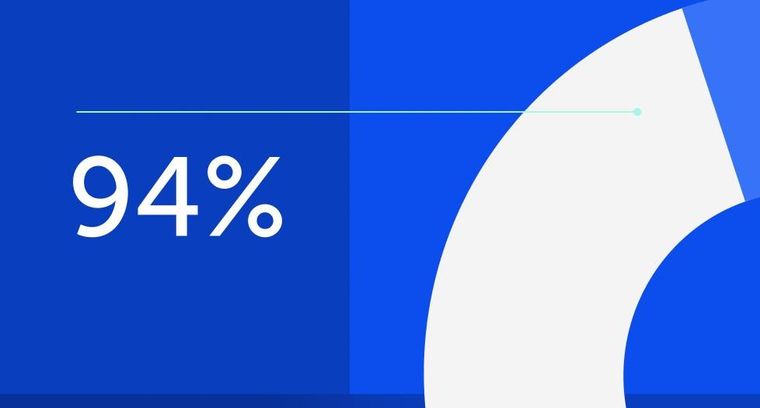
94% of researchers rate our articles as excellent or good
Learn more about the work of our research integrity team to safeguard the quality of each article we publish.
Find out more
REVIEW article
Front. Cell Dev. Biol., 16 May 2022
Sec. Cancer Cell Biology
Volume 10 - 2022 | https://doi.org/10.3389/fcell.2022.855474
Pancreatic cancer (PC) is one of the most lethal cancers with an almost 10% 5-year survival rate. Because PC is implicated in high heterogeneity, desmoplastic tumor-microenvironment, and inefficient drug-penetration, the chemotherapeutic strategy currently recommended for the treatment of PC has limited clinical benefit. Nucleic acid-based targeting therapies have become strong competitors in the realm of drug discovery and targeted therapy. A vast evidence has demonstrated that antibody-based or alternatively aptamer-based strategy largely contributed to the elevated drug accumulation in tumors with reduced systematic cytotoxicity. This review describes the advanced progress of antisense oligonucleotides (ASOs), small interfering RNAs (siRNAs), microRNAs (miRNAs), messenger RNA (mRNAs), and aptamer-drug conjugates (ApDCs) in the treatment of PC, revealing the bright application and development direction in PC therapy.
Pancreatic cancer (PC) is one of the deadliest malignancies with poor outcomes, and over 90% of PC belongs to pancreatic ductal adenocarcinoma (PDAC) (Hidalgo, 2010). The overall 5-year survival rate for PC in 2020 is only 9%, which is predicted to cause the second mortality regarding cancer-related death in the upcoming decade (Siegel et al., 2020). It is known that early detection and treatment for patients largely contribute to the therapeutic effect on PC. Unfortunately, PDAC does not cause symptoms right away and it is hard to detect the disease early. Most of the patients are found to be in the advanced stages without efficient drug administration (Kleeff et al., 2016; Leroux and Konstantinidou, 2021). For now, surgical resection is still the main treatment method against resectable PDAC, and the chemotherapeutic strategy currently recommended for the treatment of PC has limited clinical benefit (Table 1) (Mcguigan et al., 2018). The updated reports have substantiated the important influence of intertumoral heterogeneity and tumor microenvironment on PDAC-based drug discovery (Sun et al., 2020). Thus, it is an urgent requirement to generate and develop more specific inhibitors by overcoming the aforementioned defects.
The oncogene Kirsten Ras (KRAS) and tumor suppressor gene tumor protein 53 (TP53) could be detected through the genetic carcinogenesis of PDAC (Waters and Der, 2018). KRAS is the predominant isoform mutated in cancer and the isoform mutated exclusively in PDAC, driving the tumor initiation, progression, and metastasis. The increased desmoplasia, together with several cell types including cancer-associated fibroblasts (CAFs), pancreatic stellate cells (PSCs), muscle fibroblasts, and immune cells composes of the typical PDAC characteristics. Nucleic acid drugs consisting of ribonucleotide (RNA) or deoxyribonucleotide (DNA) have bright potential in gene inhibition, gene activation, immunity stimulation, and protein recognition. The nucleic acid drugs mainly include antisense oligonucleotides (ASOs) (Crooke et al., 2017), small interfering RNAs (siRNAs) (Castel and Martienssen, 2013), small activating RNAs (saRNAs), microRNAs (Hayes et al., 2014), mRNAs, ribozyme, aptamers (Kaur et al., 2018), and so on (Sullenger and Nair, 2016). From 1998 to 2020, ten oligonucleotides therapeutics have been approved by the FDA (Roberts et al., 2020). The abundant nucleic acid drugs provide diverse therapeutic strategies for overcoming the defects in intertumoral heterogeneity and tumor microenvironment. PC always suffers from multiple gene mutations, which are ideal targets for gene-silencing or alternative aptamer binding. Additionally, mRNA vaccines and related anticancer strategies have been paid more attention since the prevalence of COVID-19. The clinical trials data have demonstrated the promising prospects of mRNA vaccines against nonsmall cell lung cancer and advanced castration-resistant prostate cancer (Kubler et al., 2015; Huang et al., 2021). Notably, aptamers are single-stranded nucleic acids capable of binding to their targets through 3D complementarity, with high specificity and affinity as well as simple synthesizability and low immunogenicity. Importantly, much preclinical evidence has shown that aptamer-drug conjugates (ApDCs) are a promising therapy strategy against PC (Li F. F. et al., 2017). Theoretically, nucleic acid drugs based on tumor-specific genes and alternative tumor-specific antigens could be designed for any cancer. Thus, nucleic acid drugs might be a promising therapy strategy against PC.
In this review, we summarized the current progress of antisense oligonucleotides (ASOs), small interfering RNAs (siRNAs), microRNAs (miRNAs), messenger RNA (mRNAs), and aptamer-drug conjugates (ApDCs) in the treatment of PC and were detailedly explored to present a discussion and perspective of these therapies against PC.
Nucleic acid-based drugs can be classified into multiple types in terms of differences in chemical structure and functional mechanism, which include antisense oligonucleotides (ASOs) (Crooke et al., 2017), small interfering RNAs (siRNAs) (Castel and Martienssen, 2013), microRNAs (Hayes et al., 2014), mRNA, and aptamers (Kaur et al., 2018). From the first FDA-approved ASOs fomivirsen for clinical use in 1998 (Lundin et al., 2015), ten oligonucleotides therapeutics have been approved by the FDA as of January 2020, including six ASOs, two siRNAs, one RNA-based aptamer, and one mixture of ssDNA and dsDNA (Roberts et al., 2020). The dominating advantage of nucleic-acid based drugs is that their pharmacokinetics are based on the chemical entity, whereas their base sequence determines their target, allowing them to exhibit safety characteristic and potent pharmacodynamics.
ASOs are single-stranded RNA/DNA sequences containing usually 16–21 nucleotides in length and could specifically bind to RNA encoding the gene of interest via Watson–Crick base pair interactions in a sequence-specific manner. The inactivation of RNA induced by ASOs can be categorized broadly into two predominant termination mechanisms (Figure 1A): 1) RNase H-dependent: the destabilization by the formation of RNA/DNA duplex after recognition and binding to mature mRNA, followed by degradation by ribonuclease H1 (RNase H1), a ubiquitously expressed cellular endonuclease which specifically degrades DNA-RNA hybrids; 2) Steric-blocker: the internalization of ASOs lead to the binding to pre-mRNA, modulating translational arrest, alternative splicing, or disrupting recognition by splicing factors Bennett et al. (2017); Crooke et al. (2017); Shen and Corey (2018). RNase H-dependent manner is the dominant mechanism for ASOs and the following examples targeting PDAC are all based on this mechanism.
FIGURE 1. Typical mechanism of oligonucleotide drugs. (A) ASO-induced inactivation of mRNA could attribute to RNase H-dependent mRNA degradation or mRNA inhibition (also termed steric-blocker); (B) siRNA-indued mRNA cleavage contains the formation of siRNA-RISC complex, activated AGO2-RISC complex, followed by mRNA binding; (C) miRNA-induced mRNA cleavage contains the key formation of the miRISC complex for mRNA binding. Created with BioRender (License No: VV23OTLLHP).
The point mutations of the KRAS gene could be detected in 90% of human PCs, which indicates its important role in tumorigenesis. Therefore, targeting KRAS point mutations represents one potential way to treat pancreatic cancer. In 2001, a group investigated the invasive activity by one 17-mer phosphorothioate ASOs targeting KRAS point mutations in MIA PaCa-2, PANC-1, and BxPC-3 pancreatic cancer cells, and the results indicated that 17-mer ASOs could strongly inhibit the invasive activity of MIA PaCa-2, PANC-1 with aggressively mutated KRAS but not in BxPC-3 with a wild-type KRAS (Nakada et al., 2001). Additionally, ASOs targeting KRAS also inhibited the tumor growth and invasiveness of HaP-T1 (from the BHP-induced hamster PC model) in a dose-dependent manner, whereas mismatched ASOs were not effective in inhibiting invasion (Morioka et al., 2005). In 2013, the transfection of ASOs targeting KRAS point mutation by lipofectamine was investigated in human pancreatic carcinoma PC-2 cells. Further cell cycle assay showed majority of the cells were in the G1 phase and few were in the S phase, indicating the promotion of apoptosis of PC-2 cells induced by ASOs (Wang Y. X. et al., 2014). Another synthetic oligonucleotide with specific binding to KRAS via an ASOs-like mechanism, U1 Adaptors, also showed reduced KRAS mRNA expression up to 76% in MIA PaCa-2 cells. For the in vivo experiments, U1 adaptors were conjugated to tumor-targeting peptides such as cyclic arginylglycylaspartic acid (cRGD) resulting in the inhibition of tumor growth by averages of 68–93% (Carpizo et al., 2016).
Heat shock protein 27 (Hsp27) is correlated to several pathological processes such as cancer and its high expression could be connected to chemotherapy-exposed PC. The modified ASO (termed as OGX-427) complementary to the Hsp27 gene was subtly designed and showed great inhibition of proliferation, induction of apoptosis, and improvement of gemcitabine chemosensitivity in MIA PaCa-2 cells both in vitro and xenografted in mice (Baylot et al., 2011). Despite gene suppression related to PC, it is necessary to consider the specific tumor microenvironment of PC especially PSCs. By construction of a tumor microenvironment-responsive nanosystem with PEGylated polyethylenimine-coated gold nanoparticles, all-trans retinoic acid and antiHSP47 ASO were codelivered to reprogram PSCs. Their results indicated that the nanosystem could induce PSC quiescence with a 79% downregulation of HSP47 protein and inhibit extracellular matrix (ECM) hyperplasia, therefore promoting drug penetration to pancreatic tumors and greatly improving the antitumor efficacy (Han et al., 2018).
The vascular endothelial growth factor (VEGF) family of growth factors regulates angiogenesis and overexpression of VEGF in PDAC associated with a high angiogenesis density, improved metastasis, disease progression, and a reduced prognosis (Longo et al., 2016). One antiVEGF ASOs (AS-3) was designed and synthesized, which could normalize plasma VEGF and decrease neoangiogenesis, therefore indicating improved inhibition of tumor growth and metastasis as well as improved survival the in AsPC-1 xenograft nude mouse model. Notably, no ascites could be detected in animals treated with AS-3, demonstrating vascular permeability could be decreased by reducing VEGF expression in PC cells (Hotz et al., 2005).
Bcl-xL is an antiapoptotic factor of the B-cell lymphoma 2 (Bcl-2) family and its overexpression presence correlates with shorter patient survival. One anti-Bcl-xL ASO was shown to greatly inhibit PC cell growth and cause apoptosis with reduced Bcl-xL protein levels. This Bcl-xL ASO also enhanced the cytotoxicity of gemcitabine in five PC cells (PANC-1, MIA PaCa-2, Capan-1, AsPC-1, and T3M4) (Xu et al., 2001). To improve the internalization into cells, anti-Bcl-xL ASO was further conjugated with antennapedia and this conjugate, together with irradiation as a supplement, significantly inhibited cell growth compared with ASOs alone and achieved meaningful results in vivo for radiosensitization of PC (Masui et al., 2006). Transforming growth factor-beta 2 (TGF-β2) regulates pivotal mechanisms including carcinogenesis, especially immunosuppression and metastasis within PC. Trabedersen (AP 12009) was one kind of ASO modified by phosphorothioate linkages, which targeted human TGF-β2 mRNA and was capable of reducing TGF-β2 secretion in Hup-T3 and PA-TU-8902 pancreatic cancer cells. This anti-TGF-β2 inhibitor ASO could greatly inhibit cell growth and absolutely block cell migration. Furthermore, this ASO was evaluated in the orthotopic mouse models experiments transplanted with metastatic PC, followed by the intraperitoneal (i.p.) treatment. This anti-TGF-β2 ASO could dramatically inhibit tumor proliferation as well as metastasis and angiogenesis (Stauder et al., 2004; Schlingensiepen et al., 2011). In a further phase II clinical study, a cohort of nine patients who were at the advanced PDAC stage and received intravenous trabedersen exhibited excellent safety as well as a promising overall median survival of 13.4 months (Helmut et al., 2012). However, no more clinical data are available to date.
