- Department of Biology, Johns Hopkins University, Baltimore, MD, United States
Humans rely on visual cues to navigate the world around them. Vision begins with the detection of light by photoreceptor cells in the retina, a light-sensitive tissue located at the back of the eye. Photoreceptor types are defined by morphology, gene expression, light sensitivity, and function. Rod photoreceptors function in low-light vision and motion detection, and cone photoreceptors are responsible for high-acuity daytime and trichromatic color vision. In this review, we discuss the generation, development, and patterning of photoreceptors in the human retina. We describe our current understanding of how photoreceptors are patterned in concentric regions. We conclude with insights into mechanisms of photoreceptor differentiation drawn from studies of model organisms and human retinal organoids.
Introduction
Humans and other primates use their sense of sight as a primary mechanism for navigating their environments. The human camera eye relies on the cornea and lens to focus light onto the retina, a tissue located in the back of the eye. Vision begins with the detection of light by photoreceptor cells within the retina (Figure 1).
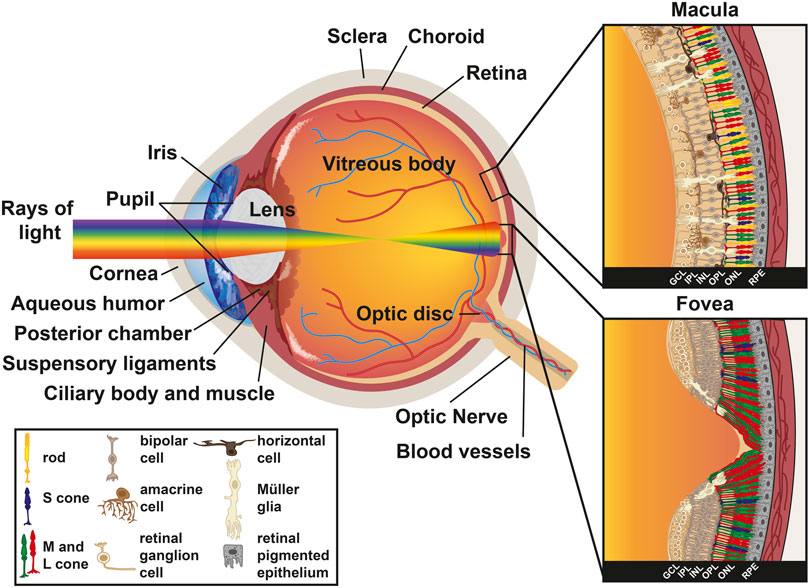
FIGURE 1. Anatomy of the human eye. Diagram of a human eye with the light path represented as a rainbow. Inset sections represent the retinal cell types present in the macula (top) and the fovea (bottom). Key describes retinal cell types.
Unlike other placental mammals, primates have trichromatic color vision and high visual acuity. Trichromacy is achieved by three distinct cone photoreceptor subtypes that enable the sensation of blue, green, and red wavelengths of light. The three cones signal through interneurons and retinal ganglion cells to the brain to perceive the colors of the visible spectrum.
The goal of this review is to describe patterning and development of the photoreceptors in the human retina. We briefly describe the major cell types of the human retina and their roles in vision. We then focus on photoreceptors, discussing how light sensitivities, cell morphologies, gene expression profiles, and functions define photoreceptor subtypes. Next, we discuss how genetic and signaling pathways influence photoreceptor subtype specification. We continue with the patterning of photoreceptor subtypes across the retina, with a particular focus on the fovea and foveola, the structures that enable high visual acuity in primates. We conclude with a description of how studies of human retinal organoids have advanced our understanding of photoreceptor subtype differentiation and how organoids serve as a promising new model to understand mechanisms of retinal development and disease.
Retinal Cell Types
The vertebrate eye contains five major neuronal types generated from a common progenitor pool: photoreceptors, bipolar cells, retinal ganglion cells (RGCs), horizontal cells, and amacrine cells. Retinal progenitor cells also generate one glial type called Müller glia. The cell types of the retina are stratified into three layers of cell bodies and two plexiform layers: 1) the outer nuclear layer (ONL) contains the photoreceptors, 2) the outer plexiform layer (OPL) contains the synapses from the photoreceptors onto interneurons, 3) the inner nuclear layer (INL) contains the cell bodies of bipolar, horizontal, and amacrine interneurons, which transmit and process visual information, and Müller glia, which provide structural support and maintain the extracellular environment, 4) the inner plexiform layer (IPL) is the location where interneurons synapse onto the retinal ganglion cells, and 5) the ganglion cell layer (GCL) primarily contains retinal ganglion cells that relay information from the eye to the brain (Figure 1).
The layers of the human retina are inverted relative to the path of light. Light passes through the GCL first, continues through the IPL, INL, and OPL, and is absorbed by photoreceptors whose nuclei lie in the ONL (Figure 1). Müller glia act as light cables that guide the yellow-green portion of the visible light spectrum (around 560 nm) through the RGCs and interneurons, directly to cone cells (Franze et al., 2007; Agte et al., 2011; Labin et al., 2014; Agte et al., 2018). Müller glia also play important roles in their interactions with photoreceptors and other neurons, acting as sinks for metabolic waste and removal of excess neurotransmitter within the retina (Newman, 1994; Tsacopoulos and Magistretti, 1996). Stray photons are absorbed in the retinal pigmented epithelium (RPE), a melanin-rich epithelial cell layer that lies behind the retina. The RPE recycles retinal, a light-sensitive chromophore required for the detection of light by the opsin protein, from all-trans-retinal into 11-cis-retinal and returns it to photoreceptors, which is essential for photoreceptor health and function (Baehr et al., 2003; Thompson and Gal, 2003). Additionally, Müller glia recycle all-trans-retinal back to 11-cis-retinal for cones exclusively (Mata et al., 2002; Kolesnikov et al., 2021). The RPE also plays a role in phagocytosis of photoreceptor outer segments as they are shed (Strauss et al., 1998; Gal et al., 2000; Finnemann, 2003). Astrocytes, another glial cell type that migrates into the retina via the optic nerve, are also present in the GCL (Stone et al., 1995).
Light information is detected when a photon is captured by a photoreceptor in the ONL. Electrical signals are transmitted back towards the inner retinal layers by bipolar cells, horizontal cells, and amacrine cells in the INL, and conveyed to RGCs in the GCL. Finally, this information is carried by RGCs to the brain.
Photoreceptors, the focus of this review, are classified as either rods or cones and have cell bodies in the ONL (Figure 1). These cell types can be distinguished by their morphologies and spectral sensitivities. Rods, with the sensitivity of single-photon detection, are the cell type responsible for low light vision and motion detection (Baylor et al., 1979). In contrast, cones are primarily involved in high-acuity daytime and color vision (Nathans et al., 1986b; Jacobs and Deegan, 1999). There is estimated to be 92 million rods (77.9–107.3 million) and 4.6 million cones (4.08–5.29 million) in the human retina (Curcio et al., 1990). In humans, the three cone subtypes are defined by the expression of opsin proteins that are optimally sensitive to different wavelengths of light. Cones contain either short-wavelength sensitive opsins (S/blue cones), medium-wavelength sensitive opsins (M/green cones), or long-wavelength sensitive opsins (L/red cones). As S cones comprise the minority (8–12%) of the cone population (Curcio et al., 1991), spatial information appears to be primarily processed by M and L cones (Lennie et al., 1993). We revisit the differentiation and patterning of photoreceptors in the human retina in much greater detail later in this review.
Photoreceptor Spectral Sensitivities, Morphologies, and Orientations
Photoreceptors are classified based on their expression of light-detecting opsin photopigments, morphologies, functions, and locations in the human retina. Light detection depends on the pathway that light takes when entering and traveling through the eye, as well as the spectral sensitivities of the photopigments expressed in rods and cones. Phototransduction begins as light enters the cornea, the transparent tissue at the most anterior region of the eye. Light then passes through the pupil. Pupil size is regulated by the iris, the pigmented part of the eye, to modulate the amount of light passing into the eye. Light then hits the lens, an ellipsoid, biconvex structure that refracts focused light onto the retina. After the lens, light passes through the aqueous humor. Ciliary muscle contraction regulates the flow of aqueous humor and lens shape. Finally, light reaches the retina and is detected by the photoreceptors (Figure 1).
In order to initiate the phototransduction cascade, photons must pass through the cell bodies of many cell types, including the photoreceptors themselves, before hitting the opsin photopigments in the photoreceptor outer segments (Figure 2). Opsin photopigments are light-sensitive G-protein coupled receptors that are covalently linked to an 11-cis-retinal chromophore through a Schiff base (Nathans et al., 1986b; Kosower, 1988; Sakmar and Khorana, 1988; Zhukovsky and Oprian, 1989). Absorption of a photon by the chromophore induces a conformational change to all-trans-retinal, which triggers the opsin to initiate the phototransduction cascade (Hubbard and Kropf, 1958). The opsin then activates transducin, a G-protein. Transducin subsequently activates PDE6, a cGMP-phosphodiesterase, which is responsible for conversion of cGMP to GMP, and sodium channels subsequently close. The decrease in the sodium current results in the release of glutamate at the synapse which signals to downstream interneurons (Grossniklaus et al., 2015). In this section, we describe how opsin expression and morphological differences among rods and cones drive functional properties of these photoreceptors.
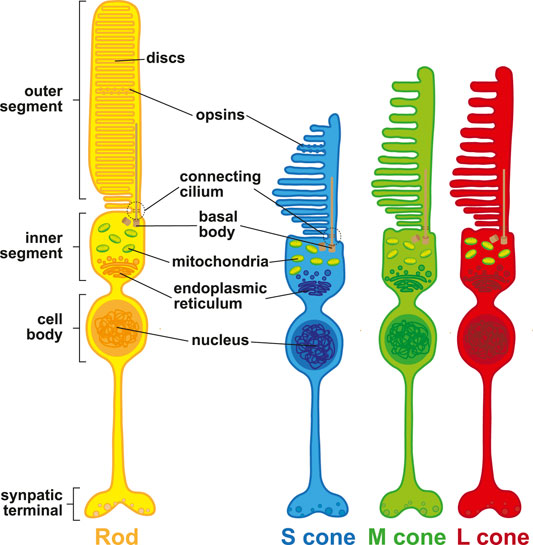
FIGURE 2. Photoreceptor morphology. Schematic representation of rod photoreceptors (yellow), S (blue), M (green), and L (red) cone photoreceptors.
Spectral Sensitivities of Opsin Proteins
The spectral sensitivity of each photoreceptor type is determined by the expression of distinct opsin proteins. Rods express rhodopsin (RHO) and cones express S-, M-, or L-opsin (OPN1SW/MW/LW). The unique spectral sensitivities of the opsins are related to differences in their protein structures, and consequentially their interaction with the light-sensitive chromophore. In humans, absorption maxima are 498 nm for rhodopsin, 420 nm for S-opsin, 534 nm for M-opsin, and 564 nm for L-opsin (Bowmaker et al., 1980) (Figure 3A). Rhodopsin is distinct from the cone opsins, sharing only 42% protein identity with S-opsin, 40% protein identity with L-opsin, and 41% protein identity with M-opsin. S-opsin shares 43% protein identity to L-opsin and 44% protein identity to M-opsin. In contrast, M- and L-opsin are highly similar, sharing 96% protein identity (Francois, 1958; Nathans et al., 1986a; Nathans et al., 1986b) with only 7 nonsynonymous amino acid changes among 20 DNA base pairs that differ between the coding sequence (Nathans et al., 1986b) (Figure 3B). Based on the differences in opsin sequence, immunohistochemistry against opsin proteins can be used to visually distinguish rhodopsin in rods, S-opsin in S cones, and M- or L-opsin in M or L cones. The generation of antibodies specific to M- and L-opsins has been hindered by the very high sequence homology (Szél et al., 1988; Lerea et al., 1989; Wang et al., 1992; Chiu and Nathans, 1994).
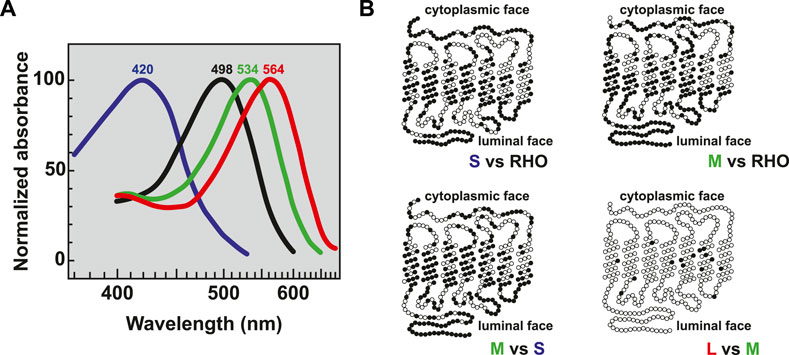
FIGURE 3. Opsin absorption and comparative morphology. (A) Relative absorption spectra of opsins with labeled peaks for S cones (blue, 420 nm), Rods (black, 498 nm), M cones (green, 534 nm), and L cones (red, 564 nm) adapted from (Bowmaker et al., 1980). (B) Pairwise comparisons of amino acids between the visual opsin proteins adapted from (Nathans et al., 1986b). Dark circles indicate amino acid differences. Sequences were optimally aligned such that no insertions or deletions were required except for comparing the carboxy termini between the green or red and blue pigments.
Photoreceptor Morphologies
At their distal end, photoreceptor cells contain an outer segment (Figure 2). The outer segment is a modified cilium that contains the light-detecting opsin photopigments and contacts the extended processes of the RPE. The outer segment is connected through a cilium to an inner segment, which is rich in mitochondria and acts as the site of photopigment and membrane disc synthesis. These discs renew in the outer segments, which are shed over the lifetime of the photoreceptor (Young, 1967). The inner segment is connected to the cell body, which contains the cell nucleus, and lastly axons which project medially into the retina.
Rods, S cones, and M/L cones are distinguished by unique morphological characteristics. Differences in the sizes and shapes of inner segments affect how light is guided through the cell to reach the outer segment (Winston, 1981). Rods have long, cylindrical inner and outer segments. Rod outer segments are stacked with parallel membranous discs independent from the plasma membrane (Sjostrand, 1953). Rod discs have unique protein compositions compared to the plasma membrane, but rhodopsin is found on both membranes (Molday and Molday, 1987). Rod inner segments can be separated into the ellipsoid, which contains a large number of mitochondria, and the myoid, which connects the inner segment to the nucleus. mRNAs for outer segment proteins are localized to the inner segment (Wensel et al., 2016).
In contrast, cones have a characteristic conical shape with tapered ends, and contain parallel membranous discs that are nearly continuous with the plasma membrane (Cohen, 1970). S cones have shorter outer segments than M/L cones. The inner segments of S cones are 10% taller than M/L cones, and their outer segments are wider relative to M/L cones (Ahnelt et al., 1987; Ahnelt et al., 1990; Curcio et al., 1991). M cones and L cones are morphologically indistinguishable from one another. Cone inner and outer segments mature and continue to develop postnatally. Inner segments begin to develop around 25 weeks of gestation. In contrast, outer segments in the fovea do not begin development until 1 week after birth and mature for years until they reach a length of ∼60 µm (Yuodelis and Hendrickson, 1986).
Photoreceptor Orientation
Nearly a century ago, Stiles and Crawford used psychophysics to show that human eyes are more sensitive to light that enters through the center of the pupil than light entering at the pupil’s edge (Crawford, 1933). Photoreceptors are oriented in the retina such that their outer segments are pointed toward the pupil to enhance light absorption and improve visual acuity in a coordinated alignment of polarized cells (Housset et al., 2021; Verschueren et al., 2022). This orientation is more pronounced in the peripheral retina (Laties et al., 1968; Laties, 1969; Laties and Enoch, 1971; Enoch, 1972). Toward the periphery, the angle between a cone cell body and its outer segment can be as great as 40° (Laties, 1969). All photoreceptors are oriented based on position, though the tilting is more significant in cones, perhaps due to the decreased sensitivity of cone cells compared to rods (Ingram et al., 2016).
Photoreceptor Subtype Specification
Multipotent progenitor cells in the retina give rise to different retinal cell types, including photoreceptors (Turner and Cepko, 1987; Wetts and Fraser, 1988; Turner et al., 1990). Cell types are born in conserved, overlapping spatiotemporal waves (Rapaport et al., 2004). In the human retina, RGCs are born first, followed by horizontal cells, cones, amacrine cells, rods, bipolar cells, and finally Müller glia (Cepko, 2014; Lu et al., 2020) (Figure 4). These cells are specified in two temporal waves or phases (Rapaport et al., 2004). During the first phase, retinal ganglion cells, horizontal cells, and cones are born. During the later phase, rods, bipolar cells, and Müller glia are born (Rapaport et al., 2004). Amacrine cells span the gap between phases (Rapaport et al., 2004). As mentioned, photoreceptors are divided between these waves, with cones being generated before rods (Carter-Dawson and LaVail, 1979; La Vail et al., 1991).
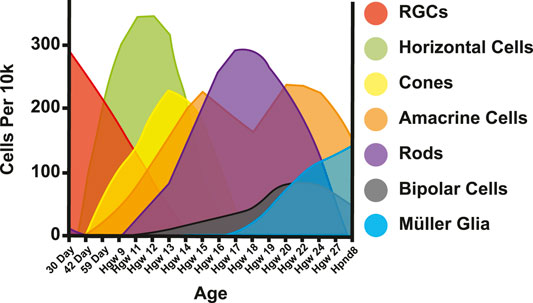
FIGURE 4. Retinal cell birth order. Adapted from (Lu et al., 2020). Cells per 10k = expected cells in 10k of cells. Day = Day post conception. Hgw = Human gestation week. Hpnd = Human postnatal day.
Photoreceptor differentiation occurs across the retina from the central fovea to the periphery in spatiotemporal waves of differentiation (La Vail et al., 1991; Xiao and Hendrickson, 2000). The specification of photoreceptors is determined by the expression of their respective opsin protein. S cones are specified first (as defined by S-opsin expression), followed by M/L cones (M/L-opsin expression) and finally, rods (rhodopsin expression) (Cepko, 2014; Eldred et al., 2018). At birth, some rods do not yet express rhodopsin, suggesting that rod generation and maturation continues postnatally (Hendrickson et al., 2008).
