- 1Department of Pathology and Laboratory Medicine, University of Wisconsin School of Medicine and Public Health, Madison, WI, United States
- 2Department of Ophthalmology and Visual Sciences, University of Wisconsin School of Medicine and Public Health, Madison, WI, United States
Integrins are a family of heterodimeric receptors composed of an α- and β-subunit that mediate cell-adhesion to a number of extracellular matrix (ECM) proteins in the Trabecular Meshwork/Schlemm’s canal (TM/SC) of the eye. Upon binding an ECM ligand, integrins transmit signals that activate a number of signaling pathways responsible for regulating actin-mediated processes (i.e phagocytosis, cell contractility, and fibronectin fibrillogenesis) that play an important role in regulating intraocular pressure (IOP) and may be involved in glaucoma. An important function of integrin-mediated signaling events is that the activity of one integrin can affect the activity of other integrins in the same cell. This creates a crosstalk that allows TM/SC cells to respond to changes in the ECM presumably induced by the mechanical forces on the TM/SC, aging and disease. In this review, we discuss how integrin crosstalk influences the function of the human TM/SC pathway. In particular, we will discuss how different crosstalk pathways mediated by either the αvβ3 or α4β1 integrins can play opposing roles in the TM when active and therefore act as on/off switches to modulate the cytoskeleton-mediated processes that regulate the outflow of aqueous humor through the TM/SC.
Introduction
The extracellular matrix (ECM) creates a microenvironment that influences gene expression and cellular behavior in tissues (Iozzo and Gubbiotti, 2018; Seetharaman and Etienne-Manneville, 2018). In the human eye, the composition and biomechanical properties (rigidity, elasticity and topology) of the ECM within the Trabecular Meshwork/Schlemm’s Canal (TM/SC) contribute to the regulation of intraocular pressure (IOP) and the pathogenesis of glaucoma (Keller and Peters, 2022). Changes in the ECM affect IOP by altering the biological processes (i.e contractility, gene expression, or phagocytosis) in the TM/SC that regulate aqueous humor flow through the anterior chamber (Rohen and Lutjen-Drecoll, 1989; Rohen et al., 1993; Vranka et al., 2015).
The TM/SC is a highly elastic tissue that stretches and recoils in response to mechanical forces. These mechanical forces are triggered by contraction of the ciliary muscle and pulsatile forces due to changes in IOP and blood pressure (Johnstone et al., 2021). Such changes in the TM/SC pathway are likely to cause force-induced changes in ECM fibrils like fibronectin (Baneyx et al., 2002; Smith et al., 2007) that can be detected by specific cell surface receptors, thus enabling the cells in the TM/SC to change their biological functions in response to mechanical and pulsatile forces.
Integrins are a major class of cell surface receptors that are able to respond to changes in the TM/SC ECM. They are a family of transmembrane heterodimeric proteins composed of an α and β subunit. Eight β subunits and eighteen α subunits can be assembled into 24 distinct integrins with unique properties (Humphries et al., 2006). The various combinations of the integrin subunits create a heterodimer that shows specificity for different ECM ligands. Currently, 12 different integrins have been identified on the cells in the TM/SC outflow pathway (Zhou et al., 1996; Zhou et al., 1999; Filla et al., 2017). Most of them appear to be expressed by all the cells in the TM/SC although there does appear to be some differences, most notably, in the levels of α2β1, α4β1 and αvβ3 integrin (Zhou et al., 1999). The expression profile and activity of these integrins in the TM/SC, however, are likely to vary. A number of factors such as the rigidity of the ECM (Seetharaman and Etienne-Manneville, 2018), ion channels and cadherins (Dieterle et al., 2021) and proteins associated with the cytoplasmic tails (Sun Z. et al., 2016) play a critical role in mediating integrin activity. In addition, since integrins are recycled on and off the cell surface and the mechanisms regulating this are cell type specific (Moreno-Layseca et al., 2019), it is possible that not all integrins will be expressed at the same time. Thus, the expression and mechanotransduction function of integrins will change in a spatiotemporal fashion as the physical and mechanical properties of the TM/SC varies.
Historically, integrins were thought to be cell adhesion receptors found in structures called focal adhesions (FAs). However, integrins are also found in other cellular structures (Zuidema et al., 2020) found in the TM/SC. For instance, α5β1 integrin is found in fibrillar adhesions. The α3β1 integrin and the α6β4 integrin can be found in cadherin-containing adherens junctions (AJs) and hemidesmosomes, respectively. Some integrins like α3β1 and αvβ3 are also found in invadiopodum/podosomes which can be found in TM cells (Aga et al., 2008; Murphy and Courtneidge, 2011; Zuidema et al., 2020) and recently the α3β1 integrin was found in tight junctions in the TM/SC (Li et al., 2020). Other integrins, such as αvβ5 integrin, can be found in clathrin-containing adhesion complexes whereas α3β1, α6β1, and α4β1 integrins can be found in tetraspanin-enriched microdomains (Zuidema et al., 2020). Thus, integrins have multiple roles in cells mediating attachment to the substrate, cell-cell adhesion, endocytosis, and the assembly of signaling and membrane complexes.
A unique feature of integrins is that their activity involves very specific conformational changes in their α- and β-subunits (Askari et al., 2009; Campbell and Humphries, 2011; Li et al., 2017; Vicente-Manzanares and Sanchez-Madrid, 2018; Kechagia et al., 2019). In their unoccupied state, the extracellular domains of the α and β integrin subunits are in a bent conformation with their cytoplasmic tails bound together by a salt bridge (Figure 1A). Upon activation, the integrin subunits undergo a conformational change and assume an upright conformation with their cytoplasmic tails separated. The integrin can be activated by engaging its ligand. This is called outside-in signaling. Alternatively, the integrin can be activated intracellularly by a secondary signaling pathway which allows the integrin to engage its ligand. This is called inside-out signaling.