AEG-35156 is a 19-mer phosphorothioate ASO targeting the caspase inhibitor X-linked inhibitor of apoptosis protein (XIAP) messenger RNA. XIAP is a representative sign to evade apoptotic cue-induced by chemotherapy in cancers including PC. Although the combination strategy of AEG35156 and gemcitabine showed promising results in preclinical models against PC, the combined therapy failed to show significant clinical activity in the phase I study in metastatic PC (Mahadevan et al., 2013). MicroRNA-21 is quite a popular cancer target, which has been demonstrated to correlate significantly with oncogenic initiation and progression. One study found that the combination of one antimicroRNA-21 ASOs (ASO-miR-21) and gemcitabine encapsulated by polyethylene glycol-polyethylenimine-magnetic iron oxide nanoparticles precoated with one target bullet fragment (scFvCD44v6) could downregulate oncogenic miR-21, thus leading to upregulation of tumor-suppressor genes programmed cell death protein 4 (PDCD4) and phosphatase and tensin homolog (PTEN) and the inhibition of aggressive invasion of PC cells in vitro. The combination strategy could induce much higher cell apoptosis when compared with a single dose of ASO or gemcitabine in vitro. Following in vivo data also demonstrated its good targeting ability as well as better efficacy in terms of tumor inhibition and liver metastasis (Li Y. Q. et al., 2017).
Among the RNA interference mechanism existing in virtually all the eukaryotic cells, short double-stranded RNAs, i.e., small interfering RNAs (siRNAs), also adopt this ubiquitous pathway to degrade target gene mRNA and suppress its expression with high specificity. siRNAs usually consist of 21–23 nucleotides as a total length with two strands, one strand is the mRNA sequence called the sense strand and the other strand is called the antisense strand (Wittrup and Lieberman, 2015). Once inside the cells, siRNA could be recognized by the RNA-induced silencing complex (RISC) to formulate the siRNA-RISC complex. Then the sense strand could be cleaved by the cleavage enzyme argonaute-2 (AGO2) while the antisense strand and AGO2-RISC could formulate the activated AGO2-RISC complex. This complex is capable of searching and binding to an mRNA that contains a complementary sequence to antisense active strand, followed by the mRNA cleavage and inactivation of mRNA (Figure 1B) (De Fougerolles et al., 2007; Tatiparti et al., 2017).
Compared with ASO drugs, the transition from siRNA to drugs could go through similar problems but not limited to the degradation by nucleases, innate immune response, and most importantly inefficient transmembrane ability (Wittrup and Lieberman, 2015). Chemical modifications and the construction of a delivery system are usually adopted to overcome the above obstacles (Kanasty et al., 2013; Yin et al., 2014; Kim et al., 2016). For the two FDA-approved siRNA drugs Patisiran and Givosiran, 2′-O-methyl and 2′-fluoro substitution of 2′-OH ribose and part of thiophosphate linkages were taken (Adams et al., 2018; Roberts et al., 2020; Scott, 2020). Unlike highly-modified ASO, siRNA, in many cases, requires an appropriate delivery system given the larger size and the negative charges (Yin et al., 2014).
Similar to ASO targeting KRAS, siRNAs also exhibited various effects in KRAS mutated PC. For example, K1/siRNA and K2/siRNA located at sites 194 and 491, respectively, were designed and transfected into MIA PaCa-2 cells, leading to increased apoptosis by inhibition of activated KRAS gene (Wang et al., 2005). But this siRNA expression cassette failed in vivo because of the short half-life and difficult transfection into cells. In 2007, another group designed and investigated three kinds of KRAS siRNA in the BxPC-3 (wild type-KRASGGT), Capan1 (KRASGTT), and PANC-1 (KRASGAT) cell lines. Their results indicated the corresponding KRAS siRNA induced a significant 80% inhibition of KRAS endogenous expression and cell proliferation at 100 nM drug concentration. In vivo, KRAS siRNAGAT containing suppression of endogenous RNA (pSUPER) vector plus gemcitabine resulted in a prolonged survival rate of 64 days and dramatically reduced the mean tumor volume compared with single agents (52 days for gemcitabine, 45 days for KRAS siRNAGAT containing pSUPER vector) (Rejiba et al., 2007). Another group investigated the combination effects of KRAS siRNA gene silencing activity and arsenic-mediated apoptosis with the aid of nanoparticles, a downregulation of KRAS mRNA and protein in PANC-1 cells (KRASGAT) treated with the combination therapy of up to 38.64 and 43.49% was achieved, respectively, resulting in enhanced inhibition of PC cell growth, migration, and invasion supplemented with the arsenic-mediated apoptosis. This combination therapy showed an improved efficiency of about 2-fold increase in the inhibition of tumor growth compared with the PBS group in the mouse models (Zeng et al., 2014). Ken-Tye Yang et al. (2015) developed one biodegradable charged polyester-based vector (BCPV) delivery system for KRAS siRNA within MIA PaCa-2 cells and found the growth, migration and invasion of the MIA PaCa-2 cells could be significantly inhibited. In mouse models, KRAS siRNA showed an improved accumulation for over 3 days and enhanced inhibition of tumor growth with the aid of BCPVs (Lin et al., 2017). Similarly, they also studied and demonstrated improved chemosensitivity of gemcitabine by BCPV-aided delivery of KRAS siRNA plus Notch1 siRNA in MIA PaCa-2 cells (Yang et al., 2017).
Survivin is also one of the cancer-related targets with high expression including PC and evidence has shown radiation would lead to increased survivin mRNA expression in all cell lines. Therefore, antisurvivin siRNA was capable of preventing the radioresistance of PC cells including AsPC-1, SUIT-2, and PANC-1 (Kami et al., 2005). This reduction of survivin mRNA expression up to 90% through survivin-specific siRNA was also observed as well as pronounced morphological changes including enlargement of cells and multinucleation (Tsuji et al., 2005). The overexpression of nestin could also be observed in many cancers including PC, which is thought to be involved in cancer invasion and metastasis. Specifically, compared with monotherapy inhibition by gemcitabine, the combination of antinestin siRNA and gemcitabine exhibited enhanced cytotoxicity up to 80% of PANC-1 cells and 85% in PK-45H cells. Administration of antinestin siRNA significantly inhibited the tumor growth and metastasis compared to the negative control siRNA group in an orthotopic implantation model (Matsuda et al., 2016).
The abnormal expression of nerve growth factor (NGF), together with its receptor proto-oncogene tropomyosin receptor kinase A (TrkA), is also connected with the aggressive and highly metastatic behavior of PC. Thus, TrkA-specific siRNA was designed and showed suppression of up to 83% of TrkA expression and kinase activity, and a much more increase of up to 5-fold of gemcitabine-induced, caspase-mediated apoptosis in PANC-1, MIA PaCa-2 and AsPC-1 (Liu et al., 2007). These results suggested TrkA might be a determinant of pancreatic adenocarcinoma chemoresistance. Xingyu Jiang et al. (Lei et al., 2017) developed a gold nanocluster-aided delivery system of NGF siRNA (GNC-siRNA) with a hydrodynamic diameter of about 70.2 nm. GNC-siRNA complex at 100 nM concentration showed 75% downregulation of NGF mRNA expression compared to 17% reduction of NGF mRNA expression by free siRNA, resulting in cancer growth and migration of PANC-1 cells. Most importantly, GNC-siRNA retarded siRNA degradation in serum conditions within 6 h resulted in a prolonged circulation lifetime of siRNA in blood and facilitated the siRNA internalization as well as the lysosomal escape. This GNC-siRNA also exhibited effective tumor regression in three pancreatic tumor animal models. Besides, the siRNA could be encapsulated by GNCs with the highest loading rate compared with other published data (226 mmol siRNA per g GNCs), which might serve as one promising delivery approach for PC treatment.
In terms of TGF-β signaling, its deregulation is usually related to tumor growth, invasion, and metastasis. Ski, as a TGF-β repressor, is an oncogenic protein and prevents related gene transcription. Anti-Ski siRNA-treated cells could restore TGF-β transcriptional activity and lead to a 5-fold decrease in tumor growth in nude mice (Heider et al., 2007). The combination of gene silencing of TGF-β1 (ppp-TGF-β) and immune activation via the cytosolic helicase retinoic acidinducible gene I (RIG-I) could remodel the immunosuppressive environment by recruiting CD8+ T cells and exert promising antineoplastic effects in PANC-1, MIA PaCa-2, and BxPC-3 cell lines as well as an orthotopic mouse model of PC. In addition, ppp-TGF-β with RIG-I activation also induced much more release of type I interferon and C-X-C motif chemokine ligand 10 (CXCL10), thus resulting in activation of CD8+ T cells and potent tumor cell apoptosis in orthotopic mouse model (Ellermeier et al., 2013). Other siRNAs targeting Bcl-2 mRNA (anti-Bcl-2 siRNA) were also proven to inhibit expression of the target gene in vitro, reaching maximum apoptosis of about 37.1% after 120 h at 10 nM concentration in YAP C PC cells. Within PC xenografts in male nude mice treated intraperitoneally, the tumor growth could significantly be inhibited but no more cytotoxicity could be observed on fibroblasts or normal tissues. However, the siRNA was rapidly metabolized and eliminated by the liver and excreted via the kidneys, thus explaining only a delayed growth of PC xenografts in vivo instead of strong suppression (Ocker et al., 2005).
Cyclooxygenase-2 (COX-2) correlates with the tumorigenesis of epithelial cancers including PC. The transfection of COX-2 siRNA (50 nM siRNA with 5 µl/2 ml Lipo) could downregulate about 70% of the expression of downstream COX-2 protein, further suppressing the vitality of Capan-2 cells but without causing significant cell apoptosis. However, anti-COX-2 siRNA could significantly inhibit tumor growth in subcutaneous xenografts nude mice (50 mm3/54.7 mg compared with 119.3 mm3/175.53 mg as the negative control) (Zhong et al., 2012). As PANC-1 cancer cells have higher nuclear factor-κB (NF-κB) and XIAP levels, antiNF-κB p65 siRNA could downregulate p65 protein and XIAP protein but not to a satisfying level. Further introduction of antiXIAP siRNA in combination with antiNF-κB p65 siRNA could effectively downregulate the expression of XIAP and p65 proteins and enhance the chemosensitivity of PC cells to gemcitabine (Cao et al., 2013).
Reprogramming tumor immune microenvironment (TIME) in PC plays a vital role because immune evasion of PC can include but is not limited to autophagy (Yamamoto et al., 2020). For instance, phosphatidylinositide 3-kinase γ (PI3K-γ) and colony-stimulating factor 1 (CSF-1)/colony stimulating factor 1 receptor (CSF-1R) pathways were thought to be involved in the infiltration and polarization of immunosuppressive cells. Therefore, dual blockade of PI3K-γ inhibitor NVP-BEZ 235 and antiCSF-1R-siRNA specifically targeting specific deactivated immune cells were developed. Therefore, the TIME could be reversed from inflammatory deactivation to inflammatory activation, further promoting a strong immune response and tumor growth in vivo (Li et al., 2020).
To facilitate the efficient delivery, one siRNA targeting polo-like kinase 1 (PLK1) was encapsulated by a novel nanosystem, which consists of cRGD modification on the surface of well-prepared ionizable lipid nanoparticles (GARP). This GARP/anti-PLK1 siRNA combination showed enhanced efficacy both in vitro and in vivo (Guo et al., 2021), which might give guidance on the implication against PDAC.
