- 1Faculty of Environment and Life, Beijing University of Technology, Beijing, China
- 2Beijing Molecular Hydrogen Research Center, Beijing, China
Ferroptosis is a newly defined programmed cell death, which by its mechanism differs from other programmed cell death processes such as apoptosis, necrosis, and autophagy. It has a unique morphology and biological properties that antioxidants and iron-chelating agents can regulate. Ferroptosis has the characteristics of iron ion deposition and dependence on lipid peroxidation. It can affect the progression of many cancers, including liver cancer, by inducing an intracellular iron-dependent accumulation of reactive oxygen species, providing new possibilities for cancer treatment. At present, great progress has been made in exploring the molecular mechanism of ferroptosis. In this review, we summarize the characteristics, mechanisms, and regulatory factors of ferroptosis in detail, discuss the progress of ferroptosis research in liver cancer, and provide directions and new ideas for the treatment of hepatocellular carcinoma.
1 Introduction
Regulatory cell death (RCD) is a common process in organisms, essential to restoring tissue homeostasis or biological balance after stress. RCD is defined as a death process dependent on specific molecular mechanisms, which can be regulated through specific pharmacological and genetic interventions to promote the selective removal of harmful cells or activate specific pathological states (Del Re et al., 2019). RCD occurs as a homeostatic mechanism during development and aging, but could also originate from disturbances in the intracellular or extracellular microenvironment (Thompson, 1995). In addition to RCD, other forms of death programs have been described (Del Re et al., 2019). In 2003, Dolma et al. discovered a nonapoptotic form of cell death induced by Erastin in tumors with RAS mutations (Dolma et al., 2003). Later in 2008, they identified two additional compounds, RSL3 and RSL5, with the same nonapoptotic cell death-inducing potential as Erastin (Yang and Stockwell, 2008). The newly discovered death program was later identified in 2012 as an intracellular iron-dependent form of cell death caused by cellular accumulation of lipid peroxides and was called ferroptosis (Dixon et al., 2012). Ferroptosis cells have unique morphological and bioenergy characteristics that differentiate them from other forms of regulated cell death such as apoptosis and necrosis (Dixon et al., 2012; Bersuker et al., 2019; Xia et al., 2019). At the subcellular level, mitochondria in ferroptosis cells are smaller, have a higher membrane density, have cristae that shrink or disappear and show rupture of the outer mitochondrial membrane (Dixon et al., 2012; Friedmann Angeli et al., 2014; Hassannia et al., 2019). Ferroptosis can be induced by various types of small molecules (Table 1), including Erastin and derivatives, sulfasalazine (SAS), glutamate, and drugs such as Sorafenib, cisplatin, artemisinin, and lanperisone (Stockwell et al., 2017; Yu et al., 2017). These molecules act on the system Xc- and reduce intracellular glutathione content resulting in a cellular redox imbalance (Gout et al., 2001; Yagoda et al., 2007; Dixon et al., 2012). Other inducers, including RAS selective lethal compound 3 (RSL3), DPI2, DPI7, directly inhibit glutathione peroxidase 4 (GPX4), resulting in accumulation of lipid peroxides (Yang et al., 2014). Furthermore, the glutathione synthesis interrupter butylthionyl sulfoxide (BSO) can also induce ferroptosis (Griffith, 1982; Shaw et al., 2011; Dixon et al., 2012; Dixon et al., 2014; Eling et al., 2015; Louandre et al., 2015; Lőrincz et al., 2015; Sun et al., 2020). Since the increase in reactive oxygen species (ROS) and iron accumulation are the two most important factors in the ferroptosis process, antioxidants such as ferrostatins-1 and liproxstatins, exogenous iron chelating agents such as DFO (Dixon et al., 2012; Friedmann Angeli et al., 2014; Yang et al., 2014; Chen et al., 2021), and endogenous compounds such as glutathione, ubiquinone, vitamin E and selenium (Dixon et al., 2012; Homma and Fujii, 2015; Manz et al., 2016; Stockwell et al., 2017) can be used as inhibitors of ferroptosis.
Ferroptosis is associated with various physiological and pathological processes (Jenkins et al., 2020; Shen et al., 2020; Jiang et al., 2021), and its pathophysiological relevance has been well documented in a growing number of diseases such as neurodegeneration (Devos et al., 2014; Chen et al., 2015; Hambright et al., 2017), fibrosis (Zhang et al., 2018; Yu et al., 2020), autoimmune (Hu et al., 2019; Kim et al., 2019) and pulmonary diseases (Park et al., 2019). Ferroptosis has also been proven to be very important for various tumors, including hepatocellular carcinoma, lung cell carcinoma, lymphoma, pancreatic ductal cell carcinoma, and renal cell carcinoma (Louandre et al., 2013; Yang et al., 2016; Chen et al., 2021; Li et al., 2021). In this review, we focus on research progress on ferroptosis in liver cancers and provide the latest information on the basic mechanisms that contribute to the regulation of ferroptosis in this cancer.
2 General Overview of the Mechanisms of Ferroptosis
Induction of ferroptosis required iron and iron-dependent peroxidation enzymes (Yang and Stockwell, 2008; Wenzel et al., 2017), phospholipids with polyunsaturated fatty acids, and inhibition of the pathways involved in the reparation of lipid peroxidation. The contribution of these three hallmarks to ferroptosis has been extensively investigated, and current knowledge of their mechanism in the process is reviewed and summarized in Figure 1 and Table 2.
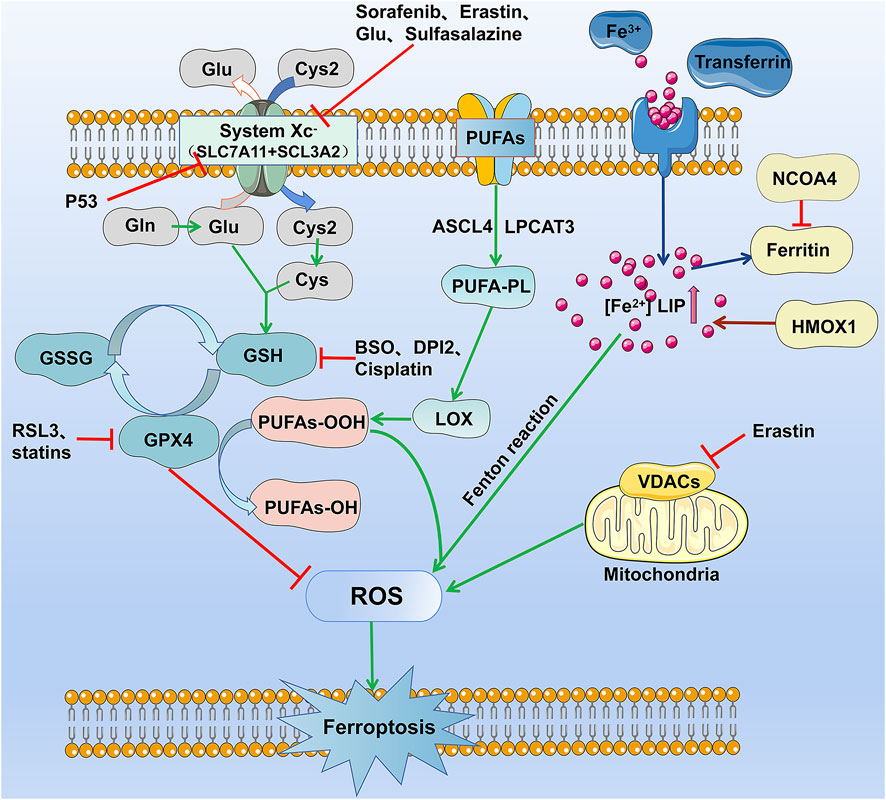
FIGURE 1. The occurrence and regulation mechanism of ferroptosis in cells. The figure highlights the five currently known mechanisms involved in ferroptosis: lipid reactive oxygen metabolism pathway, cystine glutamate transport receptor (System Xc-) metabolic pathway, iron metabolism pathway, and VDAC receptor pathway.
2.1 Regulation of Iron Homeostasis and Implications for Ferroptosis
Iron is an important factor in the formation of free radicals and lipid peroxidation and plays a pivotal role in ferroptosis. Increased iron absorption and decreased iron output make cancer cells sensitive to oxidative damage and ferroptosis (Liang et al., 2019). Transferrin and its receptors transport iron into the cell and store it in the form of ferritin, while intracellular iron is exported through ferroportin to maintain iron balance in the cell (De Domenico et al., 2008; Trujillo-Alonso et al., 2019). The labile iron pool (LIP) exists mainly in the cytoplasm in the form of Fe2+, which can directly catalyze the formation of hydroxyl radicals with strong activity through the Fenton reaction and further promote lipid peroxidation (Gaschler and Stockwell, 2017; Yan et al., 2021). In eukaryotes and most prokaryotes, iron participates in the synthesis of iron-sulfur clusters (Fe-S), heme, and other cofactors. It is mainly involved in energy metabolism, oxygen transport and metabolism, cell respiration and electron transfer, signal transduction, central nervous system myelin, various neurotransmitter formation, DNA replication and repair, enzyme reaction, and other important physiological processes (Beard, 2001; Cronin et al., 2019; Braymer et al., 2021). Furthermore, iron and its derivatives, such as heme or iron-sulfur [Fe-S] clusters, can affect the activity of enzymes that catalyze ROS production, such as NADPH oxidases (NOX), lipoxygenases (LOX), and mitochondrial electron transport complexes, which stimulate ROS production and thus lead to ferroptosis (Wang et al., 2020). In addition to its role in ferroptosis, iron is an essential trace element for normal body functioning. Iron regulates different biological processes, including the cellular metabolism of proteins and enzymes, and defects in maintaining its cellular homeostasis by iron overload or iron deficiency could lead to human disorders (De Domenico et al., 2008; Ng et al., 2020).