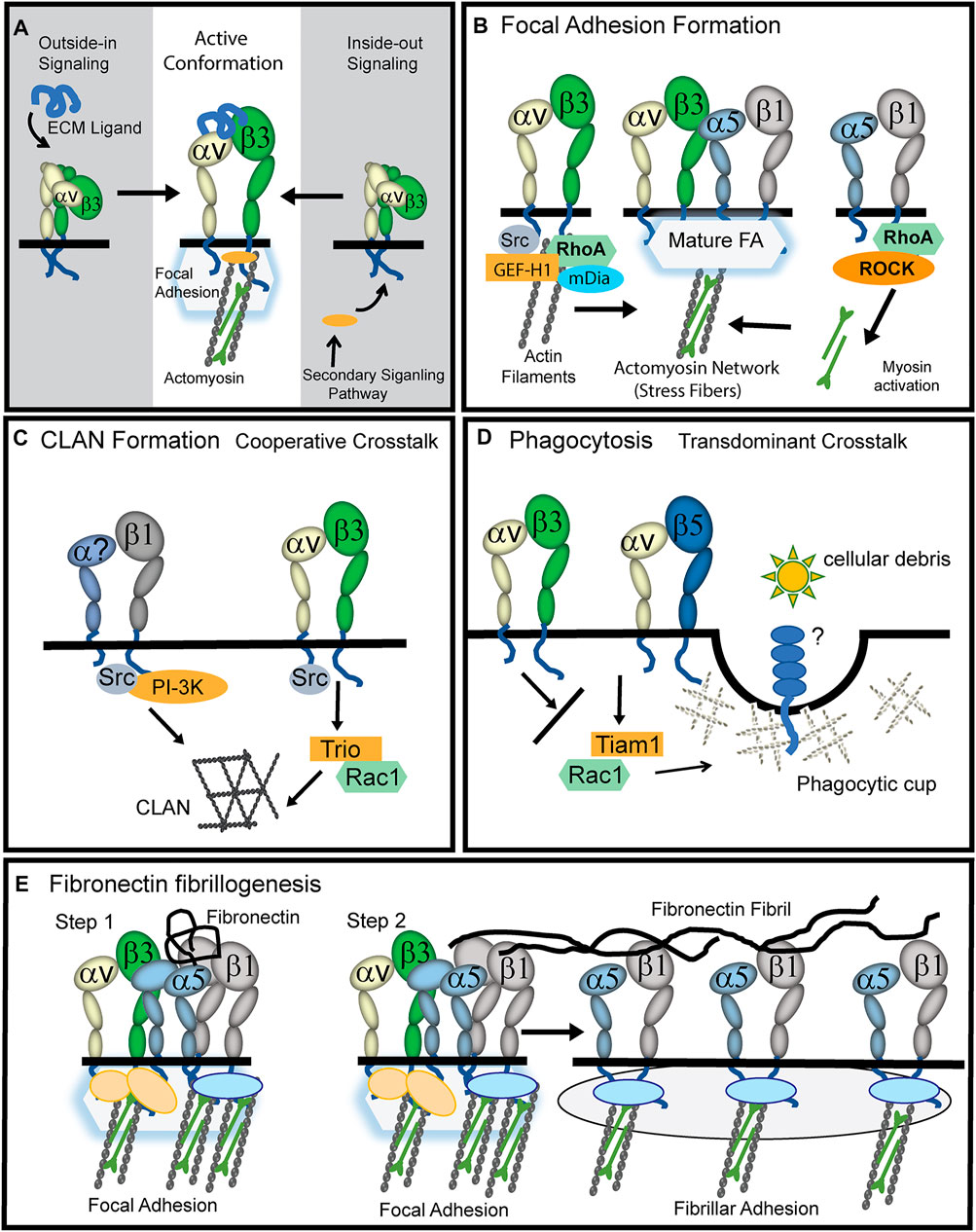
FIGURE 1. Role of activated αvβ3 integrin in TM cells. (A) The active conformation of αvβ3 integrin can be triggered by a process called outside-in signaling in which an ECM ligand binds to the extracellular domain of the heterodimer and triggers the upright conformation. Alternatively, a process called inside-out signaling can induce the active conformation. This happens when a secondary signaling pathway triggers the binding of cytoplasmic proteins (orange oval) to the cytoplasmic tails of the integrin causing the cytoplasmic tails to separate and the heterodimer to assume an upright conformation. (B) During the early stages of focal adhesion (FA) formation, actin filaments are generated when activated αvβ3 integrin signaling recruits GEF-H1, RhoA and the Rho effector mDía to nascent FAs. Stress fibers in FAs are then formed when signaling from α5β1 integrins recruit RhoA and Rho kinase (ROCK) to the FA and myosin is activated and binds to actin filaments. (C) Cooperative crosstalk between activated αvβ3 and β1 integrins forms CLANs. αvβ3 integrins utilize Src kinase to help recruit the GEF Trio and Rac1 to FAs while β1 integrins utilize Src kinase and PI3-kinase. The question mark in the α subunit indicates that α1, α2, α4, or α5 integrin subunits may be involved. (D) Transdominant crosstalk between αvβ3 and αvβ5 integrins inhibits αvβ5 integrin-mediated phagocytosis which involves the GEF Tiam1 and Rac1. αvβ3 integrin inhibits this process presumably because it prevents αvβ5 integrin from using Rac1 to form the branched actin structure used to form a phagocytic cup. (E) Upon binding of soluble fibronectin to α5β1 integrins (Step1), cooperative signaling between αvβ3 and α5β1 integrins in FAs creates the RhoA-mediated contractility needed for fibronectin fibrillogenesis (Zhang et al., 1997; Zhong et al., 1998). While αvβ3 integrins remain in FAs, presumably to help anchor stress fibers, α5β1 integrins are translocated out of FAs by contractile forces into fibrillar adhesions (Step2). This α5β1 translocation promotes the stretching of the fibronectin dimer which exposes fibronectin-fibronectin binding sites involved in fibronectin fibrillogenesis.
Upon engagement with an ECM protein, each integrin transmits signals via proteins associated with their cytoplasmic tails and the cytoskeleton. This cytoskeleton engagement allows for signals generated by mechanical forces on the ECM to be transmitted directly to the nucleus and drive transcription. Thus, integrins are conduits that convert signals from the ECM environment (rigidity, elasticity and topology) into intracellular biochemical signals that affect cytoskeleton organization, gene expression, and proliferation (Kechagia et al., 2019).
The specificity and activation of each integrin can occur within subseconds and is therefore likely to be highly regulated by the spatiotemporal expression and activation of other integrins and receptors on the cell surface (Ross et al., 2013; Seetharaman and Etienne-Manneville, 2018). This creates not only a “crosstalk” between integrins but a crosstalk between integrins and their various membrane partners such as cadherins (Canel et al., 2013), syndecans (Bass and Humphries, 2002; Morgan et al., 2007), and growth factor receptors (Ross, 2004). This crosstalk, together with the specific conformational states of the integrin, enables the integrin function to be tunable thus eliciting different biochemical responses without necessarily disrupting cell adhesion.
At least two integrins found in the TM/SC are known to have a “tunable” conformation and thus their activity is likely to be altered depending upon the composition and 3D architecture of the ECM in the TM/SC. These integrins are the αvβ3 and α4β1 integrins.
αvβ3 Integrin
αvβ3 integrin is potentially a key player in regulating outflow through the TM/SC and is found throughout the TM/SC (Gagen et al., 2013; Filla et al., 2017). Unlike other integrins in this pathway, αvβ3 integrin has a number of ligands important in regulating IOP including connective tissue growth factor (CTGF), fibronectin, and thrombospondin-1 (TSP-1). Its expression and active state can be upregulated by the glucocorticoid dexamethasone (Filla et al., 2011; Faralli et al., 2013) via a secondary effect involving the transcription factor NFATc1. CTGF may also upregulate αvβ3 integrin activity since it increases expression of the αv integrin subunit in human TM (HTM) cells (Junglass et al., 2009). αvβ3 integrin can also be activated by mechanical forces similar to those observed in the TM/SC (Johnstone et al., 2021). In particular, the αvβ3 integrin can be activated by shear stress (Tzima et al., 2001) making it likely to be activated on SC cells when shear stress is increased (Ashpole et al., 2014).
Transforming growth factor β2 (TGFβ2), which is a risk factor for primary open angle glaucoma (POAG) (Fuchshofer and Tamm, 2012), increases expression of both αv and β3 integrin subunits (Tsukamoto et al., 2018). Interestingly, αvβ3 integrin activation drives expression of TGFβ2 (Filla et al., 2021), thus setting up a potential feedback loop. Additionally, TGFβ2-induced changes in the ECM (Fleenor et al., 2006; Medina-Ortiz et al., 2013) likely trigger the expression of various ECM ligands (Flugel-Koch et al., 2004) that can activate αvβ3 integrin. For instance, TSP-1, which is upregulated by TGFβ2, can activate αvβ3 integrin signaling (Gao et al., 1996; Barazi et al., 2002; Filla et al., 2009).