MicoRNAs, abbreviated as miRNAs, are single-stranded, short noncoding RNAs containing 20–24 nucleotides. MicroRNAs are indeed involved in all physiological signaling pathways, especially in mammals (Jansson and Lund, 2012; Dong et al., 2013). The mechanism of miRNAs-mediated gene silencing is achieved by directing Argonaute (AGO) proteins to related mRNA binding sites through a base-pairing mechanism, followed by the formation of the miRNA-induced silencing complexes (miRISCs). Therefore, the downstream deadenylation of the mRNA target could be catalyzed by the further formation of poly(A)-nuclease 2-poly(A)-nuclease 3 (PAN2-PAN3) and carbon catabolite repression 4–negative on TATA-less (CCR4-NOT) complexes, thus resulting in mRNA decapping and degradation (Jonas and Izaurralde, 2015; Gebert and Macrae, 2019). Notably, mRNA is usually cleaved by siRNAs and the translation is usually repressed by miRNAs depending on the degree of related complementarity (Figure 1C) (Siomi and Siomi, 2009).
A growing number of direct and indirect evidence has suggested a strong relationship between the deregulation of miRNA and PC. For example, miR-221/222, miR-376a, and miR-301 are overexpressed in pancreatic tumors (Lee et al., 2007; Xu et al., 2015). The overexpression of miR-21 can also be detected in PC cells and miR-21 contributes to cell proliferation, invasion, and chemoresistance (Moriyama et al., 2009). In addition, elevated levels of miR-200a and miR-200b (Li et al., 2010), miR-27a (Ma et al., 2010), miR-17-5p (Yu J. et al., 2010), miR-10b (Nakata et al., 2011), miR-155 (Mikamori et al., 2017), miR-196a and miR-1246 (Xu et al., 2017), miR-501-3p (Yin et al., 2019) can be detected in tumor cells, plasma, exosomes related to PC. However, in some cases, the downregulation of miRNA could also be observed. miR-34a is commonly deactivated in human PC cells and transactivated by p53 tumor suppressor protein (Chang et al., 2007; Raver-Shapira et al., 2007). Downregulation of miR-15a (Zhang et al., and 2010), miR-124 (Wang P. et al., 2014) is also associated with proliferation, progression, and metastasis of PC.
Deregulation of miRNAs can be connected with PC, therefore, most of the articles regarding miRNA in PC focused on the potent diagnostic or prognostic use instead of therapeutic implication. Julia S. Johansen et al. screened 38 microRNAs and identified two diagnostic panels holding approximately 80% accuracy, sensitivity, and specificity (Schultz et al., 2014). The expression of miR-191, -21, and -451a derived from serum exosomal was significantly up-regulated in patients with PC, which was served as early diagnostic and progression markers of PC with an accuracy of 60–80% (Goto et al., 2018). In particular, compared with carcinoembryonic antigen (CEA) and carbohydrate antigen 19-9 (CA19-9) termed asstandard and widely-known biomarkers and the detection of PC, circulating miR-25 could also be a promising biomarker for the diagnosis of early-stage PC owing to its high specificity (Deng et al., 2016). Moreover, a clinical trial related to the detection of microRNA-25 for the diagnosis of PC is already in progress in China (Takakura et al., 2019).
As for therapeutic implications, “miRNA mimics” are double-stranded miRNA with suitable chemical modifications, which are often used for miRNA-based anticancer therapies (Rawat et al., 2019). Pierre Cordelier et al. reported the strong reduction of let-7 microRNA in PC. Restoring let-7 levels strongly suppressed KRAS expression and cell proliferation in Capan-1 cells. However, this could not inhibit the progression of tumor growth (Torrisani et al., 2009). Hsa-microRNA-520h was also proven to target ATP-binding cassette superfamily G member 2 (ABCG2) highly expressed in PC, thus leading to inhibition of cell growth especially migration and invasion in PANC-1 cells (Wang et al., 2010). Furthermore, miR-96 showed the downregulation ability of KRAS, resulting in inhibition of cancer cell and tumor growth both in vitro and in vivo (Yu S. N. et al., 2010). Notably, adenovirus-mediated delivery of miR-143 also exhibited significant inhibition of PANC-1 cancer cells and xenograft tumor growth (Hu et al., 2012). Likewise, restoring miR-150 to the normal level could also dramatically impede the tumor growth of PC cells in a manner of downregulation of MUC4 protein levels (Srivastava et al., 2011).
For miRNAs related to PC whose expression is upregulated, oligonucleotides especially antisense oligonucleotides and small molecule inhibitors were reported. A portfolio of antimirR-21 ASO and gemcitabine adopting scFv-functionalized nanoparticle exerted synergistic antitumor effects on PC cells (Li Y. Q. et al., 2017). Another antimiRNA-199a oligonucleotides adopted novel cell-penetrating peptide-based nanocomplexes as the carrier, which significantly inhibited the differentiation of hPSCs and prevented tumor heterospheroids (Schnittert et al., 2017). Anti-microRNA oligonucleotides encapsulated by human serum albumin-assistant nanosystem (EPOC and cholesterol-related liposomes) showed efficient abolishment of microRNA expression (miR-21, miR-221, miR-222), with a strong and synergistic antitumor effect plus chemotherapeutic drug sunitinib (Passadouro et al., 2014). One small molecule azobenzene has also shown specific and efficient inhibition of miR-21 expression in vitro (Gumireddy et al., 2008).
The carcinogenesis process is always accompanied by the dysregulation of various proteins and this transition requires a high level of mRNA translation. Unlike small molecules and oligonucleotides, mRNA usually consists of hundreds or thousands of nucleotides with a single strand including untranslated regions. Omacetaxine mepesuccinate (homoharringtonine) was approved by the FDA for the treatment of chronic myelogenous leukemia (CML) in 2012. Later, several drugs such as tomivosertib, selinexor, and ribavirin have also entered clinical trials via the mechanism of inhibiting mRNA translation (Pal et al., 2019).
Numerous genetic and epigenetic alterations could be detected within PC and correlate with the dysregulation of mRNA translation. For instance, the transcription factor nuclear factor erythroid-derived 2-like 2 (NRF2) is vital to stimulate the PC initiation and maintain proliferation by regulating mRNA translation, which makes the NRF2 ablation a promising strategy for the treatment of PC (Chio et al., 2016). Insulin-like growth factor 2 mRNA binding protein 2 (IGF2BP2), which is capable of binding to various transcripts and regulating RNA functions, is situated at a high level within PDAC, therefore resulting in a poor prognosis. IGF2BP2 could also promote PDAC progression through the straightforward binding of glucose transporter 1 (GLUT1) mRNA (Huang et al., 2019). The downregulation of BAG3, one of the HSP families, is correlated with poor prognostics in PDAC, which could attribute to the suppression of AGO2-related degradation of IL6 mRNA (Li et al., 2019). The pathogenic mechanism research facilitates the druggable and therapeutic strategy but remains at an extreme earlier stage. Notably, Yoshio Sakai designed one PCR-based detecting strategy to monitor the dysregulated genes related to PDAC with a high sensitivity of more than 70% independent of the stage of PDAC (Sakai et al., 2019).
mRNA vaccines have been effective against multiple cancers but with limited reports against PDAC, the major mechanism for mRNA vaccine is based on the identification of specific tumor antigen, followed by the activation of the immune system to recognize and eliminate cancer cells (Figure 2) (Dorrie et al., 2020). Recently one group has investigated and identified six potential antigens within PDAC patients, which could lay the foundation for the development of anti-PDAC mRNA vaccines (Huang et al., 2021). Another group utilized the combination of antibodies which could specifically bind and neutralize C-C motif chemokine ligand 2 (CCL2) and CCL5 (BisCCL2/5i) and mRNA encoding BisCCL2/5i within a clinical available delivery system. Their results indicated that the BisCCL2/5i mRNA nanoplatforms could dramatically activate sufficient immune response and inhibit cancer growth. Together with PD-1 ligand inhibitor (PD-Li), this combination strategy could achieve significant tumor inhibition and a longer survival time within mouse models (Wang Y. et al., 2021).
FIGURE 2. Typical mechanism of mRNA vaccine. LNP-based mRNA vaccine could induce the formation of peptide-based antigen through mRNA transcription as the blueprint. The produced antigen could stimulate the adaptive immune response to destroy the corresponding pathogen or cancer cells. Created with BioRender (License No: HK23PCSH9W).
Aptamers, usually screened by systematic evolution of ligands by exponential enrichment (SELEX) protocol, contain 20–100 nucleotides with high affinity to the target (Zhou and Rossi, 2017; Rothlisberger and Hollenstein, 2018). The binding affinity largely depends on the three-dimensional structure, including loop, bugle, pseudoknot, G-quadruplex, and kissing hairpin (Keefe et al., 2010; Dunn et al., 2017). Compared with antibodies, aptamers have a much smaller size of 6–30 kDa, preferential penetration, reduced toxicity, lower immunogenicity, and longer shelf life (Table 2) (Zhou and Rossi, 2017; Odeh et al., 2020). At present, eleven aptamers have entered clinical trials, one of which named NOX-A12 was designed for the treatment of metastatic PC (Kaur et al., 2018; Halama et al., 2020). This aptamer targets chemokine ligand 12 (CXCL12), which has been proven to play an important role in PC growth, metastasis, and particular recurrences (De Nigris et al., 2012).
Various aptamers have been investigated or developed upon the antitumor activity. For example, Luigi E. Xodo et al. found a new quadruplex oligonucleotide with TINA modification based on the promoter of the human KRAS gene. This new G-quadruplex motif lifted the Tm value and indicated a significant antiproliferative activity within PANC-1 cancer cells (Cogoi et al., 2009). Another 2′-fluoropyrimidine modified RNA aptamer (P12FR2) designed against human overexpressed pancreatic adenocarcinoma up-regulated factor (PAUF) with a high binding affinity of 77 nM was also reported, which displayed strong inhibition activity in PANC-1 cells as well as up to 60% inhibition of tumor shrinkage via intraperitoneal injection of P12FR2 (Kim et al., 2011). However, in most cases, aptamers are adopted as the specific cargo to form ApDCs to deliver different kinds of drugs to their target. In terms of exerting mechanism, the conjugated drug is supposed to release with the break of a specific linker upon the accumulation into aptamer’s target which is usually highly expressed on the surface of cancer cells (Figure 3).
FIGURE 3. Typical mechanism of ApDC. ApDC could be internalized by a specific receptor which is highly expressed among PC cells. The cytotoxic drugs can be released from cleavable and non-cleavable linkers along with the degradation of the aptamer. Created with BioRender (License No: EQ23P90T7N).
The upregulation of some specific protein receptors is common in PC cells, which enables the specific binding and internalization of aptamer together with the desired cargo as the therapeutic drug (Table 3). Rebekah R. White group developed a nuclease resistant RNA aptamer targeting EGFR for the delivery of gemcitabine-containing polymers into epidermal growth factor receptor (EGFR)-expressing cells, which, as a result, showed strong inhibition of cell proliferation (Ray et al., 2012). Alkaline phosphatase placental-like 2 (ALPPL2) is also a putative biomarker for PC. Dong-ki Lee et al. identified a nuclease-resistant RNA aptamer SQ2 with a specific affinity to ALPPL2 and made use of this aptamer to deliver 5-fluorouracil to exert inhibition of cell proliferation in Capan-1 PC cells (Dua et al., 2015). Another oligonucleotide chimera, composed of an antitransferrin receptor RNA aptamer as the targeting carrier and a DNA decoy oligonucleotide as an NF-κB inhibitor showed increased therapeutic efficacy of Doxorubicin with selectivity in pancreatic tumor cells (Porciani et al., 2015). P19, as another PC-specific RNA aptamer, was also used to conjugate active metabolites of neoadjuvant gemcitabine (dFdCMP) and 5FdU (5FdUMP) into the strand, resulting in over 50% inhibition of cell proliferation in PANC-1 and AsPC-1. The Monomethyl auristatin E (MMAE) drug was further attached to modified P19 aptamer, which significantly increased specific uptake of the aptamer-drug conjugate in tumor cells (Yoon et al., 2016a; Yoon et al., 2017a). Similarly, Monomethyl auristatin F (MMAF) was conjugated to an anti-EGFR aptamer to form E07, which could serve as one alternative in terms of specific delivery into PC cancer cells including PANC-1, MIA PaCa-2, and BxPC-3 (Kratschmer and Levy, 2018). Chung-Hsuan Chen et al. developed Ap52 aptamer targeting tumor-specific MAGE-A3111-125 peptide antigen and further phosphorothioate-modified Ap52 (ThioAp52)-mediated delivery of anticancer drug indicated the ThioAp52-Dox complex has a specific selectivity against PC cells when compared with naked Dox (Wang C. Y. et al., 2021). John J Rossi et al. used blind cell SELEX and successfully developed one P15 aptamer specifically bound to the cell surface vimentin of human pancreatic adenocarcinoma cells. Meanwhile, this P15 aptamer was associated with reduced matrix metallopeptidase 3 (MMP3) expression highly related to tumor cell invasion (Yoon et al., 2017b).
