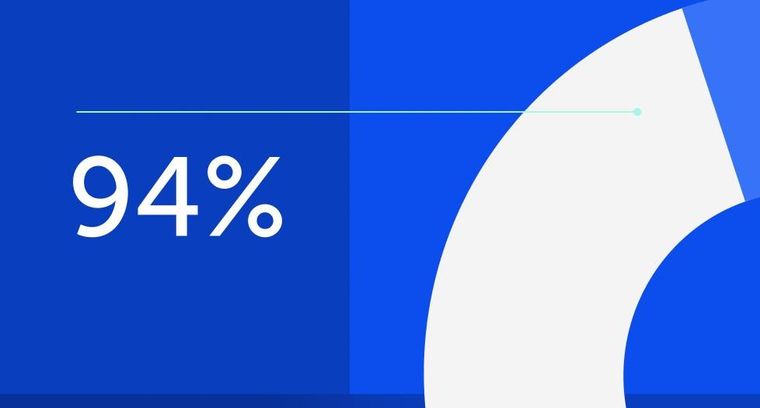
94% of researchers rate our articles as excellent or good
Learn more about the work of our research integrity team to safeguard the quality of each article we publish.
Find out more
REVIEW article
Front. Cell Dev. Biol., 28 March 2022
Sec. Cell Death and Survival
Volume 10 - 2022 | https://doi.org/10.3389/fcell.2022.840576
Pyroptosis is a novel form of programmed cell death (PCD), which is characterized by DNA fragmentation, chromatin condensation, cell swelling and leakage of cell contents. The process of pyroptosis is performed by certain inflammasome and executor gasdermin family member. Previous researches have manifested that pyroptosis is closely related to human diseases (such as inflammatory diseases) and malignant tumors, while the regulation mechanism of pyroptosis is not yet clear. Non-coding RNA (ncRNA) such as microRNA (miRNA), long non-coding RNA (lncRNA) and circular RNA (circRNA) have been widely identified in the genome of eukaryotes and played a paramount role in the development of cell function and fate after transcription. Accumulating evidences support the importance of ncRNA biology in the hallmarks of pyroptosis. However, the associations between ncRNA and pyroptosis are rarely reviewed. In this review, we are trying to summarize the regulation and function of ncRNA in cell pyroptosis, which provides a new research direction and ideas for the study of pyroptosis in different diseases.
Pyroptosis is a novel form of programmed cell death (PCD) plays a pivotal role in the survival and development of organisms, and it is also a way of self-protection (Bedoui et al., 2020). Apoptosis is the first PCD type to be discovered (D'Arcy, 2019), and the others including pyroptosis, necrosis, ferroptosis, autophagic cell death and paraptosis are gradually being revealed (Lee et al., 2016). Their main characteristics and differences are presented in Table 1. In 2005, Susan L. Fink (Fink and Cookson, 2005) et al. firstly dug out and defined the phenomenon of cell pyroptosis. Then pyroptosis is well-known as cell inflammatory necrosis (Xia et al., 2019), and it has been widely recognized as a new member of PCD in recent years. Pyroptosis is manifested by the continuous expansion of cells until the membrane ruptures, which leads to the release of cell contents and severe inflammation (Fang et al., 2020). Through comparing with other PCD, it is found that pyroptosis occurs more rapidly and is accompanied by plentiful pro-inflammatory factors (Xu et al., 2018), which constitutes the human natural immune barrier against exogenous infections and internal danger signals (Liang F. et al., 2020). Nowadays, growing small molecule substances and drugs that inhibit pyroptosis have been discovered, however, the amount of pyroptosis activator is rare (McKenzie et al., 2018; Sollberger et al., 2018; Yang J. et al., 2018; Hu et al., 2020; Humphries et al., 2020; Teng et al., 2020; Zhou et al., 2020a; Yu et al., 2021a) (Table 2). Besides, their applications are almost still in the cell research stage. Recent studies have shown that pyroptosis can participate in the process of various diseases, including tumors (Erkes et al., 2020; Zheng and Li, 2020), cardiovascular diseases (Zhaolin et al., 2019), atherosclerosis (AS) (Wu et al., 2018), diabetes (Tu et al., 2019) and so on. Therefore, an in-depth comprehension of the system about pyroptosis would be beneficial to the recognition and prevention of human diseases.
The genetic central dogma states that the genetic information encoded in DNA should first be transcribed into messenger RNA (mRNA), and then translated into functional protein (Liu et al., 2018). Increasing studies have found that the maladjustment of the genetic central dogma will result in the occurrence of many diseases. Previous works have revealed that about 90% of the genes in the eukaryotic genome are transcribed genes. Of interest, only 1–2% of these transcribed genes encode proteins, most of them are transcribed into non-coding RNA (ncRNA) (Liu et al., 2021a). Although ncRNA lacks the potential to encode proteins, they can affect the expression of many molecular targets to drive specific cellular biological reactions and destinies. Therefore, ncRNA acts as key adjustment factor in physiological and pathological process. For example, ncRNA is particularly related to cancer, and have been identified as carcinogenic driving factor and tumor inhibitory factor in certain cancer (Anastasiadou et al., 2018). Furthermore, studies have indicated that ncRNA can affect cell fate decision (Schwarzer et al., 2017), as well as PCD (Su et al., 2016) via regulating gene transcriptional or post-transcriptional level. At present, it is shared for ncRNA to regulate apoptosis in various cells and tissues (Villanova et al., 2018; Hu et al., 2019; He et al., 2020), but the regulation of pyroptosis is remain uncovered. The exploration of ncRNA may be a new strategy of pyroptosis research, which is worthy of exhaustive analysis and clarification.
In this review, we mainly investigated the effects of various lncRNAs, miRNAs and circRNAs on pyroptosis in different diseases. In addition, we also revealed the role of ceRNA network in pyroptosis (Figure 1). This will facilitate the diagnosis and treatment of complex diseases.
FIGURE 1. The abstract image of this review. In this review, we summarized the regulation and function of ncRNA such as lncRNA, microRNA and circRNA in pyroptosis, which provides novel research direction and strategy for the study of pyroptosis in different human diseases.
Initially, research suggested that pyroptosis was related to the antibacterial response of immune cells (Sauer et al., 2010). So far, pyroptosis is usually induced by caspase-1-dependent canonical inflammasome and caspase-4/5/11-dependent noncanonical inflammasome pathways. It is worth noting that caspase-3 and caspase-8 (apoptosis-related caspase proteins) can also trigger pyroptosis. More attractively, some new mechanisms that induce pyroptosis have recently been discovered such as granzyme-mediated pyroptosis. We summarize the current mechanisms of pyroptosis in the Figure 2 according to the literatures.
FIGURE 2. The main molecular mechanism of pyroptosis. In the caspase-1-dependent canonical inflammasome pathway, various inflammasomes are stimulated by cellular signals (such as PAMPs and DAMPs), which activate the inflammasomes and caspase-1. The activated caspase-1 cleaves GSDMD and pro-IL-1β/pro-IL-18, and finally mature IL-1β and IL-18 flow out of the GSDMD pore formed by the N-GSDMD oligomerization. In the caspase-4/5/11-dependent noncanonical inflammasome pathway, cytosolic LPS directly activates caspase-4/5/11, and the corresponding activated caspases will cleave GSDMD and eventually trigger pyroptosis. In the caspase-3-mediated pathway, chemotherapy drugs can directly activate caspase-3/GSDME-mediated pyroptosis. And molecular targeted therapies will elicit mitochondrial dysfunction and activate caspase-9, which eventually promote caspase-3/GSDME-mediated pyroptosis. In addition, the stimulation of high bile acid levels can result in MPT, then promoting Apaf-1/caspase-4/11 pyroptosome assembly, and ultimately causing caspase-3/GSDME-dependent pyroptosis. Caspase-3 can also be activated via caspase-8 when death ligands/receptors are stimulated. In the caspase-8-mediated pathway, in the response to LPS (such as Yersinia), the inhibition of TAK1/IKK complex activates caspase-8, and then triggers GSDMD-mediated pyroptosis. Moreover, under hypoxic conditions, PD-L1 binds to p-Stat3 in the nucleus, converting TNF-α-induced apoptosis into caspase-8/GSDMC-mediated pyroptosis. Besides, the metabolite α-KG can increase ROS production, promoting the assembly of DR6/pro-caspase-8/GSDMC receptosome, and activated caspase-8 cleaves GSDMC, leading to pyroptosis. In granzyme-mediated pathway, the CAR T cells rapidly activate caspase-3 by releasing GzmB, then GSDME-mediated pyroptosis is induced, and GzmB can directly act on GSDME. The GzmA derived by NK cells and lymphocytes could cleave GSDMB to result in pyroptosis.
There are five main types of pattern recognition receptors (PRRs) involved in pathogen recognition: Toll-like receptors (TLRs), Nod-like receptors (NLRs), RIG-I-like receptors (RLRs), C-type lectin receptors (CLRs) and DNA sensors (Shekarian et al., 2019). Pathogen-associated molecular patterns (PAMPs) and damage associated molecular patterns (DAMPs) are two molecules closely related to innate immunity. When cells and tissues are damaged or under hypoxia condition, DAMPs are released into the blood circulation or intercellular spaces, while PAMPs are the molecular structures on pathogens. Both PAMPs and DAMPs are recognized by PRRs, which triggers immune response (Zindel and Kubes, 2020). The innate immune system is activated when PAMPs or DAMPs are burst, then the inflammasomes gradually formed in certain cells such as monocytes, macrophages and dendritic cells, because they can express more inflammation-related genes (Vande Walle and Lamkanfi, 2016). The canonical inflammasome is a polyprotein complex that contains a sensor protein, an adaptor protein named apoptosis-associated speck-like protein containing CARD (ASC), and an effector protein pro-caspase-1 (Man and Kanneganti, 2015; Rathinam and Fitzgerald, 2016). Currently, common sensor proteins mainly include NLRP1, NLRP3, NLRP6, NLRP12, NLRC4, AIM2, Pyrin, etc. In the canonical inflammasome-mediated pyroptosis pathway, once the sensor proteins receive signals, their oligomerization occurs rapidly, and the inflammasomes assembly are triggered, which leads to the polymerization of the adaptor protein ASC. Importantly, ASC is a double adaptor protein molecule which contains caspase recruitment domain (CARD) and pyrin domain (PYD). NLR containing PYD domain and pro-caspase-1 containing CARD domain are linked by ASC through the interaction of CARD-CARD and PYD-PYD. Followed by pro-caspase-1 in dimer form is cleaved into p10 and p20 subunits to form activated caspase-1, which induces the maturation of IL-1β and IL-18 from their precursors. In addition, activated caspase-1 cleaves and activates GSDMD to promote the occurrence of cell pyroptosis (Broz and Dixit, 2016; McKenzie et al., 2020). It seems that the formation of caspase-1-dependent canonical inflammasome is a key step in the driver of pyroptosis, and affecting the assembly and activation of inflammasome can be a classical way to regulate cell pyroptosis.
