- 1Department of Basic and Clinical Neuroscience, Institute of Psychiatry, Psychology and Neuroscience, King’s College London, Maurice Wohl Clinical Neuroscience Institute, London, United Kingdom
- 2UK Dementia Research Institute at King’s College London, London, United Kingdom
- 3Departamento de Bioquímica y Biología Molecular y Genética, Facultad de Enfermería y Terapia Ocupacional, Universidad de Extremadura, Cáceres, Spain
- 4Centro de Investigación Biomédica en Red de Enfermedades Neurodegenerativas (CIBERNED), Madrid, Spain
- 5Instituto Universitario de Investigación Biosanitaria de Extremadura (INUBE), Cáceres, Spain
Amyotrophic lateral sclerosis and frontotemporal dementia are neurodegenerative disorders that lie on a disease spectrum, sharing genetic causes and pathology, and both without effective therapeutics. Two pathways that have been shown to play major roles in disease pathogenesis are autophagy and RNA homeostasis. Intriguingly, there is an increasing body of evidence suggesting a critical interplay between these pathways. Autophagy is a multi-stage process for bulk and selective clearance of malfunctional cellular components, with many layers of regulation. Although the majority of autophagy research focuses on protein degradation, it can also mediate RNA catabolism. ALS/FTD-associated proteins are involved in many stages of autophagy and autophagy-mediated RNA degradation, particularly converging on the clearance of persistent pathological stress granules. In this review, we will summarise the progress in understanding the autophagy-RNA homeostasis interplay and how that knowledge contributes to our understanding of the pathobiology of ALS/FTD.
Introduction
Amyotrophic lateral sclerosis (ALS) is the most common form of motor neuron disease and is known to be clinically, genetically and pathologically linked to frontotemporal dementia (FTD), the second most common form of presenile dementia after Alzheimer’s disease. Thus, both disorders relate to one another on a disease spectrum for which there is currently no cure nor disease-modifying treatment available. A considerable number of studies have provided evidence for a link between the dysregulation of RNA homeostasis and defective autophagy in ALS/FTD. Furthermore, recent literature from the autophagy and RNA research fields has provided intriguing connections between these key cellular mechanisms, with implications for our understanding of ALS/FTD pathogenesis.
Autophagy is an evolutionarily conserved lysosomal catabolic pathway that ensures nutrient recycling and removal of unwanted substrates. Consequently, autophagy plays a crucial homeostatic role for maintaining healthy and functional cells, enabling adaptation to changing cellular demands and protection from stress. Even though most studies have focused on autophagy-mediated protein catabolism, accumulating data supports autophagy-mediated RNA degradation as an important additional cellular RNA quality control mechanism. RNA, RNA-binding proteins (RBPs) and RNA-protein complexes (such as RNA granules) can all be degraded by autophagy, although the mechanisms of these processes and their biological consequences have not yet been fully determined and this is now an active field of research. Furthermore, new non-conventional functions of autophagy machinery proteins impacting key aspects of RNA homeostasis have been revealed.
The dysregulation of RNA and protein homeostasis is a major contributor to ALS/FTD pathogenesis. Notably, abnormal accumulation of insoluble protein inclusions in neurons and neuroglia in affected brain regions is a key hallmark of ALS/FTD. These inclusions are frequently characterized by the presence of RBPs involved in RNA processing, such as TAR-DNA binding protein (TDP-43), and proteins from the autophagy machinery, like the selective autophagy receptor, p62. Moreover, ALS/FTD genetics have also uncovered a substantial number of genes involved in autophagy and RNA metabolism. Thus, autophagy and RNA homeostasis are two key dysregulated molecular processes and there is an emerging perspective of an interplay between them in disease.
In this review, we will first provide an overview of important aspects of ALS/FTD and autophagy and then present novel findings regarding conventional and non-conventional functions of the autophagy machinery in RNA catabolism. We will then summarize key evidence for defective autophagy in ALS/FTD, dissecting the relevance of disease-associated proteins in the different stages of this process and defective transcriptional and post-transcriptional regulation. Finally, we will discuss the emerging impact of the interplay between autophagy and RNA homeostasis with particular reference to the complexities in C9orf72-ALS/FTD.
An Overview of Amyotrophic Lateral Sclerosis/Frontotemporal Dementia
ALS and FTD are characterized by progressive degeneration of neurons in affected areas of the nervous system: motor neurons in the motor cortex, brainstem, and spinal cord in ALS and cortical neurons in the frontal and temporal lobes in FTD (Abramzon et al., 2020). Degeneration in these areas results in the clinically observed symptoms of loss of motor control in ALS and behavioural and language dysfunctions in FTD. Despite being clinically distinct, symptoms of both diseases are regularly observed in the same person or segregating within affected families (Abramzon et al., 2020). FTD and ALS are also linked by a common pathological signature, with the vast majority of familial and sporadic cases of ALS and a significant proportion of FTD displaying pathological accumulation of the RBP TDP-43 into cytoplasmic inclusions in neurons and also in glial cells (Neumann et al., 2007). Apart from the clinical and pathological overlap, genetics also unifies both disorders. Classic familial inheritance is observed in 5%–10% of ALS cases and in 30%–50% of FTD cases; whereas some genes like SOD1 or MAPT are predominantly linked to ALS or FTD, respectively, disease-causing variants in a number of genes have been identified in ALS/FTD families (see Table 1). Of particular note, in 2011, the discovery of a pathogenic G4C2 hexanucleotide repeat expansion in a non-coding region of C9orf72 gene causing both ALS and FTD, strengthened the genetic link between these neurodegenerative disorders (DeJesus-Hernandez et al., 2011; Renton et al., 2011). The C9orf72 mutation is the most common cause of familial ALS/FTD with around 40% and 25% of familial ALS or FTD carrying the C9orf72 repeat expansion, respectively, and accounting for around 6% of sporadic cases in both as well (Majounie et al., 2012).
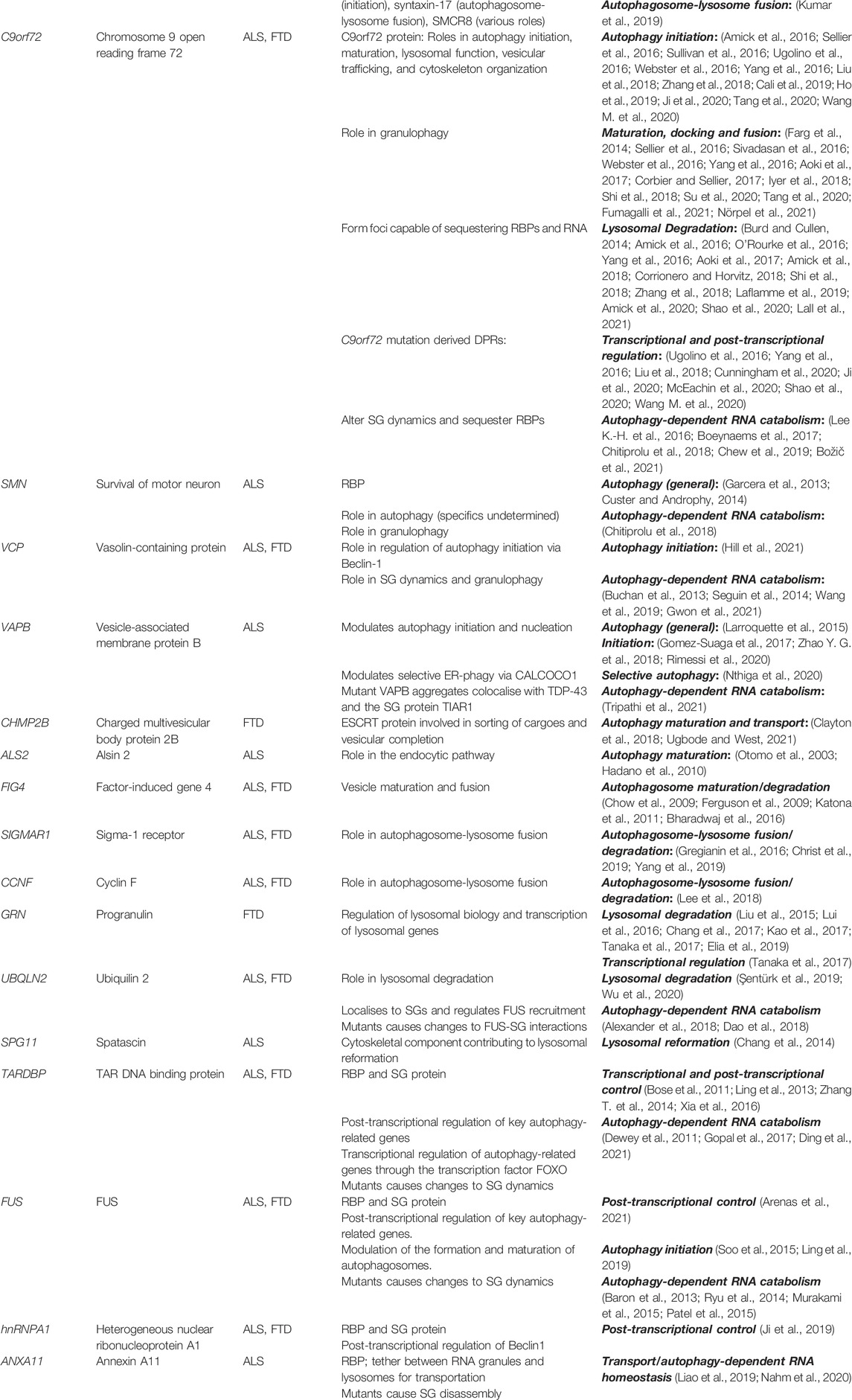
TABLE 1. Autophagy-related ALS/FTD genes and their putative direct or indirect functions in dysfunctional autophagy-RNA homeostasis interplay.
The findings that sporadic and familial ALS/FTD are phenotypically and pathologically indistinguishable and that pathogenic variants in many ALS/FTD-linked genes are described in patients without a family history of disease highlight the value of studying the monogenic, heritable forms (Shepheard et al., 2021). Although ALS/FTD-associated genes do not fit easily into a single cellular pathway, they direct the focus to two key cellular processes: RNA metabolism and autophagy degradation, as many of the ALS/FTD-linked genes determined are implicated in these biological pathways. The first category of ALS/FTD-linked genes fall into those encoding for RBPs, like TARDBP (Sreedharan et al., 2008), FUS (Vance et al., 2009), hnRNPA1 (Kim et al., 2013), ANXA11 (Smith et al., 2017) and SMN (Blauw et al., 2012), implicated in RNA metabolism (see Table 1). RBPs bind to RNA at specific sequences or secondary structures to facilitate several steps of the RNA life cycle, both in the nucleus and cytoplasm. Under physiological conditions, the majority of ALS/FTD-associated RBPs are largely nuclear; however, under pathological conditions, such as when they are affected by ALS/FTD-associated mutations, they are often mislocalised to the cytoplasm and form large insoluble inclusions (Maziuk et al., 2017; Nussbacher et al., 2019).
Mislocalisation and aggregation of mutant RBPs impact their normal functions regulating RNA metabolism. This can result in a loss of function effect on their respective nuclear or cytoplasmic mRNA targets, but aggregation of ALS/FTD-related RBPs can also favour pathological interactions and sequestration of other RBPs or RNA (Nussbacher et al., 2019). An additional gain of function patho-mechanism may derive from the formation of aberrant RNA granules. This mechanism relies on the intrinsic aggregation-prone properties of RBPs; apart from RNA recognition motifs and nuclear import and export sequences, a feature of all RBPs is the presence of low complexity domains (LCDs). These domains allow transient multivalent interactions facilitating the formation of membraneless RNA-protein complexes, such as stress granules (SGs), which require the capacity to form and dissolve rapidly. Many ALS/FTD-causing mutations in RBPs occur in LCDs, increasing the tendency to form poorly dynamic and solid-like complexes which may form the seed for subsequent aggregation (reviewed in Mann and Donnelly, 2021; Solomon et al., 2021). Additionally, mutations in the stress granule components TIA1 and ataxin-2 (ATXN2) have also been associated with ALS (Polymenidou et al., 2011; Sephton et al., 2011; Mackenzie et al., 2017). As already noted, the most frequent RBP which aggregates in ALS/FTD is TDP-43 although mutations in TARDBP itself only underly 1%–4% of ALS/FTD cases, showing that alternative mechanisms can drive RBPs to aggregate (Sreedharan et al., 2008). Similarly, inclusions of the RBP FUS are found in brain tissue of some patients with ALS/FTD both with and without FUS mutations (Vance et al., 2009; van Langenhove et al., 2010).
A second category of ALS/FTD-linked genes fall in those encoding for proteins involved in the autophagy-lysosomal pathway (see Table 1). The products of these genes, such as those belonging to the autophagy machinery, like ubiquilin-2 (gene: UBQLN2) (Deng et al., 2011), optineurin (OPTN) (Maruyama et al., 2010), and p62 (SQSTM1) (Fecto et al., 2011; Rubino et al., 2012), are also identified in both neuronal and glial inclusions in post-mortem ALS/FTD patient tissue from both familial and sporadic cases, and sometimes colocalise with the above mentioned RBPs (Ramesh and Pandey, 2017). While this is also the case for two genes causing FTD (CHMP2B (Skibinski et al., 2005) and GRN), mutations in the MAPT gene predominantly lead to FTD with a different brain pathology, characterized by abnormal hyperphosphorylated tau filaments. Tauopathy is a feature of many diseases and, while autophagy also seems to be a key player in dealing with tau aggregates (Spillantini and Goedert, 2013), we will not discuss this within this review.
Similar to other ALS/FTD genes, the brain pathology of C9orf72 ALS/FTD patients shows typical TDP-43 and p62 inclusions in neurons and glial cells (Al-Sarraj et al., 2011; Murray et al., 2011; Cooper-Knock et al., 2012). However, C9orf72 patients additionally show unique RNA and protein pathologies stemming from the nature of the mutation. The large intronic repeat expansion mutation in C9orf72 is transcribed into sense and antisense repeat RNA which form aggregates termed RNA foci in neurons and glia (Mizielinska et al., 2013). Although in a non-coding genomic region, the sense and antisense repeat RNA can also be translated via an unconventional mechanism into dipeptide-repeat proteins (DPRs) which form protein aggregates in C9orf72-ALS/FTD patient brain and spinal cord (reviewed in Balendra and Isaacs, 2018). The mutation also causes haploinsufficiency of the encoded C9orf72 protein itself. Research is revealing multiples roles for the C9orf72 protein in autophagy, and although its loss does not seem to be the dominant mechanism for neurodegeneration, recent literature supports a model where a combination of C9orf72 haploinsufficiency and gain of function repeat RNA and DPR toxic mechanisms drive disease pathogenesis via a synergistic effect (reviewed in Shi et al., 2018).
Thus, ALS and FTD genetics and pathology have a strong association with dysfunction of RNA homeostasis and autophagy pathways and indicate them as key disease mechanisms that warrant further review.
Autophagy
Autophagy is particularly important in neurons and neuroglia where unique autophagy signalling and functions operate (Stavoe and Holzbaur, 2019; Belgrad et al., 2020; Sung and Jimenez-Sanchez, 2020). Neuronal autophagy is temporally and spatially fine-tuned to face their post-mitotic and long-lived nature and to accommodate their highly polarized structure. In neurons, autophagy plays a key role maintaining neuronal homeostasis as the major degradative pathway for the clearance of larger targets, such as aggregates and dysfunctional organelles. Furthermore, autophagy regulates specific neuronal functions, such as neurodevelopment, including axonal outgrowth and synapse formation, and neuronal activity and plasticity, which underlies the unique function of neurons to pass signals along neuronal networks and enables nervous system functionality. Unsurprisingly, defective neuronal autophagy has been widely linked to proteotoxic stress, aging and neurodegeneration (Stavoe and Holzbaur, 2019).
Neuroglia, comprising microglia, astrocytes, and oligodendrocyte lineage cells, are the other major brain cell types and in recent years have emerged as key players in brain development, physiology and metabolism. However, functions of autophagy in glial cells are less well known than in neurons (Strohm and Behrends, 2020). For example, in microglia, the resident mono-phagocytic cells in the CNS, autophagy participates in immune-related processes such as inflammasome activation (Houtman et al., 2019) and has been shown to be crucial for synaptic homeostasis in mice (Kim et al., 2017). Astrocytes, which help neuronal survival via the release of neurotrophic factors (Li et al., 2019), secrete bioactive molecules in extracellular vesicles in a process that involves divergent secretory organelles, including lysosomes and secretory autophagic vesicles (Verkhratsky et al., 2016), as further explained later. Lastly, in oligodendrocytes, autophagy is involved in myelination, which is one of the crucial functions of these cells (Belgrad et al., 2020). As briefly exemplified here, autophagy contributes to the biology of different cell types in the brain, and thus understanding how autophagy differs or is differentially regulated in neurons and neuroglia is an area of research that warrants the increasing attention it is receiving (Fleming et al., 2022).
However, across all cell types, the autophagy process shares general common features, reviewed here. Three distinct autophagy pathways can be described depending on a cargo’s delivery route to the lysosome: chaperone-mediated autophagy (CMA), microautophagy, and macroautophagy. CMA utilizes cytosolic chaperone proteins, typically the heat shock protein family A (Hsp70) member 8 (HSPA8/HSC70), to bind soluble proteins and direct them to the lysosome. CMA protein targets contain a KFERQ-like recognition motif and are delivered across the lysosomal membrane one-by-one by the lysosomal-associated membrane protein 2A (LAMP2A), which acts as a receptor to translocate them to the lysosomal lumen for degradation (Tekirdag and Cuervo, 2018). During microautophagy cytoplasmic entities destined for degradation are directly taken up by lysosomes or late endosomes (endosomal microautophagy). Although it is the least studied form of autophagy, microautophagy has now proven able to be selective (reviewed in Tekirdag and Cuervo, 2018).
Macroautophagy, herein referred to as autophagy, is the most prevalent and well-characterized. Since it is by far the most studied autophagic pathway in the context of RNA catabolism and ALS/FTD, we will focus on this process. Autophagy involves de novo formation of cytoplasmic double-membrane organelles termed autophagosomes. Autophagosomes sequester cellular components, undergo further maturation steps and finally fuse with lysosomes leading to the degradation of the autophagic cargo by hydrolases. The resultant membranes and nutrients are recycled back to the cytosol for reuse (Figure 1). Thus, each stage of the autophagy process, with its own set of regulatory factors, provide a potential point of dysfunction in disease. Here we provide more details on the autophagy steps implicated in ALS/FTD pathogenesis, providing a context to the disease-associated changes reviewed later.
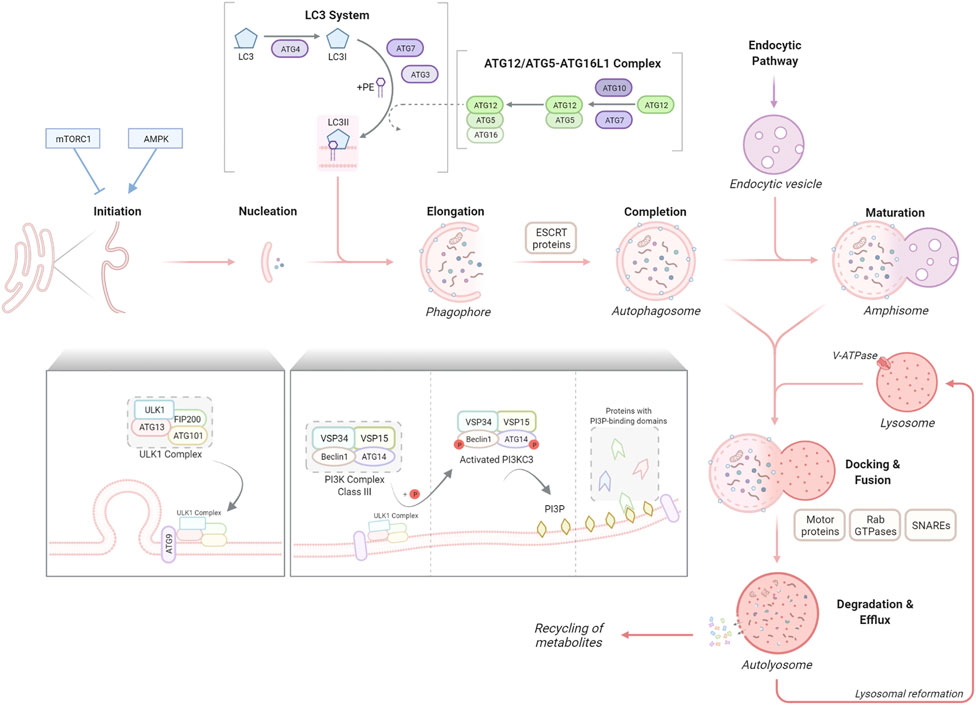
FIGURE 1. The stages of macroautophagy (autophagy). Autophagy initiation is tightly regulated commonly by two well-known kinases, mTORC1 and AMPK1, which inhibit or promote the activity of the ULK1 complex respectively. The ULK1 complex, consisting of ULK1, ATG13, FIP200 and ATG101, translocates to the autophagosome formation site with the transmembrane spanning ATG9 to stimulate nucleation of the phagophore membrane. The ULK1 complex activates the PI3K complex class III, formed of VSP34, VSP15, Beclin1 and ATG14, resulting in the production of PI3P and recruitment of proteins with PI3P-binding domains. The phagophore elongates, sequestering autophagic cargo for degradation. This elongation process is regulated by two ubiquitin-like reactions, the ATG12/ATG5–ATG16L1 complex formation and the conjugation of LC3 to the lipid anchor PE to form LC3-II, facilitated by ATG7 and other proteins. ESCRT proteins enable the elongation and fusing of phagophore edges to form the autophagosome which can undergo further maturation through fusion with endocytic vesicles to form amphisomes. Motor proteins, RAB GTPases and SNAREs facilitate the fusion of autophagosomes/amphisomes with lysosomes to form autolysosomes, within which cargo is degraded into metabolites and released for cellular recycling.
The signalling mechanisms leading to autophagy activation under different types of cellular stressors have been extensively investigated (Yu L. et al., 2018). Two well-known kinases are implicated in the control of this tightly regulated the initiation process: the nutrient sensor, mTOR (mammalian target of rapamycin) complex 1 (mTORC1) and the energy-sensing AMP-activated protein kinase (AMPK), whose inhibition or activation, respectively, triggers autophagy. The ULK1 (Unc-51-like autophagy-activating kinase) complex, which comprises ULK1 itself and ATG13, FIP200 (FAK family kinase interacting protein of 200 kDa; also known as RB1CC1) and ATG101, integrates upstream mTORC1 and AMPK signalling to coordinate the induction of the first steps of the autophagy process (Saxton and Sabatini, 2017).
