- 1Department of Breast and Thyroid Surgery, Renmin Hospital of Wuhan University, Wuhan, Hubei, China
- 2Department of Pathology, Renmin Hospital of Wuhan University, Wuhan, Hubei, China
- 3Department of Clinical Laboratory, Renmin Hospital of Wuhan University, Wuhan, Hubei, China
- 4Tongji University Cancer Center, Shanghai Tenth People’s Hospital of Tongji University, School of Medicine, Tongji University, Shanghai, China.
Immune responses in nonlymphoid tissues play a vital role in the maintenance of homeostasis. Lots of evidence supports that tissue-specific immune cells provide defense against tumor through the localization in different tissue throughout the body, and can be regulated by diverse factors. Accordingly, the distribution of nervous tissue is also tissue-specific which is essential in the growth of corresponding organs, and the occurrence and development of tumor. Although there have been many mature perspectives on the neuroendocrine regulation in tumor microenvironment, the neuroendocrine regulation of tissue-specific immune cells has not yet been summarized. In this review, we focus on how tissue immune responses are influenced by autonomic nervous system, sensory nerves, and various neuroendocrine factors and reversely how tissue-specific immune cells communicate with neuroendocrine system through releasing different factors. Furthermore, we pay attention to the potential mechanisms of neuroendocrine-tissue specific immunity axis involved in tumors. This may provide new insights for the immunotherapy of tumors in the future.
1 Introduction
Tumors develop in complicated tissue environments, which they rely on for growth, invasion and metastasis (Quail and Joyce, 2013). The tumor microenvironment (TME) is a heterogeneous ecosystem composed of all the structures at the site and those that are recruited to the area—immune cells, mesenchymal stem cells, vascular vessels, nerves and matrix components (Hanahan and Coussens, 2012). Tumors are derived from the complex interactions that occur between them (Gysler and Drapkin, 2021). With the constant exploration of new ways to treat tumors, therapies targeting the TME have emerged as a promising approach for cancer treatment for the past few years (Bejarano et al., 2021). Research has progressed, but there are remaining unknowns. The composition of the TME is being elucidated, with the neurological, immunological and microbiological components being identified. However, other characteristics and the dynamic evolution of the TME require further in-depth exploration.
Tissue-specific immunity occurs when immune cells establish permanent tissue residency in different organs, thus creating a defensive system (Klose and Artis, 2020). Numerous immune cells reside in nonlymphoid tissues. These tissue-resident populations do not recirculate and adopt a unique phenotype that is distinct from immune cells in the blood or lymphatic system (Mackay and Kallies, 2017). The origin of tissue-resident immune cells is complex and includes tissue innate immune cells and immune cells that have migrated from the peripheral blood. Innate immune cells, including macrophages, dendritic cells (DCs) and innate lymphoid cells (ILCs), exhibit tissue-specific subset compositions in the lung, skin, intestines and comprise the early responders to pathogen encounters. Adaptive immune cells, such as T cells and B cells, also have tissue-resident subsets that help to build immune memory. For example, tissue-resident memory T cells persist as tissue-resident populations in mucosal and exocrine sites, while memory B cells predominate in the intestines. In general, the human immune system is localized in a tissue-specific manner in diverse sites (Weisberg et al., 2021). Functionally, tissue-specific immunity is observed to play a part in tumor development through various regulatory mechanisms (Lei et al., 2020; Liu et al., 2022a).
Recently, the contribution of nerves to the pathogenesis of malignancies has emerged (Zahalka and Frenette, 2020). Several landmark studies have demonstrated that the nervous system plays an active role in tumorigenesis (Reavis et al., 2020). Meanwhile, some studies have suggested that tumor innervation is associated with accelerated tumor progression in multiple cancers (Albo et al., 2011; Magnon et al., 2013; Huang et al., 2014; Kappos et al., 2018; Renz et al., 2018). The nervous system is composed of the central nervous system (CNS) and the peripheral nervous system (PNS). The CNS is the main part of the nervous system and is composed of the spinal cord and brain, which play a part in tumor development. Studies have reported that chronic stress causes disorders in the CNS and promotes tumor initiation and progression (Saul et al., 2005; Goldfarb et al., 2011; Levi et al., 2011; Partecke et al., 2016). β-adrenergic activation by the cAMP–PKA signaling pathway, e.g., β-adrenergic receptors (ARs) stimulated by norepinephrine (NE), is a major mechanism by which stress can enhance tumor development and increase vascularization (Thaker et al., 2006). Furthermore, immune regulation also plays a vital role in stress-induced tumor development (Dhabhar, 2009). Studies have shown that chronic stress causes a large-scale alteration of immune cells in tissues and induces suppression or dysregulation of immune function (Dhabhar et al., 2012; Dhabhar, 2014). The PNS emanates from the CNS and is divided into the autonomic systems and somatosensory, which are responsible for communicating with all parts of the body. Autonomic nerve density was reported to be associated with tumor prognosis and progression (Silverman et al., 2021). In terms of mechanism, infiltrating sympathetic nerves facilitated tumor progression and invasion through β-adrenergic activation (Pon et al., 2016). Adrenergic nerves also facilitate tumor growth by releasing NE to stimulate angiogenesis via VEGF signaling (Zahalka et al., 2017). In light of neural receptors are expressed by many immune cell types, it follows that immune cells may also participate in oncogenesis through adrenergic signaling (Silverman et al., 2021). Neuroendocrine factors contain neurotransmitters, neuropeptides and many other factors secreted by the nervous system mediate stimulatory or inhibitory functions by binding to their respective receptors. In recent decades, many discoveries have elucidated their regulatory roles in tissues and organs (Jiang et al., 2020a). For instance, studies in nerve growth factor (NGF) has reported that this factor acts not only on the PNS and CNS but also on nonneuronal and cancer cells (Aloe et al., 2016). NGF and its receptor TrkA have been implicated in the development of many aggressive cancers. However, NGF pathway has been proved to be critical to inflammation control and the immune response in tumor (Campos et al., 2007; Retamales-Ortega et al., 2017; Wehkamp et al., 2018; Triaca et al., 2019; Jiang et al., 2020b). Nerves regulate multiple components of the TME. Importantly, with the development of high-throughput sequencing techniques including single-cell RNA sequencing and spatial transcriptomics (Liu et al., 2022b), researchers found that immune and neuronal cells are often colocalized to form neuroimmune cell units (Huh and Veiga-Fernandes, 2020). And, both immune cells and neurons express receptors to sense neurotransmitters and cytokines, allowing direct interactions between the two systems (Seillet and Jacquelot, 2019).
Relatively few studies have investigated the functional role of neuroendocrine in carcinogenesis and regulation of the TME. Here, we summarize the mechanisms of neuroendocrine regulation of tissue-specific immunity based on the neuroendocrine signal transmission medium and how tissue-specific immune cells communicate with nerves by releasing different factors. We then focus on the role of neuroendocrine regulation in tumor immunity and how it informs prognosis and treatment.
2 Neuroendocrine regulation in tissue-specific immunity
The nervous system is composed of various components such as the CNS, sympathetic nerves, parasympathetic nerves, and sensory nerve nociceptors, and it mediates immune cells primarily by secreting neuroendocrine factors such as neurotransmitters and neuropeptides. These molecules can regulate tissue-specific immunity by binding to their corresponding receptors expressed on immune cells in various tissues. Here, we summarize the neuroendocrine regulation of tissue-specific immunity, categorized by signaling mediator.
2.1 Adrenergic regulation
The adrenergic system is composed of NE and epinephrine, which are the primary neurotransmitters secreted by postganglionic sympathetic neurons. They regulate cellular function through ARs, including the α1-, α2-, β1-, β2-, and β3-ARs (Tanner et al., 2021). The immune effects of adrenergic signaling are primarily transmitted by β2-ARs, which are expressed on nearly all major immune cell types (Marino and Cosentino, 2013). NE has been shown to mediate the cell trafficking and effector activities of immune cells via β2-AR.
NE affects the function of the CNS. Microglia are the most common resident innate immune cells in the CNS and monitor brain homeostasis by producing ligands that support neuronal survival, pruning non-functional synapses and removing dying neurons (Nayak et al., 2014; Werneburg et al., 2017). Studies have shown that NE upregulates the expression of the amyloid beta peptide (Aβ) receptor mFPR2 through activation of β2-AR in microglia, which helps to maintain the adequate uptake and clearance of Aβ(42). Moreover, increased secretion of NE suppresses the microglial response and reduces the upregulation of both anti- and pro-inflammatory cytokines through β2-adrenergic signaling (Lechtenberg et al., 2019). In general, NE can modulate microglial motility, which can affect the function of microglia in some pathogenic situations (Gyoneva and Traynelis, 2013; Umpierre and Wu, 2020).
Tissue-resident macrophages, ILCs, tissue-resident T cells and NK cells are common tissue-resident immune cells in the periphery. They act in a tissue-specific manner in adipose tissue, liver, lung and gut, where NE has been shown to modulate these cells via β2-adrenergic signaling. In adipose tissue, adipose tissue macrophages (ATMs) and ILC2s are common resident immune cells that act as dominant initiators of type 2 inflammation and tissue repair (Cording et al., 2016). Some studies have shown that sympathetic nerves maintain an anti-inflammatory state in mice by inhibiting TNF-α level in macrophages (Tang et al., 2015). Additionally, NE can promote extracellular fatty acid uptake and storage as triglycerides and reduce free fatty acid release from triglyceride-laden macrophages (Petkevicius et al., 2021a). However, another study found that the sympathetic nervous system exerts an indirect effect on ATMs through the modulation of adipocyte function instead of modulating the phenotype of ATMs directly (Petkevicius et al., 2021b). Similar findings were also found for ILC2s, in which PDGFRA + adipose stem cell (ASC) could serve as a messenger between the immune cells and nervous system. The sympathetic nerve can act on ASCs via β2-AR to control the level of glial-derived neurotrophic factor and indirectly regulate the activity of adipose tissue ILC2s via the neurotrophic factor receptor RET (50). Likewise, β-adrenergic signaling was also reported to regulate the production of IL-33 by a DPP4+PDGFRB + ASC subpopulation and enhance ILC2 accumulation indirectly (Shan et al., 2021).
In the intestine, tissue-specific immune cells include muscularis macrophages (MMs) and ILC2s. By studying the transcriptional profile, gut-innervating sympathetic neurons have been found to polarize MMs towards a protectively M2 phenotype through β2-AR (52). In murine models of enteric infections, MMs upregulate a neuroprotective program via β2-AR and constrain neuronal death through an arginase 1-polyamine axis (Matheis et al., 2020). ILC2s participate in multiple intestinal physiological processes, including tissue repair, metabolic homeostasis, allergic inflammation and host defense against infections (Cardoso et al., 2017). Research has shown that β2-AR deficiency results in exaggerated ILC2 responses and type 2 inflammation in the intestine (Moriyama et al., 2018). Another study also demonstrated that sympathetic innervation constrains the effects of innate immune responses on microbes in the gut through the β2-AR pathway (Willemze et al., 2019). β2-AR has been proven to mediate negative regulation of ILC2s through the inhibition of cell proliferation and effector function (Moriyama et al., 2018). A recent study reported that colonic sympathetic nerves can also exert an indirect effect on immune cells through endothelial MAdCAM-1. In murine models, activation of local sympathetic nerves decelerated colitis and reduced the abundance of immune cell (Schiller et al., 2021). Hepatic invariant NKT (iNKT) cells are tissue-specific immune cells that primarily reside in the liver (Bendelac et al., 2007). One study showed that the immunosuppressive function of iNKT cells was mediated through NE (59).
In summary, adrenergic signaling regulates a variety of tissue-resident immune cells in the CNS and PNS in which plays an anti-inflammatory and immunosuppressive role (Figure 1, Table 1). Thus, adrenergic regulation is an important neuroendocrine modulating method for tissue-specific immunity.
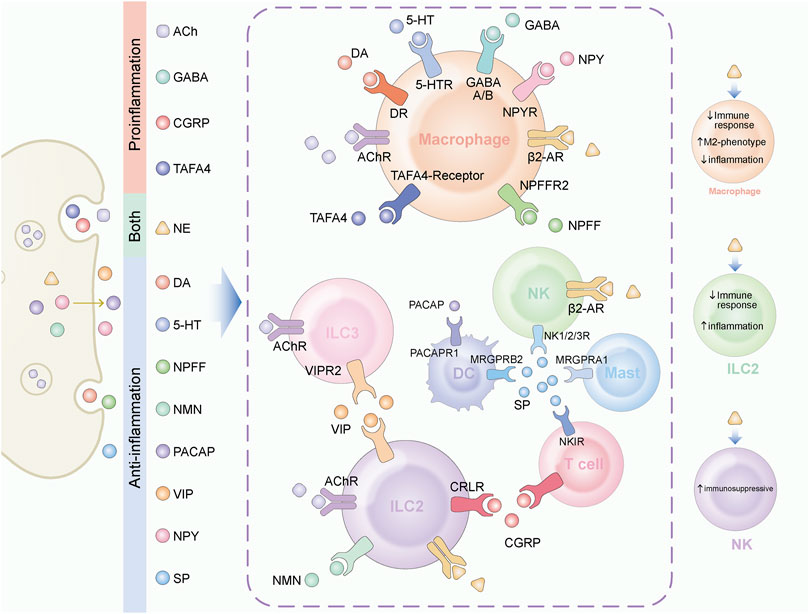
FIGURE 1. Neuroendocrine factors play various role in tissue immune regulation. A variety of neuroendocrine factors play regulatory roles in tissue-resident immune cells. The target cells and their receptors for different factors are displayed in the figure. On the left, neuroendocrine factors are classified according to their function. ACh, GABA, CGRP and TAFA4 mainly play a pro-inflammatory function. DA, 5-HT, NPFF, NMU, PACAP, VIP, NPY, and SP mainly play an anti-inflammatory function. NE plays different roles in different target cells. On the right side of the figure, we marked the functions of NE.
