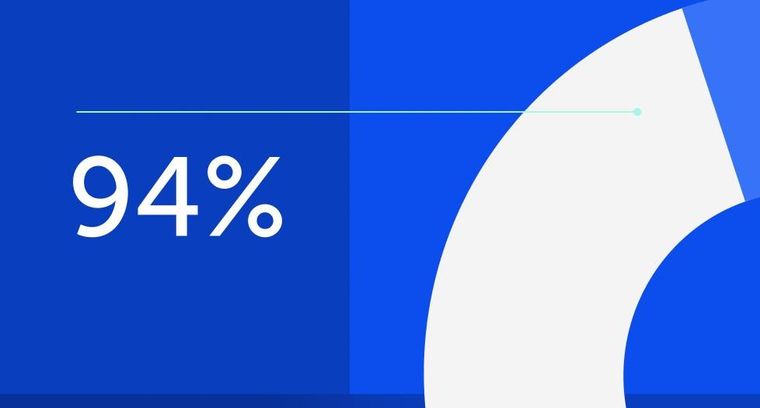
94% of researchers rate our articles as excellent or good
Learn more about the work of our research integrity team to safeguard the quality of each article we publish.
Find out more
METHODS article
Front. Cell Dev. Biol., 30 May 2022
Sec. Stem Cell Research
Volume 10 - 2022 | https://doi.org/10.3389/fcell.2022.898560
This article is part of the Research TopicCell-Based Neurodegenerative Disease ModelingView all 6 articles
α-Synuclein (αSyn) is a small, disordered protein that becomes aggregated in Lewy body diseases, such as Parkinson’s disease (PD) and dementia with Lewy bodies (DLB). Human induced pluripotent stem cells (hiPSCs) potentially provide a tractable disease model to monitor early molecular changes associated with PD/DLB. We and others have previously derived hiPSC lines from patients with duplication and triplication of the SNCA gene, encoding for αSyn. It is now recognised that to perform meaningful disease modelling with these hiPSC lines, it is critical to generate isogenic control cell lines that lack the disease causing mutations. In order to complement the existing and emerging hiPSC models for PD/DLB, we have generated an allelic series of αSyn over-expressing hESC lines on the same isogenic background. An unresolved question is whether pluripotent stem cell lines, with elevated levels of αSyn, can undergo efficient differentiation into dopaminergic and cortical neurons to model PD and DLB, respectively. We took advantage of our isogenic collection of hESC lines to determine if increased expression of αSyn affects neural induction and neuronal differentiation. Clonal hESC lines with significantly different levels of αSyn expression proliferated normally and maintained expression of pluripotent markers, such as OCT4. All cell lines efficiently produced PAX6+ neuroectoderm and there was no correlation between αSyn expression and neural induction efficiency. Finally, global transcriptomic analysis of cortical differentiation of hESC lines with low or high levels of αSyn expression demonstrated robust and similar induction of cortical neuronal expression profiles. Gene expression differences observed were unrelated to neural induction and neuronal differentiation. We conclude that elevated expression of αSyn in human pluripotent stem cells does not adversely affect their neuronal differentiation potential and that collections of isogenic cell lines with differing levels of αSyn expression are valid and suitable models to investigate synucleinopathies.
Multiple lines of evidence have implicated αSyn as a major pathological driver in PD (Polymeropoulos et al., 1997; Spillantini et al., 1997; Singleton et al., 2003; Chartier-Harlin et al., 2004). The genetic forms of PD have led to the development of patient-derived and engineered pluripotent stem cell models to gain mechanistic insights into synucleinopathies (Singh Dolt et al., 2017). A key question for the validity of such models is whether mutant or elevated αSyn expression disrupts early neural induction or neuronal differentiation, thereby limiting later phenotypic analysis and disease modelling. Current data in the literature is conflicting, with some studies proposing that high levels of αSyn alter cell fate and differentiation (Schneider et al., 2007; Oliveira et al., 2015), whereas others have found no evidence for impaired neurogenesis (Devine et al., 2011; Prots et al., 2018; Brazdis et al., 2020). Furthermore, αSyn is known to bind DNA and alter expression of other genes, however the exact mechanism of this affect is not known (Surguchev and Surguchov, 2017). In this study, we aimed to generate an isogenic collection of hESC lines with differing levels of αSyn expression and use this to systematically investigate if elevated αSyn expression affects cortical neuron differentiation. If either the efficiency of neural induction or fidelity of neuronal differentiation is affected, then this may be a source of bias in studies comparing cultured neurons with differential αSyn expression. Isogenic cellular models provide the best experimental system to test this directly.
Studies utilising αSyn null mouse models showed no major differences in neuronal development and overall brain structure (Abeliovich et al., 2000; Specht and Schoepfer, 2001; Greten-Harrison et al., 2010). However, subtle differences in dopaminergic neurotransmission were reported and these were age-dependent (Al-Wandi et al., 2010; Anwar et al., 2011; Connor-Robson et al., 2016). Rodent models employing transgenic over-expression of wild-type αSyn have reported impaired adult neurogenesis, but have not determined whether this is a neurodevelopmental defect from birth or a toxic effect of αSyn over-expression (Winner et al., 2012). We have previously shown that hiPSCs with an SNCA triplication mutation have a two-fold increase in αSyn protein levels, and this did not significantly impair dopaminergic neuronal differentiation (Devine et al., 2011). This was also shown by other independent groups using hiPSCs harbouring the SNCA triplication (Byers et al., 2011; Lin et al., 2016) and SNCA duplication mutations (Prots et al., 2018; Brazdis et al., 2020). However, other studies using SNCA triplication mutation-derived hiPSCs reported reduced neuronal differentiation capacity and impaired neurite outgrowth (Flierl et al., 2014; Oliveira et al., 2015). Furthermore, other studies using lentiviral-mediated Dox-inducible expression of αSyn, in human iPSC-derived neuronal progenitors, reported altered cell fate and impaired differentiation of neural stem cells into neurons (Schneider et al., 2007; Zasso et al., 2018). The cellular and rodent data described so far report conflicting roles for αSyn in neurogenesis, highlighting the need for work to clarify this point.
In this study, we generated an allelic series of clonal isogenic hESC lines expressing a broad range of αSyn, including lines expressing the protein at supraphysiological levels. We then set out to determine whether elevated αSyn expression impairs cortical neuron differentiation of human pluripotent stem cells. We show, using marker analysis during multiple rounds of differentiation and unbiased transcriptomic analysis, that cortical neuron differentiation is not impaired by increased expression of αSyn. The strength of our approach is the use of an isogenic collection of hESC lines with differing and sustained αSyn expression during differentiation, robust cortical neuron differentiation protocols and unbiased analysis of the transcriptome before and after differentiation. This study provides a valuable collection of cell lines for the neuroscience community and provides important evidence for the validity of hESC/iPSC disease models with elevated levels of αSyn expression.
An allelic series of clonal transgenic hESC lines was established using a human SNCA cDNA expression cassette driven by the pCAG promoter (Figure 1A), reported to maintain stable and ubiquitous transgene expression across diverse cell types (Hitoshi et al., 1991; Liew et al., 2007). Multiple puromycin-resistant clones were established on a parental Shef4 hESC line and examined for SNCA expression using qRT-PCR (Figure 1B). In an undifferentiated state, 11 clones (S5, S7, S9, S10, S12, S13, S17, S20, S21, S22, and S34) had similar SNCA expression to Shef4, whereas 18 clones (S2, S4, S6, S8, S11, S14, S15, S16, S18, S19, S23, S24, S30, S32, S33, S35, S36, and S37) had elevated expression of SNCA (4-fold–30-fold) relative to the parental cell line (Figure 1B). V2 and V39 control lines, transfected with a control plasmid lacking SNCA, expressed similar levels of SNCA to the parental cell line, Shef4. Clones were selected based on stability of SNCA mRNA expression and placed into low (Shef4, S9, S34) and high (S8, S37) groups. Each of these lines similarly expressed the pluripotency marker, OCT4, and maintained hESC morphology in their undifferentiated state (Figure 1C). Elevated expression of αSyn protein was confirmed for selected clones using immunohistochemistry and western blotting (Figures 1C,D).
