- 1Hunan Cancer Hospital and the Affiliated Cancer Hospital of Xiangya School of Medicine, Central South University, Changsha, China
- 2Hypertension Center, FuWai Hospital, State Key Laboratory of Cardiovascular Disease, National Center for Cardiovascular Diseases, Peking Union Medical College, Chinese Academy of Medical Sciences, Beijing, China
- 3Department of Dermatology, National Clinical Research Center for Geriatric Disorders, Xiangya Hospital, Central South University, Changsha, China
- 4The Key Laboratory of Carcinogenesis of the Chinese Ministry of Health, Cancer Research Institute and School of Basic Medical Sciences, Central South University, Changsha, China
- 5The Key Laboratory of Carcinogenesis and Cancer Invasion of the Chinese Ministry of Education, Cancer Research Institute and School of Basic Medical Sciences, Central South University, Changsha, China
Chemotherapy is one of the primary treatments for most human cancers. Despite great progress in cancer therapeutics, chemotherapy continues to be important for improving the survival of cancer patients, especially for those who has unresectable metastatic tumors or fail to respond to immunotherapy. However, intrinsic or acquired chemoresistance results in tumor recurrence, which remains a major obstacle in anti-cancer treatment. The high prevalence of chemoresistant cancer makes it urgent to deepen our understanding on chemoresistance mechanisms and to develop novel therapeutic strategies. Multiple mechanisms, including drug efflux, enhanced DNA damage reparability, increased detoxifying enzymes levels, presence of cancer stem cells (CSCs), epithelial mesenchymal transition (EMT), autophagy, ferroptosis and resistance to apoptosis, underlie the development of chemoresistance. Recently, accumulating evidence suggests that lipid metabolism alteration is closely related to drug resistance in tumor. Targeting lipid metabolism in combination with traditional chemotherapeutic drugs is a promising strategy to overcome drug resistance. Therefore, this review compiles the current knowledge about aberrant lipid metabolism in chemoresistant cancer, mainly focusing on aberrant fatty acid metabolism, and presents novel therapeutic strategies targeting altered lipid metabolism to overcome chemoresistance in cancer.
1 Introduction
Cancer is fundamentally a disorder of uncontrolled cell growth, continual unregulated proliferation, blockade of differentiation and resistant to cell death caused by genetic and epigenetic abnormalities (Greaves and Maley, 2012). Despite great progress in diagnostic methods and therapeutic strategies, therapy failure leads to the death of a large number of patients. Besides immunotherapy which is another new approach that stimulates immune cells to enhance their anticancer activity, chemotherapy remains to be one of the primary methods for cancer treatment. There are different kinds of chemotherapeutic agents including alkylators, antibiotics, antimetabolites, topoisomerases inhibitors, mitosis inhibitors and others (Espinosa et al., 2003; Pan et al., 2016). However, apart from the cell toxicity for normal cells, the effect of chemotherapeutic treatments is limited due to chemoresistance, known as multidrug resistance (MDR). It is broadly recognized that drug resistance is the main cause of tumor recurrence and a major obstacle against tumor control. Overall, there exist two types of chemoresistance in cancer: one is intrinsic drug resistance which is mainly due to high cellular heterogeneity within the tumor bulk and the other is acquired drug resistance which is often induced by therapy (Pan et al., 2016). Until recently, several mechanisms of drug resistance have been highlighted (shown in Figure 1), including increased drug efflux, altered cell cycle, enhanced DNA damage repairmen, insensitivity to death stimuli and tumor dormancy (Szakacs et al., 2006; Assaraf et al., 2019). Notably, metabolic reprogramming has been emphasized to be a novel characteristic preventing cancer cells from chemotherapy-induced death (Hirpara et al., 2019). Aberrant lipid metabolism observed in chemoresistant malignant cells plays an important role in cancer survival and poor prognosis (Wang T. et al., 2018; Lee M. Y. et al., 2018). However, we are far away from comprehensive understanding the underlying mechanisms. Besides, here exists a strong need to identify novel therapeutic strategies to treat chemoresistant tumors. Hence, this review highlights the current knowledge about the role of aberrant lipid metabolism mainly focusing on fatty acids (FAs) in cancer chemoresistance and provides novel strategies targeting specific enzymes or other pathways.
2 Mechanisms Involved in Chemoresistance
2.1 Increased Drug Efflux
Enhanced drug efflux is one of the most widely studied mechanisms contributing to drug resistance, which is mainly regulated by the ATP binding cassette (ABC) superfamily to reduce the intracellular drug levels (Eckford and Sharom, 2009). The ABC transporters contain seven sub-families designated A to G (Kathawala et al., 2015). Members of ABC transporters are frequently overexpressed in human cancers, which links to drug resistance and unfavorable clinical outcome (Fletcher et al., 2010). For instance, ABCF2 overexpressed in cisplatin-resistant ovarian cancer cells (Bao et al., 2017) and ABCC5 overexpressed in gemcitabine-resistant pancreatic cancer cells (Hagmann et al., 2010) inducing the poor prognosis of the treatments. Selonsertib (GS-4997), targeting apoptosis signal-regulating kinase 1 (ASK1), reversed chemoresistance by suppressing drug efflux activity of ABCB1 and ABCG2 transporters (Ji et al., 2019). This study showed that selonsertib interacted with substrate-binding sites to inhibit efflux function and increase intracellular accumulation of anticancer drugs thereby sensitizing cancer cells.
2.2 DNA Damage Repairment
Alkylating agents such as platinum agents kill cancer cells by inducing DNA damage (Jeggo et al., 2016). DNA damage repair is utilized by cells to overcome the multiple DNA damages occurring endogenously and exogenously, which is also the strategy for cancer to adapt and resist to the chemotherapies. For instance, up-regulation of RAD6, a ubiquitin-conjugating enzyme regarded as a DNA repair protein, facilitated DNA damage repair and induced carboplatin-resistance (Somasagara et al., 2017). Rac1, a GTP binding protein, also triggered DNA damage repair by increased nucleotides metabolism to support neoadjuvant chemotherapy (NAC) resistant breast tumors, while silencing Rac1 expression by nanoparticles resensitized the cancer cells (Li et al., 2020). As a crucial mechanism of cancer chemoresistance, DNA repair capacity is considered as a hallmark to predict the prognosis of cancer patients.
2.3 Altered Cell Cycle
The dysregulation of cell cycle is intensively involved in tumorigenesis and cancer chemoresistance. On the one hand, cell cycle arrest often leads to insensitivity of tumor chemotherapy. Though fast-dividing cancer cells give anti-cancer drugs more opportunities to contact and damage the nuclear DNA (Zhao, 2016), cancer cell is likely to stay quiescent and enter G0 phase to avoid being killed by anti-cancer drugs. It is considered to be one of the reasons why cancer stem cells (CSCs) challenge the treatment of chemotherapy. CSCs are relatively quiescent cells to stay in the “safe” states evading the attack of anti-cancer drugs (Zhao, 2016). On the other hand, the dysregulation at cell cycle checkpoints makes cell lose the ability to prevent genomic instability and halt cell cycle progression, leading to cancer chemoresistance consequently (Horibe et al., 2015).
2.4 Autophagy
Autophagy is a conserved metabolic recycling mechanism intensively implicated in cancer development (Levy et al., 2017). Autophagy may exert a tumor suppressive role at early stage by eliminating damaged organelles and protein aggregates, safeguarding genome stability. Paradoxically, when a tumor occurs, autophagy has the opposite effects (Levy et al., 2017). Cancer cells often have higher basal level of autophagy when compared to its normal counterparts. Under the stress condition induced by chemotherapy in tumor cells, autophagy usually appears to have effects on promotion of cell survival and resistance of anti-cancer drugs (Huang et al., 2016). Verteporfin, an autophagy inhibitor, sensitizes pancreatic ductal adenocarcinoma to gemcitabine, suggesting that inhibition of autophagy is a promising approach to overcome chemoresistance (Donohue et al., 2013).
2.5 Epithelial Mesenchymal Transition
Epithelial mesenchymal transition (EMT) refers to a biological process originally observed during embryogenesis, in which epithelial cells turn into mesenchymal phenotype cell by specific program (Lamouille et al., 2014). EMT presents in cancer cells to promote stemness, migration, invasion and dissemination (Ye and Weinberg, 2015). Whereas, mesenchymal-epithelial transition (MET), the reverse process of EMT, induced the cessation of migration and facilitated metastatic colonization of cancer cells to form the new tumor (Nieto et al., 2016). Transcriptional and translational/post-translational control regulates EMT at multilayer levels mainly including SNAIL, TWIST and ZEB families (Brabletz et al., 2018). Emerging evidence suggests that EMT contributes to chemoresistance in cancers. In pancreatic ductal adenocarcinoma, EMT program was found to protect cancer cells from cytotoxicity of gemcitabine by suppressing proliferation and drug transporters, therefore inducing chemoresistance (Zheng et al., 2015). EMT is also considered to play an important role in the formation of a stem cell phenotype (Alison et al., 2012). In brief, EMT regulates chemoresistance by promotion of CSCs signaling pathways, avoidance of drug-induced apoptosis and microenvironment remodeling (Du and Shim, 2016).
2.6 Cancer Stem Cells
Cancer stem cells (CSCs) are sub-populations of cells which possess the ability of self-renewal and differentiation, appearing in most tumors (Alison et al., 2012). CSCs have the ability to undergo asymmetric cell division or symmetrical division to facilitate heterogeneity in cancer (Alison et al., 2012). Importantly, CSCs, considered as the “root of cancer” to promote cancer progression and metastasis, are responsible for chemoresistance and poor prognosis in cancer treatments. For instance, pancreatic cancer (Cioffi et al., 2015), hepatic cancer (Govaere et al., 2016) and colorectal cancer (Fesler et al., 2017) were found chemoresistance associated with CSCs.
2.7 Refractory to Apoptosis/Cell Death Stimuli
Apoptosis is an intrinsic programmed cell death process contributing to the regulation of important physiological and pathological processes. In apoptosis, activation of caspases, breakdown of DNA and protein and changes of membrane are main biochemical changes (Wong, 2011). Both intrinsic mitochondrial pathway and extrinsic death receptor pathway activate caspases which are both initiators and executioners in the mechanism of apoptosis (Wong, 2011). Apoptosis is recognized as a positive strategy to induce death of cancer cells (Ichim and Tait, 2016). However, malignant cells evade apoptosis to facilitate carcinogenesis and chemoresistance. The inhibition of caspases, suppression of death receptor signaling and disturbed balance of apoptotic proteins including BcI-2 proteins, Mcl-1 proteins, p53 protein, inhibitor of apoptosis proteins (IAPs) are responsible for cancer development and chemoresistance (Wong, 2011; Mohammad et al., 2015; Ichim and Tait, 2016). For instance, cisplatin-resistant ovarian cancer (Yang et al., 2008) and oxaliplatin-resistant colorectal cancer (Choi et al., 2018) was attributed to inhibition of apoptosis-induced death thereby resulting in chemoresistance.
2.8 Tumor Dormancy
Tumor dormancy is recently recognized as a tactics of malignant cells to survive, recur and metastasize. In the dormancy state, cancer cells are clinically undetectable, undergoing G1–G0 growth arrest and surviving the exposure to cytotoxic drugs (Yumoto et al., 2014). The dormancy is also a characteristic of self-renew cancer cells like cancer stem sell. Dormancy-competent cancer stem cells are proposed as a neoplastic subpopulation controlling alternative of dormancy and growth (Crea et al., 2015). Remaining in dormancy period suggests these cells are able to regulate their cell cycle. Besides, angiogenic switch, immune escape and cellular dormancy mechanisms contribute to tumor dormancy (Yumoto et al., 2014; Jahanban-Esfahlan et al., 2019). Strikingly, the microenvironment changes play a pivotal role in reactivating dormant cancer cell after chemotherapy to develop chemoresistance (Yumoto et al., 2014; De Angelis et al., 2019). Disturbing their niche to wake up dormant cells then killed by anticancer drugs or to sustain tumor dormancy infinitely is considered to be a strategy to defeat tumor-dormancy-induced chemoresistance (Yumoto et al., 2014; De Angelis et al., 2019).
3 Metabolic Reprogramming in Cancer
Metabolic reprogramming is one of emerging hallmarks of cancer (Hanahan and Weinberg, 2011). Tumor cells reside in a microenvironment characterized by hyper-oxidative, hypoxic, acidic and nutrient-poor conditions (Najafi et al., 2019). Metabolic alterations benefit malignant cells to adapt to microenvironment to maintain their survival and growth (Pavlova and Thompson, 2016). More than a century ago, Otto Warburg observed that tumor cells preferred glycolysis to mitochondrial oxidative phosphorylation (OXPHOS) even in oxygen rich conditions, a phenomenon named aerobic glycolysis (Warburg, 1956b; a). This perturbed metabolism imparts tumor cells to make a balance between energy supply and accumulation of glycolytic intermediates for building blocks and NADPH production (Koppenol et al., 2011). Importantly, Warburg effect leads to chemoresistance through epigenetic and genetic changes to activate survival pathway and prevent cell death (Icard et al., 2018). In addition to glucose metabolism alterations, the reprograming of lipid metabolism and perturbation of amino acids metabolisms are also intensively implicated in cancer development and progression (Pavlova and Thompson, 2016). Glutamine, an abundant amino acid in the body and a central precursor for protein in proliferating cells, plays important roles in maintaining intestinal function and amino acid homeostasis (Cluntun et al., 2017). In cancer cells, deregulated uptake of glutamine is a hallmark that cancer cells display a higher consumption of glutamine to satisfy their proliferation (Pavlova and Thompson, 2016). Interestingly, there is an underlying association among glycolysis, rewired amino acids metabolism and altered lipid metabolism which are still being elucidated. For instance, the enzymes in Warburg effect have been reported to be involved in the aberrant lipid metabolism. Pyruvate kinase M2 (PKM2), a major glycolytic enzyme in Warburg effect facilitating chemoresistance (Li et al., 2015; Shanmugasundaram et al., 2017), interacts with sterol regulatory element-binding proteins (SREBPs) to increase nuclear SREBP-1a’s abundance and activate lipogenic gene expression as well as lipid biosynthesis subsequently (Zhao et al., 2018). Besides, altered amino acids metabolism was regulated by transcription factor MYC (Wise et al., 2008), which is also attributed to lipid metabolism reprogramming (Eberlin et al., 2014). Moreover, intermediate substances associate the Warburg effect with the lipid biosynthesis. Glucose metabolism imports citrate to FA metabolism, which is the intersection of the two major metabolic pathways as an intermediate in the Krebs cycle (Currie et al., 2013). A new thesis of mitochondrial OXPHOS and respiration highlighted the significant role of mitochondria in resistant cancer cells (Bosc et al., 2017). The dysfunction of the mitochondria, as a signaling center converging most catabolic and anabolic pathways, contributed to cell survival and chemoresistance.
