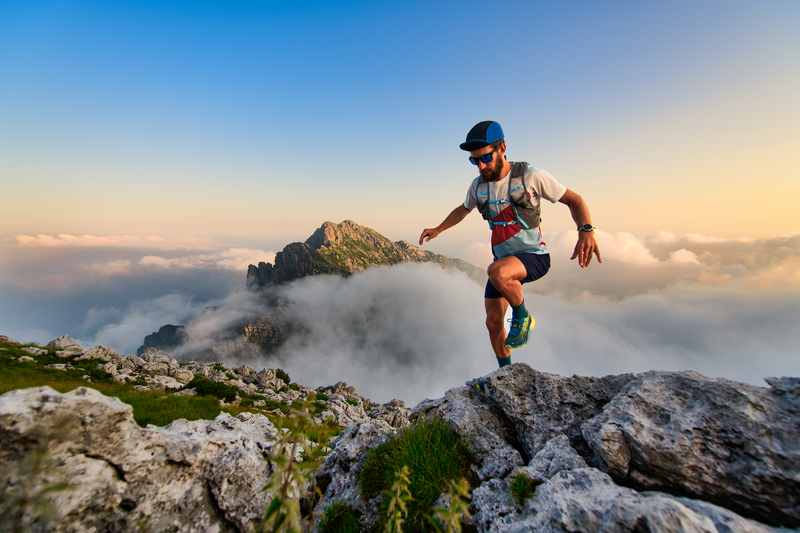
95% of researchers rate our articles as excellent or good
Learn more about the work of our research integrity team to safeguard the quality of each article we publish.
Find out more
MINI REVIEW article
Front. Cell Dev. Biol. , 11 March 2022
Sec. Stem Cell Research
Volume 10 - 2022 | https://doi.org/10.3389/fcell.2022.854740
This article is part of the Research Topic Developmental Models 2.0 View all 16 articles
The study of human intestinal biology in healthy and diseased conditions has always been challenging. Primary obstacles have included limited tissue accessibility, inadequate in vitro maintenance and ethical constrains. The development of three-dimensional organoid cultures has transformed this entirely. Intestinal organoids are self-organized three-dimensional structures that partially recapitulate the identity, cell heterogeneity and cell behaviour of the original tissue in vitro. This includes the capacity of stem cells to self-renew, as well as to differentiate towards major intestinal lineages. Therefore, over the past decade, the use of human organoid cultures has been instrumental to model human intestinal development, homeostasis, disease, and regeneration. Intestinal organoids can be derived from pluripotent stem cells (PSC) or from adult somatic intestinal stem cells (ISC). Both types of organoid sources harbour their respective strengths and weaknesses. In this mini review, we describe the applications of human intestinal organoids, discussing the differences, advantages, and disadvantages of PSC-derived and ISC-derived organoids.
The small intestine is a major organ that regulates digestive function and nutrient absorption. It is structured into crypts and protruding finger-like domains called villi (Figure 1A). The whole intestine is lined with a highly specialized epithelium, supported by a complex underlying mesenchyme (Guiu and Jensen, 2015; Gehart and Clevers, 2019; McCarthy et al., 2020a). During homeostasis, the human intestinal epithelium undergoes complete renewal every 5–7 days (Barker, 2014). This highly regenerative nature is supported by multipotent intestinal stem cells (ISCs), residing at the bottom of the crypts (Barker et al., 2007). ISCs can be identified by the expression of the R-spondin (RSPO) receptor LGR5, which plays a major role in the regulation of WNT/RSPO-signalling (Clevers, 2013). The proliferative ISC population in the crypt is maintained by neutral competition for supportive signals, including high WNT concentration, Notch signals and EGF (Snippert et al., 2010; Sato et al., 2011a). These primarily originate from Paneth cells, differentiated secretory cells that migrate away from differentiated villi regions to become interspersed with ISCs (van Es et al., 2005; Sato et al., 2011a). Paneth cells are also specialised in the secretion of antimicrobial agents such as lysozyme, α-defensins and phospolipase-A2 (Porter et al., 2002). Nevertheless, ablation experiments in mice showed that Paneth cells are redundant (Kim et al., 2012; Van Es et al., 2019). While Notch-signalling can be provided by secretory lineage precursor cells, WNT and EGF signals are also supplied by multiple cell types of the subepithelial mesenchyme (Blumberg et al., 2008; Van Es et al., 2019; McCarthy et al., 2020a).
FIGURE 1. Cellular composition of intestinal tissue and organoids. (A) Different cell types that make up the crypt-villi structure in vivo small intestinal tissue. (B) Comparative overview of the cellular composition of pluripotent stem cell-derived human intestinal organoids or hIOs and tissue-derived organoids or enteroids. TAZ, Transit Amplifying Zone. Created with BioRender.com.
One specific CD81+/Pdgfra + mesenchymal subpopulation, termed trophocytes is primarily located below the crypt epithelium and can support mouse intestinal cells in vitro without any additional factors (McCarthy et al., 2020a) (Figure 1A). Aside from expression of canonical Wnt ligands and Wnt-promoting Rspo proteins, trophocytes are also typified by their expression of BMP inhibitors Gremlin 1 and Noggin (McCarthy et al., 2020a). Another subpopulation, Foxl1+/Pdgfra + subepithelial myofibroblasts (SEMFs), also termed telocytes, form a loosely interconnected sheat directly below the intestinal epithelium via their far-reaching cell extensions (Shoshkes-Carmel et al., 2018). Their distribution is non-uniform as they have higher concentrations near the villus base, as well as in villus tips (Shoshkes-Carmel et al., 2018). Depending on this distribution, telocytes express multiple Bmp factors and non-canonical Wnt factors which generally counteract canonical Wnt-signalling (Shoshkes-Carmel et al., 2018; McCarthy et al., 2020b). However, telocytes also express canonical Wnt agonists, and specific inhibition of Wnt secretion in mouse telocytes ultimately caused a dramatic reduction in ISC proliferation (Shoshkes-Carmel et al., 2018). The mesenchyme further harbours a large heterogenous population of Pdgfralow-mesenchymal cells which can express both Bmp factors, Wnt agonists and antagonists, further contributing to a complex mesenchymal environment (McCarthy et al., 2020a; Kim JE. et al., 2020). Specific subpopulations of Gfap + enteric glial cells (EGCs), localised near crypts, also provide key yet redundant Wnt factors to ISCs (Baghdadi et al., 2022). In case of injury however, these EGCs expand and are critical to efficient intestinal regeneration (Baghdadi et al., 2022). Various types of immune cells also reside in the intestinal mesenchyme and tissue-resident lymphoid cells have been implicated in the regulation of intestinal homeostasis and regeneration by secretion of interleukin factors (Lindemans et al., 2015; Zhu et al., 2019). Furthermore, endothelial cells may also be involved in ISC maintenance, as specific inhibition of endothelial cell apoptosis was sufficient to recover crypt homeostasis following irradiation damage (Paris et al., 2001). Lymphatic endothelial cells were also shown to express relatively high levels of Rspo3, contributing to the maintenance of intestinal homeostasis (Ogasawara et al., 2018).
Generally, factors secreted by Paneth cells and mesenchymal cells create a high WNT/RSPO-signalling environment towards the bottom of the crypt, while a high BMP-signalling environment is present towards the villi. Both gradients decrease towards the transition zone, creating a zonation with segregated environments that guide cell type identity (Sato and Clevers, 2013; Moor et al., 2018). As ISC daughter cells move from the bottom of the crypt to the villi domain, they move through these different molecular gradients, generating increasing maturation of specialized cell types (Moor et al., 2018; Shoshkes-Carmel et al., 2018; McCarthy et al., 2020a).
