- Laboratory of Chromosome Biology, Graduate School of Frontier Biosciences, Osaka University, Suita, Japan
The assembly of a functional kinetochore on centromeric chromatin is necessary to connect chromosomes to the mitotic spindle, ensuring accurate chromosome segregation. This connecting function of the kinetochore presents multiple internal and external structural challenges. A microtubule interacting outer kinetochore and centromeric chromatin interacting inner kinetochore effectively confront forces from the external spindle and centromere, respectively. While internally, special inner kinetochore proteins, defined as “linkers,” simultaneously interact with centromeric chromatin and the outer kinetochore to enable association with the mitotic spindle. With the ability to simultaneously interact with outer kinetochore components and centromeric chromatin, linker proteins such as centromere protein (CENP)-C or CENP-T in vertebrates and, additionally CENP-QOkp1-UAme1 in yeasts, also perform the function of force propagation within the kinetochore. Recent efforts have revealed an array of linker pathways strategies to effectively recruit the largely conserved outer kinetochore. In this review, we examine these linkages used to propagate force and recruit the outer kinetochore across evolution. Further, we look at their known regulatory pathways and implications on kinetochore structural diversity and plasticity.
1 Introduction
The kinetochore is a macromolecular protein complex that forms on centromeric chromatin and couples forces from the mitotic spindle to facilitate accurate chromosome segregation (Figure 1A). Initial electron microscopy (EM) observations of the kinetochore architecture identified an inner and outer plate that was separated by a translucent layer (Luykx, 1965; Brinkley and Stubblefield, 1966). Spindle microtubules were observed to terminate on the outer plate. In recent years, we broadly distinguish the plates as protein networks of the inner, proximal to centromeric chromatin, and outer kinetochore, proximal to spindle microtubules (Figure 1B). What manifests itself as the translucent layer is yet not understood. Additionally, even after more than 50 years of studying the kinetochore a wholistic structural picture of this elegant structure has not been understood, while researchers in the field are just beginning to understand its plasticity. However, recent cryo-EM studies of the reconstituted inner kinetochore complex in budding yeast and humans are providing a strong platform for understanding kinetochore architecture and its evolutionary divergence (Hinshaw and Harrison, 2019; Yan et al., 2019; Pesenti et al., 2022; Yatskevich et al., 2022), Although the primary function of the kinetochore is to form load-bearing attachments, the kinetochore has also to control the feedback mechanism for the correction of inaccurate microtubule attachments through the recruitment of the components involved in the error correction mechanism and the spindle assembly checkpoint (SAC) (Foley and Kapoor, 2013; Joglekar and Kukreja, 2017; Lara-Gonzalez et al., 2021). Additionally, kinetochores are required to ensure their self-preservation across generations at the centromere through CENP-A replenishment (Black and Cleveland, 2011; McKinley and Cheeseman, 2016; Mellone and Fachinetti, 2021). To achieve these functional goals the kinetochore consists of more than 100 proteins, including around 30 core structural components (Figure 1B) (Cheeseman, 2014; Fukagawa and Earnshaw, 2014; Musacchio and Desai, 2017). This functionally conserved protein complex assembles on a variable centromeric platform that is not only some of the most rapidly evolving DNA sequences in the genome but is also diverse in terms of its organization, a contradiction termed the centromere paradox (Henikoff, 2001). Centromeric DNA varies, from the short ∼125 bp sequence-defined point centromeres of budding yeast to several Mb long repetitive regional centromeres in humans. Although chromosomes containing centromeres at a single locus, called monocentric chromosomes, were the first discovered, more recently systems ranging from plants to insects have been identified as consisting of kinetochore attachment sites across the length of a chromosome, defined as holocentric chromosomes (Boveri and Fischer, 1888; Clarke and Carbon, 1980; Buscaino et al., 2010; Fukagawa and Earnshaw, 2014; Guin et al., 2020).
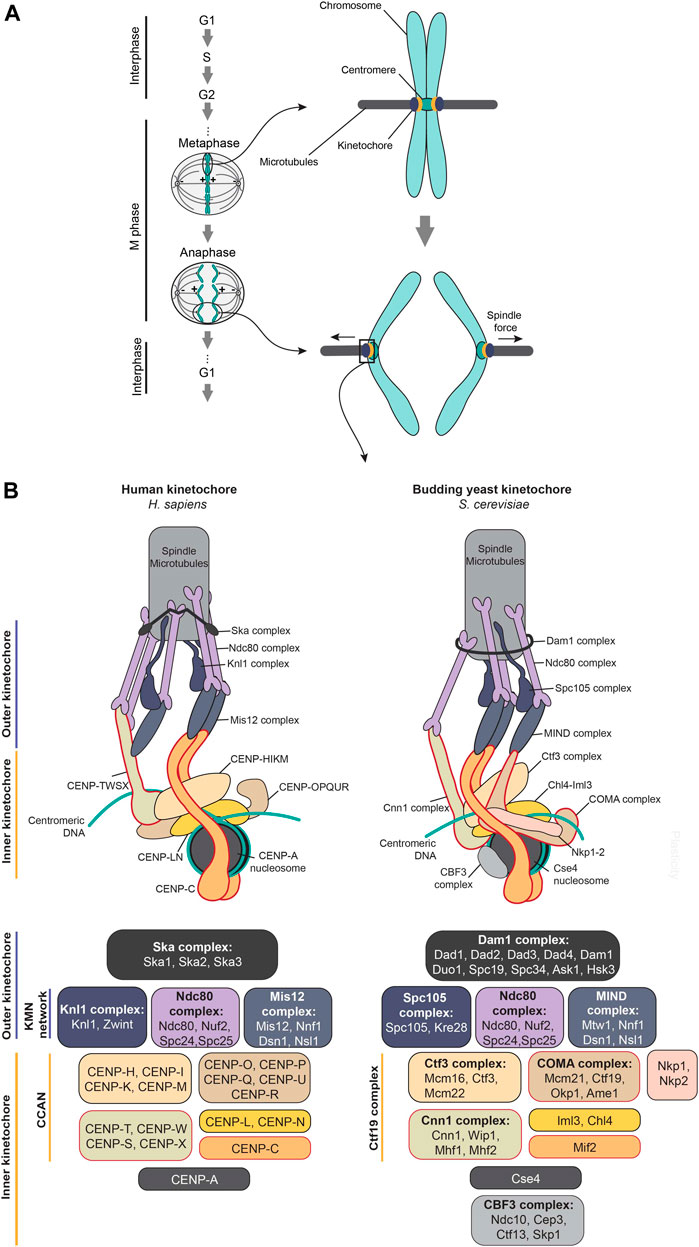
FIGURE 1. Schematic of kinetochore composition and architecture in human and budding yeast systems. (A) A functional kinetochore is assembled in M-phase on centromeric chromatin and facilitates interaction with spindle microtubules to ensure accurate chromosome segregation. (B) The kinetochore ensemble comprises of the inner and outer kinetochore networks. The constitutive centromere associated network (CCAN) at the inner kinetochore ensures the adequate recruitment of the outer kinetochore KMN network through specific linker proteins such as CENP-T and CENP-C in vertebrates and also CENP-QOkp1-UAme1 in budding yeast (highlighted in a bold maroon border). Although kinetochores across eukaryotes function to ensure accurate chromosome segregation, plasticity across its composition and architecture is observed which is more pronounced amongst inner kinetochore components. Homologous complexes between human and budding yeast kinetochore components have the same color codes. Kinetochore homologs have been mentioned in the corresponding positions.
In the last ∼30 years, great progress has been made towards the identification of kinetochore components, analysis of sub-complex functions, and their organization at the kinetochore across several model systems. Through these studies, the structural components of the kinetochore can be broadly classified into inner and outer layers. The centromere-specific histone H3 variant and hereditary factor CENP-A and the constitutive centromere associated network (CCAN) form the centromeric chromatin proximal inner layer (De Rop et al., 2012; Fukagawa and Earnshaw, 2014; McKinley and Cheeseman, 2016). While the outer kinetochore is comprised of the microtubule interaction facilitating KMN (Knl1, Mis12, and Ndc80 complexes) network (Figure 1B) (Musacchio and Desai, 2017).
The 16-member CCAN consists of CENP-C, CENP-L-N, CENP-H-I-K-M, CENP-T-W-S-X, and CENP-O-P-Q-U-R subcomplexes in vertebrates (Figure 1B) (Hara and Fukagawa, 2017). Although having distinct sub-complex functions, overall, the CCAN works to recuit and maintain centromeric CENP-A, mediate chromosome congression, and recruit the outer kinetochore components. Key CCAN components with the ability to simultaneously interact with centromeric chromatin and the outer kinetochore, such as CENP-T, CENP-C, or CENP-QOkp1-UAme1 in yeasts are defined as linkers in this review. Although being assisted by other kinetochore components in various capacities, it is only these known linker proteins that not only can recruit the outer kinetochore but also propagate spindle forces from them and transmit it to the underlying centromeric chromatin (Figures 1B, 2A–C). Thereby, these linker pathways form critical pillars to establish a functional kinetochore.
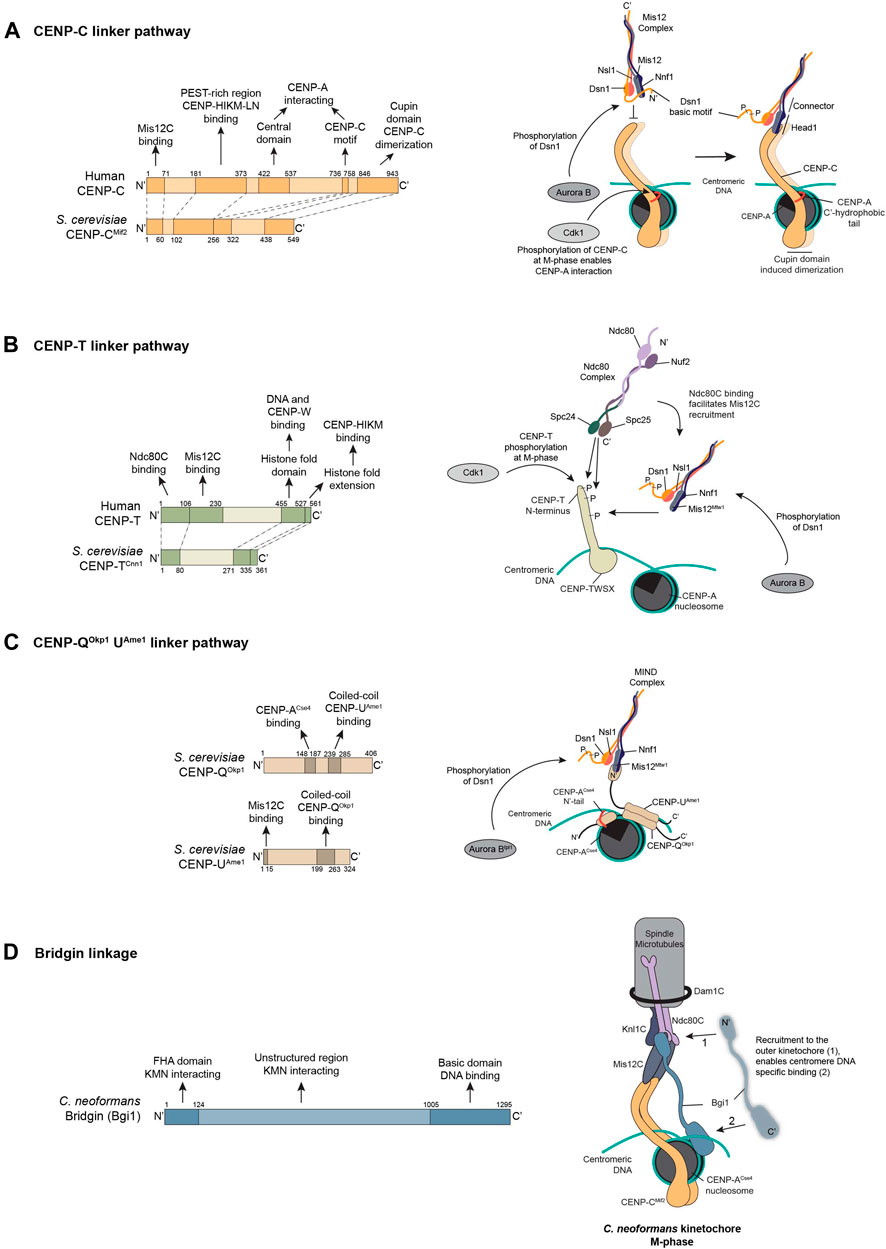
FIGURE 2. Linker pathways connect the outer kinetochore to centromeric chromatin. (A) The CENP-C linker pathway originates through the interactions of CENP-C with the C-terminal hydrophobic tail of CENP-A at the inner kinetochore. Subsequently, CENP-C through its N-terminal motif interacts with the Mis12-Nnf1 head of the Mis12C. This interaction is weakened/inhibited by the Dsn1 basic motif that binds to Mis12 and diminishes interaction with CENP-C in its unphosphorylated form. Aurora B-dependent phosphorylation alleviates this autoinhibition. (B) CENP-T complex interacts with centromeric linker DNA through a nucleosome-like structure formed by the histone-fold domains of CENP-T-W-S-X. At the N-terminus, in the human CENP-T, two Ndc80C recruitment sites exist which is under the control of Cdk1 phosphorylation. This Ndc80C binding subsequently facilitates the recruitment of the Mis12C. The phosphorylated form of Mis12C by Aurora B preferentially binds to CENP-T. (C) CENP-QOkp1-UAme1 has been described to serve as a linker pathway in budding yeast exclusively. While CENP-QOkp1 interacts with the CENP-ACse4 N-terminal tail, CENP-QAme1 has been described to interact with the Mis12Mtw1-Nnf1 head similar to CENP-CMif2 ensuring the recruitment of the Mis12CMIND. (D) In C. neoformans, CENP-CMif2 is the only conventional linker pathway described. Interestingly, a Ki-67-like protein named bridgin (Bgi1) was identified which is recruited to the outer kinetochore by the KMN network. This kinetochore-specific recruitment facilitates Bgi1 to subsequently interact with centromeric chromatin through its basic C-terminal motif. Thus generating a linkage between the outer kinetochore and centromeric chromatin.
The outer kinetochore functions as the primary site for spindle microtubule-binding and is chiefly made up of the 10-member KMN network comprising the Knl1 complex (Knl1C), Mis12 complex (Mis12C), and Ndc80 complex (Ndc80C) (Lampert and Westermann, 2011; Musacchio and Desai, 2017). More recently, the structure of several of these sub-networks of proteins has been resolved and the kinetochore particle reconstituted, although several questions persist (Akiyoshi et al., 2010; Dimitrova et al., 2016; Petrovic et al., 2016; Weir et al., 2016; Hinshaw and Harrison, 2019; Yan et al., 2019; Pesenti et al., 2022; Yatskevich et al., 2022). Interestingly, the single kinetochore module present on the budding yeast point centromere seems to occur multivalently across larger regional centromeres where they are observed to form multiple kinetochore-microtubule attachments (Figure 1B). What started as a study in understanding a functionally conserved chromosome segregation machinery in defined model systems has grown today into an exploration towards understanding the diversity and rapid evolution of this system while retaining its functional conservation (Drinnenberg et al., 2016). With the improvement in genomic sequencing and bioinformatic tools, it is evident that the outer kinetochore components are rather well conserved across eukaryotic evolution (D’Archivio et al., 2017; Hooff et al., 2017; Plowman et al., 2019). By contrast, the platform that recruits it, the inner kinetochore, has undergone greater diversity, and components at the inner kinetochore have evolved a multitude of strategies to recruit the outer kinetochore to establish a functional kinetochore (Meraldi et al., 2006; Hooff et al., 2017). The last eukaryotic common ancestor (LECA) is suspected to contain a full compliment of the CCAN, including known linker components (Tromer et al., 2019). However, it is clear from recent comparative genomic studies that some CCAN components are often subsequently lost during evolution. Through this, the CENP-C linker pathway arises as the most conserved across evolution. The CENP-QOkp1-UAme1 linker pathway is exclusive to budding yeast. While components of the CENP-T pathway are recurrently lost across eukaryotic evolution (Hooff et al., 2017; Plowman et al., 2019). Being highly diverged, identification of homologs for these CCAN components is challenging and further analysis may identify additional homologs. However, biochemical analysis of Kinetoplastids kinetochores has revealed a whole new complement of kinetochore components validating the loss of all known kinetochore proteins (Akiyoshi and Gull, 2014; Ishii and Akiyoshi, 2022). Further predictions in Metamonads, Diplonemids and Euglenids suggest a similar loss event of most known kinetochore components (Butenko et al., 2020; Salas-Leiva et al., 2021; Tromer et al., 2021). Driven by recent exciting findings, in this review we explore the plasticity and mechanisms of how the inner kinetochore is set up during mitotic progression to recruit the essential outer kinetochore from available biochemical, and comparative genomic studies.
2 Outer Kinetochore and the KMN Network
The 10-member KMN network consisting of the Knl1C, Mis12C, and Ndc80C is predicted to form the most structurally conserved section of the kinetochore (Figure 1B). With Nuf2 and Ndc80, components of the Ndc80C, being the best conserved of them and only predicted to be lost in certain species of the group Euglenazoa and Metamonada, while the Mis12C is additionally lost in Apicomplexans (D’Archivio et al., 2017; Hooff et al., 2017; Plowman et al., 2019; Butenko et al., 2020; Brusini et al., 2021a; Salas-Leiva et al., 2021). The KMN network conservation is likely a consequence of its function in forming the primary interface with the near-ubiquitous segregation force generator, the spindle microtubules. The KMN network not only facilitates end-on attachments but also tracks depolymerizing microtubules, in turn transducing spindle forces to move chromosomes. Although functioning together to form accurate kinetochore-microtubule attachments, components of the KMN network: the Knl1C, comprising Knl1 and Zwint-1, the Mis12C, comprising Mis12, Dsn1, Nnf1, and Nsl1, and the Ndc80C, comprising of Spc24, Spc25, Nuf2, and Ndc80 (Figure 1B), have distinct functional roles. A number of extensive reviews have discussed the structure and function of the KMN network and thus we will only briefly describe the complexes (Lampert and Westermann, 2011; Cheeseman, 2014; Musacchio and Desai, 2017).
The 4-member Ndc80C, which is an ∼55 nm long-heterotetramer, forms the main microtubule contact site (Figure 1B) (Cheeseman et al., 2006; DeLuca et al., 2006). The complex is comprised of two dimers Nuf2-Ndc80, and Spc24-Spc25 that are held together by the overlapping α-helical coiled-coil domains in the C-termini of Nuf2-Ndc80 and N-termini of Spc24-Spc25 (Wei et al., 2007; Cheeseman and Desai, 2008; Ciferri et al., 2008). The Nuf2 and Ndc80 subunits each contain calponin-homology (CH) domains at their N-termini that are tightly packed in the Ndc80C structure to mediate microtubule binding (Ciferri et al., 2008; Valverde et al., 2016; Wei et al., 2007). Additionally, the highly disordered basic N-terminal tail of Ndc80 has been implicated in microtubule interactions (Ciferri et al., 2008; Guimaraes et al., 2008; Miller et al., 2008). Kinetochore targeting of the Ndc80C is mediated by Spc24-Spc25 through interactions with either CENP-T or Dsn1-Nsl1 subunits of the Mis12C (Figure 2B) (Petrovic et al., 2010; Gascoigne et al., 2011; Bock et al., 2012; Schleiffer et al., 2012; Hori et al., 2013; Malvezzi et al., 2013; Nishino et al., 2013; Petrovic et al., 2016).
