- 1Department of Clinical and Experimental Cardiology, Heart Center, Amsterdam UMC, University of Amsterdam, Amsterdam, Netherlands
- 2Division of Pharmacoepidemiology and Clinical Pharmacology, Utrecht Institute for Pharmaceutical Sciences, Utrecht University, Utrecht, Netherlands
- 3Department of Cardiology, Herlev and Gentofte Hospital, University of Copenhagen, Gentofte, Denmark
- 4Department of Medical Biology, Amsterdam Cardiovascular Sciences, Amsterdam UMC, University of Amsterdam, Amsterdam, Netherlands
- 5Netherlands Heart Institute, Utrecht, Netherlands
Aim: To assess the risk of sudden cardiac arrest (SCA) associated with the use of carbamazepine (CBZ) and establish the possible underlying cellular electrophysiological mechanisms.
Methods: The SCA risk association with CBZ was studied in general population cohorts using a case–control design (n = 5,473 SCA cases, 21,866 non-SCA controls). Effects of 1–100 µM CBZ on action potentials (APs) and individual membrane currents were determined in isolated rabbit and human cardiomyocytes using the patch clamp technique.
Results: CBZ use was associated with increased risk of SCA compared with no use (adjusted odds ratio 1.90 [95% confidence interval: 1.12–3.24]). CBZ reduced the AP upstroke velocity of rabbit and human cardiomyocytes, without prominent changes in other AP parameters. The reduction occurred at ≥30 µM and was frequency-dependent with a more pronounced reduction at high stimulus frequencies. The cardiac sodium current (INa) was reduced at ≥30 μM; this was accompanied by a hyperpolarizing shift in the voltage-dependency of inactivation. The recovery from inactivation was slower, which is consistent with the more pronounced AP upstroke velocity reduction at high stimulus frequencies. The main cardiac K+ and Ca2+ currents were unaffected, except reduction of L-type Ca2+ current by 100 µM CBZ.
Conclusion: CBZ use is associated with an increased risk of SCA in the general population. At concentrations of 30 µM and above, CBZ reduces AP upstroke velocity and INa in cardiomyocytes. Since the concentration of 30 µM is well within the therapeutic range (20–40 µM), we conclude that CBZ increases the risk of SCA by a reduction of the cardiac INa.
1 Introduction
Sudden cardiac arrest (SCA) is a global public health problem with an annual incidence of 40–100 per 100,000 individuals (Fishman et al., 2010; Hayashi et al., 2015). SCA accounts for 50% of deaths from cardiovascular disease and 15–20% of all deaths in industrialized societies (Zipes and Wellens 1998; Wong et al., 2019). Most cases of SCA are caused by cardiac arrhythmias (ventricular fibrillation (VF) or ventricular tachycardia (VT)). Such arrhythmias may arise from functional changes in the ion channels that underlie the cardiac action potential (AP) (Antzelevitch and Burashnikov 2011). These functional changes may be evoked by various drugs used for the treatment of cardiac or non-cardiac conditions. This is best known for drugs that affect cardiac repolarization (QT prolonging drugs) (Haverkamp et al., 2000). However, there is increasing recognition that it also applies to drugs that affect cardiac depolarization (Bardai et al., 2013). An example of such drugs are anti-epileptic drugs (AEDs) (Bardai et al., 2015). Some AEDs are primarily developed for blocking neuronal ion channels, e.g., voltage-gated Na+, Ca2+ or K+ channels, while other AEDs act by impacting on neurotransmitters such as γ-aminobutyric acid (Davies 1995; Sills and Rogawski 2020). Importantly, neuronal and cardiac ion channel isoforms are highly homologous (Heinemann et al., 1994; Fozzard and Hanck 1996). Thus, AEDs may not only affect neuronal electrical activity but may also act on cardiac ion channels, thereby causing cardiac arrhythmias (Danielsson et al., 2005). Accordingly, the increased SCA risk of epilepsy patients may be partly explained by AED use (Bardai et al., 2015).
Carbamazepine (CBZ) is a prime example of such drugs, because it has high efficacy in the treatment of epilepsy (Pellock 2000) through various mechanism, including block of neuronal Na+ channels (Ragsdale and Avoli 1998; Catterall 1999; Sun et al., 2006; Lasoń et al., 2013). CBZ may also impact on cardiac electrophysiology as suggested by several CBZ-related case reports and retrospective studies, which report bradycardia, sinoatrial and atrioventricular block, QRS interval prolongation, cardiac arrhythmias, and cardiac arrest, as summarized in Table 1 (Beermann et al., 1975; Hamilton 1978; Herzberg 1978; Boesen et al., 1983; Leslie et al., 1983; Benassi et al., 1987; Kasarskis et al., 1992; Hojer et al., 1993; Schmidt and Schmitz-Buhl 1995; Koutsampasopoulos et al., 2014). Still, the underlying electrophysiological mechanism is not completely understood. Our current study has two aims: 1) to establish whether CBZ is associated with increased SCA risk in a large dataset from a cohort that was specifically designed to study SCA in the general population; 2) to establish the effects of CBZ on cardiac APs and individual membrane currents of rabbit and human cardiomyocytes using patch clamp methodology.
2 Methods and Materials
2.1 Epidemiological Studies
We studied the SCA risk associated with CBZ use in a case–control design. Cases were patients who suffered out-of-hospital SCA with presumed cardiac causes in the Amsterdam Resuscitation Studies (ARREST) registry. ARREST is an ongoing, prospective, population-based registry that we designed to study the occurrence and outcome of out-of-hospital SCA in the general population. Patients are collected in collaboration with dispatch centers, ambulance personnel, pharmacies and hospitals in one contiguous study region in the Netherlands (2.6 million inhabitants, urban and rural areas), thereby assuring collection of >95% of all out-of-hospital SCA patients in the study region and minimizing inclusion bias (Blom et al., 2014). Each out-of-hospital SCA case was matched with up to five non-SCA controls based on age, sex and index-date (SCA-date). Non-SCA controls were randomly drawn from the general population using the PHARMO Database Network (Kuiper et al., 2020), which contains, among other things, complete medication data from the community pharmacists across the Netherlands.
Drug dispensing records for drugs prescription were obtained from computerized databases of pharmacists. Use of CBZ was defined as having a drug-dispensing record within 90 days prior to index-date. We chose a period of 90 days, since, in the Netherlands, prescription length for drugs used for chronic disease is 90 days.
For all cases and controls, we included cardiovascular disease and diabetes mellitus in our analyses because these are known risk factors for SCA. We derived cardiovascular disease and diabetes mellitus by using medication use as proxies as we did previously (Eroglu et al., 2020). Cardiovascular disease was defined by use of β-adrenoceptor blockers, calcium channel blockers, diuretics, renin-angiotensin system inhibitors, diuretics, antithrombotics, nitrates and statins. Diabetes mellitus was defined by use of antidiabetics. Patients were considered users of cardiovascular drugs and antidiabetics if there was any drug-dispensing record within 6 months prior to index-date.
2.2 Cellular Electrophysiological Studies
2.2.1 Cell Preparations
Full details of rabbit ventricular and human atrial cell isolation procedures are provided in the Supplementary Material. The investigation using rabbits conformed to the Guide for the Care and Use of Laboratory Animals (NIH Publication 85–23, 1996) and was approved by the institutional animal experiments committee. The human atrial cardiomyocytes were isolated from explanted hearts of male patients with end-stage heart failure caused by ischemic cardiomyopathy. All patients were in New York Heart Association functional class IV and received standard therapy for chronic heart failure (Supplementary Table S1). Informed consent was obtained before heart transplantation, and the protocol complied with institutional guidelines.
2.2.2 Action Potentials
APs were measured at 36 ± 0.2°C in modified Tyrode’s solution containing (in mM): NaCl 140, KCl 5.4, CaCl2 1.8, MgCl2 1.0, glucose 5.5, HEPES 5.0; pH 7.4 (NaOH). Patch pipettes were filled with solution composed of (in mM): K-gluconate 125, KCl 20, NaCl 5.0, K2ATP 2.0, HEPES 10; pH 7.2 (KOH). Detailed recording procedures are provided in the Supplementary Material. APs were evoked at stimulation rates of 0.2–4 Hz using square 3-ms current pulses through the patch pipette. To reduce variability in the moment of AP upstroke, stimulus amplitude was chosen such that the AP upstroke originated just before the end of the stimulus, as we described previously (Remme et al., 2006). The maximal AP upstroke velocity (dV/dtmax) was determined from the first derivative of the AP upstroke from which the approximately constant initial dV/dt in response to the stimulus pulse was subtracted (Figure 1A, inset). In addition, we analyzed resting membrane potential (RMP), AP amplitude (APA), and AP duration at 90% repolarization (APD90), as also shown in Figure 1A. AP parameters from 10 consecutive APs were averaged.