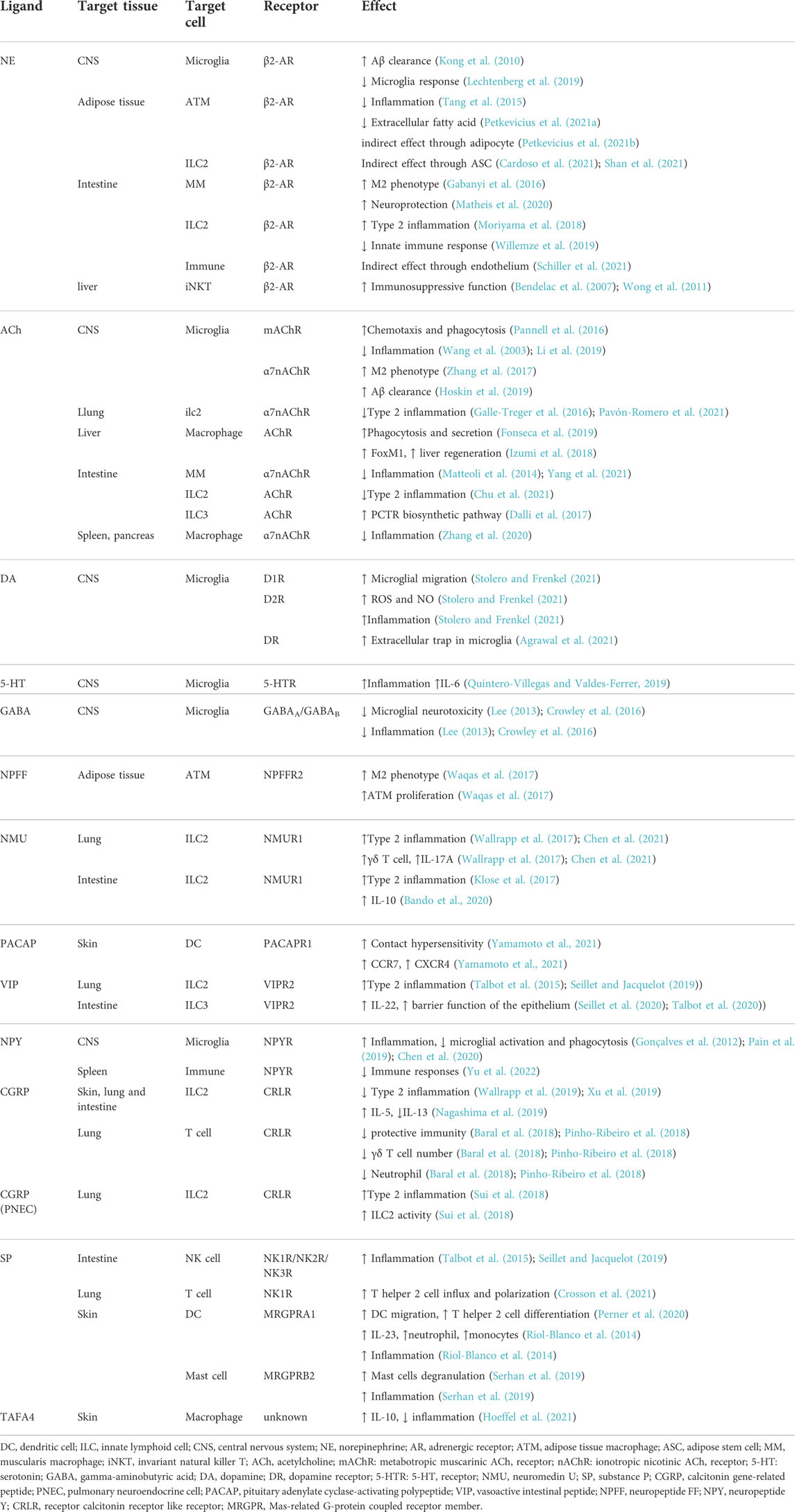
TABLE 1. Summary of central and peripheral neuroendocrine factors regulating tissue specific immunity.
2.2 Cholinergic regulation
The cholinergic system, which is found in both neuronal and nonneuronal cells, is a network that performs various complex functions in the body. It is composed of acetylcholine (ACh), cholinergic receptors (AChRs), acetylcholinesterase enzyme and choline acetyltransferase enzyme (Halder and Lal, 2021). ACh is the classical neurotransmitter in the cholinergic system. The receptor of ACh is divided into ionotropic nicotinic ACh receptors (nAChRs) and metabotropic muscarinic ACh receptors (mAChRs).
ACh affects the function of the CNS. AChRs are expressed on microglia in the CNS. mAChR stimulation modulates microglial chemotaxis and phagocytic activity via IFN-γ activation (Pannell et al., 2016). Regarding nAChRs, α7nAChR is an essential regulator of inflammation (Wang et al., 2003). The anti-inflammatory ACh response in microglia is mediated through α7nAChR. This response helps to lower inflammatory cytokine levels and microglial activation (Li et al., 2019). Similarly, ACh inhibits LPS-induced IL-1β and IL-6 elevation and promote IL-4 and IL-10 production through α7nAChRs to promote the M2 phenotype (Zhang et al., 2017). In addition, ACh stimulation of α7nAChR in microglia also enhances Aβ clearance (Hoskin et al., 2019).
Tissue-resident macrophages and ILCs are common tissue-resident immune cells in the periphery. They act in a tissue-specific manner in the liver, lung, gut, where ACh has been shown to modulate these cells via ACh-AChR signaling. In the lung, ILC2s are common resident immune cells. The ACh-α7nAChR axis in ILC2s decreases the synthesis of TNF-α, IL-1, and IL-6 (66). However, ILC2 transcription factor GATA-3 and the inflammatory modulator NF-κB is diminished. In general, ACh-α7nAChR signaling in ILC2s helps to promote anti-inflammatory function (Galle-Treger et al., 2016). In the liver, hepatic macrophages are the common resident immune cells. The vagus nerve regulates the secretory and phagocytic activity of resident macrophages via cholinergic signaling (Fonseca et al., 2019). ACh-AChR signal-mediated IL-6 production in hepatic macrophages upregulates the expression of FoxM1 in hepatocytes, leading to liver regeneration (Izumi et al., 2018). In the intestine, tissue-specific immune cells include MMs, ILC2s and ILC3s. The vagus nerve regulates the anti-inflammatory effect of intestinal MMs via α7nAChR-mediated JAK2/STAT3 signaling pathway (Matteoli et al., 2014; Yang et al., 2021). The ACh-AChR axis in ILC2s also causes anti-inflammatory effects by promoting ILC2 cytokine production (Chu et al., 2021). Moreover, AChs upregulate the PCTR biosynthetic pathway in ILC3s(Dalli et al., 2017). Besides, the vague nerve is also reported to modulate anti-inflammatory effect through the α7nAChR on macrophages in spleen and pancreas (Zhang et al., 2020).
In summary, cholinergic signaling facilitates the behavior of tissue-resident macrophages and promotes the anti-inflammatory function of ILC2s in the nervous system (Figure 1, Table 1). Thus, cholinergic regulation is another important neuroendocrine modulating method for tissue-specific immunity.
2.3 Regulation via other neurotransmitters
In addition to NE, epinephrine and ACh, neurotransmitters also include dopamine (DA), serotonin (5-HT) and gamma-aminobutyric acid (GABA). Research on the neuroendocrine regulation of immune cells by these other neurotransmitters has mainly focused on microglia. Microglia express several receptors for DA, 5-HT and GABA (Younger et al., 2019; Stolero and Frenkel, 2021).
DA is a neurotransmitter synthesized in both the CNS and PNS. It is mainly distributed in the CNS and has been detected in the liver, spleen, pancreas and gut (Peters et al., 2014; Klein et al., 2019). Dopamine receptors (DRs), including D1R, D2R, D3R, D4R, and D5R, are present on microglia and some other macrophages (Thomas Broome et al., 2020). DA promotes microglial migration, increases NO and ROS production through D1R, and the secretion of proinflammatory cytokines, such as IL-1β and IL-6 through D2R (75). Also, DA can induce the formation of DNA-based extracellular traps in microglia which participate in immunosurveillance and pathogen clearance (Agrawal et al., 2021) (Figure 1, Table 1).
5-HT, a biogenic amine synthesized from tryptophan, is a well-known neurotransmitter in the CNS which plays a critical roles in mood stability, sleep patterns and pain tolerance. The effects of 5-HT are regulated through 5-HT receptors. It also exist as an important signaling molecule in the periphery, especially in the gastrointestinal tract (Ye et al., 2021). 5-HT plays a role in CNS inflammation and repair. Administering 5-HT to microglia induces inflammatory initiation and IL-6 production (Quintero-Villegas and Valdés-Ferrer, 2019) (Figure 1, Table 1).
GABA is known as a main inhibitory amino acid neurotransmitter in the CNS. The physiological roles of GABA are related to the modulation of synaptic transmission. Two distinct classes of GABA receptors have been identified, including ionotropic receptor GABAA and metabotropic receptor GABAB (Ngo and Vo, 2019). Microglia are known to express both GABAA and GABAB. GABA has been shown to decrease microglial neurotoxicity and exert an anti-inflammatory effect (Lee, 2013; Crowley et al., 2016) (Figure 1, Table 1).
There are few studies on the role of these neurotransmitters in peripheral tissue-specific immunity. However, there is evidence for their presence in the periphery; for instance, the airway epithelium contains pulmonary neuroendocrine cells filled with 5-HT and GABA neuropeptides (Magnon, 2021). Some peripheral tissue-specific immune cells have been reported to express corresponding neurotransmitter receptors, providing the possibility for neuroendocrine interactions between them.
2.4 Regulation via neuropeptides
Neuropeptides are peptide substances produced by neurons that play key roles in modulating cell function by binding to specific receptors. Examples of neuropeptides include neuromedin U (NMU), neuropeptide calcitonin gene-related peptide (CGRP), substance P (SP), neuropeptide TAFA4, pituitary adenylate cyclase-activating polypeptide (PACAP), vasoactive intestinal peptide (VIP), neuropeptide FF (NPFF), neuropeptide Y (NPY) and somatostatin (SST). Neuropeptides and their receptors have regionally restricted distributions in the nervous system (Hoyer and Bartfai, 2012). Transcriptomic analyses have indicated that immune cells express numerous neuropeptide receptors (Seillet and Jacquelot, 2019). Thus, neuropeptides may play a vital role in mediating tissue-specific immunity.
2.4.1 Neuropeptides secreted by the central nervous system and autonomic nerves
NPFF is a CNS octapeptide that plays a part in pain modulation and opiate tolerance. NPFF has two receptors, NPFF receptor 1 and 2 (NPFFR1, NPFFR2) (Bonini et al., 2000). In peripheral tissues, only NPFFR2 is expressed in ATMs (Waqas et al., 2017). NPFF promotes M2 phenotype and increase the proliferation of ATMs. Specifically, NPFF suppressed the expression of the E3 ubiquitin ligase RNF128, which promote the stability of phosphorylated STAT6 and increased expression of gene related to M2 macrophage. NPFF induced ATM proliferation accompanied with the increase of NDRG2 expression and suppression of MAFB expression (Waqas et al., 2017) (Figure 1, Table 1).
NMU is a neuropeptide widely distributed in the human body and has two receptors, NMU receptor 1 and 2 (NMUR1, NMUR2). NMU mainly functions on ILC2s through NMUR1 (Martinez and O'Driscoll, 2015). NMU stimulates ILC2 activation and promotes type 2 cytokine responses that can induce antimicrobial and inflammatory responses (Klose et al., 2017). In the intestine, NMU increases IL-10 production in intestinal ILC2s. However, the function of ILC2-derived IL-10 in the intestine remains unknown (Bando et al., 2020). NMU can also amplify lung inflammation driven by ILC2s. IL-9 derived from ILC2 plays an important role in increasing the abundance of γδ T cell and IL-17A production (Wallrapp et al., 2017; Chen et al., 2021).
PACAP and VIP are two highly related neuropeptides widely distributed in organisms and have immunomodulatory actions. The receptors of PACAP and VIP are expressed by numerous immune cell types (Abad and Tan, 2018). The neuropeptide PACAP is an antimicrobial peptide induced in the brain in response to bacterial and fungal infection (Lee et al., 2021). PACAPR1 is a main receptor of PACAP expressed on DC. PACAP induces cutaneous DC functions and promotes the development of contact hypersensitivity through PACAPR1. The expression of CCR7 and CXCR4 of DCs was enhanced by PACAP in vitro (Yamamoto et al., 2021).
