspects of cellular functions including DNA repair (Sengupta and Harris, 2005; Williams and Schumacher, 2016), cell cycle arrest (Chen, 2016) and apoptosis (Aubrey et al., 2018) etc. This topic is too broad to be covered in one section and many of these topics have been separately reviewed elsewhere. Here we would like to highlight three aspects of nuclear phosphoinositide-mediated p53 regulation in human diseases.
The first is the role of PIP4Ks in tumor suppression. The question of the roles of PIP4Ks in p53-null tumors has been addressed in mouse xenograft models (Emerling et al., 2013). Those studies show that PIP4K depletion reduces tumor-dependent death in p53 null mice. At a cellular level, knockdown of PIPKIIα and PIPKIIβ increases metabolic stress and ROS, leading to senescence in a cell line lacking p53 (BT474) but not in MEFs in the same experiment. This observation indicates that partial loss of PIP4K2A and/or PIP4K2B alleles (genes encoding PIPKIIα and PIPKIIβ, respectively) may result in senescence in the context of p53-deficient tumors. Knockout of either PIP4K2B or PIP4K2A in a wildtype p53 background did not lead to a strong phenotype in both mice and tissue culture studies (Wang et al., 2019). By contrast, no mice with the PIP4K2B−/-, TP53−/− genotype emerged from the crosses, indicating synthetic lethality (Lamia et al., 2004). Additionally, PIP4K2B−/- mice showed increased sensitivity to insulin stimulated Akt activation in muscle compared to their wildtype counterparts (Lamia et al., 2004). Mice embryos with double knockout of both PIP4K2A and 2B developed normally, but died within 12 h after birth (Emerling et al., 2013). Mice with germline deletion of PIP4K2C also appeared normal in regard to growth and viability but had increased inflammation and T-cell activation as they aged (Shim et al., 2016). Since the double KO of PIP4K2A and 2B is prenatal lethal for the mice, the Cantley laboratory crossed PIP4K2A−/-, PIP4K2B+/− mice with TP53−/− mice to investigate the effect of partial loss of PIP4Ks in a p53-null background and got viable litters. While p53-deficient mice developed spontaneous tumors in approximately 4–6 months (Jacks et al., 1994), the litters from the crosses had a dramatic reduction in tumors compared to the PIP4K2A+/+, PIP4K2B+/+, TP53−/− mice. These findings stimulated the recent development of PIP4K inhibitors, which included covalent (Manz et al., 2020; Sivakumaren et al., 2020) and non-covalent inhibitors (Chen et al., 2021b). Excitingly, the noncovalent and selective inhibitors also demonstrated selective killing of p53-null tumor cells with disruption of cell energy metabolism (Chen et al., 2021b) (see review (Fiume et al., 2015)). Together, these results indicated that expression of PIPKIIα and/or β was critical for the growth of tumors with TP53 mutations or deletions, which made PIP4Ks a promising druggable target for cancer treatment.
The second aspect is the complex formation between mutant p53, nuclear PIPKIα and PI(4,5)P2, which has direct implications in tumorigenesis (Choi et al., 2019). Recent studies from the Anderson laboratory showed that depletion of PIPKIα or inhibition of its activity using a PIPKI-α inhibitor, ISA-2011B (Semenas et al., 2014), decreased the level of mutant p53. Treatment with ISA-2011B also led to a reduction of PIPKIα. By contrast, PIPKIα knockdown or ISA-2011B had no effect on wildtype p53 protein levels under unstressed conditions. While the association between wildtype p53 and PI(4,5)P2 was promoted by DNA damage, cancer cells bearing p53 mutations showed a strong interaction between p53 and PI(4,5)P2 even in resting states. The p53-PI(4,5)P2 interaction has been mapped to the CTD of p53 but it did not provide an explanation regarding how tumor-derived p53 mutants, which often carry mutations in the DBD, were differentially regulated by PI(4,5)P2 that interacted with p53 through the CTD of p53. Deciphering the molecular functions of such stable interactions between PI(4,5)P2 and mutant p53 is important.
The differential regulation of wildtype vs mutant p53 by a nuclear PI(4,5)P2 pathway has profound implications for cancer. The stability of wildtype and mutant p53s has been reported to be regulated by distinct mechanisms (Freed-Pastor and Prives, 2012). The stable association of PI(4,5)P2 with mutant p53 is unexpected and may be an underlying mechanism for the long-term stability of mutant p53s. Although PIPKIα, mutant p53 and sHSPs are independently implicated in tumor progression (Arrigo and Gibert, 2014; Muller et al., 2014; Semenas et al., 2014; Malin et al., 2016), findings indicate that these proteins form an orchestrated molecular complex that may play a central role in tumorigenesis. In addition, αB-crystallin expression correlates with p53 protein stabilization in clinically aggressive triple-negative breast cancers (Koletsa et al., 2014) and the gene encoding PIPKIα is commonly amplified in breast cancer (Waugh, 2014), indicating that this complex may contribute to the pathogenesis of these and perhaps other cancers. Given the critical role of mutant p53 stability in its oncogenic activity (Freed-Pastor and Prives, 2012; Muller et al., 2014; Alexandrova et al., 2015), these findings indicate that the PIPKIα and PIPKIα–p53–PI(4,5)P–sHSP complexes might serve as therapeutic targets for cancer.
Finally, there is a potential role for nuclear phosphoinositides in synthetic lethality by p53-mediated DNA damage repair pathway selection. As discussed previously, we find that the rapid recruitment of p53 is a newly identified property that is independent of its transcriptional activity. The ability of p53 or its mutants to be rapidly recruited to damage sites correlates with their tumor suppressive function in vivo. The finding that p53 interacts with PI(4,5)P2 upon DNA damage brings in additional factors in this process. Because the rapid recruitment of p53 strongly relies on its CTD, it appears that the polybasic stretch of p53 is critical for its interaction with auto-PARylated PARP and subsequent p53 PARylation (Wang et al., 2017; Fischbach et al., 2018). Interestingly, the same polybasic stretch is critical for p53’s interaction with PI(4,5)P2 (Choi et al., 2019). This implies that PI(4,5)P2 might play a role in regulating the PARylation of p53, which is required for its rapid recruitment to DNA damage sites and hence repair pathway selection. Furthermore, a recent interactome study validates PARP1 as a PI(4,5)P2-binding protein (Mazloumi Gavgani et al., 2021). The interactions between PARP1, PI(4,5)P2/PI(3,4,5)P3 and p53 form a new signaling axis and how nuclear PI(4,5)P2 affects DNA repair pathway selection warrants further investigation.
Conclusion
Nuclear phosphoinositides play a role in maintaining genomic stability. Genomic stability is under constant threat from both endogenous and exogenous factors, and its disruption is a hallmark of cancer (Negrini et al., 2010; Hanahan, 2022). Replication fidelity and repair of damaged DNA ensures correct genetic information is transmitted during cell division and proliferation. These processes are critical to genomic integrity and even slight deviations can result in age-associated diseases and cancer (Hoeijmakers, 2001). Further, emerging evidence shows that many of these processes involve nuclear phosphoinositides. In this review, we have focused on the roles of nuclear phosphoinositides in regulating the DNA damage response. Our recent evidence indicates that there is a basic mechanism of DNA repair pathway selection by p53 likely involving PI(4,5)P2. The signaling of nuclear phosphoinositides in response to DNA damage and particularly their roles in p53-dependent repair pathway selection potentially provide new handles for treatments through synthetic lethality.
Author Contributions
Y-HW conceived the idea. Y-HW and MS wrote the manuscript together.
Funding
MS and Y-HW were supported by Cancer Prevention and Research Institute of Texas (CPRIT) (Grant No. CPRIT-27373).
Conflict of Interest
The authors declare that the research was conducted in the absence of any commercial or financial relationships that could be construed as a potential conflict of interest.
Publisher’s Note
All claims expressed in this article are solely those of the authors and do not necessarily represent those of their affiliated organizations, or those of the publisher, the editors and the reviewers. Any product that may be evaluated in this article, or claim that may be made by its manufacturer, is not guaranteed or endorsed by the publisher.
Acknowledgments
Y-HW and MS were grateful for the support from the CPRIT foundation.
