- 1Jilin Provincial Key Laboratory of Radiation Oncology and Therapy, The First Hospital of Jilin University, and Key Laboratory of Pathobiology, Ministry of Education, Jilin University, Changchun, China
- 2Key Laboratory of Pathobiology, Ministry of Education, Jilin University, Changchun, China
- 3Department of Radiation Oncology, The First Hospital of Jilin University, Changchun, China
- 4NHC Key Laboratory of Radiobiology, School of Public Health, Jilin University, Changchun, China
Radiotherapy (RT) is an effective treatment option for multiple thoracic malignant tumors, including lung cancers, thymic cancers, and tracheal cancers. Radiation-induced lung injury (RILI) is a serious complication of radiotherapy. Radiation causes damage to the pulmonary cells and tissues. Multiple factors contribute to the progression of Radiation-induced lung injury, including genetic alterations, oxidative stress, and inflammatory responses. Especially, radiation sources contribute to oxidative stress occurrence by direct excitation and ionization of water molecules, which leads to the decomposition of water molecules and the generation of reactive oxygen species (ROS), reactive nitrogen species (RNS). Subsequently, reactive oxygen species and reactive nitrogen species overproduction can induce oxidative DNA damage. Immune cells and multiple signaling molecules play a major role in the entire process. Mesenchymal stem cells (MSCs) are pluripotent stem cells with multiple differentiation potentials, which are under investigation to treat radiation-induced lung injury. Mesenchymal stem cells can protect normal pulmonary cells from injury by targeting multiple signaling molecules to regulate immune cells and to control balance between antioxidants and prooxidants, thereby inhibiting inflammation and fibrosis. Genetically modified mesenchymal stem cells can improve the natural function of mesenchymal stem cells, including cellular survival, tissue regeneration, and homing. These reprogrammed mesenchymal stem cells can produce the desired products, including cytokines, receptors, and enzymes, which can contribute to further advances in the therapeutic application of mesenchymal stem cells. Here, we review the molecular mechanisms of radiation-induced lung injury and discuss the potential of Mesenchymal stem cells for the prevention and treatment of radiation-induced lung injury. Clarification of these key issues will make mesenchymal stem cells a more fantastic novel therapeutic strategy for radiation-induced lung injury in clinics, and the readers can have a comprehensive understanding in this fields.
1 Introduction
Radiotherapy (RT) is an effective treatment option for thoracic malignancies. Radiation-induced lung injury (RILI) is a serious complication of RT. RILI consists of two stages: the acute phase, usually manifesting as radiation pneumonitis (RP), and the chronic phase, manifesting as radiation pulmonary fibrosis (RPF). The symptoms of RILI are mainly dry cough, severe dyspnea, and respiratory failure, resulting in death (Rajan Radha and Chandrasekharan, 2017; Hanania et al., 2019). According to reports, 5%–20% of patients will experience dyspnea after RT, and pulmonary function tests often shows significant subclinical reduction. RT for cancer of the lung, mediastinal lymphatics, and breast exhibits different incident rate of RILI, respectively 5%–50%, 5%–10%, and 1%–5% (Marks et al., 2010; Zhang et al., 2010). Higher radiation dose, older age and larger tumor diameter are significant adverse risk of RILI. The therapeutic dose of radiation that can be given in effectively control cancer are restricted in patients due to RILI. So, the patients with RILI have poor prognosis (Kong et al., 2021). RILI is concerning because of its high incidence and potentially severe consequences. Multiple factors contribute to the progression of RILI, including genetic alterations, oxidative stress, and inflammatory responses. Immune cells and multiple signaling molecules play a major role in the entire process of RILI. The current therapies for RILI lack treatment plans and guidelines.
Mesenchymal stem cells (MSCs), as cell-based treatments, have the potential to treat RILI. MSCs are pluripotent stem cells that have multiple differentiation potentials and are derived from various tissues (Samsonraj et al., 2017). MSCs have been successfully isolated from many tissues, particularly marrow-derived MSCs, which are frequently used and have established criteria for characterization (Pittenger et al., 1999). MSCs can interact with cells of the innate and acquired immune systems and migrate to injured tissues, where they can inhibit the release of pro-inflammatory cytokines and promote the survival of damaged cells by regulating signaling molecules (Uccelli et al., 2008). Owing to their ability to regulate signaling, researchers are interested in using MSCs to treat RILI.
2 Changes in lung tissue in RILI
The lung parenchyma is composed of the respiratory bronchioles, alveolar ducts, and alveoli. The alveolar stroma is composed of fibroblasts, alveolar macrophages, and the extracellular matrix (ECM). When exposed to radiation, these tissues and cells of the lung suffer damage in genes, which is considered a direct effect of radiation. Ionizing radiation can disrupt atomic structures, damage normal DNA or RNA sequences, and affect biological functions (Liu et al., 2021). Another significant change is the radiation-induced water radiolysis. The water in cells and tissues can absorb radiation and energy. The process results in excitation and ionization of water, which leads to the generation of reactive oxygen species (ROS) and reactive nitrogen species (RNS) (Azzam et al., 2012). ROS and RNS are major damage factors that can influence the normal conditions of cells and tissues. Moreover, the expression of genes related to oxidation and anti-oxidation can be affected.
Under the influence of these initial damaging factors, multiple cellular events occur. Mitotic catastrophe and apoptosis lead to endothelial cell death mainly by activating p53 or by the sphingomyelin ceramide pathway. Subsequently, apoptosis causes the release of damage-associated molecular patterns and activation of the systemic inflammatory response (Wijerathne et al., 2021). Radiation can also cause cellular senescence. Induction to senescence is considered as an important mechanism in the treatment of cancer by RT, since it causes permanent growth arrest of DNA-damaged cells preventing their reproduction and stimulates the immune system to rapidly eliminate these genetically unstable cells. However, in addition to cancer cells, normal tissue cells in the lungs are also significantly affected by cell senescence, and the excessive secretion of senescence-associated secretion phenotype causes subsequent damage (He et al., 2019). Some recent studies have found that radiation can induced ferroptosis by promoting lipid peroxidation (Lei et al., 2020a).
These changes lead to a subsequent inflammatory response. Inflammatory cells release large amounts of signaling molecules, leading to severe inflammation and immune system disorders (Giuranno et al., 2019; Wei et al., 2021). Subsequently, lung tissue fibrosis progresses with an increase in cytokine levels and the expression of collagen genes. These continuous stress factors go beyond the usual regulatory range of cellular and organismal homeostasis, eventually leading to pulmonary fibrosis (Petkau, 1987). This review elaborates on the progress of cells and organs and the adjustment of signaling molecules and immune systems in RILI (Figures 1, 2).
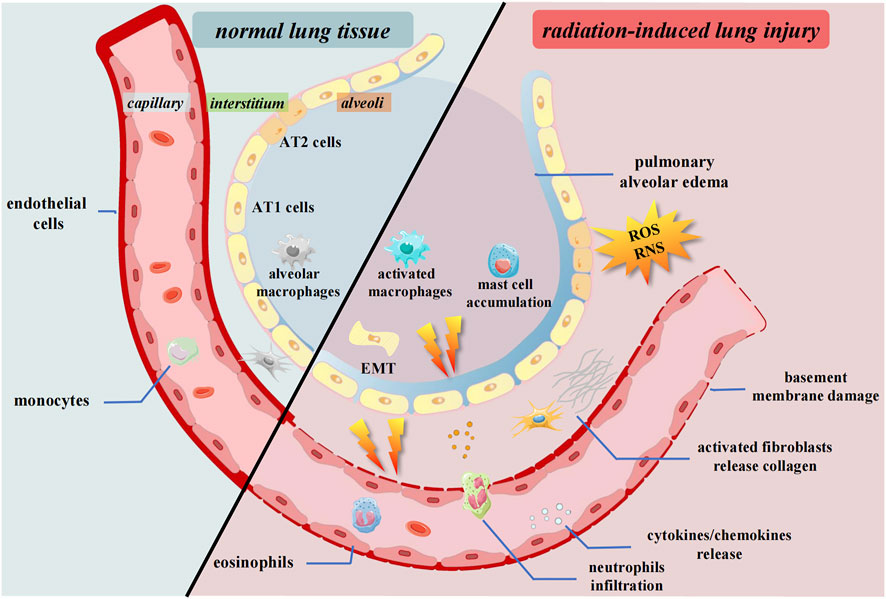
FIGURE 1. Pulmonary tissue changes after irradiation. Radiation causes the release of cytokines and chemokines. The inflammatory cells and fibrosis associated cells are activated and infiltrate to interstitium. ROS and RNS accumulate in the microenvironment. The epithelium undergoes EMT process. AT1 cells, Alveolar type 1 cells; AT2 cells, Alveolar type 2 cells; ROS, Reactive oxygen species; RNS, Reactive nitrogen species; EMT, Epithelial-mesenchymal transition.
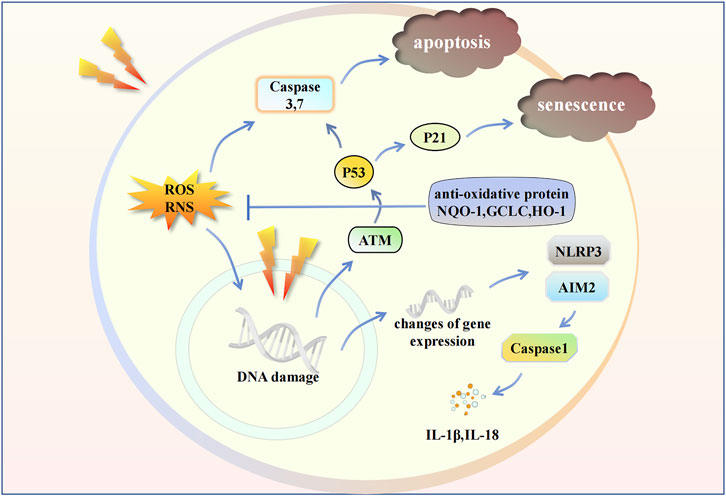
FIGURE 2. Radiation influences some intracellular signaling pathways in endothelial cell. Radiation leads to the changes in gene expression and damage in gene structure. ROS and RNS cause apoptosis. Inflammasomes induce the release of cytokines. The activation of ATM/P53/P21 signaling pathway cause cell senescence. IL, Interleukin; ATM, Ataxia telangiectasia mutated; P21, Cyclin-dependent kinase inhibitor 1A; NQO-1, NAD(P)H Quinone Dehydrogenase 1; GCLC, Glutamate-Cysteine Ligase Catalytic Subunit; HO-1, Heme oxygenase-1.