In terms of lipid metabolism, among the diverse categories of lipid, FA is regarded to be fundamentally involved in the development of malignant biological properties in cancer cells (Currie et al., 2013). In the process of lipid biosynthesis, citrate needs several steps to convert carbons to bioactive FAs. Then, the free FA is esterified to triacylglycerol (TAG) and storage in lipid droplets (LDs). As an integral part of metabolism, lipid metabolism, especially aberrant FA metabolism, is emphasized in tumorigenesis and cancer chemoresistance.
4 Fatty Acid Metabolism and Chemoresistance
4.1 Lipid Biosynthesis
The lipid biosynthesis is an intricate process which is controlled by complex, cross-linked regulatory networks including enormous regulatory factors and regulatory pathways (Grunt, 2018). Enhanced lipogenesis contributes to cancer growth, metastasis and acquisition of drug resistance resulting in unfavorable clinical outcome in cancer patients. Activation of FA synthesis in cancer can be achieved by transcriptional induction. Sterol regulatory element binding proteins (SREBPs) is a master regulators in lipogenetic regulation in cancer (Shao and Espenshade, 2012). SREBPs transcriptionally activates enzymes involved in lipogenesis processes in cancer cells (Liu et al., 2017), including ATP citrate lyase (ACLY), acetyl-CoA carboxylase (ACC), fatty acid synthase (FASN), and acyl-CoA synthetase (ACS) which play significant roles in the process of lipid biosynthesis (Currie et al., 2013) (seen Figure 2). These enzymes are potentially involved in the interaction between the altered lipogenesis and chemoresistance (Roehrig and Schulze, 2016). So far, several attempts are being made to focus on the rewired lipid biosynthesis in order to overcome cancer chemoresistance.
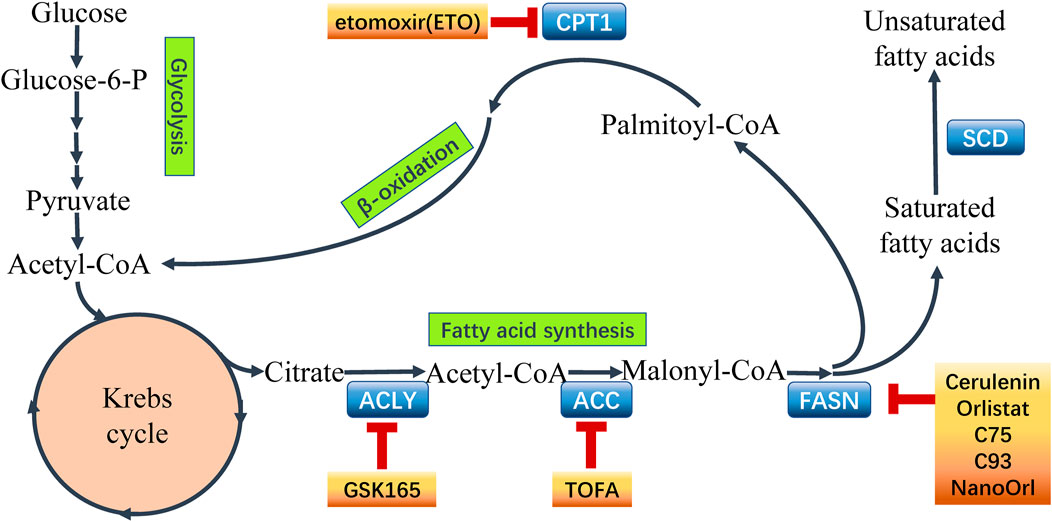
FIGURE 2. Fatty acid metabolism and points of intervention to overcome chemoresistance. Fatty acid metabolism plays a critical role in chemoresistant cancer cells. Aberrant metabolism contributes to the survival and proliferation of malignant cells. Importantly, the key enzymes catalyzing the processes in fatty acid metabolism have been recognized to express abnormally in cancer chemoresistance. Hence, it is a novel strategy using inhibitors to target the key enzymes to intervene the aberrant metabolism and overcome chemoresistance in cancer. Some common inhibitors of the key enzymes are shown above. ATP-citrate lyase (ACLY), acetyl-CoA carboxylase (ACC), fatty acid synthase (FASN), stearoyl-CoA desaturase (SCD), carnitine palmitoyltransferase 1 (CPT1).
4.1.1 ACLY
ACLY is the rate-limiting enzyme for the first-step of de novo lipogenesis. It is a cytosolic homotetrameric enzyme that catalyzes mitochondrial derived citrate into acetyl CoA, the precursor of FA synthesis, thus connecting glucose metabolism to lipid metabolism (Zaidi et al., 2012). ACLY is reported to be up-regulated in many cancer cells such as glioblastoma (Beckner et al., 2010), breast cancer (Lucenay et al., 2016; Wang et al., 2017), colorectal cancer (Zhou et al., 2013), osteosarcoma (Xin et al., 2016), prostate cancer (Xin et al., 2016), cervical cancer (Xin et al., 2016) and lung cancer (Xin et al., 2016), indicating overexpression of ACLY is a hallmark of cancer. Inhibition of ACLY by RNA interference or chemical inhibitor SB-204990 inhibited cancer proliferation (Hatzivassiliou et al., 2005). Activation of Akt pathway may either directly activate ACLY by phosphorylating ACLY protein (Potapova et al., 2000; Berwick et al., 2002), or transcriptionally enhance the expression level of ACLY by SREBP (Porstmann et al., 2005), thus triggering the production of cytosolic acetyl-CoA for lipogenesis and protein acetylation reactions (Hoxhaj and Manning, 2020). The mTORC2/AKt/ACLY pathway (Martinez Calejman et al., 2020) and the PI3K/Akt/ACLY pathway (Lee et al., 2014) are involved in histone acetylation, which plays important roles in gene regulation and DNA damage repair dependent on acetyl-CoA provided by ACLY (Sivanand et al., 2017). The ACLY is highlighted in histone acetylation and carcinogenesis (Carrer et al., 2019), indicating that oncogenic metabolic reprogramming regulated the epigenome (Lee et al., 2014). ACLY is also responsible for increased cancer stemness (Hanai et al., 2013; Zhang et al., 2022), cancer cell adhesion and migration (Lee J. V. et al., 2018; Wen et al., 2019). These characteristics render cancer cells capability in growth as well as chemoresistance.
Zhou et al. revealed that upregulation of ACLY confers colorectal cancer cells resistance to SN38, an active ingredient converted from irinotecan (Zhou et al., 2013). ACLY promotes resistance to MAPK inhibitors by increased histone acetylation facilitating MITF-PGC1α axis transcription in melanoma (Guo et al., 2020). In castration-resistant prostate cancer cells, combining androgen receptor (AR) antagonist with ACLY inhibition promoted AMPK activation and lead to suppression of AR levels to induce apoptosis (Shah et al., 2016). Besides, blocking ACLY sensitized cancer cells to chemotherapy by suppressing stem cell features (Hanai et al., 2013). Due to the complicated association between ACLY and signal pathways, combinatory targeting ACLY with other critical molecules may be more effective than using ACLY knockdown alone (Zhou et al., 2013). For example, inhibiting ACLY alone was unefficient to restore chemo-sensitivity to SN38 since compensatory activation of AKT pathway was induced when ACLY was suppressed (Zhou et al., 2013). Combining ACLY inhibition with tyrosine kinase or PI3K/Akt inhibitors could notably abrogate the effects of PI3K/Akt activation in metabolism (Hatzivassiliou et al., 2005). Dual blockade of MAPK and PI3K/AKT pathways by combining ACLY knockdown and statin treatment sensitized EGF receptor resistant in non-small cell lung cancer (NSCLC) (Hanai et al., 2012).
4.1.2 FASN
FASN is the enzyme catalyzing the terminal steps in the de novo biosynthesis of long-chain FAs (Menendez and Lupu, 2007). FASN consumes acetyl-CoA, malonyl-CoA and NADPH to catalyze the biosynthesis of palmitate, sustaining altered metabolic state for cancer growth and survival (Jones and Infante, 2015). Palmitate is an important product in de novo FA synthesis which possesses vital biological function (Menendez and Lupu, 2007). Palmitate and additional FAs are considered precursors of cellular lipid synthesis for membrane fluidity and function, and substrates of post-translational protein regulation for protein localization and activity (Ventura et al., 2015). Palmitate interacts with lipid rafts, which are specialized plasma membrane microdomains rich in lipid-modified membrane-associated proteins and lipids, to play important roles in receiving, localizing, and transmitting (Simons and Sampaio, 2011). FASN inhibition may affect membrane structure such as lipid rafts, thus disrupting signal transduction networks and biological processes. However, activation of FASN is a hallmark of cancer contributing to tumor growth, invasion and stemness (Yasumoto et al., 2016).
Dysfunction of FASN is reported as a new characteristic in chemoresistance in malignant cells. Studies demonstrated that FASN levels were increased in paclitaxel-resistant hepatocellular carcinoma (HCC) (Meena et al., 2013), taxane-resistant prostate cancer (Souchek et al., 2017), cisplatin-resistant squamous cell carcinoma (Huang et al., 2012), paclitaxel-resistant laryngeal cancer (Xu et al., 2013), gemcitabine-resistant pancreatic cancer (Yang et al., 2011) and carboplatin/paclitaxel-resistant ovarian cancer (Ueda et al., 2010).
In resistant breast cancer cells, increased FASN-catalyzed endogenous FA biogenesis was related to HER2 overexpression (Vazquez-Martin et al., 2008; Puig et al., 2011; Ligorio et al., 2021). In trastuzumab-resistant HER2-positive breast cancer cells, overexpression of FASN was facilitated by Pin1 via regulating EGF signaling pathway to activate the sterol regulatory element-binding protein-1c (SREBP1c) promoter (Yun et al., 2014). Inducing SREBP-1 gene to activate the Akt and HIF1 in breast cancer also increased the expression of FASN, which partly conduced to hypoxia-induced chemoresistance to cyclophosphamide (Furuta et al., 2008). Simultaneous blocking FASN and HER2 pathways showed significantly anti-tumor effect for breast cancer cells resistant to anti-HER2 drugs (Blancafort et al., 2015). In addition, FASN promotes cancer chemoresistance by regulation of apoptosis. FASN overexpression caused palmitate overproduction, thus supporting anti-apoptosis (Liu et al., 2008). Also, FASN overexpression inhibited TNF-α expression, which in turn suppressed NF-κB as well as neutral sphingomyelinase (nSMase) to reduce ceramide production, suppress caspase 8 activation, and inhibit apoptosis (Liu et al., 2013).
In gastric cancer cells, MaCC1, the upstream regulator of FASN, decreased the chemosensitivity to oxaliplatin by enhancing the expression of FASN (Duan et al., 2017). Moreover, in cisplatin-resistant NSCLC, increased FASN expression was regulated by a FASN-TGFβ1-FASN positive feedback loop which mediated epithelial mesenchymal transition (EMT) (Yang et al., 2016). Activation of EGFR/FASN-Akt signaling axis was found in acquired TKI-resistant EGFR mutated NSCLC cells (Ali et al., 2018). In addition to EGFR mutated cancer cells, Akt pathway was related to chemoresistance in FASN-overexpressed gastrointestinal stromal tumors (GISTs) with KIT mutated and the activation of oncogenic pathway like PI3K/AKT/mTOR pathway promoted chemoresistance to imatinib (Li et al., 2017). In gemcitabine-resistant pancreatic cancer cells, a notably increase in FASN expression was closely associated with regulating ER stress, which resulted in apoptosis, and stemness (Tadros et al., 2017). In vemurafenib-resistant BRAF-mutant melanoma, active SREBP-1, the downstream target of BRAF signaling, was the characteristic to sustain lipogenesis and up-regulate the expression of FASN (Talebi et al., 2018).
Targeting FASN by FASN inhibitors is useful to sensitize cancer cells to chemotherapies (seen Figure 2). Since the FASN oncogenicity involves versatile molecules in anti-apoptosis, lipid raft function and central oncogenic pathway activation (Li et al., 2017), the inhibition of FASN can regulate important biological processes. For instance, PIK3/AKT/mTOR pathway, β-catenin signaling and expression of oncogenic effectors like c-Myc were inhibited by FASN inhibitors (Ventura et al., 2015; Li et al., 2017). Proton pump inhibitors (PPIs) effectively suppressed FASN to induce apoptosis and sensitized to doxorubicin in breast cancer (Wang et al., 2021). Meanwhile, through FASN inhibition, the perturbation of lipid raft, an important membrane structure, destroyed the signal transduction networks to affect cell growth and death (Ventura et al., 2015; Li et al., 2017). New FASN inhibitors with less in-vivo toxicity like TVB-3166 exhibited potent anti-tumor effects in chemoresistant cancer cells (Ventura et al., 2015).
4.1.3 ACC
ACC, including ACC1 and ACC2, catalyzes acetyl-CoA to form malonyl-CoA, which is the rate-limiting step to synthesize long-chain FA (Ventura et al., 2015). AMPK is a major regulator of ACC in cancer development. AMPK phosphorylated transcriptional regulator such as SREBP1c to inhibit ACC activity and control FA synthesis (Steinberg and Carling, 2019). Importantly, the levels of acetyl-CoA and malonyl-CoA affected the initiation of FA synthesis or fatty acid oxidation (FAO) (Carracedo et al., 2013). FAO leaded to the accumulation of cytoplasmic acetyl-CoA needed for the initiation of the FA synthesis (Caro et al., 2012). The ACC-catalyzed production malonyl-CoA was reported to be an allosteric inhibitor of a key enzyme of FAO--CPT1 (McGarry et al., 1983). Additionally, genetic manipulation of ACC1 or ACC2 disturbed the balance of FA synthesis and FAO in cancer cells (Jeon et al., 2012). It was reported that overexpression of ACC1 in NSCLC plays a crucial role in maintaining cancer cell growth and viability (Svensson et al., 2016). Blocking ACC inhibits tumor growth of NSCLC (Svensson et al., 2016).