NPY is a neuropeptide widely distributed in the human body and is secreted mainly by the CNS and sympathetic nerves, where it is co-released with NE. NPY exerts its effects through interacting with NPY receptors (NPYRs), among which NPY1R is the most abundantly expressed NPYR receptor in immune cells (Dimitrijevic and Stanojevic, 2013). NPY inhibits microglial activation and phagocytosis and affects the direction of migration and phagocytosis. NPY also inhibits cytokine secretion of microglia, especially the secretion of proinflammatory factors (Gonçalves et al., 2012; Pain et al., 2019; Chen et al., 2020). Besides, NPY was reported to attenuate the splenic immune response as well (Yu et al., 2022) (Figure 1, Table 1).
Regarding VIP, intestinal ILC3s and pulmonary ILC2s highly express VIP receptor 2 (VIPR2). In the intestine, VIP activates ILC3s, enhancing the production of IL-22 and the barrier function of the epithelium (Seillet et al., 2020). This dynamic neuroimmune circuit in the intestine is regulated by feeding and circadian mechanisms (Talbot et al., 2020) (Figure 1, Table 1).
SST is a neuropeptide with generally inhibitory function which commonly produced by endocrine cells and the CNS(Weckbecker et al., 2003). SST mediates its biological functions via SST receptors (subtype 1–5), which was found expressing in multiple immune cells (Møller et al., 2003). However, existing studies have shown that SST only affects immune cells in peripheral blood of normal tissues. For instance, SST reduced the secretion of INF-γ from peripheral blood mononuclear cell and inhibit the production of immunoglobulin by B lymphocytes. Also, the chemotaxis of peripheral blood monocytes is inhibited by this peptide (Pintér et al., 2006).
2.4.2 Neuropeptides secreted by sensory nerves
Nociceptor sensory neurons are responsible for the detection of potentially damaging stimuli and elicit defensive behaviors (Chiu et al., 2013). The neuropeptides CGRP and SP are nociceptor mediators that act in the skin, lung and intestine. TAFA4 is a neuropeptide secreted by sensory neurons in skin. VIP mentioned above can also be secreted by nociceptors. Tissue-resident macrophages, ILC2s, NK cells, DCs and γδ T cells are common tissue-resident immune cells reside within epithelial layers of the lung, skin, and gut (Zheng et al., 2013).
CGRP regulates a variety of immune cells which express the receptor, calcitonin receptor-like receptor (CRLR). Broadly speaking, CGRP is a negative regulator of ILC2 responses, which is necessary for suppressing ILC2 expansion and maintaining homeostasis of the type 2 immune machinery (Wallrapp et al., 2019; Xu et al., 2019). Additionally, CGRP in concert with NMU is reported to promote IL-5 but constrain IL-13 expression (Nagashima et al., 2019). In the lung, nociceptors suppress protective immunity through the release of CGRP. Specifically, CGRP suppressed the recruitment and surveillance of neutrophils (Pinho-Ribeiro et al., 2018), and reduced lung γδ T cell numbers, which act as first responders to infection (Baral et al., 2018). On the contrary, pulmonary neuroendocrine cells (PNECs) are a kind of rare airway epithelial cells that reside near airway branches. CGRP secreted by PNECs enhances ILC2 activity and the production of cytokines (Sui et al., 2018).
In the intestine, precursor and immature NK cells exhibit tissue-resident signatures (Dogra et al., 2020). SP is released from nociceptors after stimulation through the neurokinin receptors NK1R, NK2R and NK3R and has been shown to regulate cell migration and proliferation to generate neurogenic inflammation (Seillet and Jacquelot, 2019). In the airways, lung nociceptor neurons release SP, and amplify T helper 2 cell influx and polarization via NK1R (Crosson et al., 2021). In skin, SP interacts with DCs and mast cells through the Mas-related G-protein coupled receptor member (MRGPR). Sensory neurons release SP, which acts through MRGPRA1 to induce CD301b+ DC migration to the draining lymph node (dLN). Migrated DCs initiate T helper-2 cell differentiation in the dLNs(Perner et al., 2020). One study reported that nociceptors induce the recruitment of neutrophils and monocytes, driving skin inflammation. Nociceptors promote the production of IL-23 by dermal DCs. IL-23 then acts on IL-23R+ γδT17 cells to promote the secretion of IL-17F and IL-22 (119). Nociceptors can also amplify skin inflammation by inducing the degranulation of mast cells that are contiguous to the nociceptors and activating MRGPRB2 on the mast cells (Serhan et al., 2019).
TAFA4 is a neuropeptide secreted by sensory neurons in skin. TAFA4 reduce inflammation and cell infiltration by inducing IL-10 production from Tim4+ dermal macrophages (Flayer and Sokol, 2021; Soler Palacios and Gutiérrez‐González, 2022). TAFA4 can also affect the inflammatory mechanisms of other macrophage subsets, including CD206+ dermal macrophages and peritoneal macrophages (Hoeffel et al., 2021). In the lung, nociceptors can induce the production of VIP which in turn stimulates ILC2s to promote the occurrence of inflammation (Talbot et al., 2015; Seillet and Jacquelot, 2019).
In a word, sensory nerves function primarily in their widely distributed areas including skin, lung and gut. Neuropeptides secreted by sensory neurons play diverse roles in immune responses against various pathogens (Figure 1, Table 1).
3 Tissue-specific immune effects on nerves
Tissue-specific immune cells regulate nerves by releasing various factors such as cytokines, chemokines, small-molecule peptides and neurotrophic factors.
Among the tissue-specific immune cells, tissue-resident macrophages exhibit a rich regulatory role in different tissues. In adipose tissue, ATM expresses neurotrophic factors to promote white adipose tissue innervation (Xie et al., 2022). Also, ATM reportedly controls brown adipose tissue innervation (Wolf et al., 2017). In subcutaneous fat, a new subset of immune cells called cholinergic adipose macrophages (ChAMs) has been identified. ChAMs secrete ACh to regulate thermogenic activation via β2-AR (Knights et al., 2021). ATM can also mediate sympathetic neurons through ROBO1 receptor. Slit3 is a macrophage cytokine secreted by ATM. It binds to the ROBO1 receptor to stimulate Ca2+/calmodulin-dependent protein kinase II signaling and NE release, which enhances adipocyte thermogenesis (Wang et al., 2021). Additionally, MMs regulate peristaltic activity of the colon by secreting bone morphogenetic protein 2 (BMP2), which activates the expression of BMP receptor on enteric neurons. Enteric neurons, in turn, secrete a growth factor required for macrophage development called colony stimulatory factor 1 (CSF1), (Muller et al., 2014). Sympathetic neuron-associated macrophages are another previously undescribed population of resident macrophages that mediate NE clearance via SLC6A2 and MAOA expression (Pirzgalska et al., 2017). A nerve- and airway-associated macrophage (NAM) subset has been identified in the lung. Their closely associated nerves have sympathetic fibers. However, the relationship between NAMs and their associated nerves is unclear (Ural et al., 2020).
In the CNS, microglia perform negative feedback control of neuronal activity. The suppression of neuronal activation depends on the ability to sense and catabolize extracellular ATP of microglia (Badimon et al., 2020). ILC2s also play a part. In the lung, nociceptors can sense IL-5 released by activated immune cells, and IL-5 further induces VIP production. VIP then stimulates ILC2s, creating an inflammatory signaling loop that promotes allergic inflammation (Talbot et al., 2015; Seillet and Jacquelot, 2019). ACh is a broadly distributed signaling molecule that is not only produced by neurons, but also by numerous immune cells. Several immune cell types respond to ACh signaling and can also produce ACh directly (Cox et al., 2020).
4 Neuroendocrine tissue-specific immunity axis in cancer
It is generally accepted that tumor innervation correlates with tumor progression across multiple solid tumor types (Albo et al., 2011; Huang et al., 2014; Kappos et al., 2018; Renz et al., 2018). Specifically, autonomic nervous infiltration was discovered to affect the development and dissemination of breast cancer, head and neck carcinoma, prostate cancer, liver cancer, lung cancer, pancreatic cancer, cholangiocarcinoma, colorectal cancer, ovarian cancer, glioma and gastric cancer (Magnon et al., 2013; Zhao et al., 2014; Partecke et al., 2016; Pon et al., 2016; Zahalka et al., 2017; Faulkner et al., 2019; Sha et al., 2019). While sensory neurons were found to play a part in tumor development of melanoma and pancreatic cancer (Saloman et al., 2016; Prazeres et al., 2020). Meanwhile, high intra-tumoral nerve density is associated with poor prognosis and high recurrence in multiple cancers (Silverman et al., 2021). Studies have shown that the mechanism by which tumor innervation affects cancer can be divided into three parts: direct stimulation of tumor proliferation, promotion of vascularization, and indirect modulation of tumors through immune changes. In this context, we discuss the effect of neuroendocrine regulation on tissue-specific immunity in the TME.
In breast cancer, sympathetic innervation accelerates tumor progression, while parasympathetic innervation decelerates tumor progression in the TME (26). The sympathetic nervous system affects stress-induced cancer behaviors, including initiation, progression and metastasis, by modulating tumor-associated immune cells via the NE-βAR signaling pathway in four ways. First, β-adrenergic signaling represses an effector phenotype of CD8+ T cells in the TME. Reducing β-adrenergic signaling induced an immunologically active TME in tumor-bearing animal models (Bucsek et al., 2017). Second, parasympathetic innervation constrains the function of immune checkpoints. Sympathetic nerve denervation and parasympathetic neurostimulation reduced the level of PD-1, PD-L1, and FOXP3 on CD4+ or CD8+ T cells in murine models of breast cancer. This effect was also proved in human breast cancer samples (Kamiya et al., 2019). Next, the NE-βAR signaling pathway increased the infiltration of macrophages in the TME and induced the differentiation to a prometastatic M2 macrophage phenotype. Stress-induced NE-βAR signaling activation induced a metastasis to distant tissues in mouse breast cancer models (Sloan et al., 2010). Finally, NE-βAR signaling pathway promotes breast cancer metastasis by recruiting myeloid-derived suppressor cells (MDSCs). MDSCs are a population of cells with immunosuppressive phenotype. In murine models of breast cancer, chronic stress leaded to the elevation of MDSCs, accelerated breast cancer metastasis, and upregulated IL-6 expression and JAK/STAT3 signaling pathways (an et al., 2021). Another study reveals that the expression level of β2-AR on MDSCs increases with tumor growth. β2-adrenergic signaling increases oxidative phosphorylation, increase fatty acid oxidation, decreases glycolysis, and also increases autophagy and activates the arachidonic acid cycle (Mohammadpour et al., 2021). A recent study revealed that in brain metastases from breast cancer, neuronal exposure induces synaptic mediators and neurotransmitter signaling in tumors (Deshpande et al., 2022). This research suggests that neuroendocrine regulation in brain metastases seems to be more direct, and whether immune cells in brain metastases TME are regulated by neuroendocrine factors might be the direction of future research.
Likewise, in hepatocellular carcinoma, β-adrenergic signaling promotes tumor growth by mobilizing MDSCs to tumor tissues. In the hepatocellular carcinoma mouse models, stress enhanced tumor progression. Specifically, β-adrenergic signaling changed the spleen structure, and caused a redistribution of MDSCs to tumors. Also, the author found that splenectomy could constrain tumor growth and prevent increasement of macrophages in tumor tissues in stressed mice (Jiang et al., 2019). Another study pointed out the possible mechanism of this mobilization. The recruitment of MDSCs was modulated through β-adrenergic-activated CXCL5-CXCR2-Erk signaling cascades. Chronic stress upregulated the expression of CXCR2 and pErk1/2 in MDSCs, and the expression of CXCL5. In vitro, T-cell proliferation was obviously constrained in NE treated medium. In vivo, β-adrenergic blockade reversed the acceleration of tumor growth induced by chronic stress and CXCL5-CXCR2-Erk signaling pathway was suppressed (Cao et al., 2021).
Moreover, in prostate cancer, the density of sympathetic fibers in tumors is associated with poor clinical outcomes (Magnon et al., 2013). It was observed that prostate cancer patients with depression showed higher tumor-associated macrophage infiltration. NPY is co-released with NE in sympathetic nerves. Animal experiments revealed that NPY released from NE-treated prostate cancer cells promotes macrophage trafficking and IL-6 release, which subsequently activates the STAT3 signaling pathway (Cheng et al., 2019). Analyses of clinical prostate cancer samples have also suggested that elevated NPY promotes prostate cancer development and is associated with poor prognosis and therapy resistance (Rasiah et al., 2006; Ding et al., 2021). Additionally, NK cells are mobilized by epinephrine through the β-adrenergic signaling pathway, which can reduce the tumor initiation and recurrence. In mouse tumor models, epinephrine induced selective mobilization of IL-6-sensitive NK cells while IL-6 inhibited the infiltration and activation of NK cells in TME (Pedersen et al., 2016).
Yet, different perspectives have been raised in pancreatic cancer. Chronic stress is observed to increase pancreatic cancer progression and can be antagonized by β-AR blockade in some studies (Partecke et al., 2016; Renz et al., 2018). However, a recently published study demonstrated that sympathetic nerves ablation increases tumor growth and spread by increasing intra-tumoral CD163+ macrophage numbers. In the mouse model of pancreatic cancer, the sympathetic nerves exert a protective function during the early stage of tumor. The author mentioned that the converse response to sympathectomy in pancreatic cancer may be shaped by the particular TME (Guillot et al., 2022). Otherwise, ablation of sensory neurons of pancreatic cancer reportedly slows initiation and progression of cancer. However, the study did not mention its impact on tumor immunity (Saloman et al., 2016).