In addition to the canonical inflammasome-mediated pyroptosis, noncanonical inflammasome can also trigger pyroptosis. The activation of inflammasomes usually follow one of these two pathways and depend on their activated caspases. The activation of canonical inflammasome depends on caspase-1, while noncanonical inflammasome activation is related to mouse-derived caspase-11 and human-derived caspase-4, 5. The Gram-negative bacteria (such as E. coli) can stimulate caspase-4, 5, 11 to induce the pyroptosis of macrophages (Muendlein et al., 2020). Bacterial LPS, a main immunogenic component of the outer leaflet of the cell wall of Gram-negative bacteria, is a necessary PAMP that gives rise to inflammation in Gram-negative bacterial infections (Matikainen et al., 2020). Recent findings suggest that in human epithelial cells guanylate-binding proteins 1 (GBP1) may act as a cytosolic LPS sensor and assembles a platform for caspase-4 recruitment and activation at LPS-containing membranes. It is regarded as the first step of non-canonical inflammasome signaling (Santos et al., 2020). When LPS leaks into the cytoplasm, it can directly bind to the CARD domain of caspase-11 (caspase-4, 5 in human) to induce its oligomerization and activation and then promote pyroptosis, interestingly, this process does not need to be mediated by activated caspase-1 (Vande Walle and Lamkanfi, 2016). Surprisingly, activated caspase-11 can also activate caspase-1 under certain circumstances (Aachoui et al., 2015). It can be seen that there is a crosstalk between caspase-1-dependent and caspase-11-dependent pyroptosis.
Notably, GSDME (also referred to as DFNA5) can also be cleaved and activated by caspase-3 to form soluble membrane pores. Studies have demonstrated that chemotherapeutic drugs can activate caspase-3-mediated cleavage of GSDME, thereby inducing pyroptosis (Wang et al., 2017). In addition, caspase-3/GSDME pathway is believed to participate in pyroptosis under caspase-9-mediated apoptosis stimulation (Lu et al., 2018). Apoptotic protease activating factor-1 (Apaf-1) plays a crucial role in apoptosis with the participation of mitochondria (Dorstyn et al., 2018). Of interest, Xu W. et al. (2021) discovered a new mechanism of caspase-3/GSDME-dependent pyroptosis, which is induced by Apaf-1/caspase-4/11 pyroptosome assembly activated through mitochondrial permeability transition (MPT). Attractively, this mechanism process is induced by bile acids rather than LPS stimulation. Further exploration determined that low-dose bile acids promoted mitochondrial outer membrane permeabilization (MOMP) and activated caspase-9-mediated apoptosis after a long time, while high-dose bile acids induced MPT and stimulated Apaf-1 more strongly, prompting Apaf-1 pyroptosome assembly selectively and caused pyroptosis. Can Apaf-1 trigger both pyroptosis and apoptosis in an intermediate state of mitochondrial stress? Hence, clarifying the dynamic changes of mitochondria may be the key point for the selection of different death pathways.
In the response to Yersinia infection, the activities of transforming growth factor β-activated kinase 1 (TAK1) and IκB kinase complex (IKK) are blocked, while the caspase-8 pathway is activated, which causing GSDMD to be cleaved and activated at the same location, finally, pyroptosis is triggered (Orning et al., 2018). Moreover, another finding indicate that in extrinsic and intrinsic apoptosis, caspase-1 and caspase-8 cleave GSDMD to trigger pyroptosis, importantly, extrinsic and intrinsic apoptosis both activate pannexin-1 to drive NLRP3 inflammasome assembly, further promoting pyroptosis (Chen K. W. et al., 2019). Among several kinds of caspase family, caspase-1 seems to be the strongest driving factor for GSDMD cleavage, and caspase-8 is the weakest driving factor. It is possible that caspase-8 acts as a backup measure when other caspase family members are damaged (Newton et al., 2019). Encouragingly, the researchers found that PD-L1 together with p-Stat3 transformed tumor cell apoptosis induced by TNFα into pyroptosis. More crucially, under hypoxic conditions, PD-L1 promoted GSDMC-mediated pyroptosis, which is specifically cleaved by caspase-8 rather than caspase-6 (Hou et al., 2020). Recent study suggests that in the acidic environment of tumor cells, the metabolic enzyme MDH1 reduces α-KG to L-2HG, increasing the level of reactive oxygen species (ROS) and inducing the oxidation and internalization of DR6 (the plasma membrane-localized death receptor), and then pro-caspase-8 and GSDMC are recruited to the internalized DR6 receptosome, which facilitates the activated-caspase-8 to cleave GSDMC, leading to pyroptosis ultimately (Zhang J.-y. et al., 2021).
Of interest, evidence demonstrated that chimeric antigen receptor (CAR) T cells rapidly activated caspase-3 in target cells by releasing granzyme B (GzmB), which cleaved GSDME and caused pyroptosis (Liu Y. et al., 2020). Shockingly, another study reported that GzmB could directly cleave GSDME to trigger pyroptosis, while caspase-3 was not indispensable for GzmB-mediated pyroptosis (Zhang Z. et al., 2020). GSDMB is highly expressed in certain tissues, especially the digestive tract epithelium. In 2020, Zhou et al. firstly discovered that natural killer cells (NKs) and cytotoxic T lymphoid (CTLs) cells killed GSDMB-positive cells via pyroptosis. The specific reason was that lymphocyte-derived granzyme A (GzmA) cleaved GSDMB at Lys229/Lys244 site (Zhou et al., 2020b).
Kayagaki et al. (2015), Shi et al. (2015) determined that GSDMD was the shared substrate protein of caspase-l, caspase-4, caspase-5 and caspase-11 almost at the same time. GSDMD is cleaved by caspase-1/4/5/11 to induce pyroptosis at the 272FLTD275 site (273LLSD276 in mouse GSDMD). In addition, GSDMD is divided into an N-terminal p30 (GSDMD-N domain) and a C-terminal p20 (GSDMD-C domain). GSDMD-N can specifically bind to phosphorylated phosphatidylinositol or cardiolipin, which are specific phospholipids on eukaryotic and prokaryotic cell membranes respectively. GSDMD-N molecules bind to the cell membrane in this way and rapidly undergo oligomerization, and then 16 of these molecules form pores with an about 10–14 nm inner diameter. The inflammatory factors IL-1β, IL-18 and K+ produced via the inflammasome pathway are secreted out of the cell through these pores. At the same time, plenty of Ca2+ flows into the cell, resulting in increased osmotic pressure and electrochemical gradient, and a host of extracellular water enters the cell, causing the rupture and death of cell (Ding et al., 2016). Liu et al. (2017) have detected that NF-κB/GSDMD signaling is essential for the formation of NLRP3 inflammasome and the release of inflammatory cytokines during the melatonin inhibiting adipocytes pyroptosis. Furthermore, Kayagaki et al. (2019) indicated that in bone marrow–derived macrophages (BMDMs), interferon regulatory factor 2 (IRF2), a member of the transcription factors IRF family, could bind to regulatory elements in the GSDMD promoter to directly drive GSDMD transcription and trigger pyroptosis.
GSDMD belongs to the gasdermin family, which also includes GSDMA, GSDMB, GSDMC, GSDME and GSDMF (also referred to as DFNB59) (Tanaka et al., 2013). Except for GSDMF, all others have a pore-forming N-terminal domain and a C-terminal regulatory domain. These two domains are thought to be activated through cleavage and separation (Ding and Wang et al., 2016; Feng et al., 2018). In view of the death execution activity of the gasdermin family proteins, NCCD (The Nomenclature Committee on Cell Death) updated the definition of pyroptosis in 2018. The concept of pyroptosis is redefined as a regulated cell death (RCD) that is the formation of gasdermin family-dependent plasma membrane pores, and the inflammatory caspase activation is not indispensable. Therefore, a more comprehensive investigation of the mechanism and regulation of the gasdermin family will contribute to understand the pyroptosis mediated by gasdermin in the future, which will provide a theoretical basis for the treatment of pyroptosis-related diseases.
There are numerous types of ncRNAs, which can be divided into two categories according to their length: those larger than 200 nt are called lncRNA, those smaller than 200 nt are called small ncRNA, and those below 50 nt can also be called tiny ncRNA (such as small interfering RNA [siRNA], miRNA, and [piwi-interactiing RNA] piRNA). In another way of classification, ncRNAs can also be divided into housekeeping ncRNA (such as ribosomal RNA [rRNA], transfer RNA [tRNA]) and regulatory ncRNA (such as miRNA, piRNA, lncRNA, tRNA-derived stress-induced RNA and tRNA-derived small RNA [tRF]) according to their expression and functional characteristics (Chen H. et al., 2019; Seal et al., 2020). The content of the housekeeping ncRNA is constitutive, which is essential for cell survival. The regulatory ncRNA is usually short-lived and regulates processes such as transcription and translation (Vivek and Kumar, 2021). Although ncRNA species are abundant, their roles in pyroptosis have been gradually revealed in recent years. Next, we reviewed the three regulatory ncRNAs involved in pyroptosis, including lncRNA, miRNA and circular RNA (circRNA) and the possible related mechanisms, and we also discussed the role of competing endogenous RNA (ceRNA) related to lncRNA and circRNA during pyroptosis process. These would provide a new research direction for the study of pyroptosis. We summarized the target, function, corresponding tissues and diseases of ncRNA involved in pyroptosis (Figure 3; Table 3).
FIGURE 3. The role of ncRNAs on pyroptosis in different tissues. In different organs, various ncRNAs regulate the pyroptosis process by affecting the corresponding target genes.