Recently, ACC is emphasized in cancer chemoresistance. In cisplatin-resistant lung cancer cells which had high ROS and low thioredoxin-1 (TRX1) levels, ACC presented a significantly high basal level (Wangpaichitr et al., 2012). The up-regulation of the antioxidative factor TRX1 caused an attenuated level of ACC (Wangpaichitr et al., 2012). In cetuximab-resistant head and neck squamous cell carcinoma (HNSCC), an increased level of total ACC regulated by post-translational mechanism compensated the AMPK activation-induced ACC inhibition, thus promoting cancer cells to survive the cetuximab (Luo et al., 2017). Co-targeting ACC with TOFA, an ACC allosteric inhibitor, significantly sensitized cetuximab-resistant HNSCC xenografts to cetuximab (Luo et al., 2017).
4.2 Lipid Desaturation
Lipid desaturation is an integral modification in cancer cells to limit lipotoxicity and generate unsaturated FAs (Ackerman and Simon, 2014). Monounsaturated fatty acid (MUFA) is the major product of lipid desaturation to regulate the membrane fluidity, normal cell function and cancer development (Hendrich and Michalak, 2003; Calder, 2015; Igal, 2016). Stearoyl-CoA desaturase (SCD) is the key fatty acyl △9-desaturing enzyme to convert MUFA from saturated fatty acid (SFA). Dysfunction of SCD in cancer yields an altered level of MUFA, which breaks the balance of MUFA/SFA ratio and change the cellular lipid composition, fluidity and signal transduction to promote cell survival and induce cancer chemoresistance. Dietaries involving caloric restriction and a ketogenic diet could impair SCD activity in cancer (Lien et al., 2021).
4.2.1 SCD1
SCD is upregulated in several cancers. Overexpression of SCD enhanced cell viability in breast and prostate cancers (Peck et al., 2016). Elevated level of SCD1 was involved in poor prognosis of bladder cancer (Piao et al., 2018) and clear cell renal cell carcinoma (von Roemeling et al., 2013; Wang et al., 2016). In lung cancer, EGFR-mediated phosphorylation of Y55, a mutant of tyrosine residue, enhanced SCD1 stability and activity, which led to enhanced MUFA synthesis and accelerated cell growth (Zhang et al., 2017). In addition, 17β-estradiol (17β-ED) up-regulated SCD-1 via activating SREBP-1c, which increased MUFA/SFA ratio in estrogen-sensitive breast cancer (Belkaid et al., 2015). Inhibition of SREBP1 down-regulates SCD1, which is a potential approach to treat pancreatic cancer (Siqingaowa et al., 2017). Due to the elevated SCD1 activity, cancer cells contain aberrant higher levels of MUFA, which is considered as a hallmark of cancer manifesting a distinctive transformation of lipogenesis (Igal, 2011). The relative abundance of MUFAs of membrane lipids protects cancer cells from ER stress (Griffiths et al., 2013) and apoptosis (Peck et al., 2016).
Overexpression of SCD1 is also responsible for chemoresistance in malignant cells. Studies revealed that up-regulation of SCD1 contributed to liver tumor-initiating cells and sorafenib resistance by regulating ER stress-mediated differentiation (Ma et al., 2017). FBJ murine osteosarcoma viral oncogene homolog B (FOSB) also mediated the SCD-dependent acquire-resistance in glioblastoma cell lines (Oatman et al., 2021). Inhibiting SCD1 activated ER stress response and enhanced autophagy to overcome chemoresistance in cisplatin-resistant lung cancer stem cells (Pisanu et al., 2017). Furthermore, SCD regulated lipid desaturation contributes to maintaining stemness. For example, SCD1 was identified to be an upstream activator of β-catenin and YAP/TAZ pathways (Pisanu et al., 2017). Higher expression of SCD1 increased the activity of YAP/TAZ, a stem cell pathway, which supported the stemness and adapted to BRAF inhibitor (vemurafenib) and MEK inhibitor (binimetinib) treatments in BRAF-mutated melanoma (Pisanu et al., 2018). Moreover, in TMZ-resistant glioma cells, up-regulation of SCD1 accounted for promoting Akt/GSK3β/β-catenin signaling (Dai et al., 2017). JNK and PI3K signaling and SREBP-1 promoted SCD expression to lead chemoresistance in HCC (Bansal et al., 2014). Interestingly, SCD is involved in ferroptosis. In ovarian cancer cells and stem cells, over-expression of SCD1 assisted cancer cells to survive ferroptosis (Tesfay et al., 2019). In this study, coenzyme Q10, an endogenous membrane antioxidant involved in ferroptosis, was decreased by SCD1 inhibition thus inducing ferroptosis and apoptosis. Ferroptosis is a novel and unique non-apoptotic cell death form, which is different from apoptosis, necrosis and autophagy, involving iron-dependent ROS formation and lipid oxidation (Yang and Stockwell, 2016). Activation of ferroptosis observed in cancer contributed to destroying the cancer cells (Dixon et al., 2012). However, cancer cells were equipped with potential mechanism to survive the ferroptosis (Lee et al., 2020). During ferroptosis, polyunsaturated fatty acids (PUFAs) in cellular membrane react with ROS to induce lipid peroxidation (Xie et al., 2016). Two lipid metabolism-associated enzymes, lysophosphatidylcholine acyltransferase 3 (ACSL4) and lysophosphatidylcholine acyltransferase 3 (LPCAT3) participate in the formation of membrane phospholipids from PUFAs such as arachidonic acid (AA) or adrenic acid (AdA), thus protecting cancer cells against ferroptosis (Chen et al., 2021; Lee et al., 2021). Exogenous MUFAs activated by ACSL3 could inhibit membrane lipid ROS accumulation and suppress ferroptosis in cancer cells (Magtanong et al., 2019). In the context of chemoresistance, ferroptosis plays an important role. Induction of ferroptosis was reported to overcome drug resistance in head and neck cancer, glioblastoma multiforme and colorectal cancer (Kazan et al., 2017). SCD1 was regulated by complicated pathways associating tumor suppressors such as p53 in ferroptosis (Gnanapradeepan et al., 2018; Angeli et al., 2019). The SCD1 inhibition altered the membrane phospholipid composition, thus influencing ferroptosis (Tesfay et al., 2019). The content of MUFA chains could be displaced by increased PUFA chains, thus facilitating membrane lipid oxidation and ferroptosis (Tesfay et al., 2019; Snaebjornsson et al., 2020).
SCD inhibition suppressed cell proliferation and resensitized cancer cells to chemotherapy-induced apoptosis (Bansal et al., 2014). However, to inhibit lipid desaturation, SCD inhibition is not always efficient. Strikingly, during SCD inhibition, other enzyme such as FA desaturase 2 (FADS2) was utilized by cancer cells to fulfil their requirements for MUFA (Vriens et al., 2019). Plus, the uptake of ample exogenous unsaturated FAs compensated the shortage of lipid desaturation by SCD inhibition (Snaebjornsson et al., 2020).
4.3 Lipid Droplets
The lipid droplet (LD), recognized as a multi-functional organelle in most eukaryotic cells, is composed of a neutral lipid core mainly including triacylglycerols (TAGs) and sterol esters (SEs) with a phospholipid monolayer membrane (Farese and Walther, 2009; Walther et al., 2017; Zhang and Liu, 2017). LD fulfills different roles according to cell types and physiological states. It functions as a repository for energy storage and generation, or membrane synthesis (Farese and Walther, 2009; Walther et al., 2017). Accumulation of LDs is emerging as a new hallmark of cancer and cancer stemness.
There exists a close relation between FA metabolism and LDs. The excess FAs generated by lipogenic enzymes are then esterified and stored as TAG and cholesterol esters (CE) in LDs, which help cancer cells evade the harmful effect of the excess free FAs and survive under starvation by liberating FAs from LDs to mobilize into mitochondria for oxidation (Rambold et al., 2015). LD has a complicated relationship with LD-related proteins, enzymes and other organelles such as endoplasmic reticulum (ER), nucleus, mitochondria and peroxisomes (Olzmann and Carvalho, 2019). ER contributes to LD formation by synthesizing and packaging neutral lipids in lens-like structures to bud from its membrane (Barneda and Christian, 2017). The continuity in their membranes supports the growth of LD, which also suggests the direct connection between LD and ER (Barneda and Christian, 2017). This ER-LD contact contributed to the rapid lipid exchange during lipid metabolism between ER and LD (Farese and Walther, 2009). A form of this contact was a membrane bridge which supports connection and allowed TG synthesis enzymes migrate to LD surface (Walther et al., 2017), which may participate in the local synthesis of TG (Farese and Walther, 2009). In addition to the connection with ER, LD interacted closely with mitochondria to transfer FAs for β-oxidation (Rambold et al., 2015).
It has been recently demonstrated that aberrant accumulation of LDs appeared in KRAS and BRAF mutated colon cancer cells, which had poor response to erlotinib, an EGFR-inhibition treatment (Yosef et al., 2015). Besides, progestin facilitated accumulation of LDs in PR+ breast cancers, which in turn caused docetaxel sequestered in enlarged LDs to prevent docetaxel binding to microtubule (Schlaepfer et al., 2012). Similarly, sequestration of prodrug CHR2863 (a hydrophobic aminopeptidase inhibitor) in accumulated LDs suppressed the conversion from CHR2863 to its acid metabolite CHR6768 to promote chemoresistance in myeloid leukemia cells (Verbrugge et al., 2016). Besides, ponatinib, a multi-tyrosine kinase inhibitor, was deposited into increased LDs to influence intracellular pharmacokinetics and then induce chemoresistance in lung cancer (Englinger et al., 2020). Collectively, it has been suggested that drugs with dominant hydrophobicity may be sequestrated into the accumulative LDs to block the cell-killing effects of chemotherapies, which is utilized by cancer cells to accumulate LDs sequestering anti-cancer agents to avoid death. This may be a mechanism for cancer cells to reduce cytoplasmic drug concentrations, and targeting LD integrity will be a novel strategy to deal with the chemoresistance of lipophilic anticancer drugs.
In addition to the hydrophobic characteristic of drugs, the participation of other enzymes, molecules and organelles contributes to LD-associated chemoresistance. In ovarian and cervical cancer, a higher level of LDs was found in resistant cell lines, which was associated with the increased activity of 6-phosphofructo-2-kinase/fructose-2,6-biphosphatase 3 enzyme (PFKFB3), a glycolytic regulator that also regulated a key step to control glycolytic rate by mediating fructose-2,6-bisphosphate (F2,6BP) levels (Mondal et al., 2019). PFK158, a PFKFB3 inhibitor sensitized cancer cells by inducing LDs inhibition, which was related to an up-regulation of autophagic flux to boost lipophagy and induce apoptosis for suppression of tumorigenesis (Mondal et al., 2019). Moreover, an LD-localized enzyme called lysophosphatidylcholine acyltransferase 2 (LPCAT2) was reported to contribute to LD-induced chemoresistance of 5-fluorouracil and oxaliplatin by inhibiting caspase cascade activation as well as ER stress responses mechanistically (Cotte et al., 2018).
Since LDs contain different neutral lipids, it is important to clarify the precise effect of these components and networks. Not only FA metabolism but also cholesterol metabolism is emphasized in LD-induced chemoresistance. It has been shown that enhanced LDs and cholesterol esters were observed in carboplatin-resistant laryngeal carcinoma cells (Rak et al., 2014). Moreover, both increased cholesterol esters and triglycerides accumulating in LDs promoted breast cancer cells to survive the tamoxifen treatment (Hultsch et al., 2018). In this study, downregulated lysosomal protein cathepsin D and enhanced lysosomal-associated-membrane-proteins 1 and 2 suppressed lysosomal membrane permeabilization to prevent tamoxifen-resistant breast cancer cells from lysosomal cell death.
4.4 Lipid Catabolism
Lipid catabolism, also known as β-oxidation, yields ATP, NADH and acetyl-CoA to maintain energy balance, reduce oxidative stress and support (histone) protein acetylation (Grunt, 2018). Enhanced FAO not only increases ATP production but also provides NADH and FADH2 thus rendering metabolic advantages to tumor cells (Carracedo et al., 2013). FAO product Acetyl-CoA enters various metabolic pathways and contributes to FA synthesis, TCA cycle, and protein acetylation (Yoshii et al., 2015). The carnitine palmitoyltransferases (CPTs), two forms of mitochondrial membrane-bound enzymes named CPT1 and CPT2, function to uptake FAs across the mitochondrial membrane and play critical role in the regulation of FAO in cells (seen Figure 2). Recent studies have recognized that FAO occupies a critical position in rewiring FA metabolism to contribute to therapy resistance in cancer cells.
4.4.1 CPT
CPT1 is the key enzyme located on outer mitochondrial membrane to regulate the entry of FAs into the mitochondria via converting FA-CoAs to FA carnitines (Currie et al., 2013). CPT1a, CPT1b and CPT1c are three subtypes of CPT family showing specific tissue distribution (Qu et al., 2016). CPT1 antagonized apoptosis and supported cancer cell to survive the metabolic stress (Qu et al., 2016). CPT1a contributed to metastasis of colorectal cancer cell by anoikis inhibition (Wang Y.-n. et al., 2018). CPT1b was upregulated by phosphatidylinositol transfer protein cytoplasmic 1 (PITPNC1) to promote FAO and anoikis resistance thus facilitating omental metastasis of gastric cancer (Tan et al., 2018). In addition, CPT1 is responsible for cancer chemoresistance. The high levels of CPT-1a in pancreatic ductal adenocarcinoma (PDAC) patients was associated with chemoresistance by rewiring cancer lipid metabolism to escape from energy stress (Luo et al., 2016). Activation of FAO provides cancer cells sufficient energy to survive the metabolic stress induced by chemotherapies. The mammary-adipocyte-derived leptin activated STAT3 signaling to promote stemness and chemoresistance through transcriptionally induction of CPT-1b and activating FAO in breast cancer cells (Wang T. et al., 2018). Silencing STAT3 impaired FAO and inhibited self-renewal, whereas stimulating FAO by bezafibrate rescued self-renewal in breast cancer stem cells (Wang T. et al., 2018). Blocking FAO by CPT1 inhibitor etomoxir re-sensitized breast cancer cells to chemotherapy, and inhibited BCSCs in mouse breast tumors in vivo (Wang T. et al., 2018). Furthermore, using etomoxir suppressed hypoxia-activated-FAO pathway in anti-angiogenic drug (AAD) treated tumors, and it was considered that the loss of CPT1a function enhanced AAD sensitivity in steatotic liver cancer (Iwamoto et al., 2018) (seen Figure 2).