The cytosolic iron pool can be affected in various ways. For example, inhibition of nitrogen fastening 1 (NFS1), a cysteine desulfurase, sensitizes cells to ferroptosis by increasing the transferrin receptor (TFRC) and decreasing ferritin levels (FTH) by decompressing cysteine sulfur to produce a group of iron-sulfur (Alvarez et al., 2017). The lysosomal degradation of ferritin can lead to a large amount of cytosolic iron accumulation. This process involves Nuclear Receptor Coactivator 4 (NCOA4), which can bind and transport ferritin to autophagosomes where ferritin is degraded and lysosomal iron exported in the cytosol. Therefore, inhibition of lysosomal activity or silencing of NCOA4 can inhibit ferroptosis (Mancias et al., 2014; Quiles del Rey and Mancias, 2019; Mou et al., 2021). HMOX1 catalyzes the degradation of heme to Fe2+, biliverdin, and carbon monoxide, enhancing ferroptosis by increasing LIP (Kwon et al., 2015; Chang et al., 2018; Conrad and Pratt, 2019). Besides, HMOX1 can also impact cell protection through its antioxidant activity (Sun et al., 2016; Conrad and Pratt, 2019). The circulating peptide hormone Hepcidin, a beta-defensin-like peptide coded by the HAMP gene and secreted primary by hepatocytes, is a principal regulator of systemic iron homeostasis (Verga Falzacappa and Muckenthaler, 2005; De Domenico et al., 2008; Yang et al., 2020). Hepcidin acts as a negative regulator of iron transport into plasma by binding to the ferroportin, causing its internalization and lysosomal degradation (Nemeth et al., 2004; De Domenico et al., 2007), leading to an increase in the level of cytosolic iron that explains the contribution of hepcidin to ferroptosis regulation (Yang et al., 2020).
2.2 System XC− and Ferroptosis
Cysteine/Glutamate antiporter referred to as System XC− is a heterodimer composed of SLC7A11 and SLC3A2, a sulfide-linked system, and an important target for the induction of ferroptosis. System XC− facilitates the import of cystine and glutamate export in a 1:1 ratio. Cystine that enters the cell via the antiporter is reduced to cysteine and used in the biosynthesis of reduced glutathione (GSH) (Jiang et al., 2021). GSH is a tripeptide of glutamate, cysteine, and glycine, synthesized by the consecutive action of the cytoplasmic enzymes glutamate-cysteine ligase (GCL) and glutathione synthase (GSS), respectively (Meister, 1995). GSH is the most abundant antioxidant in mammalian cells, and it prevents the cellular accumulation of reactive oxygen species (ROS). Therefore, depletion of cysteine by deletion of SLC7A11 or through inhibition of the system Xc− using chemical probes such as Erastin or Sorafenib results in a decrease in the level of GSH, impaired glutathione peroxidase 4 (GPX4) activity, accumulation of ROS, and subsequent lipid peroxidation, required for the execution of ferroptosis-mediated cell death (Yang et al., 2014; Badgley et al., 2020; Stockwell and Jiang, 2020).
2.3 Lipid Peroxidation in Ferroptosis
Lipid peroxidation is the hallmark of ferroptosis. It is a complex biological process of oxidative degradation of lipids observed in plants and animals. It is a chain of enzymatic and nonenzymatic reactions initiated by hydrogen abstraction or the addition of oxygen radicals, resulting in the oxidative damage of polyunsaturated fatty acids (PUFA) (Yin et al., 2011). Nonenzymatic lipid peroxidation is a free radical-driven chain reaction in which reactive oxygen species (ROS) trigger polyunsaturated fatty acid oxidation (Vigor et al., 2014) and are facilitated by Fe2+ (Repetto et al., 2010; Gaschler and Stockwell, 2017). During this process, hydroxyl radicals extract hydrogen from polyunsaturated fatty acids to produce carbon-centered phospholipid radicals that subsequently react with oxygen to form lipid peroxide radicals (PLOO·) (Hassannia et al., 2019). The PLOO can propagate the chain reaction by extracting another hydrogen from adjacent polyunsaturated fatty acids to form lipid hydroperoxide (PLOOH) and a new lipid free radical, triggering another chain reaction of lipid peroxidation (Yin et al., 2011; Gaschler and Stockwell, 2017; Maiorino et al., 2018; Conrad and Pratt, 2019). In contrast, enzymatic lipid peroxidation is mediated in a controlled manner by the activity of LOXs (Gaschler and Stockwell, 2017; Conrad and Pratt, 2019) and cyclooxygenases (COXs) (Rouzer and Marnett, 2003). LOX, a non-heme iron dioxygenase, and COXs catalyze the dioxygenation of free and esterified PUFA to produce various lipid hydroperoxides, PLOOH. In mammalian cells, linoleic acid (LA) and arachidonic acid (AA) are the most abundant PUFA and substrates for LOX. Free PUFA can be esterified by activation of the acyl-coenzyme A synthase long-chain family member 4 (ACSL4) and bound to membrane phospholipids by lysophosphatidylcholine acyltransferase 3 (LPCAT3). ACSL4 up-regulation is considered a biomarker and contributor to ferroptosis (Doll et al., 2017; Lu et al., 2018). In the presence of ferrous iron, PLOOH can be broken down to the alkoxy lipid radical (PLO), which promotes further spread of lipid peroxidation by binding to another PUFA; on the other hand, PLOOH can break down into 4-hydroxynonenal (4-HNE) or malondialdehyde (MDA), causing the formation of adducts that disrupts the structure and/or function of proteins. Peroxidation of phospholipid and production of 4-HNE or MDA can cause membrane instability and permeability, leading to cell death. When inhibition of lipid peroxidation is out of control, this iron and oxygen catalyzed chain process leads to membrane destruction and to ferroptosis (Yin et al., 2011; Gaschler and Stockwell, 2017; Conrad and Pratt, 2019).
2.4 GPX4 and GPX4-Independent Regulation of Ferroptosis
Although the importance of the GSH-GPX4 axis in ferroptosis, recent work has uncovered GPX4-independent mechanisms that control ferroptosis. These mechanisms are summarized in the sections below.
2.4.1 GSH-Glutathione Peroxidase 4(GPX4) Axis
GSH-GPX4 axis is considered the main system that controls ferroptosis in mammals. Glutathione peroxidase 4 (GPX4) can reduce reactive phospholipid hydroperoxides (PL-OOH) to nonreactive phospholipid alcohols (PL-OH), which can interrupt free radical chain reactions, inhibit lipid peroxidation, and thus suppress ferroptosis. GPX4 does so using its catalytic selenocysteine residue and two electrons donated by GSH or low-molecular thiols or protein thiols (Maiorino et al., 2018; Jiang et al., 2021). GSH depletion caused by cysteine deprivation directly inactivates GPX4 and leads to subsequent ferroptosis. Moreover, alteration of GPX4 activity by pharmacological inhibitors such as RSL3 or Altretamine or genetic methods leads to rapid accumulation of lipid ROS, which can cause ferroptosis (Shen et al., 2018; Weiland et al., 2019; Jiang et al., 2021).
2.4.2 Ferroptosis Suppressor Protein 1 (FSP1)
FSP1, known for its role in transporting and folding mitochondrial intermembrane proteins (Reinhardt et al., 2020), was found to protect cells from ferroptosis induced by inhibition or genetic deletion of GPX4 (Bersuker et al., 2019; Doll et al., 2019). The anti-ferroptosis function of FSP1 is based on its NADH:ubiquinone oxidoreductase activity (Elguindy and Nakamaru-Ogiso, 2015), through which it suppresses lipid peroxidation by reducing ubiquinone to ubiquinol, which in turn may directly reduce lipid radicals to end lipid autoxidation or indirectly by regenerating the antioxidant, vitamin E (Elguindy and Nakamaru-Ogiso, 2015; Bersuker et al., 2019; Doll et al., 2019).
2.4.3 Voltage-Dependent Anion Channel (VDAC)
The first described inducer of ferroptosis, Erastin, binds directly to two isoforms of the VDAC family, VDAC2 and VDAC3, and this interaction was required for Erastin-mediated lethality (Yagoda et al., 2007). Located in the outer mitochondrial membrane, VDAC mediates and controls the exchange of ions and metabolites between mitochondria and cytoplasm in eukaryotic cells through dynamic gating interaction. When VDAC is closed, mitochondrial transport function is restricted, metabolism is inhibited, and a low ATP/ADP ratio is maintained, thus reducing oxidative stress (Maldonado et al., 2010; Lemasters, 2017). The opening of VDAC mediates the entry of respiratory substrates, ADP, phosphoric acid, and other substances into mitochondria, leading to increased mitochondrial metabolism, reduced glycolysis, and increased ROS production (Lemasters, 2017). Erastin combines with VDAC2 and VDAC3 in the outer mitochondrial membrane to change membrane permeability, slow the oxidation of NADH, and change the ion selectivity of the channel, allowing only cations to enter mitochondria (Yagoda et al., 2007; Maldonado et al., 2013); this leads to increased ROS production and increased lipid peroxidation, which in turn causes ferroptosis (Yagoda et al., 2007; Maldonado et al., 2013).