Other P19- and P1-CCAAT/enhancer-binding Protein α (C/EBPα)-saRNA conjugates also indicated strong suppression of PANC-1 cell proliferation and reduced tumor size up to 30% with no observed toxicity in gemcitabine-resistant AsPC-1 cells mouse-xenografts (Yoon et al., 2016b). The C/EBPα-saRNA could significantly inhibit tumor growth in vivo. Furthermore, this C/EBPα-saRNA was conjugated to another TR 14 RNA aptamer targeting transferrin with a highly-potent affinity of 0.01317 nM. Even though the TR 14 RNA aptamer was truncated to only 22 nucleotides (tTR 14) it could show comparable characteristics. The potent anti-tumor activity regarding tumor shrinkage up to the 85% in advanced PDAC mouse model could be observed after administration of tTR 14-C/EBPα-saRNA conjugate supplemented with gemcitabine, while gemcitabine alone could reduce the tumor volume ∼70% (Yoon et al., 2019).
AS1411 is an aptamer targeting nucleolin which is usually overexpressed by PC cancer cells. Won Jun Kang et al. incorporated gemcitabine into AS1411 to form APTA-12, resulting in improved in vitro cytotoxicity and inhibition of tumor growth in Capan-1 tumor-bearing mice (Park et al., 2018). Weihong Tan et al. identified an ssDNA aptamer termed XQ-2d based on cell-SELEX technology and demonstrated the XQ-2d mediated delivery of doxorubicin showed increased uptake by a variety of cancers including PC (Wu et al., 2019).
In addition, nanoparticles are often taken as efficient codelivery vehicles together with aptamers in PC. Dual drugs containing gemcitabine and curcumin conjugated to AS1411 and magnetic PLGA nanoparticles exhibited strong inhibition of cell growth in PANC-1 and MIA PaCa-2 (Sivakumar et al., 2013). Triptolide, as a superior anticancer agent was also conjugated to AS1411 followed by the preparation of AS1411-PEG-PDLLA micelle loading TP (AS-PPT). AS-PPT exhibited more antitumor activity than triptolide and showed increased overall survival in mouse models compared with the clinical anticancer drug Gemzar (Wang et al., 2016). One DNA aptamer termed AP1153 was successfully selected and exhibited a highly potent Kd value of 15 pM to G-protein-coupled cholecystokinin B receptor (CCKBR). AP1153 was further conjugated to the surface of nanoparticles and this delivery system could significantly penetrate pancreatic tumors in animal models (Clawson et al., 2017).
Notably, Chen Jiang et al. conjugated ECM-derived tenascin-C targeting aptamer (GBI-10) to a stroma-permeable cell-penetrating peptide (CPP). In the stroma, tenascin-C can detach and the exposed CPP can facilitate pancreatic tumor penetration endocytosis. The intracellular high redox environment provided the potential to trigger the controlled release of chemotherapy drugs. The in vitro cytotoxicity was relatively mild; however, the in vivo antitumor efficacy was excellent together with decreased systemic toxicity (He et al., 2018).
Up to date, patients with advanced and metastatic PDAC only own a median survival from 6 to 12 months (Conroy et al., 2018). Cytotoxic chemotherapy gemcitabine, together with nab-paclitaxel and FOLFIRINOX make up the standard and prime treatment of PC. However, chemotherapy usually leaves patients suffering from various adverse effects and poor prognoses. Many studies have paid much attention to the development of small molecules inhibitors for PDAC treatments. For example, one tyrosine kinase receptor inhibitor pazopanib showed promising results in a phase II study for patients with advanced, well-differentiated neuroendocrine tumors (NETs) (Phan et al., 2015). However, NETs are scarce (<10%) and require further phase III study. Some other studies have focused on the inhibition of vital signaling pathways related to PDAC, such as focal adhesion kinase (FAK) inhibitor, signal transducer, and activator of transcription 3 (STAT3). Although FAK inhibitor against PDAC has entered clinical trial, drug resistance and a lack of deep understanding of the mechanism could still hinder further development (Jiang et al., 2020). Notably, one group developed one dendrimer nanocarrier to deliver doxorubicin, resulting in better responses against 35 major PC cell lines than free drug, which might offer an insight into the nanosystem-aided therapy (Liu et al., 2021).
The overall progress is usually heavily-limited compared with the patient survival relative to current chemotherapy (Sun et al., 2020). This lack of enhancement could attribute to the superabundant extracellular matrix with desmoplastic fibrotic stroma accounting for 80% of tumor volume, which in turn limits the effective delivery of therapeutical chemotherapy agents into pancreatic tumors and correlates with the poor prognosis (Kota et al., 2017). Thus, in recent years, many researchers have concentrated on the characteristics of the tumor microenvironment especially in the stroma environment and inside cellular communication led by CAFs, PSCs, muscle fibroblasts, and immune cells. Unfortunately, PEGPH20, sonic hedgehog inhibitors and matrix metalloproteinase (MMP) inhibitors targeting and reshaping the tumor stroma microenvironment followed by the drug administration have shown limited clinical efficacy (Vennin et al., 2018; Ho et al., 2020). Recently, targeting Pin1 has been demonstrated to completely eliminate or sustain remissions of aggressive PDAC in diverse model systems by firstly remodeling the original tumor microenvironment into a less desmoplastic and immune responsive tumor microenvironment followed by antiPD-1 and gemcitabine therapy (Koikawa et al., 2021). On the other hand, current preclinical models of PC are poor and could not reflect reality, which usually leads to failure in clinical studies. Reducing stromal collagen and hyaluronan production and targeting the vitamin D receptors overexpressed in the stroma to facilitate the chemotherapy administration were also blocked by the same limitation. In this way, the desmoplastic fibrotic stroma within the tumor microenvironment is one unavoidable obstacle that remains to be settled and should be considered as a priority when confronted with the drug discovery of PC.
Compared with conventional drugs, nucleic acid drugs afford a versatile approach to treat a wider range of diseases from the underlying causes. The delivery system is vital in enhancing the druggability of nucleic acid drugs and there are already some clinical examples based on N-acetylgalactosamine (GalNAc) conjugation, lipid nanoparticles (LNPs), and adeno-associated virus (AAV) vector systems (Kulkarni et al., 2021; Van Den Berg et al., 2021; Vargason et al., 2021). Unfortunately, there is no clinically available therapeutic RNAi for PC up to date. The point mutations of the KRAS gene could be detected in over 90% of human PCs and the introduction of anti-KRAS ASO and siRNA have shown ideal knock-out efficiency and tumor inhibition in orthotopic mouse models, but few clinical trials could be identified to give fundamental support for the treatment of PC. ASOs targeting cell proliferation factors including XIAP, protein Kinase A (PKA), cell signaling (HRAS), resistance to chemotherapy (Hsp27), and cancer stroma (TGFβ2) all failed to show antitumor effects in clinical trials (Yamakawa et al., 2019). Some other siRNAs targeting mutated KRAS in combination with gemcitabine treatment showed potential efficacy in PC patients in early-phase trials but no more progress has been reported (Yamakawa et al., 2019). For the FDA-approved siRNA/ASO drugs, all candidates are used for rare disease treatment and most of them are highly modified because of the highly-charged characteristic and the serum instability. The utility of highly optimized chemical modifications, conjugation to multiple ligands, and nanoparticle carriers significantly have improved the delivery efficiency. Nevertheless, due to the sophisticated tumor microenvironment and the desmoplastic fibrotic stroma of PC, it becomes much more complex to efficiently deliver RNAi therapeutics into the pancreatic tumors. Therefore, the selection and development of best-fit chemical modifications, and delivery systems capable of overcoming the inherent shortcomings and potential targets for RNAi within PC and even stroma largely contribute to the relevant drug discovery (Vetvicka et al., 2021). mRNA vaccines and translation inhibitors as well as therapeutical vaccines represent novel and promising strategies against PC, but more preclinical and clinical evidence necessary for the drug discovery of PC are still required.
Compared with antibodies, aptamers have a smaller molecular weight but own considerable affinity owing to the complex tertiary folded structures, which can be chemically synthesized and modified with more availability and flexibility enabling the long shelf life and low immunogenicity characteristics favorable for clinical implications and precision medicine. Since the first approval of aptamer drug pegaptanib for the treatment of wet-age-related macular degeneration, an increasing number of aptamer therapeutics for oncology are in clinical trials (Morita et al., 2018). ApDCs, as one combination of aptamers and cytotoxic molecules, have shown promising results in preclinical models against PC. It has been almost one decade since the first model of the aptamer-drug conjugates or chimeras while ADCs have already entered third generations over two decades since the first approval of Mylotarg (Beck et al., 2017; Xuan et al., 2018). The overall drug research and development of ApDCs have largely lagged behind ADCs and no ApDCs have gone far to clinical trials. The screening and discovery of new high-affinity and specificity targeting aptamers towards PC, together with the combination of reshaping or remodeling the pancreatic tumor microenvironment, might hold promise for the future treatment of PC.
HD wrote the manuscript. RA, YM, and XW helped in revising the manuscript. FL, AL, YM, and GZ supervised the preparation of the manuscript. All authors contributed to the article and approved the submitted version.
This study was supported by the Guangdong Basic and Applied Basic Research Foundation (2020A1515110630), the Hong Kong General Research Fund (HKBU 12114416, HKBU 12101117, HKBU 12100918, HKBU 12101018, HKBU 12103519, and HKBU 14100218), the National Key R&D Program of China (2018YFA0800804).
Authors FL, YM, AL, GZ were employed by the “Institute of Precision Medicine and Innovative Drug Discovery”.
The remaining authors declare that the research was conducted in the absence of any commercial or financial relationships that could be construed as a potential conflict of interest.
All claims expressed in this article are solely those of the authors and do not necessarily represent those of their affiliated organizations, or those of the publisher, the editors, and the reviewers. Any product that may be evaluated in this article, or claim that may be made by its manufacturer, is not guaranteed or endorsed by the publisher.