Compared with CPT1, CPT2 is little known. CPT2 converts FA carnitines back to FA-CoAs located inside the matrix (Lodhi and Semenkovich, 2014). As the other genetically distinct mitochondrial membrane-bound enzyme regulating FAO, CPT2 appears to play a controversial role in chemoresistance. Lower expression of CPT2 is associated with tumor histological differentiation and venous invasion (Lin et al., 2018). Silencing CPT2 not only enhances the tumorigenic activity and metastatic potential, but also induces chemoresistance to cisplatin in hepatoma cells (Lin et al., 2018). Decrease of CPT2 leads to upregulation of SCD1 and enhanced lipogenesis in HCC cells, indicating that CPT2 may be a key regulator in SCD1-mediated lipid metabolism in drug resistant cancer cells (Lin et al., 2018). To explain the contradiction that CPT1 and CPT2, which are both key enzymes to promote FAO, play opposite roles in cancer chemoresistance, it is speculated that in some certain conditions CPTs are suppressed to attenuate lipid degradation and maintain sufficient lipid for cancer progression (Lin et al., 2018), although the mechanism is unclear. Conversely, in oxaliplatin-treated gastrointestinal cancer cells, higher expression of CPT2 resulted in poor chemotherapy outcomes (Wang et al., 2020). Mechanistically, nuclear factor of activated T cells 3 (NFATc3) promoted CPT2 expression transcriptionally, and inhibiting CPT2 resensitized cancer cells to oxaliplatin (Wang et al., 2020).
Importantly, Chia-Lin Chen et al. demonstrated that NANOG, reprogramming of mitochondrial metabolism, contributed to human tumor-initiating stem-like cells (TICs) oncogenicity and chemoresistance (Chen et al., 2016). NANOG, which mediated oncogenic pathways including stimulating fatty acid oxidation (FAO) and suppressed oxidative phosphorylation (OXPHOS) through mitochondrial metabolic reprogramming, inhibited chemotherapy-induced apoptosis by suppressing ROS production to promote chemoresistance of tumor-initiating stem-like cells (TICs) (Chen et al., 2016). Moreover, in CD36+ chemoresistant leukemic stem cells (LSCs), a higher FAO rate was found, indicating a close relationship between FAO and chemoresistance (Ye et al., 2016). Besides, Thomas Farge et al. illustrated that CD36–FAO–OXPHOS axis in AML was responsible for cytarabine (AraC) resistance, with an upregulation of CD36 and FAO to maintain high OXPHOS state to survive the chemotherapy (Farge et al., 2017).
In addition to CPT1 and CPT2, master factors involved in lipid metabolism, including peroxisome proliferator-activated receptors (PPARs) and peroxisome proliferator-activated receptor gamma co-activator-1 (PGC-1), are associated with chemoresistance via modulating FAO. There are three isoforms of PPARs, including alpha, beta/delta and gamma belonging to the nuclear receptor superfamily which are modulated differentially by endogenous ligands, drugs and other xenobiotics (Peters et al., 2012). In terms of the role of PPARs in cancer development, it remains controversial that PPARs promote or inhibit cancer growth under certain conditions with complex underlying mechanisms [reviewed in Peters et al. (2012), Peters et al. (2015)]. PPARα was found to regulate the transcription of CPT II gene (Barrero et al., 2003), which confirmed that PPARs have an important effect on controlling FAO enzymes expression. In glucocorticoid (GC)-resistant chronic lymphocytic leukemia (CLL), GCs enhanced PPARα expression and stimulated PPARα-mediated FAO, promoting cell survival upon metabolic stress (Tung et al., 2013). Besides PPARs, the nuclear cofactors regulating mitochondrial oxidative metabolism including transcriptional co-activators PGC-1 family also contribute to cancer chemoresistance. For instance, PML-PGC-1α axis was activated by oxidative stress in high-grade serous ovarian cancer (HGSOC) with high-OXPHOS features, which promoted the sensitivity to conventional chemotherapies (Gentric et al., 2018). PGC-1α promoted metformin-resistance in lung metastatic breast cancer and stimulated bioenergetic flexibility (Andrzejewski et al., 2017).
5 Strategies Targeting FA Metabolism to Overcome Chemoresistance
Recent investigation into the altered FA metabolism in chemoresistant cancer cells (seen Figure 3) has revealed a new insight to address cancer therapy by targeting these key enzymes and pathways in different processes of FA metabolism (seen Figure 2). A summary of the small molecules targeting the key enzymes in FA metabolism are shown in Supplementary Table S1.
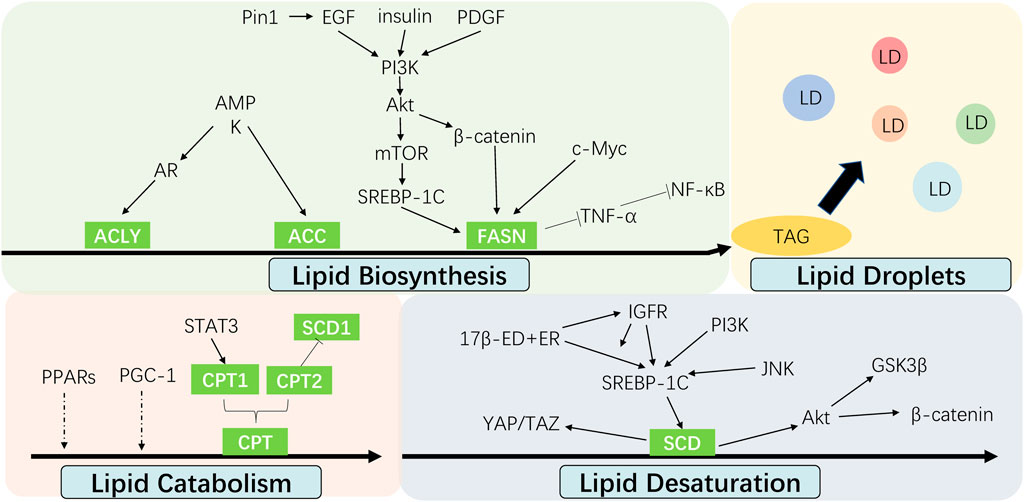
FIGURE 3. Interaction between cell signalings and altered fatty acid metabolism in cancer chemoresistance. The alteration of fatty acid metabolism including lipid biosynthesis, lipid desaturation, lipid droplets formation and lipid catabolism contributes to cancer chemoresistance, which are regulated by multiple signal molecules.
Apart from the chemotherapy, immunotherapy is a breakthrough for cancer treatment, which activates natural defenses to eliminate malignant cells (Zhang and Zhang, 2020). PD-1/PD-L1 antibody and chimeric antigen receptor T cells therapy (CART) are immunotherapies developed and approved for clinical use (O'Donnell et al., 2019; Riley et al., 2019; Kennedy and Salama, 2020). It also shed new light into conventional chemotherapeutics. Cioccoloni et al. (2020) found that the overexpressed FASN was associated with the increased PD-L1 expression in a human T-cell leukemia line, and blocking the FASN by Orlistat impaired the expression of the PD-L1. PD-L1 with highly palmitoylation was observed in cisplatin-resistant BC bladder cancer cells (Shahid et al., 2020). The expression and palmitoylation of FASN could increase PD-1 expression and palmitoylation (Shahid et al., 2020). These close associations between lipid metabolism and PD-L1 expression have opened up the opportunity to use the combination of chemotherapies and immunotherapies to overcome cancer chemoresistance.
To improve the bioavailability and tolerance of drugs, recent advances in nanotechnology promote the development of nanoparticle-based drug delivery systems, which is widely used in cancer chemotherapy (Lepeltier et al., 2020; Su et al., 2021). Nanoparticles have advantages including good biocompatibility, low toxicity, long circulation time, controlled release, tumor targeting ability, etc., which is a promising strategy to treat chemoresistant cancer precisely. Additionally, exosomes, the natural subcellular vesicles, is recognized as novel drug carriers. The modified exosomes that wrapping small-molecule chemical drugs can reach the target cells to achieve personalized medicine (Liu et al., 2021). Dan Lin et al. found that iRGD-modified exosomes reversed oxaliplatin resistance of colon by the successful targeted delivery of CPT1A siRNA (Lin et al., 2021).
6 Conclusion and Perspective
Lipid metabolism alteration in cancer has provided opportunities for cancer treatment. This review focus on the current knowledge of altered lipid metabolism especially the fatty acids metabolism. We have exhibited various experimental findings and theoretical arguments to show that the alteration of lipid metabolism has crucial effect on chemoresistance in cancer cells. However, many elusive challenges still exist due to the unclear mechanism and the pitfalls of these inhibitors. First, different cancer types may present different sensitivity to the inhibitors, which need biomarkers to predict sensitivity in chemoresistant malignant cells to guide the treatment. The high plasticity of the metabolic network in chemoresistant cancer also bother the treatment by inducing compensatory routes of biosynthesis to maintain survival status, which emphasizes the advantage of combination of multiple treatment targets or approaches. In addition, although some molecules have entered clinical trials such as FASN inhibitor Omeprazole (Sardesai et al., 2021), most of the studies were performed in vitro with several limitations and more data in vivo is necessary. Drug efficacy and safety need to be further tested by clinical trials.
Author Contributions
RY wrote the manuscript. BX and MY supervised, reviewed and revised the manuscript.
Funding
This study was supported in part by grants from The National Natural Science Foundation of China (82072596, 81772902, 81872278, 81703131, 82173339, 82172766), the National “111” Project (Project #111-2-12), the Hunan Provincial Key Research and Development Program (2022SK2026), the Natural Science Foundation of Hunan Province, China (2020JJ4920, 2020JJ4838, 2020JJ4766, 2020JJ3055), the Scientific Research Project of Hunan Provincial Health Commission (20201067, 20201040), and the Independent Exploration and Innovation Program of Central South University (2019zzts895, 2019zzts326, 2021zzts0928). We thank our colleagues at the cancer research institute of central south university for constructive discussion.
Conflict of Interest
The authors declare that the research was conducted in the absence of any commercial or financial relationships that could be construed as a potential conflict of interest.
Publisher’s Note
All claims expressed in this article are solely those of the authors and do not necessarily represent those of their affiliated organizations, or those of the publisher, the editors and the reviewers. Any product that may be evaluated in this article, or claim that may be made by its manufacturer, is not guaranteed or endorsed by the publisher.
