- 1Department of Pathophysiology, College of Basic Medical Sciences, Dalian Medical University, Dalian, China
- 2Department of Maternal, Child and Adolescent Health, School of Public Health, Anhui Medical University, MOE Key Laboratory of Population Health Across Life Cycle, NHC Key Laboratory of Study on Abnormal Gametes and Reproductive Tract, Anhui Provincial Key Laboratory of Population Health and Aristogenics, Hefei, Anhui, China
- 3Department of Nephrology, Anhui Provincial Children’s Hospital, Hefei, Anhui, China
Aquaporin-8 (AQP8), a member of the aquaporin family, is strongly expressed in follicular granulosa cells, which could affect the hormone secretion level in females. AQP8, as a membrane protein, could mediate H2O2 into cells, thereby triggering various biological events. The deficiency of Aqp8 increases female fertility, resulting from the decrease in follicular atresia. The low cell death rate is related to the apoptosis of granulosa cells. However, the mechanism by which AQP8 regulates the autophagy of granulosa cells remains unclear. Thus, this study aimed to explore the effect of AQP8 on autophagy in follicular atresia. We found that the expression of the autophagy marker light-chain protein 3 was significantly downregulated in the granulosa cells of Aqp8-knockout (Aqp8−/−) mice, compared with wild-type (Aqp8+/+) mice. Immunofluorescence staining and transmission electron microscopic examination indicated that the number of autophagosomes in the granulosa cells of Aqp8−/− mice decreased. Using a follicular granulosa cell autophagy model, namely a follicular atresia model, we verified that the concentration of H2O2 significantly increased during the autophagy of granulosa cells, consistent with the Aqp8 mRNA level. Intracellular H2O2 accumulation was modulated by endogenous AQP8 expression level, indicating that AQP8-mediated H2O2 was involved in the autophagy of granulosa cells. AQP8 deficiency impaired the elevation of H2O2 concentration through phosphorylated tyrosine activation. In addition, we carried out the analysis of transcriptome sequencing datasets in the ovary and found there were obvious differences in principal components, differentially expressed genes (DEGs) and KEGG pathways, which might be involved in AQP8-regulated follicular atresia. Taken together, these findings indicated that AQP8-mediated H2O2 transport could mediate the autophagy of granulosa cells. AQP8 might be a potential target for diseases related to ovarian insufficiency.
Highlight
AQP8-mediated H2O2 was involved in the autophagy of granulosa cells.
AQP8 deficiency could impair the elevation of H2O2 concentration through P-Tyr activation.
AQP8-mediated extracellular H2O2 may promote follicular atresia.
Introduction
Aquaporin-8 (AQP8), as a transport facilitator, can control cellular oxidative stress by regulating H2O2 levels (Marchissio et al., 2012; Bertolotti et al., 2013). H2O2 is a reactive oxygen species (ROS) that serves as a secondary messenger in various signal transduction pathways. ROS and antioxidant deficiencies are involved in ovarian aging (Qian et al., 2016). Although high ROS levels are cytotoxic, low ROS levels are essential for cell physiology and survival. H2O2 transiently modulates tyrosine phosphatases and kinases and inhibits phosphatases to activate certain kinases (Finkel, 2011). H2O2 is generated via several approaches, such as oxidative phosphorylation in the mitochondria, oxidative protein folding in the endoplasmic reticulum, and NADPH oxidases in the plasma membrane (Kakihana et al., 2012). Some members of the aquaporin (AQP) family can transport H2O2 (Bienert et al., 2007; Miller et al., 2010). For instance, H2O2 can enter lymphoid cells through AQP8 and activate related growth factor signaling (Bertolotti et al., 2016). However, the mechanism by which AQP8 regulates the autophagy of ovary granulosa cells through H2O2 transport remains unclear.
Members of the AQP family can selectively and efficiently transport water molecules, and some of them transport small molecules, such as glycerol, urea, and H2O2, simultaneously. They are widely distributed in various animal tissues and serve important physiological functions. At present, few studies have concentrated on the roles of AQP in the reproductive system and various pathological processes (Sha et al., 2011; Zhang et al., 2012). Deletion of AQP3 affects sperm motility (Chen et al., 2011). Estrogen regulate the expression of AQP5 and AQP8 to abnormal embryo implantation (Zhang et al., 2015). A clinical study reported that the decreased uterine acceptance in patients with ovarian hyperstimulation is associated with decreased AQP2 expression in the endometrium (Zhang et al., 2016). And in yeast model, AQP8 can promote H2O2 transport (Bienert et al., 2007). Subsequently, studies have confirmed that AQP3 and AQP8 feature highly efficient H2O2 transport functions by systematically analyzing the H2O2 transport of various rat AQP family members overexpressed in HEK-293 cells (Miller et al., 2010). A recent study has shown that the migration of chemotactic T cells in skin contact hypersensitivity requires AQP3-mediated H2O2 transport (Hara-Chikuma et al., 2012). TNF stimulates the hyper H2O2 production of Nox2 on keratinized cell membranes, whereas the AQP3 synergistic transport of H2O2 regulating protein phosphatase 2A activates NF-KB signals to induce psoriasis (Hara-Chikuma et al., 2015). These results prove that some AQPs mediate the transport of H2O2 and regulate its harmful or beneficial functions.
We previously reported that Aqp8−/− mice have a more litter size (Su et al., 2010), with an increase in efficient follicles (Su et al., 2013). However, the mechanisms underlying these phenomena remain unclear. Previous studies have found that AQP8 is abundantly expressed in mouse (Su et al., 2010), rat (McConnell et al., 2002) and human (Li et al., 2013) ovaries. Thus, in this study, using Aqp8−/− mice and the follicular atresia model, we measured the intracellular H2O2 concentration and Aqp8 expression level in granulosa cells and investigated the role of AQP8-transported H2O2 in the autophagy of granulosa cells.
Material and methods
Animal experiment
Aqp8−/− mice (C57BL/6 genetic background) were generated by targeted gene disruption (Yang et al., 2005). For this experiment, 5–6-week-old female mice were used. Mice were allowed free access to water and food in 12 h light and 12 h dark conditions. All animal experiments were reviewed and approved by the Committee on the Ethics of Animal Research of Dalian Medical University.
Follicular atresia mouse model
Aqp8+/+ and Aqp8−/− were intraperitoneally injected with 0.1 ml pregnant mare serum gonadotropin (PMSG) (1000 IU in 10 ml 0.9% NaCl, Ningbo a second hormone factory) or vehicle control (0.9% NaCl, 0.1 ml) at 16:00 into the mice. Mice were killed by cervical dislocation 0, 1, 2, 3, 4, and 5 days after PMSG treatment, and the ovary samples were excised. The ovaries were used for the collection of granulosa cells.
Mouse primary granulosa cell collection and culture
Ovaries were excised from mice and placed in DMEM/F12 (GIBCO-BRL, 11039021) that was supplemented with 10% fetal bovine serum (GIBCO-BRL), 10 mg/ml of streptomycin sulfate (Sigma), and 75 mg/ml of penicillin G (Sigma). Granulosa cells were harvested by follicle puncture using a 25-gauge needle. After follicle puncture, granulosa cells were suspended in the appropriate solution for immunoblotting or H2O2 concentration.
For in vitro culture of granulosa cells under serum-free conditions, ovaries were collected, and granulosa cells were collected by follicle puncture, as described previously. The cells were seeded in 24-well plates and were allowed to attach overnight. The next morning, the medium and unattached cells were removed and replaced with serum-free media. After 24 h, the granulosa cells were fixed for immunofluorescence.
