- Research Laboratory on Human Reproduction, Faculté de Médecine, Université libre de Bruxelles, Brussels, Belgium
When mammalian spermatozoa are released in the female reproductive tract, they are incapable of fertilizing the oocyte. They need a prolonged exposure to the alkaline medium of the female genital tract before their flagellum gets hyperactivated and the acrosome reaction can take place, allowing the sperm to interact with the oocyte. Ionic fluxes across the sperm membrane are involved in two essential aspects of capacitation: the increase in intracellular pH and the membrane hyperpolarization. In particular, it has been shown that the SLO3 potassium channel and the sNHE sodium-proton exchanger, two sperm-specific transmembrane proteins, are necessary for the capacitation process to occur. As the SLO3 channel is activated by an increase in intracellular pH and sNHE is activated by hyperpolarization, they act together as a positive feedback system. Mathematical modeling provides a unique tool to capture the essence of a molecular mechanism and can be used to derive insight from the existing data. We have therefore developed a theoretical model formalizing the positive feedback loop between SLO3 and sHNE in mouse epididymal sperm to see if this non-linear interaction can provide the core mechanism explaining the existence of uncapacited and capacitated states. We show that the proposed model can fully explain the switch between the uncapacitated and capacited states and also predicts the existence of a bistable behaviour. Furthermore, our model indicates that SLO3 inhibition, above a certain threshold, can be effective to completely abolish capacitation.
1 Introduction
Despite continuous research in reproductive biology over the last two decades, the prevalence of couple infertility (over 12 months) remains around 15%, among which 30% is due to a male infertility factor (Hajder et al., 2016). The sperm count has been continuously decreasing for 40 years, raising the alarm for a major fertility crisis by the midst of the 21st century and the need for increased research in male infertility (Barratt et al., 2017; Levine et al., 2017; Duffy et al., 2020). Our knowledge of the molecular regulation of sperm motility and its fertilization potential is still incomplete and the etiology of a number of human male infertility cases remains unknown. Therefore, whether in search of new male fertility screening methods or novel contraceptive solutions, a deeper understanding of the molecular events regulating sperm functions is needed. These functions notably depend on ion homeostasis, which is controlled by ion channels and transporters. Many of these proteins or their regulatory subunits are expressed exclusively in sperm cells, making them ideal pharmacological targets (Wang et al., 2021).
Before mammalian spermatozoa are able to fertilize the oocyte, they need to spend some time in the female genital tract. In human this duration must be of several hours while in mouse it is around an hour. During this transit, spermatozoa are exposed to a diversity of environmental and intracellular signals allowing sperm to acquire a special form of motility, known as hyperactivation, and the ability to undergo the acrosome reaction. This process is called capacitation and since its discovery (Austin, 1951; Chang, 1951), in vitro studies of mammalian sperm showed that the presence of albumin and bicarbonate in the physiological incubation medium is essential for capacitation to occur (Lee and Storey, 1986; Stival et al., 2015).
Mammalian sperm capacitation is characterized by an increase in intracellular pH (pHi) (Zeng et al., 1996), membrane hyperpolarization (Arnoult et al., 1999) and a calcium influx from the extracellular medium (Ruknudin and Silver, 1990), and since nearly three decades now, pharmacological and genetic studies have revealed the presence of sperm-specific proteins that are essential for male fertility and necessary for the sperm to reach capacitation: The sNHE sodium-proton exchanger, the SLO3 potassium channel and the CATSPER calcium channel. The absence of any of the corresponding genes leads to male infertility without any systemic abnormality, in accordance with the fact that these proteins are expressed only in the sperm (Ren et al., 2001; Wang et al., 2003; Santi et al., 2010).
The calcium influx, increase in pHi and hyperpolarization observed during capacitation are dependent on the activity of CATSPER, sNHE and SLO3 (Carlson et al., 2003; Wang et al., 2003; Santi et al., 2010), and these actors operate all together as CATSPER and SLO3 are activated by an elevation of pHi and sNHE is activated by hyperpolarization (Schreiber et al., 1998; Kirichok et al., 2006; Windler et al., 2018). These considerations led Chávez et al. (2014) to the proposal of the existence of a positive feedback loop between the activation of sNHE and SLO3, leading to a high pHi and membrane hyperpolarization, that could promote the pHi-dependent CATSPER’s activity during capacitation.
In order to test this hypothesis and find if capacitation can indeed be controlled by the feedback loop between sNHE and SLO3, we propose a mathematical model for the capacitation process based on these two essential molecular actors of capacitation. The model also includes the known effect of PKA-dependent phosphorylation on SLO3, and even though the capacitation process includes other regulatory changes, the focus is here set on a minimal number of actors that could be at the core of a capacitation switch. Using this minimal model we then investigate the conditions necessary for the incubation medium to promote capacitation and ask whether this process of capacitation can be reversed back. Finally we also investigate the most effective way of preventing capacitation by inhibition of sNHE and SLO3.
2 Materials and Methods
Our model is a minimal two variables model describing the evolution in time of the intracellular pH (pHi) and the transmembrane electrical potential (Vm) of a mouse epididymal spermatozoon, which includes the feedback between the increase in pHi and hyperpolarization resulting from the activations of sNHE and SLO3.
The first Eq. 1 links the evolution of pHi to the different mechanisms related to proton homeostasis (Figure 1A), where the β factor is the total pHi buffer capacity of the sperm cell. The second Eq. 2 is based on the conservation of the electrical charge and shows that the evolution of the transmembrane potential depends on the contributions of SLO3 and a leak current. The factor Cm is the membrane’s capacitance of the sperm cell. All the actors taken into account in the two equations of the model are schematized on Figure 1A.