To initiate autophagosome nucleation, the active ULK1 complex, together with the only multiple transmembrane-spanning ATG protein, ATG9, are translocated to autophagosome formation sites (Mercer et al., 2018); these have been described at different cellular compartments including the plasma membrane, Golgi, mitochondria, lipid droplets, but in particular at the ER (reviewed by Gómez-Sánchez et al., 2021). This translocation is followed by the recruitment of the VPS34/class III PI3K complex (comprising VPS34/PIK3C3, VPS15, Beclin1, and ATG14). Through phosphorylation of members of this complex, the ULK1 promotes PI3KC3 activity, generating the phospholipid PI3P (phosphatidylinositol 3-phosphate) to enable vesicle elongation. PI3P incorporation promotes the recruitment of proteins with PI3P-binding domains, such as FYVE domain-containing proteins (e.g. ALFY, autophagy FYVE-linked protein), which helps in the maturation of the new structure, the phagophore.
Two ubiquitin-like protein cascades, which are highly conserved, are required for the next steps. Two ubiquitin-like proteins, ATG12 and LC3 are conjugated to ATG5 or the lipid phosphatidylethanolamine (PE), respectively. Similar to ubiquitin, covalent conjugation of these proteins is achieved through a cascade of activities that are catalysed by E1 activating enzymes, E2 conjugating enzymes, and E3 ligases (Mizushima, 2020). Briefly, the ATG4 protease cleaves LC3 by removing C-terminal amino acids and exposing a glycine residue. This glycine is used in the first cascade mediated by the E1-like ATG7, followed by ATG3, an E2-like enzyme. Next, in the second cascade, the ATG12/ATG5–ATG16L1 complex, (elongation complex), is formed, involving the conjugation of ATG12 to ATG5 by the E1-like ATG7 and the E2-like ATG10 and the association of ATG16L1 with ATG5. The elongation complex, considered to have E3-like activity, determines the lipidation site of LC3 a process required for the association of LC3 with the autophagosomal membrane (Fujita et al., 2008). During this, LC3 is covalently bound to PE in the membrane (Mizushima, 2020). As the phagophore expands, LC3-PE (also known as LC3-II) is incorporated into the membrane. Consequently, the level of LC3-II can be used to determine the number of autophagosomes in cells (Klionsky et al., 2021). Finally, the edges of the phagophore fuse (vesicle completion) to form the autophagosome, which engulf the cargo for degradation. The endosomal sorting complexes required for transport (ESCRT) machinery is required for this step (Takahashi et al., 2018; Flower et al., 2020). Membrane-bound LC3-PE controls critical steps during the autophagy process apart from the autophagosome closure, such as movement of the autophagosomes or its fusion with the lysosomes, and degradation of the autolysosome inner membrane (Mercer et al., 2018). LC3 is also a key molecule for the binding to adapter proteins in selective autophagy, as further reviewed later.
Autophagosomes undergo fusion with endolysosomal compartments, including early endosomes, multivesicular bodies and late endosomes to form amphisomes (maturation). Autophagosomes/amphisomes are transported along microtubules to the perinuclear region where they fuse with lysosomes to form autolysosomes. The steps of docking and fusion involve a large set of molecules, including cytoskeleton components and related motor proteins, Rab GTPases, SNAREs and other proteins (Kriegenburg et al., 2018). Most of the molecular machinery that is responsible for these events involve proteins that are shared with the endocytic pathway (Mercer et al., 2018). Finally, the enclosed cargo is degraded by lysosomal hydrolases, allowing for the release and recycling of the newly generated metabolites and lysosomal reformation. An acidic environment in the lysosomal lumen, generated by the vacuolar H+ ATPase (V-ATPase) at the lysosomal membrane, is essential for this degradation (Yu L. et al., 2018).
Autophagy is also controlled at the transcriptional regulation level (di Malta et al., 2019). The transcription factor TFEB (transcription factor EB) and related MiTF/TFE (microphthalmia-associated transcription factor/transcription factor E) family members recognize and bind to coordinated lysosomal expression and regulation (CLEAR) motifs in the promoter region of many lysosomal and autophagy genes, leading to an increase in their transcription and a global enhancement of autophagy flux (Sardiello et al., 2009; Palmieri et al., 2011; Settembre et al., 2012; Martina et al., 2014; Ploper et al., 2015). We here highlight the role of the master regulator TFEB. TFEB shuttles between the nucleus and the cytoplasm, which is mainly regulated by mTORC1 and Rag GTPases on the lysosomal membrane (Puertollano et al., 2018). In response to amino acid signals, Rag GTPases recruit TFEB to the lysosome where it is phosphorylated by mTORC1, inhibiting its nuclear translocation and activation (Puertollano et al., 2018). Therefore basally, TFEB remains in its inactive phosphorylated form in the cytosol. Upon nutrient deprivation, or other cellular stressors, such as lysosomal damage or oxidative stress (Jia et al., 2018; Wang H. et al., 2020), TFEB gets dephosphorylated (activated) and translocates into the nucleus. Here, it enhances the expression of important genes for the autophagy-lysosomal pathway such as lysosomal proteins, for example, LAMP1/2 and hydrolases, and key proteins of the autophagy machinery such as beclin1 or p62 (Puertollano et al., 2018).
New studies have provided evidence for an additional regulatory layer of the autophagy process at the post-transcriptional level, governed by RBPs which regulate the processing and translation of specific autophagy-related transcripts (Sakellariou et al., 2021). Recently, noncoding RNAs have also been added to this regulatory function, furthering the interplay of autophagy and RNA (Xu et al., 2017; Yang et al., 2017; Zhao et al., 2020). However, although post-transcriptional/co-translational regulation of autophagy has received increasing attention in recent years, it is still poorly understood.
Autophagy-Dependent RNA Catabolism
Apart from the conventional RNA decay pathways (reviewed by Houseley and Tollervey, 2009; Tatosyan et al., 2020), autophagy-mediated RNA degradation complements these quality control mechanisms to remove obsolete/defective RNA molecules. Additionally, RBPs and RNA-protein complexes, such as RNA granules, are also autophagy substrates, indirectly affecting RNA homeostasis. RNA degradation by autophagy was initially suggested in early observations from the 80–90s (Sameshima et al., 1981).These pioneering studies demonstrated increased RNA degradation during starvation-induced autophagy. In agreement with a lysosomal RNA degradation pathway, several acid ribonucleases (RNases) have been identified within lysosomes (Schröder et al., 2010). Interestingly, loss-of-function mutations in the RNase RNASET2 leads to familial cystic leukoencephalopathy and displays lysosomal accumulation of ribosomal RNA in neurons and undigested substrates in microglia (Haud et al., 2011; Hamilton et al., 2020). Although the role of autophagy-dependent RNA catabolism has not been fully characterized, recent studies have described three different mechanisms - RNautophagy, ribophagy and granulophagy (Figure 2).
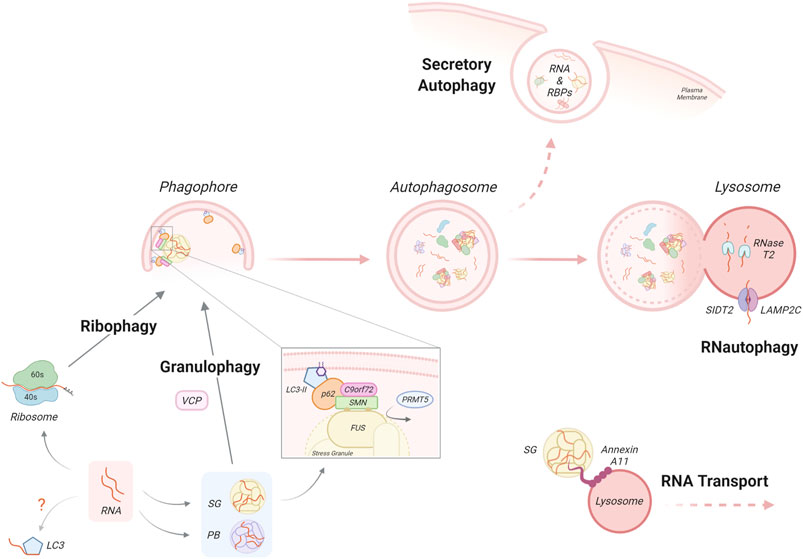
FIGURE 2. Autophagy-dependent RNA homeostasis. Autophagy-dependent RNA catabolism involves the degradation of RNA, RNA-binding proteins and RNA granules within the lysosomal lumen by RNases such as RNase T2 and lysosomal acidic hydrolases. Through RNautophagy, mRNA molecules are taken up directly into the lysosome through SIDT2 or LAMP2 proteins, in an ATP-dependent manner. Unbound RNA can also directly interact with LC3 via its arginine-rich motif however the impact of this requires further investigation. Ribophagy is a mechanism for autophagy dependent ribosome clearance by which ribosomes are engulfed within the autophagosome and delivered to the lysosome for degradation. mRNA can associate with RNA-binding proteins and accumulate in dynamic RNA granules such as stress granules (SG) or P-bodies (PB). Granulophagy is the process by which SGs are recruited for selective autophagy, which may involve the protein VCP. An additional mechanism of SG recruitment has been proposed, by which the PRMT5-dependent symmetric arginine methylation of SG components, such as FUS, allows for their recognition by the p62/C9orf72 complex via the SMN protein. Autophagy-dependent RNA catabolism is also impacted by the transport and secretion of RNA/RNA complexes. RNA transport involves SGs and other RNA complexes hitchhiking on motile vesicles, a process that involves the docking of RNA granules via molecular tethers. Notably, the ALS-associated RBP Annexin A11 is a component of SGs and can also bind lysosomes that are transported along microtubules. The autophagy machinery is also involved in the non-canonical autophagy function of secretory autophagy. By this process, the contents of autophagosomes, which may contain RNA and RBPs, bypass degradation and instead are directly secreted from the cell.
Uptake of mRNA molecules into lysosomes by the autophagy mechanism of RNautophagy does not require the formation of a double-membrane vesicle but instead resembles CMA (Schröder et al., 2010; Haud et al., 2011; Hamilton et al., 2020). During RNautophagy, the uptake occurs by direct binding of RNA to the lysosomal membrane proteins LAMP2C or SIDT2 (SID1 transmembrane family member 2), which act as nucleic acid receptors in an ATP-dependent manner (Fujiwara et al., 2013). The cytoplasmic region of LAMP2C/SIDT2 binds to RNA/DNA via its arginine-rich motif and possesses binding preference for stretches of poly-guanosines (Fujiwara et al., 2015; Hase et al., 2015; Hase et al., 2020). This binding may be regulated by other RNA-interacting proteins such as RBPs or DNA-binding proteins in the cytosol. Indeed, the ALS/FTD linked RBP hnRNPA1 was found to interact with LAMP2C in an RNA-dependent manner, in addition to a range of other RBPs (Fujiwara et al., 2013). In vitro studies have identified other lysosomal membrane proteins as RNA interactors, such as the mammalian CMA effector HSPA8 and the yeast lysosomal membrane V-ATPase subunit Vma1; however, the biological significance of these findings in terms of RNA-mediated autophagy degradation has not yet been determined (Castello et al., 2012). Indeed, further work is required to understand the substrates, sensors and regulation in RNautophagy. At present, the relative contributions of conventional RNA decay and this autophagy-mediated pathway in mRNA degradation are unclear.
Ribosomal RNA (rRNA) is the primary component of ribosomes and represents about 80% of total cellular RNA (Darnell, 1968; Warner, 1999; Weinberg et al., 2016). There are several pathways of eukaryotic ribosomal RNA decay (reviewed in Inada, 2020). However, ribosomes can also be targeted and removed by autophagy (An and Harper, 2020). Indeed, in electron microscopy studies, ribosomes are frequently detected inside autophagic structures in starved cells, leading to the general notion that ribosomes are non-selective cargo during bulk autophagy (Eskelinen et al., 2011). However, a growing body of evidence suggests that selective autophagic mechanisms directed to ribosomes (ribophagy) are employed in both yeast and mammalian cells (Beese et al., 2020). In mammalian cells, the protein NUFIP1 (nuclear fragile X mental retardation-interacting protein 1) has been identified as a ribophagy receptor for the 60S subunit, interacting with LC3B and ribosomes in response to nutrient stress (Wyant et al., 2018). However, a recent work has challenged this role, finding that NUFIP1 deletion has no effects on ribophagy flux (An et al., 2020). Indeed, a study from the same group has suggested that the overall contribution of ribophagy to ribosome homeostasis during nutrient stress is small relative to other pathways, such as the ubiquitin-proteasome system (An and Harper, 2018; An et al., 2020).
mRNAs exiting translation often accumulate in distinct cytoplasmic RNA-protein complexes, such as SGs and P-bodies. These are cytoplasmic membraneless organelles comprised of RNA and RBPs with key roles in the regulation of gene expression and cellular homeostasis (Alberti and Carra, 2018). SGs are assembled during a stress response, when global translation initiation is inhibited; thus, they are transient, reversible structures and their formation constitutes an adaptation mechanism for maintaining RNA metabolism homeostasis during unfavourable environmental conditions. Indeed, several studies have shown a pro-survival role for SGs (Reineke and Neilson, 2019; Samir et al., 2019). Interestingly, recent evidence suggests that translation of mRNAs can take place at the boundary of SGs, arguing against an exclusive role for SGs in inhibition of protein synthesis (Mateju et al., 2020). Physiologically, once the stress is resolved, SGs can be cleared by several mechanisms including disassembly by molecular chaperones (Ganassi et al., 2016; Mateju et al., 2017; Mediani et al., 2021), clearance by the ubiquitin–proteasome system (Turakhiya et al., 2018) and by selective autophagy – thus termed granulophagy (recently reviewed in Alberti and Carra, 2018). Under basal conditions only 5%–10% of SGs are degraded by granulophagy. However, autophagy-dependent SG degradation increases dramatically during stress and disease (Buchan et al., 2013; Mateju et al., 2017; Chitiprolu et al., 2018), which will be discussed later.
Autophagic clearance of SGs has been implicated by their recruitment of key autophagic proteins, such as p62 and LC3 (Buchan et al., 2013; Ganassi et al., 2016). A key study demonstrated that the molecular chaperone Cdc48 (human homologue: VCP), an ALS/FTD associated protein, is critical to autophagic degradation of SGs in yeast (Buchan et al., 2013). However, another study has shown that ULK1/2 localises to SGs where it phosphorylates VCP and increases its potential to drive SG disassembly in mammalian cells (Wang et al., 2019), which was not shared by other effectors of autophagy. Therefore, demonstrating both autophagy dependent and independent roles for classical autophagy proteins in SG clearance. Generally, selective autophagy involves the targeting of cargo by ubiquitination; this modification is recognized by the ubiquitin-associated domain of autophagy receptors (Conway et al., 2020), such as p62 and optineurin, which are also associated with ALS/FTD. However, one study has reported an alternative C9orf72 protein-mediated ubiquitin-independent granulophagy (Chitiprolu et al., 2018). Instead, symmetric arginine methylation of SG components by PRMT5 (protein arginine methyltransferase 5) was necessary for the selective targeting of SGs. This non-canonical signal was specifically recognized by p62 in a complex with C9orf72 via the Tudor protein SMN (survival motor neuron protein). Interestingly, impaired autophagy can also lead to anomalies in SG formation and morphology, which may be due to aberrant recruitment of defective ribosomal proteins (Seguin et al., 2014). These abnormal SGs contained noncanonical components, such as the 60S ribosomal subunit, which is normally absent. Furthermore, p62 and the autophagy receptor CALCOCO1 (also known as NDP52) have been implicated in autophagy-mediated degradation of retrotransposon RNA, localized to both RNA granules, P-bodies and SGs. This autophagy-mediated control in retrotransposon RNA degradation affects their insertion within the genome, which might be relevant for tempering somatic mosaicism (Guo et al., 2014). These selective mechanisms share the essential autophagy steps and machinery reviewed above, and so any impact on them may have a concomitant effect on RNA-selective autophagy degradation.
In addition, there are noncanonical roles for autophagy in RNA metabolism. Secretory autophagy is a newly defined autophagy function that bypasses the degradative process to allow the secretion of autophagic structures (Xu et al., 2018); of note, nucleic acids and RBPs have been found to be secreted by this pathway (Leidal et al., 2020). Interestingly, this process was also apparent in glial cells. Therefore, in addition to a known role for secretory autophagy in the release of aggregation-prone proteins (Lee J.-G. et al., 2016), the autophagy pathway may be implicated in non-cell autonomous control through excretion of nucleobases and RBPs (van Niel et al., 2018; Xu et al., 2018). LC3 has been reported to be responsible for the specific cargo loading of RBPs and small noncoding RNAs into vesicles to be secreted (Leidal et al., 2020). Interestingly, many RBPs are found to contain LC3-interacting (LIR) consensus motifs (Leidal et al., 2020). Furthermore, LC3 possesses a direct RNA-binding capacity through its own arginine-rich motif (Kraft et al., 2016), which has already been shown to play a role in the translational regulation of certain RNA (Zhou et al., 1997).
Another emerging role for autophagy in RNA metabolism is related to RNA transport, which is especially relevant for maintaining highly polarized cells such as neurons (Salogiannis and Reck-Peterson, 2017). The transport of RNA from neuronal soma to distal sites such as synapses allows local protein synthesis which is essential for the precise spatiotemporal control required for neuronal function. mRNAs associate with RBPs and are transported as RNA-protein complexes. Recent works have described a transport mechanism for RNA granules, known as “hitchhiking”, where they are indirectly transported along microtubules by docking onto endosomes, lysosomes or other membrane-bound organelles (Pohlmann et al., 2015; Gershoni-Emek et al., 2018; Cioni et al., 2019; Liao et al., 2019; Olgeiser et al., 2019). The ALS-related RBP Annexin A11 which is a component of SGs but also has phosphoinositide-binding capacity is reported to act as a molecular tether between RNA granules and lysosomes (Markmiller et al., 2018; Liao et al., 2019). Thus, due to the integration with the endocytic and lysosomal pathways, autophagy may also influence RNA transport.
Dysfunctional Autophagy in Amyotrophic Lateral Sclerosis/Frontotemporal Dementia
Several stages of the autophagy pathway, in addition to selective autophagy mechanisms, are affected by ALS/FTD-linked genes (Figure 3). Most of these are ubiquitous expressed, including in neurons and neuroglia, and although the majority of studies have focussed on the role of these genes in neurons, some neuroglia specific roles have been reported. Here, we summarize the roles of these ALS/FTD genes relative to the specific stages of autophagy.
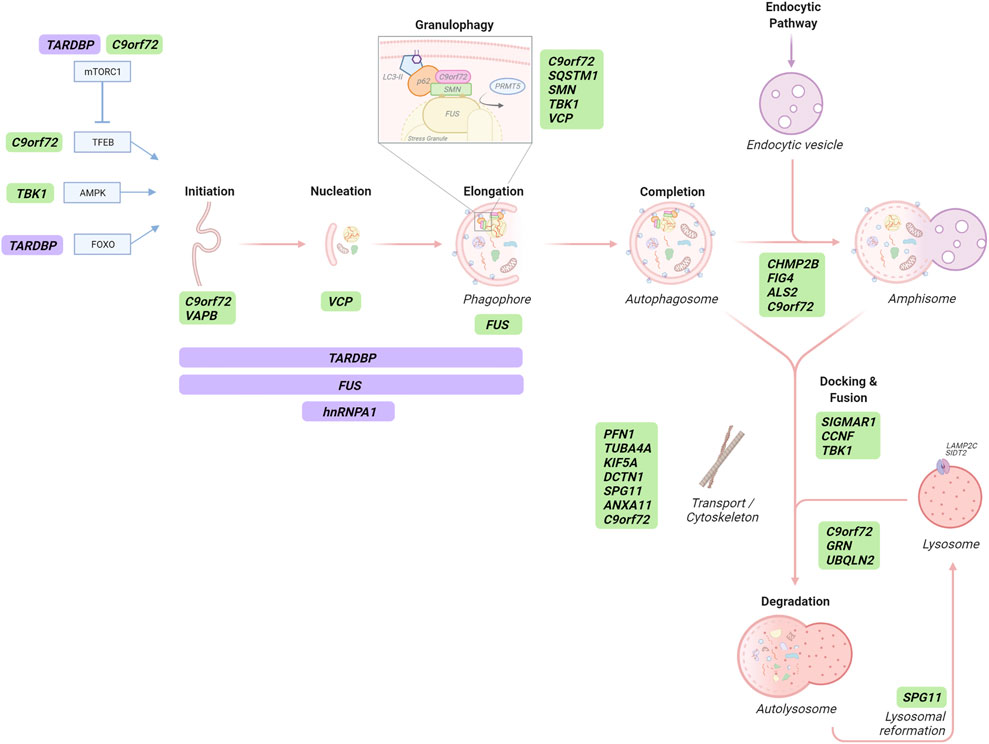
FIGURE 3. The role of ALS/FTD-associated genes in autophagy-dependent RNA catabolism. Several stages of the autophagy pathway, in addition to selective autophagic degradation of stress granules, are affected by ALS/FTD-linked gene (green) products, some of which are RBPs that transcriptionally and/or post-transcriptionally regulate autophagy-related proteins (purple). Autophagy initiation is transcriptionally regulated by TFEB, AMPK and FOXO which can be affected by C9orf72 protein and repeat RNA/DPRs, TBK1 and TDP43 (TARDBP), respectively. TFEB activity is modulated by mTORC1 which is also regulated by C9orf72 and TDP43 proteins. C9orf72, VAPB, VCP and FUS proteins play roles in different stages of autophagosome formation. Proteins participating in autophagosome formation are also post-transcriptionally regulated by TDP43, FUS and hnRNPA1 proteins. Selective SG degradation - termed granulophagy - can be mediated by C9orf72, p62 (SQSTM1), SMN, TBK1 and VCP proteins. CHMP2B, FIG4, ALS2 and the C9orf72 protein participate in the maturation of autophagosomes to form amphisomes in the intersection with the endocytic pathway. SIGMAR1, CCNF and TBK1 gene products are required for appropriate autophagosome-lysosome membrane fusion. Retrograde transport of autophagic structures contributes to proper autophagy flux and it is affected by ALS/FTD-linked PFN1, TUBA4A, KIF5A, DCTN1 and SPG11 genes, coding for proteins of the vesicle transport machinery. Likewise, the C9orf72 protein is proposed to contribute vesicular trafficking, and annexin A11 (ANXA11) plays a role in RNA granule-lysosomal trafficking. Appropriate lysosomal degradative capacity of autophagic cargo is affected by ALS/FTD-linked proteins encoded by C9orf72, GRN and UBQLN2. Finally, spatacsin (SPG11) is involved in lysosomal reformation.
