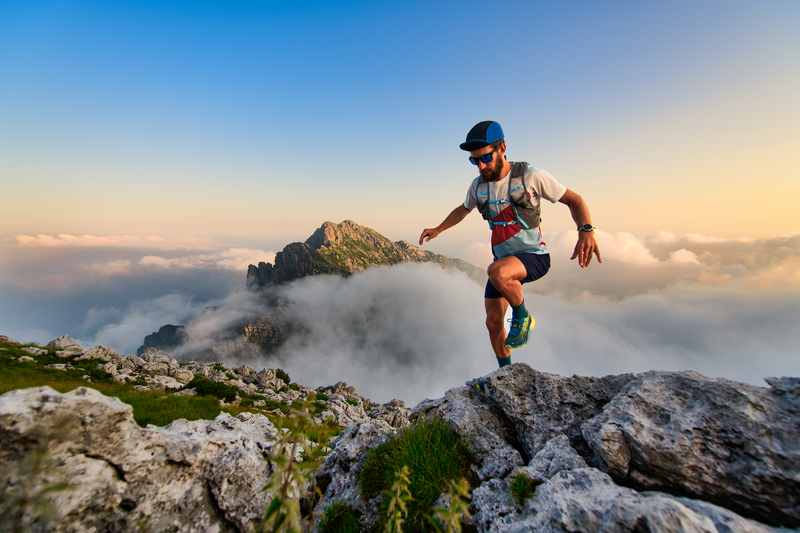
95% of researchers rate our articles as excellent or good
Learn more about the work of our research integrity team to safeguard the quality of each article we publish.
Find out more
ORIGINAL RESEARCH article
Front. Cell Dev. Biol. , 21 November 2022
Sec. Cell Adhesion and Migration
Volume 10 - 2022 | https://doi.org/10.3389/fcell.2022.1083130
This article is part of the Research Topic Mechanobiology of Organoid Systems View all 6 articles
Mechanical microenvironment and cellular senescence of trabecular meshwork cells (TMCs) are suspected to play a vital role in primary open-angle glaucoma pathogenesis. However, central questions remain about the effect of shear stress on TMCs and how aging affects this process. We have investigated the effect of shear stress on the biomechanical properties and extracellular matrix regulation of normal and senescent TMCs. We found a more significant promotion of Fctin formation, a more obvious realignment of F-actin fibers, and a more remarkable increase in the stiffness of normal cells in response to the shear stress, in comparison with that of senescent cells. Further, as compared to normal cells, senescent cells show a reduced extracellular matrix turnover after shear stress stimulation, which might be attributed to the different phosphorylation levels of the extracellular signal-regulated kinase. Our results suggest that TMCs are able to sense and respond to the shear stress and cellular senescence undermines the mechanobiological response, which may lead to progressive failure of cellular TM function with age.
As the second major cause of blindness globally (Bourne, 2006; Cook and Foster, 2012), glaucoma is a group of eye diseases that lead to optic nerve damage and consequent irreversible visual loss (Tian et al., 2017). Glaucoma is often classified into several types, among which primary open-angle glaucoma (POAG) is one of the most common ones (Weinreb and Khaw, 2004). Nowadays, POAG affects approximately 57.5 million people worldwide (Wiggs and Pasquale, 2017; Allison et al., 2020), and it is predicted that approximately 111.8 million people will suffer from glaucoma by 2040 (Tham et al., 2014).
Although the molecular mechanism of glaucoma pathogenesis is poorly understood, elevated intraocular pressure (IOP) resulting from increased resistance to aqueous humor outflow in the TM conventional outflow pathway is considered one of the main risk factors for POAG (Gabelt and Kaufman, 2005; Mcmonnies, 2017; Buffault et al., 2020; Li and Song, 2020). Located in the anterior chamber angle of the eye, TM is a mechanosensitive tissue that mediates 80–90% of aqueous outflow (Yuan et al., 2016; Yarishkin et al., 2021). TMCs are able to adjust the aqueous outflow facility through remodeling the actin cytoskeleton (Clark et al., 2005; Rao et al., 2005; Rao et al., 2017), or changing extracellular matrix (ECM) turnover and subsequent ECM replacement rates by modulating matrix metalloproteinases (MMPs) activity (Bradley et al., 2001; Keller et al., 2009; Pattabiraman and Rao, 2010; Vranka and Acott, 2017). Impaired regulation of these cellular functions leads to IOP dysregulation and has been advocated as a pathogenic factor of POAG (Last et al., 2011; Vahabikashi et al., 2019). Although the underlying mechanisms remain elusive, it has been reported that the extracellular signal-regulated kinase (ERK) pathway is involved in regulating the production of MMPs in TM (Alexander and Acott, 2003). Studies indicate that the ERK pathway can affect the secretion of MMPs in TMCs, which may lead to an aberrant accumulation of ECM and consequently elevated IOP that eventually develops glaucoma (Shearer and Crosson, 2001; Conley et al., 2004).
The bulk flow of aqueous humor driven by IOP imposes shear stress on the conventional outflow pathway (Wudunn, 2009; Yarishkin et al., 2021). This shear stress is predicted to be in the range of 2–25 dyn/cm2, which could be higher due to the elevated IOP in glaucoma (Ethier et al., 2004). Existing studies suggested that the TMCs could respond to shear stress imposed by aqueous humor flow (Carreon et al., 2017), thus providing a means of regulatory feedback to control IOP (Johnstone, 2004; Yarishkin et al., 2021). Recently, it has been proven that the shear stress-induced change of TMCs may be involved in the increase in outflow resistance in glaucoma. For example, Patel et al. found that impaired TRPV4-eNOS signaling activated by increased fluid shear stress in TMCs contributes to elevated IOP in glaucoma (Patel et al., 2021). Meanwhile, Yarishkin et al. reported that the shear stress could activate Piezo1, leading to an increased number of focal cell-matrix contacts of human TMC, a determinant of mechanically induced aqueous humor outflow which in turn contributes to TM stiffness (Yarishkin et al., 2021). But the effects of shear stress on the important cellular functions of TMC, such as cytoskeleton remodeling, cell migration, and MMP production, remain unknown so far.
The risk of developing POAG clearly increases with age (Yoshida et al., 2001; Friedman et al., 2004; Cook and Foster, 2012). Aging is a process associated with the accumulation of damages that brings about a progressive decline in cellular and physiological function, which can attenuate the cellular capacity to feel and respond to stress and then increase the risk of degenerative diseases. According to the oxidative stress theory, the accumulation of negative effects induced by reactive oxygen species (ROS) results in progressive loss of functions in aging (Liguori et al., 2018). The TM is the most sensitive tissue to oxidative damage in the anterior chamber (Izzotti et al., 2009). Senescence of the TMCs is assumed as a major risk factor in the development or progression of POAG (Liton et al., 2008; Liton et al., 2009). Numerous studies have demonstrated that cell senescence could alter the morphology (Saccà et al., 2016), cytoskeleton (Zhou et al., 1999), phenotype (Liton et al., 2005), and functions (Alvarado et al., 2005; Zhao et al., 2016) of the TMCs. However, how cell senescence influences the TMCs’ response to shear stress is barely known.
Here, we investigated the effects of senescence on the responses of porcine trabecular meshwork (PTM) cells to shear stress. Our results showed that the mechanotransduction of PTM cells could be altered by cell senescence. A more remarkable realignment of F-actin fibers, a more significant promotion of Fctin formation, and a greater increase in cell stiffness in response to the shear stress were observed in normal PTM cells compared with senescent PTM cells. Shear stress enhanced the capability of cell migration of normal PTM cells whereas decreased that of senescent PTM cells. Moreover, senescent PTM cells exhibited altered changes in ECM turnover-related protein after the shear stress stimulation in comparison with normal PTM cells, which may be associated with the difference in phosphorylation levels of ERK. Our findings indicate that the cell senescence compromises the physiological responses of PTM cells to shear stress.
Primary cultures of PTM cells were prepared from porcine eyes obtained from the local abattoir within less than 4 h postmortem. Briefly, the TM was dissected from surrounding tissue as previously described (Obazawa et al., 2004; Liton et al., 2008). The tissue was then placed in collagen I-coated 35-mm dishes and cultivated in a TM culture medium which consists of low-glucose Dulbecco’s modified Eagle’s medium (DMEM; Hyclone, United States) supplemented with l-glutamine and 110 mg/L sodium pyruvate, 100 mM nonessential amino acids, 100 U/mL penicillin, 100 mg/ml streptomycin sulfate and 20% fetal bovine serum (FBS) (Li et al., 2007). All the reagents were obtained from Invitrogen (Carlsbad, CA). After one passage, serum was reduced to 10% for routine cultivation. Cells were maintained and propagated at 37 °C in humidified air with 5% CO2. When the cells reached confluency, they were subcultivated 1:3. The passages three to four of the PTM cell were used in our study. More than 20 different porcine cell lines were studied. For each experiment, we used at least three different cell lines. The PTM cells used in this study were characterized as previously described (Polansky et al., 2000; Snider et al., 2015; Keller et al., 2018). Briefly, cultured cells at passage three were grown to confluence and then treated with 100 nM dexamethasone for 7 days before the myocilin mRNA expression level was examined. Also, cells at passage three were carefully seeded on the prepared slide and the expression of fibronectin (FN) and laminin (LN) was evaluated by immunochemical staining.