The genetic network specifying photoreceptor fate is well-studied. The transcription factor paired box 6 (PAX6) is expressed in multipotent progenitor cells and is essential for the specification of retinal cell fates (Marquardt et al., 2001). The receptor Notch is expressed in retinal progenitors to maintain progenitor state (Nelson et al., 2007). As progenitor cells exit the cell cycle and differentiate into photoreceptor fates, Notch expression ceases and cells express the orthodenticle homeobox 2 (OTX2) transcription factor (Jadhav et al., 2006; Muranishi et al., 2011). OTX2 is essential for photoreceptor fate specification and its absence leads to an amacrine-like fate (Nishida et al., 2003). OTX2 activates downstream genes including visual system homeobox 2 (VSX2) (Kim et al., 2008), PR/SET domain 1 (PRDM1) (Brzezinski et al., 2010; Katoh et al., 2010), and cone-rod homeobox protein (CRX) (Nishida et al., 2003). VSX2 is upregulated in bipolar cells (Kim et al., 2008) and represses rod photoreceptor genes (Dorval et al., 2006; Livne-Bar et al., 2006). PRDM1 stabilizes photoreceptor fate and prevents immature cells from differentiating into bipolar cells (Brzezinski et al., 2010) by repressing VSX2 (Katoh et al., 2010). As PRDM1 levels increase, it negatively feeds back onto OTX2, ultimately turning off its own expression and driving rod fate (Wang et al., 2014). Finally, CRX is present in all photoreceptors (Chen et al., 1997). CRX is important for photoreceptor maintenance, as CRX mutations result in cone-rod dystrophy (Freund et al., 1997) or Leber’s congenital amaurosis (Swaroop et al., 1999), which causes photoreceptor degeneration and vision loss. Conversely, overexpression of CRX increases rods at the expense of Müller glia and amacrine cells (Furukawa et al., 1997).
Rod Specification
Upon acquiring photoreceptor fate, photoreceptors choose between cone and rod fates. RAR-related orphan receptor B (RORB) is a nuclear hormone receptor expressed in all photoreceptors. RORB acts upstream of Neural retina-specific leucine zipper protein (NRL), a critical regulator of rod fate (Jia et al., 2009; Kautzmann et al., 2011; Fu et al., 2014). NRL is necessary and sufficient for rod fate, as its loss results in a complete absence of rods and an increase in S cone-like photoreceptors (Mears et al., 2001; Akimoto et al., 2006), and ectopic expression leads to a loss of cones and an increase in rods (Oh et al., 2007). NRL directly binds and represses the promoters of both thyroid hormone receptor beta (THRB) and S-opsin, genes essential for cone specification (Oh et al., 2007). NRL also activates nuclear receptor subfamily 2 group E member 3 (NR2E3), which represses cone fate and activates a subset of rod genes including rhodopsin (Chen et al., 2005; Peng et al., 2005; Cheng et al., 2006; Oh et al., 2008). NR2E3 mutations result in improper lamination and enhanced S cone syndrome, with an increase in S cones and a number of visual defects (Haider et al., 2000; Jacobson et al., 2004).
Retinoic acid (RA) has also been implicated in the promotion of rod fate. In zebrafish, exogenous RA causes an increase in rods with a loss of cones (Hyatt et al., 1996). RA drives rhodopsin expression in cultured cells (Kelley et al., 1994; Kelley et al., 1999) and NRL expression in retinoblastoma cells and primary cell culture (Khanna et al., 2006). Regulation of NRL by RA may be direct as the NRL promoter contains a retinoic acid response element (RARE) (Khanna et al., 2006). Along with these regulators, taurine and sonic hedgehog (SHH) also promote rod fate in cultured cells (Altshuler et al., 1993; Levine et al., 1997).
Cone Specification
Like rods, cones are specified by a distinct regulatory logic. One cut homeobox 1 (ONECUT1), a member of the Cut homeobox family of transcription factors, acts with OTX2 to promote cone fate by activating expression of nuclear thyroid hormone receptor THRB/B2, an early marker of cone fate (Sjoberg et al., 1992; Ng et al., 2009; Emerson et al., 2013). Discriminating the roles of THRB (including THRB1 and THRB2) versus the THRB2 isoform in human and mouse has been complicated (Ng et al., 2001; Roberts et al., 2006; Applebury et al., 2007; Weiss et al., 2012; Eldred et al., 2018), as THRB2 lies interior in the THRB locus. THRB is a thyroid hormone receptor that acts as a transcription factor at thyroid response elements (TREs). In the absence of thyroid hormone, THRB is bound by corepressors. When thyroid hormone is present, it binds THRB, which induces the replacement of corepressors by coactivators, ultimately regulating gene expression (McNerney and Johnston, 2021). Thyroid hormone receptors can homodimerize or heterodimerize with retinoid X receptor gamma (RXRG), a nuclear receptor expressed in cones (Mori et al., 2001), to regulate aspects of cone subtype specification (Roberts et al., 2005). In mouse, heterodimers of RXRG and THRB play a role in establishing the S-cone pattern. Since the expression dynamics of these transcription factors are similar in human and mouse retinal development, this heterodimer pair might play a similar role in human cone patterning (Roberts et al., 2005). Additionally, POU2F2 has been shown in mouse to repress NRL and promote cone fate (Javed et al., 2020).
Cone Subtype Specification: S Versus M/L Cone Fates
Human cones choose one of three subtype fates: S/blue, M/green, or L/red. Cone subtypes are specified in a two-step process. In the first decision, cones choose either the S or M/L cone fate. The second decision occurs between M and L cone fates.
Thyroid hormone signaling regulates the first choice between S and M/L cone fates. Thyroid hormone has two main forms: the active form, T3, which binds with high affinity to thyroid hormone receptors, and T4, the less active, circulating form (Samuels et al., 1974; Schroeder and Privalsky, 2014; McNerney and Johnston, 2021). Thyroid hormones cannot diffuse freely across cell membranes but rather require transporters such as MCT8 (Dumitrescu et al., 2004; Friesema et al., 2004). Moreover, deiodinase enzymes locally modulate levels of T3 and T4. Specifically, deiodinase 3 (DIO3) degrades both T3 and T4, while deiodinase 2 (DIO2) converts T4 into the active T3 (Dentice et al., 2013). DIO3 and DIO2 are expressed dynamically in the developing human retina as well as in the retinas of other species (Trimarchi et al., 2008; Ng et al., 2010; Guo et al., 2014; Bagci et al., 2015).
Foundational work in model organisms established a role for thyroid hormone signaling in cone subtype specification (Sjoberg et al., 1992; Ng et al., 2001; Roberts et al., 2006; Applebury et al., 2007; Trimarchi et al., 2008; Suzuki et al., 2013). Mice lacking THRB2 show a complete loss of M cones and only express S-opsin in cones (Ng et al., 2001; Roberts et al., 2006). Similarly, THRB knockouts display altered cone subtype ratios in fish (Suzuki et al., 2013; Mackin et al., 2019). Thyroid hormone signaling is also sufficient to regulate cone fates, as increasing T3 induces M-opsin expression in mice (Roberts et al., 2006; Glaschke et al., 2010; Glaschke et al., 2011). Moreover, knockdown of MCT8, a thyroid hormone transporter, altered cone subtype ratios in chicken (Vancamp et al., 2019).
Regulators of the thyroid hormone pathway are expressed in waves during development of the chicken retina. During early neurogenesis, a wave of DIO3 expression in cone progenitors occurs from the center to the periphery (Trimarchi et al., 2008). As DIO3 expression fades, TRß expression initiates, but is limited to a subset of progenitor cells (Trimarchi et al., 2008). A wave of DIO2 expression in a subset of progenitor cells in the outer neuroblastic layer, an early, proliferative region of the retina, marks the beginning of photoreceptor differentiation, beginning as a ventral to dorsal gradient that gradually becomes restricted to the ventral retina. Simultaneously, a horizontal stripe of DIO3 expression occurs across the central retina (Trimarchi et al., 2008). Over time, this DIO3 expression expands to the periphery (Trimarchi et al., 2008). Lastly, DIO3 expression is lost in a wave from the center to the periphery, concurrent with progenitor cell differentiation into Müller glia (Trimarchi et al., 2008). Following these waves of expression, DIO3 is expressed in progenitors, TRα (another thyroid hormone receptor) is expressed in most cells of the retina, and DIO2 is expressed in a subset of photoreceptors (Trimarchi et al., 2008). Together, these studies across multiple model organisms show that thyroid hormone signaling is spatially and temporally dynamic, and fundamental for retina development and photoreceptor differentiation.
Consistent with these studies in model organisms, clinical evidence supports a role for thyroid hormone signaling in cone subtype differentiation in the human retina. Premature infants with low ratios of T3 to T4 have an increased incidence of color vision deficiencies (Dowdeswell et al., 1995; Rovet and Simic, 2008; Simic et al., 2010; Yassin et al., 2019). Additionally, patients with mutations in THRB display altered color perception (Weiss et al., 2012). More recently, DIO2 was found to be expressed in retinal precursor cells in the human macula during development (Lu et al., 2020). These studies implicate thyroid hormone signaling in human cone subtype specification.
To directly address the role of thyroid hormone signaling in human cone subtype specification, we studied human retinal organoids. Human retinal organoids are model retinas that are differentiated from human stem cells and grown in a dish. They recapitulate the temporal dynamics of human cone subtype specification during fetal development (Nakano et al., 2012; Zhong et al., 2014; Kaewkhaw et al., 2015; Wahlin et al., 2017; Eldred et al., 2018; Phillips et al., 2018). In human retinas and organoids, S cones are generated before M/L cones. THRB mutant organoids have S cones only, whereas addition of T3 in wild type organoids produces M/L cone-rich organoids. Moreover, the addition of T3 to THRB mutant organoids generates organoids with all S cones, indicating that T3 acts through THRB to specify M/L cones (Eldred et al., 2018). Thus, thyroid hormone signaling is necessary and sufficient to promote M/L cone fates and suppress S cone fates in humans.
The expression of thyroid hormone regulators in human retinas and retinal organoids is consistent with a temporal role of thyroid hormone signaling in the generation of S cones before M/L cones during human retinal development. DIO3, the enzyme which degrades T3, is expressed early, around the time when S cones are specified (Hoshino et al., 2017; Eldred et al., 2018). In contrast, DIO2, which synthesizes T3, is expressed later in development, when M/L cones are specified (Hoshino et al., 2017; Eldred et al., 2018). These studies suggest that expression of thyroid hormone regulators is temporally regulated to decrease thyroid hormone signaling early to specify S cones and increase signaling late to specify M/L cones.
Along with the role of thyroid hormone, PIAS3 is activated by TRB2 and RXRY in mice. PIAS3, as a result, is expressed at higher levels in M cones over S cones. PIAS3 then acts in M cones to SUMOylate RORA to repress S-opsin. PIAS3 can also act to enhance the effect of T3 driven M-opsin expression (Onishi et al., 2010). Interestingly, PIAS3-dependent SUMOylation of NR2E3 within rod cells can strongly prevent the expression of S cone genes (Onishi et al., 2009), suggesting multiple distinct roles for PIAS3 in retinal cell type specification.
The COUP family of transcription factors also plays a role in the specification of cone subtype specification in mice. Mice have a gradient of cone subtypes with more M cones in the dorsal retina and more S cones in the ventral retina (Wikler et al., 1996; Eldred et al., 2020). COUP-TFI suppresses M-opsin ventrally while COUP-TFI and COUP-TFII suppress S-opsin dorsally. In human cell lines, COUP-TFs repress S-opsin expression, suggesting these two transcription factors also play a crucial role in cone subtype specification (Satoh et al., 2009).
SALL3 also plays an important role in cone subtype specification. SALL3 is expressed in the S cones of mice and drives expression of S-opsin. SALL3 null mice showed a downregulation of S-opsin and phototransduction genes (de Melo et al., 2011). Though PIAS3, the COUP transcription factors, and SALL3 have not been directly implicated in human cone subtype specification, they are promising candidates to evaluate for roles through genetic manipulation in human retinal organoids.
Cone Subtype Specification and Differences: M Versus L Cone Fates
Cones that adopt the M/L cone fate then choose between M or L cone fates. The only known difference between M and L cones is the expression of their respective opsin photopigment (Yamaguchi et al., 1997; Hagstrom et al., 2000; Peng et al., 2019). The M-opsin and L-opsin genes are located in a tandem array on the distal portion of the q arm of the X chromosome (Francois, 1958; Nathans et al., 1986a; Vollrath et al., 1988; Feil et al., 1990). As a result, regulation of these genes occurs at only one locus due to hemizygosity in males and X-inactivation in females (Wang et al., 1992).
The M- and L-opsin genes are regulated by a shared DNA element called the locus control region (LCR), which is required for the expression of both genes (Nathans et al., 1989; Wang et al., 1992). The LCR is an ancient promoter element that predates mammals with ancestral origins in fish (Tsujimura et al., 2007). Within mammals, the LCR is highly conserved among diverse species including humans, cows, and mice, and predates the duplication of M-opsin to generate L-opsin (Wang et al., 1992), yet the promoters of the opsin genes are not conserved.
The number and arrangement of M- and L-opsin gene copies relative to the LCR is variable. In the most common arrangement, the L-opsin gene is proximal to the LCR, followed by multiple copies of the M-opsin gene. M-opsin genes are most often found in a range of 1–5 copies with a mode of 2 (Drummond-Borg et al., 1989). No matter the number of M-opsin genes in the array, only the first M-opsin is expressed (Jorgensen et al., 1990; Winderickx et al., 1992; Yamaguchi et al., 1997; Hayashi et al., 1999). In addition to the proximal copy of the L-opsin gene, L-opsin genes are also sometimes observed in the distal array (Ueyama et al., 2015).
Two nonexclusive models for M and L cone specification have been proposed: the “stochastic” model and the “temporal” model (Smallwood et al., 2002). In the stochastic model, M and L cones randomly choose between these two fates. In this model, the regulatory LCR DNA element loops to either the M-opsin promoter or the L-opsin promoter to stably and exclusively drive expression of one opsin (Wang et al., 1999; Smallwood et al., 2002). This model is supported by transgene experiments in mice suggesting that the order and proximity of the opsin genes relative to the LCR controls the probability of opsin expression (Smallwood et al., 2002). These experiments suggest that the distal M promoter interacts with the LCR more effectively than the proximal L promoter.
In the temporal model, also known as the standard model, M cones are generated before L cones. Functional microspectrophotometry, multifocal-ERG imaging, and mRNA expression studies suggest that the retinal periphery has a higher proportion of L cones to M cones (La Vail et al., 1991; Mollon and Bowmaker, 1992; Hagstrom et al., 1998; Bumsted and Hendrickson, 1999; Martin et al., 2000; Otake et al., 2000; Xiao and Hendrickson, 2000; Roorda et al., 2001; Carroll et al., 2002; Bowmaker et al., 2003; Cornish et al., 2004; Neitz et al., 2006; Kuchenbecker et al., 2008). Since the retina develops from the center to the periphery, the earliest cells lie nearest to the fovea and the last-born cells are in the periphery. The higher proportion of L cones in the periphery suggest that L cones are the last cells to differentiate. Our analysis of M- and L-opsin expression suggested that M-opsin is expressed first in human fetal development, consistent with temporal regulation of the M/L cone fate decision (Hadyniak et al., 2021).
Experimental interrogation of the mechanism controlling M versus L cone subtype specification has been limited in large part due to the difficulty differentiating between the two cone subtypes. As the proteins are too similar to distinguish by antibodies, sequencing experiments and adaptive optics have been the primary methods used to understand the ratios of these two cone subtypes (Hofer et al., 2005). We recently took advantage of new advances in RNA in situ hybridization to directly visualize M and L cones and conduct spatiotemporal analysis of cone development (Hadyniak et al., 2021). This RNA in situ hybridization approach relies on using two partially overlapping oligos with three key nucleotide differences that enable binding specifically to the M- or L-opsin mRNAs.
Experimentally tractable model systems to study M versus L cone specification are lacking as the L cone subtype is unique to humans and non-human primates amongst placental mammals. Human retinal organoids provide a promising model to test mechanisms of human-specific developmental mechanisms. Treatment of retinal organoids with exogenous retinoic acid during early stages of development promotes M cone fate at the expense of L cones (Hadyniak et al., 2021), providing the first cue to a pathway responsible for the differences in M and L cone fates.
Variation in the Ratios of Cone Subtypes
The percentage of S cones (8–12%) relative to M/L cones in the human retina is consistent (de Monasterio et al., 1985; Ahnelt et al., 1987; Szél et al., 1988; Lerea et al., 1989; Ahnelt et al., 1990; Nork et al., 1990; Curcio et al., 1991). In contrast, the ratio of L:M cones (historically reported as L before M) in the human retina varies dramatically across the population from 1:4 to 16.5:1, with an average ratio of 2:1 (De Vries, 1946; Rushton and Baker, 1964; Dartnall et al., 1983; Cicerone and Nerger, 1989; Jacobs and Neitz, 1993; Yamaguchi et al., 1997; Hagstrom et al., 1998; Brainard et al., 1999; Roorda and Williams, 1999; Deeb et al., 2000; Kremers et al., 2000; Otake et al., 2000; Carroll et al., 2002; Hofer et al., 2005; McMahon et al., 2008). The range of variability in L:M cones implicates a level of biological plasticity as individuals who have extreme L:M ratios retain normal color vision.
Evidence suggests that changes in the nucleotide sequences in the L/M-opsin loci are associated with variation in the ratio (Gunther et al., 2008), but the differences between the L and M promoters did not account for this variation (McMahon et al., 2004). Our studies identified changes in the non-coding antisense RNA of NR2F2, a transcription factor activated by retinoic acid, that are associated with changes in the L:M ratio, consistent with the role for RA and its sufficiency to generate M cones early in retinal organoids (Hadyniak et al., 2021).
In contrast to the highly variable L:M ratio in humans, the ratio in non-human primates is more reproducible and more or less equal, ranging from 0.6:1 to 1.17:1 (Marc and Sperling, 1977; Mollon and Bowmaker, 1992; Packer et al., 1996), suggesting evolutionary differences in mechanisms of L and M cone differentiation between primates.
