- 1Buck Institute for Research on Aging, Novato, CA, United States
- 2Leonard Davis School of Gerontology, University of Southern California, Los Angeles, CA, United States
A major recent advance in cell biology is the mechanistic and kinetic understanding of biogenesis of many membrane-less condensates. As membrane-less condensates and membrane-bound organelles are two major approaches used by the eukaryotic cells to organize cellular contents, it is not surprising that these membrane-less condensates interact with the membrane-bound organelles and are dynamically regulated by the cellular signaling, metabolic states, and proteostasis network. In this review, I will discuss recent progress in the biogenesis of membrane-less condensates and their connections with well-studied membrane-bound organelles. Future work will reveal the molecular and functional connectome among different condensates and membrane-bound organelles.
Introduction
One major goal of cell biology is to understand the principles and mechanistic details behind the self-organization of cellular contents into individual functional units of different scales. For example, most cell biologists study the biogenesis and functions of membrane-bound organelles in eukaryotic cells, which spatiotemporally and dynamically compartmentalize unique parts of proteome, nucleic acids, lipids, and metabolites to efficiently and specifically carry out different cellular functions. The studies on each individual organelles are now gradually continued by the efforts to elucidate the interaction among different membrane-bound organelles, mainly motivated by the intention to better understand cellular physiology as most cellular functions are fulfilled by multiple steps across different organelles. Although the prototypical organelles are membrane-bound, membrane-less cellular compartments (now often called condensates) have been observed since the very beginning of cell biology research (Montgomery, 1898; Wilson, 1899). The recent years have seen an explosive interest in these membrane-less condensates in the light of their biogenesis through liquid-liquid phase separation (LLPS). With the maturation of the theories behind the biogenesis of membrane-less condensates, it is of great interest to study the inter-connectome of these cellular structures, with and without membrane, to fully understand how the contents and information exchange between them to achieve certain cellular functions. Here I review the biogenesis mechanisms of the membrane-less condensates and their known interactions with membrane-bound organelles.
The Biogenesis of Membrane-Less Condensates
Our interest on the membrane-less condensates dates back to the discovery of nucleolus (Montgomery, 1898; Wilson, 1899) and centrosomes (Boveri, 1888). This also makes nucleolus and centrosome the most studied condensates. While nucleolus and centrosome represent the common condensates found in almost all eukaryotic cells, other membrane-less condensates are usually found in specific cell types, developmental stages, or induced by certain stresses. For example, post-synaptic density is found in the neurons (Zeng et al., 2016), paraspeckles are found in some epithelial cells (Nakagawa et al., 2011), germline P granules are formed during C. elegans embryonic development (Brangwynne et al., 2009), and stress granules (SG) are induced by various stress conditions (Collier and Schlesinger, 1986; Anderson and Kedersha, 2008; Gwon et al., 2021). Except for a few cases, such as the centrosome and post-synaptic density, most of the condensates consist of both proteins and RNA.
The biogenesis of various condensates was initially studied separately to understand the key components and the protein-protein/protein-RNA interactions inside. For example, the formation of SGs was proposed to be nucleated by specific protein-mRNA interaction that forms oligomers which are crosslinked by PABP-1 into microscopically visible SGs (Anderson and Kedersha, 2008). Similarly, a number of nuclear bodies, including nucleolus, histone locus bodies (HLBs), Cajal body, Nuclear splicing speckles, paraspeckle, and nuclear stress bodies (nSB) were nucleated by specific RNAs which recruit additional proteins to form microscopically visible granules (Mao et al., 2011; Shevtsov and Dundr, 2011; Falahati et al., 2016; Falahati and Wieschaus, 2017; Erhardt and Stoecklin, 2020). However, most of these condensates contain hundreds of proteins that their recruitment and interactions remain uncharacterized. For example, nucleolus selectively enrich >700 different nuclear proteins via unknown mechanisms.
The recent resurgence of interest on LLPS provides a fresh perspective on the selective enrichment of different components in a membrane-less condensate. Inspired by the examples such as the P granule and nucleolus with liquid properties (Brangwynne et al., 2011, 2009), a surge of publications revisited different membrane-less condensates and propose that LLPS drives the selective condensation and enrichment of different proteins and mRNAs into each membrane-less compartment. LLPS arises from the supersaturation of molecules: given a specific condition, a molecule in a solution will partition into two separate high-concentration and low-concentration phases when its concentration rises above the critical concentration (Banani et al., 2017). It has been shown that multivalent interactions, either from multidomain proteins or intrinsically disordered regions (IDRs), drive the condensation of molecules (Banani et al., 2017). In this model, multivalent proteins, or scaffold proteins, crosslink with each other to setup the framework which recruits client proteins with lower valency (Banani et al., 2016). Although classical cases of LLPS driven by multidomain proteins have been reported, such as the Nephrin-Nck-N-WASP (Li et al., 2012), most of the condensation events are made possible by the IDRs-mediated multivalent weak interactions. Indeed, proteomes of different membrane-less condensates are enriched in IDRs, such as RNA-binding proteins, which can search multiple conformations (Crick et al., 2006; Mukhopadhyay et al., 2007; Tran et al., 2008; Darling et al., 2018) and form weak intermolecular interactions through the cation-pi, electrostatic, and polypeptide backbone interactions (Xiang et al., 2015; Hughes et al., 2018). It is important to note that although most studies focused on the IDRs-mediated multivalent protein-protein interactions (PPIs), many condensates are dominated by RNAs (Van Treeck and Parker, 2018; Roden and Gladfelter, 2021). For example, the G3BP1 and RNA in the SG is about 1 mg/ml and 64 mg/ml, respectively (Guillén-Boixet et al., 2020). As mRNAs are at least three times longer than polypeptides, it has been suggested that multivalent RNA-RNA and RNA-protein interactions likely dominate the nucleation and condensation of molecules (Zhang et al., 2015; Langdon et al., 2018; Van Treeck and Parker, 2018; Roden and Gladfelter, 2021). These multivalent RNAs and scaffold proteins provide the attractive model in which different client proteins can be recruited to the membrane-less condensates through non-specific weak interactions and LLPS, thus potentially explain the selective recruitment of hundreds of different proteins.