LncRNA, transcript length exceeds 200 nt, is believed to have multiple functions, including cis or trans transcriptional regulation, nuclear domain organization and protein or RNA molecule regulation (Bridges et al., 2021). The functions are closely related to their subcellular location. In the nucleus, lncRNA regulates gene expression at the epigenetic and transcriptional levels, and in the cytoplasm, it influences gene expression at the post-transcriptional and translational spheres (Yang Z. et al., 2019). Dramatically, studies have also manifested that some transcripts such as lncRNA actually encode small proteins (Kopp and Mendell, 2018). It can be seen that the actual function of lncRNA has not yet been fully understood. Gradually, researchers paid attention to the regulation of lncRNA on cell fate. According to reports, lncRNA is involved in the regulation of cell death pathways such as apoptosis (Xiong et al., 2021), necrosis (Zhou et al., 2021), senescence (Montes et al., 2021)and autophagy (Zhou C. et al., 2020). Recently, the function of lncRNA on pyroptosis also has been continuously revealed.
lncRNA Neat1 is transcribed from a multitude of endocrine tumor formation sites. Findings (Zhang et al., 2019) demonstrated that in LPS-stimulated mouse immortal bone marrow-derived macrophages (iBMDM), lncRNA Neat1 boosted the activation of inflammasomes NLRP3, NLRC4 and AIM2, especially NLRP3, and enhanced caspase-1 activation to induce pyroptosis. Mechanistically, lncRNA Neat1 binds to pro-caspase-1 and promotes the assembly of inflammasomes. So that it can also stabilize mature caspase-1 and increase the activity of caspase-1 protease. According to research, lnc-LFAR1 is rich in liver without Kozak sequence, which is vital for the initiation of mRNA translation. Zhang et al. investigated that the silencing of lnc-LFAR1 alleviated the pro-inflammatory activation of M1 macrophages and restrained NLRP3 inflammasome-mediated pyroptosis stimulated by CCl4 and BDL ligation in vivo. Moreover, knockdown of lnc-LFAR1 also blocked LPS/ATP- and LPS/Niger-induced NLRP3 inflammasome-mediated pyroptosis in vitro. These data confirmed that lnc-LFAR1 played a crucial role in the containment of macrophage activation and pyroptosis, which provides an attractive therapeutic target for liver fibrosis. LncRNA NEXN-AS1 is a newly identified lncRNA with reduced expression in atherosclerotic plaques. It was found to interact with the nuclear protein BAZ1A to regulate its neighboring gene NEXN (Zhang K. et al., 2020). Wu et al. examined that atorvastatin could improve AS by inhibiting lncRNA NEXN-as1/NEXN-mediated pyroptosis. However, the research on lncRNA NEXN-AS1-mediated pyroptosis is still unclear, and it is worth exploring the underlying molecular mechanism (Wu et al., 2020). In another probe on AS (Liu X.-H. et al., 2021), researchers discovered that lncRNA RP11-490M8.1 blocked LPS-induced pyroptosis of Human Umbilical Vein Endothelial Cells (HUVECs) through the TLR4/NF-κb signal axis, implying that lncRNA RP11-490M8.1 may act as a therapeutic target for AS. Nevertheless, this work did not explore which gasdermin protein ultimately affected by lncRNA RP11-490M8.1, and did not identify it in-depth in animal models. Ma et al. indicated that the inhibition of lncRNA RP1-85F18.6 expression could accelerate the occurrence of colorectal cancer (CRC) cells pyroptosis by increasing the lysis of GSDMD (Ma et al., 2018). Not long ago, Chen et al. confirmed that siRNA designed for lncRNA AA388235, a kind of mouse-specific lncRNA, could induce the death of human CRC cells, yet had no effect on normal human cells. More attractively, the siRNA targeting mouse-specific lncRNA AA388235 could facilitate tumor cells pyroptosis through the caspase-3/GSDME signaling pathway, while in the absence of GSDME, tumor cells would undergo apoptosis (Chen Y.-R. et al., 2021). Therefore, species-specific lncRNA may be used as a sequence target to design siRNA for tumor treatment, which provides a new method for tumor drug design. Besides, researchers pointed out that in the diabetes-cerebral I/R model and microglia cells treated with high glucose followed by hypoxia/reoxygenation (H/R), lncRNA-Fendrr was highly expressed. LncRNA-Fendrr could prevent the ubiquitination and degradation of NLRC4 protein through the E3 ubiquitin ligase HERC2, thereby pushing the pyroptosis of microglia. This infers that lncRNA-Fendrr may be a potential target for the treatment of diabetic brain I/R damage (Wang L.-Q. et al., 2021). Above all, these studies not only further determine the vital role of lncRNA in pyroptosis, but also provide a fresh direction for the identification of disease markers.
MiRNA is a type of small ncRNA with a length of approximately 21 nt (Xie et al., 2021). Foremost, the primary transcription product (pri-miRNA) of the miRNA gene is cleaved by RNase III Drosha in the nucleus to become the precursor miRNA (pre-miRNA). After the initial cleavage, pre-miRNA is transferred from the nucleus to the cytoplasm under the action of the transporter exportin-5, and then another Rnase III Dicer further cleaves it to produce mature miRNA. These mature miRNAs together with other proteins form a RISC (RNA-induced silencing complex) complex, which leads to the degradation of target mRNA or translation inhibition (Campbell et al., 2022). Recently, growing works have suggested that miRNA is largely involved in the pyroptosis, and it is of great significance to detect the role of miRNA in pyroptosis.
Xu et al. conducted microarray analysis on normal and degenerative nucleus pulposus (NP) and identified miRNA-141 as a key miRNA in intervertebral disk (IVD) degeneration. Further mechanism showed that miRNA-141 accelerated the ROS production and the activation of TXNIP/NLRP3 signaling pathway to induce nucleus pulposus cells (NPCs) pyroptosis along with extracellular matrix (ECM) catabolism (Xu Q. et al., 2020). Therefore, the miRNA-141-regulated pyroptosis may be a new therapeutic strategy for IVD degeneration. Evidences have implied that miRNA-148a have wealthy functions and is involved in prominent biological processes such as lipid metabolism (Cheng et al., 2017), inflammation (Humphries and Shmuel-Galia et al., 2020) and cell adhesion (Li et al., 2019). In the research of alcoholic liver disease (ALD), Heo et al. denoted that alcohol reduced the expression of miR-148a in hepatocytes by decreasing the expression of forkhead box protein O1 (FOXO1), and then promoted TXNIP expression and the activation of NLRP3 inflammasomes, thereby stimulating hepatocytes pyroptosis (Heo et al., 2019). The work provides a brand-new strategy for reducing the incidence of ALD. Huang et al. applied TargetScan, an RNA target prediction software, to conduct an online search. Results showed that miR-125b-2-3p contained a nucleotide sequence complementary to the highly conserved seed sequence in NLRP1 mRNA 3-UTR. Further exploration suggested that STF083010 (IRE1α inhibitor) could restrain neuronal pyroptosis regulated by miR-125b-2-3p/NLRP1 pathway in the neonatal hypoxic ischemic encephalopathy (HIE) rat model (Huang et al., 2020). Besides, miR-181b-5p has shear sensitivity in aortic valve endothelial cells and side specificity in porcine aortic valve respectively (Heath et al., 2018). Researchers in the AS study demonstrated that when HUVECs were induced by low shear stress, the expression of miR-181b-5p decreased, which increased STAT-3 expression and activated the NLRP3 inflammasome, eventually, the caspase-1-dependent pyroptosis was triggered (Xu X. et al., 2021). Thus, in-depth exploration of miR-181b-5p-mediated pyroptosis may be a promising way to treat AS. MiR-103 is widely expressed in various tissues, whose gene coding region is located on human chromosome 5 (Xu et al., 2015). Another outcome in AS indicated that miR-103 was reduced in H2O2-treated human coronary artery endothelial cells (HCAECs), which resulted in an up-regulation of Bcl-2/adenovirus E1B 19 kDa interacting protein (BNIP3) and the suppression of end-stage autophagy. As autolysosome synthesis is blocked, NLRP3 and caspase-1 increased, and cell pyroptosis was accelerated (Wang Y. et al., 2020). This phenomenon denoted that miR-103 might become a key point in the crosstalk between autophagy and pyroptosis, which offers an unusual basis for the regulation of pyroptosis. In general, these studies reveal the miRNAs regulate pyroptosis and expand the research field of pyroptosis.
CircRNA that possesses regulatory functions has a closed loop structure and exists in an army of eukaryotic transcriptomes. Most circRNAs are derived from exons, and a small part is directly formed by introns cyclization. CircRNA is conserved in diverse species and it has specific expression in multiple tissues and different developmental stages. Besides, circRNA is divided into four categories, including exon circRNA (ecRNA), circular intron RNA (ciRNA), exon-intron circRNA (EIciRNA) and tRNA intron circular RNA (tricRNA) (Chen L. et al., 2021). Because circRNA is not sensitive to nucleases, it is more stable than linear RNA, which makes circRNA has obvious advantages in the development and application of new clinical diagnostic markers. CircRNA plays a pivotal role in various biological processes, such as autophagy (Liang G. et al., 2020), ferroptosis (Liu Z. et al., 2020), tumorigenesis (Guarnerio et al., 2019), tumor metabolism (Yu et al., 2019) and drug resistance (Liu J. et al., 2020). Amusingly, researchers have also presented new insights on circRNA in pyroptosis. Although there are a few reports in this area, the role of circRNA in pyroptosis is worthy of further detection.
Liu et al. analyzed that hsa_circ_0001836 was up-regulated in glioma cells, and its knockdown inhibited cell viability and proliferation. Engagingly, the reduction of hsa_circ_0001836 promoted pyroptosis of glioma cells. In mechanism, hsa_circ_0001836 knockdown may increase the expression of NLRP1 through DNA demethylation, thereby triggering cell pyroptosis (Liu et al., 2021c). The findings indicated that hsa_circ_0001836 might be a potential therapeutic target for the treatment of glioma. Wen et al. used circRNA chip analysis and found a series of dysregulated circRNAs in HK-2 cells stressed by glucose. Among the candidate circRNAs, circACTR2 was up-regulated and might be involved in inflammation and pyroptosis. Knockdown of circACTR2 could significantly reduce the production of interleukin (IL)-1β, the type IV collagen and fibronectin, even vitally blocked the occurrence of pyroptosis (Wen et al., 2020). The results manifested that circACTR2 could effectively regulate renal tubular cell pyroptosis, inflammation and fibrosis induced by high glucose. Whereas, the mechanism of circACTR2 in pyroptosis has not yet been elucidated, which is worthy of further clarification. Moreover, Guo et al. reported that IFN regulatory factor-1 (IRF-1) inhibited the pyroptosis and inflammation of macrophages in acute coronary syndrome (ACS) and AS via promoting the N6-methyladenosine (m6A) modification of circ_0029589 (Guo M. et al., 2020). The novel pyroptosis regulation mechanism provides great potentiality for the prevention and treatment of ACS and AS. Researches on circRNA in pyroptosis are limited, and further exploration is needed.
CeRNA is not a novel kind of RNA molecule, but a newly discovered regulatory mechanism. Recent studies have emphasized that genes have multiple modes of transcriptional regulation (Vivek and Kumar, 2021). One of the crucial regulatory factors, miRNA, can reverse-regulate gene expression by suppressing the translation of target genes or degrading target genes. In the actual regulation process, there is not only the simple miRNA-mRNA silencing mechanism, but also more complex regulatory network. Some ncRNAs also have binding sites with miRNA, which act as miRNA sponge in cells, thereby releasing miRNA. The inhibitory effect increases the expression level of target genes of miRNA, therefore a huge ceRNA network (ceRNET) is constructed. This mechanism of action is called the ceRNA mechanism (Li et al., 2021). CeRNA provides individuals with a new perspective for transcriptome exploration, which contributes to explain quite a few biological phenomena more comprehensively. To date, there has been growing learning on ceRNA, including cell pyroptosis. Underneath, we introduce the role of lncRNA and circRNA-related ceRNA in pyroptosis.
