- 1Center for Molecular Cardiology, University of Zurich, Schlieren, Switzerland
- 2Internal Medicine, Angiology and Atherosclerosis, Department of Medicine and Surgery, University of Perugia, Perugia, Italy
- 3First Clinic of Internal Medicine, Department of Internal Medicine, University of Genoa, Genoa, Italy
- 4IRCCS Ospedale Policlinico San Martino Genoa—Italian Cardiovascular Network, Genoa, Italy
- 5Department of Cardiology, University Heart Center, University Hospital Zurich, Zurich, Switzerland
- 6Department of Research and Education, University Hospital Zurich, Zurich, Switzerland
The improvements in healthcare services and quality of life result in a longer life expectancy and a higher number of aged individuals, who are inevitably affected by age-associated cardiovascular (CV) diseases. This challenging demographic shift calls for a greater effort to unravel the molecular mechanisms underlying age-related CV diseases to identify new therapeutic targets to cope with the ongoing aging "pandemic". Essential for protection against external pathogens and intrinsic degenerative processes, the inflammatory response becomes dysregulated with aging, leading to a persistent state of low-grade inflammation known as inflamm-aging. Of interest, inflammation has been recently recognized as a key factor in the pathogenesis of CV diseases, suggesting inflamm-aging as a possible driver of age-related CV afflictions and a plausible therapeutic target in this context. This review discusses the molecular pathways underlying inflamm-aging and their involvement in CV disease. Moreover, the potential of several anti-inflammatory approaches in this context is also reviewed.
Introduction
Life expectancy is steadily increasing due to improvements in medical treatment and living conditions, resulting in a growing portion of elderly individuals. Aging is an inevitable biological phenomenon characterized by a progressive deterioration of physiological functions. Consequently, aging is globally recognized as an independent risk factor for different diseases, including those of the CV system. Thus, the ever-increasing number of elderly people will eventually result in a “pandemic” of age-dependent CV disorders (Camici and Liberale, 2017). A better understanding of the specific role of aging in the development of CV pathologies and the molecular mechanisms underlying this association is, therefore, a matter of top priority.
Under physiological conditions, inflammation protects against external pathogens and intrinsic degenerative processes (Chen et al., 2018). Nevertheless, dysregulation of the immune system, as seen during aging, triggers a persistent state of low-grade inflammation, which has been recognized as an important driver for the development of age-related diseases (Ferrucci and Fabbri, 2018; Liberale et al., 2022; Tansey et al., 2022). This phenomenon, referred to as inflamm-aging, has been linked to a higher risk of CV events and has been increasingly recognized as a determinant of CV outcome (Liberale et al., 2020), This review summarizes the current knowledge about inflamm-aging and its involvement in the pathogenesis of age-related CV diseases. Finally, the potential of inflammation as a therapeutic target is also discussed based on available experimental and clinical evidence.
Molecular Determinants of Inflamm-Aging
Inflamm-aging was firstly theorized by Franceschi et al. in the 2000s as a phenomenon involved in the age-related deterioration of physiological processes. Defined as a chronic low-grade sterile inflammation, inflamm-aging was suggested to result from persistent antigenic load and stress (Franceschi et al., 2000). Since then, this concept has been intensively studied to identify its molecular mechanisms and how it contributes to age-dependent diseases. Even though the exact mechanisms of inflamm-aging are not yet fully elucidated, some pathologic features have been identified (Figure 1).
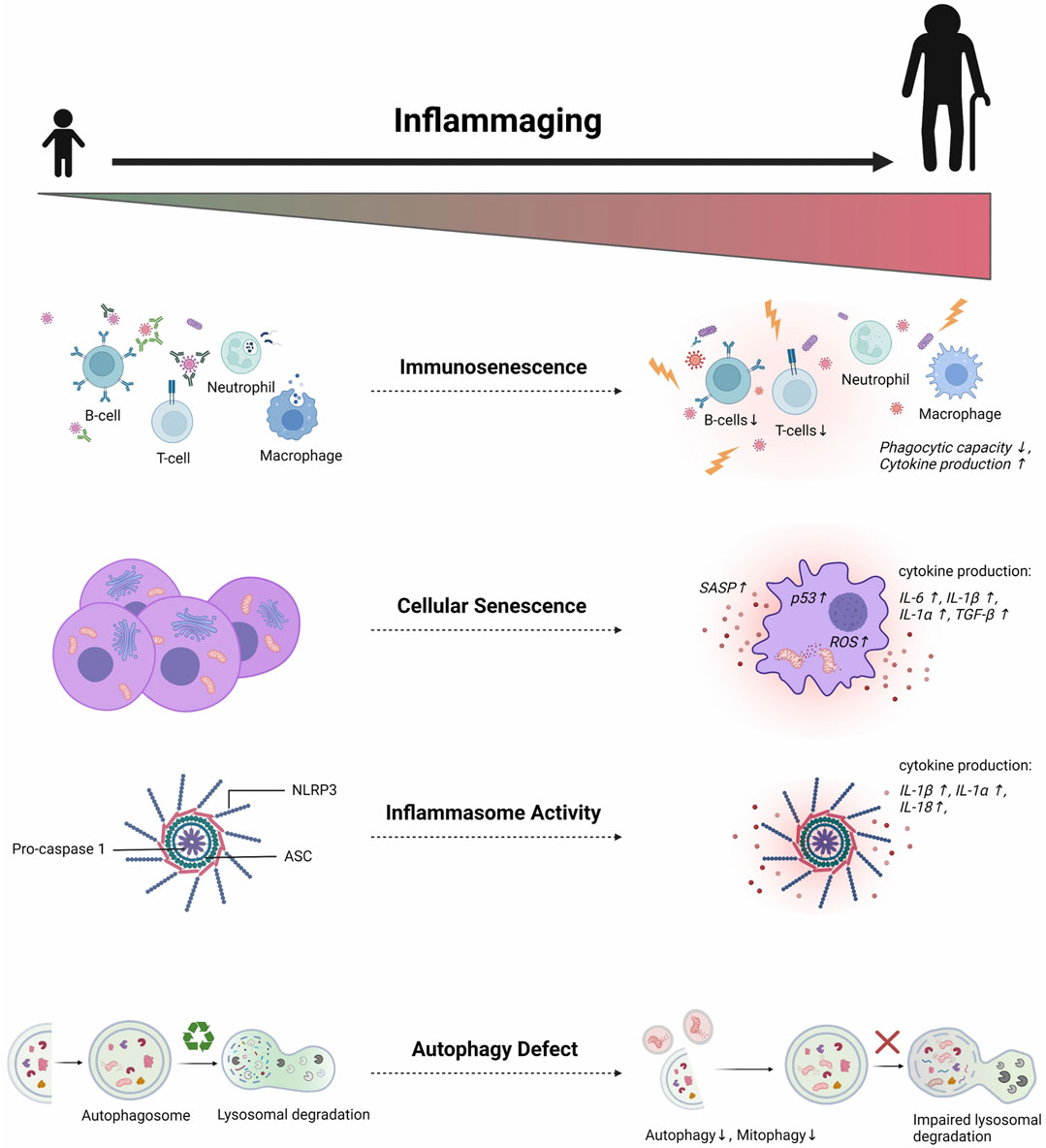
FIGURE 1. Multifactorial mechanisms of inflamm-aging. Several molecular pathways are involved in triggering age-related chronic low-grade inflammation. Aging affects both innate and adaptive immune systems, leading to sustained low-grade immune activation and reduced sensitivity to appropriate immunogenic stimuli. Such deterioration contributes to inadequate senescent cell clearance. Consequently, the accumulation of senescent cells induces chronic exposure to inflammatory SASP proteins. Aberrant inflammasome activation also occurs in aging due to defects in autophagy and mitophagy, thus further perpetuating the age-related pro-inflammatory milieu. SASP = senescence-associated secretory phenotype; ROS = Reactive oxygen species; NLRP3 = Nod-like receptor protein 3; ASC = Apoptosis speck-like protein; IL = Interleukin (Created with BioRender.com)
Cellular Senescence
Senescence is a phase of cell cycle, characterized by an irreversible replicative arrest (Olivieri et al., 2018; Sanada et al., 2018). Firstly reported by Hayflick and Moorhead, this phenomenon is a hallmark of aging and is driven by various intrinsic and extrinsic stressors, such as telomere shortening (Hayflick and Moorhead, 1961). As non-coding repetitive nucleotide sequences are located at the end of chromosomes, telomeres prevent chromosomal end-to-end fusion and instability (Vaiserman and Krasnienkov, 2021). In proliferating cells, telomeres shorten gradually at each cell division until they reach a critical length. Short telomeres cause chromosomal fusion and activation of transcription factor p53, ultimately leading to replicative senescence (RS) and apoptosis. (Toussaint et al., 2002; de Magalhães and Passos, 2018). On the other side, stress-induced premature senescence (SIPS) is a cellular phenotype developing in response to endogenous and exogenous stressors, such as oxidative stress, inflammation, and irradiation (Raghuram and Mishra, 2014), independently of telomere shortening (De Magalhães et al., 2002).
Being involved in physiological processes such as tissue regeneration and tumor suppression (Prata et al., 2018), senescence is increasingly considered a fundamental contributor to inflamm-aging. Indeed, senescent cells display a senescence-associated secretory phenotype (SASP), characterized by increased expression and release of pro-inflammatory cytokines, chemokines, and proteases, such as Interleukin (IL)-6, IL-1β, IL-1α, and TGF-β through NFkB (Van Deursen, 2014; Ferrucci and Fabbri, 2018; Rea et al., 2018; Docherty et al., 2020).
Molecular features of SASP are extremely heterogenous, depending on cell types and inducing factors. Nevertheless, the physiological goal of SASP is activating the immune cells to promote the clearance of senescent cells (Docherty et al., 2020). Aging of the immune system results in an inadequate elimination of senescent cells, which exponentially accumulate in multiple organs and thereby lead to a sustained pro-inflammatory condition (Ferrucci and Fabbri, 2018; Rea et al., 2018). Accordingly, accelerated senescence and an increased number of senescent cells characterize different chronic degenerative diseases, including atherosclerosis, cancer, and heart failure (Stojanovic et al., 2020).
Immunosenescence
Immunosenescence refers to a wide range of age-associated disorders of the immune system. Affecting both innate and adaptive immune responses, immunosenescence impact all functions of the immune system including defense against pathogens and aberrant cells, and long-term immunity (Weiskopf et al., 2009; Shaw et al., 2013). As a consequence, the elderly are exposed to a higher risk of acute infections, re-activation of chronic infections, cancers and vaccine failure (Weiskopf et al., 2009).