αvβ3 integrins likely play a role in mechanosensing in the TM/SC pathway. Studies in fibroblasts found that integrins containing the αv integrin subunit in FAs play an important role in modulating cellular responses to forces on the ECM microenvironment (Schiller et al., 2013). αvβ3 integrin localization into FAs is driven by forces on the actomyosin network (Wang and Ha, 2013), and it is considered to be one of the more stable components of FAs (Morgan et al., 2013). This is in contrast to α5β1 integrin which ultimately leaves FAs and translocates to fibrillar adhesions (Katz et al., 2000). αvβ3 integrin is, therefore, a key factor in the mechanosensing role of FAs and its localization in FAs is a prerequisite for myofibroblast differentiation (Hinz, 2006; Hinz and Gabbiani, 2010; Hinz et al., 2012) which is thought to drive ECM changes associated with POAG (Fuchshofer and Tamm, 2012).
Another important feature of αvβ3 integrin is that it has a weaker bond strength and faster binding and unbinding rate compared to α5β1 integrin within FAs (Roca-Cusachs et al., 2009). This makes αvβ3 integrin better able to sense and modulate changes in the contractile properties of cells as the TM/SC stretches and recoils, possibly acting as an on/off switch to control the contractile properties of the TM/SC cytoskeleton. This presumably would involve force-induced changes in the interactions between αvβ3 integrin and its ECM ligands (Seetharaman and Etienne-Manneville, 2018) that may occur as the TM/SC stretches and recoils. Other factors that may affect mechanosensing through integrins in the TM/SC include lipid rafts (Radel et al., 2007; Sun X. et al., 2016), cross talk with ion channels and cadherins (Dieterle et al., 2021) and the mechanical link (clutch bond) formed between integrins and actin binding proteins (Sun Z. et al., 2016).
αvβ3 integrin is responsible for regulating a number of different actin-containing structures. It is responsible for the Src/Rac1 driven polymerization of branched Arp2/3-containing actin networks in lamellipodium (Danen et al., 2002) and crosslinked actin networks (CLANs) in HTM cells (Filla et al., 2009). It is also involved in RhoA mediated formation of podosomes in osteoclasts (Chellaiah, 2006) and the RhoA-mDia polymerization of actin filaments during early stages of cell adhesion (Figure 2A). Eventually, these actin filaments formed by αvβ3 integrin-RhoA-mDia signaling develop into stress fibers in mature FAs when RhoA/ROCK activity generated by α5β1 integrin drives the phosphorylation of myosin (Huveneers and Danen, 2009).
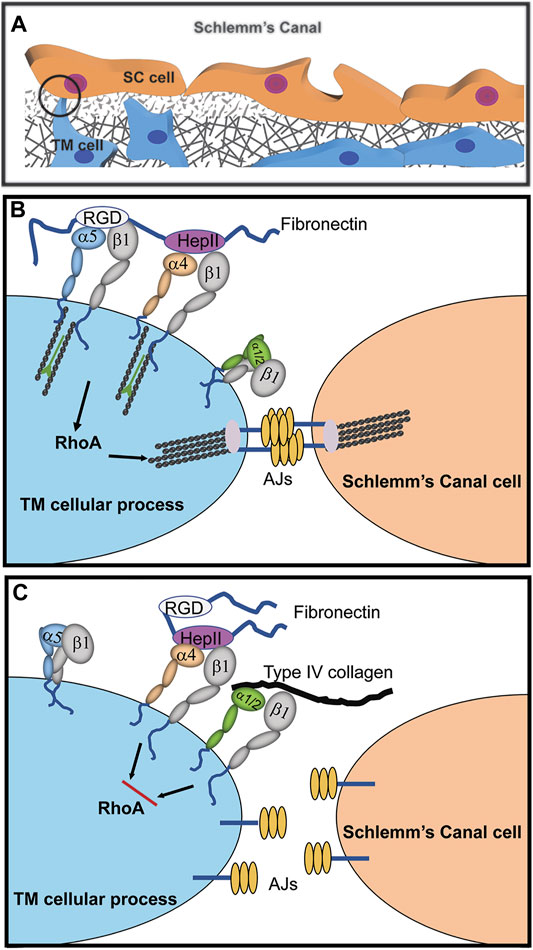
FIGURE 2. Cooperative signaling with α4β1 integrin controls assembly of adherens junctions (AJs). (A) Cadherin-containing AJs are likely to be found in the cellular processes connecting juxtacanalicular trabecular meshwork (JCT) and SC cells as well as in cellular processes connecting JCT cells to each other and with JCT cells connected to the cells lining the trabecular beams of the meshwork (Johnstone et al., 2021). (B) Fibronectin in the ECM promotes cooperative signaling between α4β1 and α5β1 integrins that activates RhoA-mediated stress fiber formation. Crosstalk between this integrin signaling complex and cadherins in AJs stabilizes cell-cell interactions. (C) The presence of collagen in the ECM binding to either α1β1 or α2β1 integrins disrupts α4β1 integrin signaling with α5β1 integrin. This creates an α1/α2β1 and α4β1 integrin complex that inhibits RhoA activity, thus causing a disruption in the formation of stress fibers and AJs.
In the following section we will discuss what is known about how αvβ3 integrin crosstalk influences the formation of various actin-containing structures required for phagocytosis, fibronectin fibrillogenesis and CLAN formation in the TM/SC pathway. In each case, the role of αvβ3 integrin appears to be determined by the guanine nucleotide exchange factors (GEFs) recruited into the integrin signaling complex.
Role of αvβ3 Integrin in Phagocytosis
In vitro studies show that αvβ3 integrin triggers Rac1-mediated signaling events in HTM cells associated with glaucoma. For instance, activation of αvβ3 integrin decreased phagocytosis (Gagen et al., 2013; Peotter et al., 2016). Phagocytosis is needed to clear cellular debris from the outflow pathway, and dysregulation of phagocytosis is associated with elevated IOP in glucocorticoid-induced glaucoma (Matsumoto and Johnson, 1997a; Matsumoto and Johnson, 1997b). Normally, an αvβ5 integrin driven Rac1 process regulates phagocytosis in HTM cells (Gagen et al., 2013) as observed in retinal pigmented epithelial cells (Mao and Finnemann, 2012). The inhibition of phagocytosis by αvβ3 integrin activation is considered a “transdominant inhibition” (Diaz-Gonzalez et al., 1996; Gonzalez et al., 2010) of αvβ5 integrin activity (Figure 1D). Studies suggest the inhibition was due to a switch in the use of the GEFs (Trio or Tiam1) regulating the activation of Rac1 (Peotter et al., 2016; Faralli et al., 2019a) since αvβ5 integrin expression was unimpaired by upregulation of an activated αvβ3 integrin (Gagen et al., 2013).
Role of αvβ3 Integrin in Fibronectin Fibrillogenesis
αvβ3 integrin activation in cultured HTM cells increased the deposition of the alternatively spliced form of fibronectin called EDA + fibronectin (Filla et al., 2019) that is associated with the ECM changes observed in POAG and thought to contribute to elevated IOP (Roberts et al., 2020). This enhanced EDA + fibronectin fibrillogenesis may be due to cooperativity between αvβ3 integrin-Rac1 mediated actin polymerization and the α5β1 integrin/Rho signaling pathway forming stable FAs that promote the RhoA-mediated contractile forces needed for fibrillogenesis (Figure 1E). Thus, during fibrillogenesis, αvβ3 integrin maintains the FAs, while the contractile forces of the actomyosin fibers drive α5β1 integrin translocation into fibrillar adhesions; pulling and stretching the fibronectin as they form fibrils.