Although the IDR-based multivalent weak interaction and LLPS now are the default explanations for the condensation of membrane-less compartments, we should note that the traditional specific protein-protein/protein-RNA interactions among folded protein and RNA domains also play critical roles (McSwiggen et al., 2019b). For example, although some of the nucleolar proteins can automatically associate and condense into microscopically visible structure, their spatiotemporal localization are nucleated by specific protein-RNA interactions (Falahati et al., 2016). Additionally, some nucleolar proteins are recruited through active process instead of thermodynamic LLPS (Falahati and Wieschaus, 2017). Thus, for a membrane-less condensate that shows phase separation behavior for some of its components, there are many proteins that are recruited via alternative mechanisms. In another case, live-cell single-molecule imaging revealed that transcription factors (TFs) form hubs via multivalent interactions between IDRs without showing LLPS, which happens only when the TFs are overexpressed (Boija et al., 2018; Chong et al., 2018). A recent study on Herpes Simplex Virus replication compartment (RC) showed that although RC displays hallmarks of LLPS, including roundness, fission and fusion, and speedy fluorescence recovery, single particle tracking suggested that RC is formed through non-specific protein-DNA interaction without forming two different phases (McSwiggen et al., 2019a).
The contribution of both thermodynamic LLPS and other alternative mechanisms to the formation of membrane-less condensates are probably best illustrated by the heterogeneity within the condensates formed in vivo, while LLPS alone predicts largely homogenous constitution throughout the condensate. Super resolution studies showed that SGs, P granules, paraspeckles, and RCs have anisotropic properties across the compartment (West et al., 2016; Jain et al., 2016; Wheeler et al., 2016; McSwiggen et al., 2019a; Wang et al., 2014). In the case of SGs, the condensates are composed of stable cores surrounded by a phase separated shell (Jain et al., 2016) (Figure 1). Although such stable cores can be explained by the aging of liquid condensates following LLPS (Molliex et al., 2015; Patel et al., 2015; Xiang et al., 2015), evidence showed that weak nonspecific interactions underlying LLPS are not required for the formation of stable cores, and importantly, the size of these stable cores does not change overtime (Wheeler et al., 2016). In addition, early results showed that the formation of microscopically visible SGs relies on multiple cellular factors, including dynein and kinesin (Loschi et al., 2009; Kwon et al., 2007). The dependence of SG formation on these motors contradicts to the LLPS-aging model and instead, is consistent with a model in which the stable cores of SGs are nucleated through active process into which additional factors are recruited via LLPS (Figure 1). This LLPS independent mechanism seems not unique to SG, as the isolation of endogenously formed p-bodies (PB) and nucleoli into protein-free buffers does not cause the dissolution of these condensates as predicted by LLPS and previously showed with in vitro reconstituted condensates (Hubstenberger et al., 2017; Hayes et al., 2018). Consistently, while LLPS predicts a dynamic exchange of components between different phases, quantification of different PB components revealed that some of the key components show little to no exchange with the surrounding cytosol (Xing et al., 2020). Similarly, the LLPS-mediated condensations of endocytic factors and ZO proteins are initiated by scaffold proteins (Syp1 or tight junction receptors) vis a LLPS-independent process (Beutel et al., 2019; Bergeron-Sandoval et al., 2021).
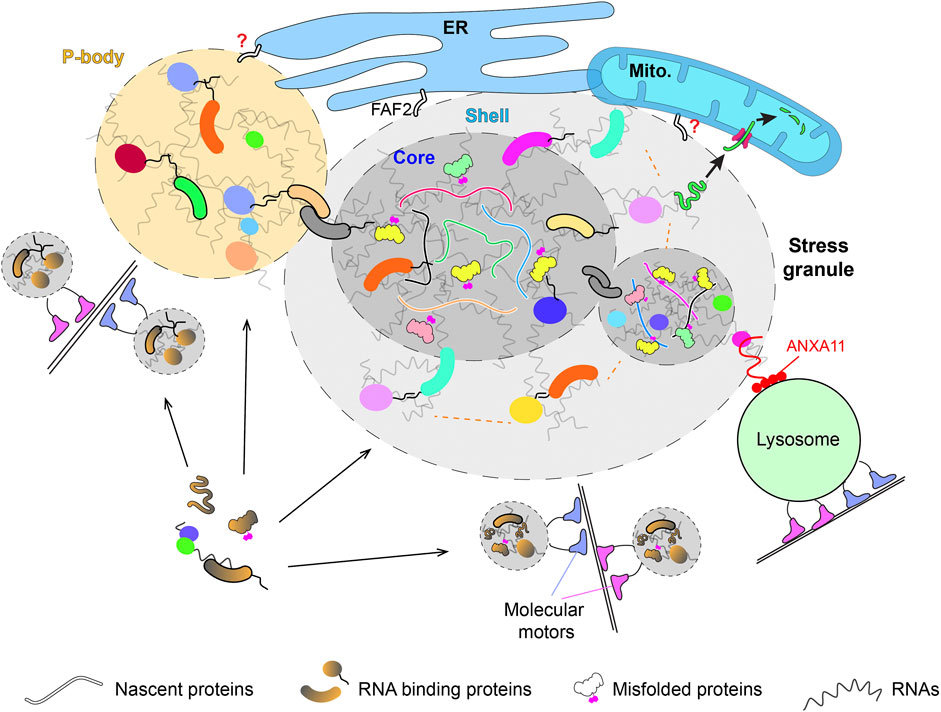
FIGURE 1. The biogenesis and interaction of membrane-less condensates with organelles. P-body and stress granule interact with membrane-bound organelles in different organisms. The spatially localized biogenesis of these condensates suggests certain key membrane-bound molecules initiate their assembly. The recruitment of different components to the condensates happens via both LLPS and alternative mechanisms, such as the active transport by molecular motors. The abundant RNAs in these condensates likely dominate the multivalent weak interactions required to assemble and stabilize these membrane-less structures with help from IDP-containing scaffold proteins. The heterogeneity of endogenously assembled condensates is reflected by the anisotropic properties within each individual condensate (e.g., shell and core in SG) and the heterogenous molecular composition across different condensates in the same cell. The recruitment of nascent and misfolded proteins into the core of SG likely solidifies the structure. The condensate-membrane interaction allows some misfolded proteins to get imported into and degraded inside the mitochondria or hitchhike on lysosomes for long-distance transport.