PTM cells were subjected to normobaric hyperoxia conditions as previously described (Saretzki et al., 1998; Liton et al., 2008). Confluent cultures of PTM cells at passage three were grown for 2 weeks at 40% O2 and 5% CO2 in a triple-gas incubator (China Innovation Instrument, Ningbo, China). Control cultures were grown under 5% CO2 and atmospheric oxygen concentration. The senescence β-galactosidase staining kit (Beyotime, Shanghai, China) was used according to the manufacturer’s protocol to stain senescent PTM cells. The staining was visualized with a Nikon Eclipse inverted microscope system. The percentage of senescent cells was calculated as the number of cells that contained the blue β-galactosidase staining divided by the total number of cells in the field of view.
Cell cycle was determined by flow cytometry as previously described (Zheng et al., 2016). Cells were incubated with 20 μg/ml DNase-free RNase A (Beyotime, Shanghai, China) at 37°C, washed with PBS (phosphate buffer saline) and stained with propidium iodide (PI) (Beyotime, Shanghai, China). Cell cycle was analyzed using BD CellQuest Pro software by FACSCalibur™ (BD, Franklin Lakes, NJ, United States).
A parallel plate flow chamber was utilized to produce shear stress as described previously (Zheng et al., 2016; Huang et al., 2019). The PTM cells cultured on glass slides were placed in the flow block that can provide controlled shear stress τ = 6Qμ/(wh2), where the shear stress (τ) depended on the flow rate (Q), the viscosity of the culture media (μ), as well as the width (w) and height 8) of the flow channel. The whole device was incubated at 37°C in a humidified atmosphere of 5% CO2 in the air. A steady laminar shear flow of 25 dyn/cm2 was loaded.
Cells were fixed in 4% paraformaldehyde, then were permeabilized with 0.1% Triton X-100 in PBS and blocked in 1% bovine serum albumin. Cells were incubated in Texas red isothioc2yanate-conjugated phalloidin (Invitrogen, Carlsbad, CA) for 30 min to stain the F-actin filaments. Then the cells were incubated with DAPI (Sigma, St. Louis, MO, United States) for 5 min to label cell nuclear. The fluorescent images were taken under a confocal microscope (TCS-SP5; Leica, Solms, Germany). The angle of cell is defined as the angle between the main axis of the cell and the flow direction. The cell angles and average optical intensity of F-actin were calculated with ImageJ software (NIH Image, Bethesda, MD).
Cell stiffness was determined as described previously (Ding et al., 2015; Sancho et al., 2017; Gu et al., 2018) using the atomic force microscope (AFM, Agilent 5,500, United States) equipped with an inverted fluorescence microscope (Nikon TE 2000U). Acquisition of F-D curves was carried out on Picoview SPM System (Agilent 5,500, United States). We employed a very low loading rate of 0.25 μm/s, and an indentation depth of 300 nm. The spherical probe was applied here to determine the overall stiffness of each cell. The calibrated spring constant was given as 0.08 N/m. The probe tip was a SiO2 sphere with a diameter of 11.41 μm. Cells were selected randomly and each of them was compressed three times. More than 10 cells were measured for each treatment condition and each experiment was repeated three times. The F-D curves were fitted by the Hertz model. A MATLAB program was used to process all the data.
The migration of PTM cells was measured with a transwell migration apparatus as described previously (Hogg et al., 2000; Kim, 2016). Briefly, cells were trypsinized and resuspended at a density of 6×105 cells/ml in serum-free media. Then, the PTM cells were added into the upper wells of a transwell chamber (Corning, United States). Culture media with 10% FBS were added into the lower wells. After incubating for 24 h, cells were fixed and stained with DAPI. Migrated cells attached to the bottom of the filter were counted under a fluorescent microscope.
After removing the culture medium, PTM cells were immediately immersed in RNAlater™ (Qiagen, Valencia, CA) to preserve RNA integrity. Total RNA was isolated from PTM cultures using an RNase kit (Qiagen) according to the manufacturer’s protocol and was treated with DNase. RNA yields were determined using Ribogreen fluorescent dye (Molecular Probes). First-strand cDNA was synthesized from 0.5 µg total RNA by reverse transcription using an oligo dT primer and Superscript II reverse transcriptase (Invitrogen) according to the manufacturer’s instructions. Reverse transcription-polymerase chain reaction (RT-PCR) analyses were performed using the PCR parameters shown in Table 1. Glyceraldehyde 3-phosphate dehydrogenase (GAPDH) or β-actin was used as an internal standard of mRNA expression. The sequences of the primers used for the amplifications are listed in Table 2.
Cell lysates were prepared using RIPA solution, and protein concentration was determined with a BCA protein determination kit (Ythxbio, China). Equal amounts of protein samples (25 μg) were separated by SDS-PAGE (10.0% acrylamide gel slabs) and then transferred to PVDF membranes (Bio-Rad). The PVDF membranes were blocked with 5% BSA and incubated overnight with anti-GAPDH antibody (Beyotime, China), ERK antibody (Santa Cruz, CA), or p-ERK antibody (Santa Cruz, CA), followed by incubation with secondary antibodies conjugated to peroxidase. GAPDH was used as a loading control.
These experiments were repeated at least three times independently with different cell lines, as described above. Data were represented as the mean ± SD and were analyzed by one-way analysis of variance (one-way ANOVA). Data analysis was performed with GraphPad Prism7 (GraphPad Software Inc., United States) and SPSS 19.0 (SPSS Inc., United States). Differences were considered statistically significant at p < 0.05.
Morphologically, confluent cultures of cells exhibited the typical morphology of PTM cells, i.e., long shuttle in shape (Supplementary Figure S1B) (Mao et al., 2013; Stamer and Clark, 2017). Measured by immunohistochemical staining, the cells in this study expressed TM cells biomarker FN and LN (Supplementary Figure S1C) (Khaw et al., 1994; Ge et al., 2016; Wang et al., 2017; Huang et al., 2022). Because the neighboring cells do not respond as robustly, the induced myocilin expression in response to DEX is widely accepted as a gold standard in TM cell characterization (Polansky et al., 2000; Snider et al., 2015; Gu et al., 2018). In this study, 100 nM DEX was added for 7 days to provoke robust myocilin production, as assessed by PCR (Supplementary Figure S1D, E). By examining cell morphology, biomarkers, and myocilin induction, the identity of TM cells was established.
In this study, we adopted the normobaric hyperoxia treatment to induce senescent cells (Gille and Joenje, 1992; Liton et al., 2008). We found that these cells grown in 40% O2 exhibited morphology with enlarged cell size (Figure 1B) compared to normal PTM morphology (Figure 1A). After 2-week exposure to hyperoxia, PTM cells stained positively for the cell senescence marker β-galactosidase (Figure 1D), whereas PTM cells in control have negligible staining for this maker (Figure 1C), as shown in Figure 1E. Further, flow cytometry results showed that the proportion of G2/M phase cells appeared to decrease after exposure to hyperoxia (Figure 1H) compared to the control group (Figure 1G). Quantitative results were shown in Figure 1F.
FIGURE 1. Hyperoxia as an experimental model of senescence for porcine trabecular meshwork (PTM) cells. Morphology of PTM cells grown for 2 weeks under control (A) or hyperoxic (40% O2) conditions (B). β-galactosidase staining of PTM cells grown for 2 weeks under control (C) or hyperoxic (40% O2) conditions (D). PTM cells grown under the control conditions exhibited negligible staining for senescence marker β-galactosidase, whereas cells exposed to hyperoxia stained positive for this marker (E) (***p < 0.001). The proportion of cells in the S phase and G2 phase decreased after hyperoxia exposure compared with the control group (F) (**p < 0.01). Flow cytometry quantification of the cell cycle of PTM cells grown for 2 weeks under control (G) or hyperoxic (40% O2) conditions (H).