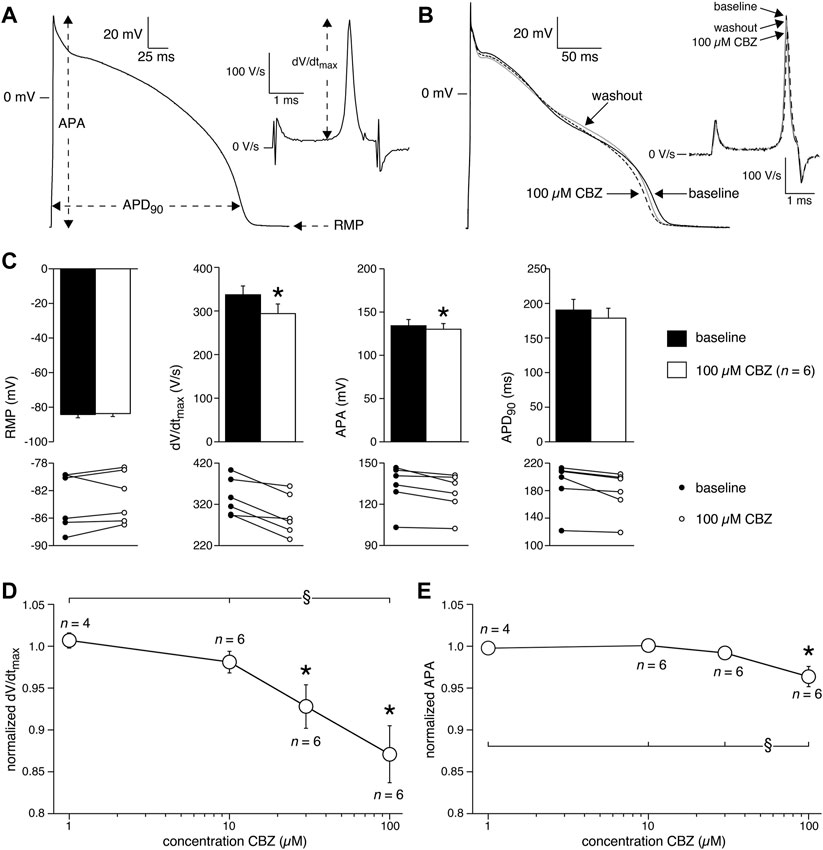
FIGURE 1. Carbamazepine (CBZ) reduces the action potential (AP) upstroke velocity and AP amplitude of rabbit ventricular cardiomyocytes. (A) AP recording illustrating the analyzed AP parameters. Inset: time derivative (dV/dt) of the AP upstroke on an expanded time scale. RMP, resting membrane potential; APA, AP amplitude; APD90, AP duration at 90% of repolarization; dV/dtmax, maximal AP upstroke velocity. (B) Superimposed representative APs at 1 Hz under baseline conditions, in presence of 100 µM CBZ, and upon washout of the drug. Inset: time derivatives of the AP upstrokes. (C) Average AP characteristics at 1 Hz (top panels) and individual (paired) data points (bottom panels). *p < 0.05 CBZ versus baseline (One-Way RM ANOVA). (D,E) Average normalized dV/dtmax (D) and APA (E) at 1 Hz in response to 1, 10, 30, and 100 µM CBZ. Values are normalized to the values measured under baseline conditions. Numbers near symbols indicate the number of cells (n) measured at a given concentration. *p < 0.05 CBZ versus baseline (One-Way RM ANOVA); §p < 0.05 CBZ 100 µM versus lower concentrations (One-Way ANOVA).
2.2.3 Membrane Current Measurements
The L-type Ca2+ current (ICa,L), inward rectifier K+ current (IK1), delayed rectifier K+ current (IK), and transient outward K+ current (Ito1) were all measured at 36 ± 0.2°C with the same solutions as used for the AP measurements. However, Ito1 was measured in the presence of CdCl2 (0.25 mM) to block INa and ICa,L, thereby also preventing activation of the outward Ca2+-activated Cl− current (Verkerk et al., 2011). Suppression of these inward and outward currents allows accurate determination of Ito1. The whole-cell sodium current (INa) in freshly isolated cardiomyocytes is an extremely large and fast activating and inactivating membrane current, which for technical reasons cannot be reliably measured at a close-to-physiological temperature and normal Na+ gradients over the cell membrane (see Berecki et al. (2010) and primary references cited therein). Therefore, we measured INa at room temperature with modified bath and pipette solutions (including an identical Na+ concentration in pipette and bath solution), which allowed specific measurements of Na+ currents only. Bath solution for INa measurements contained (in mM): NaCl 7.0, CsCl 133, CaCl2 1.8, MgCl2 1.2, glucose 11.0, HEPES 5.0, and nifedipine 0.05; pH 7.4 (CsOH). Patch pipettes for INa measurements were filled with (in mM): NaCl 3.0, CsCl 133, MgCl2 2.0, Na2ATP 2.0, TEA-Cl 2.0, EGTA 10, HEPES 5.0; pH 7.3 (CsOH). The membrane currents were measured with specific voltage clamp protocols as depicted in the insets to Figures 3–5 and described in detail in the Supplementary Material. Recording procedures and data analysis are also described in detail in the Supplementary Material.
2.2.4 Preparation of Carbamazepine
CBZ obtained from Sigma-Aldrich (St. Louis, MO, US) was freshly dissolved every day in dimethyl sulfoxide (DMSO) as 100 mM stock and diluted in the bath solution to the desired concentration just before use. APs and membrane currents were measured in the presence of the vehicle DMSO and after wash-in of CBZ (1, 10, 30, or 100 µM) in the same cardiomyocytes. In order to obtain steady-state conditions, signals were recorded after a 5 min stimulation period, i.e. under baseline conditions, and 5 min after application of CBZ.
2.3 Statistics
Data are presented as mean ± SEM. The association between CBZ and SCA was estimated by calculating the adjusted odds ratio with 95% confidence interval using conditional logistic regression by adjusting for the use of cardiovascular drugs and antidiabetics. For the patch-clamp study, comparisons were made using One-Way ANOVA, One-Way Repeated Measures (RM) ANOVA, or Two-Way RM ANOVA, followed by pairwise comparison using the Student-Newman-Keuls post hoc test. For the epidemiological study, differences in baseline values for binary variables between cases and controls were tested using a chi-square test. Differences in baseline values for continuous variables between cases and controls were tested using an independent t-test. p < 0.05 defined statistical significance.
3 Results
3.1 Carbamazepine Use and the Risk of Sudden Cardiac Arrest
We first conducted a systematic study to establish whether CBZ use is associated with increased risk of SCA in the general population. We identified 5,473 SCA cases, and matched them to 21,866 non-SCA controls. The mean age of the cases was 68.8 years and 69.9% were male. As expected, the prevalence of cardiovascular drugs and antidiabetics was higher among the cases than controls (Table 2). We observed that the proportion of CBZ users was significantly higher among cases (n = 24, 0.44%) than among controls (n = 41, 0.19%) (Table 3). After adjusting for cardiovascular drugs and antidiabetics, we found that use of CBZ was associated with increased risk of SCA compared with no use of CBZ, with an adjusted odds ratio of 1.90 (95% confidence interval: 1.12–3.24; Table 3).
3.2 Effects of Carbamazepine on Action Potentials of Rabbit Ventricular Cardiomyocytes
Next, we characterized the effects of 1, 10, 30, and 100 µM CBZ on APs elicited at 1 Hz in rabbit ventricular cardiomyocytes. Figure 1B shows typical APs under baseline conditions (solid line), in the presence of 100 µM CBZ (dashed line), and upon washout of the drug (gray line). Exposure to 100 µM CBZ resulted in substantial alterations in AP morphology in comparison to baseline conditions, particularly a decrease in dV/dtmax and APA (as measures of cardiac depolarization) and a slight decrease of APD90 (as a measure of cardiac repolarization). The effects were partially reversible upon washout of the drug. Average data are shown in the top panels of Figure 1C, with the individual (paired) data of the 6 cells tested shown in the bottom panels. These data indicate that dV/dtmax and APA were significantly decreased by 12.9 ± 3.3% (294 ± 22 (CBZ) vs. 337 ± 20 V/s (baseline)) and 3.6 ± 1.2% (128 ± 6.5 (CBZ) vs. 133 ± 7.2 mV (baseline)), respectively. The effects of CBZ on dV/dtmax and APA were concentration dependent (Figures 1D,E). At 100 µM CBZ, RMP was unaffected (–83.0 ± 1.7 (CBZ) vs. –83.5 ± 1.8 mV (baseline)) and the small effect on APD90 (177 ± 14 (CBZ) vs. 189 ± 15 ms (baseline)) did not reach the level of statistical significance (Figure 1C). Similarly, no statistically significant effects on RMP and APD90 were observed at other stimulus frequencies or at lower CBZ concentrations (Supplementary Figure S1).