The Interactions Between Membrane-Less Condensates and Membrane-Bound Organelles
Many membrane-less condensates are spatially close to or interact with each other, such as paraspeckles vs. nuclear speckles and PB vs. SG (Kedersha et al., 2005; Kedersha and Anderson, 2007; Fox and Lamond, 2010; Sanders et al., 2020). The mechanisms behind the physical interactions of different condensates are currently under extensive exploration, with recent results highlighting the contribution of overlapping PPI networks between different condensates to their physical connectivity (Sanders et al., 2020). There are also cases where the membrane-less condensate binds to the membrane-bound organelles. For example, the P granules tightly associate with the nuclear membrane and the nuclear pore complex in the germ cells of C. elegans (Pitt et al., 2000). A TIS11B-enriched protein-RNA condensate (TIS granule) interacts with endoplasmic reticulum (ER) (Ma and Mayr, 2018). In addition, the intercellular junctions, such as tight junctions between epithelium cells and nephrin between podocytes, form plasma membrane associated protein condensates that recruit downstream effectors (Banjade and Rosen, 2014; Beutel et al., 2019; Case et al., 2019). A recent paper reported the liquid phase separation behind the recruitment of multiple components of the endocytic coat on the plasma membrane which drive the deformation and internalization of plasma membrane during endocytosis (Bergeron-Sandoval et al., 2021).
PB and SG are archetypal membrane-less condensates used to study the interaction between membrane-less and membrane-bound structures (Figure 1). Early studies in yeast revealed that PB tend to dock on ER (Kilchert et al., 2010). Proteomics study of the PB interacting proteins discovered two ER-associated proteins (Scp160 and Bfr1) that known to interact with polysome (Weidner et al., 2014). However, Scp160 and Bfr1 are not required for the localization of PB to the ER. PB was also found to dynamically associate with ER in mammalian cells (Lee et al., 2020). This recent study showed that the translational capacity on the cisternal ER sheets correlates with the amount of PB and their ER association. As PB are storage sites of dormant mRNAs, it was speculated that the contact between PB and ER allows mRNA to shuffle between repressive and active translation status (Lee et al., 2020). However, PBs did not tend to associate with the cisternal ER where the mRNA is abundant and stripping mRNA/polysomes from ER by puromycin did not detach PB from ER. As the transcriptome inside PB does not enrich mRNAs related to ER or secretory pathway (Hubstenberger et al., 2017), it remains unclear regarding why and how PB interact with ER.
SGs also interact with membrane-bound organelles. Early studies in yeast showed that SGs, or protein aggregates/Q-bodies, that induced by different stresses are associated with ER and mitochondria (Escusa-Toret et al., 2013; Zhou et al., 2014; Böckler et al., 2017). Recent studies in mammalian cells showed that SGs also associate with membrane-bound organelles, such as lysosomes and ER (Lee et al., 2020; Liao et al., 2019; Gwon et al., 2021). The molecular identities of SG-ER/mitochondria/lysosome interaction remain largely unknown. In the case of SG-lysosome interaction, proteomics study identified ANXA11 as a molecular tether that can dynamically couple SGs with lysosomes (Liao et al., 2019) (Figure 1). Although the SGs were shown to have limited interaction with ER in one study (Liao et al., 2019), a separate study showed that SGs are tightly tethered by ER via FAF2 which marks the fission of SGs (Lee et al., 2020; Gwon et al., 2021) (Figure 1). The fission events of SGs are rare compared to their fusion events, which dominating the LLPS and liquid condensates, highlighting the heterogeneity of SGs in vivo that differ in both compositions and physical properties (Khong et al., 2017). Similarly, PBs also show heterogeneity in vivo with individual PB recruits mRNA independently (Wang et al., 2018) and interacts with ER with different dynamics (Lee et al., 2020). These heterogeneities further support the model that these membrane-less condensates are assembled via a combination of LLPS and alternative mechanisms (Figure 1).
The Functions of Condensates-Organelles Interaction
Many molecular and cellular functions have been proposed for different condensates, such as regulating biochemical reactions (Su et al., 2016; Du and Chen, 2018), sequestration of molecules (Frottin et al., 2019; Youn et al., 2019), compartmentalizing/vectorizing the complex modification of molecules (Riback et al., 2020), and buffering stochastic cellular noises (Klosin et al., 2020). It is important to note that due to the multivalent nature of phase separating molecules, most of the studies used extensive mutations/truncations to remove multivalent interacting sites on key scaffold proteins to block their phase separation. These large-scale mutations likely have pleiotropic effects on other functions of the scaffold protein, which is usually a hub in the network of specific PPIs with hundreds of other molecules in addition to its IDR-mediated weak interactions required for LLPS (Hubstenberger et al., 2017; Sanders et al., 2020; Yang et al., 2020). Furthermore, there are examples that the formation of membrane-less condensates is not required for the related functions. For example, removing NEAT-1, the scaffold of paraspeckles, has no effect in the cells and tissues (Nakagawa et al., 2011). Blocking SG formation did not affect the stress-induced translation repression (Kedersha et al., 2016), and the dissolution of SG is not required to restore translation during recovery (Loschi et al., 2009). Furthermore, long-term exposure to the same stressors causes cellular adaptation that prevents the formation of SGs (Domnauer et al., 2021). Similarly, formation of PB is not required for mRNA decay (Decker et al., 2007). Although multiphase nucleolus is proposed to vectorize the assembly of ribosomes in eukaryotes (Riback et al., 2020), there is no such multiphase structure in prokaryotes for ribosome biogenesis. Similarly, previous studies reported a mitochondrial “RNA granule” that recruits several accessory proteins to assemble mitochondrial ribosome (Barrientos, 2015). Instead of LLPS via IDR-mediated weak multivalent interactions, recent study showed that these accessory proteins fold and co-assemble with ribosome intermediate (Cheng et al., 2021). Future studies are required to address the complexity of native condensates and the discrepancy among different studies (Lyon et al., 2021).