2.1 Changes in genes and expression
Radiation leads to direct DNA damage, including base deletions, and single- and double-strand breaks (Yan et al., 2022). The first protein activated by the DNA damage response is “ataxia telangiectasia mutated,” which can activate downstream P53 and P21, inducing cell senescence (Lei et al., 2020b). Moreover, DNA damage contributes to the inflammatory microenvironment and stimulates signaling molecules such as type 1 interferon (IFN) and interleukin (IL)-1 (Wang et al., 2020; Cinat et al., 2021). Radiation also inhibits transcriptional regulation, including mRNA processing, DNA-dependent regulation of transcription, chromatin modification, and RNA splicing (Mathew et al., 2011). Changes in the quantity and quality of gene expression may be the earliest events in the body’s response to radiation and this variation leads to subsequent biological processes (Thakur et al., 2021). Such genes are associated with organismal injury, cellular movement, immune cell trafficking, and inflammatory response. The upregulated genes were mainly SERPINA3, ATP12A, LRRN4, GJB2, and CLDN10, while the downregulated genes were PNMT, TOX3, FAM107A, RXRG, and LPA. The major upstream regulators of these genes are associated with angiotensinogen, IL-1, and transforming growth factor-β (TGF-β), indicating that these molecules play key roles in the progression of RILI. Radiation also causes discrepancies in circRNAs and miRNAs in contrast to normal pulmonary tissues (Li et al., 2021). The main target mRNAs of these differentially expressed circRNAs and miRNAs are related to T cell proliferation, chemokine receptor activity, and the B-cell receptor signaling pathway. Thus, the migration of inflammatory cells and the release of cytokines, along with the immune response, play a critical role after radiation. Although most current studies are based on mouse models, there are some studies on RILI in non-human primate (NHP) models, which are much similar to human than rodents. The microarray expression profiling of NHP models shows that the differentially expressed genes are mainly related to expression of inflammatory cytokines, complement factors, apoptosis-related molecules (Cui et al., 2021).
2.2 Changes in oxidation and anti-oxidation
The continuous production of ROS after RT affects the homeostasis of progeny cells and bystander cells, resulting in a wide range of oxidative damage, including protein carbonylation and lipid peroxidation, which increases the chance of spontaneous gene mutations and neoplastic transformation (Buonanno et al., 2011a; Buonanno et al., 2011b; Shen et al., 2018). These biological processes primarily damage genes and cause cellular dysfunctions. ROS can lead to the generation of oxidative lipids in the pulmonary endothelial cells. Oxygenated lipids in endothelial cells, as major biomarkers, can reflect the damage process of damage. It also correlates with signaling pathways involved in cell proliferation, apoptosis, programmed cell death, inflammation, and immune surveillance (Tang et al., 2002; Cole et al., 2003; Greenberg et al., 2006; Haworth and Levy, 2007). Research has shown that cardiolipin and phosphatidylserine are the main phospholipids that are significantly oxidized (Tyurina et al., 2011). Caspase 3/7 activity is accompanied by the degree of oxidation of cardiolipin and phosphatidylserine after radiation. These oxidative phospholipids may trigger apoptosis. Apart from the influence of ROS, some major oxidative damage of RILI comes from RNS, which includes nitric oxide (NO) and peroxynitrite. NO is a free radical that can chain react with superoxide, producing the potent oxidizing agent peroxynitrite. NO and peroxynitrite can induce lipid peroxidation in biological membranes, nitration, and hydroxylation of aromatic acid residues, and sulfhydryl oxidation of proteins (Giaid et al., 2003). They mainly influence the alveolar epithelial cells and specific surfactant apoproteins. When radiation-induced oxidative stress damage occurs, the body must enhance its antioxidant biological function to defend itself against such damage. Nuclear factor E2-related factor 2 (Nrf2) is an effective cellular antioxidant protein that triggers a cascade of downstream antioxidant signals. Nrf2 is upregulated after irradiation and increases the expression of antioxidant response elements, which mediate phase II antioxidant enzymes in pulmonary tissue (Lee et al., 2007; Mathew et al., 2013). Genes related to antioxidant function are activated, including NQO1, GCLC, HO–1, Gpx1, Prdx1, and Txn1. Recently, researchers discovered that the gene MIF and its encoding molecule, macrophage migratory inhibition factor, a multifunctional pleiotropic cytokine, had a positive relationship with Nrf2 and downstream antioxidized enzymes (Mathew et al., 2013).
2.3 Changes in cytokines, inflammasomes, and immune cells
When the lung tissue is irradiated, an inflammatory response occurs. The alveolar epithelium and vascular endothelium are damaged. Simultaneously, due to increased microvascular permeability, immune cells are recruited to the injured site by chemokines. Many immune cells are activated to produce inflammatory signaling molecules, which lead to the initiation of additional paracrine and autocrine loops between several cells (Hanania et al., 2019).
Cytokines, as the most important biomarkers, are considered to predict the progression of RILI. Radiation can cause changes in multiple cytokines, including IL, IFN-γ, TGF-β, tumor necrosis factor-α (TNF-α), granulocyte colony-stimulating factor (G-CSF), and granulocyte macrophage colony-stimulating factor (GM-CSF). Most of these cytokines are associated with RILI (Ao et al., 2009). Inflammatory cytokines are key mediators of the pathogenesis of RP. The levels of pro-inflammatory cytokines in the serum, such as IL-1α and IL-6, are significantly elevated during all stages of RILI. Subsequently, the same changes in IL-6 were confirmed in bronchoalveolar lavage fluid (Chen et al., 2001). IL-7, IL-12, IL-15, IL-16 were also found to be significantly changed at different time points after radiation in NHP model (Cui et al., 2020). These changes in cytokines can persist for several months after completing RT, which illustrates that these signaling molecule events extend the damage response (Barthelemy-Brichant et al., 2004). Radiation can elevate TNF-α expression in lung tissue (Rübe et al., 2002). TNF-α, as a proinflammatory cytokine, can stimulate the expression of adhesion molecules to recruit leukocytes and induce the production of prostaglandins and other mediators of inflammation. Besides inflammatory cytokines, other cytokines also vary prominently during RILI. Radiation can upregulate TGF-β expression, leading to RILI progression (Rube et al., 2000). TGF-β plays a significant role in regulating inflammatory cell infiltration, collagen deposition, autophagy, apoptosis, and EMT, and is a key molecule in the process of fibrogenesis in cells and ECM (Ikushima and Miyazono, 2010; Eser and Jänne, 2018). TGF-β, an upstream regulator, can regulate many molecules in the TGF-β/Smad pathway, such as α-smooth muscle actin, collagen I, and tissue inhibitors of matrix metalloproteinases (Meng et al., 2016). Multiple regulators targeting TGF-β can alleviate RILI (Gauter-Fleckenstein et al., 2010; Xue et al., 2013; Kim et al., 2020; Ying et al., 2021). Lipoxin A4, a bioactive product of arachidonic acid, can target the TGF-β/Smad pathway to exert anti-inflammatory and antifibrotic properties in RILI (Kim et al., 2020). Pirfenidone and MnTE-2-PyP5+ also alleviated RILI by regulating TGF-β (Gauter-Fleckenstein et al., 2010; Ying et al., 2021). G-CSF and GM-CSF levels in the lungs are elevated after radiation (Ao et al., 2009). G-CSF is responsible for activating and recruiting neutrophils to the lung, which may contribute to pulmonary damage (Gandhi et al., 2019). GM-CSF functions similar to G-CSF and stimulates the activation and proliferation of macrophages, which prompts the progression of inflammation (Shiomi and Usui, 2015). However, some researchers believe that GM-CSF exerts a protective effect against RILI. GM-CSF can maintain normal pulmonic homeostasis and it is essential for biological immunity processes (Hu et al., 2020). Hence, its role in RILI is not yet well understood. Chemokines also influence RILI. Chemokine C-C motif ligand (CCL) is associated with epithelial senescence and vascular dysfunction after radiation (Wiesemann et al., 2019). Radiation increases CCL2 levels, which contributes to the immune regulation and migration of myeloid cells. In addition, thoracic irradiation caused CCL3 and CCL8 production in vivo. These chemokines bind to CC chemokine receptors (CCR), which promote injury, inflammation, and mortality by driving leukocyte recruitment (Yang et al., 2011; Kim et al., 2020).
Inflammasomes have recently been found to play a crucial role in RILI. Inflammasomes can influence the release of certain cytokines. One such inflammasome, AIM2, can sense potentially dangerous cytoplasmic DNA with its oligonucleotide/oligosaccharide-binding domain, and it can interact with apoptosis-associated speck-like protein through its amino-terminal pyrin domain to activate caspase-1 (Fernandes-Alnemri et al., 2009). A study found that radiation caused AIM2 translocation to the nucleus to sense damaged double-stranded DNA, followed by AIM2 oligomerization, assembly, and activation (Gao et al., 2019). Subsequently, caspase-1 is then activated, and IL-1β is released abundantly, triggering inflammation. Some researchers have revealed that the NLRP3 inflammasome is increased by irradiation. Subsequently, mature cleaved capase-1 was significantly upregulated and the proportion of IL-1β and IL-18 was dramatically elevated, which led to a more severe inflammatory response in the later stage (Wu et al., 2019).