The ∼20 nm long rod-shaped Mis12C, also known as the MIND complex in S. cerevisiae, forms the scaffold enabling the nucleation of the KMN network via harboring binding sites for both Ndc80C and Knl1C (Figure 1B) (Maskell et al., 2010; Petrovic et al., 2010). The Mis12C heterotetramer is formed by dimers of Dsn1-Nsl1, and Mis12-Nnf1. The subunits are structural paralogs having high helical content (Figure 2A). Linear motifs close to the C-termini of Nsl1 and Dsn1 provide binding sites to the RWD domains present in the Ndc80C subunits of Spc24-Spc25. The Nsl1 C-terminal tail in addition to an extended interface generated by the C-terminal four-helix bundle in the stalk of the Mis12C enables interaction with the Knl1C (Petrovic et al., 2010; Dimitrova et al., 2016; Petrovic et al., 2016).
Knl1C is a heterodimer of Knl1 (Spc105 in fungi) and Zwint-1 or its homolog Kre28/Sos7 in fungi. Knl1 is a largely disordered protein with a coiled-coil region followed by the tandem RWD domains at its C-terminus (Figure 1B). The RWD domain facilitating protein-protein interactions is a recurring module at the kinetochore, with up to eight kinetochore proteins harbouring it (Schmitzberger and Harrison, 2012; Tromer et al., 2019). The coiled-coil domain on Knl1 plays host to the interaction with Zwint-1, while the RWD domain mediates interactions with the Mis12C (Petrovic et al., 2010; Petrovic et al., 2014; Petrovic et al., 2016). The largely unstructured N-terminal region of Knl1 comprises an array of protein docking sites, recruiting proteins such as PP1 and Bub1-Bub3 critical in regulating kinetochore dynamics, activating SAC, and in error correction (Kiyomitsu et al., 2007; Cheeseman and Desai, 2008). Towards SAC activation, Bub1-Bub3 is recruited through Met-Glu-Leu-Thr (MELT) repeats following the phosphorylation of the conserved Thr residue by Mps1 kinase (Joglekar, 2016). Additionally, residues in the extreme N-terminus are also involved in microtubule-binding and bundling which is required for checkpoint silencing (Espeut et al., 2012; Bajaj et al., 2018).
Outside of the KMN network, the outer kinetochore also consists of accessory factors that aid in tracking microtubules during the M-phase (Cheeseman, 2014). The functionally analogous 10-member Dam1 complex (Dam1C) or the 3-member Ska complex (SkaC) performs this function and is found to be widespread, but exceptionally inverse in their conservation across eukaryotic evolution (Figure 1B) (Hooff et al., 2017). The Dam1C is abundantly conserved across fungi, while the SkaC is observed in other systems. It is also suggested that components of the Dam1 complex are analogs of the Ska complex, likely existing in the LECA (van Hooff et al., 2017). Being out of the scope of this review further insights into these complexes can be found in other publications (Jeyaprakash et al., 2012; Cheeseman, 2014; Abad et al., 2016; van Hooff et al., 2017; Jenni and Harrison, 2018).
Although being largely conserved across eukaryotic evolution and playing a critical role in microtubule binding and SAC recruitment, a single ubiquitously conserved mechanism to ensure KMN network recruitment at the kinetochore is not observed rather a diversity of linkages has been reported across eukaryotes (discussed below), with systems regularly hosting multiple pathways.
3 Inner Kinetochore and the CCAN
The inner kinetochore comprises the centromere-specific histone H3 variant CENP-A and the 16-member CCAN in vertebrates (Figure 1B) (Hara and Fukagawa, 2017). In the budding yeast system, the CCAN is referred to as the Ctf19 complex (Ctf19C), wherein homologs for CENP-M and CENP-R are absent (Figure 1B). The Ctf19C in addition contains Nkp1-Nkp2 proteins, which share ancestry with the Mis12CMIND (Figure 1B) (Biggins, 2013; Tromer et al., 2019). While CENP-A marks an active centromere in most species, recent studies have identified species in which CENP-A is lost and does not exist on a centromeric locus that forms a functional kinetochore. Of the recently identified CENP-A-deficient systems, Bombyx mori and Mucor circinelloides have retained CCAN components (Navarro-Mendoza et al., 2019; Cortes-Silva et al., 2020), while kinetoplastids have in addition lost all known kinetochore components (Akiyoshi and Gull, 2014; Ishii and Akiyoshi, 2022). CENP-A, when present, forms a nucleosome structure in which the canonical histone H3 is replaced with CENP-A. Much debate has surrounded as to what makes the CENP-A chromatin “special,” summarized in other studies (McKinley and Cheeseman, 2016; Ali-Ahmad and Sekulić, 2020; Mitra et al., 2020a). CCAN components CENP-C and CENP-N in vertebrates directly bind to the CENP-A nucleosome to assemble the whole CCAN structure in the centromeric chromatin (Carroll et al., 2009; Kato et al., 2013; Watanabe et al., 2019; Ariyoshi et al., 2021), and CENP-UAme1-QOkp1 in budding yeast also directly binds to the CENP-ACse4 nucleosome (Anedchenko et al., 2019; Fischböck-Halwachs et al., 2019). This recognition of centromeric chromatin by CCAN factors facilitates the formation of the kinetochore. How the kinetochore assembles on CENP-A deficient centromeres is unknown and a critical question in this field.
Cryo-EM structures of the human (Pesenti et al., 2022; Yatskevich et al., 2022) and yeast (Hinshaw and Harrison, 2019; Yan et al., 2019) CCAN complexes have greatly aided in our understanding of its structure-function. The CCAN is observed as a defined complex where its subunits interdigitate rather than forming a network of binary interactions. Further, the Y-shaped opening of the budding yeast CCANCtf19C cradles the CENP-ACse4 nucleosomes on either side in a ratio of 2:1. A conserved feature across systems is the strong binding to linker DNA by the CENP-L-N channel. Reminiscent of canonical nucleosomes the CENP-T-W-S-X and CENP-H-I-K modules partially wrap linker DNA. Thus, the CCAN through its tight entrapment of linker DNA provides insights into how the strong push-pull forces of the mitotic spindle are handled by the inner kinetochore. While the formation of the CCAN on centromeric chromatin is critical, it is insufficient for a full kinetochore activity. This requires the recruitment of the microtubule interacting outer kinetochore by linker members of the CCAN.
4 Linkages Connecting the Outer Kinetochore to Centromeric Chromatin
As linkers, CENP-C, CENP-T, or CENP-QOkp1-UAme1 function to recruit the outer kinetochore either as a complete KMN network unit, through the interaction of the Mis12C with CENP-C/-T/-QOkp1-UAme1, or only the Ndc80C through the direct interaction with the N-terminal region of CENP-T (Figures 2A–C). In addition, these linker pathways also function to effectively transmit forces from the spindle-bound outer kinetochore to chromosomes, manifesting as “stretch” in the disordered regions in CENP-T and CENP-C (Hara et al., 2018; Suzuki et al., 2011; Suzuki et al., 2014; Uchida et al., 2021; Ye et al., 2016). At the inner kinetochore, the linker pathways of CENP-C and CENP-QOkp1-UAme1 contact centromeric chromatin through CENP-ACse4 (Figures 2A,C) (Anedchenko et al., 2019; Ariyoshi et al., 2021; Fischböck-Halwachs et al., 2019; Kato et al., 2013). While, the CENP-T complex has been described to bind centromeric DNA and induce supercoiling in it (Figure 2B) (Hori et al., 2008a; Nishino et al., 2012; Takeuchi et al., 2014; Pesenti et al., 2022; Yatskevich et al., 2022). The multifaceted roles of linker proteins at the kinetochores make them key candidates for regulatory action (Hara and Fukagawa, 2019). Thus, linker proteins function as a critical junction between centromeric chromatin and the outer kinetochore. Here we summarize the detailed understanding of each of the defined linkages across model systems (Figures 2A–D).
4.1 The CENP-C Pathway
CENP-C, along with CENP-A and CENP-B, was identified as an antigen detected by the sera from patients diagnosed with the autoimmune syndrome CREST (Calcinosis, Reynaud’s syndrome, Esophageal dysmotility, Sclerodactyly, Telangiectasia) (Moroi et al., 1980). The following works characterized CENP-C as a centromere protein and later an inner kinetochore component required for cell cycle progression and in the maintenance of the kinetochore’s trilaminate structure (Earnshaw and Rothfield, 1985; Saitoh et al., 1992; Tomkiel et al., 1994; Fukagawa and Brown, 1997; Kalitsis et al., 1998). Thus, cementing CENP-C as the first described component of the kinetochore.
CENP-C has multiple conserved domains which enable interaction with the outer kinetochore, multiple CCAN components, and the CENP-A nucleosome (Figure 2A). These include the N-terminal domain for Mis12C binding (Gascoigne et al., 2011; Hori et al., 2013; Petrovic et al., 2016; Przewloka et al., 2011; Screpanti et al., 2011), a middle conserved region that binds CENP-H-I-K-M and -L-N (Klare et al., 2015; McKinley et al., 2015; Nagpal et al., 2015), two CENP-A binding motifs in humans (Carroll et al., 2010; Kato et al., 2013; Guo et al., 2017), with one each in chicken and budding yeast (Cohen et al., 2008; Watanabe et al., 2019; Ariyoshi et al., 2021), and an extreme C-terminal cupin domain involved in CENP-C dimerization (Figure 2A) (Cohen et al., 2008; Trazzi et al., 2009). Owing to its extensive interaction at the kinetochore, CENP-C has been proposed to form a central hub for kinetochore assembly in human cells (Klare et al., 2015; Weir et al., 2016).
4.1.1 CENP-C Dynamics at the Inner Kinetochore
CENP-C was first described to have DNA binding capability (Yang et al., 1996) and later shown to interact specifically with the CENP-A nucleosome by recognizing its C-terminal hydrophobic tail, distinguishing it from canonical histone H3 (Figure 2A) (Westermann et al., 2003; Xiao et al., 2017; Kato et al., 2013). A recent work using in vitro reconstituted human full-length CENP-C from the Musacchio lab describes it to dimerize and bind simultaneously to two CENP-A nucleosomes in humans (Walstein et al., 2021). Interestingly this interaction of CENP-C with CENP-A was shown to be transient, occurring exclusively in M-phase, controlled by CDK1 phosphorylation in both chicken and human cells (Figure 2A) (Nagpal et al., 2015; Watanabe et al., 2019; Ariyoshi et al., 2021). Strikingly, chicken CENP-C contains a single CENP-A binding site, the CENP-C motif, which happens to be dispensable for cell viability. Although this motif is dispensable in human cells too, simultaneous disruption of a second CENP-A interacting motif, the central domain, is not (Watanabe et al., 2019). Unlike in the vertebrate systems, the sole CENP-ACse4 contact site, the CENP-C motif, is essential in budding yeast (Cohen et al., 2008; Hornung et al., 2014). This difference in CENP-C requirement at the inner kinetochore across systems further manifests itself in the localization hierarchy of CCAN components. A greater dependence of the CENP-H-I-K-M and CENP-T-W-S-X complexes on CENP-C is observed in budding yeast, human and Xenopus systems over chicken cells, but is not correlated with its function as a linker protein (see subsequent sections) (Westermann et al., 2003; Hori et al., 2008a; Krizaic et al., 2015; McKinley et al., 2015; Weir et al., 2016).
Although not constitutively bound to the CENP-A nucleosome, CENP-C is suggested to be recruited to the kinetochore in G1 in coordination with CENP-A recruitment in Xenopus (Krizaic et al., 2015). Outside M-phase, CENP-C interacts with CENP-H-I-K-M and CENP-L-N through its middle conserved region. This interaction retains CENP-C stably at the kinetochore in humans cells, while is described to be dynamic in chicken cells (Hemmerich et al., 2008; Nagpal et al., 2015; Watanabe et al., 2022). Additionally, CENP-B has been shown to preserve CENP-C anchoring at the centromere in the absence of CENP-A contacts in human cells (Hoffmann et al., 2016).
4.1.2 CENP-C Interaction With the Outer Kinetochore
Once anchored at the centromere, CENP-C can function to recruit and transmit KMN network-generated force (Ye et al., 2016). CENP-C through its ∼45-residue N-terminal motif contacts the Mis12C (Przewloka et al., 2011; Screpanti et al., 2011; Hornung et al., 2014; Liu et al., 2016; Richter et al., 2016). In the budding yeast, Kluyveromyces lactis, and in the fruit fly Drosophila melanogaster, CENP-C contacts the N-terminal head domain of Mis12 and Nnf1. Whereas in the human homolog a composite site comprising the head domain and helical connector of Dsn1-Nsl1 was shown to be required. Through these interactions, each CENP-C molecule has the potential to recruit a single KMN unit at the kinetochore (Figure 2A) (Dimitrova et al., 2016; Petrovic et al., 2016; Richter et al., 2016).
This interaction between CENP-C and the Mis12C is regulated by Aurora B kinase-dependent phosphorylation. In an intra-Mis12C manner the N-terminal basic motif residing in a disordered region of Dsn1 masks the CENP-C interaction site on the Mis12C in its unphosphorylated state. This limits the interaction between CENP-C and the Mis12C. Upon phosphorylation by Aurora B kinase, the inhibitory motif moves away from the interaction site, allowing for ∼150-fold increased binding affinity of the Mis12C to CENP-C (Figure 2A) (Welburn et al., 2010; Kim and Yu, 2015; Dimitrova et al., 2016; Petrovic et al., 2016). As a part of this regulatory cycle, the phosphorylation is suggested to be countered by PP1, more so during M-phase induced stretch when the Mis12C is further away from the influence of centromere-localized Aurora B. This may further be magnified in anaphase when Aurora B relocalizes to the midzone (Hara et al., 2018; Lang et al., 2018). Interestingly, the D. melanogaster Dsn1 homolog lacks the basic inhibitory motif in its N-terminal region, thus eliminating this autoinhibition at its kinetochore.
Consistent with the ability of the CENP-C N-terminus to recruit a full complement of the KMN network, artificial tethering experiments of this region to a non-centromeric locus by the LacO-LacI system in chicken DT40 cells and human cell lines were able to ensure the normal segregation of the tethered chromosome (Gascoigne et al., 2011; Hori et al., 2013). Importantly no other CCAN components were localized to the tethered site. This suggests that at the ectopic loci the CENP-C N-terminus was necessary and sufficient for recruiting the KMN network and in forming functional kinetochore-microtubule attachments through the extensive multicopy tethering system (Gascoigne et al., 2011; Hori et al., 2013). Thus, to prevent the unregulated recruitment of a functional outer kinetochore unit on rogue CENP-C at a non-centromeric locus, the Westermann lab recently reported that CENP-CMif2 autoinhibition prevents interaction with the Mis12CMIND in the absence of CENP-ACse4 binding in budding yeast cells (Killinger et al., 2020).
4.2 The CENP-T Pathway
CENP-T was identified as a component of the CENP-A containing centromere complex (Obuse et al., 2004; Foltz et al., 2006; Izuta et al., 2006). Subsequent studies from our lab established it as a key component of the inner kinetochore CCAN network required for the recruitment of KMN network components (Figure 2B) (Hori et al., 2008a; Nishino et al., 2012, 2013; Hara et al., 2018; Takenoshita et al., 2022). Initially, the budding yeast kinetochore was thought to lack CENP-T and be divergent from vertebrate kinetochores. However, robust computations analysis identified the CENP-T homolog as the budding yeast protein Cnn1 (Schleiffer et al., 2012). Interestingly, Cnn1 was identified before the discovery of the vertebrate CENP-T as “copurified with Nnf1” (De Wulf et al., 2003) and was suggested to be a point centromere-specific protein (Meraldi et al., 2006). The difficulty in recognizing CENP-T homologs, similar to other CCAN components, highlights their sequence divergence across evolution (Hooff et al., 2017).
CENP-T is the dominant protein of its namesake complex. A functional CENP-T complex has been shown to comprise the CENP-T-W (Hori et al., 2008a) and CENP-S-X (Amano et al., 2009) dimers. Each subunit contains a histone fold (Nishino et al., 2012). Phylogenetic analysis has suggested that CENP-T-W-S-X may have arisen after two duplication events of CENP-S-T and CENP-X-W, which is interconnected with the origin of the eukaryotic transcription and DNA repair machinery (Tromer et al., 2019). This is owing to the role of CENP-S-X in the Fanconi Anaemia pathway (Singh et al., 2010; Yan et al., 2010).
4.2.1 CENP-T Dynamics at the Inner Kinetochore
The CENP-T-W and CENP-S-X dimers can form a heterotetrameric nucleosome-like structure that can bind DNA and induce positive super-coiling, unlike conventional nucleosomes that generate negative supercoils at budding yeast mini-chromosomes (Furuyama and Henikoff, 2009; Nishino et al., 2012; Takeuchi et al., 2014; Pesenti et al., 2022; Yatskevich et al., 2022). Loss of CENP-S-X has shown not to strongly affect CENP-T-W levels at the kinetochore although CENP-S is sufficient to recruit CENP-T to an ectopic locus (Amano et al., 2009; Nishino et al., 2012).
Although being constitutively localized at centromeric chromatin, CENP-T has been shown to be rapidly turned over and not stability inherited at the human kinetochore (Prendergast et al., 2011). In budding yeast, CENP-TCnn1 levels rapidly increase post anaphase onset which is regulated by multiple mitotic kinases (Bock et al., 2012). Loading of CENP-T-W at the human kinetochore has been suggested to take place in the S and G2 phase of the cell cycle, independent of DNA replication and CENP-A presence but requiring the H2A/B chaperone, FACT (Hoffmann et al., 2016; Prendergast et al., 2011, Prendergast et al., 2016).
Although the precise mechanism of CENP-T regulation and deposition at the kinetochore is not understood, studies have shown that the interaction of CENP-T-W with the CENP-H-I-K complex is critical for its stable retention. A major binding interface is formed between the conserved CENP-T C-terminal histone-fold extension α-helix and a CENP-K α-helix (Hori et al., 2008a; Nishino et al., 2012; Basilico et al., 2014; McKinley et al., 2015; Pekgöz Altunkaya et al., 2016; Hinshaw and Harrison, 2020; Zhang et al., 2020; Pesenti et al., 2022; Yatskevich et al., 2022). Although CENP-T interaction with CENP-H-I-K is essential, it is insufficient for CENP-T complex kinetochore localization, as point mutations that affect DNA binding in CENP-T-W also completely abolish CENP-T localization (Nishino et al., 2012; McKinley et al., 2015). Additionally, CENP-A tails have been shown to affect CENP-T kinetochore levels in fission yeast and in human cells during centromere establishment (Folco et al., 2015; Logsdon et al., 2015). Thus, addressing how the synergy between CCAN contacts and DNA binding helps maintain and recruit CENP-T at the kinetochore requires further investigation and would aid greatly in a holistic view of the inner kinetochore assembly. In addition to CCAN factors, recent studies have also implicated the SUMO protease SENP6 in maintaining CCAN protein levels including CENP-T and CENP-C at the kinetochore, consequently affecting CENP-A maintenance (Mukhopadhyay et al., 2010; Liebelt et al., 2019; Wagner et al., 2019; Mitra et al., 2020b).