References
Alexandrova, E. M., Yallowitz, A. R., Li, D., Xu, S., Schulz, R., Proia, D. A., et al. (2015). Improving Survival by Exploiting Tumour Dependence on Stabilized Mutant P53 for Treatment. Nature 523, 352–356. doi:10.1038/nature14430
Alvarez-Venegas, R., Sadder, M., Hlavacka, A., Baluška, F., Xia, Y., Lu, G., et al. (2006). The Arabidopsis Homolog of Trithorax, ATX1, Binds Phosphatidylinositol 5-phosphate, and the Two Regulate a Common Set of Target Genes. Proc. Natl. Acad. Sci. U.S.A. 103, 6049–6054. doi:10.1073/pnas.0600944103
Andrin, C., McDonald, D., Attwood, K. M., Rodrigue, A., Ghosh, S., Mirzayans, R., et al. (2012). A Requirement for Polymerized Actin in DNA Double-Strand Break Repair. Nucleus 3, 384–395. doi:10.4161/nucl.21055
Arrigo, A.-P., and Gibert, B. (2014). HspB1, HspB5 and HspB4 in Human Cancers: Potent Oncogenic Role of Some of Their Client Proteins. Cancers 6, 333–365. doi:10.3390/cancers6010333
Aubrey, B. J., Kelly, G. L., Janic, A., Herold, M. J., and Strasser, A. (2018). How Does P53 Induce Apoptosis and How Does This Relate to P53-Mediated Tumour Suppression? Cell Death Differ. 25, 104–113. doi:10.1038/cdd.2017.169
Balla, A., and Balla, T. (2006). Phosphatidylinositol 4-kinases: Old Enzymes with Emerging Functions. Trends Cell Biol. 16, 351–361. doi:10.1016/j.tcb.2006.05.003
Banfić, H., Žižak, M., Divecha, N., and Irvine, R. F. (1993). Nuclear Diacylglycerol Is Increased during Cell Proliferation In Vivo. Biochem. J. 290, 633–636.
Barlow, C. A., Laishram, R. S., and Anderson, R. A. (2010). Nuclear Phosphoinositides: a Signaling Enigma Wrapped in a Compartmental Conundrum. Trends Cell Biol. 20, 25–35. doi:10.1016/j.tcb.2009.09.009
Bassi, C., Ho, J., Srikumar, T., Dowling, R. J. O., Gorrini, C., Miller, S. J., et al. (2013). Nuclear PTEN Controls DNA Repair and Sensitivity to Genotoxic Stress. Science 341, 395–399. doi:10.1126/science.1236188
Bawa-Khalfe, T., Yang, F.-M., Ritho, J., Lin, H.-K., Cheng, J., and Yeh, E. T. H. (2017). SENP1 Regulates PTEN Stability to Dictate Prostate Cancer Development. Oncotarget 8, 17651–17664. doi:10.18632/oncotarget.13283
Belin, B. J., Lee, T., and Mullins, R. D. (2015). Correction: DNA Damage Induces Nuclear Actin Filament Assembly by Formin-2 and Spire-1/2 that Promotes Efficient DNA Repair. eLife 4. doi:10.7554/eLife.11935
Bertrand, P., Rouillard, D., Boulet, A., Levalois, C., Soussi, T., and Lopez, B. S. (1997). Increase of Spontaneous Intrachromosomal Homologous Recombination in Mammalian Cells Expressing a Mutant P53 Protein. Oncogene 14, 1117–1122. doi:10.1038/sj.onc.1200931
Bidlingmaier, S., Wang, Y., Liu, Y., Zhang, N., and Liu, B. (2011). Comprehensive Analysis of Yeast Surface Displayed cDNA Library Selection Outputs by Exon Microarray to Identify Novel Protein-Ligand Interactions. Mol. Cell. Proteomics 10, M110.005116. doi:10.1074/mcp.m110.005116
Blind, R. D., Suzawa, M., and Ingraham, H. A. (2012). Direct Modification and Activation of a Nuclear Receptor-Pip₂ Complex by the Inositol Lipid Kinase IPMK. Sci. Signal 5, ra44. doi:10.1126/scisignal.2003111
Boehme, K. A., Kulikov, R., and Blattner, C. (2008). p53 Stabilization in Response to DNA Damage Requires Akt/PKB and DNA-PK. Proc. Natl. Acad. Sci. U.S.A. 105, 7785–7790. doi:10.1073/pnas.0703423105
Boronenkov, I. V., Loijens, J. C., Umeda, M., and Anderson, R. A. (1998). Phosphoinositide Signaling Pathways in Nuclei Are Associated with Nuclear Speckles Containing Pre-mRNA Processing Factors. MBoC 9, 3547–3560. doi:10.1091/mbc.9.12.3547
Bua, D. J., Martin, G. M., Binda, O., and Gozani, O. (2013). Nuclear Phosphatidylinositol-5-Phosphate Regulates ING2 Stability at Discrete Chromatin Targets in Response to DNA Damage. Sci. Rep. 3, 2137. doi:10.1038/srep02137
Bunce, M. W., Boronenkov, I. V., and Anderson, R. A. (2008). Coordinated Activation of the Nuclear Ubiquitin Ligase Cul3-SPOP by the Generation of Phosphatidylinositol 5-Phosphate. J. Biol. Chem. 283, 8678–8686. doi:10.1074/jbc.m710222200
Caridi, C. P., Plessner, M., Grosse, R., and Chiolo, I. (2019). Nuclear Actin Filaments in DNA Repair Dynamics. Nat. Cell Biol. 21, 1068–1077. doi:10.1038/s41556-019-0379-1
Castano, E., Yildirim, S., Fáberová, V., Krausová, A., Uličná, L., Paprčková, D., et al. (2019). Nuclear Phosphoinositides-Versatile Regulators of Genome Functions. Cells 8. doi:10.3390/cells8070649
Certo, M. T., Ryu, B. Y., Annis, J. E., Garibov, M., Jarjour, J., and Rawlings, D. J. (2011). Tracking Genome Engineering Outcome at Individ-ual DNA Breakpoints. Nat. Methods 8, 671–676.
Chakrabarti, R., Bhowmick, D., Bhargava, V., Bhar, K., and Siddhanta, A. (2013). Nuclear Pool of Phosphatidylinositol 4 Phosphate 5 Kinase 1α Is Modified by polySUMO-2 during Apoptosis. Biochem. Biophysical Res. Commun. 439, 209–214. doi:10.1016/j.bbrc.2013.08.058
Chakrabarti, R., Sanyal, S., Ghosh, A., Bhar, K., Das, C., and Siddhanta, A. (2015). Phosphatidylinositol-4-phosphate 5-Kinase 1α Modulates Ribosomal RNA Gene Silencing through its Interaction with Histone H3 Lysine 9 Trimethylation and Heterochromatin Protein HP1-α. J. Biol. Chem. 290, 20893–20903. doi:10.1074/jbc.m114.633727
Chen, J. (2016). The Cell-Cycle Arrest and Apoptotic Functions of P53 in Tumor Initiation and Progression. Cold Spring Harb. Perspect. Med. 6, a026104. doi:10.1101/cshperspect.a026104
Chen, M., Choi, S., Wen, T., Chen, C., Thapa, N., Cryns, V. L., et al. (2021). A P53-Phosphoinositide Signalosome Regulates Nuclear Akt Activation. BioRxiv. doi:10.1101/2021.1109.1117.460854
Chen, M., Wen, T., Horn, H. T., Chandrahas, V. K., Thapa, N., Choi, S., et al. (2020). The Nuclear Phosphoinositide Response to Stress. Cell Cycle 19, 268–289. doi:10.1080/15384101.2019.1711316
Chen, M. Z., Zhu, X., Sun, H.-Q., Mao, Y. S., Wei, Y., Yamamoto, M., et al. (2009). Oxidative Stress Decreases Phosphatidylinositol 4,5-Bisphosphate Levels by Deactivating Phosphatidylinositol- 4-phosphate 5-Kinase β in a Syk-dependent Manner. J. Biol. Chem. 284, 23743–23753. doi:10.1074/jbc.m109.036509
Chen, S., Chandra Tjin, C., Gao, X., Xue, Y., Jiao, H., Zhang, R., et al. (2021). Pharmacological Inhibition of PI5P4Kα/β Disrupts Cell Energy Metabolism and Selectively Kills P53-Null Tumor Cells. Proc. Natl. Acad. Sci. 118, e2002486118. doi:10.1073/pnas.2002486118
Chen, W.-Y., Lee, W.-C., Hsu, N.-C., Huang, F., and Chung, B.-C. (2004). SUMO Modification of Repression Domains Modulates Function of Nuclear Receptor 5A1 (Steroidogenic Factor-1). J. Biol. Chem. 279, 38730–38735. doi:10.1074/jbc.m405006200
Chi, Y., Zhou, B., Wang, W.-Q., Chung, S.-K., Kwon, Y.-U., Ahn, Y.-H., et al. (2004). Comparative Mechanistic and Substrate Specificity Study of Inositol Polyphosphate 5-Phosphatase Schizosaccharomyces pombe Synaptojanin and SHIP2. J. Biol. Chem. 279, 44987–44995. doi:10.1074/jbc.m406416200