The quantified alignment of PTM cells was depicted as a proportion of cells within ±30° range with respect to the flow axis. For normal PTM cells, it was shown in Figures 2A,B that the percentage of cells aligned with the angle of orientation ranging from -30° to 30° with respect to the flow axis (0°) was significantly increased after 12-h exposure to shear stress of 25 dyn/cm2 compared with the static group (no shear stress). In contrast, for senescent PTM cells, the percentage of cells did not vary significantly after exposure to sheer stress compared with the static group (Figures 2A,B). These results suggested that, after exposure to shear stress of 25 dyn/cm2 for 12 h, normal PTM cells tended to orient in the direction of the flow, while senescent PTM cells did not respond in the same way.
FIGURE 2. Effect of senescence on cytoskeleton and cell stiffness of PTM cells in response to shear stress. (A) Fluorescence images of normal and senescent PTM cells under static conditions (no shear stress) or subjected to shear stress of 25 dyn/cm2. Red: phalloidin, Blue: DAPI. (B) Alignment of the cytoskeleton of normal and senescent PTM cells under static conditions or subjected to shear stress (*p < 0.05). (C) F-actin content of normal and senescent PTM cells under static conditions or subjected to shear stress (**p < 0.01, ***p < 0.001). (D) Cell stiffness of normal and senescent PTM cells under static conditions or subjected to shear stress (*p < 0.05). SS stands for shear stress.
As shown in Figure 2C, these senescent PTM cells demonstrated a significant increase in F-actin content compared with normal PTM cells. When exposing cells to shear stress, F-actin content was significantly improved in both normal and senescent PTM cells. Correspondingly, AFM results indicated that the senescence of PTM cells led to an increase in cell stiffness (Figure 2D). After being subjected to the shear stress, both normal and senescent PTM cells exhibited increased cell stiffness. Quantitatively, we observed an increase in stiffness by 67.44% and 36.14% for normal and senescent PTM cells, respectively, after exposure to the shear stress. As expected, simultaneously exposing PTM cells to hyperoxia and shear stress led to the most remarkable change in cell stiffness compared with the control (Figure 2D). Our findings are consistent with existing results which suggested that the cell stiffness is positively correlative to the F-actin content (Starodubtseva, 2011).
Transwell assays were performed to determine the migration ability of normal and senescent PTM cells subjected to the shear stress. We found that the senescent PTM cells had a significantly lower migration rate compared with normal PTM cells. After 12-h exposure to the shear stress, the migration ability of normal PTM cells significantly increased compared with the static group. However, the opposite was true for the senescent PTM cells (Figures 3A,B).
FIGURE 3. Effect of senescence on cell migration of PTM cells in response to shear stress. (A) Transwell migration assay for normal and senescent PTM cells under static conditions or subjected to shear stress (B) Migration activity of normal and senescent PTM cells under static conditions or subjected to shear stress (*p < 0.05, ***p < 0.001). SS stands for shear stress.
Results from RT-PCR indicated that, for normal PTM cells, the mRNA expressions of MMP-1, MMP-2, TIMP-1, and TIMP-2 were significantly up-regulated after 12-h exposure to the shear stress compared to the static group. However, the mRNA expressions of these specific proteins, except TIMP-1, were significantly down-regulated for senescent PTM cells (Figures 4A–E). In addition, the mRNA expression of collagen I (COLA-1) and collagen IV (COLA-4) did not change for both normal and senescent PTM cells after exposure to the shear stress (Figures 4F–I).
FIGURE 4. Effect of senescence on matrix metalloproteinases (MMP), tissue inhibitors of metalloproteinases (TIMP), and collagen mRNA expression of PTM cells in response to shear stress. (A–E) PCR quantification of MMP-1, MMP-2, TIMP-1 and TIMP-2 mRNA expression of normal and senescent PTM cells under static conditions or subjected to shear stress (*p < 0.05, **p < 0.01). (F, H) PCR quantification of collagen I (COLA-1) mRNA expression of normal and senescent PTM cells under static conditions or subjected to shear stress. (G, I) PCR quantification of collagen IV (COLA-4) mRNA expression of normal and senescent PTM cells under static conditions or subjected to shear stress. SS stands for shear stress.
We also studied the expression and phosphorylation of ERK using Western blot analysis. For normal PTM cells, the p-ERK/total ERK ratio increased significantly after 12-h exposure to the shear stress compared with the static group. In contrast, a significant decrease in p-ERK/total ERK ratio was observed for senescent PTM cells in response to the 12-h exposure to the shear stress (Figures 5A,B). To further study the effect of senescence on the ERK expression and phosphorylation, we investigated the exposure time-dependence of normal and senescent PTM cells by considering short-term shear stress. As shown in Figures 5C,D, for normal PTM cells, the p-ERK/total ERK ratio remained roughly unchanged after exposure to the shear stress for 10 min and 30 min compared to the static group. However, the p-ERK/total ERK ratio decreased significantly to the value measured for 12 h after exposure to the shear stress even for 10 min for the case of senescent PTM cells (Figures 5C,D). These results indicated that the alteration in ERK phosphorylation in response to the shear stress occurred at a different time point in normal or senescent PTM cells.
FIGURE 5. Effect of senescence on the extracellular signal-regulated kinase (ERK) and p-ERK expression of PTM cells in response to shear stress. (A,B) Western blot analysis of the p-ERK and ERK expression of normal and senescent PTM cells under static conditions or subjected to 12-h shear stress (*p < 0.05). (C,D) Western blot analysis of the p-ERK/total ERK protein expression of normal and senescent PTM cells under static conditions or subjected to 10-min and 30-min shear stress (**p < 0.01). SS stands for shear stress.
In this study, we investigated the effects of senescence on the responses of PTM cells to the shear stress. We found for the first time that mechanotransduction of PTM cells could be altered by cell senescence. Our studies addressed the possible correlation of the senescence-induced change in cytoskeletal rearrangement, F-actin remodeling, migration, and MMP/TIMP expression in response to shear stress with the pathogenesis of POAG.
It is commonly accepted that the TMCs can remodel the cytoskeleton in response to mechanical stress such as mechanical stretch or ECM stiffness in order to moderate their capability of draining aqueous humor (Starodubtseva, 2011; Li et al., 2022). In our study, following exposure to the shear stress of 25 dyn/cm2, normal PTM cells showed clearly a cytoskeletal rearrangement, i.e., the F-actin fibers tended to realign along the direction of the flow, in sharp contrast to the random cytoskeletal arrangement for cells grown under static conditions. The cytoskeletal network’s structure is essential for transmitting force stimulation and perceiving the mechanical microenvironment cues (Janmey and Mcculloch, 2007; Du et al., 2022). It may play a crucial role in several biological functions of TMCs, such as cell contraction, cell migration, and phagocytosis (Rottner et al., 2017; Mylvaganam et al., 2021). Hence, we propose the cytoskeletal rearrangement of TMCs as an important means for responding to the shear stress and regulating the aqueous humor outflow. Existing studies have proved that the shear stress can cause cytoskeletal arrangement for several different cell types (Galbraith et al., 1998; Kadi et al., 2007; Huang et al., 2010; Risca et al., 2012; Cheng et al., 2013; Molladavoodi et al., 2017; Son et al., 2020), and the changes in cytoskeleton and cellular functions after shear stress stimulation may be mediated by FAK (Girard and Nerem, 1995; Fabry et al., 2011; Cheng et al., 2013; Sun et al., 2018), ERK pathway (Fabry et al., 2011; Sun et al., 2018), Rho pathway (Tzima et al., 2002), and transient receptor potential melastatin type 7 (TRPM7) channel (Liu et al., 2015; Xiao et al., 2015). These uncovered mechanisms may also hold for the case of TMCs. An exciting result in our study was that the shear stress-induced reorganization of the cytoskeleton was weakened for the senescent PTM cells. Existing studies indicated that the cytoskeletal arrangement of TMCs in glaucomatous eyes of elderly human is more random and disordered than that in control eyes (Read et al., 2007; Huang et al., 2022). Moreover, glaucomatous TMCs derived from POAG donors are insensitive to shear stress (Patel et al., 2021). Therefore, we postulate that the impairment of rearrangement in senescent TMCs under mechanical stimulation may partly contribute to the aging-related glaucoma pathogenesis.
