- Molecular Cell Biology, Groningen Biomolecular Sciences and Biotechnology Institute, University of Groningen, Groningen, Netherlands
In the yeast Hansenula polymorpha the peroxisomal membrane protein Pex11 and three endoplasmic reticulum localized proteins of the Pex23 family (Pex23, Pex24 and Pex32) are involved in the formation of peroxisome-ER contact sites. Previous studies suggested that these contacts are involved in non-vesicular lipid transfer and important for expansion of the peroxisomal membrane. The absence of Pex32 results in a severe peroxisomal phenotype, while cells lacking Pex11, Pex23 or Pex24 show milder defects and still are capable to form peroxisomes and grow on methanol. We performed transposon mutagenesis on H. polymorpha pex11 cells and selected mutants that lost the capacity to grow on methanol and are severely blocked in peroxisome formation. This strategy resulted in the identification of Vps13, a highly conserved contact site protein involved in bulk lipid transfer. Our data show that peroxisome formation and function is normal in cells of a vps13 single deletion strain. However, Vps13 is essential for peroxisome biogenesis in pex11. Notably, Vps13 is also required for peroxisome formation in pex23 and pex24 cells. These data suggest that Vps13 is crucial for peroxisome formation in cells with reduced peroxisome-endoplasmic reticulum contact sites and plays a redundant function in lipid transfer from the ER to peroxisomes.
Introduction
Peroxisomes are ubiquitous organelles. Their function and abundance continuously changes in response to cellular needs (Smith and Aitchison, 2013). During peroxisome growth, the organelles incorporate matrix and membrane proteins a well as membrane lipids. In mammals, membrane contact sites (MCSs) between peroxisomes and the endoplasmic reticulum (ER) function in non-vesicular transport of lipids from the ER to the peroxisomal membrane. These MCSs contain peroxisome bound members of the Acyl-CoA binding domain containing proteins (ACBDs), ACBD5 and ACBD4, and the ER-localized VAP proteins VAPA and VAPB. VAP proteins are highly conserved ER membrane proteins that play a role in various processes, including lipid transport (Lev et al., 2008). At mammalian peroxisome-ER contact sites ACBD5/ACBD4 interact through a FFAT-like motif with both VAP proteins (Costello et al., 2017; Hua et al., 2017; Islinger et al., 2020). Recent studies showed that human VPS13D, a bulk lipid transporter, is also important for peroxisome biogenesis and transport of lipids from the ER to peroxisomes (Baldwin et al., 2021; Guillen-Samander et al., 2021).
Studies in Saccharomyces cerevisiae revealed that also in this organism the peroxisomal membrane can receive membrane lipids via non-vesicular transport (Raychaudhuri and Prinz, 2008). These lipids may derive from various sources including the ER, the vacuole, the mitochondrion and the Golgi apparatus (Rosenberger et al., 2009; Flis et al., 2015). Also, in S. cerevisiae peroxisomes form contact sites with many other cellular membranes (Shai et al., 2016; Shai et al., 2018). However, proteins involved in non-vesicular lipid transport to yeast peroxisomes have not been identified yet. In the yeast Hansenula polymorpha peroxisomes can form various MCSs. Contacts have been described with the plasma membrane, the ER, mitochondria and vacuoles (Wu et al., 2019). ER-localized peroxins of the Pex23 family (Pex23, Pex24 and Pex32) together with the peroxisomal membrane protein (PMP) Pex11 play a role in the formation of peroxisome-ER contacts (Wu et al., 2020). Similarly, members of the S. cerevisiae Pex23 family (called Pex28, Pex29, Pex30, Pex31 and Pex32 (Jansen et al., 2021)) have been implicated in the formation of peroxisome ER contact sites (David et al., 2013; Mast et al., 2016). S. cerevisiae Pex23 family proteins also have been implicated in other processes, such as the regulation of pre-peroxisomal vesicle (PPV) formation from the ER (David et al., 2013; Joshi et al., 2016; Mast et al., 2016; Wang et al., 2018) and the biogenesis of lipid bodies (Joshi et al., 2018). Studies in S. cerevisiae suggested that Inp1, a protein essential for retention of peroxisomes in yeast mother cells, plays a role in the formation of peroxisome-ER contact sites (Knoblach et al., 2013). However, recent studies showed that Inp1 associates peroxisomes to the plasma membrane (Hulmes et al., 2020; Krikken et al., 2020).
The absence of H. polymorpha Pex23, Pex24 or Pex32 leads to reduction, but not a complete loss, of peroxisome-ER contacts. This reduction is accompanied by a decrease in the cellular peroxisomal membrane surface, suggesting that these peroxisome-ER contacts are important for lipid transfer.
The absence of the H. polymorpha Pex32 causes the most severe peroxisome-ER MCS defect, which is accompanied by mislocalization of a portion of the peroxisomal matrix proteins to the cytosol. As a consequence pex32 cells are unable to grown on media containing methanol as sole carbon source (van der Klei et al., 2006). pex23, pex24 and pex11 cells show milder peroxisomal defects and still are capable to grow on methanol, although the doubling times are increased (Krikken et al., 2009; Wu et al., 2020).
We hypothesized that these weaker phenotypes are due to functional redundancy of proteins of the peroxisome-ER MCS. To identify these redundant proteins, we performed transposon mutagenesis of H. polymorpha pex11 cells and selected mutants that fully lost the capacity to grow on methanol. This screen resulted in the identification of Vps13, a highly conserved protein that is responsible for lipid transport and localizes to multiple MCSs in eukaryotic cells. We show that like cells of the pex11 vps13 double deletion strain, also pex23 vps13 and pex24 vps13 cells are unable to utilize methanol. In these double deletion strains very small peroxisomes still occur. PMPs are normally sorted to these organelles, but the bulk of the matrix proteins mislocalize to the cytosol. This suggests that peroxisomes can still form but are unable to grow and incorporate all matrix proteins. Cells of a single vps13 deletion strain contain normal peroxisomes and grow on methanol like wild type control cells.
These data indicate that Vps13 is essential for peroxisome growth in cells that have reduced peroxisome-ER MCSs.
Materials and Methods
Strains and Growth Conditions
The H. polymorpha and S. cerevisiae strains used in this study are listed in the Supplementary Table S1and S2. H. polymorpha cells were grown in batch cultures at 37°C on mineral media (MM) (van Dijken et al., 1976) containing 0.5% glucose, 0.5% methanol or a mixture of 0.5% methanol and 0.05% glycerol (MM-M/G) as carbon sources and 0.25% ammonium sulfate as nitrogen sources. S. cerevisiae cells were grown at 30°C on media containing 0.5% glucose and 0.25% ammonium sulfate. When required, amino acids were added to the media to a final concentration of 30 μg/ml. Escherichia coli DH5α and DB3.1 were used for cloning.