Sensory innervation was reported to constrain melanoma progression. Ablation of sensory nerves lead to worse outcomes in melanoma-bearing mice (Prazeres et al., 2020). In a recent study, Costa et al. (2021) informs that sensory neuron activity may slow the melanoma progression by inducing tumor immunosurveillance. Specifically, in sensory neuron-overactivated melanoma mice, the number of MDSCs and neutrophils significantly decreased, while tumor-infiltrating DCs, CD8 + T cells, CD4 + T cells, γδ T cells and NK cell was detected to increase. The expression of immune checkpoint molecules such as PD-1 and CTLA-4 decreased. And it induces a Th17-immune response in the melanoma microenvironment. Furthermore, the author validated these results in a large human melanoma cohort. They found that SCN10A (encoding Nav1.8), a key gene of sensory neurons, has higher expression in patients with better prognosis. The enrichment of tumor-infiltrating immune cells showed an increase of DCs, CD8 + T cells, CD4 + T cells and NK cell in patients with better prognosis. Conversely, an earlier published study by Keskinov et al. (2016) reported that the dorsal root ganglia (DRG) of sensory nerves contribute to melanoma progression by recruiting MDSCs which help to create an oncogenic TME. Experimental results suggested that, in vitro, melanoma cells can activate DRG neurons and increase the expression of chemokines that attract MDSC. In vivo, in the presence of DRG cells, tumor growth was accelerated and the number of MDSC increased. To sum up, for sensory neuron modulation, Costa et al. (2021) used a designer drug to selectively activate or inhibit sensory neurons within the tumor in vivo, whereas Keskinov et al. (2016) used DRG cells injection in mice. Besides, Costa et al. (2021) focused on multiple kinds of cells involved in tumor immunity including T cells, DCs, and MDSCs, while Keskinov et al. (2016) focused only on the alteration of MDSCs. Furthermore, Costa et al. (2021) proved their findings through in vivo assays and bioinformatic validations, while Keskinov et al. (2016) validated through in vitro and in vivo assays. In our opinion, there are several possible reasons for the opposite results produced by the two studies. First, the nerve fibers at work are different. It speculates that it is inappropriate to choose DRG cells as a representation of sensory neurons. The DRG is an enlargement of the dorsal root that houses somata of sensory neurons. But DRGs can also receive signals from the autonomic nervous system by connecting to the sympathetic nerve via rami communicantes nerves (Esposito et al., 2019). Consequently, the tumor-promoting effects of DRGs mentioned in the study of Keskinov et al. (2016) is likely to be partially mediated by the autonomic nerves. The oncogenic role of the autonomic nervous system in multiple cancers has been mentioned above. Apart from that, the neuroendocrine factors at work are different. Signals from sensory neurons in normal tissues can be transmitted to immune cells through various factors such as CGRP, SP, and VIP. But neither study involved exploration on factors that signal between neurons and immune cells. Although Costa et al. (2021) observed an elevation of CGRP level after sensory nerve activation, this mechanism has not been confirmed. Whether sensory nerves also interact with immune cells through these substances in the melanoma microenvironment might be a direction of future research. We believe that further explorations of neuroendocrine factors involved in immune regulation in melanoma may answer the reasons for the opposite conclusions of the two studies above.
An analysis of clinical samples reveals that GABA is associated with poor prognosis in lung cancer and colon adenocarcinoma (Huang et al., 2022). GABA is reported to have an anti-tumor immunity function through two different pathways. One study informed GABA promotes the differentiation from monocyte to anti-inflammatory macrophages that secrete IL-10. GABA also inhibit CD8+ T cell killer function (Zhang et al., 2021). Another study suggested GABA activates the GABAB receptor to stimulate tumor cell proliferation and suppress CD8+ T cell infiltration in TME. In mouse models, targeting GABAB overcomed resistance to anti-PD-1 immune checkpoint blockade therapy (Huang et al., 2022).
Regarding neuropeptides, a systematic review found evidence that the elevation of SP and NK-1R are oncogenic events in head and neck carcinogenesis and probably act in the early stages of tumorigenesis (Gonzalez-Moles et al., 2021). Proline rich polypeptide 1, (PRP-1) is a neuropeptide secreted by the brain neurosecretory cells which causes inhibition of chondrosarcoma cell growth (Galoyan, 2000; Galoian et al., 2009). A study proves that PRP-1 functions via toll like receptor family TLR1/2, TLR6 and mucin MUC5B which are responsible for innate immunity pattern recognition. This result suggests that the anti-tumor effect of PRP-1 might be related to immune cells in TME. But the author also suggested that the receptors predominantly located in the tumor nucleus (Galoian et al., 2018). Another research demonstrated that PRP-1 can also inhibit cancer stem cell proliferation in chondrosarcoma (Granger et al., 2020). In addition, somatostatin analog (SSA) is the analog of SST which is now widely used in the treatment of neuroendocrine neoplasms. Long-acting repeatable of SSA decrease the level of total regulatory T cells and MDSCs, as well as the expression of PD1, CTLA4 and ENTPD1 in neuroendocrine neoplasms which exert an anti-tumor immunosurveillance function (von Arx et al., 2020). SSA was also reported to decrease cell proliferation and tumor growth in multiple cancers, such as lung cancer, breast cancer, colon carcinoma and endometrial carcinomas (Kahán et al., 1999; Engel et al., 2005; Treszl et al., 2009; Hohla et al., 2010). However, if these oncogenic effects are associated with tumor-associated immune cells has not yet been discussed. The function of neuropeptides in tumor-specific immunity requires further research.
In brief, under the regulation of neuroendocrine factors, some components of TME play the role of immunosurveillance, such as effective T cells, NK cells, and the immune checkpoint molecules produced by them. Yet some other components play the opposite pro-tumor effects including MDSCs, regulatory T cells and tumor-associated macrophages. Immunosurveillance effects are negatively regulated by β-adrenergic signaling in breast, prostate and liver cancers. In lung and colon cancer, immunosurveillance is negative regulated by sensory nerves. Pancreatic cancer immunosurveillance is positive regulated by β-adrenergic signaling, however negative regulated by sensory nerves. Melanoma is mainly mediated by sensory nerves, but the direction of regulation is controversial. Lastly, immunosurveillance in neuroendocrine neoplasm is positive regulated by SST.
From the perspective of therapy, there are also many researches emerged in recent years. Since tumor innervation plays an active role in cancer initiation and progression, neuron ablation emerged as a treatment strategy mentioned a lot. Some studies demonstrate that surgical or pharmacological denervation attenuate tumor growth in a tissue-specific manner. For instance, sensory neuron ablation is proved to favor melanoma progression, but slows the initiation and progression of pancreatic cancer (Saloman et al., 2016; Prazeres et al., 2020). And denervation suppresses gastric and breast tumorigenesis (Zhao et al., 2014). Although method to manoeuvre intra-tumoral innervations is not yet mature for clinical treatment, it provides a promising new avenue for anti-cancer therapy (Prazeres et al., 2020).
Apart from neuron ablation, antagonize β2-AR seems to be a more mature approach to block the neuroendocrine signal. Propranolol is a nonselective beta blocker used for cardiovascular indications for decades (Srinivasan, 2019). However, in recent years, propranolol has been applicated in the treatment of some kinds of tumors successfully, such as angiosarcoma (Wagner et al., 2018). Retrospective studies have observed a correlation between β blocker usage and increased overall survival among cancer patients (Nagaraja et al., 2013). Blockade of β-AR reduces tumor progression and upregulates the response to anti-CTLA4 therapy contributes to the formation of an immunosuppressive TME. In mouse fibrosarcoma models, propranolol increased the number of T cells, reduced the number of intra-tumoral MDSCs and altered the gene expression profile of tumor-associated macrophages significantly in the TME. Similar phenomenon was observed in murine models of colon cancer (Fjæstad et al., 2022). Specifically, one study demonstrates that NE-βAR pathway helps to maintain the level and the suppressive function of MDSCs. In murine breast cancer models, β2-adrenergic signaling modulated the expression of immunosuppressive molecules such as arginase-I and PD-L1 and suppressed the proliferation of T cells. Also, the author pointed out that the regulatory functions of β2-AR signaling in MDSCs is activated by STAT3 phosphorylation. The β2-AR-mediated increase in MDSC is dependent on Fas-FasL interactions. Besides, the immunosuppressive function of MDSCs can be decelerated by β-AR antagonists (Mohammadpour et al., 2019). Propranolol was also reported to reduce stress-induced elevation of regulatory T cells in breast cancer patients (Zhou et al., 2016). Another study report that β2-adrenergic signaling contributes to an exhausted phenotype in T cells and induce metabolic dysfunction in the TME. In mouse melanoma and colon cancer models, it is observed that by using propranolol, tumor growth rate was slowed accompanied by a significantly decrease of tumor-infiltrating T cells that express exhaustion related genes and an increase in progenitor exhausted T cells. Also, β-AR blockade in mice increases oxidative phosphorylation and glycolysis in tumor-infiltrating lymphocyte (Qiao et al., 2021). Therefore, β-AR antagonists such as propranolol could be a potentially efficacious therapy approach of tumors in the future.
In traditional Chinese medicine, moxibustion, a treatment modality with a long history, has been used to treat cancer-related symptoms in clinical practice for years. However, few people have explored its mechanism of action. Interestingly, a recent study informed that grain-sized moxibustion significantly reduced tumor growth in lung cancer. Grain-sized moxibustion performed at the acupoint of Zusanli promotes anti-tumor immunity of NK cell by inhibiting adrenergic signaling. The acupoint of Zusanli is an important acupoint involved in the interaction between neuroendocrine systems and immune cells (Zhang et al., 2018). In vitro, grain-sized moxibustion increased the proportion, infiltration and activation of NK cells, yet it didn’t affect T cells. Additionally, the grain-sized moxibustion mediated NK cells activation can be reduced by β-blocker treatment (Hu et al., 2021). To sum up, we describe the role of moxibustion in neuroendocrine regulation of lung cancer, which indicated that traditional Chinese medicine is still an ancient but promising therapeutic regimen for cancers.
5 Conclusion
Many studies have shown that tumor innervation is associated with tumor initiation, progression and a worse prognosis, and neuroendocrine regulation is the main mechanism by which nerves modulate tumor cells and other non-nerve cells. Several studies have focused on the neuroendocrine regulation of tumor cells; however, immune cells are also a vital part of the TME. Various tumor-associated immune cells have complex functions that can greatly impact tumor development, prognosis and treatment. Therefore, this review discusses the existing research on the neuroendocrine regulation of tissue-specific immunity. The regulatory mechanisms of different immune cell types in various tissues were summarized according to the classification of regulatory factors. Adrenergic and cholinergic signaling are two common regulatory pathways. In central and peripheral tissues, sympathetic nerves mainly modulate tissue-resident immune cells through the NE-β2A pathway, exerting anti-inflammatory and immunosuppressive roles. The parasympathetic nervous system regulates resident macrophages and ILCs mainly by secreting ACh from the vagus nerve to promote macrophage activity and anti-inflammatory effects. Other neurotransmitters are secreted primarily in the CNS and regulate microglia activity. Neuropeptides are another type of neuroendocrine factor that is secreted by central, autonomic and sensory neurons. These neuropeptides have various functions in tissues. Furthermore, many tissue-resident immune cells secrete cytokines and other factors to regulate nerves. We also summarized the available studies on neuroendocrine immunomodulatory regulation in different kinds of tumors. Some studies focused on autonomic nervous regulation in cancers and have helped to explain why chronic stress promotes tumor development from an immune aspect. Yet, many tumors are also regulated by sensory nerves and other factors. After that we discuss the neuroendocrine regulation of tumor immunity from the perspective of immune cell function and tumor therapy.
There are still knowledge gaps in the field of neuroendocrine regulation of immune cells in the TME. Many receptors of neuroendocrine factors are expressed on macrophages, T cells, and other cells that play important roles in tumor immunity. Some regulatory pathways have been studied in nontumor tissues, while their roles in tumors have not been studied. Moreover, a variety of cancers have been reported to be affected by tumor innervation, but the neuron-immune interactions in most cancers remain unclear. Thus, we also covered some articles that only discussed neuroendocrine regulation from the perspective of TME. Although they did not mention the neuroendocrine regulation of immune cells, we believe that some of them can provide ideas for the following research.
Due to the tissue specificity of immune cells, individualized immunotherapy in tumors may bring greater benefits to patients. For example, some studies have found that β2-AR blockade can inhibit NE-mediated tumor progression, but it has not been applied clinically in most kinds of tumors. Therefore, enriching the research focusing on these aspects may provide new insights for personalized tumor immunotherapy.
Author contributions
QW, BL, and S-QL are responsible for collecting and collating documents. S-QL and BL are responsible for writing this review, while QW and S-RS are responsible for the revision, and J-JL and SS are responsible for editing and submission. All authors read and approved the final manuscript.
Funding
This work was supported by the Fundamental Research Funds for the Central Universities (Grant No. 2042021kf0102) to BL and (Grant No. 2042021kf0083) to QW. This work was also supported by a National Natural Science Foundation of China (NSFC) grant (Grant NO: 81903166) to SS.