2.4.4 Protein Kinases in Ferroptosis
Ferroptosis involves multiple signaling pathways that can dictate cell susceptibility to ferroptosis under specific biological conditions. For example, the activation of the Ras-RAF-MEK-ERK pathway is necessary for Erastin-induced cell death in tumor cells harboring activating mutations in the RAS-RAF-MEK pathway (Xie et al., 2016) but not in acute myeloid leukemia where only inhibition of p38 and JNK was associated with resistance to Erastin-induced cell death (Yagoda et al., 2007; Yu et al., 2015) or in human pancreatic islet-like cells where p38 and JNK activation was necessary for Erastin induced ferroptosis to occur (Li and Leung, 2020) or to cold-induced ferroptosis in multiple cell lines (Hattori et al., 2017). A wide variety of agents activate AMP-activated protein kinase (AMPK) (Hawley et al., 2010), and the stress condition underlying this activation is determinant for the AMPK function during ferroptosis (Zhu et al., 2019; Zhao et al., 2020b; Lee et al., 2020; Yao et al., 2021). Recently, activation of the cellular energy sensor AMPK under energy stress induced by glucose depravation was found to block ferroptosis by impairing the biosynthesis of PUFAs, essential for lipid peroxidation that drives ferroptosis (Lee et al., 2020; Yao et al., 2021). The same AMPK activation, but this time under non-metabolic stress conditions, was required for ferroptosis induction in several experimental conditions (Zhu et al., 2019; Zhao et al., 2020b). In addition to MAPKs and AMPK, several other kinases have been reported as a positive or negative regulators of ferroptosis (Zhang et al., 2020a; Zhao et al., 2020a; Zhang et al., 2021; Zhong et al., 2021).
2.4.5 Cell Cycle Regulators in Ferroptosis
The tumor suppressor p53, encoded by the TP53 gene, is a key regulator of cell cycle, senescence, and apoptosis and plays an important role in the occurrence and development of tumors (Liu J. et al., 2020). Beyond the functions mentioned above, p53 is believed to also control ferroptosis through complex mechanisms involving transcriptional and post-transcriptional modifications and is reviewed in detail elsewhere (Gnanapradeepan et al., 2018; Kang et al., 2019; Liu J. et al., 2020). Moreover, several direct targets of p53, including SLC7A11, GLS2, PTGS2, and SAT1, have been discovered to play a role in ferroptosis. The tumor suppressor p53 could act as an inducer or inhibitor of ferroptosis depending on cell types, energy state, and p53 status (Gnanapradeepan et al., 2018). Besides p53, the loss of function of the retinoblastoma (Rb) protein, well known for its ability to regulate the activity of the transcription factors E2F, was found to promote Sorafenib induced ferroptosis in hepatocellular carcinoma (Louandre et al., 2015). Furthermore, p21 encoded by the CDKN1A gene was a barrier to ferroptosis independent of p53 (Venkatesh et al., 2020). These data suggest a probable implication of cell cycle regulators in the ferroptosis process (Jiang et al., 2015).
3 Research Progress on Ferroptosis in HCC
Current research has shown that ferroptosis could be induced in many cancers such as hepatocellular carcinoma, lung cell carcinoma, lymphoma, pancreatic ductal cell carcinoma, and renal cell carcinoma and could be considered a therapeutic strategy. HCC is one of the most common primary malignant tumors and the third leading cause of cancer-related death (Siegel et al., 2019). Generally, surgical resection and liver transplantation can treat liver cancer if diagnosed in its early stages. However, in advanced stages, only Sorafenib is currently approved by the FDA for advanced HCC (Roxburgh and Evans, 2008; Villanueva, 2019). Many other therapies have been tested in clinical trials for the past decades, but most of them did not receive approval for HCC patients. Even some of the approved drugs later failed to inhibit tumor growth due to the emergence of resistance mechanisms. Therefore, it is important to find new and better treatment strategies for patients with HCC, which continues to increase. Ferroptosis, which is considered the most promising tumor growth inhibitor, can affect the occurrence and development of HCC by regulating intracellular iron levels and intracellular reactive oxygen species, providing new treatment options for patients with liver cancer (Couri and Pillai, 2019). This section focuses mainly on current research progress that evaluated ferroptosis in HCC and highlights the mechanisms involved in the process (Figure 2 and Table 3).
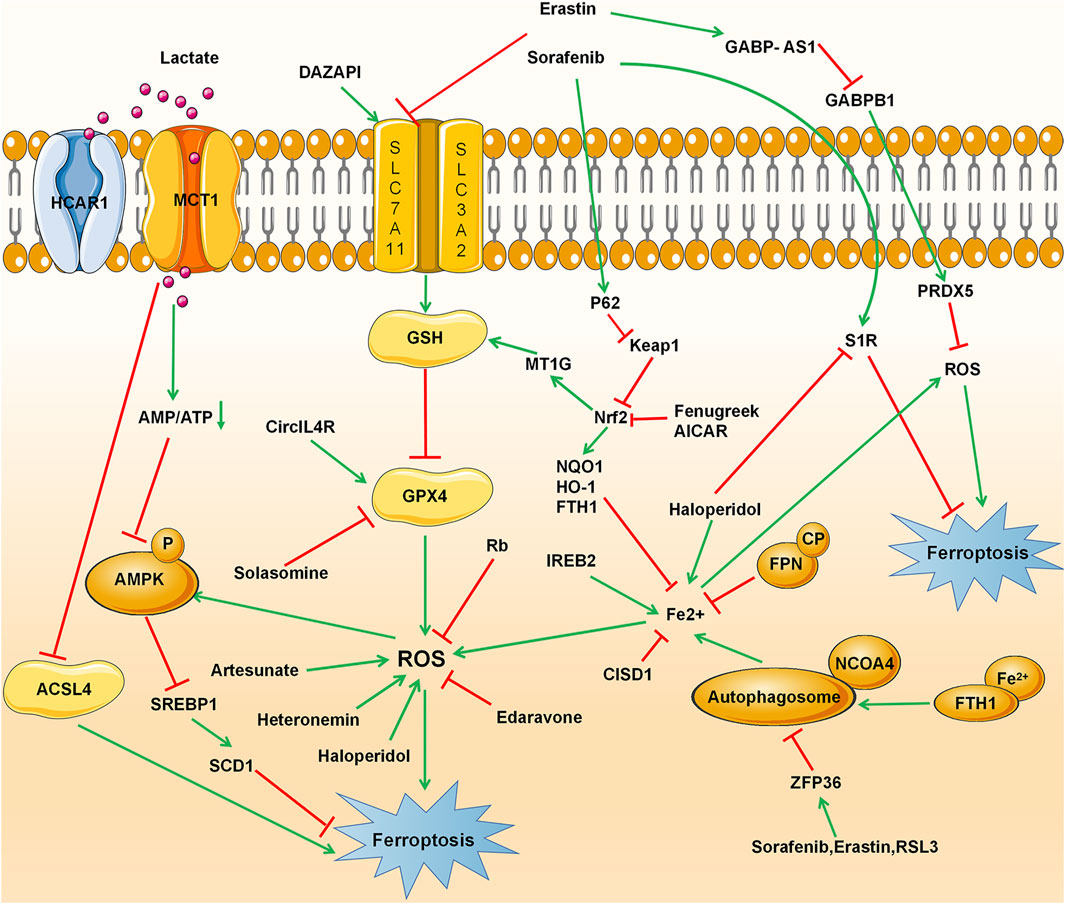
FIGURE 2. Ferroptosis signaling pathway in hepatocellular carcinoma (HCC), including conventional drivers and suppressors, non-coding RNAs, RNA-binding proteins, ACSL4, and metallothionein.
3.1 Conventional Drivers and Suppressors of Ferroptosis in HCC
Currently, a large number of studies have shown that in addition to traditional ferroptosis inducers such as inhibitors of GPX4 and system Xc- (Table1), many other substances can induce and play an important role in the ferroptosis process in HCC. Some of these substances may act alone, while others must be combined with conventional ferroptosis inducers or chemotherapies. Recently, a marine terpenoid, heteronemin, has been found to inhibit HCC cell lines HA22T and HA59T through ROS-MAPK-mediated apoptosis and ferroptosis (Chang et al., 2021). At the same time, Saponin Formosanin C, a natural compound isolated from Paris formosana Hayata, has been found to induce ferroptosis in HepG2 cells with higher levels of NCOA4 and lower levels of ferritin heavy chain 1 (FTH1) (Lin et al., 2020). Solasonine, obtained from Solanum melongena, has been proposed to act as a GPX4 inhibitor that promotes HCC cell lines HepG2 and HepRG ferroptosis by destroying the glutathione peroxidase 4-induced glutathione redox system (Jin et al., 2020). In a study led by Li et al., Artesunate, a clinically well-tolerated compound, synergized with sorafenib in inducing ferroptosis in HCC cell lines Huh7, SNU-449, and SNU-182 (Li et al., 2021). Sun et al. has shown that the combination therapy of Quiescin sulfhydryl oxidase 1 (QSOX1) and sorafenib sensitized HCC cells to oxidative stress by inhibiting activation of the master antioxidant transcription factor NRF2 and proposed QSOX1 to serve as a new therapeutic target in HCC or other types of EGFR-dependent tumors (Sun et al., 2021). Other approaches combining Auranofin and BSO or Erastin and BSO have shown a beneficial effect in Huh7 cells by promoting ferroptosis induced by inhibition of GPX4 (Lippmann et al., 2020). The psychotropic drugs haloperidol, which binds to the sigma 1 receptor (S1R), has been found to improve erastin and sorafenib-induced ferroptosis by increasing cellular levels of Fe2+ and lipid peroxidation and decreasing the level of cellular GSH (Bai et al., 2017).
In addition to inducing ferroptosis, preventing its inhibition could also be an alternative strategy for HCC treatment. Shang et al. have shown that Ceruloplasmin (CP), a copper-containing glycoprotein and member of the multicopper oxidase family (Vashchenko and MacGillivray, 2013), can suppress erastin and RSL3 by regulating iron homeostasis and lipid reactive oxygen species in HCC cell lines HepG2 and Hep3B (Shang et al., 2020). Lactate, commonly found in the microenvironment of aerobic glycolytic cancer (de la Cruz-López et al., 2019; Kim and DeBerardinis, 2019; Pérez-Tomás and Pérez-Guillén, 2020), was recently identified to enhance resistance to ferroptosis damage induced by ferroptosis inducers RSL3 and erastin when it is uptake into HCC cells through the monocarboxylate transporter 1 (MCT1). These lactate-rich cancer cells has been found to deactivate AMP-activated protein kinase (AMPK), leading to upregulation of sterol regulatory element-binding protein 1 (SREBP1) and downstream stearoyl-coenzyme A (CoA) desaturase-1 (SCD1), which enhance the production of monounsaturated fatty acids with anti-ferroptosis properties (Zhao et al., 2020b). Several other ferroptosis inhibitors have also been observed in HCC, among which the Sigma-1 receptor (S1R) that regulates the accumulation of reactive oxygen species through NRF2 (Bai et al., 2019), or the O-GlcNAcylated c-Jun that controls GSH synthesis (Chen et al., 2019) and Edaravone, a clinically approved free radical scavenger for the treatment of acute ischemic stroke and amyotrophic lateral sclerosis (Homma et al., 2019) have been showed to protect mouse hepatoma Hepa 1-6 cells from ferroptosis.