Plasmids and Molecular Techniques
GFP-SKL and DsRed-SKL are peroxisomal matrix markers appended with the peroxisomal targeting signal -SKL. In H. polymorpha the encoding genes were expressed under control of the TEF1 or AOX promoter, in S. cerevisiae under control of the MET25 promoter. For expression of H. polymorpha genes encoding various peroxisomal membrane proteins under control of their endogenous promoter, approximately 500 nucleotides upstream from the ORF were included. For the expression under control of a strong promoter the full length gene was cloned. All plasmids were linearized and integrated in the genome as described before (Faber et al., 1994). Plasmids used in this study are listed in Supplementary Table S3. All deletions were confirmed by Southern blotting. For DNA and amino acid sequence analysis, the Clone Manager 5 program (Scientific and Educational Software, Durham, NC) was used. Transposon mutagenesis of H. polymorpha pex11 DsRed-SKL, isolation of total genomic DNA and sequencing of genomic insert was performed as described before (van Dijk et al., 2001).
DNA restriction enzymes were used as recommended by the suppliers (Thermo Scientific or New England Biolabs). Polymerase chain reactions (PCR) for cloning were carried out with Phusion High-Fidelity DNA Polymerase (Thermo Scientific). Colony PCR was carried out using Phire polymerase (Thermo Scientific). For DNA and amino acid sequence analysis, the Clone Manager 5 program (Scientific and Educational Software, Durham, NC) was used.
Microscopy
Fluorescence microscopy images were captured using an Axio Scope A1 (Carl Zeiss) with a 100 × 1.30 NA Plan Neofluar objective, Micro-Manager 1.4 software and a Coolsnap HQ2 camera (Photometrics). GFP was visualized with a 470/40 nm band pass excitation filter, a 495 nm dichromatic mirror, and a 525/50 nm band-pass emission filter. mCherry was visualized with a 587/25 nm band pass excitation filter, a 605 nm dichromatic mirror, and a 647/70 nm band-pass emission filter. DsRed fluorescence was visualized with a 546/12 nm bandpass excitation filter, a 560 nm dichromatic mirror, and a 575–640 nm bandpass emission filter. Image analysis was carried out using ImageJ and Adobe Photoshop CS6 software.
To quantify peroxisomes random images of cells were taken as a stack using a confocal microscope (LSM510, Carl Zeiss) and photomultiplier tubes (Hamamatsu Photonics) and Zen 2009 software (Carl Zeiss). Z-Stack images were made containing 14 optical slices and the GFP signal was visualized by excitation with a 488 nm argon ion laser (Lasos), and a 500–550 nm bandpass emission filter. Peroxisomes were quantified using a custom made plugin for ImageJ (Thomas et al., 2015).
Electron microscopy was performed as described previously (Knoops et al., 2014). For morphological analysis cells were fixed in 1.5% potassium permanganate, post-stained with 0.5% uranyl acetate and embedded in Epon. Immuno-EM was performed as described previously (Knoops et al., 2014) using anti-Pex14 antibodies (Komori et al., 1997) followed by goat-anti-mouse antibodies conjugated to 6 nm gold (Aurion, Netherlands). Ultrathin sections were viewed in a Philips CM12 TEM.
Results
Vps13 is Required for Peroxisome Biogenesis in Yeast pex11 Cells
Previously, we showed that like H. polymorpha pex11, pex23 and pex24 cells still contain peroxisomes (Krikken et al., 2009; Wu et al., 2020). However, peroxisome numbers are reduced accompanied by the occurrence of organelles with a relatively large diameter. We confirmed these observations by quantitative analysis of fluorescence microscopy (FM) images of these three deletion strains. The percentage of relatively large peroxisomes (diameter >1 µm) is significantly enhanced in pex11, pex23 and pex24 cells (Figures 1A,B). The lowest peroxisome numbers were observed in pex11 cells (Figures 1A,C), which we selected to perform transposon mutagenesis.
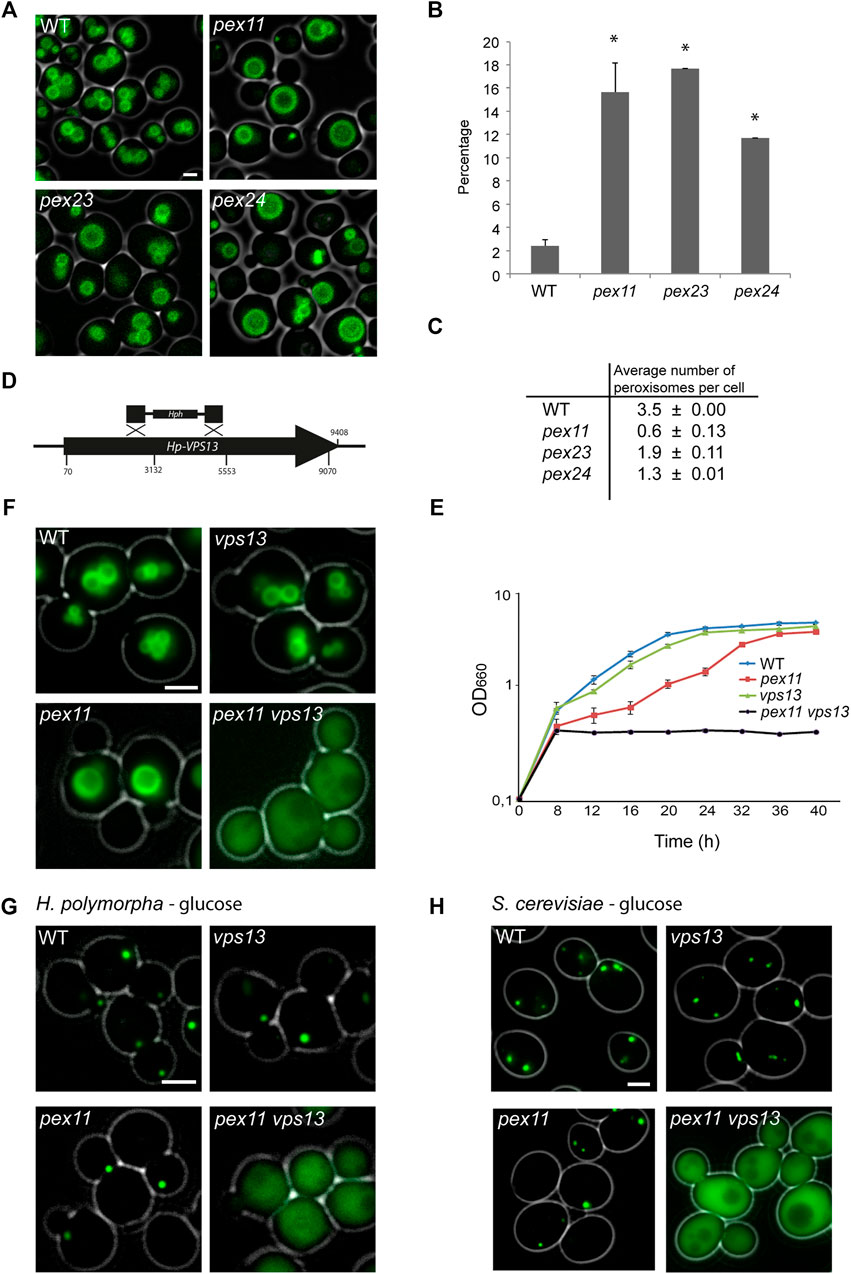
