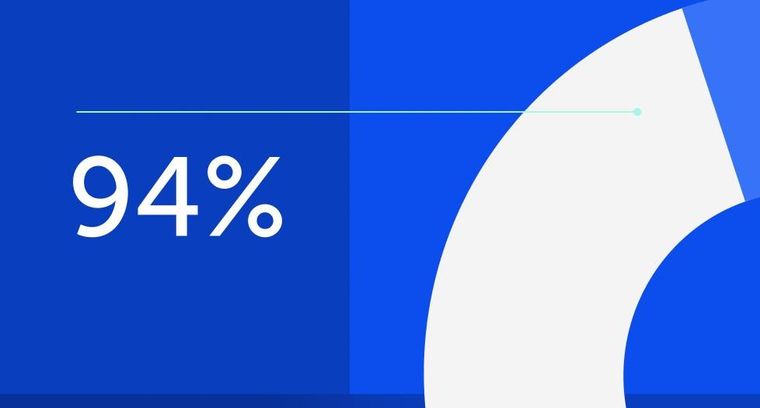
94% of researchers rate our articles as excellent or good
Learn more about the work of our research integrity team to safeguard the quality of each article we publish.
Find out more
ORIGINAL RESEARCH article
Front. Cell Dev. Biol., 23 May 2022
Sec. Stem Cell Research
Volume 10 - 2022 | https://doi.org/10.3389/fcell.2022.890574
This article is part of the Research TopicAdult Stem Cells for Regenerative Medicine: From Cell Fate to Clinical ApplicationsView all 14 articles
Telomerase activity is essential for the self-renewal and potential of embryonic, induced pluripotent, and cancer stem cells, as well as a few somatic stem cells, such as human urine-derived stem cells (USCs). However, it remains unclear how telomerase activity affects the regeneration potential of somatic stem cells. The objective of this study was to determine the regenerative significance of telomerase activity, particularly to retain cell surface marker expression, multipotent differentiation capability, chromosomal stability, and in vivo tumorigenic transformation, in each clonal population of human primary USCs. In total, 117 USC specimens from 10 healthy male adults (25–57 years of age) were obtained. Polymerase chain reaction amplification of a telomeric repeat was used to detect USCs with positive telomerase activity (USCsTA+). A total of 80 USCsTA+ (70.2%) were identified from 117 USC clones, but they were not detected in the paired normal bladder smooth muscle cell and bone marrow stromal cell specimens. In the 20–40 years age group, approximately 75% of USC clones displayed positive telomerase activity, whereas in the 50 years age group, 59.2% of the USC clones expressed positive telomerase activity. USCsTA+ extended to passage 16, underwent 62.0 ± 4.8 population doublings, produced more cells, and were superior for osteogenic, myogenic, and uroepithelial differentiation compared to USCsTA−. Importantly, USCs displayed normal chromosome and no oncological transformation after being implanted in vivo. Overall, as a safe cell source, telomerase-positive USCs have a robust regenerative potential in cell proliferation and multipotent differentiation capacity.
Telomerase activity (TA) is closely related to the longevity of pluripotent stem cells (Huang et al., 2014; Li et al., 2020), embryonic stem cells (ESCs) (Hiyama and Hiyama, 2007), induced pluripotent stem cells (iPSCs) (Wang et al., 2012), and tumor cells (Hiyama and Hiyama, 2007). In normal somatic cells, the activity of telomerase extends telomeric repeats and is usually reduced after birth. Interestingly, TA often cannot be detected in most human mesenchymal stem cells (MSCs) (Zimmermann et al., 2003; Hiyama and Hiyama, 2007), whereas low levels of telomerase are identified in some somatic stem cells from the hematopoietic system (Thongon et al., 2021), intestinal mucosa, and epidermal basal layers (Hiyama and Hiyama, 2007). Human MSCs, such as bone marrow-derived stem cells (BMSCs) (Bernardo et al., 2007; Hiyama and Hiyama, 2007), adipose-derived stem cells (ASCs) (Nava et al., 2015b), and skeletal muscle progenitor cells (SMPCs), often display telomerase negativity, although these stem cells have the MSC phenotype (SH2+, SH3+, SH4+, CD29+, CD44+, CD14−, CD34−, and CD45−) and can differentiate into adipocytes, chondrocytes, and osteoblasts (Zimmermann et al., 2003). A possible reason for the negative telomerase result is the occurrence of alternative lengthening of telomeres (ALT) in MSCs, which is an alternative telomere length-maintaining mechanism (Lafferty-Whyte et al., 2009).
Our previous study was the first to demonstrate that progenitor/stem cells exist in the urine, thus we proposed the name urine-derived stem cells (USCs) (Zhang et al., 2008; Bodin et al., 2010; Wu et al., 2011; Bharadwaj et al., 2013). These cells can be easily isolated from urine samples via a non-invasive approach (Kang et al., 2015), which offers clear advantages over the stem cells harvested from other sources, like bone marrow or adipose aspirates. Clonal USC populations can be readily generated from a single cell by limiting dilution of the starting mixed culture. Each micro-colony will proliferate into a clonal population with many cells (approximately 6.4 × 107 cells) within 4 weeks. Urine collected over 24 h from one individual will generate approximately 140 clones (or 10 clones/200 mL urine), which will expand to greater than 1 × 108 cells by passage three within 3 weeks. Cells do not require tissue dissociation procedures with digestive enzymes, which help to preserve cell viability (Lang et al., 2013). Despite their proliferative capacity, USCs display low expression of gene markers of stem cells (SOX2, OCT3/4, c-MYC, and KLF4) (Bharadwaj et al., 2013) whereas providing a robust proliferative potential reaching up to p16 in a >60-day culture. Understanding the impact of TA on cellular proliferation in vitro and the potential regenerative capacity in vivo is essential to evaluate the suitability of using USCs for tissue repair, disease modeling, and drug development.
It is well known that telomerase activity is critical for the self-renewal or cell proliferation capacity in stem cells, however, the role of TA in multiple differentiation potential is controversial. The aim of this study was to determine the role of TA in maintaining stemness with cell longevity, proliferation capacity, multipotent differentiation potential, cell surface marker expression, karyotype stability, and the risk of in vivo teratoma formation in human primary urine-derived stem cells (USCs).
This study was approval by the Wake Forest University Health Sciences Institutional Review Board. A total of 50 urine samples were collected from 10 healthy male individuals ranging from 25 to 57 years of age and divided into four age groups (20, 30, 40, 50 years of age). A total of 117 USCs clones were isolated, expanded, and characterized as previously described (Zhang et al., 2008; Bodin et al., 2010; Wu et al., 2011). Briefly, USCs were grown in culture media composed of keratinocyte serum-free medium (KSFM) and embryonic fibroblast medium (EFM) mixed at a ratio of 1:1 (Zhang et al., 2008). Only wells in 24 plates that contained single cell were scored and used for further experimentation.