FIGURE 1. Establishment of clonal hESC lines over-expressing ⍺Syn. (A) Schematic of pCAG-SNCA-IRES-Venus and pCAG-IRES-Venus constructs transfected into Shef4 hESCs to generate clonal lines. (B) Quantitative RT-PCR measuring total SNCA expression (mRNA) levels in multiple self-renewing undifferentiated transgenic clones generated from parental Shef4 hESC lines. Data was normalised to 18S rRNA levels, and shown relative to expression in the parental hESC Shef4 line. Each bar represents the mean and standard deviation of three technical replicates. (C) Representative immunocytochemistry images of undifferentiated hESC clones derived from the parental cell line Shef4, and transgenic clonal lines S9, S34, S8, and S37, stained for DAPI (blue), OCT4 (red), and αSyn (green). Scale bar = 50 µm. (D) Western blot for total αSyn and ß-actin in undifferentiated clonal lines for Shef4 and S9 (low αSyn), and S8 and S37 (high αSyn) clones.
The impact of αSyn on cell proliferation was assessed using an MTS assay. Over a 10-day period, six low αSyn lines (S9, S12, S13, S17, S22, S34) and 3 high αSyn lines (S8, S36, S37) were examined and each showed a similar rate of proliferation (Supplementary Figure S1). There was no significant difference in the proliferation rate of low and high αSyn groups and linear regression analysis showed no correlation between SNCA expression and proliferation rate of the examined cell lines (R2 = 0.003, p = 0.884).
Multiple clonal lines were differentiated into cortical neural progenitors using a dual SMAD inhibition protocol (Figure 2A) (Chambers et al., 2009; Shi et al., 2012). RNA was isolated and qRT-PCR used to quantify NCAM and MAPT expression at day 11 and compared to SNCA expression at the start of differentiation (Figure 2B). There was no correlation in day 11 NCAM (R2 = 0.008, p = 0.761) nor day 11 MAPT (R2 = 0.019, p = 0.461) levels relative to SNCA expression for the clonal lines (Figure 2C). Western blotting at day 11 of differentiation also confirmed differentiatial protein expression of αSyn in low and high αSyn cell lines (Figure 2D). hESC lines with low or high αSyn expression differentiated equally well to form PAX6-positive neuroectoderm by day 12 (Figure 3A). A direct comparison of Shef4 and selected clonal hESC lines with human iPSC lines from a healthy control (NAS2) and triplication SNCA patient (AST18) showed robust and similar expression of cortical progenitor markers PAX6, OTX1/2, and Vimentin at day 12 of neural induction (Supplementary Figure S2). By day 26 of cortical neuron differentiation the mRNA expression of PAX6 and the cortical marker, TBR1, were similarly induced in the parental Shef4 cell line and two high αSyn clones (S8, S37) (Figure 3B). Furthermore, by day 45, there was similar protein expression of early cortical markers, TBR1 and CTIP2, in low and high αSyn groups (Figure 3C). Despite the difference in αSyn levels, both groups formed neuronal networks with the same degree of efficiency based on βIII-Tubulin immunostaining (Figure 3D). This data provides evidence that αSyn expression levels do not influence neuronal induction and differentiation potential across multiple clonal hESC lines.
FIGURE 2. Level of αSyn does not impair neural induction. (A) Schematic summarising key stages of the cortical neuron differentiation protocol. Cell lifts were carried out at days 12, 17, and 25. Dual SMAD inhibition using SB431542 (SB) and LDN-193189 (LDN) was employed early to drive neural induction and cortical identity, and the growth factors BDNF and GDNF were used to promote neuronal maturation after day 25. (B) Quantitative RT-PCR data measuring total SNCA expression in undifferentiated transgenic Shef4 cell lines, and NCAM and MAPT mRNA levels at day 11 of differentiation. Data shown as relative fold-change to expression in the parental Shef4 line, and error bars represent the standard error of the mean (SEM). (C) Linear regression analysis of SNCA, NCAM, and MAPT expression levels was performed (p = 0.174 and p = 0.191 for NCAM and MAPT, respectively). (D) Western blot for αSyn and ß-actin of whole cell lysates from cortical neuroectoderm (day 11) differentiated from transgenic hESC lines.
FIGURE 3. Level of αSyn does not impair cortical neuron differentiation. (A) Representative immunocytochemistry images at day 12 of low αSyn and high αSyn hESCs differentiated into neuroectoderm, immunostained for the cortical progenitor marker PAX6. Scale bar = 50 µm. (B) Quantitative RT-PCR for PAX6 and TBR1 expression for Shef4 (control), S8 (high αSyn), and S37 (high αSyn) samples at day 0 (self-renewing hESCs) and day 26 of cortical differentiation. Mean expression levels are shown relative to the expression of TBP. (C) Representative immunocytochemistry images of both low αSyn and high αSyn immature neurons (day 45) stained for the deep cortical layer markers TBR1 (green), CTIP2 (red), and βIII-Tubulin (blue), as well as merged images. Scale bar = 50 µm. (D) Immunocytochemistry images of neuronal networks representing both low αSyn and high αSyn hESCs differentiated into mature neurons (day 83), immunostained for the neuronal marker βIII-Tubulin (blue) and DAPI (white). Scale bar = 100 µm. Percentage neurons was calculated by quantifying DAPI levels relative to βIII-Tubulin. Significance performed using a Welch’s t-test. N = number of biological replicates (differentiated cell line) and n = technical replicates (number of wells per cell line) (N = 2, n = 5 for low αSyn; N = 3, n = 8 for high αSyn).
To determine if transgenic SNCA expression was maintained during differentiation, total and transgenic SNCA levels were measured by qRT-PCR (Figure 4A). The primers for total SNCA were designed to target the coding region and primers for transgenic SNCA targeted the IRES region (Figure 4A). Total and transgenic levels of human SNCA were measured in self-renewing hESCs (day 0) and day 25 differentiated cortical cells. Day 25 was a suitable time point as the neural induction period has been completed and immature cortical neurons are forming. Total SNCA expression at day 0 was significantly higher in the high αSyn vs. low αSyn hESC group (Figure 4B). Importantly, at day 25, the elevated expression of SNCA was maintained between the high αSyn vs. low αSyn cortical neuron group (p < 0.01). Total SNCA levels did not significantly increase over each time point, day 0 vs. day 25, for both high αSyn and low αSyn groups. Transgenic SNCA levels in undifferentiated hESCs were significantly higher in the high αSyn vs. low αSyn group (Figure 4B) (p < 0.01). Day 25 high αSyn vs. low αSyn cortical neurons also had higher transgenic SNCA (p < 0.01). Transgenic SNCA levels did not significantly increase or decrease between day 0 and day 25 for the high αSyn and low αSyn groups. Immunostaining and western blotting confirmed that high αSyn expression was maintained following cortical neuron differentiation (Figures 4C,D). At day 72 of cortical differentiation the hiPSC line, AST18, containing an SNCA triplication mutation exhibited a 3-fold increase in αSyn expression compared to a control hiPSC line, NAS2, derived from a 1st-degree relative (Devine et al., 2011) (Figure 4D). The S37 transgenic cell line maintained high αSyn expression similar to AST18 neurons, while the S36 hESC line, that expressed the most SNCA at day 0 (Figure 1B), now had low αSyn expression by day 72 of differentiation, suggesting significant transgene silencing (Figure 4D). This is not unexpected as transgene expression is contingent on several factors, including site of integration, number of copies of the transgene, as well as transgene silencing over cell passage or differentiation (Liu et al., 2009).
FIGURE 4. Overexpression of αSyn is maintained during cortical neuron differentiation. (A) Schematic showing the regions of SNCA cDNA amplified to measure “Total SNCA,” and location of primers targeting the internal ribosome entry site (IRES) region to measure “Transgenic SNCA” levels. (B) Quantitative RT-PCR for total SNCA and transgenic SNCA expression for both low αSyn and high αSyn samples at day 0 (self-renewing hESCs) and day 25 (immature differentiated cortical neurons). Mean expression levels were quantified relative to the expression of TBP and error bars represent SEM. N = number of biological replicates (differentiated cell line) and n = technical replicates (number of wells per cell line). For both total and transgenic αSyn, low αSyn (N = 4, n = 4) and high αSyn (N = 6 and n = 6). Statistical comparisons were performed using the Welch’s t-test (** = 2-tailed p < 0.01). (C) Representative immunocytochemistry images of differentiated neuronal cells (day 34) derived from transgenic Shef4 clonal lines S9 (low) and S37 (high), stained for DAPI (blue), βIII-Tubulin (red), and αSyn (green). White box delineates the zoomed in area. Scale bar = 30 µm. (D) Western blot (left) for total αSyn and ß-actin in cortical neurons (day 72) differentiated from transgenic hESC and hiPSC cell lines. αSyn levels quantified in ImageJ (right) for all cell lines relative to expression in Shef4-derived neurons.