FIGURE 1. VPS13 is required for peroxisome formation in pex11 cells. H. polymorpha cells were grown for 16 h on methanol medium unless indicated otherwise. (A) FM analysis of the indicated H. polymorpha strains producing the peroxisomal membrane marker PMP47-GFP. Scale bar 2 µm. (B) Percentage of peroxisomes with a diameter >1 μm. 2 × 660 peroxisomes from two independent cultures were quantified. Two-tailed Student’s t test was performed. *, p < 0.05. pex11: 0.016, pex23: 0.003, pex24: 0.005. The error bars represent standard deviation (SD). (C) Average number of peroxisomes per cell (±SD) of the indicated strains. 2 × 660 cells from two independent cultures were quantified. (D) Schematic representation of the H. polymorpha VPS13 gene, showing the four positions where transposon insertion occurred as well as the region that was replaced (nt 2430–5436) by Zeor to disrupt VPS13. (E) Growth curves of the indicated H. polymorpha strains on methanol medium. Error bars represent SD (n = 2). (F) FM images of the indicated H. polymorpha strains producing GFP-SKL. Cells were grown for 16 h on medium containing a mixture of methanol and glycerol. Scale bar: 2 µm. (G) FM images of the indicated strains grown on glucose medium (pex11.PTEFGFP-SKL, vps13PTEFGFP-SKL and pex11 vps13PTEFGFP-SKL). (H) FM analysis of the indicated S. cerevisiae strains producing GFP-SKL and grown on glucose. Scale bars: 2 μm.
Transformants were isolated that were still capable to grow on glucose, but not on methanol (Mut−), indicative for severe peroxisome biogenesis defects. FM analysis revealed that out of the 100 Mut− strains obtained, 42 displayed mislocalization of the red fluorescent peroxisomal matrix marker DsRed-SKL. Sequencing of the genomic regions flanking the integrated pREMI-Z cassette resulted in the identification of 17 different genes (Supplementary Table S4). As expected these included various PEX genes, because mutations in most PEX genes result in a Mut− phenotype due to mislocalization of matrix proteins. In 9 of the 17 identified mutants the transposon was integrated in VPS13. In four mutants the transposon was integrated at different positions in the VPS13 open reading frame, whereas in the remaining five mutants deletions or truncations of the VPS13 gene occurred (Figure 1D).
To validate this result, we constructed a pex11 vps13 double mutant (Figure 1D). Cells of this strain, but not of the pex11 or vps13 single deletion strains, were unable to grow on methanol (Figure 1E). Moreover, peroxisomal matrix proteins were mislocalized to the cytosol of cells in the pex11 vps13 double deletion strain, but not in pex11 or vps13 cells. This phenotype was observed both when cells were grown in peroxisome inducing media (methanol; Figure 1F) or peroxisome repressing media (glucose; Figure 1G).
In S. cerevisiae essentially the same observations were made: the peroxisome matrix marker GFP-SKL was properly imported into peroxisomes of pex11 and vps13 cells, but mislocalized to the cytosol in pex11 vps13 double deletion cells (Figure 1H).
Summarizing, in H. polymorpha and S. cerevisiae deletion of VPS13 in a pex11 deletion strain strongly affects peroxisome biogenesis.
pex11 vps13 Cells Contain Small, Import Competent Peroxisomes
Electron microscopy (EM) revealed that H. polymorpha pex11 vps13 cells harbor clusters of very small peroxisomes (Figures 2AI,II), which contain the peroxisomal membrane marker Pex14 (Figure 2AIII). In the larger organelles small alcohol oxidase (AO) crystalloids could be observed, indicating that these peroxisomes still are capable of importing of matrix protein (Figure 2AIII, inset). The bulk of the AO protein mislocalizes to the cytosol, as evident from the large AO crystalloids in the cytosol of the double mutant (Figure 2AIV). Fluorescence microscopy showed that Pex14-mCherry localized in spots in pex11 vps13 cells, which most likely represent the clusters of small peroxisomes observed by EM (Figure 2B). All other PMPs, C-terminally tagged with GFP and produced under the control of their endogenous promoters, co-localized with Pex14-mCherry (Figure 2B). Based on these data we conclude that PMPs normally localize to the membranes of the small peroxisomes in pex11 vps13 cells. The small peroxisomes are competent to import a minor fraction of the matrix proteins, but the bulk of the matrix proteins mislocalizes to the cytosol.