A host of studies demonstrate that lncRNA participates in pyroptosis via coordinating with miRNA and protein-encoding mRNA. LncRNA acts as a ceRNA by competitively occupying the shared binding sequence of miRNA, thereby isolating miRNA and changing the expression of its downstream target genes. Such ceRNA networks formed by lncRNA/miRNA/mRNA interactions have been widely discovered in a multitude of biological processes, including epithelial-to-mesenchymal transition (EMT) (Yang X.-Z. et al., 2018), inflammation (Cao et al., 2020) and angiogenesis (Guo Z. et al., 2020). Below we summarize the quintessential examples of ceRNA networks related to lncRNA, which are involved in the underlying molecular mechanisms of pyroptosis.
LncRNA-H19 is one of the earliest discovered lncRNA and miR-21 exists widely in various organisms and mammalian tissues (Gamaev et al., 2021; Rossi et al., 2021). There is growing evidences have suggested that their regulatory effects are closely related to a variety of biological functions (Zhou et al., 2017; Morgoulis et al., 2019; Yu et al., 2021b). Wan et al. first used transcriptome sequencing technology to find that the level of lncRNA-H19 was obviously up-regulated during retinal ischemia/reperfusion (I/R) injury. Through further exploration, it was shown that lncRNA-H19 promoted programmed cell death protein 4 (PDCD4) expression by sponging miR-21 to form ceRNET, and then the balance of NLRP3/6 inflammasomes were regulated. Afterwards, caspase-1 was activated to cleave GSDMD and finally triggered microglia pyroptosis (Kai et al., 2020). This presents that the regulation of lncRNA-H19-related ceRNA-mediated pyroptosis may become a new idea to prevent I/R injury-related diseases. The research results of Xu et al. stated that during spinal cord injury (SCI), TLR4 was activated and promoted the expression of lncRNA-F630028O10Rik. This lncRNA acted as the ceRNA of the miR-1231-5p/Col1a1 axis and enhanced the microglia pyroptosis by activating the PI3K/AKT pathway after SCI (Xu S. et al., 2020). In the study of AS, Zhang et al. found that lncRNA MEG3 was enriched in endothelial cells and played a role of ceRNA on miR-223. Moreover, they proved that melatonin exerted an anti-pyroptosis effect by suppressing the lncRNA MEG3/miR-223/NLRP3 axis (Zhang et al., 2018). Surprisingly, another research denoted that lncRNA MEG3/miR-485/AIM2 axis promoted cell pyroptosis by activating caspase-1 signaling during cerebral I/R (Liang J. et al., 2020). Fan et al. treated cardiac fibroblasts with 30 mmol/L glucose, and the following results demonstrated that lncRNA KCNQ1OT1 acted as a ceRNA, which modulated the expression of caspase-1 by sponging miR-214-3p to trigger pyroptosis (Yang F. et al., 2018). Amusingly, in diabetic corneal endothelial keratopathy, the lncRNA KCNQ1OT1/miR-214 axis can also be found. Researchersreported that lncRNA KCNQ1OT1 promoted pyroptosis induced by high glucose through targeting miR-214/caspase-1 signaling (Zhang Y. et al., 2020). Ren et al. demonstrated that in cisplatin-treated gastric cancer (GC) cells, lncRNA ADAMTS9-AS2 activated NLRP3 inflammasome through sponging miR-223-3p to promote pyroptosis, which inhibited GC progress and made cisplatin-resistant GC (CR-GC) cells sensitive to cisplatin (Ren et al., 2020). The data offers new insights into the molecular mechanism of cisplatin resistance in GC cells, and is profitable to develop new clinical therapeutic drugs. In diabetic nephropathy (DN) study, Xie et al. pointed out that lncRNA GAS5 reduced the oxidative stress and renal tubular cells pyroptosis stimulated by high glucose. Mechanistically, lncRNA GAS5 prevented NLRP3-mediated pyroptosis through sponging miR-452-5p (Xie et al., 2019). This provides a new perspective for the treatment of. In another DN exploration, Wang et al. found that lncRNA ANRIL prevented TXNIP expression by acting as a sponge of miR-497, thereby giving rise to pyroptosis and kidney damage (Wang and Zhao, 2021). In high concentration of uric acid (HUA)-induced renal injury, Chi et al. proved that lncRNA-HOTAIR promoted endothelial cell pyroptosis by regulating the miR-22/NLRP3 signaling pathway (Chi et al., 2021). In addition, Zhang et al. also reported that lncRNA ORLNC1 induced the pyroptosis of bone marrow mesenchymal stem cells (BMSCs) induced by CML [Nε-(carboxymethyl) lysine, the most common advanced glycation end-products (AGEs)] through targeting the miR-200b-3p/FOXO3 axis (Zhang L. et al., 2021). The above researches confirmed that lncRNA could be used as ceRNA to participate in the process of pyroptosis, which broaden horizons for the pyroptosis exploration.
Currently, increasing reports have denoted that circRNA-related ceRNA is involved in physiological process. CircRNA can compete with miRNA to affect the stability of target RNA or their translation, thereby regulating gene expression at the transcriptional level (Wang L. et al., 2021). Evidences suggested that circRNA can be used as ceRNA to participate in biological processes, such as tumor cell proliferation (Cheng et al., 2019), apoptosis (Sang et al., 2019), invasion (Luo et al., 2020) and migration (Wang et al., 2019). It is worth noting that the function of circRNA as ceRNA in pyroptosis has also been continuously presented.
Ge et al. treated human aortic endothelial cells (HAECs) with oxidized low-density lipoprotein (ox-LDL) to construct the AS model. They found that circ_0090231 promoted cell pyroptosis in vitro. What’s more, they identified circ_0090231 as a sponge of miR-635, and its knockdown reversed the effect of circ_0090231. Finally, it showed that the circ_0090231/miR-635/NLRP3 axis affected the development of AS by regulating cell pyroptosis (Ge et al., 2021). Yan et al. indicated that circHIPK3 derived from stem cell-derived exosomes could interact with miR-421 to regulate the expression of FOXO3a, resulting in the down-regulation of NLRP3 and caspase-1, ultimately inhibiting the pyroptosis of skeletal muscle cells and enhancing the repair of ischemic hind limbs (Yan et al., 2020). Dramatically, another study also hinted that the role of circHIPK3 in acinar cells pyroptosis. They demonstrated that circHIPK3 promoted pyroptosis by regulating the miR-193a-5p/GSDMD axis (Wang J. et al., 2020). Obviously, the roles of circHIPK3 in pyroptosis are opposite in the two reports. In the exploration of diabetic cardiomyopathy (DCM), Yang et al. emphasized that hsa_circ_0076631, named caspase-1-associated circRNA (CACR), was significantly increased in the serum of diabetic patients, and the silencing of CACR could reduce caspase-1 expression by sponging miR-214-3p, thereby restraining inflammation and pyroptosis of cardiomyocytes (Yang F. et al., 2019). Jiang et al. proved that in hypoxia-induced pulmonary arterial smooth muscle cells (PASMCs), circ-Calm4 increased the expression of programmed cell death protein 6 (PDCD6) through sponging miR-124-3p, and ultimately triggered pyroptosis (Jiang et al., 2021). Although the current research on circRNA-related ceRNA in pyroptosis is insufficient, it is believed that the achievements in this field would be even greater in over time.
Overall, this review reveal how ncRNAs accurately regulate the cell pyroptosis processes in different tissues or cells. The above findings show that although ncRNAs lack the potential of proteins, they can regulate pyroptosis by a variety of mechanisms. Increasing researches have stated that ncRNAs can be used to predict the diagnosis and prognosis of cancer or other diseases (Rincon-Riveros et al., 2021). Perhaps ncRNAs can also become pivotal indications of pyroptosis-related diseases. Then the expression of the pyroptosis-related genes can be adjusted through acting on the consensus sequence of ncRNAs specifically. Therefore, various ncRNAs, as potential targets, can provide new possibilities for clinical treatment by affecting pyroptosis. However, the specific mechanisms of targeting ncRNA need to be further confirmed.
Since the emergence of pyroptosis, it has aroused strong interest of researchers, and reached a lot of achievements. Evidences have confirmed that pyroptosis is closely related to many diseases, such as tumors (Erkes et al., 2020; Zheng and Li, 2020), cardiovascular diseases (Zhaolin et al., 2019), AS (Wu et al., 2018) and diabetes (Tu et al., 2019). In some cases, pyroptosis releases the cytoplasmic contents of dying host cells, thereby providing a powerful signal to initiate the inflammatory cascade. Local inflammation leads to the recruitment and activation of immune cells, which ultimately helps the host clear pathogens. Even so, in the context of chronic autoinflammatory diseases and sepsis, uncontrolled pyroptosis is extremely destructive. This raises a series of questions: Which pathological conditions will cause cell pyroptosis? When pyroptosis should be suppressed or promoted? Where are the inflammatory factors released from pyroptosis cells going? These are all paramount issues that need to be resolved urgently. Nevertheless, the analysis of the pyroptosis mechanism and its exact role in the body still remain uncovered.
Notably, the exploration of ncRNA has achieved many gratifying outcomes, involving the process of a variety of diseases, such as liver fibrosis, kidney damages, tumors and neurodegenerative diseases. What is remarkable is that increasing evidences show that ncRNA plays a pivotal role in pyroptosis, which opens up a novel way for the pyroptosis mechanism. However, due to the wide varieties of ncRNA, it is arduous to explore the specific mechanism of ncRNA in pyroptosis. Obviously, there are various differentially expressed ncRNAs-induced pyroptosis in the same disease. At present, it is still difficult to determine which ncRNA is the most effective intervention method of pyroptosis. Furthermore, how to trigger specifically the abnormal cells (such as tumor cells) pyroptosis by regulating ncRNA is still facing a huge challenge. Another serious problem is that different cell death modes such as pyroptosis, apoptosis and autophagy might be under the control of the same ncRNA. How does ncRNA keep a balance between pyroptosis and other death pathways requiring more in-depth exploration. The influence of ncRNA on pyroptosis is complex, especially in different cells and diseases. How to exactly intervene different ncRNAs-mediated pyroptosis still requires more experiments to investigate.