Adams, D., Gonzalez-Duarte, A., O’Riordan, W. D., Yang, C.-C., Ueda, M., and Kristen, A. V. (2018). Patisiran, an RNAi Therapeutic, for Hereditary Transthyretin Amyloidosis. N. Engl. J. Med. 379 (1), 11–21. doi:10.1056/NEJMoa1716153
Baylot, V., Andrieu, C., Katsogiannou, M., Taieb, D., Garcia, S., Giusiano, S., et al. (2011). OGX-427 Inhibits Tumor Progression and Enhances Gemcitabine Chemotherapy in Pancreatic Cancer. Cell Death Dis 2, e221. doi:10.1038/cddis.2011.104
Beck, A., Goetsch, L., Dumontet, C., and Corvaia, N. (2017). Strategies and Challenges for the Next Generation of Antibody Drug Conjugates. Nat. Rev. Drug Discov. 16 (5), 315–337. doi:10.1038/nrd.2016.268
Bennett, C. F., Baker, B. F., Pham, N., Swayze, E., and Geary, R. S. (2017). Pharmacology of Antisense Drugs. Annu. Rev. Pharmacol. Toxicol. 57 (1), 81–105. doi:10.1146/annurev-pharmtox-010716-104846
Cao, L. P., Song, J. L., Yi, X. P., and Li, Y. X. (2013). Double Inhibition of NF-Κb and XIAP via RNAi Enhances the Sensitivity of Pancreatic Cancer Cells to Gemcitabine. Oncol. Rep. 29 (4), 1659–1665. doi:10.3892/or.2013.2246
Carpizo, D., Tsang, A., Yu, X., Yi, L., Dudgeon, C., Donohue, K., et al. (2016). Therapeutic Suppression of the KRAS-MYC Oncogenic axis in Human Pancreatic Cancer Xenografts with U1 Adaptor Oligonucleotide/RGD Peptide Conjugates. Mol. Ther. 24, S104. doi:10.1016/s1525-0016(16)33071-4
Castel, S. E., and Martienssen, R. A. (2013). RNA Interference in the Nucleus: Roles for Small RNAs in Transcription, Epigenetics and beyond. Nat. Rev. Genet. 14 (2), 100–112. doi:10.1038/nrg3355
Chang, T. C., Wentzel, E. A., Kent, O. A., Ramachandran, K., Mullendore, M., Lee, K. H., et al. (2007). Transactivation of miR-34a by P53 Broadly Influences Gene Expression and Promotes Apoptosis. Mol. Cel 26 (5), 745–752. doi:10.1016/j.molcel.2007.05.010
Chio, I. I. C., Jafarnejad, S. M., Ponz-Sarvise, M., Park, Y., Rivera, K., Palm, W., et al. (2016). NRF2 Promotes Tumor Maintenance by Modulating mRNA Translation in Pancreatic Cancer. Cell 166 (4), 963–976. doi:10.1016/j.cell.2016.06.056
Clawson, G. A., Abraham, T., Pan, W. H., Tang, X. M., Linton, S. S., Mcgovern, C. O., et al. (2017). A Cholecystokinin B Receptor-specific DNA Aptamer for Targeting Pancreatic Ductal Adenocarcinoma. Nucleic Acid Ther. 27 (1), 23–35. doi:10.1089/nat.2016.0621
Cogoi, S., Paramasivam, M., Filichev, V., Geci, I., Pedersen, E. B., and Xodo, L. E. (2009). Identification of a New G-Quadruplex Motif in the KRAS Promoter and Design of Pyrene-Modified G4-Decoys with Antiproliferative Activity in Pancreatic Cancer Cells. J. Med. Chem. 52 (2), 564–568. doi:10.1021/jm800874t
Conroy, T., Hammel, P., Hebbar, M., Ben Abdelghani, M., Wei, A. C., Raoul, J. L., et al. (2018). FOLFIRINOX or Gemcitabine as Adjuvant Therapy for Pancreatic Cancer. N. Engl. J. Med. 379 (25), 2395–2406. doi:10.1056/NEJMoa1809775
Crooke, S. T., Wang, S. Y., Vickers, T. A., Shen, W., and Liang, X. H. (2017). Cellular Uptake and Trafficking of Antisense Oligonucleotides. Nat. Biotechnol. 35 (3), 230–237. doi:10.1038/nbt.3779
De Fougerolles, A., Vornlocher, H. P., Maraganore, J., and Lieberman, J. (2007). Interfering with Disease: a Progress Report on siRNA-Based Therapeutics. Nat. Rev. Drug Discov. 6 (6), 443–453. doi:10.1038/nrd2310
De Nigris, F., Schiano, C., Infante, T., and Napoli, C. (2012). CXCR4 Inhibitors: Tumor Vasculature and Therapeutic Challenges. Recent Pat. Anticancer Drug Discov. 7 (3), 251–264. doi:10.2174/157489212801820039
Deng, T., Yuan, Y. Z., Zhang, C. N., Zhang, C. Y., Yao, W. Y., Wang, C., et al. (2016). Identification of Circulating MiR-25 as a Potential Biomarker for Pancreatic Cancer Diagnosis. Cell. Physiol. Biochem. 39 (5), 1716–1722. doi:10.1159/000447872
Dong, H. F., Lei, J. P., Ding, L., Wen, Y. Q., Ju, H. X., and Zhang, X. J. (2013). MicroRNA: Function, Detection, and Bioanalysis. Chem. Rev. 113 (8), 6207–6233. doi:10.1021/cr300362f
Dorrie, J., Schaft, N., Schuler, G., and Schuler-Thurner, B. (2020). Therapeutic Cancer Vaccination with Ex Vivo RNA-Transfected Dendritic Cells-An Update. Pharmaceutics 12 (2), 92–115. doi:10.3390/pharmaceutics12020092
Dua, P., Sajeesh, S., Kim, S., and Lee, D. K. (2015). ALPPL2 Aptamer-Mediated Targeted Delivery of 5-Fluoro-2 '-Deoxyuridine to Pancreatic Cancer. Nucleic Acid Ther. 25 (4), 180–187. doi:10.1089/nat.2014.0516
Dunn, M. R., Jimenez, R. M., and Chaput, J. C. (2017). Analysis of Aptamer Discovery and Technology. Nat. Rev. Chem. 1 (10), s41570-017-0076. doi:10.1038/s41570-017-0076
Ellermeier, J., Wei, J. W., Duewell, P., Hoves, S., Stieg, M. R., Adunka, T., et al. (2013). Therapeutic Efficacy of Bifunctional siRNA Combining TGF-Β1 Silencing with RIG-I Activation in Pancreatic Cancer. Cancer Res. 73 (6), 1709–1720. doi:10.1158/0008-5472.CAN-11-3850
Gebert, L. F. R., and Macrae, I. J. (2019). Regulation of microRNA Function in Animals. Nat. Rev. Mol. Cel Biol. 20 (1), 21–37. doi:10.1038/s41580-018-0045-7
Goto, T., Fujiya, M., Konishi, H., Sasajima, J., Fujibayashi, S., Hayashi, A., et al. (2018). An Elevated Expression of Serum Exosomal microRNA-191,-21,-451a of Pancreatic Neoplasm Is Considered to Be Efficient Diagnostic Marker. BMC Cancer 18, 116–127. doi:10.1186/s12885-018-4006-5
Gumireddy, K., Young, D. D., Xiong, X., Hogenesch, J. B., Huang, Q. H., and Deiters, A. (2008). Small-molecule Inhibitors of microRNA miR-21 Function. Angew. Chem. Int. Ed. 47 (39), 7482–7484. doi:10.1002/anie.200801555
Guo, S., Li, K., Hu, B., Li, C. H., Zhang, M. J., Hussain, A., et al. (2021). Membrane-destabilizing Ionizable Lipid Empowered Imaging-Guided siRNA Delivery and Cancer Treatment. Exploration 1 (1), 35–49. doi:10.1002/exp.20210008
Halama, N., Williams, A., Suarez-Carmona, M., Schreiber, J., Hohmann, N., Pruefer, U., et al. (2020). Phase I/II Study with CXCL12 Inhibitor NOX-A12 and Pembrolizumab in Patients with Microsatellite-Stable, Metastatic Colorectal or Pancreatic Cancer. Ann. Oncol. 31, S944. doi:10.1016/j.annonc.2020.08.2020
Han, X. X., Li, Y. Y., Xu, Y., Zhao, X., Zhang, Y. L., Yang, X., et al. (2018). Reversal of Pancreatic Desmoplasia by Re-educating Stellate Cells with a Tumour Microenvironment-Activated Nanosystem. Nat. Commun. 9, 3390. doi:10.1038/s41467-018-05906-x
Hayes, J., Peruzzi, P. P., and Lawler, S. (2014). MicroRNAs in Cancer: Biomarkers, Functions and Therapy. Trends Mol. Med. 20 (8), 460–469. doi:10.1016/j.molmed.2014.06.005
He, X., Chen, X. L., Liu, L. S., Zhang, Y., Lu, Y. F., Zhang, Y. J., et al. (2018). Sequentially Triggered Nanoparticles with Tumor Penetration and Intelligent Drug Release for Pancreatic Cancer Therapy. Adv. Sci. 5 (5), 1701070. doi:10.1002/advs.201701070
Heider, T. R., Lyman, S., Schoonhoven, R., and Behrns, K. E. (2007). Ski Promotes Tumor Growth through Abrogation of Transforming Growth Factor-Beta Signaling in Pancreatic Cancer. Ann. Surg. 246 (1), 61–68. doi:10.1097/SLA.0b013e318070cafa
Helmut, O., Seufferlein, T., Luger, T., Schmid, R., Von Wichert, G., Endlicher, E., et al. (2012). Final Results of a Phase I/II Study in Patients with Pancreatic Cancer, Malignant Melanoma, and Colorectal Carcinoma with Trabedersen. J. Clin. Oncol. 30, 4034. doi:10.1200/jco.2012.30.15_suppl.4034
Hidalgo, M. (2010). Pancreatic Cancer. N. Engl. J. Med. 362 (17), 1605–1617. doi:10.1056/NEJMra0901557
Ho, W. J., Jaffee, E. M., and Zheng, L. (2020). The Tumour Microenvironment in Pancreatic Cancer - Clinical Challenges and Opportunities. Nat. Rev. Clin. Oncol. 17 (9), 527–540. doi:10.1038/s41571-020-0363-5
Hotz, H. G., Hines, O. J., Masood, R., Hotz, B., Foitzik, T., Buhr, H. J., et al. (2005). VEGF Antisense Therapy Inhibits Tumor Growth and Improves Survival in Experimental Pancreatic Cancer. Surgery 137 (2), 192–199. doi:10.1016/j.surg.2004.07.015
Hu, Y. J., Ou, Y. L., Wu, K. M., Chen, Y. X., and Sun, W. J. (2012). miR-143 Inhibits the Metastasis of Pancreatic Cancer and an Associated Signaling Pathway. Tumor Biol. 33 (6), 1863–1870. doi:10.1007/s13277-012-0446-8
Huang, S., Wu, Z., Cheng, Y. Y., Wei, W. Z., and Hao, L. L. (2019). Insulin-like Growth Factor 2 mRNA Binding Protein 2 Promotes Aerobic Glycolysis and Cell Proliferation in Pancreatic Ductal Adenocarcinoma via Stabilizing GLUT1 mRNA. Acta Biochim. Biophys. Sin. 51 (7), 743–752. doi:10.1093/abbs/gmz048
Huang, X., Zhang, G., Tang, T. Y., and Liang, T. B. (2021). Identification of Tumor Antigens and Immune Subtypes of Pancreatic Adenocarcinoma for mRNA Vaccine Development. Mol. Cancer 20 (1), 44–62. doi:10.1186/s12943-021-01310-0
Jansson, M. D., and Lund, A. H. (2012). MicroRNA and Cancer. Mol. Oncol. 6 (6), 590–610. doi:10.1016/j.molonc.2012.09.006
Jiang, H., Liu, X. T., Knolhoff, B. L., Hegde, S., Lee, K. B., Jiang, H. M., et al. (2020). Development of Resistance to FAK Inhibition in Pancreatic Cancer Is Linked to Stromal Depletion. Gut 69 (1), 122–132. doi:10.1136/gutjnl-2018-317424
Jonas, S., and Izaurralde, E. (2015). NON-CODING RNA towards a Molecular Understanding of microRNA-Mediated Gene Silencing. Nat. Rev. Genet. 16 (7), 421–433. doi:10.1038/nrg3965
Kami, K., Doi, R., Koizumi, M., Toyoda, E., Mori, T., Ito, D., et al. (2005). Downregulation of Survivin by siRNA Diminishes Radioresistance of Pancreatic Cancer Cells. Surgery 138 (2), 299–305. doi:10.1016/j.surg.2005.05.009
Kanasty, R., Dorkin, J. R., Vegas, A., and Anderson, D. (2013). Delivery Materials for siRNA Therapeutics. Nat. Mater. 12 (11), 967–977. doi:10.1038/nmat3765
Kaur, H., Bruno, J. G., Kumar, A., and Sharma, T. K. (2018). Aptamers in the Therapeutics and Diagnostics Pipelines. Theranostics 8 (15), 4016–4032. doi:10.7150/thno.25958