Live cell station imaging of autophagic vacuoles
The adenovirus expressing GFP-LC3B (Ad-GFP-LC3B, C3006, Beyotime) was transfected into GCs grown on coverslips. After 24 h, cells were rinsed using PBS and then exposed to 2 h of H2O2 incubation. The distribution and fluorescence emitted by GFP-LC3B puncta were then observed under a live cell station (GE). Experiments were repeated three times.
Western blot analysis
Protein was isolated by the previous description (Huang et al., 2020a). The freshly isolated granulosa cells were lysed with ice-cold radioimmunoprecipitation assay (RIPA) buffer that was supplemented with a protease inhibitor PMSF (Beyotime). To facilitate the complete solubilization of the cellular proteins, the cell lysates were incubated on ice for 30 min and then centrifuged (13000 g at 4°C for 30 min). The protein concentration was tested by using a BCA Protein Assay Kit. The whole-cell lysates (20 mg/lane) were separated by sodium dodecyl sulfate (SDS)–polyacrylamide gel electrophoresis and transferred to a polyvinylidene difluoride (PVFD) membrane (Millipore). After the nonspecific binding sites were blocked with 5% skim milk, the membrane was treated with the anti-LC3 rabbit polyclonal antibody (1:2000, Novus Biologicals), cleaved caspase-3 rabbit polyclonal antibody (diluted 1:1000, Cell Signaling Technology), and β-actin (1:1000, Beyotime) overnight at 4°C. The primary antibodies for Akt, pAkt-Ser473, Bax, Bcl-2, and P-Tyr were obtained from Cell Signaling Technology (Beverly, MA). The primary antibodies for Atg3 were obtained from Proteintech (Wuhan, China), while Beclin-1 was obtained from Santa Cruz Biotechnology (Santa Cruz, CA, United States). The immunoreactive bands were demonstrated by incubation with horseradish peroxidase (HRP)-conjugated goat anti-rabbit IgG (1:10000, Zhongshan Biotechnology) at room temperature for 1 h. The peroxidase activity was visualized with the enhanced chemiluminescence detection system (WBKLS500, Millipore). Integrated optical intensities of the immunoreactive protein bands were detected by using the DNR bioimaging system MicroChemi 4.2 and the quantified analysis by ImageJ software, normalized to β-actin values.
Transmission electron microscopy test
To identify autophagic vacuoles at the ultrastructural level, the ovary was fixed with 2.5% glutaraldehyde in 0.1 M cacodylate buffer (pH 7.4) for more than 24 h at 4°C, rinsed in cacodylate buffer, postfixed in 1% OsO4 in cacodylate buffer, dehydrated, and embedded in Epon. Ultrathin sections were briefly contrasted with uranyl acetate and photographed with a transmission electron microscope (Hitachi 7100 Japan).
Measurement of the intracellular hydrogen peroxide level
Intracellular hydrogen peroxide was measured by using the Hydrogen Peroxide Assay Kit (Beyotime, Nanjing, China). Briefly, 100–200 μl of hydrogen peroxide was added to detect the ratio of lysate to lysate. One million granulosa cells were treated with 100–200 μl of the hydrogen peroxide lysate, followed by sufficient homogenization to break and lyse the cells. It was then centrifuged at 12000 g at 4°C for 3–5 min, and the supernatant was collected. Then, 50 µl of the sample or standard was treated with 100 µl of the hydrogen peroxide detection reagent at room temperature (15–30°C). And then, it was immediately measured at A560 nm.
To evaluate intracellular H2O2 levels, 1×106 cells/ml were washed twice in HBSS and incubated with 20 µM 2′,7′-dichlorofluorescein diacetate (DCFH-DA) (sigma). Granulosa cells were incubated for 30 min at 37°C. DCFH-DA was a small nonpolar and nonfluorescent molecule that diffuses into the cells, where it is enzymatically deacetylated by intracellular esterases to a polar nonfluorescent compound, which is oxidized to the highly green fluorescent 2,7-dichlorofluorescein (DCF). DCF fluorescence was measured using a multiwell plate reader (Synergy Neo HTS multimode microplate reader, BioTek) at excitation and emission wavelengths of 485 and 535 nm, respectively.
RNA extraction and real-time PCR assay
Total RNA was isolated by the previous description (Huang et al., 2020b) using the TRIzol reagent (10296010, Invitrogen) and quantified using a NanoVueTM Plus Spectrophotometer (GE Healthcare, Buckinghamshire, United Kingdom). The A260/A280 ratio of the optical density was measured using the NanoVueTM Plus Spectrophotometer. The ratio was between 1.9 and 2.1 for all samples, indicating a good quality of RNA purity and yield. cDNA was synthesized from 2 µg of total RNA using the RT reagent Kit with gDNA Eraser (TaKaRa, RR047A). The qPCR solution contained 1 µl of cDNA, 1 µl of specific primers, and 10 µl of a 2X PCR SuperMix (AS111, TransGen Biotech) in a final volume of 20 µl. All primers were produced by lifespan (Milan, Germany) and listed as follows in the 5′-3′ direction: Aqp8-F: ACACCAATGTGTAGTATGGACCT; Aqp8-R: TGACCGATAGACATCCGATGAAG; β-actin-F: TGGAATCCTGTGGCATCCATGAAAC; β-actin-R: TAAAACGCAGCTCAGTAACAGTCCG.
The reaction conditions were as follows: polymerase activation and DNA denaturation (one cycle at 95°C for 30 s); denaturation, annealing, and extension (40 cycles at 95°C for 10 s and 60°C for 30 s); melting curve (65°C, with the temperature, gradually increased 0.5°C up to 95°C). mRNA expression was normalized to the level of β-actin mRNA. Changes in mRNA expression were calculated according to the 2−△△Ct method. The amplification of β-actin mRNA was utilized to normalize the data.
Analysis of transcriptome sequencing datasets
Raw transcriptome sequencing data were stored in FASTQ document format by Bcl2fastq (v2.17.1.14). Sequencing data quality was assessed by FastQC (v0.10.1). The raw data were preprocessed, the low-quality data were filtered, and the contamination and joint sequences were removed by cutadapt (version 1.9.1). Then, gene expressions were calculated by HTSeq (V 0.6.1) with FPKM (Fragments per Kilobase per Million reads) (Mortazavi et al., 2008). Differentially expressed genes (DEGs) in the ovary (group: control and PMSG with treatment for 2 days or 4 days) were analyzed by using the R package edgeR (v3.4.6) using |fold change|>2 and a p-value < 0.05.
Statistical analysis
Statistical analysis was performed by analysis of variance (ANOVA). Significant differences between treatment groups were determined by Duncan’s multiple range tests. p < 0.05 was considered statistically significant. For statistical comparisons between two groups, an independent-sample t-test was used.
Results
Autophagy and apoptosis decrease in Aqp8−/− mouse follicular granulosa cells
Microtubule-associated light-chain protein 3 (LC3) is an autophagy marker used to evaluate granulosa cell autophagy. Granulosa cells freshly isolated from Aqp8+/+ and Aqp8−/− mice were used. The results of Western blot analysis using specific antibodies against the autophagy marker LC3 and apoptosis marker cleaved caspase-3 were shown in Figure 1. Densitometric analysis normalized by the β-actin content indicated that the protein contents of LC3 and cleaved caspase-3 significantly decreased compared with the control, suggesting that AQP8 was involved in the development of follicular granulosa cells via autophagy and apoptosis.