The upstroke of APs in working cardiomyocytes is mainly due to INa (see Berecki et al. (2010) and primary references cited therein), which suggests that the CBZ-induced decrease in dV/dtmax is due to blockade of INa. It is well-known that drugs may block INa in a voltage- and use-dependent manner (Bagal et al., 2015). The latter means that the amount of block may increase upon higher stimulus frequencies. Figure 2A shows typical AP time derivatives under baseline and 100 µM CBZ conditions at stimulus frequencies ranging from 0.2 to 4 Hz, while Figure 2B summarizes the average effects on dV/dtmax at 100 µM CBZ as well as lower concentrations. An increase in stimulus frequency resulted in a significantly lower dV/dtmax at every concentration tested (Figure 2B, filled squares; see also Supplementary Figure S2), consistent with a reduced INa recovery from inactivation at fast pacing rates (Berecki et al., 2010). In addition, the CBZ-induced decrease in dV/dtmax is more pronounced at higher stimulus frequencies (Figure 2B, open circles). For example, 100 µM CBZ decreased dV/dtmax by 14.1 ± 3.4% (294 ± 20 (CBZ) vs. 342 ± 18 V/s (baseline)) at 0.2 Hz, but by as much as 41.5 ± 12% (143 ± 21 (CBZ) vs. 264 ± 33 V/s (baseline)) at 4 Hz. Because APA and dV/dtmax are both importantly determined by INa (Krishnan and Antzelevitch 1991; Berecki et al., 2010), it is not surprising that the APA shows a largely similar concentration and frequency dependency as dV/dtmax (Figures 2B,C).
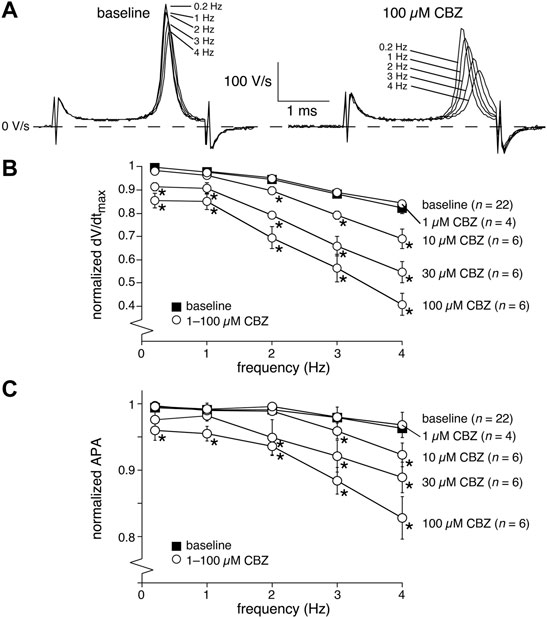
FIGURE 2. Carbamazepine (CBZ) reduces the AP upstroke velocity and amplitude of rabbit ventricular cardiomyocytes in a frequency dependent manner. (A) Superimposed typical time derivatives (dV/dt) during the AP upstroke phase at stimulus frequencies ranging from 0.2 to 4 Hz under baseline conditions (left) and in presence of 100 µM CBZ (right). (B) Average dV/dtmax under baseline conditions (filled squares) and in response to 1–100 µM CBZ (open circles) at stimulus frequencies ranging from 0.2 to 4 Hz. Values are normalized to the highest dV/dtmax measured at 0.2–4 Hz under baseline conditions. *p < 0.05 CBZ versus baseline (Two-Way RM ANOVA). See Supplementary Figure S2 for statistical significance of the frequency dependent effects. (C) Average APA under baseline conditions (filled squares) and in response to 1–100 µM CBZ (open circles) at stimulus frequencies ranging from 0.2 to 4 Hz. Values are normalized to the highest APA measured at 0.2–4 Hz under baseline conditions. *p < 0.05 CBZ versus baseline (Two-Way RM ANOVA).
3.3 Effects of Carbamazepine on Membrane Currents of Rabbit Ventricular Cardiomyocytes
We next studied the effects of CBZ on the main membrane currents underlying cardiac APs in rabbit ventricular cardiomyocytes. First, we focused on the main current underlying the AP depolarization, i.e., INa. Figure 3A shows typical INa recordings (at −80 to 0 mV) and Figure 3B shows the average current-voltage (I-V) relationships of INa under baseline conditions and in the presence of 100 µM CBZ. CBZ significantly decreased INa in the voltage range from −45 to +10 mV, e.g., by 30.3 ± 6.7% at −30 mV (67.8 ± 6.7% (CBZ) vs. 97.3 ± 2.0% (baseline) of the maximal peak amplitude under baseline conditions). Figure 3C shows the dose-dependency of the CBZ effects on INa and demonstrates that INa was also significantly reduced by 30 µM CBZ. Figure 3D shows the steady-state activation and inactivation curves for INa under baseline conditions and in the presence of 100 µM CBZ. While CBZ did not affect the voltage dependency of activation, the voltage dependency of inactivation was significantly shifted to more negative membrane potentials. On average, the negative shift in V1/2 was 6.2 ± 1.3 mV (−90.4 ± 1.8 (CBZ) vs. −84.3 ± 1.0 mV (baseline)), while the slope of the inactivation curve was not significantly different between baseline (−5.0 ± 0.9 mV) and CBZ (−5.4 ± 0.7 mV). Figures 3E,F, show the recovery from inactivation of INa, with in Figure 3E typical INa recordings (bottom) obtained in response to a double-pulse protocol (top) with an interpulse interval of 100 ms, and in Figure 3F the average data with all interpulse intervals tested. CBZ results in a severe delay in the recovery from inactivation. For example, with an interpulse interval of 100 ms, recovery from inactivation was as large as 78.1 ± 4.5% at baseline, but only 15.4 ± 3.1% in the presence of CBZ.
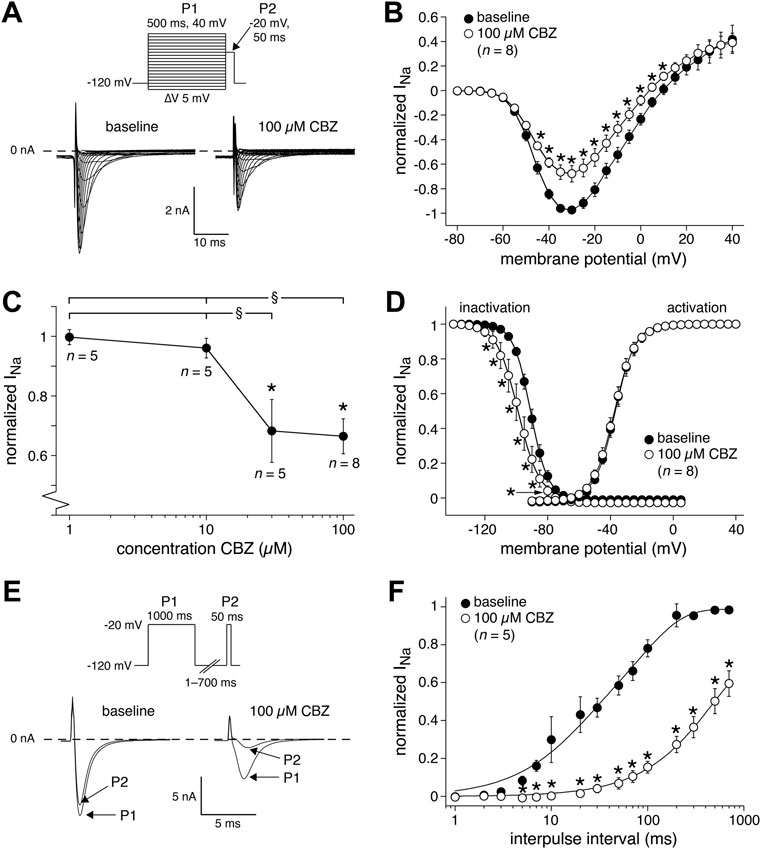
FIGURE 3. Carbamazepine (CBZ) reduces the sodium current (INa) of rabbit ventricular cardiomyocytes in a use-dependent manner. (A) Typical INa recordings between −80 and 0 mV under baseline conditions and in presence of 100 µM CBZ. Inset: double-pulse voltage clamp protocol used to measure current-voltage (I–V) relationships (B) as well as the voltage dependency of (in)activation (D). Cycle length was 5 s. (B) Average I-V relationship of INa under baseline conditions and in presence of 100 µM CBZ. INa was normalized to the maximal peak amplitude under baseline conditions, but peak current was set to −1 to retain the well-known inward direction of INa. *p < 0.05 CBZ versus baseline (Two-Way RM ANOVA). (C) Concentration dependency of the CBZ effects on INa amplitude at −35 mV *p < 0.05 CBZ versus baseline (One-Way RM ANOVA); §p < 0.05 higher versus lower CBZ concentrations (One-Way ANOVA). (D) Voltage dependency of (in)activation. Solid lines are Boltzmann fits to the average data. *p < 0.05 CBZ versus baseline (Two-Way RM ANOVA). (E,F) Recovery from INa inactivation measured with a double-pulse protocol (E, inset). (E) Typical INa recordings under baseline conditions and in presence of 100 µM CBZ with an interpulse interval of 100 ms. (F) Average data. Solid lines are double-exponential fits to the average data. *p < 0.05 CBZ versus baseline (Two-Way RM ANOVA).