Keefe, A. D., Pai, S., and Ellington, A. (2010). Aptamers as Therapeutics. Nat. Rev. Drug Discov. 9 (7), 537–550. doi:10.1038/nrd3141
Kim, H. J., Kim, A., Miyata, K., and Kataoka, K. (2016). Recent Progress in Development of siRNA Delivery Vehicles for Cancer Therapy. Adv. Drug Del. Rev. 104, 61–77. doi:10.1016/j.addr.2016.06.011
Kim, Y. H., Sung, H. J., Kim, S., Kim, E. O., Lee, J. W., Moon, J. Y., et al. (2011). An RNA Aptamer that Specifically Binds Pancreatic Adenocarcinoma Up-Regulated Factor Inhibits Migration and Growth of Pancreatic Cancer Cells. Cancer Lett. 313 (1), 76–83. doi:10.1016/j.canlet.2011.08.027
Kleeff, J., Korc, M., Apte, M., La Vecchia, C., Johnson, C. D., Biankin, A. V., et al. (2016). Pancreatic Cancer. Nat. Rev. Dis. Primers 2, 16022. doi:10.1038/nrdp.2016.22
Koikawa, K., Kibe, S., Suizu, F., Sekino, N., Kim, N., Manz, T. D., et al. (2021). Targeting Pin1 Renders Pancreatic Cancer Eradicable by Synergizing with Immunochemotherapy. Cell 184 (18), 4753–4771. doi:10.1016/j.cell.2021.07.020
Kota, J., Hancock, J., Kwon, J., and Korc, M. (2017). Pancreatic Cancer: Stroma and its Current and Emerging Targeted Therapies. Cancer Lett. 391, 38–49. doi:10.1016/j.canlet.2016.12.035
Kratschmer, C., and Levy, M. (2018). Targeted Delivery of Auristatin-Modified Toxins to Pancreatic Cancer Using Aptamers. Mol. Ther. Nucleic Acids 10, 227–236. doi:10.1016/j.omtn.2017.11.013
Kubler, H., Scheel, B., Gnad-Vogt, U., Miller, K., Schultze-Seemann, W., Vom Dorp, F., et al. (2015). Self-adjuvanted mRNA Vaccination in Advanced Prostate Cancer Patients: a First-In-Man Phase I/IIa Study. J. Immunother. Cancer 3, 26. doi:10.1186/s40425-015-0068-y
Kulkarni, J. A., Witzigmann, D., Thomson, S. B., Chen, S., Leavitt, B. R., Cullis, P. R., et al. (2021). The Current Landscape of Nucleic Acid Therapeutics. Nat. Nanotechnol. 16 (6), 630–643. doi:10.1038/s41565-021-00898-0
Lee, E. J., Gusev, Y., Jiang, J. M., Nuovo, G. J., Lerner, M. R., Frankel, W. L., et al. (2007). Expression Profiling Identifies microRNA Signature in Pancreatic Cancer. Int. J. Cancer 120 (5), 1046–1054. doi:10.1002/ijc.22394
Lei, Y. F., Tang, L. X., Xie, Y. Z. Y., Xianyu, Y. L., Zhang, L. M., Wang, P., et al. (2017). Gold Nanoclusters-Assisted Delivery of NGF siRNA for Effective Treatment of Pancreatic Cancer. Nat. Commun. 8, 15130. doi:10.1038/ncomms15130
Leroux, C., and Konstantinidou, G. (2021). Targeted Therapies for Pancreatic Cancer: Overview of Current Treatments and New Opportunities for Personalized Oncology. Cancers (Basel) 13 (4), 799–828. doi:10.3390/cancers13040799
Li, A., Omura, N., Hong, S. M., Vincent, A., Walter, K., Griffith, M., et al. (2010). Pancreatic Cancers Epigenetically Silence SIP1 and Hypomethylate and Overexpress miR-200a/200b in Association with Elevated Circulating miR-200a and miR-200b Levels. Cancer Res. 70 (13), 5226–5237. doi:10.1158/0008-5472.Can-09-4227
Li, C., An, M. X., Jiang, J. Y., Yao, H. B., Li, S., Yan, J., et al. (2019). BAG3 Suppresses Loading of Ago2 to IL6 mRNA in Pancreatic Ductal Adenocarcinoma. Front. Oncol. 9, 225. doi:10.3389/fonc.2019.00225
Li, F. F., Lu, J., Liu, J., Liang, C., Wang, M. L., Wang, L. Y., et al. (2017). A Water-Soluble Nucleolin Aptamer-Paclitaxel Conjugate for Tumor-specific Targeting in Ovarian Cancer. Nat. Commun. 8, 1390. doi:10.1038/s41467-017-01565-6
Li, M., Li, M. M., Yang, Y. L., Liu, Y. K., Xie, H. B., Yu, Q. W., et al. (2020). Remodeling Tumor Immune Microenvironment via Targeted Blockade of PI3K-Gamma and CSF-1/CSF-1R Pathways in Tumor Associated Macrophages for Pancreatic Cancer Therapy. J. Control Release 321, 23–35. doi:10.1016/j.jconrel.2020.02.011
Li, Y. Q., Chen, Y. T., Li, J. J., Zhang, Z. Q., Huang, C. M., Lian, G. D., et al. (2017). Co-delivery of microRNA-21 Antisense Oligonucleotides and Gemcitabine Using Nanomedicine for Pancreatic Cancer Therapy. Cancer Sci. 108 (7), 1493–1503. doi:10.1111/cas.13267
Lin, G. M., Chen, C. K., Yin, F., Yang, C. B., Tian, J. L., Chen, T., et al. (2017). Biodegradable Nanoparticles as siRNA Carriers for In Vivo Gene Silencing and Pancreatic Cancer Therapy. J. Mater. Chem. B 5 (18), 3327–3337. doi:10.1039/c6tb03116a
Liu, D. P., Zhang, Y., Dang, C. X., Ma, Q. Y., Lee, W., and Chen, W. C. (2007). siRNA Directed against TrkA Sensitizes Human Pancreatic Cancer Cells to Apoptosis Induced by Gemcitabine through an Inactivation of PI3K/Akt-dependent Pathway. Oncol. Rep. 18 (3), 673–677. doi:10.3892/or.18.3.673
Liu, J., Chen, C., Wei, T., Gayet, O., Loncle, C., Borge, L., et al. (2021). Dendrimeric Nanosystem Consistently Circumvents Heterogeneous Drug Response and Resistance in Pancreatic Cancer. Exploration 1 (1), 21–34. doi:10.1002/exp.20210003
Longo, V., Brunetti, O., Gnoni, A., Cascinu, S., Gasparini, G., Lorusso, V., et al. (2016). Angiogenesis in Pancreatic Ductal Adenocarcinoma: a Controversial Issue. Oncotarget 7 (36), 58649–58658. doi:10.18632/oncotarget.10765
Lundin, K. E., Gissberg, O., and Smith, C. I. E. (2015). Oligonucleotide Therapies: the Past and the Present. Hum. Gene Ther. 26 (8), 475–485. doi:10.1089/hum.2015.070
Ma, Y. H., Yu, S. N., Zhao, W. G., Lu, Z. H., and Chen, J. (2010). miR-27a Regulates the Growth, colony Formation and Migration of Pancreatic Cancer Cells by Targeting Sprouty2. Cancer Lett. 298 (2), 150–158. doi:10.1016/j.canlet.2010.06.012
Mahadevan, D., Chalasani, P., Rensvold, D., Kurtin, S., Pretzinger, C., Jolivet, J., et al. (2013). Phase I Trial of AEG35156 an Antisense Oligonucleotide to XIAP Plus Gemcitabine in Patients with Metastatic Pancreatic Ductal Adenocarcinoma. Am. J. Clin. Oncol. 36 (3), 239–243. doi:10.1097/COC.0b013e3182467a13
Masui, T., Hosotani, R., Ito, D., Kami, K., Koizumi, M., Mori, T., et al. (2006). Bcl-XL Antisense Oligonucleotides Coupled with Antennapedia Enhances Radiation-Induced Apoptosis in Pancreatic Cancer. Surgery 140 (2), 149–160. doi:10.1016/j.surg.2006.03.014
Matsuda, Y., Ishiwata, T., Yoshimura, H., Yamashita, S., Ushijima, T., and Arai, T. (2016). Systemic Administration of Small Interfering RNA Targeting Human Nestin Inhibits Pancreatic Cancer Cell Proliferation and Metastasis. Pancreas 45 (1), 93–100. doi:10.1097/mpa.0000000000000427
Mcguigan, A., Kelly, P., Turkington, R. C., Jones, C., Coleman, H. G., and Mccain, R. S. (2018). Pancreatic Cancer: A Review of Clinical Diagnosis, Epidemiology, Treatment and Outcomes. World J. Gastroenterol. 24 (43), 4846–4861. doi:10.3748/wjg.v24.i43.4846
Mikamori, M., Yamada, D., Eguchi, H., Hasegawa, S., Kishimoto, T., Tomimaru, Y., et al. (2017). MicroRNA-155 Controls Exosome Synthesis and Promotes Gemcitabine Resistance in Pancreatic Ductal Adenocarcinoma. Sci. Rep. 7, 42339–42353. doi:10.1038/srep42339
Morioka, C. Y., Machado, M. C., Saito, S., Nakada, Y., Matheus, A. S., Jukemura, J., et al. (2005). Suppression of Invasion of a Hamster Pancreatic Cancer Cell Line by Antisense Oligonucleotides Mutation-Matched to K-Ras Gene. In Vivo 19 (3), 535–538.
Morita, Y., Leslie, M., Kameyama, H., Volk, D. E., and Tanaka, T. (2018). Aptamer Therapeutics in Cancer: Current and Future. Cancers (Basel) 10 (3), 80–102. doi:10.3390/cancers10030080
Moriyama, T., Ohuchida, K., Mizumoto, K., Yu, J., Sato, N., Nabae, T., et al. (2009). MicroRNA-21 Modulates Biological Functions of Pancreatic Cancer Cells Including Their Proliferation, Invasion, and Chemoresistance. Mol. Cancer Ther. 8 (5), 1067–1074. doi:10.1158/1535-7163.Mct-08-0592
Nakada, Y., Saito, S., Ohzawa, K., Morioka, C. Y., Kita, K., Minemura, M., et al. (2001). Antisense Oligonucleotides Specific to Mutated K-Ras Genes Inhibit Invasiveness of Human Pancreatic Cancer Cell Lines. Pancreatology 1 (4), 314–319. doi:10.1159/000055830
Nakata, K., Ohuchida, K., Mizumoto, K., Kayashima, T., Ikenaga, N., Sakai, H., et al. (2011). MicroRNA-10b Is Overexpressed in Pancreatic Cancer, Promotes its Invasiveness, and Correlates with a Poor Prognosis. Surgery 150 (5), 916–922. doi:10.1016/j.surg.2011.06.017
Ocker, M., Neureiter, D., Lueders, M., Zopf, S., Ganslmayer, M., Hahn, E. G., et al. (2005). Variants of Bcl-2 Specific siRNA for Silencing Antiapoptotic Bcl-2 in Pancreatic Cancer. Gut 54 (9), 1298–1308. doi:10.1136/gut.2004.056192
Odeh, F., Nsairat, H., Alshaer, W., Ismail, M. A., Esawi, E., Qaqish, B., et al. (2020). Aptamers Chemistry: Chemical Modifications and Conjugation Strategies. Molecules 25 (1), 3–54. doi:10.3390/molecules25010003
Pal, I., Safari, M., Jovanovic, M., Bates, S. E., and Deng, C. C. (2019). Targeting Translation of mRNA as a Therapeutic Strategy in Cancer. Curr. Hematol. Malig. Rep. 14 (4), 219–227. doi:10.1007/s11899-019-00530-y
Park, J. Y., Cho, Y. L., Chae, J. R., Moon, S. H., Cho, W. G., Choi, Y. J., et al. (2018). Gemcitabine-incorporated G-Quadruplex Aptamer for Targeted Drug Delivery into Pancreas Cancer. Mol. Ther. Nucleic Acids 12, 543–553. doi:10.1016/j.omtn.2018.06.003
Passadouro, M., De Lima, M. C. P., and Faneca, H. (2014). MicroRNA Modulation Combined with Sunitinib as a Novel Therapeutic Strategy for Pancreatic Cancer. Int. J. Nanomedicine 9, 3203–3217. doi:10.2147/ijn.S64456
Phan, A. T., Halperin, D. M., Chan, J. A., Fogelman, D. R., Hess, K. R., Malinowski, P., et al. (2015). Pazopanib and Depot Octreotide in Advanced, Well-Differentiated Neuroendocrine Tumours: a Multicentre, Single-Group, Phase 2 Study. Lancet Oncol. 16 (6), 695–703. doi:10.1016/s1470-2045(15)70136-1
Porciani, D., Tedeschi, L., Marchetti, L., Citti, L., Piazza, V., Beltram, F., et al. (2015). Aptamer-mediated Codelivery of Doxorubicin and NF-Kappa B Decoy Enhances Chemosensitivity of Pancreatic Tumor Cells. Mol. Ther. Nucleic Acids 4, e235. doi:10.1038/mtna.2015.9