Role of αvβ3 Integrin in CLAN Formation
αvβ3 integrin is also involved in CLAN formation (Figure 1C). CLANs are interconnected networks of actin filaments that radiate outward from central hubs resembling a geodesic dome (Lazarides, 1976). CLANs were found in dexamethasone treated-cultured TM cells and in TM cells in isolated meshworks from glaucomatous donor eyes not treated with dexamethasone (Clark et al., 1995) suggesting these actin structures are involved in POAG pathogenesis (Clark et al., 2005; Hoare et al., 2009). The αvβ3 integrin is suitable to controlling CLAN formation since it readily reorganizes the actin cytoskeleton in response to lower levels of stiffness in the ECM like that induced by stretch (Balcioglu et al., 2015). The function of CLANs in the TM remains unclear, but they were originally thought to be actin stress fiber precursors in nonmuscle cells (Lazarides, 1976). If true, this suggests that increased CLAN formation could trigger increased stress fiber formation which may contribute to elevated IOP in glaucoma (Rao et al., 2001; Rao et al., 2005).
CLAN assembly, like fibronectin fibrillogenesis, also involves cooperativity between αvβ3 integrin and a β1 integrin and is dependent on the level of αvβ3 integrin expression and activity (Filla et al., 2009). However, unlike fibronectin fibrillogenesis, this crosstalk between αvβ3 and β1 integrins utilizes a Rac1-mediated signaling pathway to control actin polymerization (Figure 1D). This difference is likely due to the downstream effectors being used to activate actin polymerization. Studies in HTM cells showed that during CLAN formation, αvβ3 integrin signaling activates the Rac1 GEF Trio (Filla et al., 2009). This αvβ3 integrin/Trio/Rac1 signaling pathway converges with a β1 integrin/PI3-K signaling pathway to form CLANs. Both β1 and αvβ3 integrins are needed since low levels of CLANs are observed in HTM cells when only one of these integrins is engaged (Filla et al., 2006). Although α5β1 and αvβ3 integrins were more proficient at inducing CLANs, other β1 integrins such as α4β1 integrin and the collagen-binding integrins α1β1 and α2β1 induce CLANs in cooperation with αvβ3 integrins (Filla et al., 2006). Thus, the frequency of CLAN formation is dependent on ECM substrate composition and the engagement of specific integrins with the ECM.
Given the effects of αvβ3 integrin on phagocytosis, ECM deposition and actin polymerization, it is not surprising that αvβ3 integrin activation significantly increased IOP in C57BL/6J mice while the knockdown of αvβ3 integrin levels in the mouse TM significantly decreased IOP (Faralli et al., 2019b). αvβ3 integrin activation also significantly decreased outflow facility in porcine cultured anterior segments (Faralli et al., 2019b).
α4β1 Integrin
α4β1 is another integrin found in the TM/SC pathway (Zhou et al., 1999; Peterson et al., 2004) that has a well-documented tunable function (Chigaev et al., 2001; Chigaev et al., 2004; Chigaev et al., 2007). Although it is best known for its role in inflammation as a co-receptor for TLR4 (Kelsh-Lasher et al., 2017) and as an adhesion receptor in migrating leukocytes (Liu et al., 2000), α4β1 integrin also controls the shear force-dependent contractile properties of endothelial cells (Goldfinger et al., 2008) and the contractile properties of migrating smooth muscle and neural crests cells (Sheppard et al., 1994; Stepp et al., 1994). Among the integrins, it has a unique role in migration and FAs that is largely attributed to the α4 integrin cytoplasmic domain (Kassner et al., 1995). α4β1 integrin forms less stable interactions with the cytoskeleton within FAs similar to αvβ3 integrin which suggests that it may alter cytoskeletal dynamics in response to mechanical factors such as shear force (Goldfinger et al., 2008). This weaker interaction is regulated by paxillin binding to the α4 subunit cytoplasmic tail. When paxillin is bound to α4β1 integrin, binding to the α4β1 ligand is weakened (Liu et al., 2002). Paxillin interactions with the α4 subunit are regulated by the phosphorylation of Ser988 in the α4 cytoplasmic tail (Han et al., 2001). When Ser988 is phosphorylated, interactions between paxillin and the α4 cytoplasmic tail are inhibited.
Like αvβ3 integrin, α4β1 integrin can be activated by inside-out signaling pathways (Figure 1A). These pathways include G-protein-coupled receptors for CXCR2 and CXCR4 (Laudanna et al., 2002), phorbol esters, and calcium ionophores (Chigaev et al., 2007). In contrast, other signaling pathways such as nitric oxide/cGMP pathways in lymphoma cells can downregulate α4β1 integrin activation (Chigaev et al., 2011).
One α4β1 integrin ligand is the HeparinII (HepII) domain of fibronectin (Faralli et al., 2019c) Studies using the HepII domain found that α4β1 integrin can control the contractile properties of the TM/SC. Treating HTM cultures with the HepII domain caused the disassembly of stress fibers and decreased cell contractility in a collagen gel assay (Schwinn et al., 2010). This required the α4β1 integrin because when α4β1 integrin expression was silenced, the HepII domain had no effect on cell contractility. Perfusion of the HepII domain also decreased IOP in human (Santas et al., 2003) and monkey cultured anterior segments (Schwinn et al., 2010). Histology of the monkey anterior segments showed that treatment with the HepII domain disrupted the contractile properties of the TM/SC similar to what was observed in monkey eyes treated with the H-7 inhibitor (Sabanay et al., 2000). Additionally, there was a loss of SC cells in human anterior segments (Santas et al., 2003) possibly resulting from a disruption in cell-cell adhesions since HepII treatment disrupted AJs in HTM cultures (Gonzalez et al., 2006; Gonzalez et al., 2009). These data suggest that α4β1 integrin activation by the HepII domain affected the contractile properties of the TM/SC.
The composition of the ECM can affect the ability of the HepII domain and α4β1 integrin to disrupt the contractile properties of HTM cells (Figure 2). When TM cells were plated on type IV collagen, not only was stress fiber assembly disrupted by the HepII domain, but AJs were also disrupted (Peterson et al., 2004; Schwinn et al., 2010). Presumably this loss in stress fibers and AJs occurred because RhoA, which is needed to stabilize AJs and stress fibers, was inhibited. In contrast, when cells were plated on fibronectin, HepII domain enhanced stress fiber formation and AJs were unaffected. Since different integrins would be involved in binding to fibronectin versus type IV collagen, this suggests that crosstalk between the various integrins and α4β1 integrin differentially affect RhoA. Crosstalk between collagen-bound integrins (α1β1 or α2β1) and HepII-bound α4β1 integrin may disrupt RhoA activity whereas crosstalk between the α5β1 integrin fibronectin receptor and HepII-bound α4β1 integrin enhance RhoA activity (Peterson et al., 2004; Schwinn et al., 2010). This observation has special significance for the TM/SC because changes in ECM composition, especially an increase in fibronectin expression, are associated with increased IOP and the development of POAG. Thus, this finding demonstrates how critical integrin-ECM interactions are for modulating the functions of the TM/SC.
Discussion
Since the expression of and signaling from αvβ3 and α4β1 integrins (Peterson et al., 2004; Faralli et al., 2013) is normally low in HTM cells in vitro compared to other integrins, activation of these two integrins could be a gain of function in normal HTM cells. Interestingly, activating αvβ3 integrin as discussed above would induce many of the changes associated with reduced aqueous humor outflow while activating α4β1 integrin in TM increases outflow facility. This suggests that the level of expression and activity of these two integrins could essentially create on/off switches in the TM/SC pathway that could regulate the homeostatic properties of the tissue.
Author Contributions
Conceptualization: DP. performed experiments: JF, and MF. data analysis: JF, MF, and DP.; writing: JF, MF, and DP; editing: JF, MF, and DP; funding acquisition: DP.
Funding
This work was supported by NEI grants EY017006, EY026009, BrightFocus (DP) and a Core grant to the Department of Ophthalmology and Visual Sciences (P30 EY016665). The University of Wisconsin Translational Research Initiatives in Pathology laboratory (TRIP), supported by the UW Department of Pathology and Laboratory Medicine, UWCCC (P30 CA014520) and the Office of The Director- NIH (S10 OD023526).