The transcriptomic profile of self-renewing hESC lines (day 0) and differentiated cortical neurons (day 25) with high αSyn and low αSyn were investigated using RNA-seq analysis. A total of 17 samples were subjected to UPX 3′-sequencing to measure polyadenylated mRNA transcripts across 4 sample groups 1) low αSyn hESCs, 2) high αSyn hESCs, 3) low αSyn cortical neurons, and 4) high αSyn cortical neurons (Figure 5A). Principal Component Analysis (PCA) showed a clear segregation between hESCs and cortical neurons, represented by 83% variance on PC1 (Figure 5B). The PC2 axis was representative of αSyn-related differences between hESC and cortical neuron samples. The hESC samples were intermixed, but cortical neuron samples segregated based on αSyn expression, however, the variance along PC2 was small (5%). This is further corroborated by hierarchical clustering analysis based on all differentially expressed genes across the four sample groups (Figure 5C).
FIGURE 5. RNA-seq analysis of clonal hESC lines and differentiated cortical neurons. (A) Table of individual sample groups highlighting αSyn levels, differentiation experiment group, and the cell line of samples. N = 3 for high αSyn cortical neurons, N = 4 for both low αSyn hESCs and cortical neurons, and N = 6 for high αSyn hESCs. (B) Principal component analysis (PCA) plot of all samples. (C) Heatmap of a hierarchical cluster analysis of differential expression results, showing relative gene expression changes as either upregulated (red) or downregulated (blue). Analysis was carried out with log2-transformed raw counts. Plot encompasses all differentially expressed genes from all four DESeq2 pairwise comparisons, representing 5,157 genes in total.
A pairwise comparison of self-renewing high αSyn vs low αSyn hESC lines found only 2 genes (CBR1, CTNNA3) were significantly differentially expressed between the two groups (Figure 6A), indicating αSyn does not impact on the pluripotency transcriptome. As expected, comparisons of low αSyn cortical neurons and low αSyn hESCs, as well as high αSyn cortical neurons and high αSyn hESCs, identified large numbers of differentially expressed genes (3,429 and 4,188, respectively, Figures 6B,C; Supplementary Table S1). Seven of the top ten most significantly upregulated genes were the same for these two pairwise comparisons, including SOX5, NPAS3, MALAT1, MAP2, QKI, PCDH9, and AC10729.1 (Figure 6D). Most of these genes have a role in neurogenesis (Izant and McIntosh, 1980; Hardy et al., 1996; Wunderle et al., 1996; Strehl et al., 1998; Brunskill et al., 1999; Chen et al., 2016). Similarly, five of the top ten significantly downregulated genes were the same in the hESCs vs cortical neuron comparisons for high and low αSyn cell lines, including DPPA4, L1TD1, RBM47, XACT and DNMT3B (Figure 6D). Most of these top downregulated genes have roles in pluripotency (Madan et al., 2009; Hu et al., 2012; Vallot et al., 2013; Emani et al., 2015; Radine et al., 2020). There was a large overlap in the genes that were significantly upregulated and downregulated in cortical neuron vs hESC groups with high or low αSyn (Figure 7A). There was also a substantial overlap of gene ontology (GO) terms significantly upregulated and downregulated in the high or low αSyn comparisons (Figure 7B). The most significantly enriched KEGG pathway in both high and low αSyn cortical neuron vs. hESC comparisons for upregulated genes was “axon guidance” (KEGG:04360). Five other top ten KEGG pathways linked with upregulated genes were the same for both the high αSyn and low αSyn cortical neuron vs hESC comparisons (Figure 7C). The “ribosome” term (KEGG:03,010) was the most significant downregulated pathway common to both comparisons. Interestingly, the Parkinson’s disease KEGG pathway was downregulated in high αSyn cortical neurons vs high αSyn hESCs, due to the downregulation of several mitochondrial genes and cytochrome c oxidase genes, including NDUFB9, MT-CO2, MT-CO3, MT-ATP6, MT-ATP8, MT-CYB, COX7C, and CYCS. This may reflect a mitochondrial phenotype caused by elevated αSyn expression in cortical neurons. The data so far suggests that the process of cortical neuron differentiation induced a large number of significant gene expression changes, and that the level of αSyn in these cells does not impair this process.
FIGURE 6. RNA-seq reveals large and over-lapping gene expression changes between cortical neurons and hESCs for both low αSyn and high αSyn groups. (A–C) Volcano plots showing differentially expressed genes between (A) low αSyn hESCs and high αSyn hESCs, (B) low αSyn cortical neurons (day 25) vs hESCs (day 0), and (C) high αSyn cortical neurons (day 25) vs. hESCs (day 0). Horizontal dashed lines cross the y-axis at–log10 (0.05), representing a significance cut-off Padj value of 0.05. Vertical lines represent a fold-change cut-off of 1.2, and therefore cross the x-axis at 0.263 and−0.263.
FIGURE 7. (A) Venn diagrams comparing upregulated and downregulated differentially expressed genes from low αSyn cortical neurons vs hESCs and high αSyn cortical neurons vs hESCs comparisons. (B) Venn diagrams comparing upregulated and downregulated GO terms from low αSyn cortical neurons vs hESCs and high αSyn cortical neurons vs hESCs comparisons. (C) List of top 10 upregulated and top 10 downregulated KEGG terms by Padj value, in order of ascending Padj, for low αSyn cortical neurons vs hESCs and high αSyn cortical neurons vs hESCs (if less than 10, all terms shown). KEGG terms common to both comparisons are indicated.
The key group comparison was that of high αSyn cortical neurons vs. low αSyn cortical neurons. The volcano plot, in comparison to the cortical neurons vs. hESC plots, identified a total of 47 differentially expressed genes (Figure 8A; Supplementary Table S1). KEGG pathway analysis did not show any relevant neurogenesis-related pathways enriched in high αSyn vs low αSyn cortical neurons (Figure 8B). Selected gene expression analysis revealed that the pluripotency markers POU5F1 (OCT4) and NANOG were significantly downregulated and neurogenesis markers, ASCL1, MYT1L, and POU3F2, were significantly upregulated in cortical neuron samples relative to hESC samples for both high αSyn and low αSyn groups (Figure 8C) (Nichols et al., 1998; Chambers et al., 2003; Vierbuchen et al., 2010). Analysis of genes implicated in axon guidance, including MAP2, SLIT2, EPHB1 and NCAM1 showed no difference in cortical neurons with low or high αSyn, except for NTN1 (p < 0.01) (Figure 8D) (Kennedy et al., 1994; Izant and McIntosh, 1980; Borrell et al., 2012; Enriquez-Barreto et al., 2012). There were no significant differences in genes associated with synaptic development, in low αSyn and high αSyn cortical neurons, including CADM1, NLGN1, SNAP25, SYP, and DLG4 (Figure 8E) (Wiedenmann and Franke, 1985; Oyler et al., 1989; Cho et al., 1992; Ichtchenko et al., 1995; Stagi et al., 2010). Other markers were explored to ascertain telencephalic development. FOXG1 and PAX6, cortical progenitor markers, and CTIP2 and TLE4, markers of deep cortical layers, showed no significant differences in low and high αSyn cortical neuron groups (Figure 8F) (Raciti et al., 2013). The superficial layer marker SATB2, was not significantly different between the low and high αSyn cortical neurons, which is in keeping with its involvement in the later stages of cortical development (Mariani et al., 2012). Expression of the neuronal migration marker, DCX, also presented no significant variation between low or high αSyn cortical neurons (Figure 8F) (Gleeson et al., 1999).
FIGURE 8. RNA-seq analysis reveals that αSyn overexpression does not have a significant impact on cortical marker gene expression. (A) Volcano plot showing distribution of differentially expressed genes between high αSyn and low αSyn day 25 cortical neurons (B) Table showing all upregulated and downregulated KEGG terms for this comparison. (C–F) Plots showing normalised single gene read counts across all four sample groups for (C) pluripotency and neurogenesis markers, (D) axon guidance genes, (E) synaptic development genes, and (F) cortical progenitor, deep layer, superficial layer, and migration genes. Statistical comparisons between cortical neurons were performed using the Welch’s t-test (**p < 0.01).