Innate immunity represents the front-line host defense. It consists of different components, including physical barriers, effector cells, and soluble mediators, providing a fast primary response against invading pathogens (Hato and Dagher, 2015). Aging affects all the components of innate immunity (Weiskopf et al., 2009). In particular, reduced phagocytic capacity is considered the hallmark of immunosenescence. An impairment of the respiratory burst, a pivotal mechanism in intracellular pathogen destruction, has been observed in neutrophils of both aged mice and elderly individuals (Davila et al., 1990; Butcher et al., 2001; Weiskopf et al., 2009; Shaw et al., 2013; Takahashi et al., 2016; Ventura et al., 2017; Lloberas et al., 2019). In addition, natural killer (NK) cells, which are crucial in suppressing viral infection and tumor cells, display an age-related dysfunction, despite the increased number (Weiskopf et al., 2009; Gounder et al., 2018). In detail, aging is associated with impaired response of NK cells to IL-2, reduced IFN- γ secretion and degranulation, resulting in the attenuation of their cytotoxic function (Beli et al., 2011; Camous et al., 2012; Shaw et al., 2013). Finally, dendritic cells (DCs) display impairment of antigen uptake and presentation to T cells. Monocyte-derived DCs from aged individuals are less effective than those of younger subjects in inducing T cell proliferation and production of IFN-γ (Agrawal et al., 2017). Indeed, the ability of DCs to prime CD8+ T cells in the elderly was found to be inadequate (Briceño et al., 2016). Nevertheless, the underlying mechanisms of the impact of age on DC function are still not fully understood and to this end, additional studies will be required.
Aging also affects the adaptive immune system. T cells maturate within the thymus, an organ undergoing a volume reduction and a functional decay alongside aging (Weiskopf et al., 2009), especially thymopoiesis and the central tolerance process. As a result, T cells maturation is impaired, leading to depletion of naïve T cells and increase of self-reactive T cells, thus facilitating autoimmune responses (Thomas et al., 2020). Similar to T cells, the number and function of B cells are both reduced in the elderly. B cells produce antibodies with a lower affinity toward antigens (Weiskopf et al., 2009), and this could be due to the deficient expression of CD28 co-stimulatory molecule on T cells (Boucher et al., 1998) (Weiskopf et al., 2009; Paudel et al., 2019; Pangrazzi and Weinberger, 2020). CD28− T cells have a pro-inflammatory phenotype, and the accumulation of CD8+CD28− T cells in elderly individuals correlates with a higher risk of age-related disease and mortality (Pangrazzi and Weinberger, 2020).
Hematopoietic stem cells (HSCs) -a key component of this process-maintain the production of all the lineage of blood cells in the body, including myeloid and lymphoid cells (Aw et al., 2007; Sawai et al., 2016). However, aging leads to their exhaustion resulting in lower capacity for self-renewal and deterioration of their differentiation ability, with significant consequences on both adaptive and innate immunity. Furthermore, with age, HSCs accumulate mutagenic events, which lead to positive selection and outgrowth of selected clones (Khetarpal et al., 2019; Mooney et al., 2021). The presence of clonal mutant stem cells without the development of overt hematologic malignancy and other clonal diseases is referred to as clonal hematopoiesis of indeterminate potential (CHIP) (Tyrrell and Goldstein, 2021; Libby and Ebert, 2018; Khetarpal et al., 2019). The most frequently encountered mutated genes in CHIP are DNA methyltransferase 3 (DNMT3A), ten-eleven-translocation 1 (TET2), Janus kinase 2 (Jak2), and additional sex comb-like 1 (ASXL1) (Jaiswal et al., 2014; Mooney et al., 2021). A cross-sectional study reported that the frequency of clonal somatic mutations in peripheral blood cells increases with age, reaching a prevalence of around 10% in individuals above 70 years, whereas they are rarely found in younger individuals (Jaiswal et al., 2014). Immune effector cells derived from mutated stem cells are functionally altered and favor a pro-inflammatory milieu (Leoni et al., 2017). Indeed, individuals with clonal hematopoiesis show higher circulating IL-6, TNF-α, and MCP-1 compared to age-matched individuals (Mooney et al., 2021).
NLRP3 Inflammasome Activity
The Nod-like receptor protein 3 (NLRP3) inflammasome complex consists of NLRP3, apoptosis speck-like protein (ASC), and pro-caspase 1. Upon stimulation, NLRP3 inflammasome activates caspase-1, which cleaves the precursors of inflammatory cytokines, such as IL-1β, IL-1α, and IL-18 (Martinon et al., 2009; Rea et al., 2018; Liu et al., 2020). The activation of NLRP3 inflammasome consists of two essential steps named priming and triggering. NLRP3 priming is ignited by stress signals, including endogenous danger-associated molecular patterns (DAMPs) and exogenous pathogen-associated molecular patterns (PAMPs) molecules, which results in NF-κB activation and the consequent upregulation of NLRP3, which is otherwise low in unstimulated cells (Boaru et al., 2015; Afonina et al., 2017; Kelley et al., 2019). Upregulation of NLRP3 promotes a cascade of events (triggering), leading to the assembly of the NLRP3 inflammasome complex and its activation (Afonina et al., 2017; Kelley et al., 2019). In the absence of NLRP3 and ASC, old healthy animals present lower expression of IL-1β and IL-18 than the control group, suggesting the involvement of NLRP3 inflammasome in inflamm-aging (Youm et al., 2013). This age-related NLRP3 activation could be due to the accumulation of DAMPs along with aging, including adenosine triphosphate, uric acid, and cholesterol crystals (Youm et al., 2013).
Autophagy and mitophagy are intracellular processes dedicated to the removal of dysfunctional cytoplasmic materials and misfolded proteins and, ultimately, regulate inflammasome activation. (Barbosa et al., 2019; Biasizzo and Kopitar-Jerala, 2020). Autophagy activity is compromised in aging due to impaired epigenetic and transcriptional regulation of autophagy genes; thus, progressive accumulation of damaged organelles typically occurs (Wong et al., 2020). Mitophagy–the selective removal process of damaged mitochondria–has a major role in age-related diseases, including those of the CV system. Mitophagy preserves mitochondrial integrity and quality control along with mitochondrial fission and fusion (Chen et al., 2020). The accumulation of Parkin, an essential protein that amplifies the polyubiquitination of mitochondrial surface proteins to initiate mitophagy, is detected in aged cardiomyocytes, suggesting defective mitophagy in the aging heart (Thai et al., 2019; Ajoolabady et al., 2020). Mitophagy is impaired in aging as a result of downregulation of crucial elements, such as PTEN-induced kinase (PINK)-1 (Ajoolabady et al., 2020).
Previous studies have identified the involvement of mtROS and mtDNA, which are released by damaged mitochondria, in triggering NLRP3 inflammasome activation (Zhou et al., 2011; Elliott and Sutterwala, 2015; Grazioli and Pugin, 2018; Biasizzo and Kopitar-Jerala, 2020). Interestingly, this mechanism leads to a vicious circle, where caspase-1 further inhibits mitophagy, thus amplifying the mitochondrial damage. (Yu et al., 2014). Oxidized mtDNA was demonstrated to directly bind to NLRP3, resulting in NLRP3 inflammasome activation and increased IL-1β production (Shimada et al., 2012). Furthermore, mtROS induce NLRP3 inflammasome activity also indirectly via NF-κB pathway (Ferrucci and Fabbri, 2018).
Inflamm-Aging and CV Diseases
Chronic low-grade inflammation levels, characterizing inflamm-aging, have been increasingly recognized as a crucial aspect of different CV diseases. Indeed, evidence indicates the contribution of the inflammatory process to the pathogenesis and outcome of age-related CV diseases (Ruparelia et al., 2017; Ferrucci and Fabbri, 2018; Liberale et al., 2018, 2020; Bonetti et al., 2019). As research in this field progresses, the impact of inflamm-aging and its underlying mechanism on CV diseases begins to be elucidated.
Atherosclerosis
As the underlying pathological process of many CV diseases, atherosclerosis shows a strong association with age, and it is extremely frequent in elderly individuals. The role of inflammation in this process and its interplay with modified lipids have been increasingly recognized (Libby, 2013; Stojanovic et al., 2020; Ministrini et al., 2021a).
Atherogenesis starts at a very young age with the accumulation of modified lipoproteins within the vessel wall and escalates with advanced age. The progression of atherosclerosis is facilitated by endothelial cell dysfunction due to increased ROS generation (Ross, 1999; Ruparelia et al., 2017; Tyrrell and Goldstein, 2021). Indeed, the dysfunctional endothelium favors the uptake of lipoproteins and the recruitment of immune cells in the vascular wall, accelerating plaque formation. (Galkina and Ley, 2007). Notably, a higher number of macrophages infiltrating the wall is detected in the atherosclerotic aortas of aged mice with hyperlipidemia compared to young animals (Du et al., 2016). Also, in comparison to young animals, aged mice on high-fat diet display higher levels of IL-6 and macrophage-attracting chemokines in their arteries (Du et al., 2016). Once beneath the endothelial layer, monocytes differentiate into macrophages, internalize modified lipoproteins and transform into foam cells. Accumulated apoptotic foam cells integrate into the lipid necrotic core of the atherosclerotic plaque and increase its instability (Ruparelia et al., 2017; Rea et al., 2018). Such a process further sustains a state of chronic unresolved inflammation, as the lesion itself contains antigens that contribute to increasing pro-inflammatory mediators. Among others, cholesterol crystals induce the activation of the NLRP3 inflammasome (Stojanovic et al., 2020). Moreover, senescent endothelial cells contribute to the higher NLRP3 activity due to upregulated oxidative stress and defect of autophagy (Rea et al., 2018).
As atherosclerosis advances, an increased number of senescent cells accumulates within the lesion. These cells express SASP, consisting of various pro-inflammatory cytokines, growth factors, and proteases, which exacerbate the inflammatory reaction, promote plaque growth and cause its destabilization (Childs et al., 2015; Stojanovic et al., 2020). For instance, metalloproteinases secreted by senescent cells digest the plaque’s fibrous cap, which becomes more prone to rupture (Libby, 2013). The pro-inflammatory milieu also results in an increased risk of thrombotic occlusion, the ultimate cause of most ischemic complications of atherosclerosis (Ferrucci and Fabbri, 2018; Soysal et al., 2020). Therefore, acting as one of the sources of chronic low-grade inflammation, senescent cells can be potential therapeutic targets to regulate atherosclerosis burden in the elderly (Stojanovic et al., 2020).
On the other hand, CHIP has also been proposed as a risk factor for atherosclerosis. In particular, mutations of TET2 are associated with inflammation and atherosclerosis. TET2-knockout atherosclerotic-prone animals develop larger aortic plaques than the control group (Fuster et al., 2017). Moreover, TET2 deficient macrophages respond to atherogenic stimuli such as LDL by releasing higher amounts of inflammatory mediators, such as IL-6, IL-1β, and IL-18 (Fuster et al., 2017; Jaiswal et al., 2017; Jaiswal and Ebert, 2019). Being involved in the DNA demethylation process, TET2 facilitates the suppression of IL-6 and IL-1β, inflammatory mediators implicated in the pathogenesis of atherosclerosis (Libby and Ebert, 2018; Cong et al., 2021). Indeed, the deleterious effect of TET2 knockdown can be reversed by treatment with the NLRP3 inhibitor MCC950, indicating the pivotal role of IL-1β/NLRP3 inflammasome in TET2 atherosclerosis-related signaling (Fuster et al., 2017).