Autophagosome Formation
Since the discovery of the mutation in ALS/FTD, studies into loss of the C9orf72 protein function (predominantly the long isoform) has been widely linked to regulation of the autophagy pathway. However, opposing effects have been reported. Several studies have shown that C9orf72 forms a stable complex with WDR41 (WD repeat-containing protein 41) and SMCR8 (Smith–Magenis chromosome regions 8) (Amick et al., 2016; Sellier et al., 2016; Sullivan et al., 2016; Ugolino et al., 2016; Yang et al., 2016; Zhang et al., 2018; Tang et al., 2020); this complex regulates the early steps of autophagy by interaction with the autophagy initiation complex, ULK1-FIP200-ATG13-ATG101 (Sellier et al., 2016; Sullivan et al., 2016; Webster et al., 2016; Yang et al., 2016; Ho et al., 2019). Perturbation of this interaction by reduced or knocked out C9orf72 has been associated with disrupted autophagy (Yang et al., 2016; Ho et al., 2019). However, other studies show that C9orf72 can negatively regulate autophagy (Ugolino et al., 2016; Yang et al., 2016; Liu et al., 2018; Ji et al., 2020; Wang M. et al., 2020) via upstream modulation of mTORC1 signalling (discussed below).
A mutation in the endoplasmic reticulum (ER)-mitochondria tethering protein VAPB (vesicle-associated membrane protein-associated protein B) is a rare cause of ALS (Nishimura et al., 2004) and causes a reduction in VAPB protein stability (Mitne-Neto et al., 2011). Interestingly, a decrease in VAPB protein is also observed in the spinal cord of people with sporadic ALS (Anagnostou et al., 2010). VAPB has been linked to several pathways involved in autophagy initiation. One function is exerted through binding to the mitochondrial protein PTPIP51 (protein tyrosine phosphatase-interacting protein-51) at ER-mitochondria contact sites, acting as a tether between the two organelles (de Vos et al., 2012; Stoica et al., 2014). ER-mitochondria contacts and the VAPB-PTPIP51 tethers have been shown to regulate autophagy by controlling ER-mitochondrial calcium transfer (Gomez-Suaga et al., 2017). Indeed, loss of either VAPB or PTPIP51 decreases ER-mitochondria contacts and increases autophagic flux, whereas overexpression of VAPB or PTPIP51 tightens the ER-mitochondria contacts and inhibits autophagosome formation (Gomez-Suaga et al., 2017). These effects are also seen in the context of selective autophagy during pathogen infection (Rimessi et al., 2020). VAPB has been proposed to exert a role in the recruitment of the autophagy initiation ULK1 complex during autophagosome formation through a direct interaction with ATG proteins (Zhao Y. P. et al., 2018). It also interacts with the ER-phagy receptor CALCOCO1, linking VAPB with early steps of this selective autophagy pathway (Nthiga et al., 2020). Mutant VAPB is also able to alter autophagic flux in multiple cell lines and a mutant knock-in mouse model (Larroquette et al., 2015), and accumulation of autophagy structures colocalising with VAPB has been reported in a VAPB-ALS patient fibroblasts and muscle biopsy (Tripathi et al., 2021). However, whether this increase in autophagy structures is related to the loss of function of wild-type VAPB or the mutant protein remains unknown.
Maturation, Docking, and Fusion
C9orf72 has been shown to interact with multiple Rab GTPases (Farg et al., 2014; Sellier et al., 2016; Webster et al., 2016; Yang et al., 2016; Aoki et al., 2017). Rab GTPases are major players in membrane trafficking events, including autophagy (Marat et al., 2011); like other GTPases, cycle between an inactive GDP-bound form in the cytoplasm and a membrane-associated GTP-bound active form. A guanine nucleotide exchange factor (GEF) facilitates the exchange of nucleotides and activates the Rab, which then interacts with downstream effectors to exert its biological function. Rab inactivation requires a GTPase-activating protein (GAP) to catalyse efficient nucleotide hydrolysis (Stenmark, 2009). The C9orf72-WDR41-SMCR8 complex was initially proposed to have GEF activity on Rab GTPases, such as Rab8A and Rab39B, resulting in their activation (Sellier et al., 2016; Yang et al., 2016; Iyer et al., 2018); both of which are implicated in autophagosome maturation (Pilli et al., 2012; Szatmári and Sass, 2014; Corbier and Sellier, 2017). Importantly, a constitutively active form of Rab39b, but not Rab8a, rescued autophagy defects caused by C9orf72 depletion in cultured primary mouse cortical neurons (Sellier et al., 2016). However, recent in vitro studies have suggested opposing GAP activity (Su et al., 2020; Tang et al., 2020; Nörpel et al., 2021); clearly an area of research that requires further clarification.
Autophagosome maturation is hampered by pathogenic variants in the ALS2 gene as well. These variants have been identified in rare juvenile motor neuron diseases including ALS (Hadano et al., 2001; Yang et al., 2001). The ALS2 gene encodes for Alsin 2, a GEF for the small GTPase Rab5, a key protein in the endocytic pathway (Otomo et al., 2003). Secondary knockout of ALS2 displayed accumulation of autophagosome-like vesicles and accelerated disease progression in a mutant SOD1-ALS transgenic mouse model (Hadano et al., 2010).
Defects in the ESCRT system can cause various neurodegenerative diseases (Saksena and Emr, 2009). ESCRT-III proteins, including the ALS/FTD-associated protein CHMP2B (charged multivesicular body protein 2B) (Skibinski et al., 2005; Parkinson et al., 2006; Cox et al., 2010), are responsible for the sorting of cargoes and the completion of vesicular formation (Ugbode and West, 2021). CHMP2B-FTD is unusual in its lack of TDP-43 aggregates, presenting instead with inclusions positive for ubiquitin and p62 and lysosomal storage pathology (Holm et al., 2007; Clayton et al., 2015). The most common M178V mutation results in C-terminal truncation of CHMP2B; this results in loss of the MIT-interacting motif, which is required for interaction with Vps4, a critical ATPase in scission of vesicles into multivesicular bodies. CHMP2B mutant models and patient tissue display disrupted vesicle trafficking and accumulation of both endolysosomal and autophagy structures (Clayton et al., 2018; Ugbode and West, 2021).
A similar impact on the endo-lysosomal pathway can derive from ALS/FTD-associated loss-of-function variants in FIG4 (Factor-induced gene 4) (Chow et al., 2009). FIG4 is a phosphoinositide phosphatase involved in (PI3P) generation (Ho et al., 2012); apart from its role in autophagy, PI3P has functions in several membrane tracking events such as membrane identity, endosomal maturation and degradation (Marat and Haucke, 2016). Loss or mutation of FIG4 results in lysosomal phenotypes in several disease models (Casterton et al., 2020) which according to recent work may be independent from its role as a phosphatase (Bharadwaj et al., 2016). FIG4 null mice display p62-positive inclusions, of which most are found in astrocytes, highlighting the relevance of autophagic clearance in glial cells (Ferguson et al., 2009).
ALS/FTD can be caused by recessive mutations in the SIGMAR1 gene, which encodes the sigma-1 receptor (sig-1R) (Luty et al., 2010). Although sig-1R participates in a broad array of biological functions (Aishwarya et al., 2021), reduced autophagy flux and accumulation of autophagic structures are characteristics of several cell and mouse models of SIGMAR1-ALS/FTD (Christ et al., 2020). Likewise, pharmacological activation or overexpression of sig-1R can increase autophagy flux in vitro and in vivo (Gregianin et al., 2016; Christ et al., 2019). Recently, loss of sig-1R or expression of ALS-associated mutants has revealed specific impairment of autophagosome-lysosome membrane fusion with concomitant autophagy flux defects (Yang et al., 2019).
Similarly, mutations in CCNF account for rare cases of familial ALS-FTD (Williams et al., 2016). Cyclin F, encoded by CCNF gene, is one of the components of an E3 ubiquitin-protein ligase complex and ALS/FTD-associated mutations have been found to impair autophagosome-lysosome fusion through direct binding and increased ubiquitylation of p62/SQSTM1 (Lee et al., 2018).
Finally, in neurons, autophagosomes in distal processes are required to be transported toward the soma where mature acidic lysosomes are predominantly located, and thus impairments in retrograde transport can contribute to defective autophagy flux (Nixon, 2013). Axonal transport deficiency is observed early in many different ALS/FTD models, and therefore may play an important role in the initiation of disease (de Vos and Hafezparast, 2017). Indeed, several pathogenic variants in genes encoding vesicular transport machinery proteins, such as PFN1 (profilin) (Chen et al., 2013; Ingre et al., 2013), TUBA4A (tubulin isotype α4a) (Smith et al., 2014), KIF5A (kinesin family member 5A) (Nicolas et al., 2018) and DCTN1 (dynactin subunit 1) (Münch et al., 2004), in addition to the cytoskeleton component SPG11 (spatacsin) (Orlacchio et al., 2010), are directly associated to ALS. Defective transport linked to these genes might damage autophagy by impairing anterograde movement of lysosomes, lysosomal biogenesis and/or autophagosome clearance; indeed, mutations in dynactin have been linked to an impairment in autophagosome-lysosome fusion due to redistribution to the cell periphery (Farías et al., 2017; Laird et al., 2008; Münch et al., 2004; Yu et al., 2018). The C9orf72 protein may also play a role in both vesicular trafficking and cytoskeleton organization: by interaction with cofilin 1 and modulation of the small GTPases ARF6 and RAC1, C9orf72 has been associated to actin dynamics and axon outgrowth in cultured motor neurons (Sivadasan et al., 2016).
Lysosomal Degradation
A role for the C9orf72 protein in the lysosomal-autophagy pathway has been identified by its lysosomal localization during amino acid starvation; this localization is driven by an interaction of WDR41 within the C9orf72-WDR41-SMCR8 complex with the lysosomal amino acid transporter PQLC2 (Amick et al., 2016; Amick et al., 2018; Amick et al., 2020). In agreement, C9orf72 knockdown in cells can affect both autophagy induction and flux (Yang et al., 2016). Studies support a role for C9orf72 at the lysosome in degradation and exocytosis, due to impaired autolysosome acidification, and also transcriptional regulation (see below) and potentially lysosomal reformation (Amick et al., 2016; Corrionero and Horvitz, 2018; Zhang et al., 2018; Shao et al., 2020). Of note, although early studies reported co-localization of C9orf72 protein with several organelles of the endocytic pathway, the nucleus, the Golgi apparatus, neurites, growth cones and RNA granules (Renton et al., 2011; Farg et al., 2014; Atkinson et al., 2015; Aoki et al., 2017; Chitiprolu et al., 2018; Shi et al., 2018), a validation study of the commercial antibodies used in these studies showed that many cannot specifically recognize C9orf72 protein (Laflamme et al., 2019); however, the antibodies determined to be specific still localised C9orf72 to lysosomal and autophagy structures. This study also confirmed high expression of C9orf72 in immune cells, which links in with the strong phenotype of autoimmunity and inflammatory disease in C9orf72 knockout mice (Balendra and Isaacs, 2018).
Indeed, several studies have demonstrated that C9orf72 protein is required for proper macrophage/microglial function, with abnormal lysosomal accumulation in knockout cells (O’Rourke et al., 2016; Zhang et al., 2018; Shao et al., 2020; Lall et al., 2021). This is consistent with the microglial hyperactivation and astrogliosis that have been described in C9orf72 ALS/FTD post-mortem brain and cerebrospinal fluid (Haidet-Phillips et al., 2011; Brettschneider et al., 2012; Cooper-Knock et al., 2012; Oeckl et al., 2019). A large body of evidence now supports the notion that glial cells can contribute to ALS/FTD (Meyer et al., 2014; Madill et al., 2017). As mentioned above, microglial autophagy is crucial for synaptic homeostasis in mice (Kim et al., 2017). Indeed, the microglia in both pan and microglia-specific C9orf72-knockout mice become activated, exhibit enhanced synaptic pruning and accumulate lysosomal material (Lall et al., 2021). On the other hand, the autophagy machinery has been related to the non-canonical autophagy function of secretory autophagy, of special relevance in astrocytes, the glial cells which provide trophic support for neurons (Verkhratsky et al., 2016). Indeed, dysfunctional autophagy in astrocytes is known to contribute to neurodegeneration in a lysosomal store disorder by impairing their ability to metabolically support neurons (di Malta et al., 2012; Sung and Jimenez-Sanchez, 2020). Regarding C9orf72-ALS/FTD, a recent study has associated the mutation to altered astrocytic extracellular vesicle secretion of micro RNAs (miRNAs) that are involved in the regulation of axonal maintenance genes and restoration of miRNA activity could partially ameliorate toxicity of conditioned media from C9orf72 patient derived astrocytes or motor neurons (Varcianna et al., 2019). Since miRNAs can also act as regulators of autophagy (Shah et al., 2018), it would be interesting to analyse a possible non-autonomous control of neuronal autophagy by C9orf72 mutant astrocytes. Additionally, the mechanisms underlying C9orf72 mutant astrocyte-mediated neurotoxicity may arise from secretion of neurotoxic factors (Haidet-Phillips et al., 2011), such as inflammatory cytokines also via the secretory autophagy pathway (Colombo and Farina, 2016). Accumulation of p62 and increased secretion of lysosomal components are also observed in C9orf72 deficient macrophages, which promote a pro-inflammatory state and altered immune responses (Zhang et al., 2018; Shao et al., 2020), suggesting a role for the encoded C9orf72 protein. Collectively, these studies evidence the contribution of defective glial autophagy and non-cell autonomous patho-mechanisms via impairments in lysosomal function from loss of C9orf72 protein in C9orf72-ALS/FTD.
The C9orf72 protein has also been linked to inappropriate sorting of lysosomal hydrolase precursors by an effect on retromer trafficking via interaction with Rab7L1 (Burd and Cullen, 2014; Aoki et al., 2017). The retromer is involved in the retrograde transport of transmembrane proteins from the late endosomes to the trans-Golgi network (TGN). This includes the mannose 6-phosphate receptor (M6PR) which cycles between the TGN and endosomes to deliver lysosomal hydrolases through the endocytic pathway into lysosomes. Downregulation of C9orf72 caused dysfunctional retromer transport of M6PR and impaired autophagosome accumulation in C9orf72-ALS/FTD patient-derived fibroblasts (Aoki et al., 2017). In agreement, a recent study has shown a correlation between deficits in retromer and lysosomal functions and reduced synaptic vesicular trafficking in C9orf72 patient and C9orf72 knockout iPSC-derived motor neurons (Shi et al., 2018).
Haploinsufficiency of progranulin (PGRN) due to loss-of-function mutations in the GRN gene is a leading genetic cause of pure FTD (Baker et al., 2006; Cruts et al., 2006; Schymick et al., 2007; Cannon et al., 2013). Multiple functions have been described for progranulin, but evidence supports a major role for this glycoprotein in regulating different aspects of lysosomal biology (Kao et al., 2017). Progranulin can both facilitate lysosomal acidification and regulate lysosomal protein levels and gene expression (Tanaka et al., 2017). Reduction of progranulin resulted in increased lysosomal gene expression and protein levels, the latter of which included mature Cathepsin D, in agreement with its accumulation in PGRN-FTD patient brain (Tanaka et al., 2017). Knockdown of progranulin has however been shown to both decrease and increase autophagic flux (Liu et al., 2015; Chang et al., 2017; Elia et al., 2019). A recent work has proposed an additional loop in which the level of progranulin itself is regulated by autophagy, as a substrate (Elia et al., 2019). Thus, there is a complex interplay of progranulin with the autophagy-lysosomal pathway. Interestingly, both overexpression and knockdown of progranulin has been reported to result in the accumulation of insoluble TDP-43 and varying between cell lines, suggesting that selective autophagy may also be compromised in PGRN-FTD (Tanaka et al., 2017; Elia et al., 2019). As other ALS/FTD-associated proteins, progranulin is broadly expressed by many cell types, including neurons, astrocytes and prominent in activated microglia, regulating neuron and immune functions (Petkau and Leavitt, 2014; Elia et al., 2019). Indeed, a still not well-understood neuro-immunomodulatory action has been postulated for progranulin in microglial activation (Chitramuthu et al., 2017). Progranulin deficiency leads to alterations in lysosomal function that drive increased production of complement proteins and enhanced synaptic pruning by microglia (Lui et al., 2016). These findings suggest that progranulin-mediated neurodegeneration may be partially caused by lysosomal dysfunction and subsequent aberrant microglial activation.
Missense mutations in UBQLN2 gene cause X-linked ALS/FTD (Deng et al., 2011), and cytoplasmic inclusions of the encoded protein ubiquilin-2 have been described in degenerating motor neurons broadly in familial and sporadic ALS (Fecto and Siddique, 2011). Disruption of both proteasomal degradation and autophagy have been proposed as pathogenic mechanisms for mutant ubiquilin-2 (Casterton et al., 2020). Although ubiquilin-2 can indirectly interact with LC3 (Rothenberg et al., 2010), it seems to predominantly act instead in later stages of autolysosome degradation; two recent works have shown that ubiquilin 2 is required for lysosomal acidification, exerting a regulatory role on V-ATPase function via interaction with different subunits of the V-ATPase pump (Şentürk et al., 2019; Wu et al., 2020).
Finally, loss-of-function mutations in the SPG11 gene, encoding for the cytoskeleton component spatacsin, are a cause of juvenile-onset ALS (Orlacchio et al., 2010) and have been found to play a role during lysosomal reformation (Chang et al., 2014).
Transcriptional and Post-Transcriptional Regulation
Although TDP-43 and FUS-mediated pathogenicity is established through direct deregulation of RNA homeostasis (Ling et al., 2013), they also have roles as autophagy regulators. Several studies have implicated TDP-43 in autophagy regulation post-transcriptionally. TDP-43 can bind to and regulate the mRNAs of multiple autophagy-related genes including ATG7, SQSTM1/p62, VCP, ATG4B, DCTN1 and the mTORC1 key component Raptor/RPTOR (Bose et al., 2011; Ling et al., 2015). However, the effects of this mRNA regulation on autophagy is complex; for example, although downregulation of Raptor by TDP-43 depletion causes mTORC1 inhibition and TFEB activation which signals for autophagy induction, reduced TDP-43 also causes an accumulation of autophagosomes due to impaired autophagosome-lysosome fusion, which was suggested to be due to downregulation of dynactin 1 (DCTN1) (Xia et al., 2016). TDP-43 dysfunction results in the dysregulated expression of other ALS-associated proteins which also have roles in autophagy, including FUS, progranulin (GRN) and ataxin-2 (ATXN2). Additionally, TDP-43 can mediate the activation of FOXO transcription factors during proteostatic stress and induce the expression of genes involved in protein quality control (Zhang T. et al., 2014); FOXO regulates genes relating to both proteasomes and autophagy (Mammucari et al., 2007; Zhao et al., 2008; van der Vos et al., 2012).
Regarding the role of FUS in autophagy regulation, a recent study has found that the mRNA and protein levels of key genes involved in initial steps of the autophagy pathway, including FIP200, ATG16L1 and ATG12, are significantly lowered in FUS depleted cells (Arenas et al., 2021). Moreover, previous studies have described defective autophagy in different ALS-linked FUS-disease models, suggesting additional roles for FUS in the autophagy process. Expression of mutant FUS disrupted autophagy induction in neuronal cells by interfering with early autophagosome formation in a Rab1-dependent manner (Soo et al., 2015). Intriguingly, the expression of wild-type FUS also impaired autophagosome formation and maturation in neuronal like cells (Ling et al., 2019). Thus, further studies investigating the molecular mechanisms involved in FUS-mediated inhibition of autophagy will be needed.
Other ALS/FTD-related RBPs governing the autophagy process at a post-transcriptional level include hnRNPA1, which binds to the Beclin1 mRNA and positively regulates its expression (Ji et al., 2019). However, whether these findings suggest a mechanism by which pathogenic variants in HNRNPA1 might affect autophagy initiation, requires further investigation. Conversely VCP regulates autophagy initiation by Beclin-1 protein directly by promoting its deubiquitination and regulating the assembly of the phosphatidylinositol-3-kinase (PI3K) lipid signalling complex (Hill et al., 2021).
Loss of function studies have implied that the C9orf72 protein can also act as an indirect negative regulator of autophagy; knockdown or knockout of C9orf72 has been reported to cause mTORC1 inactivation, resulting in the nuclear translocation of the transcription factor TFEB and autophagy induction in several studies (Ugolino et al., 2016; Yang et al., 2016; Liu et al., 2018; Ji et al., 2020; Wang M. et al., 2020). Indeed, two new studies have provided additional evidence for this negative regulation of mTORC1-dependent autophagy regulation through the interaction of C9orf72 protein with Rag GTPases on the lysosomal membrane, which recruit TFEB to the lysosome for phosphorylation by mTORC1 (Ji et al., 2020; Wang M. et al., 2020). Adding more complexity to the picture, a dual role in autophagy regulation has also been suggested by a study that showed that loss of C9orf72 impairs mTORC1-mediated autophagy induction while inducing autophagy flux under basal conditions (Yang et al., 2016). Likewise, a recent study showed that C9orf72 protein overexpression may enhance autophagy initiation basally but impair autophagy under conditions of stress (Cali et al., 2019).
Furthermore, C9orf72 repeat RNA binds and sequesters several RBPs that have roles in gene regulation (reviewed by McEachin et al., 2020). Of note to autophagy, a recent study linked the known C9orf72 repeat RNA and DPR-mediated disruption of nucleocytoplasmic transport (Kim and Taylor, 2017; Solomon et al., 2021) to impaired autophagy regulation by TFEB; expression of C9orf72 repeats reduced TFEB translocation to the nucleus resulting in defective autophagy and accumulation of protein aggregates in Drosophila, an effect that appeared to be predominantly driven by repeat RNA, with only a small effect from the DPR poly(GA) (Cunningham et al., 2020). Consistently, this study described nuclear TFEB depletion in the motor cortex of ALS patients, corroborating a previous study (Wang et al., 2016). Together it is clear that the C9orf72 mutation can cause multiple effects on autophagy, predominantly via haploinsufficiency of the protein but also by gain of function repeat RNA and DPR mechanisms, and further investigations will be required to fully determine their roles in FTD/ALS pathogenesis.
Dysfunctional Autophagy-Dependent RNA Homeostasis in Amyotrophic Lateral Sclerosis/Frontotemporal Dementia
Regarding autophagy-mediated RNA homeostasis in ALS/FTD, the majority of the evidence comes from studies on SGs. As mentioned previously, the contribution of autophagy to the clearance of SGs under basal conditions is low but increases significantly upon cellular stress or in disease (Ganassi et al., 2016; Mateju et al., 2017; Mediani et al., 2021). Indeed, accumulating evidence suggests that SGs are targeted for selective autophagic degradation when granules become pathological (Sun et al., 2020). Although, SGs can be part of a pro-survival mechanism, chronic SGs prevent the normal functionality of a cell. If not efficiently cleared, persistent SGs may form the seed for aggregation of RBPs (Baradaran-Heravi et al., 2020), and chronic optogenetic induction of SGs has been shown to drive neurotoxicity (Zhang et al., 2019). Thus, under disease conditions the selective autophagic degradation of SGs may play a crucial role.
