- 1Laboratory of Gap Junctions Proteins and Parasitic Diseases (GaPaL), Instituto Antofagasta, Universidad de Antofagasta, Antofagasta, Chile
- 2Centro de Investigación en Inmunología y Biotecnología Biomédica de Antofagasta (CIIBBA), Universidad de Antofagasta, Antofagasta, Chile
- 3Centro de Fisiología y Medicina de Altura (FIMEDALT), Universidad de Antofagasta, Antofagasta, Chile
- 4Centro Interdisciplinario de Neurociencias de Valparaíso (CINV), Instituto de Neurociencias, Universidad de Valparaíso, Valparaíso, Chile
Plasma membrane ionic channels selectively permeate potassium, sodium, calcium, and chloride ions. However, large-pore channels are permeable to ions and small molecules such as ATP and glutamate, among others. Large-pore channels are structures formed by several protein families with little or no evolutionary linkages including connexins (Cxs), pannexins (Panxs), innexin (Inxs), unnexins (Unxs), calcium homeostasis modulator (CALHMs), and Leucine-rich repeat-containing 8 (LRRC8) proteins. Large-pore channels are key players in inflammatory cell response, guiding the activation of inflammasomes, the release of pro-inflammatory cytokines such as interleukin-1 beta (IL-1ß), and the release of adenosine-5′-triphosphate (ATP), which is considered a danger signal. This review summarizes our current understanding of large-pore channels and their contribution to inflammation induced by microorganisms, virulence factors or their toxins.
1 General Introduction
Plasma membrane ionic channels are necessary for fundamental cellular processes such as setting up resting membrane potentials, neuronal transmission, and the propagation of action potentials in electrically excitable cells (Bertil, 2001). Plasma membrane ionic channels selectively permeate ions (i.e., K+, Na+, Cl−, or Ca2+); However, large-pore channels are also permeable to small molecules, such as ATP, ADP, and NAD+, and glutamate, which contribute to physiological and pathophysiological responses (Kang et al., 2008; Cisterna et al., 2020; Syrjanen et al., 2021). Large-pore channels are structures formed by several protein families with no evolutionary linkages, including Cxs, Panxs, Inxs, Unxs, CALHMs, and LRRC8 proteins (Guiza et al., 2022). Despite little sequence homology, the large-pore channel members have similar transmembrane topologies with four transmembrane helices (Syrjanen et al., 2021).
Channels constituted by Cxs or Panxs are also termed hemichannels because they correspond to half of a gap junction channel (Orellana et al., 2012). The Cx gene family is present in vertebrates, and consists of 21 members in humans that differ by up to 29% in sequence identity (Laird & Lampe, 2018). Cx proteins form hexameric large-pore channels activated by proinflammatory cytokines, metabolic inhibition, depolarization, nitrosylation, dephosphorylation, and a divalent cation-free solution (Van Campenhout et al., 2021). The pannexin gene family is present in vertebrates, and consists of three members that differ by up to 75%–80% with Cxs in sequence identity (Panchin et al., 2000; Ruan et al., 2020). The Panx1 protein forms heptameric large-pore channels activated by phosphorylation by CAMKII, extracellular alkaline pH, and caspase cleavage (Penuela et al., 2013; Harcha et al., 2019; Lopez et al., 2021). Moreover, the Inx gene family is found exclusively in invertebrates and presents eight genes identified in Drosophila melanogaster, 25 in Caenorhabditis elegans, three in Hirudo verdana, and one gene in Hydra polyps (Guiza et al., 2018). Inx proteins form octameric large-pore channels activated by depolarization and mechanical stress (Guiza et al., 2018). Moreover, CALHM1 is a voltage- and Ca2+-gated channel that plays an essential role in the purinergic neurotransmission of sweet, bitter, and umami tastes (Taruno et al., 2013; Ma et al., 2016). CALHM1 form octameric large-pore channels in vertebrates, while the Caenorhabditis elegans CALHM1 assembles as non-amers, decamers, or undecamers (Demura et al., 2020; Ren et al., 2022). The VRACs are composed of LRRC8 proteins and are responsible for regulatory volume decreases after hypotonic cell swelling (Concepcion et al., 2022). Recent evidence suggests that kinetoplastid parasites have large-pore channel members formed by homologs of innexins, named unnexins, which seem to have a membrane topology similar to that of large-pore channels, and might play a critical role in infections (Guiza et al., 2022).
Cx hemichannels conduct K+, Na+, and Ca2+ (Mandal et al., 2015), and small molecules such as glutamate, glucose, NAD+, and ATP (Retamal et al., 2007; Anselmi et al., 2008; Okuda et al., 2013; Hansen et al., 2014). Panx1 hemichannels also permeate Cl−, ATP and glucose (Ma et al., 2012; Riquelme et al., 2013). VRAC channels conduct Cl− and other halide ions, but also transport a variety of organic molecules such as taurine, inositol, glutamate, as well as therapeutic agents such as cisplatin, carboplatin, and blasticidin S, and immunomodulatory cyclic dinucleotides such as 2′3′cGAMPs (Concepcion et al., 2022). Of particular interest for this review are channels formed by Cx43, CALHM1, Panx1 and innexins, which are permeable to ATP and Ca2+ ions (Locovei et al., 2006; Kang et al., 2008; Schalper et al., 2010; Ma et al., 2016; Shan et al., 2020). Because ATP externalization is the first step in the cascade of events leading to the maturation and secretion of IL-1ß and IL-18, multiple studies have proposed that large-pore channels play a relevant role in inflammation (Makarenkova and Shestopalov, 2014; Sáez and Green, 2018). Accordingly, blocking large-pore channels attenuates inflammation and prevents cell death (Makarenkova and Shestopalov, 2014; Sáez and Green, 2018).