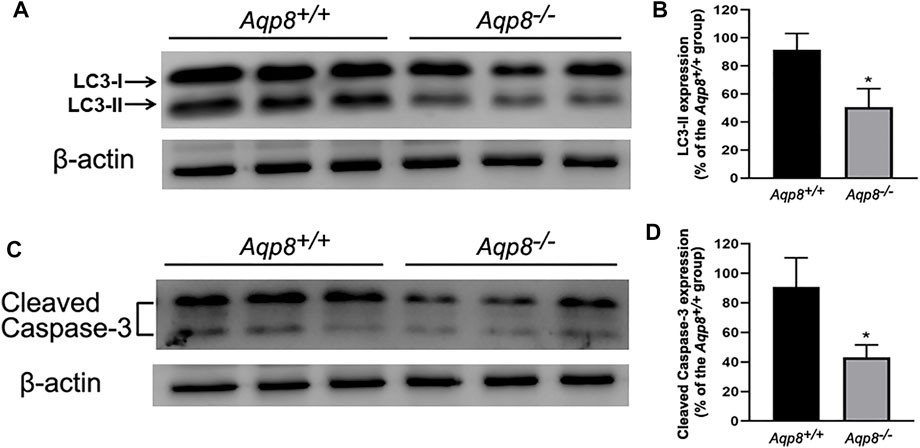
FIGURE 1. Autophagy and apoptosis decrease in Aqp8−/− mouse follicular granulosa cells. The expressions of cleaved caspase-3 and LC3-II proteins in granulosa cells from Aqp8+/+ and Aqp8−/− mice. Densitometric quantification and representative immunoblots of LC3 proteins (A,B) or cleaved caspase-3 (C,D). Experiments were repeated three times, and data were expressed as mean ± SD. *p <0.05 were considered statistically significant.
Localization of autophagosomes in granulosa cells
Transmission electron micrographs showed that autophagosomes existed in the follicular granulosa cells of Aqp8+/+ and Aqp8−/− mice. Fewer autophagosomes localized in the granulosa cells of the Aqp8−/− mice than in those of the Aqp8+/+ mice (Figure 2).
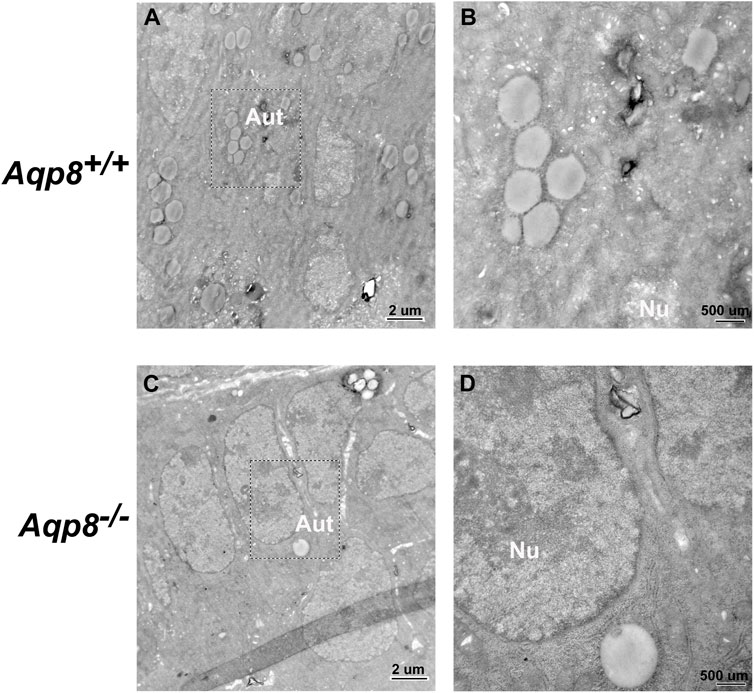
FIGURE 2. Transmission electron microscopic images of granulosa cells in Aqp8+/+ and Aqp8−/− mice. Representative images from transmission electron microscopy of granulosa cells from Aqp8+/+ (A,B) and Aqp8−/− (C,D) mice. High-magnification images indicated nucleus (Nu) and autophagosomes (Aut). The scale was indicated on the lower right corner of each image.
Aquaporin-8 channel mediates H2O2 uptake and AQP8-dependent H2O2 permeability in granulosa cells
Considering that AQP8 is expressed on granulosa cells (Su et al., 2010), we used Aqp8−/− mice to determine whether or not AQP8 is required for the efficient entry of exogenous H2O2. To investigate H2O2 transport across the plasma membrane, we incubated DFCH-DA into granulosa cells. The addition of PBS or 50 µM H2O2 clearly activated DFCH-DA to DCF, as determined by 488/510 nm shifts on a microplate reader (Figure 3A). The aforementioned experiments provided additional evidence that H2O2 cannot freely permeate through the plasma membrane and identified AQP8 as an efficient transporter. Clearly, H2O2 import was severely impaired with AQP8 silencing.
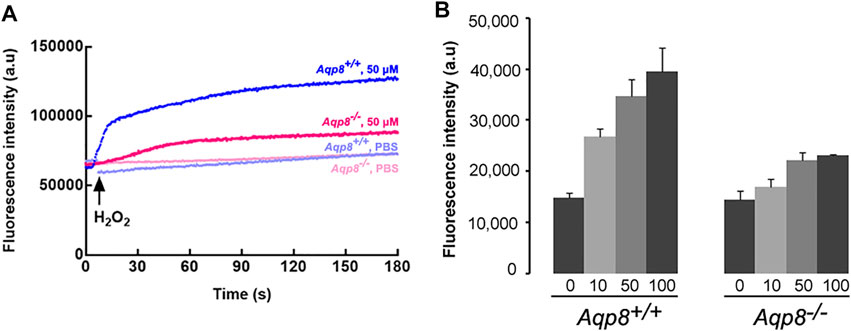
FIGURE 3. H2O2 uptake into primarily cultured granulosa cells. (A) Representative fluorescence intensity of CM-H2DCFDA. (B) Granulosa cells were incubated with H2O2 (0–100 mM), and cellular H2O2 was detected by CM-H2DCFDA fluorescence using a plate reader. Increased fluorescence intensity could be detected at 15 s after treatment with H2O2. Data were shown as mean ± SD.
Meanwhile, we investigated whether or not AQP8 can transport extracellular H2O2 into granulosa cells. Intracellular H2O2 was measured in primary granulosa cell cultures from Aqp8+/+ and Aqp8−/− mice after the extracellular addition of 10–100 μM H2O2 using the fluorescent dye CM-H2DCFDA, which reacted with ROS, including H2O2. Results showed that the cellular H2O2 level was significantly higher in the granulosa cells of the Aqp8+/+ mice than that in those of the Aqp8−/− mice (Figure 3B), indicating the involvement of AQP8 in H2O2 transport in granulosa cells. The ability of AQP8 to transport H2O2 across the plasma membrane was tested. Figure 3B showed that the basal intracellular H2O2 level was significantly affected by AQP8, revealing that the channel activity of this AQP isoform modulated the entry of physiologically produced H2O2 into the cells.
Aquaporin-8 participates in granulosa cell autophagy during follicular development and atresia
Autophagy, as an important process of programmed cell death, regulates follicular homeostasis in rats (Choi et al., 2010). Follicular development and atresia in vivo model was established in immature mice. Granulosa cell autophagy was determined by measuring the expression levels of LC3-II/LC3-I. As shown in Figure 4A, LC3-II expression in the granulosa cells was significantly downregulated 1 and 2 days after PMSG injection compared with the granulosa cells of immature mice without the treatment of exogenous gonadotropin (day 0). LC3-II expression was upregulated on day 3 and maintained until day 5. Furthermore, the expression of Aqp8 was measured. After PMSG injection, the expression of Aqp8 was significantly downregulated on days 1 and 2 and then increased on days 3, 4, and 5 (Figure 4B). Ovarian granulosa cells were isolated from each stage of the atresia model, and the concentration of H2O2 in granulosa cells was measured. The concentration of H2O2 in granulosa cells decreased during follicular development but increased during atresia (Figure 4C), suggesting that AQP8 mediated H2O2 transport and autophagy in granulosa cells to regulate follicular development and atresia.
