- 1Department of Oral Medicine, School of Basic Medical Sciences, Capital Medical University, Beijing, China
- 2Department of Physiology and Pathophysiology, School of Basic Medical Sciences, Capital Medical University, Beijing, China
- 3Undergraduate Student of 2018 Eight Program of Clinical Medicine, Peking University People’s Hospital, Beijing, China
- 4Department of Clinical Medicine, School of Basic Medical Sciences, Capital Medical University, Beijing, China
- 5Department of Laboratory Animal Research, Xuan Wu Hospital, Capital Medical University, Beijing, China
The intestine is a powerful digestive system and one of the most sophisticated immunological organs. Evidence shows that tuft cells (TCs), a kind of epithelial cell with distinct morphological characteristics, play a significant role in various physiological processes. TCs can be broadly categorized into different subtypes depending on different molecular criteria. In this review, we discuss its biological properties and role in maintaining homeostasis in the gastrointestinal tract. We also emphasize its relevance to the immune system and highlight its powerful influence on intestinal diseases, including inflammations and tumors. In addition, we provide fresh insights into future clinical diagnostic and therapeutic strategies related to TCs.
1 Introduction
TCs within the rat trachea and the gastric wall were originally discovered in the 1950s (Rhodin and Dalhamn, 1956; Jarvi and Keyrilainen, 1956). Because TCs have apical brush borders, researchers may identify them based on their distinct morphological traits. As intestinal TCs were detected above the Paneth cells at the crypt (the +4 position), they were assumed to be reserved stem cells (May et al., 2008; Dekaney et al., 2009; May et al., 2009; DelGiorno et al., 2020). Due to similarities between TCs and enteroendocrine cells, it was commonly considered that TCs were a subset of enteroendocrine cells. Recent investigations, however, have revealed that TCs are post-mitotic and short-lived, implying TCs represent a distinct secretory intestinal epithelial cell (IEC) lineage different from enteroendocrine, Paneth, goblet cells (GCs), and enterocytes (Gerbe et al., 2009). Further information on post-mitotic cells is depicted in Box 1 (Gerbe et al., 2012). Their distinct requirements for biomarkers and the transcription factor testified to their uniqueness. Although rarely discussed, there are multiple TC subtypes with distinct traits and roles. The functions of this unusual cell type, however, have yet to be properly examined. As a secretory epithelial cell lineage, TCs can secrete multiple molecules that are required for the type 2 immune response against helminth and bacterial infection.
Inflammations or neoplasms may result from TC malfunction in the digestive system. The identification of one of the TCs markers in CSC raises the hypothesis that aberrant TC proliferation is linked to intestinal neoplasms, and more study is needed to validate the underlying mechanism to treat these diseases. As a result, the goal of this review is to provide a comprehensive assessment of the characteristics and functions of TCs in the digestive tract, as well as a novel strategy for future clinical practice of intestinal inflammations and tumors.
2 History of TCs discovery
Independent investigations have documented the existence of TCs in various hollow organs since the first identification in the mouse gastrointestinal tract (Jarvi and Keyrilainen, 1956) and rat trachea (Rhodin and Dalhamn, 1956) in the 1950s. Since then, TCs have been found in several organs of various species (Jarvi et al., 1967; Luciano et al., 1968; Meyrick and Reid, 1968; Luciano et al., 1969; Chang et al., 1986; Hofer and Drenckhahn, 1992), including taste buds, pancreas, submandibular glands, and efferent ductules of testis (Jeffery, 1983; Sato and Miyoshi, 1988; Hofer and Drenckhahn, 1992; Hofer et al., 1996). (as Table 1 shown the TCs discovery milestones).
TCs have been discovered in human airways in pathologic conditions but not among healthy people (Gordon and Kattan, 1984; Cerezo and Price, 1985; DiMaio et al., 1988).
3 Morphology of TCs
Early studies in rodent models revealed that TCs possess brush boundaries formed by distinct apical bristles (Silva, 1966; Luciano and Reale, 1979; Luciano and Reale, 1997). Actin filaments sustain the microvilli, which could be visualized by phalloidin (Hofer and Drenckhahn, 1998). Since the identification of this unique cell type, researchers have given it names such as “fibrillovesicular” “peculiar” “caveolated” “brush” and “tuft”. In 2005, the term “tuft” was proposed as a moniker for this cell lineage (Reid et al., 2005). The overall morphology of TCs varies among hollow organs (Luciano and Reale, 1979; Sato, 2007), and the intestinal TC body is fashioned like a cylinder with thinner basal and apical ends (Meyrick and Reid, 1968). Although TCs in various organs have different functions, most researchers believe they belong to the same cell type.
Furthermore, using ATUM, SBF, and SEM, Hoover et al. (2017) discovered a novel tubulovesicular system in TCs. Volume rendering revealed a sophisticated network of tubules connecting the microvilli to the rough endoplasmic reticulum in TCs from the gastrointestinal tract. The tubular network may facilitate molecular interaction between TCs and the intestinal lumen or adjacent cell nuclei (Herring et al., 2018). However, unlike TCs in the alimentary tract, those in the respiratory tract lack a tubular network.
Aside from the well-known brush border, Luciano et al. discorvered lateral projections formed by basolateral membrane and microvilli in TCs. Although the protrusions might extend to neighboring cells and connect to their nuclei, transmission electron microscopy failed to corroborate the details (Luciano and Reale, 1979; Luciano and Reale, 1990). Hoover et al. (2017) revealed the nanostructure of TCs using ATUM, SBF, and SEM and dubbed the protrusions “cytospinules.” Every TC has three or four cytospinules in direct contact with the nuclear membranes of neighboring cells. Since the specific function of cytospinules has been vague, it is speculated that this direct cell-to-cell interaction might play a substantial role in intercellular comminucation (Figure 1 depicts structure and composition patterns of intestinal TCs). Moreover, although secretory cells are scarcely distributed in the intestinal epithelium, TCs may exist close to other secretory cells. It is now hypothesized that GCs receive cytokine signals when secreting mucus and expelling helminths during the “weep and sweep” process. If a TC is adjacent to a GC, would it generate a direct signal to rapidly promote GC’s role in “weep and sweep”? The detailed signaling pathway has yet to be demonstrated.
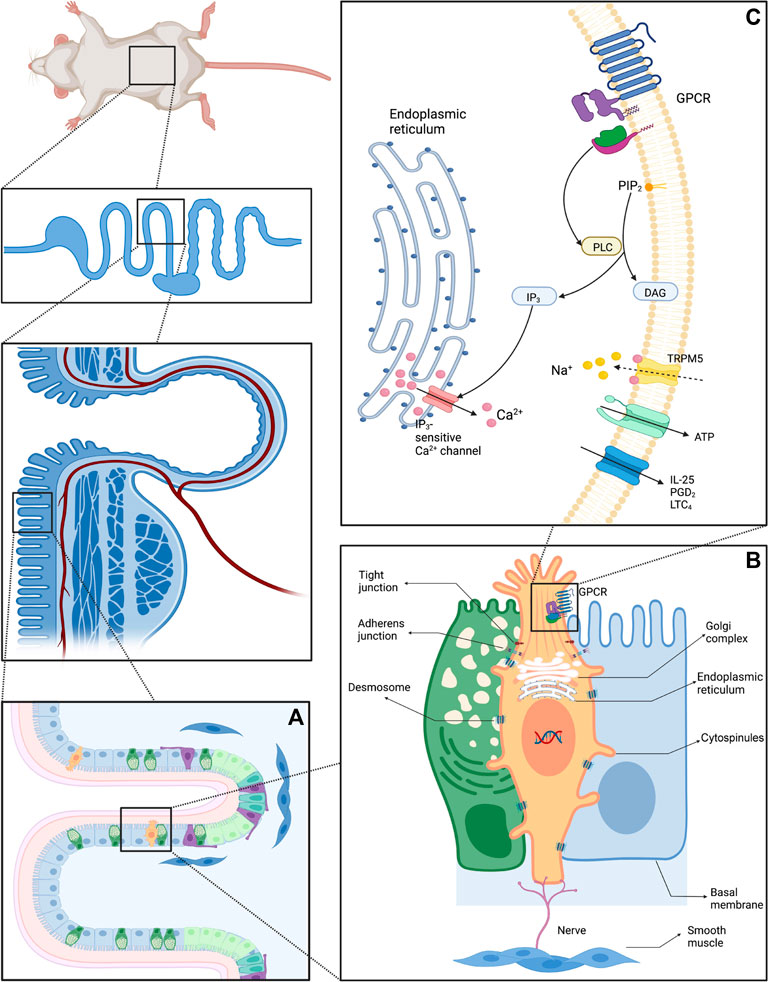
FIGURE 1. Diagram of the structure and composition patterns of IECs (A) Schematic diagram of Lgr5+ stem cells and differentiated progeny of the crypt-villus (B) Structure of intestinal TCs and adjacent enterocytes: Intestinal TCs possess distinguish morphological characteristics, especially the unique brush border. The cytospinules can directly contact the neighboring cells, serving as a bridge between the extracellular and intracellular environment. The tubular network within TCs can transport cargo via vesicles. (C) Schematic diagram of the vicinity of the TC cell membrane.
4 Intestinal TCs originate from Lgr5+ stem cells
TCs account for around 0.4% of the IECs in the murine alimentary tract (McKinley et al., 2017). TCs, in contrast to GCs, decrease progressively from the jejunum to the colon, peaking in the proximal small intestine, according to earlier research. Because the small intestine performs an important absorptive role and contains the majority of the TCs, it may be assumed that TCs are associated with intestinal absorption (Cheng et al., 2018). The differentiated TCs first appear around the 7 postnatal day and can be promptly detected a week later, relying on self-renewal stem cells.
Tsubouchi and Leblond’s experiment in the 1970s offered a vital insight into the TCs progenitors. The label first developed in the enteroendocrine cells at the base of the crypts after 3H-thymidine infusion began, and then traveled to the “+4 position,” where the TCs resided (Tsubouchi and Leblond, 1979). Genetic tracing experiments using Cre-activable Rosa26-LacZ reporter mouse and the Lgr5EGFP−IRES-CreERT2 mouse have demonstrated that Lgr5+ crypt basal columnar (CBC) cells can self-renew and differentiate into diverse cell lineages over time, implying that Lgr5+ CBC cells possess characteristics of stem cells and that TCs are generated from Lgr5+ stem cells (Gerbe et al., 2011). Yui et al. (2012) further testified the cellular origin of TCs by cultivating organoids derived from single Lgr5-EGFP cells.
It is hypothesized that intestinal stem cells (ISCs) dwell in the crypt and are continually feed differentiated progeny from the crypt to the villi. Although most differentiated daughter cells are phased out after a short existence of 3–5 days, long-lived ISCs can self-renew. Initially, cells with preserved labels at the +4 position of the crypt were thought to represent ISCs (Rea et al., 1975). Recent lineage tracing investigations, however, have discovered that CBCs positioned at locations +1 to +3 in the crypt are quickly cycling, self-renewing ISCs (Barker et al., 2007).
Although we have long assumed that the differentiation of stem cells is irreversible based on our comprehension of the hematopoietic system, a series of studies have shown that mature intestinal epithelial cells (IECs) show a strong capacity for retro-differentiation, indicating that IECs have more remarkable plasticity (de Sousa and de Sauvage, 2019). A more dynamic model, rather than the traditional view of the stratified organization of the gut, is thus better suited to explain this phenomenon, in which various differentiated cells within the intestinal epithelium can dedifferentiate and function as an alternative source of stem cells in inflammation and tumorigenesis. IECs differentiation is regulated by the interaction of growth and developmental stimuli, metabolites, and signaling pathways such as Notch, EGF, BMP, and Hippo signaling.
5 TCs qualify as a distinct secretory epithelial cell
DCLK1+ cells have long been considered to be quiescent stem cells (Giannakis et al., 2006; May et al., 2008; Dekaney et al., 2009; Jin et al., 2009; Sureban et al., 2009) and a subset of enteroendocrine cells (Formeister et al., 2009; Kokrashvili et al., 2009). However, with further studies of TCs’ characteristics, it has been confirmed that DCLK1+ TCs are a particular type of IECs (Gerbe et al., 2011).
5.1 Classification of differentiated IECs
The small intestine contains crypt-villus units that repeat. The pioneering experiments of Barker et al. (2007) demonstrated that Lgr5+ CBC stem cells are the progenitors of a variety of epithelial cells, which inhabit the base of the crypt and are intercalated between Paneth cells. Lgr5+ CBCs rapidly create transit-amplifying (TA) progenitor cells that move upwards and completely develop before entering the crypt (Barker et al., 2007). Based on morphology and expression features, differentiated epithelial cells may be generally separated into two types: secretory cells and absorptive cells (Flier and Clevers, 2009). Although as many as seven lineages of cells have been described in the intestinal epithelium, including cup cells and “membranous” (M) cells (Madara, 1982; Neutra, 1998), only five of which are usually considered. (Figure 2 depicts an IEC differentiation diagram).