Radiation leads to increases not only in the total immune cell count, but also in the category and quantity of immune cells undergo a series of changes. Immune cells, including polymorphonuclear leukocytes, lymphocytes, macrophages, and mast cells, accumulate in alveoli after radiation (Majori et al., 2000; Cappuccini et al., 2011; Kunwar et al., 2013). Interstitial macrophages decreased, while alveolar macrophages significantly increased, indicating macrophage migration. Macrophages can transform into activated macrophages with the pro-inflammatory M1 phenotype. Massive infiltration of activated neutrophils in the lungs induces thickened alveolar septa (Abernathy et al., 2015), and the total number of activated T lymphocytes increases continuously. Radiation also affects the composition of the T lymphocyte subsets recruited to the lungs. The proportions of T helper (Th) 17 cells and regulatory T cells (Tregs) increase greatly day 21 post irradiation (Cappuccini et al., 2011). Tregs are thought to possess different functions in the RP and RPF stages. Tregs are recruited by CCL2 and inhibit inflammatory responses in RP. However, Tregs contribute to RPF through several mechanisms, including promotion of fibrocyte accumulation and EMT, modulation of the Th1/Th2 balance, and suppression of Th17 responses (Guo et al., 2020).
2.4 Changes in tissue composition and organ function
Changes in elements of tissues often occur after radiation exposure. Alveolar type 1 (AT1) cells on the alveolar surface undergo apoptosis after radiation, while alveolar type 2 (AT2) cells accelerate proliferation to fill the defect. Moreover, the proliferation of AT2 cells and an unbalanced AT1:AT2 ratio leads to more pulmonary surfactant being produced. Exposing epithelial cells to radiation results in the rapid induction of a stress response in which pro-inflammatory cytokines are produced and immunological reactions take place in the airways (Rübe et al., 2005). Radiation activates the expression of fibrosis-related genes, resulting in changes in ECM composition, especially increased collagen (Lei et al., 2020b). In normal situations, the ECM of the alveolar wall is mainly composed of proteoglycans, fibronectin, laminin, entactin, and types IV and VII collagens. When exposed to radiation, these proportions will change in quality and quantity. For example, type I/III/IV collagens increase significantly, especially type IV collagen (Tsoutsou and Koukourakis, 2006). Growing evidence suggests that radiation can prompt alveolar epithelial cells to undergo epithelial-mesenchymal transition (EMT) (Kim et al., 2006). EMT is considered crucial to the pathogenesis of organ fibrosis (Hewlett et al., 2018; Wang et al., 2020). Epithelial cells acquire mesenchymal-like properties essential for the progressive accumulation of collagen and extracellular matrix proteins, ultimately leading to lung fibrosis. Furthermore, endothelial cells are affected by pulmonary dysfunction. The pulmonary endothelial cells as protective barriers of blood-vessel walls are prominently lost, and the basement membrane of endothelial cells ruptures at an early stage (Ghobadi et al., 2012). Owing to the enlarged endothelial gap, inflammatory infiltrate diffusely expands resulting in abnormal lung radiation density on CT scans (Thakur et al., 2021). Endothelial cells serve as a regulator of normal physiological function for their inducible metabolic and synthetic function. With endothelial cell loss, the medial layer becomes muscularized, the adventitia becomes thicker, and damage to the neointima occurs. This indicates the pulmonary vasculature is remodeled during RILI. Irradiation often induces radiation dose-dependent pulmonary hypertension due to changes in the pulmonary vessels. Respiration function is impaired by radiation; in particular, the pulmonary diffusion capacity decreases significantly (Elliott et al., 2019). These changes in normal pulmonary physiology are associated with the clinical symptoms of RILI.
3 MSCs alleviate RILI
Current treatments are not effective for RILI, and have many potential side effects. The treatments of RPF are primarily supportive, such as supplemental oxygen to relieve the symptoms (Hanania et al., 2019). The current clinical treatment of RP mainly involves high doses of corticosteroids and other anti-inflammatory drugs (Bledsoe et al., 2017). However, these treatments have severe problems, including inefficiency, recurrence of symptoms, and complications due to use of steroids (Sekine et al., 2006; Grennan and Wang, 2019). Several combined treatments for RILI exist, but none has been satisfactory (Raghu et al., 2012). Given the unique characteristics of MSCs, some researchers have utilized them as a potentially revolutionary treatment for RILI.
MSCs are easy to obtain, genetically stable, have low immunogenicity, and can easily pass through the immune barrier (Lee et al., 2011). The differentiation and grafting of MSCs are thought to play a substantial role in repairing damaged tissues (Prockop, 1997; Sueblinvong et al., 2008). MSCs can migrate along vascular networks. Because of their near-ubiquitous perivascular locations and rich network of projections, MSCs are ideal first responders to inflammation (Soliman et al., 2021). For RILI, inflammatory changes in the immune system occur both in the early stage and during the entire process. Therefore, MSCs as initial damage sensors and inhibitors of innate inflammation can react to changes in metabolism and microenvironment, including cytokine secretion, signaling molecule release, and related immune cell regulation (Harrell et al., 2019). The specific mechanisms associated with MSC therapy for RILI are shown (Figure 3). There are two generations of MSCs treatment for RILI. The first generation, direct administration of MSCs is a basic and effective method (Table 1), and the second generation involves modifying MSCs to act as gene therapy vehicles. Extracellular vesicles (EVs) secreted by MSCs and the culture medium of MSCs are found to have similar functions in preventing pulmonary damage. Therefore, researchers have occasionally used them to treat RILI (Xue et al., 2013; Lei et al., 2020b). The function of EVs is depend on their cargo, which can include functional mRNA, small RNA, lipids, and proteins. For example, EVs as miRNA delivery vehicles can inhibit wound inflammation by delivering miRNA21, miRNA146a, miRNA181 (Ti et al., 2016). The culture medium of MSCs is a mixture of several hundred to thousands of different proteins, cytokines, growth factors, and enzymes, which is secreted by MSCs. EVs are also included in the mixture. It is difficult to clarify all factors that play roles in mitigating the damage. Some researchers aimed to compare the treatment effects of different method. In the study of high fat-induced cardiac complications, MSCs can significantly regulate the TGF-β signaling pathway. However, culture medium has a certain activation effect on transcriptional factors suppressor of cytokine signaling 3 and peroxisome proliferator activated receptor gamma, indicating that MSCs and culture medium may have similar therapeutic effects, but their mechanisms may be different (Daltro et al., 2017). Other studies about MSCs and EVs show the same result (Huang et al., 2016). It cannot be denied that EVs and culture medium as a cell-free therapy offers more advantages, such as suitable for long-term storage, no heterologous risk. There are also some flaws in EVs and culture medium, such as no standard of isolation method (Cheng et al., 2020). In general, both EVs and culture medium are derived from MSCs. Therefore, it is difficult to decide which method is better. Compared to current drug treatment, MSCs have good prospects as a new treatment strategy.
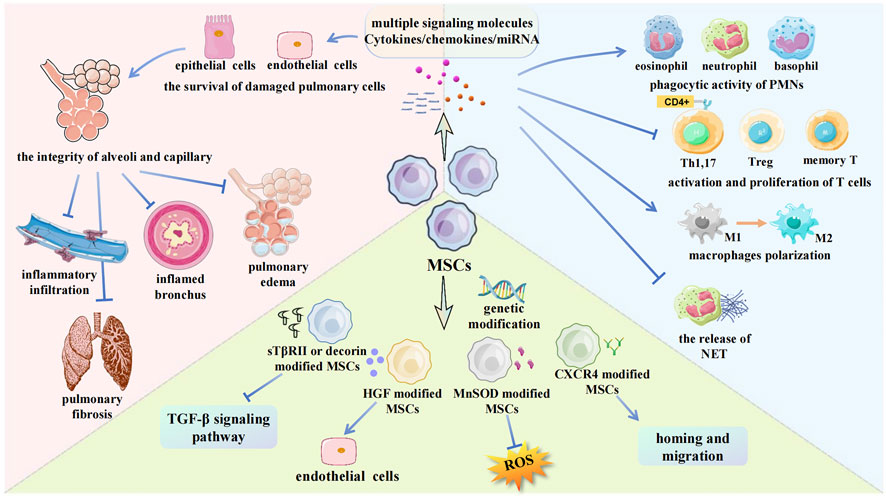
FIGURE 3. Some mechanisms about MSCs for RILI in current researches. The main methods to apply MSCs in RILI are showed in the picture. MSCs can regulate multiple signaling molecules, which protect pulmonary cells. MSCs inhibit leukocytes infiltration and regulate leukocytes function. Genetically modified MSCs can strengthen target gene expression. PMNs, Polymorphonuclear leukocytes; Treg, Regulatory T cell; sTβRII, TGF-β type II receptor; HGF, Hepatocyte growth factor; MnSOD, Manganese superoxide dismutase; CXCR4, CXC-chemokine receptor 4; TGF-β, Transforming growth factor beta.