The interactions between membrane-less condensates and membrane-bound organelles play important roles in the functions and fates of condensates. For example, the ER-associated TIS granules enrich AU-rich mRNAs and enable the interaction between the nascent membrane proteins translated inside TIS granule and SET, which sorts the nascent proteins to different subcellular localizations along the secretory pathway (Ma and Mayr, 2018). The plasma membrane-associated protein clusters increase the dwelling time of proteins inside, enabling kinetic proofreading that enhances the activities of the recruited proteins, such as the Nephrin-Nck-N-WASP for actin polymerization and LAT-Grb2-SOS for Ras activation (Case et al., 2019; Huang et al., 2019). It is important to note that membrane-association is not strictly required for both cases as condensates alone without membrane association can also activate actin assembly or Ras signaling (Li et al., 2012; Tulpule et al., 2021). In the case of ER-PB/SG association, ER tubules wrap the condensates and induce the fission of the ER-associated PB/SG (Lee et al., 2020). The lysosome-SG/RNPs interaction mediates the long-range transportation of SGs (Liao et al., 2019). This is similar to early studies in yeast where the tight-association between protein aggregates/SG and mitochondria dominate the motility of these SGs (Zhou et al., 2014; Böckler et al., 2017). It was shown that most of the SGs induced by heat shock are formed directly on the surface of mitochondria (Zhou et al., 2014), suggesting a spatially organized biogenesis of membrane-less condensates on organelles. In contrast to the long-range active transportation of the lysosome-associated SGs in mammalian cells, the mitochondrial association of SGs in yeast reduces the long-range transportation and contributes to the asymmetric retention of these SGs during mitosis (Zhou et al., 2014).
In addition to the motility of SGs, the association between SGs and mitochondria also contributes to the dissolution of SGs. It was shown in both yeast and mammalian cells that many aggregated cytosolic proteins inside SGs were solubilized by chaperones and imported into mitochondria for their degradation (Ruan et al., 2017; Li et al., 2019; Shcherbakov et al., 2019) (Figure 1). Although mitochondrial import is a selective process under physiological and heathy conditions, it is known that some neurodegenerative diseases related proteins get into mitochondria and cause mitochondrial defects (Devi et al., 2006, 2008; Hansson Petersen et al., 2008; Wang et al., 2016). The mitochondrial import of aggregated non-mitochondrial proteins indicates that misfolded proteins can hijack the mitochondrial import pathway if they are presented in the vicinity of the import channels via the mitochondria associated SGs.
It remains largely unclear how and why SGs establish connections with specific membrane-bound organelles. In the case of mitochondria-SG interactions, most of the SGs are formed on the surface of mitochondria and thus maintain their association with mitochondria (Zhou et al., 2014). As the misfolded proteins and RNAs are ubiquitously distributed in the cytosol, this membrane-associated biogenesis of SGs indicates certain spatially localized factors drives the formation of SGs, which resembles the localized nucleation of nucleolus (Falahati et al., 2016). Future studies are required to understand the in vivo biogenesis and interaction of SGs and other membrane-less condensates with organelles. It is also critical to elucidate how the biogenesis of membrane-bound organelles are regulated in a way to prevent the aggregation (formation of SGs) of organellar proteins which are aggregation-prone (Wang and Chen, 2015; Wrobel et al., 2015; Liu et al., 2022).
Conclusion
It is expected that more condensates will be described in the coming years and their interactions with membrane-bound organelles will be a spotlight of future research. Elucidation of the molecular mechanisms linking membrane-less condensates to membrane-bound organelles is critical to understand the function of such interactions. Additionally, future studies will shed light on the fate of different condensates (e.g., asymmetric segregation or degradation via autophagy) and their connections with the inter-organellar contact sites (King et al., 2020). In the end, we will understand the evolutionary perspective of the interactions between condensates and organelles, two major ways of organizing the cellular contents.
Author Contributions
CZ conceived and wrote the manuscript, designed the figure.
Funding
This work was supported by NIH Office of the Director DP5OD024598 and National Institute on Aging R03AG070478 to CZ Opinions, interpretations, conclusion, and recommendations are those of the author and are not necessarily endorsed by the any of the funder.
Conflict of Interest
The author declares that the research was conducted in the absence of any commercial or financial relationships that could be construed as a potential conflict of interest.
Publisher’s Note
All claims expressed in this article are solely those of the authors and do not necessarily represent those of their affiliated organizations, or those of the publisher, the editors and the reviewers. Any product that may be evaluated in this article, or claim that may be made by its manufacturer, is not guaranteed or endorsed by the publisher.
Acknowledgments
The authors would like to apologize to those colleagues whose work could not be cited or discussed in sufficient detail due to space limitations.
References
Anderson, P., and Kedersha, N. (2008). Stress Granules: the Tao of RNA Triage. Trends Biochem. Sci. 33, 141–150. doi:10.1016/j.tibs.2007.12.003
Banani, S. F., Lee, H. O., Hyman, A. A., and Rosen, M. K. (2017). Biomolecular Condensates: Organizers of Cellular Biochemistry. Nat. Rev. Mol. Cel Biol. 18, 285–298. doi:10.1038/nrm.2017.7
Banani, S. F., Rice, A. M., Peeples, W. B., Lin, Y., Jain, S., Parker, R., et al. (2016). Compositional Control of Phase-Separated Cellular Bodies. Cell 166, 651–663. doi:10.1016/j.cell.2016.06.010
Banjade, S., and Rosen, M. K. (2014). Phase Transitions of Multivalent Proteins Can Promote Clustering of Membrane Receptors. Elife 3, 4123. doi:10.7554/eLife.04123
Barrientos, A. (2015). Mitochondriolus: Assembling Mitoribosomes. Oncotarget 6, 16800–16801. doi:10.18632/oncotarget.4646
Bergeron-Sandoval, L.-P., Kumar, S., Heris, H. K., Chang, C. L. A., Cornell, C. E., Keller, S. L., et al. (2021). Endocytic Proteins with Prion-like Domains Form Viscoelastic Condensates that Enable Membrane Remodeling. Proc. Natl. Acad. Sci. U.S.A. 118, 89118. doi:10.1073/pnas.2113789118
Beutel, O., Maraspini, R., Pombo-García, K., Martin-Lemaitre, C., and Honigmann, A. (2019). Phase Separation of Zonula Occludens Proteins Drives Formation of Tight Junctions. Cell 179, 923–936. e11. doi:10.1016/j.cell.2019.10.011
Böckler, S., Chelius, X., Hock, N., Klecker, T., Wolter, M., Weiss, M., et al. (2017). Fusion, Fission, and Transport Control Asymmetric Inheritance of Mitochondria and Protein Aggregates. J. Cel Biol. 216, 2481–2498. doi:10.1083/jcb.201611197
Boija, A., Klein, I. A., Sabari, B. R., Dall’Agnese, A., Coffey, E. L., Zamudio, A. V., et al. (2018). Transcription Factors Activate Genes through the Phase-Separation Capacity of Their Activation Domains. Cell 175, 1842–1855. e16. doi:10.1016/j.cell.2018.10.042
Boveri, T. (1888). Die Befruchtung und Teilung des Eies von Ascaris megalocephala. Zellen-Studien 2 Jena: G. Fischer. Google Scholar.