ISCs give rise to committed daughter cells, which differentiate into distinct absorptive and secretory lineages (Wright, 2000; Schuijers and Clevers, 2012). The absorptive lineage gives rise to nutrient-absorbing enterocytes, while the secretory lineage primarily generates diverse hormone-producing enteroendocrine cells, mucus-producing goblet cells and ISC-supporting Paneth cells (Clevers and Bevins, 2013; Beumer et al., 2018; Yang and Yu, 2021). Cells that reach the top of the villi go into apoptosis and are shed into the lumen. The relatively rapid turnover, combined with the changing molecular environments demands a high degree of plasticity from intestinal epithelial cells. This has recently been demonstrated in the mouse intestine, as all major intestinal cell types can de-differentiate to a stem cell state, in reaction to proper environmental cues. Following ablation of resident ISCs, cell-specific lineage tracing strategies demonstrated regeneration of ISCs and full crypt-villi structures from Alpi + enterocytes (Tetteh et al., 2016), Lyz + Paneth cells (Yu et al., 2018) and endocrine lineage precursor cells (Van Es et al., 2012; Barriga et al., 2017; Yan et al., 2017; Ayyaz et al., 2019). In the mouse fetal intestinal epithelium, all cells, irrespective of their location and differentiation status, actively contribute to the adult ISC pool (Guiu et al., 2019). Moreover, a recent study demonstrated that, following the loss of proliferative ISCs due to injury, de-differentiation processes are the main driving force in the generation of novel ISCs as opposed to a specialized injury-resistant reserve stem cell population (Murata et al., 2020). However, cells of the secretory lineage inherently have low proliferation rates, which may make them more resistant to routinely used forms of cell damage, such as irradiation or chemotherapy (Basak et al., 2014). This may make them more likely to be sources of de-differentiation. Interestingly, de-differentiation processes occur by a shift from an adult to a more fetal-like intestinal gene expression profile, particularly involving high YAP1-signalling (Gregorieff et al., 2015; Yui et al., 2018).
Due to limited tissue accessibility and ethical considerations, the elucidation of the cellular and molecular complexity of the intestine has relied on animal and in vitro model systems. In recent years, the development of three-dimensional organoids has transformed biomedical research fields, including tissue homeostasis, regeneration and stem cell differentiation (Leushacke and Barker, 2014). In this review, we discuss the advantages and disadvantages of different organoid models.
Mouse models have greatly enhanced our understanding of biological systems and have enabled the development of numerous therapeutic strategies. Most importantly, they have allowed us to study pathologies without involving human patients. However, insights from animal models cannot guarantee extrapolation to human tissues. For instance, the timeline of embryonic intestinal development in mice proceeds past birth, while human intestinal formation is largely completed during the second trimester (Guiu and Jensen, 2015). One major difference in these developmental schedules is the formation of Paneth cells, which in human appear during the first trimester while in mouse only by day 14 postnatally (Bry et al., 1994; Rumbo and Schiffrin, 2005). Small intestinal villi in mouse are taller than in human, even though the small intestine is proportionally about three times shorter in mice (Nguyen et al., 2015). Furthermore, EGF seems to have opposite effects on the maturation of in vivo intestinal epithelium in mouse and in human (Calvert et al., 1982; Ménard et al., 1988). Importantly, stable mouse organoid lines can be derived with minimal niche factors Rspo1, Noggin and EGF, while human organoids demand additional growth factors and inhibition of p38/MAPK pathways (Sato et al., 2011b; Fujii et al., 2018). In addition, studies on animal models remain encumbered by considerations of animal ethics, limiting their scalability to high-throughput use.
In the last decade, organoids have been increasingly used as in vitro models as they improve upon several of these shortcomings. Organoids are self-organizing three-dimensional structures, consisting of both multipotent tissue-specific stem cells, as well as their more differentiated progeny (Zachos et al., 2016). Similar to stem cell lines, they can be propagated virtually indefinitely, provided the right culture conditions are met (Sato et al., 2009; 2011b). While human intestinal organoids were the first human organoid types that were successfully established in vitro, many protocols have since been optimized for organoids of many other tissue types, including pancreas, liver, kidney, stomach and lung (Huch et al., 2013a, 2013b; Dye et al., 2015; Nishinakamura, 2019; Seidlitz et al., 2021). Organoids recapitulate in vivo tissue architecture, multiple cell type heterogeneity and interactions in vitro. Therefore, they will potentially model human tissue functionality and physiology more accurately than animal models or 2D models (Lancaster and Knoblich, 2014; Kim J. et al., 2020). For instance, when sufficiently matured, human intestinal organoids recapitulate budding crypt and villi domains, harbouring proliferative ISCs and progenitors, as well as differentiated enterocytes, goblet cells and Paneth cells, respectively (Onozato et al., 2021). Although organoids contain differentiated cell types, they can be cryopreserved and expanded relatively rapidly (Clinton and McWilliams-Koeppen, 2019). Organoids have proven to be highly versatile in experimental settings, as they are compatible with routinely used imaging techniques (Mahe et al., 2013; Dekkers et al., 2019), as well as live cell imaging (Zietek et al., 2015), assessment of metabolic activity (Berger et al., 2016), genome-wide gene expression and protein analyses (Lindeboom et al., 2018; Kip et al., 2021; Norkin et al., 2021) and gene editing techniques (Koo et al., 2012; Schwank et al., 2013; Schwank and Clevers, 2016; Driehuis and Clevers, 2017). Another major advantage of organoid models is their in vitro scalability to high-throughput assays, in combination with their recapitulation of 3D tissue functionality (Lukonin et al., 2020; Sugimoto and Sato, 2022). As such, organoids have been increasingly used to study intestinal function, to model disease phenotypes and for drug discovery screening (Fair et al., 2018; Frum and Spence, 2021).
Different types of small intestinal organoids have been described and to distinguish them, a specific nomenclature has been proposed (Stelzner et al., 2012). Human small intestinal organoids can be generated by differentiation from human pluripotent stem cells (PSCs) or by derivation from isolated multipotent stem cells and progenitor cells present in vivo intestinal crypts. The former are conventionally referred to as human intestinal organoids (hIOs), while the latter are termed enteroids (Stelzner et al., 2012) (Figure 1B). Additionally, in the initial 24-h culture phase to generate enteroids, small circular structures are formed, which are referred to as enterospheres (Stelzner et al., 2012). The enterosphere phase is transversed by both enteroids and hIOs during their formation (Stelzner et al., 2012). In contrast to enteroids, hIOs recapitulate fetal small intestine developmental stages and are therefore an excellent tool for studying this process (Wells and Spence, 2014; Frum and Spence, 2021). However, they also differentiate into both epithelial and mesenchymal cells (Spence et al., 2011; Mahe et al., 2015). Multicellular organoids such as these are also sometimes referred to as “reconstituted intestinal organoids” (Stelzner et al., 2012). Thus, reconstituted intestinal organoids or hIOs may complicate our understanding of the molecular mechanisms occurring specifically between intestinal epithelial cells. Recently, Mithal and colleagues reported a novel strategy to generate mesenchyme-free organoids from human iPSCs (Mithal et al., 2020). On the other hand, the presence of mesenchymal cells does create an intestinal model which more closely resembles the in vivo tissue compared to organoids with only epithelial cells. If the goal is to study the co-dependency of both tissues during development, this is rather advantageous, as has been shown by the use of hIOs including mesenchymal cells to model fibrosis in ulcerative colitis (Sarvestani et al., 2021). However, upon establishment of hIO cultures, they largely retain fetal-like characteristics. This has been demonstrated by RNA-sequencing comparisons of hIOs with fetal and adult intestinal tissues and is likely caused by a lack of appropriate cues from the mesenchymal niche (Finkbeiner et al., 2015; McCarthy et al., 2020a).
