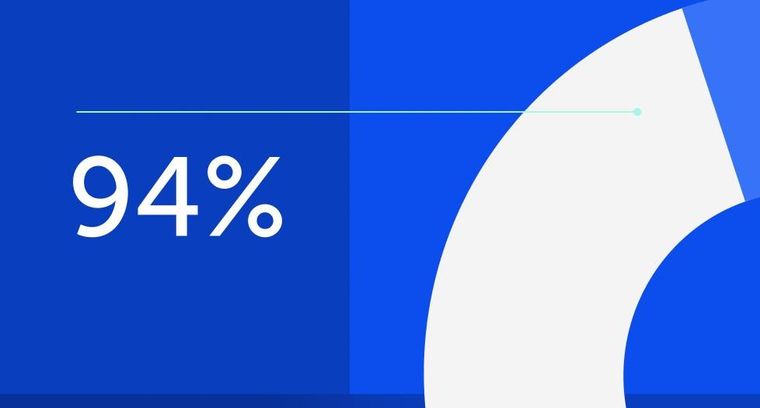
94% of researchers rate our articles as excellent or good
Learn more about the work of our research integrity team to safeguard the quality of each article we publish.
Find out more
REVIEW article
Front. Cell Dev. Biol., 23 November 2022
Sec. Cancer Cell Biology
Volume 10 - 2022 | https://doi.org/10.3389/fcell.2022.1069555
This article is part of the Research TopicTumor Metabolism: from Molecular Mechanisms to Clinical ApplicationView all 7 articles
The Warburg effect is known as the hyperactive glycolysis that provides the energy needed for rapid growth and proliferation in most tumor cells even under the condition of sufficient oxygen. This metabolic pattern can lead to a large accumulation of lactic acid and intracellular acidification, which can affect the growth of tumor cells and lead to cell death. Proton-coupled monocarboxylate transporters (MCTs) belong to the SLC16A gene family, which consists of 14 members. MCT1-4 promotes the passive transport of monocarboxylate (e.g., lactate, pyruvate, and ketone bodies) and proton transport across membranes. MCT1-4-mediated lactate shuttling between glycolytic tumor cells or cancer-associated fibroblasts and oxidative tumor cells plays an important role in the metabolic reprogramming of energy, lipids, and amino acids and maintains the survival of tumor cells. In addition, MCT-mediated lactate signaling can promote tumor angiogenesis, immune suppression and multidrug resistance, migration and metastasis, and ferroptosis resistance and autophagy, which is conducive to the development of tumor cells and avoid death. Although there are certain challenges, the study of targeted drugs against these transporters shows great promise and may form new anticancer treatment options.
Monocarboxylic acids represented by lactate, pyruvate, and ketone bodies are essential metabolites in most mammalian cells, and their dynamic absorption and redistribution are carried out through monocarboxylate transporters (Felmlee et al., 2020). There are two types of monocarboxylate transporters in the body: proton-coupled monocarboxylate transporters (MCTs) and sodium-coupled monocarboxylic monocarboxylate transporters (SMCTs). MCTs belong to the solute carrier family 16 (SLC16) or MFS superfamily, which includes 12 transmembranes (TMs) helices with intracellular N- and C-termini, a large cytosolic loops between TM6 and TM7, and two highly conserved sequences in TM1 and TM5 (Halestrap, 2012; Halestrap, 2013). Among the 14 members of the family, MCT1/SLC16A1, MCT2/SLC16A7, MCT3/SLC16A8, and MCT4/SLC16A3 convey monocarboxylate ions together with protons. These passive transporters are primarily localized at the plasma membrane, where they can operate bidirectionally depending on the concentration gradient of their substrates (Payen et al., 2020). SLC16A2 encodes the high-affinity thyroid hormone transporter (MCT8) and the SLC16A10 aromatic amino acid transporter (TAT1), while the substrates and roles of the remaining eight members are unknown (Adijanto and Philp, 2012).
