- Department of Biochemistry and Molecular Biotechnology, University of Massachusetts Chan Medical School, Worcester, MA, United States
RNA passed from parents to progeny controls several aspects of early development. The germline of the free-living nematode Caenorhabditis elegans contains many families of evolutionarily conserved RNA-binding proteins (RBPs) that target the untranslated regions of mRNA transcripts to regulate their translation and stability. In this review, we summarize what is known about the binding specificity of C. elegans germline RNA-binding proteins and the mechanisms of mRNA regulation that contribute to their function. We examine the emerging role of miRNAs in translational regulation of germline and embryo development. We also provide an overview of current technology that can be used to address the gaps in our understanding of RBP regulation of mRNAs. Finally, we present a hypothetical model wherein multiple 3′UTR-mediated regulatory processes contribute to pattern formation in the germline to ensure the proper and timely localization of germline proteins and thus a functional reproductive system.
Introduction
In sexually reproducing organisms, post-transcriptional regulation of maternal and paternal mRNAs is crucial to gametogenesis and early embryo development (Wickens, 1990; Richter, 1991; Curtis et al., 1995; DeRenzo and Seydoux, 2004; Farley and Ryder, 2008; Kang and Han, 2011). During embryogenesis, control of the levels and localization of specific mRNAs and proteins is key for axis formation and cell fate specification (Zhang et al., 2014). Components of these regulatory processes include RNA-binding proteins (RBPs) and/or regulatory small RNAs that affect the stability of the mRNA transcript and ultimately influence protein levels (Shaw et al., 2010). Delineating the mechanisms of post-transcriptional regulation and how they mediate transfer of information from parent to progeny at the mRNA level will provide a deeper understanding of reproduction and early development as well as how misregulation of such processes can lead to disease.
C. elegans is a well-suited model organism to study the biology of RBP-mRNA networks and how they regulate early development. They have a large brood size (∼300), develop from an egg to an adult in a short time (2–3 days), and are self-fertile (Corsi et al., 2015). Not only is this nematode easy to grow and maintain, but there is a plethora of genetic and biochemical techniques that enable addressing important questions in the field of RNA regulation in this organism. CRISPR/Cas9 genome editing is straightforward in the worm and has become a standard technique for genetic manipulation (Kim and Colaiacovo, 2019; Vicencio and Ceron, 2021). Many of C. elegans developmental and molecular phenotypes are easy to score and analyze (Corsi et al., 2015). Additionally, both in vitro and in vivo biochemical methods have been developed to study many aspects of protein-RNA interactions (Ryder et al., 2004; Jedamzik and Eckmann, 2009; Jungkamp et al., 2011; Wright et al., 2011; Ryder, 2016).
C. elegans exist as either sperm-producing males or hermaphrodites that produce both sperm and oocytes from the same germline. Hermaphrodites undergo spermatogenesis during the fourth larval stage and switch to oogenesis during adulthood. The gonads consist of two symmetrical arms. Each arm is a tubular syncytium in which all nuclei share a cytoplasm termed the rachis. In each arm, a distal tip cell provides a niche for progenitor germ cells to divide by mitosis and proliferate and then enter meiosis I. These meiotic nuclei start to fully cellularize in the gonadal arm bend and form oocytes (Figure 1A). As the oocytes approach the proximal end, they get fertilized by the sperm, stored in the spermatheca, and then get delivered to the uterus where the 1-cell embryo undergoes multiple cellular divisions before exiting the animal. Posterior/anterior axis determination and cell fate specification take place during early cellular divisions (Figure 1B). After the eggs hatch, the first larval stage (L1) animals undergo four molts before reaching adulthood (Hubbard and Greenstein, 2005; Goupil et al., 2017; Bauer et al., 2021).
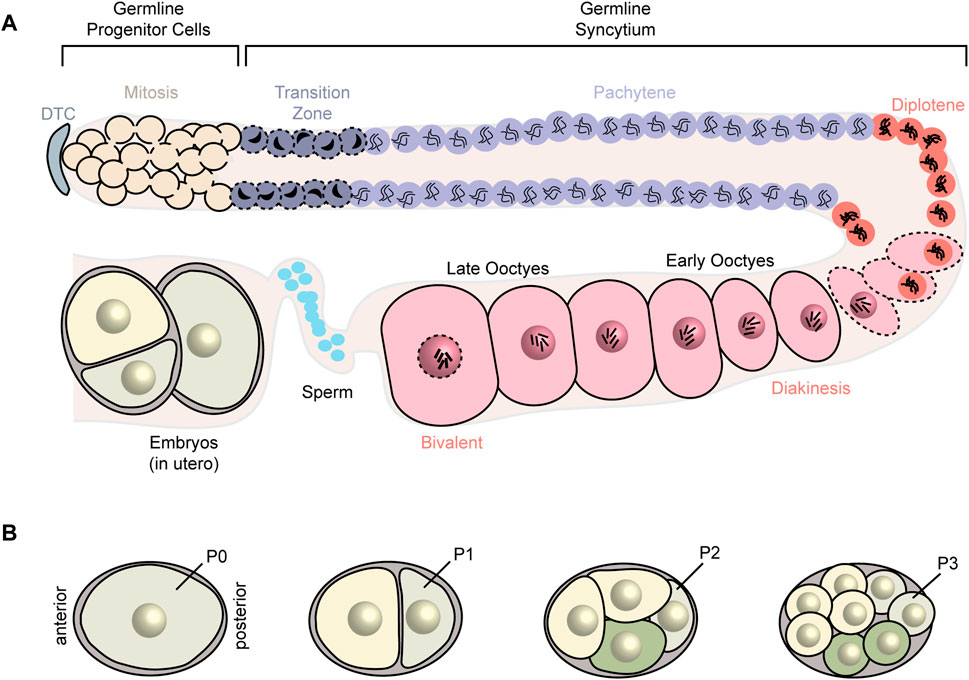
FIGURE 1. Schematic of C. elegans germline and early embryonic stages. (A) C. elegans gonads consist of two symmetrical arms (one arm represented). In each arm, a distal tip cell (DTC) provides the niche for germ cells to mitotically divide. These germ cells begin to enter meiosis I as they move farther from the DTC. The meiotically dividing cells begin to recellularize around the loop region and form early oocytes which then undergo oocyte maturation and fertilization. (B) Axis determination and cell fate specification occurs in the early embryo.
RNA-bindings proteins (RBPs) are essential to cellular function. They contribute to every stage in the journey of an RNA transcript from pre-mRNA splicing, mRNA capping and polyadenylation, to post-transcriptional regulation in the cytoplasm. RBPs vary widely in their RNA-binding domain structure, target binding specificity, and biological function (Burd and Dreyfuss, 1994). Many RNA-binding domains such as hnRNP K homology (KH) domain, RNA recognition motifs (RRM), and zinc-finger domains are highly conserved across metazoans. Some RBPs bind single stranded RNA while others bind dsRNA. Some RBPs such as the ribosomal proteins are more universally expressed and are highly abundant. Others-such as RBPs that regulate germline development-are both spatially and temporally restricted in their expression patterns (Burd and Dreyfuss, 1994; Lee and Schedl, 2006; Hentze et al., 2018).
The purpose of this review is to provide an update on how these RBPs direct mRNA regulation during germline development and embryogenesis and outline new approaches that can be used to define their relative importance to reproductive biology. In the past few decades, numerous studies have identified and characterized dozens of germline RNA-binding proteins in Caenorhabditis elegans (Figures 2, 3). Many of these proteins contain highly conserved RNA-binding domains that bind the UTRs of their target mRNAs to control different aspects of development including spermatogenesis, spermatogenesis to oogenesis switch in hermaphrodites, oogenesis, and early embryo development (Lee and Schedl, 2006). These germline RBPs can influence the fate of their target mRNAs by modifying their poly(A) tail length and thus mRNA stability, and/or controlling translational efficiency. Together, these RBP-mRNA networks maintain a functional reproductive system required for the animal to effectively reproduce.
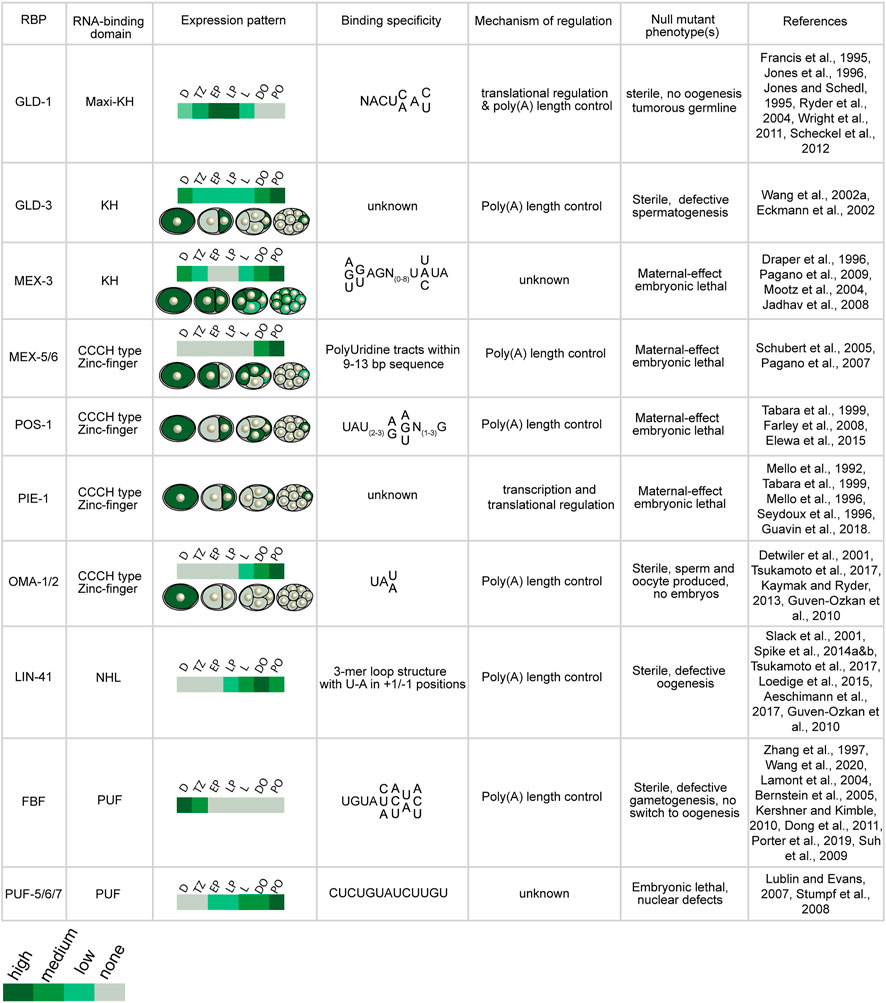
FIGURE 2. Expression and activities of germline RNA-binding proteins discussed in this manuscript. C. elegans germline RBPs vary in their RNA-binding domain, spatiotemporal expression pattern, binding specificity, and mode of post-transcriptional regulation (D: distal, TZ: transition zone, EP: early pachytene, LP: late pachytene, L: loop region, DO: distal oocytes, PO: proximal oocytes).
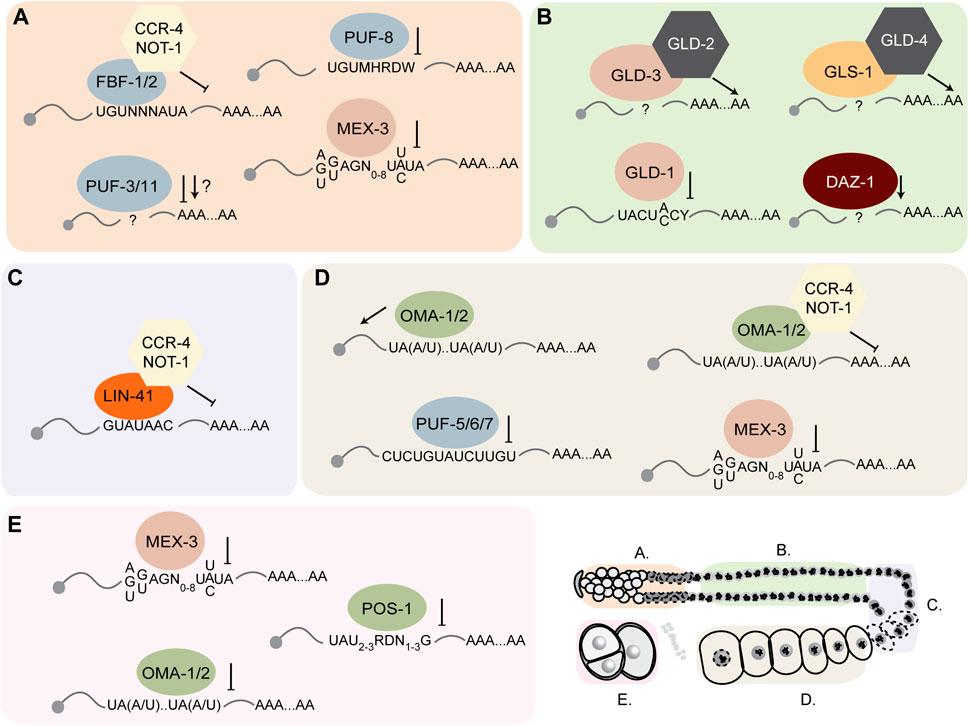
FIGURE 3. C. elegans germline RNA-binding proteins act on common and distinct maternal mRNA targets. In each region of the germline, several RBPs bind specific 3′UTR cis-regulatory elements in their target mRNA to regulate their stability or translational efficiency. (A-E) correspond to the region of the germline indicated in the key at the bottom right corner of the figure.
C. elegans RNA-binding proteins
The C. elegans genome encodes numerous RBPs. One estimate predicts that 887 of C. elegans genes encode a protein containing at least one of 17 annotated RNA binding domains (Tamburino et al., 2013). This study, designed to identify all proteins that associate with mRNA in C. elegans by interactome capture, detected 594 such RBPs. Many contain evolutionarily conserved domains that are found in other model organisms. For instance, more than half of C. elegans RBPs have homologues in S. cerevisiae (Matia-Gonzalez et al., 2015). This suggests that post-transcriptional regulation by RBPs is an ancient and evolutionarily conserved mechanism that predates metazoa.