LG conducted research and wrote manuscript. LG, XY and YH performed the design of the paper. ZJ and YL were responsible for document retrieval. LG and XY made the figures in the manuscript. ZJ, LG and YL made the tables. LG, XY and ZJ revised the manuscript. All authors have read and approved the final manuscript.
This work was supported by the Fifth Batch of Suzhou Health Talents Project (GSWS2019075) and the Suzhou Science and Technology Plan Project (SYSD2019192, SKJYD2021147).
The authors declare that the research was conducted in the absence of any commercial or financial relationships that could be construed as a potential conflict of interest.
All claims expressed in this article are solely those of the authors and do not necessarily represent those of their affiliated organizations, or those of the publisher, the editors and the reviewers. Any product that may be evaluated in this article, or claim that may be made by its manufacturer, is not guaranteed or endorsed by the publisher.
Aachoui, Y., Kajiwara, Y., Leaf, I. A., Mao, D., Ting, J. P.-Y., Coers, J., et al. (2015). Canonical Inflammasomes Drive IFN-γ to Prime Caspase-11 in Defense against a Cytosol-Invasive Bacterium. Cell Host & Microbe 18 (3), 320–332. doi:10.1016/j.chom.2015.07.016
Anastasiadou, E., Jacob, L. S., and Slack, F. J. (2018). Non-coding RNA Networks in Cancer. Nat. Rev. Cancer 18 (1), 5–18. doi:10.1038/nrc.2017.99
Bedoui, S., Herold, M. J., and Strasser, A. (2020). Emerging Connectivity of Programmed Cell Death Pathways and its Physiological Implications. Nat. Rev. Mol. Cel Biol 21 (11), 678–695. doi:10.1038/s41580-020-0270-8
Bridges, M. C., Daulagala, A. C., and Kourtidis, A. (2021). LNCcation: lncRNA Localization and Function. J. Cel Biol 220 (2). doi:10.1083/jcb.202009045
Broz, P., and Dixit, V. M. (2016). Inflammasomes: Mechanism of Assembly, Regulation and Signalling. Nat. Rev. Immunol. 16 (7), 407–420. doi:10.1038/nri.2016.58
Campbell, A. M., De La Cruz-Herrera, C. F., Marcon, E., Greenblatt, J., and Frappier, L. (2022). Epstein-Barr Virus BGLF2 Commandeers RISC to Interfere with Cellular miRNA Function. Plos Pathog. 18 (1), e1010235. doi:10.1371/journal.ppat.1010235
Cao, D.-w., Liu, M.-m., Duan, R., Tao, Y.-f., Zhou, J.-s., Fang, W.-r., et al. (2020). The lncRNA Malat1 Functions as a ceRNA to Contribute to Berberine-Mediated Inhibition of HMGB1 by Sponging miR-181c-5p in Poststroke Inflammation. Acta Pharmacol. Sin 41 (1), 22–33. doi:10.1038/s41401-019-0284-y
Chen, H., Xu, Z., and Liu, D. (2019b). Small Non‐coding RNA and Colorectal Cancer. J. Cel Mol Med 23 (5), 3050–3057. doi:10.1111/jcmm.14209
Chen, K. W., Demarco, B., Heilig, R., Shkarina, K., Boettcher, A., Farady, C. J., et al. (2019a). Extrinsic and Intrinsic Apoptosis Activate Pannexin‐1 to Drive NLRP 3 Inflammasome Assembly. EMBO J. 38 (10). doi:10.15252/embj.2019101638
Chen, L., Wang, C., Sun, H., Wang, J., Liang, Y., Wang, Y., et al. (2021b). The Bioinformatics Toolbox for circRNA Discovery and Analysis. Brief Bioinform 22 (2), 1706–1728. doi:10.1093/bib/bbaa001
Chen, Y.-R., Feng, W.-Y., Cheng, Y.-X., Zhu, H., Liu, H.-J., Gao, Y., et al. (2021a). siRNAs Targeting Mouse-specific lncRNA AA388235 Induce Human Tumor Cell Pyroptosis/Apoptosis. Front. Oncol. 11, 662444. doi:10.3389/fonc.2021.662444
Cheng, L., Zhu, Y., Han, H., Zhang, Q., Cui, K., Shen, H., et al. (2017). MicroRNA-148a Deficiency Promotes Hepatic Lipid Metabolism and Hepatocarcinogenesis in Mice. Cell Death Dis 8 (7), e2916. doi:10.1038/cddis.2017.309
Cheng, Z., Yu, C., Cui, S., Wang, H., Jin, H., Wang, C., et al. (2019). circTP63 Functions as a ceRNA to Promote Lung Squamous Cell Carcinoma Progression by Upregulating FOXM1. Nat. Commun. 10 (1), 3200. doi:10.1038/s41467-019-11162-4
Chi, K., Geng, X., Liu, C., Zhang, Y., Cui, J., Cai, G., et al. (2021). LncRNA‐HOTAIR Promotes Endothelial Cell Pyroptosis by Regulating the miR‐22/NLRP3 axis in Hyperuricaemia. J. Cel Mol Med 25 (17), 8504–8521. doi:10.1111/jcmm.16812
D’Arcy, M. S. (2019). Cell Death: a Review of the Major Forms of Apoptosis, Necrosis and Autophagy. Cell Biol Int 43 (6), 582–592. doi:10.1002/cbin.11137
Ding, J., Wang, K., Liu, W., She, Y., Sun, Q., Shi, J., et al. (2016). Pore-forming Activity and Structural Autoinhibition of the Gasdermin Family. Nature 535 (7610), 111–116. doi:10.1038/nature18590
Dorstyn, L., Akey, C. W., and Kumar, S. (2018). New Insights into Apoptosome Structure and Function. Cell Death Differ 25 (7), 1194–1208. doi:10.1038/s41418-017-0025-z
Erkes, D. A., Cai, W., Sanchez, I. M., Purwin, T. J., Rogers, C., Field, C. O., et al. (2020). Mutant BRAF and MEK Inhibitors Regulate the Tumor Immune Microenvironment via Pyroptosis. Cancer Discov. 10 (2), 254–269. doi:10.1158/2159-8290.CD-19-0672
Fang, Y., Tian, S., Pan, Y., Li, W., Wang, Q., Tang, Y., et al. (2020). Pyroptosis: A New Frontier in Cancer. Biomed. Pharmacother. 121, 109595. doi:10.1016/j.biopha.2019.109595
Feng, S., Fox, D., and Man, S. M. (2018). Mechanisms of Gasdermin Family Members in Inflammasome Signaling and Cell Death. J. Mol. Biol. 430 (18 Pt B), 3068–3080. doi:10.1016/j.jmb.2018.07.002
Fink, S. L., and Cookson, B. T. (2005). Apoptosis, Pyroptosis, and Necrosis: Mechanistic Description of Dead and Dying Eukaryotic Cells. Infect. Immun. 73 (4), 1907–1916. doi:10.1128/IAI.73.4.1907-1916.2005
Gamaev, L., Mizrahi, L., Friehmann, T., Rosenberg, N., Pappo, O., Olam, D., et al. (2021). The Pro-oncogenic Effect of the lncRNA H19 in the Development of Chronic Inflammation-Mediated Hepatocellular Carcinoma. Oncogene 40 (1), 127–139. doi:10.1038/s41388-020-01513-7
Ge, Y., Liu, W., Yin, W., Wang, X., Wang, J., Zhu, X., et al. (2021). Circular RNA Circ_0090231 Promotes Atherosclerosis In Vitro by Enhancing NLR Family Pyrin Domain Containing 3-mediated Pyroptosis of Endothelial Cells. Bioengineered 12, 10837–10848. doi:10.1080/21655979.2021.1989260
Guarnerio, J., Zhang, Y., Cheloni, G., Panella, R., Mae Katon, J., Simpson, M., et al. (2019). Intragenic Antagonistic Roles of Protein and circRNA in Tumorigenesis. Cell Res 29 (8), 628–640. doi:10.1038/s41422-019-0192-1
Guo, M., Yan, R., Ji, Q., Yao, H., Sun, M., Duan, L., et al. (2020a). IFN Regulatory Factor-1 Induced Macrophage Pyroptosis by Modulating m6A Modification of Circ_0029589 in Patients with Acute Coronary Syndrome. Int. Immunopharmacology 86, 106800. doi:10.1016/j.intimp.2020.106800
Guo, Z., Wang, X., Yang, Y., Chen, W., Zhang, K., Teng, B., et al. (2020b). Hypoxic Tumor-Derived Exosomal Long Noncoding RNA UCA1 Promotes Angiogenesis via miR-96-5p/AMOTL2 in Pancreatic Cancer. Mol. Ther. - Nucleic Acids 22, 179–195. doi:10.1016/j.omtn.2020.08.021
He, J., Huang, Z., He, M., Liao, J., Zhang, Q., Wang, S., et al. (2020). Circular RNA MAPK4 (Circ-MAPK4) Inhibits Cell Apoptosis via MAPK Signaling Pathway by Sponging miR-125a-3p in Gliomas. Mol. Cancer 19 (1), 17. doi:10.1186/s12943-019-1120-1
Heath, J. M., Fernandez Esmerats, J., Khambouneheuang, L., Kumar, S., Simmons, R., and Jo, H. (2018). Mechanosensitive microRNA-181b Regulates Aortic Valve Endothelial Matrix Degradation by Targeting TIMP3. Cardiovasc. Eng. Tech. 9 (2), 141–150. doi:10.1007/s13239-017-0296-z
Heo, M. J., Kim, T. H., You, J. S., Blaya, D., Sancho-Bru, P., and Kim, S. G. (2019). Alcohol Dysregulates miR-148a in Hepatocytes through FoxO1, Facilitating Pyroptosis via TXNIP Overexpression. Gut 68 (4), 708–720. doi:10.1136/gutjnl-2017-315123
Hou, J., Zhao, R., Xia, W., Chang, C.-W., You, Y., Hsu, J.-M., et al. (2020). PD-L1-mediated Gasdermin C Expression Switches Apoptosis to Pyroptosis in Cancer Cells and Facilitates Tumour Necrosis. Nat. Cel Biol 22 (10), 1264–1275. doi:10.1038/s41556-020-0575-z
Hu, H., Wu, J., Yu, X., Zhou, J., Yu, H., and Ma, L. (2019). Long Non-coding RNA MALAT1 Enhances the Apoptosis of Cardiomyocytes through Autophagy Inhibition by Regulating TSC2-mTOR Signaling. Biol. Res. 52 (1), 58. doi:10.1186/s40659-019-0265-0
