- 1Institut National de La Recherche Scientifique, INRS-Centre Armand-Frappier Santé Biotechnologie, Université du Québec, Laval, QC, Canada
- 2Institut des Sciences et Industries du Vivant et de l’Environnement - AgroParisTech, Université Paris-Saclay, Paris, France
Regulation of hematopoietic stem cell (HSC) self-renewal and differentiation is essential for their maintenance, and HSC polarity has been shown to play an important role in this regulation. Vangl2, a key component of the Wnt/polarity pathway, is expressed by fetal and adult HSCs, but its role in hematopoiesis and HSC function is unknown. Here we show the deletion of Vangl2 in mouse hematopoietic cells impairs HSC expansion and hematopoietic recovery post-transplant. Old Vangl2-deficient mice showed increased expansion of myeloid-biased multipotent progenitor cells concomitant with splenomegaly. Moreover, Vangl2-deficient cells were not able to effectively reconstitute the recipient bone marrow in serial transplants, or when coming from slightly older donors, demonstrating impaired self-renewal or expansion. Aged Vangl2-deficient HSCs displayed increased levels of cell cycle inhibitor p16INK4a and active β–catenin, which could contribute to their impaired function. Overall, our findings identify Vangl2 as a new regulator of hematopoiesis.
Introduction
Hematopoiesis serves to generate trillions of new blood cells every day from a small number of hematopoietic stem cells (HSC). HSCs possess the ability to self-renew and differentiate into erythroid, myeloid and lymphoid cells. This is clinically used in bone marrow transplantation (BMT), in which non-functional HSCs are replaced with healthy ones. BMT is the most effective cell therapy used to treat hematopoietic malignancies, especially blood cancers. Healthy HSCs from compatible donors are traditionally obtained from bone marrow (BM), but can also be obtained from cytokine-mobilized peripheral blood or umbilical cord blood. Understanding HSC regulators could help studying HSCs ex vivo which remains a prominent challenge today and improve BMT success by expanding the use of cord blood HSCs which are associated with fewer risks of graft-versus-host-disease (Li and Sykes, 2012).
HSCs reside in the BM niche, where they receive different cues regulating their survival and quiescence vs. proliferation. Among these, Wnt proteins (Staal et al., 2008) regulate the balance between HSC proliferation and maintenance through three interconnected pathways: the canonical Wnt/β-catenin pathway, extensively studied and known to control HSC self-renewal (Luis et al., 2012), and non-canonical Wnt/Ca2+ (Florian et al., 2012; Florian et al., 2013) and Wnt/Planar Cell Polarity (PCP) (Le Grand et al., 2009; Sugimura et al., 2012; Abidin et al., 2015; Lhoumeau et al., 2016) pathways, mostly known for stem cell maintenance. Wnt/PCP pathway was originally identified as a means to control epithelial cell polarity (Seifert and Mlodzik, 2007), but its role in HSC regulation has been more recently discovered. Previous work from our laboratory suggests a protective role for Wnt/PCP signaling in HSCs, particularly under hematopoietic stress (Heinonen et al., 2011; Abidin et al., 2015). Frizzled-6, a receptor which has a prevalent role in non-canonical Wnt/PCP signalling (Chang et al., 2016), regulates HSC self-renewal and progenitor cell survival post-transplant and is crucial for emergency hematopoietic response during inflammation (Abidin et al., 2015) and infections (Abidin et al., 2017). Others have demonstrated that mice deficient in the Wnt/PCP component Ptk-7 exhibited deregulated HSC proliferation and migration (Lhoumeau et al., 2016), while Frizzled-8 and Celsr2/Fmi maintained HSC long-term quiescence in the BM (Sugimura et al., 2012). In sum, various Wnt/PCP pathway components have been implicated in regulating HSC proliferation and maintenance.
Vangl2 is another key regulator of the Wnt/PCP signalling pathway, and fetal and adult HSCs express Vangl2 (Kwarteng et al., 2018). Moreover, Vangl2 is expressed more strongly by HSCs and progenitor cells compared to mature blood cells (Choi et al., 2019). Vangl2 is known for its crucial role in neural plate development, and mutations in this gene are associated with neural tube defects in mice (Murdoch et al., 2001) and in humans (Kibar et al., 2011). In mouse epithelial cells, Vangl2 is known to control hair bundle orientation and plays a role in Frizzled-3 and Frizzled-6 cell surface localisation in the inner ear (Montcouquiol et al., 2003; Wang et al., 2006). Despite being highly expressed by HSCs and progenitor cells and having a crucial role in the PCP pathway, nothing is known yet about the role of Vangl2 in HSC regulation and maintenance.
In this study, we show that the loss of Vangl2 in hematopoietic cells impairs post-transplant recovery and leads to BM failure following serial transplantation. There are also alterations in the multipotent progenitor cell (MPP) pool of old Vangl2-deficient mice, and old Vangl2-deficient HSCs show increased expression of the senescence-associated cell cycle inhibitor p16INK4a concomitant with increased β-catenin stabilization. These results uncover the important role of Vangl2 in HSC expansion and post-transplant recovery and open the door for further research on Vangl2 as a regulator of hematopoiesis and HSC function.
Materials and Methods
Experimental Animals
C57BL/6, B6.SJL (B6.SJL-PtprcaPepcb/BoyJ), B6.Cg-Commd10Tg(Vav1-icre)A2Kio/J (Vav-Cre), and B6;129-Vangl2tm2.1Mdea/J (Vangl2lox/lox) mice were purchased from The Jackson Laboratory (Bar Harbor, ME). Male Vav-Cre+ Vangl2lox/lox or Vav-Cre+ Vangl2lox/+ mice were crossed with Vangl2lox/+ or Vangl2lox/lox females to obtain offspring in which the Vangl2 gene is deleted from the hematopoietic stem/progenitor cell pool (Vav-Cre+ Vangl2lox/lox). Experiments were done using both male and female mice together with sex-matched, co-housed littermate controls (Vav-Cre- Vangl2lox/+ or Vav-Cre- Vangl2lox/lox). All mice were reared and housed in pathogen-free conditions in sterile ventilated supports at the animal facility of l’Institut national de la recherche scientifique (INRS) - Centre Armand-Frappier Santé Biotechnologie (Laboratoire national de biologie expérimentale). All procedures were carried out in accordance with the Canadian Council on Animal Care guidelines and were approved by INRS Institutional Animal Care Committee (CIPA).
BM Transplantation Assays
Donor (CD45.2+; C57BL/6 background, control and Vangl2Δ/Δ) and competitor (CD45.1+; B6.SJL) BM cells were analyzed by flow cytometry prior to transplant, and the quantities of total BM cells were adjusted to inject equivalent numbers of donor and competitor HSCs, similar to what has been previously described and as detailed below (Kwarteng and Heinonen, 2016; Hétu-Arbour et al., 2021a). Cells were pooled from two to three donors per experiment, and quantities of whole BM cells were normalized to the equivalent of 150 LT-HSCs (defined as CD150+ CD48− Lin− Sca1+ c-Kithi). This corresponded to approx. 1 × 106 CD45.1+ competitor BM cells and, on average, to 7 × 105 total BM cells for CD45.2+ donors. The exact number was determined independently for each donor based on LT-HSC frequency in the BM. Cells were injected into the lateral tail vein of lethally irradiated recipient mice (CD45.1+ CD45.2+; F1 offspring of C57BL/6 x B6.SJL intercrosses). Recipients were given two doses of 4.5 Gy with a 16-h interval using the RS 2000 small animal X-ray irradiator (RadSource Technologies, Suwanee, GA). For secondary transplants, an equal number (2.5 × 106) of total BM cells from two primary recipients were pooled, mixed with 1 × 106 fresh CD45.1+ competitor cells, and injected into lethally irradiated secondary recipients. For tertiary transplants, BM cells were again pooled from two secondary recipients (5 × 106 in total), but there was no added competition. Serial transplant results are shown for young primary donors, only. To analyze short and long-term reconstitution, peripheral blood was collected from the mandibular vein of recipient mice at 4, 8, 12, and 16 weeks post-transplant. Mice were euthanized 20–22 weeks post-transplant and BM/spleen were collected and prepared as described below for flow cytometry analysis.
Flow Cytometry and Imaging
BM cells were harvested by flushing tibiae and femora with PBS/0.1% BSA/0.5 mM EDTA using a syringe with a 25-gauge needle. Spleen cells were isolated by mechanically crushing the organs with the plunger of a 10cc syringe. For blood analysis, erythrocytes were lysed in hypotonic buffer (0.14M NH4Cl; 17 mM Tris-HCl, pH 7.6) for 4–6 min, or until sample was translucent and reaction was stopped with three volumes of ice-cold PBS, followed by centrifugation and washing with PBS/0.1% BSA/0.5 mM EDTA. Non-specific staining was blocked with anti-CD16/CD32 prior to staining with fluorochrome-conjugated antibodies for 30 min on ice. For cell cycle analysis, cells were first stained with surface antibodies, then fixed and permeabilized using the Foxp3 labeling kit for nuclear proteins as directed by the manufacturer (eBioscience, San Diego, CA). Samples were blocked with 2% rat serum for 30 min before intracellular staining with anti-Ki-67 for 1 h, followed by DAPI (0.25 μg/ml) for 30 min, both at room temperature. For detection of p16 expression, cells were prepared as described above, fixed, permeabilized, and then blocked with 1% BSA before intracellular staining with the recombinant anti-CDKN2A/p16INK4a antibody for 1 h at room temperature. Primary antibody was detected with Alexa Fluor 488-conjugated F(ab')2-goat anti-rabbit IgG (H + L) cross-adsorbed secondary antibody. Samples were acquired on a four-laser BD LSRFortessa flow cytometer (BD Biosciences, Mountain View, CA) and analyzed using FACS DiVa software (v. 8.1) or FlowJo (v. 10.1) software. See Table 1 for additional details on antibodies.