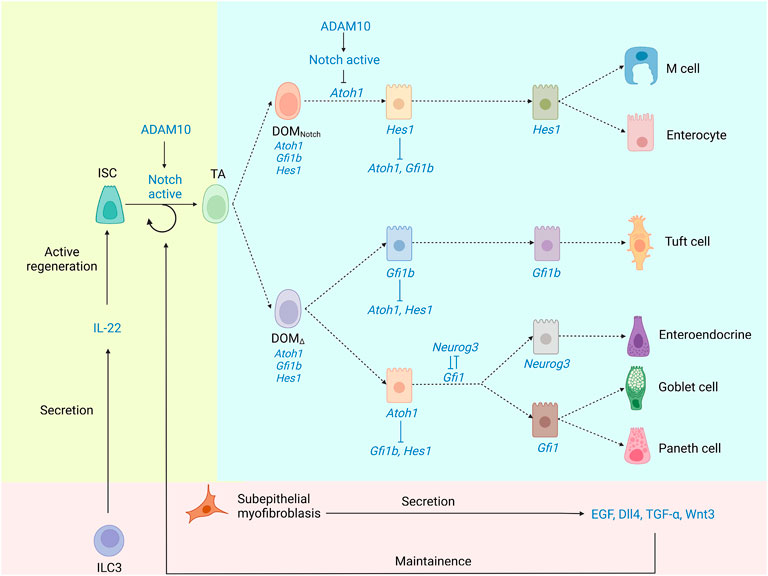
FIGURE 2. The underlying mechanism of IEC differentiation. Lgr5+ CBCs generate TA progenitor cells, which then differentiate into a spectrum of different absorptive and secretory cell lineages. Notch signaling is crucial to the maintenance of ISCs and the differentiation of TA. ADAM10, as an α-secretase, promotes Notch signaling. Atoh1 is necessary for stem cells to differentiate into secretory cells, while Hes1 acts to repress secretory cells. There is reciprocal repression between Hes1 and Atoh1. TCs are produced from Gfi1b-expressing progenitors. Progenitors differentiate into three distinct cell fates through the guidance of three characteristic transcription factors, Hes1, Atoh1, and Gfi1b. Although TC is not Atoh1-dependent, TCs are hypothesized to derive from secretory progenitors.
Absorptive epithelial cells comprise the majority of differentiated epithelial cells, while secretory cells account for only 1%. Absorptive IECs play various roles in digestion, nutrition absorption, and mucosal defense. Secretory IECs are in charge of secreting antimicrobial peptides and growth factors, as well as the controlling the gut flora and surrounding stem cells (Gerbe et al., 2011).
5.2 Using transcription factors to identify TC from other IECs
Previous research has emphasized the transcriptional start sequence, the participation of particular transcription factors, and epigenetic modification. It is assumed that a multitude of mechanisms is involved in IEC differentiation, however, it is disputed whether transcriptional modulation is involved.
5.2.1 Lateral inhibitory notch signaling in IEC fate decisions
The Notch pathway is one of the critical signaling pathways in maintaining the balance of epithelial cell proliferation and differentiation (Kimble and Simpson, 1997; Shen et al., 2004), which is best known for specifying different cell fates of neighboring cells via an evolutionarily conserved process of “lateral inhibition” (Chitnis, 1995).
5.2.1.1 The contribution of notch signaling in intestinal homeostasis and cell fate decision
Notch signaling is crucial to the maintenance of ISCs and the differentiation of TA progenitors. To maintain the stem cell pool, the Notch signaling pathway operates directly on intestinal stem cells. It also regulates the differentiation of the secretory and absorptive cells through “lateral inhibition” (Sancho et al., 2015).
Notch “active” TA progenitors are destined to be absorptive progenitors, in which Notch targets the Hes/Hey transcription factors, repressing the expression of Atoh1 and Dll-1/4 ligand (Akazawa et al., 1995; Jensen et al., 2000; Yang et al., 2001). These cells would ultimately differentiate into post-mitotic enterocytes after several rounds of proliferation. Notch “low” TA progenitors are destined to be secretory progenitors, in which low Notch activity disinhibits the expression of Atoh1 and Dll-1/4 ligand. These cells would rapidly differentiate into distinct secretory cell types (Jensen et al., 2000; Yang et al., 2001; Bjerknes and Cheng, 2005). In summary, using lateral inhibition, Notch promotes differentiation towards the absorptive lineage, whereas the Notch-low state permits differentiation towards the secretory cell lineage.
5.2.1.2 ADAM10 regulates notch signaling
As a family member of Sultidomain, a Disintegrin and Metalloproteinases (ADAMs) are involved in signal transduction processes that regulate cell migration and adhesion proteolysis (Hartmann et al., 2002). Analysis has demonstrated that ADAM10 is an α-secretase that promotes Notch signaling. The systemic Adam10-deficient mice embryos die at E 9.5 due to defective in somatic cell development, angiogenesis, and neurogenesis, similar to those of the Notch-defective mice (Hartmann et al., 2002).
5.2.1.3 Notch-signaling driven ternary switching and Gfi1b-expressing progenitors
Secretory cells are more common in Hes1-deficient epithelial cells than absorptive cells, according to Bjerknes et al. (Jensen et al., 2000), suggesting that Hes1 represses secretory cells, potentially via suppressing the expression of Atoh1 (Akazawa et al., 1995; Jensen et al., 2000; Yang et al., 2001). Therefore, the reciprocal inhibition between Hes1 and Atoh1 would lead DOM (daughters of TA progenitors/daughters of Mix) to pass through a binary switch via the Notch signaling (Fortini, 2009). (Box 2 depicts alternate nomenclature for cells in the differentiation process as well as their relationship).
Unfortunately, this model does not include TCs. Contrary to Gerbe et al., Bjerknes et al. discovered that conditional Atoh1 deletion dramatically increases TC populations, suggesting that the differentiation and survival of TCs are independent of Atoh1, but Atoh1 may be transiently expressed in TA cells before lineage commitment (Bjerknes et al., 2012). TCs are derived from progenitors that express Gfi1b. These findings support a model in which progenitors develop into three discrete cell fates under the control of three separate transcription factors, Atoh1, Hes1, and Gfi1b. Notch signaling leads Hes1 to dominate one of the two major differentiated cell lineages, producing absorptive cells. Correspondingly, Atoh1 or Gfi1b dominate the other major cell lineage, producing secretory cells or TCs, resulting in a ternary switch for cell fate determination (Bjerknes et al., 2012). The schematic diagram of the ternary switch is shown in Figure 2. TCs require another transcription factor to differentiate than enteroendocrine, GCs, and Paneth cells; non-etheless, Bjerknes et al. argue that these four lineages share many properties and also hypothesized that the secretory progenitor gives birth to the TC lineage.
To conclude, Atoh1, Hes1, and Gfi1b are components of a genetic network that forms a ternary switch in the TCs via Notch signaling.
5.2.1.4 The debatable regulation of TCs by ATOH1
Although the significance of ATOH1 in regulating enteroendocrine, Paneth, and goblet cells is well established (Yang et al., 2001), how ATOH1 regulates TCs remains controversial (Gerbe et al., 2011; Gracz et al., 2018). Recent cell lineage tracing investigations have revealed that ATOH1+ cells contain stem cell characteristics and facilitate epithelial regeneration following damage (Ishibashi et al., 2018; Tomic et al., 2018). Moreover, the Atoh1−/− mouse model published by Banerjee et al. showed that although colonic TCs depend on ATOH1, TC expansion can be observed in small intestinal in AtohKO mice, contradicting the conclusion that TCs are dependent on ATOH1 found in a prior work by Gerbe et al. (2011). It was inferred that a subset of small intestinal TCs may be independent of ATOH1 and follow a distinct path of development (Herring et al., 2018). Banerjee et al. demonstrate that ATOH1-independent TCs expand through a metabolic communication network during luminal microbiota perturbations, a specific mechanism that could be used to suppress inflammation and repair the epithelial damage caused by Crohn’s disease (CD) (Banerjee et al., 2020).
5.2.2 Other transcription factors involved in the TC differentiation
Notch regulates the cell fate decisions of TA progenitors by influencing the essential transcription factor Atoh1 (Fre et al., 2009; VanDussen et al., 2012). These events are coordinated by Notch1/2 receptors and DLL1/4 (Riccio et al., 2008; Pellegrinet et al., 2011; Carulli et al., 2015). Atoh1 target genes, such as the SAM pointed domain containing Ets transcription factor (Spdef) genes, Kruppel-like factor 4 (Klf4), SRY-box containing gene 9 (Sox9), Neurog3, and growth factor-independent 1 (Gfi1), are responsible for secretory cell type specification. Although evidence shows that Paneth cells and GCs have a common ancestor, it is unclear how multipotent secretory progenitor cells form particular secretory cell types (Barker, 2014; Sancho et al., 2015). Wnt signaling facilitates Paneth cell development by directly activating lineage-specific transcription factors and differentiation genes such as defensins (van Es and Clevers, 2005; van Es et al., 2005; Farin et al., 2012; Kim et al., 2012; San Roman et al., 2014). However, since this Wnt signaling pathway was suppressed, the Lgr5+ CBC stem cells could not be observed (Korinek et al., 1998; Pinto et al., 2003).
Therefore, Notch and Wnt activity must always be in balance with each other to ensure the survival of intestinal stem cells, the proper development of all types of epithelial cells, and the maintenance of crypt stability and intestinal function. Sox9, a Wnt signaling target, is expressed in crypt epithelial cells. TCs occur in Sox9-deficient intestinal epithelium due to adequate Sox9 expression inside differentiated TC (Bastide et al., 2007; Mori-Akiyama et al., 2007). Growth factor-independent 1b (Gfi1b) is expressed at greater levels among Trpm5-expressing TCs, according to a transcriptome comparison (Bezencon et al., 2008). The growth factor-independent 1 (Gfi1) is detected in goblet and Paneth cells (Bjerknes and Cheng, 2010), where it may block the transcription factor of the enteroendocrine cells (Neurog3) (Jenny et al., 2002; Mellitzer et al., 2010). TCs are also dependent for their development on the transcription factor Pou2f3. Pou2f3−/− mice lack intestinal TCs and have defective mucosal type 2 responses to helminth infection (Gerbe et al., 2016). The detailed requirements are listed in Table 2.
5.3 Biomarkers of TCs
The lack of identifiable biomarkers has hampered the study of TCs since their discovery in the 1950s. Identifying more viable unambiguous, and specific markers has enhanced the research situation, allowing for a more detailed examination of TCs. With updated biomarker information, far more complete research is expected.
5.3.1 Ambiguous markers of TCs
Cytokeratin 18 (Hofer and Drenckhahn, 1996), Ulex europaeus lectin 1 (Gebhard and Gebert, 1999; Gebert et al., 2000), neuronal nitric oxide synthase (Kugler et al., 1994), Villin, and fimbrin (Hofer and Drenckhahn, 1992), are either expressed ubiquitously in the intestinal epithelium, or also expressed in TCs within limited areas. (Kugler et al., 1994; Gebert et al., 2000; Jang et al., 2007; Sutherland et al., 2007; Bezencon et al., 2008; Kokrashvili et al., 2009). Taste-related biomarkers such as α-gustducin, β-endorphin, uroguanylin, and Met-Enkephalin (Perez et al., 2002; Bezencon et al., 2007; Kaske et al., 2007) are expressed within TCs. They may engage in the taste transduction (Hofer et al., 1996; Hofer and Drenckhahn, 1998). However, the exact relationship between these biomarkers and TC is not elucidated, so these markers are considered to be TCs non-specific. Given that TCs secrete such a wide variety of proteins, the complexity of TC’s functions may be far beyond our imagination. Therefore, the study of TC function is very promising and may provide us with enlightenment in many aspects.
5.3.2 Specific markers of TCs
Although TRPM5 (transient receptor potential cation channel, subfamily M, member 5) is expressed by enteroendocrine cells (Bezencon et al., 2007), it is one of the best markers for TCs, since Trmp5-expressing IECs are primarily TCs (Kaske et al., 2007) and the detailed depiction of TRPM5 is shown in Box 3.
All TCs, characterised by DCLK1 and Growth factor independent 1b (GFI1b) expression also co-expressed the Pou domain, class 2, transcription factor 3 (POU2F3) (Bjerknes et al., 2012; Gerbe and Jay, 2016). TCs also express the cyclooxygenase 1 (COX1) and cyclooxygenase 2 (COX2) enzymes (Bezencon et al., 2008). May et al. (2014) discovered that DCLK1−/− mice had altered gene expression profiles of growth and functions in TCs, proving the relevance of DCLK1 in TCs. DCLK1+ cells were formerly assumed to represent latent intestinal epithelial stem cells (Giannakis et al., 2006; May et al., 2008; Dekaney et al., 2009; Jin et al., 2009; May et al., 2009; Sureban et al., 2009). However, it was later proven to be untrue. Gerbe et al. discovered that DCLK1+ cells were distributed throughout the adult mouse’s intestinal epithelium, but only 21% of the TCs were identified in the crypt (Gerbe et al., 2009). This evidence revealed that the DCLK1+cells do not belong to stem cells. DCLK1 did not co-stain with any known markers of Paneth cells, enterocytes, GCs, or enteroendocrine cells, above which gives direct proof that the DCLK1 represented a gene signature of the intestinal TCs in mice (Bezencon et al., 2008). A 2019 study showed that DCLK1 is connected to the activation status of TCs. Still, it was not engaged in TCs growth (Yi et al., 2019), which was related to the response to intestinal epithelial damage (May et al., 2014; Westphalen et al., 2014; Qu et al., 2015). Gerbe et al. validated DCLK1 as a particular marker of post-mitotic TCs in the mouse intestinal epithelium based on repeated co-staining experiments and micro-array data (Bezencon et al., 2008; Gerbe et al., 2009). The data indicate that DCKL1+ intestinal cells are TCs rather than long-lived quiescent stem cells. Special attention should be paid to SUCNR1 expressed exclusively in mice, confirming Sucnr1 as a TC gene signature (Lei et al., 2018).
Cells expressing DCLK1, hematopoietic prostaglandin-D synthase (HPGDS), COX1, COX2, and SOX9 have more significant immunoreactivity to F-actin, -tubulin, and villin. These properties resemble the typical TC trait (May et al., 2014). The evidence presented above shows that DCLK1, HPGDS, COX1, COX2, and SOX9 coexpression is confined to TCs in the epithelium.