Since the members of the MCTs family are themselves unglycosylated, MCT1-4 requires binding the glycosylated accessory proteins (Basigin/CD147 or embigin) to maintain their activity (Payen et al., 2020). Basigin (also known as CD147 or EMMPRIN) is a molecular chaperone that assists in MCT1, 3, and 4 localization, and gp70/embigin is a molecular chaperone in MCT2. Interactions of MCT1 with gp70 and MCT2 with CD147 have also been observed, which it maybe species dependent (Ovens et al., 2010a). The stability of MCTs and their associated chaperone proteins is interdependent, as silencing of one usually reduces the expression of the other (Marchiq et al., 2015).
Different MCTs isoforms have different affinities for the same substrate (Payen et al., 2020). MCT1 has a high affinity for lactate (3–6 mM), and the direction of lactate transport driven by MCT1 depends on the gradient of lactate and proton across the membrane and metabolic state (Van Hée et al., 2017; Halestrap and Wilson, 2012). The main physiological role of MCT1 is to transport L-lactate into cells for gluconeogenesis or oxidative phosphorylation, but MCT1 is a major lactate exporter in some normal cells under certain circumstances, including white skeletal muscle fibers, red blood cells, astrocytes, oligodendrocytes, hypoxic cells, and immune cells such as activated T lymphocytes (Halestrap, 2012). In solid tumors, the oxygen utilization and distribution lead to tumor symbiosis, where lactate secreted by tumor cells in the hypoxic zone (its concentration can be as high as 40 mM (Dhup et al., 2012) is more absorbed by perivascular tumor cells through MCT1, promoting tumor growth and proliferation (Potter et al., 2016). It has also been reported that MCT1 can promote lactate export from cancer cells (Morais-Santos et al., 2015). Both MCT2 and MCT3 have been poorly studied. MCT2 is expressed in brain, liver, and renal tubules, whereas MCT3 is in the choroid plexus and retina. MCT4 has a low affinity for lactate (25–30 mM) and does not intake serum lactate (usually <2 mM). MCT4 gives cells the ability to export lactate in a high-lactate micro-environment, which it has physiological relevance to pyruvate (Contreras-Baeza et al., 2019. Current studies have shown that MCT1, MCT2 or MCT4 are widely expressed in tumor cell lines and patients with various tumor types, and the expression of MCT subtypes or CD147 is significantly increased in most tumor cells compared with the adjacent normal epithelium (Choi et al., 2014; Pinheiro et al., 2014; De Oliveira et al., 2012). (Table 1). However, compared with normal tissue, the expression of MCT2 and MCT4 in prostate tumor cells were significantly increased, while the expression of MCT1 and CD147 significantly decreased (Pertega-Gomes et al., 2011). Although the expression of MCT4 gradually increased from non-neoplastic tissues to HCC and to metastasis, the overall expression of MCT2 gradually decreased (Alves et al., 2014). In addition, high expression of MCT1, MCT2, MCT4 or CD147 is associated with advanced tumor nodal metastasis (TNM) stage and tumor prognosis, and is a marker of poor tumor prognosis (Pertega-Gomes et al., 2011; Pinheiro et al., 2016; Payen et al., 2020). (Table 2). However, MCT2 expression suggests a better prognosis in hepatocellular carcinoma (Alves et al., 2014). MCTs play an important role in the growth, metabolism, proliferation, metastasis, and immune tolerance oftumor cells (Doherty and Cleveland, 2013; Payen et al., 2020), and more effort has been devoted to the development of MCT inhibitors as potential anti-cancer agents.
Generally speaking, most cells in the body produce adenosine triphosphate (ATP) by oxidative phosphorylation (OXPHOS) in mitochondria and complete oxidation of glucose to CO2, producing 32–38 mol of ATP per mole of glucose (Puri and Juvale, 2020). However, some cells exposed to hypoxia and proliferating cells or tumor cells show a transition from oxidative phosphorylation to anaerobic glycolysis and aerobic glycolysis, respectively, which preferentially convert glucose to lactate, producing only 2 ATPs per glucose molecule. (Vander Heiden et al., 2009). The rate of glucose metabolism through aerobic glycolysis is faster relative to OXPHOS, which is 10 to 100 times faster than the complete oxidation of glucose in mitochondria (Liberti and Locasale, 2016). Most tumor cells still provide energy by hyperactive glycolysis in the presence of sufficient oxygen, which promotes their rapid growth and proliferation, namely the Warburg effect (Vaupel et al., 2019).
