- 1Independent Researcher, New Delhi, India
- 2Department of Zoology, Deshbandhu College, University of Delhi, Delhi, India
- 3Stem Cell and Gene Therapy Research Group, Institute of Nuclear Medicine and Allied Sciences (INMAS), Delhi, India
- 4Immunogenetics and Transplantation Laboratory, Department of Surgery, University of California San Francisco, San Francisco, CA, United States
Hematopoietic stem cells (HSCs) possess two important properties such as self-renewal and differentiation. These properties of HSCs are maintained through hematopoiesis. This process gives rise to two subpopulations, long-term and short-term HSCs, which have become a popular convention for treating various hematological disorders. The clinical application of HSCs is bone marrow transplant in patients with aplastic anemia, congenital neutropenia, sickle cell anemia, thalassemia, or replacement of damaged bone marrow in case of chemotherapy. The self-renewal attribute of HSCs ensures long-term hematopoiesis post-transplantation. However, HSCs need to be infused in large numbers to reach their target site and meet the demands since they lose their self-renewal capacity after a few passages. Therefore, a more in-depth understanding of ex vivo HSCs expansion needs to be developed to delineate ways to enhance the self-renewability of isolated HSCs. The multifaceted self-renewal process is regulated by factors, including transcription factors, miRNAs, and the bone marrow niche. A developed classical hierarchical model that outlines the hematopoiesis in a lineage-specific manner through in vivo fate mapping, barcoding, and determination of self-renewal regulatory factors are still to be explored in more detail. Thus, an in-depth study of the self-renewal property of HSCs is essentially required to be utilized for ex vivo expansion. This review primarily focuses on the Hematopoietic stem cell self-renewal pathway and evaluates the regulatory molecular factors involved in considering a targeted clinical approach in numerous malignancies and outlining gaps in the current knowledge.
1 Introduction
The bone marrow resident hematopoietic stem cells (HSCs) direct the process of hematopoiesis (Rieger and Schroeder, 2012). The earliest experimental evidence of the HSCs function was reported when the transplantation of bone marrow (BM) into irradiated mice gave rise to myeloid lineage cells within the spleen of the transplanted mice (Bertrand et al., 2010). The resultant restoration of the differentiated blood cell population was a presupposed function of the HSCs by their stemness, self-renewal property, and asymmetric cell division (Post and Clevers, 2019). Studies revealed that the HSCs rich niches, include BM, peripheral blood, umbilical cord, or fetal liver (Cheng et al., 2020).
Fetal BM HSCs were isolated for the first time in 1992, disclosing their property of asymmetric cell division upon functional characterization (Cheng et al., 2020). HSCs divide into two daughter cells with similar properties: long-term HSCs (LT-HSCs) and short-term (ST-HSCs) (Yang et al., 2005). LT-HSCs are a population of quiescent cells residing in the BM with a self-renewal capacity retained for greater than 6 months (Cheng et al., 2020). However, ST-HSCs are lineage-committed, depending on the niche’s intrinsic and extrinsic signals, and thus cannot sustain their self-renewal property for more than a month (Cheng et al., 2020). ST-HSCs differentiate into hematopoietic progenitor cells (HPCs), which further segregate into common myeloid progenitors (CMPs) and common lymphoid progenitors (CLPs). The CMPs give rise to granulocyte-macrophage progenitors (GMPs) and megakaryocyte-erythrocyte progenitors (MEPs). The GMPs form granulocytes, monocytes, and dendritic cells, while MEPs transform into erythrocytes and megakaryocytes. The CLPs, on the other hand, give rise to T, B, NK, and dendritic cells (Cheng et al., 2020).
Genetically modified mouse models are commonly used to delineate the hematopoietic niche and specific HSC populations because of the ability to control the time and location of somatic mutation in such models. Tie2− Cre model is a common in vivo fate mapping system specific to HSCs wherein the Tie2 gene was manipulated using the Cre/loxP system. The Tie2+ labeled LT-HSCs amplify the ST-HSCs and multipotent progenitors (MPPs) populations validating the spot of LT-HSCs at the top of the lineage map (Höfer and Rodewald, 2018). The progenitor cells lack self-renewal potency, observed in transplantation of donor stem or progenitor cells in myeloablated mice, wherein these transit-amplifying cell types were unable to restore hematopoiesis completely (Höfer and Rodewald, 2018; Busch et al., 2015). However, further quantification revealed that these progenitors possess self-renewal potential lesser than that of LT-HSCs, in addition to their transiting nature (Höfer and Rodewald, 2018; Busch et al., 2015). However, myeloid progenitor cells lack self-renewal since it is tightly regulated by various endogenous molecules, including transcription factors (TFs), cytokines, niche conditions, and epigenetic modifiers (Table 1) (Sun et al., 2014; Busch et al., 2015; Höfer et al., 2016; Raghav and Gangenahalli, 2018). Mx1-Cre is another inducible tool in which Cre recombinase is activated under the Mx1 promoter by either synthetically designed dsRNA that induces production of interferons IFN-α and IFN-β, or other immunostimulants like polyinosinic: polycytidylic acid (pIpC) (Joseph et al., 2013). This immunostimulation is specific to HSCs that have been contrived to visualize the perivascular cells located close to the endosteum, and the involvement of proteins like Ash2l required to differentiate early hematopoietic progenitor cells by epigenetic regulation (Lüscher-Firzlaff et al., 2019). Nonetheless, it does not have as wide-scale application as Tie2− Cre since this system is usually induced by IFN cytokine and has been reported as a low detectable rate of Cre induction by the endogenous IFN levels itself. It is also known to change the immunophenotype of HSCs transiently thus has been disregarded as a standard tool (Velasco-Hernandez et al., 2016). Additionally, Vav-Cre Vav-iCre strains have been exploited to study gene deletions in the hematopoietic system. Vav gene in the mice encodes for the GEF protein, expressed almost exclusively in the adult HSCs. However, the Vav gene also shows some expression levels in the testicular germ cells and placental trophoblasts. Therefore, in addition to the Vav-Cre strain, a codon improved Vav-iCre model was developed, which is less susceptible to epigenetic silencing [11]. Locking the Vav sites with a GFP gene introduced a new Vav-GFP transgenic line which selectively labels only the nucleated HSCs population. The role of Runx1 and Atg-7 driven autophagy in HSCs homeostasis was hence determined (Speck and Iruela-Arispe, 2009; Hashimoto et al., 2021).
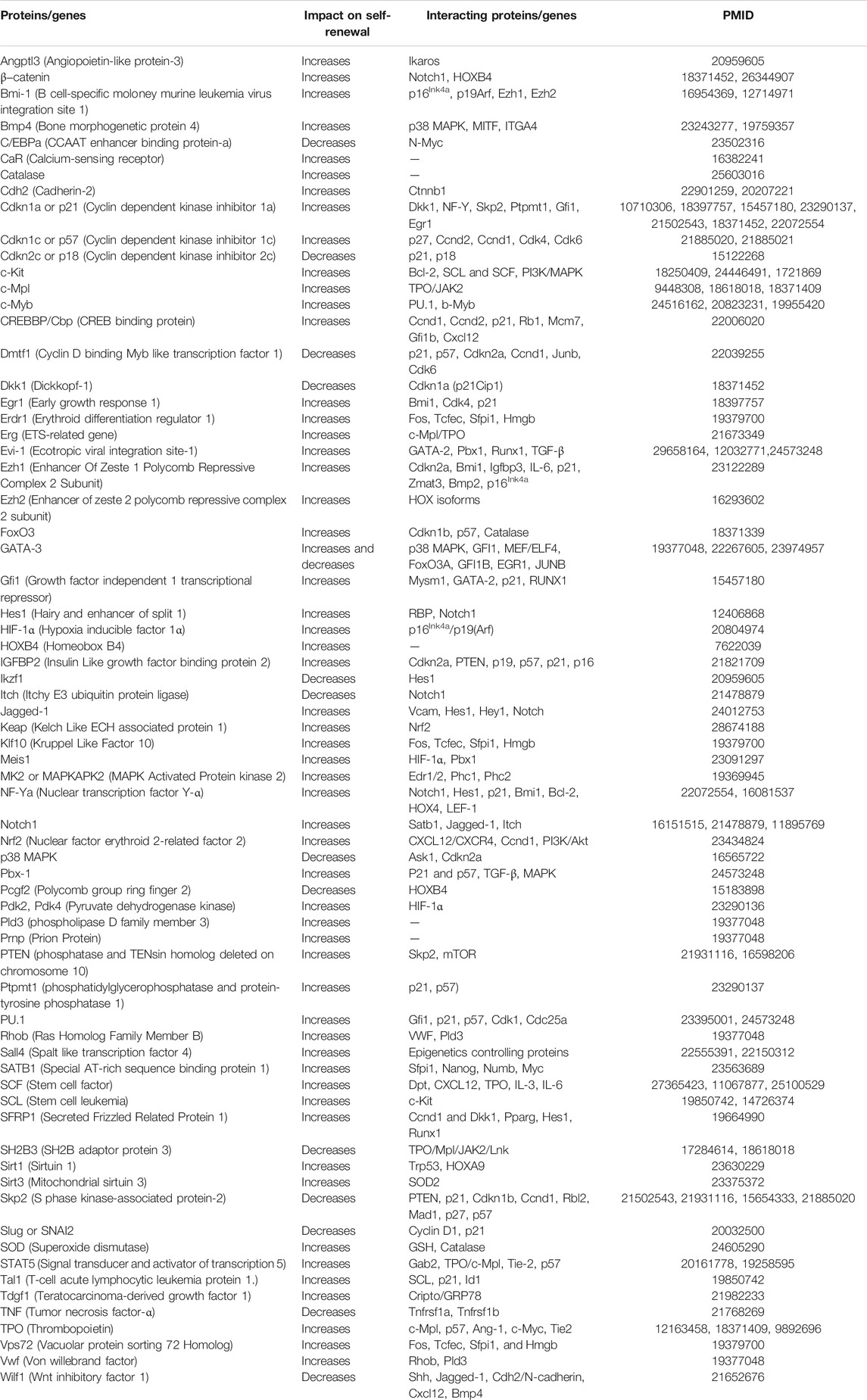
TABLE 1. Proteins/genes, their impact on self-renewal, and their interaction with other proteins/genes involved in regulating hematopoiesis.
Blood cancers account for 10% of all cancer cases. Any disruption in HSCs functioning leads to various hematological malignancies such as leukemia, myeloma, and lymphoma. These three cancer types can be further categorized into acute myeloid leukemia (AML), B-cell acute lymphoblastic leukemia (B-ALL), T-cell ALL (T-ALL), myelodysplastic syndrome (MDS), myeloproliferative neoplasm (MPN), chronic lymphocytic leukemia (CLL), and follicular lymphoma (FL) (Taylor et al., 2017).
Leukemic growth and HSCs maintenance is also regulated by the metabolic pathways. Among the 22 amino acids, leukemic niche is heavily dependent on amino acid metabolism for sustenance of leukemic stem cells (LSCs), unlike HSCs which are only affected negatively by valine deficiency (Tabe et al., 2019). The niche cells secrete certain amino acids that modulate the functioning of the HSCs and LSCs. Understanding of these metabolic patterns is fundamental to pave a way to amino-acid based leukemia targeting therapies. For instance, L-asparaginase shows excellent results in acute lymphoid leukemia (ALL) treatment. The stromal cells secrete asparagine and cysteine which support tumor metabolism as well as glutamine secretion by adipocytes. These mechanisms are considered important in TCA cycle and activation of NK and T cells (Tabe et al., 2019). Thus, their respective inhibitors asparaginase, cysteinase and GLS inhibitor have shown promising clinical outcomes in amino acid depletion and hence, tumor suppression (Fultang et al., 2021). However, a recent study established that only the LSCs are dependent on amino acid metabolism for their functionality and survival (Jones et al., 2018). Similarly, pleiotrophin, a heparin binding growth factor secreted by the human brain and marrow sinusoidal endothelial cells, supports LT-HSCs expansion and plays a critical role in self-renewal (Himburg et al., 2012). Additionally, HSCs also rely on fatty acid oxidation (FAO) for asymmetric cell division since disrupting this pathway causes loss of HSCs quiescence and enhanced differentiation leading to exhaustion of HSCs pool (Miyamoto et al., 2007; Ito and Suda, 2014; Ito et al., 2016). Peroxisome proliferator-activated receptor δ (PPARδ) acts as a transcription factor for FAO regulators and its suppression decreases ATP levels and drives HSCs more towards differentiation. Additionally, Pink/PARKIN mediate autophagy within HSCs to control FAO rates and thus, their maintenance by self-renewal and quiescence.
The self-renewability of HSCs is exploited in this aspect to serve as a therapeutic strategy to restore hematopoietic hierarchical progenitors via myeloid, lymphoid, and erythroid intermediates. LT-HSCs derived from BM, peripheral blood, or umbilical cord are used in transplantation. However, direct aspiration of these tissue-specific HSCs is considered invasive, and the doses administered to the patients need to be standardized case to case (Morrison et al., 1995; Cheng et al., 2020). Therefore, ex vivo expansion strategies to enhance the self-renewal and proliferation of HSCs need to be devised. For instance, it has been shown that stem cell factor (SCF) and Src homology 2 domain-containing protein tyrosine phosphatase 1 (SHP-1) inhibitor, 8-Hydroxy-7-(6-sulfonaphthalen-2-yl)diazenyl-quinoline-5-sulfonic acid, Disodium Salt (NSC87877) synergism activates c-Kit and inhibits c-Kit negative regulators (SHP-1/SHP-2) that can be used clinically to enhance cellular proliferation of both erythroid and megakaryoblast cells (Raghav et al., 2018a; Raghav et al., 2018b).
Moreover, extracellular vesicles (EVs) are 30 to 10,000 nm sized cargo encapsulating lipid bilayers, and can be further categorized into exosomes and microvesicles based on their size. HSCs secreted exosomes typically fall within the range 30–120 nm (Grenier-Pleau and Abraham, 2021). The EVs secreted by mesenchymal stem cells (MSCs) are cargoes for miRNAs and piRNAs, which regulate hematopoietic processes by HSCs uptake. IL-4 stimulated macrophages secrete EVs enriched with miRNA-99a/146b/378a, which reduce inflammation by NF-κB and TNF-α targeting and hence, also restrict HPCs expansion. Also, AML-derived EVs suppress HPCs clonogenicity since they are enriched with DKK-1, IL-6 and CCL3 reponsible for leukemic growth and disease progression. Thus, EV-based therapies is another new direction of clinical therapies which is being better understood these days for hematological malignancies.
A better understanding of the transcriptional process regulating the self-renewal of HSCs is essential to enhance the shelf-life and potency of HSCs so that the frequency of HSCs isolation from donors and the invasiveness of the process can be minimized. This review summarizes the intrinsic factors that affect HSCs homeostasis and lineage commitment (Figure 1).
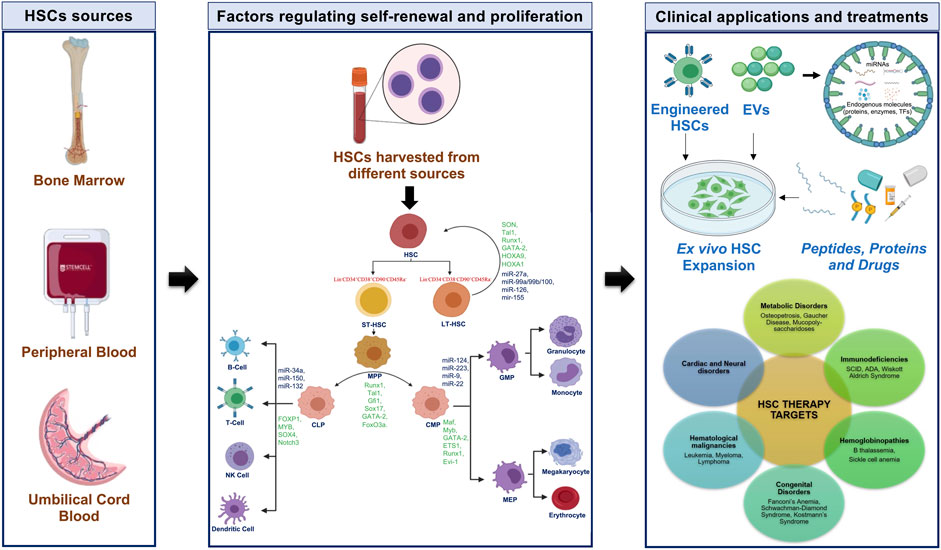
FIGURE 1. Human hematopoiesis with characteristic markers, TFs, and miRNAs with potential approaches for HSCs in clinical applications. CLP-common lymphoid progenitor; CMP-common myeloid progenitor; EVs-extracellular vesicles; GMP-granulocyte-macrophage progenitor; HSC-hematopoietic stem cell; LT-HSC-long-term HSC; MEP-megakaryocyte-erythrocyte progenitor; MPP-multipotent progenitor; NK-natural killer; ST-HSC-short-term HSC; TFs-transcription factors.
2 Hematopoiesis
In vivo evidence and colony-forming assays revealed two primary characteristics of HSCs-self-renewability and multipotency (Morrison et al., 1995; Orkin, 2000; Reya et al., 2001; Pazianos et al., 2003; Wilson et al., 2008; Foudi et al., 2009; Raghav et al., 2018a; Raghav et al., 2018b; Cheng et al., 2020; Grenier-Pleau and Abraham, 2021). The self-renewability of HSCs commands its top hierarchy in the classical lineage model of hematopoiesis.
The first evidence of the cell cycle dynamics of murine HSCs revealed that 99% of HSCs undergo cell division every 2 months, usually remaining in the G0 phase (Post and Clevers, 2019). Thereafter conducted in vivo studies revealed that dormant HSCs carry the highest self-renewal potential among all other blood cells (Wilson et al., 2008; Foudi et al., 2009). This quiescent HSCs subset arises through asymmetric division, retaining long-term self-renewal potential and producing an actively dividing progenitor subset that maintains the lineage commitment (Post and Clevers, 2019).