Second, we studied the main currents underlying the AP repolarization. Although APD90 was not significantly affected by CBZ, a potential increase (or decrease) in outward currents can be balanced by a similar increase (or decrease) in inward currents, or vice versa. Figure 4A shows typical recordings (at 0 mV) and Figure 4B shows the average I-V relationships of the inward ICa,L under baseline conditions and in the presence of 100 µM CBZ. CBZ significantly decreased the ICa,L density in the voltage range from −20 to +20 mV (Figure 4B). Figure 4C shows that ICa,L was only significantly reduced at the highest concentration of CBZ tested, i.e., 100 µM. The reduction in peak ICa,L at 0 mV was 10.3 ± 3.7% (86.0 ± 3.0% (CBZ) vs. 96.2 ± 3.2% (baseline) of the maximal peak amplitude under baseline conditions). Figure 4D shows typical recordings and Figure 4E shows the average I-V relationships of the steady-state outward K+ currents, IK and IK1, under baseline conditions and in the presence of 100 µM CBZ. Figure 4F shows the concentration dependency of IK and IK1. Neither IK nor IK1 were significantly affected by CBZ. Figure 4G shows typical recordings and Figure 4H shows the average I-V relationships of Ito1 under baseline and 100 µM CBZ conditions. Figure 4I shows the concentration dependency of Ito1. We observed no significant changes in the amplitude of Ito1 at any voltage and concentration tested.
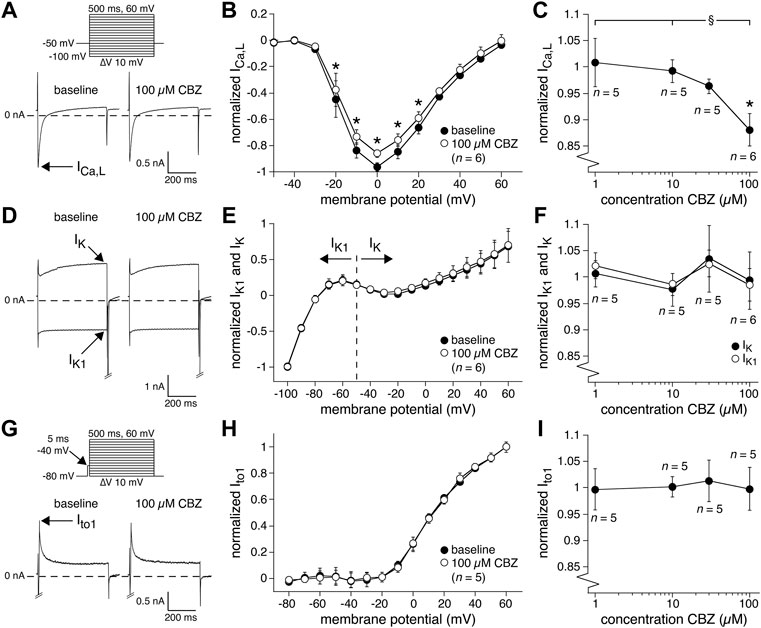
FIGURE 4. Carbamazepine (CBZ) reduces the L-type Ca2+ current of rabbit ventricular cardiomyocytes without affecting K+ currents. (A) Typical recordings of the L-type Ca2+ current (ICa,L) at 0 mV under baseline conditions and in presence of 100 µM CBZ. Inset: voltage clamp protocol used. Cycle length was 2 s. (B) Average I-V relationship of ICa,L under baseline conditions and in presence of 100 µM CBZ. ICa,L was normalized to the maximal peak amplitude under baseline conditions, but peak current was set to −1 to retain the well-known inward direction of ICa,L. *p < 0.05 CBZ versus baseline (Two-Way RM ANOVA). (C) Concentration dependency of the CBZ effect on ICa,L amplitude measured at 0 mV. *p < 0.05 CBZ versus baseline (One-Way RM ANOVA); §p < 0.05 CBZ 100 µM versus lower concentrations (One-Way ANOVA). (D) Typical recordings of the delayed rectifier K+ current (IK; at +60 mV) and inward rectifier K+ current (IK1; at −100 mV) under baseline conditions and in presence of 100 µM CBZ. Voltage clamp protocol as in panel (A). (E) Average I-V relationships of IK and IK1 under baseline conditions and in presence of 100 µM CBZ. The currents were normalized to the current measured at −100 mV (and set to −1) under baseline conditions. (F) Concentration dependency of the CBZ effect on IK1 and IK amplitude measured at −100 mV and +60 mV, respectively. (G) Typical recordings of the transient outward K+ current (Ito1) at +60 mV under baseline conditions and in presence of 100 µM CBZ. Inset: voltage clamp protocol used. Cycle length was 5 s. (H) Average I-V relationships of Ito1 under baseline conditions and in presence of 100 µM CBZ. Ito1 was normalized to the current at +60 mV under baseline conditions. (I) Concentration dependency of the CBZ effect on Ito1 amplitude at +60 mV.
3.4 Effects of Carbamazepine on Action Potentials of Human Atrial Cardiomyocytes
Having established the effects of CBZ on AP properties and membrane current of rabbit cardiomyocytes, we measured the effects of 100 µM CBZ on APs and INa density of freshly isolated human atrial cardiomyocytes to study whether these effects may also occur in the human heart. In patch clamp experiments on single isolated human atrial cardiomyocytes, the amount of quiescent, Ca2+-tolerant cells is typically low and non-depolarized cells are scarce (Verkerk et al., 2021). Here, we selected cardiomyocytes with an RMP of −75 mV or more negative, which generated stable APs after an initial 8–10 min period of continuous pacing at 1 Hz. Figure 5A shows typical APs at 1 Hz under baseline conditions and in the presence of 100 µM CBZ. Average AP parameters are summarized in the top panels of Figure 5B, with the individual (paired) data of the 5 cells tested shown in the bottom panels. Under baseline conditions, the pre-selected human atrial cardiomyocytes had an RMP of −81.9 ± 1.3 mV and a high maximum AP upstroke velocity, and the APs largely overshot the zero potential value. CBZ (100 µM) significantly reduced the AP upstroke velocity and significantly shortened AP duration, without affecting RMP or APA (Figure 5B). These effects are largely comparable to those in rabbit ventricular cardiomyocytes. For example, the AP upstroke velocity decreased significantly by 23.4 ± 6.5% (from 435 ± 58 (baseline) to 328 ± 30 V/s (CBZ)), while the APD90 was significantly decreased by 11.8 ± 3.5% (from 187 ± 49 (control) to 169 ± 51 ms (CBZ)). Furthermore, human APs showed a frequency dependency in maximum AP upstroke velocity with a decrease at higher frequencies (Figure 5C, filled circles). The frequency dependency in the presence of CBZ was more pronounced, indicating a similar use-dependent reduction of INa by CBZ (Figure 5C, open circles) as found in rabbit cardiomyocytes. Figure 5D (top panel), shows the I-V relationships of INa in human atrial cardiomyocytes under baseline conditions and in presence of 100 µM CBZ. CBZ significantly reduced INa density, without changes in V1/2 and k of activation (Figure 5D, bottom panels).