For imaging flow cytometry, BM cells were harvested by flushing tibiae, femora and iliac crests with PBS using a 25-gauge syringe needle. Samples were first enriched for HSCs and progenitor cells with the EasySep™ Mouse Hematopoietic Cell Isolation Kit (Stem Cell Technologies, Vancouver, BC, Canada) and then stained with anti-Sca1, anti-CD117 and anti-CD150 (see Table 1). Cells were washed with PBS, fixed, permeabilized and blocked with BSA as described above prior to intracellular labeling with anti-Cdc42 or anti-non-phospho (active) β‐catenin overnight. Intracellular staining was detected with R-Phycoerythrin-conjugated AffiniPure F(ab')₂ Fragment Goat Anti-Rabbit IgG (H + L) for 1 h and counterstained with DAPI (0.025 μg/ml) for 15 min. Cells were acquired with Amnis Imagestream Mark II imaging flow cytometer (EMD Millipore) and analyzed with IDEAS v6.2 software. Polarity was determined using delta centroid and modulation morphology features for Cdc42, while β‐catenin nuclear translocation was determined by similarity with DAPI (nuclear translocation feature), similar to what we have previously published (Kwarteng et al., 2018). See Table 1 for additional details on antibodies.
Colony Assays
BM single-cell suspensions were prepared in IMDM (Life Technologies) containing 10% Premium FBS (Wisent Bio Products, Saint-Bruno, QC, Canada). Using a syringe fitted with a blunt-ended needle, cells were plated in duplicates into 35-mm non-adherent Petri dishes at a density of 2 × 104 cells/dish in methylcellulose medium optimized for multilineage erythro-myeloid growth (MethoCult™ GF M3434; Stem Cell Technologies). Cultures were incubated at 37 C in 5% CO2 for 10–14 days and hematopoietic colonies were counted and identified based on morphology under an inverted microscope. Cells were harvested by pooling duplicates for flow cytometry analysis.
Statistical Analysis
Each graph represents at least three independent experiments, unless otherwise indicated. Two-tailed Student’s t test was used to determine statistical significance unless otherwise noted. A p value of 0.05 or less was considered significant.
Results
Vangl2 Regulates BM Multipotent Progenitor Cell Numbers and Spleen Size in Old Mice
To investigate the functional role of Vangl2 in homeostatic conditions, we first analyzed BM hematopoiesis using a mouse model in which the polarity gene Vangl2 is inactivated in all hematopoietic lineages using the Vav-iCre-LoxP system (Vangl2Δ/Δ mice) (de Boer et al., 2003). We analyzed mice from both sexes and different age groups by flow cytometry (Figure 1A). There were no major differences in total BM cellularity between Vangl2Δ/Δ mice and controls in any age group, although it tended toward an increase in older (18-month-old) mice (Figure 1B). However, old Vangl2Δ/Δ mice presented a significant increase in total Lin−Sca-1+cKit+ (LSK) cell numbers driven by the expansion of myeloid-biased multipotent progenitor cell populations (MPP2 and MPP3) compared to their littermate controls (Figures 1C,F). Most of these changes were not seen in young (2-month-old) and adult (6-month-old) Vangl2Δ/Δ groups, although an increase in MPP3 frequency was already detectable in adult mice (Figures 1D,E). Similar tendencies were observed in both sexes (Supplementary Figure S1A,B,C); there was a significant decrease in the number of CD150+ CD48− LT-HSCs in Vangl2Δ/Δ males compared to Vangl2Δ/Δ females, but the difference was not significant when compared to their sex-matched controls (Supplementary Figure S1C). Taken together, these results indicate that Vangl2 is dispensable for HSC emergence and their steady-state maintenance. However, the expansion of myeloid-biased MPPs in older mice suggest that Vangl2 may play a role in hematopoiesis, including age-dependent alterations in BM progenitor cell pool.
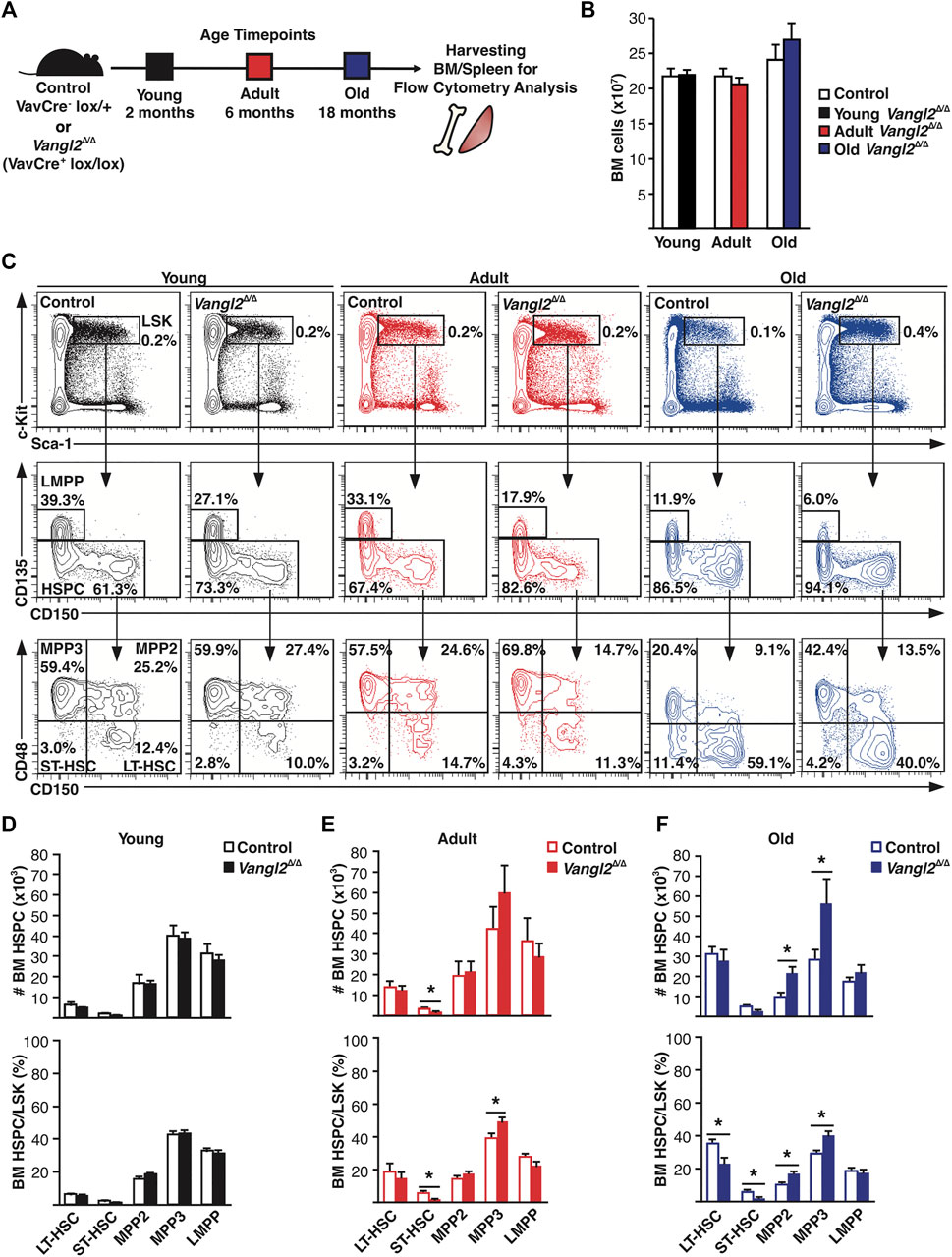
FIGURE 1. Vangl2 loss in hematopoietic cells increases myeloid-biased multipotent progenitor cell numbers in old mice. (A) Experimental design for flow cytometry analysis of young (2-month-old, in black), adult (6-month-old, in red) and old (18-month-old, in blue) VavCre+Vangl2lox/lox mice in which Vangl2 is ablated in all hematopoietic lineages (Vangl2Δ/Δ) and littermate controls. (B) Histogram represents absolute numbers of BM cells per leg (tibia + femur) for young, adult and old mice (mean +SEM). (C) Representative flow cytometry data are shown for young, adult and old mice. Populations were defined as follows: Lin−Sca1+c-Kit+ (LSK), CD135+CD48+CD150− (LMPP), CD135−CD48+CD150− (MPP3), CD135−CD48+CD150+ (MPP2), CD135−CD48−CD150− (ST-HSC) and CD135−CD48−CD150+ (LT-HSC). Numbers within panels represent cell percentage for the panel shown. (D-F) Quantitative results of HSCs and progenitor cells flow cytometry analysis are represented in (D) for young, (E) adult, and (F) old mice. Histograms represent absolute numbers of BM cells in top panels and stem/progenitor cell percentage within the LSK pool in bottom panels (mean +SEM). See also Supplementary Figure S1. Young (n = 17 control, 18 Vangl2Δ/Δ), adult (n = 10 control, 11 Vangl2Δ/Δ), old (n = 14 control, 14 Vangl2Δ/Δ), *p ≤ 0.05.
Vangl2 is highly expressed by HSCs and progenitor cells compared to mature blood cells (Choi et al., 2019). Hence, to evaluate the role of Vangl2 in differentiation, we next analyzed mature lymphoid and myeloid cells in the BM and spleen (Supplementary Figure S2A,B). We found no differences in total spleen cellularity between control and Vangl2Δ/Δ mice (Figure 2A). However, we noted a significant increase in spleen size and weight in a subset of old Vangl2Δ/Δ mice (Figure 2B). This was not driven by an overall increase in body size, as similar differences were also seen using spleen index (spleen/bodyweight ratio; Figure 2B), and it occurred more frequently in females (5/7 at 18 months and 3/6 at 6 months) than in males (1/6 and 0/6, respectively).