In contrast, intestinal crypt-derived enteroids recapitulate adult intestinal architecture and genetic profiles of specific adult intestinal epithelial cell types more faithfully in mouse, with Lgr5+ stem cells residing in crypt-like zones and differentiated cells residing in inter-crypt zones (Schuijers and Clevers, 2012). Interestingly, transient depletion of proliferative cells reverts mouse intestinal organoids to a more fetal-like state (Gregorieff et al., 2015). Of note, fetal-like hIOs can be matured further by transplantation under the kidney capsule of immune-deficient mice (Watson et al., 2014). One major factor involved in this maturation may originate from endothelial cells, as post-transplanted mature organoids obtain extensive vascularization (Frum and Spence, 2021). Moreover, the co-culture of hIOs with endothelial cells also promotes organoid maturation (Palikuqi et al., 2020). Nevertheless, the directed differentiation process of PSCs towards intestinal organoids takes quite some time (2–3 months), after which hIOs still largely resemble fetal intestinal homeostasis (McCracken et al., 2011; Frum and Spence, 2021). Though recently, Onozato and colleagues described a method to enhance organoid maturation in vitro, generating crypt-like budding and expression of villin1 (Onozato et al., 2021). Conversely, enteroids do not have to undergo sequential differentiation steps and can therefore be generated much more rapidly. Furthermore, enteroids are capable of recapitulating intestinal epithelium beyond a fetal phenotype with a higher degree of maturation (Hofer and Lutolf, 2021). However, it is important to realize that full maturation is usually not achieved with enteroids either, due to conventional culture conditions that promote proliferation. Nevertheless, due to the lack of mesenchymal cells, enteroids provide a less complex model system compared to hIOs to study epithelial cell type-specific molecular functions.
Intestinal organoid technology has greatly enhanced research into tissue homeostasis, cell-to-cell interactions, differentiation, and physiology. However, several shortcomings still limit the model in its application to clinical research.
Firstly, while organoids are comprised of multiple cell types of the corresponding tissue, they still only include multipotent stem cells and their progeny. Enteroids therefore only include epithelial cell types. While hIOs do generate mesenchymal cells surrounding the epithelial cells, this is still rather limited compared to the in vivo intestine. Generally, both types of organoids lack complex mesenchymal heterogeneity and architecture, vasculature, neuronal connections and interaction with immune cells and the intestinal microbial flora. Several groups have therefore explored possible co-culture strategies to increase organoid complexity, including co-culture with endothelial (Palikuqi et al., 2020), mesenchymal (Stzepourginski et al., 2017), immune (Bar-Ephraim et al., 2020) and glial cells (Baghdadi et al., 2022), as well as microbial (Finkbeiner et al., 2012; Wilson et al., 2015; Fatehullah et al., 2016; Han et al., 2019; Barron et al., 2020) and viral interactions (Zhao et al., 2021). Yet, current co-culture systems are still relatively simple, only combining one or few cell types simultaneously. In the future, both hIO and enteroid models will benefit from efforts to increase complexity of co-culture systems.
Intestinal organoids inherently form a lumen-enclosed structure. This reduces access to the absorptive apical side and leads to accumulation of apoptotic cells inside organoids. This potentially confounds drug screening and microbial co-culture studies (Altay et al., 2019). Recently, several publications have described methods to generate reversion of the apical side to the outside of organoids (Co et al., 2019, 2021; Li et al., 2020; Stroulios et al., 2021). For instance, Co. and colleagues described how inhibition of the interaction between organoid epithelial cell β1-integrin receptors and the protein matrix in which they are cultured (such as Matrigel), caused spontaneous reversion of polarity (Co et al., 2019). Indeed, the localisation of the matrix used to create a supportive 3D environment, causes the apical-in polarity of organoids, similar to the extracellular matrix in vivo.
Conventional organoid culture mostly involves the embedding of organoids in Matrigel, a complex basement-membrane protein matrix, isolated from Engelbreth-Holm-Swarm mouse sarcoma tumor cells (Jee et al., 2019; Aisenbrey and Murphy, 2020). Matrigel provides essential ECM components to organoids, such as laminins, collagens and fibronectins (Jee et al., 2019; Aisenbrey and Murphy, 2020). Each of these components individually are capable of supporting the formation of ISC colonies, yet optimal differentiation to organoids was shown to require a delicate balancing of differential compositions (Gjorevski et al., 2016). However, due to Matrigel’s highly complex composition, inherent variability and mouse origin, recent efforts are focussing on the design and study of synthetic hydrogel alternatives (Rezakhani et al., 2021). In fact, mechanical properties such as stiffness and degradability are equally vital to the formation, maintenance and differentiation of organoids in culture (Gjorevski et al., 2016). As such, the mechanobiological properties of the protein matrix also influence the phenotype exhibited by organoids: 1) enteroids cultured in Matrigel generate budding structures that recapitulate in vivo behaviour (Sato et al., 2009); 2) organoids cultured in collagen I matrices acquire a foetal-like state mimicking the in vivo process of regeneration (Yui et al., 2018); 3) hanging drop cultures increase inter-organoid interactions, organoid fusion and can even induce continuous peristaltic movements (Ueda et al., 2010; Panek et al., 2018). Similarly, culture in floating collagen gels also generates fusion and self-organisation of intestinal organoids into tubular structures (Sachs et al., 2017). Nevertheless, as of yet, conventional organoid culture lacks the presence of in vivo growth factors, complex extracellular matrix composition, as well as fully optimized biomechanical properties of their environment (Guiu and Jensen, 2022). Importantly, intestinal organoid lines also possess a high degree of variability, as tissue heterogeneity (for enteroids), passaging methods, access to media components and positioning within 3D matrices impose a stochastic nature to their culture (Nikolaev et al., 2020).
Recently, novel approaches have been implemented aiming to meet several of these shortcomings. Experimentation on substrate matrix properties has allowed for improved monolayer culture of intestinal organoids, allowing access to the luminal side of intestinal cells and analysis of barrier integrity (Schweinlin et al., 2016; Altay et al., 2019). Chen and colleagues utilized a hollowed-out porous silk protein scaffold, onto which intestinal epithelial and myofibroblast cells could be seeded and maintained (Chen et al., 2015). This system recapitulated complex intestinal function and architecture, including mucus production and an accessible tubular lumen which allowed analysis of intraluminal oxygen tension and bacterial interaction (Chen et al., 2015). Future advancement of 3D bioprinting technology towards scaffold-free approaches will undoubtedly improve intestinal tissue modelling even further (Murphy and Atala, 2014; Wengerter et al., 2016). Organ-on-chip engineering has also recently been implemented to form perfusable in vitro mouse intestinal organoid structures, embedded in a pre-shaped matrix scaffold. Nikolaev and colleagues described the self-organization of intestinal cells into a perfusable tube-shaped structure with crypt and villi domains and differentiation into specialised intestinal cells, inside a preformed hydrogel scaffold on a chip (Nikolaev et al., 2020). Kasendra and colleagues created an intestinal and microvascular endothelial co-culture on a chip in parallel perfusable channels. Crypt and villi structures were formed with multilineage differentiation, while interfacing with the microvascular endothelial cells (Kasendra et al., 2018). Such perfusable models allow the sequential analysis of fluid samples to quantify nutrient digestion, mucus secretion and establishment of intestinal barrier function in vitro (Kasendra et al., 2018; Nikolaev et al., 2020). Overall, this technology has the potential to increase robustness of crypt-villi architecture formation, nutrient absorption, co-culture strategies, larger scale intestinal architecture, as well as morphogen gradients. Moreover, multi-organ co-culture chip strategies have already been explored to investigate higher order tissue interactions and barrier function (Maschmeyer et al., 2015a; 2015b). However, the increasing complexity of organ-on-chip approaches may also limit high-throughput scalability and escalate associated costs.