Solid tumors are composed of two different types of cells. Normoxic or oxidative tumor cells are close to blood vessels and have high oxygen content around the cells. On the contrary, hypoxic cells or glycolytic tumor cells are far away from blood vessels and low oxygen content (Rockwell et al., 2009). Glycolytic tumor cells enhance the expression of glucose transporter 1 (GLUT1) to overcome ATP deficiency and accelerate glucose uptake. HIF- 1 facilitates the conversion of glucose to pyruvate and subsequently to lactate in the presence of lactate dehydrogenase 5 (LDH5) promoting NAD regeneration, which is required to maintain high glycolytic flux (Nagao et al., 2019). Lactate and hypoxia-stimulated HIF- 1α or WNT/β-catenin signaling prevent pyruvate from entering the TCA cycle and converting to acetyl-CoA by upregulating pyruvate dehydrogenase kinase 1 (PDK1) and then inhibiting pyruvate dehydrogenase complex (PDH), and drive tumor cells to obtain energy by leading to aerobic glycolysis (Kim et al., 2006; Vallee et al., 2017). In hepatocellular carcinoma, glycolysis is four times more potent than oxidative phosphorylation (Beyoğlu et al., 2013). Finally, MCT4 removes lactate from glycolytic tumor cells to avoid intracellular acidification. The low expression level of HIF- 1 and allosteric feedback inhibition of lactate in oxidized tumor cells result in low glycolysis efficiency (Leite et al., 2011). To meet energy requirements, oxidized tumor cells use MCT1 to take up lactate, which is oxidized by LDH- 1 to pyruvate, while NAD+ is reduced to NADH.
In many cases, mitochondrial oxidative phosphorylation (OXPHOS) still contributes to ATP production by tumor cells (Fu et al., 2017). Oxidized tumor cells utilize the breakdown products of glycolysis, including lactate, pyruvate, and NADH (which fuels the mitochondrial electron transport chain (ETC) via the apple-aspartic acid shuttle) to generate ATP through the tricarboxylic acid (TCA) cycle and mitochondrial OXPHOS (Ganapathy-Kanniappan and Geschwind, 2013; Van Hee et al., 2015). For oxidized tumor cells, oxidative lactate metabolism is more advantageous than aerobic glycolysis, and each molecule consumed by lactate generates 7.5 times more ATP produced (Payen et al., 2020).
The lactate shuttle mediated by MCT1 and MCT4 can connect cancer cells with glycolysis in Glycolytic tumor cells and mitochondrial oxidation in oxidized tumor cells as the main production mode, and make them form cooperative metabolism, promoting the occurrence and development of tumors (Wang et al., 2022) (Figure 1).