Hu, J. J., Liu, X., Xia, S., Zhang, Z., Zhang, Y., Zhao, J., et al. (2020). FDA-approved Disulfiram Inhibits Pyroptosis by Blocking Gasdermin D Pore Formation. Nat. Immunol. 21 (7), 736–745. doi:10.1038/s41590-020-0669-6
Huang, J., Lu, W., Doycheva, D. M., Gamdzyk, M., Hu, X., Liu, R., et al. (2020). IRE1α Inhibition Attenuates Neuronal Pyroptosis via miR-125/NLRP1 Pathway in a Neonatal Hypoxic-Ischemic Encephalopathy Rat Model. J. Neuroinflammation 17 (1), 152. doi:10.1186/s12974-020-01796-3
Humphries, F., Shmuel-Galia, L., Ketelut-Carneiro, N., Li, S., Wang, B., Nemmara, V. V., et al. (2020). Succination Inactivates Gasdermin D and Blocks Pyroptosis. Science 369 (6511), 1633–1637. doi:10.1126/science.abb9818
Jiang, Y., Liu, H., Yu, H., Zhou, Y., Zhang, J., Xin, W., et al. (2021). Circular RNA Calm4 Regulates Hypoxia-Induced Pulmonary Arterial Smooth Muscle Cells Pyroptosis via the Circ-Calm4/miR-124-3p/PDCD6 Axis. Atvb 41 (5), 1675–1693. doi:10.1161/ATVBAHA.120.315525
Kai, J., Yang, X., Wang, Z., Wang, F., Jia, Y., Wang, S., et al. (2020). Oroxylin a Promotes PGC-1α/Mfn2 Signaling to Attenuate Hepatocyte Pyroptosis via Blocking Mitochondrial ROS in Alcoholic Liver Disease. Free Radic. Biol. Med. 153, 89–102. doi:10.1016/j.freeradbiomed.2020.03.031
Kayagaki, N., Lee, B. L., Stowe, I. B., Kornfeld, O. S., O'Rourke, K., Mirrashidi, K. M., et al. (2019). IRF2 Transcriptionally Induces GSDMD Expression for Pyroptosis. Sci. Signal. 12 (582). doi:10.1126/scisignal.aax4917
Kayagaki, N., Stowe, I. B., Lee, B. L., O’Rourke, K., Anderson, K., Warming, S., et al. (2015). Caspase-11 Cleaves Gasdermin D for Non-canonical Inflammasome Signalling. Nature 526 (7575), 666–671. doi:10.1038/nature15541
Kopp, F., and Mendell, J. T. (2018). Functional Classification and Experimental Dissection of Long Noncoding RNAs. Cell 172 (3), 393–407. doi:10.1016/j.cell.2018.01.011
Lee, D., Kim, I. Y., Saha, S., and Choi, K. S. (2016). Paraptosis in the Anti-cancer Arsenal of Natural Products. Pharmacol. Ther. 162, 120–133. doi:10.1016/j.pharmthera.2016.01.003
Li, B., Huang, N., Wei, S., Xv, J., Meng, Q., Aschner, M., et al. (2021). lncRNA TUG1 as a ceRNA Promotes PM Exposure-Induced Airway Hyper-Reactivity. J. Hazard. Mater. 416, 125878. doi:10.1016/j.jhazmat.2021.125878
Li, Y., Li, W., Zeng, X., Tang, X., Zhang, S., Zhong, F., et al. (2019). The Role of microRNA-148a and Downstream DLGAP1 on the Molecular Regulation and Tumor Progression on Human Glioblastoma. Oncogene 38 (47), 7234–7248. doi:10.1038/s41388-019-0922-3
Liang, F., Zhang, F., Zhang, L., and Wei, W. (2020a). The Advances in Pyroptosis Initiated by Inflammasome in Inflammatory and Immune Diseases. Inflamm. Res. 69 (2), 159–166. doi:10.1007/s00011-020-01315-3
Liang, G., Ling, Y., Mehrpour, M., Saw, P. E., Liu, Z., Tan, W., et al. (2020b). Autophagy-associated circRNA circCDYL Augments Autophagy and Promotes Breast Cancer Progression. Mol. Cancer 19 (1), 65. doi:10.1186/s12943-020-01152-2
Liang, J., Wang, Q., Li, J.-Q., Guo, T., and Yu, D. (2020c). Long Non-coding RNA MEG3 Promotes Cerebral Ischemia-Reperfusion Injury through Increasing Pyroptosis by Targeting miR-485/AIM2 axis. Exp. Neurol. 325, 113139. doi:10.1016/j.expneurol.2019.113139
Liu, C. C., Jewett, M. C., Chin, J. W., and Voigt, C. A. (2018). Toward an Orthogonal central Dogma. Nat. Chem. Biol. 14 (2), 103–106. doi:10.1038/nchembio.2554
Liu, J., Dou, X., Chen, C., Chen, C., Liu, C., Xu, M. M., et al. (2020c). N 6 -methyladenosine of Chromosome-Associated Regulatory RNA Regulates Chromatin State and Transcription. Science 367 (6477), 580–586. doi:10.1126/science.aay6018
Liu, X.-H., Wu, L.-M., Wang, J.-L., Dong, X.-H., Zhang, S.-C., Li, X.-H., et al. (2021b). Long Non-coding RNA RP11-490M8.1 Inhibits Lipopolysaccharide-Induced Pyroptosis of Human Umbilical Vein Endothelial Cells via the TLR4/NF-Κb Pathway. Immunobiology 226 (5), 152133. doi:10.1016/j.imbio.2021.152133
Liu, Y., Fang, Y., Chen, X., Wang, Z., Liang, X., Zhang, T., et al. (2020a). Gasdermin E-Mediated Target Cell Pyroptosis by CAR T Cells Triggers Cytokine Release Syndrome. Sci. Immunol. 5 (43). doi:10.1126/sciimmunol.aax7969
Liu, Y., Liu, X., Lin, C., Jia, X., Zhu, H., Song, J., et al. (2021a). Noncoding RNAs Regulate Alternative Splicing in Cancer. J. Exp. Clin. Cancer Res. 40 (1), 11. doi:10.1186/s13046-020-01798-2
Liu, Y., Wu, H., Jing, J., Li, H., Dong, S., and Meng, Q. (2021c). Downregulation of Hsa_circ_0001836 Induces Pyroptosis Cell Death in Glioma Cells via Epigenetically Upregulating NLRP1. Front. Oncol. 11, 622727. doi:10.3389/fonc.2021.622727
Liu, Z., Gan, L., Xu, Y., Luo, D., Ren, Q., Wu, S., et al. (2017). Melatonin Alleviates Inflammasome-Induced Pyroptosis through Inhibiting NF-Κb/GSDMD Signal in Mice Adipose Tissue. J. Pineal Res. 63 (1), e12414. doi:10.1111/jpi.12414
Liu, Z., Wang, Q., Wang, X., Xu, Z., Wei, X., and Li, J. (2020b). Circular RNA cIARS Regulates Ferroptosis in HCC Cells through Interacting with RNA Binding Protein ALKBH5. Cell Death Discov. 6, 72. doi:10.1038/s41420-020-00306-x
Lu, H., Zhang, S., Wu, J., Chen, M., Cai, M.-C., Fu, Y., et al. (2018). Molecular Targeted Therapies Elicit Concurrent Apoptotic and GSDME-dependent Pyroptotic Tumor Cell Death. Clin. Cancer Res. 24 (23), 6066–6077. doi:10.1158/1078-0432.CCR-18-1478
Luo, Z., Rong, Z., Zhang, J., Zhu, Z., Yu, Z., Li, T., et al. (2020). Circular RNA circCCDC9 Acts as a miR-6792-3p Sponge to Suppress the Progression of Gastric Cancer through Regulating CAV1 Expression. Mol. Cancer 19 (1), 86. doi:10.1186/s12943-020-01203-8
Ma, Y., Chen, Y., Lin, C., and Hu, G. (2018). Biological Functions and Clinical Significance of the Newly Identified Long Non-coding RNA RP1-85F18.6 in C-olorectal C-ancer. Oncol. Rep. 40 (5), 2648–2658. doi:10.3892/or.2018.6694
Man, S. M., and Kanneganti, T.-D. (2015). Regulation of Inflammasome Activation. Immunol. Rev. 265 (1), 6–21. doi:10.1111/imr.12296
Matikainen, S., Nyman, T. A., and Cypryk, W. (2020). Function and Regulation of Noncanonical Caspase-4/5/11 Inflammasome. J.I. 204 (12), 3063–3069. doi:10.4049/jimmunol.2000373
McKenzie, B. A., Dixit, V. M., and Power, C. (2020). Fiery Cell Death: Pyroptosis in the Central Nervous System. Trends Neurosciences 43 (1), 55–73. doi:10.1016/j.tins.2019.11.005
McKenzie, B. A., Mamik, M. K., Saito, L. B., Boghozian, R., Monaco, M. C., Major, E. O., et al. (2018). Caspase-1 Inhibition Prevents Glial Inflammasome Activation and Pyroptosis in Models of Multiple Sclerosis. Proc. Natl. Acad. Sci. U.S.A. 115 (26), E6065–E6074. doi:10.1073/pnas.1722041115
Montes, M., Lubas, M., Arendrup, F. S., Mentz, B., Rohatgi, N., Tumas, S., et al. (2021). The Long Non-coding RNA MIR31HG Regulates the Senescence Associated Secretory Phenotype. Nat. Commun. 12 (1), 2459. doi:10.1038/s41467-021-22746-4
Morgoulis, D., Berenstein, P., Cazacu, S., Kazimirsky, G., Dori, A., Barnea, E. R., et al. (2019). sPIF Promotes Myoblast Differentiation and Utrophin Expression while Inhibiting Fibrosis in Duchenne Muscular Dystrophy via the H19/miR-675/let-7 and miR-21 Pathways. Cel Death Dis 10 (2), 82. doi:10.1038/s41419-019-1307-9
Muendlein, H. I., Jetton, D., Connolly, W. M., Eidell, K. P., Magri, Z., Smirnova, I., et al. (2020). cFLIP L Protects Macrophages from LPS-Induced Pyroptosis via Inhibition of Complex II Formation. Science 367 (6484), 1379–1384. doi:10.1126/science.aay3878
Newton, K., Wickliffe, K. E., Maltzman, A., Dugger, D. L., Reja, R., Zhang, Y., et al. (2019). Activity of Caspase-8 Determines Plasticity between Cell Death Pathways. Nature 575 (7784), 679–682. doi:10.1038/s41586-019-1752-8
Orning, P., Weng, D., Starheim, K., Ratner, D., Best, Z., Lee, B., et al. (2018). Pathogen Blockade of TAK1 Triggers Caspase-8-dependent Cleavage of Gasdermin D and Cell Death. Science 362 (6418), 1064–1069. doi:10.1126/science.aau2818