To summarize, whereas enterocytes, Paneth cells, enteroendocrine cells, and GCs are derived from Lgr5+ CDC stem cells, TCs constitute a unique cell lineage with distinct transcription factor requirements and biomarkers. Apart from Atoh1, TC differentiation is unaffected by other transcription factors in other epithelial cells. There are no shared markers between TCs and other epithelial cells.
6 Subtypes of TCs
Immunostaining of combination markers and morphological inspection are now widely employed to identify TCs; nevertheless, minor changes between TC subtypes cannot be detected with this approach. We do not yet know all of the indicators that can distinguish cell subtypes in the gut. We analyzed multiple standards for classifying TC subsets using various criteria that may aid future studies.
6.1 DCLK1/5HT-IR cells represent a novel subtype of TCs
According to double immunostaining evidence, DCLK1/5HT-IR cells contain serotonin (5HT) and are a novel subtype of DCLK1-immunoreactive (IR) TCs. These cells shrank distally from the small to the large intestine. 5-HT has a wide range of biological roles, including cognition, learning, memory, emotional control and vasoconstriction (Young, 2007). Approximately 90% of the serotonin in the human body is located in the enterochromaffin cells of the GI tract, where it also involves in the accommodation of gut homeostasis (Berger et al., 2009). In a word, DCLK1/5HT-IR cells, as a non-negligible neo-subtype of TCs, may contribute to the intestinal physiological function (Cheng et al., 2019).
6.2 Tuft 1 and Tuft 2
Transcriptome analysis revealed two additional TC subgroups: neuronal TCs (tuft 1) and immunological TCs (tuft 2). Despite the fact that DCLK1 and IL-25 are expressed by both TC subtypes, their roles are distinct (Haber et al., 2017). Tuft 1 has higher levels of neuronal gene expression profile, including Ninj1, Nrep, and Nradd. Immunological genes, such as those encoding CD45 and thymic stromal lymphopoietin (TSLP), were expressed at higher levels in tuft 2 (Haber et al., 2017). When parasite infections occur, tuft 2 outnumbers tuft 1 to form the majority of mouse gastrointestinal TCs (Haber et al., 2017).
According to a 2020 research, there may be another subtype of TC that mimics intestinal endocrine cells following the treatment of scopolamine (Middelhoff et al., 2020). The properties and functions of this novel subtype TCs need to be investigated urgently.
6.3 ATOH1-dependent and ATOH1-independent TCs
Banerjee et al. (2020) identified heterogeneous TC populations that respectively undergo ATOH1-dependent and ATOH1-independent pathways. Both ATOH1-independent and dependent TCs can be observed in the small intestine, but only ATOH1-dependent TCs can be observed in the colon. Banerjee et al. also found that ATOH1-independent TCs are a flexible cell population that can expand in the presence of luminal perturbations, whereas the ATOH1-dependent cell population is constant. Specifically, succinate drives ATOH1-dependent TC gene expression and growth in symbiotic bacteria (Stumhofer et al., 2006; Langille et al., 2013).
7 Functions of TCs
Chemosensory cells are used by most organs to respond to changes and maintain homeostasis. TCs are responsible for chemoreception and secretion, which includes sensing and processing chemical signals as well as mending the epithelium (Chandrakesan et al., 2016).
7.1 Biologically active molecules released from TCs
Previous research has revealed that TCs release various chemicals, including NO, leukotrienes, IL-25, opioids, fatty acid metabolism-related proteins, and components of the eicosanoid pathway. These molecular secretions demonstrate that TCs may perform a variety of roles in the digestive tract, as summarized in Table 3. These secretion-related activities might provide deeper insight into inflammation and tumor-related pathways. Box 4 depicts the function of TCs in various organs or tissues.
7.2 Chemoreception
Changes in nutrition, pH, and microbiota can be detected by TCs, which are found in the airway and digestive tract. Because of their physical similarities to lingual taste bud cells, TCs were assumed to have a role in chemoreception. Members of the pancreatic and intestinal taste transduction pathways support this theory (Hofer et al., 1996; Hofer and Drenckhahn, 1998). TCs express several signaling molecules, including α-gustducin (also known as the guanine nucleotide binding protein alpha transducing 3, or GNAT3) (Hofer and Drenckhahn, 1998), TRPM5 (Kaske et al., 2007), G protein-coupled taste receptor type 1 member 3 (TAS1R3), the calcium signal transducer phospholipase Cβ2 (PLCβ2) (Ogura et al., 2010), β-endorphin, uroguanylin, and Met-Enkephalin, (Perez et al., 2002; Bezencon et al., 2007; Kaske et al., 2007). According to some study, TCs are a component of the diffuse chemosensory system (Sbarbati and Osculati, 2005). Furthermore, succinate receptor 1 (SUCNR1) was found to be expressed in TCs, and Lei et al. (2018) identified SUCNR1 as a TC-specific marker in mice, suggesting that SUCNR1 might aid in detecting infectious pathogens, triggering the proliferation of TCs and GCs involving in type 2 immune response.
7.3 Tuft-ILC2 circuit mediated helminth expulsion pathway
Helminth infection is still regarded as a major worldwide health issue by scientists and practitioners, owing to its widespread occurrence and severe societal effect, particularly in less developed countries and regions. However, the early sensing and signaling mechanisms that initiate type 2 immunity against helminths remain unclear. The identification of these pathways might pave the way for the development of vaccines and medicines that target type 2 immunity. A recent study found that helminth infection can cause the synthesis of immunoregulatory substances that attract immune cells, resulting in infestations and inflammatory responses (Lightowlers and Rickard, 1988). Nontheless, the fundamental process, as well as the chemicals and cells involved, remain unclear. TCs were previously unseen to have great importance in this immunoreaction. TCs have been discovered as a significant activator of type 2 immunity in the small intestine by three distinct groups during the last decade (Gerbe et al., 2016; Howitt et al., 2016; von Moltke et al., 2016). Through a chemosensory mechanism, TCs in the small intestine detect helminths such as Heligmosomoides polygyrus, Trichinella spiralis, Nippostrongylus brasiliensis, and various species of Tritrichomonad protists.
In response to helminth infection (such as H. polygyrus), impaired epithelial cells release mediators such as leukotrienes, IL-22, and IL-33 (Artis and Grencis, 2008). Upon detecting the ligand, TCs transmit signals to the underlying lamina propria’s group 2 innate lymphoid cells (ILC2s), evoking an inflammation response. TCs are the only cell lineage that expresses IL-25 continuously (von Moltke et al., 2016). IL-25 stimulates ILC2s via the IL-17RB receptor. However, studies have observed that parasite-secreted H. polygyrus alarmin released inhibitor (HpARI) could hamper the “weep and sweep” immune response by limiting the IL-33 synthesis from injured epithelial cells (Osbourn et al., 2017). When subjected to helminth chemosensing, TCs produce cysteinyl leukotrienes (cysLTs), which rapidly activate type 2 immunity, accordng to McGinty et al.. CysLTs in collaboration with IL-25 stimulate ILC2s, and TC-specific leukotriene synthesis suppresses type 2 immunity and delays helminth clearance (McGinty et al., 2020). ILC2 activation may acquire additional signals to regulate the circuit in addition to IL-25. TCs in the colon, unlike those in the small intestine, respond to bacteria rather than parasites. Bacterial microflora can control colonic TC populations and stimulate TC growth, whereas colonic TCs have been shown to inhibit bacterial penetration and promote epithelial repair (McKinley et al., 2017; Wilen et al., 2018; Yi et al., 2019; Banerjee et al., 2020).
As a member of the chemokine family, IL-13 could stimulate secretory epithelial cells proliferation to boost mucus production and promote smooth muscle contraction to expel parasites in the intestine (Kamal et al., 2002; Gerbe et al., 2016; Howitt et al., 2016; Sharpe et al., 2018). IL-13 signals act directly on ISCs and bias their development towards the TCs and GCs, resulting in proliferation and a feed-forward loop in the tuft-ILC2 circuit. Upon the process, the quantity of TCs might rise tenfold within a few days of parasite infection (Gerbe et al., 2016; von Moltke et al., 2016). Given that IL-4 and IL-13 share a component, IL-4R, they may promote the proliferation of TCs (Gerbe et al., 2016), which also initiate smooth muscle contraction by releasing acetylcholine (ACh) and facilitate TCs to expel worms (Jonsson et al., 2007).
In mouse models, intestinal TCs appear around two weeks after birth, coinciding with epithelial changes in metabolic and nutritional behavior (Gerbe et al., 2011), as well as ILC2 and ILC3 growth (Hoyler et al., 2012), and the formation of solitary lymphoid clusters in the gut (Kiss et al., 2011). This research reveals a relationship between the ILC-epithelial cell axis and metabolic adaptation, showing that the innate immune system is important in homeostasis. A better knowledge of the innate immune system might pave the way for potential immunological advancements. The process of type 2 immune response orchestrated by TCs is shown in Figure 3.
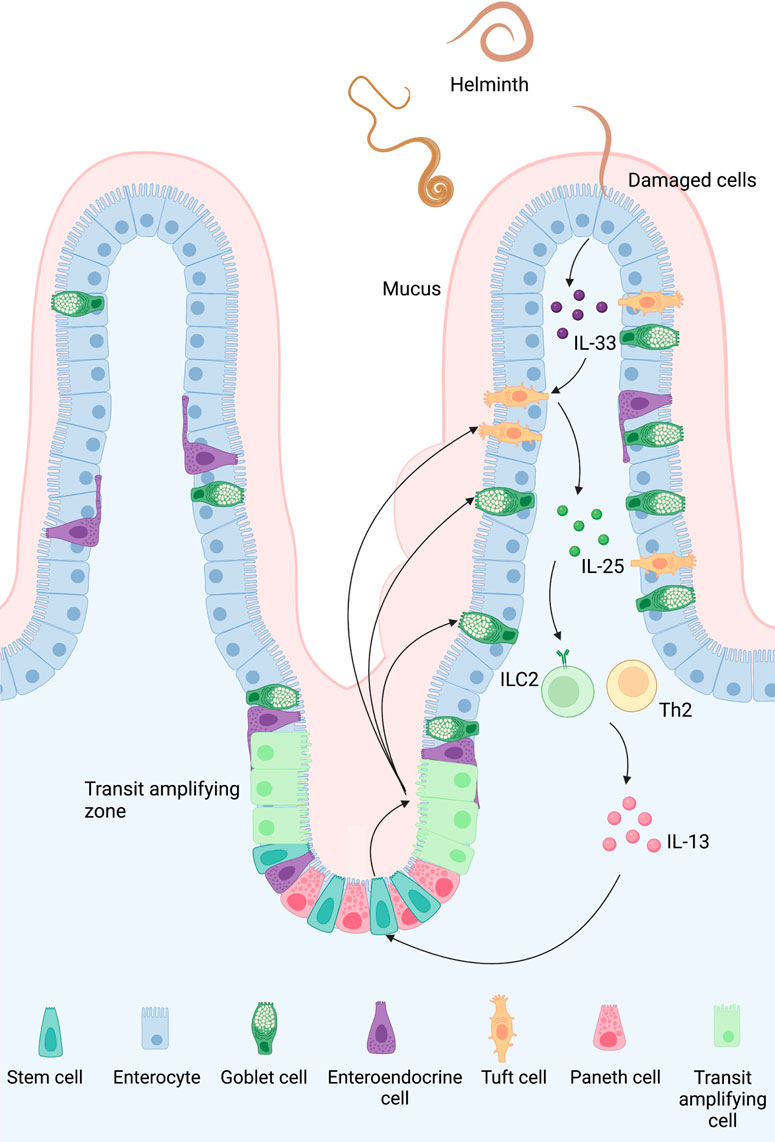
FIGURE 3. Type 2 immune response orchestrated by TCs in the small intestine: A feed-forward loop of the tuft 2-ILC2 axis can be observed in the early stage of intestinal helminth infection. Once the epithelial cells are damaged by a helminth, they release IL-33 and trigger TCs to secrete IL-25. Activated by these cytokines, ILC2 then produces IL-4 and IL-13, which promotes goblet and tuft hyperplasia and smooth muscle contraction. Although the exact mechanism of how TCs sense an infection in the first place is still unclear, TCs might sense succinate secreted by helminth and bacteria via protein-coupled succinate receptor SUCNR1. An intracellular Ca2+ flux follows the signal and opens the cation channel TRPM5, causing a Na+ influx which depolarizes TCs.
7.4 GPCR-PLCγ2-Ca2+ signaling axis involved in the elimination of bacterial infection via TCs
Researchers revealed in 2022 that, in addition to their role in the immunological response to helminth infection, TCs (tuft 2) also contribute to bacterial clearance via a Vmn2r26-mediated mechanism. Animals lacking CD45+ tuft 2 were more vulnerable to pathogenic bacteria, indicating that tuft 2 might develop and respond to harmful bacteria. Tuft 2 was also shown to recognize the microbial chemical N-undecanoylglycine via its vomeronasal receptor Vmn2r26, which can activate the GPCR-PLC2-Ca2+ signaling axis and produce prostaglandin D2 (PGD2), causing GCs to generate mucus and increases gut immunity (Xiong et al., 2022).
7.5 Potential role of TC in the amelioration of IBD
Inflammatory bowel disease (IBD), also known as ulcerative colitis (UC) and Crohn’s disease (CD), is a chronic inflammatory illness characterized by inflammation and mucosal destruction that threatens the intestine’s integrity. The primary objective of IBD treatment is to repair the inflammatory mucosa, which improves clinical symptoms, decreases disease recurrence, and increases survival without resection (Pineton de Chambrun et al., 2010; Colombel et al., 2011; Neurath and Travis, 2012).