Taken together, although human organoids provide attractive in vitro models, it is important to keep in mind they are still simplified culture models of a much more complex in vivo system (Figures 1A,B) (Guiu and Jensen, 2022). Yet, they provide a highly improved model compared to 2D culture approaches in terms of recapitulation of cell type-heterogeneity, cell type-interactions and 3D architecture. Compared to mouse models, intestinal organoids circumvent animal ethics considerations, allow high-throughput scalability and provide a human model intestinal functionality (Figure 2). As such, human intestinal organoids can represent a sufficiently complex in vitro model of intestinal tissue from fetal to adult human stages of development, which are otherwise difficult to access. Multiple recent reports have shown increased applicability and accuracy of organoid models, including maturation into budding organoids in vitro, outwards reversion of the apical side and induction of peristalsis. These improvements have increasingly benefitted potential future applications of hIOs, which could be used in a patient specific manner for drug screening and disease modelling purposes. Nevertheless, current organoid culture strategies still lack the complex interaction with in vivo growth factors, extracellular matrix composition and multi-organ physiology (Guiu and Jensen, 2022). Ultimately, future findings of interest from high-throughput screening studies using hIO or enteroid models should be validated in vivo in animal models, to provide information on the effects of the physiological environment, but also to assess whether findings from a simplified organoid structure can be recapitulated in the more complex in vivo intestinal tissue, albeit in an animal model.
FIGURE 2. Strengths and weaknesses of hIOs, enteroids and mouse models. Summary of advantages and disadvantages of PSC-derived organoids or hIOs, tissue-derived organoids or enteroids and the mouse model. Created with BioRender.com.
JT and JG contributed to the conception and design of the mini review. JT wrote the manuscript. JT, MD and JG edited sections and provided critical review. All authors read and approved the final version of the manuscript.
This study has been funded by Instituto de Salud Carlos III through the grant CP20/00115 (Co-funded by European Social Fund. ESF investing in your future and the European Regional Developtment Fund “a way to build Europe”). We thank CERCA Programme/Generalitat de Catalunya for institutional support. This study has been funded by Instituto de Salud Carlos III through the project PI21/01152 (Co-funded by European Regional Development Fund. ERDF, a way to build Europe).
The authors declare that the research was conducted in the absence of any commercial or financial relationships that could be construed as a potential conflict of interest.
All claims expressed in this article are solely those of the authors and do not necessarily represent those of their affiliated organizations, or those of the publisher, the editors and the reviewers. Any product that may be evaluated in this article, or claim that may be made by its manufacturer, is not guaranteed or endorsed by the publisher.
Aisenbrey, E. A., and Murphy, W. L. (2020). Synthetic Alternatives to Matrigel. Nat. Rev. Mater. 5, 539–551. doi:10.1038/s41578-020-0199-8
Altay, G., Larrañaga, E., Tosi, S., Barriga, F. M., Batlle, E., Fernández-Majada, V., et al. (2019). Self-organized Intestinal Epithelial Monolayers in Crypt and Villus-like Domains Show Effective Barrier Function. Sci. Rep. 9, 10140. doi:10.1038/s41598-019-46497-x
Ayyaz, A., Kumar, S., Sangiorgi, B., Ghoshal, B., Gosio, J., Ouladan, S., et al. (2019). Single-cell Transcriptomes of the Regenerating Intestine Reveal a Revival Stem Cell. Nature 569, 121–125. doi:10.1038/s41586-019-1154-y
Baghdadi, M. B., Ayyaz, A., Coquenlorge, S., Chu, B., Kumar, S., Streutker, C., et al. (2022). Enteric Glial Cell Heterogeneity Regulates Intestinal Stem Cell Niches. Cell Stem Cell 29, 86–100.e6. doi:10.1016/j.stem.2021.10.004
Bar-Ephraim, Y. E., Kretzschmar, K., and Clevers, H. (2020). Organoids in Immunological Research. Nat. Rev. Immunol. 20, 279–293. doi:10.1038/s41577-019-0248-y
Barker, N. (2014). Adult Intestinal Stem Cells: Critical Drivers of Epithelial Homeostasis and Regeneration. Nat. Rev. Mol. Cel Biol. 15, 19–33. doi:10.1038/nrm3721
Barker, N., Van Es, J. H., Kuipers, J., Kujala, P., Van Den Born, M., Cozijnsen, M., et al. (2007). Identification of Stem Cells in Small Intestine and colon by Marker Gene Lgr5. Nature 449, 1003–1007. doi:10.1038/nature06196
Barriga, F. M., Montagni, E., Mana, M., Mendez-Lago, M., Hernando-Momblona, X., Sevillano, M., et al. (2017). Mex3a Marks a Slowly Dividing Subpopulation of Lgr5+ Intestinal Stem Cells. Cell Stem Cell 20, 801–816.e7. doi:10.1016/j.stem.2017.02.007
Barron, M. R., Cieza, R. J., Hill, D. R., Huang, S., Yadagiri, V. K., Spence, J. R., et al. (2020). The Lumen of Human Intestinal Organoids Poses Greater Stress to Bacteria Compared to the Germ-free Mouse Intestine: Escherichia coli Deficient in RpoS as a Colonization Probe. mSphere 5. doi:10.1128/mSphere.00777-20
Basak, O., van de Born, M., Korving, J., Beumer, J., van der Elst, S., van Es, J. H., et al. (2014). Mapping Early Fate Determination in Lgr5+ Crypt Stem Cells Using a Novel Ki67-RFP Allele. EMBO J. 33, 2057–2068. doi:10.15252/embj.201488017
Berger, E., Rath, E., Yuan, D., Waldschmitt, N., Khaloian, S., Allgäuer, M., et al. (2016). Mitochondrial Function Controls Intestinal Epithelial Stemness and Proliferation. Nat. Commun. 7, 1–17. doi:10.1038/ncomms13171