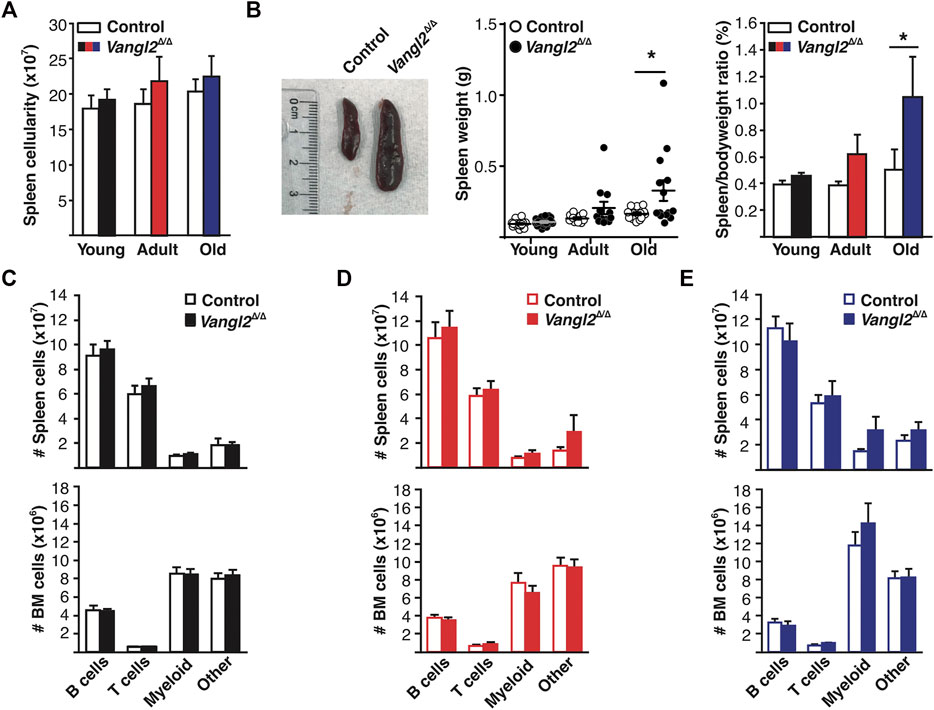
FIGURE 2. Vangl2 is dispensable for steady-state lympho-myelopoiesis, but its absence promotes splenomegaly in old mice. (A) Spleen cellularity for young (2-month-old), adult (6-month-old) and old (18-month-old) mice (mean +SEM). (B) Photograph of an enlarged Vangl2Δ/Δ spleen (left). Spleen weight for individual mice (middle; horizontal lines represent mean and error bars, SEM) and average spleen index as determined by the spleen weight (g)/bodyweight (g) ratio (right; mean +SEM). (C-E) Histograms represent absolute spleen (top panels) and BM (bottom panels) B lymphocyte (CD19+), T lymphocyte (CD3ε+), and myeloid (CD11bhi) cell numbers for (C) young mice, (D) adult mice, and (E) old mice (mean +SEM). CD19− CD3ε− CD11b−/lo cells are represented as “Other”. See also Supplementary Figure S1,S2. Young (n = 17 control, 19 Vangl2Δ/Δ), adult (n = 10 control, 11 Vangl2Δ/Δ), old (n = 14 control, 14 Vangl2Δ/Δ), *p ≤ 0.05.
Although the proportion of myeloid cells increased with age, as expected, there was no significant difference in the number of myeloid cells in the BM or spleen between Vangl2Δ/Δ mice and age-matched controls (Figures 2C–E), irrespective of sex (Supplementary Figure S1D-F). However, there was a tendency toward an increased accumulation of myeloid cells in the spleen of old Vangl2Δ/Δ mice (Figure 2E) that appeared mostly restricted to females (Supplementary Figure S1F), in line with the stronger MPP expansion in the BM of Vangl2Δ/Δ females (Supplementary Figure S1C) and the occurrence of splenomegaly.
Vangl2-Deficient Progenitor Cells Show Impaired Expansion in Culture
The increase in BM multipotent progenitors in older Vangl2Δ/Δ mice led us to investigate the proliferative activity of Vangl2Δ/Δ stem/progenitor cells. To evaluate cell cycle, we performed a Ki-67 and DAPI intracellular staining and analyzed different cell cycle phases by flow cytometry (Szade et al., 2016). As expected, we found no differences between controls and Vangl2Δ/Δ mice in young and adult groups (Figure 3A, top and middle panel). However, we did find differences in the old group in which Vangl2Δ/Δ mice had fewer quiescent LSKs and more LSKs in G1 phase (Figure 3B). However, no differences were seen within most sub-populations, except for an increase in the proportion of Vangl2Δ/Δ ST-HSCs in G0 phase (Figure 3C). This apparent discrepancy can be explained by the relative increase in the frequency of MPPs (that are actively cycling) concomitant with the relative decrease in HSCs (that are generally more quiescent) within the Vangl2Δ/Δ LSK population as compared to control LSKs (Figures 1C,F).
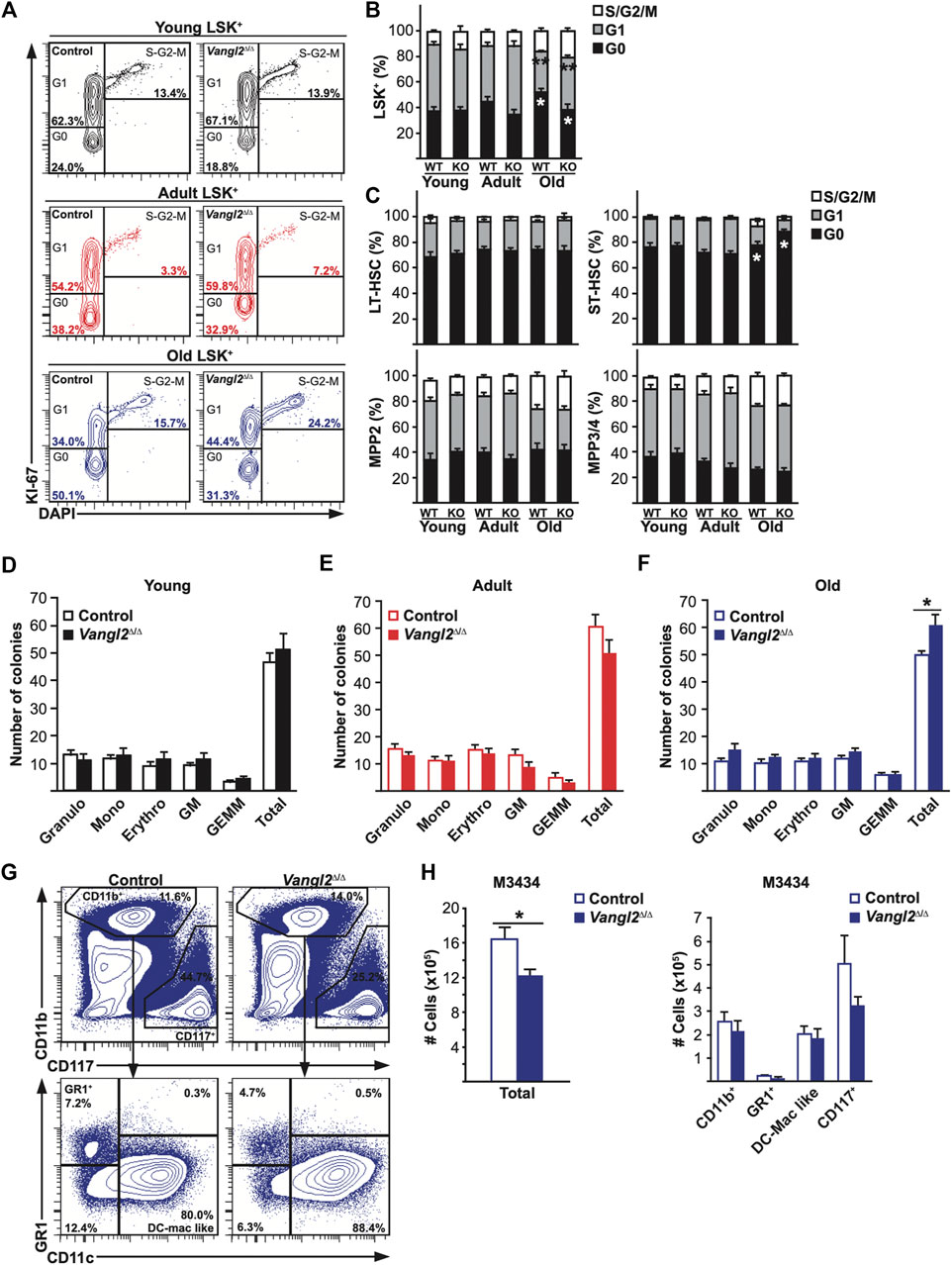
FIGURE 3. Vangl2 loss impairs old myeloid progenitor cell expansion in vitro. (A) Representative flow cytometry data are shown for LSK+ cells from young (2-month-old), adult (6-month-old) and old (18-month-old) mice. Numbers within panels represent G0, G1 and S-G2-M cell percentage for the panel shown. (B and C) Quantitative results of flow cytometry cell cycle analysis are represented for young, adult and old (B) LSK + cells and (C) LT-HSC, ST-HSC, MPP2 and MPP3/4 cells (mean +SEM). Young (n = 10 control, 12 Vangl2Δ/Δ), adult (n = 8 control, 10 Vangl2Δ/Δ), old (n = 8 control, 10 Vangl2Δ/Δ). (D-F) Number of colonies counted after 10–14 days in vitro are represented for (D) young (n = 8), (E) adult (n = 5) and (F) old (n = 5) BM cells. Granulo: granulocyte, Mono: monocyte, Erythro: erythroid, GM: granulocyte/macrophage, GEMM: granulocyte/erythroid/macrophage/megakaryocyte. (G) Representative flow cytometry data of harvested colony-forming units (H) Histograms represent cell numbers recovered per pooled duplicate Petri dishes seeded with BM cells from old mice (mean +SEM). *p ≤ 0.05, **p ≤ 0.005.
To further determine whether their differentiation in vitro was affected, we evaluated BM myeloid colony-forming units after 10–14 days of culture. As expected, we did not see any differences in young and adult mice (Figures 3D,E), but there was a slight increase in the total number of colonies from old Vangl2Δ/Δ BM (Figure 3F). Flow cytometry analysis revealed that although more colonies were generated by old Vangl2Δ/Δ mice, they consisted on average of fewer cells (Figures 3G,H). This was consistent with a decreased frequency of CD117+ cells that are normally found in colonies with high proliferative potential (Figures 3G,H). These results suggest Vangl2-deficient old progenitor cells have a decreased proliferative capacity in vitro, despite their being no significant changes in their overall cell cycle status.