2 Modulation of large-pore channels by bacteria and pathogen-associated molecular patterns and their role in bacterial diseases
Peptidoglycans (PGNs) are a cell wall component of Gram-positive bacteria considered to be a pathogen-associated molecular patterns (PAMPs) that can promote the generation of pro-inflammatory cytokines, such as tumor necrosis factor-alpha (TNF-alpha) and IL-1ß, which promote a systemic inflammatory response by activating Toll-like receptor two signaling (Amoureux et al., 2005; Robertson et al., 2010). Interestingly, PGNs can modulate large-pore channels (Robertson et al., 2010). For example, a PNG derived from Staphylococcus epidermidis (strain NCIMB 40896) has been shown to increase Cx43 hemichannel activity in HeLa-Cx43 cells, which can be prevented by LnCl3, carbenoxolone or Gap26 (Robertson et al., 2010). Moreover, carbenoxolone prevents the induction of IL-6 and TLR2 mRNA expression induced by PGN (Robertson et al., 2010).
Lipopolysaccharides (LPSs) of Gram-negative bacteria are potent proinflammatory PAMPs that can induce the expression of Cx43 or activate large-pore channels, hence promoting inflammation (Eugenin et al., 2001; Eugenin et al., 2003; Chae and Bothwell, 2019; Huang et al., 2020; Ma et al., 2020). For example, carbenoxolone (dose of 20 mg/kg for 30 min before LPS injection) has been shown to reduce the production of IL-1β, IL-6, and TNF-α, as well as tubular cell apoptosis in a model of sepsis-induced acute kidney injury (Huang et al., 2020). Silencing Panx1 was observed to decrease inflammatory cytokine production, apoptosis, NLRP3 inflammasome activation, and pro-apoptosis in LPS-treated HK-2 cells (Huang et al., 2020). Selective inhibition of Cx43 hemichannels also protects HUVEC cells from LPS-induced apoptosis (Ma et al., 2020). LPS from Escherichia coli (E. coli) (serotype O111:B4) increases Cx43 levels in the plasma membrane of HUVECs, and treatment with Gap19 reduces LPS-induced intracellular ROS and apoptotic levels in HUVECs (Ma et al., 2020). Along the same line of analysis, LPS (from E. coli serotype O111:B4) was seen to increase ethidium uptake in microglia, which can be blocked by probenecid, 10Panx1, and siRNA for Panx1 (Orellana et al., 2013a). Yet another study showed that LPS increases astroglial Cx43 hemichannel activity in acute hippocampal slices (Abudara et al., 2015). The LPS-induced Cx43 hemichannel activation in astrocytes is mediated, to a great extent, by pro-inflammatory cytokines released from activated microglia (Retamal et al., 2007). Prenatal exposure to LPS increases Cx43 and Panx1 hemichannel activity in reactive astrocytes in offspring (Avendano et al., 2015). In the periphery, LPS causes severe muscle deterioration due to higher sarcolemma permeability, and a decline in resting membrane potential (Cea et al., 2019).
In 2019, we demonstrated that mice treated for 5 h with LPS (from E. coli) induced the appearance of functional Cx hemichannels in myofibers freshly isolated from skeletal muscle (Cea et al., 2019). These results suggest that sarcolemmal dysfunction induced by endotoxemia is partially due to de novo expressions of functional Cx43-and Cx45-formed large-pore channels, which are also expressed in skeletal muscles during sepsis (Balboa et al., 2018; Cea et al., 2019). Interestingly, LPS-induced neuroinflammation was remarkably less in microglia from CALHM2 knock-out mice, suggesting the participation of microglia CALHM2 channels in the neuroinflammation produced by LPS (Cheng et al., 2021).
Shigella flexneri (S. flexneri) is the causative agent of bacillar dysentery, causing intestinal inflammation (Tran Van Nhieu et al., 2003). S. flexneri induces the activation of caspase-1, leading to pyroptotic cell death in macrophages (Suzuki et al., 2014). It is noteworthy that S. flexneri modulates large-pore channels to favor its spread and invasion (Tran Van Nhieu et al., 2003; Bonnet and Tran Van Nhieu, 2016). For example, S. flexneri (M90T strain) increases Lucifer yellow uptake in HeLa-Cx26 cells, whereas challenging with the non-invasive mxiD mutant strain induced minimal dye incorporation (Tran Van Nhieu et al., 2003). In addition, S. flexneri induces ATP release in HeLa-Cx26 cells—a response blocked by carbenoxolone, which is a derivative of 18-α-glycyrrhetinic acid (Tran Van Nhieu et al., 2003). Treatment with 18-α-glycyrrhetinic acid consistently reduced the number of infected cells per dissemination focus in HeLa-Cx26 (Tran Van Nhieu et al., 2003). Furthermore, Cx26 hemichannels facilitate gastrointestinal bacterial infection caused by E. coli (Simpson et al., 2013). A significant reduction in both cellular invasion and adherence by E. coli (E69 strain) was also demonstrated in human intestinal cell lines (Caco-2 and HT-29 cells) following treatment with Cx26 siRNA (Simpson et al., 2013). Moreover, the R143W Cx26 mutant causes a reduction in E. coli adherence (Simpson et al., 2013). Another study showed that Cx43 hemichannels are involved in the pathogenesis of Yersinia enterocolitica (Y. enterocolitica), which is a Gram-negative pathogen that causes a broad range of gastrointestinal syndromes (Velasquez-Almonacid et al., 2009). HeLa-Cx43 cells challenged with Y. enterocolitica resulted in higher bacterial uptake than parental cells (Velasquez-Almonacid et al., 2009). Y. enterocolitica also increased Lucifer yellow uptake, a response blocked by carbenoxolone in HeLa-Cx43 cells (Velasquez-Almonacid et al., 2009). Similarly, endotoxemia induces de novo expression of Cxs and upregulates Panx1 in skeletal muscles, where they are likely to form hemichannels (Balboa et al., 2018). Clostridioides difficile (C. difficile) is a Gram-positive, anaerobic toxin-producing bacillus that causes nosocomial diarrhea associated with antibiotic use (Loureiro et al., 2022). Infecting C57BL/6 mice with C. difficile (VPI10463 strain) was shown to increase levels of Panx1 in the cecum and colon (Loureiro et al., 2022). Blocking Panx1 with mimetic peptide 10Panx1 was observed to decrease caspase-3/7 activity and phosphatidylserine-annexin-V binding in toxin A- and toxin B-challenged enteric neurons and enteric glial cells (Loureiro et al., 2022).