Technical challenges may complicate the analyses of M and L cone ratios. As M and L cones could not be visualized directly in fixed tissue prior to our recent studies, measurement methods may contribute to the variability observed in humans. Methods that rely on mRNA copy number assume equal transcription between M and L cone opsin genes. Physiological methods assume that each M and L cone contributes equally to the visual response. Microspectrophotometry, using a combination of high-resolution retina imagining with retinal densitometry, provides one of the most accurate forms of individual visualization of the L/M cone ratio (Hofer et al., 2005). Our development of an in situ hybridization approach to distinguish M- and L-opsin will enable new studies of variation in fixed tissue with single cell resolution moving forward (Hadyniak et al., 2021).
Photoreceptor Developmental Disorders
Mutations in essential rod pathway genes can lead to visual disorders. NR2E3 is a transcription factor that drives rod fate, including rhodopsin gene expression (Cheng et al., 2004; Peng et al., 2005; Cheng et al., 2006). Patients with mutations in NR2E3 can present with enhanced S cone syndrome (a condition in which the retina lacks rods but produces an unusually high number of S cones), night blindness, hypersensitivity of S cones, impairment of M and L cones, loss of rod function, and a decrease in total rods (Jacobson et al., 1990; Marmor et al., 1990; Hood et al., 1995). One study identified a loss of rods along with double the typical number of cones, with 92% of those being S cones (Milam et al., 2002). These clinical data suggest that this gene is responsible for suppressing the S cone fate and driving proper rod specification (Haider et al., 2000; Haider et al., 2006).
Similarly, mutations in cone specification and development leads to human visual disorders. Color blindness affects 1 in 12 Caucasian males and 1 in 200 Caucasian females (McKusick, 2007). Mutations which cause cone-related disease and color blindness can come from essential cone genes or from mutations in the cone opsins themselves. The most severe form of color blindness is achromatopsia, marked by the true absence of color discrimination and monochromatic color vision. The two main types of achromatopsia are rod monochromacy and S cone monochromacy. Rod monochromacy is an autosomal recessive disease (Spivey, 1965; Fleischman and O'Donnell, 1981) in which individuals have normal rods with functioning rhodopsin, but no cone sensitivity (Sloan, 1954; Blackwell and Blackwell, 1961). This is typically caused by mutations in essential cone genes such as activating transcription factor 6 (ATF6), cyclic nucleotide-gated cation channel alpha-3 (CNGA3), cyclic nucleotide-gated channel subunit beta-3 (CNGB3), G protein subunit alpha transducin 2 (GNAT2), or phosphodiesterase 6C (PDE6C) (Rosenberg et al., 2004; Vincent et al., 2011; Kohl et al., 2012; Carss et al., 2017; Skorczyk-Werner et al., 2017). S cone monochromacy is an X-linked disease in which individuals have rods and S cones, but completely lack M and L cones (Blackwell and Blackwell, 1961; Alpern et al., 1965; Pokorny et al., 1970; Alpern et al., 1971; Daw and Enoch, 1973; Alpern and Pugh, 1974; Smith et al., 1978; 1979).
Another form of color blindness, dichromacy, is marked by the absence or loss of function of one cone subtype. Loss of expression of a cone subtype-specific opsin is linked to the loss of function of the respective cone subtype. The most common form of color blindness in the population is anomalous trichromacy, where individuals have altered spectral sensitivity of one opsin. Anomalous trichromacy is caused by point mutations in opsin genes or gene rearrangements (Nathans et al., 1986a).
In addition to these disorders caused by breakdowns in specification or function during development, numerous degenerative diseases affecting rods and cones cause vision loss [reviewed in (Sancho-Pelluz et al., 2008; Liu et al., 2010; Wright et al., 2010)].
Patterning of Photoreceptors Across the Human Retina
We now shift our discussion from the mechanisms that specify photoreceptor subtypes to their patterning in the human retina. Foundational work, particularly in the 1980s through the 2000s, informed our current knowledge of the spatiotemporal patterning of human photoreceptors during development. Rods outnumber cones 20:1 across the retina, but this ratio varies in different regions, with 1:1 near the center and 30:1 in the periphery (Curcio et al., 1990). The human retina is patterned into discrete, concentric regions, each with unique compositions of photoreceptor subtypes at distinct densities. Additionally, there are some local patterns of photoreceptor patterning. We first discuss local patterning, then move on to photoreceptor patterning in concentric regions, and finally discuss axial patterning in the nasal/temporal and superior/inferior axes.
Local Patterning
The adult human retina has local patterning of rods and cones. Cones are hexagonally patterned near the central fovea, and as eccentricity increases, rod populations increase and disorder the cone hexagonal pattern (Sawides et al., 2017). Rod density varies with distance from the central fovea. Around 500 μm from the fovea, rods begin to encircle cone cells (Curcio et al., 1990).
Local patterning also occurs for cone subtypes. At a distance of ∼0.5 mm from the fovea, S cones are randomly patterned (Roorda and Williams, 1999; Roorda et al., 2001). At an eccentricity of 3 mm, however, the S cone distribution becomes more regular (Curcio et al., 1991; Bumsted and Hendrickson, 1999; Martin et al., 2000). The local arrangement of M and L cones is proposed to be random in the human retina (Roorda and Williams, 1999; Otake et al., 2000; Roorda et al., 2001; Bowmaker et al., 2003), based on studies using microspectrophotometry and retinal densitometry combined with adaptive optics (Mollon and Bowmaker, 1992). However, other analysis suggests nonrandom clumping of M cones, supporting elements of regulated patterning (Roorda et al., 2001).
Concentric Patterning
The patterning of photoreceptors across the human retina can be divided into several concentric rings with distinct photoreceptor constituencies and morphologies. From the periphery to the center, the retina contains the peripheral rim, posterior pole, macula, fovea, and foveola (Figure 5). Here, we discuss the differences in photoreceptor patterning and function in these regions:
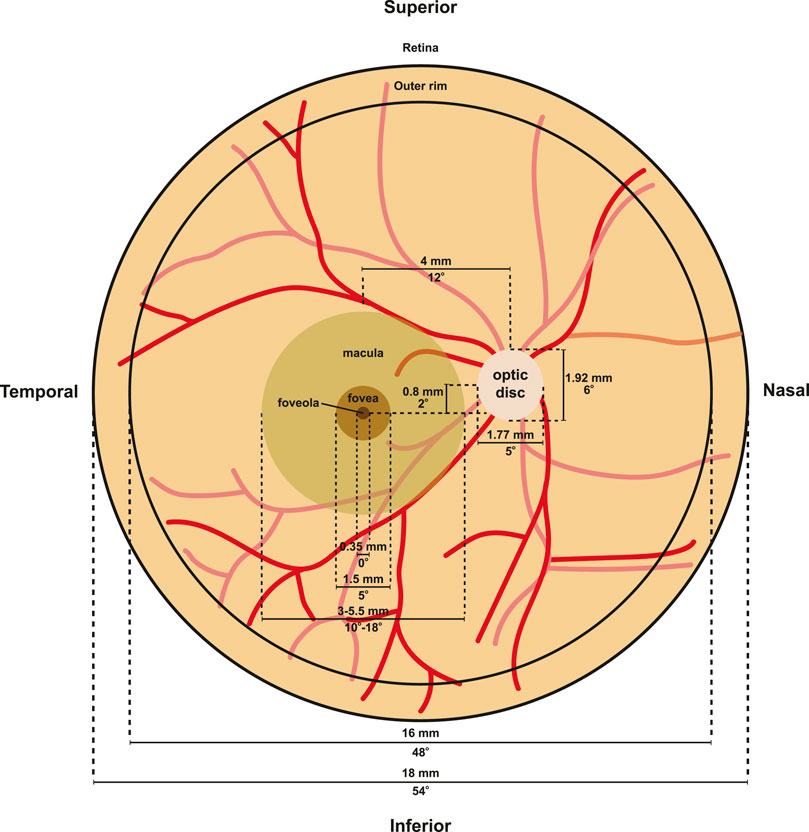
FIGURE 5. Retina morphology. Flat mount diagram of a human retina. Optic disc measurements were derived from (Arora et al., 2015), while macula, fovea, foveola, and outer rim measurements from (Schultze, 1866; Polyak, 1941; Curcio et al., 1990; Curcio et al., 1991; Hendrickson, 1992). Red lines indicate vasculature.
Periphery and Outer Rim
The peripheral retina makes up the majority of the retina (Figure 5). At eccentricities from 5 to 16 mm, cones decrease in density, reaching a low of about 2000 cones/mm2 (Farber et al., 1985; Curcio et al., 1990; Jonas et al., 1992). Rods, in contrast, increase to around 6,500 rods/mm2 outside the macula from 5 to 8 mm, and then decrease to 58,000 rods/mm2 from 8–16 mm (Curcio et al., 1990; Jonas et al., 1992). At the edge of the retina at 16–18 mm from the fovea center, cone density increases to 5,000 to 7,000 cones/mm2 (Curcio et al., 1990). At the outer rim, a 1 mm band surrounding the edge of the retina, cone density increases three-fold and rod density decreases 10-fold (Williams, 1991).
Peak rod density in the periphery appears important for low-light vision and motion detection. Though the periphery is over 90% of the retina, it does not play a substantial role in high-acuity daytime vision (Curcio et al., 1990; Quinn et al., 2019). The functional role of the cone-rich outer rim is poorly understood.
The Macula
Collectively known as the macula, the central retina is responsible for high acuity vision and perception of color in humans (Figure 5). Visual acuity is much lower in the peripheral macula compared to the central macula (fovea). The macula is 3–5.5 mm in diameter and is located approximately 4.7 mm temporal from the optic nerve (Jonas et al., 2015). The macula is characterized by a yellowish hue known as the macular pigment (Delori et al., 2001). The macular pigment contains a number of carotenoid pigment molecules including zeaxanthin, meso-zeaxanthin, and lutein (Bone et al., 1985), which limits chromatic blur to increase visual acuity (Reading and Weale, 1974) and protects the macula from photodegradation by short wavelength light (Kirschfeld, 1982).
Despite making up just 4% of the retinal surface area, the macula contains a majority of the cones and RGCs in the retina (Curcio and Allen, 1990). All three subtypes of cones are present at high density within the macula. From the central rod-free foveola, rods are introduced into this mosaic (Curcio et al., 1991). Rods quickly outnumber cones in the macula, reaching a ratio of 4:1 at approximately 0.66 mm from the center of the foveola (Curcio et al., 1990), and ending at a ratio of 8:1 throughout the macula (Curcio et al., 1990). In addition, the macula contains the largest portion of RGCs in the retina. The central retinal RGCs project to a larger proportion of the visual cortex compared to the RGCS of the peripheral retina (Tootell et al., 1988; Curcio et al., 1990).
The macula is particularly susceptible to degeneration later in life. This could be related to the high rate of metabolic stress in the region as a result of the high density of photoreceptors and retinal ganglion cells. In addition to the roles that Müller glia play in protecting the fovea from blue light and acting as optical fibers, they likely also protect the neurons, including the photoreceptors, of the retina from oxidative stress, along with supporting neuronal homeostasis and survival. Compared to peripheral Müller glia, macular Müller glia show increased expression of phosphoglycerate dehydrogenase, an important enzyme in the serine synthesis pathway (Zhang et al., 2019). When phosphoglycerate dehydrogenase was inhibited in cultured macular Müller glia, they were more susceptible to oxidative stress (Zhang et al., 2019). Additionally, Müller glia have recently been shown in mice to play a role in serine biosynthesis, which can prevent photoreceptor degeneration observed in phosphoglycerate dehydrogenase deficiencies (Shen et al., 2021). These experiments suggest that Müller glia play a critical role in the health and maintenance of the photoreceptors of the human macula.
The Fovea
The fovea is the specialized region at the center of the macula that limits light scattering and increases visual acuity (Figure 5). The fovea is located approximately 4 mm temporal and 0.8 mm inferior to the optic nerve head (Hogan et al., 1971). The fovea is about 1.0–1.5 mm wide and 200–240 μm deep, which is approximately half the thickness of the surrounding retina (Polyak, 1941), though these numbers can vary between individuals (Figure 5).
The high acuity of the fovea, including the central foveola, is enabled by the high cone density, the ratio of cones to bipolar cells to RGCs, and cortical magnification. Cortical magnification describes the process whereby the central fovea, which makes up only 0.01% of the area of the human retina, maps to 8–10% of the visual cortex (Goldstein, 2010). In comparison, the central 5 degrees of the retina (which also encompasses the foveal pit), maps to approximately 40% of the visual cortex (Tootell et al., 1988; Curcio et al., 1990). To utilize these visual adaptations, the human eyes are constantly moving such that the binocular foveas are focused on the object of interest (Goldberg, 2014).
Foveal L, M, and S cones are dense and morphologically distinct. In the fovea, cones have smaller inner and outer segment diameters compared to cones in the rest of the retina (Farber et al., 1985), Cones have an inner segment diameter of 1.6–2.2 μm in the fovea (Curcio et al., 1990) versus 6–8 μm in the periphery (Liu et al., 2018). Cones in the fovea are tightly packed in a triangular lattice.
The fovea contains the highest density of the ∼4 to 5 million cones in the retina in only 0.02% of the area and a large proportion of the total retinal ganglion cells (Curcio and Allen, 1990; Curcio et al., 1990). The peak cone density in the central fovea is ∼200,000 cones/mm2, but can range from 100,000 to 325,000 cones/mm2 (Curcio et al., 1990). This density decreases by an order of magnitude to 20,000 cones/mm2 towards the edge of the fovea at 1 mm from the central foveola. This decrease is more significant along the vertical axis than the horizontal axis, consistent with the presence of a cone streak along the horizontal meridian (Packer et al., 1989; Curcio et al., 1990). In contrast, rods appear at 100–200 μm from the foveal center and S cones peak at 15% of the total cone ratio at 200–300 μm (de Monasterio et al., 1985; Szel et al., 1988; Curcio et al., 1991; Cornish et al., 2004). Throughout the rest of the retina, S cones are present at 8–10% of the cone population. Rod density increases until rods and cones are equal in number at 400–500 μm from the foveal center. As rods enter the mosaic, cones lose their triangular lattice packing.
In addition to these photoreceptor patterns, several morphological features enhance visual acuity in the human fovea. The fovea is concaviclivate, or dish-shaped, with displacement of the majority of the retinal layers except for the cone photoreceptors (Figure 1). This displacement of inner retinal layers and absence of vasculature limits light scattering and enhances photon absorption efficiency by the outer segments of photoreceptors (Walls, 1937; Polyak et al., 1957). Chromatic aberration is also reduced by Müller glia, which act as fibers to guide light to the cones in the fovea (Franze et al., 2007). The physical shape of the foveal pit places Müller glia on top of cones, forming a cone of Müller glia on top of the foveal cone cells in the space normally occupied by the inner retinal layers (Yamada, 1969). Patients with foveal hypoplasia, a disease in which pit formation does not occur, present with reduced visual acuity, suggesting a role in pit formation for proper visual acuity in humans (Thomas et al., 2011; Wilk et al., 2014; McCafferty et al., 2015).
Differences in retinal circuitry also enhance visual acuity in the fovea. In the fovea, cones signal via elongated axons (i.e. fibers of Henle) to centrifugally displaced bipolar cells at the walls of the fovea. A single foveal M or L cone signals to two midget bipolar cells (an ON and an OFF). This signal is then transmitted to a corresponding ON or OFF midget ganglion cell such that each RGC receives input from one M or L cone, forming a “private” line (Kolb and Marshak, 2003). In the fovea, the ratio of cones to RGCs is 1:2 or 1:1 (Wassle et al., 1989; Curcio et al., 1990) consistent with a midget system of 1 cone: 2 bipolar cells: 2 RGCs (Drasdo et al., 2007). However, some RGC types may receive information from more than one cone, consistent with a measurement of 3.34 RGCs per 1 cone in the fovea (Wassle et al., 1989). The midget circuit is best characterized for M/L cones, though an S cone specific pathway has been identified in macaque (Klug et al., 2003; Patterson et al., 2019). Midget circuitry contrasts between the fovea and peripheral retina. In the peripheral retina, midget ganglion cells receive input from many more bipolar cells, and each bipolar cell receives input from multiple rod or cone photoreceptors, resulting in information from as many as 10–30 cones (Sinha et al., 2017). These midget systems allow for increased visual acuity by reducing noise as each photoreceptor has almost a direct line to an individual RGC, which transfers the information to a large visual processing system in the brain.
The Foveola
At the central base of the foveal pit lies the foveola (Figure 5), an elliptical region with an average size of 0.35 mm in horizontal diameter along the length of the ellipse (Schultze, 1866; Polyak, 1941; Curcio et al., 1990; Curcio et al., 1991; Hendrickson, 1992) and a thickness of only 100 μm (Yamada, 1969; Burris et al., 2002) compared to over 300 μm in the thickest regions at the foveal edge (Myers et al., 2015). The foveola contains the highest density of photoreceptors in the retina, ranging from 50,000 cones/mm2 (Osterberg, 1935; Farber et al., 1985; Ahnelt et al., 1987) to 180,000 cones/mm2 (Jonas et al., 1992), suggesting a high degree of variability in foveal cone density. Changes and variability in foveola cone densities are not surprising as loss of photoreceptors within the macula region occurs with aging (Gartner and Henkind, 1981).
The foveola contains M and L cones, no S cones, and no rods (Willmer, 1944; Willmer and Wright, 1945; Wald, 1967; Williams et al., 1981). Less is known about the ratio of M to L cones in the fovea, due to the similarity between these opsin subtypes and the challenges in distinguishing them. Psychophysics experiments suggest that the ratio of L to M cones is 2:1 in the fovea and foveola (Cicerone and Nerger, 1989). Direct measurements of the human retina using adaptive optics suggests that the proportion can vary greatly (Roorda and Williams, 1999).
A zone 365 μm in diameter with few to no S cones is first observed at around fetal week 15 (Xiao and Hendrickson, 2000). In adults, a range of sizes for the S cone-free region has been described, from 35–40 μm (Wald, 1967; Nork et al., 1990) (Williams et al., 1981; Curcio et al., 1991) to 300 μm (König, 1894). The size of the S cone-free zone may vary between individuals, with sparse S cone patterning within the region in some individuals, but the techniques used to determine the S cone-free region may not be able to resolve smaller regions (Williams et al., 1981). Consistent with the possible patterning of S cones in the foveola, Ahnelt and colleagues identified 3–5% of foveal cones as S cones based on morphology (Ahnelt et al., 1987).