Vangl2 Is Required for Post-transplant Peripheral Blood and BM Reconstitution in Adult Donors
Considering differences seen in homeostatic conditions in Vangl2Δ/Δ mice, we next evaluated HSC functionality under hematopoietic pressure. The gold-standard assay for HSC function is to evaluate their ability to reconstitute a lethally irradiated host so we conducted competitive bone marrow transplant assays as described (Kwarteng and Heinonen, 2016; Hétu-Arbour et al., 2021a) and followed post-transplant recovery by analyzing peripheral blood reconstitution for 16 weeks and BM recovery at 20–22 weeks by flow cytometry (Figure 4A). To evaluate the possible age-related role of Vangl2, we performed BM transplants with young (2-month-old) and adult (6-month-old) donors. We found no differences in recovery in primary recipient mice transplanted with young Vangl2Δ/Δ BM cells. However, we observed a significant decrease in overall peripheral blood chimerism after 16 weeks in mice transplanted with adult Vangl2Δ/Δ BM cells compared to their controls (Figures 4B,C; Supplementary Figure S2C). Furthermore, mice transplanted with adult Vangl2Δ/Δ BM cells showed a significant decrease in donor-derived GR1hi granulocytes (12–16 weeks post-transplant), GR1lo monocytes (8–16 weeks post-transplant), CD19+ B cells (16 weeks post-transplant) and CD3ε+ T cells (16 weeks post-transplant) in peripheral blood (Figures 4C,D). We next evaluated BM HSC and progenitor cell recovery 20 weeks post-transplant. As expected from peripheral blood analysis, no differences were seen in mice transplanted with young Vangl2Δ/Δ vs. control BM cells. However, mice transplanted with adult Vangl2Δ/Δ BM cells had a significant decrease in donor-derived BM LT-HSCs as compared to controls (Figures 4E,F). No differences were observed in mature cell numbers in BM or spleen in primary transplant recipients at 20 weeks (Supplementary Figure S3A,B).
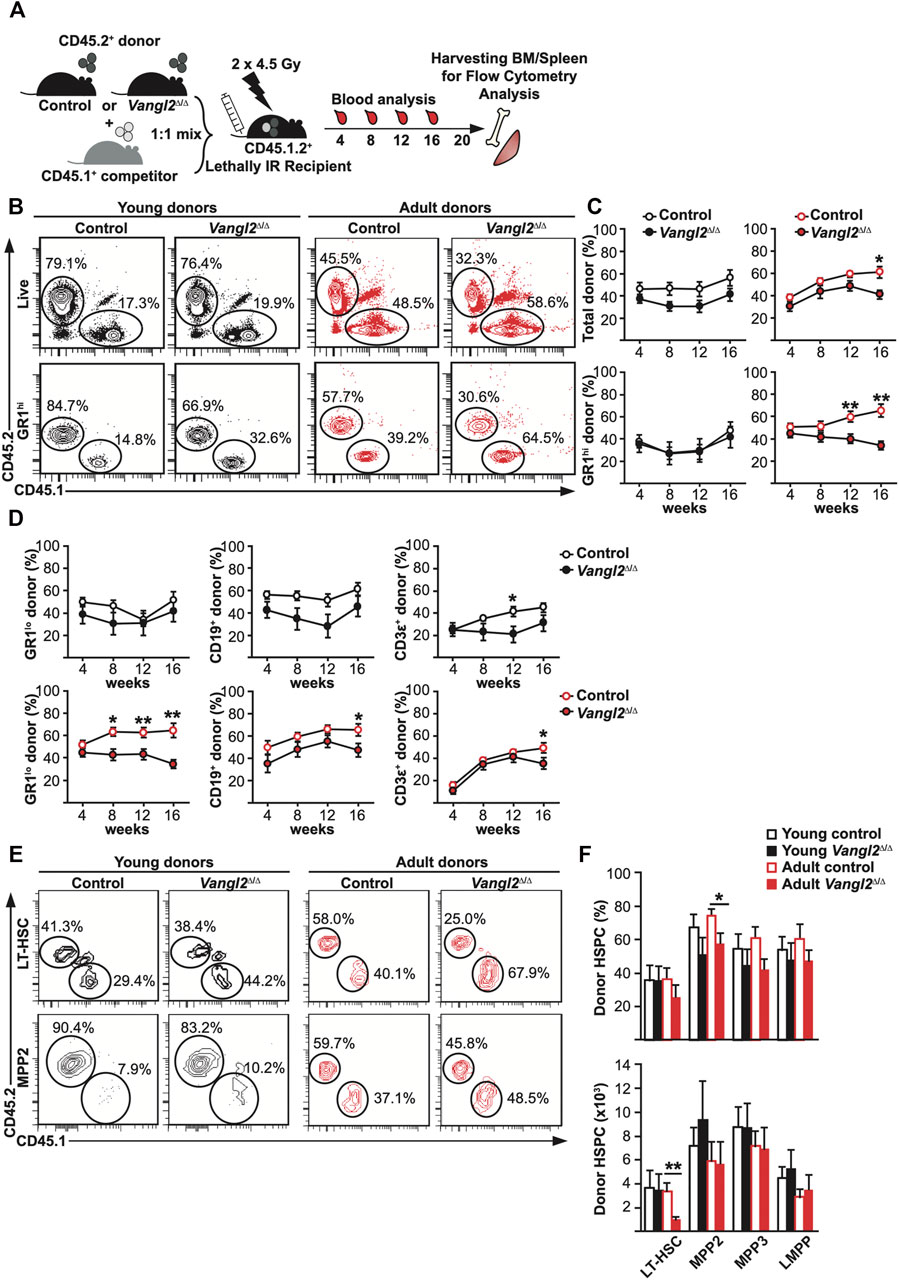
FIGURE 4. Vangl2 is required for hematopoietic reconstitution in adult donors. (A) Experimental design for primary transplants (B) Representative flow cytometry data showing the percentage of donor-derived total and GR1hi cells in recipient peripheral blood post-transplant for young (2-month-old) and adult (6-month-old) donors at 16 or 12 weeks post-transplant, respectively. (C) Graphs represent pooled results of donor-derived total and GR1hi cells in recipient peripheral blood for young and adult donors (mean +SEM). (D) Graphs represent pooled results of donor-derived GR1lo cells, CD19+ B cells and CD3ε+ T cells in recipient peripheral blood for young and adult donors (mean +SEM). (E) Representative flow cytometry data showing the percentage of CD45.2+ donor-derived or CD45.1+ competitor LT-HSCs (top panels) and MPP2s (bottom panels) in recipient BM 20 weeks post-transplant for young and adult donors. (F) Histograms represent mean of young or adult donor-derived HSPCs chimerism (top) or absolute cell numbers (bottom) in BM 20 weeks post-transplant (mean +SEM). See also Supplementary Figure S2,S3,S4. Data for transplants with young female donors are pooled from three independent experiments (n = 14–16 control, 13–15 Vangl2Δ/Δ) and for adult female donors, from two independent experiments (n = 10 control, 11 Vangl2Δ/Δ). *p ≤ 0.05, **p ≤ 0.005.
Vangl2Δ/Δ BM Cells Fail in Serial Transplants, Leading to Hematopoietic Failure
To evaluate Vangl2Δ/Δ HSC long-term self-renewal capacity, we then performed serial BM transplantation assays using primary recipients of young BM as donors (Figure 5A). Secondary transplants from primary Vangl2Δ/Δ donors showed a significant decrease in myeloid (GR1hi and GR1lo) long-term reconstitution in peripheral blood as compared to controls (Figures 5B,C in black). This was most likely due to a functional impairment in the Vangl2Δ/Δ stem/progenitor cell compartment as we did not detect any differences in the frequency or number of phenotypic donor HSCs in the primary recipient BM (Figures 4E,F in black). We also observed a significant decrease in Vangl2Δ/Δ donor-derived LT-HSC, MPP2, MPP3 and LMPP numbers in secondary recipient BM (Figures 5E,F). Vangl2Δ/Δ donor-derived mature B and T cell numbers were not decreased as compared to controls in peripheral blood, spleen or BM of secondary recipients (Supplementary Figure S3C-F), most likely due to their long half-life. This was also reflected in total donor chimerism in peripheral blood (Supplementary Figure S3C,D), which is consistent with lymphocytes representing up to 80% leukocytes in murine peripheral blood (O'Connell et al., 2015; Stahl et al., 2018). In contrast, short-lived Vangl2Δ/Δ donor-derived GR1hi granulocytes dependent on constant replacement showed a significant decrease in spleen as well as in BM (Supplementary Figure S3E,F) that on average was at least as strong as that observed in peripheral blood at slightly earlier time points (approximately two-fold). Donor chimerism in BM MPPs at 20 weeks was very similar to what was seen in the periphery for myeloid cells 16 weeks post-transplant, and this applied to both Vangl2Δ/Δ and control donors (Figures 5C,E); however, the proportion of donor-derived HSCs was significantly lower (Figures 5D,E), particularly in the case of Vangl2Δ/Δ donors. The direct contribution of LT-HSCs to steady state hematopoiesis remains under debate (Sun et al., 2014; Chapple et al., 2018), but progenitor cells with little to no transplantation capacity have been shown to support local granulocyte production for up to a year (Sun et al., 2014), which would explain our result.