Streptococcus pneumoniae, which is a major causative agent of bacterial meningitis, was shown to increase astroglial Cx43 hemichannel activity (Bello et al., 2020) (Table 1). The authors explained that purified pore-forming toxin pneumolysin promotes the Cx43-dependent release of extracellular ATP, and prolongs the increase of cytosolic Ca2+ in host cells (Bello et al., 2020).
Finally, bacteria have been shown to use innexin-formed large pore channels for their mechanism of infection in insects. For example, Vibrio alginolyticus, Vibrio parahaemolyticus, or LPS (from E. coli serotype O55:B5) can induce upregulation of Inx2 gene expression in hemocyte, gill, and hepatopancreas tissues in Scylla paramamosain (Wang et al., 2015). The authors indicated that ectopic expression of Sp-inx2 in HeLa and epithelioma papulosum cyprinid cells can induce apoptosis (Wang et al., 2015).
3 Modulation of large-pore channels by virus, and their role in viral diseases
Human immunodeficiency virus (HIV) causes a public health problem, and has claimed more than 35 million lives worldwide (Malik and Eugenin, 2019). HIV can modulate Cx43 or Panx1 hemichannels (Orellana et al., 2013b; Orellana et al., 2014). For example, HIV induces the opening of Panx1 hemichannels in CD4+ T lymphocytes (Orellana et al., 2013b). The 10Panx1 mimetic peptide inhibits HIV replication in CD4+ T lymphocytes (Orellana et al., 2013b). In a subsequent study, it was demonstrated that HIV causes the opening of Cx43 hemichannels, an effect that can be blocked by lanthanum ions, Cx43E2 antibodies, or mimetic peptide gap26 (Orellana et al., 2014). Another study showed that the simian immunodeficiency virus (SIV) causes Panx1 hemichannels to open in peripheral blood mononuclear cells isolated from SIV-infected macaques (Gorska et al., 2021). A subsequent study demonstrated that peripheral blood mononuclear cells isolated from HIV-infected individuals have a spontaneous opening of Panx1 hemichannels, which results in increased circulating ATP levels and prostaglandin E2 in the serum of all HIV-infected individuals (Velasquez et al., 2020). Moreover, the protein S from severe acute respiratory syndrome coronavirus 2 (SARS-CoV-2) induces a transient increase in ethidium uptake in human lung epithelial cells. This effect was blocked by probenecid or 10Panx1 peptide, suggesting the involvement of open Panx1 hemichannels. It should be noted that blocking Panx1 hemichannels reduces viral entry and replication in human lung epithelial cells, suggesting a critical role for Panx1 in SARS-CoV-2 infections (Luu et al., 2021) (Table 1). Moreover, the herpes simplex virus infection causes necrotic cell death in murine embryonic fibroblasts, a process that is often inversely correlated with an interferon response (Zhou et al., 2020). LRRC8A−/− cells were observed to exhibit higher viral loads after HSV-1 (KOS strain) infection, suggesting that VRACs participate in the propagation of the herpes simplex virus (Zhou et al., 2020). A comprehensive review of the structure and possible mechanisms underlying pore inhibition and modulation by targeting the intracellular leucine-rich repeat (LRR) domain has been recently reported (Pereira da silva et al., 2022).
4 Modulation of large-pore channels by parasites, and their role in parasitic diseases
Trypanosoma cruzi (T. cruzi) is a kinetoplastid parasite that causes Chagas disease in humans, which is characterized by severe cardiomyopathy and gastrointestinal motility disorders (Adesse et al., 2011). T. cruzi can also modulate intercellular communication via gap junctions in cardiac myocytes, brown adipocytes, astrocytes, and leptomeningeal cells (de Carvalho et al., 1992; Campos de Carvalho et al., 1998; Burke et al., 2014). Recently, we described that T. cruzi could modulate large-pore channels. Exposure to T. cruzi (H510 strain) was observed to increase Panx1 hemichannel activity in Hela-Panx1 (Barría et al., 2018). T. cruzi or supernatants from T. cruzi cultures (epimastigotes) also increase ethidium uptake in neonatal rat cardiac myocytes (Barría et al., 2018). 10Panx1 or probenecid prevents a T. cruzi-induced [Ca2+]i transient as well as T. cruzi invasion (Barría et al., 2018) (Table 1).
Leishmania is a protozoan parasite that causes leishmaniasis (Alvar et al., 2012). The three main clinical manifestations are cutaneous, mucocutaneous, and visceral leishmaniasis (Alvar et al., 2012). The Leishmania amazonensis (L. amazonensis) infection upregulates expression and function of the P2Y2 receptor in macrophages (Marques-da-Silva et al., 2011). Treatment with uridine triphosphate (UTP, P2Y2 agonist) reduces parasite load and triggers apoptosis in macrophages infected with L. amazonensis (Marques-da-Silva et al., 2011). The effect of apoptosis induced by UTP was not observed in L. amazonensis-infected macrophages from Panx1−/− mice (Thorstenberg et al., 2018). The authors suggest that the Panx1 and the P2X7 receptors would be involved in controlling L. amazonensis infection induced by uridine triphosphate treatment (Marques-da-Silva et al., 2011; Thorstenberg et al., 2018).
5 Conclusion and futures
The inflammatory process is a complex and multistep process, requiring the recruitment of various cells, such as fibroblasts, glial cells (Seo et al., 2021), endothelial cells (Kameritsch and Pogoda, 2020), resident leukocytes (Kameritsch and Pogoda, 2020), and mast cells, among others (Medzhitov, 2008; Medzhitov, 2021). In addition, most cells involved in inflammation express functional large-pore channels in their plasma membrane, where they play critical roles in inflammatory responses, mainly as exit routes for ATP in inflamed cells (Crespo Yanguas et al., 2017; Syrjanen et al., 2021). Upon ATP release, several enzymes degrade ATP into ADP, AMP, and adenosine, thus amplifying the response, given that they also signal through purinergic receptors (Fredholm et al., 1994; Ralevic and Burnstock, 1998; Velasquez and Eugenin, 2014). The importance of purinergic signaling in inflammation has been established in the last decades (see the following reviews: Idzko et al., 2014; Cekic and Linden, 2016; Dosch et al., 2018). However, the role of large-pore channels and purinergic signaling in inflammation generated by infectious disease has only recently been examined (Eugenin, 2014; Velasquez and Eugenin, 2014).