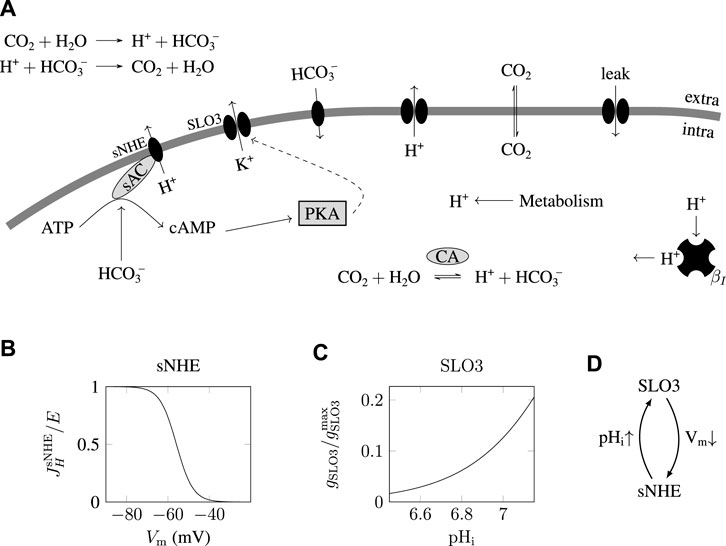
FIGURE 1. The feedback loop regulating capacitation. (A) The model takes into account the potassium channel SLO3 and the sodium-proton exchanger sNHE. SLO3 is activated downstream of cAMP production through the activation of PKA. The cAMP production by the soluble adenylyl cyclase is activated by the presence of bicarbonate (HCO3−). A leak current, regrouping all transmembrane electrical currents except through SLO3, is taken into account. HCO3− passes through the plasma membrane by a variety of transporters. The carbon dioxyde (CO2) freely and instantly crosses the cell membrane, and equilibrates with HCO3− and H+. An influx of protons resulting from the metabolism is taken into account and the intracellular pH varies due to a leak of protons through the plasma membrane. Finally the total intrinsic protons buffer βI is present. (B) sNHE activation curve against Vm. Hyperpolarization activates sNHE. In this graph, the intracellular cAMP concentration, which influences the half activation voltage, is fixed at 1 mM. The y-axis label denominator E is the maximal activity of sNHE. (C) SLO3 activation against pHi. Intracellular alkalization activates SLO3. In this graph the transmembrane potential Vm is fixed at +80 mV and the phosphorylation level PSLO3 is kept at 0 (unincubated spermatozoa). The y-axis label denominator
The first term on the right side of Eq. 1 represents the contribution of the sperm-specific sodium-proton exchanger sNHE. This contribution is always positive as the extrusion of protons has the effect of increasing the pHi. This transporter has a putative voltage sensor (Wang et al., 2003) and is activated by hyperpolarization in sea urchin sperm (Windler et al., 2018) (Figure 1B). Moreover, the half maximal activation’s voltage of sNHE,
The constant
In our model, the soluble adenylyl cyclase activation is controlled directly by the intracellular bicarbonate concentration. Intracellular calcium concentration is also known to activate this adenylyl cyclase (Litvin et al., 2003). As a first approximation, our model indirectly includes this effect of sAC’s activation by calcium through
The maximal activity of sNHE (EsNHE),
The other contributions to the variation in pHi of the sperm cell are the following:
Firstly the contribution from the flux of bicarbonates,
Secondly, a term of passive flux of protons across the membrane
The next contribution to the variations in pHi comes from the effect of changes in extracellular carbon dioxide concentration which are instantly followed by the same intracellular carbon dioxide concentration changes as the permeability of the plasma membrane to CO2 is high. So in the model, the CO2 concentration is the same on both sides of the sperm membrane and an increase CO2 results in acidification of the cell as the CO2 is converted to bicarbonates and protons. This intracellular equilibration between carbon dioxide and bicarbonate is fast as the carbonic anhydrases [CAII, Wandernoth et al. (2015)] catalyse the reversible reaction, as schematized in Figure 1A. In the extracellular medium, as the incubation medium is devoid of carbonic anhydrases as it is usually the case in vitro incubations, the equilibration should be considered not instantaneous, but for simplicity we do not include this delay in our model. We nevertheless carried out simulations using explicitly the reaction rates of the conversion of CO2 into protons and checked that the results and conclusions presented in this paper are not different (data not shown).
The last contribution to the variations in pHi is the acid loading resulting from the metabolism, taken as a constant. This acid loading, which allows the sperm to reach an equilibrium state by compensating the pHi increase due to the protons’ extruders, includes the contribution of the leak of protons from the acrosome which is an acidic organelle that has been shown to alkalize during capacitation (Nakanishi et al., 2001). The value of this parameter is adjusted so that the pHi of the sperm cell in uncapacitating conditions lies in the range of the experimental data of the literature, between 6.4 and 6.85 (Zeng et al., 1996; Carlson et al., 2007; Chávez et al., 2019).
The first term on the right side of Eq. 2 is the contribution of SLO3, considered with its auxiliary subunit LRRC52 (Yang et al., 2011), to the transmembrane electrical potential. It is activated by alkalization as shown by the Hill’s [H+]i dependence of its conductance. The H+i concentration for half occupation of SLO3 (written
The factor
where PSLO3 is the phosphorylation level of SLO3 and is chosen as a Hill equation. The pathway to SLO3 phosphorylation is initiated by the activation of the soluble adenylyl cyclase (sAC) from an increase in [HCO3−]i, which is followed by the activation of the protein kinase A (PKA) and finally leads to the auto-phosphorylation of the cSrc kinase (Stival et al., 2015) which then phosphorylates tyrosine residues of SLO3. In fact, the phosphorylation of SLO3 is slow, of a timescale of 15 min (Stival et al., 2015), but we do not include this delay in our equations because we will focus on the results concerning the steady states of the sperm cell that are reached during incubations in various media. The half maximal concentration
where the Boltzmann activation parameters were measured by Zeng et al. (2015) with electrophysiological techniques on spermatozoa at a pHi of 8. This way of modeling the voltage activation of SLO3 independently of the intracellular pH value is based on experimental activation curves obtained by Yang et al. (2011) for various membrane voltages that indicate a pHi-independent voltage activation’s behavior.
The second term on the right of Eq. 2 is regrouping into a leak current the contribution from all other electrogenic transporters of the sperm cell membrane, where the resultant leak conductance gl is a constant (the value of which is described in Supplementary Appendix). This choice of taking solely SLO3 as the effector of Vm changes during capacitation is due to the fact that we are considering the core model of a feedback loop in order to build a minimal model of capacitation. This choice is supported by the demonstration that the variation in SLO3’s conductance is responsible for the hyperpolarization of the sperm cell during capacitation (Santi et al., 2010; Chávez et al., 2013). The value of Vleak is set at −40 mV according to the transmembrane voltage obtained by Chávez et al. (2013) for SLO3’s knock-out and SLO3’s inhibited mouse spermatozoa, both giving a value around −40 mV.
Finally, it could be objected that because the Na+/HCO3− cotransporter has been suggested to induce hyperpolarization upon addition of bicarbonate (Demarco et al., 2003), the model should include this contribution explicitly. In our approach, this effect was not taken into account as this instantaneous hyperpolarization seems to be transient and disappears in minutes (Demarco et al., 2003; Santi et al., 2010; De La Vega-Beltran et al., 2012; Chávez et al., 2013; Escoffier et al., 2015; Stival et al., 2015). Yet we do not exclude the participation of the cotransporter in the subsequent capacitation-related hyperpolarization as its activity is voltage-dependent. The model could be refined by including such voltage-dependent contributions as the Na+/HCO3− cotransporter or the CFTR channel among others.
With this minimal model built around the two actors SLO3 and sNHE, we made simulations of the time evolution of the state of a sperm cell in order to check if it gives account of the capacitation process and if a positive feedback between sNHE and SLO3 could be at the core of the process. We also simulated the effects of the inhibition of SLO3 or sNHE on the state of the sperm.
The simulations, using the evolution Eqs 1, 2, were performed using the software XPPAUT 6.11 (Free Software Foundation Inc., Cambridge, United States). In all simulations, the extracellular pH (pHe) is fixed at the value of 7.4 and the temperature is set at 37°C, as usually the case in sperm incubating media. Original source code is available on GitHub1.