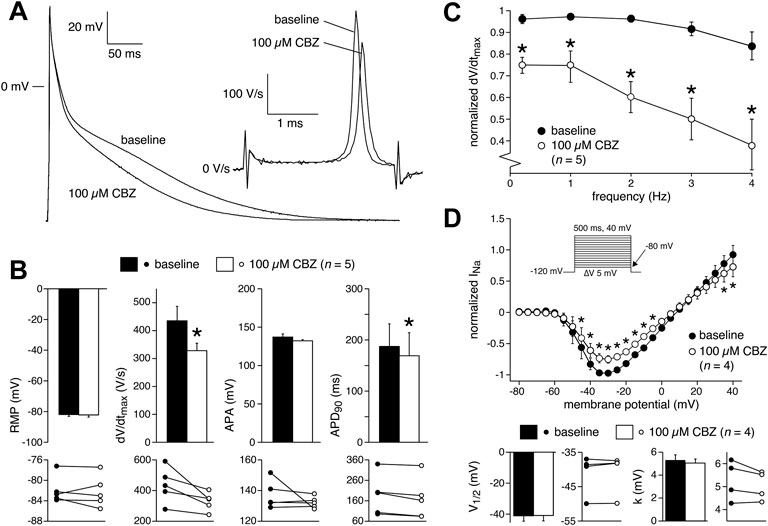
FIGURE 5. Carbamazepine (CBZ) affects human atrial electrophysiology. (A) Superimposed representative human atrial APs at a stimulus frequency of 1 Hz in control conditions and in presence of 100 µM CBZ. Inset: time derivatives during the AP upstroke phase. (B) Average AP characteristics at a stimulus frequency of 1 Hz under baseline conditions and in presence of 100 µM CBZ (top panels) and individual (paired) data points (bottom panels). *p < 0.05 CBZ versus baseline (One-Way RM ANOVA). (C) Average dV/dtmax in response to 100 µM CBZ at stimulus frequencies ranging from 0.2 to 4 Hz. Values are normalized to the highest dV/dtmax measured under baseline conditions. *p < 0.05 CBZ versus baseline (Two-Way RM ANOVA). (D) Average I-V relationship of INa under baseline conditions and in presence of 100 µM CBZ (top panel) and V1/2 and k of activation (bottom panels). Inset: voltage clamp protocol used. Cycle length was 5 s. INa was normalized to the maximal peak amplitude under baseline conditions, but peak current was set to −1 to retain the well-known inward direction of INa. *p < 0.05 CBZ versus baseline (Two-Way RM ANOVA).
4 Discussion
The main findings of the present study are: 1) CBZ use is associated with increased SCA risk in the general population; 2) CBZ reduces cardiac AP upstroke velocity and INa in human and rabbit cardiomyocytes; 3) CBZ results in a tendency to (in rabbit) and significant (in human) cardiac AP shortening and reduces ICa,L, while leaving sarcolemmal potassium currents unaltered. All of the observed effects are consistent with each other: reduction in cardiac AP upstroke velocity is well explained by reduction in INa (Berecki et al., 2010), and may, in turn, lead to reduction in cardiac excitability and conduction velocity of the excitation wavefront in the heart, as represented by CBZ-induced QRS interval prolongation (Leslie et al., 1983). It also facilitates reentrant excitation, VF/VT, and SCA, as shown for the use of class IC antiarrhythmic drugs (potent INa blockers) (Rogers et al., 1989), and in Brugada syndrome (where 20% of patients have an identifiable loss-of-function mutation in SCN5A, the gene that encodes the NaV1.5 α-subunit of the cardiac Na+ channel) (Meregalli et al., 2005). Previous case reports (Table 1) have reported findings that are consistent with these electrophysiological effects of CBZ. Accordingly, we found that CBZ use is associated with a 90% increase in the risk of SCA in the general population. These epidemiological findings are consistent with a previous study by Bardai et al. (2015) on the association of SCA with epilepsy and with the use of CBZ, which was conducted in a smaller patient set (10 cases used CBZ and 26 controls were included) and with less certain SCA ascertainment (no ECG documentation). In our study, we had no information regarding the epilepsy status. Hence, we could not adjust for epilepsy in the epidemiological analysis. This is an important limitation considering that epilepsy is associated with increased SCA risk (Surges et al., 2009). Therefore, our findings from the epidemiological analysis should be interpreted with caution. However, Bardai et al. (2015) found that the AEDs with putative cardiac INa blocking properties such as CBZ are similarly associated with an increased SCA risk. This was not only observed among patients with epilepsy, but also among patients who had no epilepsy (but used AEDs for other indications, e.g., neuralgia). Moreover, the observed association between CBZ and SCA remained unchanged after correction for epilepsy (Bardai et al., 2015). This suggested that the SCA risk associated with CBZ use resulted from the drug effect rather than from suffering epilepsy per se.
Of note, we measured the effects of different concentrations of CBZ (1–100 µM) in vitro, including concentrations corresponding to plasma levels that provide anticonvulsant effects (20–40 µM) (Bertilsson 1978). CBZ displays a high distribution volume, entering the bloodstream from tissue reserves (Charlier et al., 2021), which, together with the fine end-branches of the vasculature of the heart, would make sure that all cardiomyocytes (not only the cells on the surface) are exposed to the compounds in the blood and the extracellular fluid. Thus, the plasma CBZ concentration is a good measure of the concentration of free CBZ “seen” by the cardiomyocytes in the intact heart and in our in vitro experiments. Kennebäck et al. (1995) reported a CBZ plasma concentration of 26.1 ± 5.5 µM (mean ± SD) at a dose of 400 mg/day and 35.6 ± 5.9 μM at 800 mg/day in healthy volunteers. Correspondingly, our study showed that the CBZ-induced reduction of upstroke velocity was present at 10 μM at 2 Hz and faster, and at 30 μM at all pacing frequencies, which is thus within the range of therapeutic concentrations. Our observed reduction of upstroke velocity is consistent with findings in guinea-pig ventricular cardiomyocytes where 75 µM CBZ significantly reduced dV/dtmax at 1 Hz frequency stimulation by ≈ 13% (Delaunois et al., 2015).
We here compared our used CBZ concentrations to plasma concentrations in healthy volunteers. However, as reviewed by Bertilsson (1978), a poor correlation between the prescribed dose and the actual plasma concentration of CBZ is found in epileptic patients. Furthermore, CBZ plasma levels may be affected by several factors, among which age, pregnancy, and pharmacokinetic drug interactions, including interactions with both central nervous system and cardiovascular drugs (Bertilsson 1978; Panday et al., 2017). Consequently, CBZ plasma levels show a considerable inter-individual variability (Bertilsson 1978; Panday et al., 2017). On the one hand, plasma levels can be so low that therapeutic efficacy is lost, while on the other hand the therapeutic range of 4–10 or 4–12 μg/mL (17–42 or 17–51 μM, respectively) is exceeded in a substantial percentage of patients treated with CBZ (Shakya et al., 2008; Al-Balawi et al., 2020; Eroğlu et al., 2021; Grześk et al., 2021), which may have contributed to the observed cardiac arrhythmias of Table 1. Supratherapeutic CBZ plasma levels were found in 4.9% of their patients by Shakya et al. (2008), in 8.6% by Al-Balawi et al. (2020), in 16% by Eroğlu et al. (2021), and in 2.1% by Grześk et al. (2021).
The CBZ-induced changes in upstroke velocity support our epidemiological findings, and suggest that CBZ affects INa (Berecki et al., 2010). Indeed, we found that ≥30 µM CBZ reduced cardiac INa and that it affected various gating properties (hyperpolarizing shift in voltage dependency of inactivation and slower recovery from inactivation). Our finding is supported by previous studies on CBZ’s effects on cardiac and neuronal INa (Kuo et al., 1997; Sun et al., 2007; Sheets et al., 2008; Harmer et al., 2011; Theile and Cummins 2011). For example, Harmer et al. (2011) found an IC50 of 152 µM for NaV1.5 channels expressed in CHO cells, while IC50 values for “brain-type” Na+ channels expressed in HEK293 cells were 2.5 and 1.6 mM for NaV1.3 and NaV1.7 channels in resting state, respectively (Sheets et al., 2008). In resting state, tetrodotoxin-resistant (TTX-R) NaV1.8 channels had an IC50 of 840 µM in dorsal root ganglion cells (Sheets et al., 2008). CBZ-induced shift in voltage dependency of inactivation and slowed recovery of inactivation were also observed for NaV1.3, NaV1.7 and NaV1.8 channels (Sheets et al., 2008; Theile and Cummins 2011). This strengthens the notion that INa block is a plausible contributing mechanism of increased SCA risk associated with CBZ and likely other AEDs with similar cardiac electrophysiological effects. This notion may serve as a basis to adapt clinical procedures for prescription of CBZ with the aim of reducing SCA risk (Benassi et al., 1987). This may be achieved by identifying individuals who are vulnerable to this risk when prescription of INa blocking CBZ is considered. This may be based on identification of the clinical conditions that increase SCA risk in the context of INa block, similar to guidelines regarding the prescription of INa blocking (class IC) antiarrhythmic drugs in case of ischemic heart disease and heart failure (Greenberg et al., 1995). Also, procedures to screen for genetic vulnerability (pharmacogenetics) may be developed (Surges et al., 2009). Finally, at set out above, CBZ levels are affected by several factors and supratherapeutic CBZ levels have been found in a substantial percentage of CBZ users. Therefore, CBZ concentrations need to be closely evaluated (Panday et al., 2017; Charlier et al., 2021).