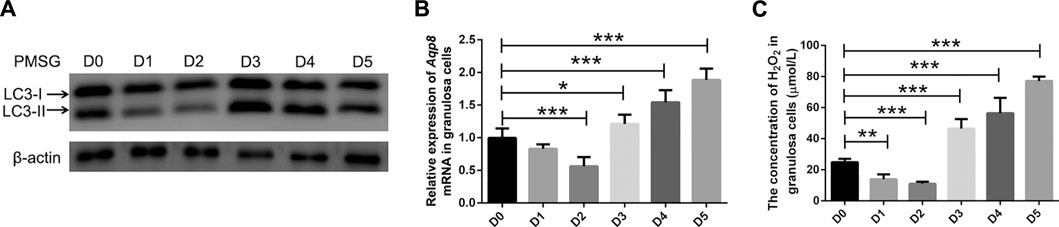
FIGURE 4. AQP8 involvement in granulosa cell autophagy during follicular development and atresia. (A) Expression of LC3-II proteins in granulosa cells from immature mice at different times (0–5 days) after pregnant mare serum gonadotropin injection (10 IU, intraperitoneally). (B) qRT-PCR was performed to measure the mRNA levels of Aqp8 in GCs. The expression of Aqp8 mRNA in granulosa cells from immature mice at different times (0–5 days) after pregnant mare serum gonadotropin injection. (C) Concentration of H2O2 in granulosa cells from immature Aqp8+/+ mice at different times (0–5 days) after pregnant mare serum gonadotropin injection (10 IU, intraperitoneally). Values are presented as the means ± SD. *p < 0.05, **p < 0.01, and ***p < 0.001.
Aquaporin-8 inhibits pAkt and phosphorylated tyrosine signaling
The mechanism by which AQP8 regulates the autophagy of granulosa cells was further explored. Results showed that AQP8 can facilitate the absorption of H2O2 into granulosa cells and mediate downstream intracellular signaling. As shown in Figure 5A, the phosphorylation level of Akt in the granular cells of the Aqp8−/− mice was increased. The expression levels of proteins related to apoptosis (Bax and caspase-3) and autophagy (Beclin-1 and Atg-3) decreased in the granulosa cells of the Aqp8−/− mice. Meanwhile, phosphorylated tyrosine signaling was activated in the granulosa cells of the Aqp8−/− mice (Figure 5B). And the absorption of H2O2 in the granulosa cells of the Aqp8+/+ mice inhibited the PI3K signaling pathway and promoted granulosa cell death and autophagy.
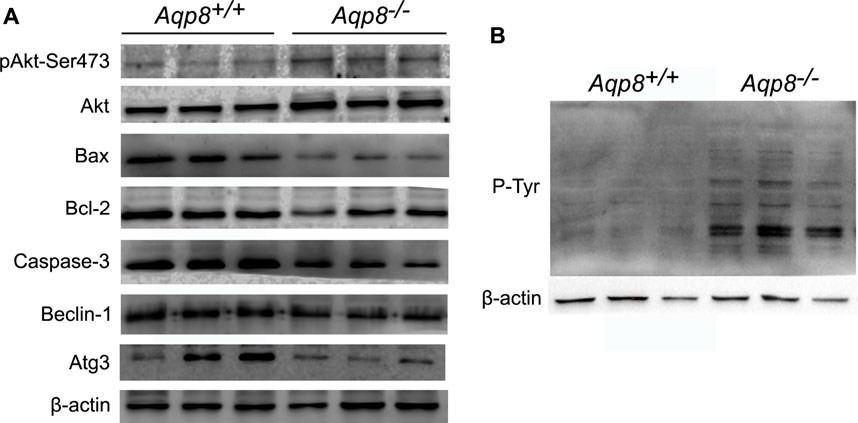
FIGURE 5. AQP8 inhibits pAkt and phosphorylated tyrosine (P-Tyr) signaling. (A) Western blot detection of the relevant signaling pathway. (B) Western blot of P-Tyr, which could be affected by H2O2.
AQP8 mediated H2O2 uptake affects granulosa intracellular autophagy
We next investigated whether or not AQP8 influences autophagy by oxidative stress. An in vitro culture experiment revealed that AQP8 was involved in granulosa cell autophagy through the transport of H2O2. Primary cultured GCs were transfected with the GFP-LC3B plasmid, incubated with or without 200 μM H2O2 for 2 h, and then rinsed with PBS. Immunofluorescence images of granulosa cells were obtained in a live cell station. As shown in Figure 6, AQP8 obviously induced autophagosome formation in the cells with H2O2 exposure, displaying that AQP8 facilitated intracellular autophagy of granulosa cells through H2O2 absorption.
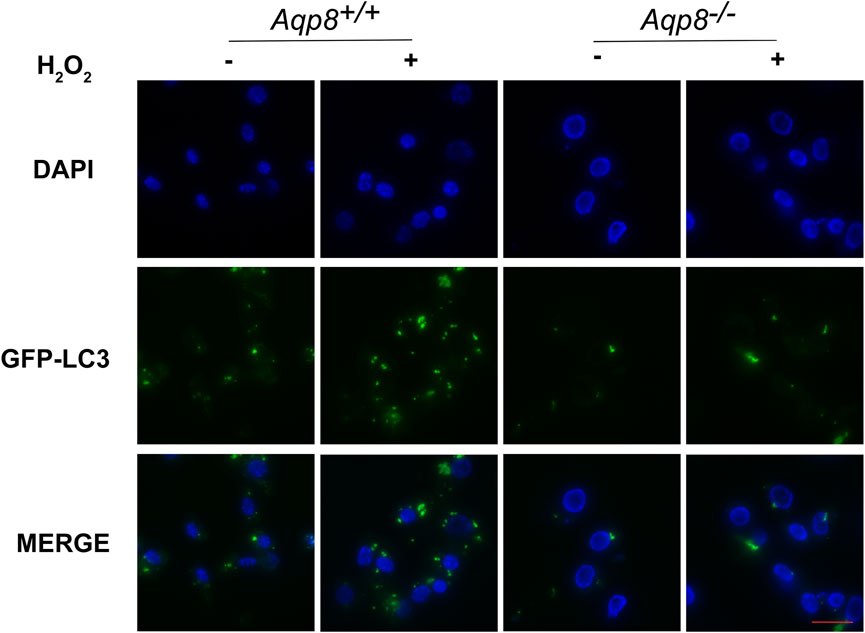
FIGURE 6. AQP8-mediated H2O2 uptake influences granulosa intracellular autophagy. GCs transfected with the GFP-LC3B plasmid for 24 h were incubated with 200 μM H2O2 for 1 h and rinsed in PBS. A live cell station was employed to observe the GFP fluorescent puncta in GCs. The nuclei were counterstained with DAPI (blue). Scale bar = 10 μm.