In this study, we generated and investigated 29 SNCA transgenic hESC lines and found no correlation between αSyn expression level and neural differentiation potential. We further addressed the hypothesis that increased αSyn expression does not impair cortical neuronal differentiation of human pluripotent stem cells. Across multiple clonal lines, multiple rounds of cortical neuron differentiation using two robust differentiation protocols and unbiased transcriptomic analysis, we show data to support this hypothesis. The level of SNCA expression, whether normal or increased, did not have a significant impact on the efficiency of cortical neuron differentiation. These findings resolve conflicting data in the field, and are highly relevant to studies utilising human pluripotent stem cells (hESCs or iPSCs) with differential αSyn expression to model synucleinopathies, such as PD, DLB and multiple system atrophy (MSA).
As expected, significant differential gene expression was observed when cortical neurons were compared to undifferentiated hESCs for both high αSyn and low αSyn hESCs. Gene ontology (GO) and KEGG pathway analysis identified significant overlap in the group comparisons with high αSyn or low αSyn hESCs and their differentiated counterparts. Furthermore, most of the top differentially expressed genes were the same in these comparisons, indicating neurogenesis proceeded in a similar manner despite the level of αSyn expression. The gene expression differences that were unique to the high αSyn hESCs or to the low αSyn hESCs could be due to a number of factors, including clonal variation, experiment-to-experiment variation, or due to a phenotype of elevated αSyn expression, such as mitochondrial dysfunction. When high αSyn and low αSyn cortical neurons were directly compared to each other, only 47 genes were differentially expressed, and pathway analysis did not reveal anything relevant to cortical neuron induction or differentiation. Furthermore, read count analysis for well-characterised genes with known roles in neurogenesis and the development of cortical identity confirmed cortical differentiation occurred similarly between the high αSyn and low αSyn groups.
Research groups, including ourselves, have used hiPSCs derived from patients with SNCA multiplication mutations to model synucleinopathies (Byers et al., 2011; Devine et al., 2011; Flierl et al., 2014; Oliveira et al., 2015; Lin et al., 2016; Prots et al., 2018; Chen et al., 2019; Brazdis et al., 2020). We previously showed, using eight clonal hiPSC lines, harbouring the SNCA triplication mutation and six hiPSC lines from a non-affected first-degree relative, that the main sources of variation in dopaminergic differentiation efficiency were due to differences between clonal lines, reprogramming efficiency, and the process of neuronal differentiation itself (Devine et al., 2011). Whilst cellular reprogramming is a powerful method for generating human disease models, reprogramming can be incomplete and can introduce coding mutations or large chromosomal abnormalities that may lead to altered differentiation potential of different clones (Mayshar et al., 2010; Boulting et al., 2011; Hussein et al., 2011). Furthermore, in these patient-derived iPSCs the size of the multiplicated region is variable and other adjacent genes such as MMRN1 may be incorporated (Ross et al., 2008). Over-expression of other coding genes in these iPSC lines presents another potential confounding factor when investigating and interpreting neuronal differentiation potential. A number of these caveats apply to studies differentiating hiPSCs with a SNCA triplication into neuronal progenitors or dopaminergic neurons (Flierl et al., 2014; Oliveira et al., 2015). In particular, limited clonal lines were examined and siRNA knock-down of SNCA “rescue” of dopaminergic differentiation was partial (Oliveira et al., 2015).
To further highlight the point regarding clonal variation and conflicting reports in the literature, recent studies have shown, using hiPSCs from patients with a SNCA duplication mutation, that cortical neurons (Prots et al., 2018) and dopaminergic neurons can be efficiently and comparably generated using these hiPSC lines (Brazdis et al., 2020). Other independent groups have also used hiPSCs with a SNCA triplication mutation to show functional dopaminergic neuron generation comparable to control hiPSC lines (Byers et al., 2011; Lin et al., 2016). We recently showed that reducing SNCA alleles in isogenic hESC lines also does not affect dopaminergic neuronal differentiation; wild type, SNCA+/− and SNCA−/− hESC lines showed no differences in differentiation into FOXA2:TH double-positive dopaminergic neurons (Chen et al., 2019). This study, and the triplication and duplication SNCA hiPSC studies (Devine et al., 2011; Brazdis et al., 2020) strongly predict that the isogenic collection of hESC lines generated here will have similar efficiencies of midbrain dopaminergic differentiation. In the studies reporting no impairment of dopaminergic neuron differentiation with a SNCA multiplications, PD-related phenotypes, including reduced synchronous firing on microelectrode recordings and increased susceptibility to oxidative stress were observed (Lin et al., 2016; Brazdis et al., 2020). While there may be αSyn-related phenotypic differences or inherent vulnerabilities due to elevated αSyn expression in mature neurons, this does not imply that the process of neuronal differentiation itself is impaired.
When αSyn was over-expressed using a lentiviral system in hESC-derived neuroectoderm impaired neuronal patterning and acute toxicity were reported (Schneider et al., 2007). Dopaminergic and GABAergic neuron populations were affected by αSyn over-expression, but this was only quantified in a single hESC line (H9), and the toxicity shown to be occurring could result in selective neuronal loss, making the interpretation of differentiation marker analysis challenging (Schneider et al., 2007). Similar caveats apply to work performed using AF22 neural stem cells with doxycycline-inducible αSyn expression. Exposure to doxycycline may cause pleotropic effects, and αSyn expression was not sustained throughout differentiation (Zasso et al., 2018).
It is important to consider that neuronal differentiation protocols often require optimisation for each clonal line and small variations in ligand concentrations, specifically CHIR99021 in the midbrain dopaminergic differentiation protocol, can impair neurogenesis. For example, in the study by Oliveira et al., 2015 the dopaminergic differentiation protocol yielded less than eight percent TH-positive neurons in the control lines. Whilst our study does not yield insight into the impact of elevated αSyn expression on dopaminergic differentiation, a subject for future studies, the cortical neuron protocols used were robust with the vast majority of cells producing cortical neurons.
The next steps for these cell lines are to investigate differential susceptibility to know triggers of synucleinopathy, such as environmental risk factors that affect autophagy and lysosomal biology, as well as compounds that inhibit mitochondrial function. We have previously shown that reduction of SNCA alleles in hESC-derived neurons protects against synucleinopathy (Chen et al., 2019), and it is predicted that increasing αSyn expression will make neurons more susceptible to conditions that promote Parkinson’s and other Lewy body conditions.
In summary, this work has produced an allelic series of isogenic clonal hESC lines with differing levels of αSyn expression, and we have demonstrated that elevated αSyn expression does not impair cortical neurogenesis. This supports the validity of using human pluripotent stem cells, such as iPSCs with SNCA multiplications and transgenic hESC lines, to model synucleinopathies. Since this collection of cell lines are isogenic and the only genetic variable is the level of αSyn expression, their utility for disease modelling and research into novel therapeutics is very significant. However, close matching of the differentiation stage and maturity of neurons from the different clones is still required for accurate interpretation of results. The practical application of these cell lines is most promising for investigating treatments that are targeted against αSyn itself or downstream effects of its over-expression.
Shef4 hESCs were provided Prof D Hay (University of Edinburgh) following MRC Steering Committee approval (SCSC11-60). The plasmid consisting of wild-type human SNCA (pcDNA3.1) was provided by Prof J Hardy (UCL). The PGK-Puro-pCAGS and FCT-IRES-Venus-pBS plasmids were provided by William Hamilton (University of Edinburgh).
IRES-Venus and human SNCA fragments were amplified by PCR (MJ Research, PTC-200 Peltier Thermal Cycler) and purified using DNA Clean and Concentrator™-5 Kit (Zymo Research, D4003) to provide 20 µl of eluted DNA. 1X BSA, 1X digestion buffer and 50 U digestion enzyme (all New England Biolabs®) were used to digest the plasmid DNA, which was then purified from agarose gel using Zymoclean™ Gel DNA Recovery Kit (Zymo Research, D4001) per manufacturer’s protocol. Purified and digested DNA was ligated to PCR products at 16°C overnight in a final volume of 20 μl using 2 U of T4 DNA ligase and 1x ligation buffer (Roche, 10481220001). Plasmid DNA was transformed into TOP10 chemically competent cells (Invitrogen, c4040-10) by the heat-shock method. Plasmid DNA was extracted using QIAprep®-Spin Miniprep kit (Qiagen, 27,104) or Maxiprep (Qiagen, 12,662) systems per manufacturer’s protocol, then desalted using Millipore centrifugal filter units (Millipore, UFC503024 24PK). The final pCAG-SNCA-IRES-Venus construct contained human SNCA, internal ribosome entry site (IRES) and Venus expression cassette under the constitutive pCAG promoter, as well as a puromycin resistance gene (Puror) driven by the PGK promoter. The control construct, pCAG-IRES-Venus, contained the same elements except for the SNCA gene (Figure 1A).