TCs serve as important sentinels in the intestine, directing host responses to particular injuries, including helminth infection (Gerbe et al., 2016; Grencis and Worthington, 2016; Howitt et al., 2016; von Moltke et al., 2016; Gerbe and Jay, 2016), as well as facilitating epithelial repair after tumorigenesis and acute injury (Westphalen et al., 2014).
Although helminth infection itself is a global health issue, it may have an impact on the treatment of CD (Summers et al., 2005; Broadhurst et al., 2010). It is widely accepted that anti-parasitic immune responses can neutralize CD’s pro-inflammatory signals (Summers et al., 2005). Banerjee et al. (2020) observed a reduction in the number of TCs in ileal tissues of mouse models and CD patients, therefore they postulated that TCs could act as a hub between parisites and the host, thus can be used to counteract pro-inflammatory signals in the gut. In pathological situation, the absence of TCs and DCLK1 causes a regeneration deficiency, resulting in impaired recovery of the epithelium (Yi et al., 2019). In addition, the helminth-induced tuft-ILC2 loop promotes mucus secretion by GCs and TCs and protects the intestinal mucosa, which may contribute to alleviating the symptoms of IBD. To conclude, TC is a clinically feasible strategy for reducing IBD symptoms and prognosis.
7.6 DCLK1 is protective against radiation enteritis and DSS enteritis
Radiotherapy has become a popular treatment in many cancers, although it has certain unavoidable adverse effects. Chronic radiation enteritis has been documented in up to one in every five patients treated with pelvic irradiation, with the real number being greater (Daly et al., 1989; Yeoh et al., 1993; Miller et al., 1999; Ooi et al., 1999). Colonic inflammation should not be ignored as it is one of the key factors for colon cancer (Kim and Chang, 2014). Experiments showed that DCLK1 ablation in the intestinal epithelium worsens the outcome during acute intestinal injury induced by radaition and dextran-sodium sulfate (DSS), since inadequate DCLK1 promotes protective intestinal epithelial regeneration (May et al., 2014; Qu et al., 2015). These findings prove that DCLK1 maintains integrity of the intestinal epithelial barrier and modulates the inflammatory response (Qu et al., 2015).
7.7 TCs involve in regulating satiety and energy metabolism
TCs are assumed to be engaged in the gut-brain axis and metabolic control due to their closeness to metabolic-regulating enteroendocrine and enteric neurons in the gut (Cheng et al., 2018). Although the underlying mechanisms of TC participation are unknown, intestinal TCs boost secretory ability while suppressing absorptive capacity during type 2 immune response, indicating that TCs are engaged in satiety mice and energy metabolism. Furthermore, the population of TCs rises in starved mice and persists even after refeeding (McKinley et al., 2017). Evidence above suggests that TCs may aid in adapting to various dietary situations (Arora et al., 2021).
8 Diseases linked to TCs
A deeper understanding of the properties and functions of TCs may bring insights into studies of TC dysfunctions. Aberrant TC numbers and secretory behaviour have been observed in inflammation, infection, and tumors of the GI tract in both mice and humans (Saqui-Salces et al., 2011). TCs are normally quiescent but can be induced to proliferate in response to inflammatory stimuli (Westphalen et al., 2014; Middelhoff et al., 2017). When TCs poliferate, they may acquire mutations from stem cells and commence cancer when exposed to inflammation and damage (Westphalen et al., 2014). When the secretory behavior of TC changes, the downstream pathways are misregulated and ultimately lead to diseases. The precise processes and cause-and-effect link between TC anomalies and illnesses, however, remain unknown.
8.1 TC as a potential target of MNV
MNV is the primary cause of acute viral gastroenteritis worldwide, with similar incidence in high and low-income nations (Mans, 2019). Evidence shows that TCs is the principal target of chronic MNV in both the small and large intestines and may enhance immune evasion (Baldridge et al., 2015; Tomov et al., 2017; Wilen et al., 2018). In mice, TCs express high levels of MNV receptor CD300lf, which acts as a target for viral infection. Viral shedding occurs several weeks after the acute phase of infection (Teunis et al., 2015; Wilen et al., 2018).
8.2 Underlying linkage of IBD and TC-secreted IL-25
IBD is a collection of chronic idiopathic inflammatory illnesses that are widespread in Europe and North America. However, with industrialization and urbanization in the last 20 years, the prevalence of IBD in China has increased, attracting the attention of clinical practitioners and strengthening research into the condition.
IBD is distinguished by hidden asymptomatic intervals and repeated bouts of various degrees of gastrointestinal inflammation (Chang, 2020; Kobayashi et al., 2020; Roda et al., 2020). Blocking the p40 subunit shared by IL-12 and IL-23 was shown to induce colitis, leading to the conclusion that both the IL-12/Th1 and IL-23/Th17 axis may be implicated in the pathophysiology of CD and UC. (Gulati and Dubinsky, 2009; Strober and Fuss, 2011; Chang, 2020).
The considerable decrease in IL-25 in both inflamed intestinal mucosa and serum in patients with dilated IBD and healthy controls, as well as non-inflamed tissues and serum in patients with quiescent UC and CD, is cause for concern. When IBD was treated with infliximab, a TNF-α inhibitor, serum IL-25 levels returned to normal (Su et al., 2013). IL-25 may have a role in the etiology of IBD. Because TC acts as the sole generator of IL-25 in the mucosa, increasing IL-25 expression by TCs is a possible treatment strategy for IBD. The particular role of TC and the location of IL-25 expression, however, remain unknown. Yet, this simply suggests a correlation between IBD and the aberrant TC decrease, not a causal link. The findings presented here, that the quantity of TC in IBD may be altered, will provide vital insights into the underlying mechanism of IBD and clinical practice in the future.
8.3 Obesity may be associated with low secretion of IL-25 by TC
Obesity is a globally increasing disease that is a risk factor for the development of a variety of ailments, including numerous cardiovascular issues and digestive system changes. In both rats and humans, diet-induced obesity is characterized by chronic low-grade systemic inflammation as well as alterations in gut flora (Lee et al., 2018). Especially, the proportion of TCs to total epithelial cells was not altered, and TC-specific expression of IL-25 and TLSP was reduced (Arora et al., 2021) along with activation of the GABAA/B receptor pathways, which is positively correlated with alterations in the expression of the TC signature genes IL-25 and TSLP (Arora et al., 2021). This may provide solution for obesity by modulating TC secretion of IL-25 and TLSP.
8.4 TC-related DCLK1 may involve in alimentary tumor
DCLK1 is recognized as a possible marker since it is over-expressed in a variety of solid malignant tumors and has been associated to malignant biological activity and poor tumor prognosis (Chandrakesan et al., 2014; Ji et al., 2018).
Under normal circumstances, the only source of DCLK1 is TCs. DCLK1 has been detected in cancer stem cells (CSCs) from esophageal, pancreatic, and colon cancers (May et al., 2009; Vega et al., 2012; Weygant et al., 2015; Cao et al., 2020), suggesting that CSCs are derived from malignant TCs. CSCs interact with the immunosuppressive tumor microenvironment (TME) and aid in the activity of stem cells. An increasing body of data suggests that DCLK1+ TCs influence the formation and progression of inflammation-related malignancies (May et al., 2009; May et al., 2010; Vega et al., 2012; Weygant et al., 2015). Konishi et al. (2019) discovered in 2019 that TCs can induce Lgr5+ stem cells in the gastrointestinal tract, hence hastening cancer growth. Recent studies have further revealed that gastrointestinal TCs can promote hepatocellular carcinoma (HCC) development by secreting IL-25 to activate macrophages in TME (Friedrich et al., 2019). This “long-distance communication channel of the gut-liver axis” adds a new dimension to the study of TC function. Although TC markers can be found in mouse adenomas, they are uncommon in human cancer cell biopsies (Gerbe et al., 2011; Saqui-Salces et al., 2011), implying that animal studies are not yet useful for speculating on the association between human cancer and TC.
DCLK1 is expressed by certain pancreatic acinar and epithelial cells. Acinar-ductal metaplasia in pancreatic acinar cells may lead to cancer; DCLK1+ pancreatic epithelial cells are involved in regeneration following injury or inflammation (according to the lineage-tracing experiment); KRAS mutation in DCLK1+ pancreatic epithelial cells in pancreatitis may lead to pancreatic cancer (Nakanishi et al., 2013). Notably, utilizing a DCLK1 kinase inhibitor can reduce these DCLK1+ cells in the pancreas (Ferguson et al., 2020). These results suggest that DCLK1 may be a potential target for pancreatic cancer in clinical practice (Cao et al., 2020).
TC has been considered as a source of mature cell-derived carcinogenesis, alongside Paneth cells. In one word, DCLK1+ TCs (Nakanishi et al., 2013) or IL17RB+ TC-like cells (Goto et al., 2019) have been shown to act as stem cells in an intestinal tumor model. Similarly, in the context of further DSS-induced inflammation, Apc deletion in DCLK1+ TCs resulted in the development of colon tumors, whereas no DCLK1-expressing cells developed tumors in the steady state. Furthermore, following an acute assault, intestinal TC can act as colon cancer beginning cells (Westphalen et al., 2014). During validation, however, multiple essential pathways may be implicated in limiting TC activity and TC-derived tumor growth. For example, NF-κB signaling activation may be necessary for non-stem cell dedifferentiation and tumor development. At present, there are still many mysteries in this field. Future research will need to address this issue (Schwitalla et al., 2013).
9 Conclusion
We focused on the characteristics and functions of this peculiar cell lineage in this review. TCs secrete various molecules, suggesting that TCs may be associated with intrinsic immunity, intestinal secretion, contraction, pain, fatty acid metabolism, etc. TCs have chemosensory capabilities since they are comparable to tongue taste bud cells. It is worth noting that TC contains SUCNR1, which may detect pathogen invasion. The tuft-ILC2 circuit promotes TC and GC proliferation in type 2 immunity, ultimately expelling pathogens (especially helminth), which is of social significance. To eliminate bacterial infection, TCs also participate in (GPCR-PLCγ2)-Ca2+ signaling axis. Furhtermore, TCs might be involved in the gut-brain axis, as well as satiety and energy metabolism.
Diseases associated with TCs are of great concern. Murine TC has been identified as a MNV target. Reduced levels of TC-secreted IL-25 may be linked to IBD, obesity, duodenal ulcer, and acute duodenitis. However, as current studies are still inadequate, there is no more evidence supporting the precise involvement of TCs in these disorders, which is a limitation of our review. Despite the drawbacks, we believe that this evaluation will be useful for future TC-related research. Future research will reveal innovative paths for the diagnosis and treatment of these diseases if the causal link between TCs and the disorders is clarified. DCLK1+ cells have been shown to induce tumor growth in the GI tract. Given that TC is the only source of intestinal DCLK1 in the physiological state in mice, it can be hypothesized that carcinogenesis is associated with aberrant TC proliferation. Once the aforesaid molecular mechanisms are elucidated, new approaches for early molecular screening and therapy of GI cancers will emerge.
Boxes
BOX 1 Post-mitotic cells
According to research, TCs are short-lived post-mitotic cells with a lifespan of at least seven days and are regularly replenished (Gerbe et al., 2011). In the “transit-amplifying” zone, the Lgr5+ stem cell transformed into shorter-lived cells. Cells continue to move but cease proliferating when they reach the crypt-villus border, resulting in a villus composed entirely of post-mitotic cells.
BOX 2 Different terminology for cells in the differentiation process and their correspondence
Bjerknes et al. define TA cells as “Mix”, so the “daughters of Mix” was abbreviated as “DOM”, which equals the “daughters of TA cells”. Bjerknes et al. refered to DOM entering different states as DOMNotch (Absoprive progenitor) and DOMΔ (Secretory progenitor), respectively.
BOX 3 TRPM5
TRPM5 is a critical component of taste transduction, such as bitter, sweet, and umami. It also has a possible role in fat taste signaling (Liu et al., 2011; Mattes, 2011). Expressed in pancreatic β-cells (Colsoul et al., 2010), TRPM5 was proposed to be related to insulin secretion and lower risk of type 2 diabetes in mice (Philippaert et al., 2017). TRPM5 is expressed in sensory cells, including solitary chemosensory cells and TCs.
BOX 4 Functions of TCs in other organs or tissues
This cell lineage behaves differently in various organs and tissues than in the gastrointestinal tract. Pancreatic TCs could reduce carcinogenesis by secreting prostaglandins (Delgiorno et al., 2014); tracheal TCs could participate in mucociliary clearance (Perniss et al., 2020); and thymic TCs could take part in the nurture of B cells, NK cells, and T cells (Bornstein et al., 2018).
Author contributions
YD, HG, and CH analyzed the reference and wrote of the manuscript. SX, BW, and SZ polished the images. FG, XY, LP, and FS analyze the literature data and revise the text part of the article. WW and JX analyzed the data and revised the manuscript. All authors discussed the manuscript and approved of the final version.
Funding
This study was supported by National Natural Science Foundation of China (No.82174056 JX; 82173795 WW).
Acknowledgments
We would like to express our special thanks to Xin Tao. Department of Applied Linguistics of Capital Medical University for the language polishing and grammar proofreading in this article, as well as the support of BioRender.com where the figures are created.
Conflict of interenst
The authors declare that the research was conducted in the absence of any commercial or financial relationships that could be construed as a potential conflict of interest.
Publisher’s note
All claims expressed in this article are solely those of the authors and do not necessarily represent those of their affiliated organizations, or those of the publisher, the editors and the reviewers. Any product that may be evaluated in this article, or claim that may be made by its manufacturer, is not guaranteed or endorsed by the publisher.