3.1 Targeting signaling molecules with MSCs
MSCs can directly secrete the anti-inflammatory cytokines IL-10 and IL-2 in an inflammatory environment (Jiang and Xu, 2020). These anti-inflammatory factors directly guide the immune system to exert anti-inflammatory effects and reduce pro-inflammatory cytokines (Zhang et al., 2019a). MSCs also regulate the expression of most inflammatory cytokines. IL-1, the main mediator of innate immunity and inflammation, is significantly reduced after MSC treatment (Garlanda et al., 2013). Moreover, the gene expression of pro-inflammatory cytokines such as IL-6 and TNF-α is significantly decreased. As a multifunctional cytokine, TGF-β can be regulated by MSCs in the RILI model, which means that MSCs also influence collagen deposition and EMT to ameliorate fibrosis (Jiang et al., 2015). Researchers have found that MSCs inhibit the canonical Wnt/β-catenin pathway by secreting Dickkopf-1. Dickkopf-1 can prevent the activation of low-density lipoprotein receptor-related protein 6, a type of Wnt3a receptor on the cell membrane, blocking the downstream signal transduction of Wnt (Shao et al., 2021). MSCs can regulate the migration of immune cells by influencing chemokines or receptors. MSCs can normalize the expression of CCL2, decreasing the directional migration ability of inflammatory cells (Klein et al., 2016). Moreover, the abnormally high proportion of monocytes and granulocytes observed during RILI was reversed by MSCs. Some evidence has shown that MSCs can alleviate RILI through miRNAs. miRNA-214-3p was discovered in EVs secreted by MSCs (Lei et al., 2020b). miRNA-214-3p suppresses endothelial cell damage and enhances radioprotective functions. MSC-derived miRNA-466f-3p could reverse EMT to inhibit fibrosis (Li et al., 2022). The possibility of species variation in the application of MSCs is considered. MSCs can reduce TNF-α and IL-1 in lung tissues and increase the partial pressure of blood oxygen in canines (Hao et al., 2018). Pathological examination also demonstrated that MSCs inhibited inflammatory cell infiltration, accompanied by collagen formation.
3.2 MSCs relieving RILI by regulating immune cells
Several studies have highlighted the critical role of MSCs in the infiltration of polymorphonuclear leukocytes. MSCs can potently suppress the activation and infiltration of neutrophils in the lung (Hong et al., 2017; Fang et al., 2018; Su et al., 2018). MSCs can direct polymorphonuclear leukocytes to ameliorate inflammation via enhanced phagocytic activity (van Dalen et al., 2019). The phagocytosis of polymorphonuclear leukocytes can clear debris and cause self-apoptosis. Then, these leukocytes are ingested by macrophages, which decrease the inflammatory response. Moreover, MSCs can drive neutrophils away from the site of inflammation and injury, which is also called the reversal of inflammatory infiltration (Shang et al., 2020). Neutrophil extracellular traps are network structures composed of chromatin, mitochondrial DNA, and proteins, which are secreted by neutrophils to resist the invasion of harmful substances. However, the overproduction of these traps causes severe tissue pathology in the context of inflammation (Rosazza et al., 2020). Researchers have found that MSCs effectively inhibit the ability of neutrophils to release these traps, which helps relieve inflammation (Navas et al., 2018).
Lymphocytes promote RILI progression, especially during the early inflammatory stage. MSCs exhibit powerful lymphocyte modulatory properties. Ren et al. (2008) found that MSCs can secrete chemokines including CCL5 and CXC-chemokine ligand 9. These chemokines can bind to CCR5 and CXC-chemokine receptor (CXCR) 3 on the surface of T cells, attracting T cells to migrate to the vicinity of MSC. MSCs express nitric oxide synthase or indoleamine 2,3-dioxygenase, which inhibit the activation and proliferation of adjacent T cells, leading to low adaptive immunity. Researchers have shown that MSCs can simultaneously inhibit the proliferation of central memory T cells and effector memory T cells, demonstrating their powerful ability to reduce inflammatory symptoms (Luque-Campos et al., 2019). MSCs can suppress the proliferation, activation, and differentiation of Th1 and Th17 cells when added at the early stages of differentiation (Luz-Crawford et al., 2013). Th1 and Th17 cell subsets are well known to promote inflammation (Lafaille, 1998; Bettelli et al., 2007). MSCs can also promote Treg differentiation through direct contact or a paracrine effect (Selmani et al., 2008; Ghannam et al., 2010). Treg cells can extensively inhibit the immune response and reduce inflammatory damage to the body.
Some researchers have focused on the EVs secreted by MSCs. Lei et al. (2020b) found that EVs secreted by MSCs can effectively reduce the retention of macrophages and neutrophils in lung tissues and the expression of inflammatory cell migration-related genes. Macrophage polarization is a key factor in RILI. MSCs can guide the polarization of macrophages via EVs (Lo Sicco et al., 2017). Then, the macrophages switch from the M1 to M2 phenotype, which means that M1 polarization-related secretion of IL-12 and TNF-α is reduced. Anti-inflammatory factors such as IL-10 are increased. In addition, MSCs cultured in a hypoxic environment seem to be more effective in regulating macrophage polarization.
3.3 Genetically modified MSCs for RILI
MSCs can trigger endogenous repair pathways, particularly the regulation of immune cells in RILI. Another ability of MSCs is that they can home to the damaged site when an injury occurs. Cytokines secreted from damaged site have been demonstrated to stimulate the migration of MSCs in vitro and induce their pathotropism in vivo which is mainly based on the activation of downstream migration-related signaling cascades. These signaling pathway mainly includes the phosphoinositide 3-kinase/AKT, stromal cell-derived factor-1 and its receptor chemokine CXCR4. Some miRNAs also determine the ability of migration (He and Zhang, 2019). Radiation can recruit MSCs to the damaged sites. Perez et al. (Perez et al., 2017) observed an apparent increase in the number of MSCs in the lungs after irradiation. Another study demonstrated that MSCs directly migrated to damaged pulmonary tissues after radiation by fluorescence endomicroscopy imaging. Moreover, irradiation prolongs the resident time of MSCs (Wang et al., 2013). Because MSCs possess the homing ability, an increasing number of researchers have used MSCs as a tool for gene therapy. They induce functional gene expression in MSCs. MSCs can then migrate to the injured site and express specific genes. Such MSCs are considered a superior to normal MSCs. Some studies on MSCs as gene vehicles for the treatment of RILI are summarized in Table 2.
Genetically modified MSCs expressing TGF-β type II receptors have been under investigation to treat RILI (Xue et al., 2013). The receptor secreted by MSCs competes with the extracellular domain of TGF-β type II receptor, resulting in the inhibition of active TGF-β in plasma. Downstream signal transduction related to fibrosis was suppressed accordingly. MSCs protected pulmonary tissue, especially AT2 cells, via a paracrine mechanism. Moreover, genetically modified MSCs can reduce alveolar damage more efficiently than MSCs. Decorin is a natural inhibitor of TGF-β, and decorin-expressing MSCs can cure RILI (Liu et al., 2018). In particular, these modified MSCs also restrict the initiation and progression of fibrosis by downregulating Tregs.
In various injury and disease models, hepatocyte growth factor (HGF) improves cell survival, promotes tissue regeneration, and suppresses chronic inflammation and fibrosis (Nakamura et al., 2011). Wang et al. (2013) found that high HGF significantly reduced alveolar hemorrhage when Hgf genetically modified MSCs were under investigation to treat RILI. The expression of profibrotic genes, such as TGF-β, Col1a1, and Col3a1 is reduced in the lung. High levels of HGF can also stimulate the release of sphingosine kinase, leading to the production of sphingosine-1-phosphate, a lipid messenger. When the messenger binds to its receptor, downstream signaling pathways associated with the regulation of immune cell trafficking and endothelial barrier function are activated (Matloubian et al., 2004; Sanna et al., 2006). Thus, genetically modified MSCs can ameliorate pulmonary damage in RILI.
Damage and senescence of endothelial cells and microvessels are closely related to RP and RPF. Restoring the expression level of superoxide dismutase (SOD) 1 can increase the viability of endothelial cells to alleviate RILI (Epperly et al., 1999; Holley et al., 2011; Klein et al., 2017). Chen et al. (2017) found that MSCs modified with the manganese SOD gene could attenuate capillary rupture, prevent telangiectasia, and maintain the integrity of microvessels. It can also regulate oxidative stress, which is highly associated with abnormal metabolism during RILI.
Currently, the stromal cell-derived factor-1/CXCR4 axis is an important signaling pathway related to the recruitment of MSCs to damaged sites (Wynn et al., 2004; Dar et al., 2005). Some researchers have constructed an MSC cell line that highly expresses CXCR4 (Zhang et al., 2019b). They found that CXCR4 overexpression enhanced the homing and migration abilities of MSCs in vivo. With increased MSC numbers in the lung tissue, morphological lung damage was obviously alleviated. This result again proves the efficacy of modified MSCs for the treatment of RILI.
4 Discussion
Multiple factors contribute to RILI progress, including changes in lung tissue, genetic alterations, oxidative stress, and inflammatory responses. MSCs exhibit powerful anti-inflammatory, anti-oxidative, and signaling effects. MSCs are considered a promising option for the treatment of RILI. In particular, therapies based on MSCs have been combined with gene modification. This new method can enhance the proliferative, migratory, and secretory properties of MSCs, providing further possibilities for RILI. However, applying MSCs still has some limitations, including difficulties in definite characterization of MSCs, and the varying properties of different sources of MSCs. Some studies also reported MSCs may not necessarily provide amelioration of fibrogenic disease in every case. Despite the considerable number of studies that have been performed, clinical management of RILI using the MSCs requires further investigation. For example, which secretory phenotype of MSCs plays a major role in their therapeutic process, and whether the MSCs responds to the RILI according to its stage of progression. Thus, the use of MSCs for the treatment of RILI requires additional data, both in vitro studies and in clinical investigations.
Author contributions
Conceptualization, YX and XJ; methodology, GH; software, JW; validation, YX and JX; formal analysis, JL; investigation, GH; resources, XJ; data curation, WL; writing-original draft preparation, GH; visualization, XJ; supervision, XJ; project administration, XJ; funding acquisition, XJ and YX.
Funding
This research was funded by the Jilin Provincial Science and Technology Foundation (Grant Number 20210509003RQ and 20210402002GH), Health talents special project of Jilin Provincial Finance Department (Grant Number JLSWSRCZX 2021-065), the Achievement Transformation Guiding Foundations of First Hospital of Jilin University (Grant Number CGZHYD202012-029).