Conflict of Interest
The authors declare that the research was conducted in the absence of any commercial or financial relationships that could be construed as a potential conflict of interest.
Publisher’s Note
All claims expressed in this article are solely those of the authors and do not necessarily represent those of their affiliated organizations, or those of the publisher, the editors and the reviewers. Any product that may be evaluated in this article, or claim that may be made by its manufacturer, is not guaranteed or endorsed by the publisher.
References
Aga, M., Bradley, J. M., Keller, K. E., Kelley, M. J., and Acott, T. S. (2008). Specialized Podosome- or Invadopodia-like Structures (PILS) for Focal Trabecular Meshwork Extracellular Matrix Turnover. Invest. Ophthalmol. Vis. Sci. 49, 5353–5365. doi:10.1167/iovs.07-1666
Ashpole, N. E., Overby, D. R., Ethier, C. R., and Stamer, W. D. (2014). Shear Stress-Triggered Nitric Oxide Release from Schlemm's Canal Cells. Invest. Ophthalmol. Vis. Sci. 55, 8067–8076. doi:10.1167/iovs.14-14722
Askari, J. A., Buckley, P. A., Mould, A. P., and Humphries, M. J. (2009). Linking Integrin Conformation to Function. J. Cel Sci 122, 165–170. doi:10.1242/jcs.018556
Balcioglu, H. E., Van Hoorn, H., Donato, D. M., Schmidt, T., and Danen, E. H. J. (2015). The Integrin Expression Profile Modulates Orientation and Dynamics of Force Transmission at Cell-Matrix Adhesions. J. Cel Sci 128, 1316–1326. doi:10.1242/jcs.156950
Baneyx, G., Baugh, L., and Vogel, V. (2002). Fibronectin Extension and Unfolding within Cell Matrix Fibrils Controlled by Cytoskeletal Tension. Proc. Natl. Acad. Sci. U.S.A. 99, 5139–5143. doi:10.1073/pnas.072650799
Barazi, H. O., Li, Z., Cashel, J. A., Krutzsch, H. C., Annis, D. S., Mosher, D. F., et al. (2002). Regulation of Integrin Function by CD47 Ligands. J. Biol. Chem. 277, 42859–42866. doi:10.1074/jbc.m206849200
Bass, M. D., and Humphries, M. J. (2002). Cytoplasmic Interactions of Syndecan-4 Orchestrate Adhesion Receptor and Growth Factor Receptor Signalling. Biochem. J. 368, 1–15. doi:10.1042/bj20021228
Campbell, I. D., and Humphries, M. J. (2011). Integrin Structure, Activation, and Interactions. Cold Spring Harbor Perspect. Biol. 3, a004994. doi:10.1101/cshperspect.a004994
Canel, M., Serrels, A., Frame, M. C., and Brunton, V. G. (2013). E-cadherin-integrin Crosstalk in Cancer Invasion and Metastasis. J. Cel Sci 126, 393–401. doi:10.1242/jcs.100115
Chellaiah, M. A. (2006). Regulation of Podosomes by Integrin αvβ3 and Rho GTPase-Facilitated Phosphoinositide Signaling. Eur. J. Cel Biol. 85, 311–317. doi:10.1016/j.ejcb.2006.01.008
Chigaev, A., Blenc, A. M., Braaten, J. V., Kumaraswamy, N., Kepley, C. L., Andrews, R. P., et al. (2001). Real Time Analysis of the Affinity Regulation of α4-Integrin. J. Biol. Chem. 276, 48670–48678. doi:10.1074/jbc.m103194200
Chigaev, A., Smagley, Y., and Sklar, L. A. (2011). Nitric oxide/cGMP Pathway Signaling Actively Down-Regulates α4β1-integrin Affinity: an Unexpected Mechanism for Inducing Cell De-adhesion. BMC Immunol. 12, 28. doi:10.1186/1471-2172-12-28
Chigaev, A., Waller, A., Zwartz, G. J., Buranda, T., and Sklar, L. A. (2007). Regulation of Cell Adhesion by Affinity and Conformational Unbending of α4β1Integrin. J. Immunol. 178, 6828–6839. doi:10.4049/jimmunol.178.11.6828
Chigaev, A., Zwartz, G. J., Buranda, T., Edwards, B. S., Prossnitz, E. R., and Sklar, L. A. (2004). Conformational Regulation of α4β1-Integrin Affinity by Reducing Agents. J. Biol. Chem. 279, 32435–32443. doi:10.1074/jbc.m404387200
Clark, A. F., Brotchie, D., Read, A. T., Hellberg, P., English-Wright, S., Pang, I.-H., et al. (2005). Dexamethasone Alters F-Actin Architecture and Promotes Cross-Linked Actin Network Formation in Human Trabecular Meshwork Tissue. Cell Motil. Cytoskeleton 60, 83–95. doi:10.1002/cm.20049
Clark, A. F., Miggans, S. T., Wilson, K., Browder, S., and McCartney, M. D. (1995). Cytoskeletal Changes in Cultured Human Glaucoma Trabecular Meshwork Cells. J. Glaucoma 4, 183–188. doi:10.1097/00061198-199506000-00007
Danen, E. H. J., Sonneveld, P., Brakebusch, C., Fässler, R., and Sonnenberg, A. (20022002). The Fibronectin-Binding Integrins α5β1 and αvβ3 Differentially Modulate RhoA-GTP Loading, Organization of Cell Matrix Adhesions, and Fibronectin Fibrillogenesis. J. Cel Biol 159, 1071–1086. doi:10.1083/jcb.200205014
Díaz-González, F., Forsyth, J., Steiner, B., and Ginsberg, M. H. (1996). Trans-dominant Inhibition of Integrin Function. MBoC 7, 1939–1951. doi:10.1091/mbc.7.12.1939
Dieterle, M. P., Husari, A., Rolauffs, B., Steinberg, T., and Tomakidi, P. (2021). Integrins, Cadherins and Channels in Cartilage Mechanotransduction: Perspectives for Future Regeneration Strategies. Expert Rev. Mol. Med. 23, e14. doi:10.1017/erm.2021.16
Faralli, J. A., Desikan, H., Peotter, J., Kanneganti, N., Weinhaus, B., Filla, M. S., et al. (2019a). Proteomic Analyses of Phagocytosis in Dexamethasone (DEX)-treated Human Trabecular Meshwork (TM) Cells Reveals a Role for Gulp1 and ABR. Mol. Vis. 25, 237–254.