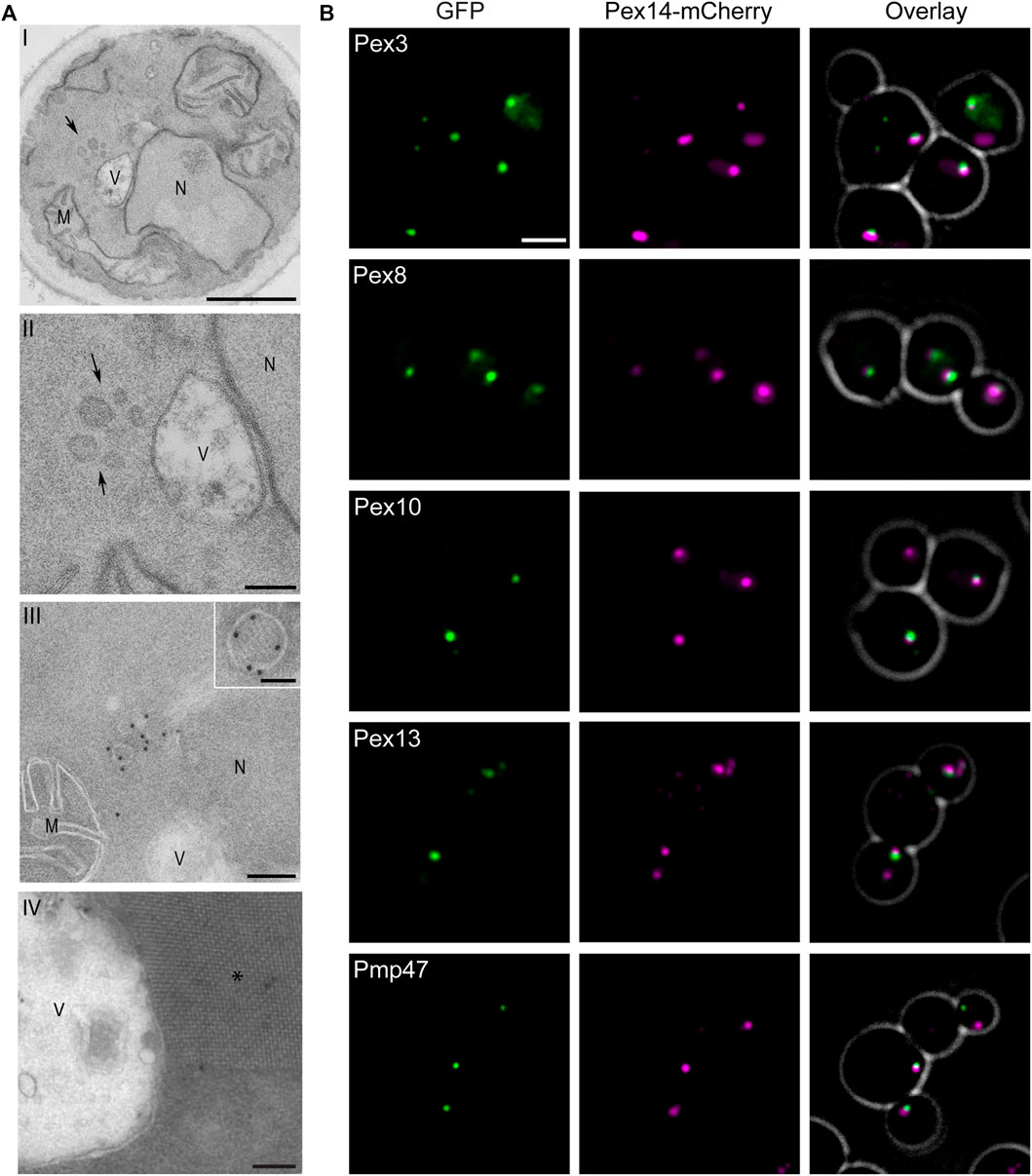
FIGURE 2. H. polymorpha pex11 vps13 cells contain small peroxisomes. (A) Electron microscopy analysis of thin sections of KMnO4-fixed pex11 vps13 cells grown for 8 h on a mixture of glycerol and methanol. Cells contain clusters of small peroxisomes (arrows). I—overview, II—magnification. III—Immunolabelling of cryosection of pex11 vps13 cells using anti-Pex14 antibodies. The inset shows a small peroxisome labelled with anti-Pex14 antibodies, containing an alcohol oxidase crystalloid. IV—Cryosection showing a large, cytosolic alchol oxidase crystalloid. Scale bars: I: 500 nm, II: 100 nm, III 100 nm, inset: 50 nm, IV—100 nm. M-mitochondrion; N—nucleus, V-vacuole. (B) FM images of pex11 vps13 cells producing Pex14-mCherry together with the indicated mGFP fusion proteins, all produced under control of the endogenous promoters. Cells were grown for 8 h on glycerol/methanol medium. Cells producing Pex10-GFP were grown for 4 h on glycerol/methanol. Scale bar: 2 µm.
VPS13 is also Required for Peroxisome Biogenesis in H. polymorpha pex23 and pex24 Cells
Next, we analyzed whether deletion of VPS13 also affects peroxisome biogenesis in pex23 and pex24 cells, two other mutants in which peroxisome-ER MCSs are reduced (Wu et al., 2020). As shown in Figure 3A, peroxisomal matrix markers mislocalized to the cytosol in pex23 vps13 and pex24 vps13 cells. In line with these observations, both double deletion strains are unable to grow on methanol, while pex23 and pex24 single deletion strains grow on methanol (Figure 3B).
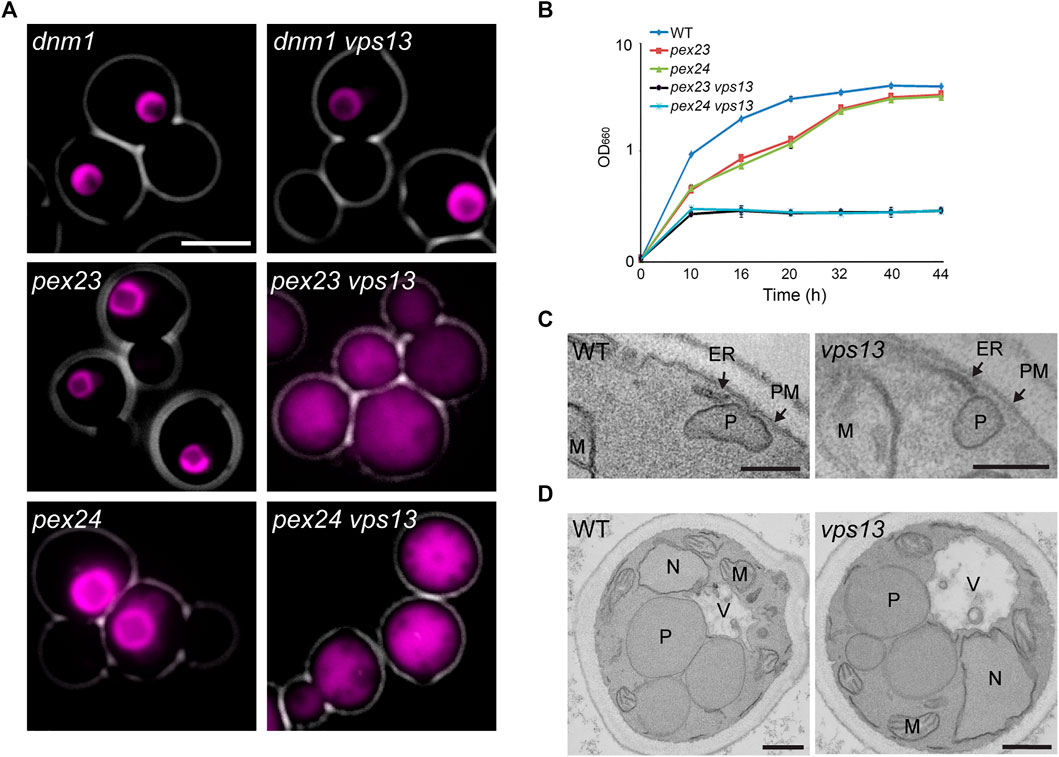
FIGURE 3. H. polymorpha pex23 vps13 and pex24 vps13 cells show a severe peroxisome biogenesis defect. (A) FM analysis of the indicated strains producing DsRed-SKL or GFP-SKL, grown for 16 h on a mixture of glycerol and methanol. Scale bar: 2 μm. (B) Growth curves of the indicated strains on medium containing methanol. The optical density is expressed as OD660. Error bars represent SD (n = 2). (C,D) Electron microscopy analysis of KMnO4 fixed WT and vps13 cells grown on glucose (C) or methanol (D). Like the WT controls vps13 cells still contain peroxisome-ER, peroxisome-plasma membrane (PM), peroxisome-mitochondrion (M) and peroxisome-vacuole (V) contacts. Scale bar in C 200 nm, size bar in D 500 nm. N—nucleus.