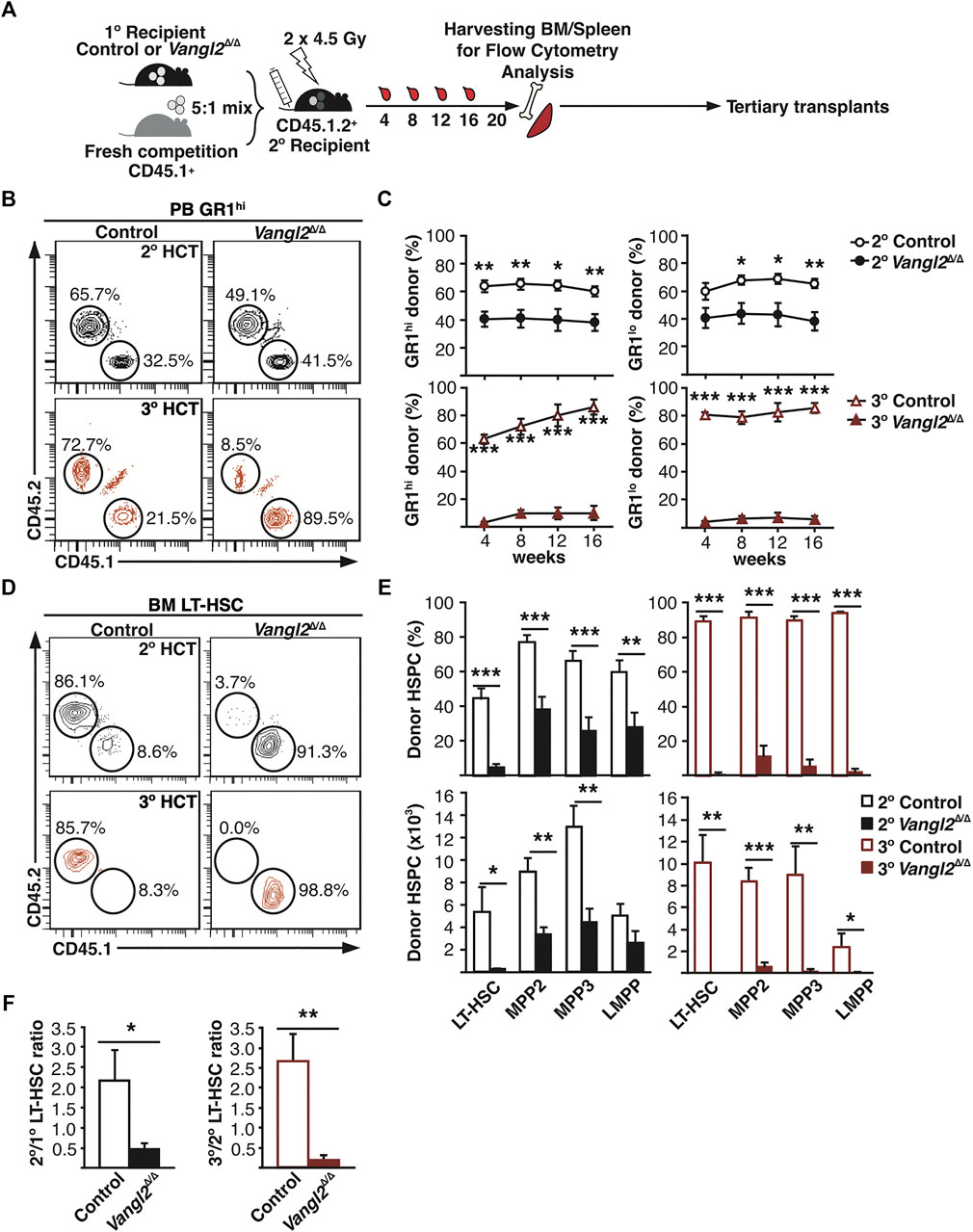
FIGURE 5. Vangl2 deficiency severely impairs hematopoietic recovery in serial transplants, leading to BM failure. (A) Experimental design for serial transplants (B) Representative flow cytometry data showing the percentage of donor-derived GR1hi cells in secondary and tertiary recipient peripheral blood 16 weeks post-transplant. (C) Graphs represent pooled results of donor-derived GR1hi granulocytes and GR1lo monocytes in secondary and tertiary recipient peripheral blood (mean +SEM). (D) Representative flow cytometry data showing the percentage of CD45.2+ donor-derived or CD45.1+ competitor LT-HSCs in BM of secondary (top panels) and tertiary recipients (bottom panels) 20 weeks post-transplant. (E) Histograms represent donor chimerism or absolute donor-derived cell numbers in secondary/tertiary recipient BM 20 weeks post-transplant (mean +SEM). (F) Donor-derived LT-HSC ratio (post-/pre-transplant) 20 weeks post-transplant. See also Supplementary Figure S2,S3,S4. *p ≤ 0.05, **p ≤ 0.005, ***p ≤ 0.0005. Secondary transplants represent two independent transplant groups (n = 9 control, 10 Vangl2Δ/Δ), while tertiary transplants represent one single group (n = 4 control, six Vangl2Δ/Δ).
To further corroborate our interpretation, tertiary transplants with Vangl2Δ/Δ BM cells displayed a nearly complete failure of hematopoietic reconstitution as little to no Vangl2Δ/Δ donor-derived cells were observed from 4–16 weeks in myeloid or lymphoid lineages (Supplementary Figure S5B,C and Supplementary Figure S3C,D in red). The low frequency of donor-derived LT-HSCs in the graft certainly contributed to the impaired reconstitution in tertiary transplants, but the proportion of donor-derived cells was even lower than what would be mathematically expected (approx. 10% CD45.2+ LT-HSCs in the secondary recipients used as BM donors), further confirming the decline not only in phenotypic but also in functional HSCs in the Vangl2Δ/Δ donor cell pool. In comparison, control donor-derived cells represented up to 80% total peripheral blood, an increase from what was seen in the BM of secondary recipients (approx. 40% CD45.2+ LT-HSCs). Similar results were seen in BM and spleen, where Vangl2Δ/Δ cells were essentially absent except for the myeloid lineage (Supplementary Figure S3E,F). This corresponded to the presence of a detectable Vangl2Δ/Δ donor-derived population only within MPP2 and MPP3 compartments in the BM (Figures 5E,F), suggesting that these cells could still maintain traces of myelopoiesis in tertiary recipients. In an attempt to account for the differences in donor cell input, especially in tertiary transplants, we compared donor LT-HSC numbers from the graft to those recovered 20 weeks post-transplant (number of cells in recipient BM/number of cells injected). We found no expansion of Vangl2Δ/Δ LT-HSCs in secondary or tertiary transplants, resulting in net loss of cells and the absence of Vangl2Δ/Δ LT-HSCs in tertiary transplants (Figure 5F). In comparison, control LT-HSCs retained their capacity to expand (approximately 2.5-fold in both cases). Similar results were obtained with male mice with the exception of slightly improved Vangl2Δ/Δ GR1hi granulocyte reconstitution in peripheral blood following primary transplants; however, donor contribution was significantly decreased in all lineages following secondary transplants (Supplementary Figure S4A,B).
Vangl2-Deficiency Increases p16INK4a Expression in LT-HSCs and Promotes β-catenin Stabilization
Since Wnt/PCP and Vangl2 signalling is known to control polarity in other cell types and because establishment of polarity is important for HSC self-renewal (Florian et al., 2018), we next investigated if Vangl2 loss impaired HSC polarity. To do so, we analyzed the cellular distribution of Cdc42, a small GTPase and polarity marker in HSCs, with imaging flow cytometry (Figure 6A) (Florian et al., 2012). We found no significant differences in polar and apolar CD150+ LSKs or Cdc42 intensity in old Vangl2Δ/Δ mice (Figures 6B,C). Similarly, no differences were found in young and adult mice, suggesting Vangl2 does not play a role in Cdc42 activation or localisation (Supplementary Figure S5A,B). However, as previously shown by others (Florian et al., 2012), cells from old mice were more frequently apolar.
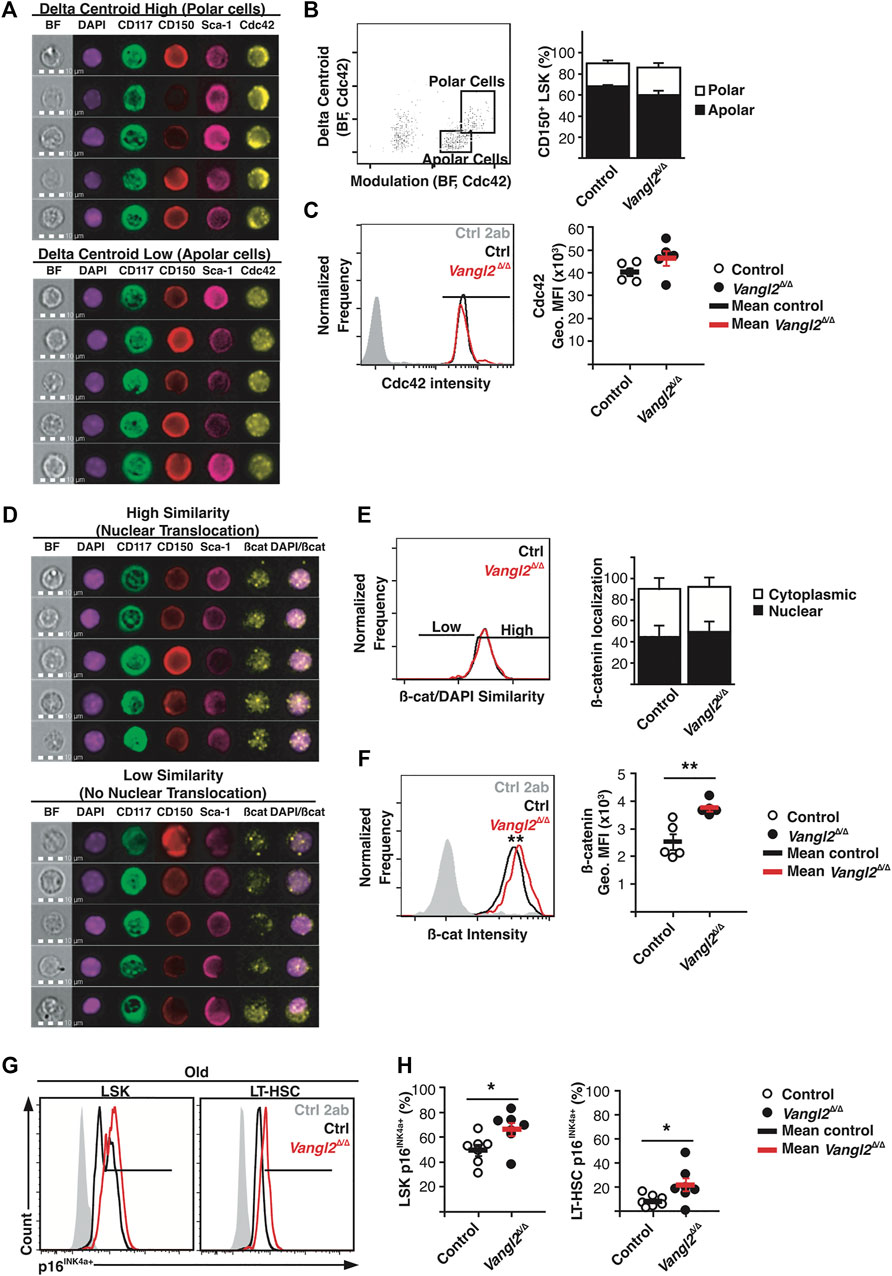
FIGURE 6. Vangl2 loss increases β-catenin activation and p16INK4a expression in old stem/progenitor cells. (A) Representative images of polar and apolar CD150+ LSK+ cells (B) Gating strategy based on Cdc42 delta centroid and morphology modulation features. Histogram represents the frequency of polar and apolar CD150+ LSK+ cells from old mice (mean +SEM). (C) Representative overlay of Cdc42 staining intensity (left) and quantification of Cdc42 geometric mean of fluorescence intensity (Geo. MFI). (D) Representative images of β-catenin (β-cat) nuclear translocation based on β-catenin/DAPI similarity analysis. (E) Gating strategy based on β-cat/DAPI similarity dilate analysis. Histogram represents cytoplasmic and nuclear β-catenin localization in old CD150+ LSK+ cells (mean +SEM). (F) Representative overlay of β-catenin staining intensity (left) and quantification of β-catenin geometric mean of fluorescence intensity (Geo. MFI) in old CD150+ LSK+ cells. (G) Representative overlay of p16INK4a expression in old LSK+ cells and LT-HSCs (H) Graphs represent the frequencies of p16+ cells within LSK+ and LT-HSC compartments (mean +SEM). See also Supplementary Figure S5. *p ≤ 0.05 (n = five to six, three independent experiments).