Like the S cone-free zone, the rod-free zone in the foveola and fovea appears to change during development. The rod-free zone decreases from over 1,600 μm in diameter at 22 weeks of gestation to 650 μm at 4 years of age (Yuodelis and Hendrickson, 1986). The final diameter of the rod free zone varies postnatally, ranging from 350 to 720 μm (Yuodelis and Hendrickson, 1986; Curcio et al., 1990). Together, these observations suggest that the S cone-free and rod-free zones of the foveola develop dynamically and are likely somewhat variable across the population.
To achieve high visual acuity, a large number of neurons are needed to transmit the signals from the many photoreceptors in the foveola. High densities of photoreceptors can connect at ratios near 1:2:2 to bipolar cells and retinal ganglion cells to signal visual information to the brain, but this can result in a local increase in retinal thickness, which is detrimental to visual acuity. The foveola is designed to simultaneously allow for an increase in cell density and direct excitation of photoreceptors by photons of light to enable increased visual acuity by decreasing light scatter through centrifugal displacement of downstream neurons (Walls, 1937; Polyak et al., 1957). In this way, light can directly hit the photoreceptors because the connecting neurons are pushed aside, generating the foveal pit. This displacement to limit light scattering along with the midget circuitry that allows for a near 1:1 relationship between photoreceptors and downstream targets, provides the human foveola with high acuity vision (Polyak, 1941; Jusuf et al., 2006).
Axial Patterning
The human retina has unique patterning of photoreceptors along the nasal-temporal and superior-inferior axes. Humans have a streak of high cone density found along the nasal-temporal axis at the horizontal meridian of the retina (Curcio et al., 1990) (Figure 6A). The nasal retina has up to 45% higher cone density compared to the temporal retina (Curcio et al., 1990).
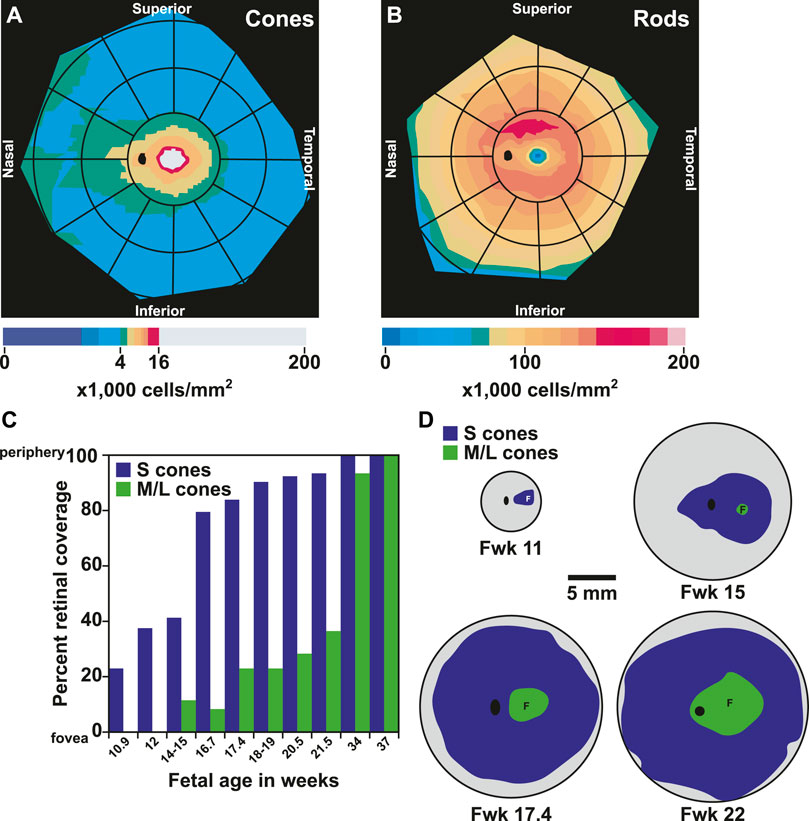
FIGURE 6. Patterning and migration of cones during development. (A) Heat map of cone density modeled from (Curcio et al., 1990). Cones range from 0 to 16,000 cells/mm2 at intervals of 1,000 cells/mm2. Densities above 16,000 cells/mm2 are represented by gray. (B) Heat map of rod density modeled from (Curcio et al., 1990). Rods range from 0 to 200,000 cells/mm2 at intervals of 12,500 cells/mm2 (C) Graph of fetal age and onset of S and M/L cones by percent retinal coverage, adapted from (Xiao and Hendrickson, 2000). (D) S and M/L specification by onset of opsin protein during fetal retinal development modeled from (Xiao and Hendrickson, 2000). Gray area is growing retinal tissue representing retinal progenitors. Fwk = fetal week. F = fovea center, black oval = optic disc.
In contrast to cone density, which is greatest in the central retina, rod density is greater and rod diameter is smaller in the temporal retina 5 mm from the center (Curcio et al., 1990). At 8 and 16 mm from the center, rods are less numerous and larger in the temporal retina compared to the nasal retina (Curcio et al., 1990) (Figure 6B). Along the superior-inferior axis, rods increase to 160,000–190,000 rods per mm2 in a hotspot in the superior retina. This hotspot occurs in an ovular ring at a similar distance from the foveal center as the optic disc (Curcio et al., 1991), and currently the purpose of the rod hotspot is not known. Rod density is lowest in the foveola as it passes the cone-rich horizontal meridian (Curcio et al., 1990). This scarcity of rods allows for the increased presence of cone cells to mediate visual acuity and color perception.
Photoreceptor Patterning and Foveal Formation During Development
As we have described, the human retina is made up of many distinct, concentric regions comprised of unique populations and densities of photoreceptor subtypes. Development of this pattern of photoreceptor topography occurs in a sequence of waves of differentiation beginning at the foveola and spreading out into the peripheral retina over gestational time. Though cone differentiation begins in the fovea, this region is the last to fully develop. Pit formation and complete displacement of the inner retinal layers is not complete until 1 or 2 years of age. Additionally, foveal cone inner and outer segments continue to grow and the cones increase in density until around the age of 10, at which point this region resembles the adult (Hendrickson, 1992).
Photoreceptor differentiation starts at the foveola with the generation of cones. The differentiation of cones and then rods spreads out to the periphery (La Vail et al., 1991; Xiao and Hendrickson, 2000). S cones are specified first, followed by M/L cones, and finally by rods (Figures 6C,D) (La Vail et al., 1991; Xiao and Hendrickson, 2000). Some rods do not yet express rhodopsin at birth, suggesting that the process of maturation could continue postnatally (Hendrickson et al., 2008).
At 10–12 weeks of gestation, the first S opsin-expressing cones are observed in the presumptive fovea in the central retina (Hendrickson, 1992). After differentiation, cones form synapses in the fovea (Yuodelis and Hendrickson, 1986). By the 14th week of gestation, cell division has ceased in the fovea (Provis et al., 1985) and the wave of cones has reached the optic disc (Xiao and Hendrickson, 2000).
Rods are first observed as NRL-expressing cells around the fovea at fetal week 10.5–12 (Xiao and Hendrickson, 2000; Hendrickson et al., 2008), and express rhodopsin at fetal week 15 (Hendrickson et al., 2008). While the adult fovea is rod-free, the presumptive fovea in the fetal retina contains NRL- and/or rhodopsin-expressing photoreceptors that are lost by fetal week 20, suggesting that these immature rods are in some way eliminated from the fovea (Bumsted et al., 1997; Bumsted and Hendrickson, 1999; Xiao and Hendrickson, 2000; Hendrickson et al., 2008).
Foveal development appears to involve two series of neuronal migrations. The first migration occurs from fetal week 24 until 15 months after birth. During this time, bipolar cells, horizontal cells, amacrine cells, and RGCs migrate peripherally, promoting pit formation (Yuodelis and Hendrickson, 1986). In addition to this displacement, central migration of cones into the foveola increases packing. As these cones migrate centrally, they become narrower and more elongated, which allows for increased density. These processes may promote displacement of rods (Hendrickson and Yuodelis, 1984; Yuodelis and Hendrickson, 1986). Migration can be visualized through the fibers of Henle, the long cone axons that form synaptic connections with their partner bipolar cells prior to migration. These axons can reach lengths of up to 300 μm (Hollenberg and Spira, 1973; Smelser et al., 1974; Hendrickson and Kupfer, 1976). As the adult foveola contains only M and L cones, it is possible that M and L cones migrate internally past the S cones (Ahnelt et al., 1987; Curcio et al., 1991), though this seems unlikely as downstream synaptic connections have already been established and would result in tangled axons. Another possibility is that these S cones are eliminated in some yet unknown mechanism. Consistent with a role for cell migration in foveal photoreceptor patterning, the densities of cones in the fovea and rods in the region neighboring the fovea increase in the absence of local mitoses (Diaz-Araya and Provis, 1992). During these migration and packing events, the rod-free zone decreases from a diameter of 1,605 μm at 22 weeks gestation (Yuodelis and Hendrickson, 1986), to an average diameter of 350 μm by adulthood (Curcio et al., 1993).
The fovea is unique to primates among mammals, making experimental studies of development and photoreceptor patterning in this region challenging. Studies of the chicken retina, which contains a region analogous to the fovea called the high acuity area (HAA), suggest mechanisms controlling human foveal development. Similar to the human fovea, the HAA is cone-rich, rod-free, and surrounded by large populations of RGCs (da Silva and Cepko, 2017). In some avian species, HAAs have a pit, lack blood vasculature, and have a unique arrangement of interneurons known as the aster (da Silva and Cepko, 2017). Patterning of the HAA in chick depends on low retinoic acid (RA) signaling (da Silva and Cepko, 2017). The RA degrading enzymes CYP26A1 and CYP26C1 are highly expressed in the HAA, whereas the RA synthesizing enzymes RALDH1/ALDH1A1 and RALDH3/ALDH1A3 are repressed, consistent with RA degradation in this region (da Silva and Cepko, 2017). RA is sufficient to increase rods in the rod-free zone, decrease retinal ganglion cell density, and disrupt the aster in the inner nuclear layer of the high-acuity area (da Silva and Cepko, 2017). The patterning of RA regulatory enzymes is conserved in the developing human retina (da Silva and Cepko, 2017). Moreover, single cell RNA sequencing showed increased CYP26A1 expression in the human fovea compared to the peripheral retina (Peng et al., 2019; Lu et al., 2020). These findings suggest that limiting RA signaling is also important for human foveal development.
Future Directions: Human Retinal Organoids Provide a Model to Study Photoreceptor Development and Patterning
We are only beginning to understand how photoreceptors are specified in the human eye. Studies in model organisms have been critical in our understanding of retinal development, photoreceptor subtype differentiation, and retinal patterning of photoreceptors. Some mechanisms of photoreceptor differentiation, including specification of rods by NRL and cone subtypes by thyroid hormone signaling, are well-conserved between humans and other vertebrates.
The next challenges lie in understanding human-specific features of photoreceptor differentiation and patterning. The advent of human retinal organoids enables the generation of human retinal tissue in a dish using embryonic stem cells or induced pluripotent stem cells. Organoids develop on human timescales and follow the same temporal dynamics of cell type differentiation seen in the human retina, making them a powerful, emergent system to study mechanisms of human photoreceptor differentiation. First developed in 2012 by Yoshiki Sasai’s group (Nakano et al., 2012), human retinal organoids provide a tractable system to study and manipulate processes unique to the human retina. Since their development, a number of methods have emerged for generating retinal organoids from gravity aggregation to 2D/3D embryoid bodies to microfluidic chips with retinal cell types (Guy et al., 2021; Marcos et al., 2021).
Human organoids allow for genetic and pharmacological manipulations of human tissue, as well as observations of human development. Retinal organoids thus hold potential for better understanding basic human biology as well as developing therapeutics for retinal degenerative diseases and injuries. scRNA-seq studies confirmed that retinal organoids specify cell types in the same temporal order as the developing human retina (Clark et al., 2019; Cowan et al., 2020; Sridhar et al., 2020). Using this system, we showed that thyroid hormone plays an important role in the specification of S vs. M/L cones (Eldred et al., 2018) and that retinoic acid regulates the generation of M vs. L cones (Hadyniak et al., 2021). NRL null human retinal organoids display a lack of rods and an increase in S cones (Kallman et al., 2020). Recently, human retinal organoids have also been shown to be a useful model for studying cis-regulatory elements and their roles in disease and normal human development. Specifically, perturbation of an enhancer (5q14.3) involved in age-related macular degeneration among other diseases resulted in organoids that showed a delay in cell type specification and a partial loss of rods (Thomas et al., 2022). These types of mechanistic discoveries were not possible prior to the development of this in vitro system. Human retinal organoids are at the forefront of understanding human developmental biology and are sure to provide huge advances in our understanding of the human retina in the coming years.
One major challenge is that human retinal organoids do not recapitulate the concentric patterning of photoreceptors observed in humans. Additionally, human retinal organoids fail to form a fovea, preventing studies of pit formation, the mechanisms that yield high populations of M and L cones, cell displacement, and migration. One solution may lie in modulation of retinal organoid protocols to adjust the concentrations of thyroid hormone and retinoic acid, two factors that drive foveal-like cone patterning in other organisms. Additionally, tissue engineering could aid in mechanically forming a foveal-like pit in the organoid structure and introducing vascularization that enables better survivability and increased size. With larger organoids, local treatment of small molecules and hormones could be administered to create retinal organoids patterned more comparably to the adult retina. Currently, organoids remain too small to allow for such experiments, resulting in experiments that generate either more “foveal-like” or “peripheral-like” organoids, instead of organoids with a combination of specialized regions.
Non-human primates, especially rhesus macaques, provide an alternative model for retinal studies. They share similar patterning of photoreceptors as well as a high-acuity macula and fovea, and as such, are good models for understanding spatiotemporal development of photoreceptors and macular degeneration. Additionally, non-human primates are amenable to genetic manipulations including CRISPR (Kang et al., 2019), optogenetics (Chaffiol et al., 2017), adeno-associated virus (AAV) (Vandenberghe et al., 2013), and transplants (Picaud et al., 2019). This makes non-human primates a very promising model for studying primate-specific retinal features and patterns that organoids have not yet been optimized to examine.
Additional challenges come from the difficulty in differentiating M versus L cones. Recent advances in in situ technologies enabled us to differentiate between these two cone subtypes in fixed tissue at a single-cone resolution (Hadyniak et al., 2021). This new advance allows us to label cones in retinal organoids and study perturbations in development, along directly visualizing cone ratios in human samples in regions of the retina. With this new technology, we found that retinoic acid signaling regulates the M to L fate decision in retinal organoids (Hadyniak et al., 2021). Improvements to this technology will enable for visualization of M- and L-opsin expression with other genes of interest in multiple retinal cell types. These advances suggest that we will learn much more about the patterning and development of photoreceptors through experimentation in human retinal organoids.
Author Contributions
KH and SH wrote and edited the manuscript. RJ edited the manuscript.
Funding
KH was supported by NSF DGE-1746891. SH was supported by 5F31EY029157-02. RJ was supported by National Eye Institute R01EY030872.
Conflict of Interest
The authors declare that the research was conducted in the absence of any commercial or financial relationships that could be construed as a potential conflict of interest.
Publisher’s Note
All claims expressed in this article are solely those of the authors and do not necessarily represent those of their affiliated organizations, or those of the publisher, the editors and the reviewers. Any product that may be evaluated in this article, or claim that may be made by its manufacturer, is not guaranteed or endorsed by the publisher.
Acknowledgments
We thank members of the Johnston lab for helpful feedback on the manuscript.
References
Agte, S., Junek, S., Matthias, S., Ulbricht, E., Erdmann, I., Wurm, A., et al. (2011). Müller Glial Cell-Provided Cellular Light Guidance through the Vital Guinea-Pig Retina. Biophysical J. 101 (11), 2611–2619. doi:10.1016/j.bpj.2011.09.062
Agte, S., Savvinov, A., Karl, A., Zayas-Santiago, A., Ulbricht, E., Makarov, V. I., et al. (2018). Müller Glial Cells Contribute to Dim Light Vision in the Spectacled caiman ( Caiman crocodilus Fuscus ): Analysis of Retinal Light Transmission. Exp. Eye Res. 173, 91–108. doi:10.1016/j.exer.2018.05.009
Ahnelt, P., Keri, C., and Kolb, H. (1990). Identification of Pedicles of Putative Blue-Sensitive Cones in the Human Retina. J. Comp. Neurol. 293 (1), 39–53. doi:10.1002/cne.902930104
Ahnelt, P. K., Kolb, H., and Pflug, R. (1987). Identification of a Subtype of Cone Photoreceptor, Likely to Be Blue Sensitive, in the Human Retina. J. Comp. Neurol. 255 (1), 18–34. doi:10.1002/cne.902550103
Akimoto, M., Cheng, H., Zhu, D., Brzezinski, J. A., Khanna, R., Filippova, E., et al. (2006). Targeting of GFP to Newborn Rods by Nrl Promoter and Temporal Expression Profiling of Flow-Sorted Photoreceptors. Proc. Natl. Acad. Sci. U.S.A. 103 (10), 3890–3895. doi:10.1073/pnas.0508214103
Alpern, M., Lee, G. B., Maaseidvaag, F., and Miller, S. S. (1971). Colour Vision in Blue-Cone 'monochromacy'. J. Physiol. 212 (1), 211–233. doi:10.1113/jphysiol.1971.sp009318
Alpern, M., Lee, G. B., and Spivey, B. E. (1965). 1 Cone Monochromatism. Arch. Ophthalmol. 74, 334–337. doi:10.1001/archopht.1965.00970040336008
Alpern, M., and Pugh, E. N. (1974). The Density and Photosensitivity of Human Rhodopsin in the Living Retina. J. Physiol. 237 (2), 341–370. doi:10.1113/jphysiol.1974.sp010485
Altshuler, D., Lo Turco, J. J., Rush, J., and Cepko, C. (1993). Taurine Promotes the Differentiation of a Vertebrate Retinal Cell Type In Vitro. Development 119 (4), 1317–1328. doi:10.1242/dev.119.4.1317
Applebury, M. L., Farhangfar, F., Glösmann, M., Hashimoto, K., Kage, K., Robbins, J. T., et al. (2007). Transient Expression of Thyroid Hormone Nuclear Receptor TRβ2 Sets S Opsin Patterning during Cone Photoreceptor Genesis. Dev. Dyn. 236 (5), 1203–1212. doi:10.1002/dvdy.21155
Arora, S., Chung, H., and Damji, K. (2015). Optic Disk Size Assessment Techniques: Photo Essay. Int. J. Opthalmol Clin. Res. 2, 1. doi:10.23937/2378-346x/1410009
Baehr, W., Wu, S. M., Bird, A. C., and Palczewski, K. (2003). The Retinoid Cycle and Retina Disease. Vis. Res. 43 (28), 2957–2958. doi:10.1016/j.visres.2003.10.001
Bagci, E., Heijlen, M., Vergauwen, L., Hagenaars, A., Houbrechts, A. M., Esguerra, C. V., et al. (2015). Deiodinase Knockdown during Early Zebrafish Development Affects Growth, Development, Energy Metabolism, Motility and Phototransduction. PLoS One 10 (4), e0123285. doi:10.1371/journal.pone.0123285
Baylor, D. A., Lamb, T. D., and Yau, K. W. (1979). Responses of Retinal Rods to Single Photons. J. Physiol. 288, 613–634.