3.2 Other Regulators of Ferroptosis in HCC
Identifying new therapeutic targets or prognostic markers is of great significance in developing a precise and better treatment for liver cancer. In addition to the conventional ferroptosis inducers and inhibitors, several other regulators have been identified and could be considered potential targets for treating HCC patients.
3.2.1 Non-Coding RNAs and RNA-Binding Proteins in Ferroptosis of HCC
MiRNAs and RNA-biding proteins are pivotal participants and regulators in the development and progression of cancers. It is imperative to fully understand their regulatory networks and explore their therapeutic potential in HCC. Bai et al. have found that MicroRNA-214-3p inhibits ATF4 in HCC cells HepG2 and Hep3B, accelerates ferroptosis, and can be used as a new therapeutic target or prognostic marker for HCC treatment (Bai et al., 2020). Similarly, ubiquitin-like modifier activating enzyme 1 (UBA1), which has been reported to participate in the development of HCC by modulating cell phenotypes and ferroptosis via the NRF2 pathway, is proposed to be a promising diagnostic and prognostic indicator for HCC (Shan et al., 2020). In addition, other non-coding RNAs such as the LncRNA GABPB1-AS1 may be key molecules that regulate the ferroptosis process of HCC cells HepG2 caused by erastin (Qi et al., 2019). Circular RNAs (circRNAs) are a new class of non-coding RNAs backspliced from pre-mRNAs (Memczak et al., 2013). Circular RNAs (circ) are usually dysregulated in human diseases, including cancers (Barrett and Salzman, 2016; Bolha et al., 2017; Chen et al., 2017; Bi et al., 2018; Su et al., 2020), and have been confirmed to be involved in various malignant behaviors of HCC (Wang et al., 2018). The circIL4R is abnormally overexpressed in HCC tissues and cells, and its knockdown prevents HCC cell tumorigenesis and accelerates ferroptosis. CircIL4R directly sponges microRNA-541-3p, and inhibition of miR-541-3p mitigated the effects of circIL4R knockdown on HCC cells; this suggests that circIL4R served as a tumor promoter and ferroptosis inhibitor in HCC by the miR-541-3p/GPX4 network (Xu et al., 2020). Recently, another circular RNA, cIARS, has been described as an important positive regulator of sorafenib-induced ferroptosis by suppressing the inhibition of autophagy mediated by the RNA binding protein ALKBH5 (Liu Z. et al., 2020).
In addition to miRNAs, RNA-binding proteins (RBP) were recently found to play roles in ferroptosis. For example, Qi Wang et al. have shown that the RNA-binding protein DAZAP1 could suppress ferroptosis in HCC cells HepG2, SMMC-7721, Hep3B, Bel-7402, Huh7 and L02 by interacting with the SLC7A11 mRNA to affect the sensitivity of HCC cells to sorafenib (Wang et al., 2021). Several other RBPs such as ZFP36 (Zhang et al., 2020b) IRP2 (Moroishi et al., 2011) have been reported to regulate ferroptosis. ELAV like RNA binding protein 1 (ELAVL1), which is highly expressed in many cancers, was a key target of ferroptosis induced by Erastin or Sorafenib in hepatic stellate cells. Up-regulation of ELAVL1 triggered by Erastin or Sorafenib promoted the production of BECN1/Beclin1 by binding to the AU-rich elements in the 3ʹ-UTR of BECN1 mRNA, thereby triggering autophagy activation, and ultimately promoting autophagic ferritin screening and ferroptosis (Zhang et al., 2018).
3.2.2 ACSL4
Acyl-CoA synthetase long-chain family member 4 (ACSL4), a ferroptosis-positive activating enzyme that esterifies free PUFA and binds to membrane phospholipids by LPCAT3, is considered a ferroptosis biomarker in hepatocellular carcinoma and has been proposed to be useful to predict the sensitivity of Sorafenib in HCC (Feng et al., 2021). Furthermore, ACLS4 was found to have a differential expression profile in hepatocellular carcinoma and gastrointestinal hepatic metastases. Therefore, it could be used to differentiate HCC from other forms of liver cancer and indicate that up-regulation of fatty acid metabolism is a potential chemotherapeutic target for the treatment of HCC (Ndiaye et al., 2020).
3.2.3 Metallothionein in Ferroptosis of HCC
Metallothionein (MT) is a family of small proteins widely expressed in eukaryotic cells. It is a low molecular weight protein and is highly rich in cysteine. It is highly induced in the reaction of different metal ions, cytokines, and free radicals and plays a critical role in detoxifying heavy metals and antioxidants (Coyle et al., 2002). Metallothionein-1G (MT-1G), a member of the MT family, was recently identified as a negative regulator of ferroptosis and a positive regulator of sorafenib resistance in HCC and could be used as a biomarker to explore the impact of Sorafenib on redox metabolism of cancer cells. Houessinon et al. found that HCC cells line Huh7 exposed to Sorafenib have enhanced expression of the metal ion protein-1 (MT1) gene due to the activity of the transcription factor NRF2, which has a binding site in an antioxidant response element found in the MT-1G promoter. The group also reported that sorafenib-treated patients have an increased level of MT1 protein, which was associated with reduced overall survival (Houessinon et al., 2016). Later, Sun et al. have sought to elucidate the mechanisms underlying the action of MT-1G on sorafenib resistance and discovered that MT-1G might facilitate sorafenib resistance by inhibiting ferroptosis (Sun et al., 2016).
4 Conclusion and Perspectives
Ferroptosis, characterized by iron and lipid peroxide-dependent cell death, has unique morphological and biological properties that have attracted widespread attention, as it can be induced in various cancers. Ferroptosis can be controlled by the key glutathione peroxidase 4 (Gpx4), antioxidants, and iron chelating agents. In addition to the conventional GSH-GPX4 axis, we have reviewed and summarized various mechanisms of GPX4 independent regulation of ferroptosis and highlighted their therapeutic potential in HCC. More aggressive phenotypes of HCC were associated with the activation of signaling pathways that regulate cell cycle progression and mutations in the TP53 gene in at least half of patients with HCC (Zucman-Rossi et al., 2015; Calderaro et al., 2019). However, whether this group of HCC patients could benefit from ferroptosis-induced therapy as reported in other cancers is unknown. Protein kinases such as mitogen-activated protein kinases whose activities are heavily impaired in HCC have also been suggested to have a role in ferroptosis in several disease models, although their implication for ferroptosis in HCC remained to be elucidated. Investigation to better understand in depth the contribution of these pathways to ferroptosis could ultimately open a new avenue to improve the outcomes of patients with HCC. Preventing chemotoxicity to healthy cells is a major concern in cancer treatment. Although inducing ferroptosis could be a reliable strategy for treating patients with HCC, emerging evidence also supports its role in the pathogenesis of other liver diseases (Wu et al., 2021; Chen et al., 2022). Therefore, an in-depth understanding of the regulatory mechanisms of ferroptosis in healthy cells versus HCC cells is required to selectively attack cancer cells while protecting all healthy tissues.
Author Contributions
XM and YA: conceptualization and supervision. SM and YA: writing initial manuscript. PZ, FX, ML, and XW: writing (review and editing). All authors contributed to the article and approved the submitted version.
Funding
This work was supported by Military Logistics Key Open Research Projects (BHJ17L018).
Conflict of Interest
The authors declare that the research was conducted in the absence of any commercial or financial relationships that could be construed as a potential conflict of interest.
Publisher’s Note
All claims expressed in this article are solely those of the authors and do not necessarily represent those of their affiliated organizations, or those of the publisher, the editors and the reviewers. Any product that may be evaluated in this article, or claim that may be made by its manufacturer, is not guaranteed or endorsed by the publisher.