The CENP-T complex being the only other histone-fold containing complex identified at the kinetochore, after CENP-A, raises the question as to how it is positioned within the kinetochore structure? Insights from in vitro studies using chicken proteins suggest that the CENP-T complex binds preferentially to an ∼100 bp linker DNA over nucleosome-bound DNA (Figure 2B) (Takeuchi et al., 2014). Indeed, the recent structure of the human CCAN described CENP-T-W-S-X together with CENP-H-I-KHead to partially wrap around linker DNA reminiscent of canonical histones (Yatskevich et al., 2022), as previously shown with DNA and the recombinant CENP-T-W-S-X complex (Nishino et al., 2012; Takeuchi et al., 2014). This is consistent with ChIP analysis, positioning CENP-T between two CENP-A nucleosomes at the regional centromeres of humans and fission yeast (Thakur et al., 2015; Thakur and Henikoff, 2016). On the other hand, in the point centromere containing budding yeast, CENP-TCnn1 is suggested to bind the core centromere particle, a region overlapping with CENP-ACse4, and not form a separate nucleosome-like particle (Pekgöz Altunkaya et al., 2016; Hinshaw and Harrison, 2020; Zhang et al., 2020). Thus, the positioning of CENP-T at the kinetochore may vary to accommodate the constraints of the system differing from point to regional centromeres which might offer an explanation towards its variable interdependencies (Hori et al., 2008a; Pekgöz Altunkaya et al., 2016; Walstein et al., 2021).
4.2.2 CENP-T Interaction With the Outer Kinetochore
CENP-T possesses a long unstructured N-terminal region, unlike the other small histone-fold containing proteins, CENP-W, -S, and -X of the CENP-T complex. The interaction of the CENP-T complex with the outer kinetochore occurs through multiple domains contained in this CENP-T N-terminal region (Figure 2B) (Bock et al., 2012; Schleiffer et al., 2012; Rago et al., 2015; Hara et al., 2018). At the extreme amino-terminal end two Ndc80C binding motifs in humans, and one each in chicken cells or budding yeast are present. Following this is the Mis12C binding site that ensures a complete KMN network ensemble on CENP-T (Emanuele et al., 2008; Nishino et al., 2013; Rago et al., 2015; Huisin’T Veld et al., 2016; Hara et al., 2018; Takenoshita et al., 2022). Both Ndc80C and Mis12C interactions with the CENP-T N terminus are regulated by multiple phosphorylation events (Figure 2B) (see below in detail). Interestingly, no Mis12CMIND binding on the budding yeast CENP-TCnn1 has been reported.
CENP-W is an integral interacting partner of CENP-T, and its absence has been shown to severely affect CENP-T at the kinetochore, inturn affecting outer kinetochore levels (Hori et al., 2008a; Bock et al., 2012; Nishino et al., 2012, Nishino et al., 2013; Schleiffer et al., 2012). Yet interestingly, in the B. mori system, biochemical searchers did not detect any homolog of CENP-W (Cortes-Silva et al., 2020). While in the Xenopus egg extract system, CENP-T and CENP-W might have variable temporal dynamics during de novo kinetochore formation (Krizaic et al., 2015).
On the other hand, CENP-S-X loss has been shown not to alter CENP-T levels at the kinetochore, yet they have been described to influence the localization of the outer kinetochore (Amano et al., 2009; Nishino et al., 2012). If this is through the CENP-T recruited KMN network is to be explored. While, in the moss system of Physcomitrella patens, the conditional knockdown of CENP-S-X results in segregation defects that phenocopies other outer kinetochore components although not exhibiting distinct kinetochore localization (Kozgunova et al., 2019). Thus, further analysis following these insights may lead to a better functional understanding of the CENP-T complex components.
Recruitment of the outer kinetochore by CENP-T in vertebrates occurs exclusively in the M-phase, with CDK1 phosphorylation-dependent binding of the Ndc80C. This binding of the Ndc80C onto CENP-T is necessary for subsequent recruitment of the Mis12C, which is also under the influence of CDK1 phosphorylation of CENP-T (Figure 2B) (Kettenbach et al., 2011; Nishino et al., 2013; Rago et al., 2015; Suzuki et al., 2015; Huisin’T Veld et al., 2016). In addition, Dsn1 of the Mis12C is also necessary to be phosphorylated by Aurora B kinase for stable binding to CENP-T (Figure 2B) (Walstein et al., 2021). In budding yeast, the Ndc80C recruitment may not be regulated by phosphorylation, but indirectly controlled through the phosphorylation-dependent increase of CENP-TCnn1 itself at anaphase onset (Bock et al., 2012; Malvezzi et al., 2013). In an interesting twist, CENP-TCnn1 has also been shown to be able to localize to the kinetochore via its N-terminal Spc24/25 interacting sequence (Thapa et al., 2015). Considering CENP-TCnn1 and the Mis12C compete for the same Spc24/25 binding site. Which pool of the Ndc80C, the N-terminal CENP-TCnn1 is recruited by is not known. Convergently, the Ndc80C binding site on CENP-T resembles that on Dsn1 (Malvezzi et al., 2013; Dimitrova et al., 2016; Hara et al., 2018). Yet, strikingly, the CDK1 phosphorylation which is required for Ndc80C recruitment on CENP-T reduces the affinity of the Ndc80C for Dsn1 (Rago et al., 2015). The intention of this regulation is not yet understood.
Previous studies propose that the Mis12C binds to CENP-T using an overlapping region that is also involved in CENP-C binding. Thus forcing CENP-T and CENP-C to compete for Mis12C binding (Huisin’T Veld et al., 2016). More recent work builds on these findings, suggesting that in addition, the Aurora B kinase-dependent phosphorylation that limits interactions between Mis12C and CENP-C might also be involved in the Mis12C-CENP-T interaction (Walstein et al., 2021). Overall, each CENP-T molecule, dependent on the system, is capable of recruiting 2 or 3 Ndc80C. One or two Ndc80C are recruited directly and an additional Ndc80C is recruited via the Mis12C binding onto CENP-T (Figure 2B) (Rago et al., 2015; Huisin’T Veld et al., 2016; Hara et al., 2018; Takenioshita et al., 2022).
To test the sufficiency of the CENP-T N-terminus in recruiting a functional outer kinetochore, tethering of this region at an ectopic site by the LacO-LacI system was performed. This tethering was found to be sufficient to segregate the chromosome harboring the tethered site in vertebrates systems, as well as in the context of an autonomous-replicating sequence (ARS) containing plasmid in budding yeast (Gascoigne et al., 2011; Schleiffer et al., 2012; Hori et al., 2013). Thus, like the CENP-C pathway, CENP-T via its N-terminus can recruit a functional unit of the outer kinetochore without the presence of any other CCAN at an ectopic locus, to ensure chromosome segregation.
4.3 The CENP-QOkp1-UAme1 Pathway in Budding Yeasts
S. cerevisiae harbors an almost complete complement of the CCANCtf19C (Figure 1B). Yet, CENP-CMif2, CENP-QOkp1, and CENP-UAme1 are the only CCANCtf19C components that are essential for viability (Biggins, 2013). CENP-QOkp1 and CENP-UAme1 form a dimeric subcomplex which along with CENP-OMcm21 and CENP-PCtf19 constitute the COMA complex (Hyland et al., 1999; Ortiz et al., 1999; Poddar et al., 1999; Cheeseman et al., 2002). CENP-QOkp1 and CENP-UAme1 contain a coiled-coil region in the C-terminal half and harbor no other distinct structural domains (Figure 2C) (Hornung et al., 2014). The Nkp1-Nkp2 heterodimer has been described to facilitate stabilization of the COMA complex through interactions with CENP-QOkp1-UAme1. The absence of Nkp1-Nkp2 in vertebrates may be substituted by its functional analog CENP-R (Schmitzberger et al., 2017; Hinshaw and Harrison, 2019; Yan et al., 2019).
Microscopic observation shows that CENP-QOkp1 and CENP-UAme1 are more abundant at the kinetochore than CENP-PCtf19, suggesting a variable functional unit of the COMA complex (Dhatchinamoorthy et al., 2017). Although CENP-OMcm21 and CENP-PCtf19 are non-essential for viability, they are required for accurate chromosome segregation (Hyland et al., 1999). CENP-QOkp1 and CENP-UAme1 lie upstream to all but CENP-CMif2 at the CCANCtf19C assembly hierarchy. Their levels increase in anaphase and are amongst the most abundant CCANCtf19C proteins at the kinetochore (Dhatchinamoorthy et al., 2017). While CENP-QOkp1-UAme1 is a linker protein in S. cerevisiae, vertebrate CENP-Q-U bind neither to outer kinetochore components nor to the CENP-A nuclesome, and the CENP-O-P-Q-U-R complex is not required for cell viability in chicken DT40 cells (Okada et al., 2006; Hori et al., 2008b; Kagawa et al., 2014), suggesting that vertebrate CENP-Q-U does not function as a linker pathway.
4.3.1 CENP-QOkp1-UAme1 Dynamics at the Inner Kinetochore
Unlike simultaneous interactions of CENP-T or CENP-C with centromeric chromatin and the outer kinetochore, a division of labor amongst the essential CENP-QOkp1 and CENP-UAme1 is observed. CENP-QOkp1 interacts with centromeric CENP-ACse4-contaning nucleosome while CENP-UAme1 interacts with the Mis12CMIND (Figure 2C) (Hornung et al., 2014; Anedchenko et al., 2019; Fischböck-Halwachs et al., 2019). CENP-QOkp1-UAme1 was initially described to interact with DNA which was not specific to the point centromeric sequences of budding yeast, suggesting other mechanisms to enable its centromere targeting (Hornung et al., 2014). Two recent studies (Anedchenko et al., 2019; Fischböck-Halwachs et al., 2019) describe in detail how CENP-QOkp1-UAme1 achieves this (Figure 2C). Fischböck-Halwachs et al. (2019) show that CENP-QOkp1 of the heterodimer was sufficient to form direct binding selectively to the CENP-ACse4 N-terminus. A 45-amino acid domain called the core domain of CENP-QOkp1 (residues 127–184) was critical for this interaction. In addition, Anedchenko et al. (2019) found cross-links between the centromeric nucleosome and CENP-UAme1 in the in vitro reconstituted complex. They also show that methylation on R37 and acetylation on K49 of CENP-ACse4 inhibit interaction with CENP-QOkp1-UAme1, suggesting a reader-like function for the heterodimer.
4.3.2 CENP-QOkp1-UAme1 Interaction With the Outer Kinetochore
Immunoprecipitation (IP) followed by mass-spectrometry (MS) analysis of CENP-UAme1 revealed Mis12Mtw1 as the most abundantly interacting KMN network component in S. cerevisiae. Follow-up studies using in vitro reconstitution described a short conserved motif containing 15 residues at the extreme N-terminal of CENP-UAme1 that was necessary and sufficient for interaction with the Mis12-Nnf1 globular head domain. The binding stoichiometry of CENP-UAme1 to the Mis12CMIND is 1:1. This interaction with the Mis12CMIND was further aided by the co-operative binding of CENP-CMif2 bringing the stoichiometry to 2:2:2 for CENP-CMif2:CENP-QOkp-1UAme1:Mis12CMIND at the kinetochore, after accounting for CENP-CMif2 dimerization (Hornung et al., 2014). Subsequent structural analysis of K. lactis proteins suggested that CENP-CMif2 and CENP-UAme1 bind noncompetitively to the globular head domain of Mis12Mtw1-Nnf1 at distinct interfaces in vitro (Dimitrova et al., 2016). However, in disagreement, a recent study from the Westermann lab (Killinger et al., 2020), shows using in vitro and in vivo assays that CENP-UAme1 and CENP-CMif2 compete with each other and do not bind to the same Mis12CMIND (Figures 2A,C). They further present evidence that instead of occupying spatially distinct sites on the Mis12C head I, they occupy largely overlapping or identical binding sites where mutations in the CENP-CMif2 binding interface of Mis12Mtw1 are lethal in cells and disrupt interactions with CENP-UAme1. This is further corroborated with N-terminal swap experiments suggesting no unique attribute of CENP- UAme1 in binding the Mis12CMIND and thus can be substituted with CENP-CMif2 (Killinger et al., 2020).
It is noteworthy that the same Aurora B kinase-dependent control of Mis12C interaction with CENP-C and CENP-T has been shown to promote the recruitment and strengthen the binding of the Mis12CMIND to CENP-UAme1 (Figure 2C) (Dimitrova et al., 2016). Contrary to this, Hamilton and others (Hamilton et al., 2020) suggest that at least in vitro CENP-UAme1 does not require the alleviation of Dsn1 autoinhibition for tight binding to Mis12C. Why the conflicting reports is not clear? Owing to the fact that mutation of residues S240 and S250 to alanine on Dsn1 renders cells harboring the URA-CEN plasmid inviable favors the model that both CENP-CMif2 and the CENP-UAme1 pathways fall under the influence of the Dsn1 autoinhibition-Aurora B regulatory system in budding yeast (Akiyoshi et al., 2013). Taken together, this suggests that the Aurora B phosphorylation-dependent removal of Dsn1 auto-inhibition is a critical step in outer kinetochore recruitment onto a linker platform and is conserved through Opisthokonta evolution (Figures 2A–C).
5 Kinetochore Architecture Based on Functional Linker Modules
Several linkages between the outer kinetochore and centromeric chromatin are reported as highlighted above (Figures 2A–C). Yet, it is unclear what evolutionary constraints drive the dominance/retention of specific pathways within each kinetochore ensemble thereby greatly influencing kinetochore architecture. In this section, we discuss some of the currently known strategies employed by organisms as well as ones that are likely, as alluded to through experimental and computational analysis. However, owing to the conservation of redundant pathways in certain systems, it is possible that the non-dominant but conserved pathways may be critical at an unresolved cellular context.
5.1 CENP-C-Pathway Dominant Kinetochores
Kinetochores containing the CENP-C module were some of the earliest described systems. The functional module of the CENP-C linker pathway consists of CENP-A-CENP-C-Mis12C. Recent findings from the Apicomplexan kinetochore however report the loss of the Mis12C, while retaining the CENP-A-CENP-C axis (Brusini et al., 2021a), suggesting plasticity of this linker module. Amongst the described model systems consisting of this functional unit, it is only in the systems of D. melanogaster and Caenorhabditis elegans that CENP-C is the major pathway (Figure 3). It so happens that CENP-C is also the exclusive linker protein present in the two systems. A recent work on the basidiomycete Cryptococcus neoformans also highlights CENP-C as the sole CCAN component retained and likely the dominant pathway albeit with certain adaptations (Figures 2D, 3) (Sridhar et al., 2021).
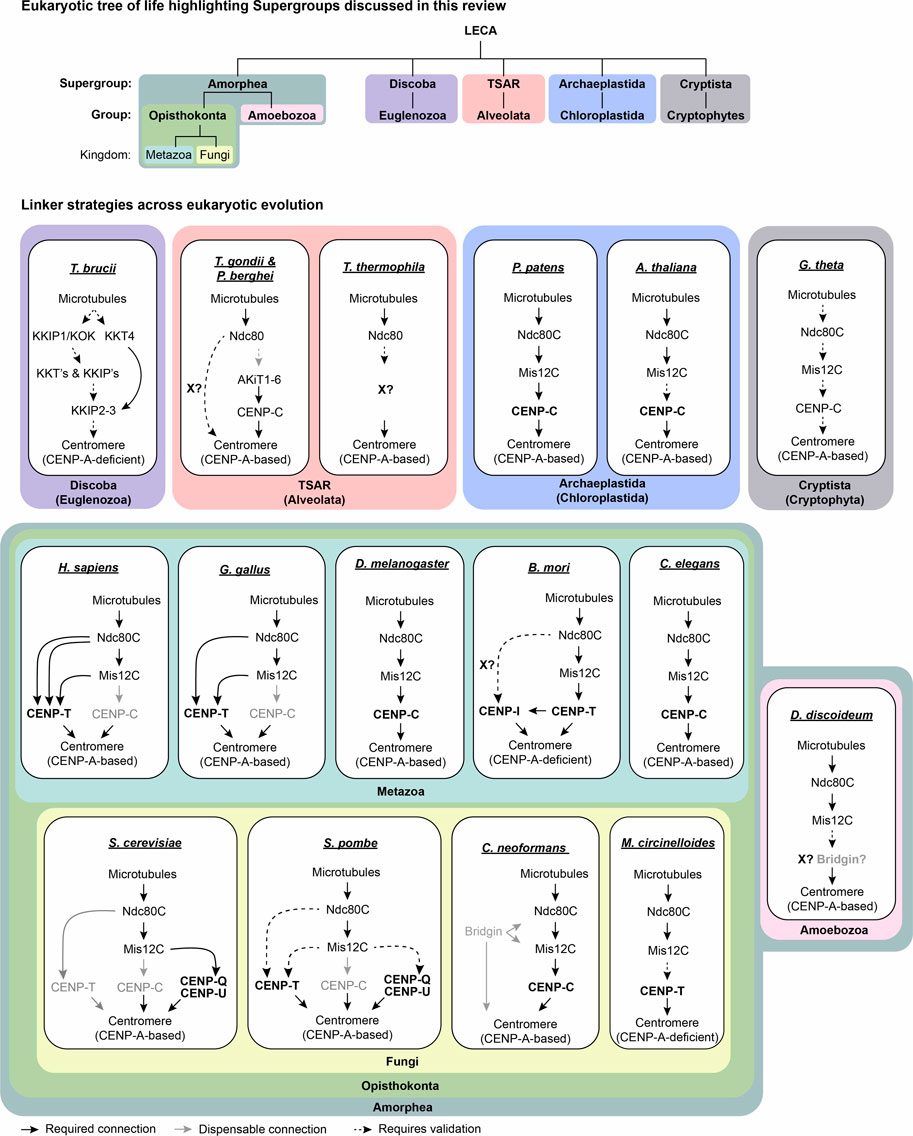
FIGURE 3. Linker strategies used across eukaryotic evolution to recruit the near-ubiquitous outer kinetochore. (Top) A cartoon highlighting the phylogenetic relationship between supergroups of representative species described in the bottom panel. (Bottom) The connection between the outer kinetochore centromeric chromatin across representative species is highlighted. The major linker pathway protein is highlighted in bold. Phylogenetic groups are mentioned in brackets where applicable. Names of kinetochore homologs are presented in the vertebrate format.
5.1.1 CENP-C Pathway Exclusive Systems
Extensive genetic and biochemical analysis of the D. melanogaster and C. elegans systems have failed to reveal other components of the CCAN but for CENP-C (Barth et al., 2014; Cheeseman et al., 2004; Goshima et al., 2007; Przewloka et al., 2007; Przewloka et al., 2011). The loss of CCAN components in a Dipteran ancestor is suggested to have taken place ∼250 million years ago (Drinnenberg and Akiyoshi, 2017). In the fruit fly, DmCENP-C is essential (Heeger et al., 2005), and interactions with the Mis12C have been shown to be critical for accurate chromosome segregation (Przewloka et al., 2011). Notably in D. melanogaster, the Mis12C component Dsn1 was suspected to be lost (Przewloka et al., 2007; Liu et al., 2016). However, more recent bioinformatic predictions suggest the previously identified DmNsl1 as the DmDsn1 homolog. Interestingly, DmDsn1 lacks the N-terminal region including the basic autoinhibitory motif (Hooff et al., 2017; Plowman et al., 2019). Additionally, two functionally redundant Nnf1 paralogs exist. Further Knl1Spc105 was required for Mis12 complex assembly (Venkei et al., 2012). It is difficult to determine if these variations arose as a consequence of CCAN loss.