Choi, S., Chen, M., Cryns, V. L., and Anderson, R. A. (2019). A Nuclear Phosphoinositide Kinase Complex Regulates P53. Nat. Cell Biol. 21, 462–475. doi:10.1038/s41556-019-0297-2
Clarke, J. H., Letcher, A. J., D'Santos, C. S., Halstead, J. R., Irvine, R. F., and Divecha, N. (2001). Inositol Lipids Are Regulated during Cell Cycle Progression in the Nuclei of Murine Erythroleukaemia Cells. Biochem. J. 357, 905–910. doi:10.1042/bj3570905
Cocco, L., Faenza, I., Fiume, R., Maria Billi, A., Gilmour, R. S., and Manzoli, F. A. (2006). Phosphoinositide-specific Phospholipase C (PI-PLC) β1 and Nuclear Lipid-dependent Signaling. Biochimica Biophysica Acta (BBA) - Mol. Cell Biol. Lipids 1761, 509–521. doi:10.1016/j.bbalip.2006.03.001
Cocco, L., Gilmour, R. S., Ognibene, A., Letcher, A. J., Manzoli, F. A., and Irvine, R. F. (1987). Synthesis of Polyphosphoinositides in Nuclei of Friend Cells. Evidence for Polyphosphoinositide Metabolism inside the Nucleus Which Changes with Cell Differentiation. Biochem. J. 248, 765–770. doi:10.1042/bj2480765
Cooke, M. S., Evans, M. D., Dizdaroglu, M., and Lunec, J. (2003). Oxidative DNA Damage: Mechanisms, Mutation, and Disease. FASEB J. 17, 1195–1214. doi:10.1096/fj.02-0752rev
Davis, W. J., Lehmann, P. Z., and Li, W. (2015). Nuclear PI3K Signaling in Cell Growth and Tumorigenesis. Front. Cell Dev. Biol. 3, 24. doi:10.3389/fcell.2015.00024
Déléris, P., Bacqueville, D., Gayral, S., Carrez, L., Salles, J. P., Perret, B., et al. (2003). SHIP-2 and PTEN Are Expressed and Active in Vascular Smooth Muscle Cell Nuclei, but Only SHIP-2 Is Associated with Nuclear Speckles. J. Biol. Chem. 278, 38884–38891. doi:10.1074/jbc.M300816200
Devries, T. A., Neville, M. C., and Reyland, M. E. (2002). Nuclear Import of PKCδ Is Required for Apoptosis: Identification of a Novel Nuclear Import Sequence. Embo J. 21, 6050–6060. doi:10.1093/emboj/cdf606
Dias, A. P., Dufu, K., Lei, H., and Reed, R. (2010). A Role for TREX Components in the Release of Spliced mRNA from Nuclear Speckle Domains. Nat. Commun. 1, 97. doi:10.1038/ncomms1103
Divecha, N., Banfić, H., and Irvine, R. F. (1993). Inositides and the Nucleus and Inositides in the Nucleus. Cell 74, 405–407. doi:10.1016/0092-8674(93)80041-c
Divecha, N., Banfić, H., and Irvine, R. F. (1991). The Polyphosphoinositide Cycle Exists in the Nuclei of Swiss 3T3 Cells under the Control of a Receptor (For IGF-I) in the Plasma Membrane, and Stimulation of the Cycle Increases Nuclear Diacylglycerol and Apparently Induces Translocation of Protein Kinase. EMBO J. 10, 3207–3214. doi:10.1002/j.1460-2075.1991.tb04883.x
Divecha, N., Letcher, A. J., Banfic, H. H., Rhee, S. G., and Irvine, R. F. (1995). Changes in the Components of a Nuclear Inositide Cycle during Differentiation in Murine Erythroleukaemia Cells. Biochem. J. 312, 63–67. doi:10.1042/bj3120063
Divecha, N., Roefs, M., Los, A., Halstead, J., Bannister, A., and D'Santos, C. (2002). Type I PIPkinases Interact with and Are Regulated by the Retinoblastoma Susceptibility Gene Product-pRB. Curr. Biol. 12, 582–587. doi:10.1016/s0960-9822(02)00769-8
Droubi, A., Bulley, S. J., Clarke, J. H., Irvine, R. F., and Jonathan, F. I. (2016). Nuclear Localizations of Phosphatidylinositol 5-phosphate 4-kinases α and β Are Dynamic and Independently Regulated during Starvation-Induced Stress. Biochem. J. 473, 2155–2163. doi:10.1042/bcj20160380
Ehm, P., Nalaskowski, M. M., Wundenberg, T., and Jücker, M. (2015). The Tumor Suppressor SHIP1 Colocalizes in Nucleolar Cavities with P53 and Components of PML Nuclear Bodies. Nucleus 6, 154–164. doi:10.1080/19491034.2015.1022701
Elong Edimo, W. S., Derua, R., Janssens, V., Nakamura, T., Vanderwinden, J.-M., Waelkens, E., et al. (2011). Evidence of SHIP2 Ser132 Phosphorylation, its Nuclear Localization and Stability. Biochem. J. 439, 391–404. doi:10.1042/bj20110173
Emerling, B. M., Poulogiannis, J. B. G., Tsukazawa, K. S., Choo-Wing, R., Wulf, G. M., Bell, E. L., et al. (2013). Depletion of a Putatively Druggable Class of Phosphatidylinositol Kinases Inhibits Growth of P53-Null Tumors. Cell 155, 844–857. doi:10.1016/j.cell.2013.09.057
Escribano‐Diaz, C., and Durocher, D. (2013). DNA Repair Pathway Choice—A PTIP of the Hat to 53BP1. EMBO Rep. 14, 665–666.
Fáberová, V., Kalasová, I., Krausová, A., and Hozák, P. (2020). Super-Resolution Localisation of Nuclear PI(4)P and Identification of its Interacting Proteome. Cells 9, 1191.
Fischbach, A., Krüger, A., Hampp, S., Assmann, G., Rank, L., Hufnagel, M., et al. (2018). The C-Terminal Domain of P53 Orchestrates the Interplay between Non-covalent and Covalent poly(ADP-Ribosyl)ation of P53 by PARP1. Nucleic Acids Res. 46, 804–822. doi:10.1093/nar/gkx1205
Fiume, R., Faenza, I., Sheth, B., Poli, A., Vidalle, M. C., Mazzetti, C., et al. (2019). Nuclear Phosphoinositides: Their Regulation and Roles in Nuclear Functions. Int. J. Mol. Sci. 20. doi:10.3390/ijms20122991
Fiume, R., Keune, W. J., Faenza, I., Bultsma, Y., Ramazzotti, G., Jones, D. R., et al. (2012). “Nuclear Phosphoinositides: Location, Regulation and Function,” in Subcellular Biochemistry (Netherlands: Springer), 335–361. doi:10.1007/978-94-007-3015-1_11
Fiume, R., Stijf-Bultsma, Y., Shah, Z. H., Keune, W. J., Jones, D. R., Jude, J. G., et al. (2015). PIP4K and the Role of Nuclear Phosphoinositides in Tumour Suppression. Biochimica Biophysica Acta (BBA) - Mol. Cell Biol. Lipids 1851, 898–910. doi:10.1016/j.bbalip.2015.02.014
Fiume, R., Teti, G., Faenza, I., and Cocco, L. (2010). Phosphoinositide-Specific Phospholipase C β1 Signal Transduction in the Nucleus. Methods Mol. Biol. 645, 143–164. doi:10.1007/978-1-60327-175-2_10
Freed-Pastor, W. A., and Prives, C. (2012). Mutant P53: One Name, Many Proteins. Genes Dev. 26, 1268–1286. doi:10.1101/gad.190678.112
Freeman, D. J., Li, A. G., Wei, G., Li, H.-H., Kertesz, N., Lesche, R., et al. (2003). PTEN Tumor Suppressor Regulates P53 Protein Levels and Activity through Phosphatase-dependent and -independent Mechanisms. Cancer Cell 3, 117–130. doi:10.1016/s1535-6108(03)00021-7
Fu, W., Ma, Q., Chen, L., Li, P., Zhang, M., Ramamoorthy, S., et al. (2009). MDM2 Acts Downstream of P53 as an E3 Ligase to Promote FOXO Ubiquitination and Degradation. J. Biol. Chem. 284, 13987–14000. doi:10.1074/jbc.m901758200
Gelato, K. A., Ong, M. M. S., Winter, S., Hiragami-Hamada, K., Sindlinger, J., Lemak, A., et al. (2014). Accessibility of Different Histone H3-Binding Domains of UHRF1 Is Allosterically Regulated by Phosphatidylinositol 5-Phosphate. Mol. Cell 54, 905–919. doi:10.1016/j.molcel.2014.04.004
Georgescu, M. M., Kirsch, K. H., Kaloudis, P., Yang, H., Pavletich, N. P., and Hanafusa, H. (2000). Stabilization and Productive Positioning Roles of the C2 Domain of PTEN Tumor Suppressor. Cancer Res. 60, 7033–7038.