Germline RBPs regulate translation of germline mRNAs through their 3′UTR or 5′UTR to control their spatiotemporal expression pattern (Zhang et al., 1997; Marin and Evans, 2003; Jadhav et al., 2008; Merritt et al., 2008; Suh et al., 2009; Farley and Ryder, 2012; Kaymak and Ryder, 2013; Nousch and Eckmann, 2013; Theil et al., 2018). Intriguingly, transcripts encoding RBPs tend to have long 3′UTRs compared to other genes; the median length estimate is 156 nucleotides compared to the overall average of 129 nucleotides. RBP genes also tend to encode multiple alternatively spliced isoforms (Tamburino et al., 2013). Both features reflect the potential for additional layers of regulation that act at the mRNA level for transcripts that encode regulatory RBPs. RBPs are also frequently regulated at the protein level. They tend to have more phosphorylation sites that can affect their function and activity (Tamburino et al., 2013). For example, the kinase MBK-2 phosphorylates the RBPs MEG-1 and MEG-3 leading to P-granule disassembly (Wang et al., 2014), and the polo-like kinase PLK-1 was shown to phosphorylate the zinc finger RBP POS-1 to promote its diffusion and therefore its asymmetric localization in the early embryo (Han et al., 2018). PAR-4, another kinase, is thought to phosphorylate and inactivate the KH domain-containing RBP MEX-3 during early embryo development to promote soma/germline asymmetry (Huang and Hunter, 2015). RBPs can also be inactivated through ubiquitin-based protein degradation during specific stages of germline development. For instance, the E3 ubiquitin ligase SEL-10 mediates degradation of the RBPs GLD-1, CPB-3 (Kisielnicka et al., 2018), and LIN-41 (Spike et al., 2018). This degradation contributes to localization of the RBP within the germline, which in the case of GLD-1 is critical to its function in promoting the early stages of meiosis. A study by Greenstein and others used CRISPR/Cas9 to target the endogenous locus of the RBP lin-41 and deleted regions enriched in putative phosphorylation sites. The deletions prevented its degradation in the late oocytes and the early embryo (Spike et al., 2018). Uncovering the role of the phosphorylation sites in the RBP’s localization by mutating them in vivo will help define their biological relevance and determine the biological impact on the animal’s development.
RBPs involved in mitotic progenitor cell self-renewal
In the distal mitotic end of the germline, there are at least six RBPs that promote and maintain mitosis and germ cell proliferation including the PUF-domain RBPs FBF-1/2, PUF-8, PUF-3, PUF-11, and the KH-domain RBP MEX-3 (Figures 2, 3A). There are eleven C. elegans puf genes, which were identified based on their sequence homology to the Drosophila Pumilio proteins and C. elegans FBF proteins (Macdonald, 1992; Zhang et al., 1997; Subramaniam and Seydoux, 2003). The evolutionarily conserved PUF-domain RBPs FBF-1/2 (fem-3 binding factor) were first discovered in a yeast three-hybrid screen used to identify proteins that bind to the fem-3 3′UTR (Zhang et al., 1997). fbf-1;fbf-2 double mutant animals are sterile and exhibit germline defects including failure to switch to oogenesis and to maintain mitosis. FBF-1/2 contribute to maintenance of mitosis by translationally repressing transcripts encoding the meiosis promoting gene gld-1 and the Nanos homolog nos-3 (Hansen et al., 2004). FBF-1/2 also contribute to maintenance of the mitotic region by 3′UTR-mediated repression of genes that encode components of the synaptonemal complex (SC), which forms between homologous chromosomes during meiosis (Merritt and Seydoux, 2010).
Although FBF-1 and FBF-2 are highly similar in their amino acid sequence (∼89%), they are proposed to have opposite functions in the mitotic region and use different mechanisms of post-transcriptional regulation (Lamont et al., 2004; Wang et al., 2020). First, FBF-1 is thought to expand the size of the mitotic region by preventing entry to meiosis while FBF-2 is thought to promote meiotic entry. Null fbf-1 mutant animals contain less mitotic germ cells than wild type animals while fbf-2 null mutant animals contain more mitotic germ cells than wild type animals (Lamont et al., 2004). Similarly, fbf-1 loss of function mutant animals contain a reduced mitotic region while fbf-2 loss of function mutant animals contain an expanded mitotic region compared to wild type animals (Lamont et al., 2004; Wang et al., 2020). Second, FBF-1 activity requires the CCR4/NOT deadenylase complex while FBF-2 does not (Wang et al., 2020). Knockdown of the CCR-4/NOT complex components in fbf-1 loss of function mutant animals does not affect the size of the mitotic region but it does lead to a shorter mitotic region in the fbf-2 loss of function mutant animals. A proposed explanation is that FBF-1 protein contains variable regions that allow it to bind the CCR-4/NOT complex that are absent in FBF-2, despite their high sequence similarity.
It has been shown that the sequence UGU is present within the otherwise variable motifs recognized by PUF proteins. The requirement of this sequence was first discovered by a combination of in vitro binding assays with purified recombinant PUF domains and isotopically labeled RNAs (Zamore et al., 1997), and subsequently confirmed in other family members from other species, both in vitro and in vivo (Nakahata et al., 2001; Tadauchi et al., 2001; Wang X. et al., 2002; Francischini and Quaggio, 2009). The PUF domain in PUF RBPs consists of forty amino acids which constitute eight repeat regions. Each repeat interacts with a base in the consensus binding sequence.
FBF-1/2 binds with high affinity and specificity to the sequence UGUNNNAUA in the 3′UTR of its target mRNAs gld-1 and fem-3 as determined by yeast three-hybrid assays and EMSA (Electrophoretic Mobility Shift Assays) (Bernstein et al., 2005) (Figures 2, 3A). Several factors contribute to the ability of FBF-1 and FBF-2 to recognize mRNAs, including their relative abundance, stacking forces between amino acid side chains and nucleotides, hydrogen bonding, steric accommodations, and base flipping. The ideal FBE also contains a cytosine upstream of the core UGU sequence (Bernstein et al., 2005; Kershner and Kimble, 2010; Dong et al., 2011). A study using iCLIP (individual-nucleotide resolution Cross-Linking and ImmunoPrecipitation) identified additional FBF target mRNAs specific to oogenic and spermatogenic germlines (Porter et al., 2019). This study showed that while some FBF targets are present in both male and hermaphroditic germlines, approximately 47% are gender-specific. As expected, some FBF targets encode RNA-binding proteins, supporting a cascade of regulation model where some RBPs govern the post-transcriptional regulation of others during germline development. Identification of all FBF target mRNAs will likely enable prediction of the biological processes in which FBF-1/2 are involved. Many of these targets remain to be validated and their 3′UTRs investigated for functional relevance of the mapped FBEs, and their overall contribution to germline development.
The PUF-domain RBPs PUF-3 and PUF-11 also contribute to maintenance of the mitotic region (Haupt et al., 2020) (Figure 3A). puf-3;puf-11 double mutant animals are fertile, but loss of both proteins in an fbf-1;fbf-2 mutant background enhances the mitotic germ cell defects observed in fbf-1/2 double mutants. PUF-3 and PUF-11 are proposed to act upstream of the meiosis promoting factor GLD-1 (Haupt et al., 2020). RNAi-mediated knockdown of puf-3 and puf-11 does not affect the tumorous germline phenotype observed in the gld-1 gld-2;fbf-1 fbf-2 mutant animals. It is possible that PUF-3 and PUF-11 promote mitosis by repressing meiotic mRNAs in addition to gld-1. It is also possible that PUF-3 and PUF-11 act as positive regulators of mitotic mRNAs translation. Revealing the binding specificity of PUF-11 and PUF-3 and mapping their network of in vivo targets will help define how these RBPs contribute to maintenance of the mitotic region. PUF-3 and PUF-11 activity could be controlled at the post-transcription or post-translational level by other germline proteins. A recent paper showed that the TRIM-NHL protein NHL-2 promotes meiotic entry by repressing PUF-3 and PUF-11. Expression levels of both proteins appeared increased in the distal mitotic region in nhl-2 null mutant animals (Brenner et al., 2022).
The final two RBPs that have been shown to contribute to maintenance of mitosis in the distal end are the PUF-domain RBP PUF-8 and the KH-domain RBP MEX-3 (Mootz et al., 2004; Ciosk et al., 2006; Ariz et al., 2009; Mainpal et al., 2011). mex-3 was first identified in a screen for maternal-effect embryonic lethal mutations (Draper et al., 1996) (Figure 2; Figure 3A). Both MEX-3 and PUF-8 act redundantly in maintenance of germ cell mitosis in the distal end. Single loss of function mutant animals of puf-8 or mex-3 contain few germ cells, but puf-8;mex-3 double mutant animals are sterile. They produce very few germ cells (less than 50) compared to wild type animals (∼430) (Ariz et al., 2009). Additionally, PUF-8 contributes to mitotic germ cell proliferation through 3′UTR-mediated positive regulation of the ER protein FARL-11, which in turn promotes Notch/GLP-1 signaling. MEX-3 is also thought to act redundantly with PUF-8 to promote expression of FARL-11 (Maheshwari et al., 2016).
MEX-3, which contains two KH (K homology) domains, binds two short motifs separated by 0–8 bases (A/G/U) (G/U)AGN(0–8)U(U/A/C)UA) as determined by in vitro binding studies performed with purified recombinant protein (Pagano et al., 2009). PUF-8 binds UGUMHRDW (M: A/C, H: A/U/C, R: A/G, D: A/U/G, W: A/T) motifs (Opperman et al., 2005; Campbell et al., 2012). It is intriguing to note that there is similarity in the motifs recognized by both proteins, but the in vivo relevance of this phenomenon remains unknown. Although it is possible to predict the target mRNAs of MEX-3 and PUF-8 based on the presence of the motifs they recognize, there is limited information concerning which mRNAs are bound by these proteins, and how binding contributes to germ cell proliferation and mitosis (Ariz et al., 2009; Pagano et al., 2009; Mainpal et al., 2011; Datla et al., 2014; Maheshwari et al., 2016). Intriguingly, spatiotemporal expression of MEX-3 is regulated through its 3′UTR in the germline (Merritt et al., 2008; Kaymak et al., 2016). Deleting majority of its 3′UTR at the endogenous locus results in significant de-repression of MEX-3 throughout the germline and a modest reduction in brood size (Albarqi and Ryder, 2021).
Taken together, there is a network of RNA-binding proteins and target mRNAs that promote and maintain germ cell proliferation in the distal end of the germline (Figure 3A). Several PUF proteins, MEX-3, and possibly NHL-2 appear to be required in combination to ensure mitosis in the distal end, presumably through regulation of an overlapping suite of critical mRNA targets. Redundancy is an emerging feature of these RBP-mRNA networks. Having multiple RBPs that act in the same pathway share target mRNAs ensures the repression or activation of gene expression and may improve the robustness of the germline under unfavorable conditions.
RBPs involved in the mitosis to meiosis switch
In the C. elegans germline, multiple RBP-mediated pathways promote the transition from mitosis to meiosis recently reviewed by Pushpa et al. (2017). Two critically important factors are the maxi KH-domain RBP GLD-1 and the RRM-containing RBP DAZ-1 (Figures 2, 3B). gld-1 was first identified in a mutagenic EMS screen looking for animals with germline defects. Dozens of gld-1 mutant alleles were identified and characterized using three-factor mapping and Nomarski microscopy. Complete loss of gld-1 function causes defects in meiotic progression (Francis et al., 1995a). Germ cells exit meiosis, return to mitosis, and continue to proliferate leading to tumor formation and sterility. Other gld-1 mutants exhibit a wide variety of phenotypes reflecting the various processes controlled by this RBP. For example, some hypomorphic alleles make oocytes that are small and defective (Jones and Schedl, 1995; Jones et al., 1996) indicating a role in oogenesis, while others make only sperm and fail to begin oogenesis. Transgenic reporter studies and immunostaining of dissected germline gonads show that GLD-1 is expressed at very low levels in the distal mitotic end (Jones et al., 1996) (Figure 2). This is presumably due to the negative regulation mediated by FBF-1/2. As FBF-1/2 levels start to decrease in the transition zone, GLD-1 levels increase in the meiotic region where its presence is necessary for promoting meiosis. The expression level decreases again in the loop region and disappears in the oocytes (Jones et al., 1996).
GLD-1 also contributes to maintenance of totipotency of the germline. Dissected gonads of a gld-1 null mutant express a neuronal fluorescent transgene in the germline. This phenotype is enhanced in a gld-1;mex-3 double mutant (Ciosk et al., 2006) indicating a role for both GLD-1 and MEX-3 in promoting the stemness of the germ cells. GLD-1 contains a maxi KH domain flanked by two conserved Qua domains. It binds ((U > N)ACU(C/A)AY) motifs in the 3′UTR and/or 5′UTR of its target mRNAs and binds as a homodimer (Lee and Schedl, 2004; Ryder et al., 2004; Wright et al., 2011; Theil et al., 2018) (Figure 2). In vitro and in vivo binding assays have identified more than 400 targets, majority of which are expressed in the germline and are involved in DNA replication, cell cycle, and mitosis (Lee and Schedl, 2001; Jungkamp et al., 2011). GLD-1 also represses genes that need to be translated only in later stages of germ cell development such as rme-2 (receptor-mediated endocytosis), a gene that encodes a yolk receptor involved in yolk endocytosis which is part of oocyte growth (Grant and Hirsh, 1999; Lee and Schedl, 2001). The contribution of many GLD-1 associated mRNAs towards the phenotypes observed upon loss of gld-1 function remains to be determined.
Deleted in azoospermia (daz-1) is a homologue of the conserved mammalian DAZL and Drosophila Boule RNA-binding proteins. DAZ-1 is required for germ cell development during oogenesis but not spermatogenesis. In daz-1 null mutant animals, germ cell nuclei arrest in the pachytene stage and fail to progress through meiosis leading to sterility (Karashima et al., 2000; Maruyama et al., 2005). It is mainly expressed in the distal mitotic end, transition zone, and at low levels in the meiotic region (Karashima et al., 2000) (Figure 2). DAZ-1 may contribute to meiotic progression by targeting other RNA-binding proteins in the meiotic region. For instance, DAZ-1 is proposed to positively regulate the expression of gld-1 through its 3′UTR (Theil et al., 2019). Knockdown of daz-1 results in repression of a gld-1 3′UTR reporter in the meiotic region. Interestingly, daz-1 null mutant animals do not exhibit defects in the mitotic germ cells which could be because DAZ-1 may be acting redundantly with other RBPs in the mitotic region; that loss of DAZ-1 alone does not have a phenotype. The binding specificity of DAZ-1 is unknown. Mapping the binding specificity of DAZ-1 will enable prediction of the network of target mRNAs. Then, genome editing tools can be used to delineate which targets are required for DAZ-1 regulation of meiosis and the mitosis to meiosis switch.