The Neon Transfection System (Invitrogen, MPK5000) was used for nucleofection of both constructs into Shef4 hESCs as per the manufacturer’s protocol. 1 μg/ml puromycin was added to the culture media for at least two weeks to isolate clones and colonies, manually picked for expansion and cryopreservation. Clones were screened for SNCA over-expression by qRT-PCR.
The MasterPure™ Complete DNA and RNA Purification kit (Epicentre, MC85200) or the RNeasy kit (Qiagen, 74,104) was used for RNA extraction. Genomic DNA was removed using DNase I (Promega, M6101). cDNA was synthesised from 500 ng total RNA using M-MLV reverse transcriptase (RT, ThermoFisher Scientific, 28025013) or Superscript IV reverse transcriptase (Invitrogen, 18090010). qRT-PCR was performed using a LightCycler™ 480 (Roche) with the following parameters: (95°C for 10 min), [(95°C for 10 s) + (60°C for 20 s)] over 45 cycles. Intron-spanning primers were designed using the universal Probe Library (UPL) Assay design centre (Roche). Primer sequences and UPL probes were total SNCA F-tgggcaagaatgaagaaggagc, R-gtggtgacgggtgtgacagc Probe 68; transgenic SNCA F-cgacctgcagttggacct, R-tgacaatgacatccactttgc Probe 163; NCAM F-gcgttggagagtccaaattc, R-gggagaaccaggagatgtcttt Probe 51; MAPT F-accacagccaccttctcct, R-cagccatcctggttcaaagt Probe 55; PAX6 F- tcaccatggcaaataacctg, R-cagcatgcaggagtatgagg Probe 20; TBR1 F-aggaagaaagaatggcctaaaa, R-ggatgcatatagacccgatttc Probe 22; TATA-box binding protein (TBP) F-atagggattccgggagtcat, R-gaacatcatggatcagaacaaca Probe 87. Each 10 μl reaction was performed in triplicate and results normalised to TBP expression.
Cell proliferation was assessed using a colorimetric assay, CellTiter96® AQueous One Solution Cell Proliferation Assay or MTS assay. In this test, MTS tetrazolium is bioreduced in viable cells (Supplementary Figure S1).
Shef4-derived transgenic hESC lines, and AST18 and NAS2 hiPSCs were expanded in culture on either Matrigel-coated 6-well plates (BD, 356234) in mTeSR1 medium (StemcellTM Technologies, 05850) or on Laminin-521 (BioLamina, LN521) coated 6-well plates in StemMACS iPS-Brew XF, human (Miltenyi Biotec, 130-107-086). 1 μg/ml puromycin (Sigma, P8833) was used in the culture media of Tg hESCs to maintain the expression of the transgenes.
Two cortical neuron differentiation protocols were used, referred to as CD protocol 1 and CD protocol 2, respectively. CD protocol 1 was adapted from the cortical neuron differentiation protocol published by Chambers et al., 2009 and CD protocol 2 was adapted from the published protocol by Shi et al., 2012. Both protocols use dual SMAD inhibition to induce cortical neuron differentiation. In CD protocol 1, neural differentiation was started by changing the culture media to neural induction media (NIM) which included 10 μM SB431542 (Tocris, 616461) and 100 nM LDN-193189 (Stemgent, 04-0019). NIM for this protocol was prepared by mixing 1:1 DMEM/F12 (Gibco, 20331-020) and Neurobasal medium (Gibco, 21103-049), supplemented with 1 ml N2 and 2 ml B27 with retinoic acid (Gibco, 17504-044). NIM was also supplemented with 2 mM L-glutamine, 0.1 mM ß-mercaptoethanol (BDH, 44143-31), 100 U/ml penicillin and 100 μg/ml streptomycin (Invitrogen, 15140-122) and 100 μM non-essential amino acids (Gibco, 1140-035). Cells were lifted using dispase at day 12, dissociated into clumps and plated on 10 μg/ml Laminin-111 (Sigma L2020-1 MG) and 15 μg/ml poly-L-ornithine-coated plates (Sigma, P4957). 100 nM LDN and 20 μg/ml FGF2 were included in the NIM, and SB431542 removed from day 12. Following 7–10 days of progenitor colony expansion, cells were lifted using accutase and plated onto Laminin-111/poly-L-ornithine-coated plates in NIM supplemented with 10 ng/ml BDNF (Peprotech, 450-02) and 10 ng/ml GDNF (Peprotech, 450-10). Half media changes were performed every 3 days during the neuronal maturation phase up to day 83.
In CD protocol 2 hESCs at 80%–90% confluency in 6-well plates were lifted with 1 ml/well UltraPure 0.5 M EDTA (Invitrogen, 15575038), counted, and transferred onto 5 μg/ml Laminin-111 coated (Biolamina, LN111-04) 24-well plates (Corning, 3527), at an initial plating density of 80,000 cells/cm2. 600 μl/well neural induction media (NIM) was used until day 4 of differentiation. NIM was composed of 50% DMEM/F12 (ThermoFisher Scientific, 21331020) and 50% Neurobasal Media (ThermoFisher Scientific, 21103049), B27 supplement with Retinoic Acid (ThermoFisher Scientific, 17504044), N2 supplement (ThermoFisher Scientific, 17502048) and 2 mM L-Glutamine (ThermoFisher Scientific, 25030123). From day 4 onwards, 50% NIM, 25% DMEM/F12 and 25% Neurobasal Media with 2 mM L-glutamine. For the first 12 days of differentiation, 10 μM SB431542 (Tocris, 616461) and 100 nM LDN-193189 (Miltenyi Biotec, 130-103-925) were added to the NIM and this media was replaced every two days. Cells were lifted at day 12 and day 17 with Collagenase Type IV (Life Technologies, 17104019) diluted in HBSS (ThermoFisher Scientific, 14025). Cell were lifted and passaged as clumps in a ratio of 1:1.5 and 1:2 at day 12 and 17, respectively. 10 μM Y27632 (Tocris) was used in the media for each cell lift. At day 25, differentiated cells were lifted with Accutase (Sigma, A6964) and cell pellets frozen using a dry ice and ethanol bath for RNA isolation.
Total RNA was isolated with RNeasy kit (QIAGEN, 74104). RNA integrity (RINe ≥ 7) was confirmed using Tapestation 4200 (Agilent). The median RINe score was 9.2. Samples were collected at day 0 and day 25, across three sets of cortical neuron differentiations (Figure 5A). The samples were processed by Qiagen Genomic Services using their QIAseq UPX 3′ Transcriptome kit and libraries were sequenced on a NextSeq500 instrument. The total number of polyadenylated 3′ transcript reads and the mean number of reads per unique molecular identifier were counted. The raw transcript counts were analysed using DESeq2 (Love et al., 2014) differential expression analysis in R studio. Pairwise analysis was used to compare hESC vs. cortical neurons with high or low αSyn. A padj cut off value of 0.05 and a log2 fold-change cut off value of 1.2, were used. KEGG pathway analysis was performed using the g:GOSt function in the CRAN gprofiler2 package (Raudvere et al., 2019). RNA-seq data has been deposited on the Gene Expression Omnibus (GEO Accession number: GSE195877).