Brangwynne, C. P., Eckmann, C. R., Courson, D. S., Rybarska, A., Hoege, C., Gharakhani, J., et al. (2009). Germline P Granules Are Liquid Droplets that Localize by Controlled Dissolution/condensation. Science 324, 1729–1732. doi:10.1126/science.1172046
Brangwynne, C. P., Mitchison, T. J., and Hyman, A. A. (2011). Active Liquid-like Behavior of Nucleoli Determines Their Size and Shape in Xenopus laevis Oocytes. Proc. Natl. Acad. Sci. U.S.A. 108, 4334–4339. doi:10.1073/pnas.1017150108
Case, L. B., Zhang, X., Ditlev, J. A., and Rosen, M. K. (2019). Stoichiometry Controls Activity of Phase-Separated Clusters of Actin Signaling Proteins. Science 363, 1093–1097. doi:10.1126/science.aau6313
Cheng, J., Berninghausen, O., and Beckmann, R. (2021). A Distinct Assembly Pathway of the Human 39S Late Pre-mitoribosome. Nat. Commun. 12, 4544. doi:10.1038/s41467-021-24818-x
Chong, S., Dugast-Darzacq, C., Liu, Z., Dong, P., Dailey, G. M., Cattoglio, C., et al. (2018). Imaging Dynamic and Selective Low-Complexity Domain Interactions that Control Gene Transcription. Science 361, 2555. doi:10.1126/science.aar2555
Collier, N. C., and Schlesinger, M. J. (1986). The Dynamic State of Heat Shock Proteins in Chicken Embryo Fibroblasts. J. Cel Biol. 103, 1495–1507. doi:10.1083/jcb.103.4.1495
Crick, S. L., Jayaraman, M., Frieden, C., Wetzel, R., and Pappu, R. V. (2006). Fluorescence Correlation Spectroscopy Shows that Monomeric Polyglutamine Molecules Form Collapsed Structures in Aqueous Solutions. Proc. Natl. Acad. Sci. U.S.A. 103, 16764–16769. doi:10.1073/pnas.0608175103
Darling, A. L., Liu, Y., Oldfield, C. J., and Uversky, V. N. (2018). Intrinsically Disordered Proteome of Human Membrane‐Less Organelles. Proteomics 18, 1700193. doi:10.1002/pmic.201700193
Decker, C. J., Teixeira, D., and Parker, R. (2007). Edc3p and a Glutamine/asparagine-Rich Domain of Lsm4p Function in Processing Body Assembly in Saccharomyces cerevisiae. J. Cel Biol. 179, 437–449. doi:10.1083/jcb.200704147
Devi, L., Prabhu, B. M., Galati, D. F., Avadhani, N. G., and Anandatheerthavarada, H. K. (2006). Accumulation of Amyloid Precursor Protein in the Mitochondrial Import Channels of Human Alzheimer's Disease Brain Is Associated with Mitochondrial Dysfunction. J. Neurosci. 26, 9057–9068. doi:10.1523/JNEUROSCI.1469-06.2006
Devi, L., Raghavendran, V., Prabhu, B. M., Avadhani, N. G., and Anandatheerthavarada, H. K. (2008). Mitochondrial Import and Accumulation of α-Synuclein Impair Complex I in Human Dopaminergic Neuronal Cultures and Parkinson Disease Brain. J. Biol. Chem. 283, 9089–9100. doi:10.1074/jbc.M710012200
Domnauer, M., Zheng, F., Li, L., Zhang, Y., Chang, C. E., Unruh, J. R., et al. (2021). Proteome Plasticity in Response to Persistent Environmental Change. Mol. Cel 81, 3294–3309. e12. doi:10.1016/j.molcel.2021.06.028
Du, M., and Chen, Z. J. (2018). DNA-induced Liquid Phase Condensation of cGAS Activates Innate Immune Signaling. Science 361, 704–709. doi:10.1126/science.aat1022
Erhardt, S., and Stoecklin, G. (2020). The Heat's on: Nuclear Stress Bodies Signal Intron Retention. EMBO J. 39, e104154. doi:10.15252/embj.2019104154
Escusa-Toret, S., Vonk, W. I. M., and Frydman, J. (2013). Spatial Sequestration of Misfolded Proteins by a Dynamic Chaperone Pathway Enhances Cellular Fitness during Stress. Nat. Cel Biol. 15, 1231–1243. doi:10.1038/ncb2838
Falahati, H., Pelham-Webb, B., Blythe, S., and Wieschaus, E. (2016). Nucleation by rRNA Dictates the Precision of Nucleolus Assembly. Curr. Biol. 26, 277–285. doi:10.1016/j.cub.2015.11.065
Falahati, H., and Wieschaus, E. (2017). Independent Active and Thermodynamic Processes Govern the Nucleolus Assembly In Vivo. Proc. Natl. Acad. Sci. U.S.A. 114, 1335–1340. doi:10.1073/pnas.1615395114
Fox, A. H., and Lamond, A. I. (2010). Paraspeckles. Cold Spring Harbor Perspect. Biol. 2, a000687. doi:10.1101/cshperspect.a000687
Frottin, F., Schueder, F., Tiwary, S., Gupta, R., Körner, R., Schlichthaerle, T., et al. (2019). The Nucleolus Functions as a Phase-Separated Protein Quality Control Compartment. Science 365, 342–347. doi:10.1126/science.aaw9157
Guillén-Boixet, J., Kopach, A., Holehouse, A. S., Wittmann, S., Jahnel, M., Schlüßler, R., et al. (2020). RNA-induced Conformational Switching and Clustering of G3BP Drive Stress Granule Assembly by Condensation. Cell 181, 346–361. e17. doi:10.1016/j.cell.2020.03.049
Gwon, Y., Maxwell, B. A., Kolaitis, R.-M., Zhang, P., Kim, H. J., and Taylor, J. P. (2021). Ubiquitination of G3BP1 Mediates Stress Granule Disassembly in a Context-specific Manner. Science 372, eabf6548. doi:10.1126/science.abf6548