3 Results
3.1 The Positive Feedback Between SLO3 and sNHE Causes Capacitation
In order to find if the model reproduces the transition from a depolarized acidic state (not promoting capacitation) to a hyperpolarized alkaline state (promoting capacitation, or “capacitating”) of the sperm, we performed a simulation of the incubation of a sperm cell as it is usually done in the laboratory, consisting of the incubation into a solution containing 15 mM bicarbonate ([HCO3−]e = 15 mM).
This protocol is represented on the top graph of Figure 2A showing a step of 15 mM of bicarbonate at time t = 2 min. The initial concentration of bicarbonate in the medium is set at [HCO3−]e = 0.2 mM, which corresponds to a solution of pH = 7.4 at equilibrium with ambient atmosphere. As indicated in the graphs of the pHi(t) and Vm(t) on Figure 2A, before the bicarbonate step (t
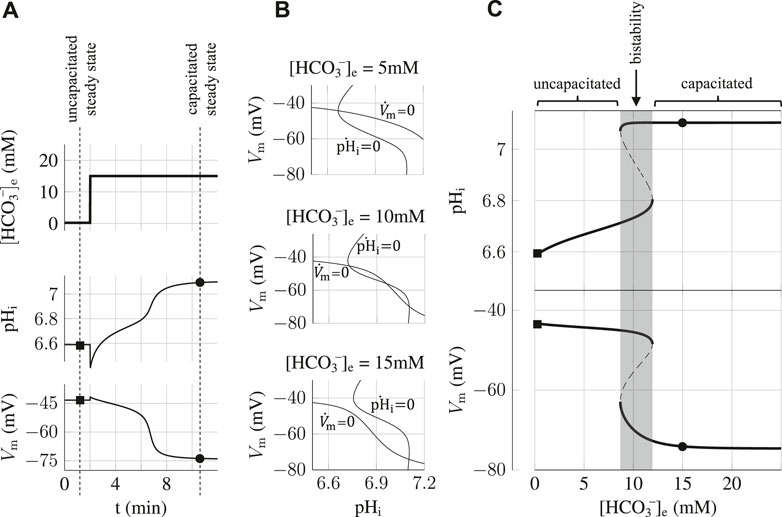
FIGURE 2. Transition between uncapacitated and capacitated state as the bicarbonate concentration is increased. (A) Time evolution of the pHi and Vm following a step from 0.2 to 15 mM extracellular bicarbonate
This simulation of the time evolution of the state of the sperm therefore shows two steady states, one at 0.2 mM HCO3−e and one at 15 mM HCO3−e, which are reported on the two diagrams of Figure 2C (black squares and bullets), one corresponding to the uncapacitated state of the sperm and one corresponding to the capacitating state of the sperm.
3.2 Bistability of the Capacitation Process
Having found two steady states of the sperm, one at 0.2 mM HCO3−e and one at 15mM HCO3−e, we wondered if these sperm states were the only possible ones for each of these extracellular bicarbonate concentration, and whether there is a threshold in [HCO3−]e above which the uncapacitated sperm will spontaneously shift to the capacitating state of low Vm and high pHi. As a positive feedback loop can give rise to bistability we can expect that for some values of the parameter [HCO3−]e we would find two possible stable states, one capacitating and one uncapacitating.
The top graph of Figure 2B shows that when [HCO3−]e = 5 mM there is only one possible steady state for the sperm. When [HCO3−]e = 15 mM (bottom graph of Figure 2B), there is also only one steady state, at the point (pHi,Vm) ≃(7.1,−75 mV). This latter state is hyperpolarized and alkaline, and so corresponds to a sperm’s capacitating state, as the one found in Figure 2A where [HCO3−]e = 15 mM.
Between these two bicarbonate concentrations of 5 and 15 mM however, at [HCO3−]e = 10 mM we find three intersections of the nullclines, which are shown in the middle graph of Figure 2B, and indicating that the sperm can be found in three different steady states, one of these three states corresponding to a capacitating state of the sperm at Vm ≃−70 mV and pHi ≃7.1. In order to gain insight of what is happening here we computed the steady states for all values of [HCO3−]e and plotted these steady states in two diagrams shown on Figure 2C, where we observe that a transition between uncapacitating states and capacitating states happens when the extracellular bicarbonate concentration crosses a threshold at around 12 mM HCO3−e. Below 8 mM HCO3−e spermatozoa are in uncapacitating states and above 12 mM HCO3−e are found the capacitating states of the sperm, and between these two appears a region (shaded in the figure) where three steady states of the sperm coexist. The analysis of the dynamics of the system shows that the steady states in the middle (dashed lines in Figure 2C) are unstable; in reality the sperm will never be found at such steady states because any imperceptible fluctuation in the physiological conditions brings the sperm state away from these unstable steady states. Therefore, in the shaded region, the sperm can be found in two different steady states; it is a phenomenon of bistability where the state of the spermatozoa will depend on what happened before they were brought to this bistable region. Indeed, if we start on the left of the diagram where [HCO3−]e is low, and increase slowly the medium’s bicarbonate concentration to the bistable region, the sperm will be found at the state (pHi,Vm) ≃(6.7,−45 mV), but if the sperm is prepared on the right of the diagram, decreasing the [HCO3−]e to the gray region will keep the sperm at the capacitating state near (7.1,−70 mV).
3.3 The Capacitation Switch has Hysteresis yet is Reversible
The bistability arising from the feedback loop between sNHE and SLO3 implies that the sperm will chose one of the two stable states according to its initial state. This hysteresis phenomenon implies that once the sperm has chosen one of the two stable states, it will remain locked in this state regardless of small changes in the surrounding physiological conditions.
That is what we show here with a simulation of an experiment where a hysteresis effect resulting from this bistability arises in the state of the sperm. The simulation, shown on Figure 3, consists in preparing the sperm cell in the bistability region at 10 mM HCO3−e before imposing a pulse of 15 mM HCO3−e that brings the sperm to a capacitating state. We find that the capacitating state is robust as the sperm remains in the hyperpolarized high pHi state when the bicarbonate concentration is brought back to its initial value of 10 mM after the pulse. This is a hysteresis effect and it shows that once the sperm cell is in the capacitating state, it is blocked in this state regardless of small variations in the physiological conditions surrounding the sperm cell. We find however that this switch is reversible as a sufficient depletion of [HCO3−]e for some time is able to bring the sperm back to the uncapacitating state.