Acknowledgments
We thank a professional language editing by American Journal Experts.
Conflict of interest
The authors declare that the research was conducted in the absence of any commercial or financial relationships that could be construed as a potential conflict of interest.
Publisher’s note
All claims expressed in this article are solely those of the authors and do not necessarily represent those of their affiliated organizations, or those of the publisher, the editors and the reviewers. Any product that may be evaluated in this article, or claim that may be made by its manufacturer, is not guaranteed or endorsed by the publisher.
References
Abad, C., and Tan, Y. V. Immunomodulatory roles of PACAP and VIP: Lessons from knockout mice. J. Mol. Neurosci. 2018;66(1):102–113.doi:10.1007/s12031-018-1150-y
Agrawal, I., Sharma, N., Saxena, S., Arvind, S., Chakraborty, D., Chakraborty, D. B., et al. Dopamine induces functional extracellular traps in microglia. iScience. 2021;24(1):101968.doi:10.1016/j.isci.2020.101968
Albo, D., Akay, C. L., Marshall, C. L., Wilks, J. A., Verstovsek, G., Liu, H., et al. Neurogenesis in colorectal cancer is a marker of aggressive tumor behavior and poor outcomes. Cancer. 2011;117(21):4834–4845.doi:10.1002/cncr.26117
Aloe, L., Rocco, M. L., Balzamino, B. O., and Micera, A. Nerve growth factor: Role in growth, differentiation and controlling cancer cell development. J. Exp. Clin. Cancer Res. 2016;35(1):116.doi:10.1186/s13046-016-0395-y
an, J., Feng, L., Ren, J., Li, Y., Li, G., Liu, C., et al. Chronic stress promotes breast carcinoma metastasis by accumulating myeloid-derived suppressor cells through activating β-adrenergic signaling. Oncoimmunology. 2021;10(1):2004659.doi:10.1080/2162402x.2021.2004659
Badimon, A., Strasburger, H. J., Ayata, P., Chen, X., Nair, A., Ikegami, A., et al. Negative feedback control of neuronal activity by microglia. Nature. 2020;586(7829):417–423.doi:10.1038/s41586-020-2777-8
Bando, J. K., Gilfillan, S., Di Luccia, B., Fachi, J. L., Sécca, C., Cella, M., et al. ILC2s are the predominant source of intestinal ILC-derived IL-10. J. Exp. Med. 2020;217(2).doi:10.1084/jem.20191520
Baral, P., Umans, B. D., Li, L., Wallrapp, A., Bist, M., Kirschbaum, T., et al. Nociceptor sensory neurons suppress neutrophil and γδ T cell responses in bacterial lung infections and lethal pneumonia. Nat. Med. 2018;24(4):417–426.doi:10.1038/nm.4501
Bejarano, L., Jordāo, M. J. C., and Joyce, J. A. Therapeutic targeting of the tumor microenvironment. Cancer Discov. 2021;11(4):933–959.doi:10.1158/2159-8290.cd-20-1808
Bendelac, A., Savage, P. B., and Teyton, L. The biology of NKT cells. Annu. Rev. Immunol. 2007;25:297–336.doi:10.1146/annurev.immunol.25.022106.141711
Bonini, J. A., Jones, K. A., Adham, N., Forray, C., Artymyshyn, R., Durkin, M. M., et al. Identification and characterization of two G protein-coupled receptors for neuropeptide FF. J. Biol. Chem. 2000;275(50):39324–39331.doi:10.1074/jbc.m004385200
Bucsek, M. J., Qiao, G., MacDonald, C. R., Giridharan, T., Evans, L., Niedzwecki, B., et al. β-Adrenergic signaling in mice housed at standard temperatures suppresses an effector phenotype in CD8+ T cells and undermines checkpoint inhibitor therapy. Cancer Res. 2017;77(20):5639–5651.doi:10.1158/0008-5472.can-17-0546
Campos, X., Muñoz, Y., Selman, A., Yazigi, R., Moyano, L., Weinstein-Oppenheimer, C., et al. Nerve growth factor and its high-affinity receptor trkA participate in the control of vascular endothelial growth factor expression in epithelial ovarian cancer. Gynecol. Oncol. 2007;104(1):168–175.doi:10.1016/j.ygyno.2006.07.007
Cao, M., Huang, W., Chen, Y., Li, G., Liu, N., Wu, Y., et al. Chronic restraint stress promotes the mobilization and recruitment of myeloid‐derived suppressor cells through β‐adrenergic‐activated CXCL5‐CXCR2‐Erk signaling cascades. Intl J. Cancer. 2021;149(2):460–472.doi:10.1002/ijc.33552
Cardoso, F., Klein Wolterink, R. G. J., Godinho-Silva, C., Domingues, R. G., Ribeiro, H., da Silva, J. A., et al. Neuro-mesenchymal units control ILC2 and obesity via a brain-adipose circuit. Nature. 2021;597(7876):410–414.doi:10.1038/s41586-021-03830-7
Cardoso, V., Chesné, J., Ribeiro, H., García-Cassani, B., Carvalho, T., Bouchery, T., et al. Neuronal regulation of type 2 innate lymphoid cells via neuromedin U. Nature. 2017;549(7671):277–281.doi:10.1038/nature23469
Chen, W., Lai, D., Li, Y., Wang, X., Pan, Y., Fang, X., et al. Neuronal-activated ILC2s promote IL-17a production in lung γδ T cells during sepsis. Front. Immunol. 2021;12:670676.doi:10.3389/fimmu.2021.670676
Chen, W. C., Liu, Y. B., Liu, W. F., Zhou, Y. Y., He, H. F., and Lin, S. Neuropeptide Y is an immunomodulatory factor: Direct and indirect. Front. Immunol. 2020;11:580378.doi:10.3389/fimmu.2020.580378
Cheng, Y., Tang, X. Y., Li, Y. X., Zhao, D. D., Cao, Q. H., Wu, H. X., et al. Depression-induced neuropeptide Y secretion promotes prostate cancer growth by recruiting myeloid cells. Clin. Cancer Res. 2019;25(8):2621–2632.doi:10.1158/1078-0432.ccr-18-2912
Chiu, I. M., Heesters, B. A., Ghasemlou, N., Von Hehn, C. A., Zhao, F., Tran, J., et al. Bacteria activate sensory neurons that modulate pain and inflammation. Nature. 2013;501(7465):52–57.doi:10.1038/nature12479
Chu, C., Parkhurst, C. N., Zhang, W., Zhou, L., Yano, H., Arifuzzaman, M., et al. The ChAT-acetylcholine pathway promotes group 2 innate lymphoid cell responses and anti-helminth immunity. Sci. Immunol. 2021;6(57).doi:10.1126/sciimmunol.abe3218
Cording, S., Medvedovic, J., Aychek, T., and Eberl, G. Innate lymphoid cells in defense, immunopathology and immunotherapy. Nat. Immunol. 2016;17(7):755–757.doi:10.1038/ni.3448
Costa, P. A. C., Silva, W. N., Prazeres, P., Picoli, C. C., Guardia, G. D. A., Costa, A. C., et al. Chemogenetic modulation of sensory neurons reveals their regulating role in melanoma progression. Acta Neuropathol. Commun. 2021;9(1):183.doi:10.1186/s40478-021-01273-9
Cox, M. A., Bassi, C., Saunders, M. E., Nechanitzky, R., Morgado‐Palacin, I., Zheng, C., et al. Beyond neurotransmission: Acetylcholine in immunity and inflammation. J. Intern Med. 2020;287(2):120–133.doi:10.1111/joim.13006
Crosson, T., Wang, J. C., Doyle, B., Merrison, H., Balood, M., Parrin, A., et al. FcεR1-expressing nociceptors trigger allergic airway inflammation. J. Allergy Clin. Immunol. 2021;147(6):2330–2342.doi:10.1016/j.jaci.2020.12.644
Crowley, T., Cryan, J. F., Downer, E. J., and O’Leary, . Inhibiting neuroinflammation: The role and therapeutic potential of GABA in neuro-immune interactions. Brain, Behav. Immun. 2016;54:260–277.doi:10.1016/j.bbi.2016.02.001
Dalli, J., Colas, R. A., Arnardottir, H., and Serhan, C. N. Vagal regulation of group 3 innate lymphoid cells and the immunoresolvent PCTR1 controls infection resolution. Immunity. 2017;46(1):92–105.doi:10.1016/j.immuni.2016.12.009
Deshpande, K., Martirosian, V., Nakamura, B. N., Iyer, M., Julian, A., Eisenbarth, R., et al. Neuronal exposure induces neurotransmitter signaling and synaptic mediators in tumors early in brain metastasis. Neuro Oncol. 2022;24(6):914–924.doi:10.1093/neuonc/noab290
Dhabhar, F. S. Effects of stress on immune function: The good, the bad, and the beautiful. Immunol. Res. 2014;58(2-3):193–210.doi:10.1007/s12026-014-8517-0
Dhabhar, F. S. Enhancing versus suppressive effects of stress on immune function: Implications for immunoprotection and immunopathology. Neuroimmunomodulation. 2009;16(5):300–317.doi:10.1159/000216188
Dhabhar, F. S., Malarkey, W. B., Neri, E., and McEwen, B. S. Stress-induced redistribution of immune cells-from barracks to boulevards to battlefields: A tale of three hormones - curt richter award winner. Psychoneuroendocrinology. 2012;37(9):1345–1368.doi:10.1016/j.psyneuen.2012.05.008
Dimitrijevic, M., and Stanojevic, S. The intriguing mission of neuropeptide Y in the immune system. Amino Acids. 2013;45(1):41–53.