Hansson Petersen, C. A., Alikhani, N., Behbahani, H., Wiehager, B., Pavlov, P. F., Alafuzoff, I., et al. (2008). The Amyloid β-peptide Is Imported into Mitochondria via the TOM Import Machinery and Localized to Mitochondrial Cristae. Proc. Natl. Acad. Sci. U.S.A. 105, 13145–13150. doi:10.1073/pnas.0806192105
Hayes, M. H., Peuchen, E. H., Dovichi, N. J., and Weeks, D. L. (2018). Dual Roles for ATP in the Regulation of Phase Separated Protein Aggregates in Xenopus Oocyte Nucleoli. Elife 7, 35224. doi:10.7554/eLife.35224
Huang, W. Y. C., Alvarez, S., Kondo, Y., Lee, Y. K., Chung, J. K., Lam, H. Y. M., et al. (2019). A Molecular Assembly Phase Transition and Kinetic Proofreading Modulate Ras Activation by SOS. Science 363, 1098–1103. doi:10.1126/science.aau5721
Hubstenberger, A., Courel, M., Bénard, M., Souquere, S., Ernoult-Lange, M., Chouaib, R., et al. (2017). P-body Purification Reveals the Condensation of Repressed mRNA Regulons. Mol. Cel 68, 144–157. e5. doi:10.1016/j.molcel.2017.09.003
Hughes, M. P., Sawaya, M. R., Boyer, D. R., Goldschmidt, L., Rodriguez, J. A., Cascio, D., et al. (2018). Atomic Structures of Low-Complexity Protein Segments Reveal Kinked β Sheets that Assemble Networks. Science 359, 698–701. doi:10.1126/science.aan6398
Jain, S., Wheeler, J. R., Walters, R. W., Agrawal, A., Barsic, A., and Parker, R. (2016). ATPase-Modulated Stress Granules Contain a Diverse Proteome and Substructure. Cell 164, 487–498. doi:10.1016/j.cell.2015.12.038
Kedersha, N., and Anderson, P. (2007). “Mammalian Stress Granules and Processing Bodies,” in Translation Initiation: Cell Biology, High‐Throughput Methods, and Chemical‐Based Approaches Methods in Enzymology (Elsevier), 61–81. doi:10.1016/S0076-6879(07)31005-7
Kedersha, N., Panas, M. D., Achorn, C. A., Lyons, S., Tisdale, S., Hickman, T., et al. (2016). G3BP-Caprin1-USP10 Complexes Mediate Stress Granule Condensation and Associate with 40S Subunits. J. Cel Biol. 212, 845–860. doi:10.1083/jcb.201508028
Kedersha, N., Stoecklin, G., Ayodele, M., Yacono, P., Lykke-Andersen, J., Fritzler, M. J., et al. (2005). Stress Granules and Processing Bodies Are Dynamically Linked Sites of mRNP Remodeling. J. Cel Biol. 169, 871–884. doi:10.1083/jcb.200502088
Khong, A., Matheny, T., Jain, S., Mitchell, S. F., Wheeler, J. R., and Parker, R. (2017). The Stress Granule Transcriptome Reveals Principles of mRNA Accumulation in Stress Granules. Mol. Cel 68, 808–820. e5. doi:10.1016/j.molcel.2017.10.015
Kilchert, C., Weidner, J., Prescianotto-Baschong, C., and Spang, A. (2010). Defects in the Secretory Pathway and High Ca2+Induce Multiple P-Bodies. MBoC 21, 2624–2638. doi:10.1091/mbc.E10-02-0099
King, C., Sengupta, P., Seo, A. Y., and Lippincott-Schwartz, J. (2020). ER Membranes Exhibit Phase Behavior at Sites of Organelle Contact. Proc. Natl. Acad. Sci. U.S.A. 117, 7225–7235. doi:10.1073/pnas.1910854117
Klosin, A., Oltsch, F., Harmon, T., Honigmann, A., Jülicher, F., Hyman, A. A., et al. (2020). Phase Separation Provides a Mechanism to Reduce Noise in Cells. Science 367, 464–468. doi:10.1126/science.aav6691
Kwon, S., Zhang, Y., and Matthias, P. (2007). The Deacetylase HDAC6 Is a Novel Critical Component of Stress Granules Involved in the Stress Response. Genes Dev. 21, 3381–3394. doi:10.1101/gad.461107
Langdon, E. M., Qiu, Y., Ghanbari Niaki, A., McLaughlin, G. A., Weidmann, C. A., Gerbich, T. M., et al. (2018). mRNA Structure Determines Specificity of a polyQ-Driven Phase Separation. Science 360, 922–927. doi:10.1126/science.aar7432
Lee, J. E., Cathey, P. I., Wu, H., Parker, R., and Voeltz, G. K. (2020). Endoplasmic Reticulum Contact Sites Regulate the Dynamics of Membraneless Organelles. Science 367, 7108. doi:10.1126/science.aay7108
Li, P., Banjade, S., Cheng, H.-C., Kim, S., Chen, B., Guo, L., et al. (2012). Phase Transitions in the Assembly of Multivalent Signalling Proteins. Nature 483, 336–340. doi:10.1038/nature10879
Li, Y., Xue, Y., Xu, X., Wang, G., Liu, Y., Wu, H., et al. (2019). A Mitochondrial FUNDC1/HSC70 Interaction Organizes the Proteostatic Stress Response at the Risk of Cell Morbidity. EMBO J. 38, 798786. doi:10.15252/embj.201798786
Liao, Y.-C., Fernandopulle, M. S., Wang, G., Choi, H., Hao, L., Drerup, C. M., et al. (2019). RNA Granules Hitchhike on Lysosomes for Long-Distance Transport, Using Annexin A11 as a Molecular Tether. Cell 179, 147–164. e20. doi:10.1016/j.cell.2019.08.050