Mutations are found in several ALS/FTD genes encoding proteins involved in selective autophagy. The ALS-associated autophagy receptor p62 is recruited to SGs upon cellular stress (Chitiprolu et al., 2018; Turakhiya et al., 2018); TBK1 (TANK-binding kinase 1; (Cirulli et al., 2015; Freischmidt et al., 2015)) phosphorylates p62 (Pilli et al., 2012; Matsumoto et al., 2015) and another autophagy receptor - optineurin (Matsumoto et al., 2015), which enhances affinity to cargo and promotes selective autophagy of damaged mitochondria or intracellular pathogens (Richter et al., 2016). There have also been reports that TBK1 regulates upstream components of the autophagy signalling cascade such as AMPK (Zhao Y. G. et al., 2018) or the autophagosome-localized SNARE protein Syntaxin-17 (Kumar et al., 2019). Interestingly, SMCR8, a C9orf72 protein binding partner, is also a substrate of TBK1 (Sellier et al., 2016), which may therefore have implications in pathogenesis from the range of roles of the C9orf72-WDR41-SMCR8 complex in autophagy. As explained above, C9orf72, p62 and SMN are also involved in an alternative ubiquitin-independent granulophagy dependent on symmetric arginine methylation of SG components. Indeed, C9orf72-ALS patient cerebellar tissue exhibits an accumulation of symmetrically arginine-methylated proteins (Chitiprolu et al., 2018), suggesting deficient turnover of SGs, and deregulation of autophagy is observed in cell culture and animal models of SMN-associated neurodegeneration (Garcera et al., 2013; Custer and Androphy, 2014). Although in most cases, studies have not investigated a direct link between granulophagy and ALS/FTD associated autophagy genes, disease associated mutations in VCP (Gitcho et al., 2009; Johnson et al., 2010) have been shown to induce persistent SGs which also contain TDP-43 (Buchan et al., 2013; Gwon et al., 2021) and depletion or inhibition of VCP impairs SG formation (Seguin et al., 2014). Interestingly, the C9orf72 DPR poly(GA) has been shown to sequester VCP (Božič et al., 2021), which may similarly inhibit VCP’s function in selective autophagy and/or SG dynamics. VAPB patient muscle biopsies also contain VAPB aggregates that colocalise with TDP-43 and the SG protein TIAR1 (Tripathi et al., 2021), suggesting impaired autophagy-dependent SG clearance.
Perturbed RNA granule dynamics has drawn much attention from the ALS-FTD field in recent years; pathological granules transition from a rapidly reversible liquid state to a more solid gel-like state which renders them less dynamic and persistent (Wolozin and Ivanov, 2019; Solomon et al., 2021). Investigation of the C9orf72 arginine-rich DPR interactomes found a wide number of RBPs and were enriched in those involved in membraneless organelles including SGs components and major ALS/FTD RBP proteins, such as TDP-43 and hnRNPA1 (Lee K.-H et al., 2016; Boeynaems et al., 2017; Chew et al., 2019). Consequently, these arginine-rich DPRs—both poly(GR) and poly(PR) - have been shown to alter SG composition and dynamics by disrupting multivalent interactions and promoting the formation of pathological SGs (reviewed by Solomon et al., 2021). Similarly, mutants of several ALS/FTD-related RBPs, such as FUS and TDP-43, promote the formation of poorly dynamic SGs (Dewey et al., 2011; Baron et al., 2013; Murakami et al., 2015; Patel et al., 2015; Gopal et al., 2017; Ding et al., 2021). SGs can also accumulate defective ribosomal proteins (Seguin et al., 2014) and misfolded proteins, such as mutant SOD1 (Mateju et al., 2017), which can drive them into a pathological state (Alberti and Carra, 2018). In line with their role in the clearance of pathological SGs, persistent mutant FUS-positive SGs were found to co-localize with LC3-II (Ryu et al., 2014). Pharmacological activation of autophagy can also reduce FUS-and TDP-43-related cytotoxicity, which was associated with a reduction in the number of TDP-43- or FUS-positive SGs (Wang et al., 2012; Boyd et al., 2014; Ryu et al., 2014; Marrone et al., 2019). The ALS/FTD protein ubiquilin 2 can also be recruited to SGs where its interaction with the RBP FUS is proposed to cause FUS dissociation from SGs, a process which is impaired in disease mutants (Alexander et al., 2018; Dao et al., 2018). Optineurin can also modulate SG dynamics and clearance in autophagy dependent and independent ways; in the latter, reduced optineurin or disease mutants lead to an upregulation of TIA1 expression causing reduced SG clearance and accumulation of ubiquitinated TDP-43 (Kakihana et al., 2021). Furthermore, SGs also participate in autophagy regulation by recruiting signalling molecules, such as the components of the mTORC1 complex, raptor and mTOR; this association regulates TORC1 inactivation-reactivation during cell stress and recovery (Takahara and Maeda, 2012; Yan et al., 2012; Wippich et al., 2013). Sequestration of these signalling molecules or other RBPs into poorly dynamic SGs may compromise the autophagy–lysosomal pathway indirectly.
Finally, autophagic degradation of SGs and other RNA granules also relies on proper trafficking. As noted previously, ALS-associated variants in ANXA11 have been shown to disrupt RNA granule-lysosome docking, impeding their transport in neurons (Liao et al., 2019) and ANXA11 mutants can impede calcium homeostasis and stress granule disassembly (Nahm et al., 2020).
In summary, although thus far relatively few studies have connected autophagy and RNA homeostasis directly, there is clear evidence for a significant impact of autophagy on RNA homeostasis in FTD/ALS, particularly in relation to the clearance of pathological SGs.
Discussion
Current knowledge reviewed here has established a multilayer understanding of autophagy regulation as well as novel non-canonical functions for the autophagy machinery. An emerging and less-well understood area of autophagy research involves its role in RNA metabolism. Here we discussed new evidence of specialized mechanisms for selective autophagy-mediated RNA degradation and other autophagy functions also affecting RNA homeostasis. We have also described how RBPs might not serve only as autophagic cargos but also exert regulatory functions on the autophagy process, adding additional complexity to the RNA-autophagy interplay.
This complex autophagy-RNA interplay is well evidenced in ALS/FTD, where dysregulated RNA homeostasis and autophagy defects are major interconnected disease mechanisms. We discussed here the role of ALS/FTD-associated proteins in the different stages of autophagy, disrupting general autophagy but also specific RNA-mediated autophagy, with SGs as major substrates in this process. The evidence presented here reinforce a unifying connection between many ALS/FTD genes and suggest a model in which pathogenic variants that dysregulate autophagic clearance and promote an accumulation of pathological RNA granules and drive cytoplasmic protein aggregation culminate in ALS/FTD.
The mechanisms associated to the C9orf72 mutation have been a focus of the ALS/FTD research field as major known cause of disease but also for its intriguing complex disease mechanisms. C9orf72 mutation studies nicely illustrate the relevance and complexity of the autophagy–RNA homeostasis interplay in health and disease. As reviewed above, as part of the C9orf72-WDR41-SMCR8 complex, the C9orf72 protein is involved in the initiation of autophagy and localised to the lysosome. Here, loss of C9orf72 results in inappropriate sorting of hydrolase precursors, impaired synaptic vesicle recycling, lysosomal accumulation, and alterations in glial secretomes. This latter finding has wide implications on the neuroimmune system and may contribute to neuroinflammation and non-cell autonomous neurotoxicity. It can also impact gene regulation of autophagy via its inactivation of mTORC and autophagosome maturation and trafficking via interaction with cofilin. Through these roles haploinsufficiency of the C9orf72 protein can affect RNA homeostasis by selective autophagy. However, the C9orf72 protein additionally impacts granulophagy by a direct role in a ubiquitin-independent pathway targeting arginine-methylated SG substrates for degradation.
In C9orf72-FTD/ALS, gain-of-function mechanisms emanating from the repeat are proposed to be the dominant cause of neurodegeneration (Mizielinska et al., 2014; Moens et al., 2019), and both repeat RNA and DPRs can affect the autophagy-RNA homeostasis interplay. Dysfunctional nucleocytoplasmic transport from both the repeat RNA and DPRs may impact the nuclear translocation of transcription factors that regulate autophagy genes (Kim and Taylor, 2017; Solomon et al., 2021); indeed, the master regulator TFEB is affected by expression of the C9orf72 repeat in Drosophila and cell models, an effect that appeared to be predominantly driven by repeat RNA with only a small effect from poly(GA) (Cunningham et al., 2020). The arginine rich DPRs bind to and disrupt a wide range of membraneless organelles (Boeynaems et al., 2017; Lee K.-H et al., 2016), including the nuclear pore through which all nucleocytoplasmic transport occurs, thus may contribute to the aforementioned disruption. However, as described above the arginine rich DPRs also bind SG components and perturb SG dynamics, which may result in the persistent pathological SGs that are targeted for degradation by autophagy. As noted, these effects are associated with the arginine rich DPRs which may mimic other arginine rich motifs such as the RGG motif which is common to RBPs involved in RNA homeostasis; similarly to the arginine rich DPRs, this motif permits interaction with both proteins and nucleic acids (Rajyaguru and Parker, 2012). This motif also undergoes regulation by arginine methylation—a signal already described to target cargo to SGs for autophagic degradation involving the C9orf72 protein (Chitiprolu et al., 2018). Indeed, the arginine rich DPRs also undergo arginine methylation, with different methylation types regulating its pathogenicity (Sakae et al., 2018; Gittings et al., 2020). Finally, the aggregates formed by the C9orf72 repeat RNA and DPRs can sequester proteins, for example the sequestration of VCP by poly(GA) (Božič et al., 2021), which promotes further dysfunction by these pathways. Gain-of-function DPR pathomechanisms can also be exacerbated by the coincident loss of C9orf72 protein levels in C9orf72-ALS/FTD which reduce their autophagic clearance. Indeed, complete knockout or reduction of C9orf72 exacerbates reduced survival in C9orf72 repeat expansion models in neuron-like cell lines, zebrafish and mice (Shao et al., 2019; Boivin et al., 2020; Zhu et al., 2020) and from DPR expression in control patient induced motor neurons (Shi et al., 2018). Conversely C9orf72 protein expression can ameliorate reduced lysosomal number, DPR accumulation and degeneration in C9orf72-ALS/FTD patient induced motor neurons (Shi et al., 2018).
Although studies have identified an effect of loss of C9orf72 protein function on clearance of DPRs, it has not yet been investigated whether it could also affect the degradation of C9orf72 repeat RNA, given the emerging evidence for the role of autophagy in RNA catabolism. Indeed, the mechanisms for the degradation of repeat RNA in repeat expansion disorders are still poorly understood. A recent study has revealed a role for the RNA exosome, which is generally involved in RNA quality control, in the degradation of the C9orf72 sense and antisense repeat transcripts (Kawabe et al., 2020). The RNA exosome exerts its functions in an autophagy/lysosomal-independent manner. Instead, the catalytic component EXOSC10 promotes the substrate loading into the tunnel of the barrel-like core of the RNA exosome complex for its degradation (Kilchert et al., 2016). However, additional mechanisms for C9orf72 expanded G4C2 repeat RNA are tempting to hypothesis when the RNautophagy receptors LAMP2C and SIDT2 have already shown to have binding preference for stretches of consecutive guanosines (Hase et al., 2015). Indeed, SIDT2 was found to interact with expanded CAG repeats in exon1 of the HTT transcript, which code for polyglutamine-expanded proteins, linked to Huntington’s disease. Importantly, overexpression of SIDT2 promoted degradation of HTT expanded transcripts, reducing the levels of polyglutamine-expanded huntingtin aggregates. Thus, C9orf72-derived expanded G4C2 repeat RNA could be directed to lysosomes for RNautophagy degradation in a similar manner. Although, in the case of the C9orf72 repeat RNA, hairpin and G-quadruplex structures will need to be considered (Fratta et al., 2012; Haeusler et al., 2014). It is also of note that the C9orf72 protein itself is a substrate for autophagy in a cell-type dependent manner (Leskelä et al., 2019).
In summary, both synergistic and feedback loops in autophagy and RNA homeostasis contribute to C9orf72-ALS/FTD pathogenesis, from gain and loss of function mechanisms. Loss of the C9orf72 protein may impact several stages of autophagy as well as granulophagy directly, which can exacerbate gain of function mechanisms by compromising DPR protein and potentially repeat RNA clearance. Then, the DPRs and repeat RNA can also impact autophagy and RNA homeostasis themselves, through effects on transcription factors, SG dynamics and potentially RNautophagy. Interestingly, a recent genome-wide data study has revealed that autophagy genes are significantly associated with ALS risk in C9orf72 expansion carriers, supporting a modifying role for autophagy from a genetic perspective as well (Saez-Atienzar et al., 2021). But it does not end there, as with the majority of ALS and a large proportion of FTD cases, the C9orf72 mutation is associated with pathology of the RBP TDP-43, and RBPs can also modulate autophagy. Indeed, the ALS/FTD-associated RBPs TDP-43, FUS and hnRNPA1 can all regulate the transcription of autophagy-related genes, with TDP-43 also able to modulate the transcription factor FOXO and FUS to both initiation and maturation of autophagosomes. Conversely, many ALS/FTD-linked genes can impact autophagy and RNA homeostasis via contribution to granulophagy, with evidence for the proteins encoded by SQSTM1 (p62) and VCP playing crucial roles.
Another point to consider is that multiple different cell types contribute to ALS/FTD pathologies (Ragagnin et al., 2019; Vahsen et al., 2021) and the autophagic-RNA homeostasis interplay may vary between these. Indeed, accumulation of insoluble protein, often RBP, inclusions are found in both neurons and neuroglia of ALS/FTD post-mortem tissue indicative of impaired protein clearance (Neumann et al., 2007). Of particular interest is also the role for secretory autophagy in the release of extracellular vesicles (di Malta et al., 2012; Verkhratsky et al., 2016) and excretion of nucleobases and RBPs (Shah et al., 2018; Varcianna et al., 2019; Leidal et al., 2020) from glial cells and their potential contribution to non-cell autonomous patho-mechanisms in ALS/FTD. However, as much of the research into the interplay of autophagy and RNA catabolism is very recent, further studies will be required to dissect contributions of different cell types to pathology via these pathways, which has implications for therapeutic targeting.
Indeed, an important point to consider is that these studies have opened a new avenue of research searching for therapeutic approaches for these fatal diseases. Autophagy has long been explored as a therapeutic target for ALS/FTD with contradictory results, depending on the ALS/FTD causing gene or disease-model. For instance, pharmacologically autophagy activation exacerbated neurotoxicity in Drosophila TARDBP (TBPH) knockout and SOD1G93A mice (Zhang et al., 2011; Hsueh et al., 2016; Xia et al., 2016; Zhou et al., 2017). While a neuroprotective role for autophagy induction was reported in several FUS and TARDBP disease models (Caccamo et al., 2009; Wang et al., 2012; Burkhardt et al., 2013; Barmada et al., 2014; Boyd et al., 2014; Cheng et al., 2015) or even in the same SOD-1 mouse model (SOD1G93A) (Fornai et al., 2008; Mancuso et al., 2014; Zhang T. et al., 2014). However thus far relatively little consideration has been given to the impact of targeting RBPs on autophagy, and we are not aware of any translational approaches for autophagy-dependent RNA catabolism in the ALS/FTD field as of yet.
In summary, we have reviewed the wide evidence for the contribution of ALS/FTD-related proteins and RNA to disrupt the different stages of autophagy. Through this dysfunction they have the potential to impact RNA catabolism in addition to their roles in direct RNA homeostasis deregulation. Of particular note is the interplay at the level of SG clearance (granulophagy), as alterations in SG dynamics and the contribution to disease pathology is of major interest to the field. Thus, future research may focus on more specific pharmacological interventions targeting pathological SGs for clearance via autophagy-dependent mechanisms. In addition, increasing our understanding of the molecular mechanisms of autophagy-dependent RNA homeostasis and the context in which these processes become dysfunctional in disease will be beneficial to design effective therapeutics particularly in ALS/FTD.
Author Contributions
SM, PG-S, and OH were involved in the investigation and editing of this manuscript. OH drafted the figures. SM and PG-S conceptualised and drafted the review in addition to acquiring the financial support for OH.
Funding
This work was supported by the UK Dementia Research Institute which receives its funding from DRI Ltd., funded by the UK Medical Research Council, Alzheimer’s Society and Alzheimer’s Research UK (OH, SM), a PhD Studentship from Motor Neuron Disease Association (OH, SM, PG-S; Mizielinska/Oct19/896-792), and by a Fellowship to PG-S from the UK Motor Neurone Disease Association (Gomez-Suaga/Oct17/967/799) and from MICINN (Spain) by the Juan de la Cierva—Incorporación programme (IJC 2019-039229-I).
Conflict of Interest
The authors declare that the research was conducted in the absence of any commercial or financial relationships that could be construed as a potential conflict of interest.
Publisher’s Note
All claims expressed in this article are solely those of the authors and do not necessarily represent those of their affiliated organizations, or those of the publisher, the editors and the reviewers. Any product that may be evaluated in this article, or claim that may be made by its manufacturer, is not guaranteed or endorsed by the publisher.
Acknowledgments
Figures were created with BioRender.com.