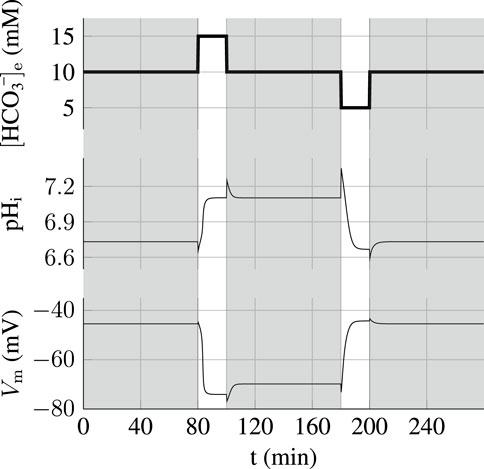
FIGURE 3. Hysteresis and reversibility of the capacitation process. After having prepared the sperm at 10 mM
We would like to insist here that a capacitating state does not mean a capacitated state, we are rather assessing the intracellular ionic state consistent with sperm incubated in capacitating conditions.
3.4 SLO3’s inhibition is More Robust Than sNHE at Preventing Capacitation
The proposed minimal model can also be used as a tool in order to predict how the capacitation process can be blocked efficiently. We simulated the time evolution of the sperm’s states when either SLO3 or sNHE is inhibited and have examined the transition between the uncapacitating and capacitating states. The result of the simulation of the incubation of sperm in 15 mM HCO3−e when SLO3 is inhibited at a level of 70% is presented on Figure 4A and shows that capacitation can not occur anymore; the sperm does not hyperpolarize and the intracellular pH shows an increase of only 0.2 unit.
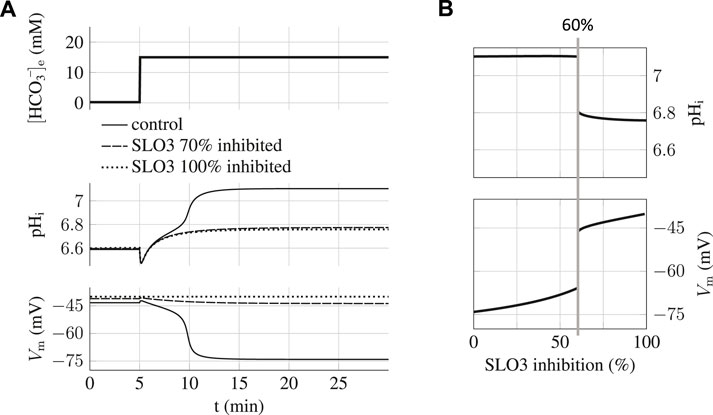
FIGURE 4. Capacitation dependence on SLO3 inhibition. (A) When SLO3 is 70% inhibited, the transition to the capacitated state does not occur and the state is blocked at the values of pHi = 6.8 and Vm = −45 mV. In the case of 100% inhibition of SLO3, the model shows no variation in Vm during capacitation. (B) Graph of the steady states of the sperm against the percentage of SLO3 inhibition. These are the steady states reached after the incubation in capacitating medium (15 mM bicarbonate) of spermatozoa previously prepared at 0.2 mM bicarbonate. A threshold appears at 60% inhibition above which both the increases in pHi and hyperpolarization are much reduced.
By carrying on the same simulations with different inhibition percentages of SLO3 we find a threshold at 60% above which this ions’ fluxes aspect of capacitation is prevented (Figure 4B); the pHi will increase no more than 0.2 unit, from 6.6 to less than 6.8, and the transmembrane potential will not hyperpolarize below −45 mV. The existence of this inhibition threshold above which the sperm does not capacitate anymore is due to the fact that the system is brought into the bistable region and will therefore remain blocked to the uncapacitated state during incubation.
When simulating an incubation at the same concentration of 15 mM HCO3−e but with 70% inhibition of sNHE, the effect on capacitation is similar to the SLO3 inhibition (Supplementary Figure S1), but when increasing the HCO3−e concentration of the incubation medium we find that the sNHE inhibition is not as effective at preventing the capacitation switch as the inhibition of SLO3. This is illustrated on Figure 5 showing the states of incubated spermatozoa as a function of the [HCO3−]e at a fixed 95% inhibition. The SLO3 inhibition keeps the state depolarized for all bicarbonate concentrations (Figure 5A) but in contrast, when [HCO3−]e crosses the threshold of 20mM, the capacitation switch is not prevented by a 95% sNHE inhibition (Figure 5B). The prevention of the hyperpolarization in the case of the SLO3’s inhibition is due to the fact that its conductance remains small compared with the leak conductance.
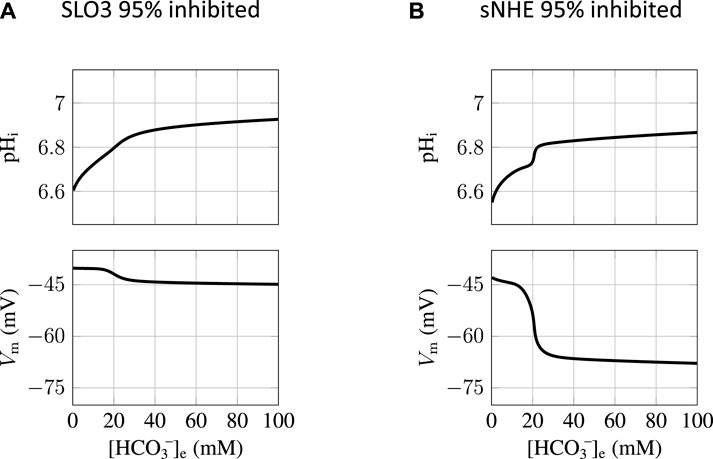
FIGURE 5. SLO3 inhibition is more robust than sNHE at preventing capacitation. (A) When SLO3 is 95% inhibited, the switch to the capacitated sperm does not occur for any HCO3−e concentration. (B) When sNHE is 95% inhibited, the capacitation switch is not prevented when the HCO3−e concentration exceeds 20 mM.
4 Discussion
In this work we have built a minimal model for the ions fluxes aspect of capacitation of mouse spermatozoa as a function of the incubating medium’s bicarbonate concentration. We modeled the sperm’s state evolution on the minimal basis of the effect of sNHE and SLO3 on pHi and Vm. By doing so, for the homeostasis of sperm pHi we included, in addition to sNHE and SLO3, the alkalizing effect of bicarbonate transporters and the acidifying effect of the metabolism of the sperm cell.
The majority of the parameters values used in the model are based on existing data, with the exception of three which remain free. These free parameters are the acid loading from the metabolism and the bicarbonate concentration for downstream half activation of sNHE or SLO3. Nevertheless, the acid loading from the metabolism, which compensates the alkalizing effects of the protons and bicarbonate transporters in order to allow pH homeostasis, is inherently physiological as the alkalizing parameters are physiological.