Non-canonical Wnt signalling can inhibit the canonical Wnt/β-catenin pathway (Nemeth et al., 2007), so we next evaluated β-catenin stabilization and localization (Figure 6D). Nuclear versus cytoplasmic β-catenin ratios were not altered in old Vangl2Δ/Δ mice (Figure 6E). Similar results were found with young and adult mice (Supplementary Figure S5C,D). However, we observed a significant increase in β-catenin mean fluorescent intensity within old Vangl2Δ/Δ CD150 + LSKs as compared to controls, suggesting a potentially higher β-catenin activity in old Vangl2Δ/Δ cells (Figure 6F, Supplementary Figure S5E).
Prolonged Wnt/β-catenin signalling is thought to induce senescence in other cell types (Gu et al., 2014), and it has been linked to HSC exhaustion and hematopoietic failure (Scheller et al., 2006). Cyclin-dependent kinase inhibitor and cell cycle regulator p16INK4a is often used as a marker of senescence, and although its endogenous expression is not uniformly associated with normal HSC aging (Janzen et al., 2006; Attema et al., 2009), enforced p16INK4a upregulation is associated with loss of HSC self-renewal (Oguro et al., 2006). There was a significant increase in p16INK4a expression among LSKs as well as LT-HSCs in old Vangl2Δ/Δ BM, suggesting p16INK4a activation within the BM progenitor cell pool in old Vangl2Δ/Δ mice (Figures 6G,H), which may contribute to their impaired recovery post-transplant.
Discussion
The importance of Wnt signalling in HSC self-renewal and hematopoiesis has been widely studied and debated, and while an equilibrium between the various intracellular pathways promotes the most optimal conditions, how this equilibrium is actually maintained remains still largely undefined. Although inhibition of Wnt binding to its receptors impairs HSC self-renewal and differentiation (Fleming et al., 2008; Renström et al., 2009; Schaniel et al., 2011), Wnt glycosylation and secretion is reportedly dispensable for adult hematopoiesis (Kabiri et al., 2015). Given the large number and potential promiscuity of Wnt ligands combined with the variety of hematopoietic and non-hematopoietic BM cells producing them, we chose to focus our study on the Wnt/PCP coreceptor Vangl2. Wnt/PCP components have been recently shown to be important in HSC maintenance and self-renewal (Sugimura et al., 2012; Abidin et al., 2015; Lhoumeau et al., 2016). Despite being highly expressed by HSCs and progenitor cells (Choi et al., 2019), Vangl2 is best known for its crucial role in neural tube development and cell polarity (Kibar et al., 2001; Murdoch et al., 2001; Montcouquiol et al., 2003). Our results demonstrate an essential role for Vangl2 in HSC expansion and hematopoietic recovery post-transplant and suggest it could play a role in regulating age-associated hematopoietic alterations.
Hematopoietic aging is associated with the accumulation of cells expressing HSC markers but having more limited self-renewal capacity on an individual basis and presenting with a bias toward myeloid differentiation (Beerman et al., 2010; Dykstra et al., 2011; de Haan and Lazare, 2018). While Vangl2 was not required for normal hematopoiesis in young mice, aging 18-month-old Vangl2Δ/Δ mouse BM contained more myeloid-biased multipotent progenitor cells, and a similar trend could be observed in 6-month-old adults as well, suggesting a gradual effect of Vangl2 deletion in hematopoiesis that increases with age. This was not associated with an overall loss of HSC or progenitor cell quiescence, in contrast to what was reported for Fzd8−/− or Celsr2/Fmi−/- HSCs (Sugimura et al., 2012), as we observed no significant increase in Ki-67+ cycling cells in any age group. To the contrary, there was a slight but significant increase in the proportion of quiescent Vangl2Δ/Δ ST-HSCs, and Vangl2Δ/Δ HSCs and MPPs expressed increased levels of the cell cycle inhibitor p16INK4a. Although p16INK4a may not play a major role in normal HSC aging, its enhanced expression impairs HSC self-renewal (Oguro et al., 2006; Attema et al., 2009). Inversely, the absence of p16INK4a specifically enhances repopulation ability of BM cells from aged donors (Janzen et al., 2006). The absence of Vangl2 might thus exacerbate the effects of HSC aging by upregulating p16INK4a. Indeed, although there was a slight increase in the frequency of myeloid colony-forming cells in Vangl2Δ/Δ BM, in line with the accrued number of myeloid-biased MPPs, these colonies consisted of fewer cells, indicative of an attenuated proliferative potential. Moreover, while young Vangl2Δ/Δ BM remained functional in primary transplants, HSCs from 6-month-old Vangl2Δ/Δ donors showed decreased expansion and long-term myeloid reconstitution, demonstrating the impact of age on Vangl2Δ/Δ HSC function. Serial transplants with young BM donors further confirmed the decreased repopulating capacity of Vangl2Δ/Δ BM cells, suggesting that cell-intrinsic Vangl2 is required for HSC expansion and self-renewal post-transplant.
We have recently published that the deletion of Wnt4 in BM stem/progenitor cells also resulted in impaired hematopoietic recovery after transplant or sublethal irradiation and corresponded to an increased expression of p16INK4a in CD150+ LSKs (Hétu-Arbour et al., 2021b). Wnt4 is a putative ligand for Vangl2 in other cell types (Messéant et al., 2017), and the similarities in the results from the two studies suggest that this could also be the case in hematopoietic cells. p16INK4a upregulation in Wnt4Δ/Δ BM cells was further associated with increased JNK activity and cellular stress (Hétu-Arbour et al., 2021b). In addition to its role in vertebrate Wnt/PCP signaling, JNK is also induced in response to different types of cellular stress, and it can regulate p16INK4a expression via JunB (Passegue and Wagner, 2000). Vangl2 has been shown to phosphorylate JNK (Gao et al., 2011; Brunt et al., 2021), but it remains to be determined if JNK activity is altered in Vangl2Δ/Δ BM cells.
HSC self-renewal is dependent not only on the frequency of cell division but also on the type of division that takes place. Young adult BM HSCs would preferentially undergo asymmetric self-renewal divisions, thus generating daughter cells ready for differentiation while at the same time maintaining the size of the stem cell pool (Wu et al., 2007; Florian and Geiger, 2010; Ting et al., 2012; Florian et al., 2013). Increased Wnt5a/Cdc42 signaling has been shown to promote functional aging via loss of HSC polarity (Florian et al., 2012; Florian et al., 2013; Florian et al., 2018), whereas Wnt/PCP receptors appear to favor HSC self-renewal (Sugimura et al., 2012; Abidin et al., 2015; Lhoumeau et al., 2016), similar to what we found here for Vangl2. It must be noted that the role of PCP signaling in establishing HSC polarity remains unclear, and we detected no striking differences in Cdc42 distribution between Vangl2Δ/Δ and control CD150+ LSKs ex vivo, although we observed an age-dependent decrease in Cdc42 polarization in both genotypes. Nevertheless, it remains possible that Vangl2-dependent differences do exist in a more restricted subset of HSCs, or that their detection requires contact with niche cells or extracellular matrix. It is also possible that the impact of Vangl2 on cell division symmetry is Cdc42-independent. Wnt/PCP signaling has been shown to promote symmetric self-renewal divisions in satellite cells by establishing an asymmetrical distribution of Vangl2 that was essential for stem cell expansion (Le Grand et al., 2009). Unfortunately, we have not been able to find a good commercially available antibody to investigate Vangl2 localization in HSCs.
HSC aging has also been linked to a decrease in canonical β-catenin-dependent Wnt signaling (Florian et al., 2013), even though the functional link between the two remains to be confirmed. Although β-catenin stabilization is necessary for BM recovery after myeloablation (Lento et al., 2014), it is generally considered dispensable for HSC maintenance at steady state (Cobas et al., 2004; Luis et al., 2011). Non-canonical Wnt signalling can inhibit the Wnt/β-catenin canonical pathway, either by preventing β-catenin stabilization or by interfering with its translocation to the nucleus (Nemeth et al., 2007). Nuclear vs. cytoplasmic distribution of β-catenin was not altered in Vangl2Δ/Δ CD150+ LSKs. However, the intensity of β-catenin staining in old Vangl2Δ/Δ cells was significantly increased, suggesting an increased activation of the canonical pathway. Although the vast majority of CD150+ LSKs are CD48−, they do not represent a homogenous population of functional HSCs, especially in older mice. Moreover, given that the increase in β-catenin was only seen in aged Vangl2Δ/Δ mice, it could also be the result of adaptative changes in the BM environment in the absence of Vangl2, rather than the lack of direct Vangl2-mediated negative signaling in HSCs. However, chronic Wnt/β-catenin signalling has been shown to lead to cell senescence in other cell types (Gu et al., 2014), and lead to loss of function and exhaustion in HSCs (Scheller et al., 2006; Luis et al., 2011). The increased stabilization of β-catenin could thus contribute to the loss of Vangl2Δ/Δ HSC self-renewal.