Telomerase activity levels were measured with the Telo TAGGG Telomerase PCR ELISA plus kit (Roche Applied Science, Mannheim, Germany), according to the manufacturer’s recommendations. HEK 293 cells were used as a positive control, and human BMSC and smooth muscle cells (SMC) were used as negative controls. Briefly, 2 × 105 cells at passage two were collected after being trypsinized and washed with cold PBS. Telomerase added the telomeric repeats (TTAGGG) in the kit to the 3’ end of the biotin-labeled synthetic P1-TS primer. These elongated products, and the internal standard (IS) included in the same reaction vessel, were amplified by PCR using the primers P1-TS and the anchor-primer P2. The PCR products were divided into two aliquots, denatured, and hybridized separately to digoxigenin (DIG) labeled detection probes specific for the telomeric repeats and for IS (P3-Std). The resulting products were immobilized via the biotin label onto a streptavidin-coated microplate. Immobilized amplicons were detected with an antibody against digoxigenin that is conjugated to horseradish peroxidase (Anti-DIG-HRP) and the sensitive peroxidase substrate TMB. Absorbance values were measured as the A450nm reading against a blank (reference wavelength A690nm) by using a spectrophotometer. Relative telomerase activity (RTA) within different samples in an experiment were obtained using the following formula (Kim et al., 1994; Kim and Wu, 1997):RTA = [(AS–AS, O)/AS, IS]/[(ATS, 8–ATS8, 0)/ATS8, IS]] × 100.
AS: absorbance of sample; AS, 0: absorbance of heat-treated sample; AS, IS: absorbance of Internal standard (IS) of the sample; ATS8: absorbance of control template (TS8); ATS8, 0: absorbance of lysis buffer; ATS8, IS: absorbance of Internal standard (IS) of the control template (TS8). The kit included the IS and TS8.
To further determine the influence of time on telomerase activity, the RTA of two pairs of USCs (age group 20–50 years), telomerase activity positive (TA+) and telomerase activity negative (TA−), after every five passages or the end passage were measured by the above protocol (Kim et al., 1994).
The USCs in passage three were seeded in 96-well plates at a density of 2,500 cells/well. The culture medium was replaced every second day. Cell proliferation was determined on days 1, 3, 5, and 7 using an MTS cell proliferation assay kit (CellTiter 96® AQueous One Solution Cell Proliferation Assay, Promega) according to the manufacturer’s instructions. Briefly, the MTS reagent was incubated with the cells in the dark for 1 h at 37°C. Following incubation, the absorbance was measured at 490 nm using a spectrophotometer (Molecular Devices Inc., Sunnyvale, CA, United States ). Triplicate measurements were conducted for each time point. The population doubling (PD) and doubling time (DT) calculations were determined based on single USCs at p0 up to the maximum passage (p16) for each clone. The USCs were trypsinized when they reached 70–80% confluence, and the cells were counted manually using a hemocytometer. The PD and DT were calculated using the following formula (Bharadwaj et al., 2011; Bharadwaj et al., 2013):
PD = ln (Nf/Ni)/ln (2) and DT = Ct/PD
Nf is the final number of cells, Ni is the initial number of cells, and Ct is the culture time.
Flow cytometry analysis for the USCs involved staining the USCs with specific labeled anti-human antibodies: CD25-PE, CD31-FITC, CD34-FITC, CD44-FITC, CD45-FITC, CD73-PE, CD90-FITC, CD105-PerCP-CY5.5, CD117-PE, CD140b-PE, and CD146-PE (BD Pharmingen™). Briefly, USCs (p4) were trypsinized, and 5.0 × 105 cells were washed with pre-chilled PBS containing 1% bovine serum albumin (BSA). The fluorescence conjugated antibodies listed above were incubated with USCs on ice for 30 min in the dark. IgG1-PE, IgG1-FITC, IgG2b-FITC, and IgG1-PerCP-CyTM5.5-conjugated isotype control antibodies were used to determine background fluorescence. Cells were washed twice with wash buffer, passed through a 70 µm filter, and analyzed using FACSCalibur™ flow cytometry (BD Biosciences, Franklin Lakes, NJ, United States).
To determine the differentiation capacity difference between USCsTA+ and USCsTA−, cells were subjected to the following induction described below, and their changes in morphology and/or histochemical staining for specific components were recorded.
Smooth muscle cell induction: Three pairs of USCsTA+ and USCsTA− (p3) from different age groups were plated at 2,000 cells/cm2 in smooth muscle differentiation media containing equal amounts of DMEM (high glucose) and EFM with 10% fetal bovine serum (FBS) and 2.5 ng/mL TGF-β1 and 5 ng/mL PDGF-BB (R&D Systems, Minneapolis, MN, United States). Cell morphology was recorded before and after growth factor additions for up to 14 days.
Uroepithelial induction: Three pairs of USCsTA+ and USCs TA− (p3) were plated at 3,000 cells/cm2 in a medium containing equal amounts of KSFM and EFM with 2% FBS and 30 ng/mL EGF (R&D Systems, Minneapolis, MN, United States ) mixed with the conditional medium of urothelial cells (UC, 1:1) for 14 days.
Osteogenic induction: USCs (p3) were seeded at a density of 4,000 cells/cm2 and cultured in serum containing DMEM low-glucose medium with 100 nM dexamethasone, 10 mM β-glycerophosphate and 50 mM ascorbic acid-2-phosphate (Wako Chemicals, Richmond, VA, United States ) for 28 days. The induced cells were harvested after 28 days and fixed in 95% ethanol before histochemical staining. For detection of calcium secreted by the osteogenic-differentiated cells, Alizarin Red S staining was conducted. Briefly, the fixed cells were incubated with 0.5% Alizarin Red S dye (pH 4.1) to sufficiently cover the cell layer for 3–5 mins. Excess dye was removed with distilled water before photo documentation.
Proteins of SMC and UC-induced USCsTA+ and USCs TA− and were extracted using RIPA lysis buffer with a proteinase inhibitor cocktail (Complete mini; Roche Applied Sciences). Protein samples (15–30 µg) were analyzed on a 6–12% SDS-PAGE, and after electrophoresis the proteins were transferred to PVDF membranes (Millipore, Billerica, MA, United States ). The membranes were blocked with skimmed milk and incubated with the primary antibody at an appropriate dilution overnight at 4°C. After secondary antibody incubation, hybridization was detected using the Western Lightning Chemiluminescence reagent (PE, Waltham, MA, United States).
To assess the barrier function of urothelial-differentiated USCsTA+ and USCsTA−, non-induced USCsTA+ and USCsTA−, SMCs, and ureter UCs, these cells were cultured on 0.4 µm Transwell inserts (353,090, Becton Dickinson) placed in 6-well dishes as previously reported (Cross et al., 2005) with minor modifications. Briefly, the inserts were coated with collagen IV (3 µg/cm2) and air-dried in a laminar hood. Cells (1 × 105/cm2) were plated in 1.5 mL of 1 mg/mL tracer-containing medium (FITC-dextran, 4 kDa, Sigma, FD4) in the insert (top chamber) and 3 mL tracer-free medium in the bottom well. Phenol-free medium was used to avoid interference from the indicator in the assay. A day before the tracer was added, the media were supplemented with 2 mM CaCl2 solution. After 3 h, 100 µL media aliquots were removed for fluorescence measurements (excitation at 490 nm and emission at 520 nm). Results were plotted as a percentage relative to the “no cell” control.