Faralli, J. A., Filla, M. S., and Peters, D. M. (2019b). Effect of αvβ3 Integrin Expression and Activity on Intraocular Pressure. Invest. Ophthalmol. Vis. Sci. 60, 1776–1788. doi:10.1167/iovs.18-26038
Faralli, J. A., Filla, M. S., and Peters, D. M. (2019c). Role of Fibronectin in Primary Open Angle Glaucoma. Cells 8, 1518. doi:10.3390/cells8121518
Faralli, J. A., Gagen, D., Filla, M. S., Crotti, T. N., and Peters, D. M. (2013). Dexamethasone Increases αvβ3 Integrin Expression and Affinity through a Calcineurin/NFAT Pathway. Biochim. Biophys. Acta (Bba) - Mol. Cel Res. 1833, 3306–3313. doi:10.1016/j.bbamcr.2013.09.020
Filla, M. S., Faralli, J. A., Desikan, H., Peotter, J. L., Wannow, A. C., and Peters, D. M. (2019). Activation of αvβ3 Integrin Alters Fibronectin Fibril Formation in Human Trabecular Meshwork Cells in a ROCK-independent Manner. Invest. Ophthalmol. Vis. Sci. 60, 3897–3913. doi:10.1167/iovs.19-27171
Filla, M. S., Faralli, J. A., Peotter, J. L., and Peters, D. M. (2017). The Role of Integrins in Glaucoma. Exp. Eye Res. 158, 124–136. doi:10.1016/j.exer.2016.05.011
Filla, M. S., Meyer, K. K., Faralli, J. A., and Peters, D. M. (2021). Overexpression and Activation of αvβ3 Integrin Differentially Affects TGFβ2 Signaling in Human Trabecular Meshwork Cells. Cells 10, 1923. doi:10.3390/cells10081923
Filla, M. S., Schwinn, M. K., Nosie, A. K., Clark, R. W., and Peters, D. M. (2011). Dexamethasone-Associated Cross-Linked Actin Network Formation in Human Trabecular Meshwork Cells Involves β3 Integrin Signaling. Invest. Ophthalmol. Vis. Sci. 52, 2952–2959. doi:10.1167/iovs.10-6618
Filla, M. S., Schwinn, M. K., Sheibani, N., Kaufman, P. L., and Peters, D. M. (2009). Regulation of Cross-Linked Actin Network (CLAN) Formation in Human Trabecular Meshwork (HTM) Cells by Convergence of Distinct β1 and β3 Integrin Pathways. Invest. Ophthalmol. Vis. Sci. 50, 5723–5731. doi:10.1167/iovs.08-3215
Filla, M. S., Woods, A., Kaufman, P. L., and Peters, D. M. (2006). β1 and β3 Integrins Cooperate to Induce Syndecan-4-Containing Cross-Linked Actin Networks in Human Trabecular Meshwork Cells. Invest. Ophthalmol. Vis. Sci. 47, 1956–1967. doi:10.1167/iovs.05-0626
Fleenor, D. L., Shepard, A. R., Hellberg, P. E., Jacobson, N., Pang, I.-H., and Clark, A. F. (2006). TGFβ2-Induced Changes in Human Trabecular Meshwork: Implications for Intraocular Pressure. Invest. Ophthalmol. Vis. Sci. 47, 226–234. doi:10.1167/iovs.05-1060
Flügel-Koch, C., Ohlmann, A., Fuchshofer, R., Welge-Lüssen, U., and Tamm, E. R. (2004). Thrombospondin-1 in the Trabecular Meshwork: Localization in Normal and Glaucomatous Eyes, and Induction by TGF-Β1 and Dexamethasone In Vitro. Exp. Eye Res. 79, 649–663. doi:10.1016/j.exer.2004.07.005
Fuchshofer, R., and Tamm, E. R. (2012). The Role of TGF-β in the Pathogenesis of Primary Open-Angle Glaucoma. Cell Tissue Res 347, 279–290. doi:10.1007/s00441-011-1274-7
Gagen, D., Filla, M. S., Clark, R., Liton, P., and Peters, D. M. (2013). Activated αvβ3 Integrin Regulates αvβ5 Integrin-Mediated Phagocytosis in Trabecular Meshwork Cells. Invest. Ophthalmol. Vis. Sci. 54, 5000–5011. doi:10.1167/iovs.13-12084
Gao, A. G., Lindberg, F. P., Dimitry, J. M., Brown, E. J., and Frazier, W. A. (1996). Thrombospondin Modulates Alpha V Beta 3 Function through Integrin-Associated Protein. J. Cel Biol 135, 533–544. doi:10.1083/jcb.135.2.533
Goldfinger, L. E., Tzima, E., Stockton, R., Kiosses, W. B., Kinbara, K., Tkachenko, E., et al. (2008). Localized α4 Integrin Phosphorylation Directs Shear Stress-Induced Endothelial Cell Alignment. Circ. Res. 103, 177–185. doi:10.1161/circresaha.108.176354
Gonzalez, A. M., Bhattacharya, R., deHart, G. W., and Jones, J. C. R. (2010). Transdominant Regulation of Integrin Function: Mechanisms of Crosstalk. Cell Signal. 22, 578–583. doi:10.1016/j.cellsig.2009.10.009
Gonzalez, J. M., Faralli, J. A., Peters, J. M., Newman, J. R., and Peters, D. M. (2006). Effect of Heparin II Domain of Fibronectin on Actin Cytoskeleton and Adherens Junctions in Human Trabecular Meshwork Cells. Invest. Ophthalmol. Vis. Sci. 47, 2924–2931. doi:10.1167/iovs.06-0038
Gonzalez, J. M., Hu, Y., Gabelt, B. A. T., Kaufman, P. L., and Peters, D. M. (2009). Identification of the Active Site in the Heparin II Domain of Fibronectin that Increases Outflow Facility in Cultured Monkey Anterior Segments. Invest. Ophthalmol. Vis. Sci. 50, 235–241. doi:10.1167/iovs.08-2143
Han, D. C., Rodriguez, L. G., and Guan, J.-L. (2001). Identification of a Novel Interaction between Integrin β1 and 14-3-3β. Oncogene 20, 346–357. doi:10.1038/sj.onc.1204068
Hinz, B. (2006). Masters and Servants of the Force:The Role of Matrix Adhesions in Myofibroblast Force Perception And Transmission. Euro J Cell Biol. 85, 175–181.
Hinz, B., and Gabbiani, G. (2010). Fibrosis: Recent Advances In Myofibroblast Biology and New Therapeutic Perspectives. F1000 Biology Reports, 2.
Hinz, B., Phan, S. H., Thannickal, V. J., Prunotto, M., Desmouliere, A., Varga, J., et al. (2012). Recent Developments In Myofibroblast Biology: Paradigms For Connective Tissue Remodeling. Am. J. Path. 180, 1340–1355.