References
Alvarez, S. W., Sviderskiy, V. O., Terzi, E. M., Papagiannakopoulos, T., Moreira, A. L., Adams, S., et al. (2017). NFS1 Undergoes Positive Selection in Lung Tumours and Protects Cells from Ferroptosis. Nature 551, 639–643. doi:10.1038/nature24637
Badgley, M. A., Kremer, D. M., Maurer, H. C., Delgiorno, K. E., Lee, H.-J., Purohit, V., et al. (2020). Cysteine Depletion Induces Pancreatic Tumor Ferroptosis in Mice. Science 368, 85–89. doi:10.1126/science.aaw9872
Bai, T., Lei, P., Zhou, H., Liang, R., Zhu, R., Wang, W., et al. (2019). Sigma-1 Receptor Protects against Ferroptosis in Hepatocellular Carcinoma Cells. J. Cell Mol. Med. 23, 7349–7359. doi:10.1111/jcmm.14594
Bai, T., Liang, R., Zhu, R., Wang, W., Zhou, L., and Sun, Y. (2020). MicroRNA-214-3p Enhances Erastin-Induced Ferroptosis by Targeting ATF4 in Hepatoma Cells. J. Cell Physiol. 235, 5637–5648. doi:10.1002/jcp.29496
Bai, T., Wang, S., Zhao, Y., Zhu, R., Wang, W., and Sun, Y. (2017). Haloperidol, a Sigma Receptor 1 Antagonist, Promotes Ferroptosis in Hepatocellular Carcinoma Cells. Biochem. Biophysical Res. Commun. 491, 919–925. doi:10.1016/j.bbrc.2017.07.136
Barrett, S. P., and Salzman, J. (2016). Circular RNAs: Analysis, Expression and Potential Functions. Development 143, 1838–1847. doi:10.1242/dev.128074
Beard, J. L. (2001). Iron Biology in Immune Function, Muscle Metabolism and Neuronal Functioning. J. Nutr. 131, 568S–580S. doi:10.1093/jn/131.2.568s
Bersuker, K., Hendricks, J. M., Li, Z., Magtanong, L., Ford, B., Tang, P. H., et al. (2019). The CoQ Oxidoreductase FSP1 Acts Parallel to GPX4 to Inhibit Ferroptosis. Nature 575, 688–692. doi:10.1038/s41586-019-1705-2
Bi, W., Huang, J., Nie, C., Liu, B., He, G., Han, J., et al. (2018). CircRNA circRNA_102171 Promotes Papillary Thyroid Cancer Progression through Modulating CTNNBIP1-dependent Activation of β-catenin Pathway. J. Exp. Clin. Cancer Res. 37, 275. doi:10.1186/s13046-018-0936-7
Bolha, L., Ravnik-Glavač, M., and Glavač, D. (2017). Circular RNAs: Biogenesis, Function, and a Role as Possible Cancer Biomarkers. Int. J. Genomics 2017, 6218353. doi:10.1155/2017/6218353
Braymer, J. J., Freibert, S. A., Rakwalska-Bange, M., and Lill, R. (2021). Mechanistic Concepts of Iron-Sulfur Protein Biogenesis in Biology. Biochimica Biophysica Acta (BBA) - Mol. Cell Res. 1868, 118863. doi:10.1016/j.bbamcr.2020.118863
Calderaro, J., Ziol, M., Paradis, V., and Zucman-Rossi, J. (2019). Molecular and Histological Correlations in Liver Cancer. J. Hepatology 71, 616–630. doi:10.1016/j.jhep.2019.06.001
Chang, L.-C., Chiang, S.-K., Chen, S.-E., Yu, Y.-L., Chou, R.-H., and Chang, W.-C. (2018). Heme Oxygenase-1 Mediates BAY 11-7085 Induced Ferroptosis. Cancer Lett. 416, 124–137. doi:10.1016/j.canlet.2017.12.025
Chang, W. T., Bow, Y. D., Fu, P. J., Li, C. Y., Wu, C. Y., Chang, Y. H., et al. (2021). A Marine Terpenoid, Heteronemin, Induces Both the Apoptosis and Ferroptosis of Hepatocellular Carcinoma Cells and Involves the ROS and MAPK Pathways. Oxid. Med. Cell Longev. 2021, 7689045. doi:10.1155/2021/7689045
Chen, J., Li, X., Ge, C., Min, J., and Wang, F. (2022). The Multifaceted Role of Ferroptosis in Liver Disease. Cell Death Differ. 29, 467–480. doi:10.1038/s41418-022-00941-0
Chen, J., Li, Y., Zheng, Q., Bao, C., He, J., Chen, B., et al. (2017). Circular RNA Profile Identifies circPVT1 as a Proliferative Factor and Prognostic Marker in Gastric Cancer. Cancer Lett. 388, 208–219. doi:10.1016/j.canlet.2016.12.006
Chen, L., Hambright, W. S., Na, R., and Ran, Q. (2015). Ablation of the Ferroptosis Inhibitor Glutathione Peroxidase 4 in Neurons Results in Rapid Motor Neuron Degeneration and Paralysis. J. Biol. Chem. 290, 28097–28106. doi:10.1074/jbc.m115.680090
Chen, X., Kang, R., Kroemer, G., and Tang, D. (2021). Targeting Ferroptosis in Pancreatic Cancer: a Double-Edged Sword. Trends Cancer 7, 891–901. doi:10.1016/j.trecan.2021.04.005
Chen, Y., Zhu, G., Liu, Y., Wu, Q., Zhang, X., Bian, Z., et al. (2019). O-GlcNAcylated C-Jun Antagonizes Ferroptosis via Inhibiting GSH Synthesis in Liver Cancer. Cell. Signal. 63, 109384. doi:10.1016/j.cellsig.2019.109384
Conrad, M., and Pratt, D. A. (2019). The Chemical Basis of Ferroptosis. Nat. Chem. Biol. 15, 1137–1147. doi:10.1038/s41589-019-0408-1
Couri, T., and Pillai, A. (2019). Goals and Targets for Personalized Therapy for HCC. Hepatol. Int. 13, 125–137. doi:10.1007/s12072-018-9919-1
Coyle, P., Philcox, J. C., Carey, L. C., and Rofe, A. M. (2002). Metallothionein: the Multipurpose Protein. Cell. Mol. Life Sci. (CMLS) 59, 627–647. doi:10.1007/s00018-002-8454-2
Cronin, S. J. F., Woolf, C. J., Weiss, G., and Penninger, J. M. (2019). The Role of Iron Regulation in Immunometabolism and Immune-Related Disease. Front. Mol. Biosci. 6, 116. doi:10.3389/fmolb.2019.00116
De Domenico, I., Mcvey Ward, D., and Kaplan, J. (2008). Regulation of Iron Acquisition and Storage: Consequences for Iron-Linked Disorders. Nat. Rev. Mol. Cell Biol. 9, 72–81. doi:10.1038/nrm2295
De Domenico, I., Ward, D. M., Langelier, C., Vaughn, M. B., Nemeth, E., Sundquist, W. I., et al. (2007). The Molecular Mechanism of Hepcidin-Mediated Ferroportin Down-Regulation. MBoC 18, 2569–2578. doi:10.1091/mbc.e07-01-0060
De La Cruz-López, K. G., Castro-Muñoz, L. J., Reyes-Hernández, D. O., García-Carrancá, A., and Manzo-Merino, J. (2019). Lactate in the Regulation of Tumor Microenvironment and Therapeutic Approaches. Front. Oncol. 9, 1143.
Del Re, D. P., Amgalan, D., Linkermann, A., Liu, Q., and Kitsis, R. N. (2019). Fundamental Mechanisms of Regulated Cell Death and Implications for Heart Disease. Physiol. Rev. 99, 1765–1817. doi:10.1152/physrev.00022.2018
Devos, D., Moreau, C., Devedjian, J. C., Kluza, J., Petrault, M., Laloux, C., et al. (2014). Targeting Chelatable Iron as a Therapeutic Modality in Parkinson's Disease. Antioxidants redox Signal. 21, 195–210. doi:10.1089/ars.2013.5593
Dixon, S. J., Lemberg, K. M., Lamprecht, M. R., Skouta, R., Zaitsev, E. M., Gleason, C. E., et al. (2012). Ferroptosis: an Iron-dependent Form of Nonapoptotic Cell Death. Cell 149, 1060–1072. doi:10.1016/j.cell.2012.03.042
Dixon, S. J., Patel, D. N., Welsch, M., Skouta, R., Lee, E. D., Hayano, M., et al. (2014). Pharmacological Inhibition of Cystine-Glutamate Exchange Induces Endoplasmic Reticulum Stress and Ferroptosis. Elife 3, e02523. doi:10.7554/eLife.02523
Doll, S., Freitas, F. P., Shah, R., Aldrovandi, M., Da Silva, M. C., Ingold, I., et al. (2019). FSP1 Is a Glutathione-independent Ferroptosis Suppressor. Nature 575, 693–698. doi:10.1038/s41586-019-1707-0
Doll, S., Proneth, B., Tyurina, Y. Y., Panzilius, E., Kobayashi, S., Ingold, I., et al. (2017). ACSL4 Dictates Ferroptosis Sensitivity by Shaping Cellular Lipid Composition. Nat. Chem. Biol. 13, 91–98. doi:10.1038/nchembio.2239
Dolma, S., Lessnick, S. L., Hahn, W. C., and Stockwell, B. R. (2003). Identification of Genotype-Selective Antitumor Agents Using Synthetic Lethal Chemical Screening in Engineered Human Tumor Cells. Cancer Cell 3, 285–296. doi:10.1016/s1535-6108(03)00050-3
Elguindy, M. M., and Nakamaru-Ogiso, E. (2015). Apoptosis-inducing Factor (AIF) and its Family Member Protein, AMID, Are Rotenone-Sensitive NADH:Ubiquinone Oxidoreductases (NDH-2). J. Biol. Chem. 290, 20815–20826. doi:10.1074/jbc.m115.641498
Eling, N., Reuter, L., Hazin, J., Hamacher-Brady, A., and Brady, N. R. (2015). Identification of Artesunate as a Specific Activator of Ferroptosis in Pancreatic Cancer Cells. Oncoscience 2, 517–532. doi:10.18632/oncoscience.160
Feng, J., Lu, P.-z., Zhu, G.-z., Hooi, S. C., Wu, Y., Huang, X.-w., et al. (2021). ACSL4 Is a Predictive Biomarker of Sorafenib Sensitivity in Hepatocellular Carcinoma. Acta Pharmacol. Sin. 42, 160–170. doi:10.1038/s41401-020-0439-x
Friedmann Angeli, J. P., Schneider, M., Proneth, B., Tyurina, Y. Y., Tyurin, V. A., Hammond, V. J., et al. (2014). Inactivation of the Ferroptosis Regulator Gpx4 Triggers Acute Renal Failure in Mice. Nat. Cell Biol. 16, 1180–1191. doi:10.1038/ncb3064
Gaschler, M. M., and Stockwell, B. R. (2017). Lipid Peroxidation in Cell Death. Biochem. Biophysical Res. Commun. 482, 419–425. doi:10.1016/j.bbrc.2016.10.086
Gnanapradeepan, K., Basu, S., Barnoud, T., Budina-Kolomets, A., Kung, C.-P., and Murphy, M. E. (2018). The P53 Tumor Suppressor in the Control of Metabolism and Ferroptosis. Front. Endocrinol. 9, 124. doi:10.3389/fendo.2018.00124
Gout, P., Buckley, A., Simms, C., and Bruchovsky, N. (2001). Sulfasalazine, a Potent Suppressor of Lymphoma Growth by Inhibition of the Xc − Cystine Transporter: a New Action for an Old Drug. Leukemia 15, 1633–1640. doi:10.1038/sj.leu.2402238
Griffith, O. W. (1982). Mechanism of Action, Metabolism, and Toxicity of Buthionine Sulfoximine and its Higher Homologs, Potent Inhibitors of Glutathione Synthesis. J. Biol. Chem. 257, 13704–13712. doi:10.1016/s0021-9258(18)33504-x
Hambright, W. S., Fonseca, R. S., Chen, L., Na, R., and Ran, Q. (2017). Ablation of Ferroptosis Regulator Glutathione Peroxidase 4 in Forebrain Neurons Promotes Cognitive Impairment and Neurodegeneration. Redox Biol. 12, 8–17. doi:10.1016/j.redox.2017.01.021
Hassannia, B., Vandenabeele, P., and Vanden Berghe, T. (2019). Targeting Ferroptosis to Iron Out Cancer. Cancer Cell 35, 830–849. doi:10.1016/j.ccell.2019.04.002
Hattori, K., Ishikawa, H., Sakauchi, C., Takayanagi, S., Naguro, I., and Ichijo, H. (2017). Cold Stress-Induced Ferroptosis Involves the ASK 1-p38 Pathway. EMBO Rep. 18, 2067–2078. doi:10.15252/embr.201744228
Hawley, S. A., Ross, F. A., Chevtzoff, C., Green, K. A., Evans, A., Fogarty, S., et al. (2010). Use of Cells Expressing γ Subunit Variants to Identify Diverse Mechanisms of AMPK Activation. Cell Metab. 11, 554–565.