Myocardial Infarction
A regenerative process of injured tissue is crucial after myocardial infarction (MI) (Karin and Clevers, 2016; Appel et al., 2021). However, the accumulation of senescent cells in the heart reduces its resilience to cardiac stress, and a gradual deterioration in tissue repair is strongly associated with aging (Owens et al., 2021). Following an ischemic event, elderly individuals display an impaired vascular response, including angiogenesis, which is driven by the inflammatory response (Fan et al., 2013). Indeed, aged animals display an inadequate inflammatory response and cardiac repair after MI, with consequent adverse remodeling and increased mortality (Bujak et al., 2008). By eliminating dead cells and initiating the healing process, an aptly and effective inflammatory response is critical for favorable cardiac repair after MI and to prevent its long-term complications, such as heart failure (HF) (Frantz et al., 2013; Rieckmann et al., 2019) I dysfunctional activation of immune cells, characterizing inflamm-aging, has been associated with a poorer prognosis after MI in the elderly (Ma et al., 2018).
Accumulating evidence demonstrates a pivotal role for macrophages in the myocardial homeostasis, in both physiologic conditions and response to injury, as they orchestrate the repair response (Nahrendorf and Swirski, 2013; Swirski and Nahrendorf, 2013). As discussed above, macrophage phagocytic capacity is impaired in aging, resulting in an unbalanced inflammatory response post-MI impacting cardiac healing (Ma et al., 2018). Specifically, impaired phagocytosis is associated with the reduced clearance of dead cardiomyocytes (Bujak et al., 2008). Moreover, a deviation of cardiac-resident macrophage subpopulations in the aging heart has been reported, showing an increased M1 subset and a reduction of M2 macrophages (Ma et al., 2018). Although a wide functional overlap exists, M1 macrophages are generally considered the pro-inflammatory subset since they secrete pro-inflammatory cytokines (i.e., TNF-α, IL-6, and IL-1β) and MMPs. In contrast, M2 macrophages release anti-inflammatory cytokines (e.g., IL-10) and pro-reparative factors, such as TGF-β and vascular endothelial growth factor (VEGF), responsible for extracellular matrix deposition, healing, and fibrosis (Ma et al., 2018; O’Rourke et al., 2019). An increased proportion of M1-to-M2 macrophages, as observed in aging animals, results in infarct size augmentation and extensive extracellular matrix remodeling (O’Rourke et al., 2019).
Inflammasome activity is also pivotal for myocardial response to ischemia. ASC is prominently expressed in the myocardium of patients with ischemic heart disease (Kawaguchi et al., 2011). Indeed, NLRP3, IL-1β, and IL-18 gene expression are markedly upregulated in the infarcted heart (Sandanger et al., 2013). In line with this finding, in vivo deletion of caspase-1 and NLRP3 yields a cardioprotective effect with smaller infarct size and preserved cardiac function (Kawaguchi et al., 2011). The release of inflammatory cytokines (i.e., IL-1β and IL-18) following inflammasome activation further impacts cardiac resident cells, promoting apoptosis and fibrosis (Mauro et al., 2019).
Heart Failure
Heart failure (HF) is the final stage of multiple structural and functional cardiac disorders, and it is one of the most common CV diseases among the elderly (Hoogen et al., 2019; Liu et al., 2020). Chronic inflammation and auto-immunity play an important role in the onset and progression of HF (Dick and Epelman, 2016; Hoogen et al., 2019), as demonstrated by the increased number of inflammatory cells, observed in the myocardium of chronic HF patients (Hoogen et al., 2019).
The role of aging as a risk factor for HF has been extensively investigated. Previous observation reported a dampened autophagy in the heart of aged animals (Xu et al., 2016). Moreover, increased telomere shortening and expression of senescence markers, such as p53 and p16Ink4a, were also observed in aging hearts, indicating a higher accumulation of senescent cells that might contribute to age-related cardiac dysfunction (Torella et al., 2004; Li et al., 2020). By developing a senescent phenotype, cardiomyocytes from old animals promote inflammation, cell death, and senescence in neighboring cells via SASP signaling (Li et al., 2020). Besides, nontypical SASP signals secretion from senescent cardiomyocytes has been observed to promote cardiac fibroblast activation. Indeed, clearance of senescent cells significantly reduces cardiac hypertrophy and fibrosis (Anderson et al., 2019).
Recently, the involvement of IL-1β in HF progression has been proposed. A previous in vivo study on outbred CD-1 mice demonstrated the induction of myocardial dysfunction by administration of recombinant IL-1β. Interestingly, the impairment was reversible, as the contractile dysfunction returned to baseline five days after the end of the treatment, suggesting the therapeutic potential for targeting IL-1β in HF (Van Tassell et al., 2013). Besides IL-1β, TNF-α, and IL-6 are involved in the pathogenesis of HF. Indeed, circulating levels of these cytokines predict survival in patients with HF (Moro-García et al., 2014). The increase of these pro-inflammatory cytokines was correlated to the increased expression of Toll-like receptor-4 (TLR-4)—similarly to what is observed in aging–both in the myocardium and in circulating monocytes from patients with chronic HF (Birks et al., 2004). Experimental models demonstrate a gradual increase in cardiac IL-6 expression along with aging. At the same time, its deficiency mitigates age-related cardiac dysfunction (Wang et al., 2020b). Interestingly, higher circulating levels of IL-6 have been reported in elderly patients with both HF and CHIP, particularly those with double somatic mutations of DNMT3A and TET2 genes (Pascual-Figal et al., 2021). Based on this observation, the involvement of age-related clonal hematopoiesis in the development of HF has been suggested. This notion is further supported by previous results from in vivo study showing that TET2 deficiency accelerates age-related cardiomyopathy with more pronounced cardiac hypertrophy and fibrosis (Wang et al., 2020a). Nonetheless, future studies will be required to investigate the role of CHIP in HF progression and its molecular mechanisms in more detail.
Aortic Aneurysm
Aortic aneurysm (AA) refers to a pathologic dilation of the aortic wall up to 1.5 times its normal diameter, with a tendency to further expand and rupture (Hellawell et al., 2021). The mortality rate among patients with AA reaches 81%, including pre- and in-hospital deaths, thus underscoring its severe nature. Age is one of the most relevant risk factors for AA. Indeed, the risk of developing AA increases significantly, up to 40% above 65 years of age (Hellawell et al., 2021).
Described initially as a noninflammatory lesion, characterized by a loss of vascular smooth muscle cells and the fragmentation of vascular connective tissue, AA is nowadays considered the result of chronic inflammation in the vessel wall, which also involves inflammasome activity. Histological sections of AA show accumulation and infiltration of macrophages and lymphocytes in the aortic media and adventitia (Yuan et al., 2021), associated with a higher expression of ASC, caspase-1, and NLRP3 (Wortmann et al., 2021). Moreover, age-associated upregulation of CASP1 and IL1B gene expression was also detected in peripheral blood mononuclear cells (PBMC) of patients with AA, suggesting a close relationship between AA and inflamm-aging (Wu et al., 2015). NLRP3 inflammasome activation in AA was proposed to be mediated by circulating homocysteine–a methionine-derived sulfur-containing amino acid whose levels increase with age (Kuo et al., 2005). Indeed, previous in vivo studies demonstrated an aggravation of AA following homocysteine supplementation by activation of adventitial fibroblast and NLRP3 inflammasome in macrophages (Liu et al., 2012; Sun et al., 2015).
Future Perspective: Targeting Age-Related Inflammation in CV Disease
With mounting evidence about the crucial contribution of inflamm-aging in the pathogenesis of CV diseases, new therapeutic approaches targeting inflammatory molecular pathways have been increasingly investigated. Several clinical trials investigated the efficacy of anti-inflammatory compounds on CV diseases (Table 1). Unfortunately, elderly individuals are often under-represented in RCTs, resulting in an important lack of knowledge and missing the opportunity to implement personalized medicine.
Given its important role in the progression of atherogenesis, MI and HF, IL-1 has emerged as a promising therapeutic target, leading to the investigation of different compounds targeting its signaling pathways, such as recombinant human IL-1 receptor antagonist (Anakinra) and human monoclonal anti-IL-1β antibodies (Canakinumab and Gevokizumab), in the setting of CV diseases (Buckley and Abbate, 2018; Liberale et al., 2021). The randomized double-blind trial Canakinumab Anti-inflammatory Thrombosis Outcomes Study (CANTOS) assessed the efficacy of canakinumab in preventing recurrent CV events in individuals with previous MI and persistent systemic inflammation (hsCRP level ≥2 mg/L). Canakinumab, administered subcutaneously every three months in addition to standard therapy, reduced hsCRP level by 41% in the highest dose arm (300 mg) compared to placebo. Further, canakinumab successfully reduced the incidence of primary endpoints (i.e., non-fatal MI, non-fatal stroke, or CV death) when administered at 150 or 300 mg at a median follow-up of 3.7 years (Ridker et al., 2017). However, canakinumab-treated patients exhibited neutropenia and showed an increased risk of fatal infections (Ridker et al., 2017), thus reducing the possibility to use such an approach for long-term treatments.
Meanwhile, promising outcomes were also observed with anakinra. With a shorter half-life (6 h), anakinra shows a better safety profile, as its dose can be easily managed to prevent adverse effects (Buckley and Abbate, 2018). Previous clinical studies in rheumatoid arthritis patients, with or without coronary artery disease, showed that a single dose of anakinra improves left ventricular and coronary vascular function (Ikonomidis et al., 2008; Ikonomidis et al., 2014). Blunted inflammatory response, defined as a reduction of hsCRP level, was also observed in ST-segment elevation MI and acute decompensated heart failure patients after 14 days of anakinra treatment (Abbate et al., 2013; Van Tassell et al., 2016). Nevertheless, secondary analysis of the MRC-ILA Heart Study showed that 14 days-long treatment with anakinra in non-ST-segment elevation MI patients does not improve long-term clinical outcomes. Instead, one year after anakinra discontinuation, a significant higher MACE incidence was observed in the treatment group, likely driven by a non-significant increase in recurrent myocardial infarction (Morton et al., 2015).