Gozani, O., Karuman, P., Jones, D. R., Ivanov, D., Cha, J., Lugovskoy, A. A., et al. (2003). The PHD Finger of the Chromatin-Associated Protein ING2 Functions as a Nuclear Phosphoinositide Receptor. Cell 114, 99–111. doi:10.1016/s0092-8674(03)00480-x
Gupta, A., Hunt, C. R., Chakraborty, S., Pandita, R. K., Yordy, J., Ramnarain, D. B., et al. (2014). Role of 53BP1 in the Regulation of DNA Double-Strand Break Repair Pathway Choice. Radiat. Res. 181, 1–8. doi:10.1667/rr13572.1
Haapaniemi, E., Botla, S., Persson, J., Schmierer, B., and Taipale, J. (2018). CRISPR-Cas9 Genome Editing Induces a P53-Mediated DNA Damage Response. Nat. Med. 24, 927–930. doi:10.1038/s41591-018-0049-z
Haberkant, P., Raijmakers, R., Wildwater, M., Sachsenheimer, T., Brügger, B., Maeda, K., et al. (2013). In Vivo Profiling and Visualization of Cellular Protein-Lipid Interactions Using Bifunctional Fatty Acids. Angew. Chem. Int. Ed. 52, 4033–4038. doi:10.1002/anie.201210178
Halstead, J. R., van Rheenen, J., Snel, M. H. J., Meeuws, S., Mohammed, S., D'Santos, C. S., et al. (2006). A Role for PtdIns(4,5)P2 and PIP5Kα in Regulating Stress-Induced Apoptosis. Curr. Biol. 16, 1850–1856. doi:10.1016/j.cub.2006.07.066
Hamann, B. L., and Blind, R. D. (2018). Nuclear Phosphoinositide Regulation of Chromatin. J. Cell Physiol. 233, 107–123. doi:10.1002/jcp.25886
Hammond, G. R. V., and Balla, T. (2015). Polyphosphoinositide Binding Domains: Key to Inositol Lipid Biology. Biochimica Biophysica Acta (BBA) - Mol. Cell Biol. Lipids 1851, 746–758. doi:10.1016/j.bbalip.2015.02.013
Hanahan, D. (2022). Hallmarks of Cancer: New Dimensions. Cancer Discov. 12, 31–46. doi:10.1158/2159-8290.cd-21-1059
Heinrich, F., Chakravarthy, S., Nanda, H., Papa, A., Pandolfi, P. P., Ross, A. H., et al. (2015). The PTEN Tumor Suppressor Forms Homodimers in Solution. Structure 23, 1952–1957. doi:10.1016/j.str.2015.07.012
Ho, T., Tan, B. X., and Lane, D. (2019). How the Other Half Lives: What P53 Does when it Is Not Being a Transcription Factor. Ijms 21, 13. doi:10.3390/ijms21010013
Hoeijmakers, J. H. J. (2001). Genome Maintenance Mechanisms for Preventing Cancer. Nature 411, 366–374. doi:10.1038/35077232
Hu, J., Yuan, Q., Kang, X., Qin, Y., Li, L., Ha, Y., et al. (2015). Resolution of Structure of PIP5K1A Reveals Molecular Mechanism for its Regulation by Dimerization and Dishevelled. Nat. Commun. 6, 8205. doi:10.1038/ncomms9205
Ihry, R. J., Worringer, K. A., Salick, M. R., Frias, E., Ho, D., Theriault, K., et al. (2018). p53 Inhibits CRISPR-Cas9 Engineering in Human Pluripotent Stem Cells. Nat. Med. 24, 939–946. doi:10.1038/s41591-018-0050-6
Irvine, R. F. (2003). Nuclear Lipid Signalling. Nat. Rev. Mol. Cell Biol. 4, 349–361. doi:10.1038/nrm1100
Jacks, T., Remington, L., Williams, B. O., Schmitt, E. M., Halachmi, S., Bronson, R. T., et al. (1994). Tumor Spectrum Analysis in P53-Mutant Mice. Curr. Biol. 4, 1–7. doi:10.1016/s0960-9822(00)00002-6
Jacobsen, R. G., Mazloumi Gavgani, F., Edson, A. J., Goris, M., Altankhuyag, A., and Lewis, A. E. (2019). Polyphosphoinositides in the Nucleus: Roadmap of Their Effectors and Mechanisms of Interaction. Adv. Biol. Regul. 72, 7–21. doi:10.1016/j.jbior.2019.04.001
Jones, D. R., Bultsma, Y., Keune, W.-J., Halstead, J. R., Elouarrat, D., Mohammed, S., et al. (2006). Nuclear PtdIns5P as a Transducer of Stress Signaling: An In Vivo Role for PIP4Kbeta. Mol. Cell 23, 685–695. doi:10.1016/j.molcel.2006.07.014
Jones, D. R., Foulger, R., Keune, W. J., Bultsma, Y., and Divecha, N. (2013). PtdIns5 P Is an Oxidative Stress‐induced Second Messenger that Regulates PKB Activation. FASEB J. 27, 1644–1656. doi:10.1096/fj.12-218842
Jungmichel, S., Sylvestersen, K. B., Choudhary, C., Nguyen, S., Mann, M., and Nielsen, M. L. (2014). Specificity and Commonality of the Phosphoinositide-Binding Proteome Analyzed by Quantitative Mass Spectrometry. Cell Rep. 6, 578–591. doi:10.1016/j.celrep.2013.12.038
Kalasova, I., Fáberová, V., Kalendová, A., Yildirim, S., Uličná, L., Venit, T., et al. (2016). Tools for Visualization of Phosphoinositides in the Cell Nucleus. Histochem Cell Biol. 145, 485–496. doi:10.1007/s00418-016-1409-8
Keune, W. J., Bultsma, Y., Sommer, L., Jones, D., and Divecha, N. (2011). Phosphoinositide Signalling in the Nucleus. Adv. Enzyme Regul. 51, 91–99. doi:10.1016/j.advenzreg.2010.09.009
Kim, E., Ahn, H., Kim, M. G., Lee, H., and Kim, S. (2017). The Expanding Significance of Inositol Polyphosphate Multikinase as a Signaling Hub. Mol. Cells 40, 315–321. doi:10.14348/molcells.2017.0066
Kitamura, A., Nakayama, Y., and Kinjo, M. (2015). Efficient and Dynamic Nuclear Localization of Green Fluorescent Protein via RNA Binding. Biochem. Biophysical Res. Commun. 463, 401–406. doi:10.1016/j.bbrc.2015.05.084
Koletsa, T., Stavridi, F., Bobos, M., Kostopoulos, I., Kotoula, V., Eleftheraki, A. G., et al. (2014). alphaB-Crystallin Is a Marker of Aggressive Breast Cancer Behavior but Does Not Independently Predict for Patient Outcome: a Combined Analysis of Two Randomized Studies. BMC Clin. Pathol. 14, 28. doi:10.1186/1472-6890-14-28
Kuluncsics, Z., Perdiz, D., Brulay, E., Muel, B., and Sage, E. (1999). Wavelength Dependence of Ultraviolet-Induced DNA Damage Distribution: Involvement of Direct or Indirect Mechanisms and Possible Artefacts. J. Photochem. Photobiol. B Biol. 49, 71–80. doi:10.1016/s1011-1344(99)00034-2
Kumar, A., Fernandez-Capetillo, O., and Carrera, A. C. (2010). Nuclear Phosphoinositide 3-kinase β Controls Double-Strand Break DNA Repair. Proc. Natl. Acad. Sci. U.S.A. 107, 7491–7496. doi:10.1073/pnas.0914242107
Lamia, K. A., Peroni, O. D., Kim, Y.-B., Rameh, L. E., Kahn, B. B., and Cantley, L. C. (2004). Increased Insulin Sensitivity and Reduced Adiposity in Phosphatidylinositol 5-Phosphate 4-Kinase β −/− Mice. Mol. Cell Biol. 24, 5080–5087. doi:10.1128/mcb.24.11.5080-5087.2004
Lee, J.-O., Yang, H., Georgescu, M.-M., Di Cristofano, A., Maehama, T., Shi, Y., et al. (1999). Crystal Structure of the PTEN Tumor Suppressor. Cell 99, 323–334. doi:10.1016/s0092-8674(00)81663-3
Lee, Y.-J., Sheu, T.-J., and Keng, P. C. (2005). Enhancement of Radiosensitivity in H1299 Cancer Cells by Actin-Associated Protein Cofilin. Biochem. Biophysical Res. Commun. 335, 286–291. doi:10.1016/j.bbrc.2005.07.073
Lewis, A. E., Sommer, L., Arntzen, M. Ø., Strahm, Y., Morrice, N. A., Divecha, N., et al. (2011). Identification of Nuclear Phosphatidylinositol 4,5-Bisphosphate-Interacting Proteins by Neomycin Extraction. Mol. Cell Proteomics 10, M110–M003376. doi:10.1074/mcp.M110.003376