Homma, T., and Fujii, J. (2015). Application of Glutathione as Anti-oxidative and Anti-aging Drugs. Cdm 16, 560–571. doi:10.2174/1389200216666151015114515
Homma, T., Kobayashi, S., Sato, H., and Fujii, J. (2019). Edaravone, a Free Radical Scavenger, Protects against Ferroptotic Cell Death In Vitro. Exp. Cell Res. 384, 111592. doi:10.1016/j.yexcr.2019.111592
Houessinon, A., François, C., Sauzay, C., Louandre, C., Mongelard, G., Godin, C., et al. (2016). Metallothionein-1 as a Biomarker of Altered Redox Metabolism in Hepatocellular Carcinoma Cells Exposed to Sorafenib. Mol. Cancer 15, 38. doi:10.1186/s12943-016-0526-2
Hu, C. L., Nydes, M., Shanley, K. L., Morales Pantoja, I. E., Howard, T. A., and Bizzozero, O. A. (2019). Reduced Expression of the Ferroptosis Inhibitor Glutathione Peroxidase-4 in Multiple Sclerosis and Experimental Autoimmune Encephalomyelitis. J. Neurochem. 148, 426–439. doi:10.1111/jnc.14604
Jenkins, N. L., James, S. A., Salim, A., Sumardy, F., Speed, T. P., Conrad, M., et al. (2020). Changes in Ferrous Iron and Glutathione Promote Ferroptosis and Frailty in Aging Caenorhabditis elegans. eLife 9, e56580. doi:10.7554/eLife.56580
Jiang, L., Kon, N., Li, T., Wang, S.-J., Su, T., Hibshoosh, H., et al. (2015). Ferroptosis as a P53-Mediated Activity during Tumour Suppression. Nature 520, 57–62. doi:10.1038/nature14344
Jiang, X., Stockwell, B. R., and Conrad, M. (2021). Ferroptosis: Mechanisms, Biology and Role in Disease. Nat. Rev. Mol. Cell Biol. 22, 266–282. doi:10.1038/s41580-020-00324-8
Jin, M., Shi, C., Li, T., Wu, Y., Hu, C., and Huang, G. (2020). Solasonine Promotes Ferroptosis of Hepatoma Carcinoma Cells via Glutathione Peroxidase 4-induced Destruction of the Glutathione Redox System. Biomed. Pharmacother. 129, 110282. doi:10.1016/j.biopha.2020.110282
Kang, R., Kroemer, G., and Tang, D. (2019). The Tumor Suppressor Protein P53 and the Ferroptosis Network. Free Radic. Biol. Med. 133, 162–168. doi:10.1016/j.freeradbiomed.2018.05.074
Kim, E. H., Wong, S.-W., and Martinez, J. (2019). Programmed Necrosis and Disease:We Interrupt Your Regular Programming to Bring You Necroinflammation. Cell Death Differ. 26, 25–40. doi:10.1038/s41418-018-0179-3
Kim, J., and Deberardinis, R. J. (2019). Mechanisms and Implications of Metabolic Heterogeneity in Cancer. Cell Metab. 30, 434–446. doi:10.1016/j.cmet.2019.08.013
Kwon, M.-Y., Park, E., Lee, S.-J., and Chung, S. W. (2015). Heme Oxygenase-1 Accelerates Erastin-Induced Ferroptotic Cell Death. Oncotarget 6, 24393–24403. doi:10.18632/oncotarget.5162
Lee, H., Zhuang, L., and Gan, B. (2020). Energy Stress Inhibits Ferroptosis via AMPK. Mol. Cell. Oncol. 7, 1761242. doi:10.1080/23723556.2020.1761242
Lemasters, J. J. (2017). Evolution of Voltage-dependent Anion Channel Function: From Molecular Sieve to Governator to Actuator of Ferroptosis. Front. Oncol. 7, 303. doi:10.3389/fonc.2017.00303
Li, X. Y., and Leung, P. S. (2020). Erastin-induced Ferroptosis Is a Regulator for the Growth and Function of Human Pancreatic Islet-like Cell Clusters. Cell Regen. 9, 16. doi:10.1186/s13619-020-00055-3
Li, Z.-j., Dai, H.-q., Huang, X.-w., Feng, J., Deng, J.-h., Wang, Z.-x., et al. (2021). Artesunate Synergizes with Sorafenib to Induce Ferroptosis in Hepatocellular Carcinoma. Acta Pharmacol. Sin. 42, 301–310. doi:10.1038/s41401-020-0478-3
Liang, C., Zhang, X., Yang, M., and Dong, X. (2019). Recent Progress in Ferroptosis Inducers for Cancer Therapy. Adv. Mater 31, e1904197. doi:10.1002/adma.201904197
Lin, P. L., Tang, H. H., Wu, S. Y., Shaw, N. S., and Su, C. L. (2020). Saponin Formosanin C-Induced Ferritinophagy and Ferroptosis in Human Hepatocellular Carcinoma Cells. Antioxidants (Basel) 9. doi:10.3390/antiox9080682
Lippmann, J., Petri, K., Fulda, S., and Liese, J. (2020). Redox Modulation and Induction of Ferroptosis as a New Therapeutic Strategy in Hepatocellular Carcinoma. Transl. Oncol. 13, 100785. doi:10.1016/j.tranon.2020.100785
Liu, J., Zhang, C., Wang, J., Hu, W., and Feng, Z. (2020a). The Regulation of Ferroptosis by Tumor Suppressor P53 and its Pathway. Int. J. Mol. Sci. 21. doi:10.3390/ijms21218387
Liu, Z., Wang, Q., Wang, X., Xu, Z., Wei, X., and Li, J. (2020b). Circular RNA cIARS Regulates Ferroptosis in HCC Cells through Interacting with RNA Binding Protein ALKBH5. Cell Death Discov. 6, 72. doi:10.1038/s41420-020-00306-x
Lőrincz, T., Jemnitz, K., Kardon, T., Mandl, J., and Szarka, A. (2015). Ferroptosis Is Involved in Acetaminophen Induced Cell Death. Pathol. Oncol. Res. 21, 1115–1121. doi:10.1007/s12253-015-9946-3
Louandre, C., Ezzoukhry, Z., Godin, C., Barbare, J.-C., Mazière, J.-C., Chauffert, B., et al. (2013). Iron-dependent Cell Death of Hepatocellular Carcinoma Cells Exposed to Sorafenib. Int. J. Cancer 133, 1732–1742. doi:10.1002/ijc.28159
Louandre, C., Marcq, I., Bouhlal, H., Lachaier, E., Godin, C., Saidak, Z., et al. (2015). The Retinoblastoma (Rb) Protein Regulates Ferroptosis Induced by Sorafenib in Human Hepatocellular Carcinoma Cells. Cancer Lett. 356, 971–977. doi:10.1016/j.canlet.2014.11.014
Lu, B., Chen, X. B., Ying, M. D., He, Q. J., Cao, J., and Yang, B. (2018). The Role of Ferroptosis in Cancer Development and Treatment Response. Front. Pharmacol. 8, 992. doi:10.3389/fphar.2017.00992
Maiorino, M., Conrad, M., and Ursini, F. (2018). GPx4, Lipid Peroxidation, and Cell Death: Discoveries, Rediscoveries, and Open Issues. Antioxidants Redox Signal. 29, 61–74. doi:10.1089/ars.2017.7115
Maldonado, E. N., Patnaik, J., Mullins, M. R., and Lemasters, J. J. (2010). Free Tubulin Modulates Mitochondrial Membrane Potential in Cancer Cells. Cancer Res. 70, 10192–10201. doi:10.1158/0008-5472.can-10-2429
Maldonado, E. N., Sheldon, K. L., Dehart, D. N., Patnaik, J., Manevich, Y., Townsend, D. M., et al. (2013). Voltage-dependent Anion Channels Modulate Mitochondrial Metabolism in Cancer Cells: Regulation by Free Tubulin and Erastin. J. Biol. Chem. 288, 11920–11929. doi:10.1074/jbc.M112.433847
Mancias, J. D., Wang, X., Gygi, S. P., Harper, J. W., and Kimmelman, A. C. (2014). Quantitative Proteomics Identifies NCOA4 as the Cargo Receptor Mediating Ferritinophagy. Nature 509, 105–109. doi:10.1038/nature13148
Manz, D. H., Blanchette, N. L., Paul, B. T., Torti, F. M., and Torti, S. V. (2016). Iron and Cancer: Recent Insights. Ann. N. Y. Acad. Sci. 1368, 149–161. doi:10.1111/nyas.13008
Meister, A. (1995). Glutathione Metabolism. Methods Enzymol. 251, 3–7. doi:10.1016/0076-6879(95)51106-7
Memczak, S., Jens, M., Elefsinioti, A., Torti, F., Krueger, J., Rybak, A., et al. (2013). Circular RNAs Are a Large Class of Animal RNAs with Regulatory Potency. Nature 495, 333–338. doi:10.1038/nature11928
Miller, K. D., Nogueira, L., Mariotto, A. B., Rowland, J. H., Yabroff, K. R., Alfano, C. M., et al. (2019). Cancer Treatment and Survivorship Statistics, 2019. CA Cancer J. Clin. 69, 363–385. doi:10.3322/caac.21565
Moroishi, T., Nishiyama, M., Takeda, Y., Iwai, K., and Nakayama, K. I. (2011). The FBXL5-IRP2 axis Is Integral to Control of Iron Metabolism In Vivo. Cell Metab. 14, 339–351. doi:10.1016/j.cmet.2011.07.011
Mou, Y., Wu, J., Zhang, Y., Abdihamid, O., Duan, C., and Li, B. (2021). Low Expression of Ferritinophagy-Related NCOA4 Gene in Relation to Unfavorable Outcome and Defective Immune Cells Infiltration in Clear Cell Renal Carcinoma. BMC Cancer 21, 18.