Ectopic targeting of the N-terminal domain of DmCENP-C was shown to be sufficient to recruit the outer kinetochore. Using FRET and Talin-rod tension-sensing assays DmCENP-C was shown to not only be sufficient for recruiting the KMN network but also in transmitting spindle forces (Ye et al., 2016).
Studies on the holocentric chromosome-containing nematode worm, C. elegans, also suggest a strong reliance on CENP-CHCP−4 for kinetochore assembly and accurate chromosome segregation, phenocopying CENP-A (Oegema et al., 2001; Desai et al., 2003). The absence of other CCAN components, a failure to identify novel components, and the importance of CENP-CHCP−4 in kinetochore assembly, together suggest CENP-CHCP−4 as a major linker pathway in C. elegans (Figure 3). Strikingly, during meiotic divisions both CENP-A and CENP-CHCP−4 have been reported to be dispensable for chromosome segregation (Monen et al., 2005). What retains the persistent outer kinetochore on these holocentric chromosomes is yet unknown.
Kinetochore composition in plants of the group Archaeplastida may resemble that of D. melanogaster or C. elegans where most CCAN components have not been identified (Hooff et al., 2017; Yamada and Goshima, 2017). However, experimental approaches to address plant kinetochore composition are limited and further analysis is required to conclude the absence of the CCAN in Archaeplastida. While CENP-S-X was identified in P. patens, kinetochore localization was not observed and no CENP-T-W homolog is reported (Figure 3) (Kozgunova et al., 2019). Similarly, while a CENP-O homolog is predicted, kinetochore localization of this protein was unclear (Kozgunova et al., 2019). CENP-C remains the only conserved linker component present. Knockdown of components of the CENP-C functional module phenocopied each other resulting in kinetochore malfunction in P. patens. Interestingly, while CENP-C has been described as a constitutive centromere localizing protein in Maize and Arabidopsis thaliana (Dawe et al., 1999; Ogura et al., 2004; Kozgunova et al., 2019), in the single-cell system of P. patens, loss of its localization from centromeres was observed soon after M-phase (Kozgunova et al., 2019).
5.1.2 The C. neoformans Kinetochore
The kinetochore composition of the pathogenic basidiomycete C. neoformans echoes that of D. melanogaster or C. elegans where it is predicted to have lost all but CENP-CMif2 of the CCAN, while retaining CENP-ACse4 and a conserved outer kinetochore (Figure 2D) (Schleiffer et al., 2012; Hooff et al., 2017; Sridhar et al., 2017). CENP-CMif2 is essential for viability, and a conditional knockout results in kinetochore collapse and increased segregation defects (Sridhar et al., 2021). To identify if additional kinetochore factors exist and to validate predictions, IP-MS studies of known kinetochore proteins CENP-CMif2, Dsn1 and Spc25 were carried out. This identified all previously predicted kinetochore proteins, including CENP-CMif2 but none of the other CCAN components. Interestingly, upon screening for other possible kinetochore proteins, an FHA-domain containing 1,295 aa long protein, named bridgin (Bgi1), was identified to specifically localize to the kinetochore from G2 to M-phase, peaking in anaphase (Figure 2D) (Sridhar et al., 2021). Sharing kinetochore localization dynamics similar to the KMN network it was observed that bridgin depended on the KMN network components for its localization. This required the FHA-domain in addition to a subsequent middle disordered region (Figure 2D). Strikingly, upon domain analysis, it was observed that the C-terminal basic region of bridgin could interact non-specifically with DNA and reconstituted nucleosomes. This was supported by the observation that chromatin-associated factors were enriched in IP-MS fractions of Bgi1 full-length over its mutant lacking the basic C-terminal region. Since in vivo, exclusive recruitment of bridgin to the kinetochore was observed, it was concluded that specific interactions with centromeric chromatin identified by native-ChIP were initiated following its recruitment by the outer kinetochore (Figure 2D) (Sridhar et al., 2021).
While bridgin is dispensable for growth, it is critical for accurate chromosome segregation, and its absence results in increased sensitivity to microtubule-depolymerizing factors. Co-evolution analysis suggested that the retention of bridgin across basidiomycetes is correlated with the loss of other potential linker pathways such as CENP-TCnn1/-QOkp1-UAme1 (Sridhar et al., 2021). While CENP-CMif2 is the dominant pathway in C. neoformans, we suspect a unique scenario where the outer kinetochore might have evolved to recruit a linkage, via bridgin, connecting it to centromeric chromatin (Figure 3). This may facilitate the reinforcement of the CENP-CMif2 pathway critically at stages of high spindle tension such as anaphase when bridgin is most abundant at the kinetochore. Further insights into the transmission of force through this linkage would be interesting.
Curiously, bridgin is not only conserved across basidiomycetous fungi but also amongst other fungal phyla. Outside fungi, bridgin-like proteins are also identified in Ameobazoa (Figure 3). Amongst metazoans, the mitotic protein Ki-67 known to behave as a biological surfactant (Cuylen et al., 2016), shares bridgin-like features, thus suggesting functional divergence from a common ancestor (Sridhar et al., 2021).
5.2 CENP-T-Pathway Dominant Kinetochores
Soon after its characterization, tethering of CENP-T was shown to be sufficient to form a functional kinetochore at an ectopic locus (Hori et al., 2013). Yet, it is only recent studies that have shed light on the CENP-T linker pathway as being a viable mechanism at the native kinetochore to transmit force in not only systems that have lost the CENP-A-CENP-C module, as in the silk moth B. mori and Mucorales fungi M. circinelloides (Figure 3) (Navarro-Mendoza et al., 2019; Cortes-Silva et al., 2020), but also in well-studied vertebrate model systems such as the case with chicken DT40 cells (Figure 3) (Hara et al., 2018). We propose the dominance of the CENP-T linker pathway in the human and Xenopus systems as well (Figure 3) (see bellow). Thus, could the CENP-T dominant pathway be a feature of vertebrate kinetochores? CENP-T-Ndc80C module forms the most conserved core of this pathway.
5.2.1 CENP-T Pathway Dominant Systems
The N-terminal regions of CENP-T and CENP-C independently were shown to be capable of forming a functional kinetochore at an ectopic locus in the chicken DT40 system. This generated artificial kinetochore enabled chromosome segregation in the absence of the native centromeric loci (Hori et al., 2013). Yet within the context of the native kinetochore, it was observed that the N-terminal Mis12C binding region of CENP-C was dispensable, and the growth of the mutant lacking the N-terminal region of CENP-C was comparable to wild-type cells. On the other hand, the Mis12C and Ndc80C binding domains of CENP-T were essential for cell viability. Further, using a tension-sensor system it was shown that CENP-T but not CENP-C could effectively transmit spindle pulling forces during M-phase (Hara et al., 2018). Further evaluating the roles of KMN network proteins on CENP-T, it was recently shown that two Ndc80C on CENP-T recruited either directly or as part of the KMN network were required for chromosome segregation (Takenoshita et al., 2022). Taken together, this suggests that in chicken DT40 cells CENP-T functions as the dominant pathway even in the presence of a functional CENP-C module. It is of interest to understand why the equivalent KMN recruitment on CENP-T and CENP-C may have variable essentialities.
Soon after the description of the CENP-T reliant chicken DT40 kinetochore, in vivo evidence for the retention of a single canonical pathway through CENP-T while losing CENP-C/-Q-U, along with CENP-A, in the B. mori and M. circinelloides was reported (Navarro-Mendoza et al., 2019; Cortes-Silva et al., 2020). Analysis of the remarkable system of B. mori by the Drinnenberg lab through IP-MS analysis of several kinetochore components identified the KMN network components except for Zwint-1. At the inner kinetochore, no homologs of CENP-A or CENP-C were detected. Homologs for CCAN components CENP-O-P and CENP-H were identified, yet with very limited homologies, thus requiring further verification. Additionally, while CENP-T, -S, and -X were identified, surprisingly no CENP-W homolog was observed, a key component influencing CENP-T localization in other systems. CENP-T itself was found to be essential for cell viability, requiring its N-terminal and histone fold domains to function. Further, CENP-T was found to be sufficient for recruiting the outer kinetochore complex. However, conditional depletion of CENP-T was insufficient to eliminate all the Ndc80C at the kinetochore, although the loss of Mis12C was almost absolute. A complete loss of the Ndc80C was observed only in CENP-I knockdown, a component of the BmCENP-K-I-M complex on which CENP-T is also dependent (Figure 3) (Cortes-Silva et al., 2020). Thus, while CENP-T may account for an essential linker pathway in B. mori, the possible contribution of other pathways either directly or indirectly influenced by CENP-I cannot be ruled out. CENP-T has been identified in other related CENP-A-deficient insects that have also lost CENP-C (Cortes-Silva et al., 2020).
In the human pathogen, M. circinelloides, of the fungal subphylum Mucoromycotina, the CCAN components including CENP-T-W-S-X, along with CENP-H-I-K-M, CENP-L-N, and CENP-O-P have been identified, while CENP-A and CENP-C are absent. CENP-T was observed to be constitutively centromere localized (Figure 3). ChIP-seq analysis of the Mis12C components shows that kinetochores form on small mosaic centromeres, exhibiting features echoing that of budding yeast and fungal regional centromeres (Navarro-Mendoza et al., 2019). With the absence of CENP-C and CENP-Q-U proteins, CENP-T is the only other linker protein identified. Thus, addressing the contribution of the histone-fold containing CENP-T complex not only towards kinetochore function but also centromere establishment should yield some exciting results.
5.2.2 Other Likely CENP-T Pathway Dominant Kinetochores:
5.2.2.1 H. sapiens
In human cell lines, CENP-C and CENP-T are essential for cell viability and are independently capable of recruiting the outer kinetochore to an ectopic locus (Gascoigne et al., 2011; Rago et al., 2015; Musacchio and Desai, 2017). Over-expression of N-terminal outer kinetochore interacting motif of CENP-C in CENP-C-deficient HeLa cells disrupted outer kinetochore assembly (Screpanti et al., 2011). While it is possible that the CENP-C pathway plays a crucial role at the kinetochore, more recent evidence points to the kinetochore in human cells as being CENP-T biased. CENP-T has been shown to recruit twice as many Ndc80C at native kinetochores in comparison to the more abundant CENP-C (Suzuki et al., 2015). Additionally, CENP-T “stretching,” an indicator of propagating spindle forces is reported during M-phase (Uchida et al., 2021). While, a majority of the CENP-C N-terminal region was found to remain within CENP-A chromatin, likely unbound to the outer kinetochore (Suzuki et al., 2014). Further, alleviating Dsn1 autoinhibition was shown to be required for effective recruitment of the Mis12C to CENP-C (Kim and Yu, 2015; Rago et al., 2015). This scenario is comparable to S. cerevisiae and chicken where the N-terminal domain of CENP-C is dispensable. Additionally, in the human system, CENP-Q-U has been shown to be incapable of interacting with the Mis12C (Pesenti et al., 2018). Although CENP-C has a greater influence over CENP-T localization in comparison to chicken cells (Watanabe et al., 2019), it is likely a feature independent of its linker function. Considered together we speculate that it is probable that human kinetochore is biased towards the CENP-T linker pathway (Figure 3).
5.2.2.2 Xenopus leavis
In egg extracts of the African clawed frog, X. leavis, CENP-C is capable of binding to CENP-A to mediate the recruitment of the Mis12C. Conserved is the feature where the outer kinetochore assembly on CENP-C was entirely dependent on phosphorylation of Dsn1 by Aurora B (Bonner et al., 2019). Although CENP-C partially influences CENP-T recruitment, both Ndc80C and Mis12C are localized at kinetochores, upon CENP-C depletion. Owing to the influence of the Dsn1 autoinhibitory motif on CENP-C recruitment and retention of outer kinetochore components upon CENP-C depletion, we favor a CENP-T biased kinetochore in X. leavis although in vivo experiments are required to conclude the same.
5.3 CENP-QOkp1-UAme1-Pathway Dominant Kinetochores
CENP-QOkp1-UAme1 dominant kinetochores have been exclusively described in the point-centromere containing budding yeast of S. cerevisiae and are likely to exist in closely related species. Although the entire suit of known linker components including CENP-TCnn1 and CENP-CMif2 has been described in budding yeast, CENP-TCnn1-null cells are viable without strong chromosome segregation phenotype and loss of the CENP-CMif2 N-terminus was shown to be well-tolerated only causing a minor growth defect in the presence of the microtubule poison benomyl (Cohen et al., 2008; Bock et al., 2012; Hornung et al., 2014). On the other hand deletions of either the Mis12CMIND interacting motif on CENP-UAme1 or the CENP-ACse4 interacting domain on CENP-QOkp1 were found to be essential for viability (Hornung et al., 2014; Fischböck-Halwachs et al., 2019). In vitro reconstitution studies reveals that CENP-QOkp1UAme1 can transmit mitotic relevant forces from the Mis12CMIND to the centromeric nucleosome, making the CENP-ACse4-CENP-QOkp1-UAme1-Mis12CMIND the functional module of this pathway (Figure 3) (Hamilton et al., 2020).
It is suggested that CENP-Q-U originated through duplication of Mis12C components early in eukaryotic evolution. Although the CENP-Q-U is conserved in vertebrates as part of the larger CENP-O-P-Q-U-R complex, it does not function as a linker (Tromer et al., 2019). While CENP-U has been shown to be essential during mouse embryogenesis and in embryonic stem (ES) cells, it is found to be dispensable for mouse fibroblast cells and in chicken DT40 cells (Okada et al., 2006; Hori et al., 2008b; Kagawa et al., 2014). No interaction of this complex with the Mis12C has been reported (Hornung et al., 2014; Pesenti et al., 2018). Thus, the essentiality of CENP-U in development and ES cells might reflect functions other than a linker role. Indeed, vertebrate CENP-Q has been attributed to have microtubule-binding activities (Amaro et al., 2010; Pesenti et al., 2018), and has also been shown to recruit the mitotic kinesin CENP-E (Bancroft et al., 2015). Further, CENP-U primed by Cdk1 is one of the main Plk1 receptor sites at the kinetochore (Kang et al., 2011; Chen et al., 2021; Nguyen et al., 2021; Singh et al., 2021).
Thus, unlike other CCAN subcomplexes, CENP-Q-U seems to have undergone drastic functional remodeling at the kinetochore. This is further exhibited in its kinetochore interdependencies, where at the vertebrate kinetochore the CENP-Q-U complex is downstream of most CCAN components except for CENP-R, including the CENP-H-I-K and CENP-T-W-S-X complexes (Hori et al., 2008b), while in budding yeast all but CENP-CMif2 depend on it (McKinley et al., 2015; Pekgöz Altunkaya et al., 2016). Taken together this makes CENP-QOkp1-UAme1 based kinetochores a unique feature of point-centromere based kinetochores.
5.4 Some Notable Kinetochores With Undefined Linker Pathways
5.4.1 S. pombe (Supergroup: Amorphea, Group: Opisthokonta)
In the fission yeast model system, homologs for CENP-TCnp20, CENP-CCnp3, and CENP-QFta7-UMis17 have been identified, in addition to a full-complement of known inner and other kinetochore proteins similar to budding yeast. In a surprise turn of events, CENP-CCnp3-null cells are viable, and observed chromosome segregation defects were largely suppressed by CENP-LFta1 over-expression (Tanaka et al., 2009). This suggests that the primary role of CENP-CCnp3 is in recruiting CENP-LFta1 to the kinetochore, although it was recently reported to function as a receptor of the Mis12C (Zhou et al., 2017). CENP-TCnp20 and CENP-QFta7-UMis17 are essential for viability (Kim et al., 2010; Tanaka et al., 2009; Hayles et al., 2013). Yet surprisingly, temperature-sensitive mutants of either CENP-TCnp20 or CENP-CCnp3 did not significantly reduce the levels of the Ndc80C at the kinetochore (Tanaka et al., 2009). Further, IP-MS of CENP-UMis17 failed to abundantly identify components of the KMN network (Shiroiwa et al., 2011). Interestingly, in S. pombe, a Nuf2 temperature-sensitive (ts) mutant has been shown to influence the localization of several inner kinetochore components including CENP-KSim4 and CENP-IMis6 (Saitoh et al., 2005), and Mis12 has been described to affect inner centromere structure. One possibility arising from these results may suggest that a redundant dependence upon linker proteins may exist between the equally abundant CENP-TCnp20 and CENP-QFta7-UMis17 at the fission yeast kinetochores (Figure 3) (Virant et al., 2021). Further analysis is required to shed light on the fission yeast kinetochore architecture.
5.4.2 Dictyostelium discoideum (Supergroup Amorphea, Group: Amoebozoa)
Amongst the group Amoebozoa, D. discoideum has been reported to contain a conserved KMN network, CENP-A, and components of the CCAN including CENP-H, -I, -K, -M, -L, -N, -O, -P, -S, and -X. Linker components including CENP-C, -T-W, and -Q-U have not been identified (Hooff et al., 2017; Plowman et al., 2019). Interestingly, a homolog of the recently identified C. neoformans kinetochore component, bridgin, has been found (Figure 3) (Sridhar et al., 2021). The only characterized kinetochore protein, CENP-AH3v1 has been shown to be incorporated into centromeric chromatin (Dubin et al., 2010). Yet, unlike other characterized CENP-A, DdCENP-A does not contain a longer loop1 region which is critical for centromere targeting in other systems (Black and Cleveland, 2011). However, it contains a long N-terminal unstructured region (Dubin et al., 2010), whose function has not been characterized.
5.4.3 Apicomplexans [Supergroup TSAR (Telonemids, Stramenopiles, Alveolates, and Rhizaria), Group: Alveolata]
Apicomplexans along with ciliates and dinoflagellates belong to the Alveolata group. They contain parasitic species which include causative agents of toxoplasmosis and malaria (del Campo et al., 2019). These systems have been reported to harbor CENP-A and a conserved Ndc80C, but little else is known (Hooff et al., 2017; Zeeshan et al., 2020). Recent biochemical analysis however revealed the presence of two distinct CENP-C genes, in addition to the previously described SEA1, in Plasmodium berghei while Toxoplasma gondii contains one, they all being essential for cell proliferation (Bushell et al., 2017; Verma and Surolia, 2014; Zhang et al., 2018; Brusini et al., 2021a). Other CCAN components are likely absent. Interestingly, the Mis12C is likely also lost, while the SkaC is retained (Hooff et al., 2017; Brusini et al., 2021a). Cross-linked IP-MS of kinetochore components revealed the presence of AKiT1-6 sub-complex that likely positions itself between CENP-C and the outer kinetochore, resembling Mis12C and Knl1C positions at the kinetochore. Although AKiT1 depends on CENP-C for its kinetochore localization in T. gondii, neither protein depletion affected Nuf2 levels (Figure 3) (Brusini et al., 2021a). Thus, it is likely that other uncharacterized AKiTs or as yet undefined factors play a role to bridge the Ndc80C onto centromeric chromatin in addition to the redundant CENP-C pathway, highlighting the existence of a possible novel linker module (Figure 3).