Ding, Y., Lee, M., Gao, Y., Bu, P., Coarfa, C., Miles, B., et al. Neuropeptide Y nerve paracrine regulation of prostate cancer oncogenesis and therapy resistance. Prostate. 2021;81(1):58–71.doi:10.1002/pros.24081
Dogra, P., Rancan, C., Ma, W., Toth, M., Senda, T., Carpenter, D. J., et al. Tissue determinants of human NK cell development, function, and residence. Cell. 2020;180(4):749–763.doi:10.1016/j.cell.2020.01.022
Engel, J. B., Schally, A. V., Halmos, G., Baker, B., Nagy, A., and Keller, G. Targeted therapy with a cytotoxic somatostatin analog, AN-238, inhibits growth of human experimental endometrial carcinomas expressing multidrug resistance protein MDR-1. Cancer. 2005;104(6):1312–1321.doi:10.1002/cncr.21327
Esposito, M. F., Malayil, R., Hanes, M., and Deer, T. Unique characteristics of the dorsal root ganglion as a target for neuromodulation. Pain Med. 2019;20(1):S23–S30.doi:10.1093/pm/pnz012
Faulkner, S., Jobling, P., March, B., Jiang, C. C., and Hondermarck, H. Tumor neurobiology and the war of nerves in cancer. Cancer Discov. 2019;9(6):702–710.doi:10.1158/2159-8290.cd-18-1398
Fjæstad, K. Y., Rømer, A. M. A., Goitea, V., Johansen, A. Z., Thorseth, M. L., Carretta, M., et al. Blockade of beta-adrenergic receptors reduces cancer growth and enhances the response to anti-CTLA4 therapy by modulating the tumor microenvironment. Oncogene. 2022;41(9):1364–1375.doi:10.1038/s41388-021-02170-0
Flayer, C. H., and Sokol, C. L. No pain, no gain: Sensory neurons heal a sunburn. Immunity. 2021;54(7):1374–1376.doi:10.1016/j.immuni.2021.06.011
Fonseca, R. C., Bassi, G. S., Brito, C. C., Rosa, L. B., David, B. A., Araújo, A. M., et al. Vagus nerve regulates the phagocytic and secretory activity of resident macrophages in the liver. Brain, Behav. Immun. 2019;81:444–454.doi:10.1016/j.bbi.2019.06.041
Gabanyi, I., Muller, P. A., Feighery, L., Oliveira, T. Y., Costa-Pinto, F. A., and Mucida, D. Neuro-immune interactions drive tissue programming in intestinal macrophages. Cell. 2016;164(3):378–391.doi:10.1016/j.cell.2015.12.023
Galle-Treger, L., Suzuki, Y., Patel, N., Sankaranarayanan, I., Aron, J. L., Maazi, H., et al. Nicotinic acetylcholine receptor agonist attenuates ILC2-dependent airway hyperreactivity. Nat. Commun. 2016;7:13202.doi:10.1038/ncomms13202
Galoian, K., Abrahamyan, S., Chailyan, G., Qureshi, A., Patel, P., Metser, G., et al. Toll like receptors TLR1/2, TLR6 and MUC5B as binding interaction partners with cytostatic proline rich polypeptide 1 in human chondrosarcoma. Int. J. Oncol. 2018;52(1):139–154.doi:10.3892/ijo.2017.4199
Galoian, K., Scully, S., McNamara, G., Flynn, P., and Galoyan, A. Antitumorigenic effect of brain proline rich polypeptide-1 in human chondrosarcoma. Neurochem. Res. 2009;34(12):2117–2121.doi:10.1007/s11064-009-0009-6
Galoyan, A. Neurochemistry of brain neuroendocrine immune system: Signal molecules. Neurochem. Res. 2000;25(9-10):1343–1355.doi:10.1023/a:1007656431612
Goldfarb, Y., Sorski, L., Benish, M., Levi, B., Melamed, R., and Ben-Eliyahu, S. Improving postoperative immune status and resistance to cancer metastasis. Ann. Surg. 2011;253(4):798–810.doi:10.1097/sla.0b013e318211d7b5
Gonçalves, J., Ribeiro, C. F., Malva, J. O., and Silva, A. P. Protective role of neuropeptide Y Y2receptors in cell death and microglial response following methamphetamine injury. Eur. J. Neurosci. 2012;36(9):3173–3183.doi:10.1111/j.1460-9568.2012.08232.x
Gonzalez-Moles, M. A., Ramos-Garcia, P., and Esteban, F. Significance of the overexpression of substance P and its receptor NK-1R in head and neck carcinogenesis: A systematic review and meta-analysis. Cancers (Basel). 2021;13(6).doi:10.3390/cancers13061349
Granger, C. J., Hoyt, A. K., Moran, A., Becker, B., Sedani, A., Saigh, S., et al. Cancer stem cells as a therapeutic target in 3D tumor models of human chondrosarcoma: An encouraging future for proline rich polypeptide‑1. Mol. Med. Rep. 2020;22(5):3747–3758.doi:10.3892/mmr.2020.11480
Guillot, J., Dominici, C., Lucchesi, A., Nguyen, H. T. T., Puget, A., Hocine, M., et al. Sympathetic axonal sprouting induces changes in macrophage populations and protects against pancreatic cancer. Nat. Commun. 2022;13(1):1985.doi:10.1038/s41467-022-29659-w
Gyoneva, S., and Traynelis, S. F. Norepinephrine modulates the motility of resting and activated microglia via different adrenergic receptors. J. Biol. Chem. 2013;288(21):15291–15302.doi:10.1074/jbc.m113.458901
Gysler, S. M., and Drapkin, R. Tumor innervation: Peripheral nerves take control of the tumor microenvironment. J. Clin. Invest. 2021;131(11).doi:10.1172/JCI147276
Halder, N., and Lal, G. Cholinergic system and its therapeutic importance in inflammation and autoimmunity. Front. Immunol. 2021;12:660342.doi:10.3389/fimmu.2021.660342
Hanahan, D., and Coussens, L. M. Accessories to the crime: Functions of cells recruited to the tumor microenvironment. Cancer Cell. 2012;21(3):309–322.doi:10.1016/j.ccr.2012.02.022
Hoeffel, G., Debroas, G., Roger, A., Rossignol, R., Gouilly, J., Laprie, C., et al. Sensory neuron-derived TAFA4 promotes macrophage tissue repair functions. Nature. 2021;594(7861):94–99.doi:10.1038/s41586-021-03563-7
Hohla, F., Buchholz, S., Schally, A. V., Krishan, A., Rick, F. G., Szalontay, L., et al. Targeted cytotoxic somatostatin analog AN-162 inhibits growth of human colon carcinomas and increases sensitivity of doxorubicin resistant murine leukemia cells. Cancer Lett. 2010;294(1):35–42.doi:10.1016/j.canlet.2010.01.018
Hoskin, J. L., Al-Hasan, Y., and Sabbagh, M. N. Nicotinic acetylcholine receptor agonists for the treatment of alzheimer's dementia: An update. Nicotine Tob. Res. 2019;21(3):370–376.doi:10.1093/ntr/nty116
Hoyer, D., and Bartfai, T. Neuropeptides and neuropeptide receptors: Drug targets, and peptide and non-peptide ligands: A tribute to Prof.Dieter seebach. Chem. Biodivers. 2012;9(11):2367–2387.doi:10.1002/cbdv.201200288
Hu, D., Shen, W., Gong, C., Fang, C., Yao, C., Zhu, X., et al. Grain‐sized moxibustion promotes NK cell antitumour immunity by inhibiting adrenergic signalling in non-small cell lung cancer. J. Cell Mol. Med. 2021;25(6):2900–2908.doi:10.1111/jcmm.16320
Huang, D., Su, S., Cui, X., Shen, X., Zeng, Y., Wu, W., et al. Nerve fibers in breast cancer tissues indicate aggressive tumor progression. Med. Baltim. 2014;93(27):e172.doi:10.1097/md.0000000000000172
Huang, Wang Y., Wang, J. W., Thompson, T., Yin, P. B., Alexander, D., Qin, , et al. Cancer-cell-derived GABA promotes β-catenin-mediated tumour growth and immunosuppression. Nat. Cell Biol. 2022;24(2):230–241.doi:10.1038/s41556-021-00820-9
Huh, J. R., and Veiga-Fernandes, H. Neuroimmune circuits in inter-organ communication. Nat. Rev. Immunol. 2020;20(4):217–228.doi:10.1038/s41577-019-0247-z
Izumi, T., Imai, J., Yamamoto, J., Kawana, Y., Endo, A., Sugawara, H., et al. Vagus-macrophage-hepatocyte link promotes post-injury liver regeneration and whole-body survival through hepatic FoxM1 activation. Nat. Commun. 2018;9(1):5300.doi:10.1038/s41467-018-07747-0
Jiang, J., Bai, J., Qin, T., Wang, Z., and Han, L. NGF from pancreatic stellate cells induces pancreatic cancer proliferation and invasion by PI3K/AKT/GSK signal pathway. J. Cell Mol. Med. 2020;24(10):5901–5910.doi:10.1111/jcmm.15265
Jiang, S. H., Hu, L. P., Wang, X., Li, J., and Zhang, Z. G. Neurotransmitters: Emerging targets in cancer. Oncogene. 2020;39(3):503–515.doi:10.1038/s41388-019-1006-0
Jiang, W., Li, Y., Li, Z. Z., Sun, J., Li, J. W., Wei, W., et al. Chronic restraint stress promotes hepatocellular carcinoma growth by mobilizing splenic myeloid cells through activating β-adrenergic signaling. Brain, Behav. Immun. 2019;80:825–838.doi:10.1016/j.bbi.2019.05.031
Kahán, Z., Nagy, A., Schally, A. V., Hebert, F., Sun, B., Groot, K., et al. Inhibition of growth of MX-1, MCF-7-MIII and MDA-MB-231 human breast cancer xenografts after administration of a targeted cytotoxic analog of somatostatin, AN-238. Int. J. Cancer. 1999;82(4):592–598.
Kamiya, A., Hayama, Y., Kato, S., Shimomura, A., Shimomura, T., Irie, K., et al. Genetic manipulation of autonomic nerve fiber innervation and activity and its effect on breast cancer progression. Nat. Neurosci. 2019;22(8):1289–1305.doi:10.1038/s41593-019-0430-3
Kappos, E. A., Engels, P. E., Tremp, M., Sieber, P. K., von Felten, S., Madduri, S., et al. Denervation leads to volume regression in breast cancer. J. Plastic, Reconstr. Aesthetic Surg. 2018;71(6):833–839.doi:10.1016/j.bjps.2018.03.012
Keskinov, A. A., Tapias, V., Watkins, S. C., Ma, Y., Shurin, M. R., and Shurin, G. V. Impact of the sensory neurons on melanoma growth in vivo. PLoS One. 2016;11(5):e0156095.doi:10.1371/journal.pone.0156095
Klein, M. O., Battagello, D. S., Cardoso, A. R., Hauser, D. N., Bittencourt, J. C., and Correa, R. G. Dopamine: Functions, signaling, and association with neurological diseases. Cell Mol. Neurobiol. 2019;39(1):31–59.doi:10.1007/s10571-018-0632-3
Klose, C. S. N., and Artis, D. Innate lymphoid cells control signaling circuits to regulate tissue-specific immunity. Cell Res. 2020;30(6):475–491.doi:10.1038/s41422-020-0323-8
Klose, C. S. N., Mahlakõiv, T., Moeller, J. B., Rankin, L. C., Flamar, A. L., Kabata, H., et al. The neuropeptide neuromedin U stimulates innate lymphoid cells and type 2 inflammation. Nature. 2017;549(7671):282–286.doi:10.1038/nature23676
Knights, A. J., Liu, S., Ma, Y., Nudell, V. S., Perkey, E., Sorensen, M. J., et al. Acetylcholine-synthesizing macrophages in subcutaneous fat are regulated by β2 -adrenergic signaling. EMBO J. 2021;40(24):e106061.doi:10.15252/embj.2020106061
Kong, Y., Ruan, L., Qian, L., Liu, X., and Le, Y. Norepinephrine promotes microglia to uptake and degrade Amyloid peptide through upregulation of mouse formyl peptide receptor 2 and induction of insulin-degrading enzyme. J. Neurosci. 2010;30(35):11848–11857.doi:10.1523/jneurosci.2985-10.2010
Lechtenberg, K. J., Meyer, S. T., Doyle, J. B., Peterson, T. C., and Buckwalter, M. S. Augmented β2-adrenergic signaling dampens the neuroinflammatory response following ischemic stroke and increases stroke size. J. Neuroinflammation. 2019;16(1):112.doi:10.1186/s12974-019-1506-4
Lee, E. Y., Chan, L. C., Wang, H., Lieng, J., Hung, M., Srinivasan, Y., et al. PACAP is a pathogen-inducible resident antimicrobial neuropeptide affording rapid and contextual molecular host defense of the brain. Proc. Natl. Acad. Sci. U. S. A. 2021;118(1).doi:10.1073/pnas.1917623117
Lee, M. Neurotransmitters and microglial-mediated neuroinflammation. Cpps. 2013;14(1):21–32.doi:10.2174/1389203711314010005
Lei, X., Lei, Y., Li, J. K., Du, W. X., Li, R. G., Yang, J., et al. Immune cells within the tumor microenvironment: Biological functions and roles in cancer immunotherapy. Cancer Lett. 2020;470:126–133.doi:10.1016/j.canlet.2019.11.009
Levi, B., Benish, M., Goldfarb, Y., Sorski, L., Melamed, R., Rosenne, E., et al. Continuous stress disrupts immunostimulatory effects of IL-12. Brain, Behav. Immun. 2011;25(4):727–735.doi:10.1016/j.bbi.2011.01.014
Li, L., Liu, Z., Jiang, Y. Y., Shen, W. X., Peng, Y. P., and Qiu, Y. H. Acetylcholine suppresses microglial inflammatory response via α7nAChR to protect hippocampal neurons. J. Integr. Neurosci. 2019;18(1):51–56.doi:10.31083/j.jin.2019.01.114
Liu, S. Q., Gao, Z. J., Wu, J., Zheng, H. M., Li, B., Sun, S., et al. Single-cell and spatially resolved analysis uncovers cell heterogeneity of breast cancer. J. Hematol. Oncol. 2022;15(1):19.doi:10.1186/s13045-022-01236-0
Liu, Z., Gao, Z., Li, B., Li, J., Ou, Y., Yu, X., et al. Lipid-associated macrophages in the tumor-adipose microenvironment facilitate breast cancer progression. OncoImmunology. 2022;11(1):2085432.doi:10.1080/2162402x.2022.2085432