We further found that the F-actin content of normal PTM cells increased under shear stress stimulation. This finding indicated that not only the cytoskeletal organization but also the formation of F-actin could be actively changed after exposure to the shear stress. It has been revealed that the TMCs showed increased stress fibers in response to mechanical stimulation such as cyclic mechanical stretch (Duffy and O’reilly, 2018). For other cell types, the F-actin formation had also been shown to correlate closely with the shear stress (Okuyama et al., 1996; Schleicher et al., 2008; Mu et al., 2015), depending on the mode and intensity of shearing (Chen et al., 2004). The dynamic regulation of F-actin polymerization of TMCs in response to the shear stress may be significant for the maintenance of outflow resistance and IOP homeostasis. Interestingly, our results showed that the shear stress stimulation led to moderate but less significant increase in F-actin content in senescent PTM cells than that in normal PTM cells. This indicated that the cell senescence impaired the capability of dynamic regulation of F-actin polymerization. It is well recognized that the F-actin is a major determinant in maintaining the cellular elastic stiffness (Fallqvist et al., 2016), and the enhancement of cell stiffness is associated with F-actin formation (Sun et al., 2017). Here, we measured the stiffness of normal and senescent PTM cells after shear stress stimulation using AFM. Our results indicated that the senescent PTM cells with more F-actin exhibited higher cell stiffness (Morgan et al., 2015) and showed a less significant increase in cell stiffness after exposure to the shear stress. Altogether, cell senescence may impair the cytoskeleton formation in TMCs after shear stress stimulation and consequently affect the regulation of cell stiffness.
The cell migration, a highly dynamic process driven by the cytoskeleton (Lin et al., 2019), was also found to be affected by the shear stress and cell senescence. More specifically, the migration of normal PTM cells increased after shear stress stimulation, while the opposite is true for the senescent PTM cells. This may be attributed to the difference in the response of cytoskeleton to the shear stress for normal and senescent cells. The relationship between the migration of TMCs and elevated IOP is not clear so far. For example, Koga et al. suggest that inhibition of migration activities might be associated with decreased aqueous outflow (Koga et al., 2006). While, Igarashi et al. suppose that inhibition of cell migration or proliferation could benefit glaucoma treatment (Igarashi et al., 2021). Although the relationship between migration of TMCs and elevated IOP remains to be established, it is widely believed that migration activities of TMCs might be associated with aqueous outflow, suggesting migration as a potential therapeutic target in treating glaucoma. We propose that normal PTM cells can sense the shear stress induced by fluid flow and accordingly moderate their functions such as migration to regulate IOP. While these responses in senescent PTMs cells are negated, which might be associated with dysregulated aqueous humor outflow and IOP.
MMPs, as a family of zinc-dependent enzymes that are involved in the ECM degradation (Clark, 1998), have been proposed to play a vital role in regulating the ECM turnover in the TM and the IOP (Alexander et al., 1991). Among those enzyme family members, TMCs are known to express MMP-1, MMP-2, and their endogenous inhibitors TIMPs, which are important for the modulation of aqueous humor outflow facility by controlling ECM turnover, cell growth, and cell migration in the TM (Sivak and Fini, 2002; Pang et al., 2003; Brew and Nagase, 2010; Ramer and Hinz, 2010). It has been demonstrated that mechanical stimulation, e.g., strain and mechanical stretch, can influence the expression of MMPs in TMCs (Wudunn, 2001; Bradley et al., 2003). In our study, we found that the expression of MMP-1 and MMP-2 in normal PTM cells increased after shear stress stimulation, which was in contrast down-regulated in senescent PTM cells. This different response to the shear stress for normal and senescent PTM cells was also observed in the cell migration as discussed above. Another finding is that the level of MMPs and TIMPs in senescent cells are higher than those in normal cells at zero stress, suggesting a higher ECM turnover rate which is believed as a pathological change in glaucomatous eyes (Camras et al., 2012; Camras et al., 2014). We further evaluated the mRNA expression of COLA-1 and COLA-4, which are vital components of the ECM in TM (Fuchshofer et al., 2007; Takahashi et al., 2014). Our results showed that cell senescence and shear stress made no difference to the COLA-1 and COLA-4 mRNA expression of PTM cells under current experimental conditions. These results together suggested that the cell senescence and shear stress altered ECM turnover by regulating MMP expression but not collagen expression. In normal cells, the increased shear stress induced by the elevated IOP (Ethier et al., 2004) led to the up-regulation of expression of MMP, which accelerates ECM degradation and helps to lower the IOP (Turturro et al., 2013; Kennedy et al., 2019). Our results suggest that cell senescence disrupted this feedback, which may eventually contribute to the development of POAG.
To illuminate the mechanism underlying the response of MMPs to the shear stress and the cell senescence, we further studied the expression and phosphorylation of ERK for normal and senescent PTM cells. Our results indicated that the ERK phosphorylation in normal PTM cells was promoted significantly after 12-h shear stress exposure, whereas remarkable suppression of ERK phosphorylation was observed in the senescent PTM cells. This response of ERK phosphorylation to the shear stress and the cell senescence was consistent with that of MMPs, suggesting that the ERK pathway might be involved in modulating MMP expression. In addition, accumulating evidence regarding shear stress-regulated ERK phosphorylation indicates that the initiation and duration of ERK phosphorylation showed cell-type- and stress-type-dependent behavior (Jo et al., 1997; Go et al., 1999; Lee D. Y. et al., 2010; Lee M. Y. et al., 2010; Fukada et al., 2017; Choi et al., 2019; Zhou et al., 2020). For example, Jo et al. found that ERK phosphorylation was up-regulated by laminar shear stress with a maximum at 5 min and a minimum at 30 min in bovine aortic endothelial cells (Jo et al., 1997). Lee et al. observed 2-fold activation of ERK in human osteoblast-like MG63 cells in response to the oscillatory shear stress, as measured from 5 min to 24 h (Lee D. Y. et al., 2010). Our results from short-term exposure to the shear stress indicated that the changes in ERK phosphorylation of the senescent PTM cells might occur at a relatively earlier time point after shearing compared to normal PTM cells.
Additionally, mechanical stress on the outflow pathways oscillates in the eye due to the fluctuation of IOP (Norouzpour and Mehdizadeh, 2012; Zou et al., 2014; Huang et al., 2015; Sherwood et al., 2019; Karimi et al., 2022). Although it is difficult to precisely measure the shear stress on TMCs with the change of IOP, it is commonly believed that the mechanotransduction properties of TM cells regulate the rhythmic IOP fluctuations and control the outflow pathway in response to rapid IOP elevations induced by stressful situations (Johnstone, 2004; Carreon et al., 2017; Turner et al., 2019). The most exciting finding in our study is that senescent PTM cells failed to respond actively to the shear stress. We believe that the senescence-induced impairment of mechanotransduction in TMCs limits the ability to modulate the pulsatile flow of the aqueous fluid while IOP fluctuates, which may eventually lead to dysregulation of IOP and glaucoma.
In conclusion, pTMCs can sense and respond to the shear stress by modifying biomechanical properties and physiological functions. However, cell senescence altered the mechanobiological response and in most cases, rendered the cells less responsive to the shear stress, which may lead to progressive failure of cellular TM function with age. Despite the importance of the mechanobiology of TMCs, our knowledge about TMCs’ behaviors in response to mechanical stress in glaucoma or aging is highly limited. This work gives us new clues to the role of senescence in regulating IOP by affecting TMC dysfunction, which would deepen our understanding of the pathophysiology of POAG.
The original contributions presented in the study are included in the article/Supplementary Material further inquiries can be directed to the corresponding authors.
JJ, LL, LZ, and YF conceived and designed this project. MZ, DL, RD, KR, and DH performed the experiments and analyzed the results. RD and MZ contributed to the figures. JJ, LL, and RD wrote the initial draft of the manuscript. YH and DL provided feedback and comments on the manuscript. All authors have approved the manuscript for publication.
This study was supported from National Natural Science Foundation of China (Grant Nos. 12232019, U20A20390, 11827803, 11902327, and 12272388), Youth Innovation Promotion Association CAS.
The authors declare that the research was conducted in the absence of any commercial or financial relationships that could be construed as a potential conflict of interest.
All claims expressed in this article are solely those of the authors and do not necessarily represent those of their affiliated organizations, or those of the publisher, the editors and the reviewers. Any product that may be evaluated in this article, or claim that may be made by its manufacturer, is not guaranteed or endorsed by the publisher.
The Supplementary Material for this article can be found online at: https://www.frontiersin.org/articles/10.3389/fcell.2022.1083130/full#supplementary-material
SUPPLEMENTARY FIGURE S1 | Morphology and myocilin (MYOC) gene expression of PTM cells. (A) Image of primary PTM cells. (B) Image of passage PTM cells. (C) Staining for fibronectin (FN) and laminin (LN) of PTM cells. (D,E) Myocilin gene expression in PTM cells after corticosteroid treatment. There was a substantial increase in myocilin expression after dexamethasone (DEX) treatment (***p < 0.001).