Supplementary Material
The Supplementary Material for this article can be found online at: https://www.frontiersin.org/articles/10.3389/fcell.2022.875318/full#supplementary-material
References
Ackerman, D., and Simon, M. C. (2014). Hypoxia, Lipids, and Cancer: Surviving the Harsh Tumor Microenvironment. Trends Cel Biol. 24 (8), 472–478. doi:10.1016/j.tcb.2014.06.001
Ali, A., Levantini, E., Teo, J. T., Goggi, J., Clohessy, J. G., Wu, C. S., et al. (2018). Fatty Acid Synthase Mediates EGFR Palmitoylation in EGFR Mutated Non‐small Cell Lung Cancer. EMBO Mol. Med. 10, e8313. doi:10.15252/emmm.201708313
Alison, M. R., Lin, W.-R., Lim, S. M. L., and Nicholson, L. J. (2012). Cancer Stem Cells: in the Line of Fire. Cancer Treat. Rev. 38 (6), 589–598. doi:10.1016/j.ctrv.2012.03.003
Andrzejewski, S., Klimcakova, E., Johnson, R. M., Tabariès, S., Annis, M. G., McGuirk, S., et al. (2017). PGC-1α Promotes Breast Cancer Metastasis and Confers Bioenergetic Flexibility against Metabolic Drugs. Cel Metab. 26 (5), 778–787. e775. doi:10.1016/j.cmet.2017.09.006
Assaraf, Y. G., Brozovic, A., Gonçalves, A. C., Jurkovicova, D., Linē, A., Machuqueiro, M., et al. (2019). The Multi-Factorial Nature of Clinical Multidrug Resistance in Cancer. Drug Resist. Updates 46, 100645. doi:10.1016/j.drup.2019.100645
Bansal, S., Berk, M., Alkhouri, N., Partrick, D. A., Fung, J. J., and Feldstein, A. (2014). Stearoyl-CoA Desaturase Plays an Important Role in Proliferation and Chemoresistance in Human Hepatocellular Carcinoma. J. Surg. Res. 186 (1), 29–38. doi:10.1016/j.jss.2013.07.001
Bao, L., Wu, J., Dodson, M., Rojo de la Vega, E. M., Ning, Y., Zhang, Z., et al. (2017). ABCF2, an Nrf2 Target Gene, Contributes to Cisplatin Resistance in Ovarian Cancer Cells. Mol. Carcinog 56 (6), 1543–1553. doi:10.1002/mc.22615
Barneda, D., and Christian, M. (2017). Lipid Droplet Growth: Regulation of a Dynamic Organelle. Curr. Opin. Cel Biol. 47, 9–15. doi:10.1016/j.ceb.2017.02.002
Barrero, M. J., Camarero, N., Marrero, P. F., and Haro, D. (2003). Control of Human Carnitine Palmitoyltransferase II Gene Transcription by Peroxisome Proliferator-Activated Receptor through a Partially Conserved Peroxisome Proliferator-Responsive Element. Biochem. J. 369 (Pt 3), 721–729. doi:10.1042/bj20020851
Beckner, M. E., Fellows-Mayle, W., Zhang, Z., Agostino, N. R., Kant, J. A., Day, B. W., et al. (2009). Identification of ATP Citrate Lyase as a Positive Regulator of Glycolytic Function in Glioblastomas. Int. J. Cancer 126 (10), 2282–2295. doi:10.1002/ijc.24918
Belkaid, A., Duguay, S. R., Ouellette, R. J., and Surette, M. E. (2015). 17β-estradiol Induces Stearoyl-CoA Desaturase-1 Expression in Estrogen Receptor-Positive Breast Cancer Cells. BMC Cancer 15, 440. doi:10.1186/s12885-015-1452-1
Berwick, D. C., Hers, I., Heesom, K. J., Moule, S. K., and Tavareá, J. M. (2002). The Identification of ATP-Citrate Lyase as a Protein Kinase B (Akt) Substrate in Primary Adipocytes. J. Biol. Chem. 277 (37), 33895–33900. doi:10.1074/jbc.M204681200
Blancafort, A., Giró-Perafita, A., Oliveras, G., Palomeras, S., Turrado, C., Campuzano, Ò., et al. (2015). Dual Fatty Acid Synthase and HER2 Signaling Blockade Shows Marked Antitumor Activity against Breast Cancer Models Resistant to Anti-HER2 Drugs. PLoS One 10 (6), e0131241. doi:10.1371/journal.pone.0131241
Bosc, C., Selak, M. A., and Sarry, J.-E. (2017). Resistance Is Futile: Targeting Mitochondrial Energetics and Metabolism to Overcome Drug Resistance in Cancer Treatment. Cel Metab. 26 (5), 705–707. doi:10.1016/j.cmet.2017.10.013
Brabletz, T., Kalluri, R., Nieto, M. A., and Weinberg, R. A. (2018). EMT in Cancer. Nat. Rev. Cancer 18 (2), 128–134. doi:10.1038/nrc.2017.118
Calder, P. C. (2015). Functional Roles of Fatty Acids and Their Effects on Human Health. JPEN J. Parenter. Enteral Nutr. 39 (1 Suppl. l), 18s–32s. doi:10.1177/0148607115595980
Caro, P., Kishan, A. U., Norberg, E., Stanley, I. A., Chapuy, B., Ficarro, S. B., et al. (2012). Metabolic Signatures Uncover Distinct Targets in Molecular Subsets of Diffuse Large B Cell Lymphoma. Cancer Cell 22 (4), 547–560. doi:10.1016/j.ccr.2012.08.014
Carracedo, A., Cantley, L. C., and Pandolfi, P. P. (2013). Cancer Metabolism: Fatty Acid Oxidation in the Limelight. Nat. Rev. Cancer 13 (4), 227–232. doi:10.1038/nrc3483
Carrer, A., Trefely, S., Zhao, S., Campbell, S. L., Norgard, R. J., Schultz, K. C., et al. (2019). Acetyl-CoA Metabolism Supports Multistep Pancreatic Tumorigenesis. Cancer Discov. 9 (3), 416–435. doi:10.1158/2159-8290.cd-18-0567
Chen, C.-L., Uthaya Kumar, D. B., Punj, V., Xu, J., Sher, L., Tahara, S. M., et al. (2016). NANOG Metabolically Reprograms Tumor-Initiating Stem-like Cells through Tumorigenic Changes in Oxidative Phosphorylation and Fatty Acid Metabolism. Cel Metab. 23 (1), 206–219. doi:10.1016/j.cmet.2015.12.004
Chen, X., Kang, R., Kroemer, G., and Tang, D. (2021). Broadening Horizons: the Role of Ferroptosis in Cancer. Nat. Rev. Clin. Oncol. 18 (5), 280–296. doi:10.1038/s41571-020-00462-0
Choi, T. G., Nguyen, M. N., Kim, J., Jo, Y. H., Jang, M., Nguyen, N. N. Y., et al. (2018). Cyclophilin B Induces Chemoresistance by Degrading Wild-type P53 via Interaction with MDM2 in Colorectal Cancer. J. Pathol. 246 (1), 115–126. doi:10.1002/path.5107
Cioccoloni, G., Aquino, A., Notarnicola, M., Caruso, M. G., Bonmassar, E., Zonfrillo, M., et al. (2020). Fatty Acid Synthase Inhibitor Orlistat Impairs Cell Growth and Down-Regulates PD-L1 Expression of a Human T-Cell Leukemia Line. J. Chemother. 32 (1), 30–40. doi:10.1080/1120009X.2019.1694761
Cioffi, M., Trabulo, S. M., Sanchez-Ripoll, Y., Miranda-Lorenzo, I., Lonardo, E., Dorado, J., et al. (2015). The miR-17-92 Cluster Counteracts Quiescence and Chemoresistance in a Distinct Subpopulation of Pancreatic Cancer Stem Cells. Gut 64 (12), 1936–1948. doi:10.1136/gutjnl-2014-308470
Cluntun, A. A., Lukey, M. J., Cerione, R. A., and Locasale, J. W. (2017). Glutamine Metabolism in Cancer: Understanding the Heterogeneity. Trends Cancer 3 (3), 169–180. doi:10.1016/j.trecan.2017.01.005
Cotte, A. K., Aires, V., Fredon, M., Limagne, E., Derangère, V., Thibaudin, M., et al. (2018). Lysophosphatidylcholine Acyltransferase 2-mediated Lipid Droplet Production Supports Colorectal Cancer Chemoresistance. Nat. Commun. 9 (1), 322. doi:10.1038/s41467-017-02732-5
Crea, F., Nur Saidy, N. R., Collins, C. C., and Wang, Y. (2015). The Epigenetic/noncoding Origin of Tumor Dormancy. Trends Mol. Med. 21 (4), 206–211. doi:10.1016/j.molmed.2015.02.005
Currie, E., Schulze, A., Zechner, R., Walther, T. C., and Farese, R. V. (2013). Cellular Fatty Acid Metabolism and Cancer. Cel Metab. 18 (2), 153–161. doi:10.1016/j.cmet.2013.05.017
Dai, S., Yan, Y., Xu, Z., Zeng, S., Qian, L., Huo, L., et al. (2017). SCD1 Confers Temozolomide Resistance to Human Glioma Cells via the Akt/GSK3β/β-Catenin Signaling Axis. Front. Pharmacol. 8, 960. doi:10.3389/fphar.2017.00960
De Angelis, M., Francescangeli, F., and Zeuner, A. (2019). Breast Cancer Stem Cells as Drivers of Tumor Chemoresistance, Dormancy and Relapse: New Challenges and Therapeutic Opportunities. Cancers 11 (10), 1569. doi:10.3390/cancers11101569
Dixon, S. J., Lemberg, K. M., Lamprecht, M. R., Skouta, R., Zaitsev, E. M., Gleason, C. E., et al. (2012). Ferroptosis: an Iron-dependent Form of Nonapoptotic Cell Death. Cell 149 (5), 1060–1072. doi:10.1016/j.cell.2012.03.042
Donohue, E., Thomas, A., Maurer, N., Manisali, I., Zeisser-Labouebe, M., Zisman, N., et al. (2013). The Autophagy Inhibitor Verteporfin Moderately Enhances the Antitumor Activity of Gemcitabine in a Pancreatic Ductal Adenocarcinoma Model. J. Cancer 4 (7), 585–596. doi:10.7150/jca.7030
Du, B., and Shim, J. (2016). Targeting Epithelial-Mesenchymal Transition (EMT) to Overcome Drug Resistance in Cancer. Molecules 21 (7), 965. doi:10.3390/molecules21070965
Duan, J., Chen, L., Zhou, M., Zhang, J., Sun, L., Huang, N., et al. (2017). MACC1 Decreases the Chemosensitivity of Gastric Cancer Cells to Oxaliplatin by Regulating FASN Expression. Oncol. Rep. 37 (5), 2583–2592. doi:10.3892/or.2017.5519
Eberlin, L. S., Gabay, M., Fan, A. C., Gouw, A. M., Tibshirani, R. J., Felsher, D. W., et al. (2014). Alteration of the Lipid Profile in Lymphomas Induced by MYC Overexpression. Proc. Natl. Acad. Sci. U.S.A. 111 (29), 10450–10455. doi:10.1073/pnas.1409778111
Eckford, P. D. W., and Sharom, F. J. (2009). ABC Efflux Pump-Based Resistance to Chemotherapy Drugs. Chem. Rev. 109 (7), 2989–3011. doi:10.1021/cr9000226
Englinger, B., Laemmerer, A., Moser, P., Kallus, S., Röhrl, C., Pirker, C., et al. (2020). Lipid Droplet‐mediated Scavenging as Novel Intrinsic and Adaptive Resistance Factor against the Multikinase Inhibitor Ponatinib. Int. J. Cancer 147, 1680–1693. doi:10.1002/ijc.32924
Espinosa, E., Zamora, P., Feliu, J., and González Barón, M. (2003). Classification of Anticancer Drugs-A New System Based on Therapeutic Targets. Cancer Treat. Rev. 29 (6), 515–523. doi:10.1016/s0305-7372(03)00116-6
Farese, R. V., and Walther, T. C. (2009). Lipid Droplets Finally Get a Little R-E-S-P-E-C-T. Cell 139 (5), 855–860. doi:10.1016/j.cell.2009.11.005
Farge, T., Saland, E., de Toni, F., Aroua, N., Hosseini, M., Perry, R., et al. (2017). Chemotherapy-Resistant Human Acute Myeloid Leukemia Cells Are Not Enriched for Leukemic Stem Cells but Require Oxidative Metabolism. Cancer Discov. 7 (7), 716–735. doi:10.1158/2159-8290.cd-16-0441
Fesler, A., Guo, S., Liu, H., Wu, N., and Ju, J. (2017). Overcoming Chemoresistance in Cancer Stem Cells with the Help of microRNAs in Colorectal Cancer. Epigenomics 9 (6), 793–796. doi:10.2217/epi-2017-0041
Fletcher, J. I., Haber, M., Henderson, M. J., and Norris, M. D. (2010). ABC Transporters in Cancer: More Than Just Drug Efflux Pumps. Nat. Rev. Cancer 10 (2), 147–156. doi:10.1038/nrc2789
Friedmann Angeli, J. P., Krysko, D. V., and Conrad, M. (2019). Ferroptosis at the Crossroads of Cancer-Acquired Drug Resistance and Immune Evasion. Nat. Rev. Cancer 19 (7), 405–414. doi:10.1038/s41568-019-0149-1
Furuta, E., Pai, S. K., Zhan, R., Bandyopadhyay, S., Watabe, M., Mo, Y.-Y., et al. (2008). Fatty Acid Synthase Gene Is Up-Regulated by Hypoxia via Activation of Akt and Sterol Regulatory Element Binding Protein-1. Cancer Res. 68 (4), 1003–1011. doi:10.1158/0008-5472.can-07-2489
Gentric, G., Kieffer, Y., Mieulet, V., Goundiam, O., Bonneau, C., Nemati, F., et al. (2019). PML-regulated Mitochondrial Metabolism Enhances Chemosensitivity in Human Ovarian Cancers. Cel Metab. 29, 156–173. doi:10.1016/j.cmet.2018.09.002
Gnanapradeepan, K., Basu, S., Barnoud, T., Budina-Kolomets, A., Kung, C.-P., and Murphy, M. E. (2018). The P53 Tumor Suppressor in the Control of Metabolism and Ferroptosis. Front. Endocrinol. 9, 7. doi:10.3389/fendo.2018.00124
Govaere, O., Wouters, J., Petz, M., Vandewynckel, Y.-P., Van den Eynde, K., Van den Broeck, A., et al. (2016). Laminin-332 Sustains Chemoresistance and Quiescence as Part of the Human Hepatic Cancer Stem Cell Niche. J. Hepatol. 64 (3), 609–617. doi:10.1016/j.jhep.2015.11.011
Greaves, M., and Maley, C. C. (2012). Clonal Evolution in Cancer. Nature 481 (7381), 306–313. doi:10.1038/nature10762
Griffiths, B., Lewis, C. A., Bensaad, K., Ros, S., Zhang, Q., Ferber, E. C., et al. (2013). Sterol Regulatory Element Binding Protein-dependent Regulation of Lipid Synthesis Supports Cell Survival and Tumor Growth. Cancer Metab. 1 (1), 3. doi:10.1186/2049-3002-1-3
Grunt, T. W. (2018). Interacting Cancer Machineries: Cell Signaling, Lipid Metabolism, and Epigenetics. Trends Endocrinol. Metab. 29 (2), 86–98. doi:10.1016/j.tem.2017.11.003
Guo, W., Ma, J., Yang, Y., Guo, S., Zhang, W., Zhao, T., et al. (2020). ATP-citrate Lyase Epigenetically Potentiates Oxidative Phosphorylation to Promote Melanoma Growth and Adaptive Resistance to MAPK Inhibition. Clin. Cancer Res. 26, 2725–2739. doi:10.1158/1078-0432.ccr-19-1359
Hagmann, W., Jesnowski, R., and Löhr, J. M. (2010). Interdependence of Gemcitabine Treatment, Transporter Expression, and Resistance in Human Pancreatic Carcinoma Cells. Neoplasia 12 (9), 740–747. doi:10.1593/neo.10576