Li, A. G., Piluso, L. G., Cai, X., Wei, G., Sellers, W. R., and Liu, X. (2006). Mechanistic Insights into Maintenance of High P53 Acetylation by PTEN. Mol. Cell 23, 575–587. doi:10.1016/j.molcel.2006.06.028
Li, W., Laishram, R. S., and Anderson, R. A. (2013). The Novel Poly(A) Polymerase Star-PAP Is a Signal-Regulated Switch at the 3′-end of mRNAs. Adv. Biol. Regul. 53, 64–76. doi:10.1016/j.jbior.2012.10.004
Li, W., Laishram, R. S., Ji, Z., Barlow, C. A., Tian, B., and Anderson, R. A. (2012). Star-PAP Control of BIK Expression and Apoptosis Is Regulated by Nuclear PIPKIα and PKCδ Signaling. Mol. Cell 45, 25–37. doi:10.1016/j.molcel.2011.11.017
Li, W., Li, W., Laishram, R. S., Hoque, M., Ji, Z., Tian, B., et al. (2017). Distinct Regulation of Alternative Polyadenylation and Gene Expression by Nuclear Poly(A) Polymerases. Nucleic Acids Res. 45, 8930–8942. doi:10.1093/nar/gkx560
Lindsay, Y., McCoull, D., Davidson, L., Leslie, N. R., Fairservice, A., Gray, A., et al. (2006). Localization of Agonist-Sensitive PtdIns(3,4,5)P3 Reveals a Nuclear Pool that Is Insensitive to PTEN Expression. J. Cell Sci. 119, 5160–5168. doi:10.1242/jcs.000133
Linke, S. P., Sengupta, S., Khabie, N., Jeffries, B. A., Buchhop, S., Miska, S., et al. (2003). p53 Interacts with hRAD51 and hRAD54, and Directly Modulates Homologous Recombination. Cancer Res. 63, 2596–2605.
Los, A. P., De Widt, J., Topham, M. K., Van Blitterswijk, W. J., and Divecha, N. (2007). Protein Kinase C Inhibits Binding of Diacylglycerol Kinase-ζ to the Retinoblastoma Protein. Biochimica Biophysica Acta (BBA) - Mol. Cell Res. 1773, 352–357. doi:10.1016/j.bbamcr.2006.12.004
Ma, J., Benitez, J. A., Li, J., Miki, S., Ponte de Albuquerque, C., Galatro, T., et al. (2019). Inhibition of Nuclear PTEN Tyrosine Phosphorylation Enhances Glioma Radiation Sensitivity through Attenuated DNA Repair. Cancer Cell 35, 504e507–518. doi:10.1016/j.ccell.2019.01.020
Maag, D., Maxwell, M. J., Hardesty, D. A., Boucher, K. L., Choudhari, N., Hanno, A. G., et al. (2011). Inositol Polyphosphate Multikinase Is a Physiologic PI3-Kinase that Activates Akt/PKB. Proc. Natl. Acad. Sci. U.S.A. 108, 1391–1396. doi:10.1073/pnas.1017831108
Malin, D., Petrovic, V., Strekalova, E., Sharma, B., and Cryns, V. L. (2016). αB-crystallin: Portrait of a Malignant Chaperone as a Cancer Therapeutic Target. Pharmacol. Ther. 160, 1–10. doi:10.1016/j.pharmthera.2016.01.012
Manz, T. D., Sivakumaren, S. C., Yasgar, A., Hall, M. D., Davis, M. I., Seo, H.-S., et al. (2020). Structure-Activity Relationship Study of Covalent Pan-Phosphatidylinositol 5-Phosphate 4-Kinase Inhibitors. ACS Med. Chem. Lett. 11, 346–352. doi:10.1021/acsmedchemlett.9b00402
Manzoli, F. A., Maraldi, N. M., Cocco, L., Capitani, S., and Facchini, A. (1977). Chromatin Phospholipids in Normal and Chronic Lymphocytic Leukemia Lymphocytes. Cancer Res. 37, 843–849.
Martelli, A. M., Fiume, R., Faenza, I., Tabellini, G., Evangelista, C., Bortul, R., et al. (2005). Nuclear Phosphoinositide Specific Phospholipase C (PI-PLC)-beta 1: a Central Intermediary in Nuclear Lipid-dependent Signal Transduction. Histol. Histopathol. 20, 1251–1260. doi:10.14670/HH-20.1251
Martelli, A. M., Billi, A. M., Manzoli, L., Faenza, I., Aluigi, M., Falconi, M., et al. (2000). Insulin Selectively Stimulates Nuclear Phosphoinositide-specific Phospholipase C (PI-PLC) β1 Activity through a Mitogen-Activated Protein (MAP) Kinase-dependent Serine Phosphorylation. FEBS Lett. 486, 230–236. doi:10.1016/s0014-5793(00)02313-9
Martelli, A. M., Gilmour, R. S., Neri, L. M., Manzoli, L., Corps, A. N., and Cocco, L. (1991). Mitogen-stimulated Events in Nuclei of Swiss 3T3 Cells Evidence for a Direct Link between Changes of Inositol Lipids, Protein Kinase C Requirement and the Onset of DNA Synthesis. FEBS Lett. 283, 243–246. doi:10.1016/0014-5793(91)80598-w
Masuda, S., Das, R., Cheng, H., Hurt, E., Dorman, N., and Reed, R. (2005). Recruitment of the Human TREX Complex to mRNA during Splicing. Genes Dev. 19, 1512–1517. doi:10.1101/gad.1302205
Matsuoka, S., Ballif, B. A., Smogorzewska, A., McDonald, E. R., Hurov, K. E., Luo, J., et al. (2007). ATM and ATR Substrate Analysis Reveals Extensive Protein Networks Responsive to DNA Damage. Science 316, 1160–1166. doi:10.1126/science.1140321
Mayo, L. D., Dixon, J. E., Durden, D. L., Tonks, N. K., and Donner, D. B. (2002). PTEN Protects P53 from Mdm2 and Sensitizes Cancer Cells to Chemotherapy. J. Biol. Chem. 277, 5484–5489. doi:10.1074/jbc.m108302200
Mazloumi Gavgani, F., Slinning, M. S., Morovicz, A. P., Arnesen, V. S., Turcu, D. C., Ninzima, S., et al. (2021). Nuclear Phosphatidylinositol 3,4,5-Trisphosphate Interactome Uncovers an Enrichment in Nucleolar Proteins. Mol. Cell. Proteomics 20, 100102. doi:10.1016/j.mcpro.2021.100102
Mellman, D. L., Gonzales, M. L., Song, C., Barlow, C. A., Wang, P., Kendziorski, C., et al. (2008). A PtdIns4,5P2-Regulated Nuclear Poly(A) Polymerase Controls Expression of Select mRNAs. Nature 451, 1013–1017. doi:10.1038/nature06666
Meyer, R., Nalaskowski, M. M., Ehm, P., Schröder, C., Naj, X., Brehm, M. A., et al. (2012). Nucleocytoplasmic Shuttling of Human Inositol Phosphate Multikinase Is Influenced by CK2 Phosphorylation. Biol. Chem. 393, 149–160. doi:10.1515/hsz-2011-0209
Milella, M., Falcone, I., Conciatori, F., Cesta Incani, U., Del Curatolo, A., Inzerilli, N., et al. (2015). PTEN: Multiple Functions in Human Malignant Tumors. Front. Oncol. 5, 24. doi:10.3389/fonc.2015.00024
Millard, C. J., Celardo, P. J. I., Gordiyenko, Y., Cowley, S. M., Robinson, C. V., Fairall, L., et al. (2013). Class I HDACs Share a Common Mechanism of Regulation by Inositol Phosphates. Mol. Cell 51, 57–67. doi:10.1016/j.molcel.2013.05.020
Ming, M., Feng, L., Shea, C. R., Soltani, K., Zhao, B., Han, W., et al. (2011). PTEN Positively Regulates UVB-Induced DNA Damage Repair. Cancer Res. 71, 5287–5295. doi:10.1158/0008-5472.can-10-4614
Ming, M., and He, Y.-Y. (2012). PTEN in DNA Damage Repair. Cancer Lett. 319, 125–129. doi:10.1016/j.canlet.2012.01.003
Moriel-Carretero, M. (2021). The Many Faces of Lipids in Genome Stability (And How to Unmask Them). Int. J. Mol. Sci. 22. doi:10.3390/ijms222312930
Muller, P. A. J., Vousden, K. H., and Vousden, H. (2014). Mutant P53 in Cancer: New Functions and Therapeutic Opportunities. Cancer Cell 25, 304–317. doi:10.1016/j.ccr.2014.01.021