Blackwell, H. R., and Blackwell, O. M. (1961). Rod and Cone Receptor Mechanisms in Typical and Atypical Congenital Achromatopsia. Vis. Res. 1 (1), 62–107. doi:10.1016/0042-6989(61)90022-0
Bone, R. A., Landrum, J. T., and Tarsis, S. L. (1985). Preliminary Identification of the Human Macular Pigment. Vis. Res. 25 (11), 1531–1535. doi:10.1016/0042-6989(85)90123-3
Bowmaker, J. K., Dartnall, H. J., and Mollon, J. D. (1980). Microspectrophotometric Demonstration of Four Classes of Photoreceptor in an Old World Primate, Macaca fascicularis. J. Physiol. 298, 131–143. doi:10.1113/jphysiol.1980.sp013071
Bowmaker, J. K., Parry, J. W. L., and Mollon, J. D. (2003). The Arrangement of Land M Cones in Human and a Primate Retina. Normal defective colour Vis., 39–50. doi:10.1093/acprof:oso/9780198525301.003.0005
Brainard, D. H., Calderone, J. B., Nugent, A. K., and Jacobs, G. H. (1999). Flicker ERG Responses to Stimuli Parametrically Modulated in Color Space. Invest. Ophthalmol. Vis. Sci. 40 (12), 2840–2847.
Brzezinski, J. A., Lamba, D. A., and Reh, T. A. (2010). Blimp1 Controls Photoreceptor versus Bipolar Cell Fate Choice during Retinal Development. Development 137 (4), 619–629. doi:10.1242/dev.043968
Bumsted, K., and Hendrickson, A. (1999). Distribution and Development of Short-Wavelength Cones Differ betweenMacaca Monkey and Human Fovea. J. Comp. Neurol. 403 (4), 502–516. doi:10.1002/(sici)1096-9861(19990125)403:4<502::aid-cne6>3.0.co;2-n
Bumsted, K., Jasoni, C., Szél, A., and Hendrickson, A. (1997). Spatial and Temporal Expression of Cone Opsins during Monkey Retinal Development. J. Comp. Neurol. 378 (1), 117–134. doi:10.1002/(sici)1096-9861(19970203)378:1<117::aid-cne7>3.0.co;2-7
Burris, C., Klug, K., Ngo, I. T., Sterling, P., and Schein, S. (2002). How Müller Glial Cells in Macaque Fovea Coat and Isolate the Synaptic Terminals of Cone Photoreceptors. J. Comp. Neurol. 453 (1), 100–111. doi:10.1002/cne.10397
Carroll, J., Neitz, J., and Neitz, M. (2002). Estimates of L:M Cone Ratio from ERG Flicker Photometry and Genetics. J. Vis. 2 (8), 1. doi:10.1167/2.8.1
Carss, K. J., Arno, G., Erwood, M., Stephens, J., Sanchis-Juan, A., Hull, S., et al. (2017). Comprehensive Rare Variant Analysis via Whole-Genome Sequencing to Determine the Molecular Pathology of Inherited Retinal Disease. Am. J. Hum. Genet. 100 (1), 75–90. doi:10.1016/j.ajhg.2016.12.003
Carter-Dawson, L. D., and LaVail, M. M. (1979). Rods and Cones in the Mouse Retina. II. Autoradiographic Analysis of Cell Generation Using Tritiated Thymidine. J. Comp. Neurol. 188 (2), 263–272. doi:10.1002/cne.901880205
Cepko, C. (2014). Intrinsically Different Retinal Progenitor Cells Produce Specific Types of Progeny. Nat. Rev. Neurosci. 15 (9), 615–627. doi:10.1038/nrn3767
Chaffiol, A., Caplette, R., Jaillard, C., Brazhnikova, E., Desrosiers, M., Dubus, E., et al. (2017). A New Promoter Allows Optogenetic Vision Restoration with Enhanced Sensitivity in Macaque Retina. Mol. Ther. 25 (11), 2546–2560. doi:10.1016/j.ymthe.2017.07.011
Chen, J., Rattner, A., and Nathans, J. (2005). The rod photoreceptor-specific nuclear receptor Nr2e3 represses transcription of multiple cone-specific genes. J. Neurosci. 25 (1), 118–129. doi:10.1523/JNEUROSCI.3571-04.2005
Chen, S., Wang, Q.-L., Nie, Z., Sun, H., Lennon, G., Copeland, N. G., et al. (1997). Crx, a Novel Otx-like Paired-Homeodomain Protein, Binds to and Transactivates Photoreceptor Cell-specific Genes. Neuron 19 (5), 1017–1030. doi:10.1016/s0896-6273(00)80394-3
Cheng, H., Aleman, T. S., Cideciyan, A. V., Khanna, R., Jacobson, S. G., and Swaroop, A. (2006). In Vivo function of the Orphan Nuclear Receptor NR2E3 in Establishing Photoreceptor Identity during Mammalian Retinal Development. Hum. Mol. Genet. 15 (17), 2588–2602. doi:10.1093/hmg/ddl185
Cheng, H., Khanna, H., Oh, E. C., Hicks, D., Mitton, K. P., and Swaroop, A. (2004). Photoreceptor-specific Nuclear Receptor NR2E3 Functions as a Transcriptional Activator in Rod Photoreceptors. Hum. Mol. Genet. 13 (15), 1563–1575. doi:10.1093/hmg/ddh173
Chiu, M. I., and Nathans, J. (1994). A Sequence Upstream of the Mouse Blue Visual Pigment Gene Directs Blue Cone-specific Transgene Expression in Mouse Retinas. Vis. Neurosci. 11 (4), 773–780. doi:10.1017/s0952523800003072
Cicerone, C. M., and Nerger, J. L. (1989). The Relative Numbers of Long-Wavelength-Sensitive to Middle-Wavelength-Sensitive Cones in the Human Fovea Centralis. Vis. Res. 29 (1), 115–128. doi:10.1016/0042-6989(89)90178-8
Clark, B. S., Stein-O’Brien, G. L., Shiau, F., Cannon, G. H., Davis-Marcisak, E., Sherman, T., et al. (2019). Single-Cell RNA-Seq Analysis of Retinal Development Identifies NFI Factors as Regulating Mitotic Exit and Late-Born Cell Specification. Neuron 102 (6), 1111–1126. doi:10.1016/j.neuron.2019.04.010
Cohen, A. I. (1970). “Further Studies on the Question of the Patency of Saccules in Outer Segments of Vertebrate Photoreceptors,” in Further Studies on the Question of the Patency of Saccules in Outer Segments of Vertebrate photoreceptorsVision Res. (Great Britain: Pergamon Press), 10, 445–IN14. doi:10.1016/0042-6989(70)90001-5Vis. Res.
Cornish, E. E., Xiao, M., Yang, Z., Provis, J. M., and Hendrickson, A. E. (2004). The Role of Opsin Expression and Apoptosis in Determination of Cone Types in Human Retina. Exp. Eye Res. 78 (6), 1143–1154. doi:10.1016/j.exer.2004.01.004
Cowan, C. S., Renner, M., De Gennaro, M., Gross-Scherf, B., Goldblum, D., Hou, Y., et al. (2020). Cell Types of the Human Retina and its Organoids at Single-Cell Resolution. Cell 182 (6), 1623–1640. e1634. doi:10.1016/j.cell.2020.08.013
Crawford, W. S. S. a. B. H. (1933). The Luminous Efficiency of Rays Entering the Eye Pupil at Different Points. Biol. Sci. 112 (778), 428–450.
Curcio, C. A., Millican, C. L., Allen, K. A., and Kalina, R. E. (1993). Aging of the Human Photoreceptor Mosaic: Evidence for Selective Vulnerability of Rods in central Retina. Invest. Ophthalmol. Vis. Sci. 34 (12), 3278–3296.
Curcio, C. A., Allen, K. A., Sloan, K. R., Lerea, C. L., Hurley, J. B., Klock, I. B., et al. (1991). Distribution and Morphology of Human Cone Photoreceptors Stained with Anti-blue Opsin. J. Comp. Neurol. 312 (4), 610–624. doi:10.1002/cne.903120411
Curcio, C. A., and Allen, K. A. (1990). Topography of Ganglion Cells in Human Retina. J. Comp. Neurol. 300 (1), 5–25. doi:10.1002/cne.903000103
Curcio, C. A., Sloan, K. R., Kalina, R. E., and Hendrickson, A. E. (1990). Human Photoreceptor Topography. J. Comp. Neurol. 292 (4), 497–523. doi:10.1002/cne.902920402
da Silva, S., and Cepko, C. L. (2017). Fgf8 Expression and Degradation of Retinoic Acid Are Required for Patterning a High-Acuity Area in the Retina. Dev. Cel 42 (1), 68–81. e66. doi:10.1016/j.devcel.2017.05.024
Dartnall, H. J., Bowmaker, J. K., and Mollon, J. D. (1983). Human Visual Pigments: Microspectrophotometric Results from the Eyes of Seven Persons. Proc. R. Soc. Lond. B. 220 (1218), 115–130. doi:10.1098/rspb.1983.0091
Daw, N. W., and Enoch, J. M. (1973). Contrast Sensitivity, Westheimer Function and Stiles-Crawford Effect in a Blue Cone Monochromat. Vis. Res. 13 (9), 1669–1680. doi:10.1016/0042-6989(73)90086-2
de Melo, J., Peng, G.-H., Chen, S., and Blackshaw, S. (2011). The Spalt Family Transcription Factor Sall3 Regulates the Development of Cone Photoreceptors and Retinal Horizontal Interneurons. Development 138 (11), 2325–2336. doi:10.1242/dev.061846
de Monasterio, F. M., McCrane, E. P., Newlander, J. K., and Schein, S. J. (1985). Density Profile of Blue-Sensitive Cones along the Horizontal meridian of Macaque Retina. Invest. Ophthalmol. Vis. Sci. 26 (3), 289–302.
Deeb, S. S., Diller, L. C., Williams, D. R., and Dacey, D. M. (2000). Interindividual and Topographical Variation of L:M Cone Ratios in Monkey Retinas. J. Opt. Soc. Am. A. 17 (3), 538–544. doi:10.1364/josaa.17.000538
Delori, F. C., Goger, D. G., Hammond, B. R., Snodderly, D. M., and Burns, S. A. (2001). Macular Pigment Density Measured by Autofluorescence Spectrometry: Comparison with Reflectometry and Heterochromatic Flicker Photometry. J. Opt. Soc. Am. A. 18 (6), 1212–1230. doi:10.1364/josaa.18.001212
Dentice, M., Marsili, A., Zavacki, A., Larsen, P. R., and Salvatore, D. (2013). The Deiodinases and the Control of Intracellular Thyroid Hormone Signaling during Cellular Differentiation. Biochim. Biophys. Acta (Bba) - Gen. Subjects 1830 (7), 3937–3945. doi:10.1016/j.bbagen.2012.05.007
Diaz Araya, C., and Provis, J. M. (1992). Evidence of Photoreceptor Migration during Early Foveal Development: a Quantitative Analysis of Human Fetal Retinae. Vis. Neurosci. 8 (6), 505–514. doi:10.1017/s0952523800005605
Dorval, K. M., Bobechko, B. P., Fujieda, H., Chen, S., Zack, D. J., and Bremner, R. (2006). CHX10 Targets a Subset of Photoreceptor Genes. J. Biol. Chem. 281 (2), 744–751. doi:10.1074/jbc.M509470200
Dowdeswell, H. J., Slater, A. M., Broomhall, J., and Tripp, J. (1995). Visual Deficits in Children Born at Less Than 32 Weeks' Gestation with and without Major Ocular Pathology and Cerebral Damage. Br. J. Ophthalmol. 79 (5), 447–452. doi:10.1136/bjo.79.5.447
Drasdo, N., Millican, C. L., Katholi, C. R., and Curcio, C. A. (2007). The Length of Henle Fibers in the Human Retina and a Model of Ganglion Receptive Field Density in the Visual Field. Vis. Res. 47 (22), 2901–2911. doi:10.1016/j.visres.2007.01.007
Drummond-Borg, M., Deeb, S. S., and Motulsky, A. G. (1989). Molecular Patterns of X Chromosome-Linked Color Vision Genes Among 134 Men of European Ancestry. Proc. Natl. Acad. Sci. U.S.A. 86 (3), 983–987. doi:10.1073/pnas.86.3.983
Dumitrescu, A. M., Liao, X.-H., Best, T. B., Brockmann, K., and Refetoff, S. (2004). A Novel Syndrome Combining Thyroid and Neurological Abnormalities Is Associated with Mutations in a Monocarboxylate Transporter Gene. Am. J. Hum. Genet. 74 (1), 168–175. doi:10.1086/380999
Eldred, K. C., Avelis, C., Johnston, R. J., and Roberts, E. (2020). Modeling Binary and Graded Cone Cell Fate Patterning in the Mouse Retina. Plos Comput. Biol. 16 (3), e1007691. doi:10.1371/journal.pcbi.1007691
Eldred, K. C., Hadyniak, S. E., Hussey, K. A., Brenerman, B., Zhang, P.-W., Chamling, X., et al. (2018). Thyroid Hormone Signaling Specifies Cone Subtypes in Human Retinal Organoids. Science 362 (6411). doi:10.1126/science.aau6348
Emerson, M. M., Surzenko, N., Goetz, J. J., Trimarchi, J., and Cepko, C. L. (2013). Otx2 and Onecut1 Promote the Fates of Cone Photoreceptors and Horizontal Cells and Repress Rod Photoreceptors. Dev. Cel 26 (1), 59–72. doi:10.1016/j.devcel.2013.06.005
Enoch, J. M. (1972). Retinal Receptor Orientation and the Role of Fiber Optics in Vision. Optom. Vis. Sci. 49 (6), 455–470. doi:10.1097/00006324-197206000-00001
Farber, D. B., Flannery, J. G., Lolley, R. N., and Bok, D. (1985). Distribution Patterns of Photoreceptors, Protein, and Cyclic Nucleotides in the Human Retina. Invest. Ophthalmol. Vis. Sci. 26 (11), 1558–1568.
Feil, R., Aubourg, P., Heilig, R., and Mandel, J. L. (1990). A 195-kb Cosmid Walk Encompassing the Human Xq28 Color Vision Pigment Genes. Genomics 6 (2), 367–373. doi:10.1016/0888-7543(90)90578-i
Finnemann, S. C. (2003). Focal Adhesion Kinase Signaling Promotes Phagocytosis of Integrin-Bound Photoreceptors. EMBO J. 22 (16), 4143–4154. doi:10.1093/emboj/cdg416
Fleischman, J. A., and O'Donnell, F. E. (1981). Congenital X-Linked Incomplete Achromatopsia. Arch. Ophthalmol. 99 (3), 468–472. doi:10.1001/archopht.1981.03930010470016
Franze, K., Grosche, J., Skatchkov, S. N., Schinkinger, S., Foja, C., Schild, D., et al. (2007). Müller Cells Are Living Optical Fibers in the Vertebrate Retina. Proc. Natl. Acad. Sci. U.S.A. 104 (20), 8287–8292. doi:10.1073/pnas.0611180104
Freund, C. L., Gregory-Evans, C. Y., Furukawa, T., Papaioannou, M., Looser, J., Ploder, L., et al. (1997). Cone-Rod Dystrophy Due to Mutations in a Novel Photoreceptor-specific Homeobox Gene () Essential for Maintenance of the Photoreceptor. Cell 91 (4), 543–553. doi:10.1016/s0092-8674(00)80440-7
Friesema, E. C., Grueters, A., Biebermann, H., Krude, H., von Moers, A., Reeser, M., et al. (2004). Association between Mutations in a Thyroid Hormone Transporter and Severe X-Linked Psychomotor Retardation. The Lancet 364 (9443), 1435–1437. doi:10.1016/S0140-6736(04)17226-7
Fu, Y., Liu, H., Ng, L., Kim, J.-W., Hao, H., Swaroop, A., et al. (2014). Feedback Induction of a Photoreceptor-specific Isoform of Retinoid-Related Orphan Nuclear Receptor β by the Rod Transcription Factor NRL. J. Biol. Chem. 289 (47), 32469–32480. doi:10.1074/jbc.M114.605774
Furukawa, T., Morrow, E. M., and Cepko, C. L. (1997). Crx, a Novel Otx-like Homeobox Gene, Shows Photoreceptor-specific Expression and Regulates Photoreceptor Differentiation. Cell 91 (4), 531–541. doi:10.1016/s0092-8674(00)80439-0
Gal, A., Li, Y., Thompson, D. A., Weir, J., Orth, U., Jacobson, S. G., et al. (2000). Mutations in MERTK, the Human Orthologue of the RCS Rat Retinal Dystrophy Gene, Cause Retinitis Pigmentosa. Nat. Genet. 26 (3), 270–271. doi:10.1038/81555
Gartner, S., and Henkind, P. (1981). Aging and Degeneration of the Human Macula. 1. Outer Nuclear Layer and Photoreceptors. Br. J. Ophthalmol. 65 (1), 23–28. doi:10.1136/bjo.65.1.23
Glaschke, A., Glösmann, M., and Peichl, L. (2010). Developmental Changes of Cone Opsin Expression but Not Retinal Morphology in the Hypothyroid Pax8 Knockout Mouse. Invest. Ophthalmol. Vis. Sci. 51 (3), 1719–1727. doi:10.1167/iovs.09-3592
Glaschke, A., Weiland, J., Del Turco, D., Steiner, M., Peichl, L., and Glosmann, M. (2011). Thyroid Hormone Controls Cone Opsin Expression in the Retina of Adult Rodents. J. Neurosci. 31 (13), 4844–4851. doi:10.1523/JNEUROSCI.6181-10.2011
Goldberg, M. (2014). “The Control of Gaze,” in Principles of Neural Science. Editors S. J. Kandel ER, T. M. Jessell, S. A. Siegelbaum, A. J. Hudspeth, and S. Mack. Fifth Edition (New York, NY: McGraw-Hill Education).