To determine the chromosomal stability of cultured USCs, the karyotypes of early (p4) and late passage (TA+ clone at p12, TA− clone at p8) cells were measured (Ge et al., 2011). Cultured cells were trypsinized with a 0.25% Trypsin-EDTA solution, resuspended in hypotonic solution (0.075 M KCl) and then fixed with methanol/acetic acid solution in a 3:1 proportion. The metaphase spread on glass slides was digested by trypsin and then stained with Giemsa stain to generate G bands along each chromosome. Standard cytogenetic analysis was performed under microscopy. Chromosomal image capture and karyotyping were performed using CytoVision®, version 3.7.
Agar assays are often used to distinguish tumor cells from non-transformed or normal cells because normal cells cannot undergo anchorage-independent growth or thrive on an agar substrate. To evaluate whether both USCsTA+ and USCsTA− induce tumorigenicity, USCs were tested on agar gels. HeLa cells and SMC were used as positive and negative controls, respectively. Briefly, 0.35% upper agar layer and 4% base agar layer were prepared in 35 mm tissue-treated dishes. Cells were seeded at the top of the upper agar at a density of 5,000 cells/well. Culture medium was changed twice a week. Cell morphology, proliferation rate, anchorage-independent growth, and cell colony formation were observed under a phase contrast microscope. After culturing for 2 weeks, all the samples were stained with 1 mL of 0.05% nitroblue tetrazolium (NBT) prepared in PBS and sterilized (0.2 micron). This was incubated overnight at 37°C. The cells that took up NBT and showed a violet color were determined to be live cells.
Experiments using nude mice were approved by the Wake Forest University Health Sciences Institutional Animal Care and Use Committee. To further determine the non-tumorigenicity of USCsTA+ in vivo, two USCsTA+ (p5) were implanted in the kidney subcapsular region of NSG (NOD.Cg-Prkdcscid-Il2rgtm1Wjl/SzJ) mice. H9 (human embryonic stem cell line) was used as a positive control. A total of 12 six-week-old female NSG mice (Jackson Labs, Bar Harbor) were used and divided into two groups: USCTA+ (3 mice/clone) and H9 groups (6 mice/group). In total, 2 × 106 cells in 20 µl of PBS were injected into the subcapsular region of the right kidney. After 8 weeks, the mice were sacrificed and bilateral kidneys were fixed in 4% paraformaldehyde, dehydrated, and embedded in paraffin. For evaluation of general graft histology and teratoma formation, routine hematoxylin and eosin (H&E) staining was performed.
A total of 80/117 USC clones (p2) from 10 individuals showed detectable TA (70.2% were USCsTA+). There were no significant differences in the positive rate for TA in the 20–40 years age group: 20 s: 30/39 (76.9%), 30s: 14/19 (73.7%), and 40s: 16/22 (72.7%) represented USC clones, respectively, but the RTA notably decreased to 16/27 (59.2%) in the 50 years age group, as shown in Figure 1 and Table 1. TA could not be detected in human BMSCs at p2.
FIGURE 1. Distribution of human USCsTA+ in different age groups. Most USCs (76.9–72.7%) retained telomerase activity in donors at age 20–40 years of age, but the ratio of USCsTA+ significantly dropped to 59.2% in the donors over 50 years. Cell extracts were prepared from 2 × 105 cells and assayed for telomerase activity, according to the manufacturer’s instructions (Telo TAAGG ELISAPLUS kit Roche). TS8 was provided as a representative positive control lysate prepared from HEK-293 cells. BMSC; human mesenchymal stromal cells of bone marrow, SMC; smooth muscle cells as controls, and A, B, C, D, E, F, G, H, J, K; individual USC clones from different aged healthy individuals (20–50 s), at passage 2 (p2). RTA is the percentage (%) of TS8 (internal positive control) after being normalized.
TABLE 1. The rate, mean, and highest telomerase activity of the isolated USCsTA+ clones for individual donors (p2) in the four different age groups.
To determine if the TA in human USCs decreased as the passage progressed, we measured the TA of two pairs of USCsTA+ and USCsTA− at early passage (p2) and late passages (p7, p11 or the last passage for USCsTA+) from the 20s and 50s groups. The strength of RTA of USCTA+ clones decreased gradually with passage (Figure 2), which seems to make a formerly TA+ clone equivalent to a TA−clone. The RTA remained at undetectable levels through all the passages of USCTA− clones.
FIGURE 2. Strength of telomerase activity declined over passage of cultured USCs. Telomerase activity of USCTA+ clones (A35 and J29) from donors of 20 and 50 years of age were determined in different passages (p2, p7, p11, and the last passage). The relative telomerase activity (RTA) of USCTA+ clones declined gradually in the passages during culture in vitro.
The USCTA+ and USCsTA− paired clones from a single donor were tested in parallel to prevent other factors from affecting the results of the comparison. The USCTA+ clone A35 which was isolated from a 27-year-old male donor, could be passed more than the USCsTA− clone A42 which was isolated from the same donor, and consistently maintained the original “rice-grain like” morphology until they reached cell senescence at p16. Similarly, the USCTA− clones steadily displayed the similar cell morphologic appearances and finally displayed a larger, flattened, and the typical “fried egg” morphology of quiescent cells at p9 (Figure 3).
FIGURE 3. Changes in the morphologic appearance of USCsTA+ and USCsTA− at different passages. A USCTA+ clone (A35, RTA: 45.6, from the donor at 20 years of age) maintained the “rice-grain”-like appearance up to p16; a USCTA- clone (A42, RTA: negative, from the same donor at an age of 20 years of age) showed a flattened cell shape or cells of larger size, and stopped growing (cell senescence) at p9, assessed by phase contrast microscopy. Magnification, ×100.
According to the cell growth curve data (Figure 4), the USCTA+ clones grew more rapidly than the USCTA− clones. Consequently, the PD of USCTA+ clones increased significantly compared to that of the USCTA− clones, regardless of the individual’s age (p < 0.001). The mean DTs of USCTA+ clones were significantly shorter than those of the USCTA− clones (Table 2; Figure 5) (p < 0.001).
FIGURE 4. Cell growth curve of USCsTA+ vs. USCsTA−. Cell proliferation was measured as the USCs were cultured for 7 days. Six individual USCTA+ clones (n = 6, p3, A35, B36, G1, E37, J29, and K5) generated significantly more cells and grew faster than USCTA− clones (n = 6, p3, A42, B31, G12, E34, J18, and K6).
TABLE 2. Population doubling and doubling time of USCTA+ vs USCTA− in the 20 and 50 years age groups in early and late passages to determine if there was a difference in telomerase activity with increasing age.