Mackay, L. K., and Kallies, A. Transcriptional regulation of tissue-resident lymphocytes. Trends Immunol. 2017;38(2):94–103.doi:10.1016/j.it.2016.11.004
Magnon, C., Hall, S. J., Lin, J., Xue, X., Gerber, L., Freedland, S. J., et al. Autonomic nerve development contributes to prostate cancer progression. Science. 2013;341(6142):1236361.doi:10.1126/science.1236361
Magnon, C. The adrenergic nerve network in cancer. Adv. Exp. Med. Biol. 2021;1329:271–294.doi:10.1007/978-3-030-73119-9_15
Marino, F., and Cosentino, M. Adrenergic modulation of immune cells: An update. Amino Acids. 2013;45(1):55–71.doi:10.1007/s00726-011-1186-6
Martinez, V. G., and O'Driscoll, L. Neuromedin U: A multifunctional neuropeptide with pleiotropic roles. Clin. Chem. 2015;61(3):471–482.doi:10.1373/clinchem.2014.231753
Matheis, F., Muller, P. A., Graves, C. L., Gabanyi, I., Kerner, Z. J., Costa-Borges, D., et al. Adrenergic signaling in muscularis macrophages limits infection-induced neuronal loss. Cell. 2020;180(1):64–78. e16.doi:10.1016/j.cell.2019.12.002
Matteoli, G., Gomez-Pinilla, P. J., Nemethova, A., Di Giovangiulio, M., Cailotto, C., van Bree, S. H., et al. A distinct vagal anti-inflammatory pathway modulates intestinal muscularis resident macrophages independent of the spleen. Gut. 2014;63(6):938–948.doi:10.1136/gutjnl-2013-304676
Mohammadpour, H., MacDonald, C. R., McCarthy, P. L., Abrams, S. I., and Repasky, E. A. β2-adrenergic receptor signaling regulates metabolic pathways critical to myeloid-derived suppressor cell function within the TME. Cell Rep. 2021;37(4):109883.doi:10.1016/j.celrep.2021.109883
Mohammadpour, H., MacDonald, C. R., Qiao, G., Chen, M., Dong, B., Hylander, B. L., et al. β2 adrenergic receptor-mediated signaling regulates the immunosuppressive potential of myeloid-derived suppressor cells. J. Clin. Invest. 2019;129(12):5537–5552.doi:10.1172/jci129502
Møller, L. N., Stidsen, C. E., Hartmann, B., and Holst, J. J. Somatostatin receptors. Biochim. Biophys. Acta. 2003;1616(1):1–84.doi:10.1016/s0005-2736(03)00235-9
Moriyama, S., Brestoff, J. R., Flamar, A. L., Moeller, J. B., Klose, C. S. N., Rankin, L. C., et al. β 2 -adrenergic receptor-mediated negative regulation of group 2 innate lymphoid cell responses. Science. 2018;359(6379):1056–1061.doi:10.1126/science.aan4829
Muller, P. A., Koscsó, B., Rajani, G. M., Stevanovic, K., Berres, M. L., Hashimoto, D., et al. Crosstalk between muscularis macrophages and enteric neurons regulates gastrointestinal motility. Cell. 2014;158(2):300–313.doi:10.1016/j.cell.2014.04.050
Nagaraja, A. S., Armaiz-Pena, G. N., Lutgendorf, S. K., and Sood, A. K. Why stress is BAD for cancer patients. J. Clin. Invest. 2013;123(2):558–560.doi:10.1172/JCI67887
Nagashima, H., Mahlakõiv, T., Shih, H. Y., Davis, F. P., Meylan, F., Huang, Y., et al. Neuropeptide CGRP limits group 2 innate lymphoid cell responses and constrains type 2 inflammation. Immunity. 2019;51(4):682–695.doi:10.1016/j.immuni.2019.06.009
Nayak, D., Roth, T. L., and McGavern, D. B. Microglia development and function. Annu. Rev. Immunol. 2014;32:367–402.doi:10.1146/annurev-immunol-032713-120240
Ngo, D. H., and Vo, T. S. An updated review on pharmaceutical properties of gamma-aminobutyric acid. Molecules. 2019;24(15).doi:10.3390/molecules24152678
Pain, S., Vergote, J., Gulhan, Z., Bodard, S., Chalon, S., and Gaillard, A. Inflammatory process in Parkinson disease: Neuroprotection by neuropeptide Y. Fundam. Clin. Pharmacol. 2019;33(5):544–548.doi:10.1111/fcp.12464
Pannell, M., Meier, M. A., Szulzewsky, F., Matyash, V., Endres, M., Kronenberg, G., et al. The subpopulation of microglia expressing functional muscarinic acetylcholine receptors expands in stroke and Alzheimer's disease. Brain Struct. Funct. 2016;221(2):1157–1172.doi:10.1007/s00429-014-0962-y
Partecke, L. I., Speerforck, S., Käding, A., Seubert, F., Kühn, S., Lorenz, E., et al. Chronic stress increases experimental pancreatic cancer growth, reduces survival and can be antagonised by beta-adrenergic receptor blockade. Pancreatology. 2016;16(3):423–433.doi:10.1016/j.pan.2016.03.005
Pavón-Romero, G. F., Serrano-Pérez, N. H., García-Sánchez, L., Ramírez-Jiménez, F., and Terán, L. M. Neuroimmune pathophysiology in asthma. Front. Cell Dev. Biol. 2021;9:663535.doi:10.3389/fcell.2021.663535
Pedersen, L., Idorn, M., Olofsson, G. H., Lauenborg, B., Nookaew, I., Hansen, R. H., et al. Voluntary running suppresses tumor growth through epinephrine- and IL-6-dependent NK cell mobilization and redistribution. Cell Metab. 2016;23(3):554–562.doi:10.1016/j.cmet.2016.01.011
Perner, C., Flayer, C. H., Zhu, X., Aderhold, P. A., Dewan, Z. N. A., Voisin, T., et al. Substance P release by sensory neurons triggers dendritic cell migration and initiates the type-2 immune response to allergens. Immunity. 2020;53(5):1063–1077.doi:10.1016/j.immuni.2020.10.001
Peters, M. A., Walenkamp, A. M., Kema, I. P., Meijer, C., de Vries, E. G., and Oosting, S. F. Dopamine and serotonin regulate tumor behavior by affecting angiogenesis. Drug Resist Updat. 2014;17(4-6):96–104.doi:10.1016/j.drup.2014.09.001
Petkevicius, K., Bidault, G., Virtue, S., Jenkins, B., van Dierendonck, X., Dugourd, A., et al. Norepinephrine promotes triglyceride storage in macrophages via beta2-adrenergic receptor activation. FASEB J. 2021;35(2):e21266.doi:10.1096/fj.202001101R
Petkevicius, K., Bidault, G., Virtue, S., Newland, S. A., Dale, M., Dugourd, A., et al. Macrophage beta2-adrenergic receptor is dispensable for the adipose tissue inflammation and function. Mol. Metab. 2021;48:101220.doi:10.1016/j.molmet.2021.101220
Pinho-Ribeiro, F. A., Baddal, B., Haarsma, R., O’Seaghdha, M., Yang, N. J., Blake, K. J., et al. Blocking neuronal signaling to immune cells treats streptococcal invasive infection. Cell. 2018;173(5):1083–1097.doi:10.1016/j.cell.2018.04.006
Pintér, E., Helyes, Z., and Szolcsányi, J. Inhibitory effect of somatostatin on inflammation and nociception. Pharmacol. Ther. 2006;112(2):440–456.doi:10.1016/j.pharmthera.2006.04.010
Pirzgalska, R. M., Seixas, E., Seidman, J. S., Link, V. M., Sánchez, N. M., Mahú, I., et al. Sympathetic neuron-associated macrophages contribute to obesity by importing and metabolizing norepinephrine. Nat. Med. 2017;23(11):1309–1318.doi:10.1038/nm.4422
Pon, C. K., Lane, J. R., Sloan, E. K., and Halls, M. L. The β 2 ‐adrenoceptor activates a positive cAMP‐calcium feedforward loop to drive breast cancer cell invasion. FASEB J. 2016;30(3):1144–1154.doi:10.1096/fj.15-277798
Prazeres, P., Leonel, C., Silva, W. N., Rocha, B. G. S., Santos, G. S. P., Costa, A. C., et al. Ablation of sensory nerves favours melanoma progression. J. Cell Mol. Med. 2020;24(17):9574–9589.doi:10.1111/jcmm.15381
Qiao, G., Chen, M., Mohammadpour, H., MacDonald, C. R., Bucsek, M. J., Hylander, B. L., et al. Chronic adrenergic stress contributes to metabolic dysfunction and an exhausted phenotype in T cells in the tumor microenvironment. Cancer Immunol. Res. 2021;9(6):651–664.doi:10.1158/2326-6066.cir-20-0445
Quail, D. F., and Joyce, J. A. Microenvironmental regulation of tumor progression and metastasis. Nat. Med. 2013;19(11):1423–1437.doi:10.1038/nm.3394
Quintero-Villegas, A., and Valdés-Ferrer, S. I. Role of 5-HT7 receptors in the immune system in health and disease. Mol. Med. 2019;26(1):2.doi:10.1186/s10020-019-0126-x
Rasiah, K. K., Kench, J. G., Gardiner-Garden, M., Biankin, A. V., Golovsky, D., Brenner, P. C., et al. Aberrant neuropeptide Y and macrophage inhibitory cytokine-1 expression are early events in prostate cancer development and are associated with poor prognosis. Cancer Epidemiol. Biomarkers Prev. 2006;15(4):711–716.doi:10.1158/1055-9965.epi-05-0752
Reavis, H. D., Chen, H. I., and Drapkin, R. Tumor innervation: Cancer has some nerve. Trends Cancer. 2020;6(12):1059–1067.doi:10.1016/j.trecan.2020.07.005
Renz, B. W., Takahashi, R., Tanaka, T., Macchini, M., Hayakawa, Y., Dantes, Z., et al. β2 adrenergic-neurotrophin feedforward loop promotes pancreatic cancer. Cancer Cell. 2018;33(1):75–90.doi:10.1016/j.ccell.2017.11.007
Retamales-Ortega, R., Oróstica, L., Vera, C., Cuevas, P., Hernández, A., Hurtado, I., et al. Role of nerve growth factor (NGF) and miRNAs in epithelial ovarian cancer. Int. J. Mol. Sci. 2017;18(3).doi:10.3390/ijms18030507
Riol-Blanco, L., Ordovas-Montanes, J., Perro, M., Naval, E., Thiriot, A., Alvarez, D., et al. Nociceptive sensory neurons drive interleukin-23-mediated psoriasiform skin inflammation. Nature. 2014;510(7503):157–161.doi:10.1038/nature13199
Saloman, J. L., Albers, K. M., Li, D., Hartman, D. J., Crawford, H. C., Muha, E. A., et al. Ablation of sensory neurons in a genetic model of pancreatic ductal adenocarcinoma slows initiation and progression of cancer. Proc. Natl. Acad. Sci. U.S.A. 2016;113(11):3078–3083.doi:10.1073/pnas.1512603113
Saul, A. N., Oberyszyn, T. M., Daugherty, C., Kusewitt, D., Jones, S., Jewell, S., et al. Chronic stress and susceptibility to skin cancer. J. Natl. Cancer Inst. 2005;97(23):1760–1767.doi:10.1093/jnci/dji401
Schiller, M., Azulay-Debby, H., Boshnak, N., Elyahu, Y., Korin, B., Ben-Shaanan, T. L., et al. Optogenetic activation of local colonic sympathetic innervations attenuates colitis by limiting immune cell extravasation. Immunity. 2021;54(5):1022–1036.doi:10.1016/j.immuni.2021.04.007
Seillet, C., and Jacquelot, N. Sensing of physiological regulators by innate lymphoid cells. Cell Mol. Immunol. 2019;16(5):442–451.doi:10.1038/s41423-019-0217-1
Seillet, C., Luong, K., Tellier, J., Jacquelot, N., Shen, R. D., Hickey, P., et al. The neuropeptide VIP confers anticipatory mucosal immunity by regulating ILC3 activity. Nat. Immunol. 2020;21(2):168–177.doi:10.1038/s41590-019-0567-y
Serhan, N., Basso, L., Sibilano, R., Petitfils, C., Meixiong, J., Bonnart, C., et al. House dust mites activate nociceptor-mast cell clusters to drive type 2 skin inflammation. Nat. Immunol. 2019;20(11):1435–1443.doi:10.1038/s41590-019-0493-z
Sha, M., Cao, J., Sun, H. Y., Tong, Y., and Xia, Q. Neuroendocrine regulation of cholangiocarcinoma: A status quo review. Biochimica Biophysica Acta (BBA) - Rev. Cancer. 2019;1872(1):66–73.doi:10.1016/j.bbcan.2019.05.005
Shan, B., Shao, M., Zhang, Q., An, Y. A., Vishvanath, L., and Gupta, R. K. Cold-responsive adipocyte progenitors couple adrenergic signaling to immune cell activation to promote beige adipocyte accrual. Genes Dev. 2021;35(19-20):1333–1338.doi:10.1101/gad.348762.121
Silverman, D. A., Martinez, V. K., Dougherty, P. M., Myers, J. N., Calin, G. A., and Amit, M. Cancer-associated neurogenesis and nerve-cancer cross-talk. Cancer Res. 2021;81(6):1431–1440.doi:10.1158/0008-5472.can-20-2793
Sloan, E. K., Priceman, S. J., Cox, B. F., Yu, S., Pimentel, M. A., Tangkanangnukul, V., et al. The sympathetic nervous system induces a metastatic switch in primary breast cancer. Cancer Res. 2010;70(18):7042–7052.doi:10.1158/0008-5472.can-10-0522
Soler Palacios, B., and Gutiérrez‐González, A. Neuron−macrophage interaction in the healing process of the skin. Allergy. 2022;77(3):1064–1066.doi:10.1111/all.15122
Srinivasan, A. V. Propranolol: A 50-year historical perspective. Ann. Indian Acad. Neurol. 2019;22(1):21–26.doi:10.4103/aian.aian_201_18
Stolero, N., and Frenkel, D. The dialog between neurons and microglia in Alzheimer's disease: The neurotransmitters view. J. Neurochem. 2021;158(6):1412–1424.doi:10.1111/jnc.15262
Sui, P., Wiesner, D. L., Xu, J., Zhang, Y., Lee, J., Van Dyken, S., et al. Pulmonary neuroendocrine cells amplify allergic asthma responses. Science. 2018;360(6393).doi:10.1126/science.aan8546