5.4.4 Tetrahymena thermophila (Supergroup TSAR, Group: Alveolata)
In the ciliate protozoan system of T. thermophila, only the centromeric determinant CENP-A and microtubule interacting Ndc80 are identified (Figure 3) (Cervantes et al., 2006; Cui and Gorovsky, 2006; Hooff et al., 2017). CENP-ACNA1 was shown to be localized as distinct spots to the peripheral centromeres in the micronucleus but absent in the macronucleus during vegetative growth. While in the meiotic prophase CENP-ACNA1 was observed to localize along the chromosome as puncta followed by a diffused phase as conjugation proceeds and subsequently reverting as distinct puncta. CENP-ACNA1 is described to be essential for accurate chromosome segregation (Cervantes et al., 2006).
5.4.5 Guillardia theta (Supergroup Cryptista, Group: Cryptophytas)
The cryptophytes are a product of secondary endosymbiosis of a red algae and a eukaryotic host. Interestingly, like other cryptophytes, G. theta still harbors the nucleus (nucleomorph) and cytoplasm of their algal endosymbiont. This nucleomorph was shown to contain CENP-A, albeit lacking the extended loop1 region. The presence of a centromere and relict mitotic apparatus is suggested despite evidence lacking for the existence of a spindle (Douglas et al., 2001). The nucleomorph exists in a highly reduced and simplified form. Thus, their nuclear genomes are repositories for thousands of endosymbiont-derived genes. While rather little is known about the kinetochores of Cryptophytes or other members of the Cryptista supergroup, recent bioinformatic analysis of the nuclear genome has identified CENP-C, CENP-I, and CENP-K of the CCAN, most components of the outer kinetochore including components of the SkaC and Dam1C (Figure 3) (Hooff et al., 2017; Plowman et al., 2019). Interestingly, the Dam1C might have been derived from the secondary endosymbiont through horizontal gene transfer (Drinnenberg and Akiyoshi, 2017; Hooff et al., 2017; van Hooff et al., 2017).
5.4.6 Kinetoplastids (Supergroup: Discoba, Group: Euglenozoa)
The kinetoplastids, which comprise a widespread sub-group of flagellated protozoans, surprised the kinetochore research field, with the identification of highly divergent kinetochores present in one of the earliest-branching eukaryotes (Akiyoshi, 2016). Unlike the other systems mentioned above, kinetochore structural components discovered in Trypanosoma brucei and other related kinetoplastids do not resemble any other reported proteins including CENP-A, but for KKIP1, a protein containing similarity to the coiled coils of Nuf2/Ndc80. Yet, chromosome segregation has been shown to rely on the conserved spindle microtubules (Akiyoshi and Gull, 2014; D’Archivio et al., 2017; Ersfeld and Gull, 1997). In contrast, they harbor a unique set of 36 kinetochore components, KKT1-20, KKT22-25, and KKIP1-12 (Akiyoshi and Gull, 2014; Nerusheva et al., 2019; Brusini et al., 2021b), where KKT16-18 were found to be similar to the axial element components of the synaptonemal complex. In addition the kinetochore shares microscopic similarity to the synaptonemal complex. These observations supports the hypothesis that kinetoplastid kinetochores repurposed parts of the meiotic machinery (Tromer et al., 2021).
Within the kinetochore, KKT2 and KKT3 contain a unique zinc-binding domain that is not only critical for their constitutive kinetochore localization but also exhibits weak DNA-binding (Akiyoshi and Gull, 2014; Marcianò et al., 2021). Recent studies further describe KKT4 as a multifunctional protein as it has been reported to be microtubule-binding with an additional DNA binding capability (Akiyoshi and Gull, 2014; Llauró et al., 2018; Ludzia et al., 2021). A recent work from the Wickstead lab shows that KKIP1 bridges the distinct inner and the outer kinetoplastid kinetochore (KOK, KKIP2-4,6,8–12) layers resulting in stretching while under metaphase tension (Figure 3) (Brusini et al., 2021b). Yet, speculating how the kinetochore is anchored in these kinetoplastids is a futile process and we await further insights into this system.
With these emerging results, we are excited and eagerly looking forward to research in this field towards unraveling the mysteries of these and other divergent kinetochores, including how the conserved outer kinetochore is linked to centromeric chromatin.
6 Discussion
In this review, we have highlighted the various strategies employed across eukaryotic evolution to ensure the recruitment and maintenance of the near ubiquitously conserved microtubule-interacting outer kinetochore (Figure 3). Tracing back the composition of the kinetochore in LECA suggests the likely presence of a full complement of known kinetochore proteins (Tromer et al., 2019). However, how was the LECA kinetochore organized? What was its actual composition and architecture? What and which linker pathways dominated? Since no eukaryote or proto-eukaryote that segregates in a LECA or pre-LECA manner has been discovered, addressing these questions is difficult. As simple prokaryotic systems only contain microtubule and centromere binding components, it is suggested that present-day kinetochore proteins, including the CCAN, were added later during eukaryogenesis. Shared ancestry with multiple other eukaryotic processes likely points to their origin sometime between the first eukaryotic common ancestor (FECA) and LECA (Tromer et al., 2019).
Factors such as the centromere drive, changes in spindle attachment dynamics, redundant linker pathways, and gene duplication events could have contributed to the kinetochore diversity observed today, originating from a full-compliment containing LECA kinetochore. Going from available phylogenies, it is needless to say that a lot of plasticity can be accommodated in this functionally conserved structure. The identification of new proto-eukaryotic, archeal or prokaryotic species might help in reconstructing kinetochore evolution.
The CENP-C functional pathway is likely the most conserved across evolution (Hooff et al., 2017; Plowman et al., 2019), yet surprisingly CENP-C-pathway dominant kinetochores reported to date are observed primarily in systems having lost other pathways. It remains a mystery as to what exactly the driving force is at kinetochores towards the maintenance of certain pathways, that in turn define their overall kinetochore architecture. Recent comparative analysis of the CENP-CMif2 and CENP-QOkp1-UAme1 linker modules in budding yeast revealed both as being able to produce similar forces, although the CENP-QOkp1-UAme1 linkage more readily bound microtubules (Hamilton et al., 2020). Linker pathways act as an oligomerization platform for the Ndc80C, where copy numbers of the Ndc80C are greater than their linker counterparts (Joglekar et al., 2008; Suzuki et al., 2015; Dhatchinamoorthy et al., 2017; Cieslinski et al., 2021; Virant et al., 2021). Indeed, it is known that multivalency of the Ndc80C is required for effective tracking and force coupling (Volkov et al., 2018; Takenoshita et al., 2022), although the critical density sufficient for kinetochore functions is unknown. The ability to facilitate the Ndc80C oligomerization may be related to the dominance of one pathway over another. Despite the diverse and active studies in the kinetochore field, there exists limited data not only in the plethora of new but also in certain popular model systems, which needs to be bridged.
Interestingly, a common theme that seems to address some of the observed variations across linker pathways utilized is the role of Dsn1-mediated autoinhibition in regulating the interaction between CENP-C and the Mis12C (Figure 2A). The presence of multiple kinetochore linker pathways, such as direct recruitment of Ndc80C by CENP-T, is one strategy to overcome the reduction in CENP-C-Mis12C interaction (Kim and Yu, 2015; Hara et al., 2018; Lang et al., 2018). Alternatively, as observed in the single linker system of D. melanogaster, containing CENP-C exclusively, the autoinhibitory motif of DmDsn1 can be lost (Przewloka et al., 2009). While in C. neoformans an additional linkage via bridgin may aid in reinforcing the CENP-C pathway (Figure 2D) (Sridhar et al., 2021). Although no such strategy is reported in C. elegans we however believe that the Dsn1 inhibition is compensated for by a large number of attachments across its holocentric chromosomes. This is in line with CENP-C tethering assays across a large repetitive locus where CENP-C is shown to be sufficient to attract a functional outer kinetochore (Gascoigne et al., 2011; Hori et al., 2013; Rago et al., 2015), yet insufficient at the native kinetochore (Hara et al., 2018). Unfortunately, evidence is lacking as to why this regulation is conserved in Opisthokonts. Further validation and testing in real-time the influence of Dsn1 autoinhibition and Aurora B phosphorylation on the transmission of spindle forces across the CENP-C-Mis12C contact during the M-phase progression would be valuable. The requirement of this regulation to prevent undue connections at an ectopic loci is blurred by the recent discovery of intra-CENP-CMif2 regulation. This preventing the recruitment of the outer kinetochore onto a CENP-CMif2 platform in the absence of bound CENP-ACse4 in S. cerevisiae (Killinger et al., 2020). Yet, given the studies across opisthokont model systems (Figure 3), the Dsn1-mediated autoinhibitory drive offers the best explanation for kinetochore architectures based on specific linker pathways. Conservation of the Aurora B phosphorylation-dependent CENP-C-Mis12C interaction is largely seen across eukaryotes, except for Excavates and Apicomplexans. Thus, this autoinhibitory-based regulation may well exist outside Opisthokonts (Plowman et al., 2019). It will be interesting to see if this hypothesis holds following future studies.
The presence of redundant including lineage-specific pathways, may allow for the loss of one or more of the linkages, as observed in basidiomycetous fungi (Sridhar et al., 2021). Once pathways known to exist in LECA are lost, the evolution of novel strategies is likely to arise as dictated by as yet unknown selective pressures during subsequent evolution (Akiyoshi, 2016; Cortes-Silva et al., 2020; Brusini et al., 2021a; Sridhar et al., 2021). Alternatively, as mentioned in D. melanogaster, the kinetochore might balance compositional changes by altering the autoinhibitory regulation as observed with the DmDsn1 losing its auto-inhibitory motif.
Further insights into the functioning of well-studied pathways across systems may be obtained by evaluvating outlying phenotypes, such as how CENP-T performs its function in the absence of a CENP-W homolog in B. mori (Cortes-Silva et al., 2020), or the observation that CENP-T and -W might have variable temporal dynamics in Xenopus egg extracts (Krizaic et al., 2015). Additionally, evaluating how CENP-S-X loss phenocopies chromosome segregation defects of other kinetochore components without exhibiting centromere enrichment in the moss system P. patens (Kozgunova et al., 2019), may highlight additional adaptations of kinetochore sub-complexes.
Interestingly, the N-terminal region of CENP-C containing the outer kinetochore binding domain is highly conserved across various systems even where it is not the dominant linker pathway (Petrovic et al., 2016; Hara et al., 2018). Similarly, kinetochores often harbor multiple redundant pathways, although a dominant pathway is known. (Bock et al., 2012; Schleiffer et al., 2012; Sridhar et al., 2021). So why the apparent paradox? One possible explanation is that the conditions in which the “dispensable” pathways are essential have not been found. For example, the CENP-C pathway might be essential in specific developmental or differentiated stages in multicellular organisms, or under specific environmental growth conditions. These could include temperature or the presence of naturally occurring microtubule modulators in the unicellular environment which might affect spindle stability. Indeed, a large number of microtubule modulators have been identified from natural sources (Stanton et al., 2011). Supporting this possibility is the observation that the loss of redundant pathways even if they non-essential for viability, are important for accurate chromosome segregation under conditions of spindle stress (Bock et al., 2012; Schleiffer et al., 2012; Hornung et al., 2014; Sridhar et al., 2021). Thus screening of kinetochore determinants under more natural conditions may address these contradictions.
With the biochemical validation of a large number of kinetochore homologs, the variation particularly amongst CCAN components is evident. It is noteworthy that recent computational predictions have been rather robust in predicting the loss or retention of known kinetochore homologs, a testament to the improvements in computational methodologies but also that of genomics (Schleiffer et al., 2012; Hooff et al., 2017; Plowman et al., 2019; Tromer et al., 2019). However, going by recent studies, robust biochemical analysis of systems is key in identifying novel kinetochore candidates that cannot be identified computationally (Akiyoshi and Gull, 2014; Cortes-Silva et al., 2020; Brusini et al., 2021a; Sridhar et al., 2021).
While we have focused on the linker components of the CCAN in this study, several other kinetochore components also exhibit evolutionary lability and may play a role in influencing CCAN architecture or linker pathway function, such as CENP-R and its functional analog Nkp1-Nkp2 in budding yeasts, the small GTPase-like protein CENP-M (Basilico et al., 2014) which is essential in vertebrates yet lost in fungi, the role of outer kinetochore accessory proteins of the Dam1 and Ska complexes (Cheeseman, 2014), the point-centromere specific CBF3 complex critical for kinetochore nucleation (Biggins, 2013), or the mystical CENP-B, that binds to a specific DNA sequence in mammals (Hoffmann et al., 2016; Musacchio and Desai, 2017).
7 Future Perspective
With the elegantly posed “Ship of Theseus” analogy (Drinnenberg et al., 2016) to describe kinetochore plasticity, going forward it would be exciting to see the compositional diversity this structure can attain across disparate systems. These include not only the kinetochores of Metamonad Carpedirmonas, Diplonemids and Euglenids likely lacking most known kinetochore components, but also the divergent Apicomplexan outer kinetochore and in CENP-A deficient systems of B. mori and M. circinelloides (Butenko et al., 2020; Brusini et al., 2021a; Salas-Leiva et al., 2021; Tromer et al., 2021). In this review, we have highlighted systems from five of the thirteen newly defined eukaryotic supergroups (Burki et al., 2020). However, it should possible to target unique and divergent systems given the availability of rather robust computational prediction tools. In spite of the likely challenges of biological dissection in certain systems, it is an area that may yield fruitful and exciting results. With the abundance of information on the various components in model systems, it would be rewarding to look into conditions of their requirement and plasticity in varying circumstances mimicking the native environment and life cycle.
In addition to the likely identification of novel linker candidates, it is equally likely that known factors may be repurposed. A prime candidate for this would be CENP-I, which has been shown to not only localize closely with the Ndc80C in humans (Suzuki et al., 2014) and directly interact with it in budding yeast, chicken DT40 and human systems (Mikami et al., 2005; Kim and Yu, 2015; Pekgöz Altunkaya et al., 2016), but also to be capable of interacting non-specifically with DNA or reconstituted nucleosomes as part of the CENP-H-I-K-M complex (Weir et al., 2016; Pesenti et al., 2022). Additionally, CENP-I and its fission yeast homolog Mis6 are shown to be required for new CENP-A deposition (Takahashi et al., 2000; Okada et al., 2006). CENP-I has also been described to be able to nucleate the formation of a functional kinetochore when tethered at an ectopic locus (Hori et al., 2013), and more recently the CENP-I homolog in B. mori has been shown to be critical in kinetochore maintenance and recruiting the outer kinetochore (Cortes-Silva et al., 2020).
Thus, the pursuit of understanding how kinetochore plasticity is accommodated towards performing a conserved function is an exciting direction the kinetochore field is working towards.
Author Contributions
SS and TF researched, wrote, and edited the manuscript.
Funding
The work in the Fukagawa lab related to the topic is funded by the grants of CREST of JST (JPMJCR21E6), JSPS KAKENHI Grant Numbers 16H06279, and 17H06167, 20H05389, 21H05752 to TF.
Conflict of Interest
The authors declare that the research was conducted in the absence of any commercial or financial relationships that could be construed as a potential conflict of interest.
Publisher’s Note
All claims expressed in this article are solely those of the authors and do not necessarily represent those of their affiliated organizations, or those of the publisher, the editors and the reviewers. Any product that may be evaluated in this article, or claim that may be made by its manufacturer, is not guaranteed or endorsed by the publisher.
Acknowledgments
The authors thank the members of the Fukagawa lab for their valuable discussions.