Muslimović, A., Nyström, S., Gao, Y., and Hammarsten, O. (2009). Numerical Analysis of Etoposide Induced DNA Breaks. PLoS ONE 4, e5859. doi:10.1371/journal.pone.0005859
Nagashima, M., Shiseki, M., Miura, K., Hagiwara, K., Linke, S. P., Pedeux, R., et al. (2001). DNA Damage-Inducible Gene p33ING2 Negatively Regulates Cell Proliferation through Acetylation of P53. Proc. Natl. Acad. Sci. U.S.A. 98, 9671–9676. doi:10.1073/pnas.161151798
Nakano, T., Akamatsu, K., Tsuda, M., Tujimoto, A., Hirayama, R., Hiromoto, T., et al. (2022). Formation of Clustered DNA Damage In Vivo upon Irradiation with Ionizing Radiation: Visualization and Analysis with Atomic Force Microscopy. Proc. Natl. Acad. Sci. U. S. A. 119, e2119132119. doi:10.1073/pnas.2119132119
Ndamukong, I., Jones, D. R., Lapko, H., Divecha, N., and Avramova, Z. (2010). Phosphatidylinositol 5-Phosphate Links Dehydration Stress to the Activity of ARABIDOPSIS TRITHORAX-LIKE Factor ATX1. PLoS ONE 5, e13396. doi:10.1371/journal.pone.0013396
Negrini, S., Gorgoulis, V. G., and Halazonetis, T. D. (2010). Genomic Instability - an Evolving Hallmark of Cancer. Nat. Rev. Mol. Cell Biol. 11, 220–228. doi:10.1038/nrm2858
Neri, L. M., Bortul, R., Borgatti, P., Tabellini, G., Baldini, G., Capitani, S., et al. (2002). Proliferating or Differentiating Stimuli Act on Different Lipid-dependent Signaling Pathways in Nuclei of Human Leukemia Cells. MBoC 13, 947–964. doi:10.1091/mbc.01-02-0086
Neri, L. M., Ricci, D., Carini, C., Marchisio, M., Capitani, S., and Bertagnolo, V. (1997). Changes of Nuclear PI-PLC γ1 during Rat Liver Regeneration. Cell. Signal. 9, 353–362. doi:10.1016/s0898-6568(96)00178-7
Ogiso, H., Nakamura, K., Yatomi, Y., Shimizu, T., and Taguchi, R. (2010). Liquid Chromatography/mass Spectrometry Analysis Revealing Preferential Occurrence of Non-arachidonate-containing Phosphatidylinositol Bisphosphate Species in Nuclei and Changes in Their Levels during Cell Cycle. Rapid Commun. Mass Spectrom. 24, 436–442. doi:10.1002/rcm.4415
Okada, M., Jang, S.-W., and Ye, K. (2008). Akt Phosphorylation and Nuclear Phosphoinositide Association Mediate mRNA Export and Cell Proliferation Activities by ALY. Proc. Natl. Acad. Sci. U.S.A. 105, 8649–8654. doi:10.1073/pnas.0802533105
Olsen, B. B., Wang, S.-Y., Svenstrup, T. H., Chen, B. P., and Guerra, B. (2012). Protein Kinase CK2 Localizes to Sites of DNA Double-Strand Break Regulating the Cellular Response to DNA Damage. BMC Mol. Biol. 13, 7. doi:10.1186/1471-2199-13-7
Osborne, S. L., Thomas, C. L., Gschmeissner, S., and Schiavo, G. (2001). Nuclear PtdIns(4,5)P2 Assembles in a Mitotically Regulated Particle Involved in Pre-mRNA Splicing. J. Cell Sci. 114, 2501–2511. doi:10.1242/jcs.114.13.2501
Payrastre, B., Nievers, M., Boonstra, J., Breton, M., Verkleij, A. J., and Van Bergen en Henegouwen, P. M. (1992). A Differential Location of Phosphoinositide Kinases, Diacylglycerol Kinase, and Phospholipase C in the Nuclear Matrix. J. Biol. Chem. 267, 5078–5084. doi:10.1016/s0021-9258(18)42732-9
Pedeux, R., Sengupta, S., Shen, J. C., Demidov, O. N., Saito, S. i., Onogi, H., et al. (2005). ING2 Regulates the Onset of Replicative Senescence by Induction of P300-dependent P53 Acetylation. Mol. Cell Biol. 25, 6639–6648. doi:10.1128/mcb.25.15.6639-6648.2005
Piazzi, M., Blalock, W. L., Bavelloni, A., Faenza, I., D'Angelo, A., Maraldi, N. M., et al. (2013). Phosphoinositide-specific Phospholipase C β 1b (PI-PLCβ1b) Interactome: Affinity Purification-Mass Spectrometry Analysis of PI-PLCβ1b with Nuclear Protein. Mol. Cell. Proteomics 12, 2220–2235. doi:10.1074/mcp.m113.029686
Planchon, S. M., Waite, K. A., and Eng, C. (2008). The Nuclear Affairs of PTEN. J. Cell Sci. 121, 249–253. doi:10.1242/jcs.022459
Raingeaud, J., Gupta, S., Rogers, J. S., Dickens, M., Han, J., Ulevitch, R. J., et al. (1995). Pro-inflammatory Cytokines and Environmental Stress Cause P38 Mitogen-Activated Protein Kinase Activation by Dual Phosphorylation on Tyrosine and Threonine. J. Biol. Chem. 270, 7420–7426. doi:10.1074/jbc.270.13.7420
Rana, R. A., Cataldi, A., Pietro, R. D., Mazzotti, G., Centurione, L., Robuffo, I., et al. (1994). Evidence for an Early and Transient Involvement of Nuclear Inositol Lipids in Subcellular Signalling Events Related to DNA Repair Processes. Cell. Signal. 6, 475–480. doi:10.1016/0898-6568(94)90095-7
Rando, O. J., Zhao, K., Janmey, P., and Crabtree, G. R. (2002). Phosphatidylinositol-dependent Actin Filament Binding by the SWI/SNF-like BAF Chromatin Remodeling Complex. Proc. Natl. Acad. Sci. U.S.A. 99, 2824–2829. doi:10.1073/pnas.032662899
Ratti, S., Evangelisti, C., Mongiorgi, S., De Stefano, A., Fazio, A., Bonomini, F., et al. (2021). "Modulating Phosphoinositide Profiles as a Roadmap for Treatment in Acute Myeloid Leukemia". Front. Oncol. 11, 678824. doi:10.3389/fonc.2021.678824
Ratti, S., Mongiorgi, S., Ramazzotti, G., Follo, M. Y., Mariani, G. A., Suh, P.-G., et al. (2017). Nuclear Inositide Signaling via Phospholipase C. J. Cell. Biochem. 118, 1969–1978. doi:10.1002/jcb.25894
Resnick, A. C., Snowman, A. M., Kang, B. N., Hurt, K. J., Snyder, S. H., and Saiardi, A. (2005). Inositol Polyphosphate Multikinase Is a Nuclear PI3-Kinase with Transcriptional Regulatory Activity. Proc. Natl. Acad. Sci. U.S.A. 102, 12783–12788. doi:10.1073/pnas.0506184102
Rose, H. G., and Frenster, J. H. (1965). Composition and Metabolism of Lipids within Repressed and Active Chromatin of Interphase Lymphocytes. Biochimica Biophysica Acta (BBA) - Lipids Lipid Metabolism 106, 577–591. doi:10.1016/0005-2760(65)90073-1
Rowland, M. M., Bostic, H. E., Gong, D., Speers, A. E., Lucas, N., Cho, W., et al. (2011). Phosphatidylinositol 3,4,5-Trisphosphate Activity Probes for the Labeling and Proteomic Characterization of Protein Binding Partners. Biochemistry 50, 11143–11161. doi:10.1021/bi201636s
Saintigny, Y., and Lopez, B. S. (2002). Homologous Recombination Induced by Replication Inhibition, Is Stimulated by Expression of Mutant P53. Oncogene 21, 488–492. doi:10.1038/sj.onc.1205040
Saintigny, Y., Rouillard, D., Chaput, B., Soussi, T., and Lopez, B. S. (1999). Mutant P53 Proteins Stimulate Spontaneous and Radiation-Induced Intrachromosomal Homologous Recombination Independently of the Alteration of the Transactivation Activity and of the G1 Checkpoint. Oncogene 18, 3553–3563. doi:10.1038/sj.onc.1202941
Schill, N. J., Anderson, R. A., and AndersonRichard, A. (2009). Two Novel Phosphatidylinositol-4-Phosphate 5-kinase Type Iγ Splice Variants Expressed in Human Cells Display Distinctive Cellular Targeting. Biochem. J. 422, 473–482. doi:10.1042/bj20090638