Concerning the half activation of sNHE and SLO3 downstream of the protein kinase A activation by bicarbonate, our parameter’s values are taken consistently with results from Stival et al. (2015) giving the percentage of hyperpolarized spermatozoa as a function of the HCO3−e concentration, which indicates a threshold below 7 mM HCO3−e above which a substantial proportion of spermatozoa do hyperpolarize during the incubation. However, the exact value of these two parameters do not affect the essence of the feedback loop and bistability in our model; it rather shifts the shaded bistability region (Figure 2C) to the right or to the left. In particular, decreasing
Our minimal model gives account of the experimentally observed hyperpolarization and alkalization of the mouse sperm’s cell during capacitation. Indeed the hyperpolarization of the sperm’s cell to −75 mV lies in the range (Vm<70 mV) found in the literature for capacitated spermatozoa (Arnoult et al., 1999; Escoffier et al., 2015). We emphasize here that our results concern individual spermatozoa that effectively capacitate, and not a “capacitated population” of spermatozoa that consists of capacitated and uncapacitated subpopulations for which the measured hyperpolarization represents the sum of the depolarized uncapacitated subpopulation and the hyperpolarized capacitated subpopulation. In such heterogenous populations, the measured hyperpolarization is around −60 mV (Arnoult et al., 1999; Stival et al., 2015).
Concerning the evolution of the sperm’s pHi during capacitation, our model shows an increase in pHi from 6.6 before incubation to 7.1 after incubation. The uncapacitated value given by the model was in fact adjusted to the value of 6.6 obtained by Chávez et al. (2019), and the capacitated value of 7.1 is in the range around 7.2 obtained recently by Ferreira et al. (2021). However, we would like to stress here that our model’s pHi values of 6.6 before capacitation and 7.1 after capacitation are not fixed and do depend on the exact value of the acid load from the metabolism which is a free parameters as mentioned here above.
Our analysis of the sperm’s steady states as a function of the bicarbonate concentration in the incubating medium showed the existence of a bistability region where the sperm can be found in two different states and we showed that once the sperm has reached the hyperpolarized and alkaline state of capacitated sperm, it will remain capacitated regardless of small fluctuations in the bicarbonate concentration. In addition, we show that this hysteresis phenomenon in the cellular response allows the encoding of transient signals, like a pulse in the external bicarbonate concentration, by long-lasting changes both in pHi and Vm. This capacitation switch can be reversed back as a sufficiently strong decrease in [HCO3−]e will bring the sperm back to an uncapacitating state. The existence of this hysteresis phenomenon resulting in a capacitation threshold which is different from the decapacitation threshold is a prediction of the model that can be tested experimentally. On a functional level, the predicted hysteresis, inducing a shift in bicarbonate sensitivity, allows the capacitation process to be more robust in presence of local variations of bicarbonate concentration. This ensures that, once capacitated, sperm cells are less sensitive to these fluctuations, providing a possible explanation for how sperm persist capacitating even when they travel through various parts the female genital tract.
The reversibility of capacitation is still a matter of debate. Even though the reversibility of capacitation could be abolished by other factors not included in the model, such as the degradation of Catsper (Ded et al., 2020), several aspects of capacitation have reversible characteristics. Indeed, various studies indicate that the responses of the sperm state to stimuli such as pulses in bicarbonate concentration, intracellular pH, intracellular calcium concentration, alkaline depolarization, and epididymal extract addition have reversible characteristics (Oliphant and Brackett, 1973; Babcock and Pfeiffer, 1987; Suarez et al., 1987; Espinosa and Darszon, 1995; Schreiber et al., 1998; Wennemuth et al., 2003). We nevertheless insist that the reversibility predicted by the present mathematical model holds for the switch between the acidic depolarized state and the alkaline hyperpolarized state of the sperm, and not for the whole process of capacitation.
Concerningthe inhibition of capacitation, when the sperm is incubated in a 15 mM bicarbonate solution we find a switch at 60% inhibition of SLO3 above which capacitation is prevented. Even though the inhibition of sNHE has similar effect on capacitation in an incubation medium of 15 mM bicarbonate, we find that inhibiting SLO3 is more effective than inhibiting sNHE when the bicarbonate concentration is increased. Indeed, a 95% inhibition of SLO3 prevents capacitation for all possible bicarbonate concentrations that could be found in the genital tract of the mammals (Maas et al., 1977), which is not the case when instead sNHE is 95% inhibited. This stronger effect of SLO3 inhibition on capacitation is not surprising as we may expect inhibition of ion channels to have a much larger impact on membrane potential than the inhibition of a carrier system like sNHE, which has a much lower turnover rate for ion transport. It appears therefore that the inhibition of SLO3 is a better candidate for the development of non-hormonal male contraception. The human sperm however is different from the mouse as hSLO3 is strongly activated by calcium and less by pH (Brenker et al., 2014). Our model could be adapted and applied to human sperm by adjusting the activation curves of SLO3 and taking into account the voltage-gated hydrogen channel 1, HV1, which has been shown to be the dominant proton conductance in human sperm (Lishko et al., 2010). However, as this transporter is not activated by hyperpolarization as it is the case for sNHE, another feedback loop could take place, between hSLO3 and Catsper, as it has been shown that the variations in hyperpolarization and intracellular calcium concentration of human spermatozoa are interconnected during capacitation (Balestrini et al., 2021).
The proposed minimal model has allowed us to identify the core molecular mechanism which is likely to control murine sperm capacitation. It may be extended to include calcium dynamics and further refined to the human case. Concerning the increase in intracellular calcium during capacitation, the feedback loop between sNHE and SLO3, which robustly switches the state of the sperm to an elevated intracellular pH, is likely to keep the pHi-sensitive Catsper channel activated during capacitation, and moreover, the reversibility of the switch implies that this capacitation’s aspect of calcium influx can be reversed back. In addition, as in a first approximation we have modeled the soluble adenylyl cyclase activity only through the increase in intracellular bicarbonate concentration and not by explicit increases in intracellular calcium concentration which are known to activate this adenylyl cyclase (Litvin et al., 2003), an extended version of the model, explicitly including Catsper, could take into account this additional feedback. In this field, a first mathematical model of mouse sperm intracellular calcium dynamics has been established by Olson et al. (2010). In their model, they explain the tail to head Ca2+ signal propagation following the activation of Catsper, as well as the subsequent sustained calcium increase in the sperm’s head. To address this, they take into account the Ca2+ release from the redundant nuclear envelope which was modeled to be indirectly gated by variations in intracellular Ca2+ concentration through the production of inositol 1,4,5-trisphosphate from the phospholipase C. Recently, the inclusion of Catsper into a more complex mathematical model of mouse sperm capacitation has been carried out by Aguado-García et al. (2021) in a comprehensive study of early steps of capacitation. Interestingly, this latter study predicts that if capacitation is impaired by in silico SLO3’s loss of function, it can not be recovered by overactivation of sNHE. On the contrary, they show that the overactivation of SLO3 can recover the capacitation response of sNHE’s loss of function. This result is now corroborated by our modeling study, highlighting that SLO3 is essential for the capacitation process. Yet we emphasize here again that our model describes the states of the sperm reached after long durations of incubations.
To conclude, using a modeling approach, we have identified a possible core molecular mechanism underlying the transition between the uncapacitated and capacitated state in mouse sperm and studied its dynamic as well as identified pharmacological targets regulating this process. These results may be relevant in the possible development of treatments of male infertility and non-hormonal male contraception, as the inhibition or stimulation of these actors of sperm’s capacitation can modulate the ability of sperm to fertilize the egg. For this, the proposed model should be extended to the human case.