Acknowledgments
We would like to thank Editage (www.editage.cn) for English language editing.
Conflict of interest
The authors declare that the research was conducted in the absence of any commercial or financial relationships that could be construed as a potential conflict of interest.
Publisher’s note
All claims expressed in this article are solely those of the authors and do not necessarily represent those of their affiliated organizations, or those of the publisher, the editors and the reviewers. Any product that may be evaluated in this article, or claim that may be made by its manufacturer, is not guaranteed or endorsed by the publisher.
Abbreviations
AT1, Alveolar type 1 cell; AT2, Alveolar type 2 cell; BAL, Bronchoalveolar lavage; ECM, Extracellular matrix; CCL, Chemokine C-C motif ligand; CCR, CC-chemokine receptor; CXCR, CXC-chemokine receptor; EMT, Epithelial-mesenchymal transition; EVs, Extracellular vesicle; G-CSF, Granulocyte colony-stimulating factor; GM-CSF, Granulocyte macrophage colony-stimulating factor; IFN, Interferon; IL, Interleukin; MSCs, Mesenchymal stem cell; NO, Nitric oxide; Nrf2, Nuclear factor E2-related factor 2; RILI, Radiation-induced lung injury; RNS, Reactive nitrogen species; ROS, Reactive oxygen species; RP, Radiation pneumonitis; RPF, Radiation pulmonary fibrosis; RT, Radiotherapy; TGF-β, Transforming growth factor-β; Th, T helper cell; TNF-α, Tumor necrosis factor-α; Treg, Regulatory T cell.
References
Abernathy, L. M., Fountain, M. D., Rothstein, S. E., David, J. M., Yunker, C. K., Rakowski, J., et al. (2015). Soy isoflavones promote radioprotection of normal lung tissue by inhibition of radiation-induced activation of macrophages and neutrophils. J. Thorac. Oncol. 10, 1703–1712. doi:10.1097/jto.0000000000000677
Ao, X., Zhao, L., Davis, M. A., Lubman, D. M., Lawrence, T. S., and Kong, F. M. (2009). Radiation produces differential changes in cytokine profiles in radiation lung fibrosis sensitive and resistant mice. J. Hematol. Oncol. 2, 6. doi:10.1186/1756-8722-2-6
Azzam, E. I., Jay-Gerin, J. P., and Pain, D. (2012). Ionizing radiation-induced metabolic oxidative stress and prolonged cell injury. Cancer Lett. 327, 48–60. doi:10.1016/j.canlet.2011.12.012
Barthelemy-Brichant, N., Bosquee, L., Cataldo, D., Corhay, J. L., Gustin, M., Seidel, L., et al. (2004). Increased IL-6 and TGF-beta1 concentrations in bronchoalveolar lavage fluid associated with thoracic radiotherapy. Int. J. Radiat. Oncol. Biol. Phys. 58, 758–767. doi:10.1016/s0360-3016(03)01614-6
Bettelli, E., Oukka, M., and Kuchroo, V. K. (2007). T(H)-17 cells in the circle of immunity and autoimmunity. Nat. Immunol. 8, 345–350. doi:10.1038/ni0407-345
Bledsoe, T. J., Nath, S. K., and Decker, R. H. (2017). Radiation pneumonitis. Clin. Chest Med. 38, 201–208. doi:10.1016/j.ccm.2016.12.004
Buonanno, M., de Toledo, S. M., and Azzam, E. I. (2011). Increased frequency of spontaneous neoplastic transformation in progeny of bystander cells from cultures exposed to densely ionizing radiation. PLoS One 6, e21540. doi:10.1371/journal.pone.0021540
Buonanno, M., de Toledo, S. M., Pain, D., and Azzam, E. I. (2011). Long-term consequences of radiation-induced bystander effects depend on radiation quality and dose and correlate with oxidative stress. Radiat. Res. 175, 405–415. doi:10.1667/rr2461.1
Cappuccini, F., Eldh, T., Bruder, D., Gereke, M., Jastrow, H., Schulze-Osthoff, K., et al. (2011). New insights into the molecular pathology of radiation-induced pneumopathy. Radiother. Oncol. 101, 86–92. doi:10.1016/j.radonc.2011.05.064
Chen, H. X., Xiang, H., Xu, W. H., Li, M., Yuan, J., Liu, J., et al. (2017). Manganese superoxide dismutase gene-modified mesenchymal stem cells attenuate acute radiation-induced lung injury. Hum. Gene Ther. 28, 523–532. doi:10.1089/hum.2016.106
Chen, Y., Rubin, P., Williams, J., Hernady, E., Smudzin, T., and Okunieff, P. (2001). Circulating IL-6 as a predictor of radiation pneumonitis. Int. J. Radiat. Oncol. Biol. Phys. 49, 641–648. doi:10.1016/s0360-3016(00)01445-0
Cheng, Y., Cao, X., and Qin, L. (2020). Mesenchymal stem cell-derived extracellular vesicles: A novel cell-free therapy for sepsis. Front. Immunol. 11, 647. doi:10.3389/fimmu.2020.00647
Cinat, D., Coppes, R. P., and Barazzuol, L. (2021). DNA damage-induced inflammatory microenvironment and adult stem cell response. Front. Cell. Dev. Biol. 9, 729136. doi:10.3389/fcell.2021.729136
Cole, A. L., Subbanagounder, G., Mukhopadhyay, S., Berliner, J. A., and Vora, D. K. (2003). Oxidized phospholipid-induced endothelial cell/monocyte interaction is mediated by a cAMP-dependent R-Ras/PI3-kinase pathway. Arterioscler. Thromb. Vasc. Biol. 23, 1384–1390. doi:10.1161/01.Atv.0000081215.45714.71
Cui, W., Hankey, K. G., Zhang, P., Bolduc, D. L., Bunger, R., Xiao, M., et al. (2020). Identifying circulating and lung tissue cytokines associated with thoracic irradiation and AEOL 10150 treatment in a nonhuman primate model. Radiat. Res. 194, 81–88. doi:10.1667/rr14310.1
Cui, W., Zhang, P., Hankey, K. G., Xiao, M., Farese, A. M., and MacVittie, T. J. (2021). AEOL 10150 alleviates radiation-induced innate immune responses in non-human primate lung tissue. Health Phys. 121, 331–344. doi:10.1097/hp.0000000000001443
Daltro, P. S., Barreto, B. C., Silva, P. G., Neto, P. C., Sousa Filho, P. H. F., Santana Neta, D., et al. (2017). Therapy with mesenchymal stromal cells or conditioned medium reverse cardiac alterations in a high-fat diet-induced obesity model. Cytotherapy 19, 1176–1188. doi:10.1016/j.jcyt.2017.07.002
Dar, A., Goichberg, P., Shinder, V., Kalinkovich, A., Kollet, O., Netzer, N., et al. (2005). Chemokine receptor CXCR4-dependent internalization and resecretion of functional chemokine SDF-1 by bone marrow endothelial and stromal cells. Nat. Immunol. 6, 1038–1046. doi:10.1038/ni1251
Elliott, J. A., O'Byrne, L., Foley, G., Murphy, C. F., Doyle, S. L., King, S., et al. (2019). Effect of neoadjuvant chemoradiation on preoperative pulmonary physiology, postoperative respiratory complications and quality of life in patients with oesophageal cancer. Br. J. Surg. 106, 1341–1351. doi:10.1002/bjs.11218
Epperly, M. W., Bray, J. A., Krager, S., Berry, L. M., Gooding, W., Engelhardt, J. F., et al. (1999). Intratracheal injection of adenovirus containing the human MnSOD transgene protects athymic nude mice from irradiation-induced organizing alveolitis. Int. J. Radiat. Oncol. Biol. Phys. 43, 169–181. doi:10.1016/s0360-3016(98)00355-1
Eser, P., and Jänne, P. A. (2018). TGFβ pathway inhibition in the treatment of non-small cell lung cancer. Pharmacol. Ther. 184, 112–130. doi:10.1016/j.pharmthera.2017.11.004
Fang, S. B., Zhang, H. Y., Jiang, A. Y., Fan, X. L., Lin, Y. D., Li, C. L., et al. (2018). Human iPSC-MSCs prevent steroid-resistant neutrophilic airway inflammation via modulating Th17 phenotypes. Stem Cell. Res. Ther. 9, 147. doi:10.1186/s13287-018-0897-y
Fernandes-Alnemri, T., Yu, J. W., Datta, P., Wu, J., and Alnemri, E. S. (2009). AIM2 activates the inflammasome and cell death in response to cytoplasmic DNA. Nature 458, 509–513. doi:10.1038/nature07710
Gandhi, K. A., Goda, J. S., Gandhi, V. V., SadAnpurwAlA, A., Jain, V. K., Joshi, K., et al. (2019). Oral administration of 3, 3'-diselenodipropionic acid prevents thoracic radiation induced pneumonitis in mice by suppressing NF-kB/IL-17/G-CSF/neutrophil axis. Free Radic. Biol. Med. 145, 8–19. doi:10.1016/j.freeradbiomed.2019.09.009
Gao, J., Peng, S., Shan, X., Deng, G., Shen, L., Sun, J., et al. (2019). Inhibition of AIM2 inflammasome-mediated pyroptosis by Andrographolide contributes to amelioration of radiation-induced lung inflammation and fibrosis. Cell. Death Dis. 10, 957. doi:10.1038/s41419-019-2195-8
Garlanda, C., Dinarello, C. A., and Mantovani, A. (2013). The interleukin-1 family: Back to the future. Immunity 39, 1003–1018. doi:10.1016/j.immuni.2013.11.010