References
Akazawa, C., Ishibashi, M., Shimizu, C., Nakanishi, S., and Kageyama, R. (1995). A mammalian helix-loop-helix factor structurally related to the product of Drosophila proneural gene atonal is a positive transcriptional regulator expressed in the developing nervous system. J. Biol. Chem. 270, 8730–8738. doi:10.1074/jbc.270.15.8730
Alexander, I., Ritchie, B. C., Maloney, J. E., and Hunter, C. R. (1975). Epithelial surfaces of the trachea and principal bronchi in the rat. Thorax 30 (2), 171–177. doi:10.1136/thx.30.2.171
Arora, P., Andersen, D., Moll, J. M., Danneskiold-Samsoe, N. B., Xu, L., Zhou, B., et al. (2021). Small intestinal tuft cell activity associates with energy metabolism in diet-induced obesity. Front. Immunol. 12, 629391. doi:10.3389/fimmu.2021.629391
Artis, D., and Grencis, R. K. (2008). The intestinal epithelium: Sensors to effectors in nematode infection. Mucosal Immunol. 1, 252–264. doi:10.1038/mi.2008.21
Baldridge, M. T., Nice, T. J., Mccune, B. T., Yokoyama, C. C., Kambal, A., Wheadon, M., et al. (2015). Commensal microbes and interferon-lambda determine persistence of enteric murine norovirus infection. Science 347, 266–269. doi:10.1126/science.1258025
Banerjee, A., Herring, C. A., Chen, B., Kim, H., Simmons, A. J., Southard-Smith, A. N., et al. (2020). Succinate produced by intestinal microbes promotes specification of tuft cells to suppress ileal inflammation. Gastroenterology 159, 2101–2115. e5. doi:10.1053/j.gastro.2020.08.029
Barker, N. (2014). Adult intestinal stem cells: Critical drivers of epithelial homeostasis and regeneration. Nat. Rev. Mol. Cell. Biol. 15, 19–33. doi:10.1038/nrm3721
Barker, N., Van Es, J. H., Kuipers, J., Kujala, P., Van Den Born, M., Cozijnsen, M., et al. (2007). Identification of stem cells in small intestine and colon by marker gene Lgr5. Nature 449, 1003–1007. doi:10.1038/nature06196
Bastide, P., Darido, C., Pannequin, J., Kist, R., Robine, S., Marty-Double, C., et al. (2007). Sox9 regulates cell proliferation and is required for Paneth cell differentiation in the intestinal epithelium. J. Cell. Biol. 178, 635–648. doi:10.1083/jcb.200704152
Berger, M., Gray, J. A., and Roth, B. L. (2009). The expanded biology of serotonin. Annu. Rev. Med. 60, 355–366. doi:10.1146/annurev.med.60.042307.110802
Bezencon, C., Le Coutre, J., and Damak, S. (2007). Taste-signaling proteins are coexpressed in solitary intestinal epithelial cells. Chem. Senses 32, 41–49. doi:10.1093/chemse/bjl034
Bezencon, C., Furholz, A., Raymond, F., Mansourian, R., Metairon, S., Le Coutre, J., et al. (2008). Murine intestinal cells expressing Trpm5 are mostly brush cells and express markers of neuronal and inflammatory cells. J. Comp. Neurol. 509, 514–525. doi:10.1002/cne.21768
Bjerknes, M., and Cheng, H. (2005). Gastrointestinal stem cells. II. Intestinal stem cells. Am. J. Physiol. Gastrointest. Liver Physiol. 289, G381–G387. doi:10.1152/ajpgi.00160.2005
Bjerknes, M., and Cheng, H. (2010). Cell Lineage metastability in Gfi1-deficient mouse intestinal epithelium. Dev. Biol. 345, 49–63. doi:10.1016/j.ydbio.2010.06.021
Bjerknes, M., Khandanpour, C., Moroy, T., Fujiyama, T., Hoshino, M., Klisch, T. J., et al. (2012). Origin of the brush cell lineage in the mouse intestinal epithelium. Dev. Biol. 362, 194–218. doi:10.1016/j.ydbio.2011.12.009
Bornstein, C., Nevo, S., Giladi, A., Kadouri, N., Pouzolles, M., Gerbe, F., et al. (2018). Single-cell mapping of the thymic stroma identifies IL-25-producing tuft epithelial cells. Nature 559, 622–626. doi:10.1038/s41586-018-0346-1
Breipohl, W., Herberhold, C., and Kerschek, R. (1977). Microridge cells in the larynx of the male white rat. Investigations by reflection scanning electron microscopy. Arch. Otorhinolaryngol. 215 (1), 1–9. doi:10.1007/BF00463186
Broadhurst, M. J., Leung, J. M., Kashyap, V., Mccune, J. M., Mahadevan, U., Mckerrow, J. H., et al. (2010). IL-22+ CD4+ T cells are associated with therapeutic trichuris trichiura infection in an ulcerative colitis patient. Sci. Transl. Med. 2, 60ra88. doi:10.1126/scitranslmed.3001500
Cao, Z., Weygant, N., Chandrakesan, P., Houchen, C. W., Peng, J., and Qu, D. (2020). Tuft and cancer stem cell marker DCLK1: A new target to enhance anti-tumor immunity in the tumor microenvironment. Cancers (Basel) 12, 3801. doi:10.3390/cancers12123801
Carulli, A. J., Keeley, T. M., Demitrack, E. S., Chung, J., Maillard, I., and Samuelson, L. C. (2015). Notch receptor regulation of intestinal stem cell homeostasis and crypt regeneration. Dev. Biol. 402, 98–108. doi:10.1016/j.ydbio.2015.03.012
Cerezo, L., and Price, G. (1985). Absence of cilia and basal bodies with predominance of brush cells in the respiratory mucosa from a patient with immotile cilia syndrome. Ultrastruct. Pathol. 8, 381–382. doi:10.3109/01913128509141528
Chandrakesan, P., Weygant, N., May, R., Qu, D., Chinthalapally, H. R., Sureban, S. M., et al. (2014). DCLK1 facilitates intestinal tumor growth via enhancing pluripotency and epithelial mesenchymal transition. Oncotarget 5, 9269–9280. doi:10.18632/oncotarget.2393
Chandrakesan, P., May, R., Weygant, N., Qu, D., Berry, W. L., Sureban, S. M., et al. (2016). Intestinal tuft cells regulate the ATM mediated DNA Damage response via Dclk1 dependent mechanism for crypt restitution following radiation injury. Sci. Rep. 6, 37667. doi:10.1038/srep37667
Chang, J. T. (2020). Pathophysiology of inflammatory bowel diseases. N. Engl. J. Med. 383, 2652–2664. doi:10.1056/NEJMra2002697
Chang, L. Y., Mercer, R. R., and Crapo, J. D. (1986). Differential distribution of brush cells in the rat lung. Anat. Rec. 216, 49–54. doi:10.1002/ar.1092160109
Cheng, X., Voss, U., and Ekblad, E. (2018). Tuft cells: Distribution and connections with nerves and endocrine cells in mouse intestine. Exp. Cell. Res. 369, 105–111. doi:10.1016/j.yexcr.2018.05.011
Cheng, X., Voss, U., and Ekblad, E. (2019). A novel serotonin-containing tuft cell subpopulation in mouse intestine. Cell. Tissue Res. 376, 189–197. doi:10.1007/s00441-018-02988-3
Chitnis, A. B. (1995). The role of Notch in lateral inhibition and cell fate specification. Mol. Cell. Neurosci. 6, 311–321. doi:10.1006/mcne.1995.1024
Colombel, J. F., Rutgeerts, P., Reinisch, W., Esser, D., Wang, Y., Lang, Y., et al. (2011). Early mucosal healing with infliximab is associated with improved long-term clinical outcomes in ulcerative colitis. Gastroenterology 141, 1194–1201. doi:10.1053/j.gastro.2011.06.054
Colsoul, B., Schraenen, A., Lemaire, K., Quintens, R., Van Lommel, L., Segal, A., et al. (2010). Loss of high-frequency glucose-induced Ca2+ oscillations in pancreatic islets correlates with impaired glucose tolerance in Trpm5-/- mice. Proc. Natl. Acad. Sci. U. S. A. 107, 5208–5213. doi:10.1073/pnas.0913107107
Daly, N. J., Izar, F., Bachaud, J. M., and Delannes, M. (1989). The incidence of severe chronic ileitis after abdominal and/or pelvic external irradiation with high energy photon beams. Radiother. Oncol. 14, 287–295. doi:10.1016/0167-8140(89)90140-0
De Sousa, E. M. F., and De Sauvage, F. J. (2019). Cellular plasticity in intestinal homeostasis and disease. Cell. Stem Cell. 24, 54–64. doi:10.1016/j.stem.2018.11.019
Dekaney, C. M., Gulati, A. S., Garrison, A. P., Helmrath, M. A., and Henning, S. J. (2009). Regeneration of intestinal stem/progenitor cells following doxorubicin treatment of mice. Am. J. Physiol. Gastrointest. Liver Physiol. 297, G461–G470. doi:10.1152/ajpgi.90446.2008
Delgiorno, K. E., Hall, J. C., Takeuchi, K. K., Pan, F. C., Halbrook, C. J., Washington, M. K., et al. (2014). Identification and manipulation of biliary metaplasia in pancreatic tumors. Gastroenterology 146, 233–244. doi:10.1053/j.gastro.2013.08.053
Delgiorno, K. E., Naeem, R. F., Fang, L., Chung, C. Y., Ramos, C., Luhtala, N., et al. (2020). Tuft cell formation reflects epithelial plasticity in pancreatic injury: Implications for modeling human pancreatitis. Front. Physiol. 11, 88. doi:10.3389/fphys.2020.00088
Dimaio, M. F., Dische, R., Gordon, R. E., and Kattan, M. (1988). Alveolar brush cells in an infant with desquamative interstitial pneumonitis. Pediatr. Pulmonol. 4, 185–191. doi:10.1002/ppul.1950040312
Farin, H. F., Van Es, J. H., and Clevers, H. (2012). Redundant sources of Wnt regulate intestinal stem cells and promote formation of Paneth cells. Gastroenterology 143, 1518–1529. doi:10.1053/j.gastro.2012.08.031
Ferguson, F. M., Nabet, B., Raghavan, S., Liu, Y., Leggett, A. L., Kuljanin, M., et al. (2020). Discovery of a selective inhibitor of doublecortin like kinase 1. Nat. Chem. Biol. 16, 635–643. doi:10.1038/s41589-020-0506-0
Flier, L. G., and Clevers, H. (2009). Stem cells, self-renewal, and differentiation in the intestinal epithelium. Annu. Rev. Physiol. 71, 241–260. doi:10.1146/annurev.physiol.010908.163145
Formeister, E. J., Sionas, A. L., Lorance, D. K., Barkley, C. L., Lee, G. H., and Magness, S. T. (2009). Distinct SOX9 levels differentially mark stem/progenitor populations and enteroendocrine cells of the small intestine epithelium. Am. J. Physiol. Gastrointest. Liver Physiol. 296, G1108–G1118. doi:10.1152/ajpgi.00004.2009
Fortini, M. E. (2009). Notch signaling: The core pathway and its posttranslational regulation. Dev. Cell. 16, 633–647. doi:10.1016/j.devcel.2009.03.010
Fre, S., Pallavi, S. K., Huyghe, M., Lae, M., Janssen, K. P., Robine, S., et al. (2009). Notch and Wnt signals cooperatively control cell proliferation and tumorigenesis in the intestine. Proc. Natl. Acad. Sci. U. S. A. 106, 6309–6314. doi:10.1073/pnas.0900427106
Friedrich, M., Jasinski-Bergner, S., Lazaridou, M. F., Subbarayan, K., Massa, C., Tretbar, S., et al. (2019). Tumor-induced escape mechanisms and their association with resistance to checkpoint inhibitor therapy. Cancer Immunol. Immunother. 68, 1689–1700. doi:10.1007/s00262-019-02373-1
Gebert, A., Al-Samir, K., Werner, K., Fassbender, S., and Gebhard, A. (2000). The apical membrane of intestinal brush cells possesses a specialised, but species-specific, composition of glycoconjugates--on-section and in vivo lectin labelling in rats, Guinea-pigs and mice. Histochem Cell. Biol. 113, 389–399. doi:10.1007/s004180000148
Gebhard, A., and Gebert, A. (1999). Brush cells of the mouse intestine possess a specialized glycocalyx as revealed by quantitative lectin histochemistry. Further evidence for a sensory function. J. Histochem Cytochem 47, 799–808. doi:10.1177/002215549904700609
Gerbe, F., and Jay, P. (2016). Intestinal tuft cells: Epithelial sentinels linking luminal cues to the immune system. Mucosal Immunol. 9, 1353–1359. doi:10.1038/mi.2016.68
Gerbe, F., Brulin, B., Makrini, L., Legraverend, C., and Jay, P. (2009). DCAMKL-1 expression identifies Tuft cells rather than stem cells in the adult mouse intestinal epithelium. Gastroenterology 137, 2179–2180. author reply 2180-2181. doi:10.1053/j.gastro.2009.06.072
Gerbe, F., Van Es, J. H., Makrini, L., Brulin, B., Mellitzer, G., Robine, S., et al. (2011). Distinct ATOH1 and Neurog3 requirements define tuft cells as a new secretory cell type in the intestinal epithelium. J. Cell. Biol. 192, 767–780. doi:10.1083/jcb.201010127
Gerbe, F., Legraverend, C., and Jay, P. (2012). The intestinal epithelium tuft cells: Specification and function. Cell. Mol. Life Sci. 69, 2907–2917. doi:10.1007/s00018-012-0984-7