In bacterial infections, PAMPs activate hemichannels formed by Cx43, Cx26, or Panx1, causing an outflow of ATP. The latter, through the activation of P2X7 receptors, induces the activation of caspase 3/7 (Loureiro et al., 2022) and the production of IL-6 (Robertson et al., 2010; Loureiro et al., 2022). By activating P2X1 receptors, it can increase the concentration of cytosolic Ca2+ ions (Wang et al., 2017). Moreover, Panx1 is a target for caspases 3 and 7, whose activation results in a constitutively open channel and, therefore, more ATP release, which generates a feedforward mechanism that could be blocked by using Panx1 hemichannels or P2X receptor blockers (Chekeni et al., 2010; Narahari et al., 2021) (Figure 1A).
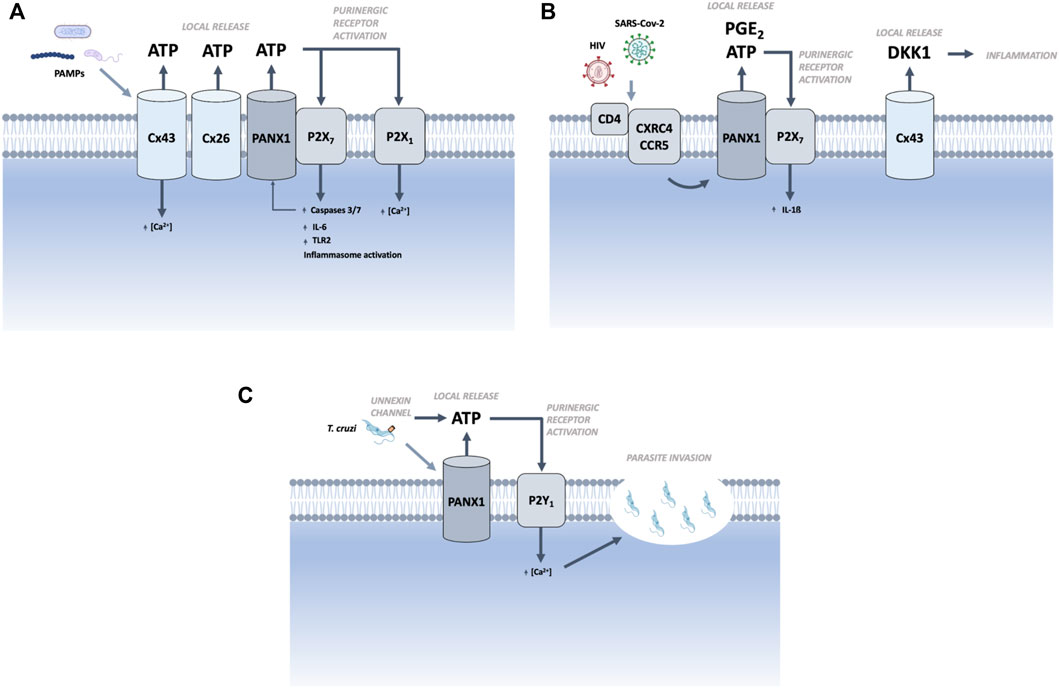
FIGURE 1. Proposed mechanism of contributions of large-pore channels to inflammation induced by microorganisms. (A) Extracellular bacteria or their toxins induce opening of Cx43, Cx26, and Panx1 hemichannels in host cell, causing an outflow of ATP. Extracellular ATP binds to specific purinergic receptors, enhancing inflammation. The opening of the Cx43 hemichannel allows for the entry of Ca2+. (B) Through the activation of CD4-CXCR4/CCR5, the virus induces the opening of Cx43 and Panx1 hemichannels in the host cell, causing an outflow of ATP, PGE2, and DKK1. (C) Trypanosomatids induce the opening of Panx1 hemichannels in the host cells, causing local ATP release. Through the activation of the P2Y1 receptor, ATP increases intracellular calcium ions and consequent invasion. The presence of hemichannels formed by unnexins in the parasite could be a tentative source of ATP. Figure was created with biorender.com.
In viral infections, SARS-CoV-2, hCoV-229E, or HIV activate Panx1 hemichannels via CD4/CXCR4 CD4/CCR5, causing an outflow of ATP, which induces the maturation of IL-1ß through the activation of P2X7 receptors (Orellana et al., 2013a; Luu et al., 2021) (Figure 1B). HIV can activate Cx43 hemichannels, inducing the release of dickkopf-1 (DKK1) proteins, which play a pivotal role in pathological inflammatory diseases (Orellana et al., 2014; Cae et al., 2019; Park et al., 2022). Therefore, Panx1 hemichannels blocker might be effective in treatments to reduce viral load.
In parasite infections, T. cruzi or T. cruzi-virulence factors have been described to activate Panx1 hemichannels in cardiac cells, causing an outflow of ATP, and increasing the concentration of cytosolic Ca2+ through the activation of P2Y1 receptors, which is necessary for parasite invasion (Barria et al., 2018) (Figure 1C). Recent evidence suggests the presence of members of large-pore channels in T. cruzi, which could be a route for ATP release, and could be considered for future research (Güiza et al., 2022).
Author contributions
JLV drafted the manuscript; MR drafted the figure; CG, JG, MR, and JCS critically revised the manuscript.
Funding
This work was partially written by JLV at the Centro Interdisciplinario de Neurociencia de Valparaíso (CINV), and was supported by the MINEDUC-UA project, code ANT 1999 (to JV), the Fondo Nacional de Desarrollo Científico y Tecnológico (FONDECYT) grant 1191329 (to JS), and as grant ICM-ANID for Project P09-022 awarded to the CINV (to JS).