Immunostaining was performed on cells cultured on 13-mm glass coverslips or in Ibidi 8-well plates. Spent medium was removed and cells fixed with 4% PFA for 15 min. Following 3 PBS (ThermoFisher Scientific) washes, the cells were permeabilised and blocked with 2% goat (or 2% donkey) serum (Sigma) in 0.1% Triton-X-100 (Fisher) in PBS for 45 min prior to overnight incubation at 4°C with primary antibodies. The primary antibodies used were ß-III tubulin (1:1,000, mouse IgG2b, Sigma T8660), CTIP2 (1:500, rat IgG2a, Abcam ab18465), PAX6 (1:40, mouse IgG1, DSHB ab528427), TBR1 (1:200, rabbit IgG, Abcam ab31940), OTX1/2 (1:1,000, rabbit IgG, Abcam ab21990), vimentin (2 μg/ml final, mouse IgM, DSHB 40E-C), and total αSyn (1:1,000, mouse IgG1, BD 610787). Secondary antibodies were applied at 1:1,000 dilution at room temperature for 1 h in the dark. These were goat anti-mouse IgG1 Alexa Fluor 488 (ThermoFisher Scientific, A21121), goat anti-mouse IgG2a Alexa Fluor 488 (ThermoFisher Scientific, A21131), donkey anti-rat IgG Alexa Fluor 488 (ThermoFisher Scientific, A21208), goat anti-mouse IgG1 Alexa Fluor 555 (ThermoFisher Scientific, A21127), goat anti-rabbit IgG Alexa Fluor 555 (ThermoFisher Scientific, A21428), donkey anti-rabbit IgG Alexa Fluor 555 (ThermoFisher Scientific, A31572), goat anti-mouse IgG2b Alexa Fluor 647 (ThermoFisher Scientific, A21242), goat anti-mouse IgM Alexa Fluor 568 (ThermoFisher Scientific, A21043), and donkey anti-mouse IgG Alexa Fluor 647 (Abcam, ab150107). Following a further three PBS washes, slides were mounted using Fluorsave (Merck, 345789). 0.1 μg/ml 4′,6-Diamidino-2-Phenylindole (DAPI, Life Technologies) was used to stain nuclei.
The Eclipse Ti (Nikon) and/or the Axio Observer (Zeiss) microscopes were used to acquire the images presented. Huygens Software (Scientific Volume Imaging) was used for deconvolution of Z stack images (at least 10 images/stack and maximum intensity pixel projection used). Fiji software was used for image analysis and quantification, with identical brightness and contrast values used for each image channel and for each experiment. Macro scripts were used to split the image channels, threshold and binarize for quantification.
RIPA Lysis Buffer (Santa Cruz, sc-24948) was used to lyse cell pellets and protein concentration determined using the Micro BCA Protein Assay kit (ThermoFisher Scientific, 232350). 10 μg protein, per sample, was mixed and incubated with 5 μl NuPAGE™ LDS Sample Buffer (ThermoFisher Scientific, NP0007) and 2 μl 1 M DTT (ThermoFisher Scientific, NP0004) prior to loading on a NuPAGE™ 4%–12% Bis-Tris Gradient Gel (ThermoFisher Scientific, NP0322BOX). SeeBlue™ Plus2 Pre-stained Protein Standard (5 μl, ThermoFisher Scientific, LC5925) was used as a standard. Following electrophoresis, protein was transferred onto a 0.45 μm nitrocellulose (Amersham Protran Premium, 10600096) or a PVDF membrane (GE Healthcare Amersham Hybond ECL, RPN68D). 0.4% PFA was used to fix the protein on the membrane. One minute of methanol (Fisher Scientific, M/3900/17) immersion was done, in addition, if a PVDF membrane was used. Membranes were immersed in blotting-grade blocker (BioRad, 1706404) in 0.1% TBS-Tween for an hour at room temperature and then the primary antibody, mouse anti-αSyn (1:1,000, BD 610787) was added overnight at 4°C. Following three washes in 0.1% TBS-Tween, 1:2,000 HRP-conjugated anti-mouse IgG (Promega) secondary antibody was applied for two hours at room temperature. Pierce™ ECL Western Blotting Substrate (ThermoFisher Scientific, 32109) was then added to the membrane for image capture using the LI-COR Odyssey imaging system (Biosciences). Antibodies were stripped with the Restore™ PLUS Western Blot Stripping Buffer (ThermoFisher Scientific, 46430). The blocking step was repeated and secondary antibody, HRP-conjugated anti-ß-Actin antibody (1:1,000, Abcam) applied. The Pierce™ ECL Western Blotting Substrate was again used prior to imaging the membrane.
Statistical tests were performed using SPSS v23 and include the Student’s t-test or t-test with Welch’s correction, linear regression analysis and the Mann-Whitney U test for non-parametric data. Each Figure legend details which test was used for each statistical comparison. Significance level cut off was p < 0.05.
The datasets presented in this study can be found in online repositories. The names of the repository/repositories and accession number(s) can be found in the article/Supplementary Material.
TK conceived the project. TK, AN, RY designed the experiments. AN and RY performed the experiments and analysed the data. RB performed bioinformatic analysis. AN and TK wrote the manuscript, and all authors reviewed and approved the manuscript.
AN was funded by the Wellcome Trust Research Training Fellowship (203646/Z/16/Z). TK was funded by an MRC grant (MR/J012831/1).
The authors declare that the research was conducted in the absence of any commercial or financial relationships that could be construed as a potential conflict of interest.
All claims expressed in this article are solely those of the authors and do not necessarily represent those of their affiliated organizations, or those of the publisher, the editors and the reviewers. Any product that may be evaluated in this article, or claim that may be made by its manufacturer, is not guaranteed or endorsed by the publisher.
The authors with to thank the Wellcome Trust and the MRC for funding this project. We are very grateful to Prof Steven Pollard for critical comments on the manuscript, and Dr James Ashmore for bioinformatics guidance. We also sincerely thank Prof John Hardy and Dr William Hamilton for pcDNA3.1-SNCA and PGK-Puro-pCAGS plasmids, respectively, and Prof David Hay for Shef4 hESCs.
The Supplementary Material for this article can be found online at: https://www.frontiersin.org/articles/10.3389/fcell.2022.898560/full#supplementary-material
Abeliovich, A., Schmitz, Y., Fariñas, I., Choi-Lundberg, D., Ho, W.-H., Castillo, P. E., et al. (2000). Mice Lacking α-Synuclein Display Functional Deficits in the Nigrostriatal Dopamine System. Neuron 25, 239–252. doi:10.1016/s0896-6273(00)80886-7
Al-Wandi, A., Ninkina, N., Millership, S., Williamson, S. J. M., Jones, P. A., and Buchman, V. L. (2010). Absence of α-synuclein Affects Dopamine Metabolism and Synaptic Markers in the Striatum of Aging Mice. Neurobiol. Aging 31, 796–804. doi:10.1016/j.neurobiolaging.2008.11.001
Anwar, S., Peters, O., Millership, S., Ninkina, N., Doig, N., Connor-Robson, N., et al. (2011). Functional Alterations to the Nigrostriatal System in Mice Lacking All Three Members of the Synuclein Family. J. Neurosci. 31, 7264–7274. doi:10.1523/JNEUROSCI.6194-10.2011
Borrell, V., Cárdenas, A., Ciceri, G., Galcerán, J., Flames, N., Pla, R., et al. (2012). Slit/Robo Signaling Modulates the Proliferation of Central Nervous System Progenitors. Neuron 76, 338–352. doi:10.1016/j.neuron.2012.08.003
Boulting, G. L., Kiskinis, E., Croft, G. F., Amoroso, M. W., Oakley, D. H., Wainger, B. J., et al. (2011). A Functionally Characterized Test Set of Human Induced Pluripotent Stem Cells. Nat. Biotechnol. 29, 279–286. doi:10.1038/nbt.1783