Even more promising results in secondary prevention of CV diseases were yielded by colchicine. The anti-inflammatory characteristics of colchicine are due to its inhibitory effect on microtubule formation. Through its effect, colchicine specifically affects different aspects of the inflammatory response, sparing from immune cell mobility to degranulation and including cell replication or cytokine release (Leung et al., 2015). Furthermore, colchicine also inhibits NLRP3 inflammasome polymerization (Martinon et al., 2009; Martínez et al., 2018). In 2013, the first Low Dose Colchicine (LoDoCo) trial was conducted on stable CAD patients, showing the beneficial effect of low-dose colchicine (0.5 mg/day) in reducing primary outcomes (i.e., the composite incidence of acute coronary syndrome, out-of-hospital cardiac arrest, or non-cardioembolic ischemic stroke) after 3-year median follow-up (Nidorf et al., 2013). However, LoDoCo was a relatively small trial. Thus, this study prompted additional trials. In 2019, the results of the large-scale Colchine Cardiovascular Outcomes Trial (COLCOT) confirmed its beneficial cardiovascular effect. At a median follow-up of 22.6 months, COLCOT demonstrated that low-dose colchicine reduces the risk of CV events in patients with a recent MI, mainly driven by a reduced incidence of stroke and urgent hospitalization for non-ST elevated MI or unstable angina (Tardif et al., 2019). More recently, the second LoDoCo trial (LoDoCo2) confirmed the encouraging results in the setting of chronic coronary disease, demonstrating a significantly lower occurrence of CV events in colchicine-treated patients compared to placebo (Nidorf et al., 2020). On the contrary, the Colchicine in Patients with Acute Coronary Study (COPS) multicenter trial reported no significant difference in primary endpoints between patients treated with low-dose oral colchicine and controls following acute coronary syndrome (ACS), whereas higher sepsis-related mortality in colchicine-treated patients was observed (Tong et al., 2020). Interestingly, a significant reduction in the primary end point in the colchicine group compared to the control was reported on the second year follow-up of this study after cessation at 12 months (Tong et al., 2021), suggesting the possible beneficial effect of the addition of low-dose colchicine to the standard medical therapy of ACS in the acute setting.
Regardless of the favorable outcomes of canakinumab and colchicine in reducing secondary CV events, other anti-inflammatory drugs show no beneficial effect. The Cardiovascular Inflammation Reduction Trial (CIRT) investigated methotrexate in patients with stable atherosclerosis against placebo and yielded neutral results, showing no effect on MACE incidence and IL-1β, IL-6, and hs-CRP levels (Ridker et al., 2019). Overall, the abovementioned results highlight the importance of understanding the specific contribution of the different inflammatory pathways in determining CV risk. Thus far, targeting the IL-1β pathway - either via direct inhibition or by intersecting the upstream signaling pathway - seems to be the most effective approach. Hence, NLRP3 inflammasome and caspase-1, the upstream mediators of IL-1β and several other inflammatory cytokines, are increasingly considered alternative therapeutical targets for CV prevention, and several inhibitory molecules against these proteins have been identified and are under investigation.
Recent in vivo studies have demonstrated the beneficial effect of MCC950 (a specific NLRP3 inhibitor) and VX-765 (a caspase-1 inhibitor) in a rodent model of MI, showing reduced infarct size, myocardial fibrosis and preserved cardiac function in MCC950 treated animals (Audia et al., 2018; Gao et al., 2019). Moreover, a reduced formation of atherosclerotic lesions presumably due to a reduction of macrophage influx was also observed in ApoE knockout mice treated with MCC950 for four weeks (Van Der Heijden et al., 2017). Collectively, these positive results highlight the potential of NLRP-3 and caspase-1 inhibitors as novel therapeutic agents for CV diseases and may set the stage for further investigations, particularly in advanced atherosclerosis.
Since senescent cells are the major source of inflammatory mediators in aging organisms, senolytics–a class of drugs that eliminates senescent cells–have been recently proposed as a pharmacological approach to prevent age-related CV afflictions, especially atherosclerosis. The first generation of senolytic drugs was identified from a transcriptome analysis in 2015 by Zhu et al., which included Dasatinib (D), a tyrosine kinase inhibitor, and Quercetin (Q), a flavonoid found in fruits and vegetables (Zhu et al., 2015; Ministrini et al., 2021b). Being approved for human use, both D and Q and their combination have been investigated as potential therapeutical compounds to treat age-related diseases (Kirkland and Tchkonia, 2020). A 3-months treatment with Dasatinib and Quercetin improves vascular function and calcification and increases p-eNOSser1177 levels in aged mice (Roos et al., 2016). Navitoclax is another potential senolytic drug that inhibits the apoptosis regulator protein B-cell lymphoma 2 (Bcl-2) (Walaszczyk et al., 2019). Aged mice receiving an intermittent treatment with Navitoclax displayed preserved cardiac function and improved survival following MI. However, the potential of senolytic agents as a new therapeutic strategy is still hampered by adverse effects. For instance, evidence of cardiotoxicity was reported in previous clinical trials following Dasatinib administration as a treatment of chronic myeloid leukemia (Chaar and Kamta, 2018). Moreover, Navitoclax administration induces severe thrombocytopenia due to Bcl-XL inhibition, which is essential for platelet survival (Schoenwaelder et al., 2011; Dookun et al., 2020).
Due to the substantial side effects displayed by different senolytics, an alternative approach targeting senescent cells using CAR T cells was recently proposed. Initially invented for cancer therapy, this approach utilizes genetically engineered T cells that express chimeric receptor proteins targeting senescence-specific surface antigens (Feucht and Abou-El-Enein, 2020). Senolytic CAR T cells have been tested in mice by targeting urokinase-type plasminogen activator receptor (uPAR), leading to the elimination of senescent cells and restoration of tissue homeostasis, thus demonstrating the therapeutic potential of this approach against age-associated disorders, including CV diseases (Amor et al., 2020; Feucht and Abou-El-Enein, 2020). Nevertheless, additional trials and investigations will be needed to translate senolytics application to the clinic, especially with respect to the identification of the best treatment strategy with minimum adverse effects (Kirkland and Tchkonia, 2020).
Conclusion
Inflammation is a complex biological process with fundamental roles in host defense, tissue healing, and regeneration. However, the chronic low-grade activation of inflammatory pathways that characterize older individuals, the so-called inflamm-aging, has been associated with reduced lifespan and age-related CV disorders. Since CV disorders are the leading cause of death in industrialized countries, improving the treatment of these diseases implies prolonging the average lifespan. Furthermore, acute CV events, particularly stroke, are associated with long-term disability, inevitably resulting in a worsening of quality of life. Long-term disability, dependence on daily living, and reduced quality of life are the most relevant backlashes of aging; thus, addressing those aspects is pivotal in promoting healthy aging. Finally, inflamm-aging is also involved in other age-related disorders, like sarcopenia (Vatic et al., 2020), cancer (Park et al., 2021), and neurocognitive impairment(Komleva et al., 2021), all having a heavy impact on lifespan and quality of life of elderly people. Therefore, targeting inflamm-aging may prolong lifespan and promote successful aging by acting on multiple levels.
Inflamm-aging develops due to senescent cell accumulation, altered function of immune cells, and increased inflammasome activity due to incremented levels of DAMPs and PAMPs. To date, wide experimental evidence validated the importance of inflamm-aging in the pathophysiology of CV diseases and the potential of targeting inflammation as pharmacological therapy. Nevertheless, none of the tested anti-inflammatory agents has yet been implemented in everyday clinical cardiology; thus, more work remains to be done to optimize these promising interventions. Ultimately, future studies are encouraged to discover further potential therapeutic targets involved in the complex mechanism of inflamm-aging. Along with other treatment strategies against different age-related alterations in molecular pathways, inflamm-aging targeted approaches will intently endure the burden of CV disease in the growing aging population.
Author Contributions
YP wrote the initial draft of the manuscript. LS and CK created the picture. SM, LL and GC critically contributed to revision and manuscript finalization. All authors contributed to manuscript revision, read, and approved the submitted version.
Funding
GC received support for his work from the Swiss National Science Foundation (310030_197510), the Swiss Heart Foundation, the Alfred and Annemarie von Sick Grants for Translational and Clinical Research Cardiology and Oncology, and the Foundation for Cardiovascular Research‐ Zurich Heart House. GC is also a recipient of a Sheikh Khalifa’s Foundation Ass. Professorship at the Faculty of Medicine. University of Zurich.
Conflict of Interest
LL and GGC are coinventors on the International Patent (WO/2020/226993) filed in April 2020 and relating to the use of antibodies which specifically bind IL-1α to reduce various sequelae of ischemia-reperfusion injury to the central nervous system. GGC is a consultant to Sovida solutions limited.
The remaining authors declare that the research was conducted in the absence of any commercial or financial relationships that could be construed as potential conflict of interest.
Publisher’s Note
All claims expressed in this article are solely those of the authors and do not necessarily represent those of their affiliated organizations, or those of the publisher, the editors and the reviewers. Any product that may be evaluated in this article, or claim that may be made by its manufacturer, is not guaranteed or endorsed by the publisher.