References
Abramzon, Y. A., Fratta, P., Traynor, B. J., and Chia, R. (2020). The Overlapping Genetics of Amyotrophic Lateral Sclerosis and Frontotemporal Dementia. Front. Neurosci. 14, 42. doi:10.3389/fnins.2020.00042
Aishwarya, R., Abdullah, C. S., Morshed, M., Remex, N. S., and Bhuiyan, M. S. (2021). Sigmar1's Molecular, Cellular, and Biological Functions in Regulating Cellular Pathophysiology. Front. Physiol. 12, 705575. doi:10.3389/fphys.2021.705575
Al-Sarraj, S., King, A., Troakes, C., Smith, B., Maekawa, S., Bodi, I., et al. (2011). p62 Positive, TDP-43 Negative, Neuronal Cytoplasmic and Intranuclear Inclusions in the Cerebellum and Hippocampus Define the Pathology of C9orf72-Linked FTLD and MND/ALS. Acta Neuropathol. 122 (6), 691–702. doi:10.1007/s00401-011-0911-2
Alberti, S., and Carra, S. (2018). Quality Control of Membraneless Organelles. J. Mol. Biol. 430 (23), 4711–4729. doi:10.1016/j.jmb.2018.05.013
Alexander, E. J., Ghanbari Niaki, A., Zhang, T., Sarkar, J., Liu, Y., Nirujogi, R. S., et al. (2018). Ubiquilin 2 Modulates ALS/FTD-linked FUS-RNA Complex Dynamics and Stress Granule Formation. Proc. Natl. Acad. Sci. U.S.A. 115 (49), E11485–E11494. doi:10.1073/pnas.1811997115
Amick, J., Roczniak-Ferguson, A., and Ferguson, S. M. (2016). C9orf72 Binds SMCR8, Localizes to Lysosomes, and Regulates mTORC1 Signaling. Mol. Biol. Cell 27 (20), 3040–3051. doi:10.1091/mbc.E16-01-0003
Amick, J., Tharkeshwar, A. K., Amaya, C., and Ferguson, S. M. (2018). WDR41 Supports Lysosomal Response to Changes in Amino Acid Availability. Mol. Biol. Cell 29 (18), 2213–2227. doi:10.1091/mbc.E17-12-0703
Amick, J., Tharkeshwar, A. K., Talaia, G., and Ferguson, S. M. (2020). PQLC2 Recruits the C9orf72 Complex to Lysosomes in Response to Cationic Amino Acid Starvation. J. Cell Biol. 219 (1), e201906076. doi:10.1083/jcb.201906076
An, H., and Harper, J. W. (2020). Ribosome Abundance Control via the Ubiquitin-Proteasome System and Autophagy. J. Mol. Biol. 432 (1), 170–184. doi:10.1016/j.jmb.2019.06.001
An, H., and Harper, J. W. (2018). Systematic Analysis of Ribophagy in Human Cells Reveals Bystander Flux during Selective Autophagy. Nat. Cell Biol. 20 (2), 135–143. doi:10.1038/s41556-017-0007-x
An, H., Ordureau, A., Körner, M., Paulo, J. A., and Harper, J. W. (2020). Systematic Quantitative Analysis of Ribosome Inventory during Nutrient Stress. Nature 583 (7815), 303–309. doi:10.1038/s41586-020-2446-y
Anagnostou, G., Akbar, M. T., Paul, P., Angelinetta, C., Steiner, T. J., and de Belleroche, J. (2010). Vesicle Associated Membrane Protein B (VAPB) is Decreased in ALS Spinal Cord. Neurobiol. Aging 31 (6), 969–985. doi:10.1016/j.neurobiolaging.2008.07.005
Aoki, Y., Manzano, R., Lee, Y., Dafinca, R., Aoki, M., Douglas, A. G. L., et al. (2017). C9orf72 and RAB7L1 Regulate Vesicle Trafficking in Amyotrophic Lateral Sclerosis and Frontotemporal Dementia. Brain: A J. Neurol. 140 (4), 887–897. doi:10.1093/brain/awx024
Arenas, A., Kuang, L., Zhang, J., Kingren, M. S., and Zhu, H. (2021). FUS Regulates Autophagy by Mediating the Transcription of Genes Critical to the Autophagosome Formation. J. Neurochem. 157 (3), 752–763. doi:10.1111/jnc.15281
Atkinson, R. A. K., Fernandez-Martos, C. M., Atkin, J. D., Vickers, J. C., and King, A. E. (2015). C9ORF72 Expression and Cellular Localization over Mouse Development. Acta Neuropathol. Commun. 3, 59. doi:10.1186/s40478-015-0238-7
Baker, M., Mackenzie, I. R., Pickering-Brown, S. M., Gass, J., Rademakers, R., Lindholm, C., et al. (2006). Mutations in Progranulin Cause Tau-Negative Frontotemporal Dementia Linked to Chromosome 17. Nature 442 (7105), 916–919. doi:10.1038/nature05016
Balendra, R., and Isaacs, A. M. (2018). C9orf72-mediated ALS and FTD: Multiple Pathways to Disease. Nat. Rev. Neurol. 14 (9), 544–558. doi:10.1038/s41582-018-0047-2
Baradaran-Heravi, Y., van Broeckhoven, C., and van der Zee, J. (2020). Stress Granule Mediated Protein Aggregation and Underlying Gene Defects in the FTD-ALS Spectrum. Neurobiol. Dis. 134, 104639. doi:10.1016/j.nbd.2019.104639
Barmada, S. J., Serio, A., Arjun, A., Bilican, B., Daub, A., Ando, D. M., et al. (2014). Autophagy Induction Enhances TDP43 Turnover and Survival in Neuronal ALS Models. Nat. Chem. Biol. 10 (8), 677–685. doi:10.1038/nchembio.1563
Baron, D. M., Kaushansky, L. J., Ward, C. L., Sama, R. R. K., Chian, R.-J., Boggio, K. J., et al. (2013). Amyotrophic Lateral Sclerosis-Linked FUS/TLS Alters Stress Granule Assembly and Dynamics. Mol. Neurodegeneration 8, 30. doi:10.1186/1750-1326-8-30
Beese, C. J., Brynjólfsdóttir, S. H., and Frankel, L. B. (2020). Selective Autophagy of the Protein Homeostasis Machinery: Ribophagy, Proteaphagy and ER-Phagy. Front. Cell Develop. Biol. 7, 373. doi:10.3389/fcell.2019.00373
Belgrad, J., de Pace, R., and Fields, R. D. (2020). Autophagy in Myelinating Glia. J. Neurosci. : Official J. Soc. Neurosci. 40 (2), 256–266. doi:10.1523/JNEUROSCI.1066-19.2019
Bharadwaj, R., Cunningham, K. M., Zhang, K., and Lloyd, T. E. (2016). FIG4 Regulates Lysosome Membrane Homeostasis Independent of Phosphatase Function. Hum. Mol. Genet. 25 (4), 681–692. doi:10.1093/hmg/ddv505
Blauw, H. M., Barnes, C. P., van Vught, P. W. J., van Rheenen, W., Verheul, M., Cuppen, E., et al. (2012). SMN1 Gene Duplications Are Associated with Sporadic ALS. Neurology 78 (11), 776–780. doi:10.1212/WNL.0b013e318249f697
Boeynaems, S., Bogaert, E., Kovacs, D., Konijnenberg, A., Timmerman, E., Volkov, A., et al. (2017). Phase Separation of C9orf72 Dipeptide Repeats Perturbs Stress Granule Dynamics. Mol. Cell 65 (6), 1044–1055.e5. doi:10.1016/j.molcel.2017.02.013
Boivin, M., Pfister, V., Gaucherot, A., Ruffenach, F., Negroni, L., Sellier, C., et al. (2020). Reduced Autophagy upon C9ORF72 Loss Synergizes with Dipeptide Repeat Protein Toxicity in G4C2 Repeat Expansion Disorders. EMBO J. 39 (4), e100574. doi:10.15252/embj.2018100574
Bose, J. K., Huang, C.-C., and Shen, C.-K. J. (2011). Regulation of Autophagy by Neuropathological Protein TDP-43. J. Biol. Chem. 286 (52), 44441–44448. doi:10.1074/jbc.M111.237115
Boyd, J. D., Lee, P., Feiler, M. S., Zauur, N., Liu, M., Concannon, J., et al. (2014). A High-Content Screen Identifies Novel Compounds that Inhibit Stress-Induced TDP-43 Cellular Aggregation and Associated Cytotoxicity. J. Biomol. Screen. 19 (1), 44–56. doi:10.1177/1087057113501553
Božič, J., Motaln, H., Janež, A. P., Markič, L., Tripathi, P., Yamoah, A., et al. (2021). Interactome Screening of C9orf72 Dipeptide Repeats Reveals VCP Sequestration and Functional Impairment by polyGA. Brain. 145, 684–699. doi:10.1093/brain/awab300
Brettschneider, J., Toledo, J. B., van Deerlin, V. M., Elman, L., McCluskey, L., Lee, V. M.-Y., et al. (2012). Microglial Activation Correlates with Disease Progression and Upper Motor Neuron Clinical Symptoms in Amyotrophic Lateral Sclerosis. PLoS One 7 (6), e39216. doi:10.1371/journal.pone.0039216
Buchan, J. R., Kolaitis, R.-M., Taylor, J. P., and Parker, R. (2013). Eukaryotic Stress Granules Are Cleared by Autophagy and Cdc48/VCP Function. Cell 153 (7), 1461–1474. doi:10.1016/j.cell.2013.05.037
Burd, C., and Cullen, P. J. (2014). Retromer: a Master Conductor of Endosome Sorting. Cold Spring Harb. Perspect. Biol. 6 (2), a016774. doi:10.1101/cshperspect.a016774
Burkhardt, M. F., Martinez, F. J., Wright, S., Ramos, C., Volfson, D., Mason, M., et al. (2013). A Cellular Model for Sporadic ALS Using Patient-Derived Induced Pluripotent Stem Cells. Mol. Cell Neurosci. 56, 355–364. doi:10.1016/j.mcn.2013.07.007
Caccamo, A., Majumder, S., Deng, J. J., Bai, Y., Thornton, F. B., and Oddo, S. (2009). Rapamycin Rescues TDP-43 Mislocalization and the Associated Low Molecular Mass Neurofilament Instability. J. Biol. Chem. 284 (40), 27416–27424. doi:10.1074/jbc.M109.031278
Cali, C. P., Patino, M., Tai, Y. K., Ho, W. Y., McLean, C. A., Morris, C. M., et al. (2019). C9orf72 Intermediate Repeats Are Associated with Corticobasal Degeneration, Increased C9orf72 Expression and Disruption of Autophagy. Acta Neuropathol. 138 (5), 795–811. doi:10.1007/s00401-019-02045-5
Cannon, A., Fujioka, S., Rutherford, N. J., Ferman, T. J., Broderick, D. F., Boylan, K. B., et al. (2013). Clinicopathologic Variability of the GRN A9D Mutation, Including Amyotrophic Lateral Sclerosis. Neurology 80 (19), 1771 LP–1777. doi:10.1212/WNL.0b013e3182919059
Castello, A., Fischer, B., Eichelbaum, K., Horos, R., Beckmann, B. M., Strein, C., et al. (2012). Insights into RNA Biology from an Atlas of Mammalian mRNA-Binding Proteins. Cell 149 (6), 1393–1406. doi:10.1016/j.cell.2012.04.031
Casterton, R. L., Hunt, R. J., and Fanto, M. (2020). Pathomechanism Heterogeneity in the Amyotrophic Lateral Sclerosis and Frontotemporal Dementia Disease Spectrum: Providing Focus Through the Lens of Autophagy. J. Mol. Biol. 432 (8), 2692–2713. doi:10.1016/j.jmb.2020.02.018
Chang, J., Lee, S., and Blackstone, C. (2014). Spastic Paraplegia Proteins Spastizin and Spatacsin Mediate Autophagic Lysosome Reformation. J. Clin. Invest. 124 (12), 5249–5262. doi:10.1172/JCI77598
Chang, M. C., Srinivasan, K., Friedman, B. A., Suto, E., Modrusan, Z., Lee, W. P., et al. (2017). Progranulin Deficiency Causes Impairment of Autophagy and TDP-43 Accumulation. J. Exp. Med. 214 (9), 2611–2628. doi:10.1084/jem.20160999
Chen, Y., Zheng, Z.-Z., Huang, R., Chen, K., Song, W., Zhao, B., et al. (2013). PFN1 Mutations Are Rare in Han Chinese Populations with Amyotrophic Lateral Sclerosis. Neurobiol. Aging 34 (7), 1922.e1–5. doi:10.1016/j.neurobiolaging.2013.01.013
Cheng, C.-W., Lin, M.-J., and Shen, C.-K. J. (2015). Rapamycin Alleviates Pathogenesis of a New Drosophila Model of ALS-TDP. J. Neurogenet. 29 (2–3), 59–68. doi:10.3109/01677063.2015.1077832
Chew, J., Cook, C., Gendron, T. F., Jansen-West, K., del Rosso, G., Daughrity, L. M., et al. (2019). Aberrant Deposition of Stress Granule-Resident Proteins Linked to C9orf72-Associated TDP-43 Proteinopathy. Mol. Neurodegeneration 14 (1), 9. doi:10.1186/s13024-019-0310-z
Chitiprolu, M., Jagow, C., Tremblay, V., Bondy-Chorney, E., Paris, G., Savard, A., et al. (2018). A Complex of C9ORF72 and P62 Uses Arginine Methylation to Eliminate Stress Granules by Autophagy. Nat. Commun. 9 (1), 2794. doi:10.1038/s41467-018-05273-7
Chitramuthu, B. P., Bennett, H. P. J., and Bateman, A. (2017). Progranulin: a New Avenue towards the Understanding and Treatment of Neurodegenerative Disease. Brain 140 (12), 3081–3104. doi:10.1093/brain/awx198
Chow, C. Y., Landers, J. E., Bergren, S. K., Sapp, P. C., Grant, A. E., Jones, J. M., et al. (2009). Deleterious Variants of FIG4, a Phosphoinositide Phosphatase, in Patients with ALS. Am. J. Hum. Genet. 84 (1), 85–88. doi:10.1016/j.ajhg.2008.12.010
Christ, M. G., Clement, A. M., and Behl, C. (2020). The Sigma-1 Receptor at the Crossroad of Proteostasis, Neurodegeneration, and Autophagy. Trends Neurosciences 43 (2), 79–81. doi:10.1016/j.tins.2019.12.002
Christ, M. G., Huesmann, H., Nagel, H., Kern, A., and Behl, C. (2019). Sigma-1 Receptor Activation Induces Autophagy and Increases Proteostasis Capacity In Vitro and In Vivo. Cells 8 (3), 211. doi:10.3390/cells8030211
Cioni, J.-M., Lin, J. Q., Holtermann, A. v., Koppers, M., Jakobs, M. A. H., Azizi, A., et al. (2019). Late Endosomes Act as mRNA Translation Platforms and Sustain Mitochondria in Axons. Cell 176 (1–2), 56–72.e15. doi:10.1016/j.cell.2018.11.030
Cirulli, E. T., Lasseigne, B. N., Petrovski, S., Sapp, P. C., Dion, P. A., Leblond, C. S., et al. (2015). Exome Sequencing in Amyotrophic Lateral Sclerosis Identifies Risk Genes and Pathways. Science 347 (6229), 1436–1441. doi:10.1126/science.aaa3650
Clayton, E. L., Milioto, C., Muralidharan, B., Norona, F. E., Edgar, J. R., Soriano, A., et al. (2018). Frontotemporal Dementia Causative CHMP2B Impairs Neuronal Endolysosomal Traffic-rescue by TMEM106B Knockdown. Brain: A J. Neurol. 141 (12), 3428–3442. doi:10.1093/brain/awy284
Clayton, E. L., Mizielinska, S., Edgar, J. R., Nielsen, T. T., Marshall, S., Norona, F. E., et al. (2015). Frontotemporal Dementia Caused by CHMP2B Mutation Is Characterised by Neuronal Lysosomal Storage Pathology. Acta Neuropathol. 130 (4), 511–523. doi:10.1007/s00401-015-1475-3
Colombo, E., and Farina, C. (2016). Astrocytes: Key Regulators of Neuroinflammation. Trends Immunol. 37 (9), 608–620. doi:10.1016/j.it.2016.06.006
Conway, O., Akpinar, H. A., Rogov, V. v., and Kirkin, V. (2020). Selective Autophagy Receptors in Neuronal Health and Disease. J. Mol. Biol. 432 (8), 2483–2509. doi:10.1016/j.jmb.2019.10.013
Cooper-Knock, J., Hewitt, C., Highley, J. R., Brockington, A., Milano, A., Man, S., et al. (2012). Clinico-Pathological Features in Amyotrophic Lateral Sclerosis with Expansions in C9ORF72. Brain A J. Neurol. 135 (Pt 3), 751–764. doi:10.1093/brain/awr365
Corbier, C., and Sellier, C. (2017). C9ORF72 is a GDP/GTP Exchange Factor for Rab8 and Rab39 and Regulates Autophagy. Small GTPases 8 (3), 181–186. doi:10.1080/21541248.2016.1212688
Corrionero, A., and Horvitz, H. R. (2018). A C9orf72 ALS/FTD Ortholog Acts in Endolysosomal Degradation and Lysosomal Homeostasis. Curr. Biol. 28 (10), 1522–1535.e5. doi:10.1016/j.cub.2018.03.063
Cox, L. E., Ferraiuolo, L., Goodall, E. F., Heath, P. R., Higginbottom, A., Mortiboys, H., et al. (2010). Mutations in CHMP2B in Lower Motor Neuron Predominant Amyotrophic Lateral Sclerosis (ALS). PLoS One 5 (3), e9872. doi:10.1371/journal.pone.0009872
Cruts, M., Gijselinck, I., van der Zee, J., Engelborghs, S., Wils, H., Pirici, D., et al. (2006). Null Mutations in Progranulin Cause Ubiquitin-Positive Frontotemporal Dementia Linked to Chromosome 17q21. Nature 442 (7105), 920–924. doi:10.1038/nature05017
Cunningham, K. M., Maulding, K., Ruan, K., Senturk, M., Grima, J. C., Sung, H., et al. (2020). TFEB/Mitf Links Impaired Nuclear Import to Autophagolysosomal Dysfunction in C9-ALS. ELife 9, e59419. doi:10.7554/eLife.59419
Custer, S. K., and Androphy, E. J. (2014). Autophagy Dysregulation in Cell Culture and Animals Models of Spinal Muscular Atrophy. Mol. Cell Neurosciences 61, 133–140. doi:10.1016/j.mcn.2014.06.006
Dao, T. P., Kolaitis, R.-M., Kim, H. J., O’Donovan, K., Martyniak, B., Colicino, E., et al. (2018). Ubiquitin Modulates Liquid-Liquid Phase Separation of UBQLN2 via Disruption of Multivalent Interactions. Mol. Cell 69 (6), 965–978.e6. doi:10.1016/j.molcel.2018.02.004
Darnell, J. E. (1968). Ribonucleic Acids from Animal Cells. Bacteriol. Rev. 32 (3), 262–290. doi:10.1128/br.32.3.262-290.1968
de Vos, K. J., and Hafezparast, M. (2017). Neurobiology of Axonal Transport Defects in Motor Neuron Diseases: Opportunities for Translational Research? Neurobiol. Dis. 105, 283–299. doi:10.1016/j.nbd.2017.02.004
de Vos, K. J., Mórotz, G. M., Stoica, R., Tudor, E. L., Lau, K.-F., Ackerley, S., et al. (2012). VAPB Interacts with the Mitochondrial Protein PTPIP51 to Regulate Calcium Homeostasis. Hum. Mol. Genet. 21 (6), 1299–1311. doi:10.1093/hmg/ddr559
DeJesus-Hernandez, M., Mackenzie, I. R., Boeve, B. F., Boxer, A. L., Baker, M., Rutherford, N. J., et al. (2011). Expanded GGGGCC Hexanucleotide Repeat in Noncoding Region of C9ORF72 Causes Chromosome 9p-Linked FTD and ALS. Neuron 72 (2), 245–256. doi:10.1016/j.neuron.2011.09.011
Deng, H.-X., Chen, W., Hong, S.-T., Boycott, K. M., Gorrie, G. H., Siddique, N., et al. (2011). Mutations in UBQLN2 Cause Dominant X-Linked Juvenile and Adult-Onset ALS and ALS/dementia. Nature 477 (7363), 211–215. doi:10.1038/nature10353
Deng, Z., Lim, J., Wang, Q., Purtell, K., Wu, S., Palomo, G. M., et al. (2020). ALS-FTLD-Linked Mutations of SQSTM1/p62 Disrupt Selective Autophagy and NFE2L2/NRF2 Anti-Oxidative Stress Pathway. Autophagy 16, 917–931. doi:10.1080/15548627.2019.1644076
Dewey, C. M., Cenik, B., Sephton, C. F., Dries, D. R., Mayer, P., Good, S. K., et al. (2011). TDP-43 Is Directed to Stress Granules by Sorbitol, a Novel Physiological Osmotic and Oxidative Stressor. Mol. Cell Biol. 31 (5), 1098–1108. doi:10.1128/MCB.01279-10
di Malta, C., Cinque, L., and Settembre, C. (2019). Transcriptional Regulation of Autophagy: Mechanisms and Diseases. Front. Cell Dev. Biol. 7, 114. doi:10.3389/fcell.2019.00114
di Malta, C., Fryer, J. D., Settembre, C., and Ballabio, A. (2012). Astrocyte Dysfunction Triggers Neurodegeneration in a Lysosomal Storage Disorder. Proc. Natl. Acad. Sci. U.S.A. 109 (35), E2334–E2342. doi:10.1073/pnas.1209577109
Ding, X., Xiang, Z., Qin, C., Chen, Y., Tian, H., Meng, L., et al. (2021). Spreading of TDP-43 Pathology via Pyramidal Tract Induces ALS-like Phenotypes in TDP-43 Transgenic Mice. Acta Neuropathol. Commun. 9 (1), 15. doi:10.1186/s40478-020-01112-3
Elia, L. P., Mason, A. R., Alijagic, A., and Finkbeiner, S. (2019). Genetic Regulation of Neuronal Progranulin Reveals a Critical Role for the Autophagy-Lysosome Pathway. J. Neurosci. 39 (17), 3332 LP–3344. doi:10.1523/JNEUROSCI.3498-17.2019
Eskelinen, E.-L., Reggiori, F., Baba, M., Kovács, A. L., and Seglen, P. O. (2011). Seeing Is Believing: the Impact of Electron Microscopy on Autophagy Research. Autophagy 7 (9), 935–956. doi:10.4161/auto.7.9.15760
Farg, M. A., Sundaramoorthy, V., Sultana, J. M., Yang, S., Atkinson, R. A. K., Levina, V., et al. (2014). C9ORF72, Implicated in Amytrophic Lateral Sclerosis and Frontotemporal Dementia, Regulates Endosomal Trafficking. Hum. Mol. Genet. 23 (13), 3579–3595. doi:10.1093/hmg/ddu068
Farías, G. G., Guardia, C. M., de Pace, R., Britt, D. J., and Bonifacino, J. S. (2017). BORC/kinesin-1 Ensemble Drives Polarized Transport of Lysosomes into the Axon. Proc. Natl. Acad. Sci. 114 (14), E2955 LP–E2964. doi:10.1073/pnas.1616363114
Fecto, F., and Siddique, T. (2011). Making Connections: Pathology and Genetics Link Amyotrophic Lateral Sclerosis with Frontotemporal Lobe Dementia. J. Mol. Neurosci. : MN 45 (3), 663–675. doi:10.1007/s12031-011-9637-9
Fecto, F., Yan, J., Vemula, S. P., Liu, E., Yang, Y., Chen, W., et al. (2011). SQSTM1 Mutations in Familial and Sporadic Amyotrophic Lateral Sclerosis. Arch. Neurol. 68 (11), 1440–1446. doi:10.1001/archneurol.2011.250