Beumer, J., Artegiani, B., Post, Y., Reimann, F., Gribble, F., Nguyen, T. N., et al. (2018). Enteroendocrine Cells Switch Hormone Expression along the Crypt-To-Villus BMP Signalling Gradient. Nat. Cel Biol. 20, 909–916. doi:10.1038/s41556-018-0143-y
Blumberg, R. S., Li, L., Nusrat, A., Parkos, C. A., Rubin, D. C., and Carrington, J. L. (2008). Recent Insights into the Integration of the Intestinal Epithelium within the Mucosal Environment in Health and Disease. Mucosal Immunol. 1, 330–334. doi:10.1038/mi.2008.29
Bry, L., Falk, P., Huttner, K., Ouellette, A., Midtvedt, T., and Gordon, J. I. (1994). Paneth Cell Differentiation in the Developing Intestine of normal and Transgenic Mice. Proc. Natl. Acad. Sci. 91, 10335–10339. doi:10.1073/pnas.91.22.10335
Calvert, R., Beaulieu, J.-F., and Ménard, D. (1982). Epidermal Growth Factor (EGF) Accelerates the Maturation of Fetal Mouse Intestinal Mucosa In Utero. Experientia 38, 1096–1097. doi:10.1007/bf01955387
Chen, Y., Lin, Y., Davis, K. M., Wang, Q., Rnjak-Kovacina, J., Li, C., et al. (2015). Robust Bioengineered 3D Functional Human Intestinal Epithelium. Sci. Rep. 5, 13708. doi:10.1038/srep13708
Clevers, H. C., and Bevins, C. L. (2013). Paneth Cells: Maestros of the Small Intestinal Crypts. Annu. Rev. Physiol. 75, 289–311. doi:10.1146/annurev-physiol-030212-183744
Clevers, H. (2013). The Intestinal Crypt, a Prototype Stem Cell Compartment. Cell 154, 274–284. doi:10.1016/j.cell.2013.07.004
Clinton, J., and McWilliams-Koeppen, P. (2019). Initiation, Expansion, and Cryopreservation of Human Primary Tissue-Derived Normal and Diseased Organoids in Embedded Three-Dimensional Culture. Curr. Protoc. Cel Biol. 82, e66. doi:10.1002/cpcb.66
Co, J. Y., Margalef-Català, M., Li, X., Mah, A. T., Kuo, C. J., Monack, D. M., et al. (2019). Controlling Epithelial Polarity: A Human Enteroid Model for Host-Pathogen Interactions. Cel Rep. 26, 2509–2520. e4. doi:10.1016/j.celrep.2019.01.108
Co, J. Y., Margalef-Català, M., Monack, D. M., and Amieva, M. R. (2021). Controlling the Polarity of Human Gastrointestinal Organoids to Investigate Epithelial Biology and Infectious Diseases. Nat. Protoc. 16, 5171–5192. doi:10.1038/s41596-021-00607-0
Dekkers, J. F., Alieva, M., Wellens, L. M., Ariese, H. C. R., Jamieson, P. R., Vonk, A. M., et al. (2019). High-resolution 3D Imaging of Fixed and Cleared Organoids. Nat. Protoc. 14, 1756–1771. doi:10.1038/s41596-019-0160-8
Driehuis, E., and Clevers, H. (2017). CRISPR/Cas 9 Genome Editing and its Applications in Organoids. Am. J. Physiology-Gastrointestinal Liver Physiol. 312, G257–G265. doi:10.1152/ajpgi.00410.2016
Dye, B. R., Hill, D. R., Ferguson, M. A., Tsai, Y. H., Nagy, M. S., Dyal, R., et al. (20152015). In Vitro generation of Human Pluripotent Stem Cell Derived Lung Organoids. Elife 4, 1–25. doi:10.7554/eLife.05098
Fair, K. L., Colquhoun, J., and Hannan, N. R. F. (2018). Intestinal Organoids for Modelling Intestinal Development and Disease. Philos. Trans. R. Soc. Lond. B Biol. Sci. 373. doi:10.1098/rstb.2017.0217
Fatehullah, A., Tan, S. H., and Barker, N. (2016). Organoids as an In Vitro Model of Human Development and Disease. Nat. Cel Biol. 18, 246–254. doi:10.1038/ncb3312
Finkbeiner, S. R., Zeng, X. L., Utama, B., Atmar, R. L., Shroyer, N. F., and Estes, M. K. (2012). Stem Cell-Derived Human Intestinal Organoids as an Infection Model for Rotaviruses. MBio 3, e00159–12. doi:10.1128/mBio.00159-12
Finkbeiner, S. R., Hill, D. R., Altheim, C. H., Dedhia, P. H., Taylor, M. J., Tsai, Y.-H., et al. (2015). Transcriptome-wide Analysis Reveals Hallmarks of Human Intestine Development and Maturation In Vitro and In Vivo. Stem Cel Rep. 4, 1140–1155. doi:10.1016/j.stemcr.2015.04.010
Frum, T., and Spence, J. R. (2021). hPSC-derived Organoids: Models of Human Development and Disease. J. Mol. Med. 99, 463–473. doi:10.1007/s00109-020-01969-w
Fujii, M., Matano, M., Toshimitsu, K., Takano, A., Mikami, Y., Nishikori, S., et al. (2018). Human Intestinal Organoids Maintain Self-Renewal Capacity and Cellular Diversity in Niche-Inspired Culture Condition. Cell Stem Cell 23, 787–793.e6. doi:10.1016/j.stem.2018.11.016
Gehart, H., and Clevers, H. (2019). Tales from the Crypt: New Insights into Intestinal Stem Cells. Nat. Rev. Gastroenterol. Hepatol. 16, 19–34. doi:10.1038/s41575-018-0081-y
Gjorevski, N., Sachs, N., Manfrin, A., Giger, S., Bragina, M. E., Ordóñez-Morán, P., et al. (2016). Designer Matrices for Intestinal Stem Cell and Organoid Culture. Nature 539, 560–564. doi:10.1038/nature20168
Gregorieff, A., Liu, Y., Inanlou, M. R., Khomchuk, Y., and Wrana, J. L. (2015). Yap-dependent Reprogramming of Lgr5+ Stem Cells Drives Intestinal Regeneration and Cancer. Nature 526, 715–718. doi:10.1038/nature15382
Guiu, J., Hannezo, E., Yui, S., Demharter, S., Ulyanchenko, S., Maimets, M., et al. (2019). Tracing the Origin of Adult Intestinal Stem Cells. Nature 570, 107–111. doi:10.1038/s41586-019-1212-5
Guiu, J., and Jensen, K. B. (2015). From Definitive Endoderm to Gut-A Process of Growth and Maturation. Stem Cell Dev. 24, 1972–1983. doi:10.1089/scd.2015.0017
Guiu, J., and Jensen, K. B. (2022). In Vivo Studies Should Take Priority when Defining Mechanisms of Intestinal Crypt Morphogenesis. Cell Mol. Gastroenterol. Hepatol. 13, 1–3. doi:10.1016/j.jcmgh.2021.06.028