The decrease in peroxisome numbers in pex11, pex23 and pex24 cells is related to reduced ER-peroxisome contacts (Wu et al., 2020). In H. polymorpha dnm1 cells peroxisome abundance is also decreased, but not due to reduced contacts but caused by a block in peroxisome fission (Nagotu et al., 2008). Hence, we reasoned that deletion of VPS13 in dnm1 cells should not lead to enhanced peroxisome biogenesis defects. Indeed, as shown in Figure 3A, the peroxisome phenotypes of dnm1 and dnm1 vps13 cells are comparable. This observation supports the redundant function of Vps13 with peroxisome-ER contacts. Peroxisome-ER contacts still occur in vps13 cells (Figure 3C). Also, in the absence of Vps13 all other peroxisomal contacts that were identified in H. polymorpha wild-type cells are normally present (peroxisome-plasmamembrane, Figure 3C; peroxisome-mitochondrion (M) and peroxisome-vacuole (V), Figure 3D).
An Artificial Peroxisome-ER Tether Suppresses the pex11 vps13, pex23 vps13 and pex24 vps13 Phenotypes
The peroxisomal defects that occur in H. polymorpha pex23 and pex24 cells are largely suppressed upon introduction of an artificial peroxisome-ER tether (Wu et al., 2020). This tether consists of full-length Pex14 and the tail anchor of the ER protein Ubc6, separated by two heme-agglutinin tags. Deletion of VPS13 enhances the peroxisomal defects in pex11, pex23 or pex24 cells (Figure 1). Introduction of the artificial peroxisome-ER tether resulted in partical suppression of the severe peroxisomal phenotypes of the pex11 vps13, pex23 vps13 and pex24 vps13 double deletion strains (Figures 4A,B). This was not observed in control strains in which Pex14, which is part of the artificial tether, was overproduced (Pex14++; Figures 4A,B). These data suggest that upon artificially enhancing peroxisome-ER contacts again, Vps13 becomes less important for peroxisome biogenesis in the three indicated double deletion strains.
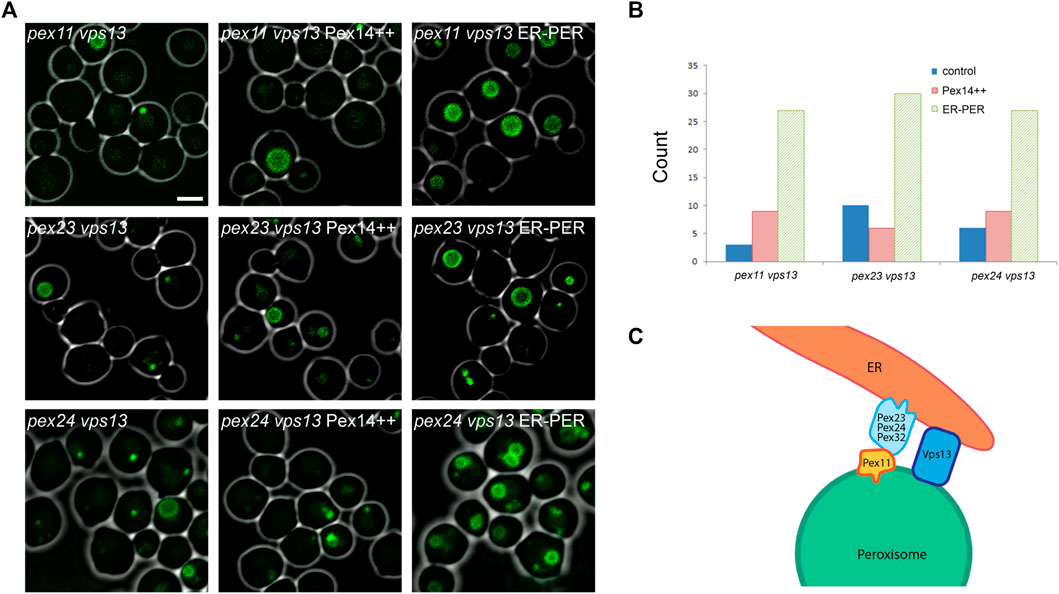
FIGURE 4. Suppression of peroxisome biogenesis defects by an artificial ER-peroxisome tether. (A) FM analysis of methanol-glycerol grown pex11 vps13, pex23 vps13 and pex24 vps13 cells producing PPMP47PMP47-GFP alone or in combination with PADH1PEX14 (Pex14++) or PADH1PEX14-HAHA-UBC6TA (ER-PER). Scale bar: 2 μm. (B) Quantitative analysis of the presence of peroxisomes in thin sections of methanol-glycerol grown cells of the indicated strains. Thin sections of KMnO4 fixed cells were analyzed by electron microscopy. The number of cell sections containing one or more peroxisomes with a diameter >200 nm were counted. 100 cell sections were analyzed per strain (n = 100). (C) Hypothetical model of the peroxisome-ER contact site in H. polymorpha, with the currently known components.
Discussion
Here we identified VPS13 as a gene required for peroxisome biogenesis in H. polymorpha cells with disturbed peroxisome-ER contacts. Previous data suggested that these contacts are involved in lipid transfer from the ER to peroxisomes to allow membrane expansion (Wu et al., 2020). This is in line with observations in S. cerevisiae indicating that the peroxisomal membrane can receive membrane lipids via non-vesicular transport (Raychaudhuri and Prinz, 2008) and that the ER is one of the lipid sources (Rosenberger et al., 2009; Flis et al., 2015).
Cells of the H. polymorpha pex11, pex23 and pex24 single deletion strains still contain peroxisomes, but show reduced growth on methanol media, due to a partial defect in peroxisome function (Wu et al., 2020). We now show that deletion of VPS13 in each of these mutants results in a complete defect in methanol growth, accompanied by much more severe peroxisome biogenesis defects, including mislocalization of the bulk of the peroxisomal matrix enzymes in the cytosol (Figures 1E,F; Figures 3A,B). The latter is unlikely to be caused by defects in the importomer. First, peroxisomal membrane proteins, including proteins of the importomer, are still normally sorted to peroxisomes (Figure 2B). Second, peroxisomes still can incorporate a minor portion of the matrix proteins (Figure 2A). Third, the matrix protein import defect of the double mutants is largely restored by the introduction of an artificial ER-peroxisome tethering protein (Figure 4). Hence, the matrix protein import defect is most likely an indirect effect of the inability of the peroxisomes to sufficiently expand as a result of reduced membrane lipid supply.
Like for H. polymorpha also the S. cerevisiae pex11 vps13 double mutant, but not the pex11 and vps13 single deletion strains, show severe peroxisome biogenesis defects, indicating that a role for Vps13 in peroxisome biogenesis is conserved in yeast (Figure 1G).