Ferguson, C. J., Lenk, G. M., and Meisler, M. H. (2009). Defective Autophagy in Neurons and Astrocytes from Mice Deficient in PI(3,5)P2. Hum. Mol. Genet. 18 (24), 4868–4878. doi:10.1093/hmg/ddp460
Fleming, A., Bourdenx, M., Fujimaki, M., Karabiyik, C., Krause, G. J., Lopez, A., et al. (2022). The Different Autophagy Degradation Pathways and Neurodegeneration. Neuron 110 (6), 935–966. doi:10.1016/j.neuron.2022.01.017
Flower, T. G., Takahashi, Y., Hudait, A., Rose, K., Tjahjono, N., Pak, A. J., et al. (2020). A Helical Assembly of Human ESCRT-I Scaffolds Reverse-Topology Membrane Scission. Nat. Struct. Mol. Biol. 27 (6), 570–580. doi:10.1038/s41594-020-0426-4
Fornai, F., Longone, P., Cafaro, L., Kastsiuchenka, O., Ferrucci, M., Manca, M. L., et al. (2008). Lithium Delays Progression of Amyotrophic Lateral Sclerosis. Proc. Natl. Acad. Sci. U.S.A. 105 (6), 2052–2057. doi:10.1073/pnas.0708022105
Fratta, P., Mizielinska, S., Nicoll, A. J., Zloh, M., Fisher, E. M. C., Parkinson, G., et al. (2012). C9orf72 Hexanucleotide Repeat Associated with Amyotrophic Lateral Sclerosis and Frontotemporal Dementia Forms RNA G-Quadruplexes. Sci. Rep. 2, 1016. doi:10.1038/srep01016
Freischmidt, A., Wieland, T., Richter, B., Ruf, W., Schaeffer, V., Müller, K., et al. (2015). Haploinsufficiency of TBK1 Causes Familial ALS and Fronto-Temporal Dementia. Nat. Neurosci. 18 (5), 631–636. doi:10.1038/nn.4000
Fujita, N., Itoh, T., Omori, H., Fukuda, M., Noda, T., and Yoshimori, T. (2008). The Atg16L Complex Specifies the Site of LC3 Lipidation for Membrane Biogenesis in Autophagy. Mol. Biol. Cell 19 (5), 2092–2100. doi:10.1091/mbc.e07-12-1257
Fujiwara, Y., Furuta, A., Kikuchi, H., Aizawa, S., Hatanaka, Y., Konya, C., et al. (2013). Discovery of a Novel Type of Autophagy Targeting RNA. Autophagy 9 (3), 403–409. doi:10.4161/auto.23002
Fujiwara, Y., Hase, K., Wada, K., and Kabuta, T. (2015). An RNautophagy/DNautophagy Receptor, LAMP2C, Possesses an Arginine-Rich Motif that Mediates RNA/DNA-binding. Biochem. Biophys. Res. Commun. 460 (2), 281–286. doi:10.1016/j.bbrc.2015.03.025
Fumagalli, L., Young, F. L., Boeynaems, S., De Decker, M., Mehta, A. R., Swijsen, A., et al. (2021). C9orf72-Derived Arginine-Containing Dipeptide Repeats Associate With Axonal Transport Machinery and Impede Microtubule-Based Motility. Sci. Adv. 7 (15), eabg3013. doi:10.1126/sciadv.abg3013
Ganassi, M., Mateju, D., Bigi, I., Mediani, L., Poser, I., Lee, H. O., et al. (2016). A Surveillance Function of the HSPB8-BAG3-HSP70 Chaperone Complex Ensures Stress Granule Integrity and Dynamism. Mol. Cell 63 (5), 796–810. doi:10.1016/j.molcel.2016.07.021
Garcera, A., Bahi, N., Periyakaruppiah, A., Arumugam, S., and Soler, R. M. (2013). Survival Motor Neuron Protein Reduction Deregulates Autophagy in Spinal Cord Motoneurons In Vitro. Cell Death Dis. 4 (6), e686. doi:10.1038/cddis.2013.209
Gershoni-Emek, N., Altman, T., Ionescu, A., Costa, C. J., Gradus-Pery, T., Willis, D. E., et al. (2018). Localization of RNAi Machinery to Axonal Branch Points and Growth Cones Is Facilitated by Mitochondria and Is Disrupted in ALS. Front. Mol. Neurosci. 11, 311. doi:10.3389/fnmol.2018.00311
Gitcho, M. A., Strider, J., Carter, D., Taylor-Reinwald, L., Forman, M. S., Goate, A. M., et al. (2009). VCP Mutations Causing Frontotemporal Lobar Degeneration Disrupt Localization of TDP-43 and Induce Cell Death. J. Biol. Chem. 284 (18), 12384–12398. doi:10.1074/jbc.M900992200
Gittings, L. M., Boeynaems, S., Lightwood, D., Clargo, A., Topia, S., Nakayama, L., et al. (2020). Symmetric Dimethylation of Poly-GR Correlates with Disease Duration in C9orf72 FTLD and ALS and Reduces Poly-GR Phase Separation and Toxicity. Acta Neuropathologica 139 (2), 407–410. doi:10.1007/s00401-019-02104-x
Gómez-Sánchez, R., Tooze, S. A., and Reggiori, F. (2021). Membrane Supply and Remodeling During Autophagosome Biogenesis. Curr. Opin. Cell Biol. 71, 112–119. doi:10.1016/j.ceb.2021.02.001
Gomez-Suaga, P., Paillusson, S., Stoica, R., Noble, W., Hanger, D. P., and Miller, C. C. J. (2017). The ER-Mitochondria Tethering Complex VAPB-PTPIP51 Regulates Autophagy. Curr. Biol. 27 (3), 371–385. doi:10.1016/j.cub.2016.12.038
Gopal, P. P., Nirschl, J. J., Klinman, E., and Holzbaur, E. L. F. (2017). Amyotrophic Lateral Sclerosis-Linked Mutations Increase the Viscosity of Liquid-like TDP-43 RNP Granules in Neurons. Proc. Natl. Acad. Sci. U.S.A 114 (12), E2466–E2475. doi:10.1073/pnas.1614462114
Gregianin, E., Pallafacchina, G., Zanin, S., Crippa, V., Rusmini, P., Poletti, A., et al. (2016). Loss-of-function Mutations in the SIGMAR1 Gene Cause Distal Hereditary Motor Neuropathy by Impairing ER-Mitochondria Tethering and Ca2+ Signalling. Hum. Mol. Genet. 25 (17), 3741–3753. doi:10.1093/hmg/ddw220
Guo, H., Chitiprolu, M., Gagnon, D., Meng, L., Perez-Iratxeta, C., Lagace, D., et al. (2014). Autophagy Supports Genomic Stability by Degrading Retrotransposon RNA. Nat. Commun. 5 (1), 5276. doi:10.1038/ncomms6276
Gwon, Y., Maxwell, B. A., Kolaitis, R.-M., Zhang, P., Kim, H. J., and Taylor, J. P. (2021). Ubiquitination of G3BP1 Mediates Stress Granule Disassembly in a Context-Specific Manner. Science 372 (6549), eabf6548. doi:10.1126/science.abf6548
Hadano, S., Hand, C. K., Osuga, H., Yanagisawa, Y., Otomo, A., Devon, R. S., et al. (2001). A Gene Encoding a Putative GTPase Regulator is Mutated in Familial Amyotrophic Lateral Sclerosis 2. Nat. Genet. 29 (2), 166–173. doi:10.1038/ng1001-166
Hadano, S., Otomo, A., Kunita, R., Suzuki-Utsunomiya, K., Akatsuka, A., Koike, M., et al. (2010). Loss of ALS2/Alsin Exacerbates Motor Dysfunction in a SOD1-Expressing Mouse ALS Model by Disturbing Endolysosomal Trafficking. PLoS One 5 (3), e9805. doi:10.1371/journal.pone.0009805
Haeusler, A. R., Donnelly, C. J., Periz, G., Simko, E. A. J., Shaw, P. G., Kim, M.-S., et al. (2014). C9orf72 Nucleotide Repeat Structures Initiate Molecular Cascades of Disease. Nature 507 (7491), 195–200. doi:10.1038/nature13124
Haidet-Phillips, A. M., Hester, M. E., Miranda, C. J., Meyer, K., Braun, L., Frakes, A., et al. (2011). Astrocytes from Familial and Sporadic ALS Patients Are Toxic to Motor Neurons. Nat. Biotechnol. 29 (9), 824–828. doi:10.1038/nbt.1957
Hamilton, N., Rutherford, H. A., Petts, J. J., Isles, H. M., Weber, T., Henneke, M., et al. (2020). The Failure of Microglia to Digest Developmental Apoptotic Cells Contributes to the Pathology of RNASET2-Deficient Leukoencephalopathy. Glia 68 (7), 1531–1545. doi:10.1002/glia.23829
Hase, K., Contu, V. R., Kabuta, C., Sakai, R., Takahashi, M., Kataoka, N., et al. (2020). Cytosolic Domain of SIDT2 Carries an Arginine-Rich Motif that Binds to RNA/DNA and Is Important for the Direct Transport of Nucleic Acids into Lysosomes. Autophagy 16 (11), 1974–1988. doi:10.1080/15548627.2020.1712109
Hase, K., Fujiwara, Y., Kikuchi, H., Aizawa, S., Hakuno, F., Takahashi, S.-I., et al. (2015). RNautophagy/DNautophagy Possesses Selectivity for RNA/DNA Substrates. Nucleic Acids Res. 43 (13), 6439–6449. doi:10.1093/nar/gkv579
Haud, N., Kara, F., Diekmann, S., Henneke, M., Willer, J. R., Hillwig, M. S., et al. (2011). rnaset2 Mutant Zebrafish Model Familial Cystic Leukoencephalopathy and Reveal a Role for RNase T2 in Degrading Ribosomal RNA. Proc. Natl. Acad. Sci. U.S.A. 108 (3), 1099–1103. doi:10.1073/pnas.1009811107
Hill, S. M., Wrobel, L., Ashkenazi, A., Fernandez-Estevez, M., Tan, K., Bürli, R. W., et al. (2021). VCP/p97 Regulates Beclin-1-dependent Autophagy Initiation. Nat. Chem. Biol. 17 (4), 448–455. doi:10.1038/s41589-020-00726-x
Ho, C. Y., Alghamdi, T. A., and Botelho, R. J. (2012). Phosphatidylinositol-3,5-bisphosphate: No Longer the Poor PIP2. Traffic 13 (1), 1–8. doi:10.1111/j.1600-0854.2011.01246.x
Ho, W. Y., Tai, Y. K., Chang, J.-C., Liang, J., Tyan, S.-H., Chen, S., et al. (2019). The ALS-FTD-Linked Gene Product, C9orf72, Regulates Neuronal Morphogenesis via Autophagy. Autophagy 15 (5), 827–842. doi:10.1080/15548627.2019.1569441
Holm, I. E., Englund, E., Mackenzie, I. R. A., Johannsen, P., and Isaacs, A. M. (2007). A Reassessment of the Neuropathology of Frontotemporal Dementia Linked to Chromosome 3. J. Neuropathol. Exp. Neurol. 66 (10), 884–891. doi:10.1097/nen.0b013e3181567f02
Houseley, J., and Tollervey, D. (2009). The many Pathways of RNA Degradation. Cell 136 (4), 763–776. doi:10.1016/j.cell.2009.01.019
Houtman, J., Freitag, K., Gimber, N., Schmoranzer, J., Heppner, F. L., and Jendrach, M. (2019). Beclin1-driven Autophagy Modulates the Inflammatory Response of Microglia via NLRP3. EMBO J. 38 (4). doi:10.15252/embj.201899430
Hsueh, K.-W., Chiou, T.-W., Chiang, S.-F., Yamashita, T., Abe, K., Borlongan, C. v., et al. (2016). Autophagic Down-Regulation in Motor Neurons Remarkably Prolongs the Survival of ALS Mice. Neuropharmacology 108, 152–160. doi:10.1016/j.neuropharm.2016.03.035
Inada, T. (2020). Quality Controls Induced by Aberrant Translation. Nucleic Acids Res. 48 (3), 1084–1096. doi:10.1093/nar/gkz1201
Ingre, C., Landers, J. E., Rizik, N., Volk, A. E., Akimoto, C., Birve, A., et al. (2013). A Novel Phosphorylation Site Mutation in Profilin 1 Revealed in a Large Screen of US, Nordic, and German Amyotrophic Lateral Sclerosis/frontotemporal Dementia Cohorts. Neurobiol. Aging 34 (6), 1708.e1–6. doi:10.1016/j.neurobiolaging.2012.10.009
Iyer, S., Subramanian, V., and Acharya, K. R. (2018). C9orf72, a Protein Associated with Amyotrophic Lateral Sclerosis (ALS) Is a Guanine Nucleotide Exchange Factor. PeerJ 6, e5815. doi:10.7717/peerj.5815
Ji, E., Lee, H., Ahn, S., Jung, M., Lee, S. H., Lee, J.-H., et al. (2019). Heterogeneous Nuclear Ribonucleoprotein A1 Promotes the Expression of Autophagy-Related Protein 6 in Human Colorectal Cancer. Biochem. Biophys. Res. Commun. 513 (1), 255–260. doi:10.1016/j.bbrc.2019.03.179
Ji, Y. J., Ugolino, J., Zhang, T., Lu, J., Kim, D., and Wang, J. (2020). C9orf72/ALFA-1 Controls TFEB/HLH-30-dependent Metabolism through Dynamic Regulation of Rag GTPases. PLoS Genet. 16 (4), e1008738. doi:10.1371/journal.pgen.1008738
Jia, J., Abudu, Y. P., Claude-Taupin, A., Gu, Y., Kumar, S., Choi, S. W., et al. (2018). Galectins Control mTOR in Response to Endomembrane Damage. Mol. Cell 70 (1), 120–135.e8. doi:10.1016/j.molcel.2018.03.009
Johnson, J. O., Mandrioli, J., Benatar, M., Abramzon, Y., van Deerlin, V. M., Trojanowski, J. Q., et al. (2010). Exome Sequencing Reveals VCP Mutations as a Cause of Familial ALS. Neuron 68 (5), 857–864. doi:10.1016/j.neuron.2010.11.036
Kakihana, T., Takahashi, M., Katsuragi, Y., Yamashita, S.-I., Sango, J., Kanki, T., et al. (2021). The optineurin/TIA1 Pathway Inhibits Aberrant Stress Granule Formation and Reduces Ubiquitinated TDP-43. IScience 24 (7), 102733. doi:10.1016/j.isci.2021.102733
Kao, A. W., McKay, A., Singh, P. P., Brunet, A., and Huang, E. J. (2017). Progranulin, Lysosomal Regulation and Neurodegenerative Disease. Nat. Rev. Neurosci. 18 (6), 325–333. doi:10.1038/nrn.2017.36
Katona, I., Zhang, X., Bai, Y., Shy, M. E., Guo, J., Yan, Q., et al. (2011). Distinct Pathogenic Processes Between Fig4-Deficient Motor and Sensory Neurons. Eur. J. Neurosci. 33, 1401–1410. doi:10.1111/j.1460-9568.2011.07651.x
Kawabe, Y., Mori, K., Yamashita, T., Gotoh, S., and Ikeda, M. (2020). The RNA Exosome Complex Degrades Expanded Hexanucleotide Repeat RNA in C9orf72 FTLD/ALS. EMBO J. 39 (19), e102700. doi:10.15252/embj.2019102700
Kilchert, C., Wittmann, S., and Vasiljeva, L. (2016). The Regulation and Functions of the Nuclear RNA Exosome Complex. Nat. Rev. Mol. Cell Biol. 17 (4), 227–239. doi:10.1038/nrm.2015.15
Kim, H.-J., Cho, M.-H., Shim, W. H., Kim, J. K., Jeon, E.-Y., Kim, D.-H., et al. (2017). Deficient Autophagy in Microglia Impairs Synaptic Pruning and Causes Social Behavioral Defects. Mol. Psychiatry 22 (11), 1576–1584. doi:10.1038/mp.2016.103
Kim, H. J., Kim, N. C., Wang, Y.-D., Scarborough, E. A., Moore, J., Diaz, Z., et al. (2013). Mutations in Prion-Like Domains in hnRNPA2B1 and hnRNPA1 Cause Multisystem Proteinopathy and ALS. Nature 495 (7442), 467–473. doi:10.1038/nature11922
Kim, H. J., and Taylor, J. P. (2017). Lost in Transportation: Nucleocytoplasmic Transport Defects in ALS and Other Neurodegenerative Diseases. Neuron 96 (2), 285–297. doi:10.1016/j.neuron.2017.07.029
Klionsky, D. J., Abdel-Aziz, A. K., Abdelfatah, S., Abdellatif, M., Abdoli, A., Abel, S., et al. (2021). Guidelines for the Use and Interpretation of Assays for Monitoring Autophagy (4th Edition)1. Autophagy 17 (1), 1–382. doi:10.1080/15548627.2020.179728010.1080/15548627.2015.1100356
Kraft, L. J., Manral, P., Dowler, J., and Kenworthy, A. K. (2016). Nuclear LC3 Associates with Slowly Diffusing Complexes that Survey the Nucleolus. Traffic 17 (4), 369–399. doi:10.1111/tra.12372
Kriegenburg, F., Ungermann, C., and Reggiori, F. (2018). Coordination of Autophagosome-Lysosome Fusion by Atg8 Family Members. Curr. Biol. 28 (8), R512–R518. doi:10.1016/j.cub.2018.02.034
Kumar, S., Gu, Y., Abudu, Y. P., Bruun, J.-A., Jain, A., Farzam, F., et al. (2019). Phosphorylation of Syntaxin 17 by TBK1 Controls Autophagy Initiation. Dev. Cell 49 (1), 130–144.e6. doi:10.1016/j.devcel.2019.01.027
Laflamme, C., McKeever, P. M., Kumar, R., Schwartz, J., Kolahdouzan, M., Chen, C. X., et al. (2019). Implementation of an Antibody Characterization Procedure and Application to the Major ALS/FTD Disease Gene C9ORF72. ELife 8, e48363. doi:10.7554/eLife.48363
Laird, F. M., Farah, M. H., Ackerley, S., Hoke, A., Maragakis, N., Rothstein, J. D., et al. (2008). Motor Neuron Disease Occurring in a Mutant Dynactin Mouse Model Is Characterized by Defects in Vesicular Trafficking. J. Neurosci.: Official J. Soc. Neurosci. 28 (9), 1997–2005. doi:10.1523/JNEUROSCI.4231-07.2008
Lall, D., Lorenzini, I., Mota, T. A., Bell, S., Mahan, T. E., Ulrich, J. D., et al. (2021). C9orf72 Deficiency Promotes Microglial-Mediated Synaptic Loss in Aging and Amyloid Accumulation. Neuron 109 (14), 2275–2291.e8. doi:10.1016/j.neuron.2021.05.020
Larroquette, F., Seto, L., Gaub, P. L., Kamal, B., Wallis, D., Larivière, R., et al. (2015). Vapb/Amyotrophic Lateral Sclerosis 8 Knock-In Mice Display Slowly Progressive Motor Behavior Defects Accompanying ER Stress and Autophagic Response. Hum. Mol. Genet. 24 (22), 6515–6529. doi:10.1093/hmg/ddv360
Lee, A., Rayner, S. L., Gwee, S. S. L., de Luca, A., Shahheydari, H., Sundaramoorthy, V., et al. (2018). Pathogenic Mutation in the ALS/FTD Gene, CCNF, Causes Elevated Lys48-Linked Ubiquitylation and Defective Autophagy. Cell Mol. Life Sci. 75 (2), 335–354. doi:10.1007/s00018-017-2632-8
Lee, J.-G., Takahama, S., Zhang, G., Tomarev, S. I., and Ye, Y. (2016). Unconventional Secretion of Misfolded Proteins Promotes Adaptation to Proteasome Dysfunction in Mammalian Cells. Nat. Cell Biol. 18 (7), 765–776. doi:10.1038/ncb3372
Lee, K.-H., Zhang, P., Kim, H. J., Mitrea, D. M., Sarkar, M., Freibaum, B. D., et al. (2016). C9orf72 Dipeptide Repeats Impair the Assembly, Dynamics, and Function of Membrane-Less Organelles. Cell 167 (3), 774–788.e17. doi:10.1016/j.cell.2016.10.002
Leidal, A. M., Huang, H. H., Marsh, T., Solvik, T., Zhang, D., Ye, J., et al. (2020). The LC3-Conjugation Machinery Specifies the Loading of RNA-Binding Proteins into Extracellular Vesicles. Nat. Cell Biol. 22 (2), 187–199. doi:10.1038/s41556-019-0450-y
Leskelä, S., Huber, N., Rostalski, H., Natunen, T., Remes, A. M., Takalo, M., et al. (2019). C9orf72 Proteins Regulate Autophagy and Undergo Autophagosomal or Proteasomal Degradation in a Cell Type-dependent Manner. Cells 8 (10), 1233. doi:10.3390/cells8101233
Li, K., Li, J., Zheng, J., and Qin, S. (2019). Reactive Astrocytes in Neurodegenerative Diseases. Aging Dis. 10 (3), 664–675. doi:10.14336/AD.2018.0720
Liao, Y.-C., Fernandopulle, M. S., Wang, G., Choi, H., Hao, L., Drerup, C. M., et al. (2019). RNA Granules Hitchhike on Lysosomes for Long-Distance Transport, Using Annexin A11 as a Molecular Tether. Cell 179 (1), 147–164.e20. doi:10.1016/j.cell.2019.08.050
Ling, J. P., Pletnikova, O., Troncoso, J. C., and Wong, P. C. (2015). TDP-43 Repression of Nonconserved Cryptic Exons Is Compromised in ALS-FTD. Science 349 (6248), 650–655. doi:10.1126/science.aab0983
Ling, S.-C., Dastidar, S. G., Tokunaga, S., Ho, W. Y., Lim, K., Ilieva, H., et al. (2019). Overriding FUS Autoregulation in Mice Triggers Gain-of-Toxic Dysfunctions in RNA Metabolism and Autophagy-Lysosome axis. ELife 8, e40811. doi:10.7554/eLife.40811
Ling, S.-C., Polymenidou, M., and Cleveland, D. W. (2013). Converging Mechanisms in ALS and FTD: Disrupted RNA and Protein Homeostasis. Neuron 79 (3), 416–438. doi:10.1016/j.neuron.2013.07.033
Liu, J., Li, H., Zhou, B., Xu, L., Kang, X., Yang, W., et al. (2015). PGRN Induces Impaired Insulin Sensitivity and Defective Autophagy in Hepatic Insulin Resistance. Mol. Endocrinol. 29 (4), 528–541. doi:10.1210/me.2014-1266
Liu, Y., Wang, T., Ji, Y. J., Johnson, K., Liu, H., Johnson, K., et al. (2018). A C9orf72-CARM1 axis Regulates Lipid Metabolism under Glucose Starvation-Induced Nutrient Stress. Genes Develop. 32 (21–22), 1380–1397. doi:10.1101/gad.315564.118
Lui, H., Zhang, J., Makinson, S. R., Cahill, M. K., Kelley, K. W., Huang, H.-Y., et al. (2016). Progranulin Deficiency Promotes Circuit-specific Synaptic Pruning by Microglia via Complement Activation. Cell 165 (4), 921–935. doi:10.1016/j.cell.2016.04.001
Luty, A. A., Kwok, J. B. J., Dobson-Stone, C., Loy, C. T., Coupland, K. G., Karlström, H., et al. (2010). Sigma Nonopioid Intracellular Receptor 1 Mutations Cause Frontotemporal Lobar Degeneration-Motor Neuron Disease. Ann. Neurol. 68 (5), 639–649. doi:10.1002/ana.22274
Mackenzie, I. R., Nicholson, A. M., Sarkar, M., Messing, J., Purice, M. D., Pottier, C., et al. (2017). TIA1 Mutations in Amyotrophic Lateral Sclerosis and Frontotemporal Dementia Promote Phase Separation and Alter Stress Granule Dynamics. Neuron 95 (4), 808–816.e9. doi:10.1016/j.neuron.2017.07.025