Alexander, J. P., and Acott, T. S. (2003). Involvement of the Erk-MAP kinase pathway in TNFalpha regulation of trabecular matrix metalloproteinases and TIMPs. Invest. Ophthalmol. Vis. Sci. 44, 164–169. doi:10.1167/iovs.01-1201
Alexander, J. P., Samples, J. R., Van Buskirk, E. M., and Acott, T. S. (1991). Expression of matrix metalloproteinases and inhibitor by human trabecular meshwork. Invest. Ophthalmol. Vis. Sci. 32, 172–180.
Allison, K., Patel, D., and Alabi, O. (2020). Epidemiology of glaucoma: The past, present, and predictions for the future. Cureus 12, e11686. doi:10.7759/cureus.11686
Alvarado, J. A., Alvarado, R. G., Yeh, R. F., Franse-Carman, L., Marcellino, G. R., and Brownstein, M. J. (2005). A new insight into the cellular regulation of aqueous outflow: How trabecular meshwork endothelial cells drive a mechanism that regulates the permeability of schlemm's canal endothelial cells. Br. J. Ophthalmol. 89, 1500–1505. doi:10.1136/bjo.2005.081307
Bourne, R. R. (2006). Worldwide glaucoma through the looking glass. Br. J. Ophthalmol. 90, 253–254. doi:10.1136/bjo.2005.083527
Bradley, J. M., Kelley, M. J., Rose, A., and Acott, T. S. (2003). Signaling pathways used in trabecular matrix metalloproteinase response to mechanical stretch. Invest. Ophthalmol. Vis. Sci. 44, 5174–5181. doi:10.1167/iovs.03-0213
Bradley, J. M., Kelley, M. J., Zhu, X., Anderssohn, A. M., Alexander, J. P., and Acott, T. S. (2001). Effects of mechanical stretching on trabecular matrix metalloproteinases. Invest. Ophthalmol. Vis. Sci. 42, 1505–1513.
Brew, K., and Nagase, H. (2010). The tissue inhibitors of metalloproteinases (TIMPs): An ancient family with structural and functional diversity. Biochim. Biophys. Acta 1803, 55–71. doi:10.1016/j.bbamcr.2010.01.003
Buffault, J., Labbé, A., Hamard, P., Brignole-Baudouin, F., Baudouin, C., and Labbe, A. (2020). The trabecular meshwork: Structure, function and clinical implications. A review of the literature. J. Fr. Ophtalmol. 43, e217–e230. doi:10.1016/j.jfo.2020.05.002
Camras, L. J., Stamer, W. D., Epstein, D., Gonzalez, P., and Yuan, F. (2014). Circumferential tensile stiffness of glaucomatous trabecular meshwork. Invest. Ophthalmol. Vis. Sci. 55, 814–823. doi:10.1167/iovs.13-13091
Camras, L. J., Stamer, W. D., Epstein, D., Gonzalez, P., and Yuan, F. (2012). Differential effects of trabecular meshwork stiffness on outflow facility in normal human and porcine eyes. Invest. Ophthalmol. Vis. Sci. 53, 5242–5250. doi:10.1167/iovs.12-9825
Carreon, T., Van Der Merwe, E., Fellman, R. L., Johnstone, M., and Bhattacharya, S. K. (2017). Aqueous outflow - a continuum from trabecular meshwork to episcleral veins. Prog. Retin. Eye Res. 57, 108–133. doi:10.1016/j.preteyeres.2016.12.004
Chen, H. Q., Tian, W., Chen, Y. S., Li, L., Raum, J., and Sung, K. L. (2004). Effect of steady and oscillatory shear stress on F-actin content and distribution in neutrophils. Biorheology 41, 655–664.
Cheng, M., Guan, X., Li, H., Cui, X., Zhang, X., Li, X., et al. (2013). Shear stress regulates late EPC differentiation via mechanosensitive molecule-mediated cytoskeletal rearrangement. PLoS One 8, e67675. doi:10.1371/journal.pone.0067675
Choi, H. Y., Yang, G. M., Dayem, A. A., Saha, S. K., Kim, K., Yoo, Y., et al. (2019). Hydrodynamic shear stress promotes epithelial-mesenchymal transition by downregulating ERK and GSK3β activities. Breast Cancer Res. 21, 6–20. doi:10.1186/s13058-018-1071-2
Clark, A. F., Brotchie, D., Read, A. T., Hellberg, P., English-Wright, S., Pang, I. H., et al. (2005). Dexamethasone alters F-actin architecture and promotes cross-linked actin network formation in human trabecular meshwork tissue. Cell Motil. Cytoskelet. 60, 83–95. doi:10.1002/cm.20049
Clark, A. F. (1998). New discoveries on the roles of matrix metalloproteinases in ocular cell biology and pathology. Invest. Ophthalmol. Vis. Sci. 39, 2514–2516.
Conley, S. M., Bruhn, R. L., Morgan, P. V., and Stamer, W. D. (2004). Selenium's effects on MMP-2 and TIMP-1 secretion by human trabecular meshwork cells. Invest. Ophthalmol. Vis. Sci. 45, 473–479. doi:10.1167/iovs.03-0767
Cook, C., and Foster, P. (2012). Epidemiology of glaucoma: what's new? Can. J. Ophthalmol. 47, 223–226. doi:10.1016/j.jcjo.2012.02.003
Ding, Y. X., Cheng, Y., Sun, Q. M., Zhang, Y. Y., You, K., Guo, Y. L., et al. (2015). Mechanical characterization of cervical squamous carcinoma cells by atomic force microscopy at nanoscale. Med. Oncol. 32, 71. doi:10.1007/s12032-015-0507-0
Du, R., Li, D., Huang, Y., Xiao, H., Xue, J., Ji, J., et al. (2022). Effect of mechanical stretching and substrate stiffness on the morphology, cytoskeleton and nuclear shape of corneal endothelial cells. Med. Nov. Technol. Devices 16, 100180. doi:10.1016/j.medntd.2022.100180
Duffy, L., and O'reilly, S. (2018). Functional implications of cross-linked actin networks in trabecular meshwork cells. Cell. Physiol. biochem. 45, 783–794. doi:10.1159/000487170
Ethier, C. R., Read, A. T., and Chan, D. (2004). Biomechanics of schlemm's canal endothelial cells: Influence on F-actin architecture. Biophys. J. 87, 2828–2837. doi:10.1529/biophysj.103.038133
Fabry, B., Klemm, A. H., Kienle, S., Schäffer, T. E., and Goldmann, W. H. (2011). Focal adhesion kinase stabilizes the cytoskeleton. Biophys. J. 101, 2131–2138. doi:10.1016/j.bpj.2011.09.043
Fallqvist, B., Fielden, M. L., Pettersson, T., Nordgren, N., Kroon, M., and Gad, A. K. B. (2016). Experimental and computational assessment of F-actin influence in regulating cellular stiffness and relaxation behaviour of fibroblasts. J. Mech. Behav. Biomed. Mat. 59, 168–184. doi:10.1016/j.jmbbm.2015.11.039
Friedman, D. S., Wolfs, R. C., O'colmain, B. J., Klein, B. E., Taylor, H. R., West, S., et al. (2004). Prevalence of open-angle glaucoma among adults in the United States. Arch. Ophthalmol. 122, 532–538. doi:10.1001/archopht.122.4.532
Fuchshofer, R., Yu, A. H., Welge-Lüssen, U., and Tamm, E. R. (2007). Bone morphogenetic protein-7 is an antagonist of transforming growth factor-beta2 in human trabecular meshwork cells. Invest. Ophthalmol. Vis. Sci. 48, 715–726. doi:10.1167/iovs.06-0226
Fukada, T., Sakajiri, H., Kuroda, M., Kioka, N., and Sugimoto, K. (2017). Fluid shear stress applied by orbital shaking induces MG-63 osteosarcoma cells to activate ERK in two phases through distinct signaling pathways. Biochem. Biophys. Rep. 9, 257–265. doi:10.1016/j.bbrep.2017.01.004
Gabelt, B. T., and Kaufman, P. L. (2005). Changes in aqueous humor dynamics with age and glaucoma. Prog. Retin. Eye Res. 24, 612–637. doi:10.1016/j.preteyeres.2004.10.003
Galbraith, C. G., Skalak, R., and Chien, S. (1998). Shear stress induces spatial reorganization of the endothelial cell cytoskeleton. Cell Motil. Cytoskelet. 40, 317–330. doi:10.1002/(SICI)1097-0169(1998)40:4<317::AID-CM1>3.0.CO;2-8
Ge, P., Navarro, I. D., Kessler, M. M., Bernier, S. G., Perl, N. R., Sarno, R., et al. (2016). The soluble guanylate cyclase stimulator IWP-953 increases conventional outflow facility in mouse eyes. Invest. Ophthalmol. Vis. Sci. 57, 1317–1326. doi:10.1167/iovs.15-18958
Gille, J. J., and Joenje, H. (1992). Cell culture models for oxidative stress: Superoxide and hydrogen peroxide versus normobaric hyperoxia. Mutat. Res. 275, 405–414. doi:10.1016/0921-8734(92)90043-o
Girard, P. R., and Nerem, R. M. (1995). Shear stress modulates endothelial cell morphology and F-actin organization through the regulation of focal adhesion-associated proteins. J. Cell. Physiol. 163, 179–193. doi:10.1002/jcp.1041630121
Go, Y. M., Patel, R. P., Maland, M. C., Park, H., Beckman, J. S., Darley-Usmar, V. M., et al. (1999). Evidence for peroxynitrite as a signaling molecule in flow-dependent activation of c-Jun NH(2)-terminal kinase. Am. J. Physiol. 277, H1647–H1653. doi:10.1152/ajpheart.1999.277.4.H1647
Gu, W., Bai, X., Ren, K., Zhao, X., Xia, S., Zhang, J., et al. (2018). Mono-fullerenols modulating cell stiffness by perturbing actin bundling. Nanoscale 10, 1750–1758. doi:10.1039/c7nr07231g
Hogg, P., Calthorpe, M., Batterbury, M., and Grierson, I. (2000). Aqueous humor stimulates the migration of human trabecular meshwork cells in vitro. Invest. Ophthalmol. Vis. Sci. 41, 1091–1098.