Vps13 was initially characterized as an S. cerevisiae protein involved in vacuolar protein sorting (Bankaitis et al., 1986). Later studies indicated that ScVps13 is required for many other processes, which all probably relate to a function in lipid transport. Vps13 is a very large (>300 kDa), highly conserved protein. In yeast, there is a single VPS13 gene, while mammals contain four VPS13 isoforms: VPS13A-D. Protein structure analysis and biochemical studies revealed the presence of a large hydrophilic groove, which can bind and transfer a variety of glycerophospholipids. Most likely Vps13 is responsible for bulk transport of lipids between membranes, because its hydrophilic groove can bind multiple lipids at once (Leonzino et al., 2021).
H. polymorpha Vps13 is very similar to S. cerevisiae Vps13 (sequence identity 40%). Also, their length is similar (3135 and 3144 residues, respectively). Like the four human Vps13 homologues and S. cerevisiae Vps13, H. polymorpha Vps13 contains a chorein domain at the N-terminus and a VAB domain folllowed by an APT1, ATG2_C and plekstrin homology domain at the C-terminus (Dziurdzik and Conibear, 2021). H. polymorpha Vps13 has 23% identity with each of the four human Vps13 proteins.
S. cerevisiae Vps13 localizes to multiple MCSs, including the Nuclear Vacuolar Junction (NVJ), the vacuole-mitochondria patch (vCLAMP) and endosome-mitochondrial contact sites (Dziurdzik and Conibear, 2021). In addition, S. cerevisiae Vps13 localizes to prospore membranes (Park and Neiman, 2012), the Golgi apparatus (Kolakowski et al., 2021) and peroxisomes (John Peter et al., 2017). Most likely H. polymorpha Vps13 also localizes to multiple organelles. Human VPS13 isoforms also localize to different MCSs and cell organelles (Dziurdzik and Conibear, 2021). Moreover, recent studies showed that human VPS13D plays a role in peroxisome formation (Baldwin et al., 2021) and localizes to these organelles (Guillen-Samander et al., 2021).
VPS13D associates to both peroxisomes and mitochondria via Miro proteins that have a dual localization at both organelles. In addition, VPS13D associates to ER localized VAP proteins. In this way VPS13D connects the ER to peroxisomes and mitochondria to mediate bulk lipid transport (Guillen-Samander et al., 2021).
We generated a functional, internally GFP-tagged H. polymorpha Vps13 variant, but unfortunately the fluorescence levels were too low to determine its localization. However, given the peroxisomal localization of S. cerevisiae Vps13 and human VPS13D it is very likely that H. polymorpha Vps13 localizes to peroxisomes as well.
We were unable to detect a peroxisomal phenotype of H. polymorpha or S. cerevisiae vps13 cells. Moreover, electron microscopy revealed that all described peroxisomal MCSs still occur in H. polymorpha vps13 cells (Figure 3C). Similarly, Baldwin and others reported that S. cerevisiae vps13 cells show no peroxisomal defects (Baldwin et al., 2021). This observation indicates that yeast Vps13 is redundant for peroxisome formation, but becomes important for lipid transport to peroxisomes, when the peroxisome-ER MCS formed by Pex23, Pex24, Pex32 and Pex11 are disturbed (Figure 4C). The defects were not only observed in methanol-grown pex11 vps13 cells, but also when cells were grown on glucose (Figure 1C). In these cells peroxisomes mostly form contacts with the plasma-membrane and the ER (Wu et al., 2019). The contacts with the plasma membrane function in peroxisome retention (Krikken et al., 2020). Therefore Vps13 possibly performs its redundant function at peroxisome-ER contacts (Figure 4C). However, given the ambiguity of Vps13 function, other models cannot be excluded yet.
Further studies are required to understand the molecular mechanisms of Vps13 function in peroxisome formation. For instance, it would be important to know whether Vps13 plays a role in lipid transport, membrane tethering or both. This could be achieved by the introduction of mutations that affect lipid transfer in Vps13. Such mutations have been reported for S. cerevisiae Vps13 (Li et al., 2020). Hence, it would be interesting to learn whether corresponding mutations in H. polymorpha Vps13 abolish peroxisome formation in pex11, pex23 and pex24 cells. Our data indicate that H. polymorpha Vps13 functions together with Pex23 and Pex24 in peroxisomal membrane expansion. Like other protein of the Pex23 family, Pex23 and Pex24 contain a dysferlin (DysF) domain (Jansen et al., 2021). DysF was first identified in dysferlin, a human protein important for membrane repair of the sarcolemma at the site of muscle injury. The function of this domain is still unknown (Bulankina and Thoms, 2020). Interestingly, S. cerevisiae Spo73, another yeast DysF domain containing protein, also functions together with Vps13. Spo73 is required for extension of the prospore membrane and interacts with Spo71, which recruits Vps13 to the prospore membrane (Parodi et al., 2015; Okumura et al., 2016). It is tempting to speculate that members of the Pex23 family and Vps13 are components of a protein complex at peroxisome-ER contacts together with a yet unknown Vps13 recruiting protein at the peroxisomal membrane. Possibly the DysF domain is involved in the formation of this complex.
Data Availability Statement
The raw data supporting the conclusion of this article will be made available by the authors, without undue reservation.
Author Contributions
WY, AA, RB, AK, and IK conceived the project, performed the experiments, analyzed the data and prepared the figures. WY, AA, and IK wrote the original draft. All contributed to reviewing and editing the manuscript.
Funding
This work was supported by grants from Netherlands Organization for Scientific Research/Chemical Sciences (NWO/CW) to AA (711.012.002), the CHINA SCHOLARSHIP COUNCIL to WY.
Conflict of Interest
The authors declare that the research was conducted in the absence of any commercial or financial relationships that could be construed as a potential conflict of interest.
Publisher’s Note
All claims expressed in this article are solely those of the authors and do not necessarily represent those of their affiliated organizations, or those of the publisher, the editors and the reviewers. Any product that may be evaluated in this article, or claim that may be made by its manufacturer, is not guaranteed or endorsed by the publisher.
Acknowledgments
We acknowledge the contributions of Srishti Devarajan for assistance in strain construction and of Anita Kram for performing the immunolabeling experiments.