Madill, M., McDonagh, K., Ma, J., Vajda, A., McLoughlin, P., O’Brien, T., et al. (2017). Amyotrophic Lateral Sclerosis Patient iPSC-Derived Astrocytes Impair Autophagy via Non-cell Autonomous Mechanisms. Mol. Brain 10 (1), 22. doi:10.1186/s13041-017-0300-4
Majounie, E., Renton, A. E., Mok, K., Dopper, E. G. P., Waite, A., Rollinson, S., et al. (2012). Frequency of the C9orf72 Hexanucleotide Repeat Expansion in Patients with Amyotrophic Lateral Sclerosis and Frontotemporal Dementia: A Cross-Sectional Study. Lancet Neurol. 11 (4), 323–330. doi:10.1016/S1474-4422(12)70043-1
Mammucari, C., Milan, G., Romanello, V., Masiero, E., Rudolf, R., del Piccolo, P., et al. (2007). FoxO3 Controls Autophagy in Skeletal Muscle In Vivo. Cell Metab. 6 (6), 458–471. doi:10.1016/j.cmet.2007.11.001
Mancuso, R., del Valle, J., Modol, L., Martinez, A., Granado-Serrano, A. B., Ramirez-Núñez, O., et al. (2014). Resveratrol Improves Motoneuron Function and Extends Survival in SOD1(G93A) ALS Mice. Neurotherapeutics 11 (2), 419–432. doi:10.1007/s13311-013-0253-y
Mann, J. R., and Donnelly, C. J. (2021). RNA Modulates Physiological and Neuropathological Protein Phase Transitions. Neuron 109 (17), 2663–2681. doi:10.1016/j.neuron.2021.06.023
Marat, A. L., Dokainish, H., and McPherson, P. S. (2011). DENN Domain Proteins: Regulators of Rab GTPases. J. Biol. Chem. 286 (16), 13791–13800. doi:10.1074/jbc.R110.217067
Marat, A. L., and Haucke, V. (2016). Phosphatidylinositol 3-Phosphates-At the Interface between Cell Signalling and Membrane Traffic. EMBO J. 35 (6), 561–579. doi:10.15252/embj.201593564
Markmiller, S., Soltanieh, S., Server, K. L., Mak, R., Jin, W., Fang, M. Y., et al. (2018). Context-Dependent and Disease-specific Diversity in Protein Interactions within Stress Granules. Cell 172 (3), 590–604.e13. doi:10.1016/j.cell.2017.12.032
Marrone, L., Drexler, H. C. A., Wang, J., Tripathi, P., Distler, T., Heisterkamp, P., et al. (2019). FUS Pathology in ALS Is Linked to Alterations in Multiple ALS-Associated Proteins and Rescued by Drugs Stimulating Autophagy. Acta Neuropathol. 138 (1), 67–84. doi:10.1007/s00401-019-01998-x
Martina, J. A., Diab, H. I., Li, H., and Puertollano, R. (2014). Novel Roles for the MiTF/TFE Family of Transcription Factors in Organelle Biogenesis, Nutrient Sensing, and Energy Homeostasis. Cell Mol. Life Sci. 71 (13), 2483–2497. doi:10.1007/s00018-014-1565-8
Maruyama, H., Morino, H., Ito, H., Izumi, Y., Kato, H., Watanabe, Y., et al. (2010). Mutations of Optineurin in Amyotrophic Lateral Sclerosis. Nature 465 (7295), 223–226. doi:10.1038/nature08971
Mateju, D., Eichenberger, B., Voigt, F., Eglinger, J., Roth, G., and Chao, J. A. (2020). Single-Molecule Imaging Reveals Translation of mRNAs Localized to Stress Granules. Cell 183 (7), 1801–1812.e13. doi:10.1016/j.cell.2020.11.010
Mateju, D., Franzmann, T. M., Patel, A., Kopach, A., Boczek, E. E., Maharana, S., et al. (2017). An Aberrant Phase Transition of Stress Granules Triggered by Misfolded Protein and Prevented by Chaperone Function. EMBO J. 36 (12), 1669–1687. doi:10.15252/embj.201695957
Matsumoto, G., Shimogori, T., Hattori, N., and Nukina, N. (2015). TBK1 Controls Autophagosomal Engulfment of Polyubiquitinated Mitochondria through p62/SQSTM1 Phosphorylation. Hum. Mol. Genet. 24 (15), 4429–4442. doi:10.1093/hmg/ddv179
Maziuk, B., Ballance, H. I., and Wolozin, B. (2017). Dysregulation of RNA Binding Protein Aggregation in Neurodegenerative Disorders. Front. Mol. Neurosci. 10, 89. doi:10.3389/fnmol.2017.00089
McEachin, Z. T., Parameswaran, J., Raj, N., Bassell, G. J., and Jiang, J. (2020). RNA-mediated Toxicity in C9orf72 ALS and FTD. Neurobiol. Dis. 145, 105055. doi:10.1016/j.nbd.2020.105055
Mediani, L., Antoniani, F., Galli, V., Vinet, J., Carrà, A. D., Bigi, I., et al. (2021). Hsp90-Mediated Regulation of DYRK3 Couples Stress Granule Disassembly and Growth via mTORC1 Signaling. EMBO Rep. 22 (5), e51740. doi:10.15252/embr.202051740
Mercer, T. J., Gubas, A., and Tooze, S. A. (2018). A Molecular Perspective of Mammalian Autophagosome Biogenesis. J. Biol. Chem. 293 (15), 5386–5395. doi:10.1074/jbc.R117.810366
Meyer, K., Ferraiuolo, L., Miranda, C. J., Likhite, S., McElroy, S., Renusch, S., et al. (2014). Direct Conversion of Patient Fibroblasts Demonstrates Non-cell Autonomous Toxicity of Astrocytes to Motor Neurons in Familial and Sporadic ALS. Proc. Natl. Acad. Sci. United States America 111 (2), 829–832. doi:10.1073/pnas.1314085111
Mitne-Neto, M., Machado-Costa, M., Marchetto, M. C. N., Bengtson, M. H., Joazeiro, C. A., Tsuda, H., et al. (2011). Downregulation of VAPB Expression in Motor Neurons Derived from Induced Pluripotent Stem Cells of ALS8 Patients. Hum. Mol. Genet. 20 (18), 3642–3652. doi:10.1093/hmg/ddr284
Mizielinska, S., Grönke, S., Niccoli, T., Ridler, C. E., Clayton, E. L., Devoy, A., et al. (2014). C9orf72 Repeat Expansions Cause Neurodegeneration in Drosophila through Arginine-Rich Proteins. Science 345 (6201), 1192–1194. doi:10.1126/science.1256800
Mizielinska, S., Lashley, T., Norona, F. E., Clayton, E. L., Ridler, C. E., Fratta, P., et al. (2013). C9orf72 Frontotemporal Lobar Degeneration Is Characterised by Frequent Neuronal Sense and Antisense RNA Foci. Acta Neuropathol. 126 (6), 845–857. doi:10.1007/s00401-013-1200-z
Mizushima, N. (2020). The ATG Conjugation Systems in Autophagy. Curr. Opin. Cell Biol. 63, 1–10. doi:10.1016/j.ceb.2019.12.001
Moens, T. G., Niccoli, T., Wilson, K. M., Atilano, M. L., Birsa, N., Gittings, L. M., et al. (2019). C9orf72 Arginine-Rich Dipeptide Proteins Interact with Ribosomal Proteins In Vivo to Induce a Toxic Translational Arrest that Is Rescued by eIF1A. Acta Neuropathol. 137 (3), 487–500. doi:10.1007/s00401-018-1946-4
Münch, C., Sedlmeier, R., Meyer, T., Homberg, V., Sperfeld, A. D., Kurt, A., et al. (2004). Point Mutations of the P150 Subunit of Dynactin (DCTN1) Gene in ALS. Neurology 63 (4), 724–726. doi:10.1212/01.wnl.0000134608.83927.b1
Murakami, T., Qamar, S., Lin, J. Q., Schierle, G. S. K., Rees, E., Miyashita, A., et al. (2015). ALS/FTD Mutation-Induced Phase Transition of FUS Liquid Droplets and Reversible Hydrogels into Irreversible Hydrogels Impairs RNP Granule Function. Neuron 88 (4), 678–690. doi:10.1016/j.neuron.2015.10.030
Murray, M. E., DeJesus-Hernandez, M., Rutherford, N. J., Baker, M., Duara, R., Graff-Radford, N. R., et al. (2011). Clinical and Neuropathologic Heterogeneity of c9FTD/ALS Associated with Hexanucleotide Repeat Expansion in C9ORF72. Acta Neuropathol. 122 (6), 673–690. doi:10.1007/s00401-011-0907-y
Nahm, M., Lim, S. M., Kim, Y.-E., Park, J., Noh, M.-Y., Lee, S., et al. (2020). ANXA11 Mutations in ALS Cause Dysregulation of Calcium Homeostasis and Stress Granule Dynamics. Sci. Transl. Med. 12 (566), eaax3993. doi:10.1126/scitranslmed.aax3993
Neumann, M., Kwong, L. K., Sampathu, D. M., Trojanowski, J. Q., and Lee, V. M.-Y. (2007). TDP-43 Proteinopathy in Frontotemporal Lobar Degeneration and Amyotrophic Lateral Sclerosis: Protein Misfolding Diseases without Amyloidosis. Arch. Neurol. 64 (10), 1388–1394. doi:10.1001/archneur.64.10.1388
Nicolas, A., Kenna, K. P., Renton, A. E., Ticozzi, N., Faghri, F., Chia, R., et al. (2018). Genome-wide Analyses Identify KIF5A as a Novel ALS Gene. Neuron 97 (6), 1268–1283.e6. doi:10.1016/j.neuron.2018.02.027
Nishimura, A. L., Mitne-Neto, M., Silva, H. C. A., Richieri-Costa, A., Middleton, S., Cascio, D., et al. (2004). A Mutation in the Vesicle-Trafficking Protein VAPB Causes Late-Onset Spinal Muscular Atrophy and Amyotrophic Lateral Sclerosis. Am. J. Hum. Genet. 75 (5), 822–831. doi:10.1086/425287
Nixon, R. A. (2013). The Role of Autophagy in Neurodegenerative Disease. Nat. Med. 19 (8), 983–997. doi:10.1038/nm.3232
Nörpel, J., Cavadini, S., Schenk, A. D., Graff-Meyer, A., Hess, D., Seebacher, J., et al. (2021). Structure of the Human C9orf72-SMCR8 Complex Reveals a Multivalent Protein Interaction Architecture. PLoS Biol. 19 (7), e3001344. doi:10.1371/journal.pbio.3001344
Nthiga, T. M., Kumar Shrestha, B., Sjøttem, E., Bruun, J.-A., Bowitz Larsen, K., Bhujabal, Z., et al. (2020). CALCOCO1 Acts with VAMP-Associated Proteins to Mediate ER-Phagy. EMBO J. 39 (15), e103649. doi:10.15252/embj.2019103649
Nussbacher, J. K., Tabet, R., Yeo, G. W., and Lagier-Tourenne, C. (2019). Disruption of RNA Metabolism in Neurological Diseases and Emerging Therapeutic Interventions. Neuron 102 (2), 294–320. doi:10.1016/j.neuron.2019.03.014
Oeckl, P., Weydt, P., Steinacker, P., Anderl-Straub, S., Nordin, F., Volk, A. E., et al. (2019). Different Neuroinflammatory Profile in Amyotrophic Lateral Sclerosis and Frontotemporal Dementia Is Linked to the Clinical Phase. J. Neurol. Neurosurg. Psychiatry 90 (1), 4–10. doi:10.1136/jnnp-2018-318868
Olgeiser, L., Haag, C., Boerner, S., Ule, J., Busch, A., Koepke, J., et al. (2019). The Key Protein of Endosomal mRNP Transport Rrm4 Binds Translational Landmark Sites of Cargo mRNAs. EMBO Rep. 20 (1), e46588. doi:10.15252/embr.201846588
Orlacchio, A., Babalini, C., Borreca, A., Patrono, C., Massa, R., Basaran, S., et al. (2010). SPATACSIN Mutations Cause Autosomal Recessive Juvenile Amyotrophic Lateral Sclerosis. Brain : A J. Neurol. 133 (Pt 2), 591–598. doi:10.1093/brain/awp325
O’Rourke, J. G., Bogdanik, L., Yáñez, A., Lall, D., Wolf, A. J., Muhammad, A. K. M. G., et al. (2016). C9orf72 Is Required for Proper Macrophage and Microglial Function in Mice. Science 351 (6279), 1324–1329. doi:10.1126/science.aaf1064
Otomo, A., Hadano, S., Okada, T., Mizumura, H., Kunita, R., Nishijima, H., et al. (2003). ALS2, a Novel Guanine Nucleotide Exchange Factor for the Small GTPase Rab5, Is Implicated in Endosomal Dynamics. Hum. Mol. Genet. 12 (14), 1671–1687. doi:10.1093/hmg/ddg184
Palmieri, M., Impey, S., Kang, H., di Ronza, A., Pelz, C., Sardiello, M., et al. (2011). Characterization of the CLEAR Network Reveals an Integrated Control of Cellular Clearance Pathways. Hum. Mol. Genet. 20 (19), 3852–3866. doi:10.1093/hmg/ddr306
Pankiv, S., Clausen, T. H., Lamark, T., Brech, A., Bruun, J. A., Outzen, H., et al. (2007). P62/Sqstm1 Binds Directly to Atg8/Lc3 to Facilitate Degradation of Ubiquitinated Protein Aggregates by Autophagy. J. Biol. Chem. 282, 24131–24145. doi:10.1074/jbc.M702824200
Parkinson, N., Ince, P. G., Smith, M. O., Highley, R., Skibinski, G., Andersen, P. M., et al. (2006). ALS Phenotypes with Mutations in CHMP2B (Charged Multivesicular Body Protein 2B). Neurology 67 (6), 1074–1077. doi:10.1212/01.wnl.0000231510.89311.8b
Patel, A., Lee, H. O., Jawerth, L., Maharana, S., Jahnel, M., Hein, M. Y., et al. (2015). A Liquid-to-Solid Phase Transition of the ALS Protein FUS Accelerated by Disease Mutation. Cell 162 (5), 1066–1077. doi:10.1016/j.cell.2015.07.047
Petkau, T. L., and Leavitt, B. R. (2014). Progranulin in Neurodegenerative Disease. Trends Neurosciences 37 (7), 388–398. doi:10.1016/j.tins.2014.04.003
Pilli, M., Arko-Mensah, J., Ponpuak, M., Roberts, E., Master, S., Mandell, M. A., et al. (2012). TBK-1 Promotes Autophagy-Mediated Antimicrobial Defense by Controlling Autophagosome Maturation. Immunity 37 (2), 223–234. doi:10.1016/j.immuni.2012.04.015
Ploper, D., Taelman, V. F., Robert, L., Perez, B. S., Titz, B., Chen, H.-W., et al. (2015). MITF Drives Endolysosomal Biogenesis and Potentiates Wnt Signaling in Melanoma Cells. Proc. Natl. Acad. Sci. 112 (5), E420 LP–E429. doi:10.1073/pnas.1424576112
Pohlmann, T., Baumann, S., Haag, C., Albrecht, M., and Feldbrügge, M. (2015). A FYVE Zinc finger Domain Protein Specifically Links mRNA Transport to Endosome Trafficking. ELife 4, e06041. doi:10.7554/eLife.06041
Polymenidou, M., Lagier-Tourenne, C., Hutt, K. R., Huelga, S. C., Moran, J., Liang, T. Y., et al. (2011). Long Pre-mRNA Depletion and RNA Missplicing Contribute to Neuronal Vulnerability from Loss of TDP-43. Nat. Neurosci. 14 (4), 459–468. doi:10.1038/nn.2779
Puertollano, R., Ferguson, S. M., Brugarolas, J., and Ballabio, A. (2018). The Complex Relationship between TFEB Transcription Factor Phosphorylation and Subcellular Localization. EMBO J. 37 (11), e98804. doi:10.15252/embj.201798804
Ragagnin, A. M. G., Shadfar, S., Vidal, M., Jamali, M. S., and Atkin, J. D. (2019). Motor Neuron Susceptibility in ALS/FTD. Front. Neurosci. 13, 532. doi:10.3389/fnins.2019.00532
Rajyaguru, P., and Parker, R. (2012). RGG Motif Proteins: Modulators of mRNA Functional States. Cell Cycle (Georgetown, Tex.) 11 (14), 2594–2599. doi:10.4161/cc.20716
Ramesh, N., and Pandey, U. B. (2017). Autophagy Dysregulation in ALS: When Protein Aggregates Get Out of Hand. Front. Mol. Neurosci. 10, 263. doi:10.3389/fnmol.2017.00263
Reineke, L. C., and Neilson, J. R. (2019). Differences between Acute and Chronic Stress Granules, and How These Differences May Impact Function in Human Disease. Biochem. Pharmacol. 162, 123–131. doi:10.1016/j.bcp.2018.10.009
Renton, A. E., Majounie, E., Waite, A., Simón-Sánchez, J., Rollinson, S., Gibbs, J. R., et al. (2011). A Hexanucleotide Repeat Expansion in C9ORF72 Is the Cause of Chromosome 9p21-Linked ALS-FTD. Neuron 72 (2), 257–268. doi:10.1016/j.neuron.2011.09.010
Richter, B., Sliter, D. A., Herhaus, L., Stolz, A., Wang, C., Beli, P., et al. (2016). Phosphorylation of OPTN by TBK1 Enhances its Binding to Ub Chains and Promotes Selective Autophagy of Damaged Mitochondria. Proc. Natl. Acad. Sci. U.S.A. 113 (15), 4039–4044. doi:10.1073/pnas.1523926113
Rimessi, A., Pozzato, C., Carparelli, L., Rossi, A., Ranucci, S., de Fino, I., et al. (2020). Pharmacological Modulation of Mitochondrial Calcium Uniporter Controls Lung Inflammation in Cystic Fibrosis. Sci. Adv. 6 (19), eaax9093. doi:10.1126/sciadv.aax9093
Rothenberg, C., Srinivasan, D., Mah, L., Kaushik, S., Peterhoff, C. M., Ugolino, J., et al. (2010). Ubiquilin Functions in Autophagy and Is Degraded by Chaperone-Mediated Autophagy. Hum. Mol. Genet. 19 (16), 3219–3232. doi:10.1093/hmg/ddq231
Rubino, E., Rainero, I., Chiò, A., Rogaeva, E., Galimberti, D., Fenoglio, P., et al. (2012). SQSTM1 Mutations in Frontotemporal Lobar Degeneration and Amyotrophic Lateral Sclerosis. Neurology 79 (15), 1556–1562. doi:10.1212/WNL.0b013e31826e25df
Ryu, H.-H., Jun, M.-H., Min, K.-J., Jang, D.-J., Lee, Y.-S., Kim, H. K., et al. (2014). Autophagy Regulates Amyotrophic Lateral Sclerosis-Linked Fused in Sarcoma-Positive Stress Granules in Neurons. Neurobiol. Aging 35 (12), 2822–2831. doi:10.1016/j.neurobiolaging.2014.07.026
Saez-Atienzar, S., Bandres-Ciga, S., Langston, R. G., Kim, J. J., Choi, S. W., Reynolds, R. H., et al. (2021). Genetic Analysis of Amyotrophic Lateral Sclerosis Identifies Contributing Pathways and Cell Types. Sci. Adv. 7 (3), eabd9036. doi:10.1126/sciadv.abd9036
Sakae, N., Bieniek, K. F., Zhang, Y.-J., Ross, K., Gendron, T. F., Murray, M. E., et al. (2018). Poly-GR Dipeptide Repeat Polymers Correlate with Neurodegeneration and Clinicopathological Subtypes in C9ORF72-Related Brain Disease. Acta Neuropathol. Commun. 6 (1), 63. doi:10.1186/s40478-018-0564-7
Sakellariou, D., Tiberti, M., Kleiber, T. H., Blazquez, L., López, A. R., Abildgaard, M. H., et al. (2021). eIF4A3 Regulates the TFEB-Mediated Transcriptional Response via GSK3B to Control Autophagy. Cell Death Differ. 28 (12), 3344–3356. doi:10.1038/s41418-021-00822-y
Saksena, S., and Emr, S. D. (2009). ESCRTs and Human Disease. Biochem. Soc. Trans. 37 (1), 167–172. doi:10.1042/BST0370167
Salogiannis, J., and Reck-Peterson, S. L. (2017). Hitchhiking: A Non-canonical Mode of Microtubule-Based Transport. Trends Cell Biol. 27 (2), 141–150. doi:10.1016/j.tcb.2016.09.005
Sameshima, M., Liebhaber, S. A., and Schlessinger, D. (1981). Dual Pathways for Ribonucleic Acid Turnover in WI-38 but Not in I-Cell Human Diploid Fibroblasts. Mol. Cell Biol. 1 (1), 75–81. doi:10.1128/mcb.1.1.75-81.1981
Samir, P., Kesavardhana, S., Patmore, D. M., Gingras, S., Malireddi, R. K. S., Karki, R., et al. (2019). DDX3X Acts as a Live-or-Die Checkpoint in Stressed Cells by Regulating NLRP3 Inflammasome. Nature 573 (7775), 590–594. doi:10.1038/s41586-019-1551-2
Sardiello, M., Palmieri, M., di Ronza, A., Medina, D. L., Valenza, M., Gennarino, V. A., et al. (2009). A Gene Network Regulating Lysosomal Biogenesis and Function. Science 325 (5939), 473–477. doi:10.1126/science.1174447
Saxton, R. A., and Sabatini, D. M. (2017). mTOR Signaling in Growth, Metabolism, and Disease. Cell 168 (6), 960–976. doi:10.1016/j.cell.2017.02.004
Schröder, B. A., Wrocklage, C., Hasilik, A., and Saftig, P. (2010). The Proteome of Lysosomes. Proteomics 10 (22), 4053–4076. doi:10.1002/pmic.201000196
Schymick, J. C., Yang, Y., Andersen, P. M., Vonsattel, J. P., Greenway, M., Momeni, P., et al. (2007). Progranulin Mutations and Amyotrophic Lateral Sclerosis or Amyotrophic Lateral Sclerosis-Frontotemporal Dementia Phenotypes. J. Neurol. Neurosurg. Psychiatry 78 (7), 754–756. doi:10.1136/jnnp.2006.109553
Seguin, S. J., Morelli, F. F., Vinet, J., Amore, D., de Biasi, S., Poletti, A., et al. (2014). Inhibition of Autophagy, Lysosome and VCP Function Impairs Stress Granule Assembly. Cell Death Differ. 21 (12), 1838–1851. doi:10.1038/cdd.2014.103
Sellier, C., Campanari, M.-L., Julie Corbier, C., Gaucherot, A., Kolb-Cheynel, I., Oulad-Abdelghani, M., et al. (2016). Loss of C9ORF72 Impairs Autophagy and Synergizes with polyQ Ataxin-2 to Induce Motor Neuron Dysfunction and Cell Death. EMBO J. 35 (12), 1276–1297. doi:10.15252/embj.201593350
Şentürk, M., Lin, G., Zuo, Z., Mao, D., Watson, E., Mikos, A. G., et al. (2019). Ubiquilins Regulate Autophagic Flux through mTOR Signalling and Lysosomal Acidification. Nat. Cell Biol. 21 (3), 384–396. doi:10.1038/s41556-019-0281-x
Sephton, C. F., Cenik, C., Kucukural, A., Dammer, E. B., Cenik, B., Han, Y., et al. (2011). Identification of Neuronal RNA Targets of TDP-43-Containing Ribonucleoprotein Complexes. J. Biol. Chem. 286 (2), 1204–1215. doi:10.1074/jbc.M110.190884
Settembre, C., Zoncu, R., Medina, D. L., Vetrini, F., Erdin, S., Erdin, S., et al. (2012). A Lysosome-to-Nucleus Signalling Mechanism Senses and Regulates the Lysosome via mTOR and TFEB. EMBO J. 31 (5), 1095–1108. doi:10.1038/emboj.2012.32