Brazdis, R.-M., Alecu, J. E., Marsch, D., Dahms, A., Simmnacher, K., Lörentz, S., et al. (2020). Demonstration of Brain Region-specific Neuronal Vulnerability in Human iPSC-Based Model of Familial Parkinson's Disease. Hum. Mol. Genet. 29, 1180–1191. doi:10.1093/hmg/ddaa039
Brunskill, E. W., Witte, D. P., Shreiner, A. B., and Potter, S. S. (1999). Characterization of Npas3 , a Novel Basic Helix-Loop-Helix PAS Gene Expressed in the Developing Mouse Nervous System. Mech. Dev. 88, 237–241. doi:10.1016/S0925-4773(99)00182-3
Byers, B., Cord, B., Nguyen, H. N., Schüle, B., Fenno, L., Lee, P. C., et al. (2011). SNCA Triplication Parkinson's Patient's iPSC-Derived DA Neurons Accumulate α-Synuclein and Are Susceptible to Oxidative Stress. PLoS One 6, e26159. doi:10.1371/journal.pone.0026159
Chambers, I., Colby, D., Robertson, M., Nichols, J., Lee, S., Tweedie, S., et al. (2003). Functional Expression Cloning of Nanog, a Pluripotency Sustaining Factor in Embryonic Stem Cells. Cell 113, 643–655. doi:10.1016/s0092-8674(03)00392-1
Chambers, S. M., Fasano, C. A., Papapetrou, E. P., Tomishima, M., Sadelain, M., and Studer, L. (2009). Highly Efficient Neural Conversion of Human ES and iPS Cells by Dual Inhibition of SMAD Signaling. Nat. Biotechnol. 27, 275–280. doi:10.1038/nbt.1529
Chartier-Harlin, M.-C., Kachergus, J., Roumier, C., Mouroux, V., Douay, X., Lincoln, S., et al. (2004). α-Synuclein Locus Duplication as a Cause of Familial Parkinson's Disease. Lancet 364, 1167–1169. doi:10.1016/S0140-6736(04)17103-1
Chen, L., Feng, P., Zhu, X., He, S., Duan, J., and Zhou, D. (2016). Long Non‐coding RNA Malat1 Promotes Neurite Outgrowth through Activation of ERK/MAPK Signalling Pathway in N2a Cells. J. Cell. Mol. Med. 20, 2102–2110. doi:10.1111/jcmm.12904
Chen, Y., Dolt, K. S., Kriek, M., Baker, T., Downey, P., Drummond, N. J., et al. (2019). Engineering Synucleinopathy‐resistant Human Dopaminergic Neurons by CRISPR ‐mediated Deletion of the SNCA Gene. Eur. J. Neurosci. 49, 510–524. doi:10.1111/ejn.14286
Cho, K.-O., Hunt, C. A., and Kennedy, M. B. (1992). The Rat Brain Postsynaptic Density Fraction Contains a Homolog of the Drosophila Discs-Large Tumor Suppressor Protein. Neuron 9, 929–942. doi:10.1016/0896-6273(92)90245-9
Connor-Robson, N., Peters, O. M., Millership, S., Ninkina, N., and Buchman, V. L. (2016). Combinational Losses of Synucleins Reveal Their Differential Requirements for Compensating Age-dependent Alterations in Motor Behavior and Dopamine Metabolism. Neurobiol. Aging 46, 107–112. doi:10.1016/j.neurobiolaging.2016.06.020
Devine, M. J., Ryten, M., Vodicka, P., Thomson, A. J., Burdon, T., Houlden, H., et al. (2011). Parkinson's Disease Induced Pluripotent Stem Cells with Triplication of the α-synuclein Locus. Nat. Commun. 2, 440. doi:10.1038/ncomms1453
Emani, M. R., Närvä, E., Stubb, A., Chakroborty, D., Viitala, M., Rokka, A., et al. (2015). The L1TD1 Protein Interactome Reveals the Importance of Post-transcriptional Regulation in Human Pluripotency. Stem Cell Rep. 4, 519–528. doi:10.1016/j.stemcr.2015.01.014
Enriquez-Barreto, L., Palazzetti, C., Brennaman, L. H., Maness, P. F., and Fairén, A. (2012). Neural Cell Adhesion Molecule, NCAM, Regulates Thalamocortical Axon Pathfinding and the Organization of the Cortical Somatosensory Representation in Mouse. Front. Mol. Neurosci. 5, 76. doi:10.3389/fnmol.2012.00076
Flierl, A., Oliveira, L. M. A., Falomir-Lockhart, L. J., Mak, S. K., Hesley, J., Soldner, F., et al. (2014). Higher Vulnerability and Stress Sensitivity of Neuronal Precursor Cells Carrying an Alpha-Synuclein Gene Triplication. PLoS One 9, e112413. doi:10.1371/journal.pone.0112413
Gleeson, J. G., Lin, P. T., Flanagan, L. A., and Walsh, C. A. (1999). Doublecortin Is a Microtubule-Associated Protein and Is Expressed Widely by Migrating Neurons. Neuron 23, 257–271. doi:10.1016/s0896-6273(00)80778-3
Greten-Harrison, B., Polydoro, M., Morimoto-Tomita, M., Diao, L., Williams, A. M., Nie, E. H., et al. (2010). αβγ-Synuclein Triple Knockout Mice Reveal Age-dependent Neuronal Dysfunction. Proc. Natl. Acad. Sci. U.S.A. 107, 19573–19578. doi:10.1073/pnas.1005005107
Hardy, R. J., Loushin, C. L., Friedrich Jr., V. L., Chen, Q., Ebersole, T. A., Lazzarini, R. A., et al. (1996). Neural Cell Type-specific Expression of QKI Proteins Is Altered inquakingviableMutant Mice. J. Neurosci. 16, 7941–7949. doi:10.1523/jneurosci.16-24-07941.1996
Hitoshi, N., Ken-ichi, Y., and Jun-ichi, M. (1991). Efficient Selection for High-Expression Transfectants with a Novel Eukaryotic Vector. Gene 108, 193–199. doi:10.1016/0378-1119(91)90434-D
Hu, N., Strobl-Mazzulla, P., Sauka-Spengler, T., and Bronner, M. E. (2012). DNA methyltransferase3A as a Molecular Switch Mediating the Neural Tube-To-Neural Crest Fate Transition. Genes Dev. 26, 2380–2385. doi:10.1101/gad.198747.112
Hussein, S. M., Batada, N. N., Vuoristo, S., Ching, R. W., Autio, R., Närvä, E., et al. (2011). Copy Number Variation and Selection during Reprogramming to Pluripotency. Nature 471, 58–62. doi:10.1038/nature09871
Ichtchenko, K., Hata, Y., Nguyen, T., Ullrich, B., Missler, M., Moomaw, C., et al. (1995). Neuroligin 1: A Splice Site-specific Ligand for β-neurexins. Cell 81, 435–443. doi:10.1016/0092-8674(95)90396-8
Izant, J. G., and McIntosh, J. R. (1980). Microtubule-associated Proteins: a Monoclonal Antibody to MAP2 Binds to Differentiated Neurons. Proc. Natl. Acad. Sci. U.S.A. 77, 4741–4745. doi:10.1073/pnas.77.8.4741
Kennedy, T. E., Serafini, T., de la Torre, J., and Tessier-Lavigne, M. (1994). Netrins Are Diffusible Chemotropic Factors for Commissural Axons in the Embryonic Spinal Cord. Cell 78, 425–435. doi:10.1016/0092-8674(94)90421-9
Liew, C.-G., Draper, J. S., Walsh, J., Moore, H., and Andrews, P. W. (2007). Transient and Stable Transgene Expression in Human Embryonic Stem Cells. Stem Cells 25, 1521–1528. doi:10.1634/stemcells.2006-0634
Lin, L., Göke, J., Cukuroglu, E., Dranias, M. R., VanDongen, A. M. J., and Stanton, L. W. (2016). Molecular Features Underlying Neurodegeneration Identified through In Vitro Modeling of Genetically Diverse Parkinson's Disease Patients. Cell Rep. 15, 2411–2426. doi:10.1016/j.celrep.2016.05.022
Liu, J., Jones, K. L., Sumer, H., and Verma, P. J. (2009). Stable Transgene Expression in Human Embryonic Stem Cells after Simple Chemical Transfection. Mol. Reprod. Dev. 76, 580–586. doi:10.1002/mrd.20983
Love, M. I., Huber, W., and Anders, S. (2014). Moderated Estimation of Fold Change and Dispersion for RNA-Seq Data with DESeq2. Genome Biol. 15, 550. doi:10.1186/s13059-014-0550-8
Madan, B., Madan, V., Weber, O., Tropel, P., Blum, C., Kieffer, E., et al. (2009). The Pluripotency-Associated Gene Dppa4 Is Dispensable for Embryonic Stem Cell Identity and Germ Cell Development but Essential for Embryogenesis. Mol. Cell. Biol. 29, 3186–3203. doi:10.1128/MCB.01970-08
Mariani, J., Simonini, M. V., Palejev, D., Tomasini, L., Coppola, G., Szekely, A. M., et al. (2012). Modeling Human Cortical Development In Vitro Using Induced Pluripotent Stem Cells. Proc. Natl. Acad. Sci. U.S.A. 109, 12770–12775. doi:10.1073/pnas.1202944109
Mayshar, Y., Ben-David, U., Lavon, N., Biancotti, J.-C., Yakir, B., Clark, A. T., et al. (2010). Identification and Classification of Chromosomal Aberrations in Human Induced Pluripotent Stem Cells. Cell Stem Cell 7, 521–531. doi:10.1016/j.stem.2010.07.017