Ex vivo expansion of HSCs has established considerable heterogeneity within the HSCs pool due to asymmetric cell division (Post and Clevers, 2019; Wilkinson et al., 2019). This leads to the production of actively proliferating MPPs that differentiate step-wise into a restricted lineage based on the signals received from the niche, including the cytokines and TFs. This phenomenon was established through an immunophenotype-based tree-like hierarchy model given by Weissman’s group, which describes the classic HSCs harboring self-renewal property retained for more than three rounds of transplantation when delivered into recipients that can sustain hematopoiesis (Kondo et al., 1997; Morrison et al., 1997; Akashi et al., 2000; Manz et al., 2002; Cheng et al., 2020). Nevertheless, lack of equilibrium between the labeled HSCs and their progeny revealed that mature blood cells are replenished by a source of progenitor cells that differ from the HSCs in their cell cycle dynamics and possess higher self-renewability (Busch et al., 2015; Cheng et al., 2020).
In summary, symmetric or asymmetric cell divisions within the HSCs pyramid control self-renewability, egression from BM, and apoptosis. The proliferation rate of HSCs is almost twice that of the differentiation rate, establishing that a sub-population of HSCs maintains its pool by replacing the lost cells either through regression or cell death. Thus, symmetric cell division is more prevalent within the BM niche than asymmetric divisions (Cheng et al., 2020).
3 Classical Hierarchical Map
3.1 In vivo Fate Mapping
In vivo fate mapping enabled quantification of the net proliferation rate of the HSCs. The output is determined by the differentiation rate that balances the net proliferation as downstream components of the lineage model are modulated by the upstream components (Busch et al., 2015; Cheng et al., 2020). This sustains the self-renewal potential, the hallmark of hematopoiesis, and safeguards the HSCs lineage pool from the emergence of malignancies since the mutation rate is higher in the rapidly proliferating progenitor cells. The downstream components can be classified as two different populations based on the surface markers present. Hierarchy-wise, LT-HSCs differentiate into ST-HSCs, which further transform into MPPs, losing their self-renewability (Kondo et al., 1997; Morrison et al., 1997; Akashi et al., 2000; Yang et al., 2005; Cheng et al., 2020).
The MPPs subsequently bifurcate into CLPs and CMPs cell types, differentiating into the lymphoid and myeloid lineages, respectively (Yang et al., 2005; Cheng et al., 2020). The CLPs produce B, T, NK, and dendritic cells lineages, while CMPs differentiate into 1) bipotent GMPs, differentiating into granulocytes and monocytes; and 2) MEPs generating megakaryocytes and erythrocytes (Zhu and Emerson, 2002; Robb, 2007; Metcalf, 2008; Zhang and Lodish, 2008; Seita and Weissman, 2010).
Inducible genetic systems have been developed for label retention assays to gain more in-depth insight into the self-renewal and differentiation patterns of HSCs (Upadhaya et al., 2018). The existence of a dormant and rare HSCs subset was studied with the H2B-GFP expression model designed to regulate the CD34-tTA transgene system (Upadhaya et al., 2018). Herein, a small subpopulation was observed to retain the label stably even after 22 months. These cells had an excellent potency for repopulation in serial transplantation, while a large chunk of the population lost the label after four rounds of cell division (Bernitz et al., 2016). This revealed the presence of the quiescent HSCs population reserved even with progressing age. This subset of HSCs is also confirmed to have elevated retinoic acid (RA) signaling, as disclosed by G-protein coupled receptor family C group 5 member C (Gprc5c) labeling. Nevertheless, more studies are needed to confirm the effects of this signaling on HSCs self-renewal (Ghiaur et al., 2013; Upadhaya et al., 2018).
Fate mapping and physiological HSCs output are determined quantitatively and qualitatively by genetic labeling and barcoding (Emmons et al., 2017; Höfer and Rodewald, 2018). While the former labeling system is suitable for delineating the kinetics of self-renewal and differentiation rates of HSCs, the latter is more apt in probing physiological progenitor-product relationships (Emmons et al., 2017). Time-resolved fate mapping also confirmed the two kinds of the hematopoietic population: the dormant LT-HSCs and the actively dividing ST-HSCs (Lajtha et al., 1962; Trumpp et al., 2010; Emmons et al., 2017; Grinenko et al., 2018). Several in vivo fate mapping models have been established for an in-depth study of the same, an important one being the Tie2-Cre mouse model, wherein labeled Tie2+ cells are heritable at different stages of ontogeny (Gomez Perdiguero et al., 2015; Emmons et al., 2017). Tie2+ HSCs are placed at the top of the lineage chart, potent enough to generate all cell types downstream and maintained within the marrow throughout the mouse’s lifespan, confirming the self-renewal function (Foudi et al., 2009; Busch et al., 2015). Additionally, paired daughter assay revealed that symmetrically dividing Tie2+ cells sustain within the BM niche for a steady-state production of the HSCs (Cao and Ma, 2011).
In addition, HSCs display spleen colony-forming unit activity as determined by transplantation into lethally irradiated mice (Emmons et al., 2017). MPPs and associated progenitor cells are single cells that produce multiple progenies within the spleen to form secondary colonies during re-transplantation. It is possible due to the underlying self-renewal property of the cells (Ema et al., 2006; Emmons et al., 2016). In contrast, the barcoding experiments use unique transposon integration sites within fetal HSCs to delineate the precursor-progeny link within the adult HSCs pool (De Lisio et al., 2011). The erythroid progenitors and granulocytes share similar barcodes, implying a shared lineage of origin throughout fetal development (Bellows et al., 2011). Around two-thirds of these barcodes are also shared by CMPs, suggesting shared lineage. However, the remaining one-third population of erythroid progenitors and granulocytes with a different set of barcodes from the CMPs is plausibly due to limited self-renewal of CMPs within BM (Baker et al., 2011; Emmons et al., 2017).
3.2 Lineage Markers
Single-cell transplantation studies have established that multi-, oligo-, bi-, and uni-potent hematopoietic progenitors co-exist within the same niche (Cheng et al., 2020). Although the classical hierarchical model helps to visualize the hematopoietic process and marker-based isolation of individual cells, the cellular schematics defining the complexity of hematopoietic maturation at genetic and epigenetic levels are difficult to predict accurately (Xavier-Ferrucio and Krause, 2018).
Classic markers for mouse HSCs are Lin−, c-Kit+, Sca1+, Flk2-, CD34− and Slamf1+, while human HSCs markers include Lin−, CD34+, CD38+ and CD90+. However, the expression levels for these markers vary between LT-HSCs and ST-HSCs (Huang et al., 2007). LT-HSCs are Slamf1+CD34-Flk2−CD34−, with levels of Lin-c-Kit+Sca1+ constant between the two population set in mice. In contrast, human LT-HSCs are CD34−CD90+ with the same expression levels in Lin−CD38−CD45Ra− in ST-HSCs. The signaling lymphocyte activation molecule (SLAM) family markers, particularly CD150 and CD229, segregate mouse HSCs based on their potency. CD229- LT-HSCs commit to myeloid lineage, while CD229+ HSCs commit to lymphoid lineage (Oguro et al., 2013). CD150hi cells, on the other hand, have higher self-renewability than CD150med HSCs and differentiate into myeloid lineage (Morita et al., 2010; Moignard et al., 2013).
Other markers identified through single-cell RNA sequencing (scRNA-seq) and single-cell assay for transposase-accessible chromatin using sequencing (scATAC-seq) revealed heterogeneity within the HSCs population (Moignard et al., 2013; Pietras et al., 2015; Wilson et al., 2015; Nestorowa et al., 2016; Buenrostro et al., 2018; Laurenti and Göttgens, 2018; Jacobsen and Nerlov, 2019). These cells are segregated into three main classes: LT-HSCs, ST-HSCs, and MPPs (Morita et al., 2010; Wilson et al., 2015; Post and Clevers, 2019). The ST-HSCs develop into MPPs, grouped into MPP1, MPP2, MPP3, and MPP4 populations as per their immunophenotypes and lineage bias. MPP1 resembles LT-HSCs with >4 months of multi-lineage reconstitution potency, while MPP2, MPP3, and MPP4 exhibit <1 month of the myeloid lineage potential (Manz et al., 2002; Wilson et al., 2008). Similarly, vWF+ platelet-primed HSCs also possess prolonged myeloid lineage bias and self-renewability (Sanjuan-Pla et al., 2013; Shin et al., 2014). The subsequent differentiated population sets have a constant expression pattern of markers Lin-c-Kit+ Sca1- in mice and Lin-CD34+CD38+CD45Ra- in humans. The difference in mouse and human CLPs is marked by Flk2+IL7Ra+CD27+ and CD10+ set of markers, respectively. Concurrently, mouse CMPs are identified as CD34+ FcgRlow and human CMPs as IL3Ralow. MEPs in mice express the markers set CD34-FcgR-, and IL3Ra- in humans, whereas GMPs express CD34−FcgR+ and IL3Ra+ in mice and humans, respectively.
The transition within these three cell types is modulated by TFs such as Runx1, Tal1/SCL, Gfi1, Sall4, Etv6, Sox17, GATA-2, and FoxO3a. NFIL3 is expressed in CLPs and is required in innate lymphoid cell development within the BM (Sui et al., 1998). Similarly, T-cell factor 1 is essential for the differentiation of CLPs into natural killer T-cell progenitors. Another important TF is PU.1, functioning downstream of Runx1, controlling myeloid and B-cell differentiation (Heintz, 2001). PU.1 also regulates T-cell differentiation through Myb, T-cell factor 7, and GATA-3, checked by the Notch signaling (Post and Clevers, 2019). A comparison of the characteristic markers in mouse and human hematopoietic cell pools has been summarized in Table 2.
4 Factors for Hematopoietic Stem Cells Stemness and Self-Renewal
HSCs constitute <0.01% cells of the total BM population and maintain the hematopoietic system’s stability, owing to their self-renewal and differentiation potency (Shin et al., 2014; Upadhaya et al., 2018). The self-renewal property of LT-HSCs sustains the hematopoietic population, while the proliferation and differentiation capacity of ST-HSCs is empirical to produce multipotent progenitors (Emmons et al., 2017).
Extrinsic and intrinsic factors control the dynamic cellular organization within the hematopoietic system. Extrinsic factors include growth factors, hypoxia, and morphogens that activate signaling pathways, whereas intrinsic factors encompassing TFs, cell cycle regulators, epigenetic proteins, and miRNAs, as summarized in Table 1 (Manz et al., 2002; Beerman and Rossi, 2014; Zhao et al., 2014; Emmons et al., 2017).
The niche in which the cells reside is rich in TFs, miRNAs, and other mediators that provide a physical microenvironment for HSCs functioning. Quiescent HSCs reside within a perivascular niche, rich in endothelial cells and mesenchymal stem cells (MSCs) (Kunisaki et al., 2013; Morrison and Scadden, 2014; Scadden, 2014; Cabezas-Wallscheid and Trumpp, 2016; Pouzolles et al., 2016). The sinusoids localize approximately 85% of HSCs near Lepr+ and CXCL12hi cells (Pouzolles et al., 2016). The hematopoietic progenitors migrate from the BM to the thymus settle near mKitL+ vascular endothelial cells, later shifting near mKitL+ cortical thymic epithelial cells (Pouzolles et al., 2016). This thymic niche is critical in supporting early progenitors’ differentiation into multiple hematopoietic lineages (Buono et al., 2016).
The BM is the primary site of hematopoiesis and this niche includes the endosteal and vascular niches (Tamma and Ribatti, 2017). The endosteal niche is localized in the inner bone shell of the trabecular and andocortical surfaces, primarily composed of osteoblasts, osteoclasts, macrophages, fibroblasts, endothelial cells and adipocytes. HSCs interact with osteoblasts via Tie2 and Ang-1 respectively, to maintain adhesion between the two cell types and thereby HSCs quiescence (Birbrair and Frenette, 2016). Immature osteoblasts with elevated Runx2 expression or those undergoing cell death lead to loss of hematopoiesis. Hence to compensate, immature osteoblasts upregulate CXCL12 surface expression to bind with CXCR4 in HSCs and support their maintenance. CXCL12 expression is however, negatively regulated by granulocyte-colony stimulating factor (G-CSF). Osteoblasts also negatively regulate HSCs pool via osteopontin surface expression.
The second type of niche is within the blood vessels called the vascular niche (Mirantes et al., 2014; Birbrair and Frenette, 2016). The vascular sinuses lined with endothelial cells are supported by the CXCL12-abundant reticular cells and MSCs, which form a reticular network and support HSCs formation, and maturation for megakaryocytic proliferation via the THPO/cMpl system. Other essential factors include FGF-4, VCAM-1 and VLA-4, which positively regulate platelet and endothelial cells maturation and proliferation. TGF-β negatively regulates HSCs proliferation, or rather promotes their quiescence, in addition to controlling c-Kit, IL6R, p21 and p57 expression levels. Additionally, hyaluronic acid produced by primitive hematopoietic cells enables HSCs migration to endosteal niche after transplantation.
4.1 Hematopoietic Regulators
Primarily, the TFs within the niche have a significant function in determining the fate of the HSCs. Several TFs have been identified for balancing the self-renewal and differentiation of HSCs (Zhu and Emerson, 2002). TFs such as Pbx-1 and Evi-1 maintain HSCs self-renewability, whereas SCL, Gfi1, PU.1, and FoxO maintain HSCs in the G0 phase. The Mef TF facilitates the quiescent HSCs transition from G0 to G1 phase (Huck et al., 2014). Other TFs that maintain the stemness of HSCs are SON, Tal1 or SCL, RUNX1, LMO2, GATA-2, HOXA9, and HOXA1, as summarized in Table 1 (Blume-Jensen et al., 1991; Shiohara et al., 1993; Rossi et al., 2007; Park et al., 2013; Wang and Ema, 2016; Bhatlekar et al., 2018).
4.1.1 c-Myb
The Myb family of TFs is involved in cell cycle regulation and maintenance of genomic integrity, composed of three isoforms, a-Myb, b-Myb, and c-Myb. The latter two are majorly responsible for regulating hematopoietic functions (Baker et al., 2014). The b-Myb is a crucial factor in controlling cell fate that maintains the self-renewability of HSCs and progenitor cells, primarily in the G0-G1 phase. Depletion and microarray studies of b-Myb revealed the accumulation of HSCs population in S/G2 phases and decreased numbers of differentiated lymphoid, erythroid, and myeloid cells (Baker et al., 2014).
Similarly, c-Myb is a key self-renewal regulator in the early stages of HSCs development which acts in conjunction with PU.1 to enhance proliferation in the earlier set of HSCs, as reported in mice and human studies. The decrease in c-Myb expression leads to HSCs lineage commitment towards erythroid cells (Soza-Ried et al., 2010; Raghav and Gangenahalli, 2018). Therefore, it indicates that both these isoforms regulate cell fate in the early stages of the cell cycle.
4.1.2 Ecotropic Viral Integration Site 1
Ecotropic viral integration site 1 (Evi1) is an oncogenic TF of the SET/PR domain protein family (Huck et al., 2014; Mirantes et al., 2014; Birbrair and Frenette, 2016). It has a developmental role in the hematopoietic system in the fetal and adult HSCs and progenitor cells. However, Evi1 deletion in mice embryos decreases HSCs numbers, whereas conditional deletion of Evi1 in adult mice disrupts HSCs development (Ficara et al., 2008; Kataoka et al., 2011). Nevertheless, Evi1 overexpression leads to myelodysplasia (Buonamici et al., 2004; Kataoka et al., 2011). Evi1 expression in LT-HSCs promotes stem cells self-renewal by interaction with downstream molecules like GATA-2, Pbx1, Runx1, and TGF-β (Yuasa et al., 2005; Senyuk et al., 2007; Sato et al., 2008; Goyama and Kurokawa, 2009; Shimabe et al., 2009). Forced and upregulated expression of Evi1 in HSCs prevents progenitor cells’ differentiation and enhances their expansion through inducing self-renewal in HSCs, independent of cell-cycle progression. Conversely, Evi1 heterozygosity causes loss of self-renewal in LT-HSCs and ST-HSCs, as reported in an Evi1+/− mice model, which had diminished colony-forming efficiency (Kataoka et al., 2011).
4.1.3 Forkhead O
The TFs belonging to Forkhead O (FoxO) family play a crucial role in diverse physiological processes, including apoptosis, regulating cell-cycle dynamics, and stress resistance (Lee and Castrillon, 2007). Four members of this family, FoxO1, FoxO3, FoxO4, and FoxO6, activate downstream PI3K-Akt signaling to sustain cell survival. Elevated FoxO levels maintain stemness within LT-HSCs by inhibiting cell cycle progression (Ludikhuize and Rodríguez Colman, 2020). FoxO1 and FoxO3 are required for metabolic regulation within LT-HSCs by glycolysis and fatty acid oxidation, keeping mitochondrial ROS levels to a sub minimal. However, mitochondrial activity increases during differentiation due to metabolic switch decreasing the FoxO levels and inducing MPP differentiation into various lineages (Ito et al., 2012; Ito et al., 2016). The loss of FoxO3 leads to defects in the long-term maintenance of the hematopoietic pool and LT-HSCs exhaustion because of absence or loss of repopulation ability (Miyamoto et al., 2007; Yalcin et al., 2008; Ito et al., 2016). Certain systemic factors also contribute to the functioning of FoxO proteins. Recent findings indicate that hyperglycemia and obesity disrupt the Akt-FoxO axis by upregulating oxidative stress that hinders the self-renewal of HSCs, decreasing the stem cell pool. Thus, specific hypoglycemic agents that can target PI3K/Akt pathway have a potential role in treating hematological deficiency disorders by upscaling the FoxO induced HSCs population (Govindarajah et al., 2020).
4.1.4 GATA
The GATA family proteins, inclusive of the three isoforms GATA-1, GATA-2, and GATA-3, are essentially identified by two highly conserved zinc fingers of the C2H2 type that recognize the motif “WGATAR” (Martin and Orkin, 1990; Trainor et al., 1996; Gao et al., 2015). GATA-1 expression is required to develop lineage-committed hematopoietic cells such as CLPs and CMPs, which differentiate into megakaryocytes and erythrocytes, primarily through EPO signaling (Whyatt et al., 1997; Rylski et al., 2003). GATA-2 is required at the early stages of HSCs, subsequently replaced by GATA-1 once the differentiation process is initiated (Bresnick et al., 2010; Suzuki et al., 2013). GATA-2 loss of function mutation leads to dysfunctional adult human cord blood progenitors with self-renewal potential (Menendez-Gonzalez et al., 2019). Conversely, LT-HSCs are enriched with GATA-3, whose activation has correlated signaling through the MAPK-p38a pathway (Yoshida and Georgopoulos, 2013). The loss of GATA-3 in steady-state quiescent HSCs does not affect its self-renewal or proliferation (Yoshida and Georgopoulos, 2013). However, under stress-activated p38a conditions, GATA-3 deletion restrains the self-renewal of LT-HSCs by balancing proliferation and differentiation without affecting the cell cycle (Frelin et al., 2013; Yoshida and Georgopoulos, 2013).