Semenas, J., Hedblom, A., Miftakhova, R. R., Sarwar, M., Larsson, R., Shcherbina, L., et al. (2014). The Role of PI3K/AKT-Related PIP5K1α and the Discovery of its Selective Inhibitor for Treatment of Advanced Prostate Cancer. Proc. Natl. Acad. Sci. U. S. A. 111, E3689–E3698. doi:10.1073/pnas.1405801111
Sengupta, S., and Harris, C. C. (2005). p53: Traffic Cop at the Crossroads of DNA Repair and Recombination. Nat. Rev. Mol. Cell Biol. 6, 44–55. doi:10.1038/nrm1546
Shah, P., Zhao, B., Qiang, L., and He, Y.-Y. (2018). Phosphorylation of Xeroderma Pigmentosum Group C Regulates Ultraviolet-Induced DNA Damage Repair. Nucleic Acids Res. 46, 5050–5060. doi:10.1093/nar/gky239
Shah, Z. H., Jones, D. R., Sommer, L., Foulger, R., Bultsma, Y., D'Santos, C., et al. (2013). Nuclear Phosphoinositides and Their Impact on Nuclear Functions. Febs J. 280, 6295–6310. doi:10.1111/febs.12543
Shen, W. H., Balajee, A. S., Wang, J., Wu, H., Eng, C., Pandolfi, P. P., et al. (2007). Essential Role for Nuclear PTEN in Maintaining Chromosomal Integrity. Cell 128, 157–170. doi:10.1016/j.cell.2006.11.042
Shen, X., Xiao, H., Ranallo, R., Wu, W.-H., and Wu, C. (2003). Modulation of ATP-dependent Chromatin-Remodeling Complexes by Inositol Polyphosphates. Science 299, 112–114. doi:10.1126/science.1078068
Shi, X., Hong, T., Walter, K. L., Ewalt, M., Michishita, E., Hung, T., et al. (2006). ING2 PHD Domain Links Histone H3 Lysine 4 Methylation to Active Gene Repression. Nature 442, 96–99. doi:10.1038/nature04835
Shim, H., Wu, C., Ramsamooj, S., Bosch, K. N., Chen, Z., Emerling, B. M., et al. (2016). Deletion of the Gene Pip4k2c , a Novel Phosphatidylinositol Kinase, Results in Hyperactivation of the Immune System. Proc. Natl. Acad. Sci. U.S.A. 113, 7596–7601. doi:10.1073/pnas.1600934113
Sierra Potchanant, E. A., Cerabona, D., Sater, Z. A., He, Y., Sun, Z., Gehlhausen, J., et al. (2017). INPP5E Preserves Genomic Stability through Regulation of Mitosis. Mol. Cell Biol. 37. doi:10.1128/mcb.00389-17
Sivakumaren, S. C., Shim, H., Zhang, T., Ferguson, F. M., Lundquist, M. R., Browne, C. M., et al. (2020). Targeting the PI5P4K Lipid Kinase Family in Cancer Using Covalent Inhibitors. Cell Chem. Biol. 27, 525–537. e526. doi:10.1016/j.chembiol.2020.02.003
Smith, C. D., and Wells, W. W. (1984). Characterization of a Phosphatidylinositol 4-phosphate-specific Phosphomonoesterase in Rat Liver Nuclear Envelopes. Archives Biochem. Biophysics 235, 529–537. doi:10.1016/0003-9861(84)90226-1
Smith, C. D., and Wells, W. W. (1983). Phosphorylation of Rat Liver Nuclear Envelopes. II. Characterization of In Vitro Lipid Phosphorylation. J. Biol. Chem. 258, 9368–9373. doi:10.1016/s0021-9258(17)44677-1
Smith, K. T., Martin-Brown, S. A., Florens, L., Washburn, M. P., and Workman, J. L. (2010). Deacetylase Inhibitors Dissociate the Histone-Targeting ING2 Subunit from the Sin3 Complex. Chem. Biol. 17, 65–74. doi:10.1016/j.chembiol.2009.12.010
Stambolic, V., MacPherson, D., Sas, D., Lin, Y., Snow, B., Jang, Y., et al. (2001). Regulation of PTEN Transcription by P53. Mol. Cell 8, 317–325. doi:10.1016/s1097-2765(01)00323-9
Steger, D. J., Haswell, E. S., Miller, A. L., Wente, S. R., and O'Shea, E. K. (2003). Regulation of Chromatin Remodeling by Inositol Polyphosphates. Science 299, 114–116. doi:10.1126/science.1078062
Stijf-Bultsma, Y., Sommer, L., Tauber, M., Baalbaki, M., Giardoglou, P., Jones, D. R., et al. (2015). The Basal Transcription Complex Component TAF3 Transduces Changes in Nuclear Phosphoinositides into Transcriptional Output. Mol. Cell 58, 453–467. doi:10.1016/j.molcel.2015.03.009
Swift, M. L., Beishline, K., Flashner, S., and Azizkhan-Clifford, J. (2021). DSB Repair Pathway Choice Is Regulated by Recruitment of 53BP1 through Cell Cycle-dependent Regulation of Sp1. Cell Rep. 34, 108840. doi:10.1016/j.celrep.2021.108840
Sztacho, M., Sobol, M., Balaban, C., Escudeiro Lopes, S. E., and Hozák, P. (2019). Nuclear Phosphoinositides and Phase Separation: Important Players in Nuclear Compartmentalization. Adv. Biol. Regul. 71, 111–117. doi:10.1016/j.jbior.2018.09.009
Taylor, V., Wong, M., Brandts, C., Reilly, L., Dean, N. M., Cowsert, L. M., et al. (2000). 5′ Phospholipid Phosphatase SHIP-2 Causes Protein Kinase B Inactivation and Cell Cycle Arrest in Glioblastoma Cells. Mol. Cell Biol. 20, 6860–6871. doi:10.1128/mcb.20.18.6860-6871.2000
Toska, E., Shandilya, J., Goodfellow, S. J., Medler, K. F., and Roberts, S. G. E. (2014). Prohibitin Is Required for Transcriptional Repression by the WT1-BASP1 Complex. Oncogene 33, 5100–5108. doi:10.1038/onc.2013.447
Trotman, L. C., and Pandolfi, P. P. (2003). PTEN and P53: Who Will Get the Upper Hand? Cancer Cell 3, 97–99. doi:10.1016/s1535-6108(03)00022-9
Ulicna, L., Kalendova, A., Kalasova, I., Vacik, T., and Hozák, P. (2018). PIP2 Epigenetically Represses rRNA Genes Transcription Interacting with PHF8. Biochimica Biophysica Acta (BBA) - Mol. Cell Biol. Lipids 1863, 266–275. doi:10.1016/j.bbalip.2017.12.008
Ungewickell, A., Hugge, C., Kisseleva, M., Chang, S.-C., Zou, J., Feng, Y., et al. (2005). The Identification and Characterization of Two Phosphatidylinositol-4,5-Bisphosphate 4-phosphatases. Proc. Natl. Acad. Sci. U.S.A. 102, 18854–18859. doi:10.1073/pnas.0509740102
Vann, R. L., Wooding, P. F. B., Irvine, F. R., and Divecha, N. (1997). Metabolism and Possible Compartmentalization of Inositol Lipids in Isolated Rat-Liver Nuclei. Biochem. J. 327, 569–576. doi:10.1042/bj3270569
Vélez-Cruz, R., Manickavinayaham, S., Biswas, A. K., Clary, R. W., Premkumar, T., Cole, F., et al. (2016). RB Localizes to DNA Double-Strand Breaks and Promotes DNA End Resection and Homologous Recombination through the Recruitment of BRG1. Genes & Dev. 30, 2500–2512. doi:10.1101/gad.288282.116
Viiri, K., Mäki, M., and Lohi, O. (2012). Phosphoinositides as Regulators of Protein-Chromatin Interactions. Sci. Signal 5, pe19. doi:10.1126/scisignal.2002917
Viiri, K. M., Jänis, J., Siggers, T., Heinonen, T. Y. K., Valjakka, J., Bulyk, M. L., et al. (2009). DNA-binding and -Bending Activities of SAP30L and SAP30 Are Mediated by a Zinc-dependent Module and Monophosphoinositides. Mol. Cell Biol. 29, 342–356. doi:10.1128/mcb.01213-08
Vítor, A. C., Huertas, P., Legube, G., and de Almeida, S. F. (2020). Studying DNA Double-Strand Break Repair: An Ever-Growing Toolbox. Front. Mol. Biosci. 7, 24.