RBPs involved in spermatogenesis
Spermatogenesis occurs in each gonadal arm in fourth larval stage males and hermaphrodites. While males continue to make sperm, hermaphrodites switch to oogenesis once they become adults. During spermatogenesis in both sexes, the primary spermatocytes are part of a syncytium sharing a cytoplasmic core termed the rachis. As the primary spermatocytes progress through meiosis, they separate from the syncytium and continue to develop and divide to form the secondary spermatocytes. The secondary spermatocytes undergo the second round of meiotic division and develop into spermatids which later become spermatozoa ready to fertilize an oocyte (L'Hernault, 2006).
In both hermaphrodites and males, GLD-1 promotes meiosis. Some gld-1 mutant alleles result in feminization of the germline (FOG) where the mutant hermaphrodites fail to make sperm and only make oocytes (Francis et al., 1995b). Others result in a masculinized (MOG) phenotype. GLD-1 and GLD-2, a cytoplasmic poly(A) polymerase, function in redundant pathways to promote meiotic entry and progression during spermatogenesis. Animals lacking both proteins fail to enter meiosis in both sexes. In males, GLD-1 and PUF-8 act redundantly to promote meiotic progression. Animals that lack both GLD-1 and PUF-8 form germline tumors in both hermaphrodites and males (Priti and Subramaniam, 2015). These germline RBPs contribute to the robustness of animal development during unfavorable conditions. puf-8 single mutant males form germline tumors at elevated temperatures but not at room temperature. The meiotic spermatocytes exit meiosis and continue to proliferate.
Additional RNA-binding factors that play a role in sperm fate include the CPEB homolog fog-1 and the TOB1 homolog fog-3, both of which have been shown to co-immunoprecipitate with a wide variety of oogenic mRNAs (Noble et al., 2016). These proteins appear to work in complex, as immunoprecipates reveal association between both factors and an overlap in their associated mRNAs. As with some gld-1 alleles, loss of either fog-1 or fog-3 causes feminization of the germline (Barton and Kimble, 1990; Ellis and Kimble, 1995). The current model proposes that a FOG-1/FOG-3 complex represses oogenic mRNAs to promote sperm fate, although it remains formally possible that they may promote the expression of a spermatogenic mRNA required for sperm fate.
RBPs involved in the spermatogenesis to oogenesis switch
RNA-binding proteins required for the switch from spermatogenesis to oogenesis include GLD-1, FBF-1/2, NOS-3, PUF-8, MEX-3, DAZ-1, and MOG proteins (Kraemer et al., 1999; Eckmann et al., 2002; Bachorik and Kimble, 2005; Otori et al., 2006; Ariz et al., 2009; Priti and Subramaniam, 2015; Hu et al., 2019) (Figure 2). The phosphatase FEM-3 and the transmembrane protein TRA-2 control this switch in C. elegans germline (Ahringer et al., 1992; Goodwin et al., 1993). FEM-3 promotes spermatogenesis while TRA-2 promotes oogenesis. During the fourth larval stage when the animals produce sperm, tra-2 mRNA is translationally repressed through its 3′UTR by GLD-1 and FOG-2 (Clifford et al., 2000). On the other hand, fem-3 mRNA is translationally repressed through its 3′UTR by FBF-1/2 and NOS-3 to promote the switch to oogenesis (Kraemer et al., 1999). NOS-3 is a homologue of Drosophila Nanos and has been shown to physically interact with FBF-1 in a yeast two-hybrid screen. Mutations in the binding site of FBF-1/2 in the 3′UTR of fem-3 or knockdown of fbf-1/2 cause a masculinization phenotype where hermaphrodites make only sperm and fail to switch to oogenesis. fem-3 mRNA is also regulated by a set of MOG genes (mog-1, mog-2, mog-3, mog-4, mog-5, mog-6). MOG-1, MOG-4, and MOG-5 are DEAH-box containing proteins. Loss of function mutants of any of these six genes causes germline masculinization. (Graham et al., 1993; Graham and Kimble, 1993; Gallegos et al., 1998; Puoti and Kimble, 2000). These proteins are thought to repress fem-3 mRNA through its 3′UTR as demonstrated by the de-repression of a fem-3 3′UTR transgene reporter in the mog mutants. While MOG-1, MOG-4, and MOG-5 are orthologues of human and yeast pre-mRNA splicing factors, their role in splicing in C. elegans remains poorly understood. prp-17, which is an orthologue of human and yeast pre-mRNA splicing factor PRP17/CDC40, was also shown to contribute to the spermatogenesis to oogenesis switch (Kerins et al., 2010). Loss of function mutants of prp-17 exhibit the germline masculinization phenotype. Additional genetic epistasis studies with other factors in the sex-determination pathway suggest that PRP-17 functions upstream of fem-3.
PUF-8 is also proposed to work with FBF-1 to control the switch to oogenesis. puf-8;fbf-1 double mutant animals have a masculinized germline (Bachorik and Kimble, 2005). However, it is unknown whether they act on the same target mRNAs. MEX-3 also appears to contribute to the spermatogenesis to oogenesis switch. A percentage (34%) of homozygous puf-8 mutant animals that are also heterozygous for mex-3 produce sperm but no oocytes. Thus, it is possible that MEX-3 partially contributes to the switch when puf-8 is compromised (Ariz et al., 2009). DAZ-1 is also proposed to act in the sex determination pathway (Otori et al., 2006). In vitro binding assays have shown that DAZ-1 can bind the 3′UTR of fbf-1/2. Moreover, FBF-1/2 levels are reduced in null mutants of daz-1. And knockdown of daz-1 in a fem-3 gain of function mutant background, which make only oocytes, results in sperm production in these mutant animals. DAZ-1 may be acting redundantly with other RBPs to control sex-determination. These observations add another layer of complexity to how RNA-binding proteins vary in their contribution to different developmental processes and mechanisms throughout germline development.
RBPs involved in oocyte development and maturation
In adult hermaphrodites, nuclei in the loop region of the germline arrest in diakinesis of meiosis I. These nuclei start to re-cellularize in the loop region to form the early oocytes (Hubbard and Greenstein, 2005) (Figure 1A). These oocytes undergo multiple changes that constitute a maturation process that entails breakdown of the nuclear envelope, assembly of meiotic spindles, and chromosome segregation. Several RBPs control meiotic nuclei cellularization in the loop region and oocyte maturation in the proximal germline including LIN-41, OMA-1/2, PUF-5, PUF-6/7, and MEX-3. LIN-41, a TRIM-NHL RNA-binding protein, starts to appear only in the loop region as meiotic nuclei start to re-cellularize (Figure 2). lin-41 was first identified as part of the heterochronic pathway that regulates temporal larval development (Slack et al., 2000). LIN-41 was shown to be necessary for repression of target mRNAs such as cdk-1 to prevent pre-mature oocyte maturation (Spike et al., 2014a). Loss of lin-41 causes de-repression of cdk-1 resulting in pre-mature cellularization of pachytene nuclei and formation of small defective oocytes (Tsukamoto et al., 2017). Additionally, LIN-41 regulates other germline mRNAs including zif-1 and rnp-1 through their 3′UTR (Spike et al., 2014b) (Figure 3C). lin-29, which encodes a transcription factor involved in seam cell self-renewal and differentiation, is also translationally repressed by LIN-41 (Aeschimann et al., 2017; Tsukamoto et al., 2017). LIN-41 recognizes a 3-mer loop structure with U-A in the +1/−1 position (Figure 2; Figure 3C). The binding specificity was revealed by RNAcompete, an in vitro-based assay (Ray et al., 2009) that was used to identify the binding specificity of several NHL-domain RNA-binding proteins (Loedige et al., 2015).
OMA-1/2 are zinc-finger RNA-binding proteins that are expressed as oocytes form (Detwiler et al., 2001; Tsukamoto et al., 2017). oma-1 and oma-2 were identified by analyzing mutants with embryonic defects. Single oma-1 or oma-2 mutants are fertile, but oma-1;oma-2 double mutant animals are sterile indicating redundant function (Detwiler et al., 2001). Oocytes produced by the double mutant animals are abnormally large, exhibit defects in the nuclear envelope, and arrest in diakinesis (Detwiler et al., 2001). Many of these defects are presumably caused by de-repression of OMA-1/2 target mRNAs, including cdc-25.3, which encodes a cell cycle phosphatase and is also targeted by LIN-41 (Spike et al., 2014a; Spike et al., 2014b; Tsukamoto et al., 2017). OMA-1/2 contain two CCCH zinc-finger domains that recognize and bind UA (A/U) motifs (Figure 2; Figure 3D) (Kaymak and Ryder, 2013). Among the targets of OMA-1/2 is the glp-1 3′UTR which contains several UA (A/U) motifs. Purified recombinant OMA-1 binds fragments of the glp-1 3′UTR in vitro and knockdown of oma-1/2 results in increased expression of a glp-1 3′UTR transgenic reporter in the oocytes (Kaymak and Ryder, 2013). Interestingly, OMA-1 also binds its target 3′UTRs with high cooperativity, suggesting that the density and distribution of the motifs might contribute to target selection. Analysis of the 3′UTRs of the mRNAs associated with epitope-tagged OMA-1 show enrichment of UA (A/U) motifs (Tsukamoto et al., 2017). OMA-1/2 have been shown to repress numerous 3′UTR reporter mRNAs in maturing oocytes (Kaymak et al., 2016), all of which contain multiple OMA-1 motifs. Both LIN-41 and OMA-1/2 regulate the development of early and late-stage oocytes through 3′UTR mediated translational regulation of target mRNAs (Figures 3C,D).
Epitope tagged LIN-41 and OMA-1 proteins were pulled down and their associated proteins and RNAs analyzed (Tsukamoto et al., 2017). LIN-41 appeared to associate with several germline RNA-binding proteins including OMA-1, GLD-1, MEX-3, and SPN-4. LIN-41 also appeared to associate with components of the cytoplasmic adenylation (GLD-2, GLD-3, RNP-8) and de-adenylation (CCF-1, NTL-1, CCR-4) complexes. Interestingly, GLD-1, MEX-3, SPN-4, and LIN-41 were also shown to associate with OMA-1. While both LIN-41 and OMA-1 appear to associate with similar protein cofactors, null mutants of lin-41 or oma-1/2 exhibit different phenotypes. lin-41 null mutant animals undergo premature nuclei cellularization (Spike et al., 2014a) while oma-1/2 null mutant animals undergo normal cellularization but fail to undergo oocyte maturation (Detwiler et al., 2001). LIN-41 and OMA-1/2 also associate with similar mRNA targets including cdc-25.3 and zif-1, both of which are translationally repressed through their 3′UTR by OMA-1/2 and LIN-41 (Guven-Ozkan et al., 2010; Spike et al., 2014b). The current model proposes that LIN-41 promotes oocyte growth and prevents oocyte maturation in the early oocytes in part by 3′UTR-mediated translational repression of mRNAs, some of which are also repressed by OMA-1/2 such as cdc-25.3, zif-1, and rnp-1. One the other hand, spn-4 and meg-1 are translationally repressed by LIN-41 in the early oocytes but positively regulated by OMA-1/2 in the late oocytes. Both SPN-4 and MEG-1 are required for early embryo development (Gomes et al., 2001; Huang et al., 2002; Leacock and Reinke, 2008; Kapelle and Reinke, 2011; Huang and Hunter, 2015).
The PUF proteins PUF-5/6/7 also contribute to late oocyte development (Lublin and Evans, 2007) (Figure 3D). puf-5 and puf-6/7 were identified in an RNAi screen of genes expressed in the germline to look for additional regulators of glp-1 mRNA. Immunostaining showed that PUF-5 starts to appear in the loop region and its levels increase throughout oocyte development until oocyte maturation where PUF-5 is absent in the most proximal oocytes. A yeast three-hybrid screen was used to identify the binding specificity of PUF-5 (Stumpf et al., 2008). PUF-5 and PUF-6 bind motifs containing two UGU trinucleotides (CUCUGUAUCUUGU) (Figure 2). Based on this consensus sequence, a set of potential mRNA targets of PUF-5 and PUF-6 were identified using bioinformatic analysis. Whether this sequence is also the consensus sequence in vivo remains unknown. PUF-5 and PUF-6/7 are proposed to repress translation of glp-1 notch receptor mRNA which is expressed in the distal mitotic end and repressed in the pachytene stage of meiosis by GLD-1. puf-5;puf-6/7 mutant animals contain maturing oocytes of variable size, pachytene nuclei rather than diakinesis nuclei, and exhibit de-repression of glp-1. Thus, PUF-5 and PUF6/7 appear to contribute to early stages of oogenesis, not mature oocytes, in part by repressing the mitotic gene glp-1. MEX-3 is also present in the maturing oocytes (Figure 2). MEX-3 alone is not essential for oogenesis. Loss of function mex-3 mutants produce oocytes that can get fertilized and produce embryos but fail to hatch. Interestingly, MEX-3 protein and RNA appears to associate with LIN-41 and OMA-1 (Tsukamoto et al., 2017), but the role of MEX-3 in oocyte maturation, if any, is unknown.