4.1.5 Growth Factor Independence
Growth factor independence 1 (Gfi1), a transcriptional repressor composed of Snail/Gfi1 (SNAG) domain and six zinc-finger motifs, is a part of the oncogenic complementation system as Bmi-1 (Moraes et al., 2002; Lecellier and Voinnet, 2004; Cellot and Sauvageau, 2005). The most studied function of Gfi1 is IL-2 dependent T-cell proliferation, in conjunction with Pim-1 and Myc TFs, and IL-6/STAT3-mediated proliferation in case of antigenic stimulation (Schmidt et al., 1998). Gfi1 depletion studies in adult HSCs revealed decreased long-term reconstitution capacity in a cell-autonomous manner. However, no significant change was observed in MEPs, suggesting their primary role in maintaining stemness and proliferation in stem cells and progenitors (Zeng et al., 2004). This function is triggered by the transactivation of GATA-2 and RUNX1 TFs by associating Gfi1 with enhancer Mysm1 (Wang et al., 2013). However, the effect of this factor on apoptosis or cell homing is yet to be studied.
4.1.6 Homeobox
The homeobox (HOX) genes are a family of highly conserved homeodomain-containing TFs that specify cell type identity in the early stages of development. These can be categorized into four separate clusters- HOXA, HOXB, HOXC, and HOXD, located on different chromosomes. The expression of HOX proteins follows a specific pattern, with the HOXA gene expressed in myeloid cells, HOXB in erythroid cells, and HOXC in lymphoid cells (Alharbi et al., 2013). HOX 1-6 genes are usually expressed within a HOX cluster in stem and progenitor cells. In contrast, the genes in order of their arrangement on the chromosome are expressed in lineage-committed cells (Sauvageau et al., 1994; Alharbi et al., 2013). Specific HOX genes have been reported to enhance stem cell and progenitor populations by inhibiting differentiation. Overexpression of HOXC4 stimulates early myeloid and erythroid progenitors to proliferate, while HOXA9, HOXB4, and HOXB6 maintain stem cell pools and support proliferation (Kroon et al., 1998; Thorsteinsdottir et al., 2002). However, these are downregulated at the time of differentiation, sustaining the regenerative potential of the LT-HSCs and ST-HSCs (Sauvageau et al., 1994; Pineault et al., 2002). STAT3 is functionally similar to HOXB4, yet they do not synergize and show independent effects (Hong et al., 2014). HOXB6 is an essential TF to maintain the self-renewal potential of the HSCs, and any mutations in this gene can end up in AML (Kappen, 2000; Fischbach et al., 2005; Vainshtein et al., 2015).
4.1.7 Mef
Mef or ELF4 is a member of the E26 transformation-specific (ETS) family of winged helix-turn-helix TFs and primarily acts on LT-HSCs (Lacorazza et al., 2006; Suico et al., 2017; Wilkinson et al., 2020). Its expression is dramatically reduced in cases of AML, implying its tumor-suppressive role (Fukushima et al., 2003). HSCs display enhanced levels of reconstitution in serial transplantation in the absence of Mef. On the contrary, Mef deficient HSCs do not show positive repopulation in primary transplantation, suggesting its main function in LT-HSCs self-renewal (Suico et al., 2017). It maintains quiescency within HSCs associated with p53, acting downstream of Gfi-1 and Necdin (Liu et al., 2009).
4.1.8 Pbx-1
Pbx-1 is a TALE class homeodomain TF regulating critical embryonic processes such as organogenesis and hematopoiesis (DiMartino et al., 2001; Kim et al., 2002; Baker et al., 2014). The proto-oncogene Pbx1 has higher expression in LT-HSCs than in ST-HSCs and MPPs (Forsberg et al., 2005; Kiel et al., 2005). Pbx1 deficiency causes downregulated self-renewal and proliferation activity of HSCs and impaired long-term engraftment. It is known to exhibit this attribute via TGF-β and MAPK signaling (Baker et al., 2014). Pbx1 forms a transcriptional complex with Prep, acting on TGF-β and regulating the expression of p21 and p57 genes to maintain HSCs quiescence (Bromleigh and Freedman, 2000; Umemoto et al., 2005). Lack of Pbx1 has been associated with severe fetal anemia and disruption in lymphoid differentiation since the absence of Pbx1 leads to premature expression of ST-HSCs genes within LT-HSCs (Baker et al., 2014).
4.1.9 PU.1
The PU.1 is another member of the ETS family of TFs, encoded by the proto-oncogene SPI1 (Tothova et al., 2007; Wilkinson et al., 2020). The expression of PU.1 is primarily linked to the differentiation and maturation of lineage-committed cells, including lymphoid, myeloid, and macrophage lineages (Dakic et al., 2005; Chang et al., 2010). Additionally, it maintains the stem cell pool in the hematopoietic system (Staber et al., 2013). Loss of functional mutation in PU.1 leads to the development of AML in adults and results in embryonic lethality (Scott et al., 1994; McKercher et al., 1996; Mueller et al., 2002; Iwasaki et al., 2005). It causes a maturation arrest in the transition of HSCs to CLP and CMP phenotypes, hence establishing PU.1 requirement for the competitive self-renewal of HSCs. However, a low level of PU.1 expression is required for HSCs maintenance, whereas an increased level causes myeloid differentiation (Iwasaki et al., 2005; Raghav and Gangenahalli, 2021). The exact mechanism of PU.1 is still under speculation; however, a recent study reported the role of non-canonical Wnt signaling in HSCs self-renewal and myeloid lineage differentiation (Welner et al., 2020).
4.1.10 Runt-Related Transcription Factor 1
Runt-related transcription factor 1 (RUNX1) is an essential TF functioning at multiple hematopoiesis stages, including definitive HSCs formation and differentiation into granulocytes, B-cell, and megakaryocytes (Guo et al., 2012; Dowdy et al., 2013; Ichikawa et al., 2013; Tober et al., 2013; Gerritsen et al., 2019). Structurally, RUNX proteins have a 128 amino acid long DNA-binding domain (at N-terminus) and a conserved five amino acid motif, “VWRPY” (at the C-terminus). The N-terminal binds to a consensus DNA sequence “TGTGGT” or “TGCGGT” and regulates hematopoiesis by modulating the expression levels of the PF4 gene, IL-3 GM-CSF cytokines, and M-CSFR cytokine receptors (Harada et al., 2013; Gerritsen et al., 2019). It plays a significant role in embryonic stage hematopoiesis by promoting the development of vascular endothelial cells. However, in adult HSCs, RUNX1 expression leads to stem cell exhaustion, indicating that its deletion would affect hematopoietic stem/progenitor cells (Osato, 2004; Haferlach et al., 2016).
Mutations in the RUNX1 gene correlate with the occurrence of AML and myelodysplasia, suggesting that this TF has a tumor-suppressive function. The conserved RUNX family has three isoforms, RUNX1, RUNX2, and RUNX3. Molecules regulating RUNX1 activity include ERK, HIPK2, cyclin-dependent kinases, or methylators like PRMT1 (Preudhomme et al., 2000; Gerritsen et al., 2019). RUNX1 was reported to function as a cytoplasmic regulator of NF-kB signaling, interacting with the IkB kinase complex. The NF-kB inhibitor BMS-345541 inhibits the proliferation of leukemia cells containing RUNX1 mutation, suggesting a potential role of NF-κb inhibitors in targeting RUNX1-related leukemia (Gerritsen et al., 2019).
4.1.11 Stem Cell Leukemia
SCL is a key factor required at different stages of HSCs development in SCL. Transcription of this gene is reportedly the highest in quiescent HSCs at the G0 phase compared to more actively proliferating HSCs in the G1/S/G2 phase (Lacombe et al., 2010; Rojas-Sutterlin et al., 2014; Haferlach et al., 2016). It regulates a smooth G0 to G1 transition of LT-HSCs by acting on its partners E47, GATA-2, and LDB1 (Rojas-Sutterlin and Hoang, 2013). SCL acts upstream of c-Kit to sustain self-renewal. However, the entire SCL-c-Kit axis is yet to be delineated (Rojas-Sutterlin and Hoang, 2013; Rojas-Sutterlin et al., 2014). Heterozygous expression of SCL exhibited repopulation efficiency limited to only 4 months of post-transplantation and impaired repopulation capacity after second transplantation (Lacombe et al., 2010). This study corroborated another study wherein, enforced SCL expression enhanced regenerative potential in secondary transplantations (Reynaud et al., 2005). SCL deletion can be compensated by Lyl1 expression, but not vice-versa (Fujiwara et al., 2004; Chan et al., 2007). In addition to its role in development, SCL controls the cellular transition from a proliferating stage to erythroid progenitors’ formation (Lacombe et al., 2010). This activity is exhibited by the upregulation of Gfi1b and Cdkn1a gene expression. The upregulated expression of cell cycle regulators Cdkn1a and Id1 maintain quiescency and mitotic index in LT-HSCs (Lacombe et al., 2010).
4.1.12 c-Kit
c-Kit maintains a balance between self-renewal and differentiation among HSCs via the c-Kit/SCF axis (Mirantes et al., 2014; Birbrair and Frenette, 2016). The c-Kitlo population stays higher in the hierarchy than c-Kithi, and the transition from c-Kitlo to c-Kithi is mediated by c-Cbl (Shiohara et al., 1993; Huck et al., 2014). It is observed that c-Kitlo HSCs exhibit long-term reconstitution with enhanced self-renewal as compared to c-Kithi. The cells expressing c-Kithi also show increased GATA-1 and decreased HOXB4 expression levels, making this population more biased towards megakaryocytic differentiation (Huck et al., 2014). SCF cytokine acts as a ligand for the c-Kit receptor to maintain self-renewal and proliferation of HSCs (Rossi et al., 2007). SCF in synergism with GM-CSF, IL-3, and EPO activates c-Kit intrinsic tyrosine kinase activity that signals the PI3K/MAPK pathway to switch to proliferation (Park et al., 2013; Raghav and Gangenahalli, 2018). Thus, for ex vivo expansion of HSCs, ablating c-Kit levels in conjunction with SCF might be a suitable strategy by upregulating the self-renewal levels while acting in synergy with GATA-1 that steer lineage-specific differentiation.
4.2 miRNAs
Some miRNAs have a functional role in self-renewal of HSCs viz. miR-27a, miR-99a/99b/100, miR-126, mir-155 and miR-33/142a-3p (Gekas and Graf, 2013). miRNAs also play a significant role in HSCs differentiation into a particular lineage. miRNAs responsible for controlling differentiation to myeloid lineage are miR-124, miR-15a/16, miR-486-3p, miR-223, mi144/451, miR-9, and miR-22. Besides, miR-34a, miR-150, and miR-132 control differentiation of HSCs into lymphoid lineage (Gekas and Graf, 2013; Xavier-Ferrucio and Krause, 2018).
The miR-99 family control HSCs quiescency and self-renewal by regulating HOXA1 levels, while miR-126 keeps HOXA9 levels under check to suppress leukemogenesis (Gekas and Graf, 2013; Bhatlekar et al., 2018). The tumor suppressor protein p53 is another TF that controls HSCs quiescency. The miR-33 and miR-142-3p are essential regulators of p53. These miRNAs are associated with decreased cell proliferation and increased apoptosis to check any leukemia incidence (Vainshtein et al., 2015; Foroutan, 2017).
The signaling pathways, Notch, Wingless-type (Wnt), Sonic hedgehog (Shh), and Smad, control the LT-HSCs population. Moreover, the IL-6 and IL-11 receptor, gp130 protein, and c-Kit play an essential role in HSCs self-renewal (Velasco-Hernandez et al., 2016; Hashimoto et al., 2021). Intracellular adaptor molecule, Lnk negatively regulates thrombopoietin (TPO), SCF, erythropoietin (EPO), IL-3, and IL-7 signaling pathways, decreasing HSCs self-renewal potency. Nevertheless, cytokines, SCF, and TPO are the two most important positive regulators of HSCs. Reportedly, the forced expression of β-catenin upregulates the expression of Notch1 and HOXB4 genes, enhancing HSCs self-renewal (Emmons et al., 2017; Xavier-Ferrucio and Krause, 2018).
4.3 Epigenetics
Another approach to regulate the HSCs expansion is via a better understanding of the epigenetic regulators. A crucial epigenetic regulator is MLLT3 or AF9, which in conjugation with DOT1L, acts as histone reader to methylate H3K79 and maintains the self-renewability of HSCs. MLLT3 levels decrease in HSCs cultured in vitro; thus, stabilizing MLLT3 levels in culture and causing a 12-fold increase in expansion of transplantable HSCs (Calvanese et al., 2019). Similarly, bromodomain PHD finger transcription factor (BPTF), an essential component of the nucleosome remodeling factor (NURF) chromatin-remodeling complex, maintains both LT-HSCs and ST-HSCs population. BPTF knockout leads to defects like anemia by suppressing Meis1, Pbx1, Mn1, and Lmo2 genes required for self-renewal (Xu et al., 2018).
Nevertheless, C/EBPα regulates multiple cell fates like directed myeloid differentiation in HSCs, maintaining self-renewal and quiescent state in LT-HSCs acting as an apoptotic inhibitor. Deleting the C/EBPα gene leads to the exhaustion of the HSCs population by recruiting epigenetic writers and erasers, a mechanism still elusive (Hasemann et al., 2014). The TF hepatic leukemia factor (HLF) also regulates HSCs quiescence and leukemogenesis. RNA-Seq data of HLF deficient mice revealed 550 differentially expressed genes, including cell cycle control genes’ upregulation. Gene ontology (GO) and gene set enrichment analysis (GSEA) study further elucidated that these upregulated genes primarily function for cell cycle regulation, DNA replication, and cellular stress response. The Chip-Seq data revealed that HLF also downregulates certain genes like GFI1 and IRF2, highlighting its dual role in promoting and inhibiting HSCs self-renewal genes (Komorowska et al., 2017).
Additionally, HSCs require a constitutive level of DNA methylation to sustain self-renewal status. The key players in this methylation include DNMT3a and DNMT3b, MLL1 and MLL5, Ezh2, Kdm2b, Sirt1, and CHD4/NuRD complex (Komorowska et al., 2017). TCF7 is an HMG box protein that regulates self-renewal in HSCs. RNA-Seq and GSEA data revealed that TCF7 is suppressed when HSCs differentiate from CD44+ to CD44− cells and thus play a dual role in promoting self-renewal and inhibiting differentiation promoting genes (Wu et al., 2012). On a similar note, DNA acetylation is also very crucial in HSCs population maintenance, as noted by DNA acetylatases KAT6A, HDAC1, and HDAC2 activity (Jiang et al., 2019). In addition, acetylation is a crucial regulator as lysine acetyltransferases (KATs) such as p300, CBP and HBO1, alter the chromatin structure for gene activation. CBP-p300, which belongs to the KAT family, regulates both differentiation and self-renewal by binding to c-Myb KIX domain and regulating its gene expression (Rodrigues et al., 2021). MOF is a different KAT that regulates erythropoiesis by modulating H4K16ac. This is counterbalanced by histone deacetylases (HDAC) like SIRT1, HDAC1 and HDAC2. Additionally, Mi2β of the NuRD complex is an SNF2-like ATPase required for self-renewal.
4.4 Negative Regulators
Within the hematopoietic niche, many growth factors and signals maintain HSCs quiescence and self-renewal stage. However, some other prevalent factors act as negative regulators, such as the immune signals that stimulate HSCs exit from quiescence and differentiation most commonly into immune regulatory cells (Caiado et al., 2021). Common trigger factors for inciting this HSCs differentiation and immune response are microbiota, bacterial or viral infection, carcinogens, inflammatory diseases and aging. For instance, the anti-viral immune response causes IFN-α production which acts as a negative HSCs regulator and triggers its differentiation into the myeloid lineage. However, bacterial response produces IFN-γ by T and NK cells which also promotes granulopoiesis (Zhang et al., 2022). Other pro-inflammatory cytokines mediate functional effects on HSCs, such as TNF-α binding with HSCs to induce apoptosis. In fact, excessive TNF-α production is linked with BM failure and myelodysplastic syndrome (MDS). Additionally, release of pathogen-associated molecular patterns (PAMPs) increases in cases of bacterial infection, which bind to the HSCs receptors like toll-like receptors (TLRs) and act as niche regulators (Barman and Goodridge, 2022). For example, human cord blood HSCs produce pro-inflammatory cytokine IL-8 via MAPK/AP-1 signalling when CpG DNA from microbes activate TLR9 present on the HSCs surface. Human BM HSCs express TLR4 and TLR7/8 instead which when activated lead to differentiation into monocytes.
Moreover, PU.1 and GATA-1 TFs are maintained in a balanced ratio to regulate the HSCs, yet their respective increase leads to differentiation into myeloid and erythroid lineages (Li, 2011). TNF-α furthermore increases PU.1 production, driving myelopoiesis. These regulators can be divided based on their extrinsic or intrinsic presence. For example, the hedgehog (Hh) signalling pathway is an extrinsic negative regulator, while factors such as MEF/EFL4, Lnk and c-Myc are intrinsic negative regulators of hematopoiesis. The differentiated niche cells play a significant role in regulating HSCs differentiation (King and Goodell, 2011). CXC-chemokine ligand 12 (CXCL12), secreted by osteoblasts in the bone marrow, binds to CXCR4 receptor on HSCs to retain them in the marrow. However, upregulated G-CSF levels during infections, suppress the CXCL12 secretion to mobilize HSCs out of the marrow and differentiate depending on the niche signal.