While INa block is a plausible mechanism underlying the higher SCA risk observed during CBZ use, there is less compelling evidence to support the notion that increased SCA risk results from changes in AP repolarization. We found mild effects of CBZ on AP repolarization as indicated by the tendency to (in rabbit cardiomyocytes) and significant (in human cardiomyocytes) APD90 shortening at 100 µM CBZ, which is above the reported plasma concentrations (Kennebäck et al., 1995). An AP shortening was also observed at 75 µM CBZ in guinea-pig ventricular myocytes at 1 Hz stimulation frequency (Delaunois et al., 2015), but QT intervals, ECG measures of the ventricular AP durations, were not affected by therapeutic doses of CBZ (Arhan et al., 2009; Amin et al., 2010; Dogan et al., 2010; Sathyaprabha et al., 2018). The mild extent of CBZ effects on AP repolarization fits with our voltage clamp experiments. We observed a lack of CBZ effects on the main cardiac repolarizing currents, IK1, IK and Ito1, consistent with previous findings in other tissues and expression systems (Wooltorton and Mathie 1993; Rundfeldt 1997; Kobayashi et al., 2009). CBZ (10–50 µM) had no effect on IK in rat isolated sympathetic neurons (Wooltorton and Mathie 1993) and NG108-15 neuronal cells (Rundfeldt 1997), while it did not affect Kir2.1 currents (Kobayashi et al., 2009), with Kir2.1 as the major Kir isoform of IK1 channels in cardiac myocytes. Although one study reported that CBZ inhibited the IKr tail current, the CBZ dosages used in that study (250–500 µM) were much higher than recommended therapeutic concentrations (Danielsson et al., 2003). We found a mild reduction of the depolarizing current ICa,L at 100 µM. Although it agrees with findings in cultured rat hippocampus neurons (Ambrósio et al., 1999) and rat sensory spinal ganglion cells (Schirrmacher et al., 1995), it is unlikely that such a decrease contributes to the SCA increase and relates to CBZ-induced changes in whole heart parameters, because the reduction is rather small and only observed at 100 μM, which is above the therapeutic plasma concentrations (Kennebäck et al., 1995). It has been demonstrated that CBZ reduced connexin43 expression in cultured cardiomyocytes (Schirrmacher et al., 1995), but more studies are required to determine the exact role of cardiac connexins in the altered ECG parameters and arrhythmias by CBZ use, and our observation of increased SCA.
The effects of CBZ on APs and INa density of freshly isolated human atrial cardiomyocytes were only tested at 100 µM due to the limited availability of Ca2+-tolerant, non-depolarized cells (Verkerk et al., 2021). We used human atrial cardiomyocytes isolated from explanted hearts of patients (with various medications) with end-stage heart failure caused by ischemic cardiomyopathy. Although such cells may be in a diseased state, the main effects of CBZ on those human atrial cardiomyocytes were largely similar to those on ventricular cardiomyocytes of control rabbits, indicating that the effects of CBZ are also present in human conditions. The K+ currents and ICa,L were measured with very general voltage clamp protocols without specific solutions and/or blockers. Although such measurements might also involve small contributions of other membrane currents, the CBZ effects were assessed in paired experiments. In addition, our findings match with CBZ findings on membrane currents in non-cardiomyocytes (Wooltorton and Mathie 1993; Rundfeldt 1997; Ambrósio et al., 1999; Danielsson et al., 2003; Sheets et al., 2008; Kobayashi et al., 2009; Theile and Cummins 2011), indicating that the CBZ effects on these (net) currents were reliably characterized.
5 Conclusion
CBZ reduces cardiac depolarization by reducing INa, and inducing an associated reduction of the AP upstroke velocity, in cardiomyocytes at therapeutic plasma concentrations. CBZ also affects cardiac repolarization, by reducing ICa,L, and an associated reduction of AP duration, but only at relatively high concentrations. These electrophysiological effects may contribute to the found increased SCA risk upon CBZ use in the general population.
Data Availability Statement
The original contributions presented in the study are included in the article/Supplementary Material, further inquiries can be directed to the corresponding author.
Ethics Statement
The studies involving human participants were reviewed and approved by The Medical Ethics Committee of Academic Medical Center Amsterdam. The patients/participants provided their written informed consent to participate in this study. The animal study was reviewed and approved by Institutional Animal Care and Use Committee of the University of Amsterdam.
Author Contributions
HT conceived and designed the study. TE structured and carried out the epidemiological studies. AV structured and designed the patch-clamp studies. LJ and AV carried out the patch-clamp experiments. RW carried out the statistical analysis of the patch-clamp data. LJ and TE drafted the first version of the manuscript. All authors contributed to manuscript revision and approved the final version.
Funding
This work has received funding from the European Union’s Horizon 2020 research and innovation program under acronym ESCAPE-NET, registered under grant agreement No. 733381, and the COST Action PARQ (grant agreement No. CA19137) supported by COST (European Co-operation in Science and Technology), and Chinese Scholarship Council.
Conflict of Interest
The authors declare that the research was conducted in the absence of any commercial or financial relationships that could be construed as a potential conflict of interest.
Publisher’s Note
All claims expressed in this article are solely those of the authors and do not necessarily represent those of their affiliated organizations, or those of the publisher, the editors and the reviewers. Any product that may be evaluated in this article, or claim that may be made by its manufacturer, is not guaranteed or endorsed by the publisher.
Acknowledgments
The authors thank Berend de Jonge for his excellent technical assistance.
Supplementary Material
The Supplementary Material for this article can be found online at: https://www.frontiersin.org/articles/10.3389/fcell.2022.891996/full#supplementary-material
References
Al-Balawi, R. S., Alshehri, M. A., Alatawi, A. S., Al Shehri, A. M., Alshehry, M. A., and Al-Gayyar, M. M. H. (2020). Measuring the Appropriateness of Carbamazepine and Valproic Acid Prescribing and Utilization Using a Newly Implemented Online System in the Tabuk Region of Saudi Arabia. Saudi Pharm. J. 28, 844–849. doi:10.1016/j.jsps.2020.06.008
Ambrósio, A. F., Silva, A. P., Malva, J. O., Soares-da-Silva, P., Carvalho, A. P., and Carvalho, C. M. (1999). Carbamazepine Inhibits L-type Ca2+ Channels in Cultured Rat Hippocampal Neurons Stimulated with Glutamate Receptor Agonists. Neuropharmacology 38, 1349–1359. doi:10.1016/s0028-3908(99)00058-1
Amin, O. S. M., Shwanni, S. S., Noori, S. F., and Hasan, A. M. (2010). Carbamazepine and the QTc Interval: Any Association? Neurol. Asia 15, 119–123. Available at: https://www.neurology-asia.org/. http://www.neurology-asia.org/articles/20102_119.pdf
Antzelevitch, C., and Burashnikov, A. (2011). Overview of Basic Mechanisms of Cardiac Arrhythmia. Card. Electrophysiol. Clin. 3, 23–45. doi:10.1016/j.ccep.2010.10.012
Arhan, E., Ayçiçek, Ş., Akaln, N., Güven, A., and Köse, G. (2009). Cardiac Effects of Carbamazepine Treatment in Childhood Epilepsy. Neurologist 15, 268–273. doi:10.1097/NRL.0b013e31818600a4
Bagal, S. K., Marron, B. E., Owen, R. M., Storer, R. I., and Swain, N. A. (2015). Voltage Gated Sodium Channels as Drug Discovery Targets. Channels 9, 360–366. doi:10.1080/19336950.2015.1079674
Bardai, A., Amin, A. S., Blom, M. T., Bezzina, C. R., Berdowski, J., Langendijk, P. N. J., et al. (2013). Sudden Cardiac Arrest Associated with Use of a Non-Cardiac Drug that Reduces Cardiac Excitability: Evidence from Bench, Bedside, and Community. Eur. Heart J. 34, 1506–1516. doi:10.1093/eurheartj/eht054
Bardai, A., Blom, M. T., Van Noord, C., Verhamme, K. M., Sturkenboom, M. C. J. M., and Tan, H. L. (2015). Sudden Cardiac Death Is Associated Both with Epilepsy and with Use of Antiepileptic Medications. Heart 101, 17–22. doi:10.1136/heartjnl-2014-305664
Beermann, B., Edhag, O., and Vallin, H. (1975). Advanced Heart Block Aggravated by Carbamazepine. Br. Heart J. 37, 668–671. doi:10.1136/hrt.37.6.668
Benassi, E., Bo, G.-P., Cocito, L., Maffini, M., and Loeb, C. (1987). Carbamazepine and Cardiac Conduction Disturbances. Ann. Neurol. 22, 280–281. doi:10.1002/ana.410220217
Berecki, G., Wilders, R., De Jonge, B., Van Ginneken, A. C. G., and Verkerk, A. O. (2010). Re-Evaluation of the Action Potential Upstroke Velocity as a Measure of the Na+ Current in Cardiac Myocytes at Physiological Conditions. PLoS One 5, e15772. doi:10.1371/journal.pone.0015772
Bertilsson, L. (1978). Clinical Pharmacokinetics of Carbamazepine. Clin. Pharmacokinet. 3, 128–143. doi:10.2165/00003088-197803020-00003
Blom, M. T., Van Hoeijen, D. A., Bardai, A., Berdowski, J., Souverein, P. C., De Bruin, M. L., et al. (2014). Genetic, Clinical and Pharmacological Determinants of Out-of-Hospital Cardiac Arrest: Rationale and Outline of the AmsteRdam Resuscitation Studies (ARREST) Registry. Open Heart 1, e000112. doi:10.1136/openhrt-2014-000112
Boesen, F., Andersen, E. B., Jensen, E. K., and Ladefoged, S. D. (1983). Cardiac Conduction Disturbances During Carbamazepine Therapy. Acta Neurol. Scand. 68, 49–52. doi:10.1111/j.1600-0404.1983.tb04814.x
Catterall, W. A. (1999). Molecular Properties of Brain Sodium Channels: An Important Target for Anticonvulsant Drugs. Adv. Neurol. 79, 441–456.