RBPs involved in cell-fate specification in early embryos
Prior to the onset of zygotic transcription, the early embryo depends on the cytoplasmic content inherited from the gametes and especially the oocyte (Figure 1B). There are several RBPs that ensure proper embryonic development including POS-1, MEX-3, MEX-5/6, PUF-5, and PUF-6/7 (Figures 2, 3). pos-1 was first identified in a molecular screen looking for mRNAs that exhibit an asymmetric pattern in the embryo and another genetic screen for maternal-effect lethal mutations. Null mutants of pos-1 are maternal-effect embryonic lethal. These animals produce dead embryos that express GLP-1 in all blastomeres and show cell fate specification defects such as germ cell precursor cells developing into somatic cells (Farley and Ryder, 2012; Elewa et al., 2015). POS-1 is present throughout the 1-cell embryo but then gets restricted to the posterior (P1) blastomere at the 2-cell stage, the EMS and P2 blastomeres at the 4-cell stage, and the P4 germline precursor cell (Figure 2). POS-1 contains two CCCH zinc finger RNA-binding motifs (Tabara et al., 1999). The POS-1 recognition motif (PRE) is UA (U2-3)(A/G)(A/G/U)(N1-3)G which was determined using in vitro binding assays with recombinant POS-1 tandem zinc finger (TZF) domain (Farley et al., 2008). Several studies have shown that POS-1 represses translation of glp-1 and skn-1 mRNAs by binding to its target motifs in their 3′UTRs, restricting their expression to the anterior blastomeres where they are required for somatic tissue specification. POS-1 also contributes to establishing the posterior-anterior asymmetry by 3′UTR-mediated repression of neg-1 mRNA (Elewa et al., 2015). Additionally, POS-1 is required for 3′UTR-dependent nos-2 expression in the P4 germline precursor cell (Jadhav et al., 2008). Epistasis experiments suggest that POS-1 acts to de-repress negative regulation by MEX-3 and SPN-4 rather than directly activating the translation of nos-2. In vitro binding assays show that POS-1 competes with SPN-4-mediated repression of nos-2. As such, the ratio of POS-1 and SPN-4 influences the ability of POS-1 to de-repress nos-2 translation in the P4 cell.
PIE-1 is another RNA-binding protein that exhibits an expression pattern similar to that of POS-1. While it appears throughout the entire 1-cell embryo, it only appears in germline precursor cells and their descendants in later embryonic stages (Figure 2). pie-1 was identified in a screen for maternal-effect mutations that cause defects in early embryo cell fate specification. It produces extra pharyngeal cells in the embryo when mutated (Mello et al., 1992). PIE-1 also contributes to germline specification in the germline precursor cells (Mello et al., 1996; Tabara et al., 1999; Tenenhaus et al., 2001; Elewa et al., 2015; Gauvin et al., 2018) in part by repressing the translation of skn-1 through its 3′UTR in the P2 blastomere at the 4-cell stage. SKN-1 is required for specification of the somatic endo-mesoderm (EMS) blastomere (Bowerman et al., 1993). In addition to its function in repressing skn-1, PIE-1 promotes germline fate by repression of transcription of zygotic mRNAs in the germline blastomeres (Seydoux et al., 1996).
MEX-5/6 are RBPs that first appear in the distal oocytes and continue to be expressed in the 1-cell embryo, the anterior blastomere in the 2 and 4-cell stages and then remain in the P-granules in the posterior blastomere and its descendants (Schubert et al., 2000) (Figure 2). mex-5 was identified in a screen for maternal-effect lethal mutations. Null mutants of mex-5 produce eggs that fail to hatch; embryos contain extra muscle cells and undergo morphogenesis defects. EMSA experiments with purified recombinant MEX-5 tandem zinc finger (TZF) showed that MEX-5 consensus binding site is a 9–13 nucleotide sequences with six or more uridines (Pagano et al., 2007). Additionally, recombinant MEX-5 TZF binds the 3′UTR of glp-1 in vitro. MEX-5 is thought to positively regulate expression of glp-1 since it is absent in mex-5; mex-6 mutant embryos or repress other RBPs that repress glp-1 (Schubert et al., 2000; Pagano et al., 2007). MEX-5 and MEX-6 have a high degree of similarity in their amino acid sequence (>50%). However, unlike mex-5, mex-6 null mutant animals produce viable eggs that hatch and develop normally. Reduction of mex-6 in a mex-5 null mutant background causes embryonic defects that are more severe than those observed in the mex-5 null mutant alone, indicating that MEX-6 contributes to embryonic development in a manner that can only be visualized in the absence of mex-5 (Schubert et al., 2000).
MEX-3, which plays a role in mitotic germ cell totipotency, also contributes to anterior cell fate specification in the early embryo (Draper et al., 1996). One key function of MEX-3 is to repress pal-1 expression through its 3′UTR (Mootz et al., 2004) (Figure 2). PAL-1, a homeodomain transcription factor, is thereby restricted to the posterior blastomeres where it contributes to the somatic tissue specification of muscle cells (Baugh et al., 2005) (Figure 3E). MEX-3 also contributes to early embryonic development by 3′UTR-mediated repression of nos-2 (Jadhav et al., 2008). PUF-5 and PUF-6/7 also play a role in early embryo development where they are required for cytokinesis, nuclear divisions, and eggshell formation (Lublin and Evans, 2007). Early embryos of puf-5;puf-6/7 mutant animal lack a fully formed eggshell, contain abnormal nuclei, and exhibit cellularization defects.
Interestingly, the consensus binding motifs of embryonic RBPs tend to overlap, which may be important to their biological activity. For example, the 3′UTR of glp-1 contains ∼100 bp region that contains overlapping binding sites for POS-1, GLD-1, MEX-3, FBF-1/2, and OMA-1/2 (Marin and Evans, 2003; Farley et al., 2008; Pagano et al., 2009; Kaymak and Ryder, 2013). Mutating GLD-1 binding motif (GBM) or the POS-1 recognition element (PRE) causes de-repression of a glp-1 3′UTR transgenic reporter in the posterior blastomere of a four cell embryo (Farley and Ryder, 2012). Although this cluster contains two nearly identical POS-1 sites (PREs) separated by only five nucleotides, only the 3’ site is required for reporter repression in animals, demonstrating that not every RBP binding site is biologically relevant. Similar to how the POS-1 and SPN-4 ratio affects nos-2 translation, a high POS-1/SPN-4 ratio represses glp-1 translation (Jadhav et al., 2008). POS-1 which is localized to the posterior blastomeres represses glp-1, thereby restricting its expression to the anterior blastomeres (Ogura et al., 2003).
Some RBPs regulate stability of their target mRNAs through poly(A) tail length control
Control of mRNA poly(A) tail length is one of the primary mechanisms of post-transcriptional control in C. elegans germline (Figure 4). In the germline, deadenylation of mRNAs does not necessarily lead to mRNA turnover. Two cytoplasmic polyA polymerases (PAP), gld-2 and gld-4, are thought to re-adenylate germline mRNAs to enhance their translation. gld-2 was identified in a forward mutagenic screen for genes required for germline development, while gld-4 was identified in a yeast two-hybrid screen (Wang L. et al., 2002; Schmid et al., 2009). Both are part of the DNA polymerase β-like superfamily of nucleotidyltransferases, and both are considered non-canonical poly(A) polymerases because they lack an RNA-targeting domain (Wang L. et al., 2002). As such, GLD-2 must be recruited to specific transcripts through interactions with RBPs, for example GLD-3, a multiple KH domain containing RBP shown to interact with GLD-2 in a yeast two-hybrid screen. The complex of GLD-2 and GLD-3 was shown to have robust poly(A) polymerase activity in vitro and both are required for germline development (Figure 4B). GLD-2 appears to be targeted to different mRNAs by different interacting partner RBPs. For example, GLD-2 was shown to interact with the RRM-domain containing protein RNP-8. Several genetic and biochemical studies have shown that GLD-2 mediates translational activation mediated by LIN-41 and OMA-1/2 in early stage and late-stage oocytes, respectively (Tsukamoto et al., 2017). The GLD-2/RNP-8 complex also functions to polyadenylate cytoplasmic mRNAs especially in the early stages of oogenesis (Kim et al., 2010). In the 2-cell and 4-cell embryos, POS-1 is thought to repress neg-1 expression in the posterior blastomere by repressing gld-2 and gld-3, although this specific model has not been tested (Elewa et al., 2015).
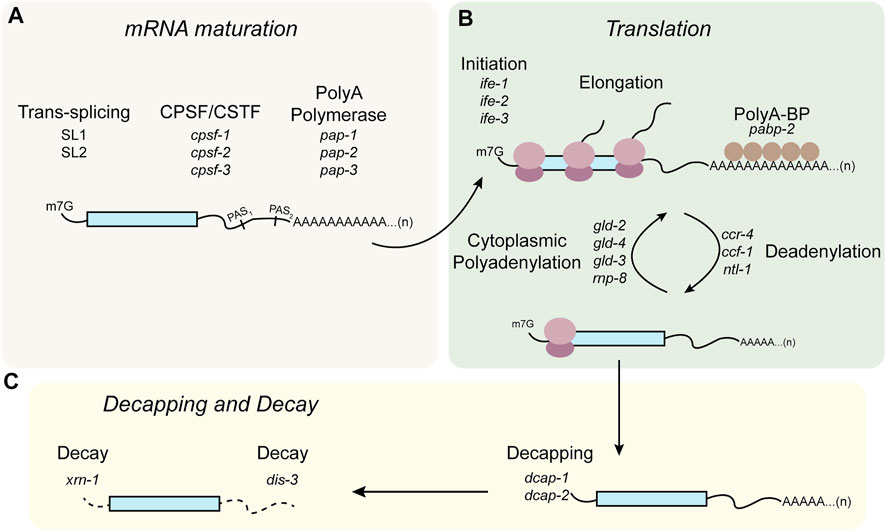
FIGURE 4. Modes of post-transcriptional regulation in C. elegans germline. (A) Pre-mRNAs undergo trans-splicing, and alternative polyadenylation. (B) In the cytoplasm, mRNAs undergo post-transcriptional regulation mediated through translation initiation or elongation factors, or cycles of polyadenylation and deadenylation. (C) mRNAs destined for degradation can undergo 5′ end decapping and decay mediated by exonucleases.
Like GLD-2, GLD-4 also lacks an RRM motif to bind its RNA target. GLD-4 binds directly to GLS-1, a P-granule component required for germline survival (Schmid et al., 2009; Nakel et al., 2016) (Figure 4B). In the mitotic region, GLD-4 and its binding partner GLS-1 activate translation of the notch receptor glp-1 mRNA through poly(A) tail lengthening (Millonigg et al., 2014). Expression of a glp-1 3′UTR reporter was shown to be absent in a gld-4 mutant background. Additionally, the size of the mitotic region was shortened in a gld-4 or gls-1 loss of function mutant. Knockdown of gld-4 also resulted in a shortened poly(A) tail length of the endogenous glp-1. GLD-2 and GLD-4 appear to both play redundant and distinct roles in the germline. Null mutant animals of gld-2 are sterile while gld-4 null mutant animals exhibit partial sterility meaning that majority of the animals have a reduced brood size, but a small percentage of the animals are completely sterile. Sterility is due to defects in meiotic progression or early stages of oogenesis (Schmid et al., 2009). In the transition zone and as nuclei enter early stages of meiosis, the GLD-2/GLD-3 complex as well as the GLD-4/GLS-1 complex mediate stabilization and translation of gld-1 mRNA by lengthening its poly(A) tail and repressing de-adenylation (Suh et al., 2006; Schmid et al., 2009).
Shortening of the poly(A) tail via de-adenylation also contributes to the post-transcriptional regulation of maternal mRNAs in the germline. The major deadenylase complex consists of CCF-1, CCR-4, and NTL-1 (Nousch et al., 2013) (Figure 4B). These components were identified by homology alignments to the yeast and human genomes in addition to RNAi and immunostaining experiments. CCF-1 is an orthologue of yeast Caf1p (ccr-4 associated factor 1) (Molin and Puisieux, 2005). CCR-4 is an orthologue of yeast Ccr4 (carbon catabolite repressor 4). CCF-1 and CCR-4 carry out the catalytic deadenylase activity while NTL-1 is a scaffolding protein required for assembly of the deadenylase complex. Although all three components are expressed throughout the germline, CCF-1 appears to be most highly expressed in the meiotic region (Nousch et al., 2013). Interestingly, loss of function mutants of ccf-1 or ntl-1 but not ccr-4 lead to reduced fertility that is caused in part by oogenesis defects. The affected animals make small defective oocytes that fail to undergo meiotic maturation. Knockdown of ccf-1 also delays the expression of OMA-1/2, which are redundantly required for oocyte maturation. In early stages of oogenesis, LIN-41 is thought to repress the translation of its target mRNAs such as spn-4 and meg-1 by promoting de-adenylation. All components of the CCR4-NOT complex appear in pull downs with epitope tagged-LIN-41 (Tsukamoto et al., 2017). In the distal mitotic end, FBF-1/2 also appear to repress translation of their target mRNAs, including gld-1 via CCF-1-mediated de-adenylation (Suh et al., 2009).
Beyond CCR4-NOT, at least two additional deadenylation complexes exist in the germline, including the PAN deadenylase complex which consists of PANL-2 and PANL-3, and the PARN deadenylase complex comprised of PARN-1 and PARN-2. Loss of function mutants of either panl-2 or panl-3 causes a modest reduction in brood size at 20°C but a significantly reduced brood size at 25°C. Loss of function mutants of parn-1 but not parn-2 exhibit a reduced brood size at 25°C (Nousch et al., 2013). How they are recruited to specific targets is unknown. The PAN complex as well as the PARN complex are only necessary for germline development and robustness in unfavorable conditions such as elevated temperature and as such are not absolutely required for reproduction. Nevertheless, it is clear that the cycle of cytoplasmic adenylation, governed both by polyadenylation and deadenylation machinery, is critical to RNA regulation in the germline and reproductive fitness (Figure 4B).