References
Abbate, A., Trankle, C. R., Buckley, L. F., Lipinski, M. J., Appleton, D., Kadariya, D., et al. (2020). Interleukin‐1 Blockade Inhibits the Acute Inflammatory Response in Patients with ST‐Segment-Elevation Myocardial Infarction. Jaha 9, e014941. doi:10.1161/JAHA.119.014941
Abbate, A., Van Tassell, B. W., Biondi-Zoccai, G., Kontos, M. C., Grizzard, J. D., Spillman, D. W., et al. (2013). Effects of Interleukin-1 Blockade with Anakinra on Adverse Cardiac Remodeling and Heart Failure after Acute Myocardial Infarction [from the virginia Commonwealth university-anakinra Remodeling Trial (2) (Vcu-art2) Pilot Study]. Am. J. Cardiol. 111, 1394–1400. doi:10.1016/j.amjcard.2013.01.287
Afonina, I. S., Zhong, Z., Karin, M., and Beyaert, R. (2017). Limiting Inflammation-The Negative Regulation of NF-Κb and the NLRP3 Inflammasome. Nat. Immunol. 18, 861–869. doi:10.1038/ni.3772
Agrawal, A., Agrawal, S., and Gupta, S. (2017). Role of Dendritic Cells in Inflammation and Loss of Tolerance in the Elderly. Front. Immunol. 8, 896. doi:10.3389/fimmu.2017.00896
Ajoolabady, A., Aslkhodapasandhokmabad, H., Aghanejad, A., Zhang, Y., and Ren, J. (2020). Mitophagy Receptors and Mediators: Therapeutic Targets in the Management of Cardiovascular Ageing. Ageing Res. Rev. 62, 101129. doi:10.1016/J.ARR.2020.101129
Amor, C., Feucht, J., Leibold, J., Ho, Y.-J., Zhu, C., Alonso-Curbelo, D., et al. (2020). Senolytic CAR T Cells Reverse Senescence-Associated Pathologies. Nature 583, 127–132. doi:10.1038/s41586-020-2403-9
Anderson, R., Lagnado, A., Maggiorani, D., Walaszczyk, A., Dookun, E., Chapman, J., et al. (2019). Length‐independent Telomere Damage Drives post‐mitotic Cardiomyocyte Senescence. EMBO J. 38, e100492. doi:10.15252/embj.2018100492
Appel, M., Frantz, S., and Campos Ramos, G. (2021). Myocardial Inflammation Comes of Age. Curr. Opin. Physiol. 19, 47–54. doi:10.1016/j.cophys.2020.09.006
Audia, J. P., Yang, X.-M., Crockett, E. S., Housley, N., Haq, E. U., O’Donnell, K., et al. (2018). Caspase-1 Inhibition by VX-765 Administered at Reperfusion in P2Y12 Receptor Antagonist-Treated Rats Provides Long-Term Reduction in Myocardial Infarct Size and Preservation of Ventricular Function. Basic Res. Cardiol. 113, 32. doi:10.1007/s00395-018-0692-z
Aw, D., Silva, A. B., and Palmer, D. B. (2007). Immunosenescence: Emerging Challenges for an Ageing Population. Immunology 120, 435–446. doi:10.1111/j.1365-2567.2007.02555.x
Barbosa, M. C., Grosso, R. A., and Fader, C. M. (2019). Hallmarks of Aging: An Autophagic Perspective. Front. Endocrinol. 9. doi:10.3389/fendo.2018.00790
Beli, E., Clinthorne, J. F., Duriancik, D. M., Hwang, I., Kim, S., and Gardner, E. M. (2011). Natural Killer Cell Function Is Altered during the Primary Response of Aged Mice to Influenza Infection. Mech. Ageing Develop. 132, 503–510. doi:10.1016/j.mad.2011.08.005
Biasizzo, M., and Kopitar-Jerala, N. (2020). Interplay between NLRP3 Inflammasome and Autophagy. Front. Immunol. 11. doi:10.3389/fimmu.2020.591803
Birks, E. J., Felkin, L. E., Banner, N. R., Khaghani, A., Barton, P. J. R., and Yacoub, M. H. (2004). Increased Toll-like Receptor 4 in the Myocardium of Patients Requiring Left Ventricular Assist Devices. J. Hear. Lung Transpl. 23 (2), 228–235. doi:10.1016/S1053-2498(03)00106-2
Boaru, S. G., Borkham-Kamphorst, E., Van De Leur, E., Lehnen, E., Liedtke, C., and Weiskirchen, R. (2015). NLRP3 Inflammasome Expression Is Driven by NF-Κb in Cultured Hepatocytes. Biochem. Biophysical Res. Commun. 458, 700–706. doi:10.1016/j.bbrc.2015.02.029
Bonetti, N. R., Diaz-Cañestro, C., Liberale, L., Crucet, M., Akhmedov, A., Merlini, M., et al. (2019). Tumour Necrosis Factor-α Inhibition Improves Stroke Outcome in a Mouse Model of Rheumatoid Arthritis. Sci. Rep. 9, 1–11. doi:10.1038/s41598-019-38670-z
Boucher, N., Dufeu-Duchesne, T., Vicaut, E., Farge, D., Effros, R. B., and Schächter, F. (1998). CD28 Expression in T Cell Aging and Human Longevity. Exp. Gerontol. 33, 267–282. doi:10.1016/S0531-5565(97)00132-0
Briceño, O., Lissina, A., Wanke, K., Afonso, G., Braun, A., Ragon, K., et al. (2016). Reduced Naïve CD 8 + T ‐cell Priming Efficacy in Elderly Adults. Aging Cell 15, 14–21. doi:10.1111/acel.12384
Buckley, L. F., and Abbate, A. (2018). Interleukin-1 Blockade in Cardiovascular Diseases: A Clinical Update. Eur. Heart J. 39, 2063–2069. doi:10.1093/eurheartj/ehy128
Bujak, M., Kweon, H. J., Chatila, K., Li, N., Taffet, G., and Frangogiannis, N. G. (2008). Aging-Related Defects Are Associated with Adverse Cardiac Remodeling in a Mouse Model of Reperfused Myocardial Infarction. J. Am. Coll. Cardiol. 51, 1384–1392. doi:10.1016/j.jacc.2008.01.011
Butcher, S. K., Chahal, H., Nayak, L., Sinclair, A., Henriquez, N. V., Sapey, E., et al. (2001). Senescence in Innate Immune Responses: Reduced Neutrophil Phagocytic Capacity and CD16 Expression in Elderly Humans. J. Leukoc. Biol. 70, 881–886. doi:10.1189/jlb.70.6.881
Camici, G. G., and Liberale, L. (2017). Aging: The Next Cardiovascular Disease? Eur. Heart J. 38, 1621–1623. doi:10.1093/eurheartj/ehx239
Camous, X., Pera, A., Solana, R., and Larbi, A. (2012). NK Cells in Healthy Aging and Age-Associated Diseases. J. Biomed. Biotechnol. 2012, 1–8. doi:10.1155/2012/195956
Chaar, M., Kamta, J., and Ait-Oudhia, S. (2018). Mechanisms, Monitoring, and Management of Tyrosine Kinase Inhibitors-Associated Cardiovascular Toxicities. Ott Vol. 11, 6227–6237. doi:10.2147/OTT.S170138
Chen, G., Kroemer, G., and Kepp, O. (2020). Mitophagy: An Emerging Role in Aging and Age-Associated Diseases. Front. Cel Dev. Biol. 8, 200. doi:10.3389/FCELL.2020.00200/BIBTEX
Chen, L., Deng, H., Cui, H., Fang, J., Zuo, Z., Deng, J., et al. (2018). Inflammatory Responses and Inflammation-Associated Diseases in Organs. Oncotarget 9, 7204–7218. doi:10.18632/oncotarget.23208
Childs, B. G., Durik, M., Baker, D. J., and Van Deursen, J. M. (2015). Cellular Senescence in Aging and Age-Related Disease: From Mechanisms to Therapy. Nat. Med. 21, 1424–1435. doi:10.1038/nm.4000
Cong, B., Zhang, Q., and Cao, X. (2021). The Function and Regulation of TET2 in Innate Immunity and Inflammation. Protein Cell 12, 165–173. doi:10.1007/s13238-020-00796-6
Davila, D. R., Edwards, C. K., Arkins, S., Simon, J., and Kelley, K. W. (1990). Interferon‐γ‐induced Priming for Secretion of Superoxide Anion and Tumor Necrosis Factor‐α Declines in Macrophages from Aged Rats. FASEB j. 4, 2906–2911. doi:10.1096/fasebj.4.11.2165948
de Magalhães, J. P., Chainiaux, F., Remacle, J., and Toussaint, O. (2002). Stress-induced Premature Senescence in BJ and hTERT-BJ1 Human Foreskin Fibroblasts. FEBS Lett. 523, 157–162. doi:10.1016/S0014-5793(02)02973-3
de Magalhães, J. P., and Passos, J. F. (2018). Stress, Cell Senescence and Organismal Ageing. Mech. Ageing Develop. 170, 2–9. doi:10.1016/j.mad.2017.07.001
Dick, S. A., and Epelman, S. (2016). Chronic Heart Failure and Inflammation. Circ. Res. 119, 159–176. doi:10.1161/CIRCRESAHA.116.308030
Docherty, M. H., Baird, D. P., Hughes, J., and Ferenbach, D. A. (2020). Cellular Senescence and Senotherapies in the Kidney: Current Evidence and Future Directions. Front. Pharmacol. 11, 755. doi:10.3389/fphar.2020.00755
Dookun, E., Passos, J. F., Arthur, H. M., and Richardson, G. D. (2020). Therapeutic Potential of Senolytics in Cardiovascular Disease. Cardiovasc. Drugs Ther. 36, 187–196. doi:10.1007/s10557-020-07075-w
Du, W., Wong, C., Song, Y., Shen, H., Mori, D., Rotllan, N., et al. (2016). Age‐associated Vascular Inflammation Promotes Monocytosis during Atherogenesis. Aging Cell 15, 766–777. doi:10.1111/acel.12488
Elliott, E. I., and Sutterwala, F. S. (2015). Initiation and Perpetuation of NLRP3 Inflammasome Activation and Assembly. Immunol. Rev. 265, 35–52. doi:10.1111/imr.12286
Fan, W., Li, C., Qin, X., Wang, S., Da, H., Cheng, K., et al. (2013). Adipose Stromal Cell and Sarpogrelate Orchestrate the Recovery of Inflammation-Induced Angiogenesis in Aged Hindlimb Ischemic Mice. Aging Cell 12, 32–41. doi:10.1111/ACEL.12014
Ferrucci, L., and Fabbri, E. (2018). Inflammageing: Chronic Inflammation in Ageing, Cardiovascular Disease, and Frailty. Nat. Rev. Cardiol. 15, 505–522. doi:10.1038/s41569-018-0064-2
Feucht, J., and Abou-El-Enein, M. (2020). Senolytic CAR T Cells in Solid Tumors and Age-Related Pathologies. Mol. Ther. 28, 2108–2110. doi:10.1016/j.ymthe.2020.08.011
Franceschi, C., Bonafè, M., Valensin, S., Olivieri, F., De Luca, M., Ottaviani, E., et al. (2000). “Inflamm-aging: An Evolutionary Perspective on Immunosenescence,” in Annals of the New York Academy of Sciences (New York, United States: New York Academy of Sciences), 908, 244–254. doi:10.1111/j.1749-6632.2000.tb06651.x