Huang, J., Camras, L. J., and Yuan, F. (2015). Mechanical analysis of rat trabecular meshwork. Soft Matter 11, 2857–2865. doi:10.1039/c4sm01949k
Huang, S., Yu, Y., Li, L., Li, S., and Liu, Z. (2022). Effects of ocular hypertension on cytoskeleton and stiffness of trabecular meshwork cells in rats. Appl. Sci. (Basel). 12, 7862. doi:10.3390/app12157862
Huang, Y., Chen, X., Che, J., Zhan, Q., Ji, J., and Fan, Y. (2019). Shear stress promotes arterial endothelium-oriented differentiation of mouse-induced pluripotent stem cells. Stem Cells Int. 2019, 1847098. doi:10.1155/2019/1847098
Huang, Y., Jia, X., Bai, K., Gong, X., and Fan, Y. (2010). Effect of fluid shear stress on cardiomyogenic differentiation of rat bone marrow mesenchymal stem cells. Arch. Med. Res. 41, 497–505. doi:10.1016/j.arcmed.2010.10.002
Igarashi, N., Honjo, M., and Aihara, M. (2021). mTOR inhibitors potentially reduce TGF-β2-induced fibrogenic changes in trabecular meshwork cells. Sci. Rep. 11, 14111. doi:10.1038/s41598-021-93580-3
Izzotti, A., Saccà, S. C., Longobardi, M., and Cartiglia, C. (2009). Sensitivity of ocular anterior chamber tissues to oxidative damage and its relevance to the pathogenesis of glaucoma. Invest. Ophthalmol. Vis. Sci. 50, 5251–5258. doi:10.1167/iovs.09-3871
Janmey, P. A., and Mcculloch, C. A. (2007). Cell mechanics: Integrating cell responses to mechanical stimuli. Annu. Rev. Biomed. Eng. 9, 1–34. doi:10.1146/annurev.bioeng.9.060906.151927
Jo, H., Sipos, K., Go, Y. M., Law, R., Rong, J., and Mcdonald, J. M. (1997). Differential effect of shear stress on extracellular signal-regulated kinase and N-terminal Jun kinase in endothelial cells. Gi2- and Gbeta/gamma-dependent signaling pathways. J. Biol. Chem. 272, 1395–1401. doi:10.1074/jbc.272.2.1395
Johnstone, M. A. (2004). The aqueous outflow system as a mechanical pump: Evidence from examination of tissue and aqueous movement in human and non-human primates. J. Glaucoma 13, 421–438. doi:10.1097/01.ijg.0000131757.63542.24
Kadi, A., De Isla, N., Lacolley, P., Stoltz, J. F., and Menu, P. (2007). Potential relation between cytoskeleton reorganization and e-NOS activity in sheared endothelial cells (Effect of rate and time of exposure). Clin. Hemorheol. Microcirc. 37, 131–140.
Karimi, A., Rahmati, S. M., Razaghi, R., Crawford Downs, J., Acott, T. S., Wang, R. K., et al. (2022). Biomechanics of human trabecular meshwork in healthy and glaucoma eyes via dynamic Schlemm's canal pressurization. Comput. Methods Programs Biomed. 221, 106921. doi:10.1016/j.cmpb.2022.106921
Keller, K. E., Aga, M., Bradley, J. M., Kelley, M. J., and Acott, T. S. (2009). Extracellular matrix turnover and outflow resistance. Exp. Eye Res. 88, 676–682. doi:10.1016/j.exer.2008.11.023
Keller, K. E., Bhattacharya, S. K., Borrás, T., Brunner, T. M., Chansangpetch, S., Clark, A. F., et al. (2018). Consensus recommendations for trabecular meshwork cell isolation, characterization and culture. Exp. Eye Res. 171, 164–173. doi:10.1016/j.exer.2018.03.001
Kennedy, S. M., Sheridan, C., Kearns, V. R., Bilir, E. K., Fan, X., Grierson, I., et al. (2019). Thrombospondin-2 is up-regulated by TGFβ2 and increases fibronectin expression in human trabecular meshwork cells. Exp. Eye Res. 189, 107820. doi:10.1016/j.exer.2019.107820
Khaw, P. T., Occleston, N. L., Schultz, G., Grierson, I., Sherwood, M. B., and Larkin, G. (1994). Activation and suppression of fibroblast function. Eye 8 (2), 188–195. doi:10.1038/eye.1994.44
Kim, J. W. (2016). Effect of nitric oxide on the expression of matrix metalloproteinase and its association with migration of cultured trabecular meshwork cells. Korean J. Ophthalmol. 30, 66–75. doi:10.3341/kjo.2016.30.1.66
Koga, T., Koga, T., Awai, M., Tsutsui, J., Yue, B. Y., and Tanihara, H. (2006). Rho-associated protein kinase inhibitor, Y-27632, induces alterations in adhesion, contraction and motility in cultured human trabecular meshwork cells. Exp. Eye Res. 82, 362–370. doi:10.1016/j.exer.2005.07.006
Last, J. A., Pan, T., Ding, Y., Reilly, C. M., Keller, K., Acott, T. S., et al. (2011). Elastic modulus determination of normal and glaucomatous human trabecular meshwork. Invest. Ophthalmol. Vis. Sci. 52, 2147–2152. doi:10.1167/iovs.10-6342
Lee, D. Y., Li, Y. S., Chang, S. F., Zhou, J., Ho, H. M., Chiu, J. J., et al. (2010a). Oscillatory flow-induced proliferation of osteoblast-like cells is mediated by alphavbeta3 and beta1 integrins through synergistic interactions of focal adhesion kinase and Shc with phosphatidylinositol 3-kinase and the Akt/mTOR/p70S6K pathway. J. Biol. Chem. 285, 30–42. doi:10.1074/jbc.M109.010512
Lee, M. Y., Wang, Y., and Vanhoutte, P. M. (2010b). Senescence of cultured porcine coronary arterial endothelial cells is associated with accelerated oxidative stress and activation of NFkB. J. Vasc. Res. 47, 287–298. doi:10.1159/000265563
Li, G., Luna, C., Liton, P. B., Navarro, I., Epstein, D. L., and Gonzalez, P. (2007). Sustained stress response after oxidative stress in trabecular meshwork cells. Mol. Vis. 13, 2282–2288.