Han, X., Lee, A., Huang, S., Gao, J., Spence, J. R., and Owyang, C. (2019). Lactobacillus Rhamnosus GG Prevents Epithelial Barrier Dysfunction Induced by Interferon-Gamma and Fecal Supernatants from Irritable Bowel Syndrome Patients in Human Intestinal Enteroids and Colonoids. Gut Microbes 10, 59–76. doi:10.1080/19490976.2018.1479625
Hofer, M., and Lutolf, M. P. (2021). Engineering Organoids. Nat. Rev. Mater. 6, 402–420. doi:10.1038/s41578-021-00279-y
Huch, M., Bonfanti, P., Boj, S. F., Sato, T., Loomans, C. J. M., Van De Wetering, M., et al. (2013a). Unlimited In Vitro Expansion of Adult Bi-potent Pancreas Progenitors through the Lgr5/R-Spondin axis. EMBO J. 32, 2708–2721. doi:10.1038/emboj.2013.204
Huch, M., Dorrell, C., Boj, S. F., Van Es, J. H., Li, V. S. W., Van De Wetering, M., et al. (2013b). In Vitro expansion of Single Lgr5+ Liver Stem Cells Induced by Wnt-Driven Regeneration. Nature 494, 247–250. doi:10.1038/nature11826
Jee, J. H., Lee, D. H., Ko, J., Hahn, S., Jeong, S. Y., Kim, H. K., et al. (2019). Development of Collagen-Based 3D Matrix for Gastrointestinal Tract-Derived Organoid Culture. Stem Cell Int 2019. doi:10.1155/2019/8472712
Kasendra, M., Tovaglieri, A., Sontheimer-Phelps, A., Jalili-Firoozinezhad, S., Bein, A., Chalkiadaki, A., et al. (2018). Development of a Primary Human Small Intestine-On-A-Chip Using Biopsy-Derived Organoids. Sci. Rep. 8, 2871. doi:10.1038/s41598-018-21201-7
Kim, J. E., Fei, L., Yin, W. C., Coquenlorge, S., Rao-Bhatia, A., Zhang, X., et al. (2020b). Single Cell and Genetic Analyses Reveal Conserved Populations and Signaling Mechanisms of Gastrointestinal Stromal Niches. Nat. Commun. 11, 334. doi:10.1038/s41467-019-14058-5
Kim, J., Koo, B.-K., and Knoblich, J. A. (2020a). Human Organoids: Model Systems for Human Biology and Medicine. Nat. Rev. Mol. Cel Biol. 21, 571–584. doi:10.1038/s41580-020-0259-3
Kim, T.-H., Escudero, S., and Shivdasani, R. A. (2012). Intact Function of Lgr5 Receptor-Expressing Intestinal Stem Cells in the Absence of Paneth Cells. Proc. Natl. Acad. Sci. 109, 3932–3937. doi:10.1073/pnas.1113890109
Kip, A. M., Soons, Z., Mohren, R., Duivenvoorden, A. A. M., Röth, A. A. J., Cillero-Pastor, B., et al. (2021). Proteomics Analysis of Human Intestinal Organoids during Hypoxia and Reoxygenation as a Model to Study Ischemia-Reperfusion Injury. Cell Death Dis. 12, 95–13. doi:10.1038/s41419-020-03379-9
Koo, B.-K., Stange, D. E., Sato, T., Karthaus, W., Farin, H. F., Huch, M., et al. (2012). Controlled Gene Expression in Primary Lgr5 Organoid Cultures. Nat. Methods 9, 81–83. doi:10.1038/nmeth.1802
Lancaster, M. A., and Knoblich, J. A. (2014). Organogenesis in a Dish: Modeling Development and Disease Using Organoid Technologies. Science 345, 1247125. doi:10.1126/science.1247125
Leushacke, M., and Barker, N. (2014). Ex Vivo culture of the Intestinal Epithelium: Strategies and Applications. Gut 63, 1345–1354. doi:10.1136/gutjnl-2014-307204
Li, Y., Yang, N., Chen, J., Huang, X., Zhang, N., Yang, S., et al. (2020). Next-Generation Porcine Intestinal Organoids: an Apical-Out Organoid Model for Swine Enteric Virus Infection and Immune Response Investigations. J. Virol. 94. doi:10.1128/JVI.01006-20
Lindeboom, R. G., van Voorthuijsen, L., Oost, K. C., Rodríguez-Colman, M. J., Luna-Velez, M. V., Furlan, C., et al. (2018). Integrative Multi-Omics Analysis of Intestinal Organoid Differentiation. Mol. Syst. Biol. 14, e8227. doi:10.15252/msb.20188227
Lindemans, C. A., Calafiore, M., Mertelsmann, A. M., O’Connor, M. H., Dudakov, J. A., Jenq, R. R., et al. (2015). Interleukin-22 Promotes Intestinal-Stem-Cell-Mediated Epithelial Regeneration. Nature 528, 560–564. doi:10.1038/nature16460
Lukonin, I., Serra, D., Challet Meylan, L., Volkmann, K., Baaten, J., Zhao, R., et al. (2020). Phenotypic Landscape of Intestinal Organoid Regeneration. Nature 586, 275–280. doi:10.1038/s41586-020-2776-9
Mahe, M. M., Sundaram, N., Watson, C. L., Shroyer, N. F., and Helmrath, M. A. (20152015). Establishment of Human Epithelial Enteroids and Colonoids from Whole Tissue and Biopsy. J. Vis. Exp. doi:10.3791/52483
Mahe, M. M., Aihara, E., Schumacher, M. A., Zavros, Y., Montrose, M. H., Helmrath, M. A., et al. (2013). Establishment of Gastrointestinal Epithelial Organoids. Curr. Protoc. Mouse Biol. 3, 217–240. doi:10.1002/9780470942390.mo130179
Maschmeyer, I., Hasenberg, T., Jaenicke, A., Lindner, M., Lorenz, A. K., Zech, J., et al. (2015a). Chip-based Human Liver-Intestine and Liver-Skin Co-cultures - A First Step toward Systemic Repeated Dose Substance Testing In Vitro. Eur. J. Pharmaceutics Biopharmaceutics 95, 77–87. doi:10.1016/j.ejpb.2015.03.002
Maschmeyer, I., Lorenz, A. K., Schimek, K., Hasenberg, T., Ramme, A. P., Hübner, J., et al. (2015b). A Four-Organ-Chip for Interconnected Long-Term Co-culture of Human Intestine, Liver, Skin and Kidney Equivalents. Lab. Chip 15, 2688–2699. doi:10.1039/c5lc00392j
McCarthy, N., Kraiczy, J., and Shivdasani, R. A. (2020a). Cellular and Molecular Architecture of the Intestinal Stem Cell Niche. Nat. Cel Biol. 22, 1033–1041. doi:10.1038/s41556-020-0567-z
McCarthy, N., Manieri, E., Storm, E. E., Saadatpour, A., Luoma, A. M., Kapoor, V. N., et al. (2020b). Distinct Mesenchymal Cell Populations Generate the Essential Intestinal BMP Signaling Gradient. Cell Stem Cell 26, 391–402.e5. doi:10.1016/j.stem.2020.01.008
McCracken, K. W., Howell, J. C., Wells, J. M., and Spence, J. R. (2011). Generating Human Intestinal Tissue from Pluripotent Stem Cells In Vitro. Nat. Protoc. 6, 1920–1928. doi:10.1038/nprot.2011.410
Ménard, D., Arsenault, P., and Pothier, P. (1988). Biologic Effects of Epidermal Growth Factor in Human Fetal Jejunum. Gastroenterology 94, 656–663.