Nichols, J., Zevnik, B., Anastassiadis, K., Niwa, H., Klewe-Nebenius, D., Chambers, I., et al. (1998). Formation of Pluripotent Stem Cells in the Mammalian Embryo Depends on the POU Transcription Factor Oct4. Cell 95, 379–391. doi:10.1016/s0092-8674(00)81769-9
Oliveira, L. M. A., Falomir-Lockhart, L. J., Botelho, M. G., Lin, K.-H., Wales, P., Koch, J. C., et al. (2015). Elevated α-synuclein Caused by SNCA Gene Triplication Impairs Neuronal Differentiation and Maturation in Parkinson's Patient-Derived Induced Pluripotent Stem Cells. Cell Death Dis. 6, e1994. doi:10.1038/cddis.2015.318
Oyler, G. A., Higgins, G. A., Hart, R. A., Battenberg, E., Billingsley, M., Bloom, F. E., et al. (1989). The Identification of a Novel Synaptosomal-Associated Protein, SNAP-25, Differentially Expressed by Neuronal Subpopulations. J. Cell Biol. 109, 3039–3052. doi:10.1083/jcb.109.6.3039
Polymeropoulos, M. H., Lavedan, C., Leroy, E., Ide, S. E., Dehejia, A., Dutra, A., et al. (1997). Mutation in the α-Synuclein Gene Identified in Families with Parkinson's Disease. Science 276, 2045–2047. doi:10.1126/science.276.5321.2045
Prots, I., Grosch, J., Brazdis, R.-M., Simmnacher, K., Veber, V., Havlicek, S., et al. (2018). α-Synuclein Oligomers Induce Early Axonal Dysfunction in Human iPSC-Based Models of Synucleinopathies. Proc. Natl. Acad. Sci. U.S.A. 115, 7813–7818. doi:10.1073/pnas.1713129115
Raciti, M., Granzotto, M., Duc, M. D., Fimiani, C., Cellot, G., Cherubini, E., et al. (2013). Reprogramming Fibroblasts to Neural-precursor-like Cells by Structured Overexpression of Pallial Patterning Genes. Mol. Cell. Neurosci. 57, 42–53. doi:10.1016/j.mcn.2013.10.004
Radine, C., Peters, D., Reese, A., Neuwahl, J., Budach, W., Jänicke, R. U., et al. (2020). The RNA-Binding Protein RBM47 Is a Novel Regulator of Cell Fate Decisions by Transcriptionally Controlling the P53-P21-axis. Cell Death Differ. 27, 1274–1285. doi:10.1038/s41418-019-0414-6
Raudvere, U., Kolberg, L., Kuzmin, I., Arak, T., Adler, P., Peterson, H., et al. (2019). g:Profiler: a Web Server for Functional Enrichment Analysis and Conversions of Gene Lists (2019 Update). Nucleic Acids Res. 47, W191–W198. doi:10.1093/nar/gkz369
Ross, O. A., Braithwaite, A. T., Skipper, L. M., Kachergus, J., Hulihan, M. M., Middleton, F. A., et al. (2008). Genomic Investigation of α-synuclein Multiplication and Parkinsonism. Ann. Neurol. 63, 743–750. doi:10.1002/ana.21380
Schneider, B. L., Seehus, C. R., Capowski, E. E., Aebischer, P., Zhang, S.-C., and Svendsen, C. N. (2007). Over-expression of Alpha-Synuclein in Human Neural Progenitors Leads to Specific Changes in Fate and Differentiation. Hum. Mol. Genet. 16, 651–666. doi:10.1093/hmg/ddm008
Shi, Y., Kirwan, P., and Livesey, F. J. (2012). Directed Differentiation of Human Pluripotent Stem Cells to Cerebral Cortex Neurons and Neural Networks. Nat. Protoc. 7, 1836–1846. doi:10.1038/nprot.2012.116
Singh Dolt, K., Hammachi, F., and Kunath, T. (2017). Modeling Parkinson's Disease with Induced Pluripotent Stem Cells Harboring α-synuclein Mutations. Brain Pathol. 27, 545–551. doi:10.1111/bpa.12526
Singleton, A. B., Farrer, M., Johnson, J., Singleton, A., Hague, S., Kachergus, J., et al. (2003). α-Synuclein Locus Triplication Causes Parkinson's Disease. Science 302, 841. doi:10.1126/science.1090278
Specht, C. G., and Schoepfer, R. (2001). Deletion of the Alpha-Synuclein Locus in a Subpopulation of C57BL/6J Inbred Mice. BMC Neurosci. 2, 11. doi:10.1186/1471-2202-2-11
Spillantini, M. G., Schmidt, M. L., Lee, V. M.-Y., Trojanowski, J. Q., Jakes, R., and Goedert, M. (1997). α-Synuclein in Lewy Bodies. Nature 388, 839–840. doi:10.1038/42166
Stagi, M., Fogel, A. I., and Biederer, T. (2010). SynCAM 1 Participates in Axo-Dendritic Contact Assembly and Shapes Neuronal Growth Cones. Proc. Natl. Acad. Sci. U.S.A. 107, 7568–7573. doi:10.1073/pnas.0911798107
Strehl, S., Glatt, K., Liu, Q. M., Glatt, H., and Lalande, M. (1998). Characterization of Two Novel Protocadherins (PCDH8andPCDH9) Localized on Human Chromosome 13 and Mouse Chromosome 14. Genomics 53, 81–89. doi:10.1006/geno.1998.5467
Surguchev, A. A., and Surguchov, A. (2017). Synucleins and Gene Expression: Ramblers in a Crowd or Cops Regulating Traffic? Front. Mol. Neurosci. 10, 224. doi:10.3389/fnmol.2017.00224
Vallot, C., Huret, C., Lesecque, Y., Resch, A., Oudrhiri, N., Bennaceur-Griscelli, A., et al. (2013). XACT, a Long Noncoding Transcript Coating the Active X Chromosome in Human Pluripotent Cells. Nat. Genet. 45, 239–241. doi:10.1038/ng.2530
Vierbuchen, T., Ostermeier, A., Pang, Z. P., Kokubu, Y., Südhof, T. C., and Wernig, M. (2010). Direct Conversion of Fibroblasts to Functional Neurons by Defined Factors. Nature 463, 1035–1041. doi:10.1038/nature08797
Wiedenmann, B., and Franke, W. W. (1985). Identification and Localization of Synaptophysin, an Integral Membrane Glycoprotein of Mr 38,000 Characteristic of Presynaptic Vesicles. Cell 41, 1017–1028. doi:10.1016/s0092-8674(85)80082-9
Winner, B., Regensburger, M., Schreglmann, S., Boyer, L., Prots, I., Rockenstein, E., et al. (2012). Role of -Synuclein in Adult Neurogenesis and Neuronal Maturation in the Dentate Gyrus. J. Neurosci. 32, 16906–16916. doi:10.1523/JNEUROSCI.2723-12.2012
Wunderle, V. M., Critcher, R., Ashworth, A., and Goodfellow, P. N. (1996). Cloning and Characterization ofSOX5,a New Member of the HumanSOXGene Family. Genomics 36, 354–358. doi:10.1006/geno.1996.0474
Keywords: human pluripotent stem cells, α-synuclein, synucleinopathy, isogenic cell lines, cortical differentiation, neurogenesis, Parkinson’s disease
Citation: Natalwala A, Behbehani R, Yapom R and Kunath T (2022) An Isogenic Collection of Pluripotent Stem Cell Lines With Elevated α-Synuclein Expression Validated for Neural Induction and Cortical Neuron Differentiation. Front. Cell Dev. Biol. 10:898560. doi: 10.3389/fcell.2022.898560
Received: 17 March 2022; Accepted: 11 May 2022;
Published: 30 May 2022.
Edited by:
Yohan Oh, Hanyang University, South KoreaReviewed by:
Andrei Surguchov, University of Kansas Medical Center, United StatesCopyright © 2022 Natalwala, Behbehani, Yapom and Kunath. This is an open-access article distributed under the terms of the Creative Commons Attribution License (CC BY). The use, distribution or reproduction in other forums is permitted, provided the original author(s) and the copyright owner(s) are credited and that the original publication in this journal is cited, in accordance with accepted academic practice. No use, distribution or reproduction is permitted which does not comply with these terms.
*Correspondence: Ammar Natalwala, YS5uYXRhbHdhbGFAdWNsLmFjLnVr; Tilo Kunath, dGlsby5rdW5hdGhAZWQuYWMudWs=
Disclaimer: All claims expressed in this article are solely those of the authors and do not necessarily represent those of their affiliated organizations, or those of the publisher, the editors and the reviewers. Any product that may be evaluated in this article or claim that may be made by its manufacturer is not guaranteed or endorsed by the publisher.
Research integrity at Frontiers
Learn more about the work of our research integrity team to safeguard the quality of each article we publish.