References
Abad, M. A., Zou, J., Medina-Pritchard, B., Nigg, E. A., Rappsilber, J., Santamaria, A., et al. (2016). Ska3 Ensures Timely Mitotic Progression by Interacting Directly with Microtubules and Ska1 Microtubule Binding Domain. Sci. Rep. 6, 34042. doi:10.1038/srep34042
Akiyoshi, B., and Gull, K. (2014). Discovery of Unconventional Kinetochores in Kinetoplastids. Cell 156, 1247–1258. doi:10.1016/j.cell.2014.01.049
Akiyoshi, B., Nelson, C. R., and Biggins, S. (2013). The Aurora B Kinase Promotes Inner and Outer Kinetochore Interactions in Budding Yeast. Genetics 194, 785–789. doi:10.1534/genetics.113.150839
Akiyoshi, B., Sarangapani, K. K., Powers, A. F., Nelson, C. R., Reichow, S. L., Arellano-Santoyo, H., et al. (2010). Tension Directly Stabilizes Reconstituted Kinetochore-Microtubule Attachments. Nature 468, 576–579. doi:10.1038/nature09594
Akiyoshi, B. (2016). The Unconventional Kinetoplastid Kinetochore: from Discovery Toward Functional Understanding. Biochem. Soc. Trans. 44, 1201–1217. doi:10.1042/bst20160112
Ali-Ahmad, A., and Sekulić, N. (2020). CENP-A Nucleosome-A Chromatin-Embedded Pedestal for the Centromere: Lessons Learned from Structural Biology. Essays Biochem. 64, 205–221. doi:10.1042/ebc20190074
Amano, M., Suzuki, A., Hori, T., Backer, C., Okawa, K., Cheeseman, I. M., et al. (2009). The CENP-S Complex Is Essential for the Stable Assembly of Outer Kinetochore Structure. J. Cell Biol. 186, 173–182. doi:10.1083/jcb.200903100
Amaro, A. C., Samora, C. P., Holtackers, R., Wang, E., Kingston, I. J., Alonso, M., et al. (2010). Molecular Control of Kinetochore-Microtubule Dynamics and Chromosome Oscillations. Nat. Cell Biol. 12, 319–329. doi:10.1038/ncb2033
Anedchenko, E. A., Samel-Pommerencke, A., Tran Nguyen, T. M., Shahnejat-Bushehri, S., Pöpsel, J., Lauster, D., et al. (2019). The Kinetochore Module Okp1CENP-Q/Ame1CENP-U Is a Reader for N-Terminal Modifications on the Centromeric Histone Cse4CENP-A. EMBO J. 38, e98991. doi:10.15252/embj.201898991
Ariyoshi, M., Makino, F., Watanabe, R., Nakagawa, R., Kato, T., Namba, K., et al. (2021). Cryo-EM Structure of the CENP-A Nucleosome in Complex with Phosphorylated CENP-C. EMBO J. 40, e105671. doi:10.15252/embj.2020105671
Bajaj, R., Bollen, M., Peti, W., and Page, R. (2018). KNL1 Binding to PP1 and Microtubules Is Mutually Exclusive. Structure 26, 1327–1336. doi:10.1016/j.str.2018.06.013
Bancroft, J., Auckland, P., Samora, C. P., and McAinsh, A. D. (2015). Chromosome Congression Is Promoted by CENP-Q- and CENP-E-Dependent Pathways. J. Cell Sci. 128, 171–184. doi:10.1242/jcs.163659
Barth, T. K., Schade, G. O. M., Schmidt, A., Vetter, I., Wirth, M., Heun, P., et al. (2014). Identification of Novel Drosophila Centromere-Associated Proteins. Proteomics 14, 2167–2178. doi:10.1002/pmic.201400052
Basilico, F., Maffini, S., Weir, J. R., Prumbaum, D., Rojas, A. M., Zimniak, T., et al. (2014). The Pseudo GTPase CENP-M Drives Human Kinetochore Assembly. Elife 3, e02978. doi:10.7554/eLife.02978
Biggins, S. (2013). The Composition, Functions, and Regulation of the Budding Yeast Kinetochore. Genetics 194, 817–846. doi:10.1534/genetics.112.145276
Black, B. E., and Cleveland, D. W. (2011). Epigenetic Centromere Propagation and the Nature of CENP-A Nucleosomes. Cell 144, 471–479. doi:10.1016/j.cell.2011.02.002
Bock, L. J., Pagliuca, C., Kobayashi, N., Grove, R. A., Oku, Y., Shrestha, K., et al. (2012). Cnn1 Inhibits the Interactions Between the KMN Complexes of the Yeast Kinetochore. Nat. Cell Biol. 14, 614–624. doi:10.1038/ncb2495
Bonner, M. K., Haase, J., Swinderman, J., Halas, H., Miller Jenkins, L. M., and Kelly, A. E. (2019). Enrichment of Aurora B Kinase at the Inner Kinetochore Controls Outer Kinetochore Assembly. J. Cell Biol. 218, 3237–3257. doi:10.1083/jcb.201901004
Boveri, T. (1888). “Die Befruchtung und Teilung des Eies von Ascaris Megalocephala,” in Zellen-Studien 2. Editor J. G. Fischer. doi:10.5962/bhl.title.6755
Brinkley, B. R., and Stubblefield, E. (1966). The Fine Structure of the Kinetochore of a Mammalian Cell In Vitro. Chromosoma 19, 28–43. doi:10.1007/bf00332792
Brusini, L., D’Archivio, S., McDonald, J., and Wickstead, B. (2021b). Trypanosome KKIP1 Dynamically Links the Inner Kinetochore to a Kinetoplastid Outer Kinetochore Complex. Front. Cell. Infect. Microbiol. 11, 641174. doi:10.3389/fcimb.2021.641174
Brusini, L., Dos, N., Pacheco, S., Soldati-favre, D., and Brochet, M. (2021a). Organization and Composition of Apicomplexan Kinetochores Reveal Plasticity in Chromosome Segregation Across Parasite Modes of Division. BioRxiv. doi:10.1101/2021.11.03.466924
Burki, F., Roger, A. J., Brown, M. W., and Simpson, A. G. B. (2020). The New Tree of Eukaryotes. Trends Ecol. Evol. 35, 43–55. doi:10.1016/j.tree.2019.08.008
Buscaino, A., Allshire, R., and Pidoux, A. (2010). Building Centromeres: Home Sweet Home or a Nomadic Existence? Curr. Opin. Genet. Dev. 20, 118–126. doi:10.1016/j.gde.2010.01.006
Bushell, E., Gomes, A. R., Sanderson, T., Anar, B., Girling, G., Herd, C., et al. (2017). Functional Profiling of a Plasmodium Genome Reveals an Abundance of Essential Genes. Cell 170, 260–272. e8. doi:10.1016/j.cell.2017.06.030
Butenko, A., Opperdoes, F. R., Flegontova, O., Horák, A., Hampl, V., Keeling, P., et al. (2020). Evolution of Metabolic Capabilities and Molecular Features of Diplonemids, Kinetoplastids, and Euglenids. BMC Biol. 18, 23. doi:10.1186/s12915-020-0754-1
Carroll, C. W., Milks, K. J., and Straight, A. F. (2010). Dual Recognition of CENP-A Nucleosomes Is Required for Centromere Assembly. J. Cell Biol. 189, 1143–1155. doi:10.1083/jcb.201001013
Carroll, C. W., Silva, M. C. C., Godek, K. M., Jansen, L. E. T., and Straight, A. F. (2009). Centromere Assembly Requires the Direct Recognition of CENP-A Nucleosomes by CENP-N. Nat. Cell Biol. 11, 896–902. doi:10.1038/ncb1899
Cervantes, M. D., Xi, X., Vermaak, D., Yao, M.-C., and Malik, H. S. (2006). The CNA1 Histone of the Ciliate Tetrahymena thermophila is Essential for Chromosome Segregation in the Germline Micronucleus. MBoC 17, 485–497. doi:10.1091/mbc.e05-07-0698
Cheeseman, I. M., Anderson, S., Jwa, M., Green, E. M., Kang, J.-s., Yates, J. R., et al. (2002). Phospho-Regulation of Kinetochore-Microtubule Attachments by the Aurora Kinase Ipl1p. Cell 111, 163–172. doi:10.1016/s0092-8674(02)00973-x
Cheeseman, I. M., Chappie, J. S., Wilson-Kubalek, E. M., and Desai, A. (2006). The Conserved KMN Network Constitutes the Core Microtubule-Binding Site of the Kinetochore. Cell 127, 983–997. doi:10.1016/j.cell.2006.09.039
Cheeseman, I. M., and Desai, A. (2008). Molecular Architecture of the Kinetochore-Microtubule Interface. Nat. Rev. Mol. Cell Biol. 9, 33–46. doi:10.1038/nrm2310
Cheeseman, I. M., Niessen, S., Anderson, S., Hyndman, F., Yates, J. R., Oegema, K., et al. (2004). A Conserved Protein Network Controls Assembly of the Outer Kinetochore and its Ability to Sustain Tension. Genes Dev. 18, 2255–2268. doi:10.1101/gad.1234104
Cheeseman, I. M. (2014). The Kinetochore. Cold Spring Harb. Perspect. Biol. 6, a015826. doi:10.1101/cshperspect.a015826
Chen, Q., Zhang, M., Pan, X., Yuan, X., Zhou, L., Yan, L., et al. (2021). Bub1 and CENP-U Redundantly Recruit Plk1 to Stabilize Kinetochore-Microtubule Attachments and Ensure Accurate Chromosome Segregation. Cell Rep. 36, 109740. doi:10.1016/j.celrep.2021.109740
Cieslinski, K., Wu, Y., Nechyporenko, L., Hörner, S. J., and Conti, D. (2021). Nanoscale Structural Organization and Stoichiometry of the Budding Yeast Kinetochore. BioArxiv. doi:10.1101/2021.12.01.469648
Ciferri, C., Pasqualato, S., Screpanti, E., Varetti, G., Santaguida, S., Dos Reis, G., et al. (2008). Implications for Kinetochore-Microtubule Attachment from the Structure of an Engineered Ndc80 Complex. Cell 133, 427–439. doi:10.1016/j.cell.2008.03.020
Clarke, L., and Carbon, J. (1980). Isolation of a Yeast Centromere and Construction of Functional Small Circular Chromosomes. Nature 287, 504–509. doi:10.1038/287504a0
Cohen, R. L., Espelin, C. W., De Wulf, P., Sorger, P. K., Harrison, S. C., and Simons, K. T. (2008). Structural and Functional Dissection of Mif2p, a Conserved DNA-Binding Kinetochore Protein. MBoC 19, 4480–4491. doi:10.1091/mbc.e08-03-0297
Cortes-Silva, N., Ulmer, J., Kiuchi, T., Hsieh, E., Cornilleau, G., Ladid, I., et al. (2020). CenH3-Independent Kinetochore Assembly in Lepidoptera Requires CCAN, Including CENP-T. Curr. Biol. 30, 561–572. doi:10.1016/j.cub.2019.12.014
Cui, B., and Gorovsky, M. A. (2006). Centromeric Histone H3 Is Essential for Vegetative Cell Division and for DNA Elimination During Conjugation in Tetrahymena Thermophila. Mol. Cell. Biol. 26, 4499–4510. doi:10.1128/mcb.00079-06
Cuylen, S., Blaukopf, C., Politi, A. Z., Müller-Reichert, T., Neumann, B., Poser, I., et al. (2016). Ki-67 Acts as a Biological Surfactant to Disperse Mitotic Chromosomes. Nature 535, 308–312. doi:10.1038/nature18610
D’Archivio, S., Wickstead, B., D’Archivio, S., and Wickstead, B. (2017). Trypanosome Outer Kinetochore Proteins Suggest Conservation of Chromosome Segregation Machinery Across Eukaryotes. J. Cell Biol. 216, 379–391.
Dawe, R. K., Reed, L. M., Yu, H.-G., Muszynski, M. G., and Hiatt, E. N. (1999). A Maize Homolog of Mammalian CENPC Is a Constitutive Component of the Inner Kinetochore. Plant Cell 11, 1227–1238. doi:10.1105/tpc.11.7.1227
De Rop, V., Padeganeh, A., and Maddox, P. S. (2012). CENP-A: The Key Player Behind Centromere Identity, Propagation, and Kinetochore Assembly. Chromosoma 121, 527–538. doi:10.1007/s00412-012-0386-5
De Wulf, P., McAinsh, A. D., and Sorger, P. K. (2003). Hierarchical Assembly of the Budding Yeast Kinetochore from Multiple Subcomplexes. Genes Dev. 17, 2902–2921. doi:10.1101/gad.1144403
del Campo, J., Heger, T. J., Rodríguez-Martínez, R., Worden, A. Z., Richards, T. A., Massana, R., et al. (2019). Assessing the Diversity and Distribution of Apicomplexans in Host and Free-Living Environments Using High-Throughput Amplicon Data and a Phylogenetically Informed Reference Framework. Front. Microbiol. 10, 2373. doi:10.3389/fmicb.2019.02373
DeLuca, J. G., Gall, W. E., Ciferri, C., Cimini, D., Musacchio, A., and Salmon, E. D. (2006). Kinetochore Microtubule Dynamics and Attachment Stability Are Regulated by Hec1. Cell 127, 969–982. doi:10.1016/j.cell.2006.09.047
Desai, A., Rybina, S., Müller-Reichert, T., Shevchenko, A., Shevchenko, A., Hyman, A., et al. (2003). KNL-1 Directs Assembly of the Microtubule-Binding Interface of the Kinetochore in C. elegans. Genes Dev. 17, 2421–2435. doi:10.1101/gad.1126303
Dhatchinamoorthy, K., Shivaraju, M., Lange, J. J., Rubinstein, B., Unruh, J. R., Slaughter, B. D., et al. (2017). Structural Plasticity of the Living Kinetochore. J. Cell Biol. 216, 3551–3570. doi:10.1083/jcb.201703152
Dimitrova, Y. N., Jenni, S., Valverde, R., Khin, Y., and Harrison, S. C. (2016). Structure of the MIND Complex Defines a Regulatory Focus for Yeast Kinetochore Assembly. Cell 167, 1014–1027. e12. doi:10.1016/j.cell.2016.10.011
Douglas, S., Zauner, S., Fraunholz, M., Beaton, M., Penny, S., Deng, L.-T., et al. (2001). The Highly Reduced Genome of an Enslaved Algal Nucleus. Nature 410, 1091–1096. doi:10.1038/35074092
Drinnenberg, I. A., and Akiyoshi, B. (2017). Evolutionary Lessons from Species with Unique Kinetochores. Prog. Mol. Subcell. Biol. 56, 111–138. doi:10.1007/978-3-319-58592-5_5
Drinnenberg, I. A., Henikoff, S., and Malik, H. S. (2016). Evolutionary Turnover of Kinetochore Proteins: A Ship of Theseus? Trends Cell Biol. 26, 498–510. doi:10.1016/j.tcb.2016.01.005
Dubin, M., Fuchs, J., Gräf, R., Schubert, I., and Nellen, W. (2010). Dynamics of a Novel Centromeric Histone Variant CenH3 Reveals the Evolutionary Ancestral Timing of Centromere Biogenesis. Nucleic Acids Res. 38, 7526–7537. doi:10.1093/nar/gkq664
Earnshaw, W. C., and Rothfield, N. (1985). Identification of a Family of Human Centromere Proteins Using Autoimmune Sera from Patients with Scleroderma. Chromosoma 91, 313–321. doi:10.1007/bf00328227
Emanuele, M. J., Lan, W., Jwa, M., Miller, S. A., Chan, C. S. M., and Stukenberg, P. T. (2008). Aurora B Kinase and Protein Phosphatase 1 Have Opposing Roles in Modulating Kinetochore Assembly. J. Cell Biol. 181, 241–254. doi:10.1083/jcb.200710019
Ersfeld, K., and Gull, K. (1997). Partitioning of Large and Minichromosomes in Trypanosoma Brucei. Science 276, 611–614. doi:10.1126/science.276.5312.611
Espeut, J., Cheerambathur, D. K., Krenning, L., Oegema, K., and Desai, A. (2012). Microtubule Binding by KNL-1 Contributes to Spindle Checkpoint Silencing at the Kinetochore. J. Cell Biol. 196, 469–482. doi:10.1083/jcb.201111107
Fischböck-Halwachs, J., Singh, S., Potocnjak, M., Hagemann, G., Solis-Mezarino, V., Woike, S., et al. (2019). The COMA Complex Interacts with Cse4 and Positions Sli15/Ipl1 at the Budding Yeast Inner Kinetochore. Elife 8, e42879. doi:10.7554/eLife.42879
Folco, H. D., Campbell, C. S., May, K. M., Espinoza, C. A., Oegema, K., Hardwick, K. G., et al. (2015). The CENP-A N-Tail Confers Epigenetic Stability to Centromeres via the CENP-T Branch of the CCAN in Fission Yeast. Curr. Biol. 25, 348–356. doi:10.1016/j.cub.2014.11.060
Foley, E. A., and Kapoor, T. M. (2013). Microtubule Attachment and Spindle Assembly Checkpoint Signalling at the Kinetochore. Nat. Rev. Mol. Cell Biol. 14, 25–37. doi:10.1038/nrm3494
Foltz, D. R., Jansen, L. E. T., Black, B. E., Bailey, A. O., Yates, J. R., and Cleveland, D. W. (2006). The Human CENP-A Centromeric Nucleosome-Associated Complex. Nat. Cell Biol. 8, 458–469. doi:10.1038/ncb1397
Fukagawa, T., and Brown, W. R. A. (1997). Efficient Conditional Mutation of the Vertebrate CENP-C Gene. Hum. Mol. Genet. 6, 2301–2308. doi:10.1093/hmg/6.13.2301
Fukagawa, T., and Earnshaw, W. C. (2014). The Centromere: Chromatin Foundation for the Kinetochore Machinery. Dev. Cell 30, 496–508. doi:10.1016/j.devcel.2014.08.016
Furuyama, T., and Henikoff, S. (2009). Centromeric Nucleosomes Induce Positive DNA Supercoils. Cell 138, 104–113. doi:10.1016/j.cell.2009.04.049
Gascoigne, K. E., Takeuchi, K., Suzuki, A., Hori, T., Fukagawa, T., and Cheeseman, I. M. (2011). Induced Ectopic Kinetochore Assembly Bypasses the Requirement for CENP-A Nucleosomes. Cell 145, 410–422. doi:10.1016/j.cell.2011.03.031
Goshima, G., Wollman, R., Goodwin, S. S., Zhang, N., Scholey, J. M., Vale, R. D., et al. (2007). Genes Required for Mitotic Spindle Assembly in Drosophila S2 Cells. Science 316, 417–421. doi:10.1126/science.1141314
Guimaraes, G. J., Dong, Y., McEwen, B. F., and DeLuca, J. G. (2008). Kinetochore-Microtubule Attachment Relies on the Disordered N-Terminal Tail Domain of Hec1. Curr. Biol. 18, 1778–1784. doi:10.1016/j.cub.2008.08.012
Guin, K., Sreekumar, L., and Sanyal, K. (2020). Implications of the Evolutionary Trajectory of Centromeres in the Fungal Kingdom. Annu. Rev. Microbiol. 74, 835–853. doi:10.1146/annurev-micro-011720-122512
Guo, L. Y., Allu, P. K., Zandarashvili, L., McKinley, K. L., Sekulic, N., Dawicki-McKenna, J. M., et al. (2017). Centromeres Are Maintained by Fastening CENP-A to DNA and Directing an Arginine Anchor-Dependent Nucleosome Transition. Nat. Commun. 8, 15775. doi:10.1038/ncomms15775
Hamilton, G. E., Helgeson, L. A., Noland, C. L., Asbury, C. L., Dimitrova, Y. N., and Davis, T. N. (2020). Reconstitution Reveals Two Paths of Force Transmission Through the Kinetochore. Elife 9, e56582. doi:10.7554/eLife.56582
Hara, M., Ariyoshi, M., Okumura, E.-i., Hori, T., and Fukagawa, T. (2018). Multiple Phosphorylations Control Recruitment of the KMN Network onto Kinetochores. Nat. Cell Biol. 20, 1378–1388. doi:10.1038/s41556-018-0230-0
Hara, M., and Fukagawa, T. (2017). “Critical Foundation of the Kinetochore: The Constitutive Centromere-Associated Network (CCAN),” in Progress in Molecular and Subcellular Biology, (Progress in Molecular and Subcellular Biology), 29–57. doi:10.1007/978-3-319-58592-5_2