FIGURE 5. Population doubling, doubling time, and in vitro survival time course of USCsTA+ vs USCsTA−. (A) Population doubling significantly increased for USCsTA+ compared to USCsTA−. (B) Doubling time was significantly shorter in USCsTA+ than in USCsTA−. (C) Cellular senescence in USCsTA− occurred earlier than that in USCsTA+ upon cell expansion. These data indicate that USCsTA+ proliferates more rapidly, generates more cells, and survives longer than USCsTA−. USCs from healthy individuals (n = 12) were cultured following plating at a single cell/well. Six individual USCTA+ clones (n = 6, p3, A35, B36, G1, E37, J29, and K5) generated significantly more cells and grew faster than USCTA− clones (n = 6, p3, A42, B31, G12, E34, J18, and K6). USCTA+ clones survived longer and had longer population doubling times. The suffixes A, B, G, C, H, D, E, F, J, and K represent the ten healthy individuals who participated in the study.
However, the relative TA of USCs was not completely coordinated with the cell proliferation capacity (PD, DT, and passage) (Table 2).
To identify the cell surface markers of USC clones, six pairs of USCsTA+ and USCsTA− were subjected to flow cytometry analysis. All the USC clones showed strong positive MSCs markers including CD44, CD90, CD73, CD105, and CD146 and were negative for hematopoietic stem cell markers including CD25, CD31, CD34, CD45, and CD117 (Figure 6; Table 3). However, there was no significant difference in the CD105 expression between USCsTA+ and USCsTA−.
FIGURE 6. USCs expressing mesenchymal stem cell surface markers. USCTA+ clones (A35, B36, G1, E37, J29, and K5) and USCTA− clones (A42, B31, G12, E34, J18, and K6) both displayed sets of mesenchymal stem cell (MSC) surface markers (CD44, CD90, CD73, CD105, and CD146), positive at similar levels, whereas both USCsTA+ and USCsTA− did not express sets of haemopoietic stem cell markers (i.e., CD31, CD34, CD45, CD25, and CD117). However, three of five USCsTA+ displayed CD105 expression, but no or weak expression in all the four USCTA− cell clones. MSC surface markers of USCs at p4 were detected via flow cytometry.
TABLE 3. Cell surface markers of USCTA+ and USCTA− clones at passage four detected using fluorescence-activated cell sorting.
The USCTA+ and USCsTA− paired clones from a single donor were tested in parallel to prevent other factors from affecting the results of the comparison. To test the difference in differentiation capability between USCsTA+ and USCsTA−, both USCsTA+ and USCsTA− (p3) were induced to osteogenic, myogenic, and uroepithelial differentiation (Table 4). Both the USCsTA+ clone A35 that was isolated from a 27 year-old male donor, and the USCsTA− clone A42 that was isolated from the same donor (p3) differentiated into the smooth muscle lineage and the urothelial lineage (Figures 7A,B). Urothelial-differentiated cells developed a cobblestone-like morphology (Figure 7Ai) and expressed the urothelial-specific proteins uroplakin-Ia and uroplakin-III and the generic epithelial cell markers CK7, CK13, and AE1/AE3 (Figure 7Aii). Furthermore, the expression of these proteins was significantly higher in the UC-induced USCsTA+ clones [A35 and J29 (from another male aged 55 years)] than in the UC-induced USCsTA− clones (A42 and J18 [from the same male aged 55 years as clone J29]) and uninduced USC clones. In an assay of cellular barrier function, the UC-induced USCsTA+ clone-A35 showed increased expression of specific tight junction protein markers (E-cadherin) compared to the UC-induced USCsTA−clone-A42 and uninduced USC clones (Figure 7Ai). In barrier function assays, both the urothelial-differentiated USCs (USCTA+ 41.0% ± 1.7% and USCTA− 44.8% ± 0.4%) showed significant reduction in leakage of fluorescent tracer through the insert in vitro (p < 0.01 and p < 0.001, respectively), which means lower permeability and higher tight junction property, compared to the non-differentiated USCs (USCTA+ 63.1% ± 4.3%, USCTA− 62.2% ± 1.1%) (Figure 7Aiii) at day 3; and similar results received at day 7 (p < 0.01), the leakage percentage of urothelial-differentiated USCs (USCTA+-A35 35.5% ± 1.2% and USCTA−-A42 38.9% ± 4.3%), less than the non-differentiated USCs (USCTA+-A35 54.8% ± 2.6%, USCTA−-A42 59.6% ± 0.5%). However, there was only a slight difference in leakage protection at day 3 between the UC-induced USCTA+-A35 and USCTA−-A42 clones (p < 0.05). In addition, no significant differences between them, although reduce in leakage, were noted in UC-induced USCTA+-A35 clone on day 7.
FIGURE 7. USCs undergo multi-potential differentiation in vitro. (A). Morphology of USCs after induction with USC induction media for 14 days. Cell morphology changed from “rice-grain-like” to a cuboidal morphology appearance. Human ureter urothelial cells (UC) were included as a positive control. Urothelial-specific proteins (Uroplakin-Ia, -III, AE1/AE3, E-cadherin, Cytokeratin 7, and Cytokeratin 13) were detected via western blotting. Specific signals (bands) were observed in lanes with proteins from induced USCsTA+ and UCs. Barrier function analysis was performed on both USCsTA+ and USCsTA− differentiated to UC-like cells for 3 and 7 days. FITC-dextran was incubated with cells grown on the insert, and media in the bottom chamber was analyzed after 3 h. Results were plotted as a percentage relative to the “no cell” control. Positive leakage prevention was observed in both urothelial-induced USCsTA+ and USCsTA− when compared to the negative control SMCs. (B) Morphology of USCs after induction with SMC induction media for 14 days. Cells were spindle-shaped after induction. Human ureter SMCs served as the positive control. Smooth muscle-specific proteins (calponin and smoothelin) were detected via immunoblotting. Specific signals (bands) were observed in lanes with proteins from the induced USCsTA+ and SMC control. (C) USCs cultured in osteogenic differentiation media were evaluated for osteogenic cell lineages. Osteogenic differentiation-day 21, positive von Kossa staining for bone minerals was observed in the induced USCsTA+ and USCsTA− D). Summary of the differentiation capacity of USCTA+ vs USCTA− clones. A35-clone (USCsTA+, RTA: 45.60) and A42 clone (USCsTA−, RTA: negative) were both isolated from one donor (male, 27 year-old); J29 (USCsTA+, RTA:46.6) and J18 (USCsTA−, RTA: negative) were isolated from another donor (male, 55 year-old).
Both myogenic differentiated USCsTA+ and USCsTA− became elongated and spindle-shaped (Figure 7Bi) and expressed smoothelin, a smooth muscle-specific protein marker, and calponin (Figure 7Bii). Moreover, the expression of smoothelin and calponin of SMC- differentiated USCsTA+-A35 were higher than the SMC-differentiated USCsTA−-A42 confirmed by western blotting (Figure 7Bii). Finally, both USCsTA+-A35 and USCsTA−-A42 were also induced to differentiate into the osteogenic lineage using our previous protocols. USCsTA+-A35 could be induced to osteocytes but not USCsTA−-A42, as confirmed by Alizarin S Red staining (Figure 7C). Moreover, both USCsTA+-A35 and USCsTA−-A42 were difficult to differentiate into adipocytes, as evidenced by Oil Red-O staining (data not shown).