RBPs regulate translation initiation in the germline
Regulation of maternal mRNAs via the 3′UTR can also involve translation initiation factor complexes that recognize the 5′ ends of mRNAs (Figure 4B). In C. elegans, more than half of the mRNA transcripts have a tri-methylated guanosine (TMG) cap at the 5′ end instead of a mono-methylated guanosine (MMG) cap. This results from a phenomenon in nematodes where one of two splice leader transcripts (SL1/2)) are spliced in trans to pre-messenger RNAs to form the mature 5′ end (Blumenthal, 1998) (Figure 4A). During translation initiation, the cap structure is recognized by eIF4E as part of the assembly process of the pre-initiation complex. Sequence homology-based searches were used to predict homologues of the human eIF4E in the C. elegans genome (Keiper et al., 2000). Initially, three isoforms were identified (IFE-1/2/3). Recombinant proteins of these isoforms were purified and used in affinity chromatography assays to determine their ability to bind analogs of the TMG cap. Two additional isoforms were identified later. Knockdown of ife-1, ife-2, ife-4, or ife-5 does not appear to affect viability, but knockdown of ife-3 causes embryonic lethality (Jankowska-Anyszka et al., 1998; Keiper et al., 2000). This appears to be due to partial redundancy, as knockdown of some isoforms simultaneously also leads to embryonic lethality. For instance, knockdown of both ife-1 and ife-2 results in 75% embryonic lethality leading to reduced brood size. The nature of the embryonic lethality remains unknown. ife-1 null mutant animals exhibit defects in oogenesis and spermatogenesis at higher temperatures and lead to sterility. In these animals, ribosome association of several germline RNA-binding proteins such as oma-1 appears to be reduced in polysome fractionation (Henderson et al., 2009). Both ife-1 and ife-3 are expressed in the germline and contribute to proper gametogenesis. They are thought to act in an opposing fashion to either repress (ife-3) or promote (ife-1) translational initiation of specific germline mRNAs. IFE-3 is proposed to associate with the RNA-binding proteins FBF and their target mRNAs (Huggins et al., 2020). Knockdown of ife-3 enhances the masculinization phenotypes of fbf-1 null mutant animals which supports its proposed role in the sex-determination pathway (Mangio et al., 2015).
Other RBPs are thought to impact translation initiation efficiency, but the mechanisms remain unclear. Polysome profiling of GLD-1 coupled with microarray analysis of GLD-1 targets showed that inhibition of translation initiation is one of the major repression mechanisms used by GLD-1 (Scheckel et al., 2012). Interestingly, GLD-1 may also play a positive role for some of its targets by stabilizing the mRNAs. For example, mutating GLD-1 binding motifs (GBMs) in an oma-2 3′UTR reporter causes a reduction in the mRNA levels of the reporter (Scheckel et al., 2012). Overall, the balance of activation and repression between IFE-1 and IFE-3, and how they are recruited to specific germline mRNAs by specific RBPs, is only just beginning to be uncovered. Exploring this pathway in detail will improve our understanding of the diverse modes of post-transcriptional regulation used in different regions of the germline.
IFET-1 is the C. elegans homologue of the eIF4E-transporter. IFET-1 contributes to germline development in the distal mitotic end, oocytes maturation, 1-cell embryo development, and P-granule formation (Li et al., 2009; Guven-Ozkan et al., 2010; Sengupta et al., 2013). ifet-1 loss of function mutant hermaphrodites are sterile unlike mutant males. Sterility is due to defects in meiotic progression. They also showed a germline masculinization phenotype (Sengupta et al., 2013). IFET-1 was also shown to be required for localization of P-granule components such as CGH-1, CAR-1, and PGL-1. Overall, IFET-1 is thought to mediate repression of mRNAs in P-granules in addition to its repressive effects in other regions of the germline.
Argonautes contribute to post-transcriptional regulation of germline mRNAs
The Argonuate family of RNA-binding proteins is conserved from human to bacteria and archaea (Hutvagner and Simard, 2008). Argonautes are essential for the biogenesis and functionality of several small RNA pathways found in the germline (Weiser and Kim, 2019). These RBPs associate with small RNAs such as microRNAs which are 21–24 nucleotide transcripts that mediate gene expression silencing (Bartel, 2004). In C. elegans, primary miRNA transcripts are cleaved by DRSH-1 to make the precursor miRNAs which then get exported to the cytoplasm where they undergo processing by DCR-1 to form the mature miRNA transcript. ALG-1 then associates with the mature miRNA to form the miRISC (miRNA Induced Silencing Complex) to which additional proteins can bind. The miRNA functions as a guide that leads the complex to the target mRNA by binding to its complementary sequence in the UTR of the mRNA. Both ALG-1 and ALG-2 are involved in germline development. Loss of function mutants of either alg-1 or alg-2 exhibit reduced fertility. These animals have a small mitotic region and fewer viable oocytes due to increased apoptosis. Both ALG-1 and ALG-2 are expressed in the distal tip cell (DTC) which provides the niche for the mitotically dividing germ cells in the distal end (Bukhari et al., 2012). Animals lacking the miRNAs let-7, lin-4, mir-237, mir-247, or mir-359 exhibit reduced fertility, a shortened mitotic region, and contain few oocytes. Using a pull-down approach followed by small RNA-seq and mass spectrometry, mir-84 was demonstrated to bind and target the 3′UTR of gld-1. Mutating the binding site of mir-84 in the gld-1 3′UTR using CRISPR/Cas9 leads to reduction in the abundance of mir-84 bound to the 3′UTR (Theil et al., 2019). More recently, a third germline expressed miRNA-associated argonaute termed ALG-5 was identified (Brown et al., 2017). ALG-5 is the fifth member of the C. elegans AGO subfamily of argonautes. It was identified by phylogenetic analysis using C. elegans, D. Melanogaster, and H. sapiens argonaute sequences. ALG-5 is expressed in the germline in the cytoplasm as well as the P-granules and its loss leads to reduced fertility. ALG-5 appears to control the timing of oogenesis. Animals deficient in alg-5 exhibit premature onset of oogenesis (Brown et al., 2017). Although miRNAs that associate with ALG-5 have been identified, the mechanisms of how ALG-5 and its associated miRNAs contribute to germline development and oogenesis remain unknown.
In the embryo, the mir-35–42 family contributes to proper embryonic and post-embryonic development. Animals that lack this family of miRNAs produce defective embryos with various phenotypes. The embryonic lethality phenotype becomes more severe at elevated temperatures (McJunkin and Ambros, 2014). Moreover, the mir-35 family is thought to play a role in sex determination in the early embryo by regulating RNA-binding proteins such as SUP-26 and NHL-2 through their 3′UTR (McJunkin and Ambros, 2017). However, the exact mechanism of post-transcriptional regulation is unknown although de-adenylation appears to be involved in the miRNA-mediated post-transcriptional regulation of embryonic mRNAs (Wu et al., 2010). The mir-51 family functions in late embryos to regulate pharyngeal development (Shaw et al., 2010). Interestingly, mir-35 and mir-51 are sufficient to rescue embryonic lethality that occurs in animals lacking Drosha and Pasha (Dexheimer et al., 2020). As such, these two miRNAs are likely the only miRNAs required prior to hatching of L1 larvae. Overall, miRNA-associated argonautes and two specific miRNA families contribute to germline development and embryogenesis. Their most important targets, and how miRNA regulation intersects with RBP-driven regulation, remains to be uncovered.
P-granules and their role in germline and embryo development
P-granules are membrane-less phase separated organelles that consist of RNAs and proteins (Brangwynne et al., 2009). P-granules are found throughout the germline where they are mainly perinuclear in early meiotic germ cells and then become more cytoplasmic in late oocytes (Updike and Strome, 2010). In the developing embryo, they start diffuse and cytoplasmic throughout the 1-cell embryo and then segregate to the germline precursor cells. PGL and GLH proteins are the core components of P-granules although dozens of other proteins have been shown to also associate with these condensates (Gruidl et al., 1996; Kawasaki et al., 1998; Kuznicki et al., 2000; Kawasaki et al., 2004). These proteins are essential to formation and maintenance of P-granules. While single pgl-1 or pgl-3 mutant animals are fertile, they become sterile at elevated temperatures. pgl-1; pgl-3 double mutant animals are sterile at both normal and elevated temperatures and exhibit defects in the germline (Kawasaki et al., 2004). Knockdown of pgl-1, pgl-3, glh-1, and glh-4 simultaneously have been shown to cause sterility (Updike et al., 2014). The sterility was due to trans-differentiation of the germ cells to somatic cells. Among the functions of P-granules is post-transcriptional regulation and mRNA surveillance mediated by argonaute proteins. Many of the RNA-binding proteins discussed in this review also localize to P-granules (Schisa et al., 2001). Interestingly, components of the polyadenylation complex including GLD-2 and GLD-4 as well as the translation initiation factor IFE-1 also localize to P-granules suggesting that post-transcriptional regulatory mechanisms occurs in these organelles (Amiri et al., 2001; Wang L. et al., 2002; Schmid et al., 2009; Updike and Strome, 2010). While segregation of P-granules contributes to germline cell fate specification in the early embryo, germ cell fate specification can occur successfully even when P-granules are segregated symmetrically among the soma and germline blastomeres (Gallo et al., 2010). P-granules tend to contain mRNA transcripts with low ribosomal occupancy. It is thought that repressed mRNAs shuttle to P-granules and then get targeted to the germ cell precursor where their translation may be necessary for germ cell fate specification in the embryo (Parker et al., 2020).
Conclusion and perspectives
By combining our knowledge of the spatiotemporal expression of germline RBPs and their proposed regulatory mechanisms, we find that potentially each region in the germline contains several RBPs that mediate post-transcriptional regulation of hundreds of target mRNAs (Figure 2). Some of these target mRNAs encode RBPs that function in a different region in the germline or even in the same region. Although our knowledge of C. elegans germline RNA-binding proteins in terms of their RNA-binding domains, binding specificity, and target mRNAs has expanded tremendously over the past few decades, we still do not know the binding specificity of many germline RNA-binding proteins such as DAZ-1, RNP-8, or GLD-3. We do not know whether the binding sites determined using in vitro methods are relevant in vivo for germline development, and in most cases the mechanisms of RBP regulation remain incompletely described. Dozens of RBPs are present in the same vicinity in various regions of the germline, but we lack the understanding of which RBPs function together on the same target mRNAs and how one RBP gains priority over another. It is possible that the concentration of each RBP and their intrinsic affinity for their sequence motifs are the primary determinants of target selection. If so, statistical effects tell us that the abundance of functional motifs in any given 3′UTR can strongly influence occupancy. However, the kinetics of RNA association and dissociation may very well contribute, and RNA structure could also play an important role. The biochemical basis of RNA target selection remains undefined for the germline RBPs, and what renders one site functional and another irrelevant, even within the same transcript, remains a mystery.
By combining the well-established in vitro methods used to determine binding specificity with modern genome editing methods such as CRISPR/Cas9, it is now possible to determine the relevance of individual RBP binding sites in the 3′UTR of the most critical maternal mRNAs. One challenge is that many of these binding sites are very short, appear in multiple copies in a single 3′UTR, and may overlap with binding sites for other RBPs. Initially, large 3′UTR deletions can be made in the endogenous locus to define the overall importance of any given 3′UTR to germline development. Smaller deletions and/or single base mutations can be made to further dissect the 3′UTR and the contribution of its different regions to the mRNA expression pattern and function in the germline once its importance is established. The 3′UTR mutations can also be made in strains in which the gene of interest is tagged at the endogenous locus with a fluorescent protein to allow for the visual determination of the impact of the 3′UTR mutations on the spatiotemporal expression pattern of the protein. To delineate the network of RBP-mRNA found in each region of the germline, sequencing-based methods such as RIP-seq and CLIP-seq have been instrumental to both define binding motifs and the occupancy of motif-containing mRNAs in worm extracts. These approaches have the advantage of simultaneously mapping the motif as well as detecting interactions that occur in animals or extracts. Additionally, RNA-centric approaches such as interactome capture can be used to identify novel proteins bound to a particular transcript to provide us with information on which RBPs act on a single 3′UTR, and how these interactions change upon mutation. The recently developed method vIPR (in Vivo Interaction by Pulldown of RNA), which entails crosslinking proteins and RNA, RNA pull down, and mass spectrometry, can be used to identify proteins as well as small RNAs bound to an mRNA of interest (Theil et al., 2019). Methods such as single molecular RNA-FISH (smRNA-FISH) can provide information about the spatial pattern of the RNA transcripts that compose these RBP-mRNA networks. Together, all these methods can provide tremendous insights into how RBP-mRNA networks function to control germline development and reproduction.
In summary, binding does not always predict regulation. Defining the rules that distinguish functional binding events from non-functional events is critical to describing how germline RBPs orchestrate proper germ cell and embryo development. Since many of the germline RBPs are highly conserved across metazoans, the rules defined in this species may well apply to others. The technology now exists to make targeted deletions and substitutions at the endogenous locus (Kim and Colaiacovo, 2019; Vicencio and Ceron, 2021). Combined with high throughput cataloging of RNA-protein networks and identification of novel RBPs, it will be possible to complete the wiring diagram of maternal mRNA regulation, a tremendous step towards understanding how information flows from parent to progeny in metazoans.
Author contributions
MA and SR conceived of the review and the scope of its contents, MA wrote the initial draft of the text and figures, and SR and MA revised and edited subsequent drafts together.
Funding
Work in the Ryder lab is supported by NIH Grant R01GM145062 to SR.
Acknowledgments
The authors would like to thank Dr. Oscar Duan, Dr. Victor Ambros, and Dr. Craig Mello for helpful conversations about RNA regulation in the worm germline.
Conflict of interest
The authors declare that the research was conducted in the absence of any commercial or financial relationships that could be construed as a potential conflict of interest.
Publisher’s note
All claims expressed in this article are solely those of the authors and do not necessarily represent those of their affiliated organizations, or those of the publisher, the editors and the reviewers. Any product that may be evaluated in this article, or claim that may be made by its manufacturer, is not guaranteed or endorsed by the publisher.
References
Aeschimann, F., Kumari, P., Bartake, H., Gaidatzis, D., Xu, L., Ciosk, R., et al. (2017). LIN41 post-transcriptionally silences mRNAs by two distinct and position-dependent mechanisms. Mol. Cell 65, 476–489 e4.
Ahringer, J., Rosenquist, T. A., Lawson, D. N., and Kimble, J. (1992). The Caenorhabditis elegans sex determining gene fem-3 is regulated post-transcriptionally. EMBO J. 11, 2303–2310.