Talbot, J., Hahn, P., Kroehling, L., Nguyen, H., Li, D., and Littman, D. R. Feeding-dependent VIP neuron-ILC3 circuit regulates the intestinal barrier. Nature. 2020;579(7800):575–580.doi:10.1038/s41586-020-2039-9
Talbot, S., Abdulnour, R. E., Burkett, P. R., Lee, S., Cronin, S. J., Pascal, M. A., et al. Silencing nociceptor neurons reduces allergic airway inflammation. Neuron. 2015;87(2):341–354.doi:10.1016/j.neuron.2015.06.007
Tang, L., Okamoto, S., Shiuchi, T., Toda, C., Takagi, K., Sato, T., et al. Sympathetic nerve activity maintains an anti-inflammatory state in adipose tissue in male mice by inhibiting TNF-α gene expression in macrophages. Endocrinology. 2015;156(10):3680–3694.doi:10.1210/en.2015-1096
Tanner, M. A., Maitz, C. A., and Grisanti, L. A. Immune cell β2-adrenergic receptors contribute to the development of heart failure. Am. J. Physiology-Heart Circulatory Physiology. 2021;321(4):H633–H649.doi:10.1152/ajpheart.00243.2021
Thaker, P. H., Han, L. Y., Kamat, A. A., Arevalo, J. M., Takahashi, R., Lu, C., et al. Chronic stress promotes tumor growth and angiogenesis in a mouse model of ovarian carcinoma. Nat. Med. 2006;12(8):939–944.doi:10.1038/nm1447
Thomas Broome, S., Louangaphay, K., Keay, K. A., Leggio, G. M., Musumeci, G., and Castorina, A. Dopamine: An immune transmitter. Neural Regen. Res. 2020;15(12):2173–2185.doi:10.4103/1673-5374.284976
Treszl, A., Schally, A. V., Seitz, S., Szalontay, L., Rick, F. G., Szepeshazi, K., et al. Inhibition of human non-small cell lung cancers with a targeted cytotoxic somatostatin analog, AN-162. Peptides. 2009;30(9):1643–1650.doi:10.1016/j.peptides.2009.06.007
Triaca, V., Carito, V., Fico, E., Rosso, P., Fiore, M., Ralli, M., et al. Cancer stem cells-driven tumor growth and immune escape: The janus face of neurotrophins. Aging. 2019;11(23):11770–11792.doi:10.18632/aging.102499
Umpierre, A. D., and Wu, L. J. Microglia research in the 100th year since its discovery. Neurosci. Bull. 2020;36(3):303–306.doi:10.1007/s12264-020-00477-8
Ural, B. B., Yeung, S. T., Damani-Yokota, P., Devlin, J. C., de Vries, M., Vera-Licona, P., et al. Identification of a nerve-associated, lung-resident interstitial macrophage subset with distinct localization and immunoregulatory properties. Sci. Immunol. 2020;5(45).doi:10.1126/sciimmunol.aax8756
von Arx, C., Rea, G., Napolitano, M., Ottaiano, A., Tatangelo, F., Izzo, F., et al. Effect of octreotide long-acting release on tregs and MDSC cells in neuroendocrine tumour patients: A pivotal prospective study. Cancers (Basel). 2020;12(9).doi:10.3390/cancers12092422
Wagner, M. J., Cranmer, L. D., Loggers, E. T., and Pollack, S. M. Propranolol for the treatment of vascular sarcomas. Jep. 2018;Vol. 10:51–58.doi:10.2147/jep.s146211
Wallrapp, A., Burkett, P. R., Riesenfeld, S. J., Kim, S. J., Christian, E., Abdulnour, R. E., et al. Calcitonin gene-related peptide negatively regulates alarmin-driven type 2 innate lymphoid cell responses. Immunity. 2019;51(4):709–723.doi:10.1016/j.immuni.2019.09.005
Wallrapp, A., Riesenfeld, S. J., Burkett, P. R., Abdulnour, R. E., Nyman, J., Dionne, D., et al. The neuropeptide NMU amplifies ILC2-driven allergic lung inflammation. Nature. 2017;549(7672):351–356.doi:10.1038/nature24029
Wang, H., Yu, M., Ochani, M., Amella, C. A., Tanovic, M., Susarla, S., et al. Nicotinic acetylcholine receptor α7 subunit is an essential regulator of inflammation. Nature. 2003;421(6921):384–388.doi:10.1038/nature01339
Wang, Y. N., Tang, Y., He, Z., Ma, H., Wang, L., Liu, Y., et al. Slit3 secreted from M2-like macrophages increases sympathetic activity and thermogenesis in adipose tissue. Nat. Metab. 2021;3(11):1536–1551.doi:10.1038/s42255-021-00482-9
Waqas, S. F. H., Hoang, A. C., Lin, Y. T., Ampem, G., Azegrouz, H., Balogh, L., et al. Neuropeptide FF increases M2 activation and self-renewal of adipose tissue macrophages. J. Clin. Invest. 2017;127(9):3559.doi:10.1172/jci95841
Weckbecker, G., Lewis, I., Albert, R., Schmid, H. A., Hoyer, D., and Bruns, C. Opportunities in somatostatin research: Biological, chemical and therapeutic aspects. Nat. Rev. Drug Discov. 2003;2(12):999–1017.doi:10.1038/nrd1255
Wehkamp, U., Stern, S., Krüger, S., Weichenthal, M., Hauschild, A., Röcken, C., et al. Co-expression of NGF and PD-L1 on tumor-associated immune cells in the microenvironment of Merkel cell carcinoma. J. Cancer Res. Clin. Oncol. 2018;144(7):1301–1308.doi:10.1007/s00432-018-2657-x
Weisberg, S. P., Ural, B. B., and Farber, D. L. Tissue-specific immunity for a changing world. Cell. 2021;184(6):1517–1529.doi:10.1016/j.cell.2021.01.042
Werneburg, S., Feinberg, P. A., Johnson, K. M., and Schafer, D. P. A microglia-cytokine axis to modulate synaptic connectivity and function. Curr. Opin. Neurobiol. 2017;47:138–145.doi:10.1016/j.conb.2017.10.002
Willemze, R. A., Welting, O., van Hamersveld, P., Verseijden, C., Nijhuis, L. E., Hilbers, F. W., et al. Loss of intestinal sympathetic innervation elicits an innate immune driven colitis. Mol. Med. 2019;25(1):1.doi:10.1186/s10020-018-0068-8
Wolf, Y., Boura-Halfon, S., Cortese, N., Haimon, Z., Sar Shalom, H., Kuperman, Y., et al. Brown-adipose-tissue macrophages control tissue innervation and homeostatic energy expenditure. Nat. Immunol. 2017;18(6):665–674.doi:10.1038/ni.3746
Wong, C. H., Jenne, C. N., Lee, W. Y., Léger, C., and Kubes, P. Functional innervation of hepatic iNKT cells is immunosuppressive following stroke. Science. 2011;334(6052):101–105.doi:10.1126/science.1210301
Xie, H., Heier, C., Meng, X., Bakiri, L., Pototschnig, I., Tang, Z., et al. An immune-sympathetic neuron communication axis guides adipose tissue browning in cancer-associated cachexia. Proc. Natl. Acad. Sci. U. S. A. 2022;119(9).doi:10.1073/pnas.2112840119
Xu, H., Ding, J., Porter, C. B. M., Wallrapp, A., Tabaka, M., Ma, S., et al. Transcriptional atlas of intestinal immune cells reveals that neuropeptide α-CGRP modulates group 2 innate lymphoid cell responses. Immunity. 2019;51(4):696–708.doi:10.1016/j.immuni.2019.09.004
Yamamoto, Y., Otsuka, A., Ishida, Y., Wong, L. S., Seidel, J. A., Nonomura, Y., et al. Pituitary adenylate cyclase-activating polypeptide promotes cutaneous dendritic cell functions in contact hypersensitivity. J. Allergy Clin. Immunol. 2021;148(3):858–866.doi:10.1016/j.jaci.2021.02.005
Yang, N. N., Yang, J. W., Ye, Y., Huang, J., Wang, L., Wang, Y., et al. Electroacupuncture ameliorates intestinal inflammation by activating α7nAChR-mediated JAK2/STAT3 signaling pathway in postoperative ileus. Theranostics. 2021;11(9):4078–4089.doi:10.7150/thno.52574
Ye, D., Xu, H., Tang, Q., Xia, H., Zhang, C., and Bi, F. The role of 5-HT metabolism in cancer. Biochimica Biophysica Acta (BBA) - Rev. Cancer. 2021;1876(2):188618.doi:10.1016/j.bbcan.2021.188618
Younger, D., Murugan, M., Rama Rao, K. V., Wu, L. J., and Chandra, N. Microglia receptors in animal models of traumatic brain injury. Mol. Neurobiol. 2019;56(7):5202–5228.doi:10.1007/s12035-018-1428-7
Yu, J., Xiao, K., Chen, X., Deng, L., Zhang, L., Li, Y., et al. Neuron-derived neuropeptide Y fine-tunes the splenic immune responses. Neuron. 2022;110(8):1327–1339.doi:10.1016/j.neuron.2022.01.010
Zahalka, A. H., Arnal-Estapé, A., Maryanovich, M., Nakahara, F., Cruz, C. D., Finley, L. W. S., et al. Adrenergic nerves activate an angio-metabolic switch in prostate cancer. Science. 2017;358(6361):321–326.doi:10.1126/science.aah5072
Zahalka, A. H., and Frenette, P. S. Nerves in cancer. Nat. Rev. Cancer. 2020;20(3):143–157.doi:10.1038/s41568-019-0237-2
Zhang, B., Vogelzang, A., Miyajima, M., Sugiura, Y., Wu, Y., Chamoto, K., et al. B cell-derived GABA elicits IL-10+ macrophages to limit anti-tumour immunity. Nature. 2021;599(7885):471–476.doi:10.1038/s41586-021-04082-1
Zhang, K., Guo, X. M., Yan, Y. W., Liu, Y. Y., Xu, Z. F., Zhao, X., et al. Applying statistical and complex network methods to explore the key signaling molecules of acupuncture regulating neuroendocrine-immune network. Evid. Based Complement. Altern. Med. 2018;2018:9260630.doi:10.1155/2018/9260630
Zhang, L., Wu, Z., Tong, Z., Yao, Q., Wang, Z., and Li, W. Vagus nerve stimulation decreases pancreatitis severity in mice. Front. Immunol. 2020;11:595957.doi:10.3389/fimmu.2020.595957
Zhang, Q., Lu, Y., Bian, H., Guo, L., and Zhu, H. Activation of the α7 nicotinic receptor promotes lipopolysaccharide-induced conversion of M1 microglia to M2. Am. J. Transl. Res. 2017;9(3):971–985.
Zhao, C. M., Hayakawa, Y., Kodama, Y., Muthupalani, S., Westphalen, C. B., Andersen, G. T., et al. Denervation suppresses gastric tumorigenesis. Sci. Transl. Med. 2014;6(250):250ra115.doi:10.1126/scitranslmed.3009569
Zheng, J., Liu, Y., Lau, Y. L., and Tu, W. γδ-T cells: An unpolished sword in human anti-infection immunity. Cell Mol. Immunol. 2013;10(1):50–57.doi:10.1038/cmi.2012.43
Zhou, L., Li, Y., Li, X., Chen, G., Liang, H., Wu, Y., et al. Propranolol attenuates surgical stress-induced elevation of the regulatory T cell response in patients undergoing radical mastectomy. J. I. 2016;196(8):3460–3469.doi:10.4049/jimmunol.1501677
Glossary
TME tumor microenvironment
DC dendritic cell
ILC innate lymphoid cell
TRM tissue-resident memory T cell
CNS central nervous system
PNS peripheral nervous system
NE norepinephrine
AR adrenergic receptor
NGF nerve growth factor
TEM effective memory T cell
ATM adipose tissue macrophage
ASC adipose stem cell
MM muscularis macrophage
iNKT invariant natural killer T
ACh acetylcholine
mAChR metabotropic muscarinic ACh receptor
nAChR ionotropic nicotinic ACh receptor
5-HT serotonin
GABA gamma-aminobutyric acid
DA dopamine
DR dopamine receptor
5-HTR 5-HT receptor
NMU neuromedin U
SP substance P
CGRP calcitonin gene-related peptide
PACAP pituitary adenylate cyclase-activating polypeptide
VIP vasoactive intestinal peptide
NPFF neuropeptide FF
NPY neuropeptide Y
CRLR receptor calcitonin receptor like receptor
PNEC pulmonary neuroendocrine cell
MRGPR Mas-related G-protein coupled receptor member
dLN draining lymph node
ChAM cholinergic adipose macrophage
BMP2 bone morphogenetic protein 2
CSF1 colony stimulatory factor
SAM Sympathetic neuron-associated macrophage
NAM nerve- and airway-associated macrophage
MDSC myeloid-derived suppressor cell
DRG dorsal root ganglia
PRP-1 proline rich polypeptide 1
SST somatostatin
SSA somatostatin analog
Keywords: neuroendocrine regulation, neurotransmitter, neuropeptide, tissue-specific immunity, cancer
Citation: Liu S-Q, Li B, Li J-J, Sun S, Sun S-R and Wu Q (2022) Neuroendocrine regulations in tissue-specific immunity: From mechanism to applications in tumor. Front. Cell Dev. Biol. 10:896147. doi: 10.3389/fcell.2022.896147
Received: 14 March 2022; Accepted: 27 July 2022;
Published: 22 August 2022.
Edited by:
Zhi-Gang Zhang, Shanghai Jiao Tong University, ChinaReviewed by:
Alexander Birbrair, Federal University of Minas Gerais, BrazilKarina Galoian, University of Miami, United States
Copyright © 2022 Liu, Li, Li, Sun, Sun and Wu. This is an open-access article distributed under the terms of the Creative Commons Attribution License (CC BY). The use, distribution or reproduction in other forums is permitted, provided the original author(s) and the copyright owner(s) are credited and that the original publication in this journal is cited, in accordance with accepted academic practice. No use, distribution or reproduction is permitted which does not comply with these terms.
*Correspondence: Sheng-Rong Sun, U3VuU1IxMzdAd2h1LmVkdS5jbg==; Qi Wu, d2Fpd2FpQHdodS5lZHUuY24=
†These authors have contributed equally to this work