Grossniklaus, H. E., Geisert, E. E., and Nickerson, J. M. (2015). “Introduction to the Retina,” in Progress in Molecular Biology and Translational Science. Editors J. F. Hejtmancik, and J. M. Nickerson (Academic Press), 383–396. doi:10.1016/bs.pmbts.2015.06.001
Gunther, K. L., Neitz, J., and Neitz, M. (2008). Nucleotide Polymorphisms Upstream of the X-Chromosome Opsin Gene Array Tune L:M Cone Ratio. Vis. Neurosci. 25 (3), 265–271. doi:10.1017/S0952523808080280
Guo, C., Chen, X., Song, H., Maynard, M. A., Zhou, Y., Lobanov, A. V., et al. (2014). Intrinsic Expression of a Multiexon Type 3 Deiodinase Gene Controls Zebrafish Embryo Size. Endocrinology 155 (10), 4069–4080. doi:10.1210/en.2013-2029
Guy, B., Zhang, J. S., Duncan, L. H., and Johnston, R. J. (2021). Human Neural Organoids: Models for Developmental Neurobiology and Disease. Dev. Biol. 478, 102–121. doi:10.1016/j.ydbio.2021.06.012
Hadyniak, S. E., Eldred, K. C., Brenerman, B., Hussey, K. A., McCoy, R. C., Sauria, M. E. G., et al. (20212021). Temporal Regulation of green and Red Cone Specification in Human Retinas and Retinal Organoids. bioRxiv 2003, 2030437763. doi:10.1101/2021.03.30.437763
Hagstrom, S. A., Neitz, J., and Neitz, M. (1998). Variations in Cone Populations for Red-green Color Vision Examined by Analysis of mRNA. Neuroreport 9 (9), 1963–1967. doi:10.1097/00001756-199806220-00009
Hagstrom, S. A., Neitz, M., and Neitz, J. (2000). Cone Pigment Gene Expression in Individual Photoreceptors and the Chromatic Topography of the Retina. J. Opt. Soc. Am. A. 17 (3), 527–537. doi:10.1364/josaa.17.000527
Haider, N. B., Demarco, P., Nystuen, A. M., Huang, X., Smith, R. S., McCall, M. A., et al. (2006). The transcription factorNr2e3functions in retinal progenitors to suppress cone cell generation. Vis. Neurosci. 23 (6), 917–929. doi:10.1017/S095252380623027X
Haider, N. B., Jacobson, S. G., Cideciyan, A. V., Swiderski, R., Streb, L. M., Searby, C., et al. (2000). Mutation of a Nuclear Receptor Gene, NR2E3, Causes Enhanced S Cone Syndrome, a Disorder of Retinal Cell Fate. Nat. Genet. 24 (2), 127–131. doi:10.1038/72777
Hayashi, T., Motulsky, A. G., and Deeb, S. S. (1999). Position of a 'green-red' Hybrid Gene in the Visual Pigment Array Determines Colour-Vision Phenotype. Nat. Genet. 22 (1), 90–93. doi:10.1038/8798
Hendrickson, A., and Kupfer, C. (1976). The Histogenesis of the Fovea in the Macaque Monkey. Invest. Ophthalmol. Vis. Sci. 15 (9), 746–756.
Hendrickson, A. (1992). A Morphological Comparison of Foveal Development in Man and Monkey. Eye 6 (Pt 2), 136–144. doi:10.1038/eye.1992.29
Hendrickson, A., Bumsted-O'Brien, K., Natoli, R., Ramamurthy, V., Possin, D., and Provis, J. (2008). Rod Photoreceptor Differentiation in Fetal and Infant Human Retina. Exp. Eye Res. 87 (5), 415–426. doi:10.1016/j.exer.2008.07.016
Hendrickson, A. E., and Yuodelis, C. (1984). The Morphological Development of the Human Fovea. Ophthalmology 91 (6), 603–612. doi:10.1016/s0161-6420(84)34247-6
Hofer, H., Carroll, J., Neitz, J., Neitz, M., and Williams, D. R. (2005). Organization of the Human Trichromatic Cone Mosaic. J. Neurosci. 25 (42), 9669–9679. doi:10.1523/JNEUROSCI.2414-05.2005
Hogan, M. J., Alvarado, J. A., and Weddell, J. E. (1971). Histology of the Human Eye: An Atlas and Textbook. Saunders.
Hollenberg, M. J., and Spira, A. W. (1973). Human Retinal Development: Ultrastructure of the Outer Retina. Am. J. Anat. 137 (4), 357–385. doi:10.1002/aja.1001370402
Hood, D. C., Cideciyan, A. V., Roman, A. J., and Jacobson, S. G. (1995). Enhanced S Cone Syndrome: Evidence for an Abnormally Large Number of S Cones. Vis. Res. 35 (10), 1473–1481. doi:10.1016/0042-6989(95)98727-q
Hoshino, A., Ratnapriya, R., Brooks, M. J., Chaitankar, V., Wilken, M. S., Zhang, C., et al. (2017). Molecular Anatomy of the Developing Human Retina. Dev. Cel 43 (6), 763–779. doi:10.1016/j.devcel.2017.10.029
Housset, M., Filion, D., Cortes, N., Vali, H., Mandato, C., Casanova, C., et al. (20212021). Light-mediated Planar Polarization of Cone Photoreceptor Cilia Contributes to Visual Acuity in Mammals. bioRxiv 0421, 440771. doi:10.1101/2021.04.21.440771
Hubbard, R., and Kropf, A. (1958). The Action of Light on Rhodopsin. Proc. Natl. Acad. Sci. U.S.A. 44 (2), 130–139. doi:10.1073/pnas.44.2.130
Hyatt, G. A., Schmitt, E. A., Fadool, J. M., and Dowling, J. E. (1996). Retinoic Acid Alters Photoreceptor Development In Vivo. Proc. Natl. Acad. Sci. U.S.A. 93 (23), 13298–13303. doi:10.1073/pnas.93.23.13298
Ingram, N. T., Sampath, A. P., and Fain, G. L. (2016). Why Are Rods More Sensitive Than Cones? J. Physiol. 594 (19), 5415–5426. doi:10.1113/JP272556
Jacobs, G. H., and Deegan, J. F. (1999). Uniformity of Colour Vision in Old World Monkeys. Proc. R. Soc. Lond. B 266 (1432), 2023–2028. doi:10.1098/rspb.1999.0881
Jacobs, G. H., and Neitz, J. (1993). “Electrophysiological Estimates of Individual Variation in the L/M Cone Ratio,” in Colour Vision Deficiencies XI: Proceedings of the Eleventh Symposium of the International Research Group on Colour Vision Deficiencies, Held in Sydney, Australia 21–23 June 1991 Including the Joint IRGCVD-AIC Meeting on Mechanisms of Colour Vision 24 June 1991. Editor B. Drum (Dordrecht: Springer Netherlands), 107–112. doi:10.1007/978-94-011-1856-9_11
Jacobson, S. G., Marmor, M. F., Kemp, C. M., and Knighton, R. W. (1990). SWS (Blue) Cone Hypersensitivity in a Newly Identified Retinal Degeneration. Invest. Ophthalmol. Vis. Sci. 31 (5), 827–838.
Jacobson, S. G., Sumaroka, A., Aleman, T. S., Cideciyan, A. V., Schwartz, S. B., Roman, A. J., et al. (2004). Nuclear Receptor NR2E3 Gene Mutations Distort Human Retinal Laminar Architecture and Cause an Unusual Degeneration. Hum. Mol. Genet. 13 (17), 1893–1902. doi:10.1093/hmg/ddh198
Jadhav, A. P., Mason, H. A., and Cepko, C. L. (2006). Notch 1 Inhibits Photoreceptor Production in the Developing Mammalian Retina. Development 133 (5), 913–923. doi:10.1242/dev.02245
Javed, A., Mattar, P., Lu, S., Kruczek, K., Kloc, M., Gonzalez-Cordero, A., et al. (2020). Pou2f1 and Pou2f2 Cooperate to Control the Timing of Cone Photoreceptor Production in the Developing Mouse Retina. Development 147 (18). doi:10.1242/dev.188730
Jia, L., Oh, E. C. T., Ng, L., Srinivas, M., Brooks, M., Swaroop, A., et al. (2009). Retinoid-related Orphan Nuclear Receptor RORβ Is an Early-Acting Factor in Rod Photoreceptor Development. Proc. Natl. Acad. Sci. U.S.A. 106 (41), 17534–17539. doi:10.1073/pnas.0902425106
Jonas, J. B., Schneider, U., and Naumann, G. O. H. (1992). Count and Density of Human Retinal Photoreceptors. Graefe's Arch. Clin. Exp. Ophthalmol. 230 (6), 505–510. doi:10.1007/BF00181769
Jonas, R. A., Wang, Y. X., Yang, H., Li, J. J., Xu, L., Panda-Jonas, S., et al. (2015). Optic Disc - Fovea Distance, Axial Length and Parapapillary Zones. The Beijing Eye Study 2011. PLoS One 10 (9), e0138701. doi:10.1371/journal.pone.0138701
Jørgensen, A. L., Deeb, S. S., and Motulsky, A. G. (1990). Molecular Genetics of X Chromosome-Linked Color Vision Among Populations of African and Japanese Ancestry: High Frequency of a Shortened Red Pigment Gene Among Afro-Americans. Proc. Natl. Acad. Sci. U.S.A. 87 (17), 6512–6516. doi:10.1073/pnas.87.17.6512
Jusuf, P. R., Martin, P. R., and Grunert, U. (2006). Random Wiring in the Midget Pathway of Primate Retina. J. Neurosci. 26 (15), 3908–3917. doi:10.1523/JNEUROSCI.4891-05.2006
Kaewkhaw, R., Kaya, K. D., Brooks, M., Homma, K., Zou, J., Chaitankar, V., et al. (2015). Transcriptome Dynamics of Developing Photoreceptors in Three-Dimensional Retina Cultures Recapitulates Temporal Sequence of Human Cone and Rod Differentiation Revealing Cell Surface Markers and Gene Networks. Stem Cells 33 (12), 3504–3518. doi:10.1002/stem.2122
Kallman, A., Capowski, E. E., Wang, J., Kaushik, A. M., Jansen, A. D., Edwards, K. L., et al. (2020). Investigating Cone Photoreceptor Development Using Patient-Derived NRL Null Retinal Organoids. Commun. Biol. 3 (1), 82. doi:10.1038/s42003-020-0808-5
Kang, Y., Chu, C., Wang, F., and Niu, Y. (2019). CRISPR/Cas9-mediated Genome Editing in Nonhuman Primates. Dis. Model. Mech. 12 (10). doi:10.1242/dmm.039982
Katoh, K., Omori, Y., Onishi, A., Sato, S., Kondo, M., and Furukawa, T. (2010). Blimp1 Suppresses Chx10 Expression in Differentiating Retinal Photoreceptor Precursors to Ensure Proper Photoreceptor Development. J. Neurosci. 30 (19), 6515–6526. doi:10.1523/JNEUROSCI.0771-10.2010
Kautzmann, M.-A. I., Kim, D. S., Felder-Schmittbuhl, M.-P., and Swaroop, A. (2011). Combinatorial Regulation of Photoreceptor Differentiation Factor, Neural Retina Leucine Zipper Gene NRL, Revealed by In Vivo Promoter Analysis. J. Biol. Chem. 286 (32), 28247–28255. doi:10.1074/jbc.M111.257246
Kelley, M. W., Turner, J. K., and Reh, T. A. (1994). Retinoic Acid Promotes Differentiation of Photoreceptors In Vitro. Development 120 (8), 2091–2102. doi:10.1242/dev.120.8.2091
Kelley, M. W., Williams, R. C., Turner, J. K., Creech-Kraft, J. M., and Reh, T. A. (1999). Retinoic Acid Promotes Rod Photoreceptor Differentiation in Rat Retina In Vivo. Neuroreport 10 (11), 2389–2394. doi:10.1097/00001756-199908020-00031
Khanna, H., Akimoto, M., Siffroi-Fernandez, S., Friedman, J. S., Hicks, D., and Swaroop, A. (2006). Retinoic Acid Regulates the Expression of Photoreceptor Transcription Factor NRL. J. Biol. Chem. 281 (37), 27327–27334. doi:10.1074/jbc.M605500200
Kim, D. S., Matsuda, T., and Cepko, C. L. (2008). A Core Paired-type and POU Homeodomain-Containing Transcription Factor Program Drives Retinal Bipolar Cell Gene Expression. J. Neurosci. 28 (31), 7748–7764. doi:10.1523/JNEUROSCI.0397-08.2008
Kirschfeld, K. (1982). Carotenoid Pigments: Their Possible Role in Protecting against Photooxidation in Eyes and Photoreceptor Cells. Proc. R. Soc. Lond. B. 216 (1202), 71–85. doi:10.1098/rspb.1982.0061
Klug, K., Herr, S., Ngo, I. T., Sterling, P., and Schein, S. (2003). Macaque Retina Contains an S-Cone OFF Midget Pathway. J. Neurosci. 23 (30), 9881–9887. doi:10.1523/jneurosci.23-30-09881.2003
Kohl, S., Coppieters, F., Meire, F., Schaich, S., Roosing, S., Brennenstuhl, C., et al. (2012). A Nonsense Mutation in PDE6H Causes Autosomal-Recessive Incomplete Achromatopsia. Am. J. Hum. Genet. 91 (3), 527–532. doi:10.1016/j.ajhg.2012.07.006
Kolb, H., and Marshak, D. (2003). The Midget Pathways of the Primate Retina. Doc Ophthalmol. 106 (1), 67–81. doi:10.1023/a:1022469002511
Kolesnikov, A. V., Kiser, P. D., Palczewski, K., and Kefalov, V. J. (2021). Function of mammalian M-cones depends on the level of CRALBP in Müller cellsARTN e202012675. J. Gen. Physiol. 153 (1). doi:10.1085/jgp.202012675
König, A. (1894). Über den menschlichen Sehpurpur und seine Bedeutung für das Sehen. In Königlich Preussischen Akademie der Wissenschaften.
Kosower, E. M. (1988). Assignment of Groups Responsible for the "opsin Shift" and Light Absorptions of Rhodopsin and Red, green, and Blue Iodopsins (Cone Pigments). Proc. Natl. Acad. Sci. U.S.A. 85 (4), 1076–1080. doi:10.1073/pnas.85.4.1076
Kremers, J., Scholl, H. P. N., Knau, H., Berendschot, T. T. J. M., Usui, T., and Sharpe, L. T. (2000). L/M Cone Ratios in Human Trichromats Assessed by Psychophysics, Electroretinography, and Retinal Densitometry. J. Opt. Soc. Am. A. 17 (3), 517–526. doi:10.1364/josaa.17.000517
Kuchenbecker, J. A., Sahay, M., Tait, D. M., Neitz, M., and Neitz, J. (2008). Topography of the Long- to Middle-Wavelength Sensitive Cone Ratio in the Human Retina Assessed with a Wide-Field Color Multifocal Electroretinogram. Vis. Neurosci. 25 (3), 301–306. doi:10.1017/S0952523808080474
La Vail, M. M., Rapaport, D. H., and Rakic, P. (1991). Cytogenesis in the Monkey Retina. J. Comp. Neurol. 309 (1), 86–114. doi:10.1002/cne.903090107
Labin, A. M., Safuri, S. K., Ribak, E. N., and Perlman, I. (2014). Müller Cells Separate between Wavelengths to Improve Day Vision with Minimal Effect upon Night Vision. Nat. Commun. 5, 4319. doi:10.1038/ncomms5319
Laties, A. M., and Enoch, J. M. (1971). An Analysis of Retinal Receptor Orientation. I. Angular Relationship of Neighboring Photoreceptors. Invest. Ophthalmol. 10 (1), 69–77.