Raver-Shapira, N., Marciano, E., Meiri, E., Spector, Y., Rosenfeld, N., Moskovits, N., et al. (2007). Transcriptional Activation of miR-34a Contributes to P53-Mediated Apoptosis. Mol. Cel 26 (5), 731–743. doi:10.1016/j.molcel.2007.05.017
Rawat, M., Kadian, K., Gupta, Y., Kumar, A., Chain, P. S. G., Kovbasnjuk, O., et al. (2019). MicroRNA in Pancreatic Cancer: from Biology to Therapeutic Potential. Genes 10 (10), 753–775. doi:10.3390/genes10100752
Ray, P., Cheek, M. A., Sharaf, M. L., Li, N., Ellington, A. D., Sullenger, B. A., et al. (2012). Aptamer-mediated Delivery of Chemotherapy to Pancreatic Cancer Cells. Nucleic Acid Ther. 22 (5), 295–305. doi:10.1089/nat.2012.0353
Rejiba, S., Wack, S., Aprahamian, M., and Hajri, A. (2007). K-ras Oncogene Silencing Strategy Reduces Tumor Growth and Enhances Gemcitabine Chemotherapy Efficacy for Pancreatic Cancer Treatment. Cancer Sci. 98 (7), 1128–1136. doi:10.1111/j.1349-7006.2007.00506.x
Roberts, T. C., Langer, R., and Wood, M. J. A. (2020). Advances in Oligonucleotide Drug Delivery. Nat. Rev. Drug Discov. 19 (10), 673–694. doi:10.1038/s41573-020-0075-7
Rothlisberger, P., and Hollenstein, M. (2018). Aptamer Chemistry. Adv. Drug Del. Rev. 134, 3–21. doi:10.1016/j.addr.2018.04.007
Sakai, Y., Honda, M., Matsui, S., Komori, O., Murayama, T., Fujiwara, T., et al. (2019). Development of Novel Diagnostic System for Pancreatic Cancer, Including Early Stages, Measuring mRNA of Whole Blood Cells. Cancer Sci. 110 (4), 1364–1388. doi:10.1111/cas.13971
Schlingensiepen, K. H., Jaschinski, F., Lang, S. A., Moser, C., Geissler, E. K., Schlitt, H. J., et al. (2011). Transforming Growth Factor-Beta 2 Gene Silencing with Trabedersen (AP 12009) in Pancreatic Cancer. Cancer Sci. 102 (6), 1193–1200. doi:10.1111/j.1349-7006.2011.01917.x
Schnittert, J., Kuninty, P. R., Bystry, T. F., Brock, R., Storm, G., and Prakash, J. (2017). Anti-microRNA Targeting Using Peptide-Based Nanocomplexes to Inhibit Differentiation of Human Pancreatic Stellate Cells. Nanomedicine 12 (12), 1369–1384. doi:10.2217/nnm-2017-0054
Schultz, N. A., Dehlendorff, C., Jensen, B. V., Bjerregaard, J. K., Nielsen, K. R., Bojesen, S. E., et al. (2014). MicroRNA Biomarkers in Whole Blood for Detection of Pancreatic Cancer. JAMA 311 (4), 392–404. doi:10.1001/jama.2013.284664
Scott, L. J. (2020). Givosiran: First Approval. Drugs 80 (3), 335–339. doi:10.1007/s40265-020-01269-0
Shen, X. L., and Corey, D. R. (2018). Chemistry, Mechanism and Clinical Status of Antisense Oligonucleotides and Duplex RNAs. Nucleic Acids Res. 46 (4), 1584–1600. doi:10.1093/nar/gkx1239
Siegel, R. L., Miller, K. D., and Jemal, A. (2020). Cancer Statistics, 2020. CA Cancer J. Clin. 70 (1), 7–30. doi:10.3322/caac.21590
Siomi, H., and Siomi, M. C. (2009). On the Road to reading the RNA-Interference Code. Nature 457 (7228), 396–404. doi:10.1038/nature07754
Sivakumar, B., Aswathy, R. G., Nagaoka, Y., Iwai, S., Venugopal, K., Kato, K., et al. (2013). Aptamer Conjugated Theragnostic Multifunctional Magnetic Nanoparticles as a Nanoplatform for Pancreatic Cancer Therapy. RSC Adv. 3 (43), 20579–20598. doi:10.1039/c3ra42645a
Srivastava, S. K., Bhardwaj, A., Singh, S., Arora, S., Wang, B., Grizzle, W. E., et al. (2011). MicroRNA-150 Directly Targets MUC4 and Suppresses Growth and Malignant Behavior of Pancreatic Cancer Cells. Carcinogenesis 32 (12), 1832–1839. doi:10.1093/carcin/bgr223
Stauder, G., Bischof, A., Egger, T., Hafner, M., Herrmuth, H., Jachimczak, P., et al. (2004). TGF-beta 2 Suppression by the Antisense Oligonucleotide AP 12009 as Treatment for Pancreatic Cancer: Preclinical Efficacy Data. J. Clin. Oncol. 22 (14), 4106. doi:10.1200/jco.2004.22.90140.4106
Sullenger, B. A., and Nair, S. (2016). From the RNA World to the Clinic. Science 352 (6292), 1417–1420. doi:10.1126/science.aad8709
Sun, J. F., Russell, C. C., Scarlett, C. J., and Mccluskey, A. (2020). Small Molecule Inhibitors in Pancreatic Cancer. RSC Med. Chem. 11 (2), 164–183. doi:10.1039/c9md00447e
Takakura, K., Kawamura, A., Torisu, Y., Koido, S., Yahagi, N., and Saruta, M. (2019). The Clinical Potential of Oligonucleotide Therapeutics against Pancreatic Cancer. Int. J. Mol. Sci. 20 (13), 3331–3348. doi:10.3390/ijms20133331
Tatiparti, K., Sau, S., Kashaw, S. K., and Iyer, A. K. (2017). siRNA Delivery Strategies: a Comprehensive Review of Recent Developments. Nanomaterials 7 (4), 77–94. doi:10.3390/nano7040077
Torrisani, J., Bournet, B., Du Rieu, M. C., Bouisson, M., Souque, A., Escourrou, J., et al. (2009). let-7 MicroRNA Transfer in Pancreatic Cancer-Derived Cells Inhibits In Vitro Cell Proliferation but Fails to Alter Tumor Progression. Hum. Gene Ther. 20 (8), 831–844. doi:10.1089/hum.2008.134
Tsuji, N., Asanuma, K., Kobayashi, D., Yagihashi, A., and Watanabe, N. (2005). Introduction of a Survivin Gene-specific Small Inhibitory RNA Inhibits Growth of Pancreatic Cancer Cells. Anticancer Res. 25 (6B), 3967–3972.
Van Den Berg, A. I. S., Yun, C. O., Schiffelers, R. M., and Hennink, W. E. (2021). Polymeric Delivery Systems for Nucleic Acid Therapeutics: Approaching the Clinic. J. Control Release 331, 121–141. doi:10.1016/j.jconrel.2021.01.014
Vargason, A. M., Anselmo, A. C., and Mitragotri, S. (2021). The Evolution of Commercial Drug Delivery Technologies. Nat. Biomed. Eng. 5 (9), 951–967. doi:10.1038/s41551-021-00698-w
Vennin, C., Murphy, K. J., Morton, J. P., Cox, T. R., Pajic, M., and Timpson, P. (2018). Reshaping the Tumor Stroma for Treatment of Pancreatic Cancer. Gastroenterology 154 (4), 820–838. doi:10.1053/j.gastro.2017.11.280
Vetvicka, D., Sivak, L., Jogdeo, C. M., Kumar, R., Khan, R., Hang, Y., et al. (2021). Gene Silencing Delivery Systems for the Treatment of Pancreatic Cancer: Where and what to Target Next? J. Control Release 331, 246–259. doi:10.1016/j.jconrel.2021.01.020
Wang, C., Liu, B., Xu, X. L., Zhuang, B., Li, H. X., Yin, J. Q., et al. (2016). Toward Targeted Therapy in Chemotherapy-Resistant Pancreatic Cancer with a Smart Triptolide Nanomedicine. Oncotarget 7 (7), 8360–8372. doi:10.18632/oncotarget.7073
Wang, C. Y., Lin, B. L., and Chen, C. H. (2021). Targeted Drug Delivery Using an Aptamer against Shared Tumor-specific Peptide Antigen of MAGE-A3. Cancer Biol. Ther. 22 (1), 12–18. doi:10.1080/15384047.2020.1833156
Wang, F., Xue, X., Wei, J., An, Y., Yao, J., Cai, H., et al. (2010). hsa-miR-520h Downregulates ABCG2 in Pancreatic Cancer Cells to Inhibit Migration, Invasion, and Side Populations. Br. J. Cancer 103 (4), 567–574. doi:10.1038/sj.bjc.6605724
Wang, P., Chen, L., Zhang, J., Chen, H., Fan, J., Wang, K., et al. (2014). Methylation-mediated Silencing of the miR-124 Genes Facilitates Pancreatic Cancer Progression and Metastasis by Targeting Rac1. Oncogene 33 (4), 514–524. doi:10.1038/onc.2012.598
Wang, W., Wang, C., Dong, J. H., Chen, X., Zhang, M., and Zhao, G. (2005). Identification of Effective siRNA against K-Ras in Human Pancreatic Cancer Cell Line MiaPaCa-2 by siRNA Expression Cassette. World J. Gastroenterol. 11 (13), 2026–2031. doi:10.3748/wjg.v11.i13.2026
Wang, Y., Tiruthani, K., Li, S. R., Hu, M. Y., Zhong, G. J., Tang, Y., et al. (2021). mRNA Delivery of a Bispecific Single-Domain Antibody to Polarize Tumor-Associated Macrophages and Synergize Immunotherapy against Liver Malignancies. Adv. Mater. 33 (23), e2007603. doi:10.1002/adma.202007603
Wang, Y. X., Gao, L., and Shao, Q. S. (2014). Apoptosis of Human Pancreatic Carcinoma PC-2 Cells by an Antisense Oligonucleotide Specific to point Mutated K-Ras. Pathol. Oncol. Res. 20 (1), 81–85. doi:10.1007/s12253-013-9661-x
Waters, A. M., and Der, C. J. (2018). KRAS: the Critical Driver and Therapeutic Target for Pancreatic Cancer. Cold Spring Harb. Perspect. Med. 8 (9), a031435. doi:10.1101/cshperspect.a031435
Wittrup, A., and Lieberman, J. (2015). Knocking Down Disease: a Progress Report on siRNA Therapeutics. Nat. Rev. Genet. 16 (9), 543–552. doi:10.1038/nrg3978
Wu, X. Q., Liu, H. L., Han, D. M., Peng, B., Zhang, H., Zhang, L., et al. (2019). Elucidation and Structural Modeling of CD71 as a Molecular Target for Cell-specific Aptamer Binding. J. Am. Chem. Soc. 141 (27), 10760–10769. doi:10.1021/jacs.9b03720
Xu, Q. H., Li, P., Chen, X., Zong, L., Jiang, Z. D., Nan, L. G., et al. (2015). miR-221/222 Induces Pancreatic Cancer Progression through the Regulation of Matrix Metalloproteinases. Oncotarget 6 (16), 14153–14164. doi:10.18632/oncotarget.3686
Xu, Y. F., Hannafon, B. N., Zhao, Y. D., Postier, R. G., and Ding, W. Q. (2017). Plasma Exosome miR-196a and miR-1246 Are Potential Indicators of Localized Pancreatic Cancer. Oncotarget 8 (44), 77028–77040. doi:10.18632/oncotarget.20332
Xu, Z. W., Friess, H., Solioz, M., Aebi, S., Korc, M., Kleeff, J., et al. (2001). Bcl-X(L) Antisense Oligonucleotides Induce Apoptosis and Increase Sensitivity of Pancreatic Cancer Cells to Gemcitabine. Int. J. Cancer 94 (2), 268–274. doi:10.1002/ijc.1447
Xuan, W. J., Peng, Y. B., Deng, Z. Y., Peng, T. H., Kuai, H. L., Li, Y. Y., et al. (2018). A Basic Insight into Aptamer-Drug Conjugates (ApDCs). Biomaterials 182, 216–226. doi:10.1016/j.biomaterials.2018.08.021
Yamakawa, K., Nakano-Narusawa, Y., Hashimoto, N., Yokohira, M., and Matsuda, Y. (2019). Development and Clinical Trials of Nucleic Acid Medicines for Pancreatic Cancer Treatment. Int. J. Mol. Sci. 20 (17), 4224–4240. doi:10.3390/ijms20174224
Yamamoto, K., Venida, A., Yano, J., Biancur, D. E., Kakiuchi, M., Gupta, S., et al. (2020). Autophagy Promotes Immune Evasion of Pancreatic Cancer by Degrading MHC-I. Nature, 581(7806), 100–105. doi:10.1038/s41586-020-2229-5