Albarqi, M. M. Y., and Ryder, S. P. (2021). The endogenous mex-3 3 UTR is required for germline repression and contributes to optimal fecundity in C. elegans. PLoS Genet. 17, e1009775. doi:10.1371/journal.pgen.1009775
Amiri, A., Keiper, B. D., Kawasaki, I., Fan, Y., Kohara, Y., Rhoads, R. E., et al. (2001). An isoform of eIF4E is a component of germ granules and is required for spermatogenesis in C. elegans. Development 128, 3899–3912. doi:10.1242/dev.128.20.3899
Ariz, M., Mainpal, R., and Subramaniam, K. (2009). C. elegans RNA-binding proteins PUF-8 and MEX-3 function redundantly to promote germline stem cell mitosis. Dev. Biol. 326, 295–304. doi:10.1016/j.ydbio.2008.11.024
Bachorik, J. L., and Kimble, J. (2005). Redundant control of the Caenorhabditis elegans sperm/oocyte switch by PUF-8 and FBF-1, two distinct PUF RNA-binding proteins. Proc. Natl. Acad. Sci. U. S. A. 102, 10893–10897. doi:10.1073/pnas.0504593102
Bartel, D. P. (2004). MicroRNAs: Genomics, biogenesis, mechanism, and function. Cell 116, 281–297. doi:10.1016/s0092-8674(04)00045-5
Barton, M. K., and Kimble, J. (1990). fog-1, a regulatory gene required for specification of spermatogenesis in the germ line of Caenorhabditis elegans. Genetics 125, 29–39. doi:10.1093/genetics/125.1.29
Bauer, J., Poupart, V., Goupil, E., Nguyen, K. C. Q., Hall, D. H., and Labbe, J. C. (2021). The initial expansion of the C. elegans syncytial germ line is coupled to incomplete primordial germ cell cytokinesis. Development 148, 1.
Baugh, L. R., Hill, A. A., Claggett, J. M., Hill-Harfe, K., Wen, J. C., Slonim, D. K., et al. (2005). The homeodomain protein PAL-1 specifies a lineage-specific regulatory network in the C. elegans embryo. Development 132, 1843–1854. doi:10.1242/dev.01782
Bernstein, D., Hook, B., Hajarnavis, A., Opperman, L., and Wickens, M. (2005). Binding specificity and mRNA targets of a C. elegans PUF protein, FBF-1. RNA 11, 447–458. doi:10.1261/rna.7255805
Blumenthal, T. (1998). Gene clusters and polycistronic transcription in eukaryotes. Bioessays 20, 480–487. doi:10.1002/(SICI)1521-1878(199806)20:6<480:AID-BIES6>3.0.CO;2-Q
Bowerman, B., Draper, B. W., Mello, C. C., and Priess, J. R. (1993). The maternal gene skn-1 encodes a protein that is distributed unequally in early C. elegans embryos. Cell 74, 443–452. doi:10.1016/0092-8674(93)80046-h
Brangwynne, C. P., Eckmann, C. R., Courson, D. S., Rybarska, A., Hoege, C., Gharakhani, J., et al. (2009). Germline P granules are liquid droplets that localize by controlled dissolution/condensation. Science 324, 1729–1732. doi:10.1126/science.1172046
Brenner, J. L., Jyo, E. M., Mohammad, A., Fox, P., Jones, V., Mardis, E., et al. (2022). TRIM-NHL protein, NHL-2, modulates cell fate choices in the C. elegans germ line. Dev. Biol. 491, 43–55. doi:10.1016/j.ydbio.2022.08.010
Brown, K. C., Svendsen, J. M., Tucci, R. M., Montgomery, B. E., and Montgomery, T. A. (2017). ALG-5 is a miRNA-associated Argonaute required for proper developmental timing in the Caenorhabditis elegans germline. Nucleic Acids Res. 45, 9093–9107. doi:10.1093/nar/gkx536
Bukhari, S. I., Vasquez-Rifo, A., Gagne, D., Paquet, E. R., Zetka, M., Robert, C., et al. (2012). The microRNA pathway controls germ cell proliferation and differentiation in C. elegans. Cell Res. 22, 1034–1045. doi:10.1038/cr.2012.31
Burd, C. G., and Dreyfuss, G. (1994). Conserved structures and diversity of functions of RNA-binding proteins. Science 265, 615–621. doi:10.1126/science.8036511
Campbell, Z. T., Bhimsaria, D., Valley, C. T., Rodriguez-Martinez, J. A., Menichelli, E., Williamson, J. R., et al. (2012). Cooperativity in RNA-protein interactions: Global analysis of RNA binding specificity. Cell Rep. 1, 570–581. doi:10.1016/j.celrep.2012.04.003
Ciosk, R., Depalma, M., and Priess, J. R. (2006). Translational regulators maintain totipotency in the Caenorhabditis elegans germline. Science 311, 851–853. doi:10.1126/science.1122491
Clifford, R., Lee, M. H., Nayak, S., Ohmachi, M., Giorgini, F., and Schedl, T. (2000). FOG-2, a novel F-box containing protein, associates with the GLD-1 RNA binding protein and directs male sex determination in the C. elegans hermaphrodite germline. Development 127, 5265–5276. doi:10.1242/dev.127.24.5265
Corsi, A. K., Wightman, B., and Chalfie, M. (2015). A transparent window into biology: A primer on Caenorhabditis elegans. United States: WormBook, 1–31.
Curtis, D., Lehmann, R., and Zamore, P. D. (1995). Translational regulation in development. Cell 81, 171–178. doi:10.1016/0092-8674(95)90325-9
Datla, U. S., Scovill, N. C., Brokamp, A. J., Kim, E., Asch, A. S., and Lee, M. H. (2014). Role of PUF-8/PUF protein in stem cell control, sperm-oocyte decision and cell fate reprogramming. J. Cell Physiol. 229, 1306–1311. doi:10.1002/jcp.24618
Derenzo, C., and Seydoux, G. (2004). A clean start: Degradation of maternal proteins at the oocyte-to-embryo transition. Trends Cell Biol. 14, 420–426. doi:10.1016/j.tcb.2004.07.005
Detwiler, M. R., Reuben, M., Li, X., Rogers, E., and Lin, R. (2001). Two zinc finger proteins, OMA-1 and OMA-2, are redundantly required for oocyte maturation in C. elegans. Dev. Cell 1, 187–199. doi:10.1016/s1534-5807(01)00026-0
Dexheimer, P. J., Wang, J., and Cochella, L. (2020). Two MicroRNAs are sufficient for embryonic patterning in C. elegans. Curr. Biol. 30, 5058–5065. e5. doi:10.1016/j.cub.2020.09.066
Dong, S., Wang, Y., Cassidy-Amstutz, C., Lu, G., Bigler, R., Jezyk, M. R., et al. (2011). Specific and modular binding code for cytosine recognition in Pumilio/FBF (PUF) RNA-binding domains. J. Biol. Chem. 286, 26732–26742. doi:10.1074/jbc.M111.244889
Draper, B. W., Mello, C. C., Bowerman, B., Hardin, J., and Priess, J. R. (1996). MEX-3 is a KH domain protein that regulates blastomere identity in early C. elegans embryos. Cell 87, 205–216. doi:10.1016/s0092-8674(00)81339-2
Eckmann, C. R., Kraemer, B., Wickens, M., and Kimble, J. (2002). GLD-3, a bicaudal-C homolog that inhibits FBF to control germline sex determination in C. elegans. Dev. Cell 3, 697–710. doi:10.1016/s1534-5807(02)00322-2
Elewa, A., Shirayama, M., Kaymak, E., Harrison, P. F., Powell, D. R., DU, Z., et al. (2015). POS-1 promotes endo-mesoderm development by inhibiting the cytoplasmic polyadenylation of neg-1 mRNA. Dev. Cell 34, 108–118. doi:10.1016/j.devcel.2015.05.024
Ellis, R. E., and Kimble, J. (1995). The fog-3 gene and regulation of cell fate in the germ line of Caenorhabditis elegans. Genetics 139, 561–577. doi:10.1093/genetics/139.2.561
Farley, B. M., Pagano, J. M., and Ryder, S. P. (2008). RNA target specificity of the embryonic cell fate determinant POS-1. RNA 14, 2685–2697. doi:10.1261/rna.1256708
Farley, B. M., and Ryder, S. P. (2012). POS-1 and GLD-1 repress glp-1 translation through a conserved binding-site cluster. Mol. Biol. Cell 23, 4473–4483. doi:10.1091/mbc.E12-03-0216
Farley, B. M., and Ryder, S. P. (2008). Regulation of maternal mRNAs in early development. Crit. Rev. Biochem. Mol. Biol. 43, 135–162. doi:10.1080/10409230801921338
Francis, R., Barton, M. K., Kimble, J., and Schedl, T. (1995a). gld-1, a tumor suppressor gene required for oocyte development in Caenorhabditis elegans. Genetics 139, 579–606. doi:10.1093/genetics/139.2.579
Francis, R., Maine, E., and Schedl, T. (1995b). Analysis of the multiple roles of gld-1 in germline development: Interactions with the sex determination cascade and the glp-1 signaling pathway. Genetics 139, 607–630. doi:10.1093/genetics/139.2.607
Francischini, C. W., and Quaggio, R. B. (2009). Molecular characterization of Arabidopsis thaliana PUF proteins-binding specificity and target candidates. FEBS J. 276, 5456–5470. doi:10.1111/j.1742-4658.2009.07230.x
Gallegos, M., Ahringer, J., Crittenden, S., and Kimble, J. (1998). Repression by the 3' UTR of fem-3, a sex-determining gene, relies on a ubiquitous mog-dependent control in Caenorhabditis elegans. EMBO J. 17, 6337–6347. doi:10.1093/emboj/17.21.6337
Gallo, C. M., Wang, J. T., Motegi, F., and Seydoux, G. (2010). Cytoplasmic partitioning of P granule components is not required to specify the germline in C. elegans. Science 330, 1685–1689. doi:10.1126/science.1193697
Gauvin, T. J., Han, B., Sun, M. J., and Griffin, E. E. (2018). PIE-1 translation in the germline lineage contributes to PIE-1 asymmetry in the early Caenorhabditis elegans embryo. G3 (Bethesda) 8, 3791–3801. doi:10.1534/g3.118.200744
Gomes, J. E., Encalada, S. E., Swan, K. A., Shelton, C. A., Carter, J. C., and Bowerman, B. (2001). The maternal gene spn-4 encodes a predicted RRM protein required for mitotic spindle orientation and cell fate patterning in early C. elegans embryos. Development 128, 4301–4314. doi:10.1242/dev.128.21.4301
Goodwin, E. B., Okkema, P. G., Evans, T. C., and Kimble, J. (1993). Translational regulation of tra-2 by its 3' untranslated region controls sexual identity in C. elegans. Cell 75, 329–339. doi:10.1016/0092-8674(93)80074-o
Goupil, E., Amini, R., Hall, D. H., and Labbe, J. C. (2017). Actomyosin contractility regulators stabilize the cytoplasmic bridge between the two primordial germ cells during Caenorhabditis elegans embryogenesis. Mol. Biol. Cell 28, 3789–3800. doi:10.1091/mbc.E17-08-0502
Graham, P. L., and Kimble, J. (1993). The mog-1 gene is required for the switch from spermatogenesis to oogenesis in Caenorhabditis elegans. Genetics 133, 919–931. doi:10.1093/genetics/133.4.919
Graham, P. L., Schedl, T., and Kimble, J. (1993). More mog genes that influence the switch from spermatogenesis to oogenesis in the hermaphrodite germ line of Caenorhabditis elegans. Dev. Genet. 14, 471–484. doi:10.1002/dvg.1020140608
Grant, B., and Hirsh, D. (1999). Receptor-mediated endocytosis in the Caenorhabditis elegans oocyte. Mol. Biol. Cell 10, 4311–4326. doi:10.1091/mbc.10.12.4311
Gruidl, M. E., Smith, P. A., Kuznicki, K. A., Mccrone, J. S., Kirchner, J., Roussell, D. L., et al. (1996). Multiple potential germ-line helicases are components of the germ-line-specific P granules of Caenorhabditis elegans. Proc. Natl. Acad. Sci. U. S. A. 93, 13837–13842. doi:10.1073/pnas.93.24.13837
Guven-Ozkan, T., Robertson, S. M., Nishi, Y., and Lin, R. (2010). zif-1 translational repression defines a second, mutually exclusive OMA function in germline transcriptional repression. Development 137, 3373–3382. doi:10.1242/dev.055327
Han, B., Antkowiak, K. R., Fan, X., Rutigliano, M., Ryder, S. P., and Griffin, E. E. (2018). Polo-like kinase couples cytoplasmic protein gradients in the C. elegans zygote. Curr. Biol. 28, 60–69. doi:10.1016/j.cub.2017.11.048
Hansen, D., Wilson-Berry, L., Dang, T., and Schedl, T. (2004). Control of the proliferation versus meiotic development decision in the C. elegans germline through regulation of GLD-1 protein accumulation. Development 131, 93–104. doi:10.1242/dev.00916
Haupt, K. A., Law, K. T., Enright, A. L., Kanzler, C. R., Shin, H., Wickens, M., et al. (2020). A PUF hub drives self-renewal in Caenorhabditis elegans germline stem cells. Genetics 214, 147–161. doi:10.1534/genetics.119.302772
Henderson, M. A., Cronland, E., Dunkelbarger, S., Contreras, V., Strome, S., and Keiper, B. D. (2009). A germline-specific isoform of eIF4E (IFE-1) is required for efficient translation of stored mRNAs and maturation of both oocytes and sperm. J. Cell Sci. 122, 1529–1539. doi:10.1242/jcs.046771
Hentze, M. W., Castello, A., Schwarzl, T., and Preiss, T. (2018). A brave new world of RNA-binding proteins. Nat. Rev. Mol. Cell Biol. 19, 327–341. doi:10.1038/nrm.2017.130
Hu, S., Skelly, L. E., Kaymak, E., Freeberg, L., Lo, T. W., Kuersten, S., et al. (2019). Multi-modal regulation of C. elegans hermaphrodite spermatogenesis by the GLD-1-FOG-2 complex. Dev. Biol. 446, 193–205. doi:10.1016/j.ydbio.2018.11.024
Huang, N. N., and Hunter, C. P. (2015). The RNA binding protein MEX-3 retains asymmetric activity in the early Caenorhabditis elegans embryo in the absence of asymmetric protein localization. Gene 554, 160–173. doi:10.1016/j.gene.2014.10.042
Huang, N. N., Mootz, D. E., Walhout, A. J., Vidal, M., and Hunter, C. P. (2002). MEX-3 interacting proteins link cell polarity to asymmetric gene expression in Caenorhabditis elegans. Development 129, 747–759. doi:10.1242/dev.129.3.747
Hubbard, E. J., and Greenstein, D. (2005). Introduction to the germ line. United States: WormBook, 1–4.