Taken together, we have shown in this study the functional importance of the key Wnt/PCP component Vangl2 in HSC self-renewal and post-transplant recovery. This loss of repopulating ability corresponds to increased β-catenin and p16INK4a levels in Vangl2-deficient HSCs from aged mice, revealing new mechanisms by which Vangl2 regulates hematopoiesis and HSC function in an age-dependent manner.
Data Availability Statement
The raw data supporting the conclusions of this article will be made available by the authors, without undue reservation.
Ethics Statement
The animal study was reviewed and approved by Comité institutionnel de la protection des animaux (CIPA) de l'Institut national de la recherche scientifique (INRS).
Author Contributions
SB: Conception and design, data collection, data analysis, visualization and manuscript writing. RH-A: Data collection and analysis. CG: Data collection and analysis. KH: Supervision and funding, conception and design, data analysis, editing and final approval of manuscript.
Funding
This work was supported by the Natural Sciences and Engineering Research Council of Canada (NSERC Discovery grant #2018–05258) and the Canada Foundation for Innovation (CFI Leaders Fund #31377). KMH is a Fonds de recherche du Québec – Santé (FRQS) Junior Research Scholar. SB was supported by a Canada Graduate Scholarship from Canadian Institutes for Health Research (CIHR). The funders had no role in study design, data collection, analysis, or interpretation.
Conflict of Interest
The authors declare that the research was conducted in the absence of any commercial or financial relationships that could be construed as a potential conflict of interest.
Publisher’s Note
All claims expressed in this article are solely those of the authors and do not necessarily represent those of their affiliated organizations, or those of the publisher, the editors and the reviewers. Any product that may be evaluated in this article, or claim that may be made by its manufacturer, is not guaranteed or endorsed by the publisher.
Acknowledgments
We acknowledge the staff of the Laboratoire national de biologie expérimentale for their assistance in animal care.
Supplementary Material
The Supplementary Material for this article can be found online at: https://www.frontiersin.org/articles/10.3389/fcell.2022.760248/full#supplementary-material
References
Abidin, B. M., Hammami, A., Stäger, S., and Heinonen, K. M. (2017). Infection-Adapted Emergency Hematopoiesis Promotes Visceral Leishmaniasis. Plos Pathog. 13 (8), e1006422. doi:10.1371/journal.ppat.1006422
Abidin, B. M., Owusu Kwarteng, E., and Heinonen, K. M. (2015). Frizzled-6 Regulates Hematopoietic Stem/Progenitor Cell Survival and Self-Renewal. J. Immunol. 195 (5), 2168–2176. doi:10.4049/jimmunol.1403213
Attema, J. L., Pronk, C. J. H., Norddahl, G. L., Nygren, J. M., and Bryder, D. (2009). Hematopoietic Stem Cell Ageing Is Uncoupled from p16INK4A-Mediated Senescence. Oncogene 28 (22), 2238–2243. doi:10.1038/onc.2009.94
Beerman, I., Bhattacharya, D., Zandi, S., Sigvardsson, M., Weissman, I. L., Bryder, D., et al. (2010). Functionally Distinct Hematopoietic Stem Cells Modulate Hematopoietic Lineage Potential during Aging by a Mechanism of Clonal Expansion. Proc. Natl. Acad. Sci. U.S.A. 107 (12), 5465–5470. doi:10.1073/pnas.1000834107
Brunt, L., Greicius, G., Rogers, S., Evans, B. D., Virshup, D. M., Wedgwood, K. C. A., et al. (2021). Vangl2 Promotes the Formation of Long Cytonemes to Enable Distant Wnt/β-Catenin Signaling. Nat. Commun. 12 (1), 2058. doi:10.1038/s41467-021-22393-9
Chang, H., Smallwood, P. M., Williams, J., and Nathans, J. (2016). The Spatio-Temporal Domains of Frizzled6 Action in Planar Polarity Control of Hair Follicle Orientation. Develop. Biol. 409 (1), 181–193. doi:10.1016/j.ydbio.2015.10.027
Chapple, R. H., Tseng, Y.-J., Hu, T., Kitano, A., Takeichi, M., Hoegenauer, K. A., et al. (2018). Lineage Tracing of Murine Adult Hematopoietic Stem Cells Reveals Active Contribution to Steady-State Hematopoiesis. Blood Adv. 2 (11), 1220–1228. doi:10.1182/bloodadvances.2018016295
Choi, J., Baldwin, T. M., Wong, M., Bolden, J. E., Fairfax, K. A., Lucas, E. C., et al. (2019). Haemopedia RNA-Seq: A Database of Gene Expression during Haematopoiesis in Mice and Humans. Nucleic Acids Res. 47 (D1), D780–D785. doi:10.1093/nar/gky1020
Cobas, M., Wilson, A., Ernst, B., Mancini, S. J. C., MacDonald, H. R., Kemler, R., et al. (2004). β-Catenin Is Dispensable for Hematopoiesis and Lymphopoiesis. J. Exp. Med. 199 (2), 221–229. doi:10.1084/jem.20031615
de Boer, J., Williams, A., Skavdis, G., Harker, N., Coles, M., Tolaini, M., et al. (2003). Transgenic Mice with Hematopoietic and Lymphoid Specific Expression of Cre. Eur. J. Immunol. 33 (2), 314–325. doi:10.1002/immu.200310005
de Haan, G., and Lazare, S. S. (2018). Aging of Hematopoietic Stem Cells. Blood 131 (5), 479–487. doi:10.1182/blood-2017-06-746412
Dykstra, B., Olthof, S., Schreuder, J., Ritsema, M., and de Haan, G. (2011). Clonal Analysis Reveals Multiple Functional Defects of Aged Murine Hematopoietic Stem Cells. J. Exp. Med. 208 (13), 2691–2703. doi:10.1084/jem.20111490
Fleming, H. E., Janzen, V., Lo Celso, C., Guo, J., Leahy, K. M., Kronenberg, H. M., et al. (2008). Wnt Signaling in the Niche Enforces Hematopoietic Stem Cell Quiescence and Is Necessary to Preserve Self-Renewal In Vivo. Cell Stem Cell 2 (3), 274–283. doi:10.1016/j.stem.2008.01.003
Florian, M. C., and Geiger, H. (2010). Concise Review: Polarity in Stem Cells, Disease, and Aging. Stem Cells 28 (9), 1623–1629. doi:10.1002/stem.481
Florian, M. C., Dörr, K., Niebel, A., Daria, D., Schrezenmeier, H., Rojewski, M., et al. (2012). Cdc42 Activity Regulates Hematopoietic Stem Cell Aging and Rejuvenation. Cell stem cell 10 (5), 520–530. doi:10.1016/j.stem.2012.04.007
Florian, M. C., Klose, M., Sacma, M., Jablanovic, J., Knudson, L., Nattamai, K. J., et al. (2018). Aging Alters the Epigenetic Asymmetry of HSC Division. Plos Biol. 16 (9), e2003389. doi:10.1371/journal.pbio.2003389
Florian, M. C., Nattamai, K. J., Dörr, K., Marka, G., Überle, B., Vas, V., et al. (2013). A Canonical to Non-canonical Wnt Signalling Switch in Haematopoietic Stem-Cell Ageing. Nature 503 (7476), 392–396. doi:10.1038/nature12631
Gao, B., Song, H., Bishop, K., Elliot, G., Garrett, L., English, M. A., et al. (2011). Wnt Signaling Gradients Establish Planar Cell Polarity by Inducing Vangl2 Phosphorylation through Ror2. Develop. Cel 20 (2), 163–176. doi:10.1016/j.devcel.2011.01.001
Gu, Z., Tan, W., Feng, G., Meng, Y., Shen, B., Liu, H., et al. (2014). Wnt/β-Catenin Signaling Mediates the Senescence of Bone Marrow-Mesenchymal Stem Cells from Systemic Lupus Erythematosus Patients through the P53/p21 Pathway. Mol. Cel Biochem 387 (1), 27–37. doi:10.1007/s11010-013-1866-5
Heinonen, K. M., Vanegas, J. R., Lew, D., Krosl, J., and Perreault, C. (2011). Wnt4 Enhances Murine Hematopoietic Progenitor Cell Expansion through a Planar Cell Polarity-Like Pathway. PLoS ONE 6 (4), e19279. doi:10.1371/journal.pone.0019279
Hétu-Arbour, R., Bouali, S., and Heinonen, K. M. (2021). “Experimental Competitive Bone Marrow Transplant Assays,” in Leukemia Stem Cells: Methods and Protocols. Editors C. Cobaleda, and I. Sánchez-García (New York, NY: Springer US), 195–214. doi:10.1007/978-1-0716-0810-4_12
Hétu-Arbour, R., Tlili, M., Bandeira Ferreira, F. L., Abidin, B. M., Kwarteng, E. O., and Heinonen, K. M. (2021). Cell-Intrinsic Wnt4 Promotes Hematopoietic Stem and Progenitor Cell Self-Renewal. Stem Cells 39 (9), 1207–1220. doi:10.1002/stem.3385
Janzen, V., Forkert, R., Fleming, H. E., Saito, Y., Waring, M. T., Dombkowski, D. M., et al. (2006). Stem-Cell Ageing Modified by the Cyclin-Dependent Kinase Inhibitor p16INK4a. Nature 443 (7110), 421–426. doi:10.1038/nature05159