Charlier, B., Coglianese, A., De Rosa, F., De Grazia, U., Operto, F. F., Coppola, G., et al. (2021). The Effect of Plasma Protein Binding on the Therapeutic Monitoring of Antiseizure Medications. Pharmaceutics 13, 1208. doi:10.3390/pharmaceutics13081208
Danielsson, B. R., Lansdell, K., Patmore, L., and Tomson, T. (2005). Effects of the Antiepileptic Drugs Lamotrigine, Topiramate and Gabapentin on hERG Potassium Currents. Epilepsy Res. 63, 17–25. doi:10.1016/j.eplepsyres.2004.10.002
Danielsson, B. R., Lansdell, K., Patmore, L., and Tomson, T. (2003). Phenytoin and Phenobarbital Inhibit Human HERG Potassium Channels. Epilepsy Res. 55, 147–157. doi:10.1016/s0920-1211(03)00119-0
Davies, J. A. (1995). Mechanisms of Action of Antiepileptic Drugs. Seizure 4, 267–271. doi:10.1016/s1059-1311(95)80003-4
Delaunois, A., Colomar, A., Depelchin, B. O., and Cornet, M. (2015). Cardiac Safety of Lacosamide: The Non-Clinical Perspective. Acta Neurol. Scand. 132, 337–345. doi:10.1111/ane.12413
Dogan, E. A., Dogan, U., Yıldız, G. U., Akıllı, H., Genc, E., Genc, B. O., et al. (2010). Evaluation of Cardiac Repolarization Indices in Well-Controlled Partial Epilepsy: 12-Lead ECG Findings. Epilepsy Res. 90, 157–163. doi:10.1016/j.eplepsyres.2010.04.008
Eroğlu, E., Harmanci, N., Yildirim, E., and Sirmagül, B. (2021). Therapeutic Drug Monitoring of Antiepileptic Drugs in Turkey: Five Years’ Experiences. Osman. J. Med. 43, 36–41. doi:10.20515/otd.767494
Eroglu, T. E., Mohr, G. H., Blom, M. T., Verkerk, A. O., Souverein, P. C., Torp-Pedersen, C., et al. (2020). Differential Effects on Out-of-Hospital Cardiac Arrest of Dihydropyridines: Real-World Data from Population-Based Cohorts Across Two European Countries. Eur. Heart J. Cardiovasc. Pharmacother. 6, 347–355. doi:10.1093/ehjcvp/pvz038
Fishman, G. I., Chugh, S. S., Dimarco, J. P., Albert, C. M., Anderson, M. E., Bonow, R. O., et al. (2010). Sudden Cardiac Death Prediction and Prevention. Circulation 122, 2335–2348. doi:10.1161/CIRCULATIONAHA.110.976092
Fozzard, H. A., and Hanck, D. A. (1996). Structure and Function of Voltage-Dependent Sodium Channels: Comparison of Brain II and Cardiac Isoforms. Physiol. Rev. 76, 887–926. doi:10.1152/physrev.1996.76.3.887
Greenberg, H. M., Dwyer, E. M., Hochman, J. S., Steinberg, J. S., Echt, D. S., and Peters, R. W. (1995). Interaction of Ischaemia and Encainide/Flecainide Treatment: A Proposed Mechanism for the Increased Mortality in CAST I. Br. Heart J. 74, 631–635. doi:10.1136/hrt.74.6.631
Grześk, G., Stolarek, W., Kasprzak, M., Grześk, E., Rogowicz, D., Wiciński, M., et al. (2021). Therapeutic Drug Monitoring of Carbamazepine: A 20-Year Observational Study. J. Clin. Med. 10, 5396. doi:10.3390/jcm10225396
Hamilton, D. V. (1978). Carbamazepine and Heart Block. Lancet 311, 1365. doi:10.1016/s0140-6736(78)92442-x
Harmer, A., Valentin, J.-P., and Pollard, C. (2011). On the Relationship Between Block of the Cardiac Na+ Channel and Drug-Induced Prolongation of the QRS Complex. Br. J. Pharmacol. 164, 260–273. doi:10.1111/j.1476-5381.2011.01415.x
Haverkamp, W., Breithardt, G., Camm, A. J., Janse, M. J., Rosen, M. R., Antzelevitch, C., et al. (2000). The Potential for QT Prolongation and Proarrhythmia by Non-Antiarrhythmic Drugs: Clinical and Regulatory Implications. Report on a Policy Conference of the European Society of Cardiology. Eur. Heart J. 21, 1216–1231. doi:10.1053/euhj.2000.2249
Hayashi, M., Shimizu, W., and Albert, C. M. (2015). The Spectrum of Epidemiology Underlying Sudden Cardiac Death. Circ. Res. 116, 1887–1906. doi:10.1161/CIRCRESAHA.116.304521
Heinemann, S. H., Schlief, T., Mori, Y., and Imoto, K. (1994). Molecular Pore Structure of Voltage-Gated Sodium and Calcium Channels. Braz. J. Med. Biol. Res. 27, 2781–2802. Available at: https://www.bjournal.org/
Herzberg, L. (1978). Carbamazepine and Bradycardia. Lancet 311, 1097–1098. doi:10.1016/s0140-6736(78)90940-6
Hojer, J., Malmlund, H.-O., and Berg, A. (1993). Clinical Features in 28 Consecutive Cases of Laboratory Confirmed Massive Poisoning with Carbamazepine Alone. J. Toxicol. Clin. Toxicol. 31, 449–458. doi:10.3109/15563659309000412
Kasarskis, E. J., Kuo, C.-S., Berger, R., and Nelson, K. R. (1992). Carbamazepine-lnduced Cardiac Dysfunction: Characterization of Two Distinct Clinical Syndromes. Arch. Intern. Med. 152, 186–191. doi:10.1001/archinte.1992.00400130184025
Kennebäck, G., Bergfeldt, L., and Tomson, T. (1995). Electrophysiological Evaluation of the Sodium-Channel Blocker Carbamazepine in Healthy Human Subjects. Cardiovasc. Drugs Ther. 9, 709–714. doi:10.1007/BF00878554
Kobayashi, T., Hirai, H., Iino, M., Fuse, I., Mitsumura, K., Washiyama, K., et al. (2009). Inhibitory Effects of the Antiepileptic Drug Ethosuximide on G Protein-Activated Inwardly Rectifying K+ Channels. Neuropharmacology 56, 499–506. doi:10.1016/j.neuropharm.2008.10.003
Koutsampasopoulos, K., Zotos, A., Papamichalis, M., and Papaioannou, K. (2014). Carbamazepine Induced Atrial Tachycardia with Complete AV Block. Hippokratia 18, 185–186. Available at: https://www.hippokratia.gr/ or more specifically. https://www.hippokratia.gr/category/volume-18-2014-issue-2/
Krishnan, S. C., and Antzelevitch, C. (1991). Sodium Channel Block Produces Opposite Electrophysiological Effects in Canine Ventricular Epicardium and Endocardium. Circ. Res. 69, 277–291. doi:10.1161/01.res.69.2.277
Kuiper, J. G., Bakker, M., Penning-Van Beest, F. J. A., and Herings, R. M. C. (2020). Existing Data Sources for Clinical Epidemiology: The PHARMO Database Network. Clin. Epidemiol. 12 12, 415–422. doi:10.2147/CLEP.S247575
Kuo, C.-C., Chen, R.-S., Lu, L., and Chen, R.-C. (1997). Carbamazepine Inhibition of Neuronal Na+ Currents: Quantitative Distinction from Phenytoin and Possible Therapeutic Implications. Mol. Pharmacol. 51, 1077–1083. doi:10.1124/mol.51.6.1077
Lasoń, W., Chlebicka, M., and Rejdak, K. (2013). Research Advances in Basic Mechanisms of Seizures and Antiepileptic Drug Action. Pharmacol. Rep. 65, 787–801. doi:10.1016/s1734-1140(13)71060-0
Leslie, P. J., Heyworth, R., and Prescott, L. F. (1983). Cardiac Complications of Carbamazepine Intoxication: Treatment by Haemoperfusion. Br. Med. J. (Clin. Res. Ed.) 286, 1018. doi:10.1136/bmj.286.6370.1018