Liu, Q., Chang, C. E., Wooldredge, A. C., Fong, B., Kennedy, B. K., and Zhou, C. (2022). Tom70-based Transcriptional Regulation of Mitochondrial Biogenesis and Aging. Elife 11, 75658. doi:10.7554/eLife.75658
Loschi, M., Leishman, C. C., Berardone, N., and Boccaccio, G. L. (2009). Dynein and Kinesin Regulate Stress-Granule and P-Body Dynamics. J. Cel Sci. 122, 3973–3982. doi:10.1242/jcs.051383
Lyon, A. S., Peeples, W. B., and Rosen, M. K. (2021). A Framework for Understanding the Functions of Biomolecular Condensates across Scales. Nat. Rev. Mol. Cel Biol. 22, 215–235. doi:10.1038/s41580-020-00303-z
Ma, W., and Mayr, C. (2018). A Membraneless Organelle Associated with the Endoplasmic Reticulum Enables 3′UTR-Mediated Protein-Protein Interactions. Cell 175, 1492–1506. doi:10.1016/j.cell.2018.10.007
Mao, Y. S., Sunwoo, H., Zhang, B., and Spector, D. L. (2011). Direct Visualization of the Co-transcriptional Assembly of a Nuclear Body by Noncoding RNAs. Nat. Cel Biol. 13, 95–101. doi:10.1038/ncb2140
McSwiggen, D. T., Hansen, A. S., Teves, S. S., Marie-Nelly, H., Hao, Y., Heckert, A. B., et al. (2019a). Evidence for DNA-Mediated Nuclear Compartmentalization Distinct from Phase Separation. Elife 8, 47098. doi:10.7554/eLife.47098
McSwiggen, D. T., Mir, M., Darzacq, X., and Tjian, R. (2019b). Evaluating Phase Separation in Live Cells: Diagnosis, Caveats, and Functional Consequences. Genes Dev. 33, 1619–1634. doi:10.1101/gad.331520.119
Molliex, A., Temirov, J., Lee, J., Coughlin, M., Kanagaraj, A. P., Kim, H. J., et al. (2015). Phase Separation by Low Complexity Domains Promotes Stress Granule Assembly and Drives Pathological Fibrillization. Cell 163, 123–133. doi:10.1016/j.cell.2015.09.015
Montgomery, T. S. H. (1898). Comparative Cytological Studies, with Especial Regard to the Morphology of the Nucleolus. J. Morphol. 15, 265–582. doi:10.1002/jmor.1050150204
Mukhopadhyay, S., Krishnan, R., Lemke, E. A., Lindquist, S., and Deniz, A. A. (2007). A Natively Unfolded Yeast Prion Monomer Adopts an Ensemble of Collapsed and Rapidly Fluctuating Structures. Proc. Natl. Acad. Sci. U.S.A. 104, 2649–2654. doi:10.1073/pnas.0611503104
Nakagawa, S., Naganuma, T., Shioi, G., and Hirose, T. (2011). Paraspeckles Are Subpopulation-specific Nuclear Bodies that Are Not Essential in Mice. J. Cel Biol. 193, 31–39. doi:10.1083/jcb.201011110
Patel, A., Lee, H. O., Jawerth, L., Maharana, S., Jahnel, M., Hein, M. Y., et al. (2015). A Liquid-To-Solid Phase Transition of the ALS Protein FUS Accelerated by Disease Mutation. Cell 162, 1066–1077. doi:10.1016/j.cell.2015.07.047
Pitt, J. N., Schisa, J. A., and Priess, J. R. (2000). P Granules in the Germ Cells of Caenorhabditis elegans Adults Are Associated with Clusters of Nuclear Pores and Contain RNA. Develop. Biol. 219, 315–333. doi:10.1006/dbio.2000.9607
Riback, J. A., Zhu, L., Ferrolino, M. C., Tolbert, M., Mitrea, D. M., Sanders, D. W., et al. (2020). Composition-dependent Thermodynamics of Intracellular Phase Separation. Nature 581, 209–214. doi:10.1038/s41586-020-2256-2
Roden, C., and Gladfelter, A. S. (2021). RNA Contributions to the Form and Function of Biomolecular Condensates. Nat. Rev. Mol. Cel Biol. 22, 183–195. doi:10.1038/s41580-020-0264-6
Ruan, L., Zhou, C., Jin, E., Kucharavy, A., Zhang, Y., Wen, Z., et al. (2017). Cytosolic Proteostasis through Importing of Misfolded Proteins into Mitochondria. Nature 543, 443–446. doi:10.1038/nature21695
Sanders, D. W., Kedersha, N., Lee, D. S. W., Strom, A. R., Drake, V., Riback, J. A., et al. (2020). Competing Protein-RNA Interaction Networks Control Multiphase Intracellular Organization. Cell 181, 306–324. e28. doi:10.1016/j.cell.2020.03.050
Shcherbakov, D., Teo, Y., Boukari, H., Cortes-Sanchon, A., Mantovani, M., Osinnii, I., et al. (2019). Ribosomal Mistranslation Leads to Silencing of the Unfolded Protein Response and Increased Mitochondrial Biogenesis. Commun. Biol. 2, 381. doi:10.1038/s42003-019-0626-9
Shevtsov, S. P., and Dundr, M. (2011). Nucleation of Nuclear Bodies by RNA. Nat. Cel Biol. 13, 167–173. doi:10.1038/ncb2157
Su, X., Ditlev, J. A., Hui, E., Xing, W., Banjade, S., Okrut, J., et al. (2016). Phase Separation of Signaling Molecules Promotes T Cell Receptor Signal Transduction. Science 352, 595–599. doi:10.1126/science.aad9964
Tran, H. T., Mao, A., and Pappu, R. V. (2008). Role of Backbone−Solvent Interactions in Determining Conformational Equilibria of Intrinsically Disordered Proteins. J. Am. Chem. Soc. 130, 7380–7392. doi:10.1021/ja710446s