Gauter-Fleckenstein, B., Fleckenstein, K., Owzar, K., Jiang, C., Reboucas, J. S., Batinic-Haberle, I., et al. (2010). Early and late administration of MnTE-2-PyP5+ in mitigation and treatment of radiation-induced lung damage. Free Radic. Biol. Med. 48, 1034–1043. doi:10.1016/j.freeradbiomed.2010.01.020
Ghannam, S., Pene, J., Moquet-Torcy, G., Torcy-Moquet, G., Jorgensen, C., and Yssel, H. (2010). Mesenchymal stem cells inhibit human Th17 cell differentiation and function and induce a T regulatory cell phenotype. J. Immunol. 185, 302–312. doi:10.4049/jimmunol.0902007
Ghobadi, G., Bartelds, B., van der Veen, S. J., Dickinson, M. G., Brandenburg, S., Berger, R. M. F., et al. (2012). Lung irradiation induces pulmonary vascular remodelling resembling pulmonary arterial hypertension. Thorax 67, 334–341. doi:10.1136/thoraxjnl-2011-200346
Giaid, A., Lehnert, S. M., Chehayeb, B., Chehayeb, D., Kaplan, I., and Shenouda, G. (2003). Inducible nitric oxide synthase and nitrotyrosine in mice with radiation-induced lung damage. Am. J. Clin. Oncol. 26, e67–e72. doi:10.1097/01.Coc.0000077940.05196.86
Giuranno, L., Ient, J., De Ruysscher, D., and Vooijs, M. A. (2019). Radiation-induced lung injury (RILI). Front. Oncol. 9, 877. doi:10.3389/fonc.2019.00877
Greenberg, M. E., Sun, M., Zhang, R., Febbraio, M., Silverstein, R., and Hazen, S. L. (2006). Oxidized phosphatidylserine-CD36 interactions play an essential role in macrophage-dependent phagocytosis of apoptotic cells. J. Exp. Med. 203, 2613–2625. doi:10.1084/jem.20060370
Guo, T., Zou, L., Ni, J., Zhou, Y., Ye, L., Yang, X., et al. (2020). Regulatory T cells: An emerging player in radiation-induced lung injury. Front. Immunol. 11, 1769. doi:10.3389/fimmu.2020.01769
Hanania, A. N., Mainwaring, W., Ghebre, Y. T., Hanania, N. A., and Ludwig, M. (2019). Radiation-induced lung injury: Assessment and management. Chest 156, 150–162. doi:10.1016/j.chest.2019.03.033
Hao, Y., Ran, Y., Lu, B., Li, J., Zhang, J., Feng, C., et al. (2018). Therapeutic effects of human umbilical cord-derived mesenchymal stem cells on canine radiation-induced lung injury. Int. J. Radiat. Oncol. Biol. Phys. 102, 407–416. doi:10.1016/j.ijrobp.2018.05.068
Harrell, C. R., Sadikot, R., Pascual, J., Fellabaum, C., Jankovic, M. G., Jovicic, N., et al. (2019). Mesenchymal stem cell-based therapy of inflammatory lung diseases: Current understanding and future perspectives. Stem Cells Int. 2019, 4236973. doi:10.1155/2019/4236973
Haworth, O., and Levy, B. D. (2007). Endogenous lipid mediators in the resolution of airway inflammation. Eur. Respir. J. 30, 980–992. doi:10.1183/09031936.00005807
He, L., and Zhang, H. (2019). MicroRNAs in the migration of mesenchymal stem cells. Stem Cell. Rev. Rep. 15, 3–12. doi:10.1007/s12015-018-9852-7
He, Y., Thummuri, D., Zheng, G., Okunieff, P., Citrin, D. E., Vujaskovic, Z., et al. (2019). Cellular senescence and radiation-induced pulmonary fibrosis. Transl. Res. 209, 14–21. doi:10.1016/j.trsl.2019.03.006
Hewlett, J. C., Kropski, J. A., and Blackwell, T. S. (2018). Idiopathic pulmonary fibrosis: Epithelial-mesenchymal interactions and emerging therapeutic targets. Matrix Biol. 71-72, 112–127. doi:10.1016/j.matbio.2018.03.021
Holley, A. K., Bakthavatchalu, V., Velez-Roman, J. M., and St Clair, D. K. (2011). Manganese superoxide dismutase: Guardian of the powerhouse. Int. J. Mol. Sci. 12, 7114–7162. doi:10.3390/ijms12107114
Hong, G. H., Kwon, H. S., Lee, K. Y., Ha, E. H., Moon, K. A., Kim, S. W., et al. (2017). hMSCs suppress neutrophil-dominant airway inflammation in a murine model of asthma. Exp. Mol. Med. 49, e288. doi:10.1038/emm.2016.135
Hu, D., Zhang, Y., Cao, R., Hao, Y., Yang, X., Tian, T., et al. (2020). The protective effects of granulocyte-macrophage colony-stimulating factor against radiation-induced lung injury. Transl. Lung Cancer Res. 9, 2440–2459. doi:10.21037/tlcr-20-1272
Huang, B., Cheng, X., Wang, H., Huang, W., la Ga Hu, Z., Wang, D., et al. (2016). Mesenchymal stem cells and their secreted molecules predominantly ameliorate fulminant hepatic failure and chronic liver fibrosis in mice respectively. J. Transl. Med. 14, 45. doi:10.1186/s12967-016-0792-1
Ikushima, H., and Miyazono, K. (2010). TGFbeta signalling: A complex web in cancer progression. Nat. Rev. Cancer 10, 415–424. doi:10.1038/nrc2853
Jiang, W., and Xu, J. (2020). Immune modulation by mesenchymal stem cells. Cell. Prolif. 53, e12712. doi:10.1111/cpr.12712
Jiang, X., Qu, C., Chang, P., Zhang, C., Qu, Y., and Liu, Y. (2015). Intravenous delivery of adipose-derived mesenchymal stromal cells attenuates acute radiation-induced lung injury in rats. Cytotherapy 17, 560–570. doi:10.1016/j.jcyt.2015.02.011
Kim, H., Park, S. H., Han, S. Y., Lee, Y. S., Cho, J., and Kim, J. M. (2020). LXA(4)-FPR2 signaling regulates radiation-induced pulmonary fibrosis via crosstalk with TGF-β/Smad signaling. Cell. Death Dis. 11, 653. doi:10.1038/s41419-020-02846-7
Kim, K. K., Kugler, M. C., Wolters, P. J., Robillard, L., Galvez, M. G., Brumwell, A. N., et al. (2006). Alveolar epithelial cell mesenchymal transition develops in vivo during pulmonary fibrosis and is regulated by the extracellular matrix. Proc. Natl. Acad. Sci. U. S. A. 103, 13180–13185. doi:10.1073/pnas.0605669103
Klein, D., Schmetter, A., Imsak, R., Wirsdorfer, F., Unger, K., Jastrow, H., et al. (2016). Therapy with multipotent mesenchymal stromal cells protects lungs from radiation-induced injury and reduces the risk of lung metastasis. Antioxid. Redox Signal. 24, 53–69. doi:10.1089/ars.2014.6183
Klein, D., Steens, J., Wiesemann, A., Schulz, F., Kaschani, F., Rock, K., et al. (2017). Mesenchymal stem cell therapy protects lungs from radiation-induced endothelial cell loss by restoring superoxide dismutase 1 expression. Antioxid. Redox Signal. 26, 563–582. doi:10.1089/ars.2016.6748
Kong, F. S., Moiseenko, V., Zhao, J., Milano, M. T., Li, L., Rimner, A., et al. (2021). Organs at risk considerations for thoracic stereotactic body radiation therapy: What is safe for lung parenchyma? Int. J. Radiat. Oncol. Biol. Phys. 110, 172–187. doi:10.1016/j.ijrobp.2018.11.028
Kunwar, A., Jain, V. K., Priyadarsini, K. I., and Haston, C. K. (2013). A selenocysteine derivative therapy affects radiation-induced pneumonitis in the mouse. Am. J. Respir. Cell. Mol. Biol. 49, 654–661. doi:10.1165/rcmb.2013-0095OC
Lafaille, J. J. (1998). The role of helper T cell subsets in autoimmune diseases. Cytokine Growth Factor Rev. 9, 139–151. doi:10.1016/s1359-6101(98)00009-4
Lee, J. W., Fang, X., Krasnodembskaya, A., Howard, J. P., and Matthay, M. A. (2011). Concise review: Mesenchymal stem cells for acute lung injury: Role of paracrine soluble factors. Stem Cells 29, 913–919. doi:10.1002/stem.643
Lee, O. H., Jain, A. K., Papusha, V., and Jaiswal, A. K. (2007). An auto-regulatory loop between stress sensors INrf2 and Nrf2 controls their cellular abundance. J. Biol. Chem. 282, 36412–36420. doi:10.1074/jbc.M706517200
Lei, G., Zhang, Y., Koppula, P., Liu, X., Zhang, J., Lin, S. H., et al. (2020). The role of ferroptosis in ionizing radiation-induced cell death and tumor suppression. Cell. Res. 30, 146–162. doi:10.1038/s41422-019-0263-3
Lei, X., He, N., Zhu, L., Zhou, M., Zhang, K., Wang, C., et al. (2020). Mesenchymal stem cell-derived extracellular vesicles attenuate radiation-induced lung injury via miRNA-214-3p. Antioxid. Redox Signal. 35, 849–862. doi:10.1089/ars.2019.7965
Li, Y., Shen, Z., Jiang, X., Wang, Y., Yang, Z., Mao, Y., et al. (2022). Mouse mesenchymal stem cell-derived exosomal miR-466f-3p reverses EMT process through inhibiting AKT/GSK3β pathway via c-MET in radiation-induced lung injury. J. Exp. Clin. Cancer Res. 128, 128. doi:10.1186/s13046-022-02351-z
Li, Y., Zou, L., Chu, L., Ye, L., Ni, J., Chu, X., et al. (2021). Identification and integrated analysis of circRNA and miRNA of radiation-induced lung injury in a mouse model. J. Inflamm. Res. 14, 4421–4431. doi:10.2147/jir.S322736