Wang, D. G., Paddock, M. N., Lundquist, M. R., Sun, J. Y., Mashadova, O., Amadiume, S., et al. (2019). PIP4Ks Suppress Insulin Signaling through a Catalytic-independent Mechanism. Cell Rep. 27, 1991–2001. e1995. doi:10.1016/j.celrep.2019.04.070
Wang, F., Marshall, C. B., Yamamoto, K., Li, G.-Y., Plevin, M. J., You, H., et al. (2008). Biochemical and Structural Characterization of an Intramolecular Interaction in FOXO3a and its Binding with P53. J. Mol. Biol. 384, 590–603. doi:10.1016/j.jmb.2008.09.025
Wang, Y.-H., Hariharan, A., Bastianello, G., Toyama, Y., Shivashankar, G. V., Foiani, M., et al. (2017). DNA Damage Causes Rapid Accumulation of Phosphoinositides for ATR Signaling. Nat. Commun. 8, 2118. doi:10.1038/s41467-017-01805-9
Wang, Y. H., Ho, T. L. F., Hariharan, A., Goh, H. C., Wong, Y. L., Verkaik, N. S., et al. (2022). Rapid Recruitment of P53 to DNA Damage Sites Directs DNA Repair Choice and Integrity. Proc. Natl. Acad. Sci. U. S. A. 119, e2113233119. doi:10.1073/pnas.2113233119
Watson, P. J., Fairall, L., Santos, G. M., and Schwabe, J. W. R. (2012). Structure of HDAC3 Bound to Co-repressor and Inositol Tetraphosphate. Nature 481, 335–340. doi:10.1038/nature10728
Watson, P. J., Millard, C. J., Riley, A. M., Robertson, N. S., Wright, L. C., Godage, H. Y., et al. (2016). Insights into the Activation Mechanism of Class I HDAC Complexes by Inositol Phosphates. Nat. Commun. 7, 11262. doi:10.1038/ncomms11262
Watt, S. A., Kular, G., Fleming, I. N., Downes, C. P., and Lucocq, J. M. (2002). Subcellular Localization of Phosphatidylinositol 4,5-bisphosphate Using the Pleckstrin Homology Domain of Phospholipase C δ1. Biochem. J. 363, 657–666. doi:10.1042/bj3630657
Waugh, M. G. (2014). Amplification of Chromosome 1q Genes Encoding the Phosphoinositide Signalling Enzymes PI4KB, AKT3, PIP5K1A and PI3KC2B in Breast Cancer. J. Cancer 5, 790–796. doi:10.7150/jca.9794
Weßbecher, I. M., Hinrichsen, I., Funke, S., Oellerich, T., Plotz, G., Zeuzem, S., et al. (2018). DNA Mismatch Repair Activity of MutLα Is Regulated by CK2-dependent Phosphorylation of MLH1 (S477). Mol. Carcinog. 57, 1723–1734. doi:10.1002/mc.22892
Wickramasinghe, V. O., Chavali, J. M. S., Jonsdottir, A. B., Rajendra, E., Grüner, T., Laskey, R. A., et al. (2013). Human Inositol Polyphosphate Multikinase Regulates Transcript-Selective Nuclear mRNA Export to Preserve Genome Integrity. Mol. Cell 51, 737–750. doi:10.1016/j.molcel.2013.08.031
Williams, A. B., and Schumacher, B. (2016). p53 in the DNA-Damage-Repair Process. Cold Spring Harb. Perspect. Med. 6, a026070. doi:10.1101/cshperspect.a026070
Wood, C. D., Thornton, T. M., Sabio, G., Davis, R. A., and Rincon, M. (2009). Nuclear Localization of P38 MAPK in Response to DNA Damage. Int. J. Biol. Sci. 5, 428–437. doi:10.7150/ijbs.5.428
Xu, R., Sen, N., Paul, B. D., Snowman, A. M., Rao, F., Vandiver, M. S., et al. (2013). Inositol Polyphosphate Multikinase Is a Coactivator of P53-Mediated Transcription and Cell Death. Sci. Signal 6, ra22. doi:10.1126/scisignal.2003405
Xu, R., and Snyder, S. H. (2013). Gene Transcription by P53 Requires Inositol Polyphosphate Multikinase as a Co-activator. Cell Cycle 12, 1819–1820. doi:10.4161/cc.25119
Yasui, M., Matsuoka, S., and Ueda, M. (2014). PTEN Hopping on the Cell Membrane Is Regulated via a Positively-Charged C2 Domain. PLoS Comput. Biol. 10, e1003817. doi:10.1371/journal.pcbi.1003817
Yata, K., Lloyd, J., Maslen, S., Bleuyard, J.-Y., Skehel, M., Smerdon, S. J., et al. (2012). Plk1 and CK2 Act in Concert to Regulate Rad51 during DNA Double Strand Break Repair. Mol. Cell 45, 371–383. doi:10.1016/j.molcel.2011.12.028
York, J. D., Odom, A. R., Murphy, R., Ives, E. B., and Wente, S. R. (1999). A Phospholipase C-dependent Inositol Polyphosphate Kinase Pathway Required for Efficient Messenger RNA Export. Science 285, 96–100. doi:10.1126/science.285.5424.96
Yu, H., Fukami, K., Watanabe, Y., Ozaki, C., and Takenawa, T. (1998). Phosphatidylinositol 4,5-bisphosphate Reverses the Inhibition of RNA Transcription Caused by Histone H1. Eur. J. Biochem. 251, 281–287. doi:10.1046/j.1432-1327.1998.2510281.x
Zhao, K., Wang, W., Rando, O. J., Xue, Y., Swiderek, K., Kuo, A., et al. (1998). Rapid and Phosphoinositol-dependent Binding of the SWI/SNF-like BAF Complex to Chromatin after T Lymphocyte Receptor Signaling. Cell 95, 625–636. doi:10.1016/s0092-8674(00)81633-5
Keywords: p53, DNA repair, DNA damage response (DDR), nuclear phosphoinositide signaling, repair pathway choice, stress response
Citation: Wang Y-H and Sheetz MP (2022) When PIP2 Meets p53: Nuclear Phosphoinositide Signaling in the DNA Damage Response. Front. Cell Dev. Biol. 10:903994. doi: 10.3389/fcell.2022.903994
Received: 25 March 2022; Accepted: 19 April 2022;
Published: 13 May 2022.
Edited by:
Federico Gulluni, University of Turin, ItalyReviewed by:
Roberta Fiume, University of Bologna, ItalyRaymond Blind, Vanderbilt University Medical Center, United States
Copyright © 2022 Wang and Sheetz. This is an open-access article distributed under the terms of the Creative Commons Attribution License (CC BY). The use, distribution or reproduction in other forums is permitted, provided the original author(s) and the copyright owner(s) are credited and that the original publication in this journal is cited, in accordance with accepted academic practice. No use, distribution or reproduction is permitted which does not comply with these terms.
*Correspondence: Michael P. Sheetz, bWlzaGVldHpAdXRtYi5lZHU=