Mithal, A., Capilla, A., Heinze, D., Berical, A., Villacorta-Martin, C., Vedaie, M., et al. (2020). Generation of Mesenchyme Free Intestinal Organoids from Human Induced Pluripotent Stem Cells. Nat. Commun. 11, 215. doi:10.1038/s41467-019-13916-6
Moor, A. E., Harnik, Y., Ben-Moshe, S., Massasa, E. E., Rozenberg, M., Eilam, R., et al. (2018). Spatial Reconstruction of Single Enterocytes Uncovers Broad Zonation along the Intestinal Villus Axis. Cell 175, 1156–1167.e15. doi:10.1016/j.cell.2018.08.063
Murata, K., Jadhav, U., Madha, S., van Es, J., Dean, J., Cavazza, A., et al. (2020). Ascl2-Dependent Cell Dedifferentiation Drives Regeneration of Ablated Intestinal Stem Cells. Cell Stem Cell 26, 377–390.e6. doi:10.1016/j.stem.2019.12.011
Murphy, S. V., and Atala, A. (2014). 3D Bioprinting of Tissues and Organs. Nat. Biotechnol. 32, 773–785. doi:10.1038/nbt.2958
Nguyen, T. L. A., Vieira-Silva, S., Liston, A., and Raes, J. (2015). How Informative Is the Mouse for Human Gut Microbiota Research? Dis. Model. Mech. 8, 1–16. doi:10.1242/dmm.017400
Nikolaev, M., Mitrofanova, O., Broguiere, N., Geraldo, S., Dutta, D., Tabata, Y., et al. (2020). Homeostatic Mini-Intestines through Scaffold-Guided Organoid Morphogenesis. Nature 585, 574–578. doi:10.1038/s41586-020-2724-8
Nishinakamura, R. (2019). Human Kidney Organoids: Progress and Remaining Challenges. Nat. Rev. Nephrol. 15, 613–624. doi:10.1038/s41581-019-0176-x
Norkin, M., Ordóñez-Morán, P., and Huelsken, J. (2021). High-content, Targeted RNA-Seq Screening in Organoids for Drug Discovery in Colorectal Cancer. Cel Rep. 35, 109026. doi:10.1016/j.celrep.2021.109026
Ogasawara, R., Hashimoto, D., Kimura, S., Hayase, E., Ara, T., Takahashi, S., et al. (2018). Intestinal Lymphatic Endothelial Cells Produce R-Spondin3. Sci. Rep. 8, 10719–9. doi:10.1038/s41598-018-29100-7
Onozato, D., Ogawa, I., Kida, Y., Mizuno, S., Hashita, T., Iwao, T., et al. (2021). Generation of Budding-like Intestinal Organoids from Human Induced Pluripotent Stem Cells. J. Pharm. Sci. 110, 2637–2650. doi:10.1016/j.xphs.2021.03.014
Palikuqi, B., Nguyen, D.-H. T., Li, G., Schreiner, R., Pellegata, A. F., Liu, Y., et al. (2020). Adaptable Haemodynamic Endothelial Cells for Organogenesis and Tumorigenesis. Nature 585, 426–432. doi:10.1038/s41586-020-2712-z
Panek, M., Grabacka, M., and Pierzchalska, M. (2018). The Formation of Intestinal Organoids in a Hanging Drop Culture. Cytotechnology 70, 1085–1095. doi:10.1007/s10616-018-0194-8
Paris, F., Fuks, Z., Kang, A., Capodieci, P., Juan, G., Ehleiter, D., et al. (2001). Endothelial Apoptosis as the Primary Lesion Initiating Intestinal Radiation Damage in Mice. Science 80-293, 293–297. doi:10.1126/science.1060191
Porter, E. M., Bevins, C. L., Ghosh, D., and Ganz, T. (2002). The Multifaceted Paneth Cell. Cell Mol. Life Sci. (Cmls) 59, 156–170. doi:10.1007/s00018-002-8412-z
Rezakhani, S., Gjorevski, N., and Lutolf, M. P. (2021). Extracellular Matrix Requirements for Gastrointestinal Organoid Cultures. Biomaterials 276, 121020. doi:10.1016/j.biomaterials.2021.121020
Rumbo, M., and Schiffrin, E. J. (2005). Intestinal Epithelial Barrier and Mucosal Immunity. Cmls, Cel. Mol. Life Sci. 62, 1288–1296. doi:10.1007/s00018-005-5033-3
Sachs, N., Tsukamoto, Y., Kujala, P., Peters, P. J., and Clevers, H. (2017). Intestinal Epithelial Organoids Fuse to Form Self-Organizing Tubes in Floating Collagen Gels. Dev 144, 1107–1112. doi:10.1242/dev.143933
Sarvestani, S. K., Signs, S., Hu, B., Yeu, Y., Feng, H., Ni, Y., et al. (2021). Induced Organoids Derived from Patients with Ulcerative Colitis Recapitulate Colitic Reactivity. Nat. Commun. 12, 1–18. doi:10.1038/s41467-020-20351-5
Sato, T., and Clevers, H. (2013). Growing Self-Organizing Mini-Guts from a Single Intestinal Stem Cell: Mechanism and Applications. Science 340 (80-), 1190–1194. doi:10.1126/science.1234852
Sato, T., Stange, D. E., Ferrante, M., Vries, R. G. J., Van Es, J. H., Van Den Brink, S., et al. (2011b). Long-term Expansion of Epithelial Organoids from Human Colon, Adenoma, Adenocarcinoma, and Barrett's Epithelium. Gastroenterology 141, 1762–1772. doi:10.1053/j.gastro.2011.07.050
Sato, T., Van Es, J. H., Snippert, H. J., Stange, D. E., Vries, R. G., Van Den Born, M., et al. (2011a). Paneth Cells Constitute the Niche for Lgr5 Stem Cells in Intestinal Crypts. Nature 469, 415–418. doi:10.1038/nature09637
Sato, T., Vries, R. G., Snippert, H. J., Van De Wetering, M., Barker, N., Stange, D. E., et al. (2009). Single Lgr5 Stem Cells Build Crypt-Villus Structures In Vitro without a Mesenchymal Niche. Nature 459, 262–265. doi:10.1038/nature07935
Schuijers, J., and Clevers, H. (2012). Adult Mammalian Stem Cells: The Role of Wnt, Lgr5 and R-Spondins. EMBO J. 31, 2685–2696. doi:10.1038/emboj.2012.149
Schwank, G., and Clevers, H. (2016). CRISPR/Cas9-Mediated Genome Editing of Mouse Small Intestinal Organoids. Methods Mol. Biol. 1422, 3–11. doi:10.1007/978-1-4939-3603-8_1
Schwank, G., Koo, B.-K., Sasselli, V., Dekkers, J. F., Heo, I., Demircan, T., et al. (2013). Functional Repair of CFTR by CRISPR/Cas9 in Intestinal Stem Cell Organoids of Cystic Fibrosis Patients. Cell Stem Cell 13, 653–658. doi:10.1016/j.stem.2013.11.002
Schweinlin, M., Wilhelm, S., Schwedhelm, I., Hansmann, J., Rietscher, R., Jurowich, C., et al. (2016). Development of an Advanced Primary Human In Vitro Model of the Small Intestine. Tissue Eng. C: Methods 22, 873–883. doi:10.1089/ten.tec.2016.0101
Seidlitz, T., Koo, B.-K., and Stange, D. E. (2021). Gastric Organoids-An In Vitro Model System for the Study of Gastric Development and Road to Personalized Medicine. Cell Death Differ 28, 68–83. doi:10.1038/s41418-020-00662-2
Shoshkes-Carmel, M., Wang, Y. J., Wangensteen, K. J., Tóth, B., Kondo, A., Massasa, E. E., et al. (2018). Subepithelial Telocytes Are an Important Source of Wnts that Supports Intestinal Crypts. Nature 557, 242–246. doi:10.1038/s41586-018-0084-4
Snippert, H. J., van der Flier, L. G., Sato, T., van Es, J. H., van den Born, M., Kroon-Veenboer, C., et al. (2010). Intestinal Crypt Homeostasis Results from Neutral Competition between Symmetrically Dividing Lgr5 Stem Cells. Cell 143, 134–144. doi:10.1016/j.cell.2010.09.016
Spence, J. R., Mayhew, C. N., Rankin, S. A., Kuhar, M. F., Vallance, J. E., Tolle, K., et al. (2011). Directed Differentiation of Human Pluripotent Stem Cells into Intestinal Tissue In Vitro. Nature 470, 105–109. doi:10.1038/nature09691