Hoare, M.-J., Grierson, I., Brotchie, D., Pollock, N., Cracknell, K., and Clark, A. F. (2009). Cross-linked Actin Networks (CLANs) in the Trabecular Meshwork of the normal and Glaucomatous Human Eye In Situ. Invest. Ophthalmol. Vis. Sci. 50, 1255–1263. doi:10.1167/iovs.08-2706
Humphries, J. D., Byron, A., and Humphries, M. J. (2006). Integrin Ligands at a Glance. J. Cel Sci 119, 3901–3903. doi:10.1242/jcs.03098
Huveneers, S., and Danen, E. H. J. (2009). Adhesion Signaling - Crosstalk between Integrins, Src and Rho. J. Cel Sci 122, 1059–1069. doi:10.1242/jcs.039446
Iozzo, R. V., and Gubbiotti, M. A. (2018). Extracellular Matrix: The Driving Force of Mammalian Diseases. Matrix Biol. 71-72, 1–9. doi:10.1016/j.matbio.2018.03.023
Johnstone, M., Xin, C., Tan, J., Martin, E., Wen, J., and Wang, R. K. (2021). Aqueous Outflow Regulation - 21st century Concepts. Prog. Retin. Eye Res. 83, 100917. doi:10.1016/j.preteyeres.2020.100917
Junglas, B., Yu, A. H. L., Welge-Lüssen, U., Tamm, E. R., and Fuchshofer, R. (2009). Connective Tissue Growth Factor Induces Extracellular Matrix Deposition in Human Trabecular Meshwork Cells. Exp. Eye Res. 88, 1065–1075. doi:10.1016/j.exer.2009.01.008
Kassner, P. D., Alon, R., Springer, T. A., and Hemler, M. E. (1995). Specialized Functional Properties of the Integrin Alpha 4 Cytoplasmic Domain. MBoC 6, 661–674. doi:10.1091/mbc.6.6.661
Katz, B.-Z., Zamir, E., Bershadsky, A., Kam, Z., Yamada, K. M., and Geiger, B. (2000). Physical State of the Extracellular Matrix Regulates the Structure and Molecular Composition of Cell-Matrix Adhesions. MBoC 11, 1047–1060. doi:10.1091/mbc.11.3.1047
Kechagia, J. Z., Ivaska, J., and Roca-Cusachs, P. (2019). Integrins as Biomechanical Sensors of the Microenvironment. Nat. Rev. Mol. Cel Biol 20, 457–473. doi:10.1038/s41580-019-0134-2
Keller, K. E., and Peters, D. M. (2022). Pathogenesis of Glaucoma: Extracellular Matrix Dysfunction in the Trabecular meshwork‐A Review. Clin. Exper Ophthalmol. 50, 163–182. doi:10.1111/ceo.14027
Kelsh-Lasher, R. M., Ambesi, A., Bertram, C., and Mckeown-Longo, P. J. (2017). Integrin α4β1 and TLR4 Cooperate to Induce Fibrotic Gene Expression in Response to Fibronectin's EDA Domain. J. Invest. Dermatol. 137, 2505–2512. doi:10.1016/j.jid.2017.08.005
Laudanna, C., Kim, J. Y., Constantin, G., and Butcher, E. C. (2002). Rapid Leukocyte Integrin Activation by Chemokines. Immunol. Rev. 186, 37–46. doi:10.1034/j.1600-065x.2002.18604.x
Lazarides, E. (1976). Actin, Alpha-Actinin, and Tropomyosin Interaction in the Structural Organization of Actin Filaments in Nonmuscle Cells. J. Cel Biol. 68, 202–219. doi:10.1083/jcb.68.2.202
Li, J., Su, Y., Xia, W., Qin, Y., Humphries, M. J., Vestweber, D., et al. (2017). Conformational Equilibria and Intrinsic Affinities Define Integrin Activation. EMBO J. 36, 629–645. doi:10.15252/embj.201695803
Li, X., Acott, T. S., Nagy, J. I., and Kelley, M. J. (2020). ZO-1 Associates with α3 Integrin and Connexin43 in Trabecular Meshwork and Schlemm's Canal Cells. Int. J. Physiol. Pathophysiol Pharmacol. 12, 1–10.
Liu, S., Kiosses, W. B., Rose, D. M., Slepak, M., Salgia, R., Griffin, J. D., et al. (2002). A Fragment of Paxillin Binds the α4Integrin Cytoplasmic Domain (Tail) and Selectively Inhibits α4-Mediated Cell Migration. J. Biol. Chem. 277, 20887–20894. doi:10.1074/jbc.m110928200
Liu, S., Rose, D. M., Han, J., and Ginsberg, M. H. (2000). α4 Integrins in Cardiovascular Development and Diseases. Trends Cardiovasc. Med. 10, 253–257. doi:10.1016/s1050-1738(00)00073-6
Mao, Y., and Finnemann, S. C. (2012). Essential Diurnal Rac1 Activation during Retinal Phagocytosis Requires αvβ5 Integrin but Not Tyrosine Kinases Focal Adhesion Kinase or Mer Tyrosine Kinase. MBoC 23, 1104–1114. doi:10.1091/mbc.e11-10-0840
Matsumoto, Y., and Johnson, D. H. (1997a). Dexamethasone Decreases Phagocytosis by Human Trabecular Meshwork Cells In Situ. Invest. Ophthalmol. Vis. Sci. 38, 1902–1907.
Matsumoto, Y., and Johnson, D. H. (1997b). Trabecular Meshwork Phagocytosis in Glaucomatous Eyes. Ophthalmologica 211, 147–152. doi:10.1159/000310782
Medina-Ortiz, W. E., Belmares, R., Neubauer, S., Wordinger, R. J., and Clark, A. F. (2013). Cellular Fibronectin Expression in Human Trabecular Meshwork and Induction by Transforming Growth Factor-β2. Invest. Ophthalmol. Vis. Sci. 54, 6779–6788. doi:10.1167/iovs.13-12298
Moreno-Layseca, P., Icha, J., Hamidi, H., and Ivaska, J. (2019). Integrin Trafficking in Cells and Tissues. Nat. Cel Biol 21, 122–132. doi:10.1038/s41556-018-0223-z
Morgan, M. R., Hamidi, H., Bass, M. D., Warwood, S., Ballestrem, C., and Humphries, M. J. (2013). Syndecan-4 Phosphorylation Is a Control Point for Integrin Recycling. Dev. Cel 24, 472–485. doi:10.1016/j.devcel.2013.01.027
Morgan, M. R., Humphries, M. J., and Bass, M. D. (2007). Synergistic Control of Cell Adhesion by Integrins and Syndecans. Nat. Rev. Mol. Cel Biol 8, 957–969. doi:10.1038/nrm2289
Murphy, D. A., and Courtneidge, S. A. (2011). The 'ins' and 'outs' of Podosomes and Invadopodia: Characteristics, Formation and Function. Nat. Rev. Mol. Cel Biol 12, 413–426. doi:10.1038/nrm3141
Peotter, J. L., Phillips, J., Tong, T., Dimeo, K., Gonzalez, J. M., and Peters, D. M. (2016). Involvement of Tiam1, RhoG and ELMO2/ILK in Rac1-Mediated Phagocytosis in Human Trabecular Meshwork Cells. Exp. Cel Res. 347, 301–311. doi:10.1016/j.yexcr.2016.08.009
Peterson, J. A., Sheibani, N., David, G., Garcia-Pardo, A., and Peters, D. M. (2004). Heparin II Domain of Fibronectin Uses Alpha4beta1 Integrin to Control Focal Adhesion and Stress Fiber Formation, Independent of Syndecan-4. J. Biol. Chem. 280, 6915–6922. doi:10.1074/jbc.M406625200
Radel, C., Carlile-Klusacek, M., and Rizzo, V. (2007). Participation of Caveolae in β1 Integrin-Mediated Mechanotransduction. Biochem. Biophysical Res. Commun. 358, 626–631. doi:10.1016/j.bbrc.2007.04.179
Rao, P. V., Deng, P., Maddala, R., Epstein, D. L., Li, C. Y., and Shimokawa, H. (2005). Expression of Dominant Negative Rho-Binding Domain of Rho-Kinase in Organ Cultured Human Eye Anterior Segments Increases Aqueous Humor Outflow. Mol. Vis. 11, 288–297.
Rao, P. V., Deng, P. F., Kumar, J., and Epstein, D. L. (2001). Modulation of Aqueous Humor Outflow Facility by the Rho Kinase-Specific Inhibitor Y-27632. Invest. Ophthalmol. Vis. Sci. 42, 1029–1037.
Roberts, A. L., Mavlyutov, T. A., Perlmutter, T. E., Curry, S. M., Harris, S. L., Chauhan, A. K., et al. (2020). Fibronectin Extra Domain A (FN-EDA) Elevates Intraocular Pressure through Toll-like Receptor 4 Signaling. Sci. Rep. 10. doi:10.1038/s41598-020-66756-6
Roca-Cusachs, P., Gauthier, N. C., Del Rio, A., and Sheetz, M. P. (2009). Clustering of α 5 β 1 Integrins Determines Adhesion Strength Whereas αVβ3 and Talin Enable Mechanotransduction. Proc. Natl. Acad. Sci. U.S.A. 106, 16245–16250. doi:10.1073/pnas.0902818106
Rohen, J. W., Lütjen-Drecoll, E., Flügel, C., Meyer, M., and Grierson, I. (1993). Ultrastructure of the Trabecular Meshwork in Untreated Cases of Primary Open-Angle Glaucoma (POAG). Exp. Eye Res. 56, 683–692. doi:10.1006/exer.1993.1085
Rohen, J. W., and Lutjen-Drecoll, E. (1989). “Morphology of Aqueous Outflow Pathways in Normal and Glaucomatous Eyes,” in The Glaucomas. Editors R. Ritch, M. B. Shields, and T. Krupin (St Louis: C. V. Mosby).