Gerbe, F., Sidot, E., Smyth, D. J., Ohmoto, M., Matsumoto, I., Dardalhon, V., et al. (2016). Intestinal epithelial tuft cells initiate type 2 mucosal immunity to helminth parasites. Nature 529, 226–230. doi:10.1038/nature16527
Giannakis, M., Stappenbeck, T. S., Mills, J. C., Leip, D. G., Lovett, M., Clifton, S. W., et al. (2006). Molecular properties of adult mouse gastric and intestinal epithelial progenitors in their niches. J. Biol. Chem. 281, 11292–11300. doi:10.1074/jbc.M512118200
Gordon, R. E., and Kattan, M. (1984). Absence of cilia and basal bodies with predominance of brush cells in the respiratory mucosa from a patient with immotile cilia syndrome. Ultrastruct. Pathol. 6, 45–49. doi:10.3109/01913128409016664
Goto, N., Fukuda, A., Yamaga, Y., Yoshikawa, T., Maruno, T., Maekawa, H., et al. (2019). Lineage tracing and targeting of IL17RB(+) tuft cell-like human colorectal cancer stem cells. Proc. Natl. Acad. Sci. U. S. A. 116, 12996–13005. doi:10.1073/pnas.1900251116
Gracz, A. D., Samsa, L. A., Fordham, M. J., Trotier, D. C., Zwarycz, B., Lo, Y. H., et al. (2018). Sox4 promotes atoh1-independent intestinal secretory differentiation toward tuft and enteroendocrine fates. Gastroenterology 155, 1508–1523. doi:10.1053/j.gastro.2018.07.023
Gregorieff, A., Stange, D. E., Kujala, P., Begthel, H., van den Born, M., Korving, J., et al. (2009). The ets-domain transcription factor Spdef promotes maturation of goblet and paneth cells in the intestinal epithelium. Gastroenterology 137 (4), 1333–1345. doi:10.1053/j.gastro.2009.06.044
Grencis, R. K., and Worthington, J. J. (2016). Tuft cells: A new flavor in innate epithelial immunity. Trends Parasitol. 32, 583–585. doi:10.1016/j.pt.2016.04.016
Gulati, A. S., and Dubinsky, M. C. (2009). Probiotics in pediatric inflammatory bowel diseases. Curr. Gastroenterol. Rep. 11, 238–247. doi:10.1007/s11894-009-0037-z
Haber, A. L., Biton, M., Rogel, N., Herbst, R. H., Shekhar, K., Smillie, C., et al. (2017). A single-cell survey of the small intestinal epithelium. Nature 551, 333–339. doi:10.1038/nature24489
Hammond, J. B., and ladeur, L. (1968). Fibrillovesicular cedlls in the fundic glands of the canine stomach: Evidence for a new cell type. Anat. Rec. 161, 393–411. doi:10.1002/ar.1091610401
Hartmann, D., De Strooper, B., Serneels, L., Craessaerts, K., Herreman, A., Annaert, W., et al. (2002). The disintegrin/metalloprotease ADAM 10 is essential for Notch signalling but not for alpha-secretase activity in fibroblasts. Hum. Mol. Genet. 11, 2615–2624. doi:10.1093/hmg/11.21.2615
Hass, N., Schwarzenbacher, K., and Breer, H. (2007). A cluster of gustducin-expressing cells in the mouse stomach associated with two distinct populations of enteroendocrine cells. Histochem Cell Biol. 128 (5), 457–471. doi:10.1007/s00418-007-0325-3
Herring, C. A., Banerjee, A., Mckinley, E. T., Simmons, A. J., Ping, J., Roland, J. T., et al. (2018). Unsupervised trajectory analysis of single-cell RNA-seq and imaging data reveals alternative tuft cell origins in the gut. Cell. Syst. 6, 37–51. doi:10.1016/j.cels.2017.10.012
Hofer, D., and Drenckhahn, D. (1992). Identification of brush cells in the alimentary and respiratory system by antibodies to villin and fimbrin. Histochemistry 98, 237–242. doi:10.1007/BF00271037
Hofer, D., and Drenckhahn, D. (1996). Cytoskeletal markers allowing discrimination between brush cells and other epithelial cells of the gut including enteroendocrine cells. Histochem Cell. Biol. 105, 405–412. doi:10.1007/BF01463662
Hofer, D., and Drenckhahn, D. (1998). Identification of the taste cell G-protein, alpha-gustducin, in brush cells of the rat pancreatic duct system. Histochem Cell. Biol. 110, 303–309. doi:10.1007/s004180050292
Hofer, D., Puschel, B., and Drenckhahn, D. (1996). Taste receptor-like cells in the rat gut identified by expression of alpha-gustducin. Proc. Natl. Acad. Sci. U. S. A. 93, 6631–6634. doi:10.1073/pnas.93.13.6631
Holzer, P. (2009). Opioid receptors in the gastrointestinal tract. Regul. Pept. 155 (1-3), 11–17. doi:10.1016/j.regpep.2009.03.012
Hoover, B., Baena, V., Kaelberer, M. M., Getaneh, F., Chinchilla, S., and Bohorquez, D. V. (2017). The intestinal tuft cell nanostructure in 3D. Sci. Rep. 7, 1652. doi:10.1038/s41598-017-01520-x
Howitt, M. R., Lavoie, S., Michaud, M., Blum, A. M., Tran, S. V., Weinstock, J. V., et al. (2016). Tuft cells, taste-chemosensory cells, orchestrate parasite type 2 immunity in the gut. Science 351, 1329–1333. doi:10.1126/science.aaf1648
Hoyler, T., Klose, C. S., Souabni, A., Turqueti-Neves, A., Pfeifer, D., Rawlins, E. L., et al. (2012). The transcription factor GATA-3 controls cell fate and maintenance of type 2 innate lymphoid cells. Immunity 37, 634–648. doi:10.1016/j.immuni.2012.06.020
Iseki, S., Kanda, T., HitoMiM., , and Ono, T. (1991). Ontogenic appearance of three fatty acid binding proteins in the rat stomach. Anat. Rec. 229 (1), 51–60. doi:10.1002/ar.1092290107
Iseki, S., and Kondo, H. (1990). An immunocytochemical study on the occurrence of liver fatty-acid-binding protein in the digestive organs of rats: Specific localization in the D cells and brush cells. Acta Anat. (Basel) 138 (1), 15–23. doi:10.1159/000146915
Ishibashi, F., Shimizu, H., Nakata, T., Fujii, S., Suzuki, K., Kawamoto, A., et al. (2018). Contribution of ATOH1(+) cells to the homeostasis, repair, and tumorigenesis of the colonic epithelium. Stem Cell. Rep. 10, 27–42. doi:10.1016/j.stemcr.2017.11.006
Isomaki, A. M. (1973). A new cell type (tuft cell) in the gastrointestinal mucosa of the rat. A transmission and scanning electron microscopic study. Acta Pathol. Microbiol. Scand. A. (240), 1–35.
Jang, H. J., Kokrashvili, Z., Theodorakis, M. J., Carlson, O. D., Kim, B. J., Zhou, J., et al. (2007). Gut-expressed gustducin and taste receptors regulate secretion of glucagon-like peptide-1. Proc. Natl. Acad. Sci. U. S. A. 104, 15069–15074. doi:10.1073/pnas.0706890104
Jarvi, O. H., Hormia, M. S., Autio, J. V., Kangas, S. J., and Tilvis, P. K. (1967). Cytologic diagnosis of pulmonary carcinoma in two hospitals. Acta Cytol. 11, 477–482.
Jarvi, O., and Keyrilainen, O. (1956). On the cellular structures of the epithelial invasions in the glandular stomach of mice caused by intramural application of 20-methylcholantren. Acta Pathol. Microbiol. Scand. Suppl. 39, 72–73. doi:10.1111/j.1600-0463.1956.tb06739.x
Jeffery, P. K. (1983). Morphologic features of airway surface epithelial cells and glands. Am. Rev. Respir. Dis. 128, S14–S20. doi:10.1164/arrd.1983.128.2P2.S14
Jensen, J., Pedersen, E. E., Galante, P., Hald, J., Heller, R. S., Ishibashi, M., et al. (2000). Control of endodermal endocrine development by Hes-1. Nat. Genet. 24, 36–44. doi:10.1038/71657
Jenny, M., Uhl, C., Roche, C., Duluc, I., Guillermin, V., Guillemot, F., et al. (2002). Neurogenin3 is differentially required for endocrine cell fate specification in the intestinal and gastric epithelium. EMBO J. 21, 6338–6347. doi:10.1093/emboj/cdf649
Ji, D., Zhan, T., Li, M., Yao, Y., Jia, J., Yi, H., et al. (2018). Enhancement of sensitivity to chemo/radiation therapy by using miR-15b against DCLK1 in colorectal cancer. Stem Cell. Rep. 11, 1506–1522. doi:10.1016/j.stemcr.2018.10.015
Jin, G., Ramanathan, V., Quante, M., Baik, G. H., Yang, X., Wang, S. S., et al. (2009). Inactivating cholecystokinin-2 receptor inhibits progastrin-dependent colonic crypt fission, proliferation, and colorectal cancer in mice. J. Clin. Invest. 119, 2691–2701. doi:10.1172/JCI38918
Jonsson, M., Norrgard, O., and Forsgren, S. (2007). Presence of a marked nonneuronal cholinergic system in human colon: Study of normal colon and colon in ulcerative colitis. Inflamm. Bowel Dis. 13, 1347–1356. doi:10.1002/ibd.20224
Kamal, M., Dehlawi, M. S., Brunet, L. R., and Wakelin, D. (2002). Paneth and intermediate cell hyperplasia induced in mice by helminth infections. Parasitology 125, 275–281. doi:10.1017/s0031182002002068
Kaske, S., Krasteva, G., Konig, P., Kummer, W., Hofmann, T., Gudermann, T., et al. (2007). TRPM5, a taste-signaling transient receptor potential ion-channel, is a ubiquitous signaling component in chemosensory cells. BMC Neurosci. 8, 49. doi:10.1186/1471-2202-8-49
Katz, J. P., Perreault, N., Goldstein, B. G., Lee, C. S., Labosky, P. A., Yang, V. W., et al. (2002). The zinc-finger transcription factor Klf4 is required for terminal differentiation of goblet cells in the colon. Development 129 (11), 2619–2628. doi:10.1242/dev.129.11.2619
Kim, E. R., and Chang, D. K. (2014). Colorectal cancer in inflammatory bowel disease: The risk, pathogenesis, prevention and diagnosis. World J. Gastroenterol. 20, 9872–9881. doi:10.3748/wjg.v20.i29.9872
Kim, T. H., Escudero, S., and Shivdasani, R. A. (2012). Intact function of Lgr5 receptor-expressing intestinal stem cells in the absence of Paneth cells. Proc. Natl. Acad. Sci. U. S. A. 109, 3932–3937. doi:10.1073/pnas.1113890109
Kimble, J., and Simpson, P. (1997). The LIN-12/Notch signaling pathway and its regulation. Annu. Rev. Cell. Dev. Biol. 13, 333–361. doi:10.1146/annurev.cellbio.13.1.333
Kiss, E. A., Vonarbourg, C., Kopfmann, S., Hobeika, E., Finke, D., Esser, C., et al. (2011). Natural aryl hydrocarbon receptor ligands control organogenesis of intestinal lymphoid follicles. Science 334, 1561–1565. doi:10.1126/science.1214914
Kobayashi, T., Siegmund, B., Le Berre, C., Wei, S. C., Ferrante, M., Shen, B., et al. (2020). Ulcerative colitis. Nat. Rev. Dis. Prim. 6, 74. doi:10.1038/s41572-020-0205-x
Kokrashvili, Z., Rodriguez, D., Yevshayeva, V., Zhou, H., Margolskee, R. F., and Mosinger, B. (2009). Release of endogenous opioids from duodenal enteroendocrine cells requires Trpm5. Gastroenterology 137, 598–606. 606 e1-2. doi:10.1053/j.gastro.2009.02.070
Konishi, M., Hayakawa, Y., and Koike, K. (2019). Role of muscarinic acetylcholine signaling in gastrointestinal cancers. Biomedicines 7, 58. doi:10.3390/biomedicines7030058
Korinek, V., Barker, N., Moerer, P., Van Donselaar, E., Huls, G., Peters, P. J., et al. (1998). Depletion of epithelial stem-cell compartments in the small intestine of mice lacking Tcf-4. Nat. Genet. 19, 379–383. doi:10.1038/1270
Kugler, P., Hofer, D., Mayer, B., and Drenckhahn, D. (1994). Nitric oxide synthase and NADP-linked glucose-6-phosphate dehydrogenase are co-localized in brush cells of rat stomach and pancreas. J. Histochem Cytochem 42, 1317–1321. doi:10.1177/42.10.7523487
Langille, M. G., Zaneveld, J., Caporaso, J. G., Mcdonald, D., Knights, D., Reyes, J. A., et al. (2013). Predictive functional profiling of microbial communities using 16S rRNA marker gene sequences. Nat. Biotechnol. 31, 814–821. doi:10.1038/nbt.2676
Lee, Y. S., Wollam, J., and Olefsky, J. M. (2018). An integrated view of immunometabolism. Cell. 172, 22–40. doi:10.1016/j.cell.2017.12.025
Lei, W., Ren, W., Ohmoto, M., Urban, J. F., Matsumoto, I., Margolskee, R. F., et al. (2018). Activation of intestinal tuft cell-expressed Sucnr1 triggers type 2 immunity in the mouse small intestine. Proc. Natl. Acad. Sci. U. S. A. 115, 5552–5557. doi:10.1073/pnas.1720758115
Lightowlers, M. W., and Rickard, M. D. (1988). Excretory-secretory products of helminth parasites: Effects on host immune responses. Parasitology 96, S123–S166. doi:10.1017/s0031182000086017