To investigate the potential susceptibility of USCsTA+ to malignant transformation, cells were tested via cytogenetic analysis, agar culture in vitro, and teratoma formation in vivo, and the results were compared to those of USCsTA− and controls.
Both TA+ and TA- USC clones in the early (p4) and late passage (USCsTA+-A35, J29 clone in p12 and USCsTA−-A42, J18 clone at p8) displayed a normal karyotype of 1 X and 1 Y chromosome, as expected for a male donor, and a normal diploid (2n = 44) complement of autosomes and a pair of sex chromosomes (Figure 8A; Table 5). No multiploidy or obvious chromosomal rearrangements in metaphase were detected by Giemsa bandings at p4 or late passage of both USC clones.
FIGURE 8. Spontaneous transformation assays of USCTA+ clones. (A) Karyotypes of USCTA+ vs. USCTA− clones. Both USCTA+ (n = 2, A35, RTA:45.6; and J29, RTA:46.6) and USCTA− (n = 2, A42 and J18) clones in the early passage (p4) and late passage (TA + clone in p12, TA-clone at p8) displayed normal complement of diploid (2n = 46). (B) In vitro agar assay of USCs. The size of the cell clones (seeded at a density of 5,000 cells/well) of USCTA+ clones (A35 and J29) and USCTA− clones (A42 and J18) remained the same between days 1 and 14 after plating on soft agar gel. However, clone size of the cancer cell line HeLa cells, as the positive control, at 14 days were significantly increased compared to that at the first day. Normal bladder smooth muscle cell clones were used as the negative control. Images were captured using a phase contrast microscope. Cell clones were stained with nitro blue tetrazolium and photographed using bright field soft agar assays for anchorage-independent cell growth of USCTA+ in vitro. (C) In vitro transformation assay of USCs. No teratoma formation was observed under the microscope 2 months after USCTA+ (two million cells/graft, n = 2, white arrow) were implanted into the capsules of the kidneys of NSG mice. There was no teratoma observable grossly (i, ii) or microscopically (iii, iv). In contrast, H9 cells as the positive control formed derivatives of all the three embryonic germ layers (v, vi). A35-clone (USCsTA+, RTA: 45.60) and A42 clone (USCsTA−, RTA: negative) were both isolated from one donor (male, 27 year-old); J29 (USCsTA+, RTA:46.6) and J18 (USCsTA−, RTA: negative) were isolated from another donor (male, 55 year-old).
TABLE 5. Conventional karyotypes of USCTA+ and USCTA- clones in the 20- and 50-years age groups at early and late passages.
USCTA+ clones-A35, J29 remained the same size on day 14 as that on day 1, after being cultured in soft agar, like colonies of SMCs. However, HeLa cancer cells formed large colonies on day 14 (Figure 8Bi). These single cells (SMC and USC) or colonies (HeLa cancer cells) were viable, which was confirmed by NBT staining (Figure 8Bii). Furthermore, no teratoma was formed when all USCsTA+-A35 were implanted in the subcapsular region of the kidney of NSG mice after 8 weeks. All human ES cell lines (H9) formed derivatives of the three embryonic germ layers (Figure 8C).
We characterized the stemness features of human USCs, including long-term survival with self-renewal capacity, multi-lineage differentiation, MSCs surface markers, expression of telomere maintenance mechanisms (TA) in in vitro culture time frames, and capacity to form teratomas. USC clones in the same individual urine sample displayed telomere heterogeneity, which could be due to USCs at different stages of the telomerase activation processes.
Stemness refers to the molecular processes underlying the fundamental stem cell properties of self-renewal and the generation of differentiated daughter cells. The stemness properties of adult-derived stem cells decline after birth, compared to those of ESCs (Hofmeister et al., 2015). Most human somatic or stem cells do not express OCT4/SOX2/KLF4/MYC. Forced expression of OCT4/SOX2/KLF4/MYC in somatic cells such as fibroblasts can reprogram cells to a pluripotent stem cell fate. USCs are multipotent, rather than pluripotent, and express low levels of OCT4/SOX2/KLF4/MYC (Bharadwaj et al., 2013). Our previous studies demonstrated that USCs possess limited stemness properties including self-renewal and multiple differentiation capacity but do not induce teratoma formation. This is different from iPSC that have higher expression levels of OCT4/SOX2/KLF4/MYC and form teratoma. In summary, USCs are multipotent and thus do not express the higher levels of OCT4/SOX2/KLF4/MYC that are observed in pluripotent stem cells.
Two methods are predominantly used to track the in vitro age of a cell culture or cell proliferation capacity. 1) The passage number implies the number of times a cell has been passaged, which is most commonly used in the laboratory. However, the cell passage number is imprecise because different laboratories may use different initial cell seeding densities, which affects the number of times cells divide in culture. 2) The PD indicate the number of cell generations the cell lineage has undergone—the number of times the cell population has doubled. PD of primary cells is a better practice for reporting cellular age in vitro, which is often used to set an acceptable upper limit for cell production, or the maximum number of cells generated in culture. In this study, we used both terms (passage number and PD number) to present cell lifespan, and there was good agreement between the two measures. USCsTA+ with higher TA could reach higher PD number or passage number, a shorter DT which indicates faster cell division or more rapid cell growth, and better proliferation capacity which indicates more cells generated than those of USCsTA+ with lower TA strength and USCsTA−. Furthermore, the TA of USCsTA+ declined with passage; thus, the proliferation potential with PD and DT of USCs gradually decreased, with the cells finally reaching senescence within 8 weeks in 2D culture and 10 weeks in 3D culture models (data not shown).
Telomerase is activated and maintains cellular immortality in ESCs or iPSCs, which plays an import role such as to protect the genome from nucleolytic degradation, unnecessary recombination, repair, and intrachromosomal fusion (Hiyama and Hiyama, 2007); however, the level of TA is low or absent in most MSCs and ASCs (Zimmermann et al., 2003; Hiyama and Hiyama, 2007) regardless of their proliferative capacity. Numbers of BMSCs are low in bone marrow nucleated cells with a frequency of colony-forming unit-fibroblasts (CFU-F) of 1: 35,700 (Lu et al., 2006). In addition, small amounts of stem cells are mixed with a large amount of stromal cells in the bone marrow, which makes it challenging to isolate true stem cells and measure their levels of TA. In contrast, USCs start with a single stem cell that forms cell clones and expands to a large number of stem cells of which most display TA. There are a couple of studies comparing hUSC to hBMSC (Sun et al., 2021) and ASC (Kang et al., 2015). In in vitro experiments, hUSC presented with better capacity for proliferation than hBMSC, while hBMSC had greater chondrogenic ability than hUSC. However, hUSC and hBMSC had similar cartilage repair effects in vivo. Results indicated that hUSC can be a stem cell alternative for cartilage regeneration, provide a powerful platform for cartilage tissue engineering, and clinical transformation (Sun et al., 2021). Similar outcomes are achieved in studies of comparison between hUSC and hASC (Kang et al., 2015). TA levels can be detected or consistently expressed in most human USCs (>70%) obtained from healthy middle-aged donors, although levels of this enzyme were lower than those of ESC. Most USCs express TA however, along with the aging process in individuals, a reduction in USC regenerative capacity occurs in the 50s age group, which also means a decrease in cell proliferation capacity along with the reduced number of USCsTA+ (59%), and relatively lower PD and DT and a decline in telomere reserve with associated lower TA. Furthermore, the number of USCsTA+ declined with increasing passage during cell proliferation. Thus, the levels of TA decreased with an increase in donor age and cell passage but did not show an increase again after long-term culture, demonstrating the safety of USC implantation with retaining chromosome stable and no oncological transformation. The TA of USCsTA+ declined with passage in vitro or throughout individual age, providing a mechanism that restricts cell over-proliferation and any tumor development.