Hara, M., and Fukagawa, T. (2019). Where Is the Right Path Heading from the Centromere to Spindle Microtubules? Cell Cycle 18, 1199–1211. doi:10.1080/15384101.2019.1617008
Hayles, J., Wood, V., Jeffery, L., Hoe, K.-L., Kim, D.-U., Park, H.-O., et al. (2013). A Genome-Wide Resource of Cell Cycle and Cell Shape Genes of Fission Yeast. Open Biol. 3, 130053. doi:10.1098/rsob.130053
Heeger, S., Leismann, O., Schittenhelm, R., Schraidt, O., Heidmann, S., and Lehner, C. F. (2005). Genetic Interactions of Separase Regulatory Subunits Reveal the Diverged Drosophila Cenp-C Homolog. Genes Dev. 19, 2041–2053. doi:10.1101/gad.347805
Hemmerich, P., Weidtkamp-Peters, S., Hoischen, C., Schmiedeberg, L., Erliandri, I., and Diekmann, S. (2008). Dynamics of Inner Kinetochore Assembly and Maintenance in Living Cells. J. Cell Biol. 180, 1101–1114. doi:10.1083/jcb.200710052
Henikoff, S., Ahmad, K., and Malik, H. S. (2001). The Centromere Paradox: Stable Inheritance with Rapidly Evolving DNA. Science 293, 1098–1102. doi:10.1126/science.1062939
Hinshaw, S. M., and Harrison, S. C. (2019). The Structure of the Ctf19c/CCAN from Budding Yeast. Elife 8, e44239. doi:10.7554/eLife.44239
Hinshaw, S. M., and Harrison, S. C. (2020). The Structural Basis for Kinetochore Stabilization by Cnn1/CENP-T. Curr. Biol. 30, 3425–3431. e3. doi:10.1016/j.cub.2020.06.024
Hoffmann, S., Dumont, M., Barra, V., Ly, P., Nechemia-Arbely, Y., McMahon, M. A., et al. (2016). CENP-A Is Dispensable for Mitotic Centromere Function After Initial Centromere/Kinetochore Assembly. Cell Rep. 17, 2394–2404. doi:10.1016/j.celrep.2016.10.084
Hooff, J. J., Tromer, E., Wijk, L. M., Snel, B., and Kops, G. J. (2017). Evolutionary Dynamics of the Kinetochore Network in Eukaryotes as Revealed by Comparative Genomics. EMBO Rep. 18, 1559–1571. doi:10.15252/embr.201744102
Hori, T., Amano, M., Suzuki, A., Backer, C. B., Welburn, J. P., Dong, Y., et al. (2008a). CCAN Makes Multiple Contacts with Centromeric DNA to Provide Distinct Pathways to the Outer Kinetochore. Cell 135, 1039–1052. doi:10.1016/j.cell.2008.10.019
Hori, T., Okada, M., Maenaka, K., and Fukagawa, T. (2008b). CENP-O Class Proteins Form a Stable Complex and Are Required for Proper Kinetochore Function. MBoC 19, 843–854. doi:10.1091/mbc.e07-06-0556
Hori, T., Shang, W.-H., Takeuchi, K., and Fukagawa, T. (2013). The CCAN Recruits CENP-A to the Centromere and Forms the Structural Core for Kinetochore Assembly. J. Cell Biol. 200, 45–60. doi:10.1083/jcb.201210106
Hornung, P., Troc, P., Malvezzi, F., Maier, M., Demianova, Z., Zimniak, T., et al. (2014). A Cooperative Mechanism Drives Budding Yeast Kinetochore Assembly Downstream of CENP-A. J. Cell Biol. 206, 509–524. doi:10.1083/jcb.201403081
Huis In 't Veld, P. J., Jeganathan, S., Petrovic, A., Singh, P., John, J., Krenn, V., et al. (2016). Molecular Basis of Outer Kinetochore Assembly on CENP-T. Elife 5, e21007. doi:10.7554/eLife.21007
Hyland, K. M., Kingsbury, J., Koshland, D., and Hieter, P. (1999). Ctf19p: A Novel Kinetochore Protein in Saccharomyces cerevisiae and a Potential Link Between the Kinetochore and Mitotic Spindle. J. Cell Biol. 145, 15–28. doi:10.1083/jcb.145.1.15
Ishii, M., and Akiyoshi, B. (2022). Plasticity in Centromere Organization and Kinetochore Composition: Lessons from Diversity. Curr. Opin. Cell Biol. 74, 47–54. doi:10.1016/j.ceb.2021.12.007
Izuta, H., Ikeno, M., Suzuki, N., Tomonaga, T., Nozaki, N., Obuse, C., et al. (2006). Comprehensive Analysis of the ICEN (Interphase Centromere Complex) Components Enriched in the CENP-A Chromatin of Human Cells. Genes Cells 11, 673–684. doi:10.1111/j.1365-2443.2006.00969.x
Jenni, S., and Harrison, S. C. (2018). Structure of the DASH/Dam1 Complex Shows its Role at the Yeast Kinetochore-Microtubule Interface. Science 360, 552–558. doi:10.1126/science.aar6436
Jeyaprakash, A. A., Santamaria, A., Jayachandran, U., Chan, Y. W., Benda, C., Nigg, E. A., et al. (2012). Structural and Functional Organization of the Ska Complex, a Key Component of the Kinetochore-Microtubule Interface. Mol. Cell 46, 274–286. doi:10.1016/j.molcel.2012.03.005
Joglekar, A. (2016). A Cell Biological Perspective on Past, Present and Future Investigations of the Spindle Assembly Checkpoint. Biology 5, 44. doi:10.3390/biology5040044
Joglekar, A. P., Bouck, D., Finley, K., Liu, X., Wan, Y., Berman, J., et al. (2008). Molecular Architecture of the Kinetochore-Microtubule Attachment Site Is Conserved Between Point and Regional Centromeres. J. Cell Biol. 181, 587–594. doi:10.1083/jcb.200803027
Joglekar, A. P., and Kukreja, A. A. (2017). How Kinetochore Architecture Shapes the Mechanisms of its Function. Curr. Biol. 27, R816–R824. doi:10.1016/j.cub.2017.06.012
Kagawa, N., Hori, T., Hoki, Y., Hosoya, O., Tsutsui, K., Saga, Y., et al. (2014). The CENP-O Complex Requirement Varies Among Different Cell Types. Chromosome Res. 22, 293–303. doi:10.1007/s10577-014-9404-1
Kalitsis, P., Fowler, K. J., Earle, E., Hill, J., and Choo, K. H. A. (1998). Targeted Disruption of Mouse Centromere Protein C Gene Leads to Mitotic Disarray and Early Embryo Death. Proc. Natl. Acad. Sci. U.S.A. 95, 1136–1141. doi:10.1073/pnas.95.3.1136
Kang, Y. H., Park, C. H., Kim, T.-S., Soung, N.-K., Bang, J. K., Kim, B. Y., et al. (2011). Mammalian Polo-Like Kinase 1-Dependent Regulation of the PBIP1-CENP-Q Complex at Kinetochores. J. Biol. Chem. 286, 19744–19757. doi:10.1074/jbc.m111.224105
Kato, H., Jiang, J., Zhou, B.-R., Rozendaal, M., Feng, H., Ghirlando, R., et al. (2013). A Conserved Mechanism for Centromeric Nucleosome Recognition by Centromere Protein CENP-C. Science 340, 1110–1113. doi:10.1126/science.1235532
Kettenbach, A. N., Schweppe, D. K., Faherty, B. K., Pechenick, D., Pletnev, A. A., and Gerber, S. A. (2011). Quantitative Phosphoproteomics Identifies Substrates and Functional Modules of Aurora and Polo-Like Kinase Activities in Mitotic Cells. Sci. Signal. 4, rs5. doi:10.1126/scisignal.2001497
Killinger, K., Böhm, M., Steinbach, P., Hagemann, G., Blüggel, M., Jänen, K., et al. (2020). Auto-Inhibition of Mif2/CENP-C Ensures Centromere-Dependent Kinetochore Assembly in Budding Yeast. EMBO J. 39, e102938. doi:10.15252/embj.2019102938
Kim, D.-U., Hayles, J., Kim, D., Wood, V., Park, H.-O., Won, M., et al. (2010). Analysis of a Genome-Wide Set of Gene Deletions in the Fission Yeast Schizosaccharomyces pombe. Nat. Biotechnol. 28, 617–623. doi:10.1038/nbt.1628
Kim, S., and Yu, H. (2015). Multiple Assembly Mechanisms Anchor the KMN Spindle Checkpoint Platform at Human Mitotic Kinetochores. J. Cell Biol. 208, 181–196. doi:10.1083/jcb.201407074
Kiyomitsu, T., Obuse, C., and Yanagida, M. (2007). Human Blinkin/AF15q14 Is Required for Chromosome Alignment and the Mitotic Checkpoint through Direct Interaction with Bub1 and BubR1. Dev. Cell 13, 663–676. doi:10.1016/j.devcel.2007.09.005
Klare, K., Weir, J. R., Basilico, F., Zimniak, T., Massimiliano, L., Ludwigs, N., et al. (2015). CENP-C Is a Blueprint for Constitutive Centromere-Associated Network Assembly within Human Kinetochores. J. Cell Biol. 210, 11–22. doi:10.1083/jcb.201412028
Kozgunova, E., Nishina, M., and Goshima, G. (2019). Kinetochore Protein Depletion Underlies Cytokinesis Failure and Somatic Polyploidization in the Moss Physcomitrella Patens. Elife 8, e43652. doi:10.7554/eLife.43652
Krizaic, I., Williams, S. J., Sánchez, P., Rodríguez-Corsino, M., Stukenberg, P. T., and Losada, A. (2015). The Distinct Functions of CENP-C and CENP-T/W in Centromere Propagation and Function in Xenopusegg Extracts. Nucleus 6, 133–143. doi:10.1080/19491034.2014.1003509
Lampert, F., and Westermann, S. (2011). A Blueprint for Kinetochores - New Insights into the Molecular Mechanics of Cell Division. Nat. Rev. Mol. Cell Biol. 12, 407–412. doi:10.1038/nrm3133
Lang, J., Barber, A., and Biggins, S. (2018). An Assay for De Novo Kinetochore Assembly Reveals a Key Role for the CENP-T Pathway in Budding Yeast. Elife 7, e37819. doi:10.7554/eLife.37819
Lara-Gonzalez, P., Pines, J., and Desai, A. (2021). Spindle Assembly Checkpoint Activation and Silencing at Kinetochores. Seminars Cell & Dev. Biol. 117, 86–98. doi:10.1016/j.semcdb.2021.06.009
Liebelt, F., Jansen, N. S., Kumar, S., Gracheva, E., Claessens, L. A., Verlaan-de Vries, M., et al. (2019). The Poly-Sumo2/3 Protease SENP6 Enables Assembly of the Constitutive Centromere-Associated Network by Group deSUMOylation. Nat. Commun. 10, 3987. doi:10.1038/s41467-019-11773-x
Liu, Y., Petrovic, A., Rombaut, P., Mosalaganti, S., Keller, J., Raunser, S., et al. (2016). Insights from the Reconstitution of the Divergent Outer Kinetochore of Drosophila melanogaster. Open Biol. 6, 150236. doi:10.1098/rsob.150236
Llauró, A., Hayashi, H., Bailey, M. E., Wilson, A., Ludzia, P., Asbury, C. L., et al. (2018). The Kinetoplastid Kinetochore Protein KKT4 Is an Unconventional Microtubule Tip-Coupling Protein. J. Cell Biol. 217, 3886–3900. doi:10.1083/jcb.201711181
Logsdon, G. A., Barrey, E. J., Bassett, E. A., DeNizio, J. E., Guo, L. Y., Panchenko, T., et al. (2015). Both Tails and the Centromere Targeting Domain of CENP-A Are Required for Centromere Establishment. J. Cell Biol. 208, 521–531. doi:10.1083/jcb.201412011
Ludzia, P., Lowe, E. D., Marcianò, G., Mohammed, S., Redfield, C., and Akiyoshi, B. (2021). Structural Characterization of KKT4, an Unconventional Microtubule-Binding Kinetochore Protein. Structure 29, 1014–1028. e8. doi:10.1016/j.str.2021.04.004
Luykx, P. (1965). The Structure of the Kinetochore in Meiosis and Mitosis in Eggs. Exp. Cell Res. 39, 643–657. doi:10.1016/0014-4827(65)90068-6
Malvezzi, F., Litos, G., Schleiffer, A., Heuck, A., Mechtler, K., Clausen, T., et al. (2013). A Structural Basis for Kinetochore Recruitment of the Ndc80 Complex via Two Distinct Centromere Receptors. EMBO J. 32, 409–423. doi:10.1038/emboj.2012.356
Marcianò, G., Ishii, M., Nerusheva, O. O., and Akiyoshi, B. (2021). Kinetoplastid Kinetochore Proteins KKT2 and KKT3 Have Unique Centromere Localization Domains. J. Cell Biol. 220, e202101022. doi:10.1083/jcb.202101022
Maskell, D. P., Hu, X.-W., and Singleton, M. R. (2010). Molecular Architecture and Assembly of the Yeast Kinetochore MIND Complex. J. Cell Biol. 190, 823–834. doi:10.1083/jcb.201002059
McKinley, K. L., and Cheeseman, I. M. (2016). The Molecular Basis for Centromere Identity and Function. Nat. Rev. Mol. Cell Biol. 17, 16–29. doi:10.1038/nrm.2015.5
McKinley, K. L., Sekulic, N., Guo, L. Y., Tsinman, T., Black, B. E., and Cheeseman, I. M. (2015). The CENP-L-N Complex Forms a Critical Node in an Integrated Meshwork of Interactions at the Centromere-Kinetochore Interface. Mol. Cell 60, 886–898. doi:10.1016/j.molcel.2015.10.027
Mellone, B. G., and Fachinetti, D. (2021). Diverse Mechanisms of Centromere Specification. Curr. Biol. 31, R1491–R1504. doi:10.1016/j.cub.2021.09.083
Meraldi, P., McAinsh, A., Rheinbay, E., and Sorger, P. (2006). Phylogenetic and Structural Analysis of Centromeric DNA and Kinetochore Proteins. Genome Biol. 7, R23. doi:10.1186/gb-2006-7-3-r23
Mikami, Y., Hori, T., Kimura, H., and Fukagawa, T. (2005). The Functional Region of CENP-H Interacts with the Nuf2 Complex that Localizes to Centromere During Mitosis. Mol. Cell. Biol. 25, 1958–1970. doi:10.1128/mcb.25.5.1958-1970.2005
Miller, S. A., Johnson, M. L., and Stukenberg, P. T. (2008). Kinetochore Attachments Require an Interaction Between Unstructured Tails on Microtubules and Ndc80Hec1. Curr. Biol. 18, 1785–1791. doi:10.1016/j.cub.2008.11.007
Mitra, S., Srinivasan, B., and Jansen, L. E. T. (2020a). Stable Inheritance of CENP-A Chromatin: Inner Strength versus Dynamic Control. J. Cell Biol. 219. doi:10.1083/jcb.202005099
Mitra, S., Bodor, D. L., David, A. F., Abdul-Zani, I., Mata, J. F., Neumann, B., et al. (2020b). Genetic Screening Identifies a SUMO Protease Dynamically Maintaining Centromeric Chromatin. Nat. Commun. 11, 501. doi:10.1038/s41467-019-14276-x
Monen, J., Maddox, P. S., Hyndman, F., Oegema, K., and Desai, A. (2005). Differential Role of CENP-A in the Segregation of Holocentric C. elegans Chromosomes During Meiosis and Mitosis. Nat. Cell Biol. 7, 1248–1255. doi:10.1038/ncb1331
Moroi, Y., Peebles, C., Fritzler, M. J., Steigerwald, J., and Tan, E. M. (1980). Autoantibody to Centromere (Kinetochore) in Scleroderma Sera. Proc. Natl. Acad. Sci. U.S.A. 77, 1627–1631. doi:10.1073/pnas.77.3.1627
Mukhopadhyay, D., Arnaoutov, A., and Dasso, M. (2010). The SUMO Protease SENP6 Is Essential for Inner Kinetochore Assembly. J. Cell Biol. 188, 681–692. doi:10.1083/jcb.200909008
Musacchio, A., and Desai, A. (2017). A Molecular View of Kinetochore Assembly and Function. Biology 6, 5. doi:10.3390/biology6010005
Nagpal, H., Hori, T., Furukawa, A., Sugase, K., Osakabe, A., Kurumizaka, H., et al. (2015). Dynamic Changes in CCAN Organization through CENP-C During Cell-Cycle Progression. MBoC 26, 3768–3776. doi:10.1091/mbc.e15-07-0531
Navarro-Mendoza, M. I., Pérez-Arques, C., Panchal, S., Nicolás, F. E., Mondo, S. J., Ganguly, P., et al. (2019). Early Diverging Fungus Mucor Circinelloides Lacks Centromeric Histone CENP-A and Displays a Mosaic of Point and Regional Centromeres. Curr. Biol. 29, 3791–3802. e6. doi:10.1016/j.cub.2019.09.024
Nerusheva, O. O., Ludzia, P., and Akiyoshi, B. (2019). Identification of Four Unconventional Kinetoplastid Kinetochore Proteins KKT22-25 in Trypanosoma Brucei. Open Biol. 9, 190236. doi:10.1098/rsob.190236
Nguyen, A. L., Fadel, M. D., and Cheeseman, I. M. (2021). Differential Requirements for the CENP-O Complex Reveal Parallel PLK1 Kinetochore Recruitment Pathways. MBoC 32, 712–721. doi:10.1091/mbc.e20-11-0751
Nishino, T., Rago, F., Hori, T., Tomii, K., Cheeseman, I. M., and Fukagawa, T. (2013). CENP-T Provides a Structural Platform for Outer Kinetochore Assembly. EMBO J. 32, 424–436. doi:10.1038/emboj.2012.348
Nishino, T., Takeuchi, K., Gascoigne, K. E., Suzuki, A., Hori, T., Oyama, T., et al. (2012). CENP-T-W-S-X Forms a Unique Centromeric Chromatin Structure with a Histone-like Fold. Cell 148, 487–501. doi:10.1016/j.cell.2011.11.061
Obuse, C., Yang, H., Nozaki, N., Goto, S., Okazaki, T., and Yoda, K. (2004). Proteomics Analysis of the Centromere Complex from HeLa Interphase Cells: UV-Damaged DNA Binding Protein 1 (DDB-1) Is a Component of the CEN-Complex, While BMI-1 Is Transiently Co-Localized with the Centromeric Region in Interphase. Genes Cells 9, 105–120. doi:10.1111/j.1365-2443.2004.00705.x
Oegema, K., Desai, A., Rybina, S., Kirkham, M., and Hyman, A. A. (2001). Functional Analysis of Kinetochore Assembly in Caenorhabditis elegans. J. Cell Biol. 153, 1209–1226. doi:10.1083/jcb.153.6.1209
Ogura, Y., Shibata, F., Sato, H., and Murata, M. (2004). Characterization of a CENP-C Homolog in Arabidopsis thaliana. Genes Genet. Syst. 79, 139–144. doi:10.1266/ggs.79.139
Okada, M., Cheeseman, I. M., Hori, T., Okawa, K., McLeod, I. X., Yates, J. R., et al. (2006). The CENP-H-I Complex Is Required for the Efficient Incorporation of Newly Synthesized CENP-A into Centromeres. Nat. Cell Biol. 8, 446–457. doi:10.1038/ncb1396
Ortiz, J., Stemmann, O., Rank, S., and Lechner, J. (1999). A Putative Protein Complex Consisting of Ctf19, Mcm21, and Okp1 Represents a Missing Link in the Budding Yeast Kinetochore. Genes & Dev. 13, 1140–1155. doi:10.1101/gad.13.9.1140
Pekgöz Altunkaya, G., Malvezzi, F., Demianova, Z., Zimniak, T., Litos, G., Weissmann, F., et al. (2016). CCAN Assembly Configures Composite Binding Interfaces to Promote Cross-Linking of Ndc80 Complexes at the Kinetochore. Curr. Biol. 26, 2370–2378. doi:10.1016/j.cub.2016.07.005
Pesenti, M. E., Prumbaum, D., Auckland, P., Smith, C. M., Faesen, A. C., Petrovic, A., et al. (2018). Reconstitution of a 26-Subunit Human Kinetochore Reveals Cooperative Microtubule Binding by CENP-OPQUR and NDC80. Mol. Cell 71, 923–939. e10. doi:10.1016/j.molcel.2018.07.038