Conflict of interest
The authors declare that the research was conducted in the absence of any commercial or financial relationships that could be construed as a potential conflict of interest.
Publisher’s note
All claims expressed in this article are solely those of the authors and do not necessarily represent those of their affiliated organizations, or those of the publisher, the editors and the reviewers. Any product that may be evaluated in this article, or claim that may be made by its manufacturer, is not guaranteed or endorsed by the publisher.
References
Abudara, V., Roux, L., Dallerac, G., Matias, I., Dulong, J., Mothet, J. P., et al. (2015). Activated microglia impairs neuroglial interaction by opening Cx43 hemichannels in hippocampal astrocytes. Glia 63, 795–811. doi:10.1002/glia.22785
Adesse, D., Goldenberg, R. C., Fortes, F. S., JasminIacobas, D. A., Iacobas, S., De carvalho, A. C. C, et al. (2011). Gap junctions and chagas disease. Adv. Parasitol. 76, 63–81. doi:10.1016/B978-0-12-385895-5.00003-7
Alvar, J., Vélez, I. D., Bern, C., Herrero, M., Desjeux, P., Cano, J., et al. (2012). Leishmaniasis worldwide and global estimates of its incidence. PLoS One 7, e35671. doi:10.1371/journal.pone.0035671
Amoureux, M. C., Rajapakse, N., Stipkovits, L., and Szathmary, S. (2005). Peptidoglycan and bacterial DNA induce inflammation and coagulation markers in synergy. Mediat. Inflamm. 2005, 118–120. doi:10.1155/MI.2005.118
Anselmi, F., Hernandez, V. H., Crispino, G., Seydel, A., Ortolano, S., Roper, S. D., et al. (2008). ATP release through connexin hemichannels and gap junction transfer of second messengers propagate Ca2+ signals across the inner ear. Proc. Natl. Acad. Sci. U. S. A. 105, 18770–18775. doi:10.1073/pnas.0800793105
Avendano, B. C., Montero, T. D., Chavez, C. E., Von Bernhardi, R., and Orellana, J. A. (2015). Prenatal exposure to inflammatory conditions increases Cx43 and Panx1 unopposed channel opening and activation of astrocytes in the offspring effect on neuronal survival. Glia 63, 2058–2072. doi:10.1002/glia.22877
Balboa, E., Saavedra-Leiva, F., Cea, L. A., Vargas, A. A., Ramírez, V., Escamilla, R., et al. (2018). Sepsis-induced channelopathy in skeletal muscles is associated with expression of non-selective channels. Shock 49, 221–228. doi:10.1097/SHK.0000000000000916
Barria, I., Guiza, J., Cifuentes, F., Zamorano, P., Sáez, J. C., Gonzalez, J., et al. (2018). Trypanosoma cruzi infection induces pannexin-1 channel opening in cardiac myocytes. Am. J. Trop. Med. Hyg. 98, 105–112. doi:10.4269/ajtmh.17-0293
Bello, C., Smail, Y., Sainte-Rose, V., Podglajen, I., Gilbert, A., Moreira, V., et al. (2020). Role of astroglial Connexin 43 in pneumolysin cytotoxicity and during pneumococcal meningitis. PLoS Pathog. 16, e1009152. doi:10.1371/journal.ppat.1009152
Bonnet, M., and Tran Van Nhieu, G. (2016). How Shigella utilizes Ca(2+) jagged edge signals during invasion of epithelial cells. Front. Cell Infect. Microbiol. 6, 16. doi:10.3389/fcimb.2016.00016
Burke, S., Nagajyothi, F., Thi, M. M., Hanani, M., Scherer, P. E., Tanowitz, H. B., et al. (2014). Adipocytes in both Brown and white adipose tissue of adult mice are functionally connected via gap junctions: Implications for chagas disease. Microbes Infect. 16, 893–901. doi:10.1016/j.micinf.2014.08.006
Campos De Carvalho, A. C., Roy, C., Hertzberg, E. L., Tanowitz, H. B., Kessler, J. A., Weiss, L. M., et al. (1998). Gap junction disappearance in astrocytes and leptomeningeal cells as a consequence of protozoan infection. Brain Res. 790, 304–314. doi:10.1016/s0006-8993(97)01523-0
Cea, L. A., Balboa, E., Vargas, A. A., Puebla, C., Branes, M. C., Escamilla, R., et al. (2019). De novo expression of functional connexins 43 and 45 hemichannels increases sarcolemmal permeability of skeletal myofibers during endotoxemia. Biochim. Biophys. Acta Mol. Basis Dis. 1865, 2765–2773. doi:10.1016/j.bbadis.2019.06.014
Cekic, C., and Linden, J. (2016). Purinergic regulation of the immune system. Nat. Rev. Immunol. 16, 177–192. doi:10.1038/nri.2016.4
Chae, W. J., and Bothwell, A. L. M. (2019). Dickkopf1: An immunomodulatory ligand and Wnt antagonist in pathological inflammation. Differentiation 108, 33–39. doi:10.1016/j.diff.2019.05.003
Chekeni, F. B., Elliott, M. R., Sandilos, J. K., Walk, S. F., Kinchen, J. M., Lazarowski, E. R., et al. (2010). Pannexin 1 channels mediate 'find-me' signal release and membrane permeability during apoptosis. Nature 467, 863–867. doi:10.1038/nature09413
Cheng, J., Dong, Y., Ma, J., Pan, R., Liao, Y., Kong, X., et al. (2021). Microglial Calhm2 regulates neuroinflammation and contributes to Alzheimer's disease pathology. Sci. Adv. 7, eabe3600. doi:10.1126/sciadv.abe3600