Hanahan, D., and Weinberg, R. A. (2011). Hallmarks of Cancer: the Next Generation. Cell 144 (5), 646–674. doi:10.1016/j.cell.2011.02.013
Hanai, J.-i., Doro, N., Sasaki, A. T., Kobayashi, S., Cantley, L. C., Seth, P., et al. (2012). Inhibition of Lung Cancer Growth: ATP Citrate Lyase Knockdown and Statin Treatment Leads to Dual Blockade of Mitogen-Activated Protein Kinase (MAPK) and Phosphatidylinositol-3-Kinase (PI3K)/AKT Pathways. J. Cel. Physiol. 227 (4), 1709–1720. doi:10.1002/jcp.22895
Hanai, J.-i., Doro, N., Seth, P., and Sukhatme, V. P. (2013). ATP Citrate Lyase Knockdown Impacts Cancer Stem Cells In Vitro. Cell Death Dis 4, e696. doi:10.1038/cddis.2013.215
Hatzivassiliou, G., Zhao, F., Bauer, D. E., Andreadis, C., Shaw, A. N., Dhanak, D., et al. (2005). ATP Citrate Lyase Inhibition Can Suppress Tumor Cell Growth. Cancer Cell 8 (4), 311–321. doi:10.1016/j.ccr.2005.09.008
Hendrich, A., and Michalak, K. (2003). Lipids as a Target for Drugs Modulating Multidrug Resistance of Cancer Cells. Cdt 4 (1), 23–30. doi:10.2174/1389450033347172
Hirpara, J., Eu, J. Q., Tan, J. K. M., Wong, A. L., Clement, M.-V., Kong, L. R., et al. (2019). Metabolic Reprogramming of Oncogene-Addicted Cancer Cells to OXPHOS as a Mechanism of Drug Resistance. Redox Biol. 25, 101076. doi:10.1016/j.redox.2018.101076
Horibe, S., Matsuda, A., Tanahashi, T., Inoue, J., Kawauchi, S., Mizuno, S., et al. (2015). Cisplatin Resistance in Human Lung Cancer Cells Is Linked with Dysregulation of Cell Cycle Associated Proteins. Life Sci. 124, 31–40. doi:10.1016/j.lfs.2015.01.011
Hoxhaj, G., and Manning, B. D. (2020). The PI3K-AKT Network at the Interface of Oncogenic Signalling and Cancer Metabolism. Nat. Rev. Cancer 20 (2), 74–88. doi:10.1038/s41568-019-0216-7
Huang, Y., Bell, L. N., Okamura, J., Kim, M. S., Mohney, R. P., Guerrero-Preston, R., et al. (2012). Phospho-ΔNp63α/SREBF1 Protein Interactions: Bridging Cell Metabolism and Cisplatin Chemoresistance. Cell Cycle 11 (20), 3810–3827. doi:10.4161/cc.22022
Huang, Z., Zhou, L., Chen, Z., Nice, E. C., and Huang, C. (2016). Stress Management by Autophagy: Implications for Chemoresistance. Int. J. Cancer 139 (1), 23–32. doi:10.1002/ijc.29990
Hultsch, S., Kankainen, M., Paavolainen, L., Kovanen, R.-M., Ikonen, E., Kangaspeska, S., et al. (2018). Association of Tamoxifen Resistance and Lipid Reprogramming in Breast Cancer. BMC Cancer 18 (1), 850. doi:10.1186/s12885-018-4757-z
Icard, P., Shulman, S., Farhat, D., Steyaert, J.-M., Alifano, M., and Lincet, H. (2018). How the Warburg Effect Supports Aggressiveness and Drug Resistance of Cancer Cells? Drug Resist. Updates 38, 1–11. doi:10.1016/j.drup.2018.03.001
Ichim, G., and Tait, S. W. G. (2016). A Fate Worse Than Death: Apoptosis as an Oncogenic Process. Nat. Rev. Cancer 16 (8), 539–548. doi:10.1038/nrc.2016.58
Igal, R. A. (2011). Roles of StearoylCoA Desaturase-1 in the Regulation of Cancer Cell Growth, Survival and Tumorigenesis. Cancers 3 (2), 2462–2477. doi:10.3390/cancers3022462
Igal, R. A. (2016). Stearoyl CoA Desaturase-1: New Insights into a central Regulator of Cancer Metabolism. Biochim. Biophys. Acta (Bba) - Mol. Cel Biol. Lipids 1861 (12 Pt A), 1865–1880. doi:10.1016/j.bbalip.2016.09.009
Iwamoto, H., Abe, M., Yang, Y., Cui, D., Seki, T., Nakamura, M., et al. (2018). Cancer Lipid Metabolism Confers Antiangiogenic Drug Resistance. Cel Metab. 28 (1), 104–117. e105. doi:10.1016/j.cmet.2018.05.005
Jahanban-Esfahlan, R., Seidi, K., Manjili, M. H., Jahanban-Esfahlan, A., Javaheri, T., and Zare, P. (2019). Tumor Cell Dormancy: Threat or Opportunity in the Fight against Cancer. Cancers 11 (8), 1207. doi:10.3390/cancers11081207
Jeggo, P. A., Pearl, L. H., and Carr, A. M. (2016). DNA Repair, Genome Stability and Cancer: a Historical Perspective. Nat. Rev. Cancer 16 (1), 35–42. doi:10.1038/nrc.2015.4
Jeon, S.-M., Chandel, N. S., and Hay, N. (2012). AMPK Regulates NADPH Homeostasis to Promote Tumour Cell Survival during Energy Stress. Nature 485(7400), 661, 665-+. doi:10.1038/nature11066
Ji, N., Yang, Y., Cai, C.-Y., Lei, Z.-N., Wang, J.-Q., Gupta, P., et al. (2019). Selonsertib (GS-4997), an ASK1 Inhibitor, Antagonizes Multidrug Resistance in ABCB1- and ABCG2-Overexpressing Cancer Cells. Cancer Lett. 440-441, 82–93. doi:10.1016/j.canlet.2018.10.007
Jones, S. F., and Infante, J. R. (2015). Molecular Pathways: Fatty Acid Synthase. Clin. Cancer Res. 21 (24), 5434–5438. doi:10.1158/1078-0432.CCR-15-0126
Kathawala, R. J., Gupta, P., Ashby, C. R., and Chen, Z.-S. (2015). The Modulation of ABC Transporter-Mediated Multidrug Resistance in Cancer: a Review of the Past Decade. Drug Resist. Updates 18, 1–17. doi:10.1016/j.drup.2014.11.002
Kazan, H. H., Urfali-Mamatoglu, C., and Gunduz, U. (2017). Iron Metabolism and Drug Resistance in Cancer. Biometals 30 (5), 629–641. doi:10.1007/s10534-017-0037-7
Kennedy, L. B., and Salama, A. K. S. (2020). A Review of Cancer Immunotherapy Toxicity. CA A. Cancer J. Clin. 70 (2), 86–104. doi:10.3322/caac.21596
Koppenol, W. H., Bounds, P. L., and Dang, C. V. (2011). Otto Warburg's Contributions to Current Concepts of Cancer Metabolism. Nat. Rev. Cancer 11 (5), 325–337. doi:10.1038/nrc3038
Lamouille, S., Xu, J., and Derynck, R. (2014). Molecular Mechanisms of Epithelial-Mesenchymal Transition. Nat. Rev. Mol. Cel Biol 15 (3), 178–196. doi:10.1038/nrm3758
Lee, H., Zandkarimi, F., Zhang, Y., Meena, J. K., Kim, J., Zhuang, L., et al. (2020). Energy-stress-mediated AMPK Activation Inhibits Ferroptosis. Nat. Cel Biol 22 (2), 225–234. doi:10.1038/s41556-020-0461-8
Lee, J.-Y., Kim, W. K., Bae, K.-H., Lee, S. C., and Lee, E.-W. (2021). Lipid Metabolism and Ferroptosis. Biology 10 (3), 184. doi:10.3390/biology10030184
Lee, J. V., Berry, C. T., Kim, K., Sen, P., Kim, T., Carrer, A., et al. (2018a). Acetyl-CoA Promotes Glioblastoma Cell Adhesion and Migration through Ca2+-NFAT Signaling. Genes Dev. 32 (7-8), 497–511. doi:10.1101/gad.311027.117
Lee, J. V., Carrer, A., Shah, S., Snyder, N. W., Wei, S., Venneti, S., et al. (2014). Akt-dependent Metabolic Reprogramming Regulates Tumor Cell Histone Acetylation. Cel Metab. 20 (2), 306–319. doi:10.1016/j.cmet.2014.06.004
Lee, M. Y., Yeon, A., Shahid, M., Cho, E., Sairam, V., Figlin, R., et al. (2018b). Reprogrammed Lipid Metabolism in Bladder Cancer with Cisplatin Resistance. Oncotarget 9 (17), 13231–13243. doi:10.18632/oncotarget.24229
Lepeltier, E., Rijo, P., Rizzolio, F., Popovtzer, R., Petrikaite, V., Assaraf, Y. G., et al. (2020). Nanomedicine to Target Multidrug Resistant Tumors. Drug Resist. Updates 52, 100704. doi:10.1016/j.drup.2020.100704
Levy, J. M. M., Towers, C. G., and Thorburn, A. (2017). Targeting Autophagy in Cancer. Nat. Rev. Cancer 17 (9), 528–542. doi:10.1038/nrc.2017.53
Li, C.-F., Fang, F.-M., Chen, Y.-Y., Liu, T.-T., Chan, T.-C., Yu, S.-C., et al. (2017). Overexpressed Fatty Acid Synthase in Gastrointestinal Stromal Tumors: Targeting a Progression-Associated Metabolic Driver Enhances the Antitumor Effect of Imatinib. Clin. Cancer Res. 23 (16), 4908–4918. doi:10.1158/1078-0432.ccr-16-2770
Li, Q., Qin, T., Bi, Z., Hong, H., Ding, L., Chen, J., et al. (2020). Rac1 Activates Non-oxidative Pentose Phosphate Pathway to Induce Chemoresistance of Breast Cancer. Nat. Commun. 11 (1), 1456. doi:10.1038/s41467-020-15308-7
Li, Q., Zhang, D., Chen, X., He, L., Li, T., Xu, X., et al. (2015). Nuclear PKM2 Contributes to Gefitinib Resistance via Upregulation of STAT3 Activation in Colorectal Cancer. Sci. Rep. 5, 16082. doi:10.1038/srep16082
Lien, E. C., Westermark, A. M., Zhang, Y., Yuan, C., Li, Z., Lau, A. N., et al. (2021). Low Glycaemic Diets Alter Lipid Metabolism to Influence Tumour Growth. Nature 599 (7884), 302–307. doi:10.1038/s41586-021-04049-2
Ligorio, F., Pellegrini, I., Castagnoli, L., Vingiani, A., Lobefaro, R., Zattarin, E., et al. (2021). Targeting Lipid Metabolism Is an Emerging Strategy to Enhance the Efficacy of Anti-HER2 Therapies in HER2-Positive Breast Cancer. Cancer Lett. 511, 77–87. doi:10.1016/j.canlet.2021.04.023
Lin, D., Zhang, H., Liu, R., Deng, T., Ning, T., Bai, M., et al. (2021). iRGD‐Modified Exosomes Effectively Deliver CPT1A siRNA to colon Cancer Cells, Reversing Oxaliplatin Resistance by Regulating Fatty Acid Oxidation. Mol. Oncol. 15 (12), 3430–3446. doi:10.1002/1878-0261.13052
Lin, M., Lv, D., Zheng, Y., Wu, M., Xu, C., Zhang, Q., et al. (2018). Downregulation of CPT2 Promotes Tumorigenesis and Chemoresistance to Cisplatin in Hepatocellular Carcinoma. Ott 11, 3101–3110. doi:10.2147/ott.s163266
Liu, H., Liu, Y., and Zhang, J.-T. (2008). A New Mechanism of Drug Resistance in Breast Cancer Cells: Fatty Acid Synthase Overexpression-Mediated Palmitate Overproduction. Mol. Cancer Ther. 7 (2), 263–270. doi:10.1158/1535-7163.mct-07-0445
Liu, H., Wu, X., Dong, Z., Luo, Z., Zhao, Z., Xu, Y., et al. (2013). Fatty Acid Synthase Causes Drug Resistance by Inhibiting TNF-α and Ceramide Production. J. Lipid Res. 54 (3), 776–785. doi:10.1194/jlr.M033811
Liu, J., Ren, L., Li, S., Li, W., Zheng, X., Yang, Y., et al. (2021). The Biology, Function, and Applications of Exosomes in Cancer. Acta Pharmaceutica Sinica. B 11 (9), 2783–2797. doi:10.1016/j.apsb.2021.01.001
Liu, Q., Luo, Q., Halim, A., and Song, G. (2017). Targeting Lipid Metabolism of Cancer Cells: A Promising Therapeutic Strategy for Cancer. Cancer Lett. 401, 39–45. doi:10.1016/j.canlet.2017.05.002
Lodhi, I. J., and Semenkovich, C. F. (2014). Peroxisomes: a Nexus for Lipid Metabolism and Cellular Signaling. Cel Metab. 19 (3), 380–392. doi:10.1016/j.cmet.2014.01.002
Lucenay, K. S., Doostan, I., Karakas, C., Bui, T., Ding, Z., Mills, G. B., et al. (2016). Cyclin E Associates with the Lipogenic Enzyme ATP-Citrate Lyase to Enable Malignant Growth of Breast Cancer Cells. Cancer Res. 76 (8), 2406–2418. doi:10.1158/0008-5472.can-15-1646
Luo, J., Hong, Y., Lu, Y., Qiu, S., Chaganty, B. K. R., Zhang, L., et al. (2017). Acetyl-CoA Carboxylase Rewires Cancer Metabolism to Allow Cancer Cells to Survive Inhibition of the Warburg Effect by Cetuximab. Cancer Lett. 384, 39–49. doi:10.1016/j.canlet.2016.09.020
Luo, J., Hong, Y., Tao, X., Wei, X., Zhang, L., and Li, Q. (2016). An Indispensable Role of CPT-1a to Survive Cancer Cells during Energy Stress through Rewiring Cancer Metabolism. Tumor Biol. 37, 15795–15804. doi:10.1007/s13277-016-5382-6
Ma, M. K. F., Lau, E. Y. T., Leung, D. H. W., Lo, J., Ho, N. P. Y., Cheng, L. K. W., et al. (2017). Stearoyl-CoA Desaturase Regulates Sorafenib Resistance via Modulation of ER Stress-Induced Differentiation. J. Hepatol. 67 (5), 979–990. doi:10.1016/j.jhep.2017.06.015
Magtanong, L., Ko, P.-J., To, M., Cao, J. Y., Forcina, G. C., Tarangelo, A., et al. (2019). Exogenous Monounsaturated Fatty Acids Promote a Ferroptosis-Resistant Cell State. Cel Chem. Biol. 26 (3), 420–432. doi:10.1016/j.chembiol.2018.11.016
Martinez Calejman, C., Trefely, S., Entwisle, S. W., Luciano, A., Jung, S. M., Hsiao, W., et al. (2020). mTORC2-AKT Signaling to ATP-Citrate Lyase Drives Brown Adipogenesis and De Novo Lipogenesis. Nat. Commun. 11 (1), 575. doi:10.1038/s41467-020-14430-w
McGarry, J. D., Mills, S. E., Long, C. S., and Foster, D. W. (1983). Observations on the Affinity for Carnitine, and Malonyl-CoA Sensitivity, of Carnitine Palmitoyltransferase I in Animal and Human Tissues. Demonstration of the Presence of Malonyl-CoA in Non-hepatic Tissues of the Rat. Biochem. J. 214 (1), 21–28. doi:10.1042/bj2140021
Meena, A. S., Sharma, A., Kumari, R., Mohammad, N., Singh, S. V., and Bhat, M. K. (2013). Inherent and Acquired Resistance to Paclitaxel in Hepatocellular Carcinoma: Molecular Events Involved. PLoS One 8 (4), e61524. doi:10.1371/journal.pone.0061524
Menendez, J. A., and Lupu, R. (2007). Fatty Acid Synthase and the Lipogenic Phenotype in Cancer Pathogenesis. Nat. Rev. Cancer 7 (10), 763–777. doi:10.1038/nrc2222
Mohammad, R. M., Muqbil, I., Lowe, L., Yedjou, C., Hsu, H.-Y., Lin, L.-T., et al. (2015). Broad Targeting of Resistance to Apoptosis in Cancer. Semin. Cancer Biol. 35, S78–S103. doi:10.1016/j.semcancer.2015.03.001