Liu, P., Shah, B. P., Croasdell, S., and Gilbertson, T. A. (2011). Transient receptor potential channel type M5 is essential for fat taste. J. Neurosci. 31, 8634–8642. doi:10.1523/JNEUROSCI.6273-10.2011
Luciano, L., Castellucci, M., and Reale, E. (1981). The brush cells of the common bile duct of the rat. This section, freeze-fracture and scanning electron microscopy. Cell Tissue Res. 218 (2), 403–420. doi:10.1007/BF00210353
Luciano, L., and Reale, E. (1979). A new morphological aspect of the brush cells of the mouse gallbladder epithelium. Cell. Tissue Res. 201, 37–44. doi:10.1007/BF00238045
Luciano, L., and Reale, E. (1990). Brush cells of the mouse gallbladder. A correlative light- and electron-microscopical study. Cell. Tissue Res. 262, 339–349. doi:10.1007/BF00309889
Luciano, L., and Reale, E. (1997). Presence of brush cells in the mouse gallbladder. Microsc. Res. Tech. 38, 598–608. doi:10.1002/(SICI)1097-0029(19970915)38:6<598:AID-JEMT4>3.0.CO;2-B
Luciano, L., Reale, E., and Ruska, H. (1968). On a "chemoreceptive" sensory cell in the tachea of the rat. Z Zellforsch Mikrosk Anat. 85, 350–375. doi:10.1007/bf00328847
Luciano, L., Reale, E., and Ruska, H. (1969). Brush cells in the alveolar epithelium of the rat lung. Z Zellforsch Mikrosk Anat. 95, 198–201. doi:10.1007/bf00968452
Madara, J. L. (1982). Cup cells: Structure and distribution of a unique class of epithelial cells in Guinea pig, rabbit, and monkey small intestine. Gastroenterology 83, 981–994. doi:10.1016/s0016-5085(82)80064-4
Mans, J. (2019). Norovirus infections and disease in lower-MiddleandLow-income countries, 1997-2018. Viruses 11, 341. doi:10.3390/v11040341
Mattes, R. D. (2011). Accumulating evidence supports a taste component for free fatty acids in humans. Physiol. Behav. 104, 624–631. doi:10.1016/j.physbeh.2011.05.002
May, R., Riehl, T. E., Hunt, C., Sureban, S. M., Anant, S., and Houchen, C. W. (2008). Identification of a novel putative gastrointestinal stem cell and adenoma stem cell marker, doublecortin and CaM kinase-like-1, following radiation injury and in adenomatous polyposis coli/multiple intestinal neoplasia mice. Stem Cells 26, 630–637. doi:10.1634/stemcells.2007-0621
May, R., Sureban, S. M., Hoang, N., Riehl, T. E., Lightfoot, S. A., Ramanujam, R., et al. (2009). Doublecortin and CaM kinase-like-1 and leucine-rich-repeat-containing G-protein-coupled receptor mark quiescent and cycling intestinal stem cells, respectively. Stem Cells 27, 2571–2579. doi:10.1002/stem.193
May, R., Sureban, S. M., Lightfoot, S. A., Hoskins, A. B., Brackett, D. J., Postier, R. G., et al. (2010). Identification of a novel putative pancreatic stem/progenitor cell marker DCAMKL-1 in normal mouse pancreas. Am. J. Physiol. Gastrointest. Liver Physiol. 299, G303–G310. doi:10.1152/ajpgi.00146.2010
May, R., Qu, D., Weygant, N., Chandrakesan, P., Ali, N., Lightfoot, S. A., et al. (2014). Brief report: Dclk1 deletion in tuft cells results in impaired epithelial repair after radiation injury. Stem Cells 32, 822–827. doi:10.1002/stem.1566
Mcginty, J. W., Ting, H. A., Billipp, T. E., Nadjsombati, M. S., Khan, D. M., Barrett, N. A., et al. (2020). Tuft-cell-derived leukotrienes drive rapid anti-helminth immunity in the small intestine but are dispensable for anti-protist immunity. Immunity 52, 528–541. doi:10.1016/j.immuni.2020.02.005
Mckinley, E. T., Sui, Y., Al-Kofahi, Y., Millis, B. A., Tyska, M. J., Roland, J. T., et al. (2017). Optimized multiplex immunofluorescence single-cell analysis reveals tuft cell heterogeneity. JCI Insight 2, e93487. doi:10.1172/jci.insight.93487
Mellitzer, G., Beucher, A., Lobstein, V., Michel, P., Robine, S., Kedinger, M., et al. (2010). Loss of enteroendocrine cells in mice alters lipid absorption and glucose homeostasis and impairs postnatal survival. J. Clin. Invest. 120, 1708–1721. doi:10.1172/JCI40794
Meyrick, B., and Reid, L. (1968). The alveolar brush cell in rat lung--a third pneumonocyte. J. Ultrastruct. Res. 23, 71–80. doi:10.1016/s0022-5320(68)80032-2
Middelhoff, M., Westphalen, C. B., Hayakawa, Y., Yan, K. S., Gershon, M. D., Wang, T. C., et al. (2017). Dclk1-expressing tuft cells: Critical modulators of the intestinal niche? Am. J. Physiol. Gastrointest. Liver Physiol. 313, G285–G299. doi:10.1152/ajpgi.00073.2017
Middelhoff, M., Nienhuser, H., Valenti, G., Maurer, H. C., Hayakawa, Y., Takahashi, R., et al. (2020). Prox1-positive cells monitor and sustain the murine intestinal epithelial cholinergic niche. Nat. Commun. 11, 111. doi:10.1038/s41467-019-13850-7
Miller, A. R., Martenson, J. A., Nelson, H., Schleck, C. D., Ilstrup, D. M., Gunderson, L. L., et al. (1999). The incidence and clinical consequences of treatment-related bowel injury. Int. J. Radiat. Oncol. Biol. Phys. 43, 817–825. doi:10.1016/s0360-3016(98)00485-4
Mori-Akiyama, Y., Van Den Born, M., Van Es, J. H., Hamilton, S. R., Adams, H. P., Zhang, J., et al. (2007). SOX9 is required for the differentiation of paneth cells in the intestinal epithelium. Gastroenterology 133, 539–546. doi:10.1053/j.gastro.2007.05.020
Nadjsombati, M. S., McGinty, J. W., Lyons-Cohen, M. R., Jaffe, J. B., DiPeso, L., Schneider, C., et al. (2018). Detection of succinate by intestinal tuft cells triggers a type 2 innate immune circuit. Immunity 49 (1), 33–41. doi:10.1016/j.immuni.2018.06.016
Nakanishi, Y., Seno, H., Fukuoka, A., Ueo, T., Yamaga, Y., Maruno, T., et al. (2013). Dclk1 distinguishes between tumor and normal stem cells in the intestine. Nat. Genet. 45, 98–103. doi:10.1038/ng.2481
Neurath, M. F., and Travis, S. P. (2012). Mucosal healing in inflammatory bowel diseases: A systematic review. Gut 61, 1619–1635. doi:10.1136/gutjnl-2012-302830
Neutra, M. R. (1998). Current concepts in mucosal immunity. V Role of M cells in transepithelial transport of antigens and pathogens to the mucosal immune system. Am. J. Physiol. 274, G785–G791. doi:10.1152/ajpgi.1998.274.5.G785
Noah, T. K., Kazanjian, A., Whitsett, J., and Shroyer, N. F. (2010). SAM pointed domain ETS factor (SPDEF) regulates terminal differentiation and maturation of intestinal goblet cells. Exp. Cell Res. 316 (3), 452–465. doi:10.1016/j.yexcr.2009.09.020
Ogura, T., Krosnowski, K., Zhang, L., Bekkerman, M., and Lin, W. (2010). Chemoreception regulates chemical access to mouse vomeronasal organ: Role of solitary chemosensory cells. PLoS One 5, e11924. doi:10.1371/journal.pone.0011924
Ooi, B. S., Tjandra, J. J., and Green, M. D. (1999). Morbidities of adjuvant chemotherapy and radiotherapy for resectable rectal cancer: An overview. Dis. Colon Rectum 42, 403–418. doi:10.1007/BF02236362
Osbourn, M., Soares, D. C., Vacca, F., Cohen, E. S., Scott, I. C., Gregory, W. F., et al. (2017). HpARI protein secreted by a helminth parasite suppresses interleukin-33. Immunity 47, 739–751. e5. doi:10.1016/j.immuni.2017.09.015
Pellegrinet, L., Rodilla, V., Liu, Z., Chen, S., Koch, U., Espinosa, L., et al. (2011). Dll1-and dll4-mediated notch signaling are required for homeostasis of intestinal stem cells. Gastroenterology 140, 1230–1240. e1-7. doi:10.1053/j.gastro.2011.01.005
Perez, C. A., Huang, L., Rong, M., Kozak, J. A., Preuss, A. K., Zhang, H., et al. (2002). A transient receptor potential channel expressed in taste receptor cells. Nat. Neurosci. 5, 1169–1176. doi:10.1038/nn952
Perniss, A., Liu, S., Boonen, B., Keshavarz, M., Ruppert, A. L., Timm, T., et al. (2020). Chemosensory cell-derived acetylcholine drives tracheal mucociliary clearance in response to virulence-associated formyl peptides. Immunity 52, 683–699. e11. doi:10.1016/j.immuni.2020.03.005
Philippaert, K., Pironet, A., Mesuere, M., Sones, W., Vermeiren, L., Kerselaers, S., et al. (2017). Steviol glycosides enhance pancreatic beta-cell function and taste sensation by potentiation of TRPM5 channel activity. Nat. Commun. 8, 14733. doi:10.1038/ncomms14733
Pineton De Chambrun, G., Peyrin-Biroulet, L., Lemann, M., and Colombel, J. F. (2010). Clinical implications of mucosal healing for the management of IBD. Nat. Rev. Gastroenterol. Hepatol. 7, 15–29. doi:10.1038/nrgastro.2009.203
Pinto, D., Gregorieff, A., Begthel, H., and Clevers, H. (2003). Canonical Wnt signals are essential for homeostasis of the intestinal epithelium. Genes. Dev. 17, 1709–1713. doi:10.1101/gad.267103
Popp, J. A., and Martin, J. T. (1984). Surface topography and distribution of cell types in the rat nasal respiratory epithelium: Scanning electron microscopic observations. Am. J. Anat. 169 (4), 425–436. doi:10.1002/aja.1001690405
Qu, D., Weygant, N., May, R., Chandrakesan, P., Madhoun, M., Ali, N., et al. (2015). Ablation of doublecortin-like kinase 1 in the colonic epithelium exacerbates dextran sulfate sodium-induced colitis. PLoS One 10, e0134212. doi:10.1371/journal.pone.0134212
Rea, T. H., Gottlieb, B., and Levan, N. E. (1975). Apparently normal skin in lepromatous leprosy: Histopathological findings. Arch. Dermatol 111, 1571–1574. doi:10.1001/archderm.111.12.1571
Reid, L., Meyrick, B., Antony, V. B., Chang, L. Y., Crapo, J. D., and Reynolds, H. Y. (2005). The mysterious pulmonary brush cell: A cell in search of a function. Am. J. Respir. Crit. Care Med. 172, 136–139. doi:10.1164/rccm.200502-203WS
Rhodin, J., and Dalhamn, T. (1956). Electron microscopy of the tracheal ciliated mucosa in rat. Z Zellforsch Mikrosk Anat. 44, 345–412. doi:10.1007/BF00345847
Riccio, O., Van Gijn, M. E., Bezdek, A. C., Pellegrinet, L., Van Es, J. H., Zimber-Strobl, U., et al. (2008). Loss of intestinal crypt progenitor cells owing to inactivation of both Notch1 and Notch2 is accompanied by derepression of CDK inhibitors p27Kip1 and p57Kip2. EMBO Rep. 9, 377–383. doi:10.1038/embor.2008.7
Roda, G., Chien Ng, S., Kotze, P. G., Argollo, M., Panaccione, R., Spinelli, A., et al. (2020). Crohn's disease. Nat. Rev. Dis. Prim. 6, 22. doi:10.1038/s41572-020-0156-2
San Roman, A. K., Jayewickreme, C. D., Murtaugh, L. C., and Shivdasani, R. A. (2014). Wnt secretion from epithelial cells and subepithelial myofibroblasts is not required in the mouse intestinal stem cell niche in vivo. Stem Cell. Rep. 2, 127–134. doi:10.1016/j.stemcr.2013.12.012
Sancho, R., Cremona, C. A., and Behrens, A. (2015). Stem cell and progenitor fate in the mammalian intestine: Notch and lateral inhibition in homeostasis and disease. EMBO Rep. 16, 571–581. doi:10.15252/embr.201540188
Saqui-Salces, M., Keeley, T. M., Grosse, A. S., Qiao, X. T., El-Zaatari, M., Gumucio, D. L., et al. (2011). Gastric tuft cells express DCLK1 and are expanded in hyperplasia. Histochem Cell. Biol. 136, 191–204. doi:10.1007/s00418-011-0831-1
Sato, A., and Miyoshi, S. (1988). Ultrastructure of the main excretory duct epithelia of the rat parotid and submandibular glands with a review of the literature. Anat. Rec. 220 (3), 239–251. doi:10.1002/ar.1092200304
Sbarbati, A., Bramanti, P., Benati, D., and Merigo, F. (2010). The diffuse chemosensory system: Exploring the iceberg toward the definition of functional roles. Prog. Neurobiol. 91 (1), 77–89. doi:10.1016/j.pneurobio.2010.01.010
Sbarbati, A., and Osculati, F. (2005). A new fate for old cells: Brush cells and related elements. J. Anat. 206, 349–358. doi:10.1111/j.1469-7580.2005.00403.x