Supplementary Material
The Supplementary Material for this article can be found online at: https://www.frontiersin.org/articles/10.3389/fcell.2022.842285/full#supplementary-material
References
Baldwin, H. A., Wang, C., Kanfer, G., Shah, H. V., Velayos-Baeza, A., Dulovic-Mahlow, M., et al. (2021). VPS13D Promotes Peroxisome Biogenesis. J. Cel Biol 220, e202001188. doi:10.1083/jcb.202001188
Bankaitis, V. A., Johnson, L. M., and Emr, S. D. (1986). Isolation of Yeast Mutants Defective in Protein Targeting to the Vacuole. Proc. Natl. Acad. Sci. 83, 9075–9079. doi:10.1073/pnas.83.23.9075
Bulankina, A. V., and Thoms, S. (2020). Functions of Vertebrate Ferlins. Cells 9, e9030534. doi:10.3390/cells9030534
Costello, J. L., Castro, I. G., Hacker, C., Schrader, T. A., Metz, J., Zeuschner, D., et al. (2017). ACBD5 and VAPB Mediate Membrane Associations between Peroxisomes and the ER. J. Cel. Biol. 216, 331–342. doi:10.1083/jcb.201607055
David, C., Koch, J., Oeljeklaus, S., Laernsack, A., Melchior, S., Wiese, S., et al. (2013). A Combined Approach of Quantitative Interaction Proteomics and Live-Cell Imaging Reveals a Regulatory Role for Endoplasmic Reticulum (ER) Reticulon Homology Proteins in Peroxisome Biogenesis. Mol. Cell Proteomics 12, 2408–2425. doi:10.1074/mcp.m112.017830
Dziurdzik, S. K., and Conibear, E. (2021). The Vps13 Family of Lipid Transporters and its Role at Membrane Contact Sites. Int. J. Mol. Sci. 22, e22062905. doi:10.3390/ijms22062905
Faber, K. N., Haima, P., Harder, W., Veenhuis, M., and Ab, G. (1994). Highly-efficient Electrotransformation of the Yeast Hansenula Polymorpha. Curr. Genet. 25, 305–310. doi:10.1007/bf00351482
Flis, V. V., Fankl, A., Ramprecht, C., Zellnig, G., Leitner, E., Hermetter, A., et al. (2015). Correction: Phosphatidylcholine Supply to Peroxisomes of the Yeast Saccharomyces cerevisiae. PloS one 10, e0140080. doi:10.1371/journal.pone.0140080
Guillen-Samander, A., Leonzino, M., Hanna, M. G., Tang, N., Shen, H., and De Camilli, P. (2021). VPS13D Bridges the ER to Mitochondria and Peroxisomes via Miro. J. Cel. Biol. 220, e202010004. doi:10.1083/jcb.202010004
Hua, R., Cheng, D., Coyaud, É., Freeman, S., Di Pietro, E., Wang, Y., et al. (2017). VAPs and ACBD5 Tether Peroxisomes to the ER for Peroxisome Maintenance and Lipid Homeostasis. J. Cel. Biol. 216, 367–377. doi:10.1083/jcb.201608128
Hulmes, G. E., Hutchinson, J. D., Dahan, N., Nuttall, J. M., Allwood, E. G., Ayscough, K. R., et al. (2020). The Pex3-Inp1 Complex Tethers Yeast Peroxisomes to the Plasma Membrane. J. Cel Biol 219, e201906021. doi:10.1083/jcb.201906021
Islinger, M., Costello, J. L., Kors, S., Soupene, E., Levine, T. P., Kuypers, F. A., et al. (2020). The Diversity of ACBD Proteins - from Lipid Binding to Protein Modulators and Organelle Tethers. Biochim. Biophys. Acta (Bba) - Mol. Cel Res. 1867, 118675. doi:10.1016/j.bbamcr.2020.118675
Jansen, R. L. M., Santana-Molina, C., van den Noort, M., Devos, D. P., and van der Klei, I. J. (2021). Comparative Genomics of Peroxisome Biogenesis Proteins: Making Sense of the PEX Proteins. Front. Cel Dev. Biol. 9, 654163. doi:10.3389/fcell.2021.654163
John Peter, A. T., Herrmann, B., Antunes, D., Rapaport, D., Dimmer, K. S., and Kornmann, B. (2017). Vps13-Mcp1 Interact at Vacuole-Mitochondria Interfaces and Bypass ER-Mitochondria Contact Sites. J. Cel. Biol. 216, 3219–3229. doi:10.1083/jcb.201610055
Joshi, A. S., Huang, X., Choudhary, V., Levine, T. P., Hu, J., and Prinz, W. A. (2016). A Family of Membrane-Shaping Proteins at ER Subdomains Regulates Pre-peroxisomal Vesicle Biogenesis. J. Cel. Biol. 215, 515–529. doi:10.1083/jcb.201602064
Joshi, A. S., Nebenfuehr, B., Choudhary, V., Satpute-Krishnan, P., Levine, T. P., Golden, A., et al. (2018). Lipid Droplet and Peroxisome Biogenesis Occur at the Same ER Subdomains. Nat. Commun. 9, 2940. doi:10.1038/s41467-018-05277-3
Knoblach, B., Sun, X., Coquelle, N., Fagarasanu, A., Poirier, R. L., and Rachubinski, R. A. (2013). An ER-Peroxisome Tether Exerts Peroxisome Population Control in Yeast. Embo J. 32, 2439–2453. doi:10.1038/emboj.2013.170
Knoops, K., Manivannan, S., Cepińska, M. N., Krikken, A. M., Kram, A. M., Veenhuis, M., et al. (2014). Preperoxisomal Vesicles Can Form in the Absence of Pex3. J. Cel. Biol. 204, 659–668. doi:10.1083/jcb.201310148
Kolakowski, D., Rzepnikowska, W., Kaniak-Golik, A., Zoladek, T., and Kaminska, J. (2021). The GTPase Arf1 Is a Determinant of Yeast Vps13 Localization to the Golgi Apparatus. Int. J. Mol. Sci. 22, 222212274. doi:10.3390/ijms222212274
Komori, M., Rasmussen, S. W., Kiel, J. A., Baerends, R. J., Cregg, J. M., van der Klei, I. J., et al. (1997). The Hansenula Polymorpha PEX14 Gene Encodes a Novel Peroxisomal Membrane Protein Essential for Peroxisome Biogenesis. EMBO J. 16, 44–53. doi:10.1093/emboj/16.1.44
Krikken, A. M., Wu, H., de Boer, R., Devos, D. P., Levine, T. P., and van der Klei, I. J. (2020). Peroxisome Retention Involves Inp1-dependent Peroxisome-Plasma Membrane Contact Sites in Yeast. J. Cel Biol 219, e20196023. doi:10.1083/jcb.201906023
Krikken, A. M., Veenhuis, M., and van der Klei, I. J. (2009). Hansenula Polymorpha Pex11 Cells Are Affected in Peroxisome Retention. FEBS J. 276, 1429–1439. doi:10.1111/j.1742-4658.2009.06883.x
Leonzino, M., Reinisch, K. M., and De Camilli, P. (2021). Insights into VPS13 Properties and Function Reveal a New Mechanism of Eukaryotic Lipid Transport. Biochim. Biophys. Acta (Bba) - Mol. Cel Biol. Lipids 1866, 159003. doi:10.1016/j.bbalip.2021.159003