Li, H., Raghunathan, V., Stamer, W. D., Ganapathy, P. S., and Herberg, S. (2022). Extracellular matrix stiffness and TGFβ2 regulate YAP/TAZ activity in human trabecular meshwork cells. Front. Cell Dev. Biol. 10, 844342. doi:10.3389/fcell.2022.844342
Li, L., and Song, F. (2020). Biomechanical research into lamina cribrosa in glaucoma. Natl. Sci. Rev. 7, 1277–1279. doi:10.1093/nsr/nwaa063
Liguori, I., Russo, G., Curcio, F., Bulli, G., Aran, L., Della-Morte, D., et al. (2018). Oxidative stress, aging, and diseases. Clin. Interv. Aging 13, 757–772. doi:10.2147/cia.S158513
Lin, S. Z., Bi, D., Li, B., and Feng, X. Q. (2019). Dynamic instability and migration modes of collective cells in channels. J. R. Soc. Interface 16, 20190258. doi:10.1098/rsif.2019.0258
Liton, P. B., Challa, P., Stinnett, S., Luna, C., Epstein, D. L., and Gonzalez, P. (2005). Cellular senescence in the glaucomatous outflow pathway. Exp. Gerontol. 40, 745–748. doi:10.1016/j.exger.2005.06.005
Liton, P. B., Gonzalez, P., and Epstein, D. L. (2009). The role of proteolytic cellular systems in trabecular meshwork homeostasis. Exp. Eye Res. 88, 724–728. doi:10.1016/j.exer.2008.11.002
Liton, P. B., Lin, Y., Luna, C., Li, G., Gonzalez, P., and Epstein, D. L. (2008). Cultured porcine trabecular meshwork cells display altered lysosomal function when subjected to chronic oxidative stress. Invest. Ophthalmol. Vis. Sci. 49, 3961–3969. doi:10.1167/iovs.08-1915
Liu, Y. S., Liu, Y. A., Huang, C. J., Yen, M. H., Tseng, C. T., Chien, S., et al. (2015). Mechanosensitive TRPM7 mediates shear stress and modulates osteogenic differentiation of mesenchymal stromal cells through Osterix pathway. Sci. Rep. 5, 16522. doi:10.1038/srep16522
Mao, W., Liu, Y., Wordinger, R. J., and Clark, A. F. (2013). A magnetic bead-based method for mouse trabecular meshwork cell isolation. Invest. Ophthalmol. Vis. Sci. 54, 3600–3606. doi:10.1167/iovs.13-12033
Mcmonnies, C. W. (2017). Glaucoma history and risk factors. J. Optom. 10, 71–78. doi:10.1016/j.optom.2016.02.003
Molladavoodi, S., Robichaud, M., Wulff, D., and Gorbet, M. (2017). Corneal epithelial cells exposed to shear stress show altered cytoskeleton and migratory behaviour. PLoS One 12, e0178981. doi:10.1371/journal.pone.0178981
Morgan, J. T., Raghunathan, V. K., Chang, Y. R., Murphy, C. J., and Russell, P. (2015). The intrinsic stiffness of human trabecular meshwork cells increases with senescence. Oncotarget 6, 15362–15374. doi:10.18632/oncotarget.3798
Mu, W., Wang, X., Zhang, X., Zhu, S., Sun, D., Ka, W., et al. (2015). Fluid shear stress upregulates E-Tmod41 via miR-23b-3p and contributes to F-Actin cytoskeleton remodeling during erythropoiesis. PLoS One 10, e0136607. doi:10.1371/journal.pone.0136607
Mylvaganam, S., Freeman, S. A., and Grinstein, S. (2021). The cytoskeleton in phagocytosis and macropinocytosis. Curr. Biol. 31, R619–r632. doi:10.1016/j.cub.2021.01.036
Norouzpour, A., and Mehdizadeh, A. (2012). A novel insight into keratoconus: Mechanical fatigue of the cornea. Med. Hypothesis Discov. Innov. Ophthalmol. 1, 14–17.
Obazawa, M., Mashima, Y., Sanuki, N., Noda, S., Kudoh, J., Shimizu, N., et al. (2004). Analysis of porcine optineurin and myocilin expression in trabecular meshwork cells and astrocytes from optic nerve head. Invest. Ophthalmol. Vis. Sci. 45, 2652–2659. doi:10.1167/iovs.03-0572
Okuyama, M., Ohta, Y., Kambayashi, J., and Monden, M. (1996). Fluid shear stress induces actin polymerization in human neutrophils. J. Cell. Biochem. 63, 432–441. doi:10.1002/(sici)1097-4644(19961215)63:4%3c432::Aid-jcb5%3e3.0.Co;2-u
Pang, I. H., Hellberg, P. E., Fleenor, D. L., Jacobson, N., and Clark, A. F. (2003). Expression of matrix metalloproteinases and their inhibitors in human trabecular meshwork cells. Invest. Ophthalmol. Vis. Sci. 44, 3485–3493. doi:10.1167/iovs.02-0756
Patel, P. D., Chen, Y. L., Kasetti, R. B., Maddineni, P., Mayhew, W., Millar, J. C., et al. (2021). Impaired TRPV4-eNOS signaling in trabecular meshwork elevates intraocular pressure in glaucoma. Proc. Natl. Acad. Sci. U. S. A. 118, e2022461118. doi:10.1073/pnas.2022461118
Pattabiraman, P. P., and Rao, P. V. (2010). Mechanistic basis of Rho GTPase-induced extracellular matrix synthesis in trabecular meshwork cells. Am. J. Physiol. Cell Physiol. 298, C749–C763. doi:10.1152/ajpcell.00317.2009
Polansky, J. R., Fauss, D. J., and Zimmerman, C. C. (2000). Regulation of TIGR/MYOC gene expression in human trabecular meshwork cells. Eye (Lond) 14, 503–514. doi:10.1038/eye.2000.137
Ramer, R., and Hinz, B. (2010). Cyclooxygenase-2 and tissue inhibitor of matrix metalloproteinases-1 confer the antimigratory effect of cannabinoids on human trabecular meshwork cells. Biochem. Pharmacol. 80, 846–857. doi:10.1016/j.bcp.2010.05.010
Rao, P. V., Deng, P., Sasaki, Y., and Epstein, D. L. (2005). Regulation of myosin light chain phosphorylation in the trabecular meshwork: Role in aqueous humour outflow facility. Exp. Eye Res. 80, 197–206. doi:10.1016/j.exer.2004.08.029
Rao, P. V., Pattabiraman, P. P., and Kopczynski, C. (2017). Role of the Rho GTPase/Rho kinase signaling pathway in pathogenesis and treatment of glaucoma: Bench to bedside research. Exp. Eye Res. 158, 23–32. doi:10.1016/j.exer.2016.08.023
Read, A. T., Chan, D. W., and Ethier, C. R. (2007). Actin structure in the outflow tract of normal and glaucomatous eyes. Exp. Eye Res. 84, 214–226. doi:10.1016/j.exer.2005.10.035
Risca, V. I., Wang, E. B., Chaudhuri, O., Chia, J. J., Geissler, P. L., and Fletcher, D. A. (2012). Actin filament curvature biases branching direction. Proc. Natl. Acad. Sci. U. S. A. 109, 2913–2918. doi:10.1073/pnas.1114292109
Rottner, K., Faix, J., Bogdan, S., Linder, S., and Kerkhoff, E. (2017). Actin assembly mechanisms at a glance. J. Cell Sci. 130, 3427–3435. doi:10.1242/jcs.206433
Saccà, S. C., Gandolfi, S., Bagnis, A., Manni, G., Damonte, G., Traverso, C. E., et al. (2016). From DNA damage to functional changes of the trabecular meshwork in aging and glaucoma. Ageing Res. Rev. 29, 26–41. doi:10.1016/j.arr.2016.05.012
Sancho, A., Vandersmissen, I., Craps, S., Luttun, A., and Groll, J. (2017). A new strategy to measure intercellular adhesion forces in mature cell-cell contacts. Sci. Rep. 7, 46152. doi:10.1038/srep46152
Saretzki, G., Feng, J., Von Zglinicki, T., and Villeponteau, B. (1998). Similar gene expression pattern in senescent and hyperoxic-treated fibroblasts. J. Gerontol. A Biol. Sci. Med. Sci. 53, B438–B442. doi:10.1093/gerona/53a.6.b438
Schleicher, M., Shepherd, B. R., Suarez, Y., Fernandez-Hernando, C., Yu, J., Pan, Y., et al. (2008). Prohibitin-1 maintains the angiogenic capacity of endothelial cells by regulating mitochondrial function and senescence. J. Cell Biol. 180, 101–112. doi:10.1083/jcb.200706072
Shearer, T., and Crosson, C. E. (2001). Activation of extracellular signal-regulated kinase in trabecular meshwork cells. Exp. Eye Res. 73, 25–35. doi:10.1006/exer.2001.1007
Sherwood, J. M., Stamer, W. D., and Overby, D. R. (2019). A model of the oscillatory mechanical forces in the conventional outflow pathway. J. R. Soc. Interface 16, 20180652. doi:10.1098/rsif.2018.0652
Sivak, J. M., and Fini, M. E. (2002). MMPs in the eye: Emerging roles for matrix metalloproteinases in ocular physiology. Prog. Retin. Eye Res. 21, 1–14. doi:10.1016/s1350-9462(01)00015-5
Snider, E., Pride, C., Patil, A., Stamer, W. D., and Ethier, C. R. (2015). Characterization of mesenchymal stem cells vs. trabecular meshwork cells. Invest. Ophthalmol. Vis. Sci. 56, 2256.