4.5 Oxidative Stress and Autophagy
The BM multifarious niche factors play a central role in the homeostasis of the hematopoietic process (Hu et al., 2019). Given that HSCs are placed at the top of the hierarchy, their self-renewal and differentiation potential give rise to various peripheral blood cells via hematopoietic progenitors (Miyamoto et al., 2007; Laurenti and Göttgens, 2018). One of the metabolic niche factors modulating this process is oxidative stress, governed by reactive oxygen species (ROS) (Ito et al., 2007; Suda et al., 2011). ROS formed as the byproducts of signal transduction and energy metabolism during hematopoietic regulation and can be beneficial and harmful to the cells depending on their fluctuating intracellular levels (Hu et al., 2019). However, quiescent HSCs require ROS levels to maintain their stemness, elevated ROS levels within the HSCs promote migration, and differentiation via the p190-B RhoGAP-ROS-TGF-β-p38MAPK signaling (Ito et al., 2007; Suda et al., 2011; Ludin et al., 2014; Shinohara et al., 2014; Hinge et al., 2017; Golan et al., 2018; Hu et al., 2019). However, an abnormally high ROS concentration may affect HSCs self-renewability, impair proliferative capacity, and cause DNA damage and cell cycle arrest. The two most recognized endogenous sources of intracellular ROS production are mitochondria and membrane NADPH oxidase (NOX) (Bigarella et al., 2014; Willems et al., 2015). Besides, ionizing radiation (IR) is the most widely accepted exogenous source of ROS production in the body (Li et al., 2016a). BM is the most susceptible tissue to IR. IR causes acute and long-term BM suppression, eventually leading to severe hematological symptoms. This suppression elevates ROS levels within the BM that impair HSCs functioning, induce senescence and stem cell exhaustion. This process is mediated by the p38MAPK-p16ink4a pathway (Shao et al., 2014).
The aggravated ROS concentration can be alleviated by antioxidants, such as Nrf2, a master TF regulating other antioxidant enzymes such as catalase, SOD, GPX1, and thioredoxin. Commercially available antioxidant supplements such as resveratrol, metformin, chlorophyllin, and methoxytryptamine-α-lipoic acid serve the same function, thus mitigating IR induced hematopoietic abnormalities (Zhang et al., 2013; Suryavanshi et al., 2015; Xu et al., 2015; Li et al., 2016b). Nrf2 function as an essential redox regulator of HSCs [187]. For example, in mice, Nrf2−/− HSCs exhibited significant proliferation, phenotypically similar to FoxO deficient HSCs. Hence, it was speculated that Nrf2 and FoxO work parallelly downstream of PI3K/Akt pathway to control HSCs proliferation (Tsai et al., 2013). According to a recent study, LT-HSCs differentiation and cell cycle entry is modulated by Nrf2 and further maintained by Keap1, compromising the HSCs quiescence status (Murakami et al., 2017).
Similarly, this factor is a global regulator of other stem cell types as well. It contributes towards survival, quiescence, and stemness in embryonic stem cells (ESCs), MSCs, intestinal stem cells (in Drosophila), and stress resistance in cancer stem cells (CSCs) (Paul et al., 2014; Ryoo et al., 2016; Ge et al., 2017).
Another mechanism that keeps oxidative stress under control in the BM niche and HSCs functioning intact is autophagy. Broadly, autophagy regulates remodeling during terminal differentiation, delays senescence, and balances the self-renewal and quiescent attributes of the hematopoietic population. These outcomes are modulated via the ATG genes and mTOR/AMPK signaling pathway (Mizushima and Levine, 2010). Among a total of 40 ATG genes, those identified to play a prominent role in stem cell quiescence are PINK1, ULK2, ATG8 homologs MAP1LC3A, GABARAPL1, and FoxO3 (Cheung and Rando, 2013; Murakami et al., 2017). FoxO3A, for instance, induces autophagy in HSCs through ATG4B, BNIP3, and MAP1LC3B, to protect the cells from metabolic stress during starvation (Warr et al., 2013; Riffelmacher and Simon, 2017). The highest autophagy rate is seen in HSCs, decreasing their further differentiation into progenitors (Warr et al., 2013; Watson et al., 2015; Riffelmacher and Simon, 2017). The HSCs differentiation is also defined by the metabolic switch from anaerobic glycolysis to mitochondrial oxidative phosphorylation (OXPHOS). This switching occurs because of the mTOR pathway’s inhibition, which induces autophagy and suppresses OXPHOS, contributing to HSCs quiescence (Gan et al., 2010; Gurumurthy et al., 2010; Butler et al., 2018). The HSCs labeled with Tie2-GFP transgenic reporter are capable of serial transplantation, rendering about 5% of the cells Tie2-GFP+ (Upadhaya et al., 2018). These positively labeled cells undergo proliferator-activated receptor (PPAR) signaling and mitophagy (Watson et al., 2015). The in vivo paired daughter cell assays proved that the Tie2-GFP+ cells had measurable self-renewal and differentiation capacity but decreased on Parkin knock-down, a key molecule in mitophagy. This established the link between mitophagy and self-renewal within the HSCs (Motoike et al., 2000).
Autophagy also controls the dynamics of the differentiated immune cells. For example, plasma cells have higher autophagy levels than CD19+ B cells. This is controlled through ER homeostasis via reticulophagy, thereby limiting the ROS concentration (Pengo et al., 2013). B- and T-cell differentiation into memory cells is also associated with increased autophagy rate, as detected by the quantification of LC3-II, ATG5, and ATG7 genes (Chen et al., 2014). Recent experiments suggest that BNIP3- and BNIP3L-dependent mitophagy is essential in natural killer (NK) cells to sustain self-renewal and cytoplasmic homeostasis by downplaying ROS levels (Salio et al., 2014).
5 Regulating Hematopoietic Stem Cells for Clinical Use
5.1 Engineered Hematopoietic Stem Cells
Cell engineering has shown insights into directed differentiation and TF-regulated approaches to design patient-specific HSCs. The sustained self-renewal and controlled differentiation to produce a functional and stable hematopoietic system is required for achieving therapy-grade engineered HSCs (Rowe et al., 2016). Heterochronic genes impact the HSCs in a stage-specific manner to regulate the hematopoietic system’s development, observed in transplantation assays (Rowe et al., 2016). The primary objective of TF-mediated blood engineering is to evade these heterochronic barriers’ complexity to reach adult HSCs phenotypes. The LIN28 and let-7 family of miRNA can be fine-tuned in engineered HSCs to enhance self-renewal potential for therapeutic applications (Rowe et al., 2016). However, using small molecules instead of the growth factors and cytokines might be a more economical and impactful alternative (MacRae and Peterson, 2015). For in vivo imaging, zebrafish has come up as a good model, giving better insights into the early developmental stages of hematopoiesis. Also, this has facilitated the identification of crucial factors required in these development stages. For instance, chromatin modifiers such as Bmi-1 maintain the quiescency, and Ezh1 drives HSCs to senescence in its absence (Park et al., 2003; Hidalgo et al., 2012). The gene therapy has been proven clinically efficient in targeting X-linked severe combined immune deficiency (X-SCID), adenine deaminase deficiency SCID (ADA-SCID), and chronic granulomatous disease (CGD). However, this technique also runs the risk of mutagenesis by specific proto-oncogene activation. Therefore, engineered HSCs would be a promising strategy for better clinical outcomes, a more in-depth understanding of the hematopoietic system is required to build functionally stable HSCs. Such HSCs are expected to have transfusion independence and acquire a stable immune system with diverse blood cells.
5.2 Ex vivo Hematopoietic Stem Cells Expansion
In vivo HSCs expansion is balanced by self-renewal and differentiation through various TFs and growth factors. However, in vivo persistence of HSCs within transplanted patients and their ex vivo expansion have been a significant obstacle in clinical practice. Thus, simulating a similar environment in ex vivo cultures by identifying the optimal growth factors such as thrombopoietin, SCF, Flt3 ligand, IL-6, and G-CSF is a pragmatic approach to sustain the isolated HSCs colonies outside the body (Papa et al., 2020). Several strategies have been devised using umbilical cord blood-derived HSCs (UCB-CD34+) for their ex vivo expansion (Table 3) (Papa et al., 2020).
One method to go about it is by Notch signalling activation within HSCs. Treating HSCs with an engineered Delta-like ligand like DELTA1ext−IgG (DXI) under normoxic conditions activates the Notch pathway in ST-HSCs by triggering the transcriptional activity of HES and HEY genes responsible for self-renewal (Araki et al., 2021). However, this engraftment did not sustain for long since it was ineffective on the LT-HSCs. Therefore, to counter the oxidative stress implicated on the endoplasmic reticulum under normoxic conditions, HSCs expanded ex vivo with exogenously provided DXI in hypoxia manifested an almost five fold increase in LT-HSCs compared to the untreated cells, offering a clinical potential for the long term HSCs sustenance ex vivo (Araki et al., 2021). The hypoxic condition also supports the stability of HIF-2α heterodimerization with HIF-1β. Since unbound HIF-1β under normoxia binds to aryl hydrocarbon receptor (AhR) that triggers transcription of cellular differentiation genes CYP1A1 and CYP1B1, confirming the crucial role of AhR pathway in self-renewal (Araki et al., 2021). AhR antagonists like Stem-Reginin1 (SR1) can increase the HSCs numbers by 50-fold and establish long-term engraftment in immunodeficient mice (Boitano et al., 2010). A phase I/II clinical trial was conducted wherein SR1 treatment to UCB-CD34+ cells demonstrated rapid platelet and neutrophil recovery. However, the mechanism of action is yet to be explored (Wagner et al., 2016; Zgurskaya and Rybenkov, 2020). A pyrimidoindole derivative, UM171, enhances primitive HSCs pool efficiently than SR1, independent of the pathway (Fares et al., 2014). This was characterized by the expression of endothelial protein C receptor (EPCR) over CD34+CD90+ cells as endothelial protein C receptor (EPCR) activates protease-activated receptor-1 signaling and displays a transcriptome similar to that of freshly isolated HSCs (Fares et al., 2014). Epigenetic modifiers, valproic acid (VA), and deacetylase inhibitor functionally reprogram UCB-CD34+ to acquire transcriptomic and mitochondrial profiles resembling that of primary HSCs (Fares et al., 2014; Papa et al., 2020). The high expression levels of CD90 and EPCR elevates the self-renewal and quiescence of HSCs. Similarly, p53 activity is controlled by suppressing intracellular Ca2+ levels and causes a slower cycling rate and higher self-renewal potential (Umemoto et al., 2018). Other essential factors considered for ex vivo HSCs expansion include mitochondrial activity. HSCs depend on glycolysis for energy production at the quiescent stage of self-renewal, displaying minimal mitochondrial activity (Jang and Sharkis, 2007; Romero-Moya et al., 2013; Warr and Passegué, 2013; Vannini et al., 2016). Low mitochondrial activity is linked to lower mitochondrial mass, membrane potential, and lesser ROS production. Following myeloablation, connexin-43 also mediates ROS transfer from HSCs to MSCs, keeping oxidative stress in check (Taniguchi Ishikawa et al., 2012; Qian et al., 2016). This tightly synchronized process is coordinated by mitophagy and autophagy, acting as gatekeepers of metabolic activity (Warr and Passegué, 2013). Other regulatory pathways are also involved in this process, such as SIRTs, FoxO3A, AMPK, and p53 signaling pathways (Ito et al., 2006; Jang and Sharkis, 2007; Tothova and Gilliland, 2007; Nakada et al., 2010; Taniguchi Ishikawa et al., 2012; Wanet et al., 2015; Jung et al., 2016; Qian et al., 2016;; Zhang et al., 2018).
5.3 Extracellular Vesicles
Extracellular vesicles (EVs) play a significant role within the hematopoietic niche in maintaining homeostasis through autocrine and paracrine signaling (Butler et al., 2018). Identification of disease-specific markers in the circulating exosomes aid in the early detection of certain malignancies (Butler et al., 2018). For instance, in chronic lymphoid leukemia, patient-derived exosomes have elevated CD19, CD31, CD44, CD82, HLA-A to D, levels, while low levels of CD21, CD49c, and CD63. In chronic lymphoid leukemia, upregulated levels of CD19 and CD37 have been observed; in AML patients, EVs rich in myeloblastic markers like CD34, CD33, and CD117 have been seen; while multiple myeloma patients have been screened based on EVs with CD38, CD138, CD44 and CD147 markers (Szczepanski et al., 2011; Harshman et al., 2016; Razmkhah et al., 2017). Similarly, EVs are biomarkers to identify patients with aplastic anemia, myelodysplastic syndrome, and sickle-cell anemia, based on their miRNA signatures derived from plasma EVs (Hosokawa et al., 2017; Butler et al., 2018).
For therapeutic purposes, autologous EVs have been obtained from several cell sources, including MSCs, T, and NK cells. MSCs, for example, display immunomodulatory function via miR-155 and miR-146 shuttling that inhibits T, B, and NK cells activity since these miRNAs regulate innate immune response by regulating vesicle trafficking (Di Trapani et al., 2016). Similarly, murine MSCs with CD73+ and CD34+ EVs have been shown to reverse graft-versus-host disease (GvHD) and hindlimb ischemia by suppressing Th1-mediated inflammation (Amarnath et al., 2015). Other cytokines that reduce the inflammatory response within patients include IL-10, TGF-β, and HLA-G. The chronic inflammation caused in Rab27 knockout mice is suppressed by HSCs injection or G-CSF rich EVs (Alexander et al., 2017). However, approaches for organ-specific selective loading need to be optimized before rolling it out for clinical use. Therefore, a practical therapeutic approach, and deeper understanding of the EVs-cellular interaction are needed.
5.4 Limitations
Despite the wide-scale clinical application of HSCs targeting many disorders, certain complications are associated with hematopoietic stem cell transplantation (HSCT). Early-onset side-effects include mucositis, hemorrhagic cystitis, GvHD and graft failure, pulmonary complications, thrombotic microangiopathy, and prolonged pancytopenia (Moore, 2021). On the other hand, late-onset complications arise due to chronic GvHD, ocular, endocrine, pulmonary, musculoskeletal, neurological, and immune effects, congestive heart failure, and risk of infections. Late-stage repercussions usually set in after many months or years of HSCT and even report cases of leukemia and myelodysplastic syndrome relapse.
6 Conclusion
Hematopoiesis is a complex developmental process regulated by a closely integrated network of TFs, miRNAs, epigenetic modifications, cytokines, and growth factors. These components maintain a fine balance between the self-renewal and differentiation properties of HSCs to generate a functionally stable hematopoietic pool. The BM niche components modulate the heterochromatic expression of multiple genes to maintain HSCs progression into a specific lineage, preventing leukemogenesis occurrence. Dysregulation in these networks leads to a diverse range of hematological malignancies such as ALL, chronic lymphocytic leukemia (CLL), acute myeloid leukemia (AML), chronic myeloid (CML), myeloma, aplastic and sickle-cell anemia, GvHD and lymphoma. In addition to intrinsic factors, various extrinsic factors such as ionization, obesity, and lack of exercise also impact the hematopoietic niche, causes oxidative stress and ablation of long-term renewability of HSCs. Therefore, a more lucid image of the hematopoietic development system is required to strategize natural therapies. Advanced technologies such as single-cell RNA sequencing, multiphoton intravital microscopy, the building of more pragmatic animal models like zebrafish, and high-throughput genetic screening, have deepened our insight into this system. However, a better comprehension of inflammation-upregulated HSCs and EVs trafficking might offer opportunities to discerning novel therapies for HSCs homing in an organ-specific manner to be used in clinical settings. The underlying mechanisms of action of TFs and miRNA of bone marrow niche in interconnecting therapeutic strategies are still poorly understood. It is conceivable that over the years, their role in influencing self-renewal and differentiation will become more apparent as the biological interactions of these microenvironmental factors are unraveled through a continuous moving from bench to bedside and vice versa. Engineered HSCs and controlled ex vivo expansion using extracellular vesicles can create HSCs without immunogenicity, making HSCs more suitable for therapeutic purposes as their infusion may be performed across histocompatibility locus antigen barriers without risk of rejection.
Author Contributions
PR designed and conceptualized the research; ZM and PR performed the analysis; ZM and PR wrote the original draft; ZM, MS, YV, RR, and PR wrote, reviewed, edited and proofread the manuscript.
Conflict of Interest
The authors declare that the research was conducted in the absence of any commercial or financial relationships that could be construed as a potential conflict of interest.
Publisher’s Note
All claims expressed in this article are solely those of the authors and do not necessarily represent those of their affiliated organizations, or those of the publisher, the editors and the reviewers. Any product that may be evaluated in this article, or claim that may be made by its manufacturer, is not guaranteed or endorsed by the publisher.
Acknowledgments
The authors are most grateful to NIH/NLM (U.S. National Institute of Health’s National Library of Medicine) to access free full-text scientific publications on PubMed Central (www.ncbi.nlm.nih.gov/pmc/), which was integral for the successful completion of this work. The PR thanks the editor for the invitation to write this review.