Integrated analysis of the ovary transcriptome in mice with PMSG treatment
Transcriptome analysis of the ovary was performed in mice with PMSG treatment for 2 days or 4 days. Principal components, differentially expressed genes (DEGs), and KEGG pathways were analyzed. The principal components of each group between parents and offspring showed obvious differences (Figure 7A). To further study the mechanisms underlying follicular development and atresia, we analyzed the DEGs and KEGG pathways between different groups. In total, 1,421 DEGs with |fold change|> 2 and p-value < 0.05 were annotated in the ovary (groups: control and injected with PMSG for 2 days), of which 690 genes were upregulated and 731 genes were downregulated (Figure 7B). In total, 1,237 DEGs with |fold change| > 2 and p value < 0.05 were annotated in the ovary (groups: control and injected with PMSG for 4 days), of which 622 genes were upregulated and 615 genes were downregulated (Figure 7C). In addition, DEG analyses of the three cohorts (control, injected with PMSG for 2 days or 4 days) overlapped, with 52 common DEGs obtained (Figure 7D). KEGG pathway enrichment analysis was also performed to study the pathway with significant DEG enrichment. Functional enrichment results revealed that 30 KEGG pathways of DEGs in the ovaries of the mice injected with PMSG for 2 days or 4 days were statistically significantly enriched, respectively (Figures 7E,G). The KEGG pathways in the control mice and mice injected with PMSG for 2 days included five categories: organismal systems, metabolism, human diseases, environmental information processing, and cellular processes in the ovary (Figure 7F). The KEGG pathways in the control mice and mice injected with PMSG for 4 days included four categories: organismal systems, metabolism, human diseases, and environmental information processing in the ovary (Figure 7H). Organismal systems and metabolism are the most enriched pathways, suggesting that they might play important roles in follicular development and atresia.
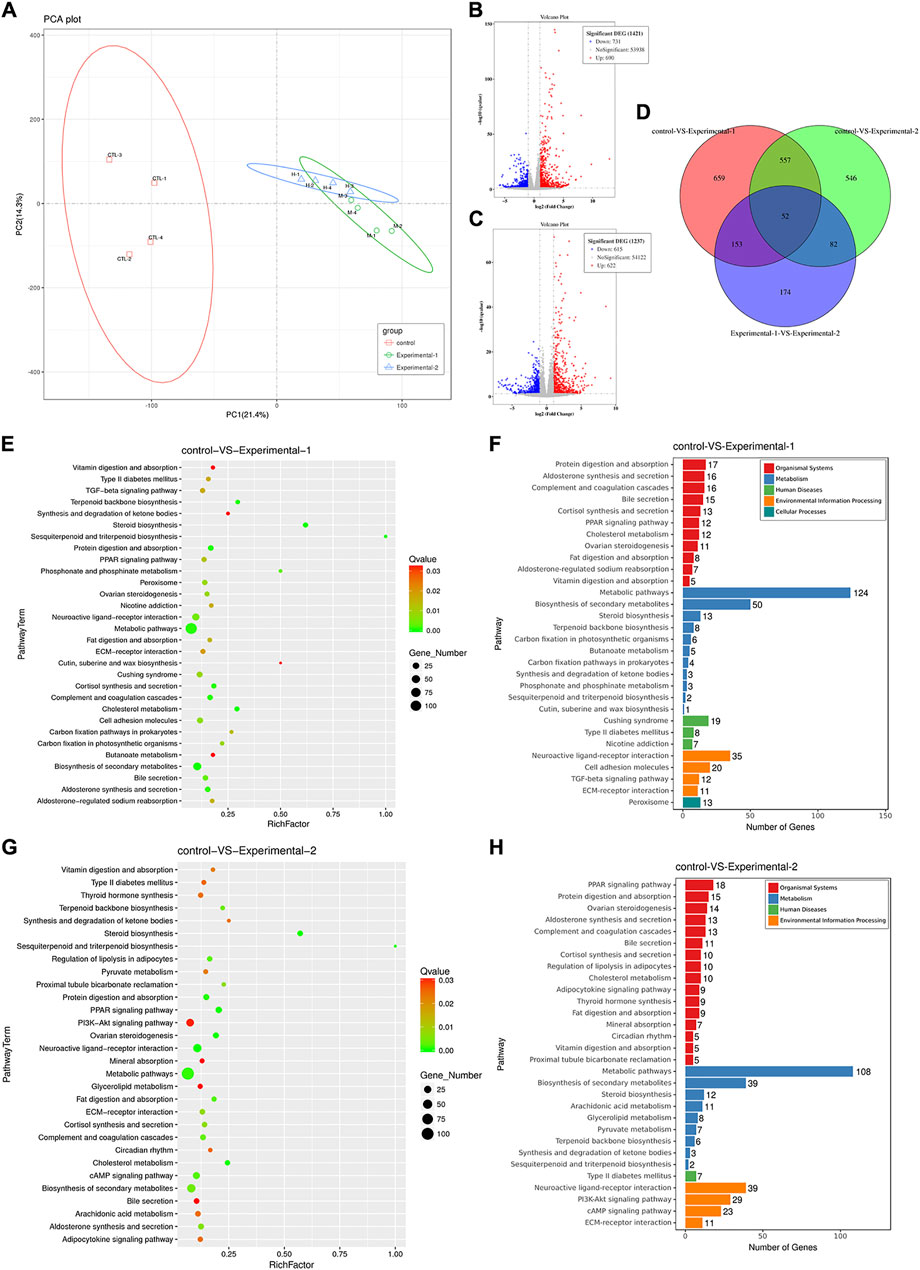
FIGURE 7. Analysis of the ovary transcriptome level after PMSG treatment. (A) Principal component analysis (PCA) was performed on the ovary (control, PMSG treatment for 2 days or 4 days), and the R function prcomp was used to analyze the samples at the transcriptome level. The volcano plot shows differentially expressed genes of three groups in the ovary with PMSG treatment for 2 days (B) and 4 days (C). The red circle represents genes that are upregulated in gene expression, the blue circle represents genes that are downregulated in gene expression, and the gray circle represents insignificant genes. (D) Venn plot shows the correlation of differential genes expressed among three groups. The bubble plot represented the enriched KEGG pathways of differentially expressed genes in the ovary with PMSG treatment for 2 days (E,F) and 4 days (G,H). The red color indicated upregulated pathways, and the blue color indicated downregulated pathways. The bubble size represents the number of genes associated with pathways in each group, and the connected color indicates the p-value across the connected groups. Control, treatment with saline; experiment 1, treatment with PMSG for 2 days; experiment 2, treatment with PMSG for 4 days.
Discussion
In the present study, we demonstrated that AQP8 induced autophagy by transporting H2O2 into granulosa cells during follicular atresia. During follicular growth and development, only less than 1% of oocytes sealed and protected by granular cells can form dominant follicles and be discharged from the body, whereas the remaining 99% of the follicles are degenerated, leading to atresia. Many studies have found that mammalian follicular development and atresia are primarily controlled by granulosa programmed cell death. In addition, at least five types of death ligands involved in programmed cell death induce particles, including TNF-alpha, Fas, TRAIL, APO-3, and PEG-5 ligands and their receptors (Manabe et al., 2004; Hurst et al., 2006; Lin and Rui, 2010). However, the follicle selection process control of the molecular mechanism of granular cell death remains unclear.