Shah, S. Z. A., Zhao, D., Hussain, T., Sabir, N., and Yang, L. (2018). Regulation of MicroRNAs-Mediated Autophagic Flux: A New Regulatory Avenue for Neurodegenerative Diseases with Focus on Prion Diseases. Front. Aging Neurosci. 10, 139. doi:10.3389/fnagi.2018.00139
Shao, Q., Liang, C., Chang, Q., Zhang, W., Yang, M., and Chen, J.-F. (2019). C9orf72 Deficiency Promotes Motor Deficits of a C9ALS/FTD Mouse Model in a Dose-dependent Manner. Acta Neuropathol. Commun. 7 (1), 32. doi:10.1186/s40478-019-0685-7
Shao, Q., Yang, M., Liang, C., Ma, L., Zhang, W., Jiang, Z., et al. (2020). C9orf72 and Smcr8 Mutant Mice Reveal MTORC1 Activation Due to Impaired Lysosomal Degradation and Exocytosis. Autophagy 16 (9), 1635–1650. doi:10.1080/15548627.2019.1703353
Shepheard, S. R., Parker, M. D., Cooper-Knock, J., Verber, N. S., Tuddenham, L., Heath, P., et al. (2021). Value of Systematic Genetic Screening of Patients with Amyotrophic Lateral Sclerosis. J. Neurol. Neurosurg. Psychiatry 92 (5), 510–518. doi:10.1136/jnnp-2020-325014
Shi, Y., Lin, S., Staats, K. A., Li, Y., Chang, W.-H., Hung, S.-T., et al. (2018). Haploinsufficiency Leads to Neurodegeneration in C9ORF72 ALS/FTD Human Induced Motor Neurons. Nat. Med. 24 (3), 313–325. doi:10.1038/nm.4490
Sivadasan, R., Hornburg, D., Drepper, C., Frank, N., Jablonka, S., Hansel, A., et al. (2016). C9ORF72 Interaction with Cofilin Modulates Actin Dynamics in Motor Neurons. Nat. Neurosci. 19 (12), 1610–1618. doi:10.1038/nn.4407
Skibinski, G., Parkinson, N. J., Brown, J. M., Chakrabarti, L., Lloyd, S. L., Hummerich, H., et al. (2005). Mutations in the Endosomal ESCRTIII-Complex Subunit CHMP2B in Frontotemporal Dementia. Nat. Genet. 37 (8), 806–808. doi:10.1038/ng1609
Smith, B. N., Ticozzi, N., Fallini, C., Gkazi, A. S., Topp, S., Kenna, K. P., et al. (2014). Exome-wide Rare Variant Analysis Identifies TUBA4A Mutations Associated with Familial ALS. Neuron 84 (2), 324–331. doi:10.1016/j.neuron.2014.09.027
Smith, B. N., Topp, S. D., Fallini, C., Shibata, H., Chen, H.-J., Troakes, C., et al. (2017). Mutations in the Vesicular Trafficking Protein Annexin A11 Are Associated with Amyotrophic Lateral Sclerosis. Sci. Transl. Med. 9 (388), eaad9157. doi:10.1126/scitranslmed.aad9157
Solomon, D. A., Smikle, R., Reid, M. J., and Mizielinska, S. (2021). Altered Phase Separation and Cellular Impact in C9orf72-Linked ALS/FTD. Front. Cell Neurosci. 15, 664151. doi:10.3389/fncel.2021.664151
Soo, K. Y., Sultana, J., King, A. E., Atkinson, R., Warraich, S. T., Sundaramoorthy, V., et al. (2015). ALS-associated Mutant FUS Inhibits Macroautophagy Which Is Restored by Overexpression of Rab1. Cell Death Discov. 1, 15030. doi:10.1038/cddiscovery.2015.30
Spillantini, M. G., and Goedert, M. (2013). Tau Pathology and Neurodegeneration. Lancet Neurol. 12 (6), 609–622. doi:10.1016/S1474-4422(13)70090-5
Sreedharan, J., Blair, I. P., Tripathi, V. B., Hu, X., Vance, C., Rogelj, B., et al. (2008). TDP-43 Mutations in Familial and Sporadic Amyotrophic Lateral Sclerosis. Science 319 (5870), 1668–1672. doi:10.1126/science.1154584
Stavoe, A. K. H., and Holzbaur, E. L. F. (2019). Autophagy in Neurons. Annu. Rev. Cell Dev. Biol. 35, 477–500. doi:10.1146/annurev-cellbio-100818-125242
Stenmark, H. (2009). Rab GTPases as Coordinators of Vesicle Traffic. Nat. Rev. Mol. Cell Biol. 10 (8), 513–525. doi:10.1038/nrm2728
Stoica, R., de Vos, K. J., Paillusson, S., Mueller, S., Sancho, R. M., Lau, K.-F., et al. (2014). ER–mitochondria Associations Are Regulated by the VAPB–PTPIP51 Interaction and Are Disrupted by ALS/FTD-associated TDP-43. Nat. Commun. 5 (1), 3996. doi:10.1038/ncomms4996
Strohm, L., and Behrends, C. (2020). Glia-specific Autophagy Dysfunction in ALS. Semin. Cell Dev. Biol. 99, 172–182. doi:10.1016/j.semcdb.2019.05.024
Su, M.-Y., Fromm, S. A., Zoncu, R., and Hurley, J. H. (2020). Structure of the C9orf72 ARF GAP Complex that is Haploinsufficient in ALS and FTD. Nature 585 (7824), 251–255. doi:10.1038/s41586-020-2633-x
Sullivan, P. M., Zhou, X., Robins, A. M., Paushter, D. H., Kim, D., Smolka, M. B., et al. (2016). The ALS/FTLD Associated Protein C9orf72 Associates with SMCR8 and WDR41 to Regulate the Autophagy-Lysosome Pathway. Acta Neuropathol. Commun. 4 (1), 51. doi:10.1186/s40478-016-0324-5
Sun, D., Wu, R., Li, P., and Yu, L. (2020). Phase Separation in Regulation of Aggrephagy. J. Mol. Biol. 432 (1), 160–169. doi:10.1016/j.jmb.2019.06.026
Sung, K., and Jimenez-Sanchez, M. (2020). Autophagy in Astrocytes and its Implications in Neurodegeneration. J. Mol. Biol. 432 (8), 2605–2621. doi:10.1016/j.jmb.2019.12.041
Szatmári, Z., and Sass, M. (2014). The Autophagic Roles of Rab Small GTPases and Their Upstream Regulators: a Review. Autophagy 10 (7), 1154–1166. doi:10.4161/auto.29395
Takahara, T., and Maeda, T. (2012). Transient Sequestration of TORC1 into Stress Granules during Heat Stress. Mol. Cell 47 (2), 242–252. doi:10.1016/j.molcel.2012.05.019
Takahashi, Y., He, H., Tang, Z., Hattori, T., Liu, Y., Young, M. M., et al. (2018). An Autophagy Assay Reveals the ESCRT-III Component CHMP2A as a Regulator of Phagophore Closure. Nat. Commun. 9 (1), 2855. doi:10.1038/s41467-018-05254-w
Tanaka, Y., Suzuki, G., Matsuwaki, T., Hosokawa, M., Serrano, G., Beach, T. G., et al. (2017). Progranulin Regulates Lysosomal Function and Biogenesis through Acidification of Lysosomes. Hum. Mol. Genet. 26 (5), 969–988. doi:10.1093/hmg/ddx011
Tang, D., Sheng, J., Xu, L., Zhan, X., Liu, J., Jiang, H., et al. (2020). Cryo-EM Structure of C9ORF72–SMCR8–WDR41 Reveals the Role as a GAP for Rab8a and Rab11a. Proc. Natl. Acad. Sci. 117 (18), 9876 LP–9883. doi:10.1073/pnas.2002110117
Tatosyan, K. A., Ustyantsev, I. G., and Kramerov, D. A. (2020). RNA Degradation in Eukaryotic Cells. Mol. Biol. 54 (4), 485–502. doi:10.1134/S0026893320040159
Tekirdag, K., and Cuervo, A. M. (2018). Chaperone-mediated Autophagy and Endosomal Microautophagy: Joint by a Chaperone. J. Biol. Chem. 293 (15), 5414–5424. doi:10.1074/jbc.R117.818237
Tripathi, P., Guo, H., Dreser, A., Yamoah, A., Sechi, A., Jesse, C. M., et al. (2021). Pathomechanisms of ALS8: Altered Autophagy and Defective RNA Binding Protein (RBP) Homeostasis Due to the VAPB P56S Mutation. Cell Death Dis. 12 (5), 466. doi:10.1038/s41419-021-03710-y
Turakhiya, A., Meyer, S. R., Marincola, G., Böhm, S., Vanselow, J. T., Schlosser, A., et al. (2018). ZFAND1 Recruits P97 and the 26S Proteasome to Promote the Clearance of Arsenite-Induced Stress Granules. Mol. Cell 70 (5), 906–919.e7. doi:10.1016/j.molcel.2018.04.021
Ugbode, C., and West, R. J. H. (2021). Lessons Learned from CHMP2B, Implications for Frontotemporal Dementia and Amyotrophic Lateral Sclerosis. Neurobiol. Dis. 147, 105144. doi:10.1016/j.nbd.2020.105144
Ugolino, J., Ji, Y. J., Conchina, K., Chu, J., Nirujogi, R. S., Pandey, A., et al. (2016). Loss of C9orf72 Enhances Autophagic Activity via Deregulated mTOR and TFEB Signaling. PLoS Genet. 12 (11), e1006443. doi:10.1371/journal.pgen.1006443
Vahsen, B. F., Gray, E., Thompson, A. G., Ansorge, O., Anthony, D. C., Cowley, S. A., et al. (2021). Non-neuronal Cells in Amyotrophic Lateral Sclerosis — from Pathogenesis to Biomarkers. Nat. Rev. Neurol. 17 (6), 333–348. doi:10.1038/s41582-021-00487-8
van der Vos, K. E., Eliasson, P., Proikas-Cezanne, T., Vervoort, S. J., van Boxtel, R., Putker, M., et al. (2012). Modulation of Glutamine Metabolism by the PI(3)K-PKB-FOXO Network Regulates Autophagy. Nat. Cell Biol. 14 (8), 829–837. doi:10.1038/ncb2536
van Langenhove, T., van der Zee, J., Sleegers, K., Engelborghs, S., Vandenberghe, R., Gijselinck, I., et al. (2010). Genetic Contribution of FUS to Frontotemporal Lobar Degeneration. Neurology 74 (5), 366–371. doi:10.1212/WNL.0b013e3181ccc732
van Niel, G., D’Angelo, G., and Raposo, G. (2018). Shedding Light on the Cell Biology of Extracellular Vesicles. Nat. Rev. Mol. Cell Biol. 19 (4), 213–228. doi:10.1038/nrm.2017.125
Vance, C., Rogelj, B., Hortobágyi, T., de Vos, K. J., Nishimura, A. L., Sreedharan, J., et al. (2009). Mutations in FUS, an RNA Processing Protein, Cause Familial Amyotrophic Lateral Sclerosis Type 6. Science 323 (5918), 1208–1211. doi:10.1126/science.1165942
Varcianna, A., Myszczynska, M. A., Castelli, L. M., O’Neill, B., Kim, Y., Talbot, J., et al. (2019). Micro-RNAs Secreted through Astrocyte-Derived Extracellular Vesicles Cause Neuronal Network Degeneration in C9orf72 ALS. EBioMedicine 40, 626–635. doi:10.1016/j.ebiom.2018.11.067
Verkhratsky, A., Matteoli, M., Parpura, V., Mothet, J.-P., and Zorec, R. (2016). Astrocytes as Secretory Cells of the central Nervous System: Idiosyncrasies of Vesicular Secretion. EMBO J. 35 (3), 239–257. doi:10.15252/embj.201592705
Wang, B., Maxwell, B. A., Joo, J. H., Gwon, Y., Messing, J., Mishra, A., et al. (2019). ULK1 and ULK2 Regulate Stress Granule Disassembly through Phosphorylation and Activation of VCP/p97. Mol. Cell 74 (4), 742–757.e8. doi:10.1016/j.molcel.2019.03.027
Wang, H., Wang, N., Xu, D., Ma, Q., Chen, Y., Xu, S., et al. (2020). Oxidation of Multiple MiT/TFE Transcription Factors Links Oxidative Stress to Transcriptional Control of Autophagy and Lysosome Biogenesis. Autophagy 16 (9), 1683–1696. doi:10.1080/15548627.2019.1704104
Wang, H., Wang, R., Xu, S., and Lakshmana, M. K. (2016). Transcription Factor EB is Selectively Reduced in the Nuclear Fractions of Alzheimer’s and Amyotrophic Lateral Sclerosis Brains. Neurosci. J. 2016, 4732837. doi:10.1155/2016/4732837
Wang, I.-F., Guo, B.-S., Liu, Y.-C., Wu, C.-C., Yang, C.-H., Tsai, K.-J., et al. (2012). Autophagy Activators rescue and Alleviate Pathogenesis of a Mouse Model with Proteinopathies of the TAR DNA-Binding Protein 43. Proc. Natl. Acad. Sci. U.S.A. 109 (37), 15024–15029. doi:10.1073/pnas.1206362109
Wang, M., Wang, H., Tao, Z., Xia, Q., Hao, Z., Prehn, J. H. M., et al. (2020). C9orf72 Associates with Inactive Rag GTPases and Regulates mTORC1-Mediated Autophagosomal and Lysosomal Biogenesis. Aging Cell 19 (4), e13126. doi:10.1111/acel.13126
Warner, J. R. (1999). The Economics of Ribosome Biosynthesis in Yeast. Trends Biochem. Sci. 24 (11), 437–440. doi:10.1016/s0968-0004(99)01460-7
Webster, C. P., Smith, E. F., Bauer, C. S., Moller, A., Hautbergue, G. M., Ferraiuolo, L., et al. (2016). The C9orf72 Protein Interacts with Rab1a and the ULK1 Complex to Regulate Initiation of Autophagy. EMBO J. 35 (15), 1656–1676. doi:10.15252/embj.201694401
Weinberg, D. E., Shah, P., Eichhorn, S. W., Hussmann, J. A., Plotkin, J. B., and Bartel, D. P. (2016). Improved Ribosome-Footprint and mRNA Measurements Provide Insights into Dynamics and Regulation of Yeast Translation. Cell Rep. 14 (7), 1787–1799. doi:10.1016/j.celrep.2016.01.043
Williams, K. L., Topp, S., Yang, S., Smith, B., Fifita, J. A., Warraich, S. T., et al. (2016). CCNF Mutations in Amyotrophic Lateral Sclerosis and Frontotemporal Dementia. Nat. Commun. 7, 11253. doi:10.1038/ncomms11253
Wild, P., Farhan, H., Mcewan, D. G., Wagner, S., Rogov, V. V., Brady, N. R., et al. (2011). Phosphorylation of the Autophagy Receptor Optineurin Restricts Salmonella Growth. Science 333, 228–233. doi:10.1126/science.1205405
Wippich, F., Bodenmiller, B., Trajkovska, M. G., Wanka, S., Aebersold, R., and Pelkmans, L. (2013). Dual Specificity Kinase DYRK3 Couples Stress Granule Condensation/dissolution to mTORC1 Signaling. Cell 152 (4), 791–805. doi:10.1016/j.cell.2013.01.033
Wolozin, B., and Ivanov, P. (2019). Stress Granules and Neurodegeneration. Nat. Rev. Neurosci. 20 (11), 649–666. doi:10.1038/s41583-019-0222-5
Wu, J. J., Cai, A., Greenslade, J. E., Higgins, N. R., Fan, C., Le, N. T. T., et al. (2020). ALS/FTD Mutations in UBQLN2 Impede Autophagy by Reducing Autophagosome Acidification through Loss of Function. Proc. Natl. Acad. Sci. 117 (26), 15230 LP–15241. doi:10.1073/pnas.1917371117
Wyant, G. A., Abu-Remaileh, M., Frenkel, E. M., Laqtom, N. N., Dharamdasani, V., Lewis, C. A., et al. (2018). NUFIP1 Is a Ribosome Receptor for Starvation-Induced Ribophagy. Science 360 (6390), 751–758. doi:10.1126/science.aar2663
Xia, Q., Wang, H., Hao, Z., Fu, C., Hu, Q., Gao, F., et al. (2016). TDP-43 Loss of Function Increases TFEB Activity and Blocks Autophagosome-Lysosome Fusion. EMBO J. 35 (2), 121–142. doi:10.15252/embj.201591998
Xu, J., Camfield, R., and Gorski, S. M. (2018). The Interplay Between Exosomes and Autophagy - Partners in Crime. J. Cell Sci. 131 (15), jcs215210. doi:10.1242/jcs.215210
Xu, Z., Yan, Y., Qian, L., and Gong, Z. (2017). Long Non-Coding RNAs Act as Regulators of Cell Autophagy in Diseases (Review). Oncol. Rep. 37 (3), 1359–1366. doi:10.3892/or.2017.5416
Yan, G., Lai, Y., and Jiang, Y. (2012). The TOR Complex 1 is a Direct Target of Rho1 GTPase. Mol. Cell 45 (6), 743–753. doi:10.1016/j.molcel.2012.01.028
Yang, H., Shen, H., Li, J., and Guo, L.-W. (2019). SIGMAR1/Sigma-1 Receptor Ablation Impairs Autophagosome Clearance. Autophagy 15 (9), 1539–1557. doi:10.1080/15548627.2019.1586248
Yang, L., Wang, H., Shen, Q., Feng, L., and Jin, H. (2017). Long Non-Coding RNAs Involved in Autophagy Regulation. Cell Death Dis. 8 (10), e3073. doi:10.1038/cddis.2017.464
Yang, M., Liang, C., Swaminathan, K., Herrlinger, S., Lai, F., Shiekhattar, R., et al. (2016). A C9ORF72/SMCR8-Containing Complex Regulates ULK1 and Plays a Dual Role in Autophagy. Sci. Adv. 2 (9), e1601167. doi:10.1126/sciadv.1601167
Yang, Y., Hentati, A., Deng, H. X., Dabbagh, O., Sasaki, T., Hirano, M., et al. (2001). The Gene Encoding Alsin, a Protein with Three Guanine-Nucleotide Exchange Factor Domains, Is Mutated in a Form of Recessive Amyotrophic Lateral Sclerosis. Nat. Genet. 29 (2), 160–165. doi:10.1038/ng1001-160
Yu, J., Lai, C., Shim, H., Xie, C., Sun, L., Long, C.-X., et al. (2018). Genetic Ablation of Dynactin p150(Glued) in Postnatal Neurons Causes Preferential Degeneration of Spinal Motor Neurons in Aged Mice. Mol. Neurodegener. 13 (1), 10. doi:10.1186/s13024-018-0242-z
Yu, L., Chen, Y., and Tooze, S. A. (2018). Autophagy Pathway: Cellular and Molecular Mechanisms. Autophagy 14 (2), 207–215. doi:10.1080/15548627.2017.1378838
Zhang, P., Fan, B., Yang, P., Temirov, J., Messing, J., Kim, H. J., et al. (2019). Chronic Optogenetic Induction of Stress Granules Is Cytotoxic and Reveals the Evolution of ALS-FTD Pathology. ELife 8, e39578. doi:10.7554/eLife.39578
Zhang, T., Baldie, G., Periz, G., and Wang, J. (2014). RNA-processing Protein TDP-43 Regulates FOXO-dependent Protein Quality Control in Stress Response. PLoS Genet. 10 (10), e1004693. doi:10.1371/journal.pgen.1004693
Zhang, X., Chen, S., Song, L., Tang, Y., Shen, Y., Jia, L., et al. (2014). MTOR-independent, Autophagic Enhancer Trehalose Prolongs Motor Neuron Survival and Ameliorates the Autophagic Flux Defect in a Mouse Model of Amyotrophic Lateral Sclerosis. Autophagy 10 (4), 588–602. doi:10.4161/auto.27710
Zhang, X., Li, L., Chen, S., Yang, D., Wang, Y., Zhang, X., et al. (2011). Rapamycin Treatment Augments Motor Neuron Degeneration in SOD1(G93A) Mouse Model of Amyotrophic Lateral Sclerosis. Autophagy 7 (4), 412–425. doi:10.4161/auto.7.4.14541
Zhang, Y., Burberry, A., Wang, J.-Y., Sandoe, J., Ghosh, S., Udeshi, N. D., et al. (2018). The C9orf72-Interacting Protein Smcr8 Is a Negative Regulator of Autoimmunity and Lysosomal Exocytosis. Genes Dev. 32 (13–14), 929–943. doi:10.1101/gad.313932.118
Zhao, J., Brault, J. J., Schild, A., and Goldberg, A. L. (2008). Coordinate Activation of Autophagy and the Proteasome Pathway by FoxO Transcription Factor. Autophagy 4 (3), 378–380. doi:10.4161/auto.5633
Zhao, P., Wong, K. I., Sun, X., Reilly, S. M., Uhm, M., Liao, Z., et al. (2018). TBK1 at the Crossroads of Inflammation and Energy Homeostasis in Adipose Tissue. Cell 172 (4), 731–743.e12. doi:10.1016/j.cell.2018.01.007
Zhao, Y. G., Liu, N., Miao, G., Chen, Y., Zhao, H., and Zhang, H. (2018). The ER Contact Proteins VAPA/B Interact with Multiple Autophagy Proteins to Modulate Autophagosome Biogenesis. Curr. Biol. 28 (8), 1234–1245.e4. doi:10.1016/j.cub.2018.03.002
Zhao, Y., Wang, Z., Zhang, W., and Zhang, L. (2020). Non-coding RNAs Regulate Autophagy Process via Influencing the Expression of Associated Protein. Prog. Biophys. Mol. Biol. 151, 32–39. doi:10.1016/j.pbiomolbio.2019.11.009
Zhou, B., Boudreau, N., Coulber, C., Hammarback, J., and Rabinovitch, M. (1997). Microtubule-associated Protein 1 Light Chain 3 Is a Fibronectin mRNA-binding Protein Linked to mRNA Translation in Lamb Vascular Smooth Muscle Cells. J. Clin. Invest. 100 (12), 3070–3082. doi:10.1172/JCI119862
Zhou, Q.-M., Zhang, J.-J., Li, S., Chen, S., and Le, W.-D. (2017). n-Butylidenephthalide Treatment Prolongs Life Span and Attenuates Motor Neuron Loss in SOD1(G93A) Mouse Model of Amyotrophic Lateral Sclerosis. CNS Neurosci. Ther. 23 (5), 375–385. doi:10.1111/cns.12681
Keywords: autophagy, RNA, amyotrophic lateral sclerosis, frontotemporal dementia, RNA-binding proteins, C9orf72, stress granules, granulophagy
Citation: Houghton OH, Mizielinska S and Gomez-Suaga P (2022) The Interplay Between Autophagy and RNA Homeostasis: Implications for Amyotrophic Lateral Sclerosis and Frontotemporal Dementia. Front. Cell Dev. Biol. 10:838402. doi: 10.3389/fcell.2022.838402
Received: 17 December 2021; Accepted: 14 April 2022;
Published: 28 April 2022.
Edited by:
Olatz Pampliega, University of the Basque Country, SpainReviewed by:
Jared Sterneckert, Technical University Dresden, GermanyBenjamin Wolozin, Boston University School of Medicine, United States
Copyright © 2022 Houghton, Mizielinska and Gomez-Suaga. This is an open-access article distributed under the terms of the Creative Commons Attribution License (CC BY). The use, distribution or reproduction in other forums is permitted, provided the original author(s) and the copyright owner(s) are credited and that the original publication in this journal is cited, in accordance with accepted academic practice. No use, distribution or reproduction is permitted which does not comply with these terms.
*Correspondence: S. Mizielinska, c2FyYWgubWl6aWVsaW5za2FAa2NsLmFjLnVr; P. Gomez-Suaga, cGdvbWV6c3VhZ2FAdW5leC5lcw==