Such a minimal model gives direction for further experimental studies aimed at understanding various aspects of sperm dynamics, and moreover, additional phosphorylation and transport mechanisms of interest could be studied by adding explicitly their contribution to this model.
Data Availability Statement
Publicly available datasets were analyzed in this study. This data can be found here: https://github.com/bdeprelle/MouseCapaModel.
Author Contributions
BP, PL, and DG designed the research, carried out the simulations and wrote the article.
Funding
This work was supported by the Université libre de Bruxelles and the Fund for Scientific Research (F.R.S.-FNRS).
Conflict of Interest
The authors declare that the research was conducted in the absence of any commercial or financial relationships that could be construed as a potential conflict of interest.
Publisher’s Note
All claims expressed in this article are solely those of the authors and do not necessarily represent those of their affiliated organizations, or those of the publisher, the editors and the reviewers. Any product that may be evaluated in this article, or claim that may be made by its manufacturer, is not guaranteed or endorsed by the publisher.
Acknowledgments
We thank Genevieve Dupont and Celia M. Santi for their remarks and suggestions during the construction of the model.
Supplementary Material
The Supplementary Material for this article can be found online at: https://www.frontiersin.org/articles/10.3389/fcell.2022.835594/full#supplementary-material
Footnotes
1https://github.com/bdeprelle/MouseCapaModel
References
Aguado-García, A., Priego-Espinosa, D. A., Aldana, A., Darszon, A., and Martínez-Mekler, G. (2021). Mathematical Model Reveals that Heterogeneity in the Number of Ion Transporters Regulates the Fraction of Mouse Sperm Capacitation. PLoS One 16, e0245816. doi:10.1371/journal.pone.0245816
Arnoult, C., Kazam, I. G., Visconti, P. E., Kopf, G. S., Villaz, M., and Florman, H. M. (1999). Control of the Low Voltage-Activated Calcium Channel of Mouse Sperm by Egg ZP3 and by Membrane Hyperpolarization during Capacitation. Proc. Natl. Acad. Sci. 96, 6757–6762. doi:10.1073/pnas.96.12.6757
Austin, C. (1951). Observations on the Penetration of the Sperm into the Mammalian Egg. Aust. Jnl. Bio. Sci. 4, 581–596. doi:10.1071/bi9510581
Babcock, D. F., and Pfeiffer, D. R. (1987). Independent Elevation of Cytosolic [Ca2+] and pH of Mammalian Sperm by Voltage-dependent and pH-Sensitive Mechanisms. J. Biol. Chem. 262, 15041–15047. doi:10.1016/s0021-9258(18)48135-5
Balestrini, P. A., Sanchez‐Cardenas, C., Luque, G. M., Baro Graf, C., Sierra, J. M., Hernández‐Cruz, A., et al. (2021). Membrane Hyperpolarization Abolishes Calcium Oscillations that Prevent Induced Acrosomal Exocytosis in Human Sperm. FASEB j. 35, e21478. doi:10.1096/fj.202002333RR
Barratt, C. L. R., Björndahl, L., De Jonge, C. J., Lamb, D. J., Osorio Martini, F., McLachlan, R., et al. (2017). The Diagnosis of Male Infertility: an Analysis of the Evidence to Support the Development of Global WHO Guidance-Challenges and Future Research Opportunities. Hum. Reprod. Update 23, 660–680. doi:10.1093/humupd/dmx021
Boron, W. F. (2004). Regulation of Intracellular pH. Adv. Physiol. Educ. 28, 160–179. doi:10.1152/advan.00045.2004
Brenker, C., Zhou, Y., Müller, A., Echeverry, F. A., Trötschel, C., Poetsch, A., et al. (2014). The Ca2+-Activated K+ Current of Human Sperm Is Mediated by Slo3. Elife 3, e01438. doi:10.7554/eLife.01438
Carlson, A. E., Hille, B., and Babcock, D. F. (2007). External Ca2+ Acts Upstream of Adenylyl Cyclase SACY in the Bicarbonate Signaled Activation of Sperm Motility. Dev. Biol. 312, 183–192. doi:10.1016/j.ydbio.2007.09.017
Carlson, A. E., Westenbroek, R. E., Quill, T., Ren, D., Clapham, D. E., Hille, B., et al. (2003). CatSper1 Required for Evoked Ca2+ Entry and Control of Flagellar Function in Sperm. Proc. Natl. Acad. Sci. 100, 14864–14868. doi:10.1073/pnas.2536658100
Chang, M. C. (1951). Fertilizing Capacity of Spermatozoa Deposited into the Fallopian Tubes. Nature 168, 697–698. doi:10.1038/168697b0
Chávez, J. C., Darszon, A., Treviño, C. L., and Nishigaki, T. (2019). Quantitative Intracellular pH Determinations in Single Live Mammalian Spermatozoa Using the Ratiometric Dye SNARF-5F. Front. Cel Dev. Biol. 7, 366. doi:10.3389/fcell.2019.00366
Chávez, J. C., de la Vega-Beltrán, J. L., Escoffier, J., Visconti, P. E., Treviño, C. L., Darszon, A., et al. (2013). Ion Permeabilities in Mouse Sperm Reveal an External Trigger for SLO3-dependent Hyperpolarization. PLoS One 8, e60578. doi:10.1371/journal.pone.0060578
Chávez, J. C., Ferreira, J. J., Butler, A., De La Vega Beltrán, J. L., Treviño, C. L., Darszon, A., et al. (2014). SLO3 K+ Channels Control Calcium Entry through CATSPER Channels in Sperm. J. Biol. Chem. 289, 32266–32275. doi:10.1074/jbc.M114.607556
Chávez, J. C., Hernández-González, E. O., Wertheimer, E., Visconti, P. E., Darszon, A., and Treviño, C. L. (2012). Participation of the Cl−/HCO3− Exchangers SLC26A3 and SLC26A6, the Cl− Channel CFTR, and the Regulatory Factor SLC9A3R1 in Mouse Sperm Capacitation1. Biol. Reprod. 86, 1–14. doi:10.1095/biolreprod.111.094037
De La Vega-Beltran, J. L., Sánchez-Cárdenas, C., Krapf, D., Hernandez-González, E. O., Wertheimer, E., Treviño, C. L., et al. (2012). Mouse Sperm Membrane Potential Hyperpolarization Is Necessary and Sufficient to Prepare Sperm for the Acrosome Reaction. J. Biol. Chem. 287, 44384–44393. doi:10.1074/jbc.M112.393488