During follicular development, granulosa cell apoptosis is not the only mechanism to control follicular atresia. Autophagy and cell necrosis have been observed in the follicles of other animals (Kovacs et al., 1992; D'Herde et al., 1996; Shao et al., 2022). In recent years, some studies have found that granulosa autophagy is also involved in follicular atresia (Choi et al., 2010; Choi et al., 2011; Matsuda et al., 2012; Zhou et al., 2019).Gonadotropin could reduce the granulosa cell autophagy by activating the PI3K/Akt signaling pathway with inhibition of mTOR (Choi et al., 2014), The expression of Beclin-1 and LC3 would increase in the ovary through the oxidative stress of mitochondrial damage after treatment with harmful chemical substances in cigarette (Gannon et al., 2013; Furlong et al., 2015). Conversely, inhibiting the expressions of Akt and mTOR proteins activate the AMPK pathway, which promotes granulosa autophagy and decreases the number of original follicles affecting reproduction (Shen et al., 2017; Shen et al., 2018). Exogenous addition of oxidizing LDL can trigger autophagy in human granulosa cells (Duerrschmidt et al., 2006). Furthermore, the circadian clock system is also involved in the granulosa cell autophagy through the regulation of nuclear receptor subfamily 1 group D member 1 (NR1D1) (Zhang et al., 2022). These findings indicate that autophagy is directly involved in the regulation of granulosa death during follicular development, whereas oxidative stress may play an important role in the regulation of granulosa autophagy.
The ROS content in mammalian cells is strictly controlled. ROS are the central elements of cell proliferation, apoptosis and other signal pathway. The H2O2 concentration decreases in the follicular fluids of animals, such as bovine (Gupta et al., 2011) and swine (Basini et al., 2008), with the follicular development, whereas the upper limit of the ROS concentration in human follicular fluids is strictly controlled (Jana et al., 2010). The balance between ROS and antioxidants in follicles plays an important role in follicular development. The physiological levels of ROS and antioxidants jointly regulate the follicular formation, and the continuous action or increase in the ROS concentration interferes with the intracellular redox reaction and leads to oxidative stress (Agarwal et al., 2008). Recent findings have shown that ROS exerts cytotoxic effects because of oxidative damage, and H2O2 activates apoptotic proteases through oxidative pressure or destroys cytochromes released by intracellular mitochondria to induce the apoptosis of Jurkat T lymphocytes (Hampton and Orrenius, 1997), which has the same effect on bovine follicular granulosa cells (Hennet et al., 2013). However, increasing evidence suggests that ROS, especially H2O2, can activate or inactivate multiple signaling pathways as signaling molecules by activating or inactivating phosphorylated proteins in cells (Rhee, 2006; Forman et al., 2010).
H2O2 is a relatively stable class of reactive oxygen molecules in vivo. H2O2 produced by NADPH oxidase on the cell surface can act as a secondary messenger responding to extracellular stimuli, such as growth factors, hormones, and cytokines (Bae et al., 1997; Rhee, 2006; Schroder and Eaton, 2008). As a signaling molecule, H2O2 activates ERK1/2 and the PI3K-Akt signaling pathway corresponding to the inhibition of PTP1B and PTEN pathways, whereas ERK and PTEN signaling pathways are crucial for ovulation (Blanc et al., 2003; Tonks, 2005). Therefore, the H2O2 concentration should be controlled and maintained to ensure its role in signal transduction. The concentration of H2O2 in non-atretic follicular fluids is higher than that in atretic follicles, suggesting that ROS in follicular fluids could regulate follicular atresia (Hennet et al., 2013). Exogenous H2O2 can activate the JNK signaling pathway and induce autophagy in mesenchymal stem cells (Liu et al., 2015). Intracellular H2O2 levels rapidly reach a certain threshold to play their molecular signaling function (Rhee, 2006). Extracellular H2O2 can enter cells through free diffusion, but free diffusion can not instantly increase the intracellular concentration of H2O2. Thus, the regulation of H2O2 transport possibly depends on selective membrane. The expression of Beclin-1 and LC3 would increase in the ovary through the oxidative stress of mitochondrial damage after treatment with harmful chemical substances in cigarette channel proteins (Rhee, 2006; Kruger et al., 2021).
The autophagy of granulosa cells increased during follicular atresia in the Aqp8+/+ mice, whereas the autophagy and apoptosis of ovarian granulosa cells significantly decreased in the Aqp8−/− mice. Primary granulosa cells of the Aqp8+/+ and Aqp8−/− mice were cultured in vitro, and different concentrations of H2O2 were added externally. The H2O2 concentration in the granulosa cells of the Aqp8+/+ mice was significantly higher than that in the granulosa cells of the Aqp8−/− mice, indicating that AQP8 can mediate the efficient transport of H2O2 outside granulosa into cells. Further studies found that the H2O2 concentration in the granulosa cells increased during follicular atresia in mice. H2O2 transported by AQP8 can act as a secondary messenger to regulate intracellular signal transmission.
In conclusion, we provided a novel mechanism by which AQP8 transported extracellular H2O2 and induced cellular autophagy in granulosa cells, resulting in the increase of follicular atresia. This regulative mechanism may shed light on the treatment of ovarian insufficiency.
Data availability statement
The original contributions presented in the study are included in the article/Supplementary Material; further inquiries can be directed to the corresponding authors.
Ethics statement
The animal study was reviewed and approved by the Committee on the Ethics of Animal Research of Dalian Medical University.
Author contributions
HZ designed this study. HZ, BH, and LJ wrote this manuscript. HZ and BH contributed to the research design. LJ and LZ conducted animal and cell experiments. BH, XC, ZZ, YL, LY, TM, and HZ revised and edited this manuscript. HZ and BH was the guarantor of this work and, as such, had full access to all the data in the study and took responsibility for the integrity of the data and the accuracy of the data analysis. All authors contributed to the manuscript and approved the submitted version.
Funding
This study was supported by the National Natural Science Foundation of China (31600943 and 82103857), the Natural Science Foundation in Higher Education of Anhui (KJ 2020A0152), the Grants for Scientific Research of BSKY (XJ2020012), and the Grants for New Technology and New Project of Anhui Provincial Children’s Hospital (2022118).
Conflict of interest
The authors declare that the research was conducted in the absence of any commercial or financial relationships that could be construed as a potential conflict of interest.
Publisher’s note
All claims expressed in this article are solely those of the authors and do not necessarily represent those of their affiliated organizations, or those of the publisher, the editors, and the reviewers. Any product that may be evaluated in this article, or claim that may be made by its manufacturer, is not guaranteed or endorsed by the publisher.
Supplementary material
The Supplementary Material for this article can be found online at: https://www.frontiersin.org/articles/10.3389/fcell.2022.897666/full#supplementary-material
Abbreviations
AQP, aquaporin; ROS, reactive oxygen species; H2O2, hydrogen peroxide; LC3, light-chain protein 3; PMSG, pregnant mare serum gonadotropin; PCR, polymerase chain reaction; Akt, protein kinase B; PCD, programmed cell death.