Mondal, S., Roy, D., Sarkar Bhattacharya, S., Jin, L., Jung, D., Zhang, S., et al. (2019). Therapeutic Targeting of PFKFB3 with a Novel Glycolytic Inhibitor PFK158 Promotes Lipophagy and Chemosensitivity in Gynecologic Cancers. Int. J. Cancer 144 (1), 178–189. doi:10.1002/ijc.31868
Najafi, M., Goradel, N. H., Farhood, B., Salehi, E., Solhjoo, S., Toolee, H., et al. (2019). Tumor Microenvironment: Interactions and Therapy. J. Cell Physiol. 234 (5), 5700–5721. doi:10.1002/jcp.27425
Nieto, M. A., Huang, R. Y.-J., Jackson, R. A., and Thiery, J. P. (2016). EMT: 2016. Cell 166 (1), 21–45. doi:10.1016/j.cell.2016.06.028
Oatman, N., Dasgupta, N., Arora, P., Choi, K., Gawali, M. V., Gupta, N., et al. (2021). Mechanisms of Stearoyl CoA Desaturase Inhibitor Sensitivity and Acquired Resistance in Cancer. Sci. Adv. 7 (7), eabd7459. doi:10.1126/sciadv.abd7459
O’Donnell, J. S., Teng, M. W. L., and Smyth, M. J. (2019). Cancer Immunoediting and Resistance to T Cell-Based Immunotherapy. Nat. Rev. Clin. Oncol. 16 (3), 151–167. doi:10.1038/s41571-018-0142-8
Olzmann, J. A., and Carvalho, P. (2019). Dynamics and Functions of Lipid Droplets. Nat. Rev. Mol. Cel Biol 20 (3), 137–155. doi:10.1038/s41580-018-0085-z
Pan, S.-T., Li, Z.-L., He, Z.-X., Qiu, J.-X., and Zhou, S.-F. (2016). Molecular Mechanisms for Tumour Resistance to Chemotherapy. Clin. Exp. Pharmacol. Physiol. 43 (8), 723–737. doi:10.1111/1440-1681.12581
Pavlova, N. N., and Thompson, C. B. (2016). The Emerging Hallmarks of Cancer Metabolism. Cel Metab. 23 (1), 27–47. doi:10.1016/j.cmet.2015.12.006
Peck, B., Schug, Z. T., Zhang, Q., Dankworth, B., Jones, D. T., Smethurst, E., et al. (2016). Inhibition of Fatty Acid Desaturation Is Detrimental to Cancer Cell Survival in Metabolically Compromised Environments. Cancer Metab. 4, 6. doi:10.1186/s40170-016-0146-8
Peters, J. M., Gonzalez, F. J., and Müller, R. (2015). Establishing the Role of PPARβ/δ in Carcinogenesis. Trends Endocrinol. Metab. 26 (11), 595–607. doi:10.1016/j.tem.2015.09.004
Peters, J. M., Shah, Y. M., and Gonzalez, F. J. (2012). The Role of Peroxisome Proliferator-Activated Receptors in Carcinogenesis and Chemoprevention. Nat. Rev. Cancer 12 (3), 181–195. doi:10.1038/nrc3214
Piao, C., Cui, X., Zhan, B., Li, J., Li, Z., Li, Z., et al. (2018). Inhibition of Stearoyl CoA Desaturase-1 Activity Suppresses Tumour Progression and Improves Prognosis in Human Bladder Cancer. J. Cel Mol Med 23, 2064–2076. doi:10.1111/jcmm.14114
Pisanu, M. E., Maugeri-Saccà, M., Fattore, L., Bruschini, S., De Vitis, C., Tabbì, E., et al. (2018). Inhibition of Stearoyl-CoA Desaturase 1 Reverts BRAF and MEK Inhibition-Induced Selection of Cancer Stem Cells in BRAF-Mutated Melanoma. J. Exp. Clin. Cancer Res. 37 (1), 318. doi:10.1186/s13046-018-0989-7
Pisanu, M. E., Noto, A., De Vitis, C., Morrone, S., Scognamiglio, G., Botti, G., et al. (2017). Blockade of Stearoyl-CoA-Desaturase 1 Activity Reverts Resistance to Cisplatin in Lung Cancer Stem Cells. Cancer Lett. 406, 93–104. doi:10.1016/j.canlet.2017.07.027
Porstmann, T., Griffiths, B., Chung, Y.-L., Delpuech, O., Griffiths, J. R., Downward, J., et al. (2005). PKB/Akt Induces Transcription of Enzymes Involved in Cholesterol and Fatty Acid Biosynthesis via Activation of SREBP. Oncogene 24 (43), 6465–6481. doi:10.1038/sj.onc.1208802
Potapova, I. A., El-Maghrabi, M. R., Doronin, S. V., and Benjamin, W. B. (2000). Phosphorylation of Recombinant Human ATP:Citrate Lyase by cAMP-dependent Protein Kinase Abolishes Homotropic Allosteric Regulation of the Enzyme by Citrate and Increases the Enzyme Activity. Allosteric Activation of ATP:Citrate Lyase by Phosphorylated Sugars. Biochemistry 39 (5), 1169–1179. doi:10.1021/bi992159y
Puig, T., Aguilar, H., Cufí, S., Oliveras, G., Turrado, C., Ortega-Gutiérrez, S., et al. (2011). A Novel Inhibitor of Fatty Acid Synthase Shows Activity against HER2+ Breast Cancer Xenografts and Is Active in Anti-HER2 Drug-Resistant Cell Lines. Breast Cancer Res. 13 (6), R131. doi:10.1186/bcr3077
Qu, Q., Zeng, F., Liu, X., Wang, Q. J., and Deng, F. (2016). Fatty Acid Oxidation and Carnitine Palmitoyltransferase I: Emerging Therapeutic Targets in Cancer. Cel Death Dis 7, e2226. doi:10.1038/cddis.2016.132
Rak, S., De Zan, T., Stefulj, J., Kosović, M., Gamulin, O., and Osmak, M. (2014). FTIR Spectroscopy Reveals Lipid Droplets in Drug Resistant Laryngeal Carcinoma Cells through Detection of Increased Ester Vibrational Bands Intensity. Analyst 139 (13), 3407–3415. doi:10.1039/c4an00412d
Rambold, A. S., Cohen, S., and Lippincott-Schwartz, J. (2015). Fatty Acid Trafficking in Starved Cells: Regulation by Lipid Droplet Lipolysis, Autophagy, and Mitochondrial Fusion Dynamics. Developmental Cel 32 (6), 678–692. doi:10.1016/j.devcel.2015.01.029
Riley, R. S., June, C. H., Langer, R., and Mitchell, M. J. (2019). Delivery Technologies for Cancer Immunotherapy. Nat. Rev. Drug Discovdrug Discov. 18 (3), 175–196. doi:10.1038/s41573-018-0006-z
Röhrig, F., and Schulze, A. (2016). The Multifaceted Roles of Fatty Acid Synthesis in Cancer. Nat. Rev. Cancer 16 (11), 732–749. doi:10.1038/nrc.2016.89
Sardesai, S. D., Thomas, A., Gallagher, C., Lynce, F., Ottaviano, Y. L., Ballinger, T. J., et al. (2021). Inhibiting Fatty Acid Synthase with Omeprazole to Improve Efficacy of Neoadjuvant Chemotherapy in Patients with Operable TNBC. Clin. Cancer Res. : Official J. Am. Assoc. Cancer Res. 27 (21), 5810–5817. doi:10.1158/1078-0432.CCR-21-0493
Schlaepfer, I. R., Hitz, C. A., Gijón, M. A., Bergman, B. C., Eckel, R. H., and Jacobsen, B. M. (2012). Progestin Modulates the Lipid Profile and Sensitivity of Breast Cancer Cells to Docetaxel. Mol. Cell Endocrinol. 363 (1-2), 111–121. doi:10.1016/j.mce.2012.08.005
Shah, S., Carriveau, W. J., Li, J., Campbell, S. L., Kopinski, P. K., Lim, H.-W., et al. (2016). Targeting ACLY Sensitizes Castration-Resistant Prostate Cancer Cells to AR Antagonism by Impinging on an ACLY-AMPK-AR Feedback Mechanism. Oncotarget 7 (28), 43713–43730. doi:10.18632/oncotarget.9666
Shahid, M., Kim, M., Jin, P., Zhou, B., Wang, Y., Yang, W., et al. (2020). S-palmitoylation as a Functional Regulator of Proteins Associated with Cisplatin Resistance in Bladder Cancer. Int. J. Biol. Sci. 16 (14), 2490–2505. doi:10.7150/ijbs.45640
Shanmugasundaram, K., Nayak, B. K., Friedrichs, W. E., Kaushik, D., Rodriguez, R., and Block, K. (2017). NOX4 Functions as a Mitochondrial Energetic Sensor Coupling Cancer Metabolic Reprogramming to Drug Resistance. Nat. Commun. 8, 1. doi:10.1038/s41467-017-01106-1
Shao, W., and Espenshade, P. J. (2012). Expanding Roles for SREBP in Metabolism. Cel Metab. 16 (4), 414–419. doi:10.1016/j.cmet.2012.09.002
Simons, K., and Sampaio, J. L. (2011). Membrane Organization and Lipid Rafts. Cold Spring Harbor Perspect. Biol. 3 (10), a004697. doi:10.1101/cshperspect.a004697
Siqingaowa, , , Sekar, S., Gopalakrishnan, V., and Taghibiglou, C. (2017). Sterol Regulatory Element-Binding Protein 1 Inhibitors Decrease Pancreatic Cancer Cell Viability and Proliferation. Biochem. Biophysical Res. Commun. 488 (1), 136–140. doi:10.1016/j.bbrc.2017.05.023
Sivanand, S., Rhoades, S., Jiang, Q., Lee, J. V., Benci, J., Zhang, J., et al. (2017). Nuclear Acetyl-CoA Production by ACLY Promotes Homologous Recombination. Mol. Cel 67 (2), 252–265. doi:10.1016/j.molcel.2017.06.008
Snaebjornsson, M. T., Janaki-Raman, S., and Schulze, A. (2020). Greasing the Wheels of the Cancer Machine: The Role of Lipid Metabolism in Cancer. Cel Metab. 31 (1), 62–76. doi:10.1016/j.cmet.2019.11.010
Somasagara, R. R., Spencer, S. M., Tripathi, K., Clark, D. W., Mani, C., Madeira da Silva, L., et al. (2017). RAD6 Promotes DNA Repair and Stem Cell Signaling in Ovarian Cancer and Is a Promising Therapeutic Target to Prevent and Treat Acquired Chemoresistance. Oncogene 36 (48), 6680–6690. doi:10.1038/onc.2017.279
Souchek, J. J., Davis, A. L., Hill, T. K., Holmes, M. B., Qi, B., Singh, P. K., et al. (2017). Combination Treatment with Orlistat-Containing Nanoparticles and Taxanes Is Synergistic and Enhances Microtubule Stability in Taxane-Resistant Prostate Cancer Cells. Mol. Cancer Ther. 16 (9), 1819–1830. doi:10.1158/1535-7163.mct-17-0013
Steinberg, G. R., and Carling, D. (2019). AMP-activated Protein Kinase: the Current Landscape for Drug Development. Nat. Rev. Drug Discov. 18 (7), 527–551. doi:10.1038/s41573-019-0019-2
Su, Z., Dong, S., Zhao, S.-C., Liu, K., Tan, Y., Jiang, X., et al. (2021). Novel Nanomedicines to Overcome Cancer Multidrug Resistance. Drug Resist. Updates 58, 100777. doi:10.1016/j.drup.2021.100777
Svensson, R. U., Parker, S. J., Eichner, L. J., Kolar, M. J., Wallace, M., Brun, S. N., et al. (2016). Inhibition of Acetyl-CoA Carboxylase Suppresses Fatty Acid Synthesis and Tumor Growth of Non-small-cell Lung Cancer in Preclinical Models. Nat. Med. 22 (10), 1108–1119. doi:10.1038/nm.4181
Szakács, G., Paterson, J. K., Ludwig, J. A., Booth-Genthe, C., and Gottesman, M. M. (2006). Targeting Multidrug Resistance in Cancer. Nat. Rev. Drug Discov. 5 (3), 219–234. doi:10.1038/nrd1984
Tadros, S., Shukla, S. K., King, R. J., Gunda, V., Vernucci, E., Abrego, J., et al. (2017). De Novo Lipid Synthesis Facilitates Gemcitabine Resistance through Endoplasmic Reticulum Stress in Pancreatic Cancer. Cancer Res. 77 (20), 5503–5517. doi:10.1158/0008-5472.can-16-3062
Talebi, A., Dehairs, J., Rambow, F., Rogiers, A., Nittner, D., Derua, R., et al. (2018). Sustained SREBP-1-dependent Lipogenesis as a Key Mediator of Resistance to BRAF-Targeted Therapy. Nat. Commun. 9, 2500. doi:10.1038/s41467-018-04664-0
Tan, Y., Lin, K., Zhao, Y., Wu, Q., Chen, D., Wang, J., et al. (2018). Adipocytes Fuel Gastric Cancer Omental Metastasis via PITPNC1-Mediated Fatty Acid Metabolic Reprogramming. Theranostics 8 (19), 5452–5468. doi:10.7150/thno.28219
Tesfay, L., Paul, B. T., Konstorum, A., Deng, Z., Cox, A. O., Lee, J., et al. (2019). Stearoyl-CoA Desaturase 1 Protects Ovarian Cancer Cells from Ferroptotic Cell Death. Cancer Res. 79 (20), 5355–5366. doi:10.1158/0008-5472.can-19-0369
Tung, S., Shi, Y., Wong, K., Zhu, F., Gorczynski, R., Laister, R. C., et al. (2013). PPARα and Fatty Acid Oxidation Mediate Glucocorticoid Resistance in Chronic Lymphocytic Leukemia. Blood 122 (6), 969–980. doi:10.1182/blood-2013-03-489468
Ueda, S. M., Yap, K. L., Davidson, B., Tian, Y., Murthy, V., Wang, T.-L., et al. (2010). Expression of Fatty Acid Synthase Depends on NAC1 and Is Associated with Recurrent Ovarian Serous Carcinomas. J. Oncol. 2010, 1–12. doi:10.1155/2010/285191
Vazquez-Martin, A., Ortega-Delgado, F. J., Fernandez-Real, J. M., and Menendez, J. A. (2008). The Tyrosine Kinase Receptor HER2 (erbB-2): from Oncogenesis to Adipogenesis. J. Cel. Biochem. 105 (5), 1147–1152. doi:10.1002/jcb.21917
Ventura, R., Mordec, K., Waszczuk, J., Wang, Z., Lai, J., Fridlib, M., et al. (2015). Inhibition of De Novo Palmitate Synthesis by Fatty Acid Synthase Induces Apoptosis in Tumor Cells by Remodeling Cell Membranes, Inhibiting Signaling Pathways, and Reprogramming Gene Expression. Ebiomedicine 2 (8), 808–824. doi:10.1016/j.ebiom.2015.06.020
Verbrugge, S. E., Al, M., Assaraf, Y. G., Kammerer, S., Chandrupatla, D. M. S. H., Honeywell, R., et al. (2016). Multifactorial Resistance to Aminopeptidase Inhibitor Prodrug CHR2863 in Myeloid Leukemia Cells: Down-Regulation of Carboxylesterase 1, Drug Sequestration in Lipid Droplets and Pro-survival Activation ERK/Akt/mTOR. Oncotarget 7 (5), 5240–5257. doi:10.18632/oncotarget.6169
von Roemeling, C. A., Marlow, L. A., Wei, J. J., Cooper, S. J., Caulfield, T. R., Wu, K., et al. (2013). Stearoyl-CoA Desaturase 1 Is a Novel Molecular Therapeutic Target for clear Cell Renal Cell Carcinoma. Clin. Cancer Res. 19 (9), 2368–2380. doi:10.1158/1078-0432.ccr-12-3249
Vriens, K., Christen, S., Parik, S., Broekaert, D., Yoshinaga, K., Talebi, A., et al. (2019). Evidence for an Alternative Fatty Acid Desaturation Pathway Increasing Cancer Plasticity. Nature 566 (7744), 403–406. doi:10.1038/s41586-019-0904-1
Walther, T. C., Chung, J., and Farese, R. V. (2017). “Lipid Droplet Biogenesis,”. Editor R. Schekman, 33, 491–510. doi:10.1146/annurev-cellbio-100616-060608Annu. Rev. Cel Dev. Biol.