References
Akashi, K., Traver, D., Miyamoto, T., and Weissman, I. L. (2000). A Clonogenic Common Myeloid Progenitor that Gives Rise to All Myeloid Lineages. Nature 404 (6774), 193–197. doi:10.1038/35004599
Alexander, M., Ramstead, A. G., Bauer, K. M., Lee, S.-H., Runtsch, M. C., Wallace, J., et al. (2017). Rab27-dependent Exosome Production Inhibits Chronic Inflammation and Enables Acute Responses to Inflammatory Stimuli. J.I. 199 (10), 3559–3570. doi:10.4049/jimmunol.1700904
Alharbi, R. A., Pettengell, R., Pandha, H. S., and Morgan, R. (2013). The Role of HOX Genes in normal Hematopoiesis and Acute Leukemia. Leukemia 27 (5), 1000–1008. doi:10.1038/leu.2012.356
Amarnath, S., Foley, J. E., Farthing, D. E., Gress, R. E., Laurence, A., Eckhaus, M. A., et al. (2015). Bone Marrow-Derived Mesenchymal Stromal Cells Harness Purinergenic Signaling to Tolerize Human Th1 Cells In Vivo. Vivo. Stem Cell 33 (4), 1200–1212. doi:10.1002/stem.1934
Araki, D., Fu, J. F., Huntsman, H., Cordes, S., Seifuddin, F., Alvarado, L. J., et al. (2021). NOTCH-mediated Ex Vivo Expansion of Human Hematopoietic Stem and Progenitor Cells by Culture under Hypoxia. Stem Cel. Rep. 16 (9), 2336–2350. doi:10.1016/j.stemcr.2021.08.001
Baker, J. M., De Lisio, M., and Parise, G. (2011). Endurance Exercise Training Promotes Medullary Hematopoiesis. FASEB j. 25 (12), 4348–4357. doi:10.1096/fj.11-189043
Baker, S. J., Ma'ayan, A., Lieu, Y. K., John, P., Reddy, M. V. R., Chen, E. Y., et al. (2014). B-myb Is an Essential Regulator of Hematopoietic Stem Cell and Myeloid Progenitor Cell Development. Proc. Natl. Acad. Sci. 111 (8), 3122–3127. doi:10.1073/pnas.1315464111
Barman, P. K., and Goodridge, H. S. (2022). Microbial Sensing by Hematopoietic Stem and Progenitor Cells. Stem Cells. doi:10.1093/stmcls/sxab007
Beerman, I., and Rossi, D. J. (2014). Epigenetic Regulation of Hematopoietic Stem Cell Aging. Exp. Cel. Res. 329 (2), 192–199. doi:10.1016/j.yexcr.2014.09.013
Bellows, C. F., Zhang, Y., Simmons, P. J., Khalsa, A. S., and Kolonin, M. G. (2011). Influence of BMI on Level of Circulating Progenitor Cells. Obesity 19 (8), 1722–1726. doi:10.1038/oby.2010.347
Bernitz, J. M., Kim, H. S., MacArthur, B., Sieburg, H., and Moore, K. (2016). Hematopoietic Stem Cells Count and Remember Self-Renewal Divisions. Cell 167 (5), 1296–1309. doi:10.1016/j.cell.2016.10.022
Bertrand, J. Y., Cisson, J. L., Stachura, D. L., and Traver, D. (2010). Notch Signaling Distinguishes 2 Waves of Definitive Hematopoiesis in the Zebrafish Embryo. Blood J. Am. Soc. Hematol. 115 (14), 2777–2783. doi:10.1182/blood-2009-09-244590
Bhatlekar, S., Fields, J. Z., and Boman, B. M. (2018). Role of HOX Genes in Stem Cell Differentiation and Cancer. Stem Cell Int 2018, 3569493. doi:10.1155/2018/3569493
Bigarella, C. L., Liang, R., and Ghaffari, S. (2014). Stem Cells and the Impact of ROS Signaling. Development 141 (22), 4206–4218. doi:10.1242/dev.107086
Birbrair, A., and Frenette, P. S. (2016). Niche Heterogeneity in the Bone Marrow. Ann. N.Y. Acad. Sci. 1370 (1), 82–96. doi:10.1111/nyas.13016
Blume-Jensen, P., Claesson-Welsh, L., Siegbahn, A., Zsebo, K. M., Westermark, B., and Heldin, C. H. (1991). Activation of the Human C-Kit Product by Ligand-Induced Dimerization Mediates Circular Actin Reorganization and Chemotaxis. EMBO J. 10 (13), 4121–4128. doi:10.1002/j.1460-2075.1991.tb04989.x
Boitano, A. E., Wang, J., Romeo, R., Bouchez, L. C., Parker, A. E., Sutton, S. E., et al. (2010). Aryl Hydrocarbon Receptor Antagonists Promote the Expansion of Human Hematopoietic Stem Cells. Science 329 (5997), 1345–1348. doi:10.1126/science.1191536
Bresnick, E. H., Lee, H.-Y., Fujiwara, T., Johnson, K. D., and Keles, S. (2010). GATA Switches as Developmental Drivers. J. Biol. Chem. 285 (41), 31087–31093. doi:10.1074/jbc.r110.159079
Bromleigh, V. C., and Freedman, L. P. (2000). p21 Is a Transcriptional Target of HOXA10 in Differentiating Myelomonocytic Cells. Genes Dev. 14 (20), 2581–2586. doi:10.1101/gad.817100
Buenrostro, J. D., Corces, M. R., Lareau, C. A., Wu, B., Schep, A. N., Aryee, M. J., et al. (2018). Integrated Single-Cell Analysis Maps the Continuous Regulatory Landscape of Human Hematopoietic Differentiation. Cell 173 (6), 1535–1548. doi:10.1016/j.cell.2018.03.074
Buonamici, S., Li, D., Chi, Y., Zhao, R., Wang, X., Brace, L., et al. (2004). EVI1 Induces Myelodysplastic Syndrome in Mice. J. Clin. Invest. 114 (5), 713–719. doi:10.1172/jci21716
Buono, M., Facchini, R., Matsuoka, S., Thongjuea, S., Waithe, D., Luis, T. C., et al. (2016). A Dynamic Niche Provides Kit Ligand in a Stage-specific Manner to the Earliest Thymocyte Progenitors. Nat. Cel Biol 18 (2), 157–167. doi:10.1038/ncb3299
Busch, K., Klapproth, K., Barile, M., Flossdorf, M., Holland-Letz, T., Schlenner, S. M., et al. (2015). Fundamental Properties of Unperturbed Haematopoiesis from Stem Cells In Vivo. Nature 518 (7540), 542–546. doi:10.1038/nature14242
Butler, J. T., Abdelhamed, S., and Kurre, P. (2018). Extracellular Vesicles in the Hematopoietic Microenvironment. Haematologica 103 (3), 382–394. doi:10.3324/haematol.2017.183335
Cabezas-Wallscheid, N., and Trumpp, A. (2016). Potency Finds its Niches. Science 351 (6269), 126–127. doi:10.1126/science.aae0325
Caiado, F., Pietras, E. M., and Manz, M. G. (2021). Inflammation as a Regulator of Hematopoietic Stem Cell Function in Disease, Aging, and Clonal Selection. J. Exp. Med. 218 (7), e20201541. doi:10.1084/jem.20201541
Calvanese, V., Nguyen, A. T., Bolan, T. J., Vavilina, A., Su, T., Lee, L. K., et al. (2019). MLLT3 Governs Human Haematopoietic Stem-Cell Self-Renewal and Engraftment. Nature 576 (7786), 281–286. doi:10.1038/s41586-019-1790-2
Cao, Y., and Ma, J. (2011). Body Mass Index, Prostate Cancer-specific Mortality, and Biochemical Recurrence: a Systematic Review and Meta-Analysis. Cancer Prev. Res. 4 (4), 486–501. doi:10.1158/1940-6207.capr-10-0229
Cellot, S., and Sauvageau, G. (2005). Gfi-1: Another Piece in the HSC Puzzle. Trends Immunology 26 (2), 68–71. doi:10.1016/j.it.2004.12.005
Chan, W. Y. I., Follows, G. A., Lacaud, G., Pimanda, J. E., Landry, J.-R., Kinston, S., et al. (2007). The Paralogous Hematopoietic Regulators Lyl1 and Scl Are Coregulated by Ets and GATA Factors, but Lyl1 Cannot rescue the Early Scl-/- phenotypeThe Paralogous Hematopoietic Regulators Lyl1 and Scl Are Coregulated by Ets and Gata Factors, but Lyl1 Cannot rescue the Early Scl–/–Phenotype. Blood 109 (5), 1908–1916. doi:10.1182/blood-2006-05-023226
Chang, H.-C., Sehra, S., Goswami, R., Yao, W., Yu, Q., Stritesky, G. L., et al. (2010). The Transcription Factor PU.1 Is Required for the Development of IL-9-producing T Cells and Allergic Inflammation. Nat. Immunol. 11 (6), 527–534. doi:10.1038/ni.1867
Chen, M., Hong, M. J., Sun, H., Wang, L., Shi, X., Gilbert, B. E., et al. (2014). Essential Role for Autophagy in the Maintenance of Immunological Memory against Influenza Infection. Nat. Med. 20 (5), 503–510. doi:10.1038/nm.3521
Cheng, H., Zheng, Z., and Cheng, T. (2020). New Paradigms on Hematopoietic Stem Cell Differentiation. Protein Cell 11 (1), 34–44. doi:10.1007/s13238-019-0633-0
Cheung, T. H., and Rando, T. A. (2013). Molecular Regulation of Stem Cell Quiescence. Nat. Rev. Mol. Cel Biol 14 (6), 329–340. doi:10.1038/nrm3591
Dakic, A., Metcalf, D., Di Rago, L., Mifsud, S., Wu, L., and Nutt, S. L. (2005). PU.1 Regulates the Commitment of Adult Hematopoietic Progenitors and Restricts Granulopoiesis. J. Exp. Med. 201 (9), 1487–1502. doi:10.1084/jem.20050075
De Lisio, M., Kaczor, J. J., Phan, N., Tarnopolsky, M. A., Boreham, D. R., and Parise, G. (2011). Exercise Training Enhances the Skeletal Muscle Response to Radiation-Induced Oxidative Stress. Muscle Nerve 43 (1), 58–64. doi:10.1002/mus.21797
Di Trapani, M., Bassi, G., Midolo, M., Gatti, A., Takam Kamga, P., Cassaro, A., et al. (2016). Differential and Transferable Modulatory Effects of Mesenchymal Stromal Cell-Derived Extracellular Vesicles on T, B and NK Cell Functions. Sci. Rep. 6, 24120. doi:10.1038/srep24120
DiMartino, J. F., Selleri, L., Traver, D., Firpo, M. T., Rhee, J., Warnke, R., et al. (2001). The Hox Cofactor and Proto-Oncogene Pbx1 Is Required for Maintenance of Definitive Hematopoiesis in the Fetal Liver. Blood J. Am. Soc. Hematol. 98 (3), 618–626. doi:10.1182/blood.v98.3.618
Dowdy, C. R., Frederick, D., Zaidi, S. K., Colby, J. L., Lian, J. B., Van Wijnen, A. J., et al. (2013). A Germline point Mutation in Runx1 Uncouples its Role in Definitive Hematopoiesis from Differentiation. Exp. Hematol. 41 (11), 980–991. doi:10.1016/j.exphem.2013.06.006
Ema, H., Morita, Y., Yamazaki, S., Matsubara, A., Seita, J., Tadokoro, Y., et al. (2006). Adult Mouse Hematopoietic Stem Cells: Purification and Single-Cell Assays. Nat. Protoc. 1 (6), 2979–2987. doi:10.1038/nprot.2006.447
Emmons, R., Niemiro, G. M., and De Lisio, M. (2016). Exercise as an Adjuvant Therapy for Hematopoietic Stem Cell Mobilization. Stem Cell Int 2016, 7131359. doi:10.1155/2016/7131359
Emmons, R., Niemiro, G. M., and De Lisio, M. (2017). Hematopoiesis with Obesity and Exercise: Role of the Bone Marrow Niche. Exerc. Immunol. Rev. 23, 82–95.
Fares, I., Chagraoui, J., Gareau, Y., Gingras, S., Ruel, R., Mayotte, N., et al. (2014). Pyrimidoindole Derivatives Are Agonists of Human Hematopoietic Stem Cell Self-Renewal. Science 345 (6203), 1509–1512. doi:10.1126/science.1256337
Ficara, F., Murphy, M. J., Lin, M., and Cleary, M. L. (2008). Pbx1 Regulates Self-Renewal of Long-Term Hematopoietic Stem Cells by Maintaining Their Quiescence. Cell stem cell 2 (5), 484–496. doi:10.1016/j.stem.2008.03.004
Fischbach, N. A., Rozenfeld, S., Shen, W., Fong, S., Chrobak, D., Ginzinger, D., et al. (2005). HOXB6 Overexpression in Murine Bone Marrow Immortalizes a Myelomonocytic Precursor In Vitro and Causes Hematopoietic Stem Cell Expansion and Acute Myeloid Leukemia In Vivo. Blood 105 (4), 1456–1466. doi:10.1182/blood-2004-04-1583
Forsberg, E. C., Prohaska, S. S., Katzman, S., Heffner, G. C., Stuart, J. M., and Weissman, I. L. (2005). Differential Expression of Novel Potential Regulators in Hematopoietic Stem Cells. Plos Genet. 1 (3), e28. doi:10.1371/journal.pgen.0010028
Foudi, A., Hochedlinger, K., Van Buren, D., Schindler, J. W., Jaenisch, R., Carey, V., et al. (2009). Analysis of Histone 2B-GFP Retention Reveals Slowly Cycling Hematopoietic Stem Cells. Nat. Biotechnol. 27 (1), 84–90. doi:10.1038/nbt.1517
Frelin, C., Herrington, R., Janmohamed, S., Barbara, M., Tran, G., Paige, C. J., et al. (2013). GATA-3 Regulates the Self-Renewal of Long-Term Hematopoietic Stem Cells. Nat. Immunol. 14 (10), 1037–1044. doi:10.1038/ni.2692
Fujiwara, Y., Chang, A. N., Williams, A. M., and Orkin, S. H. (2004). Functional Overlap of GATA-1 and GATA-2 in Primitive Hematopoietic Development. Blood 103 (2), 583–585. doi:10.1182/blood-2003-08-2870
Fukushima, T., Miyazaki, Y., Tsushima, H., Tsutsumi, C., Taguchi, J., Yoshida, S., et al. (2003). The Level of MEF but Not ELF-1 Correlates with FAB Subtype of Acute Myeloid Leukemia and Is Low in Good Prognosis Cases. Leuk. Res. 27 (5), 387–392. doi:10.1016/s0145-2126(02)00214-x
Fultang, L., Gneo, L., De Santo, C., and Mussai, F. J. (2021). Targeting Amino Acid Metabolic Vulnerabilities in Myeloid Malignancies. Front. Oncol. 11, 674720. doi:10.3389/fonc.2021.674720
Gan, B., Hu, J., Jiang, S., Liu, Y., Sahin, E., Zhuang, L., et al. (2010). Lkb1 Regulates Quiescence and Metabolic Homeostasis of Haematopoietic Stem Cells. Nature 468 (7324), 701–704. doi:10.1038/nature09595
Gao, J., Chen, Y. H., and Peterson, L. C. (2015). GATA Family Transcriptional Factors: Emerging Suspects in Hematologic Disorders. Exp. Hematol. Oncol. 4 (1), 1–7. doi:10.1038/s41467-018-04188-7
Ge, W., Zhao, K., Wang, X., Li, H., Yu, M., He, M., et al. (2017). iASPP Is an Antioxidative Factor and Drives Cancer Growth and Drug Resistance by Competing with Nrf2 for Keap1 Binding. Cancer Cell 32 (5), 561–573. doi:10.1016/j.ccell.2017.09.008
Gekas, C., and Graf, T. (2013). CD41 Expression marks Myeloid-Biased Adult Hematopoietic Stem Cells and Increases with Age. Blood J. Am. Soc. Hematol. 121 (22), 4463–4472. doi:10.1182/blood-2012-09-457929
Gerritsen, M., Yi, G., Tijchon, E., Kuster, J., Schuringa, J. J., Martens, J. H. A., et al. (2019). RUNX1 Mutations Enhance Self-Renewal and Block Granulocytic Differentiation in Human In Vitro Models and Primary AMLs. Blood Adv. 3 (3), 320–332. doi:10.1182/bloodadvances.2018024422
Ghiaur, G., Yegnasubramanian, S., Perkins, B., Gucwa, J. L., Gerber, J. M., and Jones, R. J. (2013). Regulation of Human Hematopoietic Stem Cell Self-Renewal by the Microenvironment's Control of Retinoic Acid Signaling. Proc. Natl. Acad. Sci. 110 (40), 16121–16126. doi:10.1073/pnas.1305937110
Golan, K., Kumari, A., Kollet, O., Khatib-Massalha, E., Subramaniam, M. D., Ferreira, Z. S., et al. (2018). Daily Onset of Light and Darkness Differentially Controls Hematopoietic Stem Cell Differentiation and Maintenance. Cell Stem Cell 23 (4), 572–585. doi:10.1016/j.stem.2018.08.002