Human MSCs as a heterogeneous cell population are confirmed by a set of cell surface makers, instead of a single marker. MSCs often maintain their immunophenotypic characteristics stably throughout the culture term. The ASCs did not show immunophenotypic alterations with passage and retained a consistently high expression level of MSC surface markers and were negative for HSC at early (p4) and late passage (p8) markers (Nava et al., 2015a). In this study, we observed there were USCs at different stages of activation. One was the activating USCs with positive telomerase activity; one is activated cells (relatively old) with negative TA; but both USCsTA+ and USCsTA− in p4 showed the most MSC surface markers (CD44, CD73, CD90, CD105, CD146, and HLA-A, B, C) and lacked hematopoietic markers (CD25. CD31, CD34, CD45, HLA-DR/DP/DQ, and HLA-G). Our data demonstrated that USCs are a good starting population because of their lack of immunological reactivity. Importantly, through the most exhaustive head-to-head characterization of multiple clones that each start a single USC clonal population from multiple donors, these results allow us to highlight the intrinsic differences between commonly used starting cell sources.
In addition, CD105 is one unique marker associated with differentiation potential (Dominici et al., 2006). For example, CD105+ ASCs were more prone to differentiation into chondrocytes than CD105− ASCs (Ishimura et al., 2008; Jiang et al., 2010). CD105+ MSCs were also more efficient in the infarcted heart (Gaebel et al., 2011), with stronger proliferative and colony formation abilities than CD105− MSCs (Lv et al., 2012). However, CD105− ASCs were more osteogenic (Jiang et al., 2010) and showed strong immunomodulation capacity (Anderson et al., 2013). Interestingly, five of the six USCTA+ clones strongly expressed CD105, and four of the six USCTA− clones either did not express CD105 or were weakly positive. Compared to other CD markers, CD105+ appeared to correlate with stemness. The correlation between CD105+ USC and USCTA+ of stem cells appears to have different capabilities. It is worth further investigation into the mechanism of correlation in both TA and CD105 marker cells with such different capabilities.
Telomerase and telomeres are strongly associated with cell renewal and proliferation, but it is controversial whether telomerase is associated with cell differentiation in MSCs (Zimmermann et al., 2003; Hiyama and Hiyama, 2007). The epigenetic nature of telomeres appears to depend on different human cell linages (Dogan and Forsyth, 2021). In adult human stem cells, both BMSCs and ASCs lack telomerase, but can retain their functional characteristics and multiple differentiation potential (Zimmermann et al., 2003; Hiyama and Hiyama, 2007). Interestingly, overexpression of telomerase resulted in telomere elongation, and TERT-transfected cells continued to proliferate and formed bone in vivo (Simonsen et al., 2002). However, mouse MSCs with their TA knocked down failed to differentiate into adipocytes or chondrocytes, even at early passage (Liu et al., 2004). Similarly, increased TA enhanced self-renewal ability, proliferation, and differentiation efficiency in TERT-overexpressing ES cells (Armstrong et al., 2005). High TA or the expression of TERT can therefore be regarded as a marker of undifferentiated ES cells. Downregulation of TA in differentiating EC cells was reported to be closely correlated with histone deacetylation and DNA methylation of the TERT gene (Lopatina et al., 2003). In this study, USCsTA+ performed better in terms of multiple differentiation capacity in osteogenic, myogenic, and uroepithelial differentiation than USCsTA-, indicating that telomerase is required for not only cell proliferation but also multiple differentiation in human USCs, which is a guarantee for future studies to determine whether USCTA+ induce better in vivo tissue regeneration than USCTA−.
As telomerase is related to both normal stem cells and tumor stem cells, it is necessary to determine the alteration of karyotypes, with the in vitro agar assay and the in vivo risk of tumor formation of telomerase-positive cells for their safe transplantation application. Giemsa-based chromosomal banding and staining techniques are important for cytogenetics. USCs maintain a normal diploid chromosome recognized as 46 during long-term culture or overexpansion for up to p16 or 68 PD (268). Cytogenetic analyses showed USCs can safely expand in vitro (p4, p8, and p16) with no sign of immortalization or development of chromosomal abnormalities.
Cloning techniques with semisolid medium, such as agar gel for evaluating cell growth, are commonly used to study the biology of cancer cells due to their anchorage-independent growth requirements. The ability of cancer cells to proliferate without firm attachment (i.e., anchorage independence) is one of the best in vitro indicators of tumorigenicity. Importantly, USCsTA+ do not present a propensity for spontaneous oncogenic transformation 60 days after in vivo implantation. The tumorigenic potential of USCsTA+ was not found in vitro or in vivo. Thus, USCs as a new source of seed cells, which are non-invasive, highly proliferative, and abundant, can be used for tissue engineering and regenerative medicine.
TA appears to be related to the stemness of USCs (Table 6). USCsTA+ survived for a significantly longer period with intact morphological appearance, rapid proliferation, and ample cell generation, and possessed more potent differentiation capacity than USCsTA−. Both human USCsTA+ and USCsTA− can be safely expanded in vitro maintaining normal karyotype and showed tumor-free formation after in vivo implantation, which makes them appropriate sources for cell-based therapies. Thus, TA could be an independent predictive factor for the regenerative capacity of USCs. In addition, human USCsTA+ provides sufficient cell numbers for drug testing. TA is a good indicator of stemness (cell renewal and differentiation potential) of human adult stem cells, but a collection of primary cultured cells is required, which is cumbersome. Therefore, simpler and low-cost methods of measuring TA and telomeres in stem cells are highly desired.
This study demonstrated that human primary urinary stem cells with positive TA act as a distinct subpopulation with potential regeneration capacity in both cell proliferation and multiple differentiation. USCsTA+ can more efficiently give rise to osteogenic, skeletal myogenic, smooth muscle, and urothelial cell lines than USCsTA−. Importantly, despite that USCs display TA, they do not form teratoma, which provides a safe cell source for clinical application. In addition, the number of USCsTA+ decline with increasing age. Future investigations should focus on understanding the role that physiological factors play in regulating both the temporal pattern of USCsTA+ and their influence on the ability of these cells to participate in better tissue repair. Determining the requirements for the effect of TA on the paracrine effect of USC has important implications for understanding the anti-inflammatory, fibrosis inhibition, and redox effect of USCsTA+. It will be beneficial to better understand alterations in this cell subpopulation throughout the human lifespan, and how they translate into, aging, renal dysfunction, drug-induced nephrotoxicity, or cancer, among others.