Ded, L., Hwang, J. Y., Miki, K., Shi, H. F., and Chung, J.-J. (2020). 3D In Situ Imaging of the Female Reproductive Tract Reveals Molecular Signatures of Fertilizing Spermatozoa in Mice. Elife 9, e62043. doi:10.7554/eLife.62043
Demarco, I. A., Espinosa, F., Edwards, J., Sosnik, J., de la Vega-Beltrán, J. L., Hockensmith, J. W., et al. (2003). Involvement of a Na+/HCO 3−Cotransporter in Mouse Sperm Capacitation Cotransporter in Mouse Sperm Capacitation. J. Biol. Chem. 278, 7001–7009. doi:10.1074/jbc.M206284200
Duffy, J. M. N., Adamson, G. D., Benson, E., Bhattacharya, S., Bhattacharya, S., Bofill, M., et al. (2020). Top 10 Priorities for Future Infertility Research: an International Consensus Development Study. Hum. Reprod. 35, 2715–2724. doi:10.1093/humrep/deaa242
Escoffier, J., Krapf, D., Navarrete, F., Darszon, A., and Visconti, P. E. (2012). Flow Cytometry Analysis Reveals a Decrease in Intracellular Sodium during Sperm Capacitation. J. Cel Sci 125, 473–485. doi:10.1242/jcs.093344
Escoffier, J., Navarrete, F., Haddad, D., Santi, C. M., Darszon, A., and Visconti, P. E. (2015). Flow Cytometry Analysis Reveals that Only a Subpopulation of Mouse Sperm Undergoes Hyperpolarization during Capacitation1. Biol. Reprod. 92, 121. doi:10.1095/biolreprod.114.127266
Espinosa, F., and Darszon, A. (1995). Mouse Sperm Membrane Potential: Changes Induced by Ca2+. FEBS Lett. 372, 119–125. doi:10.1016/0014-5793(95)00962-9
Ferreira, J. J., Lybaert, P., Puga-Molina, L. C., and Santi, C. M. (2021). Conserved Mechanism of Bicarbonate-Induced Sensitization of Catsper Channels in Human and Mouse Sperm. Front. Cel Dev. Biol. 9, 733653. doi:10.3389/fcell.2021.733653
Garbers, D. L., Tubb, D. J., and Hyne, R. V. (1982). A Requirement of Bicarbonate for Ca2+-Induced Elevations of Cyclic AMP in guinea Pig Spermatozoa. J. Biol. Chem. 257, 8980–8984. doi:10.1016/s0021-9258(18)34229-7
Hajder, M., Hajder, E., and Husic, A. (2016). The Effects of Total Motile Sperm Count on Spontaneous Pregnancy Rate and Pregnancy after Iui Treatment in Couples with Male Factor and Unexplained Infertility. Med. Arh 70, 39–43. doi:10.5455/medarh.2016.70.39-43
Hernández-González, E. O., Treviño, C. L., Castellano, L. E., de la Vega-Beltrán, J. L., Ocampo, A. Y., Wertheimer, E., et al. (2007). Involvement of Cystic Fibrosis Transmembrane Conductance Regulator in Mouse Sperm Capacitation. J. Biol. Chem. 282, 24397–24406. doi:10.1074/jbc.M701603200
Jansen, V., Alvarez, L., Balbach, M., Strünker, T., Hegemann, P., Kaupp, U. B., et al. (2015). Controlling Fertilization and cAMP Signaling in Sperm by Optogenetics. Elife 4, e05161. doi:10.7554/eLife.05161
Kirichok, Y., Navarro, B., and Clapham, D. E. (2006). Whole-cell Patch-Clamp Measurements of Spermatozoa Reveal an Alkaline-Activated Ca2+ Channel. Nature 439, 737–740. doi:10.1038/nature04417
Lee, M. A., and Storey, B. T. (1986). Bicarbonate Is Essential for Fertilization of Mouse Eggs: Mouse Sperm Require it to Undergo the Acrosome Reaction. Biol. Reprod. 34, 349–356. doi:10.1095/biolreprod34.2.349
Levine, H., Jørgensen, N., Martino-Andrade, A., Mendiola, J., Weksler-Derri, D., Mindlis, I., et al. (2017). Temporal Trends in Sperm Count: a Systematic Review and Meta-Regression Analysis. Hum. Reprod. Update 23, 646–659. doi:10.1093/humupd/dmx022
Lishko, P. V., Botchkina, I. L., Fedorenko, A., and Kirichok, Y. (2010). Acid Extrusion from Human Spermatozoa Is Mediated by Flagellar Voltage-Gated Proton Channel. Cell 140, 327–337. doi:10.1016/j.cell.2009.12.053
Litvin, T. N., Kamenetsky, M., Zarifyan, A., Buck, J., and Levin, L. R. (2003). Kinetic Properties of "Soluble" Adenylyl Cyclase. J. Biol. Chem. 278, 15922–15926. doi:10.1074/jbc.M212475200
Luque, G. M., Dalotto‐Moreno, T., Martín‐Hidalgo, D., Ritagliati, C., Puga Molina, L. C., Romarowski, A., et al. (2018). Only a Subpopulation of Mouse Sperm Displays a Rapid Increase in Intracellular Calcium during Capacitation. J. Cel Physiol 233, 9685–9700. doi:10.1002/jcp.26883
Maas, D. H. A., Storey, B. T., and Mastroianni, L. (1977). Hydrogen Ion and Carbon Dioxide Content of the Oviductal Fluid of the Rhesus Monkey (Macaca Mulatta)**Supported by United States Public Health Service Grant HD-06274 and by a grant from the National Foundation-March of Dimes.††Presented at the Ninth World Congress on Fertility and Sterility and the Thirty-Third Annual Meeting of the American Fertility Society, April 12 to 16, 1977, Miami Beach, Fla. Fertil. Sterility 28, 981–985. doi:10.1016/s0015-0282(16)42801-3
Magid, E., and Turbeck, B. O. (1968). The Rates of the Spontaneous Hydration of CO2 and the Reciprocal Reaction in Neutral Aqueous Solutions between 0° and 38°. Biochim. Biophys. Acta (Bba) - Gen. Subjects 165, 515–524. doi:10.1016/0304-4165(68)90232-8
Nakanishi, T., Ikawa, M., Yamada, S., Toshimori, K., and Okabe, M. (2001). Alkalinization of Acrosome Measured by GFP as a pH Indicator and its Relation to Sperm Capacitation. Dev. Biol. 237, 222–231. doi:10.1006/dbio.2001.0353
Oliphant, G., and Brackett, B. G. (1973). Capacitation of Mouse Spermatozoa in Media with Elevated Ionic Strength and Reversible Decapacitation with Epididymal Extracts**Supported by Grant RR00340, National Institutes of Health Grants HDO6274-01 and HD00130, Career Development Award HD15861, and a grant from the Ford Foundation. Fertil. Sterility 24, 948–955. doi:10.1016/s0015-0282(16)40094-4
Olson, S. D., Suarez, S. S., and Fauci, L. J. (2010). A Model of Catsper Channel Mediated Calcium Dynamics in Mammalian Spermatozoa. Bull. Math. Biol. 72, 1925–1946. doi:10.1007/s11538-010-9516-5