Pesenti, M. E., Raisch, T., Conti, D., Walstein, K., Hoffmann, I., Vogt, D., et al. (2022). Structure of the Human Inner Kinetochore CCAN Complex and its Significance for Human Centromere Organization. Mol. Cell. doi:10.1016/j.molcel.2022.04.027
Petrovic, A., Keller, J., Liu, Y., Overlack, K., John, J., Dimitrova, Y. N., et al. (2016). Structure of the MIS12 Complex and Molecular Basis of its Interaction with CENP-C at Human Kinetochores. Cell 167, 1028–1040. e15. doi:10.1016/j.cell.2016.10.005
Petrovic, A., Mosalaganti, S., Keller, J., Mattiuzzo, M., Overlack, K., Krenn, V., et al. (2014). Modular Assembly of RWD Domains on the Mis12 Complex Underlies Outer Kinetochore Organization. Mol. Cell 53, 591–605. doi:10.1016/j.molcel.2014.01.019
Petrovic, A., Pasqualato, S., Dube, P., Krenn, V., Santaguida, S., Cittaro, D., et al. (2010). The MIS12 Complex Is a Protein Interaction Hub for Outer Kinetochore Assembly. J. Cell Biol. 190, 835–852. doi:10.1083/jcb.201002070
Plowman, R., Singh, N., Tromer, E. C., Payan, A., Duro, E., Spanos, C., et al. (2019). The Molecular Basis of Monopolin Recruitment to the Kinetochore. Chromosoma 128, 331–354. doi:10.1007/s00412-019-00700-0
Poddar, A., Roy, N., and Sinha, P. (1999). MCM21 and MCM22, Two Novel Genes of the Yeast Saccharomyces cerevisiae Are Required for Chromosome Transmission. Mol. Microbiol. 31, 349–360. doi:10.1046/j.1365-2958.1999.01179.x
Prendergast, L., Müller, S., Liu, Y., Huang, H., Dingli, F., Loew, D., et al. (2016). The CENP-T/-W Complex Is a Binding Partner of the Histone Chaperone FACT. Genes Dev. 30, 1313–1326. doi:10.1101/gad.275073.115
Prendergast, L., van Vuuren, C., Kaczmarczyk, A., Doering, V., Hellwig, D., Quinn, N., et al. (2011). Premitotic Assembly of Human CENPs -T and -W Switches Centromeric Chromatin to a Mitotic State. PLoS Biol. 9, e1001082. doi:10.1371/journal.pbio.1001082
Przewloka, M. R., Venkei, Z., Bolanos-Garcia, V. M., Debski, J., Dadlez, M., and Glover, D. M. (2011). CENP-C Is a Structural Platform for Kinetochore Assembly. Curr. Biol. 21, 399–405. doi:10.1016/j.cub.2011.02.005
Przewloka, M. R., Venkei, Z., and Glover, D. M. (2009). Searching for Drosophila Dsn1 Kinetochore Protein. Cell Cycle 8, 1292–1293. doi:10.4161/cc.8.8.8159
Przewloka, M. R., Zhang, W., Costa, P., Archambault, V., D'Avino, P. P., Lilley, K. S., et al. (2007). Molecular Analysis of Core Kinetochore Composition and Assembly in Drosophila melanogaster. PLoS One 2, e478. doi:10.1371/journal.pone.0000478
Rago, F., Gascoigne, K. E., and Cheeseman, I. M. (2015). Distinct Organization and Regulation of the Outer Kinetochore KMN Network Downstream of CENP-C and CENP-T. Curr. Biol. 25, 671–677. doi:10.1016/j.cub.2015.01.059
Richter, M. M., Poznanski, J., Zdziarska, A., Czarnocki-cieciura, M., Lipinszki, Z., Dadlez, M., et al. (2016). Network of Protein Interactions within the Drosophila Inner Kinetochore. Open Biol. 6, 150238. doi:10.1098/rsob.150238
Saitoh, H., Tomkiel, J., Cooke, C. A., Ratrie, H., Maurer, M., Rothfield, N. F., et al. (1992). CENP-C, an Autoantigen in Scleroderma, Is a Component of the Human Inner Kinetochore Plate. Cell 70, 115–125. doi:10.1016/0092-8674(92)90538-n
Saitoh, S., Ishii, K., Kobayashi, Y., and Takahashi, K. (2005). Spindle Checkpoint Signaling Requires the Mis6 Kinetochore Subcomplex, Which Interacts with Mad2 and Mitotic Spindles. MBoC 16, 3666–3677. doi:10.1091/mbc.e05-01-0014
Salas-Leiva, D. E., Tromer, E. C., Curtis, B. A., Jerlström-Hultqvist, J., Kolisko, M., Yi, Z., et al. (2021). Genomic Analysis Finds No Evidence of Canonical Eukaryotic DNA Processing Complexes in a Free-Living Protist. Nat. Commun. 12, 6003. doi:10.1038/s41467-021-27605-w
Schleiffer, A., Maier, M., Litos, G., Lampert, F., Hornung, P., Mechtler, K., et al. (2012). CENP-T Proteins Are Conserved Centromere Receptors of the Ndc80 Complex. Nat. Cell Biol. 14, 604–613. doi:10.1038/ncb2493
Schmitzberger, F., and Harrison, S. C. (2012). RWD Domain: A Recurring Module in Kinetochore Architecture Shown by a Ctf19-Mcm21 Complex Structure. EMBO Rep. 13, 216–222. doi:10.1038/embor.2012.1
Schmitzberger, F., Richter, M. M., Gordiyenko, Y., Robinson, C. V., Dadlez, M., and Westermann, S. (2017). Molecular Basis for Inner Kinetochore Configuration through RWD Domain-Peptide Interactions. EMBO J. 36, 3458–3482. doi:10.15252/embj.201796636
Screpanti, E., De Antoni, A., Alushin, G. M., Petrovic, A., Melis, T., Nogales, E., et al. (2011). Direct Binding of Cenp-C to the Mis12 Complex Joins the Inner and Outer Kinetochore. Curr. Biol. 21, 391–398. doi:10.1016/j.cub.2010.12.039
Shiroiwa, Y., Hayashi, T., Fujita, Y., Villar-Briones, A., Ikai, N., Takeda, K., et al. (2011). Mis17 Is a Regulatory Module of the Mis6-Mal2-Sim4 Centromere Complex that Is Required for the Recruitment of CenH3/CENP-A in Fission Yeast. PLoS One 6, e17761. doi:10.1371/journal.pone.0017761
Singh, P., Pesenti, M. E., Maffini, S., Carmignani, S., Hedtfeld, M., Petrovic, A., et al. (2021). BUB1 and CENP-U, Primed by CDK1, Are the Main PLK1 Kinetochore Receptors in Mitosis. Mol. Cell 81, 67–87. e9. doi:10.1016/j.molcel.2020.10.040
Singh, T. R., Saro, D., Ali, A. M., Zheng, X.-F., Du, C.-h., Killen, M. W., et al. (2010). MHF1-MHF2, a Histone-Fold-Containing Protein Complex, Participates in the Fanconi Anemia Pathway via FANCM. Mol. Cell 37, 879–886. doi:10.1016/j.molcel.2010.01.036
Sridhar, S., Dumbrepatil, A., Sreekumar, L., Sankaranarayanan, S. R., Guin, K., and Sanyal, K. (2017). Centromere and Kinetochore: Essential Components for Chromosome Segregation. Gene Regul. Epigenetics Horm. Signal., 259–288. doi:10.1002/9783527697274.ch9
Sridhar, S., Hori, T., Nakagawa, R., Fukagawa, T., and Sanyal, K. (2021). Bridgin Connects the Outer Kinetochore to Centromeric Chromatin. Nat. Commun. 12, 146. doi:10.1038/s41467-020-20161-9
Stanton, R. A., Gernert, K. M., Nettles, J. H., and Aneja, R. (2011). Drugs that Target Dynamic Microtubules: A New Molecular Perspective. Med. Res. Rev. 31, 443–481. doi:10.1002/med.20242
Suzuki, A., Badger, B. L., and Salmon, E. D. (2015). A Quantitative Description of Ndc80 Complex Linkage to Human Kinetochores. Nat. Commun. 6, 8161. doi:10.1038/ncomms9161
Suzuki, A., Badger, B. L., Wan, X., DeLuca, J. G., and Salmon, E. D. (2014). The Architecture of CCAN Proteins Creates a Structural Integrity to Resist Spindle Forces and Achieve Proper Intrakinetochore Stretch. Dev. Cell 30, 717–730. doi:10.1016/j.devcel.2014.08.003
Suzuki, A., Hori, T., Nishino, T., Usukura, J., Miyagi, A., Morikawa, K., et al. (2011). Spindle Microtubules Generate Tension-Dependent Changes in the Distribution of Inner Kinetochore Proteins. J. Cell Biol. 193, 125–140. doi:10.1083/jcb.201012050
Takahashi, K., Chen, E. S., and Yanagida, M. (2000). Requirement of Mis6 Centromere Connector for Localizing a CENP-A-Like Protein in Fission Yeast. Science 288, 2215–2219. doi:10.1126/science.288.5474.2215
Takenoshita, Y., Hara, M., and Fukagawa, T. (2022). Recruitment of Two Ndc80 Complexes via the CENP-T Pathway Is Sufficient for Kinetochore Functions. Nat. Commun. 12, 851. doi:10.1038/s41467-022-28403-8
Takeuchi, K., Nishino, T., Mayanagi, K., Horikoshi, N., Osakabe, A., Tachiwana, H., et al. (2014). The Centromeric Nucleosome-Like CENP-T-W-S-X Complex Induces Positive Supercoils into DNA. Nucleic Acids Res. 42, 1644–1655. doi:10.1093/nar/gkt1124
Tanaka, K., Li Chang, H., Kagami, A., and Watanabe, Y. (2009). CENP-C Functions as a Scaffold for Effectors with Essential Kinetochore Functions in Mitosis and Meiosis. Dev. Cell 17, 334–343. doi:10.1016/j.devcel.2009.08.004
Thakur, J., and Henikoff, S. (2016). CENPT Bridges Adjacent CENPA Nucleosomes on Young Human α-Satellite Dimers. Genome Res. 26, 1178–1187. doi:10.1101/gr.204784.116
Thakur, J., Talbert, P. B., and Henikoff, S. (2015). Inner Kinetochore Protein Interactions with Regional Centromeres of Fission Yeast. Genetics 201, 543–561. doi:10.1534/genetics.115.179788
Thapa, K. S., Oldani, A., Pagliuca, C., De Wulf, P., and Hazbun, T. R. (2015). The Mps1 Kinase Modulates the Recruitment and Activity of Cnn1CENP-T at Saccharomyces cerevisiae Kinetochores. Genetics 200, 79–90. doi:10.1534/genetics.115.175786
Tomkiel, J., Cooke, C. A., Saitoh, H., Bernat, R. L., and Earnshaw, W. C. (1994). CENP-C Is Required for Maintaining Proper Kinetochore Size and for a Timely Transition to Anaphase. J. Cell Biol. 125, 531–545. doi:10.1083/jcb.125.3.531
Trazzi, S., Perini, G., Bernardoni, R., Zoli, M., Reese, J. C., Musacchio, A., et al. (2009). The C-Terminal Domain of CENP-C Displays Multiple and Critical Functions for Mammalian Centromere Formation. PLoS One 4, e5832. doi:10.1371/journal.pone.0005832
Tromer, E. C., van Hooff, J. J. E., Kops, G. J. P. L., and Snel, B. (2019). Mosaic Origin of the Eukaryotic Kinetochore. Proc. Natl. Acad. Sci. U.S.A. 116, 12873–12882. doi:10.1073/pnas.1821945116
Tromer, E. C., Wemyss, T. A., Ludzia, P., Waller, R. F., and Akiyoshi, B. (2021). Repurposing of Synaptonemal Complex Proteins for Kinetochores in Kinetoplastida. Open Biol. 11, 210049. doi:10.1098/rsob.210049
Uchida, K. S. K., Jo, M., Nagasaka, K., Takahashi, M., Shindo, N., Shibata, K., et al. (2021). Kinetochore Stretching-Mediated Rapid Silencing of the Spindle-Assembly Checkpoint Required for Failsafe Chromosome Segregation. Curr. Biol. 31, 1581–1591. e3. doi:10.1016/j.cub.2021.01.062
Valverde, R., Ingram, J., and Harrison, S. C. (2016). Conserved Tetramer Junction in the Kinetochore Ndc80 Complex. Cell Rep. 17, 1915–1922. doi:10.1016/j.celrep.2016.10.065
van Hooff, J. J. E., Snel, B., and Kops, G. J. P. L. (2017). Unique Phylogenetic Distributions of the Ska and Dam1 Complexes Support Functional Analogy and Suggest Multiple Parallel Displacements of Ska by Dam1. Genome Biol. Evol. 9, 1295–1303. doi:10.1093/gbe/evx088
Venkei, Z., Przewloka, M. R., Ladak, Y., Albadri, S., Sossick, A., Juhasz, G., et al. (2012). Spatiotemporal Dynamics of Spc105 Regulates the Assembly of the Drosophila Kinetochore. Open Biol. 2, 110032. doi:10.1098/rsob.110032
Verma, G., and Surolia, N. (2014). The Dimerization Domain of Pf CENP-C Is Required for its Functions as a Centromere Protein in Human Malaria Parasite Plasmodium Falciparum. Malar. J. 13, 475. doi:10.1186/1475-2875-13-475
Virant, D., Vojnovic, I., Winkelmeier, J., and Endesfelder, M. (2021). Unraveling the Kinetochore Nanostructure in Schizosaccharomyces pombe Using Multi-Color Single-Molecule Localization Microscopy. BioRxiv 2021. doi:10.1101/2021.12.01.469981
Volkov, V. A., Dogterom, M., and Musacchio, A. (2018). Huis in ’t VeldMultivalency of NDC80 in the Outer Kinetochore Is Essential to Track Shortening Microtubules and Generate Forces. Elife 7, e36764. doi:10.7554/elife.36764
Wagner, K., Kunz, K., Piller, T., Tascher, G., Hölper, S., Stehmeier, P., et al. (2019). The SUMO Isopeptidase SENP6 Functions as a Rheostat of Chromatin Residency in Genome Maintenance and Chromosome Dynamics. Cell Rep. 29, 480–494. e5. doi:10.1016/j.celrep.2019.08.106
Walstein, K., Petrovic, A., Pan, D., Hagemeier, B., Vogt, D., Vetter, I. R., et al. (2021). Assembly Principles and Stoichiometry of a Complete Human Kinetochore Module. Sci. Adv. 7, 34193424. doi:10.1126/sciadv.abg1037
Watanabe, R., Hara, M., Okumura, E.-i., Hervé, S., Fachinetti, D., Ariyoshi, M., et al. (2019). CDK1-Mediated CENP-C Phosphorylation Modulates CENP-A Binding and Mitotic Kinetochore Localization. J. Cell Biol. 218, 4042–4062. doi:10.1083/jcb.201907006
Watanabe, R., Hirano, Y., Hara, M., Hiraoka, Y., and Fukagawa, T. (2022). Mobility of Kinetochore Proteins Measured by FRAP Analysis in Living Cells. Chromosom. Res. 30, 43–57. doi:10.1007/s10577-021-09678-x
Wei, R. R., Al-Bassam, J., and Harrison, S. C. (2007). The Ndc80/HEC1 Complex Is a Contact Point for Kinetochore-Microtubule Attachment. Nat. Struct. Mol. Biol. 14, 54–59. doi:10.1038/nsmb1186
Weir, J. R., Faesen, A. C., Klare, K., Petrovic, A., Basilico, F., Fischböck, J., et al. (2016). Insights from Biochemical Reconstitution into the Architecture of Human Kinetochores. Nature 537, 249–253. doi:10.1038/nature19333
Welburn, J. P. I., Vleugel, M., Liu, D., Yates, J. R., Lampson, M. A., Fukagawa, T., et al. (2010). Aurora B Phosphorylates Spatially Distinct Targets to Differentially Regulate the Kinetochore-Microtubule Interface. Mol. Cell 38, 383–392. doi:10.1016/j.molcel.2010.02.034
Westermann, S., Cheeseman, I. M., Anderson, S., Yates, J. R., Drubin, D. G., and Barnes, G. (2003). Architecture of the Budding Yeast Kinetochore Reveals a Conserved Molecular Core. J. Cell Biol. 163, 215–222. doi:10.1083/jcb.200305100
Xiao, H., Wang, F., Wisniewski, J., Shaytan, A. K., Ghirlando, R., FitzGerald, P. C., et al. (2017). Molecular Basis of CENP-C Association with the CENP-A Nucleosome at Yeast Centromeres. Genes Dev. 31, 1958–1972. doi:10.1101/gad.304782.117
Yamada, M., and Goshima, G. (2017). Mitotic Spindle Assembly in Land Plants: Molecules and Mechanisms. Biology 6, 6. doi:10.3390/biology6010006
Yan, K., Yang, J., Zhang, Z., McLaughlin, S. H., Chang, L., Fasci, D., et al. (2019). Structure of the Inner Kinetochore CCAN Complex Assembled onto a Centromeric Nucleosome. Nature 574, 278–282. doi:10.1038/s41586-019-1609-1
Yan, Z., Delannoy, M., Ling, C., Daee, D., Osman, F., Muniandy, P. A., et al. (2010). A Histone-Fold Complex and FANCM Form a Conserved DNA-Remodeling Complex to Maintain Genome Stability. Mol. Cell 37, 865–878. doi:10.1016/j.molcel.2010.01.039
Yang, C. H., Tomkiel, J., Saitoh, H., Johnson, D. H., and Earnshaw, W. C. (1996). Identification of Overlapping DNA-Binding and Centromere-Targeting Domains in the Human Kinetochore Protein CENP-C. Mol. Cell. Biol. 16, 3576–3586. doi:10.1128/mcb.16.7.3576
Yatskevich, S., Muir, K. W., Bellini, D., Zhang, Z., Yang, J., Tischer, T., et al. (2022). Structure of the Human Inner Kinetochore Bound to a Centromeric CENP-A Nucleosome. Science 376, 844–852. doi:10.1126/science.abn3810
Ye, A. A., Cane, S., and Maresca, T. J. (2016). Chromosome Biorientation Produces Hundreds of Piconewtons at a Metazoan Kinetochore. Nat. Commun. 7, 13221. doi:10.1038/ncomms13221
Zeeshan, M., Pandey, R., Ferguson, D. J. P., Tromer, E. C., Markus, R., Abel, S., et al. (2020). Real-Time Dynamics of Plasmodium NDC80 Reveals Unusual Modes of Chromosome Segregation During Parasite Proliferation. J. Cell Sci. 134, jcs245753. doi:10.1242/jcs.245753
Zhang, M., Wang, C., Otto, T. D., Oberstaller, J., Liao, X., Adapa, S. R., et al. (2018). Uncovering the Essential Genes of the Human Malaria Parasite Plasmodium Falciparum by Saturation Mutagenesis. Science 360, eaap7847. doi:10.1126/science.aap7847
Zhang, Z., Bellini, D., and Barford, D. (2020). Crystal Structure of the Cenp-HIKHead-TW Sub-Module of the Inner Kinetochore CCAN Complex. Nucleic Acids Res. 48, 11172–11184. doi:10.1093/nar/gkaa772
Keywords: chromosome segregation, mitosis, kinetochore, centromere, evolution, CCAN, constitutive centromere associated network, mitotic spindle
Citation: Sridhar S and Fukagawa T (2022) Kinetochore Architecture Employs Diverse Linker Strategies Across Evolution. Front. Cell Dev. Biol. 10:862637. doi: 10.3389/fcell.2022.862637
Received: 26 January 2022; Accepted: 23 May 2022;
Published: 20 June 2022.
Edited by:
Nikolina Sekulic, University of Oslo, NorwayReviewed by:
Eelco Tromer, University of Groningen, NetherlandsInes Drinnenberg, Institut Curie, France
Copyright © 2022 Sridhar and Fukagawa. This is an open-access article distributed under the terms of the Creative Commons Attribution License (CC BY). The use, distribution or reproduction in other forums is permitted, provided the original author(s) and the copyright owner(s) are credited and that the original publication in this journal is cited, in accordance with accepted academic practice. No use, distribution or reproduction is permitted which does not comply with these terms.
*Correspondence: Shreyas Sridhar, c3NyaWRoYXJAZmJzLm9zYWthLXUuYWMuanA=; Tatsuo Fukagawa, ZnVrYWdhd2EudGF0c3VvLmZic0Bvc2FrYS11LmFjLmpw