Frantz, S., Hofmann, U., Fraccarollo, D., Schäfer, A., Kranepuhl, S., Hagedorn, I., et al. (2013). Monocytes/macrophages Prevent Healing Defects and Left Ventricular Thrombus Formation after Myocardial Infarction. FASEB j. 27, 871–881. doi:10.1096/fj.12-214049
Fuster, J. J., MacLauchlan, S., Zuriaga, M. A., Polackal, M. N., Ostriker, A. C., Chakraborty, R., et al. (2017). Clonal Hematopoiesis Associated with TET2 Deficiency Accelerates Atherosclerosis Development in Mice. Science 355, 842–847. doi:10.1126/science.aag1381
Galkina, E., and Ley, K. (2007). Vascular Adhesion Molecules in Atherosclerosis. Atvb 27, 2292–2301. doi:10.1161/ATVBAHA.107.149179
Gao, R., Shi, H., Chang, S., Gao, Y., Li, X., Lv, C., et al. (2019). The Selective NLRP3-Inflammasome Inhibitor MCC950 Reduces Myocardial Fibrosis and Improves Cardiac Remodeling in a Mouse Model of Myocardial Infarction. Int. Immunopharmacology 74, 105575. doi:10.1016/j.intimp.2019.04.022
Gounder, S. S., Abdullah, B. J. J., Radzuanb, N. E. I. B. M., Zain, F. D. B. M., Sait, N. B. M., Chua, C., et al. (2018). Effect of Aging on NK Cell Population and Their Proliferation at Ex Vivo Culture Condition. Anal. Cell Pathol. 2018, 1–6. doi:10.1155/2018/7871814
Grazioli, S., and Pugin, J. (2018). Mitochondrial Damage-Associated Molecular Patterns: From Inflammatory Signaling to Human Diseases. Front. Immunol. 9, 1. doi:10.3389/fimmu.2018.00832
Hato, T., and Dagher, P. C. (2015). How the Innate Immune System Senses Trouble and Causes Trouble. Cjasn 10, 1459–1469. doi:10.2215/CJN.04680514
Hayflick, L., and Moorhead, P. S. (1961). The Serial Cultivation of Human Diploid Cell Strains. Exp. Cel Res. 25, 585–621. doi:10.1016/0014-4827(61)90192-6
Hellawell, H. N., Mostafa, A. M. H. A. M., Kyriacou, H., Sumal, A. S., and Boyle, J. R. (2021). Abdominal Aortic Aneurysms Part One: Epidemiology, Presentation and Preoperative Considerations. J. Perioper. Pract. 31, 175045892095401–280. doi:10.1177/1750458920954014
Hoogen, P., Jager, S. C. A., Huibers, M. M. H., Schoneveld, A. H., Puspitasari, Y. M., Valstar, G. B., et al. (2019). Increased Circulating IgG Levels, Myocardial Immune Cells and IgG Deposits Support a Role for an Immune Response in Pre‐ and End‐stage Heart Failure. J. Cel Mol Med 23, 7505–7516. doi:10.1111/jcmm.14619
Ikonomidis, I., Lekakis, J. P., Nikolaou, M., Paraskevaidis, I., Andreadou, I., Kaplanoglou, T., et al. (2008). Inhibition of Interleukin-1 by Anakinra Improves Vascular and Left Ventricular Function in Patients with Rheumatoid Arthritis. Circulation 117, 2662–2669. doi:10.1161/CIRCULATIONAHA.107.731877
Ikonomidis, I., Tzortzis, S., Andreadou, I., Paraskevaidis, I., Katseli, C., Katsimbri, P., et al. (2014). Increased Benefit of Interleukin-1 Inhibition on Vascular Function, Myocardial Deformation, and Twisting in Patients with Coronary Artery Disease and Coexisting Rheumatoid Arthritis. Circ. Cardiovasc. Imaging 7, 619–628. doi:10.1161/CIRCIMAGING.113.001193
Jaiswal, S., and Ebert, B. L. (2019). Clonal Hematopoiesis in Human Aging and Disease. Science 366, eaan4673. doi:10.1126/science.aan4673
Jaiswal, S., Fontanillas, P., Flannick, J., Manning, A., Grauman, P. V., Mar, B. G., et al. (2014). Age-Related Clonal Hematopoiesis Associated with Adverse Outcomes. N. Engl. J. Med. 371, 2488–2498. doi:10.1056/nejmoa1408617
Jaiswal, S., Natarajan, P., Silver, A. J., Gibson, C. J., Bick, A. G., Shvartz, E., et al. (2017). Clonal Hematopoiesis and Risk of Atherosclerotic Cardiovascular Disease. N. Engl. J. Med. 377, 111–121. doi:10.1056/nejmoa1701719
Karin, M., and Clevers, H. (2016). Reparative Inflammation Takes Charge of Tissue Regeneration. Nature 529, 307–315. doi:10.1038/nature17039
Kawaguchi, M., Takahashi, M., Hata, T., Kashima, Y., Usui, F., Morimoto, H., et al. (2011). Inflammasome Activation of Cardiac Fibroblasts Is Essential for Myocardial Ischemia/reperfusion Injury. Circulation 123, 594–604. doi:10.1161/CIRCULATIONAHA.110.982777
Kelley, N., Jeltema, D., Duan, Y., and He, Y. (2019). The NLRP3 Inflammasome: An Overview of Mechanisms of Activation and Regulation. Ijms 20, 3328. doi:10.3390/ijms20133328
Khetarpal, S. A., Qamar, A., Bick, A. G., Fuster, J. J., Kathiresan, S., Jaiswal, S., et al. (2019). Clonal Hematopoiesis of Indeterminate Potential Reshapes Age-Related CVD. J. Am. Coll. Cardiol. 74, 578–586. doi:10.1016/j.jacc.2019.05.045
Kirkland, J. L., and Tchkonia, T. (2020). Senolytic Drugs: from Discovery to Translation. J. Intern. Med. 288, 518–536. doi:10.1111/joim.13141
Komleva, Y., Chernykh, A., Lopatina, O., Gorina, Y., Lokteva, I., Salmina, A., et al. (2021). Inflamm-Aging and Brain Insulin Resistance: New Insights and Role of Life-Style Strategies on Cognitive and Social Determinants in Aging and Neurodegeneration. Front. Neurosci. 14. doi:10.3389/FNINS.2020.618395
Kuo, H.-K., Sorond, F. A., Chen, J.-H., Hashmi, A., Milberg, W. P., and Lipsitz, L. A. (2005). The Role of Homocysteine in Multisystem Age-Related Problems: A Systematic Review. J. Gerontol. A. Biol. Sci. Med. Sci. 60, 1190–1201. doi:10.1093/GERONA/60.9.1190
Leoni, C., Montagner, S., Rinaldi, A., Bertoni, F., Polletti, S., Balestrieri, C., et al. (2017). Dnmt3a Restrains Mast Cell Inflammatory Responses. Proc. Natl. Acad. Sci. U.S.A. 114, E1490–E1499. doi:10.1073/pnas.1616420114
Leung, Y. Y., Yao Hui, L. L., and Kraus, V. B. (2015). Colchicine-Update on Mechanisms of Action and Therapeutic Uses. Semin. Arthritis Rheum. 45, 341–350. doi:10.1016/j.semarthrit.2015.06.013
Li, H., Hastings, M. H., Rhee, J., Trager, L. E., Roh, J. D., and Rosenzweig, A. (2020). Targeting Age-Related Pathways in Heart Failure. Circ. Res. 126, 533–551. doi:10.1161/CIRCRESAHA.119.315889
Libby, P., and Ebert, B. L. (2018). CHIP (Clonal Hematopoiesis of Indeterminate Potential). Circulation 138, 666–668. doi:10.1161/CIRCULATIONAHA.118.034392
Libby, P. (2013). Mechanisms of Acute Coronary Syndromes and Their Implications for Therapy. N. Engl. J. Med. 368, 2004–2013. doi:10.1056/nejmra1216063
Liberale, L., Badimon, L., Montecucco, F., Lüscher, T. F., Libby, P., and Camici, G. G. (2022). Inflammation, Aging, and Cardiovascular Disease. J. Am. Coll. Cardiol. 79, 837–847. doi:10.1016/J.JACC.2021.12.017
Liberale, L., Diaz-Cañestro, C., Bonetti, N. R., Paneni, F., Akhmedov, A., Beer, J. H., et al. (2018). Post-ischaemic Administration of the Murine Canakinumab-Surrogate Antibody Improves Outcome in Experimental Stroke. Eur. Heart J. 39, 3511–3517. doi:10.1093/eurheartj/ehy286
Liberale, L., Ministrini, S., Carbone, F., Camici, G. G., and Montecucco, F. (2021). Cytokines as Therapeutic Targets for Cardio- and Cerebrovascular Diseases. Basic Res. Cardiol. 116, 23. doi:10.1007/S00395-021-00863-X
Liberale, L., Montecucco, F., Tardif, J.-C., Libby, P., and Camici, G. G. (2020). Inflamm-ageing: the Role of Inflammation in Age-dependent Cardiovascular Disease. Eur. Heart J. 41, 2974–2982. doi:10.1093/eurheartj/ehz961
Liu, D., Richardson, G., Benli, F. M., Park, C., de Souza, J. V., Bronowska, A. K., et al. (2020). Inflammageing in the Cardiovascular System: Mechanisms, Emerging Targets, and Novel Therapeutic Strategies. Clin. Sci. 134, 2243–2262. doi:10.1042/CS20191213
Liu, Z., Luo, H., Zhang, L., Huang, Y., Liu, B., Ma, K., et al. (2012). Hyperhomocysteinemia Exaggerates Adventitial Inflammation and Angiotensin II−Induced Abdominal Aortic Aneurysm in Mice. Circ. Res. 111, 1261–1273. doi:10.1161/CIRCRESAHA.112.270520
Lloberas, J., Tur, J., Vico, T., and Celada, A. (2019). “Molecular and Cellular Aspects of Macrophage Aging,” in Handbook of Immunosenescence. Editors T. Fulop, C. Franceschi, K. Hirokawa, and G. Pawelec (Cham: Springer International Publishing). doi:10.1007/978-3-319-99375-1_46
Ma, Y., Mouton, A. J., and Lindsey, M. L. (2018). Cardiac Macrophage Biology in the Steady-State Heart, the Aging Heart, and Following Myocardial Infarction. Translational Res. 191, 15–28. doi:10.1016/j.trsl.2017.10.001
Martínez, G. J., Celermajer, D. S., and Patel, S. (2018). The NLRP3 Inflammasome and the Emerging Role of Colchicine to Inhibit Atherosclerosis-Associated Inflammation. Atherosclerosis 269, 262–271. doi:10.1016/j.atherosclerosis.2017.12.027
Martinon, F., Mayor, A., and Tschopp, J. (2009). The Inflammasomes: Guardians of the Body. Annu. Rev. Immunol. 27, 229–265. doi:10.1146/annurev.immunol.021908.132715
Mauro, A. G., Bonaventura, A., Mezzaroma, E., Quader, M., and Toldo, S. (2019). NLRP3 Inflammasome in Acute Myocardial Infarction. J. Cardiovasc. Pharmacol. 74, 175–187. doi:10.1097/FJC.0000000000000717