Liu, D., Kong, F., Yuan, Y., Seth, P., Xu, W., Wang, H., et al. (2018). Decorin-modified umbilical cord mesenchymal stem cells (MSCs) attenuate radiation-induced lung injuries via regulating inflammation, fibrotic factors, and immune responses. Int. J. Radiat. Oncol. Biol. Phys. 101, 945–956. doi:10.1016/j.ijrobp.2018.04.007
Liu, Z., Dong, L., Zheng, Z., Liu, S., Gong, S., Meng, L., et al. (2021). Mechanism, prevention, and treatment of radiation-induced salivary gland injury related to oxidative stress. Antioxidants(Basel) 35, 849-862. doi:10.3390/antiox10111666
Lo Sicco, C., Reverberi, D., Balbi, C., Ulivi, V., Principi, E., Pascucci, L., et al. (2017). Mesenchymal stem cell-derived extracellular vesicles as mediators of anti-inflammatory effects: Endorsement of macrophage polarization. Stem Cells Transl. Med. 6, 1018–1028. doi:10.1002/sctm.16-0363
Luque-Campos, N., Contreras-Lopez, R. A., Jose Paredes-Martinez, M., Torres, M. J., Bahraoui, S., Wei, M., et al. (2019). Mesenchymal stem cells improve rheumatoid arthritis progression by controlling memory T cell response. Front. Immunol. 10, 798. doi:10.3389/fimmu.2019.00798
Luz-Crawford, P., Kurte, M., Bravo-Alegria, J., Contreras, R., Nova-Lamperti, E., Tejedor, G., et al. (2013). Mesenchymal stem cells generate a CD4+CD25+Foxp3+ regulatory T cell population during the differentiation process of Th1 and Th17 cells. Stem Cell. Res. Ther. 4, 65. doi:10.1186/scrt216
Majori, M., Poletti, V., Curti, A., CorradiM., , FalconeF., , and Pesci, A. (2000). Bronchoalveolar lavage in bronchiolitis obliterans organizing pneumonia primed by radiation therapy to the breast. J. Allergy Clin. Immunol. 105, 239–244. doi:10.1016/s0091-6749(00)90071-x
Marks, L. B., Bentzen, S. M., Deasy, J. O., Kong, F. M. S., Bradley, J. D., Vogelius, I. S., et al. (2010). Radiation dose-volume effects in the lung. Int. J. Radiat. Oncol. Biol. Phys. 76, S70–S76. doi:10.1016/j.ijrobp.2009.06.091
Mathew, B., Huang, Y., Jacobson, J. R., Berdyshev, E., Gerhold, L. M., Wang, T., et al. (2011). Simvastatin attenuates radiation-induced murine lung injury and dysregulated lung gene expression. Am. J. Respir. Cell. Mol. Biol. 44, 415–422. doi:10.1165/rcmb.2010-0122OC
Mathew, B., Jacobson, J. R., Siegler, J. H., Moitra, J., Blasco, M., Xie, L., et al. (2013). Role of migratory inhibition factor in age-related susceptibility to radiation lung injury via NF-E2-related factor-2 and antioxidant regulation. Am. J. Respir. Cell. Mol. Biol. 49, 269–278. doi:10.1165/rcmb.2012-0291OC
Matloubian, M., Lo, C. G., Cinamon, G., Lesneski, M. J., Xu, Y., Brinkmann, V., et al. (2004). Lymphocyte egress from thymus and peripheral lymphoid organs is dependent on S1P receptor 1. Nature 427, 355–360. doi:10.1038/nature02284
Meng, X. M., Nikolic-Paterson, D. J., and Lan, H. Y. (2016). TGF-Β: The master regulator of fibrosis. Nat. Rev. Nephrol. 12, 325–338. doi:10.1038/nrneph.2016.48
Nakamura, T., Sakai, K., and Matsumoto, K. (2011). Hepatocyte growth factor twenty years on: Much more than a growth factor. J. Gastroenterol. Hepatol. 26 Suppl 1, 188–202. doi:10.1111/j.1440-1746.2010.06549.x
Navas, A., Magana-Guerrero, F. S., Dominguez-Lopez, A., Chavez-Garcia, C., Partido, G., Graue-Hernandez, E. O., et al. (2018). Anti-inflammatory and anti-fibrotic effects of human amniotic membrane mesenchymal stem cells and their potential in corneal repair. Stem Cells Transl. Med. 7, 906–917. doi:10.1002/sctm.18-0042
Perez, J. R., Ybarra, N., Chagnon, F., Serban, M., Lee, S., Seuntjens, J., et al. (2017). Tracking of mesenchymal stem cells with fluorescence endomicroscopy imaging in radiotherapy-induced lung injury. Sci. Rep. 7, 40748. doi:10.1038/srep40748
Petkau, A. (1987). Role of superoxide dismutase in modification of radiation injury. Br. J. Cancer. Suppl. 8, 87–95.
Pittenger, M. F., Mackay, A. M., Beck, S. C., Jaiswal, R. K., Douglas, R., Mosca, J. D., et al. (1999). Multilineage potential of adult human mesenchymal stem cells. Science 284, 143–147. doi:10.1126/science.284.5411.143
Prockop, D. J. (1997). Marrow stromal cells as stem cells for nonhematopoietic tissues. Science 276, 71–74. doi:10.1126/science.276.5309.71
Raghu, G., Anstrom, K. J., King, T. E., Lasky, J. A., and Martinez, F. J. (2012). Prednisone, azathioprine, and N-acetylcysteine for pulmonary fibrosis. N. Engl. J. Med. 366, 1968–1977. doi:10.1056/NEJMoa1113354
Rajan Radha, R., and Chandrasekharan, G. (2017). Pulmonary injury associated with radiation therapy - assessment, complications and therapeutic targets. Biomed. Pharmacother. 89, 1092–1104. doi:10.1016/j.biopha.2017.02.106
Ren, G., Zhang, L., Zhao, X., Xu, G., Zhang, Y., Roberts, A. I., et al. (2008). Mesenchymal stem cell-mediated immunosuppression occurs via concerted action of chemokines and nitric oxide. Cell. Stem Cell. 2, 141–150. doi:10.1016/j.stem.2007.11.014
Rosazza, T., Warner, J., and Sollberger, G. (2020). NET formation - mechanisms and how they relate to other cell death pathways. Febs J. 288, 3334–3350. doi:10.1111/febs.15589
Rube, C. E., Uthe, D., Schmid, K. W., Richter, K. D., Wessel, J., Schuck, A., et al. (2000). Dose-dependent induction of transforming growth factor beta (TGF-beta) in the lung tissue of fibrosis-prone mice after thoracic irradiation. Int. J. Radiat. Oncol. Biol. Phys. 47, 1033–1042. doi:10.1016/s0360-3016(00)00482-x
Rübe, C. E., Uthe, D., Wilfert, F., Ludwig, D., Yang, K., Konig, J., et al. (2005). The bronchiolar epithelium as a prominent source of pro-inflammatory cytokines after lung irradiation. Int. J. Radiat. Oncol. Biol. Phys. 61, 1482–1492. doi:10.1016/j.ijrobp.2004.12.072
Rübe, C. E., Wilfert, F., Uthe, D., Schmid, K. W., Knoop, R., Willich, N., et al. (2002). Modulation of radiation-induced tumour necrosis factor alpha (TNF-alpha) expression in the lung tissue by pentoxifylline. Radiother. Oncol. 64, 177–187. doi:10.1016/s0167-8140(02)00077-4
Samsonraj, R. M., Raghunath, M., Nurcombe, V., Hui, J. H., van Wijnen, A. J., and Cool, S. M. (2017). Concise review: Multifaceted characterization of human mesenchymal stem cells for use in regenerative medicine. Stem Cells Transl. Med. 6, 2173–2185. doi:10.1002/sctm.17-0129
Sanna, M. G., Wang, S. K., Gonzalez-Cabrera, P. J., Don, A., Marsolais, D., Matheu, M. P., et al. (2006). Enhancement of capillary leakage and restoration of lymphocyte egress by a chiral S1P1 antagonist in vivo. Nat. Chem. Biol. 2, 434–441. doi:10.1038/nchembio804
Sekine, I., Sumi, M., Ito, Y., Nokihara, H., Yamamoto, N., Kunitoh, H., et al. (2006). Retrospective analysis of steroid therapy for radiation-induced lung injury in lung cancer patients. Radiother. Oncol. 80, 93–97. doi:10.1016/j.radonc.2006.06.007
Selmani, Z., Naji, A., Zidi, I., Favier, B., Gaiffe, E., Obert, L., et al. (2008). Human leukocyte antigen-G5 secretion by human mesenchymal stem cells is required to suppress T lymphocyte and natural killer function and to induce CD4+CD25highFOXP3+ regulatory T cells. Stem Cells 26, 212–222. doi:10.1634/stemcells.2007-0554
Shang, Q., Chu, Y., Li, Y., Han, Y., Yu, D., Liu, R., et al. (2020). Adipose-derived mesenchymal stromal cells promote corneal wound healing by accelerating the clearance of neutrophils in cornea. Cell. Death Dis. 11, 707. doi:10.1038/s41419-020-02914-y
Shao, L., Zhang, Y., Shi, W., Ma, L., Xu, T., Chang, P., et al. (2021). Mesenchymal stromal cells can repair radiation-induced pulmonary fibrosis via a DKK-1-mediated Wnt/β-catenin pathway. Cell. Tissue Res. 384, 87–97. doi:10.1007/s00441-020-03325-3
Shen, Y., Jiang, X., Meng, L., Xia, C., Zhang, L., and Xin, Y. (2018). Transplantation of bone marrow mesenchymal stem cells prevents radiation-induced artery injury by suppressing oxidative stress and inflammation. Oxid. Med. Cell. Longev. 2018, 5942916. doi:10.1155/2018/5942916