Huggins, H. P., Subash, J. S., Stoffel, H., Henderson, M. A., Hoffman, J. L., Buckner, D. S., et al. (2020). Distinct roles of two eIF4E isoforms in the germline of Caenorhabditis elegans. J. Cell Sci. 133, jcs237990. doi:10.1242/jcs.237990
Hutvagner, G., and Simard, M. J. (2008). Argonaute proteins: Key players in RNA silencing. Nat. Rev. Mol. Cell Biol. 9, 22–32. doi:10.1038/nrm2321
Jadhav, S., Rana, M., and Subramaniam, K. (2008). Multiple maternal proteins coordinate to restrict the translation of C. elegans nanos-2 to primordial germ cells. Development 135, 1803–1812. doi:10.1242/dev.013656
Jankowska-Anyszka, M., Lamphear, B. J., Aamodt, E. J., Harrington, T., Darzynkiewicz, E., Stolarski, R., et al. (1998). Multiple isoforms of eukaryotic protein synthesis initiation factor 4E in Caenorhabditis elegans can distinguish between mono- and trimethylated mRNA cap structures. J. Biol. Chem. 273, 10538–10542. doi:10.1074/jbc.273.17.10538
Jedamzik, B., and Eckmann, C. R. (2009). Analysis of RNA-protein complexes by RNA coimmunoprecipitation and RT-PCR analysis from Caenorhabditis elegans. Cold Spring Harb. Protoc. 2009, pdbprot5300. doi:10.1101/pdb.prot5300
Jones, A. R., Francis, R., and Schedl, T. (1996). GLD-1, a cytoplasmic protein essential for oocyte differentiation, shows stage- and sex-specific expression during Caenorhabditis elegans germline development. Dev. Biol. 180, 165–183. doi:10.1006/dbio.1996.0293
Jones, A. R., and Schedl, T. (1995). Mutations in gld-1, a female germ cell-specific tumor suppressor gene in Caenorhabditis elegans, affect a conserved domain also found in Src-associated protein Sam68. Genes Dev. 9, 1491–1504. doi:10.1101/gad.9.12.1491
Jungkamp, A. C., Stoeckius, M., Mecenas, D., Grun, D., Mastrobuoni, G., Kempa, S., et al. (2011). In vivo and transcriptome-wide identification of RNA binding protein target sites. Mol. Cell 44, 828–840. doi:10.1016/j.molcel.2011.11.009
Kang, M. K., and Han, S. J. (2011). Post-transcriptional and post-translational regulation during mouse oocyte maturation. BMB Rep. 44, 147–157. doi:10.5483/BMBRep.2011.44.3.147
Kapelle, W. S., and Reinke, V. (2011). C. elegans meg-1 and meg-2 differentially interact with nanos family members to either promote or inhibit germ cell proliferation and survival. Genesis 49, 380–391. doi:10.1002/dvg.20726
Karashima, T., Sugimoto, A., and Yamamoto, M. (2000). Caenorhabditis elegans homologue of the human azoospermia factor DAZ is required for oogenesis but not for spermatogenesis. Development 127, 1069–1079. doi:10.1242/dev.127.5.1069
Kawasaki, I., Amiri, A., Fan, Y., Meyer, N., Dunkelbarger, S., Motohashi, T., et al. (2004). The PGL family proteins associate with germ granules and function redundantly in Caenorhabditis elegans germline development. Genetics 167, 645–661. doi:10.1534/genetics.103.023093
Kawasaki, I., Shim, Y. H., Kirchner, J., Kaminker, J., Wood, W. B., and Strome, S. (1998). PGL-1, a predicted RNA-binding component of germ granules, is essential for fertility in C. elegans. Cell 94, 635–645. doi:10.1016/s0092-8674(00)81605-0
Kaymak, E., Farley, B. M., Hay, S. A., Li, C., Ho, S., Hartman, D. J., et al. (2016). Efficient generation of transgenic reporter strains and analysis of expression patterns in Caenorhabditis elegans using library MosSCI. Dev. Dyn. 245, 925–936. doi:10.1002/dvdy.24426
Kaymak, E., and Ryder, S. P. (2013). RNA recognition by the Caenorhabditis elegans oocyte maturation determinant OMA-1. J. Biol. Chem. 288, 30463–30472. doi:10.1074/jbc.M113.496547
Keiper, B. D., Lamphear, B. J., Deshpande, A. M., Jankowska-Anyszka, M., Aamodt, E. J., Blumenthal, T., et al. (2000). Functional characterization of five eIF4E isoforms in Caenorhabditis elegans. J. Biol. Chem. 275, 10590–10596. doi:10.1074/jbc.275.14.10590
Kerins, J. A., Hanazawa, M., Dorsett, M., and Schedl, T. (2010). PRP-17 and the pre-mRNA splicing pathway are preferentially required for the proliferation versus meiotic development decision and germline sex determination in Caenorhabditis elegans. Dev. Dyn. 239, 1555–1572. doi:10.1002/dvdy.22274
Kershner, A. M., and Kimble, J. (2010). Genome-wide analysis of mRNA targets for Caenorhabditis elegans FBF, a conserved stem cell regulator. Proc. Natl. Acad. Sci. U. S. A. 107, 3936–3941. doi:10.1073/pnas.1000495107
Kim, H. M., and Colaiacovo, M. P. (2019). CRISPR-Cas9-Guided genome engineering in Caenorhabditis elegans. Curr. Protoc. Mol. Biol. 129, e106. doi:10.1002/cpmb.106
Kim, K. W., Wilson, T. L., and Kimble, J. (2010). GLD-2/RNP-8 cytoplasmic poly(A) polymerase is a broad-spectrum regulator of the oogenesis program. Proc. Natl. Acad. Sci. U. S. A. 107, 17445–17450. doi:10.1073/pnas.1012611107
Kisielnicka, E., Minasaki, R., and Eckmann, C. R. (2018). MAPK signaling couples SCF-mediated degradation of translational regulators to oocyte meiotic progression. Proc. Natl. Acad. Sci. U. S. A. 115, E2772–E2781. doi:10.1073/pnas.1715439115
Kraemer, B., Crittenden, S., Gallegos, M., Moulder, G., Barstead, R., Kimble, J., et al. (1999). NANOS-3 and FBF proteins physically interact to control the sperm-oocyte switch in Caenorhabditis elegans. Curr. Biol. 9, 1009–1018. doi:10.1016/s0960-9822(99)80449-7
Kuznicki, K. A., Smith, P. A., Leung-Chiu, W. M., Estevez, A. O., Scott, H. C., and Bennett, K. L. (2000). Combinatorial RNA interference indicates GLH-4 can compensate for GLH-1; these two P granule components are critical for fertility in C. elegans. Development 127, 2907–2916. doi:10.1242/dev.127.13.2907
Lamont, L. B., Crittenden, S. L., Bernstein, D., Wickens, M., and Kimble, J. (2004). FBF-1 and FBF-2 regulate the size of the mitotic region in the C. elegans germline. Dev. Cell 7, 697–707. doi:10.1016/j.devcel.2004.09.013
Leacock, S. W., and Reinke, V. (2008). MEG-1 and MEG-2 are embryo-specific P-granule components required for germline development in Caenorhabditis elegans. Genetics 178, 295–306. doi:10.1534/genetics.107.080218
Lee, M. H., and Schedl, T. (2001). Identification of in vivo mRNA targets of GLD-1, a maxi-KH motif containing protein required for C. elegans germ cell development. Genes Dev. 15, 2408–2420. doi:10.1101/gad.915901
Lee, M. H., and Schedl, T. (2004). Translation repression by GLD-1 protects its mRNA targets from nonsense-mediated mRNA decay in C. elegans. Genes Dev. 18, 1047–1059. doi:10.1101/gad.1188404
Li, W., Debella, L. R., Guven-Ozkan, T., Lin, R., and Rose, L. S. (2009). An eIF4E-binding protein regulates katanin protein levels in C. elegans embryos. J. Cell Biol. 187, 33–42. doi:10.1083/jcb.200903003
Loedige, I., Jakob, L., Treiber, T., Ray, D., Stotz, M., Treiber, N., et al. (2015). The crystal structure of the NHL domain in complex with RNA reveals the molecular basis of Drosophila brain-tumor-mediated gene regulation. Cell Rep. 13, 1206–1220. doi:10.1016/j.celrep.2015.09.068
Lublin, A. L., and Evans, T. C. (2007). The RNA-binding proteins PUF-5, PUF-6, and PUF-7 reveal multiple systems for maternal mRNA regulation during C. elegans oogenesis. Dev. Biol. 303, 635–649. doi:10.1016/j.ydbio.2006.12.004
Macdonald, P. M. (1992). The Drosophila pumilio gene: An unusually long transcription unit and an unusual protein. Development 114, 221–232. doi:10.1242/dev.114.1.221
Maheshwari, R., Pushpa, K., and Subramaniam, K. (2016). A role for post-transcriptional control of endoplasmic reticulum dynamics and function in C. elegans germline stem cell maintenance. Development 143, 3097–3108. doi:10.1242/dev.134056
Mainpal, R., Priti, A., and Subramaniam, K. (2011). PUF-8 suppresses the somatic transcription factor PAL-1 expression in C. elegans germline stem cells. Dev. Biol. 360, 195–207. doi:10.1016/j.ydbio.2011.09.021
Mangio, R. S., Votra, S., and Pruyne, D. (2015). The canonical eIF4E isoform of C. elegans regulates growth, embryogenesis, and germline sex-determination. Biol. Open 4, 843–851. doi:10.1242/bio.011585
Marin, V. A., and Evans, T. C. (2003). Translational repression of a C. elegans Notch mRNA by the STAR/KH domain protein GLD-1. Development 130, 2623–2632. doi:10.1242/dev.00486
Maruyama, R., Endo, S., Sugimoto, A., and Yamamoto, M. (2005). Caenorhabditis elegans DAZ-1 is expressed in proliferating germ cells and directs proper nuclear organization and cytoplasmic core formation during oogenesis. Dev. Biol. 277, 142–154. doi:10.1016/j.ydbio.2004.08.053
Matia-Gonzalez, A. M., Laing, E. E., and Gerber, A. P. (2015). Conserved mRNA-binding proteomes in eukaryotic organisms. Nat. Struct. Mol. Biol. 22, 1027–1033. doi:10.1038/nsmb.3128
Mcjunkin, K., and Ambros, V. (2017). A microRNA family exerts maternal control on sex determination in C. elegans. Genes Dev. 31, 422–437. doi:10.1101/gad.290155.116
Mcjunkin, K., and Ambros, V. (2014). The embryonic mir-35 family of microRNAs promotes multiple aspects of fecundity in Caenorhabditis elegans. G3 (Bethesda) 4, 1747–1754. doi:10.1534/g3.114.011973
Mello, C. C., Draper, B. W., Krause, M., Weintraub, H., and Priess, J. R. (1992). The pie-1 and mex-1 genes and maternal control of blastomere identity in early C. elegans embryos. Cell 70, 163–176. doi:10.1016/0092-8674(92)90542-k
Mello, C. C., Schubert, C., Draper, B., Zhang, W., Lobel, R., and Priess, J. R. (1996). The PIE-1 protein and germline specification in C. elegans embryos. Nature 382, 710–712. doi:10.1038/382710a0
Merritt, C., Rasoloson, D., Ko, D., and Seydoux, G. (2008). 3' UTRs are the primary regulators of gene expression in the C. elegans germline. Curr. Biol. 18, 1476–1482. doi:10.1016/j.cub.2008.08.013
Merritt, C., and Seydoux, G. (2010). The Puf RNA-binding proteins FBF-1 and FBF-2 inhibit the expression of synaptonemal complex proteins in germline stem cells. Development 137, 1787–1798. doi:10.1242/dev.050799
Millonigg, S., Minasaki, R., Nousch, M., Novak, J., and Eckmann, C. R. (2014). GLD-4-mediated translational activation regulates the size of the proliferative germ cell pool in the adult C. elegans germ line. PLoS Genet. 10, e1004647. doi:10.1371/journal.pgen.1004647
Molin, L., and Puisieux, A. (2005). C. elegans homologue of the Caf1 gene, which encodes a subunit of the CCR4-NOT complex, is essential for embryonic and larval development and for meiotic progression. Gene 358, 73–81. doi:10.1016/j.gene.2005.05.023
Mootz, D., Ho, D. M., and Hunter, C. P. (2004). The STAR/Maxi-KH domain protein GLD-1 mediates a developmental switch in the translational control of C. elegans PAL-1. Development 131, 3263–3272. doi:10.1242/dev.01196
Nakahata, S., Katsu, Y., Mita, K., Inoue, K., Nagahama, Y., and Yamashita, M. (2001). Biochemical identification of Xenopus Pumilio as a sequence-specific cyclin B1 mRNA-binding protein that physically interacts with a Nanos homolog, Xcat-2, and a cytoplasmic polyadenylation element-binding protein. J. Biol. Chem. 276, 20945–20953. doi:10.1074/jbc.M010528200
Nakel, K., Bonneau, F., Basquin, C., Habermann, B., Eckmann, C. R., and Conti, E. (2016). Structural basis for the antagonistic roles of RNP-8 and GLD-3 in GLD-2 poly(A)-polymerase activity. RNA 22, 1139–1145. doi:10.1261/rna.056598.116
Noble, D. C., Aoki, S. T., Ortiz, M. A., Kim, K. W., Verheyden, J. M., and Kimble, J. (2016). Genomic analyses of sperm fate regulator targets reveal a common set of oogenic mRNAs in Caenorhabditis elegans. Genetics 202, 221–234. doi:10.1534/genetics.115.182592
Nousch, M., and Eckmann, C. R. (2013). Translational control in the Caenorhabditis elegans germ line. Adv. Exp. Med. Biol. 757, 205–247. doi:10.1007/978-1-4614-4015-4_8
Nousch, M., Techritz, N., Hampel, D., Millonigg, S., and Eckmann, C. R. (2013). The Ccr4-Not deadenylase complex constitutes the main poly(A) removal activity in C. elegans. J. Cell Sci. 126, 4274–4285. doi:10.1242/jcs.132936
Ogura, K., Kishimoto, N., Mitani, S., Gengyo-Ando, K., and Kohara, Y. (2003). Translational control of maternal glp-1 mRNA by POS-1 and its interacting protein SPN-4 in Caenorhabditis elegans. Development 130, 2495–2503. doi:10.1242/dev.00469
Opperman, L., Hook, B., Defino, M., Bernstein, D. S., and Wickens, M. (2005). A single spacer nucleotide determines the specificities of two mRNA regulatory proteins. Nat. Struct. Mol. Biol. 12, 945–951. doi:10.1038/nsmb1010
Otori, M., Karashima, T., and Yamamoto, M. (2006). The Caenorhabditis elegans homologue of deleted in azoospermia is involved in the sperm/oocyte switch. Mol. Biol. Cell 17, 3147–3155. doi:10.1091/mbc.e05-11-1067
Pagano, J. M., Farley, B. M., Essien, K. I., and Ryder, S. P. (2009). RNA recognition by the embryonic cell fate determinant and germline totipotency factor MEX-3. Proc. Natl. Acad. Sci. U. S. A. 106, 20252–20257. doi:10.1073/pnas.0907916106
Pagano, J. M., Farley, B. M., Mccoig, L. M., and Ryder, S. P. (2007). Molecular basis of RNA recognition by the embryonic polarity determinant MEX-5. J. Biol. Chem. 282, 8883–8894. doi:10.1074/jbc.M700079200
Parker, D. M., Winkenbach, L. P., Boyson, S., Saxton, M. N., Daidone, C., AL-Mazaydeh, Z. A., et al. (2020). mRNA localization is linked to translation regulation in the Caenorhabditis elegans germ lineage. Development 147, 1.