Ross, R. (2004). Molecular and Mechanical Synergy: Cross-Talk between Integrins and Growth Factor Receptors. Cardiovasc. Res. 63, 381–390. doi:10.1016/j.cardiores.2004.04.027
Ross, T. D., Coon, B. G., Yun, S., Baeyens, N., Tanaka, K., Ouyang, M., et al. (2013). Integrins in Mechanotransduction. Curr. Opin. Cel Biol. 25, 613–618. doi:10.1016/j.ceb.2013.05.006
Sabanay, I., Gabelt, B. T., Tian, B., Kaufman, P. L., and Geiger, B. (2000). H-7 Effects on the Structure and Fluid Conductance of Monkey Trabecular Meshwork. Arch. Ophthalmol. 118, 955–962.
Santas, A. J., Bahler, C., Peterson, J. A., Filla, M. S., Kaufman, P. L., Tamm, E. R., et al. (2003). Effect of Heparin II Domain of Fibronectin on Aqueous Outflow in Cultured Anterior Segments of Human Eyes. Invest. Ophthalmol. Vis. Sci. 44, 4796–4801. doi:10.1167/iovs.02-1083
Schiller, H. B., Hermann, M.-R., Polleux, J., Vignaud, T., Zanivan, S., Friedel, C. C., et al. (2013). β1- and αv-class Integrins Cooperate to Regulate Myosin II during Rigidity Sensing of Fibronectin-Based Microenvironments. Nat. Cel Biol 15, 625–636. doi:10.1038/ncb2747
Schwinn, M. K., Gonzalez, J. M., Gabelt, B. A. T., Sheibani, N., Kaufman, P. L., and Peters, D. M. (2010). Heparin II Domain of Fibronectin Mediates Contractility through an α4β1 Co-signaling Pathway. Exp. Cel Res. 316, 1500–1512. doi:10.1016/j.yexcr.2010.03.010
Seetharaman, S., and Etienne-Manneville, S. (2018). Integrin Diversity Brings Specificity in Mechanotransduction. Biol. Cel 110, 49–64. doi:10.1111/boc.201700060
Sheppard, A. M., Onken, M. D., Rosen, C. D., Noakes, P. G., and Dean, D. C. (1994). Expanding Roles for α4 Integrin and its Ligands in Development. Cell Adhes. Commun. 2, 27–43. doi:10.3109/15419069409014200
Smith, M. L., Gourdon, D., Little, W. C., Kubow, K. E., Eguiluz, R. A., Luna-Morris, S., et al. (2007). Force-induced Unfolding of Fibronectin in the Extracellular Matrix of Living Cells. Plos Biol. 5, e268. doi:10.1371/journal.pbio.0050268
Stepp, M. A., Urry, L. A., and Hynes, R. O. (1994). Expression of α4 Integrin mRNA and Protein and Fibronectin in the Early Chicken Embryo. Cel Adhes. Commun. 2, 359–375. doi:10.3109/15419069409014210
Sun, X., Fu, Y., Gu, M., Zhang, L., Li, D., Li, H., et al. (2016a). Activation of Integrin α5 Mediated by Flow Requires its Translocation to Membrane Lipid Rafts in Vascular Endothelial Cells. Proc. Natl. Acad. Sci. U.S.A. 113, 769–774. doi:10.1073/pnas.1524523113
Sun, Z., Guo, S. S., and Fässler, R. (2016b). Integrin-mediated Mechanotransduction. J. Cel Biol 215, 445–456. doi:10.1083/jcb.201609037
Tsukamoto, T., Kajiwara, K., Nada, S., and Okada, M. (2018). Src Mediates TGF‐β‐induced Intraocular Pressure Elevation in Glaucoma. J. Cel Physiol 234, 1730–1744. doi:10.1002/jcp.27044
Tzima, E., Angel Del Pozo, M., Shattil, S. J., Chien, S., and Schwartz, M. A. (2001). Activation of Integrins in Endothelial Cells by Fluid Shear Stress Mediates Rho-dependent Cytoskeletal Alignment. EMBO J. 20, 4639–4647. doi:10.1093/emboj/20.17.4639
Vicente-Manzanares, M., and Sánchez-Madrid, F. (2018). Targeting the Integrin Interactome in Human Disease. Curr. Opin. Cel Biol. 55, 17–23. doi:10.1016/j.ceb.2018.05.010
Vranka, J. A., Kelley, M. J., Acott, T. S., and Keller, K. E. (2015). Extracellular Matrix in the Trabecular Meshwork: Intraocular Pressure Regulation and Dysregulation in Glaucoma. Exp. Eye Res. 133, 112–125. doi:10.1016/j.exer.2014.07.014
Wang, X., and Ha, T. (2013). Defining Single Molecular Forces Required to Activate Integrin and Notch Signaling. Science 340, 991–994. doi:10.1126/science.1231041
Zhang, Q., Checovich, W. J., Peters, D. M., Albrecht, R. M., and Mosher, D. F. (1994). Modulation of Cell Surface Fibronectin Assembly Sites By Lysophosphatidic Acid. J. Cell Biol. 127, 1447–1459. doi:10.1083/jcb.127.5.1447
Zhong, C., Chrzanowska Wodnicka, M., Brown, J., Shaub, A., Belkin, A. M., and Burridge, K. (1998). Rho-Mediated Contractility Exposes A Cryptic Site In Fibronectin And Induces Fibronectin Matrix Assembly. J. Cell Biol. 141, 539–551. doi:10.1083/jcb.141.2.539
Zhou, L., Zhang, S. R., and Yue, B. Y. (1996). Adhesion of Human Trabecular Meshwork Cells to Extracellular Matrix Proteins. Roles and Distribution of Integrin Receptors. Invest. Ophthalmol. Vis. Sci. 37, 104–113.
Zhou, L., Maruyama, I., Li, Y., Cheng, E. L., and Yue, B. Y. J. T. (1999). Expression of Integrin Receptors in the Human Trabecular Meshwork. Curr. Eye Res. 19, 395–402. doi:10.1076/ceyr.19.5.395.5297
Keywords: trabecular meshwork, glaucoma, integrin, extracelluar matix, crosstalk
Citation: Faralli JA, Filla MS and Peters DM (2022) Integrin Crosstalk and Its Effect on the Biological Functions of the Trabecular Meshwork/Schlemm’s Canal. Front. Cell Dev. Biol. 10:886702. doi: 10.3389/fcell.2022.886702
Received: 28 February 2022; Accepted: 05 April 2022;
Published: 29 April 2022.
Edited by:
Claudia Tanja Mierke, Leipzig University, GermanyReviewed by:
Ted Acott, Oregon Health and Science University, United StatesPadmanabhan Paranji Pattabiraman, Purdue University Indianapolis, United States
Vijaykrishna Raghunathan, University of Houston, United States
Copyright © 2022 Faralli, Filla and Peters. This is an open-access article distributed under the terms of the Creative Commons Attribution License (CC BY). The use, distribution or reproduction in other forums is permitted, provided the original author(s) and the copyright owner(s) are credited and that the original publication in this journal is cited, in accordance with accepted academic practice. No use, distribution or reproduction is permitted which does not comply with these terms.
*Correspondence: Donna M. Peters, ZG1wZXRlcjJAd2lzYy5lZHU=
†These authors share first authorship