Putnam, R. W. (2012). Intracellular pH Regulation. San Diego: Academic Press, 303–321. Chapter 17 - Intracellular pH Regulation. doi:10.1016/b978-0-12-387738-3.00017-2
Ren, D., Navarro, B., Perez, G., Jackson, A. C., Hsu, S., Shi, Q., et al. (2001). A Sperm Ion Channel Required for Sperm Motility and Male Fertility. Nature 413, 603–609. doi:10.1038/35098027
Ruknudin, A., and Silver, I. A. (1990). Ca2+ Uptake during Capacitation of Mouse Spermatozoa and the Effect of an Anion Transport Inhibitor on Ca2+ Uptake. Mol. Reprod. Dev. 26, 63–68. doi:10.1002/mrd.1080260110
Santi, C. M., Martínez-López, P., de la Vega-Beltrán, J. L., Butler, A., Alisio, A., Darszon, A., et al. (2010). The SLO3 Sperm-specific Potassium Channel Plays a Vital Role in Male Fertility. FEBS Lett. 584, 1041–1046. doi:10.1016/j.febslet.2010.02.005
Schreiber, M., Wei, A., Yuan, A., Gaut, J., Saito, M., and Salkoff, L. (1998). Slo3, a Novel pH-Sensitive K+ Channel from Mammalian Spermatocytes. J. Biol. Chem. 273, 3509–3516. doi:10.1074/jbc.273.6.3509
Stival, C., La Spina, F. A., Baró Graf, C., Arcelay, E., Arranz, S. E., Ferreira, J. J., et al. (2015). Src Kinase Is the Connecting Player between Protein Kinase a (PKA) Activation and Hyperpolarization through SLO3 Potassium Channel Regulation in Mouse Sperm. J. Biol. Chem. 290, 18855–18864. doi:10.1074/jbc.M115.640326
Suarez, S. S., Vincenti, L., and Ceglia, M. W. (1987). Hyperactivated Motility Induced in Mouse Sperm by Calcium Ionophore A23187 Is Reversible. J. Exp. Zool. 244, 331–336. doi:10.1002/jez.1402440218
Vyklicka, L., and Lishko, P. V. (2020). Dissecting the Signaling Pathways Involved in the Function of Sperm Flagellum. Curr. Opin. Cel Biol. 63, 154–161. doi:10.1016/j.ceb.2020.01.015
Wandernoth, P. M., Mannowetz, N., Szczyrba, J., Grannemann, L., Wolf, A., Becker, H. M., et al. (2015). Normal Fertility Requires the Expression of Carbonic Anhydrases II and IV in Sperm. J. Biol. Chem. 290, 29202–29216. doi:10.1074/jbc.M115.698597
Wang, D., King, S. M., Quill, T. A., Doolittle, L. K., and Garbers, D. L. (2003). A New Sperm-specific Na+/H+ Exchanger Required for Sperm Motility and Fertility. Nat. Cel Biol 5, 1117–1122. doi:10.1038/ncb1072
Wang, H., McGoldrick, L. L., and Chung, J.-J. (2021). Sperm Ion Channels and Transporters in Male Fertility and Infertility. Nat. Rev. Urol. 18, 46–66. doi:10.1038/s41585-020-00390-9
Wennemuth, G., Carlson, A. E., Harper, A. J., and Babcock, D. F. (2003). Bicarbonate Actions on Flagellar and Ca2+-Channel Responses:initial Events in Sperm Activation. Development 130, 1317–1326. doi:10.1242/dev.00353
Windler, F., Bönigk, W., Körschen, H. G., Grahn, E., Strünker, T., Seifert, R., et al. (2018). The Solute Carrier SLC9C1 Is a Na+/H+-exchanger Gated by an S4-type Voltage-Sensor and Cyclic-Nucleotide Binding. Nat. Commun. 9, 2809. doi:10.1038/s41467-018-05253-x
Xu, W. M., Shi, Q. X., Chen, W. Y., Zhou, C. X., Ni, Y., Rowlands, D. K., et al. (2007). Cystic Fibrosis Transmembrane Conductance Regulator Is Vital to Sperm Fertilizing Capacity and Male Fertility. Proc. Natl. Acad. Sci. 104, 9816–9821. doi:10.1073/pnas.0609253104
Yang, C., Zeng, X.-H., Zhou, Y., Xia, X.-M., and Lingle, C. J. (2011). LRRC52 (Leucine-rich-repeat-containing Protein 52), a Testis-specific Auxiliary Subunit of the Alkalization-Activated Slo3 Channel. Proc. Natl. Acad. Sci. 108, 19419–19424. doi:10.1073/pnas.1111104108
Yeung, C. H., Anapolski, M., and Cooper, T. G. (2002). Measurement of Volume Changes in Mouse Spermatozoa Using an Electronic Sizing Analyzer and a Flow Cytometer: Validation and Application to an Infertile Mouse Model. J. Androl. 23, 522–528. doi:10.1002/j.1939-4640.2002.tb02274.x
Zeng, X.-H., Yang, C., Xia, X.-M., Liu, M., and Lingle, C. J. (2015). SLO3 Auxiliary Subunit LRRC52 Controls Gating of Sperm KSPER Currents and Is Critical for normal Fertility. Proc. Natl. Acad. Sci. USA 112, 2599–2604. doi:10.1073/pnas.1423869112
Zeng, Y., Oberdorf, J. A., and Florman, H. M. (1996). pH Regulation in Mouse Sperm: Identification of Na+-, Cl−-, and[formula]Dependent and Arylaminobenzoate-dependent Regulatory Mechanisms and Characterization of Their Roles in Sperm Capacitation-dependent and Arylaminobenzoate-dependent Regulatory Mechanisms and Characterization of Their Roles in Sperm Capacitation. Dev. Biol. 173, 510–520. doi:10.1006/dbio.1996.0044
Keywords: sperm, capacitation, SNHE, SLO3, hyperpolarization, mathematical model, bistability
Citation: de Prelle B, Lybaert P and Gall D (2022) A Minimal Model Shows that a Positive Feedback Loop Between sNHE and SLO3 can Control Mouse Sperm Capacitation. Front. Cell Dev. Biol. 10:835594. doi: 10.3389/fcell.2022.835594
Received: 14 December 2021; Accepted: 21 February 2022;
Published: 25 March 2022.
Edited by:
Hongmin Qin, Texas A&M University, United StatesReviewed by:
Lonny Ray Levin, Cornell University, United StatesAlberto Darszon, Universidad Nacional Autónoma de México, Mexico
Copyright © 2022 de Prelle, Lybaert and Gall. This is an open-access article distributed under the terms of the Creative Commons Attribution License (CC BY). The use, distribution or reproduction in other forums is permitted, provided the original author(s) and the copyright owner(s) are credited and that the original publication in this journal is cited, in accordance with accepted academic practice. No use, distribution or reproduction is permitted which does not comply with these terms.
*Correspondence: Bertrand de Prelle, YmVydHJhbmQuZGUucHJlbGxlLmRlLmxhLm5pZXBwZUB1bGIuYmU=; David Gall, ZGdhbGxAdWxiLmFjLmJl
†These authors have contributed equally to this work