Rathinam, V. A. K., and Fitzgerald, K. A. (2016). Inflammasome Complexes: Emerging Mechanisms and Effector Functions. Cell 165 (4), 792–800. doi:10.1016/j.cell.2016.03.046
Ren, N., Jiang, T., Wang, C., Xie, S., Xing, Y., Piao, D., et al. (2020). LncRNA ADAMTS9-AS2 Inhibits Gastric Cancer (GC) Development and Sensitizes Chemoresistant GC Cells to Cisplatin by Regulating miR-223-3p/NLRP3 axis. Aging 12 (11), 11025–11041. doi:10.18632/aging.103314
Rincón-Riveros, A., Morales, D., Rodríguez, J. A., Villegas, V. E., and López-Kleine, L. (2021). Bioinformatic Tools for the Analysis and Prediction of ncRNA Interactions. Ijms 22 (21), 11397. doi:10.3390/ijms222111397
Rossi, M., Altomare, E., Botta, C., Gallo Cantafio, M. E., Sarvide, S., Caracciolo, D., et al. (2021). miR-21 Antagonism Abrogates Th17 Tumor Promoting Functions in Multiple Myeloma. Leukemia 35 (3), 823–834. doi:10.1038/s41375-020-0947-1
Sang, Y., Chen, B., Song, X., Li, Y., Liang, Y., Han, D., et al. (2019). circRNA_0025202 Regulates Tamoxifen Sensitivity and Tumor Progression via Regulating the miR-182-5p/FOXO3a Axis in Breast Cancer. Mol. Ther. 27 (9), 1638–1652. doi:10.1016/j.ymthe.2019.05.011
Santos, J. C., Boucher, D., Schneider, L. K., Demarco, B., Dilucca, M., Shkarina, K., et al. (2020). Human GBP1 Binds LPS to Initiate Assembly of a Caspase-4 Activating Platform on Cytosolic Bacteria. Nat. Commun. 11 (1), 3276. doi:10.1038/s41467-020-16889-z
Sauer, J.-D., Witte, C. E., Zemansky, J., Hanson, B., Lauer, P., and Portnoy, D. A. (2010). Listeria Monocytogenes Triggers AIM2-Mediated Pyroptosis upon Infrequent Bacteriolysis in the Macrophage Cytosol. Cell Host & Microbe 7 (5), 412–419. doi:10.1016/j.chom.2010.04.004
Schwarzer, A., Emmrich, S., Schmidt, F., Beck, D., Ng, M., Reimer, C., et al. (2017). The Non-coding RNA Landscape of Human Hematopoiesis and Leukemia. Nat. Commun. 8 (1), 218. doi:10.1038/s41467-017-00212-4
Seal, R. L., Chen, L. L., Griffiths‐Jones, S., Lowe, T. M., Mathews, M. B., O'Reilly, D., et al. (2020). A Guide to Naming Human Non‐coding RNA Genes. EMBO J. 39 (6), e103777. doi:10.15252/embj.2019103777
Shekarian, T., Valsesia-Wittmann, S., Brody, J., Michallet, M. C., Depil, S., Caux, C., et al. (2019). Pattern Recognition Receptors: Immune Targets to Enhance Cancer Immunotherapy. Ann. Oncol. 30 (12), 2017. doi:10.1093/annonc/mdz225
Shi, J., Zhao, Y., Wang, K., Shi, X., Wang, Y., Huang, H., et al. (2015). Cleavage of GSDMD by Inflammatory Caspases Determines Pyroptotic Cell Death. Nature 526 (7575), 660–665. doi:10.1038/nature15514
Sollberger, G., Choidas, A., Burn, G. L., Habenberger, P., Di Lucrezia, R., Kordes, S., et al. (2018). Gasdermin D Plays a Vital Role in the Generation of Neutrophil Extracellular Traps. Sci. Immunol. 3 (26). doi:10.1126/sciimmunol.aar6689
Su, Y., Wu, H., Pavlosky, A., Zou, L.-L., Deng, X., Zhang, Z.-X., et al. (2016). Regulatory Non-coding RNA: New Instruments in the Orchestration of Cell Death. Cel Death Dis 7 (8), e2333. doi:10.1038/cddis.2016.210
Tanaka, S., Mizushina, Y., Kato, Y., Tamura, M., and Shiroishi, T. (2013). Functional Conservation of Gsdma Cluster Genes Specifically Duplicated in the Mouse Genome. G3 (Bethesda) 3 (10), 1843–1850. doi:10.1534/g3.113.007393
Teng, J.-F., Mei, Q.-B., Zhou, X.-G., Tang, Y., Xiong, R., Qiu, W.-Q., et al. (2020). Polyphyllin VI Induces Caspase-1-Mediated Pyroptosis via the Induction of ROS/NF-κB/NLRP3/GSDMD Signal Axis in Non-small Cell Lung Cancer. Cancers 12 (1), 193. doi:10.3390/cancers12010193
Tu, Y., Guo, C., Song, F., Huo, Y., Geng, Y., Guo, M., et al. (2019). Mild Hypothermia Alleviates Diabetes Aggravated Cerebral Ischemic Injury via Activating Autophagy and Inhibiting Pyroptosis. Brain Res. Bull. 150, 1–12. doi:10.1016/j.brainresbull.2019.05.003
Vande Walle, L., and Lamkanfi, M. (2016). Pyroptosis. Curr. Biol. 26 (13), R568–R572. doi:10.1016/j.cub.2016.02.019
Villanova, L., Careccia, S., De Maria, R., and Fiori, M. (2018). Micro-Economics of Apoptosis in Cancer: ncRNAs Modulation of BCL-2 Family Members. Ijms 19 (4), 958. doi:10.3390/ijms19040958
Vivek, A. T., and Kumar, S. (2021). Computational Methods for Annotation of Plant Regulatory Non-coding RNAs Using RNA-Seq. Brief Bioinform 22 (4). doi:10.1093/bib/bbaa322
Wang, J., Li, X., Liu, Y., Peng, C., Zhu, H., Tu, G., et al. (2020b). CircHIPK3 Promotes Pyroptosis in Acinar Cells through Regulation of the miR-193a-5p/GSDMD Axis. Front. Med. 7, 88. doi:10.3389/fmed.2020.00088
Wang, J., and Zhao, S.-M. (2021). LncRNA-antisense Non-coding RNA in the INK4 Locus Promotes Pyroptosis via miR-497/thioredoxin-Interacting Protein axis in Diabetic Nephropathy. Life Sci. 264, 118728. doi:10.1016/j.lfs.2020.118728
Wang, L.-Q., Zheng, Y.-Y., Zhou, H.-J., Zhang, X.-X., Wu, P., and Zhu, S.-M. (2021a). LncRNA-Fendrr Protects against the Ubiquitination and Degradation of NLRC4 Protein through HERC2 to Regulate the Pyroptosis of Microglia. Mol. Med. 27 (1), 39. doi:10.1186/s10020-021-00299-y
Wang, L., Yi, J., Lu, L.-y., Zhang, Y.-y., Wang, L., Hu, G.-s., et al. (2021b). Estrogen-induced circRNA, circPGR, Functions as a ceRNA to Promote Estrogen Receptor-Positive Breast Cancer Cell Growth by Regulating Cell Cycle-Related Genes. Theranostics 11 (4), 1732–1752. doi:10.7150/thno.45302
Wang, Y.-G., Wang, T., Ding, M., Xiang, S.-H., Shi, M., and Zhai, B. (2019). hsa_circ_0091570 Acts as a ceRNA to Suppress Hepatocellular Cancer Progression by Sponging Hsa-miR-1307. Cancer Lett. 460, 128–138. doi:10.1016/j.canlet.2019.06.007
Wang, Y., Gao, W., Shi, X., Ding, J., Liu, W., He, H., et al. (2017). Chemotherapy Drugs Induce Pyroptosis through Caspase-3 Cleavage of a Gasdermin. Nature 547 (7661), 99–103. doi:10.1038/nature22393
Wang, Y., Song, X., Li, Z., Liu, N., Yan, Y., Li, T., et al. (2020a). MicroRNA-103 Protects Coronary Artery Endothelial Cells against H2O2-Induced Oxidative Stress via BNIP3-Mediated End-Stage Autophagy and Antipyroptosis Pathways. Oxidative Med. Cell Longevity 2020, 1–15. doi:10.1155/2020/8351342
Wen, S., Li, S., Li, L., and Fan, Q. (2020). circACTR2: A Novel Mechanism Regulating High Glucose-Induced Fibrosis in Renal Tubular Cells via Pyroptosis. Biol. Pharm. Bull. 43 (3), 558–564. doi:10.1248/bpb.b19-00901
Wu, L.-M., Wu, S.-G., Chen, F., Wu, Q., Wu, C.-M., Kang, C.-M., et al. (2020). Atorvastatin Inhibits Pyroptosis through the lncRNA NEXN-AS1/NEXN Pathway in Human Vascular Endothelial Cells. Atherosclerosis 293, 26–34. doi:10.1016/j.atherosclerosis.2019.11.033
Wu, X., Zhang, H., Qi, W., Zhang, Y., Li, J., Li, Z., et al. (2018). Nicotine Promotes Atherosclerosis via ROS-NLRP3-Mediated Endothelial Cell Pyroptosis. Cel Death Dis 9 (2), 171. doi:10.1038/s41419-017-0257-3
Xia, X., Wang, X., Cheng, Z., Qin, W., Lei, L., Jiang, J., et al. (2019). The Role of Pyroptosis in Cancer: Pro-cancer or Pro-"host"? Cel Death Dis 10 (9), 650. doi:10.1038/s41419-019-1883-8
Xie, C., Wu, W., Tang, A., Luo, N., and Tan, Y. (2019). lncRNA GAS5/miR-452-5p Reduces Oxidative Stress and Pyroptosis of High-Glucose-Stimulated Renal Tubular Cells. Dmso Vol. 12, 2609–2617. doi:10.2147/DMSO.S228654
Xie, D., Chen, M., Niu, J., Wang, L., Li, Y., Fang, X., et al. (2021). Phase Separation of SERRATE Drives Dicing Body Assembly and Promotes miRNA Processing in Arabidopsis. Nat. Cel Biol 23 (1), 32–39. doi:10.1038/s41556-020-00606-5
Xiong, M., Wu, M., Dan Peng, P., Huang, W., Chen, Z., Ke, H., et al. (2021). LncRNA DANCR Represses Doxorubicin-Induced Apoptosis through Stabilizing MALAT1 Expression in Colorectal Cancer Cells. Cel Death Dis 12 (1), 24. doi:10.1038/s41419-020-03318-8
Xu, M.-C., Gao, X.-F., Ruan, C., Ge, Z.-R., Lu, J.-D., Zhang, J.-J., et al. (20152015). miR-103 Regulates Oxidative Stress by Targeting the BCL2/Adenovirus E1B 19 kDa Interacting Protein 3 in HUVECs. Oxidative Med. Cell Longevity 2015, 1–11. doi:10.1155/2015/489647