Lev, S., Halevy, D. B., Peretti, D., and Dahan, N. (2008). The VAP Protein Family: from Cellular Functions to Motor Neuron Disease. Trends Cell Biology 18, 282–290. doi:10.1016/j.tcb.2008.03.006
Li, P., Lees, J. A., Lusk, C. P., and Reinisch, K. M. (2020). Cryo-EM Reconstruction of a VPS13 Fragment Reveals a Long Groove to Channel Lipids between Membranes. J. Cel Biol 219, e1161. doi:10.1083/jcb.202001161
Mast, F. D., Jamakhandi, A., Saleem, R. A., Dilworth, D. J., Rogers, R. S., Rachubinski, R. A., et al. (2016). Peroxins Pex30 and Pex29 Dynamically Associate with Reticulons to Regulate Peroxisome Biogenesis from the Endoplasmic Reticulum. J. Biol. Chem. 291, 15408–15427. doi:10.1074/jbc.m116.728154
Nagotu, S., Saraya, R., Otzen, M., Veenhuis, M., and van der Klei, I. J. (2008). Peroxisome Proliferation in Hansenula Polymorpha Requires Dnm1p Which Mediates Fission but Not De Novo Formation. Biochim. Biophys. Acta (Bba) - Mol. Cel Res. 1783, 760–769. doi:10.1016/j.bbamcr.2007.10.018
Okumura, Y., Nakamura, T. S., Tanaka, T., Inoue, I., Suda, Y., Takahashi, T., et al. (2016). The Dysferlin Domain-Only Protein, Spo73, Is Required for Prospore Membrane Extension in Saccharomyces cerevisiae. mSphere 1, e15. doi:10.1128/mSphere.00038-15
Park, J. S., and Neiman, A. M. (2012). VPS13 Regulates Membrane Morphogenesis during Sporulation in Saccharomyces cerevisiae. J. Cel Sci 125, 3004–3011. doi:10.1242/jcs.105114
Parodi, E. M., Roesner, J. M., and Huang, L. S. (2015). SPO73 and SPO71 Function Cooperatively in Prospore Membrane Elongation during Sporulation in Saccharomyces cerevisiae. PloS one 10, e0143571. doi:10.1371/journal.pone.0143571
Raychaudhuri, S., and Prinz, W. A. (2008). Nonvesicular Phospholipid Transfer between Peroxisomes and the Endoplasmic Reticulum. Proc. Natl. Acad. Sci. 105, 15785–15790. doi:10.1073/pnas.0808321105
Rosenberger, S., Connerth, M., Zellnig, G., and Daum, G. (2009). Phosphatidylethanolamine Synthesized by Three Different Pathways Is Supplied to Peroxisomes of the Yeast Saccharomyces cerevisiae. Biochim. Biophys. Acta (Bba) - Mol. Cel Biol. Lipids 1791, 379–387. doi:10.1016/j.bbalip.2009.01.015
Shai, N., Schuldiner, M., and Zalckvar, E. (2016). No Peroxisome Is an Island - Peroxisome Contact Sites. Biochim. Biophys. Acta (Bba) - Mol. Cel Res. 1863, 1061–1069. doi:10.1016/j.bbamcr.2015.09.016
Shai, N., Yifrach, E., van Roermund, C. W. T., Cohen, N., Bibi, C., IJlst, L., et al. (2018). Systematic Mapping of Contact Sites Reveals Tethers and a Function for the Peroxisome-Mitochondria Contact. Nat. Commun. 9, 1761. doi:10.1038/s41467-018-03957-8
Smith, J. J., and Aitchison, J. D. (2013). Peroxisomes Take Shape. Nat. Rev. Mol. Cel Biol 14, 803–817. doi:10.1038/nrm3700
Thomas, A. S., Krikken, A. M., van der Klei, I. J., and Williams, C. P. (2015). Phosphorylation of Pex11p Does Not Regulate Peroxisomal Fission in the Yeast Hansenula Polymorpha. Sci. Rep. 5, 11493. doi:10.1038/srep11493
van der Klei, I. J., Yurimoto, H., Sakai, Y., and Veenhuis, M. (2006). The Significance of Peroxisomes in Methanol Metabolism in Methylotrophic Yeast. Biochim. Biophys. Acta (Bba) - Mol. Cel Res. 1763, 1453–1462. doi:10.1016/j.bbamcr.2006.07.016
van Dijk, R., Faber, K., Hammond, A., Glick, B., Veenhuis, M., and Kiel, J. (2001). Tagging Hansenula Polymorpha Genes by Random Integration of Linear DNA Fragments (RALF). Mol. Gen. Genomics 266, 646–656. doi:10.1007/s004380100584
van Dijken, L. P., Otto, R., and Harder, W. (1976). Growth of Hansenula Polymorpha in a Methanol-Limited Chemostat. Arch. Microbiol. 111, 137–144. doi:10.1007/bf00446560
Wang, S., Idrissi, F.-Z., Hermansson, M., Grippa, A., Ejsing, C. S., and Carvalho, P. (2018). Seipin and the Membrane-Shaping Protein Pex30 Cooperate in Organelle Budding from the Endoplasmic Reticulum. Nat. Commun. 9, 2939. doi:10.1038/s41467-018-05278-2
Wu, F., de Boer, R., Krikken, A. M., Akşit, A., Bordin, N., Devos, D. P., et al. (2020). Pex24 and Pex32 Are Required to Tether Peroxisomes to the ER for Organelle Biogenesis, Positioning and Segregation in Yeast. J. Cel Sci 133, 24683. doi:10.1242/jcs.246983
Keywords: peroxisome, yeast, contact site, VPS13, endoplasmic reticulum
Citation: Yuan W, Akşit A, de Boer R, Krikken AM and van der Klei IJ (2022) Yeast Vps13 is Crucial for Peroxisome Expansion in Cells With Reduced Peroxisome-ER Contact Sites. Front. Cell Dev. Biol. 10:842285. doi: 10.3389/fcell.2022.842285
Received: 23 December 2021; Accepted: 28 January 2022;
Published: 17 February 2022.
Edited by:
Michael Schrader, University of Exeter, United KingdomReviewed by:
Andrés Guillén-Samander, Yale University, United StatesFred David Mast, Seattle Children’s Research Institute, United States
Copyright © 2022 Yuan, Akşit, de Boer, Krikken and van der Klei. This is an open-access article distributed under the terms of the Creative Commons Attribution License (CC BY). The use, distribution or reproduction in other forums is permitted, provided the original author(s) and the copyright owner(s) are credited and that the original publication in this journal is cited, in accordance with accepted academic practice. No use, distribution or reproduction is permitted which does not comply with these terms.
*Correspondence: Ida J. van der Klei, aS5qLnZhbi5kZXIua2xlaUBydWcubmw=
†These authors have contributed equally to this work