Kabiri, Z., Numata, A., Kawasaki, A., Edison, , , Tenen, D. G., and Virshup, D. M. (2015). Wnts are Dispensable for Differentiation and Self-Renewal of Adult Murine Hematopoietic Stem Cells. Blood 126 (9), 1086–1094. doi:10.1182/blood-2014-09-598540
Kibar, Z., Salem, S., Bosoi, C., Pauwels, E., De Marco, P., Merello, E., et al. (2011). Contribution of VANGL2 Mutations to Isolated Neural Tube Defects. Clin. Genet. 80 (1), 76–82. doi:10.1111/j.1399-0004.2010.01515.x
Kibar, Z., Vogan, K. J., Groulx, N., Justice, M. J., Underhill, D. A., and Gros, P. (2001). Ltap, a Mammalian Homolog of Drosophila Strabismus/Van Gogh, Is Altered in the Mouse Neural Tube Mutant Loop-Tail. Nat. Genet. 28 (3), 251–255. doi:10.1038/90081
Kwarteng, E. O., and Heinonen, K. M. (2016). Competitive Transplants to Evaluate Hematopoietic Stem Cell Fitness. J. Vis. Exp. 114, 54345. doi:10.3791/54345
Kwarteng, E. O., Hétu-Arbour, R., and Heinonen, K. M. (2018). Frontline Science: Wnt/β-Catenin Pathway Promotes Early Engraftment of Fetal Hematopoietic Stem/progenitor Cells. J. Leukoc. Biol. 103 (3), 381–393. doi:10.1002/jlb.1hi0917-373r
Le Grand, F., Jones, A. E., Seale, V., Scimè, A., and Rudnicki, M. A. (2009). Wnt7a Activates the Planar Cell Polarity Pathway to Drive the Symmetric Expansion of Satellite Stem Cells. Cell stem cell 4 (6), 535–547. doi:10.1016/j.stem.2009.03.013
Lento, W., Ito, T., Zhao, C., Harris, J. R., Huang, W., Jiang, C., et al. (2014). Loss of β-Catenin Triggers Oxidative Stress and Impairs Hematopoietic Regeneration. Genes Dev. 28 (9), 995–1004. doi:10.1101/gad.231944.113
Lhoumeau, A.-C., Arcangeli, M.-L., De Grandis, M., Giordano, M., Orsoni, J.-C., Lembo, F., et al. (2016). Ptk7-Deficient Mice Have Decreased Hematopoietic Stem Cell Pools as a Result of Deregulated Proliferation and Migration. J. Immunol. 196 (10), 4367–4377. doi:10.4049/jimmunol.1500680
Li, H. W., and Sykes, M. (2012). Emerging Concepts in Haematopoietic Cell Transplantation. Nat. Rev. Immunol. 12 (6), 403–416. doi:10.1038/nri3226
Luis, T. C., Ichii, M., Brugman, M. H., Kincade, P., and Staal, F. J. T. (2012). Wnt Signaling Strength Regulates normal Hematopoiesis and its Deregulation Is Involved in Leukemia Development. Leukemia 26 (3), 414–421. doi:10.1038/leu.2011.387
Luis, T. C., Naber, B. A. E., Roozen, P. P. C., Brugman, M. H., de Haas, E. F. E., Ghazvini, M., et al. (2011). Canonical Wnt Signaling Regulates Hematopoiesis in a Dosage-Dependent Fashion. Cell Stem Cell 9 (4), 345–356. doi:10.1016/j.stem.2011.07.017
Messéant, J., Ezan, J., Delers, P., Glebov, K., Marchiol, C., Lager, F., et al. (2017). Wnt Proteins Contribute to Neuromuscular junction Formation through Distinct Signaling Pathways. Development 144 (9), 1712–1724. doi:10.1242/dev.146167
Montcouquiol, M., Rachel, R. A., Lanford, P. J., Copeland, N. G., Jenkins, N. A., and Kelley, M. W. (2003). Identification of Vangl2 and Scrb1 as Planar Polarity Genes in Mammals. Nature 423 (6936), 173–177. doi:10.1038/nature01618
Murdoch, J. N., Doudney, K., Paternotte, C., Copp, A. J., and Stanier, P. (2001). Severe Neural Tube Defects in the Loop-Tail Mouse Result from Mutation of Lpp1, a Novel Gene Involved in Floor Plate Specification. Hum. Mol. Genet. 10 (22), 2593–2601. doi:10.1093/hmg/10.22.2593
Nemeth, M. J., Topol, L., Anderson, S. M., Yang, Y., and Bodine, D. M. (2007). Wnt5a Inhibits Canonical Wnt Signaling in Hematopoietic Stem Cells and Enhances Repopulation. Proc. Natl. Acad. Sci. U.S.A. 104 (39), 15436–15441. doi:10.1073/pnas.0704747104
O'Connell, K. E., Mikkola, A. M., Stepanek, A. M., Vernet, A., Hall, C. D., Sun, C. C., et al. (2015). Practical Murine Hematopathology: A Comparative Review and Implications for Research. Comp. Med. 65 (2), 96–113.
Oguro, H., Iwama, A., Morita, Y., Kamijo, T., van Lohuizen, M., and Nakauchi, H. (2006). Differential Impact of Ink4a and Arf on Hematopoietic Stem Cells and Their Bone Marrow Microenvironment in Bmi1-Deficient Mice. J. Exp. Med. 203 (10), 2247–2253. doi:10.1084/jem.20052477
Passegue, E., and Wagner, E. F. (2000). JunB Suppresses Cell Proliferation by Transcriptional Activation of p16(INK4a) Expression. EMBO J. 19 (12), 2969–2979. doi:10.1093/emboj/19.12.2969
Renström, J., Istvanffy, R., Gauthier, K., Shimono, A., Mages, J., Jardon-Alvarez, A., et al. (2009). Secreted Frizzled-Related Protein 1 Extrinsically Regulates Cycling Activity and Maintenance of Hematopoietic Stem Cells. Cell stem cell 5 (2), 157–167. doi:10.1016/j.stem.2009.05.020
Schaniel, C., Sirabella, D., Qiu, J., Niu, X., Lemischka, I. R., and Moore, K. A. (2011). Wnt-Inhibitory Factor 1 Dysregulation of the Bone Marrow Niche Exhausts Hematopoietic Stem Cells. Blood 118 (9), 2420–2429. doi:10.1182/blood-2010-09-305664
Scheller, M., Huelsken, J., Rosenbauer, F., Taketo, M. M., Birchmeier, W., Tenen, D. G., et al. (2006). Hematopoietic Stem Cell and Multilineage Defects Generated by Constitutive β-Catenin Activation. Nat. Immunol. 7 (10), 1037–1047. doi:10.1038/ni1387
Seifert, J. R. K., and Mlodzik, M. (2007). Frizzled/PCP Signalling: A Conserved Mechanism Regulating Cell Polarity and Directed Motility. Nat. Rev. Genet. 8 (2), 126–138. doi:10.1038/nrg2042
Staal, F. J. T., Luis, T. C., and Tiemessen, M. M. (2008). WNT Signalling in the Immune System: WNT Is Spreading its Wings. Nat. Rev. Immunol. 8, 581–593. doi:10.1038/nri2360
Stahl, F. R., Jung, R., Jazbutyte, V., Ostermann, E., Tödter, S., Brixel, R., et al. (2018). Laboratory Diagnostics of Murine Blood for Detection of Mouse Cytomegalovirus (MCMV)-Induced Hepatitis. Sci. Rep. 8 (1), 14823. doi:10.1038/s41598-018-33167-7
Sugimura, R., He, X. C., Venkatraman, A., Arai, F., Box, A., Semerad, C., et al. (2012). Noncanonical Wnt Signaling Maintains Hematopoietic Stem Cells in the Niche. Cell 150 (2), 351–365. doi:10.1016/j.cell.2012.05.041
Sun, J., Ramos, A., Chapman, B., Johnnidis, J. B., Le, L., Ho, Y.-J., et al. (2014). Clonal Dynamics of Native Haematopoiesis. Nature 514 (7522), 322–327. doi:10.1038/nature13824
Szade, K., Bukowska-Strakova, K., Zukowska, M., Jozkowicz, A., and Dulak, J. (2016). “Analysis of Cell Cycle Status of Murine Hematopoietic Stem Cells,” in Stem Cell Heterogeneity: Methods and Protocols. Editor K. Turksen (New York, NY: Springer New York), 91–99. doi:10.1007/7651_2016_361
Ting, S. B., Deneault, E., Hope, K., Cellot, S., Chagraoui, J., Mayotte, N., et al. (2012). Asymmetric Segregation and Self-Renewal of Hematopoietic Stem and Progenitor Cells with Endocytic Ap2a2. Blood 119 (11), 2510–2522. doi:10.1182/blood-2011-11-393272
Wang, Y., Guo, N., and Nathans, J. (2006). The Role of Frizzled3 and Frizzled6 in Neural Tube Closure and in the Planar Polarity of Inner-Ear Sensory Hair Cells. J. Neurosci. 26 (8), 2147–2156. doi:10.1523/jneurosci.4698-05.2005
Keywords: hematopoietic stem cells, Vangl2, wnt/planar cell polarity pathway, bone marrow transplants, hematopoietic recovery
Citation: Bouali S, Hétu-Arbour R, Gardet C and Heinonen KM (2022) Vangl2 Promotes Hematopoietic Stem Cell Expansion. Front. Cell Dev. Biol. 10:760248. doi: 10.3389/fcell.2022.760248
Received: 17 August 2021; Accepted: 28 February 2022;
Published: 24 March 2022.
Edited by:
Christoph Schaniel, Icahn School of Medicine at Mount Sinai, United StatesReviewed by:
Yoon-A Kang, Columbia University Irving Medical Center, United StatesSaghi Ghaffari, Icahn School of Medicine at Mount Sinai, United States
Julia Fröbel, Fritz Lipmann Institute (FLI), Germany
Copyright © 2022 Bouali, Hétu-Arbour, Gardet and Heinonen. This is an open-access article distributed under the terms of the Creative Commons Attribution License (CC BY). The use, distribution or reproduction in other forums is permitted, provided the original author(s) and the copyright owner(s) are credited and that the original publication in this journal is cited, in accordance with accepted academic practice. No use, distribution or reproduction is permitted which does not comply with these terms.
*Correspondence: Krista M. Heinonen, a3Jpc3RhLmhlaW5vbmVuQGlucnMuY2E=