Meregalli, P., Wilde, A. A. M., and Tan, H. L. (2005). Pathophysiological Mechanisms of Brugada Syndrome: Depolarization Disorder, Repolarization Disorder, or More? Cardiovasc. Res. 67, 367–378. doi:10.1016/j.cardiores.2005.03.005
Panday, D. R., Panday, K. R., Basnet, M., Kafle, S., Shah, B., and Rauniar, G. P. (2017). Therapeutic Drug Monitoring of Carbamazepine. Int. J. Neurorehabilitation Eng. 4, 245. doi:10.4172/2376-0281.1000245
Pellock, J. M. (2000). Treatment of Epilepsy in the New Millennium. Pharmacotherapy 20, 129S–138S. doi:10.1592/phco.20.12.129s.35252
Ragsdale, D. S., and Avoli, M. (1998). Sodium Channels as Molecular Targets for Antiepileptic Drugs. Brain Res. Rev. 26, 16–28. doi:10.1016/s0165-0173(97)00054-4
Remme, C. A., Verkerk, A. O., Nuyens, D., Van Ginneken, A. C. G., Van Brunschot, S., Belterman, C. N. W., et al. (2006). Overlap Syndrome of Cardiac Sodium Channel Disease in Mice Carrying the Equivalent Mutation of Human SCN5A -1795insD. Circulation 114, 2584–2594. doi:10.1161/CIRCULATIONAHA.106.653949
Rogers, W. J., Epstein, A. E., Arciniegas, J. G., Dailey, S. M., Kay, G. N., Little, R. E., et al. (1989). Preliminary Report: Effect of Encainide and Flecainide on Mortality in a Randomized Trial of Arrhythmia Suppression after Myocardial Infarction. N. Engl. J. Med. 321, 406–412. doi:10.1056/NEJM198908103210629
Rundfeldt, C. (1997). The New Anticonvulsant Retigabine (D-23129) Acts as an Opener of K+ Channels in Neuronal Cells. Eur. J. Pharmacol. 336, 243–249. doi:10.1016/s0014-2999(97)01249-1
Sathyaprabha, T. N., Koot, L. A. M., Hermans, B. H. M., Adoor, M., Sinha, S., Kramer, B. W., et al. (2018). Effects of Chronic Carbamazepine Treatment on the ECG in Patients with Focal Seizures. Clin. Drug Investig. 38, 845–851. doi:10.1007/s40261-018-0677-6
Schirrmacher, K., Mayer, A., Walden, J., Düsing, R., and Bingmann, D. (1995). Effects of Carbamazepine on Membrane Properties of Rat Sensory Spinal Ganglion Cells In Vitro. Eur. Neuropsychopharmacol. 5, 501–507. doi:10.1016/0924-977x(95)80010-y
Schmidt, S., and Schmitz-Buhl, M. (1995). Signs and Symptoms of Carbamazepine Overdose. J. Neurol. 242, 169–173. doi:10.1007/BF00936891
Shakya, G., Malla, S., Shakya, K. N., and Shrestha, R. (2008). Therapeutic Drug Monitoring of Antiepileptic Drugs. J. Nepal Med. Assoc. 47, 94–97. doi:10.31729/jnma.294 Available at: https://www.jnma.com.np/jnma/index.php/jnma/issue/view/41. https://www.chemguide.co.uk/analysis/masspec/howitworks.html
Sheets, P. L., Heers, C., Stoehr, T., and Cummins, T. R. (2008). Differential Block of Sensory Neuronal Voltage-Gated Sodium Channels by Lacosamide [(2R)-2-(acetylamino)-N-Benzyl-3-Methoxypropanamide], Lidocaine, and Carbamazepine. J. Pharmacol. Exp. Ther. 326, 89–99. doi:10.1124/jpet.107.133413
Sills, G. J., and Rogawski, M. A. (2020). Mechanisms of Action of Currently Used Antiseizure Drugs. Neuropharmacology 168, 107966. doi:10.1016/j.neuropharm.2020.107966
Sun, G.-C., Werkman, T. R., Battefeld, A., Clare, J. J., and Wadman, W. J. (2007). Carbamazepine and Topiramate Modulation of Transient and Persistent Sodium Currents Studied in HEK293 Cells Expressing the Nav1.3 α-Subunit. Epilepsia 48, 774–782. doi:10.1111/j.1528-1167.2007.01001.x
Sun, G.-C., Werkman, T. R., and Wadman, W. J. (2006). Kinetic Changes and Modulation by Carbamazepine on Voltage-Gated Sodium Channels in Rat CA1 Neurons after Epilepsy. Acta Pharmacol. Sin. 27, 1537–1546. doi:10.1111/j.1745-7254.2006.00452.x
Surges, R., Thijs, R. D., Tan, H. L., and Sander, J. W. (2009). Sudden Unexpected Death in Epilepsy: Risk Factors and Potential Pathomechanisms. Nat. Rev. Neurol. 5, 492–504. doi:10.1038/nrneurol.2009.118
Theile, J. W., and Cummins, T. R. (2011). Inhibition of Navβ4 Peptide-Mediated Resurgent Sodium Currents in Nav1.7 Channels by Carbamazepine, Riluzole, and Anandamide. Mol. Pharmacol. 80, 724–734. doi:10.1124/mol.111.072751
Verkerk, A. O., Baartscheer, A., De Groot, J. R., Wilders, R., and Coronel, R. (2011). Etiology-Dependency of Ionic Remodeling in Cardiomyopathic Rabbits. Int. J. Cardiol. 148, 154–160. doi:10.1016/j.ijcard.2009.10.047
Verkerk, A. O., Marchal, G. A., Zegers, J. G., Kawasaki, M., Driessen, A. H. G., Remme, C. A., et al. (2021). Patch-Clamp Recordings of Action Potentials from Human Atrial Myocytes: Optimization Through Dynamic Clamp. Front. Pharmacol. 12, 649414. doi:10.3389/fphar.2021.649414
Wong, C. X., Brown, A., Lau, D. H., Chugh, S. S., Albert, C. M., Kalman, J. M., et al. (2019). Epidemiology of Sudden Cardiac Death: Global and Regional Perspectives. Heart Lung Circ. 28, 6–14. doi:10.1016/j.hlc.2018.08.026
Wooltorton, J. R. A., and Mathie, A. (1993). Block of Potassium Currents in Rat Isolated Sympathetic Neurones by Tricyclic Antidepressants and Structurally Related Compounds. Br. J. Pharmacol. 110, 1126–1132. doi:10.1111/j.1476-5381.1993.tb13931.x
Keywords: anti-epileptic drugs, sudden cardiac arrest, risk association, cardiomyocytes, sodium current, action potentials
Citation: Jia L, Eroglu TE, Wilders R, Verkerk AO and Tan HL (2022) Carbamazepine Increases the Risk of Sudden Cardiac Arrest by a Reduction of the Cardiac Sodium Current. Front. Cell Dev. Biol. 10:891996. doi: 10.3389/fcell.2022.891996
Received: 08 March 2022; Accepted: 09 09 May 20222022;
Published: 03 June 2022.
Edited by:
Alessia Remigante, University of Messina, ItalyReviewed by:
Michael Pusch, National Research Council (CNR), ItalyRoberta De Zio, University of Bari Aldo Moro, Italy
Copyright © 2022 Jia, Eroglu, Wilders, Verkerk and Tan. This is an open-access article distributed under the terms of the Creative Commons Attribution License (CC BY). The use, distribution or reproduction in other forums is permitted, provided the original author(s) and the copyright owner(s) are credited and that the original publication in this journal is cited, in accordance with accepted academic practice. No use, distribution or reproduction is permitted which does not comply with these terms.
*Correspondence: Hanno L. Tan, aC5sLnRhbkBhbXN0ZXJkYW11bWMubmw=
†These authors have contributed equally to this work and share first authorship