References
Agarwal, A., Gupta, S., Sekhon, L., and Shah, R. (2008). Redox considerations in female reproductive function and assisted reproduction: from molecular mechanisms to health implications. Antioxid. Redox Signal. 10, 1375–1403. doi:10.1089/ars.2007.1964
Bae, Y. S., Kang, S. W., Seo, M. S., Baines, I. C., Tekle, E., Chock, P. B., et al. (1997). Epidermal growth factor (EGF)-induced generation of hydrogen peroxide. Role in EGF receptor-mediated tyrosine phosphorylation. J. Biol. Chem. 272, 217–221. doi:10.1074/jbc.272.1.217
Basini, G., Simona, B., Santini, S. E., and Grasselli, F. (2008). Reactive oxygen species and anti-oxidant defences in swine follicular fluids. Reprod. Fertil. Dev. 20, 269–274. doi:10.1071/rd07147
Bertolotti, M., Bestetti, S., Garcia-Manteiga, J. M., Medrano-Fernandez, I., Dal Mas, A., Malosio, M. L., et al. (2013). Tyrosine kinase signal modulation: a matter of H2O2 membrane permeability? Antioxid. Redox Signal. 19, 1447–1451. doi:10.1089/ars.2013.5330
Bertolotti, M., Farinelli, G., Galli, M., Aiuti, A., and Sitia, R. (2016). AQP8 transports NOX2-generated H2O2 across the plasma membrane to promote signaling in B cells J. Leukoc. Biol.. doi:10.1189/jlb.2AB0116-045R
Bienert, G. P., Moller, A. L., Kristiansen, K. A., Schulz, A., Moller, I. M., Schjoerring, J. K., et al. (2007). Specific aquaporins facilitate the diffusion of hydrogen peroxide across membranes. J. Biol. Chem. 282, 1183–1192. doi:10.1074/jbc.M603761200
Blanc, A., Pandey, N. R., and Srivastava, A. K. (2003). Synchronous activation of ERK 1/2, p38mapk and PKB/Akt signaling by H2O2 in vascular smooth muscle cells: potential involvement in vascular disease (review). Int. J. Mol. Med. 11, 229–234. doi:10.3892/ijmm.11.2.229
Chen, Q., Peng, H., Lei, L., Zhang, Y., Kuang, H., Cao, Y., et al. (2011). Aquaporin3 is a sperm water channel essential for postcopulatory sperm osmoadaptation and migration. Cell Res. 21, 922–933. doi:10.1038/cr.2010.169
Choi, J. Y., Jo, M. W., Lee, E. Y., Yoon, B. K., and Choi, D. S. (2010). The role of autophagy in follicular development and atresia in rat granulosa cells. Fertil. Steril. 93, 2532–2537. doi:10.1016/j.fertnstert.2009.11.021
Choi, J., Jo, M., Lee, E., and Choi, D. (2011). Induction of apoptotic cell death via accumulation of autophagosomes in rat granulosa cells. Fertil. Steril. 95, 1482–1486. doi:10.1016/j.fertnstert.2010.06.006
Choi, J., Jo, M., Lee, E., and Choi, D. (2014). AKT is involved in granulosa cell autophagy regulation via mTOR signaling during rat follicular development and atresia. Reproduction 147, 73–80. doi:10.1530/REP-13-0386
D'Herde, K., De Prest, B., and Roels, F. (1996). Subtypes of active cell death in the granulosa of ovarian atretic follicles in the quail (Coturnix coturnix japonica). Reprod. Nutr. Dev. 36, 175–189. doi:10.1051/rnd:19960203
Duerrschmidt, N., Zabirnyk, O., Nowicki, M., Ricken, A., Hmeidan, F. A., Blumenauer, V., et al. (2006). Lectin-like oxidized low-density lipoprotein receptor-1-mediated autophagy in human granulosa cells as an alternative of programmed cell death. Endocrinology 147, 3851–3860. doi:10.1210/en.2006-0088
Finkel, T. (2011). Signal transduction by reactive oxygen species. J. Cell Biol. 194, 7–15. doi:10.1083/jcb.201102095
Forman, H. J., Maiorino, M., and Ursini, F. (2010). Signaling functions of reactive oxygen species. Biochemistry 49, 835–842. doi:10.1021/bi9020378
Furlong, H. C., Stampfli, M. R., Gannon, A. M., and Foster, W. G. (2015). Cigarette smoke exposure triggers the autophagic cascade via activation of the AMPK pathway in mice. Biol. Reprod. 93, 93. doi:10.1095/biolreprod.115.132183
Gannon, A. M., Stampfli, M. R., and Foster, W. G. (2013). Cigarette smoke exposure elicits increased autophagy and dysregulation of mitochondrial dynamics in murine granulosa cells. Biol. Reprod. 88, 63. doi:10.1095/biolreprod.112.106617
Gupta, S., Choi, A., Yu, H. Y., Czerniak, S. M., Holick, E. A., Paolella, L. J., et al. (2011). Fluctuations in total antioxidant capacity, catalase activity and hydrogen peroxide levels of follicular fluid during bovine folliculogenesis. Reprod. Fertil. Dev. 23, 673–680. doi:10.1071/RD10270
Hampton, M. B., and Orrenius, S. (1997). Dual regulation of caspase activity by hydrogen peroxide: implications for apoptosis. FEBS Lett. 414, 552–556. doi:10.1016/s0014-5793(97)01068-5
Hara-Chikuma, M., Chikuma, S., Sugiyama, Y., Kabashima, K., Verkman, A. S., Inoue, S., et al. (2012). Chemokine-dependent T cell migration requires aquaporin-3-mediated hydrogen peroxide uptake. J. Exp. Med. 209, 1743–1752. doi:10.1084/jem.20112398
Hara-Chikuma, M., Satooka, H., Watanabe, S., Honda, T., Miyachi, Y., Watanabe, T., et al. (2015). Aquaporin-3-mediated hydrogen peroxide transport is required for NF-κB signalling in keratinocytes and development of psoriasis. Nat. Commun. 6, 7454. doi:10.1038/ncomms8454
Hennet, M. L., Yu, H. Y., and Combelles, C. M. (2013). Follicular fluid hydrogen peroxide and lipid hydroperoxide in bovine antral follicles of various size, atresia, and dominance status. J. Assist. Reprod. Genet. 30, 333–340. doi:10.1007/s10815-012-9925-5
Huang, B., Zhao, H., Huang, C., Wu, L., Xiang, L., Chen, J., et al. (2020a). CMKLR1 deficiency attenuates androgen-induced lipid accumulation in mice. Am. J. Physiol. Endocrinol. Metab. 318, E371–E380. doi:10.1152/ajpendo.00176.2019
Huang, B., Zhu, W., Zhao, H., Zeng, F., Wang, E., Wang, H., et al. (2020b). Placenta-Derived osteoprotegerin is required for glucose homeostasis in gestational diabetes mellitus. Front. Cell Dev. Biol. 8, 563509. doi:10.3389/fcell.2020.563509
Hurst, P. R., Mora, J. M., and Fenwick, M. A. (2006). Caspase-3, TUNEL and ultrastructural studies of small follicles in adult human ovarian biopsies. Hum. Reprod. 21, 1974–1980. doi:10.1093/humrep/del109
Jana, S. K., Narendra Babu, K., Chattopadhyay, R., Chakravarty, B., and Chaudhury, K. (2010). Upper control limit of reactive oxygen species in follicular fluid beyond which viable embryo formation is not favorable. Reprod. Toxicol. 29, 447–451. doi:10.1016/j.reprotox.2010.04.002
Kakihana, T., Nagata, K., and Sitia, R. (2012). Peroxides and peroxidases in the endoplasmic reticulum: integrating redox homeostasis and oxidative folding. Antioxid. Redox Signal. 16, 763–771. doi:10.1089/ars.2011.4238
Kovacs, J., Forgo, V., and Peczely, P. (1992). The fine structure of the follicular cells in growing and atretic ovarian follicles of the domestic goose. Cell Tissue Res. 267, 561–569. doi:10.1007/BF00319379