Stelzner, M., Helmrath, M., Dunn, J. C. Y., Henning, S. J., Houchen, C. W., Kuo, C., et al. (2012). A Nomenclature for Intestinal In Vitro Cultures. Am. J. Physiology-Gastrointestinal Liver Physiol. 302, G1359–G1363. doi:10.1152/ajpgi.00493.2011
Stroulios, G., Stahl, M., Elstone, F., Chang, W., Louis, S., Eaves, A., et al. (2021). Culture Methods to Study Apical-specific Interactions Using Intestinal Organoid Models. J. Vis. Exp. 2021. doi:10.3791/62330
Stzepourginski, I., Nigro, G., Jacob, J.-M., Dulauroy, S., Sansonetti, P. J., Eberl, G., et al. (2017). CD34+ Mesenchymal Cells Are a Major Component of the Intestinal Stem Cells Niche at Homeostasis and after Injury. Proc. Natl. Acad. Sci. USA 114, E506–E513. doi:10.1073/pnas.1620059114
Sugimoto, S., and Sato, T. (2022). Organoid vs In Vivo Mouse Model: Which Is Better Research Tool to Understand the Biologic Mechanisms of Intestinal Epithelium? Cell Mol. Gastroenterol. Hepatol. 13, 195–197. doi:10.1016/j.jcmgh.2021.06.027
Tetteh, P. W., Basak, O., Farin, H. F., Wiebrands, K., Kretzschmar, K., Begthel, H., et al. (2016). Replacement of Lost Lgr5-Positive Stem Cells through Plasticity of Their Enterocyte-Lineage Daughters. Cell Stem Cell 18, 203–213. doi:10.1016/j.stem.2016.01.001
Ueda, T., Yamada, T., Hokuto, D., Koyama, F., Kasuda, S., Kanehiro, H., et al. (2010). Generation of Functional Gut-like Organ from Mouse Induced Pluripotent Stem Cells. Biochem. Biophysical Res. Commun. 391, 38–42. doi:10.1016/j.bbrc.2009.10.157
van Es, J. H., Jay, P., Gregorieff, A., van Gijn, M. E., Jonkheer, S., Hatzis, P., et al. (2005). Wnt Signalling Induces Maturation of Paneth Cells in Intestinal Crypts. Nat. Cel Biol. 7, 381–386. doi:10.1038/ncb1240
Van Es, J. H., Sato, T., Van De Wetering, M., Lyubimova, A., Yee Nee, A. N., Gregorieff, A., et al. (2012). Dll1+ Secretory Progenitor Cells Revert to Stem Cells upon Crypt Damage. Nat. Cel Biol. 14, 1099–1104. doi:10.1038/ncb2581
Van Es, J. H., Wiebrands, K., López-Iglesias, C., Van De Wetering, M., Zeinstra, L., Van Den Born, M., et al. (2019). Enteroendocrine and Tuft Cells Support Lgr5 Stem Cells on Paneth Cell Depletion. Proc. Natl. Acad. Sci. USA 116, 26599–26605. doi:10.1073/pnas.1801888117
Watson, C. L., Mahe, M. M., Múnera, J., Howell, J. C., Sundaram, N., Poling, H. M., et al. (2014). An In Vivo Model of Human Small Intestine Using Pluripotent Stem Cells. Nat. Med. 20, 1310–1314. doi:10.1038/nm.3737
Wells, J. M., and Spence, J. R. (2014). How to Make an Intestine. Dev 141, 752–760. doi:10.1242/dev.097386
Wengerter, B. C., Emre, G., Park, J. Y., and Geibel, J. (2016). Three-dimensional Printing in the Intestine. Clin. Gastroenterol. Hepatol. 14, 1081–1085. doi:10.1016/j.cgh.2016.05.008
Wilson, S. S., Tocchi, A., Holly, M. K., Parks, W. C., and Smith, J. G. (2015). A Small Intestinal Organoid Model of Non-invasive Enteric Pathogen-Epithelial Cell Interactions. Mucosal Immunol. 8, 352–361. doi:10.1038/mi.2014.72
Wright, N. A. (2000). Epithelial Stem Cell Repertoire in the Gut: Clues to the Origin of Cell Lineages, Proliferative Units and Cancer. Int. J. Exp. Pathol. 81, 117–143. doi:10.1046/j.1365-2613.2000.00146.x
Yan, K. S., Gevaert, O., Zheng, G. X. Y., Anchang, B., Probert, C. S., Larkin, K. A., et al. (2017). Intestinal Enteroendocrine Lineage Cells Possess Homeostatic and Injury-Inducible Stem Cell Activity. Cell Stem Cell 21, 78–90. e6. doi:10.1016/j.stem.2017.06.014
Yang, S., and Yu, M. (2021). Role of Goblet Cells in Intestinal Barrier and Mucosal Immunity. Jir Vol. 14, 3171–3183. doi:10.2147/jir.s318327
Yu, S., Tong, K., Zhao, Y., Balasubramanian, I., Yap, G. S., Ferraris, R. P., et al. (2018). Paneth Cell Multipotency Induced by Notch Activation Following Injury. Cell Stem Cell 23, 46–59.e5. doi:10.1016/j.stem.2018.05.002
Yui, S., Azzolin, L., Maimets, M., Pedersen, M. T., Fordham, R. P., Hansen, S. L., et al. (2018). YAP/TAZ-Dependent Reprogramming of Colonic Epithelium Links ECM Remodeling to Tissue Regeneration. Cell Stem Cell 22, 35–49.e7. doi:10.1016/j.stem.2017.11.001
Zachos, N. C., Kovbasnjuk, O., Foulke-Abel, J., In, J., Blutt, S. E., de Jonge, H. R., et al. (2016). Human Enteroids/colonoids and Intestinal Organoids Functionally Recapitulate normal Intestinal Physiology and Pathophysiology. J. Biol. Chem. 291, 3759–3766. doi:10.1074/jbc.r114.635995
Zhao, X., Li, C., Liu, X., Chiu, M. C., Wang, D., Wei, Y., et al. (2021). Human Intestinal Organoids Recapitulate Enteric Infections of Enterovirus and Coronavirus. Stem Cel Rep. 16, 493–504. doi:10.1016/j.stemcr.2021.02.009
Zhu, P., Zhu, X., Wu, J., He, L., Lu, T., Wang, Y., et al. (2019). IL-13 Secreted by ILC2s Promotes the Self-Renewal of Intestinal Stem Cells through Circular RNA circPan3. Nat. Immunol. 20, 183–194. doi:10.1038/s41590-018-0297-6
Keywords: organoids, 3D models, intestine, human intestinal organoids, enteroids
Citation: Taelman J, Diaz M and Guiu J (2022) Human Intestinal Organoids: Promise and Challenge. Front. Cell Dev. Biol. 10:854740. doi: 10.3389/fcell.2022.854740
Received: 14 January 2022; Accepted: 24 February 2022;
Published: 11 March 2022.
Edited by:
Keiichiro Suzuki, Osaka University, JapanReviewed by:
Nandor Nagy, Semmelweis University, HungaryCopyright © 2022 Taelman, Diaz and Guiu. This is an open-access article distributed under the terms of the Creative Commons Attribution License (CC BY). The use, distribution or reproduction in other forums is permitted, provided the original author(s) and the copyright owner(s) are credited and that the original publication in this journal is cited, in accordance with accepted academic practice. No use, distribution or reproduction is permitted which does not comply with these terms.
*Correspondence: Jordi Guiu, amd1aXVAaWRpYmVsbC5jYXQ=
Disclaimer: All claims expressed in this article are solely those of the authors and do not necessarily represent those of their affiliated organizations, or those of the publisher, the editors and the reviewers. Any product that may be evaluated in this article or claim that may be made by its manufacturer is not guaranteed or endorsed by the publisher.
Research integrity at Frontiers
Learn more about the work of our research integrity team to safeguard the quality of each article we publish.