Gomez Perdiguero, E., Klapproth, K., Schulz, C., Busch, K., Azzoni, E., Crozet, L., et al. (2015). Tissue-resident Macrophages Originate from Yolk-Sac-Derived Erythro-Myeloid Progenitors. Nature 518 (7540), 547–551. doi:10.1038/nature13989
Govindarajah, V., Lee, J.-M., Solomon, M., Goddard, B., Nayak, R., Nattamai, K., et al. (2020). FOXO Activity Adaptation Safeguards the Hematopoietic Stem Cell Compartment in Hyperglycemia. Blood Adv. 4 (21), 5512–5526. doi:10.1182/bloodadvances.2020001826
Goyama, S., and Kurokawa, M. (2009). Pathogenetic Significance of Ecotropic Viral Integration Site-1 in Hematological Malignancies. Cancer Sci. 100 (6), 990–995. doi:10.1111/j.1349-7006.2009.01152.x
Grenier-Pleau, I., and Abraham, S. A. (2021). Extracellular Vesicles Tell All: How Vesicle-Mediated Cellular Communication Shapes Hematopoietic Stem Cell Biology with Increasing Age. Exp. Hematol. 101-102, 7–15. doi:10.1016/j.exphem.2021.08.004
Grinenko, T., Eugster, A., Thielecke, L., Ramasz, B., Krüger, A., Dietz, S., et al. (2018). Hematopoietic Stem Cells Can Differentiate into Restricted Myeloid Progenitors before Cell Division in Mice. Nat. Commun. 9 (1), 1–10. doi:10.1038/s41467-018-04188-7
Guo, H., Ma, O., Speck, N. A., and Friedman, A. D. (2012). Runx1 Deletion or Dominant Inhibition Reduces Cebpa Transcription via Conserved Promoter and Distal Enhancer Sites to Favor Monopoiesis over Granulopoiesis. Blood J. Am. Soc. Hematol. 119 (19), 4408–4418. doi:10.1182/blood-2011-12-397091
Gurumurthy, S., Xie, S. Z., Alagesan, B., Kim, J., Yusuf, R. Z., Saez, B., et al. (2010). The Lkb1 Metabolic Sensor Maintains Haematopoietic Stem Cell Survival. Nature 468 (7324), 659–663. doi:10.1038/nature09572
Haferlach, T., Stengel, A., Eckstein, S., Perglerová, K., Alpermann, T., Kern, W., et al. (2016). The New Provisional WHO Entity 'RUNX1 Mutated AML' Shows Specific Genetics but No Prognostic Influence of Dysplasia. Leukemia 30 (10), 2109–2112. doi:10.1038/leu.2016.150
Harada, Y., Inoue, D., Ding, Y., Imagawa, J., Doki, N., Matsui, H., et al. (2013). RUNX1/AML1 Mutant Collaborates with BMI1 Overexpression in the Development of Human and Murine Myelodysplastic Syndromes. Blood J. Am. Soc. Hematol. 121 (17), 3434–3446. doi:10.1182/blood-2012-06-434423
Harshman, S. W., Canella, A., Ciarlariello, P. D., Agarwal, K., Branson, O. E., Rocci, A., et al. (2016). Proteomic Characterization of Circulating Extracellular Vesicles Identifies Novel Serum Myeloma Associated Markers. J. Proteomics 136, 89–98. doi:10.1016/j.jprot.2015.12.016
Hasemann, M. S., Lauridsen, F. K. B., Waage, J., Jakobsen, J. S., Frank, A.-K., Schuster, M. B., et al. (2014). C/EBPα Is Required for Long-Term Self-Renewal and Lineage Priming of Hematopoietic Stem Cells and for the Maintenance of Epigenetic Configurations in Multipotent Progenitors. Plos Genet. 10 (1), e1004079. doi:10.1371/journal.pgen.1004079
Hashimoto, M., Umemoto, T., Nakamura-Ishizu, A., Matsumura, T., Yokomizo, T., Sezaki, M., et al. (2021). Autophagy Is Dispensable for the Maintenance of Hematopoietic Stem Cells in Neonates. Blood Adv. 5 (6), 1594–1604. doi:10.1182/bloodadvances.2020002410
Heintz, N. (2001). BAC to the Future: the Use of Bac Transgenic Mice for Neuroscience Research. Nat. Rev. Neurosci. 2 (12), 861–870. doi:10.1038/35104049
Hidalgo, I., Herrera-Merchan, A., Ligos, J. M., Carramolino, L., Nuñez, J., Martinez, F., et al. (2012). Ezh1 Is Required for Hematopoietic Stem Cell Maintenance and Prevents Senescence-like Cell Cycle Arrest. Cell stem cell 11 (5), 649–662. doi:10.1016/j.stem.2012.08.001
Himburg, H. A., Harris, J. R., Ito, T., Daher, P., Russell, J. L., Quarmyne, M., et al. (2012). Pleiotrophin Regulates the Retention and Self-Renewal of Hematopoietic Stem Cells in the Bone Marrow Vascular Niche. Cel Rep. 2 (4), 964–975. doi:10.1016/j.celrep.2012.09.002
Hinge, A., Xu, J., Javier, J., Mose, E., Kumar, S., Kapur, R., et al. (2017). p190-B RhoGAP and Intracellular Cytokine Signals Balance Hematopoietic Stem and Progenitor Cell Self-Renewal and Differentiation. Nat. Commun. 8 (1), 1–14. doi:10.1038/ncomms14382
Höfer, T., Busch, K., Klapproth, K., and Rodewald, H.-R. (2016). Fate Mapping and Quantitation of Hematopoiesis In Vivo. Annu. Rev. Immunol. 34, 449–478. doi:10.1146/annurev-immunol-032414-112019
Höfer, T., and Rodewald, H.-R. (2018). Differentiation-based Model of Hematopoietic Stem Cell Functions and Lineage Pathways. Blood 132 (11), 1106–1113. doi:10.1182/blood-2018-03-791517
Hong, S. H., Yang, S. J., Kim, T. M., Shim, J. S., Lee, H. S., Lee, G. Y., et al. (2014). Molecular Integration of HoxB4 and STAT3 for Self‐Renewal of Hematopoietic Stem Cells: A Model of Molecular Convergence for Stemness. Stem Cells 32 (5), 1313–1322. doi:10.1002/stem.1631
Hosokawa, K., Kajigaya, S., Feng, X., Desierto, M. J., Fernandez Ibanez, M. d. P., Rios, O., et al. (2017). A Plasma microRNA Signature as a Biomarker for Acquired Aplastic Anemia. Haematologica 102 (1), 69–78. doi:10.3324/haematol.2016.151076
Hu, L., Zhang, Y., Miao, W., and Cheng, T. (2019). Reactive Oxygen Species and Nrf2: Functional and Transcriptional Regulators of Hematopoiesis. Oxid Med. Cel Longev 2019, 5153268. doi:10.1155/2019/5153268
Huang, X., Cho, S., and Spangrude, G. J. (2007). Hematopoietic Stem Cells: Generation and Self-Renewal. Cell Death Differ 14 (11), 1851–1859. doi:10.1038/sj.cdd.4402225
Huck, V., Gorzelanny, C., Schneider, M., and Schneider, S. (2014). The various states of von Willebrand factor and their function in physiology and pathophysiology. Thromb. Haemost. 111 (04), 598–609. doi:10.1160/th13-09-0800
Ichikawa, M., Yoshimi, A., Nakagawa, M., Nishimoto, N., Watanabe-Okochi, N., and Kurokawa, M. (2013). A Role for RUNX1 in Hematopoiesis and Myeloid Leukemia. Int. J. Hematol. 97 (6), 726–734. doi:10.1007/s12185-013-1347-3
Ito, K., Carracedo, A., Weiss, D., Arai, F., Ala, U., Avigan, D. E., et al. (2012). A PML-PPAR-δ Pathway for Fatty Acid Oxidation Regulates Hematopoietic Stem Cell Maintenance. Nat. Med. 18 (9), 1350–1358. doi:10.1038/nm.2882
Ito, K., Hirao, A., Arai, F., Takubo, K., Matsuoka, S., Miyamoto, K., et al. (2006). Reactive Oxygen Species Act through P38 MAPK to Limit the Lifespan of Hematopoietic Stem Cells. Nat. Med. 12 (4), 446–451. doi:10.1038/nm1388
Ito, K., and Suda, T. (2014). Metabolic Requirements for the Maintenance of Self-Renewing Stem Cells. Nat. Rev. Mol. Cel Biol 15 (4), 243–256. doi:10.1038/nrm3772
Ito, K., Takubo, K., Arai, F., Satoh, H., Matsuoka, S., Ohmura, M., et al. (2007). Regulation of Reactive Oxygen Species byAtmIs Essential for Proper Response to DNA Double-Strand Breaks in Lymphocytes. J. Immunol. 178 (1), 103–110. doi:10.4049/jimmunol.178.1.103
Ito, K., Turcotte, R., Cui, J., Zimmerman, S. E., Pinho, S., Mizoguchi, T., et al. (2016). Self-renewal of a Purified Tie2 + Hematopoietic Stem Cell Population Relies on Mitochondrial Clearance. Science 354 (6316), 1156–1160. doi:10.1126/science.aaf5530
Iwasaki, H., Somoza, C., Shigematsu, H., Duprez, E. A., Iwasaki-Arai, J., Mizuno, S.-i., et al. (2005). Distinctive and Indispensable Roles of PU.1 in Maintenance of Hematopoietic Stem Cells and Their Differentiation. Blood 106 (5), 1590–1600. doi:10.1182/blood-2005-03-0860
Jacobsen, S. E. W., and Nerlov, C. (2019). Haematopoiesis in the Era of Advanced Single-Cell Technologies. Nat. Cel Biol 21 (1), 2–8. doi:10.1038/s41556-018-0227-8
Jang, Y.-Y., and Sharkis, S. J. (2007). A Low Level of Reactive Oxygen Species Selects for Primitive Hematopoietic Stem Cells that May Reside in the Low-Oxygenic Niche. Blood 110 (8), 3056–3063. doi:10.1182/blood-2007-05-087759
Jiang, P., Wang, H., Zheng, J., Han, Y., Huang, H., and Qian, P. (2019). Epigenetic Regulation of Hematopoietic Stem Cell Homeostasis. Blood Sci. 1 (1), 19–28. doi:10.1097/bs9.0000000000000018
Jones, C. L., Stevens, B. M., D'Alessandro, A., Reisz, J. A., Culp-Hill, R., Nemkov, T., et al. (2018). Inhibition of Amino Acid Metabolism Selectively Targets Human Leukemia Stem Cells. Cancer cell 34 (5), 724–740. doi:10.1016/j.ccell.2018.10.005
Joseph, C., Quach, J. M., Walkley, C. R., Lane, S. W., Lo Celso, C., and Purton, L. E. (2013). Deciphering Hematopoietic Stem Cells in Their Niches: a Critical Appraisal of Genetic Models, Lineage Tracing, and Imaging Strategies. Cell stem cell 13 (5), 520–533. doi:10.1016/j.stem.2013.10.010
Jung, H., Kim, D. O., Byun, J. E., Kim, W. S., Kim, M. J., Song, H. Y., et al. (2016). Thioredoxin-interacting Protein Regulates Haematopoietic Stem Cell Ageing and Rejuvenation by Inhibiting P38 Kinase Activity. Nat. Commun. 7 (1), 1–12. doi:10.1038/ncomms13674
Kappen, C. (2000). Disruption of the Homeobox Gene Hoxb-6 in Mice Results in Increased Numbers of Early Erythrocyte Progenitors. Am. J. Hematol. 65 (2), 111–118. doi:10.1002/1096-8652(200010)65:2<111::aid-ajh4>3.0.co;2-z
Kataoka, K., Sato, T., Yoshimi, A., Goyama, S., Tsuruta, T., Kobayashi, H., et al. (2011). Evi1 Is Essential for Hematopoietic Stem Cell Self-Renewal, and its Expression marks Hematopoietic Cells with Long-Term Multilineage Repopulating Activity. J. Exp. Med. 208 (12), 2403–2416. doi:10.1084/jem.20110447
Kiel, M. J., YilmazÖ, Ö. H., Iwashita, T., Yilmaz, O. H., Terhorst, C., and Morrison, S. J. (2005). SLAM Family Receptors Distinguish Hematopoietic Stem and Progenitor Cells and Reveal Endothelial Niches for Stem Cells. cell 121 (7), 1109–1121. doi:10.1016/j.cell.2005.05.026
Kim, S. K., Selleri, L., Lee, J. S., Zhang, A. Y., Gu, X., Jacobs, Y., et al. (2002). Pbx1 Inactivation Disrupts Pancreas Development and in Ipf1-Deficient Mice Promotes Diabetes Mellitus. Nat. Genet. 30 (4), 430–435. doi:10.1038/ng860
King, K. Y., and Goodell, M. A. (2011). Inflammatory Modulation of HSCs: Viewing the HSC as a Foundation for the Immune Response. Nat. Rev. Immunol. 11 (10), 685–692. doi:10.1038/nri3062
Komorowska, K., Doyle, A., Wahlestedt, M., Subramaniam, A., Debnath, S., Chen, J., et al. (2017). Hepatic Leukemia Factor Maintains Quiescence of Hematopoietic Stem Cells and Protects the Stem Cell Pool during Regeneration. Cel Rep. 21 (12), 3514–3523. doi:10.1016/j.celrep.2017.11.084
Kondo, M., Weissman, I. L., and Akashi, K. (1997). Identification of Clonogenic Common Lymphoid Progenitors in Mouse Bone Marrow. Cell 91 (5), 661–672. doi:10.1016/s0092-8674(00)80453-5
Kroon, E., Krosl, J., Thorsteinsdottir, U., Baban, S., Buchberg, A. M., and Sauvageau, G. (1998). Hoxa9 Transforms Primary Bone Marrow Cells through Specific Collaboration with Meis1a but Not Pbx1b. EMBO J. 17 (13), 3714–3725. doi:10.1093/emboj/17.13.3714
Kunisaki, Y., Bruns, I., Scheiermann, C., Ahmed, J., Pinho, S., Zhang, D., et al. (2013). Arteriolar Niches Maintain Haematopoietic Stem Cell Quiescence. Nature 502 (7473), 637–643. doi:10.1038/nature12612
Lacombe, J., Herblot, S., Rojas-Sutterlin, S., Haman, A., Barakat, S., Iscove, N. N., et al. (2010). Scl Regulates the Quiescence and the Long-Term Competence of Hematopoietic Stem Cells. Blood 115 (4), 792–803. doi:10.1182/blood-2009-01-201384
Lacorazza, H. D., Yamada, T., Liu, Y., Miyata, Y., Sivina, M., Nunes, J., et al. (2006). The Transcription Factor MEF/ELF4 Regulates the Quiescence of Primitive Hematopoietic Cells. Cancer cell 9 (3), 175–187. doi:10.1016/j.ccr.2006.02.017
Lajtha, L. G., Oliver, R., and Gurney, C. W. (1962). Kinetic Model of a Bone-Marrow Stem-Cell Population. Br. J. Haematol. 8 (4), 442–460. doi:10.1111/j.1365-2141.1962.tb06548.x
Laurenti, E., and Göttgens, B. (2018). From Haematopoietic Stem Cells to Complex Differentiation Landscapes. Nature 553 (7689), 418–426. doi:10.1038/nature25022
Lecellier, C.-H., and Voinnet, O. (2004). RNA Silencing: No Mercy for Viruses? Immunol. Rev. 198 (1), 285–303. doi:10.1111/j.0105-2896.2004.00128.x
Lee, B. H., Castrillon, D. H., Cullen, D. E., McDowell, E. P., Lazo-Kallanian, S., Williams, I. R., et al. (2007). FoxOs Are Critical Mediators of Hematopoietic Stem Cell Resistance to Physiologic Oxidative Stress. Cell 128, 325–339.
Li, D., Tian, Z., Tang, W., Zhang, J., Lu, L., Sun, Z., et al. (2016). The Protective Effects of 5-Methoxytryptamine-α-Lipoic Acid on Ionizing Radiation-Induced Hematopoietic Injury. Ijms 17 (6), 935. doi:10.3390/ijms17060935
Li, J., Cai, D., Yao, X., Zhang, Y., Chen, L., Jing, P., et al. (2016). Protective Effect of Ginsenoside Rg1 on Hematopoietic Stem/Progenitor Cells through Attenuating Oxidative Stress and the Wnt/β-Catenin Signaling Pathway in a Mouse Model of D-Galactose-Induced Aging. Ijms 17 (6), 849. doi:10.3390/ijms17060849
Li, J. (2011). Quiescence Regulators for Hematopoietic Stem Cell. Exp. Hematol. 39 (5), 511–520. doi:10.1016/j.exphem.2011.01.008
Liu, Y., Elf, S. E., Miyata, Y., Sashida, G., Liu, Y., Huang, G., et al. (2009). p53 Regulates Hematopoietic Stem Cell Quiescence. Cell stem cell 4 (1), 37–48. doi:10.1016/j.stem.2008.11.006
Ludikhuize, M. C., and Rodríguez Colman, M. J. (2020). Metabolic Regulation of Stem Cells and Differentiation: A Forkhead Box O Transcription Factor Perspective. Antioxidants & Redox Signaling.