Laties, A. M., Liebman, P. A., and Campbell, C. E. M. (1968). Photoreceptor Orientation in the Primate Eye. Nature 218(5137), 172–173. doi:10.1038/218172a0
Laties, M.D., A. M. (1969). Histological Techniques for Study of Photoreceptor Orientation. Tissue and Cell 1 (1), 63–81. doi:10.1016/s0040-8166(69)80006-6
Lennie, P., Pokorny, J., and Smith, V. C. (1993). Luminance. J. Opt. Soc. Am. A. 10 (6), 1283–1293. doi:10.1364/josaa.10.001283
Lerea, C. L., Bunt-Milam, A. H., and Hurley, J. B. (1989). α Transducin Is Present in Blue-, green-, and Red-Sensitive Cone Photoreceptors in the Human Retina. Neuron 3 (3), 367–376. doi:10.1016/0896-6273(89)90261-4
Levine, E. M., Roelink, H., Turner, J., and Reh, T. A. (1997). Sonic Hedgehog Promotes Rod Photoreceptor Differentiation in Mammalian Retinal CellsIn Vitro. J. Neurosci. 17 (16), 6277–6288. doi:10.1523/jneurosci.17-16-06277.1997
Liu, J., Jung, H., Dubra, A., and Tam, J. (2018). Cone Photoreceptor Cell Segmentation and Diameter Measurement on Adaptive Optics Images Using Circularly Constrained Active Contour Model. Invest. Ophthalmol. Vis. Sci. 59 (11), 4639–4652. doi:10.1167/iovs.18-24734
Liu, Q., Zhang, Q., and Pierce, E. A. (2010). Photoreceptor Sensory Cilia and Inherited Retinal Degeneration. Adv. Exp. Med. Biol. 664, 223–232. doi:10.1007/978-1-4419-1399-9_26
Livne-Bar, I., Pacal, M., Cheung, M. C., Hankin, M., Trogadis, J., Chen, D., et al. (2006). Chx10 Is Required to Block Photoreceptor Differentiation but Is Dispensable for Progenitor Proliferation in the Postnatal Retina. Proc. Natl. Acad. Sci. U.S.A. 103 (13), 4988–4993. doi:10.1073/pnas.0600083103
Lu, Y., Shiau, F., Yi, W., Lu, S., Wu, Q., Pearson, J. D., et al. (2020). Single-Cell Analysis of Human Retina Identifies Evolutionarily Conserved and Species-specific Mechanisms Controlling Development. Dev. Cel 53 (4), 473–491. doi:10.1016/j.devcel.2020.04.009
Mackin, R. D., Frey, R. A., Gutierrez, C., Farre, A. A., Kawamura, S., Mitchell, D. M., et al. (2019). Endocrine Regulation of Multichromatic Color Vision. Proc. Natl. Acad. Sci. U.S.A. 116 (34), 16882–16891. doi:10.1073/pnas.1904783116
Marc, R. E., and Sperling, H. G. (1977). Chromatic Organization of Primate Cones. Science 196 (4288), 454–456. doi:10.1126/science.403607
Marcos, L. F., Wilson, S. L., and Roach, P. (2021). Tissue Engineering of the Retina: from Organoids to Microfluidic Chips. J. Tissue Eng. 12, 204173142110598. doi:10.1177/20417314211059876
Marmor, M. F., Jacobson, S. G., Foerster, M. H., Kellner, U., and Weleber, R. G. (1990). Diagnostic Clinical Findings of a New Syndrome with Night Blindness, Maculopathy, and Enhanced S Cone Sensitivity. Am. J. Ophthalmol. 110 (2), 124–134. doi:10.1016/s0002-9394(14)76980-6
Marquardt, T., Ashery-Padan, R., Andrejewski, N., Scardigli, R., Guillemot, F., and Gruss, P. (2001). Pax6 Is Required for the Multipotent State of Retinal Progenitor Cells. Cell 105 (1), 43–55. doi:10.1016/s0092-8674(01)00295-1
Martin, P. R., Grünert, U., Chan, T. L., and Bumsted, K. (2000). Spatial Order in Short-Wavelength-Sensitive Cone Photoreceptors: a Comparative Study of the Primate Retina. J. Opt. Soc. Am. A. 17 (3), 557–567. doi:10.1364/josaa.17.000557
Mata, N. L., Radu, R. A., Clemmons, R. S., and Travis, G. H. (2002). Isomerization and Oxidation of Vitamin A in Cone-Dominant Retinas. Neuron 36 (1), 69–80. doi:10.1016/s0896-6273(02)00912-1
McCafferty, B. K., Wilk, M. A., McAllister, J. T., Stepien, K. E., Dubis, A. M., Brilliant, M. H., et al. (2015). Clinical Insights into Foveal Morphology in Albinism. J. Pediatr. Ophthalmol. Strabismus 52 (3), 167–172. doi:10.3928/01913913-20150427-06
McKusick, V. A. (2007). Mendelian Inheritance in Man and its Online Version, OMIM. Am. J. Hum. Genet. 80 (4), 588–604. doi:10.1086/514346
McMahon, C., Carroll, J., Awua, S., Neitz, J., and Neitz, M. (2008). The L:M Cone Ratio in Males of African Descent with normal Color Vision. J. Vis. 8 (2), 5–9. doi:10.1167/8.2.5
McMahon, C., Neitz, J., and Neitz, M. (2004). Evaluating the Human X-Chromosome Pigment Gene Promoter Sequences as Predictors of L:M Cone Ratio Variation. J. Vis. 4 (3), 7–208. doi:10.1167/4.3.7
McNerney, C., and Johnston, R. J. (2021). Thyroid Hormone Signaling Specifies Cone Photoreceptor Subtypes during Eye Development: Insights from Model Organisms and Human Stem Cell-Derived Retinal Organoids. Vitam Horm. 116, 51–90. doi:10.1016/bs.vh.2021.03.001
Mears, A. J., Kondo, M., Swain, P. K., Takada, Y., Bush, R. A., Saunders, T. L., et al. (2001). Nrl Is Required for Rod Photoreceptor Development. Nat. Genet. 29 (4), 447–452. doi:10.1038/ng774
Milam, A. H., Rose, L., Cideciyan, A. V., Barakat, M. R., Tang, W.-X., Gupta, N., et al. (2002). The Nuclear Receptor NR2E3 Plays a Role in Human Retinal Photoreceptor Differentiation and Degeneration. Proc. Natl. Acad. Sci. U.S.A. 99 (1), 473–478. doi:10.1073/pnas.022533099
Molday, R. S., and Molday, L. L. (1987). Differences in the Protein Composition of Bovine Retinal Rod Outer Segment Disk and Plasma Membranes Isolated by a Ricin-Gold-Dextran Density Perturbation Method. J. Cel Biol 105 (6 Pt 1), 2589–2601. doi:10.1083/jcb.105.6.2589
Mollon, J. D., and Bowmaker, J. K. (1992). The Spatial Arrangement of Cones in the Primate Fovea. Nature 360 (6405), 677–679. doi:10.1038/360677a0
Mori, M., Ghyselinck, N. B., Chambon, P., and Mark, M. (2001). Systematic Immunolocalization of Retinoid Receptors in Developing and Adult Mouse Eyes. Invest. Ophthalmol. Vis. Sci. 42 (6), 1312–1318.
Muranishi, Y., Terada, K., Inoue, T., Katoh, K., Tsujii, T., Sanuki, R., et al. (2011). An Essential Role for RAX Homeoprotein and NOTCH-HES Signaling in Otx2 Expression in Embryonic Retinal Photoreceptor Cell Fate Determination. J. Neurosci. 31 (46), 16792–16807. doi:10.1523/JNEUROSCI.3109-11.2011
Myers, C. E., Klein, B. E. K., Meuer, S. M., Swift, M. K., Chandler, C. S., Huang, Y., et al. (2015). Retinal Thickness Measured by Spectral-Domain Optical Coherence Tomography in Eyes without Retinal Abnormalities: the Beaver Dam Eye Study. Am. J. Ophthalmol. 159 (3), 445–456. doi:10.1016/j.ajo.2014.11.025
Nakano, T., Ando, S., Takata, N., Kawada, M., Muguruma, K., Sekiguchi, K., et al. (2012). Self-formation of Optic Cups and Storable Stratified Neural Retina from Human ESCs. Cell Stem Cell 10 (6), 771–785. doi:10.1016/j.stem.2012.05.009
Nathans, J., Davenport, C. M., Maumenee, I. H., Lewis, R. A., Hejtmancik, J. F., Litt, M., et al. (1989). Molecular Genetics of Human Blue Cone Monochromacy. Science 245 (4920), 831–838. doi:10.1126/science.2788922
Nathans, J., Piantanida, T. P., Eddy, R. L., Shows, T. B., and Hogness, D. S. (1986a). Molecular Genetics of Inherited Variation in Human Color Vision. Science 232 (4747), 203–210. doi:10.1126/science.3485310
Nathans, J., Thomas, D., and Hogness, D. S. (1986b). Molecular Genetics of Human Color Vision: the Genes Encoding Blue, green, and Red Pigments. Science 232 (4747), 193–202. doi:10.1126/science.2937147
Neitz, M., Balding, S. D., McMahon, C., Sjoberg, S. A., and Neitz, J. (2006). Topography of Long- and Middle-Wavelength Sensitive Cone Opsin Gene Expression in Human and Old World Monkey Retina. Vis. Neurosci. 23 (3-4), 379–385. doi:10.1017/S095252380623325X
Nelson, B. R., Hartman, B. H., Georgi, S. A., Lan, M. S., and Reh, T. A. (2007). Transient Inactivation of Notch Signaling Synchronizes Differentiation of Neural Progenitor Cells. Dev. Biol. 304 (2), 479–498. doi:10.1016/j.ydbio.2007.01.001
Newman, E. A. (1994). A Physiological Measure of Carbonic Anhydrase in Müller Cells. Glia 11 (4), 291–299. doi:10.1002/glia.440110402
Ng, L., Hurley, J. B., Dierks, B., Srinivas, M., Saltó, C., Vennström, B., et al. (2001). A Thyroid Hormone Receptor that Is Required for the Development of green Cone Photoreceptors. Nat. Genet. 27 (1), 94–98. doi:10.1038/83829
Ng, L., Lyubarsky, A., Nikonov, S. S., Ma, M., Srinivas, M., Kefas, B., et al. (2010). Type 3 Deiodinase, a Thyroid-Hormone-Inactivating Enzyme, Controls Survival and Maturation of Cone Photoreceptors. J. Neurosci. 30 (9), 3347–3357. doi:10.1523/JNEUROSCI.5267-09.2010
Ng, L., Ma, M., Curran, T., and Forrest, D. (2009). Developmental Expression of Thyroid Hormone Receptor β2 Protein in Cone Photoreceptors in the Mouse. Neuroreport 20 (6), 627–631. doi:10.1097/WNR.0b013e32832a2c63
Nishida, A., Furukawa, A., Koike, C., Tano, Y., Aizawa, S., Matsuo, I., et al. (2003). Otx2 Homeobox Gene Controls Retinal Photoreceptor Cell Fate and Pineal Gland Development. Nat. Neurosci. 6 (12), 1255–1263. doi:10.1038/nn1155
Nork, T. M., McCormick, S. A., Chao, G. M., and Odom, J. V. (1990). Distribution of Carbonic Anhydrase Among Human Photoreceptors. Invest. Ophthalmol. Vis. Sci. 31 (8), 1451–1458.
Oh, E. C. T., Cheng, H., Hao, H., Jia, L., Khan, N. W., and Swaroop, A. (2008). Rod Differentiation Factor NRL Activates the Expression of Nuclear Receptor NR2E3 to Suppress the Development of Cone Photoreceptors. Brain Res. 1236, 16–29. doi:10.1016/j.brainres.2008.01.028
Oh, E. C. T., Khan, N., Novelli, E., Khanna, H., Strettoi, E., and Swaroop, A. (2007). Transformation of Cone Precursors to Functional Rod Photoreceptors by bZIP Transcription Factor NRL. Proc. Natl. Acad. Sci. U.S.A. 104 (5), 1679–1684. doi:10.1073/pnas.0605934104
Onishi, A., Peng, G.-H., Chen, S., and Blackshaw, S. (2010). Pias3-dependent SUMOylation Controls Mammalian Cone Photoreceptor Differentiation. Nat. Neurosci. 13 (9), 1059–1065. doi:10.1038/nn.2618
Onishi, A., Peng, G.-H., Hsu, C., Alexis, U., Chen, S., and Blackshaw, S. (2009). Pias3-dependent SUMOylation Directs Rod Photoreceptor Development. Neuron 61 (2), 234–246. doi:10.1016/j.neuron.2008.12.006
Otake, S., Gowdy, P. D., and Cicerone, C. M. (2000). The Spatial Arrangement of L and M Cones in the Peripheral Human Retina. Vis. Res. 40 (6), 677–693. doi:10.1016/s0042-6989(99)00202-3
Packer, O., Hendrickson, A. E., and Curcio, C. A. (1989). Photoreceptor Topography of the Retina in the Adult Pigtail Macaque (Macaca nemestrina). J. Comp. Neurol. 288 (1), 165–183. doi:10.1002/cne.902880113
Packer, O., Williams, D., and Bensinger, D. (1996). Photopigment Transmittance Imaging of the Primate Photoreceptor Mosaic. J. Neurosci. 16 (7), 2251–2260. doi:10.1523/jneurosci.16-07-02251.1996
Patterson, S. S., Kuchenbecker, J. A., Anderson, J. R., Bordt, A. S., Marshak, D. W., Neitz, M., et al. (2019). An S-Cone Circuit for Edge Detection in the Primate Retina. Sci. Rep. 9 (1), 11913. doi:10.1038/s41598-019-48042-2
Peng, G.-H., Ahmad, O., Ahmad, F., Liu, J., and Chen, S. (2005). The photoreceptor-specific nuclear receptor Nr2e3 interacts with Crx and exerts opposing effects on the transcription of rod versus cone genes. Hum. Mol. Genet. 14 (6), 747–764. doi:10.1093/hmg/ddi070
Peng, Y.-R., Shekhar, K., Yan, W., Herrmann, D., Sappington, A., Bryman, G. S., et al. (2019). Molecular Classification and Comparative Taxonomics of Foveal and Peripheral Cells in Primate Retina. Cell 176 (5), 1222–1237. doi:10.1016/j.cell.2019.01.004
Phillips, M. J., Jiang, P., Howden, S., Barney, P., Min, J., York, N. W., et al. (2018). A Novel Approach to Single Cell RNA-Sequence Analysis Facilitates In Silico Gene Reporting of Human Pluripotent Stem Cell-Derived Retinal Cell Types. Stem Cells 36 (3), 313–324. doi:10.1002/stem.2755
Picaud, S., Dalkara, D., Marazova, K., Goureau, O., Roska, B., and Sahel, J.-A. (2019). The Primate Model for Understanding and Restoring Vision. Proc. Natl. Acad. Sci. U.S.A. 116, 26280–26287. doi:10.1073/pnas.1902292116
Pokorny, J., Smith, V. C., and Swartley, R. (1970). Threshold Measurements of Spectral Sensitivity in a Blue Monocone Monochromat. Invest. Ophthalmol. 9 (10), 807–813.
Polyak, S. L., Kluver, H., and Klüver, H. (1957). The Vertebrate Visual System: Its Origin, Structure, and Function and its Manifestations in Disease with an Analysis of its Role in the Life of Animals and in the Origin of Man, Preceded by a Historical Review of Investigations of the Eye, and of the Visual Pathways and Centers of the Brain. University of Chicago Press.
Polyak, S. L. (1941). The Retina: The Anatomy and the Histology of the Retina in Man, Ape, and Monkey, Including the Consideration of Visual Functions, the History of Physiological Optics, and the Histological Laboratory Technique. Chicago: University of Chicago Press.
Provis, J. M., van Driel, D., Billson, F. A., and Russell, P. (1985). Development of the Human Retina: Patterns of Cell Distribution and Redistribution in the Ganglion Cell Layer. J. Comp. Neurol. 233 (4), 429–451. doi:10.1002/cne.902330403
Quinn, N., Csincsik, L., Flynn, E., Curcio, C. A., Kiss, S., Sadda, S. R., et al. (2019). The Clinical Relevance of Visualising the Peripheral Retina. Prog. Retin. Eye Res. 68, 83–109. doi:10.1016/j.preteyeres.2018.10.001
Rapaport, D. H., Wong, L. L., Wood, E. D., Yasumura, D., and LaVail, M. M. (2004). Timing and Topography of Cell Genesis in the Rat Retina. J. Comp. Neurol. 474 (2), 304–324. doi:10.1002/cne.20134
Reading, V. M., and Weale, R. A. (1974). Macular Pigment and Chromatic Aberration. J. Opt. Soc. Am. 64 (2), 231–234. doi:10.1364/josa.64.000231
Roberts, M. R., Hendrickson, A., McGuire, C. R., and Reh, T. A. (2005). Retinoid X Receptor γ Is Necessary to Establish the S-Opsin Gradient in Cone Photoreceptors of the Developing Mouse Retina. Invest. Ophthalmol. Vis. Sci. 46 (8), 2897–2904. doi:10.1167/iovs.05-0093
Roberts, M. R., Srinivas, M., Forrest, D., Morreale de Escobar, G., and Reh, T. A. (2006). Making the Gradient: Thyroid Hormone Regulates Cone Opsin Expression in the Developing Mouse Retina. Proc. Natl. Acad. Sci. U.S.A. 103 (16), 6218–6223. doi:10.1073/pnas.0509981103
Roorda, A., Metha, A. B., Lennie, P., and Williams, D. R. (2001). Packing Arrangement of the Three Cone Classes in Primate Retina. Vis. Res 41 (10-11), 1291–1306. doi:10.1016/s0042-6989(01)00043-8
Roorda, A., and Williams, D. R. (1999). The Arrangement of the Three Cone Classes in the Living Human Eye. Nature 397 (6719), 520–522. doi:10.1038/17383
Rosenberg, T., Baumann, B., Kohl, S., Zrenner, E., Jorgensen, A. L., and Wissinger, B. (2004). Variant Phenotypes of Incomplete Achromatopsia in Two Cousins withGNAT2Gene Mutations. Invest. Ophthalmol. Vis. Sci. 45 (12), 4256–4262. doi:10.1167/iovs.04-0317
Rovet, J., and Simic, N. (2008). The Role of Transient Hypothyroxinemia of Prematurity in Development of Visual Abilities. Semin. Perinatology 32 (6), 431–437. doi:10.1053/j.semperi.2008.09.009
Rushton, W. A., and Baker, H. D. (1964). Red--grees Sensitivity in normal Vision. Vis. Res 4 (1), 75–85. doi:10.1016/0042-6989(64)90034-3
Sakmar, T. P., and Khorana, H. G. (1988). Total Synthesis and Expression of a Gene for the α-subunit of Bovine Rod Outer Segment Guanine Nucleotide-Binding Protein (Transducin). Nucl. Acids Res. 16 (14A), 6361–6372. doi:10.1093/nar/16.14.6361
Samuels, H. H., Tsai, J. S., Casanova, J., and Stanley, F. (1974). Thyroid Hormone Action In Vitro CHARACTERIZATION of SOLUBILIZED NUCLEAR RECEPTORS from RAT LIVER and CULTURED GH1 CELLS. J. Clin. Invest. 54 (4), 853–865. doi:10.1172/JCI107825
Sancho-Pelluz, J., Arango-Gonzalez, B., Kustermann, S., Romero, F. J., van Veen, T., Zrenner, E., et al. (2008). Photoreceptor Cell Death Mechanisms in Inherited Retinal Degeneration. Mol. Neurobiol. 38 (3), 253–269. doi:10.1007/s12035-008-8045-9
Satoh, S., Tang, K., Iida, A., Inoue, M., Kodama, T., Tsai, S. Y., et al. (2009). The Spatial Patterning of Mouse Cone Opsin Expression Is Regulated by Bone Morphogenetic Protein Signaling through Downstream Effector COUP-TF Nuclear Receptors. J. Neurosci. 29 (40), 12401–12411. doi:10.1523/JNEUROSCI.0951-09.2009
Sawides, L., de Castro, A., and Burns, S. A. (2017). The Organization of the Cone Photoreceptor Mosaic Measured in the Living Human Retina. Vis. Res. 132, 34–44. doi:10.1016/j.visres.2016.06.006
Schroeder, A. C., and Privalsky, M. L. (2014). Thyroid Hormones, T3 and T4, in the Brain. Front. Endocrinol. 5, 40. doi:10.3389/fendo.2014.00040
Schultze, M. (1866). Zur Anatomie und Physiologie der Retina. Archiv F. Mikrosk. Anatomie 2 (1), 175–286. doi:10.1007/BF02962033
Shen, W., Lee, S. R., Mathai, A. E., Zhang, R., Du, J., Yam, M. X., et al. (2021). Effect of Selectively Knocking Down Key Metabolic Genes in Müller Glia on Photoreceptor Health. Glia 69 (8), 1966–1986. doi:10.1002/glia.24005
Simic, N., Westall, C., Astzalos, E. V., and Rovet, J. (2010). Visual Abilities at 6 Months in Preterm Infants: Impact of Thyroid Hormone Deficiency and Neonatal Medical Morbidity. Thyroid 20 (3), 309–315. doi:10.1089/thy.2009.0128
Sinha, R., Hoon, M., Baudin, J., Okawa, H., Wong, R. O. L., and Rieke, F. (2017). Cellular and Circuit Mechanisms Shaping the Perceptual Properties of the Primate Fovea. Cell 168 (3), 413–426. doi:10.1016/j.cell.2017.01.005
Sjoberg, M., Vennstrom, B., and Forrest, D. (1992). Thyroid Hormone Receptors in Chick Retinal Development: Differential Expression of mRNAs for Alpha and N-Terminal Variant Beta Receptors. Development 114 (1), 39–47. doi:10.1242/dev.114.1.39
Sjöstrand, F. S. (1953). The Ultrastructure of the Outer Segments of Rods and Cones of the Eye as Revealed by the Electron Microscope. J. Cel. Comp. Physiol. 42 (1), 15–44. doi:10.1002/jcp.1030420103
Skorczyk-Werner, A., Chiang, W.-C., Wawrocka, A., Wicher, K., Jarmuż-Szymczak, M., Kostrzewska-Poczekaj, M., et al. (2017). Autosomal Recessive Cone-Rod Dystrophy Can Be Caused by Mutations in the ATF6 Gene. Eur. J. Hum. Genet. 25 (11), 1210–1216. doi:10.1038/ejhg.2017.131
Sloan, L. L. (1954). Congenital Achromatopsia: A Report of 19 Cases*. J. Opt. Soc. Am. 44 (2), 117–128. doi:10.1364/josa.44.000117
Smallwood, P. M., Wang, Y., and Nathans, J. (2002). Role of a Locus Control Region in the Mutually Exclusive Expression of Human Red and green Cone Pigment Genes. Proc. Natl. Acad. Sci. U.S.A. 99 (2), 1008–1011. doi:10.1073/pnas.022629799
Smelser, G. K., Ozanics, V., Rayborn, M., and Sagun, D. (1974). Retinal Synaptogenesis in the Primate. Invest. Ophthalmol. 13 (5), 340–361.