Tulpule, A., Guan, J., Neel, D. S., Allegakoen, H. R., Lin, Y. P., Brown, D., et al. (2021). Kinase-mediated RAS Signaling via Membraneless Cytoplasmic Protein Granules. Cell 184, 2649–2664. e18. doi:10.1016/j.cell.2021.03.031
Van Treeck, B., and Parker, R. (2018). Emerging Roles for Intermolecular RNA-RNA Interactions in RNP Assemblies. Cell 174, 791–802. doi:10.1016/j.cell.2018.07.023
Wang, C., Schmich, F., Srivatsa, S., Weidner, J., Beerenwinkel, N., and Spang, A. (2018). Context-dependent Deposition and Regulation of mRNAs in P-Bodies. Elife 7, 29815. doi:10.7554/eLife.29815
Wang, J. T., Smith, J., Chen, B.-C., Schmidt, H., Rasoloson, D., Paix, A., et al. (2014). Regulation of RNA Granule Dynamics by Phosphorylation of Serine-Rich, Intrinsically Disordered Proteins in C Elegans. Elife 3, e04591. doi:10.7554/eLife.04591
Wang, W., Wang, L., Lu, J., Siedlak, S. L., Fujioka, H., Liang, J., et al. (2016). The Inhibition of TDP-43 Mitochondrial Localization Blocks its Neuronal Toxicity. Nat. Med. 22, 869–878. doi:10.1038/nm.4130
Wang, X., and Chen, X. J. (2015). A Cytosolic Network Suppressing Mitochondria-Mediated Proteostatic Stress and Cell Death. Nature 524, 481–484. doi:10.1038/nature14859
Weidner, J., Wang, C., Prescianotto-Baschong, C., Estrada, A. F., and Spang, A. (2014). The Polysome-Associated Proteins Scp160 and Bfr1 Prevent P Body Formation under normal Growth Conditions. J. Cel Sci. 127, 1992–2004. doi:10.1242/jcs.142083
West, J. A., Mito, M., Kurosaka, S., Takumi, T., Tanegashima, C., Chujo, T., et al. (2016). Structural, Super-resolution Microscopy Analysis of Paraspeckle Nuclear Body Organization. J. Cel Biol. 214, 817–830. doi:10.1083/jcb.201601071
Wheeler, J. R., Matheny, T., Jain, S., Abrisch, R., and Parker, R. (2016). Distinct Stages in Stress Granule Assembly and Disassembly. Elife 5, 18413. doi:10.7554/eLife.18413
Wrobel, L., Topf, U., Bragoszewski, P., Wiese, S., Sztolsztener, M. E., Oeljeklaus, S., et al. (2015). Mistargeted Mitochondrial Proteins Activate a Proteostatic Response in the Cytosol. Nature 524, 485–488. doi:10.1038/nature14951
Xiang, S., Kato, M., Wu, L. C., Lin, Y., Ding, M., Zhang, Y., et al. (2015). The LC Domain of hnRNPA2 Adopts Similar Conformations in Hydrogel Polymers, Liquid-like Droplets, and Nuclei. Cell 163, 829–839. doi:10.1016/j.cell.2015.10.040
Xing, W., Muhlrad, D., Parker, R., and Rosen, M. K. (2020). A Quantitative Inventory of Yeast P Body Proteins Reveals Principles of Composition and Specificity. Elife 9, 56525. doi:10.7554/eLife.56525
Yang, P., Mathieu, C., Kolaitis, R.-M., Zhang, P., Messing, J., Yurtsever, U., et al. (2020). G3BP1 Is a Tunable Switch that Triggers Phase Separation to Assemble Stress Granules. Cell 181, 325–345. e28. doi:10.1016/j.cell.2020.03.046
Youn, J.-Y., Dyakov, B. J. A., Zhang, J., Knight, J. D. R., Vernon, R. M., Forman-Kay, J. D., et al. (2019). Properties of Stress Granule and P-Body Proteomes. Mol. Cel 76, 286–294. doi:10.1016/j.molcel.2019.09.014
Zeng, M., Shang, Y., Araki, Y., Guo, T., Huganir, R. L., and Zhang, M. (2016). Phase Transition in Postsynaptic Densities Underlies Formation of Synaptic Complexes and Synaptic Plasticity. Cell 166, 1163–1175. e12. doi:10.1016/j.cell.2016.07.008
Zhang, H., Elbaum-Garfinkle, S., Langdon, E. M., Taylor, N., Occhipinti, P., Bridges, A. A., et al. (2015). RNA Controls Polyq Protein Phase Transitions. Mol. Cel 60, 220–230. doi:10.1016/j.molcel.2015.09.017
Keywords: membrane-bound organelles, membrane-less condensates, stress granules, protein aggregates, phase separation
Citation: Zhou C (2022) The Molecular and Functional Interaction Between Membrane-Bound Organelles and Membrane-Less Condensates. Front. Cell Dev. Biol. 10:896305. doi: 10.3389/fcell.2022.896305
Received: 14 March 2022; Accepted: 07 April 2022;
Published: 25 April 2022.
Edited by:
Kristopher Burkewitz, Vanderbilt University, United StatesReviewed by:
Etienne Morel, INSERM U1151 Institut Necker Enfants Malades, FranceVladimir N. Uversky, University of South Florida, United States
Copyright © 2022 Zhou. This is an open-access article distributed under the terms of the Creative Commons Attribution License (CC BY). The use, distribution or reproduction in other forums is permitted, provided the original author(s) and the copyright owner(s) are credited and that the original publication in this journal is cited, in accordance with accepted academic practice. No use, distribution or reproduction is permitted which does not comply with these terms.
*Correspondence: Chuankai Zhou, a3pob3VAYnVja2luc3RpdHV0ZS5vcmc=