Cisterna, B. A., Vargas, A. A., Puebla, C., Fernández, P., Escamilla, R., Lagos, C. F., et al. (2020). Active acetylcholine receptors prevent the atrophy of skeletal muscles and favor reinnervation. Nat. Commun. 11, 1073. doi:10.1038/s41467-019-14063-8
Concepcion, A. R., Wagner, L. E., Zhu, J., Tao, A. Y., Yang, J., Khodadadi-Jamayran, A., et al. (2022). The volume-regulated anion channel LRRC8C suppresses T cell function by regulating cyclic dinucleotide transport and STING-p53 signaling. Nat. Immunol. 23, 287–302. doi:10.1038/s41590-021-01105-x
Crespo Yanguas, S., Willebrords, J., Johnstone, S. R., Maes, M., Decrock, E., De Bock, M., et al. (2017). Pannexin1 as mediator of inflammation and cell death. Biochim. Biophys. Acta Mol. Cell Res. 1864, 51–61. doi:10.1016/j.bbamcr.2016.10.006
De Carvalho, A. C., Tanowitz, H. B., Wittner, M., Dermietzel, R., Roy, C., Hertzberg, E. L., et al. (1992). Gap junction distribution is altered between cardiac myocytes infected with Trypanosoma cruzi. Circ. Res. 70, 733–742. doi:10.1161/01.res.70.4.733
Demura, K., Kusakizako, T., Shihoya, W., Hiraizumi, M., Nomura, K., Shimada, H., et al. (2020). Cryo-EM structures of calcium homeostasis modulator channels in diverse oligomeric assemblies. Sci. Adv. 6, eaba8105. doi:10.1126/sciadv.aba8105
Dosch, M., Gerber, J., Jebbawi, F., and Beldi, G. (2018). Mechanisms of ATP release by inflammatory cells. Int. J. Mol. Sci. 19, 1222. doi:10.3390/ijms19041222
Eugenín, E. A., Brañes, M. C., Berman, J. W., and Sáez, J. C. (2003). TNF-alpha plus IFN-gamma induce connexin43 expression and formation of gap junctions between human monocytes/macrophages that enhance physiological responses. J. Immunol. 170, 1320–1328. doi:10.4049/jimmunol.170.3.1320
Eugenín, E. A., Eckardt, D., Theis, M., Willecke, K., Bennett, M. V. L., and Sáez, J. C. (2001). Microglia at brain stab wounds express connexin 43 and in vitro form functional gap junctions after treatment with interferon-gamma and tumor necrosis factor-alpha. Proc. Natl. Acad. Sci. U.S.A. 98, 4190–4195. doi:10.1073/pnas.051634298
Eugenin, E. A. (2014). Role of connexin/pannexin containing channels in infectious diseases. FEBS Lett. 588, 1389–1395. doi:10.1016/j.febslet.2014.01.030
Fredholm, B. B., Abbracchio, M. P., Burnstock, G., Daly, J. W., Harden, T. K., Jacobson, K. A., et al. (1994). Nomenclature and classification of purinoceptors. Pharmacol. Rev. 46, 143–156.
Gorska, A. M., Donoso, M., Valdebenito, S., Prideaux, B., Queen, S., Scemes, E., et al. (2021). Human immunodeficiency virus-1/simian immunodeficiency virus infection induces opening of pannexin-1 channels resulting in neuronal synaptic compromise: A novel therapeutic opportunity to prevent NeuroHIV. J. Neurochem. 158, 500–521. doi:10.1111/jnc.15374
Guiza, J., Barria, I., Saez, J. C., and Vega, J. L. (2018). Innexins: Expression, regulation, and functions. Front. Physiol. 9, 1414. doi:10.3389/fphys.2018.01414
Guiza, J., Garcia, A., Arriagada, J., Gutierrez, C., Gonzalez, J., Marquez-Miranda, V., et al. (2022). Unnexins: Homologs of innexin proteins in Trypanosomatidae parasites. J. Cell Physiol. 237, 1547–1560. doi:10.1002/jcp.30626
Hansen, D. B., Braunstein, T. H., Nielsen, M. S., and MacAulay, N. (2014). Distinct permeation profiles of the connexin 30 and 43 hemichannels. FEBS Lett. 588, 1446–1457. doi:10.1016/j.febslet.2014.01.036
Harcha, P. A., López, X., Sáez, P. J., Fernández, P., Barría, I., Martínez, A. D., et al. (2019). Pannexin-1 channels are essential for mast cell degranulation triggered during type I hypersensitivity reactions. Front. Immunol. 10, 2703. doi:10.3389/fimmu.2019.02703
Huang, G., Bao, J., Shao, X., Zhou, W., Wu, B., Ni, Z., et al. (2020). Inhibiting pannexin-1 alleviates sepsis-induced acute kidney injury via decreasing NLRP3 inflammasome activation and cell apoptosis. Life Sci. 254, 117791. doi:10.1016/j.lfs.2020.117791
Idzko, M., Ferrari, D., and Eltzschig, H. K. (2014). Nucleotide signalling during inflammation. Nature 509, 310–317. doi:10.1038/nature13085
Kameritsch, P., and Pogoda, K. (2020). The role of connexin 43 and pannexin 1 during acute inflammation. Front. Physiol. 11, 594097. doi:10.3389/fphys.2020.594097
Kang, J., Kang, N., Lovatt, D., Torres, A., Zhao, Z., Lin, J., et al. (2008). Connexin 43 hemichannels are permeable to ATP. J. Neurosci. 28, 4702–4711. doi:10.1523/JNEUROSCI.5048-07.2008
Laird, D. W., and Lampe, P. D. (2018). Therapeutic strategies targeting connexins. Nat. Rev. Drug Discov. 17, 905–921. doi:10.1038/nrd.2018.138