Shiomi, A., and Usui, T. (2015). Pivotal roles of GM-CSF in autoimmunity and inflammation. Mediat. Inflamm. 2015, 568543. doi:10.1155/2015/568543
Soliman, H., Theret, M., Scott, W., Hill, L., Underhill, T. M., Hinz, B., et al. (2021). Multipotent stromal cells: One name, multiple identities. Cell. Stem Cell. 28, 1690–1707. doi:10.1016/j.stem.2021.09.001
Su, X., Yang, L., Yin, Y., Huang, J., Qiao, F., Fang, Y., et al. (2018). Bone marrow mesenchymal stem cells tune the differentiation of myeloid-derived suppressor cells in bleomycin-induced lung injury. Stem Cell. Res. Ther. 9, 253. doi:10.1186/s13287-018-0983-1
Sueblinvong, V., Loi, R., Eisenhauer, P. L., Bernstein, I. M., Suratt, B. T., Spees, J. L., et al. (2008). Derivation of lung epithelium from human cord blood-derived mesenchymal stem cells. Am. J. Respir. Crit. Care Med. 177, 701–711. doi:10.1164/rccm.200706-859OC
Tang, D. G., La, E., Kern, J., and Kehrer, J. P. (2002). Fatty acid oxidation and signaling in apoptosis. Biol. Chem. 383, 425–442. doi:10.1515/bc.2002.046
Thakur, P., DeBo, R., Dugan, G. O., Bourland, J. D., Michalson, K. T., Olson, J. D., et al. (2021). Clinicopathologic and transcriptomic analysis of radiation-induced lung injury in nonhuman primates. Int. J. Radiat. Oncol. Biol. Phys. 111, 249–259. doi:10.1016/j.ijrobp.2021.03.058
Ti, D., Hao, H., Fu, X., and Han, W. (2016). Mesenchymal stem cells-derived exosomal microRNAs contribute to wound inflammation. Sci. China. Life Sci. 59, 1305–1312. doi:10.1007/s11427-016-0240-4
Tsoutsou, P. G., and Koukourakis, M. I. (2006). Radiation pneumonitis and fibrosis: Mechanisms underlying its pathogenesis and implications for future research. Int. J. Radiat. Oncol. Biol. Phys. 66, 1281–1293. doi:10.1016/j.ijrobp.2006.08.058
Tyurina, Y. Y., Tyurin, V. A., Kapralova, V. I., Wasserloos, K., Mosher, M., Epperly, M. W., et al. (2011). Oxidative lipidomics of γ-radiation-induced lung injury: Mass spectrometric characterization of cardiolipin and phosphatidylserine peroxidation. Radiat. Res. 175, 610–621. doi:10.1667/rr2297.1
Uccelli, A., Moretta, L., and Pistoia, V. (2008). Mesenchymal stem cells in health and disease. Nat. Rev. Immunol. 8, 726–736. doi:10.1038/nri2395
van Dalen, S. C. M., Blom, A. B., Walgreen, B., Sloetjes, A. W., Helsen, M. M. A., Geven, E. J. W., et al. (2019). IL-1β-Mediated activation of adipose-derived mesenchymal stromal cells results in pmn reallocation and enhanced phagocytosis: A possible mechanism for the reduction of osteoarthritis pathology. Front. Immunol. 10, 1075. doi:10.3389/fimmu.2019.01075
Wang, B., Wei, J., Meng, L., Wang, H., Qu, C., Chen, X., et al. (2020). Advances in pathogenic mechanisms and management of radiation-induced fibrosis. Biomed. Pharmacother. 121, 109560. doi:10.1016/j.biopha.2019.109560
Wang, H., Yang, Y. F., Zhao, L., Xiao, F. J., Zhang, Q. W., Wen, M. L., et al. (2013). Hepatocyte growth factor gene-modified mesenchymal stem cells reduce radiation-induced lung injury. Hum. Gene Ther. 24, 343–353. doi:10.1089/hum.2012.177
Wang, R., Zhu, C. z., Qiao, P., Liu, J., Zhao, Q., Wang, K. j., et al. (2014). Experimental treatment of radiation pneumonitis with human umbilical cord mesenchymal stem cells. Asian pac. J. Trop. Med. 7, 262–266. doi:10.1016/s1995-7645(14)60034-1
Wei, J., Zhao, Q., Zhang, Y., Shi, W., Wang, H., Zheng, Z., et al. (2021). Sulforaphane-mediated Nrf2 activation prevents radiation-induced skin injury through inhibiting the oxidative-stress-activated DNA damage and NLRP3 inflammasome. Antioxidants(Basel) 10, 1850. doi:10.3390/antiox10111850
Wiesemann, A., Ketteler, J., Slama, A., Wirsdorfer, F., Hager, T., Rock, K., et al. (2019). Inhibition of radiation-induced Ccl2 signaling protects lungs from vascular dysfunction and endothelial cell loss. Antioxid. Redox Signal. 30, 213–231. doi:10.1089/ars.2017.7458
Wijerathne, H., Langston, J. C., Yang, Q., Sun, S., Miyamoto, C., Kilpatrick, L. E., et al. (2021). Mechanisms of radiation-induced endothelium damage: Emerging models and technologies. Radiother. Oncol. 158, 21–32. doi:10.1016/j.radonc.2021.02.007
Wu, X., Ji, H., Wang, Y., Gu, C., Gu, W., Hu, L., et al. (2019). Melatonin Alleviates Radiation-Induced Lung Injury via Regulation of miR-30e/NLRP3 Axis. Oxid. Med. Cell. Longev. 2019, 4087298. doi:10.1155/2019/4087298
Wynn, R. F., Hart, C. A., Corradi-Perini, C., O'Neill, L., Evans, C. A., Wraith, J. E., et al. (2004). A small proportion of mesenchymal stem cells strongly expresses functionally active CXCR4 receptor capable of promoting migration to bone marrow. Blood 104, 2643–2645. doi:10.1182/blood-2004-02-0526
Xia, C., Chang, P., Zhang, Y., Shi, W., Liu, B., Ding, L., et al. (2016). Therapeutic effects of bone marrow-derived mesenchymal stem cells on radiation-induced lung injury. Oncol. Rep. 35, 731–738. doi:10.3892/or.2015.4433
Xue, J., Li, X., Lu, Y., Gan, L., Zhou, L., Wang, Y., et al. (2013). Gene-modified mesenchymal stem cells protect against radiation-induced lung injury. Mol. Ther. 21, 456–465. doi:10.1038/mt.2012.183
Yan, Y., Fu, J., Kowalchuk, R. O., Wright, C. M., Zhang, R., Li, X., et al. (2022). Exploration of radiation-induced lung injury, from mechanism to treatment: A narrative review. Transl. Lung Cancer Res. 11, 307–322. doi:10.21037/tlcr-22-108
Yang, X., Walton, W., Cook, D. N., Hua, X., Tilley, S., Haskell, C. A., et al. (2011). The chemokine, CCL3, and its receptor, CCR1, mediate thoracic radiation-induced pulmonary fibrosis. Am. J. Respir. Cell. Mol. Biol. 45, 127–135. doi:10.1165/rcmb.2010-0265OC
Ying, H., Fang, M., Hang, Q. Q., Chen, Y., Qian, X., and Chen, M. (2021). Pirfenidone modulates macrophage polarization and ameliorates radiation-induced lung fibrosis by inhibiting the TGF-β1/Smad3 pathway. J. Cell. Mol. Med. 25, 8662–8675. doi:10.1111/jcmm.16821
Zhang, C., Zhu, Y., Wang, J., Hou, L., and Li, W. (2019). CXCR4-Overexpressing umbilical cord mesenchymal stem cells enhance protection against radiation-induced lung injury. Stem Cells Int. 2019, 2457082. doi:10.1155/2019/2457082
Zhang, C., Zhu, Y., Zhang, Y., Gao, L., Zhang, N., and Feng, H. (2015). Therapeutic potential of umbilical cord mesenchymal stem cells for inhibiting myofibroblastic differentiation of irradiated human lung fibroblasts. Tohoku J. Exp. Med. 236, 209–217. doi:10.1620/tjem.236.209
Zhang, J., Ma, J., Zhou, S., Hubbs, J. L., Wong, T. Z., Folz, R. J., et al. (2010). Radiation-induced reductions in regional lung perfusion: 0.1-12 year data from a prospective clinical study. Int. J. Radiat. Oncol. Biol. Phys. 76, 425–432. doi:10.1016/j.ijrobp.2009.02.005
Keywords: radiation-induced lung injury (RILI), mesenchymal stem cells (MSCs), gene modification, inflammatory response, cytokines, oxidative stress
Citation: Hou G, Li J, Liu W, Wei J, Xin Y and Jiang X (2022) Mesenchymal stem cells in radiation-induced lung injury: From mechanisms to therapeutic potential. Front. Cell Dev. Biol. 10:1100305. doi: 10.3389/fcell.2022.1100305
Received: 16 November 2022; Accepted: 30 November 2022;
Published: 12 December 2022.
Edited by:
Yu Xiao, Zhongnan Hospital, Wuhan University, ChinaReviewed by:
Yanyong Yang, Second Military Medical University, ChinaWanchang Cui, University of Maryland, Baltimore, United States
Mengxue Yu, Zhongnan Hospital, Wuhan University, China
Copyright © 2022 Hou, Li, Liu, Wei, Xin and Jiang. This is an open-access article distributed under the terms of the Creative Commons Attribution License (CC BY). The use, distribution or reproduction in other forums is permitted, provided the original author(s) and the copyright owner(s) are credited and that the original publication in this journal is cited, in accordance with accepted academic practice. No use, distribution or reproduction is permitted which does not comply with these terms.
*Correspondence: Ying Xin, eGlueUBqbHUuZWR1LmNu; Xin Jiang, amlhbmd4QGpsdS5lZHUuY24=