Ministrini, S., Carbone, F., and Montecucco, F. (2021a). Updating Concepts on Atherosclerotic Inflammation: From Pathophysiology to Treatment. Eur. J. Clin. Invest. 51, e13467. doi:10.1111/ECI.13467
Ministrini, S., Puspitasari, Y. M., Beer, G., Liberale, L., Montecucco, F., and Camici, G. G. (2021b). Sirtuin 1 in Endothelial Dysfunction and Cardiovascular Aging. Front. Physiol. 12, 1589. doi:10.3389/FPHYS.2021.733696/BIBTEX
Mooney, L., Goodyear, C. S., Chandra, T., Kirschner, K., Copland, M., Petrie, M. C., et al. (2021). Clonal Haematopoiesis of Indeterminate Potential: Intersections between Inflammation, Vascular Disease and Heart Failure. Clin. Sci. 135, 991–1007. doi:10.1042/CS20200306
Moro-García, M. A., Echeverría, A., Galán-Artímez, M. C., Suárez-García, F. M., Solano-Jaurrieta, J. J., Avanzas-Fernández, P., et al. (2014). Immunosenescence and Inflammation Characterize Chronic Heart Failure Patients with More Advanced Disease. Int. J. Cardiol. 174, 590–599. doi:10.1016/j.ijcard.2014.04.128
Morton, A. C., Rothman, A. M. K., Greenwood, J. P., Gunn, J., Chase, A., Clarke, B., et al. (2015). The Effect of Interleukin-1 Receptor Antagonist Therapy on Markers of Inflammation in Non-ST Elevation Acute Coronary Syndromes: the MRC-ILA Heart Study. Eur. Heart J. 36, 377–384. doi:10.1093/eurheartj/ehu272
Nahrendorf, M., and Swirski, F. K. (2013). Monocyte and Macrophage Heterogeneity in the Heart. Circ. Res. 112, 1624–1633. doi:10.1161/CIRCRESAHA.113.300890
Nicholls, S. J., Kastelein, J. J. P., Schwartz, G. G., Bash, D., Rosenson, R. S., Cavender, M. A., et al. (2014). Varespladib and Cardiovascular Events in Patients with an Acute Coronary Syndrome. Jama 311, 252–262. doi:10.1001/jama.2013.282836
Nidorf, S. M., Eikelboom, J. W., Budgeon, C. A., and Thompson, P. L. (2013). Low-dose Colchicine for Secondary Prevention of Cardiovascular Disease. J. Am. Coll. Cardiol. 61, 404–410. doi:10.1016/j.jacc.2012.10.027
Nidorf, S. M., Fiolet, A. T. L., Mosterd, A., Eikelboom, J. W., Schut, A., Opstal, T. S. J., et al. (2020). Colchicine in Patients with Chronic Coronary Disease. N. Engl. J. Med. 383, 1838–1847. doi:10.1056/nejmoa2021372
O'Rourke, S. A., Dunne, A., and Monaghan, M. G. (2019). The Role of Macrophages in the Infarcted Myocardium: Orchestrators of ECM Remodeling. Front. Cardiovasc. Med. 6, 101. doi:10.3389/fcvm.2019.00101
O’Donoghue, M. L., Braunwald, E., White, H. D., Steen, D. P., Lukas, M. A., Tarka, E., et al. (2014). Effect of Darapladib on Major Coronary Events after an Acute Coronary Syndrome. Jama 312, 1006–1015. doi:10.1001/jama.2014.11061
O’Donoghue, M. L., Glaser, R., Cavender, M. A., Aylward, P. E., Bonaca, M. P., Budaj, A., et al. (2016). Effect of Losmapimod on Cardiovascular Outcomes in Patients Hospitalized with Acute Myocardial Infarction. Jama 315, 1591–1599. doi:10.1001/jama.2016.3609
Olivieri, F., Prattichizzo, F., Grillari, J., and Balistreri, C. R. (2018). Cellular Senescence and Inflammaging in Age-Related Diseases. Mediators Inflamm. 2018, 1–6. doi:10.1155/2018/9076485
Owens, W. A., Walaszczyk, A., Spyridopoulos, I., Dookun, E., and Richardson, G. D. (2021). Senescence and Senolytics in Cardiovascular Disease: Promise and Potential Pitfalls. Mech. Ageing Develop. 198, 111540. doi:10.1016/J.MAD.2021.111540
Pangrazzi, L., and Weinberger, B. (2020). T Cells, Aging and Senescence. Exp. Gerontol. 134, 110887. doi:10.1016/j.exger.2020.110887
Park, S. S., Choi, Y. W., Kim, J.-H., Kim, H. S., and Park, T. J. (2021). Senescent Tumor Cells: an Overlooked Adversary in the Battle against Cancer. Exp. Mol. Med. 53, 1834–1841. doi:10.1038/S12276-021-00717-5
Pascual-Figal, D. A., Bayes-Genis, A., Díez-Díez, M., Hernández-Vicente, Á., Vázquez-Andrés, D., de la Barrera, J., et al. (2021). Clonal Hematopoiesis and Risk of Progression of Heart Failure with Reduced Left Ventricular Ejection Fraction. J. Am. Coll. Cardiol. 77, 1747–1759. doi:10.1016/j.jacc.2021.02.028
Paudel, S., Sharma, P., and Puri, N. (2019). “Immunosenescence, Inflammaging, and Their Implications for Cancer and Anemia,” in Models, Molecules and Mechanisms in Biogerontology (Singapore: Springer), 297–319. doi:10.1007/978-981-13-3585-3_14
Prata, L. G. P. L., Ovsyannikova, I. G., Tchkonia, T., and Kirkland, J. L. (2018). Senescent Cell Clearance by the Immune System: Emerging Therapeutic Opportunities. Semin. Immunol. 40, 101275. doi:10.1016/j.smim.2019.04.003
Raghuram, G. V., and Mishra, P. K. (2014). Stress Induced Premature Senescence: A New Culprit in Ovarian Tumorigenesis? Indian J. Med. Res. 140 Suppl, S120–9. Available at:/pmc/articles/PMC4345742/(Accessed May 5, 2021).
Rea, I. M., Gibson, D. S., McGilligan, V., McNerlan, S. E., Alexander, H. D., and Ross, O. A. (2018). Age and Age-Related Diseases: Role of Inflammation Triggers and Cytokines. Front. Immunol. 9, 1. doi:10.3389/fimmu.2018.00586
Ridker, P. M., Everett, B. M., Pradhan, A., MacFadyen, J. G., Solomon, D. H., Zaharris, E., et al. (2019). Low-Dose Methotrexate for the Prevention of Atherosclerotic Events. N. Engl. J. Med. 380, 752–762. doi:10.1056/NEJMoa1809798
Ridker, P. M., Everett, B. M., Thuren, T., MacFadyen, J. G., Chang, W. H., Ballantyne, C., et al. (2017). Antiinflammatory Therapy with Canakinumab for Atherosclerotic Disease. N. Engl. J. Med. 377, 1119–1131. doi:10.1056/nejmoa1707914
Rieckmann, M., Delgobo, M., Gaal, C., Büchner, L., Steinau, P., Reshef, D., et al. (2019). Myocardial Infarction Triggers Cardioprotective Antigen-specific T Helper Cell Responses. J. Clin. Invest. 129, 4922–4936. doi:10.1172/JCI123859
Roos, C. M., Zhang, B., Palmer, A. K., Ogrodnik, M. B., Pirtskhalava, T., Thalji, N. M., et al. (2016). Chronic Senolytic Treatment Alleviates Established Vasomotor Dysfunction in Aged or Atherosclerotic Mice. Aging Cell 15, 973–977. doi:10.1111/acel.12458
Ross, R. (1999). Atherosclerosis - an Inflammatory Disease. N. Engl. J. Med. 340, 115–126. doi:10.1056/NEJM199901143400207
Ruparelia, N., Chai, J. T., Fisher, E. A., and Choudhury, R. P. (2017). Inflammatory Processes in Cardiovascular Disease: A Route to Targeted Therapies. Nat. Rev. Cardiol. 14, 133–144. doi:10.1038/nrcardio.2016.185
Sanada, F., Taniyama, Y., Muratsu, J., Otsu, R., Shimizu, H., Rakugi, H., et al. (2018). Source of Chronic Inflammation in Aging. Front. Cardiovasc. Med. 5, 12. doi:10.3389/fcvm.2018.00012
Sandanger, Ø., Ranheim, T., Vinge, L. E., Bliksøen, M., Alfsnes, K., Finsen, A. V., et al. (2013). The NLRP3 Inflammasome Is Up-Regulated in Cardiac Fibroblasts and Mediates Myocardial Ischaemia-Reperfusion Injury. Cardiovasc. Res. 99, 164–174. doi:10.1093/CVR/CVT091
Sawai, C. M., Babovic, S., Upadhaya, S., Knapp, D. J. H. F., Lavin, Y., Lau, C. M., et al. (2016). Hematopoietic Stem Cells Are the Major Source of Multilineage Hematopoiesis in Adult Animals. Immunity 45, 597–609. doi:10.1016/j.immuni.2016.08.007
Schoenwaelder, S. M., Jarman, K. E., Gardiner, E. E., Hua, M., Qiao, J., White, M. J., et al. (2011). Bcl-xL-inhibitory BH3 Mimetics Can Induce a Transient Thrombocytopathy that Undermines the Hemostatic Function of Platelets. Blood 118, 1663–1674. doi:10.1182/blood-2011-04-347849
Shaw, A. C., Goldstein, D. R., and Montgomery, R. R. (2013). Age-dependent Dysregulation of Innate Immunity. Nat. Rev. Immunol. 13, 875–887. doi:10.1038/nri3547
Shimada, K., Crother, T. R., Karlin, J., Dagvadorj, J., Chiba, N., Chen, S., et al. (2012). Oxidized Mitochondrial DNA Activates the NLRP3 Inflammasome during Apoptosis. Immunity 36, 401–414. doi:10.1016/j.immuni.2012.01.009
Soysal, P., Arik, F., Smith, L., Jackson, S. E., and Isik, A. T. (2020). “Inflammation, Frailty and Cardiovascular Disease,” in Frailty and Cardiovascular Diseases. Advances in Experimental Medicine and Biology. Editors N. Veronese (Cham: Springer). doi:10.1007/978-3-030-33330-0_7
Stähli, B. E., Tardif, J.-C., Carrier, M., Gallo, R., Emery, R. W., Robb, S., et al. (2016). Effects of P-Selectin Antagonist Inclacumab in Patients Undergoing Coronary Artery Bypass Graft Surgery. J. Am. Coll. Cardiol. 67, 344–346. doi:10.1016/j.jacc.2015.10.071
Stojanović, S. D., Fiedler, J., Bauersachs, J., Thum, T., and Sedding, D. G. (2020). Senescence-induced Inflammation: An Important Player and Key Therapeutic Target in Atherosclerosis. Eur. Heart J. 41, 2983–2996. doi:10.1093/eurheartj/ehz919
Sun, W., Pang, Y., Liu, Z., Sun, L., Liu, B., Xu, M., et al. (2015). Macrophage Inflammasome Mediates Hyperhomocysteinemia-Aggravated Abdominal Aortic Aneurysm. J. Mol. Cell Cardiol. 81, 96–106. doi:10.1016/J.YJMCC.2015.02.005