Locovei, S., Bao, L., and Dahl, G. (2006). Pannexin 1 in erythrocytes: Function without a gap. Proc. Natl. Acad. Sci. U. S. A. 103, 7655–7659. doi:10.1073/pnas.0601037103
López, X., Palacios-Prado, N., Güiza, J., Escamilla, R., Fernández, P., Vega, J. L., et al. (2021). A physiologic rise in cytoplasmic calcium ion signal increases pannexin1 channel activity via a C-terminus phosphorylation by CaMKII. Proc. Natl. Acad. Sci. U. S. A. 118, e2108967118. doi:10.1073/pnas.2108967118
Loureiro, A. V., Moura-Neto, L. I., Martins, C. S., Silva, P. I. M., Lopes, M. B. S., Leitao, R. F. C., et al. (2022). Role of Pannexin-1-P2X7R signaling on cell death and pro-inflammatory mediator expression induced by Clostridioides difficile toxins in enteric glia. Front. Immunol. 13, 956340. doi:10.3389/fimmu.2022.956340
Luu, R., Valdebenito, S., Scemes, E., Cibelli, A., Spray, D. C., Rovegno, M., et al. (2021). Pannexin-1 channel opening is critical for COVID-19 pathogenesis. iScience 24, 103478. doi:10.1016/j.isci.2021.103478
Ma, W., Compan, V., Zheng, W., Martin, E., North, R. A., Verkhratsky, A., et al. (2012). Pannexin 1 forms an anion-selective channel. Pflugers Arch. 463, 585–592. doi:10.1007/s00424-012-1077-z
Ma, Z., Tanis, J. E., Taruno, A., and Foskett, J. K. (2016). Calcium homeostasis modulator (CALHM) ion channels. Pflugers Arch. 468, 395–403. doi:10.1007/s00424-015-1757-6
Ma, J. W., Ji, D. D., Li, Q. Q., Zhang, T., and Luo, L. (2020). Inhibition of connexin 43 attenuates oxidative stress and apoptosis in human umbilical vein endothelial cells. BMC Pulm. Med. 20, 19. doi:10.1186/s12890-019-1036-y
Makarenkova, H. P., and Shestopalov, V. I. (2014). The role of pannexin hemichannels in inflammation and regeneration. Front. Physiol. 5, 63. doi:10.3389/fphys.2014.00063
Malik, S., and Eugenin, E. A. (2019). Role of connexin and pannexin containing channels in HIV infection and NeuroAIDS. Neurosci. Lett. 695, 86–90. doi:10.1016/j.neulet.2017.09.005
Mandal, A., Shahidullah, M., and Delamere, N. A. (2015). Calcium entry via connexin hemichannels in lens epithelium. Exp. Eye Res. 132, 52–58. doi:10.1016/j.exer.2015.01.012
Medzhitov, R. (2008). Origin and physiological roles of inflammation. Nature 454, 428–435. doi:10.1038/nature07201
Medzhitov, R. (2021). The spectrum of inflammatory responses. Science 374, 1070–1075. doi:10.1126/science.abi5200
Narahari, A. K., Kreutzberger, A. J., Gaete, P. S., Chiu, Y. H., Leonhardt, S. A., Medina, C. B., et al. (2021). ATP and large signaling metabolites flux through caspase-activated Pannexin 1 channels. Elife 10, e64787. doi:10.7554/eLife.64787
Okuda, H., Nishida, K., Higashi, Y., and Nagasawa, K. (2013). NAD(+) influx through connexin hemichannels prevents poly(ADP-ribose) polymerase-mediated astrocyte death. Life Sci. 92, 808–814. doi:10.1016/j.lfs.2013.02.010
Orellana, J. A., Montero, T. D., and Von Bernhardi, R. (2013a). Astrocytes inhibit nitric oxide-dependent Ca(2+) dynamics in activated microglia: Involvement of ATP released via pannexin 1 channels. Glia 61, 2023–2037. doi:10.1002/glia.22573
Orellana, J. A., Sáez, J. C., Bennett, M. V., Berman, J. W., Morgello, S., and Eugenin, E. A. (2014). HIV increases the release of dickkopf-1 protein from human astrocytes by a Cx43 hemichannel-dependent mechanism. J. Neurochem. 128, 752–763. doi:10.1111/jnc.12492
Orellana, J. A., Velasquez, S., Williams, D. W., Sáez, J. C., Berman, J. W., and Eugenin, E. A. (2013b). Pannexin1 hemichannels are critical for HIV infection of human primary CD4+ T lymphocytes. J. Leukoc. Biol. 94, 399–407. doi:10.1189/jlb.0512249
Orellana, J. A., von Bernhardi, R., Giaume, C., and Sáez, J. C. (2012). Glial hemichannels and their involvement in aging and neurodegenerative diseases. Rev. Neurosci. 23, 163–177. doi:10.1515/revneuro-2011-0065
Panchin, Y., Kelmanson, I., Matz, M., Lukyanov, K., Usman, N., and Lukyanov, S. (2000). A ubiquitous family of putative gap junction molecules. Curr. Biol. 10, R473–R474. doi:10.1016/s0960-9822(00)00576-5
Park, M. H., Shin, J. H., Bothwell, A. L. M., and Chae, W. J. (2022). Dickkopf proteins in pathological inflammatory diseases. J. Leukoc. Biol. 111, 893–901. doi:10.1002/JLB.3RI0721-385R
Penuela, S., Gehi, R., and Laird, D. W. (2013). The biochemistry and function of pannexin channels. Biochim. Biophys. Acta 1828, 15–22. doi:10.1016/j.bbamem.2012.01.017
Pereira Da Silva, E. A., Martin-Aragon Baudel, M., Navedo, M. F., and Nieves-Cintron, M. (2022). Ion channel molecular complexes in vascular smooth muscle. Front. Physiol. 13, 999369. doi:10.3389/fphys.2022.999369
Ralevic, V., and Burnstock, G. (1998). Receptors for purines and pyrimidines. Pharmacol. Rev. 50, 413–492.