Ndiaye, H., Liu, J. Y., Hall, A., Minogue, S., Morgan, M. Y., and Waugh, M. G. (2020). Immunohistochemical Staining Reveals Differential Expression of ACSL3 and ACSL4 in Hepatocellular Carcinoma and Hepatic Gastrointestinal Metastases. Biosci. Rep. 40. doi:10.1042/BSR20200219
Nemeth, E., Tuttle, M. S., Powelson, J., Vaughn, M. B., Donovan, A., Ward, D. M., et al. (2004). Hepcidin Regulates Cellular Iron Efflux by Binding to Ferroportin and Inducing its Internalization. Science 306, 2090–2093. doi:10.1126/science.1104742
Ng, S. W., Norwitz, S. G., Taylor, H. S., and Norwitz, E. R. (2020). Endometriosis: The Role of Iron Overload and Ferroptosis. Reprod. Sci. 27, 1383–1390. doi:10.1007/s43032-020-00164-z
Park, E. J., Park, Y. J., Lee, S. J., Lee, K., and Yoon, C. (2019). Whole Cigarette Smoke Condensates Induce Ferroptosis in Human Bronchial Epithelial Cells. Toxicol. Lett. 303, 55–66. doi:10.1016/j.toxlet.2018.12.007
Pérez-Tomás, R., and Pérez-Guillén, I. (2020). Lactate in the Tumor Microenvironment: An Essential Molecule in Cancer Progression and Treatment. Cancers (Basel) 12.
Qi, W., Li, Z., Xia, L., Dai, J., Zhang, Q., Wu, C., et al. (2019). LncRNA GABPB1-AS1 and GABPB1 Regulate Oxidative Stress during Erastin-Induced Ferroptosis in HepG2 Hepatocellular Carcinoma Cells. Sci. Rep. 9, 16185. doi:10.1038/s41598-019-52837-8
Quiles Del Rey, M., and Mancias, J. D. (2019). NCOA4-Mediated Ferritinophagy: A Potential Link to Neurodegeneration. Front. Neurosci. 13.
Reinhardt, C., Arena, G., Nedara, K., Edwards, R., Brenner, C., Tokatlidis, K., et al. (2020). AIF Meets the CHCHD4/Mia40-dependent Mitochondrial Import Pathway. Biochim. Biophys. Acta Mol. Basis Dis. 1866, 165746. doi:10.1016/j.bbadis.2020.165746
Repetto, M. G., Ferrarotti, N. F., and Boveris, A. (2010). The Involvement of Transition Metal Ions on Iron-dependent Lipid Peroxidation. Arch. Toxicol. 84, 255–262. doi:10.1007/s00204-009-0487-y
Rouzer, C. A., and Marnett, L. J. (2003). Mechanism of Free Radical Oxygenation of Polyunsaturated Fatty Acids by Cyclooxygenases. Chem. Rev. 103, 2239–2304. doi:10.1021/cr000068x
Roxburgh, P., and Evans, T. R. (2008). Systemic Therapy of Hepatocellular Carcinoma: Are We Making Progress? Adv. Ther. 25, 1089–1104. doi:10.1007/s12325-008-0113-z
Shan, Y., Yang, G., Huang, H., Zhou, Y., Hu, X., Lu, Q., et al. (2020). Ubiquitin-Like Modifier Activating Enzyme 1 as a Novel Diagnostic and Prognostic Indicator that Correlates with Ferroptosis and the Malignant Phenotypes of Liver Cancer Cells. Front. Oncol. 10, 592413.
Shang, Y., Luo, M., Yao, F., Wang, S., Yuan, Z., and Yang, Y. (2020). Ceruloplasmin Suppresses Ferroptosis by Regulating Iron Homeostasis in Hepatocellular Carcinoma Cells. Cell Signal 72, 109633. doi:10.1016/j.cellsig.2020.109633
Shaw, A. T., Winslow, M. M., Magendantz, M., Ouyang, C., Dowdle, J., Subramanian, A., et al. (2011). Selective Killing of K-Ras Mutant Cancer Cells by Small Molecule Inducers of Oxidative Stress. Proc. Natl. Acad. Sci. U. S. A. 108, 8773–8778. doi:10.1073/pnas.1105941108
Shen, Q., Liang, M., Yang, F., Deng, Y. Z., and Naqvi, N. I. (2020). Ferroptosis Contributes to Developmental Cell Death in Rice Blast. New Phytol. 227, 1831–1846. doi:10.1111/nph.16636
Shen, Z., Song, J., Yung, B. C., Zhou, Z., Wu, A., and Chen, X. (2018). Emerging Strategies of Cancer Therapy Based on Ferroptosis. Adv. Mater 30, e1704007. doi:10.1002/adma.201704007
Stockwell, B. R., Friedmann Angeli, J. P., Bayir, H., Bush, A. I., Conrad, M., Dixon, S. J., et al. (2017). Ferroptosis: A Regulated Cell Death Nexus Linking Metabolism, Redox Biology, and Disease. Cell 171, 273–285. doi:10.1016/j.cell.2017.09.021
Stockwell, B. R., and Jiang, X. (2020). The Chemistry and Biology of Ferroptosis. Cell Chem. Biol. 27, 365–375. doi:10.1016/j.chembiol.2020.03.013
Su, Y., Feng, W., Shi, J., Chen, L., Huang, J., and Lin, T. (2020). circRIP2 Accelerates Bladder Cancer Progression via miR-1305/Tgf-Β2/smad3 Pathway. Mol. Cancer 19, 23. doi:10.1186/s12943-019-1129-5
Sun, J., Zhou, C., Zhao, Y., Zhang, X., Chen, W., Zhou, Q., et al. (2021). Quiescin Sulfhydryl Oxidase 1 Promotes Sorafenib-Induced Ferroptosis in Hepatocellular Carcinoma by Driving EGFR Endosomal Trafficking and Inhibiting NRF2 Activation. Redox Biol. 41, 101942.
Sun, X., Ou, Z., Chen, R., Niu, X., Chen, D., Kang, R., et al. (2016). Activation of the P62-Keap1-NRF2 Pathway Protects against Ferroptosis in Hepatocellular Carcinoma Cells. Hepatology 63, 173–184. doi:10.1002/hep.28251
Sun, Y., Chen, P., Zhai, B., Zhang, M., Xiang, Y., Fang, J., et al. (2020). The Emerging Role of Ferroptosis in Inflammation. Biomed. Pharmacother. 127, 110108. doi:10.1016/j.biopha.2020.110108
Thompson, C. B. (1995). Apoptosis in the Pathogenesis and Treatment of Disease. Science 267, 1456–1462.
Trujillo-Alonso, V., Pratt, E. C., Zong, H., Lara-Martinez, A., Kaittanis, C., Rabie, M. O., et al. (2019). FDA-approved Ferumoxytol Displays Anti-leukaemia Efficacy against Cells with Low Ferroportin Levels. Nat. Nanotechnol. 14, 616–622. doi:10.1038/s41565-019-0406-1
Vashchenko, G., and Macgillivray, R. T. A. (2013). Multi-Copper Oxidases and Human Iron Metabolism. Nutrients 5, 2289–2313.
Venkatesh, D., Stockwell, B. R., and Prives, C. (2020). p21 Can Be a Barrier to Ferroptosis Independent of P53. Aging (Albany NY) 12, 17800–17814. doi:10.18632/aging.103961
Verga Falzacappa, M. V., and Muckenthaler, M. U. (2005). Hepcidin: Iron-Hormone and Anti-microbial Peptide. Gene 364, 37–44.