Kruger, C., Waldeck-Weiermair, M., Kaynert, J., Pokrant, T., Komaragiri, Y., Otto, O., et al. (2021). AQP8 is a crucial H2O2 transporter in insulin-producing RINm5F cells. Redox Biol. 43, 101962. doi:10.1016/j.redox.2021.101962
Li, Y., Liu, H., Zhao, H., Xu, C., Zhao, Y., Ma, J., et al. (2013). Association of AQP8 in women with PCOS. Reprod. Biomed. Online 27, 419–422. doi:10.1016/j.rbmo.2013.07.001
Lin, P., and Rui, R. (2010). Effects of follicular size and FSH on granulosa cell apoptosis and atresia in porcine antral follicles. Mol. Reprod. Dev. 77, 670–678. doi:10.1002/mrd.21202
Liu, G. Y., Jiang, X. X., Zhu, X., He, W. Y., Kuang, Y. L., Ren, K., et al. (2015). ROS activates JNK-mediated autophagy to counteract apoptosis in mouse mesenchymal stem cells in vitro. Acta Pharmacol. Sin. 36, 1473–1479. doi:10.1038/aps.2015.101
Manabe, N., Goto, Y., Matsuda-Minehata, F., Inoue, N., Maeda, A., Sakamaki, K., et al. (2004). Regulation mechanism of selective atresia in porcine follicles: regulation of granulosa cell apoptosis during atresia. J. Reprod. Dev. 50, 493–514. doi:10.1262/jrd.50.493
Marchissio, M. J., Frances, D. E., Carnovale, C. E., and Marinelli, R. A. (2012). Mitochondrial aquaporin-8 knockdown in human hepatoma HepG2 cells causes ROS-induced mitochondrial depolarization and loss of viability. Toxicol. Appl. Pharmacol. 264, 246–254. doi:10.1016/j.taap.2012.08.005
Matsuda, F., Inoue, N., Manabe, N., and Ohkura, S. (2012). Follicular growth and atresia in mammalian ovaries: regulation by survival and death of granulosa cells. J. Reprod. Dev. 58, 44–50. doi:10.1262/jrd.2011-012
McConnell, N. A., Yunus, R. S., Gross, S. A., Bost, K. L., Clemens, M. G., and Hughes, F. M. (2002). Water permeability of an ovarian antral follicle is predominantly transcellular and mediated by aquaporins. Endocrinology 143, 2905–2912. doi:10.1210/endo.143.8.8953
Miller, E. W., Dickinson, B. C., and Chang, C. J. (2010). Aquaporin-3 mediates hydrogen peroxide uptake to regulate downstream intracellular signaling. Proc. Natl. Acad. Sci. U. S. A. 107, 15681–15686. doi:10.1073/pnas.1005776107
Mortazavi, A., Williams, B. A., Mccue, K., Schaeffer, L., and Wold, B. (2008). Mapping and quantifying mammalian transcriptomes by RNA-Seq. Nat. Methods 5, 621–628. doi:10.1038/nmeth.1226
Qian, Y., Shao, L., Yuan, C., Jiang, C. Y., Liu, J., Gao, C., et al. (2016). Implication of Differential peroxiredoxin 4 expression with age in ovaries of mouse and human for ovarian aging. Curr. Mol. Med. 16, 243–251. doi:10.2174/1566524016666160225151647
Rhee, S. G. (2006). Cell signaling. H2O2, a necessary evil for cell signaling. Science 312, 1882–1883. doi:10.1126/science.1130481
Schroder, E., and Eaton, P. (2008). Hydrogen peroxide as an endogenous mediator and exogenous tool in cardiovascular research: issues and considerations. Curr. Opin. Pharmacol. 8, 153–159. doi:10.1016/j.coph.2007.12.012
Sha, X. Y., Xiong, Z. F., Liu, H. S., Di, X. D., and Ma, T. H. (2011). Maternal-fetal fluid balance and aquaporins: from molecule to physiology. Acta Pharmacol. Sin. 32, 716–720. doi:10.1038/aps.2011.59
Shao, T., Ke, H., Liu, R., Xu, L., Han, S., Zhang, X., et al. (2022). Autophagy regulates differentiation of ovarian granulosa cells through degradation of WT1. Autophagy, 1–15. doi:10.1080/15548627.2021.2005415
Shen, M., Jiang, Y., Guan, Z., Cao, Y., Li, L., Liu, H., et al. (2017). Protective mechanism of FSH against oxidative damage in mouse ovarian granulosa cells by repressing autophagy. Autophagy 13, 1364–1385. doi:10.1080/15548627.2017.1327941
Shen, M., Cao, Y., Jiang, Y., Wei, Y., and Liu, H. (2018). Melatonin protects mouse granulosa cells against oxidative damage by inhibiting FOXO1-mediated autophagy: implication of an antioxidation-independent mechanism. Redox Biol. 18, 138–157. doi:10.1016/j.redox.2018.07.004
Su, W., Qiao, Y., Yi, F., Guan, X., Zhang, D., Zhang, S., et al. (2010). Increased female fertility in aquaporin 8-deficient mice. IUBMB Life 62, 852–857. doi:10.1002/iub.398
Su, W., Guan, X., Zhang, D., Sun, M., Yang, L., Yi, F., et al. (2013). Occurrence of multi-oocyte follicles in aquaporin 8-deficient mice. Reprod. Biol. Endocrinol. 11, 88. doi:10.1186/1477-7827-11-88
Tonks, N. K. (2005). Redox redux: revisiting PTPs and the control of cell signaling. Cell 121, 667–670. doi:10.1016/j.cell.2005.05.016
Yang, B., Song, Y., Zhao, D., and Verkman, A. S. (2005). Phenotype analysis of aquaporin-8 null mice. Am. J. Physiol. Cell Physiol. 288, C1161–C1170. doi:10.1152/ajpcell.00564.2004
Zhang, D., Tan, Y. J., Qu, F., Sheng, J. Z., and Huang, H. F. (2012). Functions of water channels in male and female reproductive systems. Mol. Asp. Med. 33, 676–690. doi:10.1016/j.mam.2012.02.002
Zhang, Y., Chen, Q., Zhang, H., Wang, Q., Li, R., Jin, Y., et al. (2015). Aquaporin-dependent excessive intrauterine fluid accumulation is a major contributor in hyper-estrogen induced aberrant embryo implantation. Cell Res. 25, 139–142. doi:10.1038/cr.2014.139
Zhang, D., Xu, G., Zhang, R., Zhu, Y., Gao, H., Zhou, C., et al. (2016). Decreased expression of aquaporin 2 is associated with impaired endometrial receptivity in controlled ovarian stimulation. Reprod. Fertil. Dev. 28, 499–506. doi:10.1071/RD13397
Zhang, J., Zhao, L., Li, Y., Dong, H., Zhang, H., Zhang, Y., et al. (2022). Circadian clock regulates granulosa cell autophagy through NR1D1-mediated inhibition of ATG5. Am. J. Physiol. Cell Physiol. 322, C231–C245. doi:10.1152/ajpcell.00267.2021
Keywords: AQP8, hydrogen peroxide, granulosa cell, autophagy, follicular atresia
Citation: Huang B, Jin L, Zhang L, Cui X, Zhang Z, Lu Y, Yu L, Ma T and Zhang H (2022) Aquaporin-8 transports hydrogen peroxide to regulate granulosa cell autophagy. Front. Cell Dev. Biol. 10:897666. doi: 10.3389/fcell.2022.897666
Received: 16 March 2022; Accepted: 25 July 2022;
Published: 23 August 2022.
Edited by:
Rujuan Zuo, Oslo University Hospital, NorwayCopyright © 2022 Huang, Jin, Zhang, Cui, Zhang, Lu, Yu, Ma and Zhang. This is an open-access article distributed under the terms of the Creative Commons Attribution License (CC BY). The use, distribution or reproduction in other forums is permitted, provided the original author(s) and the copyright owner(s) are credited and that the original publication in this journal is cited, in accordance with accepted academic practice. No use, distribution or reproduction is permitted which does not comply with these terms.
*Correspondence: He Zhang, emhhbmdoZTA2MjFAMTYzLmNvbQ==
†These authors have contributed equally to this work