Porter, D. F., Prasad, A., Carrick, B. H., Kroll-Connor, P., Wickens, M., and Kimble, J. (2019). Toward identifying subnetworks from FBF binding landscapes in Caenorhabditis spermatogenic or oogenic germlines. G3 (Bethesda) 9, 153–165. doi:10.1534/g3.118.200300
Priti, A., and Subramaniam, K. (2015). PUF-8 functions redundantly with GLD-1 to promote the meiotic progression of spermatocytes in Caenorhabditis elegans. G3 (Bethesda) 5, 1675–1684. doi:10.1534/g3.115.019521
Puoti, A., and Kimble, J. (2000). The hermaphrodite sperm/oocyte switch requires the Caenorhabditis elegans homologs of PRP2 and PRP22. Proc. Natl. Acad. Sci. U. S. A. 97, 3276–3281. doi:10.1073/pnas.97.7.3276
Pushpa, K., Kumar, G. A., and Subramaniam, K. (2017). Translational control of germ cell decisions. Results Probl. Cell Differ. 59, 175–200. doi:10.1007/978-3-319-44820-6_6
Ray, D., Kazan, H., Chan, E. T., Pena Castillo, L., Chaudhry, S., Talukder, S., et al. (2009). Rapid and systematic analysis of the RNA recognition specificities of RNA-binding proteins. Nat. Biotechnol. 27, 667–670. doi:10.1038/nbt.1550
Richter, J. D. (1991). Translational control during early development. Bioessays 13, 179–183. doi:10.1002/bies.950130406
Ryder, S. P., Frater, L. A., Abramovitz, D. L., Goodwin, E. B., and Williamson, J. R. (2004). RNA target specificity of the STAR/GSG domain post-transcriptional regulatory protein GLD-1. Nat. Struct. Mol. Biol. 11, 20–28. doi:10.1038/nsmb706
Ryder, S. P. (2016F1000). Protein-mRNA interactome capture: Cartography of the mRNP landscape. F1000Res. 5, 2627. doi:10.12688/f1000research.9404.1
Scheckel, C., Gaidatzis, D., Wright, J. E., and Ciosk, R. (2012). Genome-wide analysis of GLD-1-mediated mRNA regulation suggests a role in mRNA storage. PLoS Genet. 8, e1002742. doi:10.1371/journal.pgen.1002742
Schisa, J. A., Pitt, J. N., and Priess, J. R. (2001). Analysis of RNA associated with P granules in germ cells of C. elegans adults. Development 128, 1287–1298. doi:10.1242/dev.128.8.1287
Schmid, M., Kuchler, B., and Eckmann, C. R. (2009). Two conserved regulatory cytoplasmic poly(A) polymerases, GLD-4 and GLD-2, regulate meiotic progression in C. elegans. Genes Dev. 23, 824–836. doi:10.1101/gad.494009
Schubert, C. M., Lin, R., DE Vries, C. J., Plasterk, R. H., and Priess, J. R. (2000). MEX-5 and MEX-6 function to establish soma/germline asymmetry in early C. elegans embryos. Mol. Cell 5, 671–682. doi:10.1016/s1097-2765(00)80246-4
Sengupta, M. S., Low, W. Y., Patterson, J. R., Kim, H. M., Traven, A., Beilharz, T. H., et al. (2013). ifet-1 is a broad-scale translational repressor required for normal P granule formation in C. elegans. J. Cell Sci. 126, 850–859. doi:10.1242/jcs.119834
Seydoux, G., Mello, C. C., Pettitt, J., Wood, W. B., Priess, J. R., and Fire, A. (1996). Repression of gene expression in the embryonic germ lineage of C. elegans. Nature 382, 713–716. doi:10.1038/382713a0
Shaw, W. R., Armisen, J., Lehrbach, N. J., and Miska, E. A. (2010). The conserved miR-51 microRNA family is redundantly required for embryonic development and pharynx attachment in Caenorhabditis elegans. Genetics 185, 897–905. doi:10.1534/genetics.110.117515
Slack, F. J., Basson, M., Liu, Z., Ambros, V., Horvitz, H. R., and Ruvkun, G. (2000). The lin-41 RBCC gene acts in the C. elegans heterochronic pathway between the let-7 regulatory RNA and the LIN-29 transcription factor. Mol. Cell 5, 659–669. doi:10.1016/s1097-2765(00)80245-2
Spike, C. A., Coetzee, D., Eichten, C., Wang, X., Hansen, D., and Greenstein, D. (2014a). The TRIM-NHL protein LIN-41 and the OMA RNA-binding proteins antagonistically control the prophase-to-metaphase transition and growth of Caenorhabditis elegans oocytes. Genetics 198, 1535–1558. doi:10.1534/genetics.114.168831
Spike, C. A., Coetzee, D., Nishi, Y., Guven-Ozkan, T., Oldenbroek, M., Yamamoto, I., et al. (2014b). Translational control of the oogenic program by components of OMA ribonucleoprotein particles in Caenorhabditis elegans. Genetics 198, 1513–1533. doi:10.1534/genetics.114.168823
Spike, C. A., Huelgas-Morales, G., Tsukamoto, T., and Greenstein, D. (2018). Multiple mechanisms inactivate the LIN-41 RNA-binding protein to ensure a robust oocyte-to-embryo transition in Caenorhabditis elegans. Genetics 210, 1011–1037. doi:10.1534/genetics.118.301421
Stumpf, C. R., Kimble, J., and Wickens, M. (2008). A Caenorhabditis elegans PUF protein family with distinct RNA binding specificity. RNA 14, 1550–1557. doi:10.1261/rna.1095908
Subramaniam, K., and Seydoux, G. (2003). Dedifferentiation of primary spermatocytes into germ cell tumors in C. elegans lacking the pumilio-like protein PUF-8. Curr. Biol. 13, 134–139. doi:10.1016/s0960-9822(03)00005-8
Suh, N., Crittenden, S. L., Goldstrohm, A., Hook, B., Thompson, B., Wickens, M., et al. (2009). FBF and its dual control of gld-1 expression in the Caenorhabditis elegans germline. Genetics 181, 1249–1260. doi:10.1534/genetics.108.099440
Suh, N., Jedamzik, B., Eckmann, C. R., Wickens, M., and Kimble, J. (2006). The GLD-2 poly(A) polymerase activates gld-1 mRNA in the Caenorhabditis elegans germ line. Proc. Natl. Acad. Sci. U. S. A. 103, 15108–15112. doi:10.1073/pnas.0607050103
Tabara, H., Hill, R. J., Mello, C. C., Priess, J. R., and Kohara, Y. (1999). pos-1 encodes a cytoplasmic zinc-finger protein essential for germline specification in C. elegans. Development 126, 1–11. doi:10.1242/dev.126.1.1
Tadauchi, T., Matsumoto, K., Herskowitz, I., and Irie, K. (2001). Post-transcriptional regulation through the HO 3'-UTR by Mpt5, a yeast homolog of Pumilio and FBF. EMBO J. 20, 552–561. doi:10.1093/emboj/20.3.552
Tamburino, A. M., Ryder, S. P., and Walhout, A. J. (2013). A compendium of Caenorhabditis elegans RNA binding proteins predicts extensive regulation at multiple levels. G3 (Bethesda) 3, 297–304. doi:10.1534/g3.112.004390
Tenenhaus, C., Subramaniam, K., Dunn, M. A., and Seydoux, G. (2001). PIE-1 is a bifunctional protein that regulates maternal and zygotic gene expression in the embryonic germ line of Caenorhabditis elegans. Genes Dev. 15, 1031–1040. doi:10.1101/gad.876201
Theil, K., Herzog, M., and Rajewsky, N. (2018). Post-transcriptional regulation by 3' UTRs can Be masked by regulatory elements in 5' UTRs. Cell Rep. 22, 3217–3226. doi:10.1016/j.celrep.2018.02.094
Theil, K., Imami, K., and Rajewsky, N. (2019). Identification of proteins and miRNAs that specifically bind an mRNA in vivo. Nat. Commun. 10, 4205. doi:10.1038/s41467-019-12050-7
Tsukamoto, T., Gearhart, M. D., Spike, C. A., Huelgas-Morales, G., Mews, M., Boag, P. R., et al. (2017). LIN-41 and OMA ribonucleoprotein complexes mediate a translational repression-to-activation switch controlling oocyte meiotic maturation and the oocyte-to-embryo transition in Caenorhabditis elegans. Genetics 206, 2007–2039. doi:10.1534/genetics.117.203174
Updike, D. L., Knutson, A. K., Egelhofer, T. A., Campbell, A. C., and Strome, S. (2014). Germ-granule components prevent somatic development in the C. elegans germline. Curr. Biol. 24, 970–975. doi:10.1016/j.cub.2014.03.015
Updike, D., and Strome, S. (2010). P granule assembly and function in Caenorhabditis elegans germ cells. J. Androl. 31, 53–60. doi:10.2164/jandrol.109.008292
Vicencio, J., and Ceron, J. (2021). A living organism in your CRISPR toolbox: Caenorhabditis elegans is a rapid and efficient model for developing CRISPR-cas technologies. CRISPR J. 4, 32–42. doi:10.1089/crispr.2020.0103
Wang, J. T., Smith, J., Chen, B. C., Schmidt, H., Rasoloson, D., Paix, A., et al. (2014). Regulation of RNA granule dynamics by phosphorylation of serine-rich, intrinsically disordered proteins in C. elegans. Elife 3, e04591. doi:10.7554/eLife.04591
Wang, L., Eckmann, C. R., Kadyk, L. C., Wickens, M., and Kimble, J. (2002a). A regulatory cytoplasmic poly(A) polymerase in Caenorhabditis elegans. Nature 419, 312–316. doi:10.1038/nature01039
Wang, X., Ellenbecker, M., Hickey, B., Day, N. J., Osterli, E., Terzo, M., et al. (2020). Antagonistic control of Caenorhabditis elegans germline stem cell proliferation and differentiation by PUF proteins FBF-1 and FBF-2, 9.Elife.
Wang, X., Mclachlan, J., Zamore, P. D., and Hall, T. M. (2002b). Modular recognition of RNA by a human pumilio-homology domain. Cell 110, 501–512. doi:10.1016/s0092-8674(02)00873-5
Weiser, N. E., and Kim, J. K. (2019). Multigenerational regulation of the Caenorhabditis elegans chromatin landscape by germline small RNAs. Annu. Rev. Genet. 53, 289–311. doi:10.1146/annurev-genet-112618-043505
Wickens, M. (1990). In the beginning is the end: Regulation of poly(A) addition and removal during early development. Trends Biochem. Sci. 15, 320–324. doi:10.1016/0968-0004(90)90022-4
Wright, J. E., Gaidatzis, D., Senften, M., Farley, B. M., Westhof, E., Ryder, S. P., et al. (2011). A quantitative RNA code for mRNA target selection by the germline fate determinant GLD-1. EMBO J. 30, 533–545. doi:10.1038/emboj.2010.334
Wu, E., Thivierge, C., Flamand, M., Mathonnet, G., Vashisht, A. A., Wohlschlegel, J., et al. (2010). Pervasive and cooperative deadenylation of 3'UTRs by embryonic microRNA families. Mol. Cell 40, 558–570. doi:10.1016/j.molcel.2010.11.003
Zamore, P. D., Williamson, J. R., and Lehmann, R. (1997). The Pumilio protein binds RNA through a conserved domain that defines a new class of RNA-binding proteins. RNA 3, 1421–1433.
Zhang, B., Gallegos, M., Puoti, A., Durkin, E., Fields, S., Kimble, J., et al. (1997). A conserved RNA-binding protein that regulates sexual fates in the C. elegans hermaphrodite germ line. Nature 390, 477–484. doi:10.1038/37297
Keywords: RNA-binding proteins, nematode, germline, post-transcriptional regulation, embryogenesis, oogenesis, translation
Citation: Albarqi MMY and Ryder SP (2023) The role of RNA-binding proteins in orchestrating germline development in Caenorhabditis elegans. Front. Cell Dev. Biol. 10:1094295. doi: 10.3389/fcell.2022.1094295
Received: 09 November 2022; Accepted: 19 December 2022;
Published: 04 January 2023.
Edited by:
Cristina Montiel Duarte, Nottingham Trent University, United KingdomReviewed by:
Elif Sarinay Cenik, The University of Texas at Austin, United StatesKuppuswamy Subramaniam, Indian Institute of Technology Madras, India
Ekaterina Voronina, University of Montana, United States
Copyright © 2023 Albarqi and Ryder. This is an open-access article distributed under the terms of the Creative Commons Attribution License (CC BY). The use, distribution or reproduction in other forums is permitted, provided the original author(s) and the copyright owner(s) are credited and that the original publication in this journal is cited, in accordance with accepted academic practice. No use, distribution or reproduction is permitted which does not comply with these terms.
*Correspondence: Sean P. Ryder, c2Vhbi5yeWRlckB1bWFzc21lZC5lZHU=
†Present address: Mennatallah M. Y. Albarqi, Stanford Cancer Institute, Stanford University, Palo Alto, CA, United States