Ren, Y., Li, Y., Wang, Y., Wen, T., Lu, X., Chang, S., et al. (2022). Cryo-EM structure of the heptameric calcium homeostasis modulator 1 channel. J. Biol. Chem. 298, 101838. doi:10.1016/j.jbc.2022.101838
Retamal, M. A., Froger, N., Palacios-Prado, N., Ezan, P., Sáez, P. J., Sáez, J. C., et al. (2007). Cx43 hemichannels and gap junction channels in astrocytes are regulated oppositely by proinflammatory cytokines released from activated microglia. J. Neurosci. 27, 13781–13792. doi:10.1523/JNEUROSCI.2042-07.2007
Riquelme, M. A., Cea, L. A., Vega, J. L., Boric, M. P., Monyer, H., Bennett, M. V., et al. (2013). The ATP required for potentiation of skeletal muscle contraction is released via pannexin hemichannels. Neuropharmacology 75, 594–603. doi:10.1016/j.neuropharm.2013.03.022
Robertson, J., Lang, S., Lambert, P. A., and Martin, P. E. (2010). Peptidoglycan derived from Staphylococcus epidermidis induces Connexin43 hemichannel activity with consequences on the innate immune response in endothelial cells. Biochem. J. 432, 133–143. doi:10.1042/BJ20091753
Ruan, Z., Orozco, I. J., Du, J., and Lu, W. (2020). Structures of human pannexin 1 reveal ion pathways and mechanism of gating. Nature 584, 646–651. doi:10.1038/s41586-020-2357-y
Sáez, J. C., and Green, C. (2018). Involvement of connexin hemichannels in the inflammatory response of chronic diseases. Int. J. Mol. Sci. 19, 2469. doi:10.3390/ijms19092469
Schalper, K. A., Sánchez, H. A., Lee, S. C., Altenberg, G. A., Nathanson, M. H., and Sáez, J. C. (2010). Connexin 43 hemichannels mediate the Ca2+ influx induced by extracellular alkalinization. Am. J. Physiol. Cell Physiol. 299, C1504–C1515. doi:10.1152/ajpcell.00015.2010
Seo, J. H., Dalal, M. S., and Contreras, J. E. (2021). Pannexin-1 channels as mediators of neuroinflammation. Int. J. Mol. Sci. 22, 5189. doi:10.3390/ijms22105189
Shan, Y., Ni, Y., and Gao, Z. (2020). Pannexin-1 channel regulates ATP release in epilepsy. Neurochem. Res. 45, 965–971. doi:10.1007/s11064-020-02981-9
Simpson, C., Kelsell, D. P., and Marches, O. (2013). Connexin 26 facilitates gastrointestinal bacterial infection in vitro. Cell Tissue Res. 351, 107–116. doi:10.1007/s00441-012-1502-9
Suzuki, S., Franchi, L., He, Y., Munoz-Planillo, R., Mimuro, H., Suzuki, T., et al. (2014). Shigella type III secretion protein MxiI is recognized by Naip2 to induce Nlrc4 inflammasome activation independently of Pkcδ. PLoS Pathog. 10, e1003926. doi:10.1371/journal.ppat.1003926
Syrjanen, J., Michalski, K., Kawate, T., and Furukawa, H. (2021). On the molecular nature of large-pore channels. J. Mol. Biol. 433, 166994. doi:10.1016/j.jmb.2021.166994
Taruno, A., Vingtdeux, V., Ohmoto, M., Ma, Z., Dvoryanchikov, G., Li, A., et al. (2013). CALHM1 ion channel mediates purinergic neurotransmission of sweet, bitter and umami tastes. Nature 495, 223–226. doi:10.1038/nature11906
Tran Van Nhieu, G., Clair, C., Bruzzone, R., Mesnil, M., Sansonetti, P., and Combettes, L. (2003). Connexin-dependent inter-cellular communication increases invasion and dissemination of Shigella in epithelial cells. Nat. Cell Biol. 5, 720–726. doi:10.1038/ncb1021
Van Campenhout, R., Gomes, A. R., De Groof, T. W. M., Muyldermans, S., Devoogdt, N., and Vinken, M. (2021). Mechanisms underlying connexin hemichannel activation in disease. Int. J. Mol. Sci. 22, 3503. doi:10.3390/ijms22073503
Velasquez Almonacid, L. A., Tafuri, S., Dipineto, L., Matteoli, G., Fiorillo, E., Della Morte, R., et al. (2009). Role of connexin-43 hemichannels in the pathogenesis of Yersinia enterocolitica. Vet. J. 182, 452–457. doi:10.1016/j.tvjl.2008.08.011
Velasquez, S., and Eugenin, E. A. (2014). Role of Pannexin-1 hemichannels and purinergic receptors in the pathogenesis of human diseases. Front. Physiol. 5, 96. doi:10.3389/fphys.2014.00096
Velasquez, S., Prevedel, L., Valdebenito, S., Gorska, A. M., Golovko, M., Khan, N., et al. (2020). Circulating levels of ATP is a biomarker of HIV cognitive impairment. EBioMedicine 51, 102503. doi:10.1016/j.ebiom.2019.10.029
Wang, S. P., Chen, F. Y., Dong, L. X., Zhang, Y. Q., Chen, H. Y., Qiao, K., et al. (2015). A novel innexin2 forming membrane hemichannel exhibits immune responses and cell apoptosis in Scylla paramamosain. Fish. Shellfish Immunol. 47, 485–499. doi:10.1016/j.fsi.2015.09.028
Wang, X., Qin, W., Xu, X., Xiong, Y., Zhang, Y., Zhang, H., et al. (2017). Endotoxin-induced autocrine ATP signaling inhibits neutrophil chemotaxis through enhancing myosin light chain phosphorylation. Proc. Natl. Acad. Sci. U. S. A. 114, 4483–4488. doi:10.1073/pnas.1616752114
Keywords: connexin, pannexin, innexin, LRRC8, CALHM, infectious disease
Citation: Vega JL, Gutiérrez C, Rojas M, Güiza J and Sáez JC (2023) Contribution of large-pore channels to inflammation induced by microorganisms. Front. Cell Dev. Biol. 10:1094362. doi: 10.3389/fcell.2022.1094362
Received: 10 November 2022; Accepted: 22 December 2022;
Published: 09 January 2023.
Edited by:
Marc Mesnil, University of Poitiers, FranceReviewed by:
Verónica Abudara, Universidad de la República, UruguayCopyright © 2023 Vega, Gutiérrez, Rojas, Güiza and Sáez. This is an open-access article distributed under the terms of the Creative Commons Attribution License (CC BY). The use, distribution or reproduction in other forums is permitted, provided the original author(s) and the copyright owner(s) are credited and that the original publication in this journal is cited, in accordance with accepted academic practice. No use, distribution or reproduction is permitted which does not comply with these terms.
*Correspondence: José L. Vega, am9zZWx1aXMudmVnYUB1YW50b2YuY2w=