Xu, Q., Xing, H., Wu, J., Chen, W., and Zhang, N. (2020a). miRNA-141 Induced Pyroptosis in Intervertebral Disk Degeneration by Targeting ROS Generation and Activating TXNIP/NLRP3 Signaling in Nucleus Pulpous Cells. Front. Cel Dev. Biol. 8, 871. doi:10.3389/fcell.2020.00871
Xu, S., Wang, J., Jiang, J., Song, J., Zhu, W., Zhang, F., et al. (2020b). TLR4 Promotes Microglial Pyroptosis via lncRNA-F630028O10Rik by Activating PI3K/AKT Pathway after Spinal Cord Injury. Cel Death Dis 11 (8), 693. doi:10.1038/s41419-020-02824-z
Xu, W., Che, Y., Zhang, Q., Huang, H., Ding, C., Wang, Y., et al. (2021a). Apaf-1 Pyroptosome Senses Mitochondrial Permeability Transition. Cel Metab. 33 (2), 424–436. doi:10.1016/j.cmet.2020.11.018
Xu, X., Yang, Y., Wang, G., Yin, Y., Han, S., Zheng, D., et al. (2021b). Low Shear Stress Regulates Vascular Endothelial Cell Pyroptosis through miR‐181b‐5p/STAT‐3 axis. J. Cel Physiol 236 (1), 318–327. doi:10.1002/jcp.29844
Xu, Y.-J., Zheng, L., Hu, Y.-W., and Wang, Q. (2018). Pyroptosis and its Relationship to Atherosclerosis. Clinica Chim. Acta 476, 28–37. doi:10.1016/j.cca.2017.11.005
Yan, B., Zhang, Y., Liang, C., Liu, B., Ding, F., Wang, Y., et al. (2020). Stem Cell-Derived Exosomes Prevent Pyroptosis and Repair Ischemic Muscle Injury through a Novel exosome/circHIPK3/FOXO3a Pathway. Theranostics 10 (15), 6728–6742. doi:10.7150/thno.42259
Yang, F., Li, A., Qin, Y., Che, H., Wang, Y., Lv, J., et al. (2019b). A Novel Circular RNA Mediates Pyroptosis of Diabetic Cardiomyopathy by Functioning as a Competing Endogenous RNA. Mol. Ther. - Nucleic Acids 17, 636–643. doi:10.1016/j.omtn.2019.06.026
Yang, F., Qin, Y., Lv, J., Wang, Y., Che, H., Chen, X., et al. (2018c). Silencing Long Non-coding RNA Kcnq1ot1 Alleviates Pyroptosis and Fibrosis in Diabetic Cardiomyopathy. Cel Death Dis 9 (10), 1000. doi:10.1038/s41419-018-1029-4
Yang, J., Liu, Z., Wang, C., Yang, R., Rathkey, J. K., Pinkard, O. W., et al. (2018a). Mechanism of Gasdermin D Recognition by Inflammatory Caspases and Their Inhibition by a Gasdermin D-Derived Peptide Inhibitor. Proc. Natl. Acad. Sci. U.S.A. 115 (26), 6792–6797. doi:10.1073/pnas.1800562115
Yang, X.-Z., Cheng, T.-T., He, Q.-J., Lei, Z.-Y., Chi, J., Tang, Z., et al. (2018b). LINC01133 as ceRNA Inhibits Gastric Cancer Progression by Sponging miR-106a-3p to Regulate APC Expression and the Wnt/β-Catenin Pathway. Mol. Cancer 17 (1), 126. doi:10.1186/s12943-018-0874-1
Yang, Z., Jiang, S., Shang, J., Jiang, Y., Dai, Y., Xu, B., et al. (2019a). LncRNA: Shedding Light on Mechanisms and Opportunities in Fibrosis and Aging. Ageing Res. Rev. 52, 17–31. doi:10.1016/j.arr.2019.04.001
Yu, H., Qi, N., and Zhou, Q. (2021b). LncRNA H19 Inhibits Proliferation and Migration of Airway Smooth Muscle Cells Induced by PDGF-BB through miR-21/PTEN/Akt Axis. Jaa Vol. 14, 71–80. doi:10.2147/JAA.S291333
Yu, H., Yao, S., Zhou, C., Fu, F., Luo, H., Du, W., et al. (2021a). Morroniside Attenuates Apoptosis and Pyroptosis of Chondrocytes and Ameliorates Osteoarthritic Development by Inhibiting NF-Κb Signaling. J. Ethnopharmacology 266, 113447. doi:10.1016/j.jep.2020.113447
Yu, T., Wang, Y., Fan, Y., Fang, N., Wang, T., Xu, T., et al. (2019). CircRNAs in Cancer Metabolism: a Review. J. Hematol. Oncol. 12 (1), 90. doi:10.1186/s13045-019-0776-8
Zhang, J.-y., Zhou, B., Sun, R.-y., Ai, Y.-l., Cheng, K., Li, F.-n., et al. (2021a). The Metabolite α-KG Induces GSDMC-dependent Pyroptosis through Death Receptor 6-activated Caspase-8. Cel Res 31 (9), 980–997. doi:10.1038/s41422-021-00506-9
Zhang, K., Shi, Z., Zhang, M., Dong, X., Zheng, L., Li, G., et al. (2020b). Silencing lncRNA Lfar1 Alleviates the Classical Activation and Pyoptosis of Macrophage in Hepatic Fibrosis. Cel Death Dis 11 (2), 132. doi:10.1038/s41419-020-2323-5
Zhang, L., Li, S., Li, J., and Li, Y. (2021b). LncRNA ORLNC1 Promotes Bone Marrow Mesenchyml Stem Cell Pyroptosis Induced by Advanced Glycation End Production by Targeting miR-200b-3p/Foxo3 Pathway. Stem Cel Rev Rep 17, 2262–2275. doi:10.1007/s12015-021-10247-2
Zhang, P., Cao, L., Zhou, R., Yang, X., and Wu, M. (2019). The lncRNA Neat1 Promotes Activation of Inflammasomes in Macrophages. Nat. Commun. 10 (1), 1495. doi:10.1038/s41467-019-09482-6
Zhang, Y., Liu, X., Bai, X., Lin, Y., Li, Z., Fu, J., et al. (2018). Melatonin Prevents Endothelial Cell Pyroptosis via Regulation of Long Noncoding RNA MEG3/miR-223/NLRP3 axis. J. Pineal Res. 64 (2), e12449. doi:10.1111/jpi.12449
Zhang, Y., Song, Z., Li, X., Xu, S., Zhou, S., Jin, X., et al. (2020c). Long Noncoding RNA KCNQ1OT1 Induces Pyroptosis in Diabetic Corneal Endothelial Keratopathy. Am. J. Physiology-Cell Physiol. 318 (2), C346–C359. doi:10.1152/ajpcell.00053.2019
Zhang, Z., Zhang, Y., Xia, S., Kong, Q., Li, S., Liu, X., et al. (2020a). Gasdermin E Suppresses Tumour Growth by Activating Anti-tumour Immunity. Nature 579 (7799), 415–420. doi:10.1038/s41586-020-2071-9
Zhaolin, Z., Guohua, L., Shiyuan, W., and Zuo, W. (2019). Role of Pyroptosis in Cardiovascular Disease. Cell Prolif 52 (2), e12563. doi:10.1111/cpr.12563
Zheng, Z., and Li, G. (2020). Mechanisms and Therapeutic Regulation of Pyroptosis in Inflammatory Diseases and Cancer. Ijms 21 (4), 1456. doi:10.3390/ijms21041456
Zhou, C., Yi, C., Yi, Y., Qin, W., Yan, Y., Dong, X., et al. (2020c). LncRNA PVT1 Promotes Gemcitabine Resistance of Pancreatic Cancer via Activating Wnt/β-Catenin and Autophagy Pathway through Modulating the miR-619-5p/Pygo2 and miR-619-5p/ATG14 Axes. Mol. Cancer 19 (1), 118. doi:10.1186/s12943-020-01237-y
Zhou, Y., Sheng, B., Xia, Q., Guan, X., and Zhang, Y. (2017). Association of Long Non-coding RNA H19 and microRNA-21 Expression with the Biological Features and Prognosis of Non-small Cell Lung Cancer. Cancer Gene Ther. 24 (8), 317–324. doi:10.1038/cgt.2017.20
Zhou, Y., Zhang, F., Xu, F., Wang, Q., Wu, J., Peng, W., et al. (2021). lncRNA NEAT1 Regulates CYP1A2 and Influences Steroid-Induced Necrosis. Open Life Sci. 16 (1), 969–980. doi:10.1515/biol-2021-0097
Zhou, Z., He, H., Wang, K., Shi, X., Wang, Y., Su, Y., et al. (2020b). Granzyme A from Cytotoxic Lymphocytes Cleaves GSDMB to Trigger Pyroptosis in Target Cells. Science 368, 6494. doi:10.1126/science.aaz7548
Zhou, Z., Li, X., Qian, Y., Liu, C., Huang, X., and Fu, M. (2020a). Heat Shock Protein 90 Inhibitors Suppress Pyroptosis in THP-1 Cells. Biochem. J. 477 (20), 3923–3934. doi:10.1042/BCJ20200351
Keywords: inflammasomes, gasdermin, non-coding RNA, mechanism, pyroptosis
Citation: Gao L, Jiang Z, Han Y, Li Y and Yang X (2022) Regulation of Pyroptosis by ncRNA: A Novel Research Direction. Front. Cell Dev. Biol. 10:840576. doi: 10.3389/fcell.2022.840576
Received: 21 December 2021; Accepted: 14 March 2022;
Published: 28 March 2022.
Edited by:
Yvonne Tay, National University of Singapore, SingaporeCopyright © 2022 Gao, Jiang, Han, Li and Yang. This is an open-access article distributed under the terms of the Creative Commons Attribution License (CC BY). The use, distribution or reproduction in other forums is permitted, provided the original author(s) and the copyright owner(s) are credited and that the original publication in this journal is cited, in accordance with accepted academic practice. No use, distribution or reproduction is permitted which does not comply with these terms.
*Correspondence: Xiang Yang, eXh0Y201MjZAMTYzLmNvbQ==
Disclaimer: All claims expressed in this article are solely those of the authors and do not necessarily represent those of their affiliated organizations, or those of the publisher, the editors and the reviewers. Any product that may be evaluated in this article or claim that may be made by its manufacturer is not guaranteed or endorsed by the publisher.
Research integrity at Frontiers
Learn more about the work of our research integrity team to safeguard the quality of each article we publish.