Yang, C. B., Chan, K. K., Lin, W. J., Soehartono, A. M., Lin, G. M., Toh, H., et al. (2017). Biodegradable Nanocarriers for Small Interfering Ribonucleic Acid (siRNA) Co-delivery Strategy Increase the Chemosensitivity of Pancreatic Cancer Cells to Gemcitabine. Nano Res. 10 (9), 3049–3067. doi:10.1007/s12274-017-1521-7
Yang, C. B., Hu, R., Anderson, T., Wang, Y. C., Lin, G. M., Law, W. C., et al. (2015). Biodegradable Nanoparticle-Mediated K-Ras Down Regulation for Pancreatic Cancer Gene Therapy. J. Mater. Chem. B 3 (10), 2163–2172. doi:10.1039/c4tb01623h
Yin, H., Kanasty, R. L., Eltoukhy, A. A., Vegas, A. J., Dorkin, J. R., and Anderson, D. G. (2014). Non-viral Vectors for Gene-Based Therapy. Nat. Rev. Genet. 15 (8), 541–555. doi:10.1038/nrg3763
Yin, Z., Ma, T. T., Huang, B. W., Lin, L. H., Zhou, Y., Yan, J. H., et al. (2019). Macrophage-derived Exosomal microRNA-501-3p Promotes Progression of Pancreatic Ductal Adenocarcinoma through the TGFBR3-Mediated TGF-Beta Signaling Pathway. J. Exp. Clin. Cancer Res. 38, 310–330. doi:10.1186/s13046-019-1313-x
Yoon, S., Armstrong, B., Habib, N., and Rossi, J. J. (2017a). Blind SELEX Approach Identifies RNA Aptamers that Regulate EMT and Inhibit Metastasis. Mol. Cancer Res. 15 (7), 811–820. doi:10.1158/1541-7786.Mcr-16-0462
Yoon, S., Huang, K. W., Andrikakou, P., Vasconcelos, D., Swiderski, P., Reebye, V., et al. (2019). Targeted Delivery of C/EBP Alpha-saRNA by RNA Aptamers Shows Anti-tumor Effects in a Mouse Model of Advanced PDAC. Mol. Ther. Nucleic Acids 18, 142–154. doi:10.1016/j.omtn.2019.08.017
Yoon, S., Huang, K. W., Habib, N., and Rossi, J. (2016a). Potent Anti-tumor Effects of ApDCs (Aptamer Drug Conjugates) for Targeted Therapeutics in Pancreatic Cancer. Mol. Ther. 24, S265. doi:10.1016/s1525-0016(16)33479-7
Yoon, S., Huang, K. W., Reebye, V., Mintz, P., Tien, Y. W., Lai, H. S., et al. (2016b). Targeted Delivery of C/EBP Alpha -saRNA by Pancreatic Ductal Adenocarcinoma-specific RNA Aptamers Inhibits Tumor Growth In Vivo. Mol. Ther. 24 (6), 1106–1116. doi:10.1038/mt.2016.60
Yoon, S., Huang, K. W., Reebye, V., Spalding, D., Przytycka, T. M., Wang, Y. J., et al. (2017b). Aptamer-drug Conjugates of Active Metabolites of Nucleoside Analogs and Cytotoxic Agents Inhibit Pancreatic Tumor Cell Growth. Mol. Ther. Nucleic Acids 6, 80–88. doi:10.1016/j.omtn.2016.11.008
Yu, J., Ohuchida, K., Mizumoto, K., Fujita, H., Nakata, K., and Tanaka, M. (2010). MicroRNA miR-17-5p Is Overexpressed in Pancreatic Cancer, Associated with a Poor Prognosis and Involved in Cancer Cell Proliferation and Invasion. Cancer Biol. Ther. 10 (8), 748–757. doi:10.4161/cbt.10.8.13083
Yu, S. N., Lu, Z. H., Liu, C. Z., Meng, Y. X., Ma, Y. H., Zhao, W. G., et al. (2010). miRNA-96 Suppresses KRAS and Functions as a Tumor Suppressor Gene in Pancreatic Cancer. Cancer Res. 70 (14), 6015–6025. doi:10.1158/0008-5472.Can-09-4531
Zeng, L. J., Li, J. G., Wang, Y., Qian, C. C., Chen, Y. T., Zhang, Q. B., et al. (2014). Combination of siRNA-Directed Kras Oncogene Silencing and Arsenic-Induced Apoptosis Using a Nanomedicine Strategy for the Effective Treatment of Pancreatic Cancer. Nanomed. Nanotechnol. Biol. Med. 10 (2), 463–472. doi:10.1016/j.nano.2013.08.007
Zhang, X. J., Ye, H., Zeng, C. W., He, B., Zhang, H., and Chen, Y. Q. (2010). Dysregulation of miR-15a and miR-214 in Human Pancreatic Cancer. J. Hematol. Oncol. 3, 46. doi:10.1186/1756-8722-3-46
Zhong, Y. Q., Xia, Z. S., Liu, J., Lin, Y., and Zan, H. (2012). The Effects of Cyclooxygenase-2 Gene Silencing by siRNA on Cell Proliferation, Cell Apoptosis, Cell Cycle and Tumorigenicity of Capan-2 Human Pancreatic Cancer Cells. Oncol. Rep. 27 (4), 1003–1010. doi:10.3892/or.2011.1595
Zhou, J. H., and Rossi, J. (2017). Aptamers as Targeted Therapeutics: Current Potential and Challenges. Nat. Rev. Drug Discov. 16 (3), 181–202. doi:10.1038/nrd.2016.199
AAV Adeno-associated virus
ApDC Aptamer-drug conjugate
5-FU 5-Fluorouracil
ABCG2 ATP-binding cassette super-family G member 2
ADC Antibody-drug conjugate
AGO2 Argonaute RISC Catalytic Component 2
ALPPL2 Alkaline phosphatase placental-like 2
AP1153 One DNA aptamer targeting CCKBR
APTA-12 Gemcitabine-incorporated AS1411
AS1411 One DNA aptamer targeting nucleolin
ASO Antisense oligonucleotide
AsPC-1 Human pancreatic tumor cell line
AS-PPT AS1411-PEG-PDLLA micelle
BAG3 BAG family molecular chaperone regulator
Bcl-2 B-cell lymphoma 2
Bcl-xL B-cell lymphoma-extra large
BCPV Biodegradable charged polyester-based vector
BxPC-3 Human pancreatic adenocarcinoma cell line
C/EBPα CCAAT/Enhancer-binding Protein α
CA19-9 Carbohydrate antigen 19-9
CAF Cancer-associated fibroblast
Capan-1 Human pancreatic ductal adenocarcinoma cell line
CCKBR G-protein-coupled cholecystokinin B receptor
CCL2 C-C motif chemokine ligand 2
CCL5 C-C motif chemokine ligand 5
CCR4-NOT Carbon catabolite repression 4–negative on TATA-less
CD8+ T cells Cytotoxic T lymphocytes cells
CEA Carcinoembryonic antigen
CML Chronic myelogenous leukemia
COVID-19 Coronavirus Disease 2019
COX-2 Cyclooxygenase-2
CPNSPs Coprinopsis psychromorbida
CPP Cell-penetrating peptide
cRGD Cyclic arginylglycylaspartic acid
CSF-1 Colony stimulating factor 1
CSF-1R Colony stimulating factor 1 receptor
CXCL10 C-X-C motif chemokine ligand 10
CXCL12 Chemokine ligand 12
dFdCMP Gemcitabine monophosphate
DM1 Mertansine
DOX 4-Substituted-2,5-dimethoxyamphetamines
ECM Extracellular matrix
EGFR Epidermal growth factor receptor
FAK Focal adhesion kinase
FDA U.S. food and drug administration
FOLFIRINOX Folinic acid, fluorouracil, irinotecan and oxaliplatin
GalNAc N-acetylgalactosamine
GBI-10 One DNA aptamer targeting tenascin-C
GLUT1 Glucose transporter 1
HaP-T1 N-nitrosobis-(2-hydroxypropyl) amine-induced hamster pancreatic tumor
Hsp Heat shock protein
IC50 Half-maximal inhibitory concentration
IGF2BP2 Insulin-like growth factor 2 mRNA binding protein 2
IL-6 Interleukin 6
KRAS Kirsten rat sarcoma virus
let-7 Lethal-7 gene
LNP lipid nanoparticle
MAGE-A3 Melanoma-associated antigen 3
MIA PaCa-2 Hypotriploid human pancreatic cancer cell line
miRNA microRNA
MMAE Monomethyl auristatin E
MMAF Monomethyl auristatin F
MMP Matrix metalloproteinase
MMP3 Matrix metallopeptidase 3
mRNA Messenger RNA
MUC4 Mucin 4
NETs Neuroendocrine tumors
NF-κB Nuclear factor-κB
NGF Nerve growth factor
NRF2 Nuclear factor erythroid-derived 2-like 2
P12FR2 2′-fluoropyrimidine modified RNA aptamer
PAN2-PAN3 Poly (A)-nuclease 2-poly (A)-nuclease 3
PANC-1 Human pancreatic ductal cancer cell line
PAUF Pancreatic adenocarcinoma up-regulated factor
PC Pancreatic cancer
PCR Polymerase chain reaction
PD-1 Programmed cell death protein 1
PDAC Pancreatic ductal adenocarcinoma
PDCD4 Programmed cell death protein 4
PD-Li Programmed cell death ligand inhibitor
PDLLA Poly (DL-lactide)
PLK1 Polo-like kinase 1
PEG Polyethylene glycol
PEGPH20 Pegvorhyaluronidase alfa
PI3K-γ Phosphatidylinositide 3-kinase γ
PK-45H Human pancreatic carcinoma cell line
PKA Protein Kinase A
PLGA Poly (lactic-co-glycolic acid)
PSC Pancreatic stellate cell
pSUPER Suppression of endogenous RNA
PTEN Phosphatase and tensin homolog
RCT Randomized controlled trial
RIG-I Retinoic acid-inducible gene I
RISC RNA-induced silencing complex
RNAi RNA interference
RNase H1 Ribonuclease H1
saRNA Small activating RNA
SELEX Systematic evolution of ligands by exponential enrichment
siRNA Small interfering RNA
STAT3 Signal transducer and activator of transcription 3
SUIT-2 Human pancreatic cancer cell line from liver metastasis
T3M4 Human pancreatic carcinoma cell line
TGF Transforming growth factor
TIME Tumor immune microenvironment
TP53 Tumor protein 53
TrkA Tropomyosin receptor kinase A
tTR 14 An RNA aptamer targeting transferrin
VEGF Vascular endothelial growth factor
XIAP X-linked inhibitor of apoptosis protein
XQ-2d One DNA aptamer targeting PDAC
Keywords: pancreatic cancer, nucleic acid drugs, targeted therapy, aptamer-drug conjugates, antisense oligonucleotides, small interfering RNAs
Citation: Dai H, Abdullah R, Wu X, Li F, Ma Y, Lu A and Zhang G (2022) Pancreatic Cancer: Nucleic Acid Drug Discovery and Targeted Therapy. Front. Cell Dev. Biol. 10:855474. doi: 10.3389/fcell.2022.855474
Received: 15 January 2022; Accepted: 07 April 2022;
Published: 16 May 2022.
Edited by:
Brigitte M. Pützer, University Medicine, GermanyReviewed by:
Yuanyu Huang, Beijing Institute of Technology, ChinaCopyright © 2022 Dai, Abdullah, Wu, Li, Ma, Lu and Zhang. This is an open-access article distributed under the terms of the Creative Commons Attribution License (CC BY). The use, distribution or reproduction in other forums is permitted, provided the original author(s) and the copyright owner(s) are credited and that the original publication in this journal is cited, in accordance with accepted academic practice. No use, distribution or reproduction is permitted which does not comply with these terms.
*Correspondence: Yuan Ma, eXVhbm0wOTNAMTYzLmNvbQ==; Aiping Lu, YWlwaW5nbHVAaGtidS5lZHUuaGs=; Ge Zhang, emhhbmdnZUBoa2J1LmVkdS5oaw==
Disclaimer: All claims expressed in this article are solely those of the authors and do not necessarily represent those of their affiliated organizations, or those of the publisher, the editors and the reviewers. Any product that may be evaluated in this article or claim that may be made by its manufacturer is not guaranteed or endorsed by the publisher.
Research integrity at Frontiers
Learn more about the work of our research integrity team to safeguard the quality of each article we publish.