Ludin, A., Gur-Cohen, S., Golan, K., Kaufmann, K. B., Itkin, T., Medaglia, C., et al. (2014). Reactive Oxygen Species Regulate Hematopoietic Stem Cell Self-Renewal, Migration and Development, as Well as Their Bone Marrow Microenvironment. Antioxid. Redox Signaling 21 (11), 1605–1619. doi:10.1089/ars.2014.5941
Lüscher-Firzlaff, J., Chatain, N., Kuo, C. C., Braunschweig, T., Bochyńska, A., Ullius, A., et al. (2019). Hematopoietic Stem and Progenitor Cell Proliferation and Differentiation Requires the Trithorax Protein Ash2l. Sci. Rep. 9 (1), 1–16. doi:10.1038/s41598-019-44720-3
MacRae, C. A., and Peterson, R. T. (2015). Zebrafish as Tools for Drug Discovery. Nat. Rev. Drug Discov. 14 (10), 721–731. doi:10.1038/nrd4627
Manz, M. G., Miyamoto, T., Akashi, K., and Weissman, I. L. (2002). Prospective Isolation of Human Clonogenic Common Myeloid Progenitors. Proc. Natl. Acad. Sci. 99 (18), 11872–11877. doi:10.1073/pnas.172384399
Martin, D. I., and Orkin, S. H. (1990). Transcriptional Activation and DNA Binding by the Erythroid Factor GF-1/nf-E1/Eryf 1. Genes Dev. 4 (11), 1886–1898. doi:10.1101/gad.4.11.1886
McKercher, S. R., Torbett, B. E., Anderson, K. L., Henkel, G. W., Vestal, D. J., Baribault, H., et al. (1996). Targeted Disruption of the PU.1 Gene Results in Multiple Hematopoietic Abnormalities. EMBO J. 15 (20), 5647–5658. doi:10.1002/j.1460-2075.1996.tb00949.x
Menendez-Gonzalez, J. B., Vukovic, M., Abdelfattah, A., Saleh, L., Almotiri, A., Thomas, L.-a., et al. (2019). Gata2 as a Crucial Regulator of Stem Cells in Adult Hematopoiesis and Acute Myeloid Leukemia. Stem Cel. Rep. 13 (2), 291–306. doi:10.1016/j.stemcr.2019.07.005
Metcalf, D. (2008). Hematopoietic Cytokines. Blood J. Am. Soc. Hematol. 111 (2), 485–491. doi:10.1182/blood-2007-03-079681
Mirantes, C., Passegué, E., and Pietras, E. M. (2014). Pro-inflammatory Cytokines: Emerging Players Regulating HSC Function in normal and Diseased Hematopoiesis. Exp. Cel. Res. 329 (2), 248–254. doi:10.1016/j.yexcr.2014.08.017
Miyamoto, K., Araki, K. Y., Naka, K., Arai, F., Takubo, K., Yamazaki, S., et al. (2007). Foxo3a Is Essential for Maintenance of the Hematopoietic Stem Cell Pool. Cell stem cell 1 (1), 101–112. doi:10.1016/j.stem.2007.02.001
Mizushima, N., and Levine, B. (2010). Autophagy in Mammalian Development and Differentiation. Nat. Cel Biol 12 (9), 823–830. doi:10.1038/ncb0910-823
Moignard, V., Macaulay, I. C., Swiers, G., Buettner, F., Schütte, J., Calero-Nieto, F. J., et al. (2013). Characterization of Transcriptional Networks in Blood Stem and Progenitor Cells Using High-Throughput Single-Cell Gene Expression Analysis. Nat. Cel Biol 15 (4), 363–372. doi:10.1038/ncb2709
Moore, T. (2021). Hematopoietic Stem Cell Transplantation. (Medscape), 1–33. Available at: https://emedicine.medscape.com/article/208954-overview
Moraes, M. P., Mayr, G. A., Mason, P. W., and Grubman, M. J. (2002). Early protection against Homologous challenge after a Single Dose of Replication-Defective Human Adenovirus Type 5 Expressing Capsid Proteins of Foot-And-Mouth Disease Virus (FMDV) Strain A24. Vaccine 20 (11-12), 1631–1639. doi:10.1016/s0264-410x(01)00483-2
Morita, Y., Ema, H., and Nakauchi, H. (2010). Heterogeneity and Hierarchy within the Most Primitive Hematopoietic Stem Cell Compartment. J. Exp. Med. 207 (6), 1173–1182. doi:10.1084/jem.20091318
Morrison, S. J., and Scadden, D. T. (2014). The Bone Marrow Niche for Haematopoietic Stem Cells. Nature 505 (7483), 327–334. doi:10.1038/nature12984
Morrison, S. J., Uchida, N., and Weissman, I. L. (1995). The Biology of Hematopoietic Stem Cells. Annu. Rev. Cel Dev. Biol. 11 (1), 35–71. doi:10.1146/annurev.cb.11.110195.000343
Morrison, S. J., Wandycz, A. M., Hemmati, H. D., Wright, D. E., and Weissman, I. L. (1997). Identification of a Lineage of Multipotent Hematopoietic Progenitors. Development 124 (10), 1929–1939. doi:10.1242/dev.124.10.1929
Motoike, T., Loughna, S., Perens, E., Roman, B. L., Liao, W., Chau, T. C., et al. (2000). Universal GFP Reporter for the Study of Vascular Development. genesis 28 (2), 75–81. doi:10.1002/1526-968x(200010)28:2<75::aid-gene50>3.0.co;2-s
Mueller, B. U., Pabst, T., Osato, M., Asou, N., Johansen, L. M., Minden, M. D., et al. (2002). Heterozygous PU.1 Mutations Are Associated with Acute Myeloid Leukemia. Blood J. Am. Soc. Hematol. 100 (3), 998–1007. doi:10.1182/blood.v100.3.998
Murakami, S., Suzuki, T., Harigae, H., Romeo, P. H., Yamamoto, M., and Motohashi, H. (2017). NRF2 Activation Impairs Quiescence and Bone Marrow Reconstitution Capacity of Hematopoietic Stem Cells. Mol. Cel Biol 37. doi:10.1128/MCB.00086-17
Nakada, D., Saunders, T. L., and Morrison, S. J. (2010). Lkb1 Regulates Cell Cycle and Energy Metabolism in Haematopoietic Stem Cells. Nature 468 (7324), 653–658. doi:10.1038/nature09571
Nestorowa, S., Hamey, F. K., Pijuan Sala, B., Diamanti, E., Shepherd, M., Laurenti, E., et al. (2016). A Single-Cell Resolution Map of Mouse Hematopoietic Stem and Progenitor Cell Differentiation. J. Am. Soc. Hematol. 128 (8), e20–e31. doi:10.1182/blood-2016-05-716480
Oguro, H., Ding, L., and Morrison, S. J. (2013). SLAM Family Markers Resolve Functionally Distinct Subpopulations of Hematopoietic Stem Cells and Multipotent Progenitors. Cell stem cell 13 (1), 102–116. doi:10.1016/j.stem.2013.05.014
Orkin, S. H. (2000). Diversification of Haematopoietic Stem Cells to Specific Lineages. Nat. Rev. Genet. 1 (1), 57–64. doi:10.1038/35049577
Osato, M. (2004). Point Mutations in the RUNX1/AML1 Gene: Another Actor in RUNX Leukemia. Oncogene 23 (24), 4284–4296. doi:10.1038/sj.onc.1207779
Papa, L., Djedaini, M., and Hoffman, R. (2020). Ex Vivo HSC Expansion Challenges the Paradigm of Unidirectional Human Hematopoiesis. Ann. N.Y. Acad. Sci. 1466 (1), 39–50. doi:10.1111/nyas.14133
Park, C., Shin, J., Yalcin, S., and Hu, W. (2013). Low C-Kit Expression Identifies Hematopoietic Stem Cells with Enhanced Self-Renewal Potential. Exp. Hematol. 41 (8), S43. doi:10.1016/j.exphem.2013.05.171
Park, I.-k., Qian, D., Kiel, M., Becker, M. W., Pihalja, M., Weissman, I. L., et al. (2003). Bmi-1 Is Required for Maintenance of Adult Self-Renewing Haematopoietic Stem Cells. Nature 423 (6937), 302–305. doi:10.1038/nature01587
Paul, M. K., Bisht, B., Darmawan, D. O., Chiou, R., Ha, V. L., Wallace, W. D., et al. (2014). Dynamic Changes in Intracellular ROS Levels Regulate Airway Basal Stem Cell Homeostasis through Nrf2-dependent Notch Signaling. Cell stem cell 15 (2), 199–214. doi:10.1016/j.stem.2014.05.009
Pazianos, G., Uqoezwa, M., and Reya, T. (2003). The Elements of Stem Cell Self-Renewal: a Genetic Perspective. Biotechniques 35 (6), 1240–1247. doi:10.2144/03356ss03
Pengo, N., Scolari, M., Oliva, L., Milan, E., Mainoldi, F., Raimondi, A., et al. (2013). Plasma Cells Require Autophagy for Sustainable Immunoglobulin Production. Nat. Immunol. 14 (3), 298–305. doi:10.1038/ni.2524
Pietras, E. M., Reynaud, D., Kang, Y.-A., Carlin, D., Calero-Nieto, F. J., Leavitt, A. D., et al. (2015). Functionally Distinct Subsets of Lineage-Biased Multipotent Progenitors Control Blood Production in normal and Regenerative Conditions. Cell stem cell 17 (1), 35–46. doi:10.1016/j.stem.2015.05.003
Pineault, N., Helgason, C. D., Lawrence, H. J., and Humphries, R. K. (2002). Differential Expression of Hox, Meis1, and Pbx1 Genes in Primitive Cells throughout Murine Hematopoietic Ontogeny. Exp. Hematol. 30 (1), 49–57. doi:10.1016/s0301-472x(01)00757-3
Post, Y., and Clevers, H. (2019). Defining Adult Stem Cell Function at its Simplest: the Ability to Replace Lost Cells through Mitosis. Cell Stem Cell 25 (2), 174–183. doi:10.1016/j.stem.2019.07.002
Pouzolles, M., Oburoglu, L., Taylor, N., and Zimmermann, V. S. (2016). Hematopoietic Stem Cell Lineage Specification. Curr. Opin. Hematol. 23 (4), 311–317. doi:10.1097/moh.0000000000000260
Preudhomme, C., Warot-Loze, D., Roumier, C., Grardel-Duflos, N., Garand, R., Lai, J. L., et al. (2000). High Incidence of Biallelic point Mutations in the Runt Domain of the AML1/PEBP2αB Gene in Mo Acute Myeloid Leukemia and in Myeloid Malignancies with Acquired Trisomy 21. Blood J. Am. Soc. Hematol. 96 (8), 2862–2869. doi:10.1182/blood.v96.8.2862.h8002862_2862_2869
Qian, P., He, X. C., Paulson, A., Li, Z., Tao, F., Perry, J. M., et al. (2016). The Dlk1-Gtl2 Locus Preserves LT-HSC Function by Inhibiting the PI3K-mTOR Pathway to Restrict Mitochondrial Metabolism. Cell Stem Cell 18 (2), 214–228. doi:10.1016/j.stem.2015.11.001
Raghav, P. K., Singh, A. K., and Gangenahalli, G. (2018). Stem Cell Factor and NSC87877 Combine to Enhance C-Kit Mediated Proliferation of Human Megakaryoblastic Cells. PloS one 13, e0206364. doi:10.1371/journal.pone.0206364
Raghav, P. K., and Gangenahalli, G. (2018). Hematopoietic Stem Cell Molecular Targets and Factors Essential for Hematopoiesis. J. Stem Cel Res Ther 8 (441), 2. doi:10.4172/2157-7633.1000441
Raghav, P. K., and Gangenahalli, G. (2021). PU.1 Mimic Synthetic Peptides Selectively Bind with GATA-1 and Allow C-Jun PU.1 Binding to Enhance Myelopoiesis. Ijn Vol. 16, 3833–3859. doi:10.2147/ijn.s303235
Raghav, P. K., Singh, A. K., and Gangenahalli, G. (2018). Stem Cell Factor and NSC87877 Synergism Enhances C-Kit Mediated Proliferation of Human Erythroid Cells. Life Sci. 214, 84–97. doi:10.1016/j.lfs.2018.09.055
Razmkhah, F., Soleimani, M., Mehrabani, D., Karimi, M. H., Amini Kafi-abad, S., Ramzi, M., et al. (2017). Leukemia Microvesicles Affect Healthy Hematopoietic Stem Cells. Tumour Biol. 39 (2), 1010428317692234. doi:10.1177/1010428317692234
Reya, T., Morrison, S. J., Clarke, M. F., and Weissman, I. L. (2001). Stem Cells, Cancer, and Cancer Stem Cells. nature 414 (6859), 105–111. doi:10.1038/35102167
Reynaud, D., Ravet, E., Titeux, M., Mazurier, F., Rénia, L., Dubart-Kupperschmitt, A., et al. (2005). SCL/TAL1 Expression Level Regulates Human Hematopoietic Stem Cell Self-Renewal and Engraftment. Blood 106 (7), 2318–2328. doi:10.1182/blood-2005-02-0557
Rieger, M. A., and Schroeder, T. (2012). Hematopoiesis. Cold Spring Harb Perspect. Biol. 4. doi:10.1101/cshperspect.a008250
Riffelmacher, T., and Simon, A. K. (2017). Mechanistic Roles of Autophagy in Hematopoietic Differentiation. Febs J. 284 (7), 1008–1020. doi:10.1111/febs.13962
Robb, L. (2007). Cytokine Receptors and Hematopoietic Differentiation. Oncogene 26 (47), 6715–6723. doi:10.1038/sj.onc.1210756
Rodrigues, C. P., Shvedunova, M., and Akhtar, A. (2021). Epigenetic Regulators as the Gatekeepers of Hematopoiesis. Trends Genet. 37 (2), 125–142. doi:10.1016/j.tig.2020.09.015
Rojas-Sutterlin, S., and Hoang, T. (2013). Tumor Dormancy, Quiescence, and Senescence, Volume 1. Dordrecht: Springer, 87–105. doi:10.1007/978-94-007-5958-9_8Hematopoietic Stem Cell Quiescence and Long Term Maintenance: Role of SCL/TAL1
Rojas-Sutterlin, S., Lecuyer, E., and Hoang, T. (2014). Kit and Scl Regulation of Hematopoietic Stem Cells. Curr. Opin. Hematol. 21 (4), 256–264. doi:10.1097/moh.0000000000000052
Romero-Moya, D., Bueno, C., Montes, R., Navarro-Montero, O., Iborra, F. J., López, L. C., et al. (2013). Cord Blood-Derived CD34+ Hematopoietic Cells with Low Mitochondrial Mass Are Enriched in Hematopoietic Repopulating Stem Cell Function. Haematologica 98 (7), 1022–1029. doi:10.3324/haematol.2012.079244
Rossi, D. J., Seita, J., Czechowicz, A., Bhattacharya, D., Bryder, D., and Weissman, I. L. (2007). Hematopoietic Stem Cell Quiescence Attenuates DNA Damage Response and Permits DNA Damage Accumulation during Aging. Cell cycle 6 (19), 2371–2376. doi:10.4161/cc.6.19.4759
Rowe, R. G., Mandelbaum, J., Zon, L. I., and Daley, G. Q. (2016). Engineering Hematopoietic Stem Cells: Lessons from Development. Cell stem cell 18 (6), 707–720. doi:10.1016/j.stem.2016.05.016
Rylski, M., Welch, J. J., Chen, Y.-Y., Letting, D. L., Diehl, J. A., Chodosh, L. A., et al. (2003). GATA-1-mediated Proliferation Arrest during Erythroid Maturation. Mol. Cel Biol 23 (14), 5031–5042. doi:10.1128/mcb.23.14.5031-5042.2003
Ryoo, I. G., Lee, S. H., Kwak, M. K., and Redox modulating Nrf2, (2016). Redox Modulating NRF2: A Potential Mediator of Cancer Stem Cell Resistance. Oxid Med. Cel Longev 2016, 2428153. doi:10.1155/2016/2428153
Salio, M., Puleston, D. J., Mathan, T. S. M., Shepherd, D., Stranks, A. J., Adamopoulou, E., et al. (2014). Essential Role for Autophagy during Invariant NKT Cell Development. Proc. Natl. Acad. Sci. USA 111 (52), E5678–E5687. doi:10.1073/pnas.1413935112
Sanjuan-Pla, A., Macaulay, I. C., Jensen, C. T., Woll, P. S., Luis, T. C., Mead, A., et al. (2013). Platelet-biased Stem Cells Reside at the apex of the Haematopoietic Stem-Cell Hierarchy. Nature 502 (7470), 232–236. doi:10.1038/nature12495
Sato, T., Goyama, S., Nitta, E., Takeshita, M., Yoshimi, M., Nakagawa, M., et al. (2008). Evi-1 Promotes Para-Aortic Splanchnopleural Hematopoiesis through Up-Regulation of GATA-2 and Repression of TGF-B Signaling. Cancer Sci. 99 (7), 1407–1413. doi:10.1111/j.1349-7006.2008.00842.x
Sauvageau, G., Lansdorp, P. M., Eaves, C. J., Hogge, D. E., Dragowska, W. H., Reid, D. S., et al. (1994). Differential Expression of Homeobox Genes in Functionally Distinct CD34+ Subpopulations of Human Bone Marrow Cells. Proc. Natl. Acad. Sci. 91 (25), 12223–12227. doi:10.1073/pnas.91.25.12223
Scadden, D. T. (2014). Nice Neighborhood: Emerging Concepts of the Stem Cell Niche. Cell 157 (1), 41–50. doi:10.1016/j.cell.2014.02.013
Schmidt, T., Karsunky, H., Rödel, B., Zevnik, B., Elsässer, H.-P., and Möröy, T. (1998). Evidence Implicating Gfi-1 and Pim-1 in Pre-T-cell Differentiation Steps Associated with β-selection. Embo J. 17 (18), 5349–5359. doi:10.1093/emboj/17.18.5349
Scott, E. W., Simon, M. C., Anastasi, J., and Singh, H. (1994). Requirement of Transcription Factor PU.1 in the Development of Multiple Hematopoietic Lineages. Science 265 (5178), 1573–1577. doi:10.1126/science.8079170
Seita, J., and Weissman, I. L. (2010). Hematopoietic Stem Cell: Self-Renewal versus Differentiation. Wires Syst. Biol. Med. 2 (6), 640–653. doi:10.1002/wsbm.86
Senyuk, V., Sinha, K. K., Li, D., Rinaldi, C. R., Yanamandra, S., and Nucifora, G. (2007). Repression of RUNX1 Activity by EVI1: a New Role of EVI1 in Leukemogenesis. Cancer Res. 67 (12), 5658–5666. doi:10.1158/0008-5472.can-06-3962
Shao, L., Luo, Y., and Zhou, D. (2014). Hematopoietic Stem Cell Injury Induced by Ionizing Radiation. Antioxid. Redox Signaling 20 (9), 1447–1462. doi:10.1089/ars.2013.5635
Shimabe, M., Goyama, S., Watanabe-Okochi, N., Yoshimi, A., Ichikawa, M., Imai, Y., et al. (2009). Pbx1 Is a Downstream Target of Evi-1 in Hematopoietic Stem/progenitors and Leukemic Cells. Oncogene 28 (49), 4364–4374. doi:10.1038/onc.2009.288
Shin, J. Y., Hu, W., Naramura, M., and Park, C. Y. (2014). High C-Kit Expression Identifies Hematopoietic Stem Cells with Impaired Self-Renewal and Megakaryocytic Bias. J. Exp. Med. 211 (2), 217–231. doi:10.1084/jem.20131128
Shinohara, A., Imai, Y., Nakagawa, M., Takahashi, T., Ichikawa, M., and Kurokawa, M. (2014). Intracellular Reactive Oxygen Species Mark and Influence the Megakaryocyte-Erythrocyte Progenitor Fate of Common Myeloid Progenitors. Stem Cells 32 (2), 548–557. doi:10.1002/stem.1588
Shiohara, M., Koike, K., and Nakahata, T. (1993). Synergism of Interferon-Gamma and Stem Cell Factor on the Development of Murine Hematopoietic Progenitors in Serum-free Culture.doi:10.1182/blood.v81.6.1435.bloodjournal8161435
Soza-Ried, C., Hess, I., Netuschil, N., Schorpp, M., and Boehm, T. (2010). Essential Role of C-Myb in Definitive Hematopoiesis Is Evolutionarily Conserved. Proc. Natl. Acad. Sci. 107 (40), 17304–17308. doi:10.1073/pnas.1004640107