Smith, V. C., Pokorny, J., and Newell, F. W. (1979). Autosomal Recessive Incomplete Achromatopsia with Deutan Luminosity. Am. J. Ophthalmol. 87 (3), 393–402. doi:10.1016/0002-9394(79)90083-7
Smith, V. C., Pokorny, J., and Newell, F. W. (1978). Autosomal Recessive Incomplete Achromatopsia with Protan Luminosity Function. Ophthalmologica 177 (4), 197–207. doi:10.1159/000308767
Spivey, B. E. (1965). The X-Linked Recessive Inheritance of Atypical Monochromatism. Arch. Ophthalmol. 74, 327–333. doi:10.1001/archopht.1965.00970040329007
Sridhar, A., Hoshino, A., Finkbeiner, C. R., Chitsazan, A., Dai, L., Haugan, A. K., et al. (2020). Single-Cell Transcriptomic Comparison of Human Fetal Retina, hPSC-Derived Retinal Organoids, and Long-Term Retinal Cultures. Cel Rep. 30 (5), 1644–1659. e1644. doi:10.1016/j.celrep.2020.01.007
Stone, J., Makarov, F., and Holländer, H. (1995). The Glial Ensheathment of the Soma and Axon Hillock of Retinal Ganglion Cells. Vis. Neurosci. 12 (2), 273–279. doi:10.1017/s0952523800007951
Strauss, O., Stumpff, F., Mergler, S., Wienrich, M., and Wiederholt, M. (1998). The Royal College of Surgeons Rat: an Animal Model for Inherited Retinal Degeneration with a Still Unknown Genetic Defect. Cells Tissues Organs 162 (2-3), 101–111. doi:10.1159/000046474
Suzuki, S. C., Bleckert, A., Williams, P. R., Takechi, M., Kawamura, S., and Wong, R. O. L. (2013). Cone Photoreceptor Types in Zebrafish Are Generated by Symmetric Terminal Divisions of Dedicated Precursors. Proc. Natl. Acad. Sci. U.S.A. 110 (37), 15109–15114. doi:10.1073/pnas.1303551110
Swaroop, A., Wang, Q. L., Wu, W., Cook, J., Coats, C., Xu, S., et al. (1999). Leber Congenital Amaurosis Caused by a Homozygous Mutation (R90W) in the Homeodomain of the Retinal Transcription Factor CRX: Direct Evidence for the Involvement of CRX in the Development of Photoreceptor Function. Hum. Mol. Genet. 8 (2), 299–305. doi:10.1093/hmg/8.2.299
Szél, Á., Diamanstein, T., and Röhlich, P. (1988). Identification of the Blue-Sensitive Cones in the Mammalian Retina by Anti-visual Pigment Antibody. J. Comp. Neurol. 273 (4), 593–602. doi:10.1002/cne.902730413
Thomas, E. D., Timms, A. E., Giles, S., Harkins-Perry, S., Lyu, P., Hoang, T., et al. (2022). Cell-specific Cis-Regulatory Elements and Mechanisms of Non-coding Genetic Disease in Human Retina and Retinal Organoids. Dev. Cel. doi:10.1016/j.devcel.2022.02.018
Thomas, M. G., Kumar, A., Mohammad, S., Proudlock, F. A., Engle, E. C., Andrews, C., et al. (2011). Structural Grading of Foveal Hypoplasia Using Spectral-Domain Optical Coherence Tomography. Ophthalmology 118 (8), 1653–1660. doi:10.1016/j.ophtha.2011.01.028
Thompson, D. A., and Gal, A. (2003). Genetic Defects in Vitamin A Metabolism of the Retinal Pigment Epithelium. Dev. Ophthalmol. 37, 141–154. doi:10.1159/000072044
Tootell, R., Switkes, E., Silverman, M., and Hamilton, S. (1988). Functional Anatomy of Macaque Striate Cortex. II. Retinotopic Organization. J. Neurosci. 8 (5), 1531–1568. doi:10.1523/jneurosci.08-05-01531.1988
Trimarchi, J. M., Harpavat, S., Billings, N. A., and Cepko, C. L. (2008). Thyroid Hormone Components Are Expressed in Three Sequential Waves during Development of the Chick Retina. BMC Dev. Biol. 8, 101. doi:10.1186/1471-213X-8-101
Tsacopoulos, M., and Magistretti, P. (1996). Metabolic Coupling between Glia and Neurons. J. Neurosci. 16 (3), 877–885. doi:10.1523/jneurosci.16-03-00877.1996
Tsujimura, T., Chinen, A., and Kawamura, S. (2007). Identification of a Locus Control Region for Quadruplicated green-sensitive Opsin Genes in Zebrafish. Proc. Natl. Acad. Sci. U.S.A. 104 (31), 12813–12818. doi:10.1073/pnas.0704061104
Turner, D. L., and Cepko, C. L. (1987). A Common Progenitor for Neurons and Glia Persists in Rat Retina Late in Development. Nature 328 (6126), 131–136. doi:10.1038/328131a0
Turner, D. L., Snyder, E. Y., and Cepko, C. L. (1990). Lineage-independent Determination of Cell Type in the Embryonic Mouse Retina. Neuron 4 (6), 833–845. doi:10.1016/0896-6273(90)90136-4
Ueyama, H., Muraki, S., Tanabe, S., Yamade, S., and Ogita, H. (2015). A New Subset of Deutan Colour Vision Defect Associated with an L/M Visual Pigment Gene Array of normal Order and −71C Substitution in the Japanese Population. J. Biochem. 158 (3), 197–204. doi:10.1093/jb/mvv034
Vancamp, P., Bourgeois, N. M. A., Houbrechts, A. M., and Darras, V. M. (2019). Knockdown of the Thyroid Hormone Transporter MCT8 in Chicken Retinal Precursor Cells Hampers Early Retinal Development and Results in a Shift towards More UV/blue Cones at the Expense of green/red Cones. Exp. Eye Res. 178, 135–147. doi:10.1016/j.exer.2018.09.018
Vandenberghe, L. H., Bell, P., Maguire, A. M., Xiao, R., Hopkins, T. B., Grant, R., et al. (2013). AAV9 Targets Cone Photoreceptors in the Nonhuman Primate Retina. PLoS One 8 (1), e53463. doi:10.1371/journal.pone.0053463
Verschueren, A., Boucherit, L., Ferrari, U., Fouquet, S., Nouvel-Jaillard, C., Paques, M., et al. (2022). Planar Polarity in Primate Cone Photoreceptors: a Potential Role in Stiles Crawford Effect Phototropism. Commun. Biol. 5 (1), 89. doi:10.1038/s42003-021-02998-y
Vincent, A., Wright, T., Billingsley, G., Westall, C., and Héon, E. (2011). Oligocone Trichromacy Is Part of the Spectrum ofCNGA3-Related Cone System Disorders. Ophthalmic Genet. 32 (2), 107–113. doi:10.3109/13816810.2010.544366
Vollrath, D., Nathans, J., and Davis, R. W. (1988). Tandem Array of Human Visual Pigment Genes at Xq28. Science 240 (4859), 1669–1672. doi:10.1126/science.2837827
Wahlin, K. J., Maruotti, J. A., Sripathi, S. R., Ball, J., Angueyra, J. M., Kim, C., et al. (2017). Photoreceptor Outer Segment-like Structures in Long-Term 3D Retinas from Human Pluripotent Stem Cells. Sci. Rep. 7 (1), 766. doi:10.1038/s41598-017-00774-9
Wald, G. (1967). Blue-Blindness in the Normal Fovea*. J. Opt. Soc. Am. 57 (11), 1289–1301. doi:10.1364/josa.57.001289
Walls, G. L. (1937). SIGNIFICANCE OF THE FOVEAL DEPRESSION. Arch. Ophthalmol. 18 (6), 912–919. doi:10.1001/archopht.1937.00850120046005
Wang, S., Sengel, C., Emerson, M. M., and Cepko, C. L. (2014). A Gene Regulatory Network Controls the Binary Fate Decision of Rod and Bipolar Cells in the Vertebrate Retina. Dev. Cel 30 (5), 513–527. doi:10.1016/j.devcel.2014.07.018
Wang, Y., Macke, J. P., Merbs, S. L., Zack, D. J., Klaunberg, B., Bennett, J., et al. (1992). A Locus Control Region Adjacent to the Human Red and green Visual Pigment Genes. Neuron 9 (3), 429–440. doi:10.1016/0896-6273(92)90181-c
Wang, Y., Smallwood, P. M., Cowan, M., Blesh, D., Lawler, A., and Nathans, J. (1999). Mutually Exclusive Expression of Human Red and green Visual Pigment-Reporter Transgenes Occurs at High Frequency in Murine Cone Photoreceptors. Proc. Natl. Acad. Sci. U.S.A. 96 (9), 5251–5256. doi:10.1073/pnas.96.9.5251
Wässle, H., Grünert, U., Röhrenbeck, J., and Boycott, B. B. (1989). Cortical Magnification Factor and the Ganglion Cell Density of the Primate Retina. Nature 341 (6243), 643–646. doi:10.1038/341643a0
Weiss, A. H., Kelly, J. P., Bisset, D., and Deeb, S. S. (2012). Reduced L- and M- and Increased S-Cone Functions in an Infant with Thyroid Hormone Resistance Due to Mutations in theTHRβ2gene. Ophthalmic Genet. 33 (4), 187–195. doi:10.3109/13816810.2012.681096
Wensel, T. G., Zhang, Z., Anastassov, I. A., Gilliam, J. C., He, F., Schmid, M. F., et al. (2016). Structural and Molecular Bases of Rod Photoreceptor Morphogenesis and Disease. Prog. Retin. Eye Res. 55, 32–51. doi:10.1016/j.preteyeres.2016.06.002
Wetts, R., and Fraser, S. E. (1988). Multipotent Precursors Can Give Rise to All Major Cell Types of the Frog Retina. Science 239 (4844), 1142–1145. doi:10.1126/science.2449732
Wikler, K. C., Szel, A., and Jacobsen, A.-L. (1996). Positional Information and Opsin Identity in Retinal Cones. J. Comp. Neurol. 374 (1), 96–107. doi:10.1002/(SICI)1096-9861(19961007)374:1<96::AID-CNE7>3.0.CO;2-I
Wilk, M. A., McAllister, J. T., Cooper, R. F., Dubis, A. M., Patitucci, T. N., Summerfelt, P., et al. (2014). Relationship between Foveal Cone Specialization and Pit Morphology in Albinism. Invest. Ophthalmol. Vis. Sci. 55 (7), 4186–4198. doi:10.1167/iovs.13-13217
Williams, D. R., MacLeod, D. I. A., and Hayhoe, M. M. (1981). Foveal Tritanopia. Vis. Res. 21 (9), 1341–1356. doi:10.1016/0042-6989(81)90241-8
Williams, R. W. (1991). The Human Retina Has a Cone-Enriched Rim. Vis. Neurosci. 6 (4), 403–406. doi:10.1017/s0952523800006647
Willmer, E. N., and Wright, W. D. (1945). Colour Sensitivity of the Fovea Centralis. Nature 156 (3952), 119–121. doi:10.1038/156119a0
Winderickx, J., Battisti, L., Motulsky, A. G., and Deeb, S. S. (1992). Selective Expression of Human X Chromosome-Linked green Opsin Genes. Proc. Natl. Acad. Sci. U.S.A. 89 (20), 9710–9714. doi:10.1073/pnas.89.20.9710
Winston, R. (1981). “The Visual Receptor as a Light Collector,” in Vertebrate Photoreceptor Optics (New York: Springer-Verlag). doi:10.1007/978-3-540-38507-3_8
Wright, A. F., Chakarova, C. F., Abd El-Aziz, M. M., and Bhattacharya, S. S. (2010). Photoreceptor Degeneration: Genetic and Mechanistic Dissection of a Complex Trait. Nat. Rev. Genet. 11 (4), 273–284. doi:10.1038/nrg2717
Xiao, M., and Hendrickson, A. (2000). Spatial and Temporal Expression of Short, Long/medium, or Both Opsins in Human Fetal Cones. J. Comp. Neurol. 425 (4), 545–559. doi:10.1002/1096-9861(20001002)425:4<545::aid-cne6>3.0.co;2-3
Yamada, E. (1969). Some Structural Features of the Fovea Centralis in the Human Retina. Arch. Ophthalmol. 82 (2), 151–159. doi:10.1001/archopht.1969.00990020153002
Yamaguchi, T., Motulsky, A. G., and Deeb, S. S. (1997). Visual Pigment Gene Structure and Expression in Human Retinae. Hum. Mol. Genet. 6 (7), 981–990. doi:10.1093/hmg/6.7.981
Yassin, S. A., Al-Dawood, A. J., Al-Zamil, W. M., Al-Ghamdi, M. A., and Al-Khudairy, Z. N. (2019). Comparative Study of Visual Dysfunctions in 6-10-Year-Old Very Preterm- and Full-Term-Born Children. Int. Ophthalmol. 39 (7), 1437–1443. doi:10.1007/s10792-018-0959-2
Young, R. W. (1967). The Renewal of Photoreceptor Cell Outer Segments. J. Cel Biol 33 (1), 61–72. doi:10.1083/jcb.33.1.61
Yuodelis, C., and Hendrickson, A. (1986). A Qualitative and Quantitative Analysis of the Human Fovea during Development. Vis. Res. 26 (6), 847–855. doi:10.1016/0042-6989(86)90143-4
Zhang, T., Zhu, L., Madigan, M. C., Liu, W., Shen, W., Cherepanoff, S., et al. (2019). Human Macular Müller Cells Rely More on Serine Biosynthesis to Combat Oxidative Stress Than Those from the Periphery. Elife 8. doi:10.7554/eLife.43598
Zhong, X., Gutierrez, C., Xue, T., Hampton, C., Vergara, M. N., Cao, L.-H., et al. (2014). Generation of Three-Dimensional Retinal Tissue with Functional Photoreceptors from Human iPSCs. Nat. Commun. 5, 4047. doi:10.1038/ncomms5047
Keywords: retina, photoreceptor, cone, rod, human, macula, thyroid hormone, retinoic acid
Citation: Hussey KA, Hadyniak SE and Johnston RJ (2022) Patterning and Development of Photoreceptors in the Human Retina. Front. Cell Dev. Biol. 10:878350. doi: 10.3389/fcell.2022.878350
Received: 17 February 2022; Accepted: 25 March 2022;
Published: 14 April 2022.
Edited by:
Tom Reh, University of Washington, United StatesReviewed by:
Patrick W. Keeley, University of California, Santa Barbara, United StatesMichel Cayouette, Montreal Clinical Research Institute (IRCM), Canada
Copyright © 2022 Hussey, Hadyniak and Johnston. This is an open-access article distributed under the terms of the Creative Commons Attribution License (CC BY). The use, distribution or reproduction in other forums is permitted, provided the original author(s) and the copyright owner(s) are credited and that the original publication in this journal is cited, in accordance with accepted academic practice. No use, distribution or reproduction is permitted which does not comply with these terms.
*Correspondence: Robert J. Johnston, Jr., cm9iZXJ0am9obnN0b25Aamh1LmVkdQ==
†These authors have contributed equally to this work