Wang, C. J., Li, D., Danielson, J. A., Zhang, E. H., Dong, Z., Miller, K. D., et al. (2021). Proton Pump Inhibitors Suppress DNA Damage Repair and Sensitize Treatment Resistance in Breast Cancer by Targeting Fatty Acid Synthase. Cancer Lett. 509, 1–12. doi:10.1016/j.canlet.2021.03.026
Wang, D., Yin, L., Wei, J., Yang, Z., and Jiang, G. (2017). ATP Citrate Lyase Is Increased in Human Breast Cancer, Depletion of Which Promotes Apoptosis. Tumour Biol. 39 (4), 101042831769833. doi:10.1177/1010428317698338
Wang, H., Zhang, Y., Lu, Y., Song, J., Huang, M., Zhang, J., et al. (2016). The Role of Stearoyl-Coenzyme A Desaturase 1 in clear Cell Renal Cell Carcinoma. Tumor Biol. 37 (1), 479–489. doi:10.1007/s13277-015-3451-x
Wang, T., Fahrmann, J. F., Lee, H., Li, Y.-J., Tripathi, S. C., Yue, C., et al. (2018a). JAK/STAT3-Regulated Fatty Acid β-Oxidation Is Critical for Breast Cancer Stem Cell Self-Renewal and Chemoresistance. Cel Metab. 27 (1), 136–150. e135. doi:10.1016/j.cmet.2017.11.001
Wang, Y.-n., Zeng, Z.-l., Lu, J., Wang, Y., Liu, Z.-x., He, M.-m., et al. (2018b). CPT1A-mediated Fatty Acid Oxidation Promotes Colorectal Cancer Cell Metastasis by Inhibiting Anoikis. Oncogene 37 (46), 6025–6040. doi:10.1038/s41388-018-0384-z
Wang, Y., Lu, J.-H., Wang, F., Wang, Y.-N., He, M.-M., Wu, Q.-N., et al. (2020). Inhibition of Fatty Acid Catabolism Augments the Efficacy of Oxaliplatin-Based Chemotherapy in Gastrointestinal Cancers. Cancer Lett. 473, 74–89. doi:10.1016/j.canlet.2019.12.036
Wangpaichitr, M., Sullivan, E. J., Theodoropoulos, G., Wu, C., You, M., Feun, L. G., et al. (2012). The Relationship of Thioredoxin-1 and Cisplatin Resistance: its Impact on ROS and Oxidative Metabolism in Lung Cancer Cells. Mol. Cancer Ther. 11 (3), 604–615. doi:10.1158/1535-7163.mct-11-0599
Warburg, O. (1956a). On Respiratory Impairment in Cancer Cells. Science 124 (3215), 269–270. doi:10.1126/science.124.3215.269
Warburg, O. (1956b). On the Origin of Cancer Cells. Science 123 (3191), 309–314. doi:10.1126/science.123.3191.309
Wen, J., Min, X., Shen, M., Hua, Q., Han, Y., Zhao, L., et al. (2019). ACLY Facilitates colon Cancer Cell Metastasis by CTNNB1. J. Exp. Clin. Cancer Res. 38 (1), 401. doi:10.1186/s13046-019-1391-9
Wise, D. R., DeBerardinis, R. J., Mancuso, A., Sayed, N., Zhang, X.-Y., Pfeiffer, H. K., et al. (2008). Myc Regulates a Transcriptional Program that Stimulates Mitochondrial Glutaminolysis and Leads to Glutamine Addiction. Proc. Natl. Acad. Sci. U.S.A. 105 (48), 18782–18787. doi:10.1073/pnas.0810199105
Wong, R. S. (2011). Apoptosis in Cancer: from Pathogenesis to Treatment. J. Exp. Clin. Cancer Res. 30, 14. doi:10.1186/1756-9966-30-87
Xie, Y., Hou, W., Song, X., Yu, Y., Huang, J., Sun, X., et al. (2016). Ferroptosis: Process and Function. Cell Death Differ 23 (3), 369–379. doi:10.1038/cdd.2015.158
Xin, M., Qiao, Z., Li, J., Liu, J., Song, S., Zhao, X., et al. (2016). miR-22 Inhibits Tumor Growth and Metastasis by Targeting ATP Citrate Lyase: Evidence in Osteosarcoma, Prostate Cancer, Cervical Cancer and Lung Cancer. Oncotarget 7 (28), 44252–44265. doi:10.18632/oncotarget.10020
Xu, C. Z., Xie, J., Jin, B., Chen, X. W., Sun, Z. F., Wang, B. X., et al. (2013). Gene and microRNA Expression Reveals Sensitivity to Paclitaxel in Laryngeal Cancer Cell Line. Int. J. Clin. Exp. Pathol. 6 (7), 1351–1361.
Yang, L., Zhang, F., Wang, X., Tsai, Y., Chuang, K.-H., Keng, P. C., et al. (2016). A FASN-TGF-Β1-FASN Regulatory Loop Contributes to High EMT/metastatic Potential of Cisplatin-Resistant Non-small Cell Lung Cancer. Oncotarget 7 (34), 55543–55554. doi:10.18632/oncotarget.10837
Yang, W. S., and Stockwell, B. R. (2016). Ferroptosis: Death by Lipid Peroxidation. Trends Cel Biol. 26 (3), 165–176. doi:10.1016/j.tcb.2015.10.014
Yang, X., Fraser, M., Abedini, M. R., Bai, T., and Tsang, B. K. (2008). Regulation of Apoptosis-Inducing Factor-Mediated, Cisplatin-Induced Apoptosis by Akt. Br. J. Cancer 98 (4), 803–808. doi:10.1038/sj.bjc.6604223
Yang, Y., Liu, H., Li, Z., Zhao, Z., Yip-Schneider, M., Fan, Q., et al. (2011). Role of Fatty Acid Synthase in Gemcitabine and Radiation Resistance of Pancreatic Cancers. Int. J. Biochem. Mol. Biol. 2 (1), 89–98.
Yasumoto, Y., Miyazaki, H., Vaidyan, L. K., Kagawa, Y., Ebrahimi, M., Yamamoto, Y., et al. (2016). Inhibition of Fatty Acid Synthase Decreases Expression of Stemness Markers in Glioma Stem Cells. Plos One 11 (1), e0147717. doi:10.1371/journal.pone.0147717
Ye, H., Adane, B., Khan, N., Sullivan, T., Minhajuddin, M., Gasparetto, M., et al. (2016). Leukemic Stem Cells Evade Chemotherapy by Metabolic Adaptation to an Adipose Tissue Niche. Cell Stem Cell 19 (1), 23–37. doi:10.1016/j.stem.2016.06.001
Ye, X., and Weinberg, R. A. (2015). Epithelial-Mesenchymal Plasticity: A Central Regulator of Cancer Progression. Trends Cel Biol. 25 (11), 675–686. doi:10.1016/j.tcb.2015.07.012
Yosef, H. K., Mavarani, L., Maghnouj, A., Hahn, S., El-Mashtoly, S. F., and Gerwert, K. (2015). In Vitro prediction of the Efficacy of Molecularly Targeted Cancer Therapy by Raman Spectral Imaging. Anal. Bioanal. Chem. 407 (27), 8321–8331. doi:10.1007/s00216-015-8875-z
Yoshii, Y., Furukawa, T., Saga, T., and Fujibayashi, Y. (2015). Acetate/acetyl-CoA Metabolism Associated with Cancer Fatty Acid Synthesis: Overview and Application. Cancer Lett. 356 (2 Pt A), 211–216. doi:10.1016/j.canlet.2014.02.019
Yumoto, K., Eber, M. R., Berry, J. E., Taichman, R. S., and Shiozawa, Y. (2014). Molecular Pathways: Niches in Metastatic Dormancy. Clin. Cancer Res. 20 (13), 3384–3389. doi:10.1158/1078-0432.ccr-13-0897
Yun, H. J., Kim, J. Y., Kim, G., and Choi, H. S. (2014). Prolyl-isomerase Pin1 Impairs Trastuzumab Sensitivity by Up-Regulating Fatty Acid Synthase Expression. Anticancer Res. 34 (3), 1409–1416.
Zaidi, N., Swinnen, J. V., and Smans, K. (2012). ATP-citrate Lyase: a Key Player in Cancer Metabolism. Cancer Res. 72 (15), 3709–3714. doi:10.1158/0008-5472.can-11-4112
Zhang, C., and Liu, P. (2017). The Lipid Droplet: A Conserved Cellular Organelle. Protein Cell 8 (11), 796–800. doi:10.1007/s13238-017-0467-6
Zhang, J., Song, F., Zhao, X., Jiang, H., Wu, X., Wang, B., et al. (2017). EGFR Modulates Monounsaturated Fatty Acid Synthesis through Phosphorylation of SCD1 in Lung Cancer. Mol. Cancer 16 (1), 127. doi:10.1186/s12943-017-0704-x
Zhang, M., Peng, R., Wang, H., Yang, Z., Zhang, H., Zhang, Y., et al. (2022). Nanog Mediated by FAO/ACLY Signaling Induces Cellular Dormancy in Colorectal Cancer Cells. Cel Death Dis 13 (2), 159. doi:10.1038/s41419-022-04606-1
Zhang, Y., and Zhang, Z. (2020). The History and Advances in Cancer Immunotherapy: Understanding the Characteristics of Tumor-Infiltrating Immune Cells and Their Therapeutic Implications. Cell Mol Immunol 17 (8), 807–821. doi:10.1038/s41423-020-0488-6
Zhao, J. (2016). Cancer Stem Cells and Chemoresistance: The Smartest Survives the Raid. Pharmacol. Ther. 160, 145–158. doi:10.1016/j.pharmthera.2016.02.008
Zhao, X., Zhao, L., Yang, H., Li, J., Min, X., Yang, F., et al. (2018). Pyruvate Kinase M2 Interacts with Nuclear Sterol Regulatory Element-Binding Protein 1a and Thereby Activates Lipogenesis and Cell Proliferation in Hepatocellular Carcinoma. J. Biol. Chem. 293, 6623–6634. doi:10.1074/jbc.RA117.000100
Zheng, X., Carstens, J. L., Kim, J., Scheible, M., Kaye, J., Sugimoto, H., et al. (2015). Epithelial-to-mesenchymal Transition Is Dispensable for Metastasis but Induces Chemoresistance in Pancreatic Cancer. Nature 527 (7579), 525–530. doi:10.1038/nature16064
Keywords: drug resistance, Cancer chemoresistance, fatty acid metabolism, lipid metabolism, multi-drug resistance
Citation: Yang R, Yi M and Xiang B (2022) Novel Insights on Lipid Metabolism Alterations in Drug Resistance in Cancer. Front. Cell Dev. Biol. 10:875318. doi: 10.3389/fcell.2022.875318
Received: 14 February 2022; Accepted: 13 April 2022;
Published: 13 May 2022.
Edited by:
Markus Ritter, Paracelsus Medical University, AustriaReviewed by:
Dino Bekric, Paracelsus Medical University, AustriaParmanand Malvi, Yale University School of Medicine, United States
Copyright © 2022 Yang, Yi and Xiang. This is an open-access article distributed under the terms of the Creative Commons Attribution License (CC BY). The use, distribution or reproduction in other forums is permitted, provided the original author(s) and the copyright owner(s) are credited and that the original publication in this journal is cited, in accordance with accepted academic practice. No use, distribution or reproduction is permitted which does not comply with these terms.
*Correspondence: Mei Yi, eWlfbWVpQGNzdS5lZHUuY24=; Bo Xiang, eGlhbmdib2xpbkBjc3UuZWR1LmNu