Son, K., Small, M., Sehmi, R., and Janssen, L. (2020). The eosinophil actin cytoskeleton undergoes rapid rearrangement in response to fluid shear stress. J. Leukoc. Biol. 108, 129–137. doi:10.1002/jlb.1ma0320-349rr
Stamer, W. D., and Clark, A. F. (2017). The many faces of the trabecular meshwork cell. Exp. Eye Res. 158, 112–123. doi:10.1016/j.exer.2016.07.009
Starodubtseva, M. N. (2011). Mechanical properties of cells and ageing. Ageing Res. Rev. 10, 16–25. doi:10.1016/j.arr.2009.10.005
Sun, J., Luo, Q., Liu, L., and Song, G. (2018). tRNA-derived small non-coding RNAs in human disease. Cancer Lett. 427, 1–7. doi:10.1016/j.canlet.2018.01.015
Sun, J., Luo, Q., Liu, L., Yang, X., Zhu, S., and Song, G. (2017). Salinomycin attenuates liver cancer stem cell motility by enhancing cell stiffness and increasing F-actin formation via the FAK-ERK1/2 signalling pathway. Toxicology 384, 1–10. doi:10.1016/j.tox.2017.04.006
Takahashi, E., Inoue, T., Fujimoto, T., Kojima, S., and Tanihara, H. (2014). Epithelial mesenchymal transition-like phenomenon in trabecular meshwork cells. Exp. Eye Res. 118, 72–79. doi:10.1016/j.exer.2013.11.014
Tham, Y. C., Li, X., Wong, T. Y., Quigley, H. A., Aung, T., and Cheng, C. Y. (2014). Global prevalence of glaucoma and projections of glaucoma burden through 2040: A systematic review and meta-analysis. Ophthalmology 121, 2081–2090. doi:10.1016/j.ophtha.2014.05.013
Tian, H., Li, L., and Song, F. (2017). Study on the deformations of the lamina cribrosa during glaucoma. Acta Biomater. 55, 340–348. doi:10.1016/j.actbio.2017.03.028
Turner, D. C., Edmiston, A. M., Zohner, Y. E., Byrne, K. J., Seigfreid, W. P., Girkin, C. A., et al. (2019). Transient intraocular pressure fluctuations: Source, magnitude, frequency, and associated mechanical energy. Invest. Ophthalmol. Vis. Sci. 60, 2572–2582. doi:10.1167/iovs.19-26600
Turturro, S., Sunoqrot, S., Ying, H., Hong, S., and Yue, B. (2013). Sustained release of matrix metalloproteinase-3 to trabecular meshwork cells using biodegradable PLGA microparticles. Mol. Pharm. 10, 3023–3032. doi:10.1021/mp4001052
Tzima, E., Del Pozo, M. A., Kiosses, W. B., Mohamed, S. A., Li, S., Chien, S., et al. (2002). Activation of Rac1 by shear stress in endothelial cells mediates both cytoskeletal reorganization and effects on gene expression. Embo J. 21, 6791–6800. doi:10.1093/emboj/cdf688
Vahabikashi, A., Park, C. Y., Perkumas, K., Zhang, Z., Deurloo, E. K., Wu, H., et al. (2019). Probe sensitivity to cortical versus intracellular cytoskeletal network stiffness. Biophys. J. 116, 518–529. doi:10.1016/j.bpj.2018.12.021
Vranka, J. A., and Acott, T. S. (2017). Pressure-induced expression changes in segmental flow regions of the human trabecular meshwork. Exp. Eye Res. 158, 67–72. doi:10.1016/j.exer.2016.06.009
Wang, C., Li, L., and Liu, Z. (2017). Experimental research on the relationship between the stiffness and the expressions of fibronectin proteins and adaptor proteins of rat trabecular meshwork cells. BMC Ophthalmol. 17, 268. doi:10.1186/s12886-017-0662-5
Weinreb, R. N., and Khaw, P. T. (2004). Primary open-angle glaucoma. Lancet 363, 1711–1720. doi:10.1016/s0140-6736(04)16257-0
Wiggs, J. L., and Pasquale, L. R. (2017). Genetics of glaucoma. Hum. Mol. Genet. 26, R21–r27. doi:10.1093/hmg/ddx184
Wudunn, D. (2009). Mechanobiology of trabecular meshwork cells. Exp. Eye Res. 88, 718–723. doi:10.1016/j.exer.2008.11.008
Wudunn, D. (2001). The effect of mechanical strain on matrix metalloproteinase production by bovine trabecular meshwork cells. Curr. Eye Res. 22, 394–397. doi:10.1076/ceyr.22.5.394.5500
Xiao, E., Yang, H. Q., Gan, Y. H., Duan, D. H., He, L. H., Guo, Y., et al. (2015). Brief reports: TRPM7 senses mechanical stimulation inducing osteogenesis in human bone marrow mesenchymal stem cells. Stem Cells 33, 615–621. doi:10.1002/stem.1858
Yarishkin, O., Phuong, T. T. T., Baumann, J. M., De Ieso, M. L., Vazquez-Chona, F., Rudzitis, C. N., et al. (2021). Piezo1 channels mediate trabecular meshwork mechanotransduction and promote aqueous fluid outflow. J. Physiol. 599, 571–592. doi:10.1113/jp281011
Yoshida, M., Okada, E., Mizuki, N., Kokaze, A., Sekine, Y., Onari, K., et al. (2001). Age-specific prevalence of open-angle glaucoma and its relationship to refraction among more than 60, 000 asymptomatic Japanese subjects. J. Clin. Epidemiol. 54, 1151–1158. doi:10.1016/s0895-4356(01)00388-2
Yuan, F., Schieber, A. T., Camras, L. J., Harasymowycz, P. J., Herndon, L. W., and Allingham, R. R. (2016). Mathematical modeling of outflow facility increase with trabecular meshwork bypass and schlemm canal dilation. J. Glaucoma 25, 355–364. doi:10.1097/ijg.0000000000000248
Zhao, J., Wang, S., Zhong, W., Yang, B., Sun, L., and Zheng, Y. (2016). Oxidative stress in the trabecular meshwork (Review). Int. J. Mol. Med. 38, 995–1002. doi:10.3892/ijmm.2016.2714
Zheng, L., Chen, L., Chen, Y., Gui, J., Li, Q., Huang, Y., et al. (2016). The effects of fluid shear stress on proliferation and osteogenesis of human periodontal ligament cells. J. Biomech. 49, 572–579. doi:10.1016/j.jbiomech.2016.01.034
Zhou, H., Tu, Q., Zhang, Y., Xie, H. Q., Shuai, Q. Y., Huang, X. C., et al. (2020). Shear stress improves the endothelial progenitor cell function via the CXCR7/ERK pathway axis in the coronary artery disease cases. BMC Cardiovasc. Disord. 20, 403. doi:10.1186/s12872-020-01681-0
Zhou, L., Li, Y., and Yue, B. Y. (1999). Oxidative stress affects cytoskeletal structure and cell-matrix interactions in cells from an ocular tissue: The trabecular meshwork. J. Cell. Physiol. 180, 182–189. doi:10.1002/(SICI)1097-4652(199908)180:2<182::AID-JCP6>3.0.CO;2-X
Keywords: shear stress, senescence, trabecular meshwork cell, glaucoma, mechanobiology
Citation: Du R, Li D, Zhu M, Zheng L, Ren K, Han D, Li L, Ji J and Fan Y (2022) Cell senescence alters responses of porcine trabecular meshwork cells to shear stress. Front. Cell Dev. Biol. 10:1083130. doi: 10.3389/fcell.2022.1083130
Received: 28 October 2022; Accepted: 04 November 2022;
Published: 21 November 2022.
Edited by:
Yunfeng Chen, University of Texas Medical Branch at Galveston, United StatesCopyright © 2022 Du, Li, Zhu, Zheng, Ren, Han, Li, Ji and Fan. This is an open-access article distributed under the terms of the Creative Commons Attribution License (CC BY). The use, distribution or reproduction in other forums is permitted, provided the original author(s) and the copyright owner(s) are credited and that the original publication in this journal is cited, in accordance with accepted academic practice. No use, distribution or reproduction is permitted which does not comply with these terms.
*Correspondence: Long Li, bGlsb25nQGxubS5pbWVjaC5hYy5jbg==; Jing Ji, MDk3MTRAYnVhYS5lZHUuY24=; Yubo Fan, eXVib2ZhbkBidWFhLmVkdS5jbg==
Disclaimer: All claims expressed in this article are solely those of the authors and do not necessarily represent those of their affiliated organizations, or those of the publisher, the editors and the reviewers. Any product that may be evaluated in this article or claim that may be made by its manufacturer is not guaranteed or endorsed by the publisher.
Research integrity at Frontiers
Learn more about the work of our research integrity team to safeguard the quality of each article we publish.