Speck, N. A., and Iruela-Arispe, M. L. (2009). Conditional Cre/LoxP Strategies for the Study of Hematopoietic Stem Cell Formation. Blood Cell Mol. Dis. 43 (1), 6–11. doi:10.1016/j.bcmd.2009.01.010
Staber, P. B., Zhang, P., Ye, M., Welner, R. S., Nombela-Arrieta, C., Bach, C., et al. (2013). Sustained PU.1 Levels Balance Cell-Cycle Regulators to Prevent Exhaustion of Adult Hematopoietic Stem Cells. Mol. Cel. 49 (5), 934–946. doi:10.1016/j.molcel.2013.01.007
Suda, T., Takubo, K., and Semenza, G. L. (2011). Metabolic Regulation of Hematopoietic Stem Cells in the Hypoxic Niche. Cell stem cell 9 (4), 298–310. doi:10.1016/j.stem.2011.09.010
Sui, X., Krantz, S. B., You, M., and Zhao, Z. (1998). Synergistic Activation of MAP Kinase (ERK1/2) by Erythropoietin and Stem Cell Factor Is Essential for Expanded Erythropoiesis. J. Am. Soc. Hematol. 92 (4), 1142–1149. doi:10.1182/blood.v92.4.1142.416k42_1142_1149
Suico, M. A., Shuto, T., and Kai, H. (2017). Roles and Regulations of the ETS Transcription Factor ELF4/MEF. J. Mol. Cel Biol 9 (3), 168–177. doi:10.1093/jmcb/mjw051
Sun, J., Ramos, A., Chapman, B., Johnnidis, J. B., Le, L., Ho, Y.-J., et al. (2014). Clonal Dynamics of Native Haematopoiesis. Nature 514 (7522), 322–327. doi:10.1038/nature13824
Suryavanshi, S., Sharma, D., Checker, R., Thoh, M., Gota, V., Sandur, S. K., et al. (2015). Amelioration of Radiation-Induced Hematopoietic Syndrome by an Antioxidant Chlorophyllin through Increased Stem Cell Activity and Modulation of Hematopoiesis. Free Radic. Biol. Med. 85, 56–70. doi:10.1016/j.freeradbiomed.2015.04.007
Suzuki, M., Kobayashi-Osaki, M., Tsutsumi, S., Pan, X., Ohmori, S. Y., Takai, J., et al. (2013). GATA Factor Switching from GATA2 to GATA1 Contributes to Erythroid Differentiation. Genes Cells 18 (11), 921–933. doi:10.1111/gtc.12086
Szczepanski, M. J., Szajnik, M., Welsh, A., Whiteside, T. L., and Boyiadzis, M. (2011). Blast-derived Microvesicles in Sera from Patients with Acute Myeloid Leukemia Suppress Natural Killer Cell Function via Membrane-Associated Transforming Growth Factor- 1. Haematologica 96 (9), 1302–1309. doi:10.3324/haematol.2010.039743
Tabe, Y., Lorenzi, P. L., and Konopleva, M. (2019). Amino Acid Metabolism in Hematologic Malignancies and the Era of Targeted Therapy. Blood J. Am. Soc. Hematol. 134 (13), 1014–1023. doi:10.1182/blood.2019001034
Tamma, R., and Ribatti, D. (2017). Bone Niches, Hematopoietic Stem Cells, and Vessel Formation. Ijms 18 (1), 151. doi:10.3390/ijms18010151
Taniguchi Ishikawa, E., Gonzalez-Nieto, D., Ghiaur, G., Dunn, S. K., Ficker, A. M., Murali, B., et al. (2012). Connexin-43 Prevents Hematopoietic Stem Cell Senescence through Transfer of Reactive Oxygen Species to Bone Marrow Stromal Cells. Proc. Natl. Acad. Sci. 109 (23), 9071–9076. doi:10.1073/pnas.1120358109
Taylor, J., Xiao, W., and Abdel-Wahab, O. (2017). Diagnosis and Classification of Hematologic Malignancies on the Basis of Genetics. Blood 130 (4), 410–423. doi:10.1182/blood-2017-02-734541
Thorsteinsdottir, U., Mamo, A., Kroon, E., Jerome, L., Bijl, J., Lawrence, H. J., et al. (2002). Overexpression of the Myeloid Leukemia-associatedHoxa9 Gene in Bone Marrow Cells Induces Stem Cell Expansion. Blood J. Am. Soc. Hematol. 99 (1), 121–129. doi:10.1182/blood.v99.1.121
Tober, J., Yzaguirre, A. D., Piwarzyk, E., and Speck, N. A. (2013). Distinct Temporal Requirements for Runx1 in Hematopoietic Progenitors and Stem Cells. Development 140 (18), 3765–3776. doi:10.1242/dev.094961
Tothova, Z., and Gilliland, D. G. (2007). FoxO Transcription Factors and Stem Cell Homeostasis: Insights from the Hematopoietic System. Cell stem cell 1 (2), 140–152. doi:10.1016/j.stem.2007.07.017
Tothova, Z., Kollipara, R., Huntly, B. J., Lee, B. H., Castrillon, D. H., Cullen, D. E., et al. (2007). FoxOs Are Critical Mediators of Hematopoietic Stem Cell Resistance to Physiologic Oxidative Stress. Cell 128 (2), 325–339. doi:10.1016/j.cell.2007.01.003
Trainor, C. D., Omichinski, J. G., Vandergon, T. L., Gronenborn, A. M., Clore, G. M., and Felsenfeld, G. (1996). A Palindromic Regulatory Site within Vertebrate GATA-1 Promoters Requires Both Zinc Fingers of the GATA-1 DNA-Binding Domain for High-Affinity Interaction. Mol. Cel Biol 16 (5), 2238–2247. doi:10.1128/mcb.16.5.2238
Trumpp, A., Essers, M., and Wilson, A. (2010). Awakening Dormant Haematopoietic Stem Cells. Nat. Rev. Immunol. 10 (3), 201–209. doi:10.1038/nri2726
Tsai, J. J., Dudakov, J. A., Takahashi, K., Shieh, J.-H., Velardi, E., Holland, A. M., et al. (2013). Nrf2 Regulates Haematopoietic Stem Cell Function. Nat. Cel Biol 15 (3), 309–316. doi:10.1038/ncb2699
Umemoto, T., Hashimoto, M., Matsumura, T., Nakamura-Ishizu, A., and Suda, T. (2018). Ca2+-mitochondria axis Drives Cell Division in Hematopoietic Stem Cells. J. Exp. Med. 215 (8), 2097–2113. doi:10.1084/jem.20180421
Umemoto, T., Yamato, M., Nishida, K., Yang, J., Tano, Y., and Okano, T. (2005). p57Kip2 Is Expressed in Quiescent Mouse Bone Marrow Side Population Cells. Biochem. biophysical Res. Commun. 337 (1), 14–21. doi:10.1016/j.bbrc.2005.09.008
Upadhaya, S., Reizis, B., and Sawai, C. M. (2018). New Genetic Tools for the In Vivo Study of Hematopoietic Stem Cell Function. Exp. Hematol. 61, 26–35. doi:10.1016/j.exphem.2018.02.004
Vainshtein, A., Veenman, L., Shterenberg, A., Singh, S., Masarwa, A., Dutta, B., et al. (2015). Quinazoline-based Tricyclic Compounds that Regulate Programmed Cell Death, Induce Neuronal Differentiation, and Are Curative in Animal Models for Excitotoxicity and Hereditary Brain Disease. Cell Death Discov. 1 (1), 1–17. doi:10.1038/cddiscovery.2015.27
Vannini, N., Girotra, M., Naveiras, O., Nikitin, G., Campos, V., Giger, S., et al. (2016). Specification of Haematopoietic Stem Cell Fate via Modulation of Mitochondrial Activity. Nat. Commun. 7 (1), 13125–13129. doi:10.1038/ncomms13125
Velasco-Hernandez, T., Säwén, P., Bryder, D., and Cammenga, J. (2016). Potential Pitfalls of the Mx1-Cre System: Implications for Experimental Modeling of normal and Malignant Hematopoiesis. Stem Cel. Rep. 7 (1), 11–18. doi:10.1016/j.stemcr.2016.06.002
Wagner, J. E., Brunstein, C. G., Boitano, A. E., DeFor, T. E., McKenna, D., Sumstad, D., et al. (2016). Phase I/II Trial of StemRegenin-1 Expanded Umbilical Cord Blood Hematopoietic Stem Cells Supports Testing as a Stand-Alone Graft. Cell stem cell 18 (1), 144–155. doi:10.1016/j.stem.2015.10.004
Wanet, A., Arnould, T., Najimi, M., and Renard, P. (2015). Connecting Mitochondria, Metabolism, and Stem Cell Fate. Stem Cell Dev. 24 (17), 1957–1971. doi:10.1089/scd.2015.0117
Wang, T., Nandakumar, V., Jiang, X.-X., Jones, L., Yang, A.-G., Huang, X. F., et al. (2013). The Control of Hematopoietic Stem Cell Maintenance, Self-Renewal, and Differentiation by Mysm1-Mediated Epigenetic Regulation. Blood J. Am. Soc. Hematol. 122 (16), 2812–2822. doi:10.1182/blood-2013-03-489641
Wang, Z., and Ema, H. (2016). Mechanisms of Self-Renewal in Hematopoietic Stem Cells. Int. J. Hematol. 103 (5), 498–509. doi:10.1007/s12185-015-1919-5
Warr, M. R., Binnewies, M., Flach, J., Reynaud, D., Garg, T., Malhotra, R., et al. (2013). FOXO3A Directs a Protective Autophagy Program in Haematopoietic Stem Cells. Nature 494 (7437), 323–327. doi:10.1038/nature11895
Warr, M. R., and Passegué, E. (2013). Metabolic Makeover for HSCs. Cell Stem Cell 12 (1), 1–3. doi:10.1016/j.stem.2012.12.005
Watson, A. S., Riffelmacher, T., Stranks, A., Williams, O., De Boer, J., Cain, K., et al. (2015). Autophagy Limits Proliferation and Glycolytic Metabolism in Acute Myeloid Leukemia. Cel Death Discov 1 (1), 1–10. doi:10.1038/cddiscovery.2015.8
Welner, R. S., Bararia, D., Levantini, E., Camacho, V., Patel, S., Matkins, V. R., and Tenen, D. G. (2020). Pu, . 1 Mediates Hematopoietic Stem Cell Self-Renewal during Cellular Stress by Non-canonical Wnt Signaling.
Whyatt, D., Karis, A., Harkes, I., Verkerk, A., Gillemans, N., Elefanty, A., et al. (1997). The Level of the Tissue‐specific Factor GATA‐1 Affects the Cell‐cycle Machinery. Genes Funct. 1 (1), 11–24. doi:10.1046/j.1365-4624.1997.00003.x
Wilkinson, A. C., Igarashi, K. J., and Nakauchi, H. (2020). Haematopoietic Stem Cell Self-Renewal In Vivo and Ex Vivo. Nat. Rev. Genet. 21 (9), 541–554. doi:10.1038/s41576-020-0241-0
Wilkinson, A. C., Ishida, R., Kikuchi, M., Sudo, K., Morita, M., Crisostomo, R. V., et al. (2019). Long-term Ex Vivo Haematopoietic-Stem-Cell Expansion Allows Nonconditioned Transplantation. Nature 571 (7763), 117–121. doi:10.1038/s41586-019-1244-x
Willems, P. H. G. M., Rossignol, R., Dieteren, C. E. J., Murphy, M. P., and Koopman, W. J. H. (2015). Redox Homeostasis and Mitochondrial Dynamics. Cel Metab. 22 (2), 207–218. doi:10.1016/j.cmet.2015.06.006
Wilson, A., Laurenti, E., Oser, G., van der Wath, R. C., Blanco-Bose, W., Jaworski, M., et al. (2008). Hematopoietic Stem Cells Reversibly Switch from Dormancy to Self-Renewal during Homeostasis and Repair. Cell 135 (6), 1118–1129. doi:10.1016/j.cell.2008.10.048
Wilson, N. K., Kent, D. G., Buettner, F., Shehata, M., Macaulay, I. C., Calero-Nieto, F. J., et al. (2015). Combined Single-Cell Functional and Gene Expression Analysis Resolves Heterogeneity within Stem Cell Populations. Cell stem cell 16 (6), 712–724. doi:10.1016/j.stem.2015.04.004
Wu, J. Q., Seay, M., Schulz, V. P., Hariharan, M., Tuck, D., Lian, J., et al. (2012). Tcf7 Is an Important Regulator of the Switch of Self-Renewal and Differentiation in a Multipotential Hematopoietic Cell Line. Plos Genet. 8 (3), e1002565. doi:10.1371/journal.pgen.1002565
Xavier-Ferrucio, J., and Krause, D. S. (2018). Concise Review: Bipotent Megakaryocytic-Erythroid Progenitors: Concepts and Controversies. Stem cells 36 (8), 1138–1145. doi:10.1002/stem.2834
Xu, B., Cai, L., Butler, J. M., Chen, D., Lu, X., Allison, D. F., et al. (2018). The Chromatin Remodeler BPTF Activates a Stemness Gene-Expression Program Essential for the Maintenance of Adult Hematopoietic Stem Cells. Stem Cel. Rep. 10 (3), 675–683. doi:10.1016/j.stemcr.2018.01.020
Xu, G., Wu, H., Zhang, J., Li, D., Wang, Y., Wang, Y., et al. (2015). Metformin Ameliorates Ionizing Irradiation-Induced Long-Term Hematopoietic Stem Cell Injury in Mice. Free Radic. Biol. Med. 87, 15–25. doi:10.1016/j.freeradbiomed.2015.05.045
Yalcin, S., Zhang, X., Luciano, J. P., Mungamuri, S. K., Marinkovic, D., Vercherat, C., et al. (2008). Foxo3 Is Essential for the Regulation of Ataxia Telangiectasia Mutated and Oxidative Stress-Mediated Homeostasis of Hematopoietic Stem Cells. J. Biol. Chem. 283 (37), 25692–25705. doi:10.1074/jbc.m800517200
Yang, L., Bryder, D., Adolfsson, J., Nygren, J., Månsson, R., Sigvardsson, M., et al. (2005). Identification of Lin-Sca1+kit+CD34+Flt3- Short-Term Hematopoietic Stem Cells Capable of Rapidly Reconstituting and Rescuing Myeloablated Transplant Recipients. Blood 105 (7), 2717–2723. doi:10.1182/blood-2004-06-2159
Yoshida, T., and Georgopoulos, K. (2013). GATA-3 Controls Self-Renewal in Stressed HSCs. Nat. Immunol. 14 (10), 1032–1033. doi:10.1038/ni.2715
Yuasa, H., Oike, Y., Iwama, A., Nishikata, I., Sugiyama, D., Perkins, A., et al. (2005). Oncogenic Transcription Factor Evi1 Regulates Hematopoietic Stem Cell Proliferation through GATA-2 Expression. Embo J. 24 (11), 1976–1987. doi:10.1038/sj.emboj.7600679
Zeng, H., Yücel, R., Kosan, C., Klein-Hitpass, L., and Möröy, T. (2004). Transcription Factor Gfi1 Regulates Self-Renewal and Engraftment of Hematopoietic Stem Cells. Embo J. 23 (20), 4116–4125. doi:10.1038/sj.emboj.7600419
Zgurskaya, H. I., and Rybenkov, V. V. (2020). Permeability Barriers of Gram‐negative Pathogens. Ann. N.Y. Acad. Sci. 1459 (1), 5–18. doi:10.1111/nyas.14134
Zhang, C. C., and Lodish, H. F. (2008). Cytokines Regulating Hematopoietic Stem Cell Function. Curr. Opin. Hematol. 15 (4), 307–311. doi:10.1097/moh.0b013e3283007db5
Zhang, D., Gao, X., Li, H., Borger, D. K., Wei, Q., Yang, E., et al. (2022). The Microbiota Regulates Hematopoietic Stem Cell Fate Decisions by Controlling Iron Availability in Bone Marrow. Cell Stem Cell. doi:10.1016/j.stem.2021.12.009
Zhang, H., Menzies, K. J., and Auwerx, J. (2018). The Role of Mitochondria in Stem Cell Fate and Aging. Development 145. doi:10.1242/dev.143420
Zhang, H., Zhai, Z., Wang, Y., Zhang, J., Wu, H., Wang, Y., et al. (2013). Resveratrol Ameliorates Ionizing Irradiation-Induced Long-Term Hematopoietic Stem Cell Injury in Mice. Free Radic. Biol. Med. 54, 40–50. doi:10.1016/j.freeradbiomed.2012.10.530
Zhao, M., Perry, J. M., Marshall, H., Venkatraman, A., Qian, P., He, X. C., et al. (2014). Megakaryocytes Maintain Homeostatic Quiescence and Promote post-injury Regeneration of Hematopoietic Stem Cells. Nat. Med. 20 (11), 1321–1326. doi:10.1038/nm.3706
Zhu, J., and Emerson, S. G. (2002). Hematopoietic Cytokines, Transcription Factors and Lineage Commitment. Oncogene 21 (21), 3295–3313. doi:10.1038/sj.onc.1205318
Glossary
AhR Aryl Hydrocarbon Receptor
AML Acute Myeloid Leukemia
B-ALL B-cell Acute Lymphoblastic Leukemia
BM Bone Marrow
CLL Chronic Lymphocytic Leukemia
CLPs Common Lymphoid Progenitors
CMPs Common Myeloid Progenitors
CSCs Cancer Stem Cells
EPCR Endothelial Protein C Receptor
EPO Erythropoietin
ESCs Embryonic Stem Cells
EVs Extracellular Vesicles
FL Follicular Lymphoma
G-CSF Granulocyte Colony Stimulating Factor
GMPs Granulocyte-Macrophage Progenitors
GvHD Graft-Versus-Host Disease
HPCs Hematopoietic Progenitor Cells
HSCs Hematopoietic stem cells
IL Interleukin
IR Ionizing Radiation
LT-HSCs Long-term HSCs
MDS Myelodysplastic Syndrome
MEPs Megakaryocyte-Erythrocyte Progenitors
MPN Myeloproliferative Neoplasm
MPPs Multipotent Progenitors
MSCs Mesenchymal Stem Cells
NK Natural Killer
NOX NAPDH Oxidase
OXPHOS Oxidative Phosphorylation
PPAR Proliferator-Activated Receptor
RA Retinoic Acid
ROS Reactive Oxygen Species
scATAC-seq Single-Cell Assay For Transposase-Accessible Chromatin Using Sequencing
SCF Stem Cell Factor
scRNA-seq Single-Cell RNA Sequencing
SLAM Signaling Lymphocyte Activation Molecule
SR1 Stem-Reginin1
ST-HSCs Short-term HSCs
T-ALL T-cell Acute Lymphoblastic Leukemia
TFs Transcription Factors
TPO Thrombopoietin
UCB-CD34+ Umbilical Cord Blood Derived HSCs
VA Valproic Acid
Keywords: Hematopoietic Stem Cells (HSCs), hematopoiesis, self-renewal, differentiation, transcription factors, extracellular vesicles (EVs), Graft-versus-Host Disease (GvHD), miRNAs
Citation: Mann Z, Sengar M, Verma YK, Rajalingam R and Raghav PK (2022) Hematopoietic Stem Cell Factors: Their Functional Role in Self-Renewal and Clinical Aspects. Front. Cell Dev. Biol. 10:664261. doi: 10.3389/fcell.2022.664261
Received: 04 February 2021; Accepted: 14 February 2022;
Published: 24 March 2022.
Edited by:
Anindita Basak, Harvard Medical School, United StatesReviewed by:
Lijian Shao, University of Illinois at Chicago, United StatesMeng Zhao, Sun Yat-sen University, China
Toshio Suda, National University of Singapore, Singapore
Copyright © 2022 Mann, Sengar, Verma, Rajalingam and Raghav. This is an open-access article distributed under the terms of the Creative Commons Attribution License (CC BY). The use, distribution or reproduction in other forums is permitted, provided the original author(s) and the copyright owner(s) are credited and that the original publication in this journal is cited, in accordance with accepted academic practice. No use, distribution or reproduction is permitted which does not comply with these terms.
*Correspondence: Pawan Kumar Raghav, cHducmdodkBnbWFpbC5jb20=, UGF3YW4uUmFnaGF2QHVjc2YuZWR1
†ORCID: Zoya Mann, orcid.org/0000-0003-4210-3801; Raja Rajalingam, orcid.org/0000-0001-8821-7877; Pawan Kumar Raghav, orcid.org/0000-0002-2440-7134
‡These authors have contributed equally to this work