The original contributions presented in the study are included in the article/Supplementary Material, further inquiries can be directed to the corresponding author.
The studies involving human participants were reviewed and approved by the All human tissue samples were approved Human urine-derived stem cells (USC): All human tissue samples were approved for acquisition by the Wake Forest University Institutional Review Board. The patients/participants provided their written informed consent to participate in this study. The animal study was reviewed and approved by Experiments using nude mice were approved by the Wake Forest University Health Sciences Institutional Animal Care and Use Committee.
YZ supervision; YS and GL analyzed the data; YS, GL, RW, DM, XS, JM, XG, and AA contributed materials, reagents, analytic tools; YS, GL, and YZ wrote the original manuscript. All authors have read, edited, and approved the manuscript for publication.
This work is partially supported by Research Grants from the National Institutes of Health NIDDK (R21DK071791) (R56DK100669), NIAID (R21AI152832), and (R03AI165170) (YZ).
The authors declare that the research was conducted in the absence of any commercial or financial relationships that could be construed as a potential conflict of interest.
The handling editor JL declared a shared parent affiliation with the authors GL, RW at the time of review
All claims expressed in this article are solely those of the authors and do not necessarily represent those of their affiliated organizations, or those of the publisher, the editors and the reviewers. Any product that may be evaluated in this article, or claim that may be made by its manufacturer, is not guaranteed or endorsed by the publisher.
Anderson, P., Carrillo-Gálvez, A. B., García-Pérez, A., Cobo, M., and Martín, F. (2013). CD105 (Endoglin)-negative Murine Mesenchymal Stromal Cells Define a New Multipotent Subpopulation with Distinct Differentiation and Immunomodulatory Capacities. PLoS One 8, e76979. doi:10.1371/journal.pone.0076979
Armstrong, L., Saretzki, G., Peters, H., Wappler, I., Evans, J., Hole, N., et al. (2005). Overexpression of Telomerase Confers Growth Advantage, Stress Resistance, and Enhanced Differentiation of ESCs toward the Hematopoietic Lineage. Stem Cells 23, 516–529. doi:10.1634/stemcells.2004-0269
Bernardo, M. E., Zaffaroni, N., Novara, F., Cometa, A. M., Avanzini, M. A., Moretta, A., et al. (2007). Human Bone Marrow-Derived Mesenchymal Stem Cells Do Not Undergo Transformation after Long-Term In Vitro Culture and Do Not Exhibit Telomere Maintenance Mechanisms. Cancer Res. 67, 9142–9149. doi:10.1158/0008-5472.can-06-4690
Bharadwaj, S., Liu, G., Shi, Y., Markert, C., Andersson, K.-E., Atala, A., et al. (2011). Characterization of Urine-Derived Stem Cells Obtained from Upper Urinary Tract for Use in Cell-Based Urological Tissue Engineering. Tissue Eng. Part A 17, 2123–2132. doi:10.1089/ten.tea.2010.0637
Bharadwaj, S., Liu, G., Shi, Y., Wu, R., Yang, B., He, T., et al. (2013). Multipotential Differentiation of Human Urine-Derived Stem Cells: Potential for Therapeutic Applications in Urology. Stem Cells 31, 1840–1856. doi:10.1002/stem.1424
Bodin, A., Bharadwaj, S., Wu, S., Gatenholm, P., Atala, A., and Zhang, Y. (2010). Tissue-engineered Conduit Using Urine-Derived Stem Cells Seeded Bacterial Cellulose Polymer in Urinary Reconstruction and Diversion. Biomaterials 31, 8889–8901. doi:10.1016/j.biomaterials.2010.07.108
Cross, W. R., Eardley, I., Leese, H. J., and Southgate, J. (2005). A Biomimetic Tissue from Cultured Normal Human Urothelial Cells: Analysis of Physiological Function. Am. J. Physiology-Renal Physiology 289, F459–F468. doi:10.1152/ajprenal.00040.2005
Dogan, F., and Forsyth, N. R. (2021). Epigenetic Features in Regulation of Telomeres and Telomerase in Stem Cells. Emerg. Top. Life Sci. 5, 497–505. doi:10.1042/etls20200344
Dominici, M., Le Blanc, K., Mueller, I., Slaper-Cortenbach, I., Marini, F. C., Krause, D. S., et al. (2006). Minimal Criteria for Defining Multipotent Mesenchymal Stromal Cells. The International Society for Cellular Therapy Position Statement. Cytotherapy 8, 315–317. doi:10.1080/14653240600855905
Gaebel, R., Furlani, D., Sorg, H., Polchow, B., Frank, J., Bieback, K., et al. (2011). Cell Origin of Human Mesenchymal Stem Cells Determines a Different Healing Performance in Cardiac Regeneration. PLoS One 6, e15652. doi:10.1371/journal.pone.0015652
Ge, J., Cai, H., and Tan, W. S. (2011). Chromosomal Stability during Ex Vivo Expansion of UCB CD34+ Cells. Cell Prolif. 44, 550–557. doi:10.1111/j.1365-2184.2011.00779.x
Hiyama, E., and Hiyama, K. (2007). Telomere and Telomerase in Stem Cells. Br. J. Cancer 96, 1020–1024. doi:10.1038/sj.bjc.6603671
Hofmeister, L. H., Costa, L., Balikov, D. A., Crowder, S. W., Terekhov, A., Sung, H.-J., et al. (2015). Patterned Polymer Matrix Promotes Stemness and Cell-Cell Interaction of Adult Stem Cells. J. Biol. Eng. 9, 18. doi:10.1186/s13036-015-0016-x
Huang, Y., Liang, P., Liu, D., Huang, J., and Songyang, Z. (2014). Telomere Regulation in Pluripotent Stem Cells. Protein Cell 5, 194–202. doi:10.1007/s13238-014-0028-1
Ishimura, D., Yamamoto, N., Tajima, K., Ohno, A., Yamamoto, Y., Washimi, O., et al. (2008). Differentiation of Adipose-Derived Stromal Vascular Fraction Culture Cells into Chondrocytes Using the Method of Cell Sorting with a Mesenchymal Stem Cell Marker. Tohoku J. Exp. Med. 216, 149–156. doi:10.1620/tjem.216.149
Jiang, T., Liu, W., Lv, X., Sun, H., Zhang, L., Liu, Y., et al. (2010). Potent In Vitro Chondrogenesis of CD105 Enriched Human Adipose-Derived Stem Cells. Biomaterials 31, 3564–3571. doi:10.1016/j.biomaterials.2010.01.050
Kang, H. S., Choi, S. H., Kim, B. S., Choi, J. Y., Park, G.-B., Kwon, T. G., et al. (2015). Advanced Properties of Urine Derived Stem Cells Compared to Adipose Tissue Derived Stem Cells in Terms of Cell Proliferation, Immune Modulation and Multi Differentiation. J. Korean Med. Sci. 30, 1764–1776. doi:10.3346/jkms.2015.30.12.1764