Vigor, C., Bertrand-Michel, J., Pinot, E., Oger, C., Vercauteren, J., Le Faouder, P., et al. (2014). Non-enzymatic Lipid Oxidation Products in Biological Systems: Assessment of the Metabolites from Polyunsaturated Fatty Acids. J. Chromatogr. B Anal. Technol. Biomed. Life Sci. 964, 65–78. doi:10.1016/j.jchromb.2014.04.042
Villanueva, A. (2019). Hepatocellular Carcinoma. N. Engl. J. Med. 380, 1450–1462. doi:10.1056/NEJMra1713263
Wang, M., Yu, F., and Li, P. (2018). Circular RNAs: Characteristics, Function and Clinical Significance in Hepatocellular Carcinoma. Cancers (Basel) 10. doi:10.3390/cancers10080258
Wang, Q., Guo, Y., Wang, W., Liu, B., Yang, G., Xu, Z., et al. (2021). RNA Binding Protein DAZAP1 Promotes HCC Progression and Regulates Ferroptosis by Interacting with SLC7A11 mRNA. Exp. Cell Res. 399, 112453.
Wang, Y., Wei, Z., Pan, K., Li, J., and Chen, Q. (2020). The Function and Mechanism of Ferroptosis in Cancer. Apoptosis 25, 786–798. doi:10.1007/s10495-020-01638-w
Weiland, A., Wang, Y., Wu, W., Lan, X., Han, X., Li, Q., et al. (2019). Ferroptosis and its Role in Diverse Brain Diseases. Mol. Neurobiol. 56, 4880–4893. doi:10.1007/s12035-018-1403-3
Wenzel, S. E., Tyurina, Y. Y., Zhao, J., St Croix, C. M., Dar, H. H., Mao, G., et al. (2017). PEBP1 Wardens Ferroptosis by Enabling Lipoxygenase Generation of Lipid Death Signals. Cell 171, 628–e26. e626. doi:10.1016/j.cell.2017.09.044
Wu, J., Wang, Y., Jiang, R., Xue, R., Yin, X., Wu, M., et al. (2021). Ferroptosis in Liver Disease: New Insights into Disease Mechanisms. Cell Death Discov. 7, 276.
Xia, X., Fan, X., Zhao, M., and Zhu, P. (2019). The Relationship between Ferroptosis and Tumors: A Novel Landscape for Therapeutic Approach. Curr. Gene Ther. 19, 117–124. doi:10.2174/1566523219666190628152137
Xie, Y., Hou, W., Song, X., Yu, Y., Huang, J., Sun, X., et al. (2016). Ferroptosis: Process and Function. Cell Death Differ. 23, 369–379. doi:10.1038/cdd.2015.158
Xu, Q., Zhou, L., Yang, G., Meng, F., Wan, Y., Wang, L., et al. (2020). CircIL4R Facilitates the Tumorigenesis and Inhibits Ferroptosis in Hepatocellular Carcinoma by Regulating the miR-541-3p/GPX4 axis. Cell Biol. Int. 44, 2344–2356. doi:10.1002/cbin.11444
Yagoda, N., von Rechenberg, M., Zaganjor, E., Bauer, A. J., Yang, W. S., Fridman, D. J., et al. (2007). RAS-RAF-MEK-dependent Oxidative Cell Death Involving Voltage-dependent Anion Channels. Nature 447, 864–868. doi:10.1038/nature05859
Yan, B., Ai, Y., Sun, Q., Ma, Y., Cao, Y., Wang, J., et al. (2021). Membrane Damage during Ferroptosis Is Caused by Oxidation of Phospholipids Catalyzed by the Oxidoreductases POR and CYB5R1. Mol. Cell 81, 355–369. e310.
Yang, L., Wang, H., Yang, X., Wu, Q., An, P., Jin, X., et al. (2020). Auranofin Mitigates Systemic Iron Overload and Induces Ferroptosis via Distinct Mechanisms. Signal Transduct. Target Ther. 5, 138. doi:10.1038/s41392-020-00253-0
Yang, W. S., Kim, K. J., Gaschler, M. M., Patel, M., Shchepinov, M. S., and Stockwell, B. R. (2016). Peroxidation of Polyunsaturated Fatty Acids by Lipoxygenases Drives Ferroptosis. Proc. Natl. Acad. Sci. U. S. A. 113, E4966–E4975. doi:10.1073/pnas.1603244113
Yang, W. S., SriRamaratnam, R., Welsch, M. E., Shimada, K., Skouta, R., Viswanathan, V. S., et al. (2014). Regulation of Ferroptotic Cancer Cell Death by GPX4. Cell 156, 317–331. doi:10.1016/j.cell.2013.12.010
Yang, W. S., and Stockwell, B. R. (2008). Synthetic Lethal Screening Identifies Compounds Activating Iron-dependent, Nonapoptotic Cell Death in Oncogenic-RAS-Harboring Cancer Cells. Chem. Biol. 15, 234–245. doi:10.1016/j.chembiol.2008.02.010
Yao, X., Li, W., Fang, D., Xiao, C., Wu, X., Li, M., et al. (2021). Emerging Roles of Energy Metabolism in Ferroptosis Regulation of Tumor Cells. Adv. Sci. (Weinheim, Baden-Wurttemberg, Ger. 8, e2100997.
Yin, H., Xu, L., and Porter, N. A. (2011). Free Radical Lipid Peroxidation: Mechanisms and Analysis. Chem. Rev. 111, 5944–5972. doi:10.1021/cr200084z
Yu, H., Guo, P., Xie, X., Wang, Y., and Chen, G. (2017). Ferroptosis, a New Form of Cell Death, and its Relationships with Tumourous Diseases. J. Cell Mol. Med. 21, 648–657. doi:10.1111/jcmm.13008
Yu, Y., Jiang, L., Wang, H., Shen, Z., Cheng, Q., Zhang, P., et al. (2020). Hepatic Transferrin Plays a Role in Systemic Iron Homeostasis and Liver Ferroptosis. Blood 136, 726–739. doi:10.1182/blood.2019002907
Yu, Y., Xie, Y., Cao, L., Yang, L., Yang, M., Lotze, M. T., et al. (2015). The Ferroptosis Inducer Erastin Enhances Sensitivity of Acute Myeloid Leukemia Cells to Chemotherapeutic Agents. Mol. Cell Oncol. 2, e1054549. doi:10.1080/23723556.2015.1054549
Zhang, H. Y., Zhang, B. W., Zhang, Z. B., and Deng, Q. J. (2020). Circular RNA TTBK2 Regulates Cell Proliferation, Invasion and Ferroptosis via miR-761/ITGB8 axis in Glioma. Eur. Rev. Med. Pharmacol. Sci. 24, 2585–2600. doi:10.26355/eurrev_202003_20528
Zhang, X., Yu, K., Ma, L., Qian, Z., Tian, X., Miao, Y., et al. (2021). Endogenous Glutamate Determines Ferroptosis Sensitivity via ADCY10-dependent YAP Suppression in Lung Adenocarcinoma. Theranostics 11, 5650–5674.
Zhang, Z., Guo, M., Li, Y., Shen, M., Kong, D., Shao, J., et al. (2020). RNA-binding Protein ZFP36/TTP Protects against Ferroptosis by Regulating Autophagy Signaling Pathway in Hepatic Stellate Cells. Autophagy 16, 1482–1505. doi:10.1080/15548627.2019.1687985
Zhang, Z., Yao, Z., Wang, L., Ding, H., Shao, J., Chen, A., et al. (2018). Activation of Ferritinophagy Is Required for the RNA-Binding Protein ELAVL1/HuR to Regulate Ferroptosis in Hepatic Stellate Cells. Autophagy 14, 2083–2103. doi:10.1080/15548627.2018.1503146
Zhao, Y., Li, J., Guo, W., Li, H., and Lei, L. (2020). Periodontitis-level Butyrate-Induced Ferroptosis in Periodontal Ligament Fibroblasts by Activation of Ferritinophagy. Cell Death Discov. 6, 119. doi:10.1038/s41420-020-00356-1
Zhao, Y., Li, M., Yao, X., Fei, Y., Lin, Z., Li, Z., et al. (2020). HCAR1/MCT1 Regulates Tumor Ferroptosis through the Lactate-Mediated AMPK-SCD1 Activity and its Therapeutic Implications. Cell Rep. 33, 108487. doi:10.1016/j.celrep.2020.108487
Zhong, X., Zhang, Z., Shen, H., Xiong, Y., Shah, Y. M., Liu, Y., et al. (2021). Hepatic NF-Κb-Inducing Kinase and Inhibitor of NF-Κb Kinase Subunit α Promote Liver Oxidative Stress, Ferroptosis, and Liver Injury. Hepatol. Commun. 5, 1704–1720.
Zhu, H. Y., Huang, Z. X., Chen, G. Q., Sheng, F., and Zheng, Y. S. (2019). Typhaneoside Prevents Acute Myeloid Leukemia (AML) through Suppressing Proliferation and Inducing Ferroptosis Associated with Autophagy. Biochem. Biophys. Res. Commun. 516, 1265–1271. doi:10.1016/j.bbrc.2019.06.070
Keywords: ferroptosis, iron, lipid peroxidation, hepatocellular carcinoma, iron homeostasis
Citation: Ma S, Adzavon YM, Wen X, Zhao P, Xie F, Liu M and Ma X (2022) Novel Insights in the Regulatory Mechanisms of Ferroptosis in Hepatocellular Carcinoma. Front. Cell Dev. Biol. 10:873029. doi: 10.3389/fcell.2022.873029
Received: 10 February 2022; Accepted: 05 May 2022;
Published: 19 May 2022.
Edited by:
Chunying Li, Fourth Military Medical University, ChinaReviewed by:
Maura Poli, University of Brescia, ItalyJinke Wang, Southeast University, China
Weinan Guo, Fourth Military Medical University, China
Copyright © 2022 Ma, Adzavon, Wen, Zhao, Xie, Liu and Ma. This is an open-access article distributed under the terms of the Creative Commons Attribution License (CC BY). The use, distribution or reproduction in other forums is permitted, provided the original author(s) and the copyright owner(s) are credited and that the original publication in this journal is cited, in accordance with accepted academic practice. No use, distribution or reproduction is permitted which does not comply with these terms.
*Correspondence: Yao Mawulikplimi Adzavon, eWFvYWR6YXZvbkBianV0LmVkdS5jbg==