Schutz, B., Jurastow, I., Bader, S., Ringer, C., von Engelhardt, J., Chubanov, V., et al. (2015). Chemical coding and chemosensory properties of cholinergic brush cells in the mouse gastrointestinal and biliary tract. Front. Physiol. 6, 87. doi:10.3389/fphys.2015.00087
Schwitalla, S., Fingerle, A. A., Cammareri, P., Nebelsiek, T., Goktuna, S. I., Ziegler, P. K., et al. (2013). Intestinal tumorigenesis initiated by dedifferentiation and acquisition of stem-cell-like properties. Cell. 152, 25–38. doi:10.1016/j.cell.2012.12.012
Sharpe, C., Thornton, D. J., and Grencis, R. K. (2018). A sticky end for gastrointestinal helminths; the role of the mucus barrier. Parasite Immunol. 40, e12517. doi:10.1111/pim.12517
Shen, Q., Goderie, S. K., Jin, L., Karanth, N., Sun, Y., Abramova, N., et al. (2004). Endothelial cells stimulate self-renewal and expand neurogenesis of neural stem cells. Science 304, 1338–1340. doi:10.1126/science.1095505
Shroyer, N. F., Helmrath, M. A., Wang, V. Y. C., Antalffy, B., Henning, S. J., and Zoghbi, H. Y. (2007). Intestine-specific ablation of mouse atonal homolog 1 (Math1) reveals a role in cellular homeostasis. Gastroenterology 132 (7), 2478–2488. doi:10.1053/j.gastro.2007.03.047
Silva, D. G. (1966). The fine structure of multivesicular cells with large microvilli in the epithelium of the mouse colon. J. Ultrastruct. Res. 16, 693–705. doi:10.1016/s0022-5320(66)80015-1
Strober, W., and Fuss, I. J. (2011). Proinflammatory cytokines in the pathogenesis of inflammatory bowel diseases. Gastroenterology 140, 1756–1767. doi:10.1053/j.gastro.2011.02.016
Stumhofer, J. S., Laurence, A., Wilson, E. H., Huang, E., Tato, C. M., Johnson, L. M., et al. (2006). Interleukin 27 negatively regulates the development of interleukin 17-producing T helper cells during chronic inflammation of the central nervous system. Nat. Immunol. 7, 937–945. doi:10.1038/ni1376
Su, J., Chen, T., Ji, X. Y., Liu, C., Yadav, P. K., Wu, R., et al. (2013). IL-25 downregulates Th1/Th17 immune response in an IL-10-dependent manner in inflammatory bowel disease. Inflamm. Bowel Dis. 19, 720–728. doi:10.1097/MIB.0b013e3182802a76
Summers, R. W., Elliott, D. E., Urban, J. F., Thompson, R., and Weinstock, J. V. (2005). Trichuris suis therapy in Crohn's disease. Gut 54, 87–90. doi:10.1136/gut.2004.041749
Sureban, S. M., May, R., Ramalingam, S., Subramaniam, D., Natarajan, G., Anant, S., et al. (2009). Selective blockade of DCAMKL-1 results in tumor growth arrest by a Let-7a MicroRNA-dependent mechanism. Gastroenterology 137, 649–659. 659 e1-2. doi:10.1053/j.gastro.2009.05.004
Sutherland, K., Young, R. L., Cooper, N. J., Horowitz, M., and Blackshaw, L. A. (2007). Phenotypic characterization of taste cells of the mouse small intestine. Am. J. Physiol. Gastrointest. Liver Physiol. 292, G1420–G1428. doi:10.1152/ajpgi.00504.2006
Teunis, P. F., Sukhrie, F. H., Vennema, H., Bogerman, J., Beersma, M. F., and Koopmans, M. P. (2015). Shedding of norovirus in symptomatic and asymptomatic infections. Epidemiol. Infect. 143, 1710–1717. doi:10.1017/S095026881400274X
Tomic, G., Morrissey, E., Kozar, S., Ben-Moshe, S., Hoyle, A., Azzarelli, R., et al. (2018). Phospho-regulation of ATOH1 is required for plasticity of secretory progenitors and tissue regeneration. Cell. Stem Cell. 23, 436–443. doi:10.1016/j.stem.2018.07.002
Tomov, V. T., Palko, O., Lau, C. W., Pattekar, A., Sun, Y., Tacheva, R., et al. (2017). Differentiation and protective capacity of virus-specific CD8(+) T cells suggest murine norovirus persistence in an immune-privileged enteric niche. Immunity 47, 723–738. doi:10.1016/j.immuni.2017.09.017
Tsubouchi, S., and Leblond, C. P. (1979). Migration and turnover of entero-endocrine and caveolated cells in the epithelium of the descending colon, as shown by radioautography after continuous infusion of 3H-thymidine into mice. Am. J. Anat. 156, 431–451. doi:10.1002/aja.1001560403
Van Es, J. H., and Clevers, H. (2005). Notch and Wnt inhibitors as potential new drugs for intestinal neoplastic disease. Trends Mol. Med. 11, 496–502. doi:10.1016/j.molmed.2005.09.008
Van Es, J. H., de Geest, N., van de Born, M., Clevers, H., and Hassan, B. A. (2010). Intestinal stem cells lacking the Math1 tumour suppressor are refractory to Notch inhibitors. Nat. Commun. 1, 18. doi:10.1038/ncomms1017
Van Es, J. H., Van Gijn, M. E., Riccio, O., Van Den Born, M., Vooijs, M., Begthel, H., et al. (2005). Notch/gamma-secretase inhibition turns proliferative cells in intestinal crypts and adenomas into goblet cells. Nature 435, 959–963. doi:10.1038/nature03659
Vandussen, K. L., Carulli, A. J., Keeley, T. M., Patel, S. R., Puthoff, B. J., Magness, S. T., et al. (2012). Notch signaling modulates proliferation and differentiation of intestinal crypt base columnar stem cells. Development 139, 488–497. doi:10.1242/dev.070763
Vega, K. J., May, R., Sureban, S. M., Lightfoot, S. A., Qu, D., Reed, A., et al. (2012). Identification of the putative intestinal stem cell marker doublecortin and CaM kinase-like-1 in Barrett's esophagus and esophageal adenocarcinoma. J. Gastroenterol. Hepatol. 27, 773–780. doi:10.1111/j.1440-1746.2011.06928.x
Von Moltke, J., Ji, M., Liang, H. E., and Locksley, R. M. (2016). Tuft-cell-derived IL-25 regulates an intestinal ILC2-epithelial response circuit. Nature 529, 221–225. doi:10.1038/nature16161
Wattel, W., and Geuze, J. J. (1978). The cells of the rat gastric groove and cardia. An ultrastructural and carbohydrate histochemical study, with special reference to the fibrillovesicular cells. Cell Tissue Res. 186 (3), 375–391. doi:10.1007/BF00224928
Westphalen, C. B., Asfaha, S., Hayakawa, Y., Takemoto, Y., Lukin, D. J., Nuber, A. H., et al. (2014). Long-lived intestinal tuft cells serve as colon cancer-initiating cells. J. Clin. Invest. 124, 1283–1295. doi:10.1172/JCI73434
Weygant, N., Qu, D., May, R., Tierney, R. M., Berry, W. L., Zhao, L., et al. (2015). DCLK1 is a broadly dysregulated target against epithelial-mesenchymal transition, focal adhesion, and stemness in clear cell renal carcinoma. Oncotarget 6, 2193–2205. doi:10.18632/oncotarget.3059
Weyrauch, K. D. (1979). Ultrastructure of the tuft cell in some epithelia of the domestic ruminants (author’s transl). Anat. Anz. 146 (2), 141–151.
Wilen, C. B., Lee, S., Hsieh, L. L., Orchard, R. C., Desai, C., Hykes, B. L., et al. (2018). Tropism for tuft cells determines immune promotion of norovirus pathogenesis. Science 360, 204–208. doi:10.1126/science.aar3799
Xiong, Z., Zhu, X., Geng, J., Xu, Y., Wu, R., Li, C., et al. (2022). Intestinal Tuft-2 cells exert antimicrobial immunity via sensing bacterial metabolite N-undecanoylglycine. Immunity 55, 686–700.e7. doi:10.1016/j.immuni.2022.03.001
Yang, Q., Bermingham, N. A., Finegold, M. J., and Zoghbi, H. Y. (2001). Requirement of Math1 for secretory cell lineage commitment in the mouse intestine. Science 294, 2155–2158. doi:10.1126/science.1065718
Yeoh, E., Razali, M., and O'Brien, P. C. (1993). Radiation therapy for early stage seminoma of the testis. Analysis of survival and gastrointestinal toxicity in patients treated with modern megavoltage techniques over 10 years. Australas. Radiol. 37, 367–369. doi:10.1111/j.1440-1673.1993.tb00097.x
Yi, J., Bergstrom, K., Fu, J., Shan, X., Mcdaniel, J. M., Mcgee, S., et al. (2019). Dclk1 in tuft cells promotes inflammation-driven epithelial restitution and mitigates chronic colitis. Cell. Death Differ. 26, 1656–1669. doi:10.1038/s41418-018-0237-x
Young, S. N. (2007). How to increase serotonin in the human brain without drugs. J. Psychiatry Neurosci. 32, 394–399.
Yui, S., Nakamura, T., Sato, T., Nemoto, Y., Mizutani, T., Zheng, X., et al. (2012). Functional engraftment of colon epithelium expanded in vitro from a single adult Lgr5⁺ stem cell. Nat. Med. 18, 618–623. doi:10.1038/nm.2695
Glossary
TC Tuft cell
GC Goblet cell
RSEM Reflection scanning electron microscopy
TEM Transmission electron microscopy
SEM Scanning Electron Microscopy
SBF Serial Block-Face
ATUM Automated Tape-collecting Ultra-Microtome
IEC Intestinal epithelial cell
Lgr5 Leucine rich repeat containing G protein-coupled receptor 5
HES1 Hes family bHLH transcription factor 1
ATOH1 Atonal bHLH transcription factor 1
CBC Crypt basal columnar
ISCs Intestinal stem cells
IEC Intestinal epithelial cell
TA cells Transit amplifying cells (Mix)
M cell membranous cell
Dll1 Delta like canonical Notch ligand 1
Dll4 Delta like canonical Notch ligand 4
ErbB Erb-b2 receptor tyrosine kinase
EGF Epidermal growth factor
BMP Bone morphogenetic protein
Tcf4 Transcription factor 4
Hes Hes family bHLH transcription factor
Gfi1b Growth factor independent 1B transcriptional repressor
Atoh1 Atonal homologue 1 gene, also known as Math1
ADAMs a Disintegrin and Metalloproteinases
ADAM10 ADAM metallopeptidase domain 10
Spdef SAM pointed domain containing Ets transcription factor
Klf4 Kruppel-like factor 4
Sox9 SRY-box containing gene 9
Neurog3 Neurogenin 3
Gfi1 Growth factor-independent 1
TRPM5 Transient receptor potential cation channel, and subfamily M, member 5
COX1 Cyclooxygenase 1
COX2 Cyclooxygenase 2
DCLK1 Doublecortin like kinase 1
SUCNR1 Succinate receptor 1
HPGDS Hematopoietic prostaglandin-D synthase
5HT Serotonin
IL-25 Interleukin 25
TSLP thymic stromal lymphopoietin
NO Nitric oxide
GNAT3 Guanine nucleotide binding protein alpha transducing 3/α-gustducin
ILC2 Group 2 innate lymphoid cell
HpARI H. polygyrus alarmin released inhibitor
IL-33 Interleukin 33
cysLTs cysteinyl leukotrienes
IL-4 Interleukin 4
IL-13 Interleukin 13
ACh Acetylcholine
GPCR G protein-coupled receptor
PLC Phospholipase C
PGD2 prostaglandin D2
MNV murine norovirus
CD300lf CD300 molecule like family member 1
UC Ulcerative colitis
CD Crohn’s disease
PRR Pattern recognition receptors
TNF-α Tumor necrosis factor-α
GABA γ-aminobutyric acid
IL17RB Interleukin 17 receptor B
NF-κB Nuclear factor kappa B subunit 1
CSCs cancer stem cells
IBD inflammatory bowel disease
GI Gastrointestinal
DOM Daughters of TA cells/daughters of Mix
DOMNotch Absoprive progenitor
DOMΔ Secretory progenitor
NK cells Natural killer cells
HCC Hepatocellular carcinoma
Keywords: tuft cell, intestinal epithelial cell, type 2 immune response, helminth infection, tumor
Citation: Du Y, Gao H, He C, Xin S, Wang B, Zhang S, Gong F, Yu X, Pan L, Sun F, Wang W and Xu J (2023) An update on the biological characteristics and functions of tuft cells in the gut. Front. Cell Dev. Biol. 10:1102978. doi: 10.3389/fcell.2022.1102978
Received: 23 November 2022; Accepted: 27 December 2022;
Published: 10 January 2023.
Edited by:
Yuyong Tan, The second Xiangya Hospital, Central South University, ChinaReviewed by:
Jun Yi, Xiangya Hospital, Central South University, ChinaPeihua Jiang, Monell Chemical Senses Center, United States
Copyright © 2023 Du, Gao, He, Xin, Wang, Zhang, Gong, Yu, Pan, Sun, Wang and Xu. This is an open-access article distributed under the terms of the Creative Commons Attribution License (CC BY). The use, distribution or reproduction in other forums is permitted, provided the original author(s) and the copyright owner(s) are credited and that the original publication in this journal is cited, in accordance with accepted academic practice. No use, distribution or reproduction is permitted which does not comply with these terms.
*Correspondence: Jingdong Xu, eHVfamRkQGNjbXUuZWR1LmNu