Kim, N. W., Piatyszek, M. A., Prowse, K. R., Harley, C. B., West, M. D., Ho, P. L. C., et al. (1994). Specific Association of Human Telomerase Activity with Immortal Cells and Cancer. Science 266, 2011–2015. doi:10.1126/science.7605428
Kim, N., and Wu, F. (1997). Advances in Quantification and Characterization of Telomerase Activity by the Telomeric Repeat Amplification Protocol (TRAP). Nucleic Acids Res. 25, 2595–2597. doi:10.1093/nar/25.13.2595
Lafferty-Whyte, K., Cairney, C. J., Will, M. B., Serakinci, N., Daidone, M.-G., Zaffaroni, N., et al. (2009). A Gene Expression Signature Classifying Telomerase and ALT Immortalization Reveals an hTERT Regulatory Network and Suggests a Mesenchymal Stem Cell Origin for ALT. Oncogene 28, 3765–3774. doi:10.1038/onc.2009.238
Lang, R., Liu, G., Shi, Y., Bharadwaj, S., Leng, X., Zhou, X., et al. (2013). Self-renewal and Differentiation Capacity of Urine-Derived Stem Cells after Urine Preservation for 24 hours. PLoS One 8, e53980. doi:10.1371/journal.pone.0053980
Li, F., Ge, Y., Liu, D., and Songyang, Z. (2020). The Role of Telomere-Binding Modulators in Pluripotent Stem Cells. Protein Cell 11, 60–70. doi:10.1007/s13238-019-0651-y
Liu, L., Digirolamo, C. M., Navarro, P. A. A. S., Blasco, M. A., and Keefe, D. L. (2004). Telomerase Deficiency Impairs Differentiation of Mesenchymal Stem Cells. Exp. Cell Res. 294, 1–8. doi:10.1016/j.yexcr.2003.10.031
Lopatina, N. G., Poole, J. C., Saldanha, S. N., Hansen, N. J., Key, J. S., Pita, M. A., et al. (2003). Control Mechanisms in the Regulation of Telomerase Reverse Transcriptase Expression in Differentiating Human Teratocarcinoma Cells. Biochem. Biophysical Res. Commun. 306, 650–659. doi:10.1016/s0006-291x(03)01033-7
Lu, L. L., Liu, Y. J., Yang, S. G., Zhao, Q. J., Wang, X., Gong, W., et al. (2006). Isolation and Characterization of Human Umbilical Cord Mesenchymal Stem Cells with Hematopoiesis-Supportive Function and Other Potentials. haematologica 91, 1017–1026.
Lv, X.-J., Zhou, G.-D., Liu, Y., Liu, X., Chen, J.-N., Luo, X.-S., et al. (2012). In Vitro proliferation and Differentiation of Adipose-Derived Stem Cells Isolated Using Anti-cd105 Magnetic Beads. Int. J. Mol. Med. 30, 826–834. doi:10.3892/ijmm.2012.1063
Nava, M. B., Catanuto, G., Pennati, A. E., Rocco, N., Spano, A., Villa, R., et al. (2015b). Lack of Activation of Telomere Maintenance Mechanisms in Human Adipose Stromal Cells Derived from Fatty Portion of Lipoaspirates. Plast. Reconstr. Surg. 135, 114e–123e. doi:10.1097/PRS.0000000000001008
Nava, M. B., Catanuto, G., Pennati, A. E., Rocco, N., Spano, A., Villa, R., et al. (2015a). Lack of Activation of Telomere Maintenance Mechanisms in Human Adipose Stromal Cells Derived from Fatty Portion of Lipoaspirates. Plastic Reconstr. Surg. 135, 114e–123e. doi:10.1097/prs.0000000000001008
Simonsen, J. L., Rosada, C., Serakinci, N., Justesen, J., Stenderup, K., Rattan, S. I. S., et al. (2002). Telomerase Expression Extends the Proliferative Life-Span and Maintains the Osteogenic Potential of Human Bone Marrow Stromal Cells. Nat. Biotechnol. 20, 592–596. doi:10.1038/nbt0602-592
Sun, J., Xing, F., Zou, M., Gong, M., Li, L., and Xiang, Z. (2021). Comparison of Chondrogenesis-Related Biological Behaviors between Human Urine-Derived Stem Cells and Human Bone Marrow Mesenchymal Stem Cells from the Same Individual. Stem Cell Res. Ther. 12, 366. doi:10.1186/s13287-021-02370-1
Thongon, N., Ma, F., Santoni, A., Marchesini, M., Fiorini, E., Rose, A., et al. (2021). Hematopoiesis under Telomere Attrition at the Single-Cell Resolution. Nat. Commun. 12, 6850. doi:10.1038/s41467-021-27206-7
Wang, F., Yin, Y., Ye, X., Liu, K., Zhu, H., Wang, L., et al. (2012). Molecular Insights into the Heterogeneity of Telomere Reprogramming in Induced Pluripotent Stem Cells. Cell Res. 22, 757–768. doi:10.1038/cr.2011.201
Wu, S., Wang, Z., Bharadwaj, S., Hodges, S. J., Atala, A., and Zhang, Y. (2011). Implantation of Autologous Urine Derived Stem Cells Expressing Vascular Endothelial Growth Factor for Potential Use in Genitourinary Reconstruction. J. Urology 186, 640–647. doi:10.1016/j.juro.2011.03.152
Zhang, Y., Mcneill, E., Tian, H., Soker, S., Andersson, K.-E., Yoo, J. J., et al. (2008). Urine Derived Cells Are a Potential Source for Urological Tissue Reconstruction. J. Urology 180, 2226–2233. doi:10.1016/j.juro.2008.07.023
Keywords: telomerase, urine-derived stem cells, longevity, tissue regeneration, differentiation
Citation: Shi Y, Liu G, Wu R, Mack DL, Sun XS, Maxwell J, Guan X, Atala A and Zhang Y (2022) Differentiation Capacity of Human Urine-Derived Stem Cells to Retain Telomerase Activity. Front. Cell Dev. Biol. 10:890574. doi: 10.3389/fcell.2022.890574
Received: 06 March 2022; Accepted: 29 April 2022;
Published: 23 May 2022.
Edited by:
Jingting Li, Sun Yat-sen University, ChinaReviewed by:
Jyotirmoy Ghosh, National Institute of Animal Nutrition and Physiology (ICAR), IndiaCopyright © 2022 Shi, Liu, Wu, Mack, Sun, Maxwell, Guan, Atala and Zhang. This is an open-access article distributed under the terms of the Creative Commons Attribution License (CC BY). The use, distribution or reproduction in other forums is permitted, provided the original author(s) and the copyright owner(s) are credited and that the original publication in this journal is cited, in accordance with accepted academic practice. No use, distribution or reproduction is permitted which does not comply with these terms.
*Correspondence: Yuanyuan Zhang, eXpoYW5nQHdha2VoZWFsdGguZWR1
†These authors have contributed equally to this work and share first authorship
Disclaimer: All claims expressed in this article are solely those of the authors and do not necessarily represent those of their affiliated organizations, or those of the publisher, the editors and the reviewers. Any product that may be evaluated in this article or claim that may be made by its manufacturer is not guaranteed or endorsed by the publisher.
Research integrity at Frontiers
Learn more about the work of our research integrity team to safeguard the quality of each article we publish.