Swirski, F. K., and Nahrendorf, M. (2013). Macrophage-stem Cell Crosstalk after Myocardial Infarction. J. Am. Coll. Cardiol. 62, 1902–1904. doi:10.1016/j.jacc.2013.07.058
Takahashi, R., Totsuka, S., Ishigami, A., Kobayashi, Y., and Nagata, K. (2016). Attenuated Phagocytosis of Secondary Necrotic Neutrophils by Macrophages in Aged and SMP30 Knockout Mice. Geriatr. Gerontol. Int. 16, 135–142. doi:10.1111/ggi.12436
Tansey, M. G., Wallings, R. L., Houser, M. C., Herrick, M. K., Keating, C. E., and Joers, V. (2022). Inflammation and Immune Dysfunction in Parkinson Disease. Nat. Rev. Immunol., 1–17. doi:10.1038/s41577-022-00684-6
Tardif, J.-C., Kouz, S., Waters, D. D., Bertrand, O. F., Diaz, R., Maggioni, A. P., et al. (2019). Efficacy and Safety of Low-Dose Colchicine after Myocardial Infarction. N. Engl. J. Med. 381, 2497–2505. doi:10.1056/NEJMoa1912388
Tardif, J.-C., McMurray, J. J., Klug, E., Small, R., Schumi, J., Choi, J., et al. (2008). Effects of Succinobucol (AGI-1067) after an Acute Coronary Syndrome: a Randomised, Double-Blind, Placebo-Controlled Trial. The Lancet 371, 1761–1768. doi:10.1016/S0140-6736(08)60763-1
Tardif, J.-C., Tanguay, J.-F., Wright, S. R., Duchatelle, V., Petroni, T., Grégoire, J. C., et al. (2013). Effects of the P-Selectin Antagonist Inclacumab on Myocardial Damage after Percutaneous Coronary Intervention for Non-ST-segment Elevation Myocardial Infarction. J. Am. Coll. Cardiol. 61, 2048–2055. doi:10.1016/j.jacc.2013.03.003
Thai, P. N., Seidlmayer, L. K., Miller, C., Ferrero, M., Dorn, G. W., Schaefer, S., et al. (2019). Mitochondrial Quality Control in Aging and Heart Failure: Influence of Ketone Bodies and Mitofusin-Stabilizing Peptides. Front. Physiol. 10, 382. doi:10.3389/FPHYS.2019.00382/BIBTEX
The STABILITY Investigators (2014). Darapladib for Preventing Ischemic Events in Stable Coronary Heart Disease. N. Engl. J. Med. 370, 1702–1711. doi:10.1056/nejmoa1315878
Thomas, R., Wang, W., and Su, D.-M. (2020). Contributions of Age-Related Thymic Involution to Immunosenescence and Inflammaging. Immun. Ageing 17, 1–17. doi:10.1186/s12979-020-0173-8
Tong, D. C., Bloom, J. E., Quinn, S., Nasis, A., Hiew, C., Roberts-Thomson, P., et al. (2021). Colchicine in Patients with Acute Coronary Syndrome: Two-Year Follow-Up of the Australian COPS Randomized Clinical Trial. Circulation 144, 1584–1586. doi:10.1161/CIRCULATIONAHA.121.054610
Tong, D. C., Quinn, S., Nasis, A., Hiew, C., Roberts-Thomson, P., Adams, H., et al. (2020). Colchicine in Patients with Acute Coronary Syndrome. Circulation 142, 1890–1900. doi:10.1161/CIRCULATIONAHA.120.050771
Torella, D., Rota, M., Nurzynska, D., Musso, E., Monsen, A., Shiraishi, I., et al. (2004). Cardiac Stem Cell and Myocyte Aging, Heart Failure, and Insulin-like Growth Factor-1 Overexpression. Circ. Res. 94, 514–524. doi:10.1161/01.RES.0000117306.10142.50
Toussaint, O., Dumont, P., Remacle, J., Dierick, J.-F., Pascal, T., Frippiat, C., et al. (2002). Stress-Induced Premature Senescence or Stress-Induced Senescence-like Phenotype: OneIn VivoReality, Two Possible Definitions? The Scientific World JOURNAL 2, 230–247. doi:10.1100/tsw.2002.100
Tyrrell, D. J., and Goldstein, D. R. (2021). Ageing and Atherosclerosis: Vascular Intrinsic and Extrinsic Factors and Potential Role of IL-6. Nat. Rev. Cardiol. 18, 58–68. doi:10.1038/s41569-020-0431-7
Vaiserman, A., and Krasnienkov, D. (2021). Telomere Length as a Marker of Biological Age: State-Of-The-Art, Open Issues, and Future Perspectives. Front. Genet. 11, 630186. doi:10.3389/fgene.2020.630186
Van Der Heijden, T., Kritikou, E., Venema, W., Van Duijn, J., Van Santbrink, P. J., Slütter, B., et al. (2017). NLRP3 Inflammasome Inhibition by MCC950 Reduces Atherosclerotic Lesion Development in Apolipoprotein E-Deficient Mice-Brief Report. Atvb 37, 1457–1461. doi:10.1161/ATVBAHA.117.309575
Van Deursen, J. M. (2014). The Role of Senescent Cells in Ageing. Nature 509, 439–446. doi:10.1038/nature13193
Van Tassell, B. W., Abouzaki, N. A., Oddi Erdle, C., Carbone, S., Trankle, C. R., Melchior, R. D., et al. (2016). Interleukin-1 Blockade in Acute Decompensated Heart Failure. J. Cardiovasc. Pharmacol. 67, 544–551. doi:10.1097/FJC.0000000000000378
Van Tassell, B. W., Seropian, I. M., Toldo, S., Mezzaroma, E., and Abbate, A. (2013). Interleukin-1β Induces a Reversible Cardiomyopathy in the Mouse. Inflamm. Res. 62, 637–640. doi:10.1007/s00011-013-0625-0
Vatic, M., von Haehling, S., and Ebner, N. (2020). Inflammatory Biomarkers of Frailty. Exp. Gerontol. 133, 110858. doi:10.1016/J.EXGER.2020.110858
Ventura, M. T., Casciaro, M., Gangemi, S., and Buquicchio, R. (2017). Immunosenescence in Aging: Between Immune Cells Depletion and Cytokines Up-Regulation. Clin. Mol. Allergy 15, 21. doi:10.1186/s12948-017-0077-0
Walaszczyk, A., Dookun, E., Redgrave, R., Tual‐Chalot, S., Victorelli, S., Spyridopoulos, I., et al. (2019). Pharmacological Clearance of Senescent Cells Improves Survival and Recovery in Aged Mice Following Acute Myocardial Infarction. Aging Cell 18, e12945. doi:10.1111/acel.12945
Wang, Y., Sano, S., Yura, Y., Ke, Z., Sano, M., Oshima, K., et al. (2020a). Tet2-mediated Clonal Hematopoiesis in Nonconditioned Mice Accelerates Age-Associated Cardiac Dysfunction. JCI Insight 5, e135204. doi:10.1172/jci.insight.135204
Wang, Y., Zhu, S., Wei, W., Tu, Y., Chen, C., Song, J., et al. (2020b). Interleukin-6 Knockout Reverses Macrophage Differentiation Imbalance and Alleviates Cardiac Dysfunction in Aging Mice. aging 12, 20184–20197. doi:10.18632/aging.103749
Weiskopf, D., Weinberger, B., and Grubeck-Loebenstein, B. (2009). The Aging of the Immune System. Transpl. Int. 22, 1041–1050. doi:10.1111/j.1432-2277.2009.00927.x
Wong, S. Q., Kumar, A. V., Mills, J., and Lapierre, L. R. (2020). Autophagy in Aging and Longevity. Hum. Genet. 139, 277–290. doi:10.1007/s00439-019-02031-7
Wortmann, M., Peters, A. S., Erhart, P., Körfer, D., Böckler, D., and Dihlmann, S. (2021). Inflammasomes in the Pathophysiology of Aortic Disease. Cells 10, 2433. doi:10.3390/CELLS10092433
Wu, X., Hakimi, M., Wortmann, M., Zhang, J., Böckler, D., and Dihlmann, S. (2015). Gene Expression of Inflammasome Components in Peripheral Blood Mononuclear Cells (PBMC) of Vascular Patients Increases with Age. Immun. Ageing 12, 15–5. doi:10.1186/S12979-015-0043-Y/FIGURES/2
Xu, X., Pang, J., Chen, Y., Bucala, R., Zhang, Y., and Ren, J. (2016). Macrophage Migration Inhibitory Factor (MIF) Deficiency Exacerbates Aging-Induced Cardiac Remodeling and Dysfunction Despite Improved Inflammation: Role of Autophagy Regulation. Sci. Rep. 6, 1–15. doi:10.1038/srep22488
Youm, Y.-H., Grant, R. W., McCabe, L. R., Albarado, D. C., Nguyen, K. Y., Ravussin, A., et al. (2013). Canonical Nlrp3 Inflammasome Links Systemic Low-Grade Inflammation to Functional Decline in Aging. Cel Metab. 18, 519–532. doi:10.1016/j.cmet.2013.09.010
Yu, J., Nagasu, H., Murakami, T., Hoang, H., Broderick, L., Hoffman, H. M., et al. (2014). Inflammasome Activation Leads to Caspase-1-dependent Mitochondrial Damage and Block of Mitophagy. Proc. Natl. Acad. Sci. U.S.A. 111, 15514–15519. doi:10.1073/PNAS.1414859111
Yuan, Z., Lu, Y., Wei, J., Wu, J., Yang, J., and Cai, Z. (2021). Abdominal Aortic Aneurysm: Roles of Inflammatory Cells. Front. Immunol. 11, 3758. doi:10.3389/FIMMU.2020.609161/BIBTEX
Zhou, R., Yazdi, A. S., Menu, P., and Tschopp, J. (2011). A Role for Mitochondria in NLRP3 Inflammasome Activation. Nature 469, 221–225. doi:10.1038/nature09663
Keywords: inflammaging, cardiovascular disease, aging, inflammation, senescence
Citation: Puspitasari YM, Ministrini S, Schwarz L, Karch C, Liberale L and Camici GG (2022) Modern Concepts in Cardiovascular Disease: Inflamm-Aging. Front. Cell Dev. Biol. 10:882211. doi: 10.3389/fcell.2022.882211
Received: 23 February 2022; Accepted: 13 April 2022;
Published: 18 May 2022.
Edited by:
Xiaoqiang Tang, West China Second University Hospital, Sichuan University, ChinaReviewed by:
Jun Ren, University of Washington, United StatesHou-Zao Chen, Chinese Academy of Medical Sciences and Peking Union Medical College, China
Copyright © 2022 Puspitasari, Ministrini, Schwarz, Karch, Liberale and Camici. This is an open-access article distributed under the terms of the Creative Commons Attribution License (CC BY). The use, distribution or reproduction in other forums is permitted, provided the original author(s) and the copyright owner(s) are credited and that the original publication in this journal is cited, in accordance with accepted academic practice. No use, distribution or reproduction is permitted which does not comply with these terms.
*Correspondence: Giovanni G. Camici, Z2lvdmFubmkuY2FtaWNpQHV6aC5jaA==