FIGURE 1. Model depicting MCT-mediated lactate transport in cancer: metabolic crosstalk, immunosuppression, tumor angiogenesis and anti-apoptosis. (A) MCT1-4-mediated lactate shuttling between glycolytic tumor cells and oxidized tumor cells Firstly, glycolytic tumor cells and stromal cells in the hypoxic region produce lactate and hydrogen ions by glycolysis, which are excreted into the tumor microenvironment by MCT4, and then taken up by oxidized tumor cells expressing MCT1. It is reduced to pyruvate and NANPH by LDH and enters the TCA. In addition, MCT1-mediated lactate intake can lead to the disruption of AMP/ATP balance and the inactivation of AMPK, and up-regulate the expression of SCD1 through the AMPK-SREBP1 pathway to promote the biosynthesis of MUFAs. MUFAs are substrates for the synthesis of various lipids. Finally, lactate ingestion via MCT1 stimulates glutamine uptake and catabolism by inhibiting PHD, stabilizing HIF-2α, transactivating c-Myc, and upregulating ASCT2 and GLS1 expression. (B) MCT-mediated lactate transport inhibits immune cells MCT-mediated lactate transport affects the maturation of DCS by reducing the efficiency of NF-κB binding to DNA, promotes the differentiation of peripheral blood mononuclear cells in MDSCs, and reduces the activity of NK cells. MCT-mediated lactate intake promotes the translocation of activated TNF-1 to the nucleus and up-regulates the expression of PD-1 in Tregs, but inhibits in effector T cells. PD-1 blockade activates PD-1-expressing Treg cells and inhibits effector T cells. In addition, lactate enters neutrophils through MCT1 and induces the expression of PD-L1 through NF-κB/COX-2 pathway, which reduces the cytotoxicity of T cells. (C) MCT-mediated lactate transport promotes tumor angiogenesis In oxidized tumor cells and endothelial cells, lactate is taken up via MCT1 and oxidized to pyruvate, and then inhibits PHD and catalyzes the hydroxylation of HIF-1α, which stimulates the transcription of vascular VEGF-a, VEGFR2 and bFGF. In addition, pyruvate can regulate the expression and activity of IκKβ, leading to phosphorylation and subsequent proteasome degradation of IκBα, nuclear translocation of NF-κB and transcription of the proangiogenic factor IL-8. VEGF-a, VEGFR2, bFGF and IL-8 are all pro-angiogenic factors with good characteristics.
The metabolic synergy of tumor tissue also involves the glycolysis of stromal cells, such as cancer-associated fibroblasts (CAFs), and the lactate released by MCT4 provides the raw material for the oxidative metabolism of cancer cells (Wilde et al., 2017). This symbiotic relationship between cancer cells and stromal cells is called the “reverse Warburg effect” (Benny et al., 2020).
Lactate shuttle mediated by MCT is not merely a metabolic waste product but a nutrient with multiple regulatory roles in the tumor microenvironment, and it is particularly relevant to lipid biosynthesis as a major carbon source (Faubert et al., 2017; Chen et al., 2016).
Increasing lactate concentration in the tumor microenvironment could facilitate MCT1-mediated lactate uptake on the cytoplasmic membrane, which would promote ATP production and decrease the AMP: ATP ratio in the intracellular compartment (Yan et al., 2021). The lactate-induced disruption of AMP: ATP balance would further deactivate the energy sensor AMP-activated protein kinase (AMPK), leading to upregulating the expression of the sterol regulatory element-binding protein 1 (SREBP1) and increasing the stearoyl-coenzyme A (CoA) desaturase- 1 (SCD1) expression through the AMPK- SREBP1 pathway (Li et al., 2022). It has also been reported that SCD1-catalyzed monounsaturated fatty acids (MUFAs) may replace polyunsaturated fatty acids (PUFAs) in the lipid membrane and reduce the accumulation of cytotoxic lipid ROS (Zhao et al., 2020). SCD1 promotes the biosynthesis of MUFAs (i.e., palmitoleate and oleate) from their SFA precursors (i.e., palmitate and stearate). MUFAs are substrates for the synthesis of various lipids, including phospholipids (PLs), diacylglycerol (DAGs), triacylglycerol (TAGs), and cholesteryl esters (CEs), which are essential components of biological membranes as well as cellular energy sources and signaling molecules (Ascenzi et al., 2021) (Figure 1). It is confirmed that MCT1 inhibition with AZD3965 inhibits phospholipid biosynthesis and decreases choline-phospholipid metabolism in vivo tumors (Beloueche-Babari et al., 2020).
Otherwise, MCT1 knockdown has significantly reduced the production of phospholipids containing MUFAs and down-regulated the expression of Coenzyme Q10 (CoQ10), an important enzyme in lipid metabolism and the downstream product of SCD1, while the pro-ferroptosis phosphatidylethanolamine (PE) has increased in varying degrees (Tesfay et al., 2019).
In addition, MCT1 is widely distributed in various tissues and cells. In the intestinal epithelium, its localization at both apical and basolateral membranes has been considered to promote the absorption of short-chain fatty acids (SCFAs) produced by the anaerobic intestinal bacterial fermentation of dietary fiber and resistant starch (Van Rymenant et al., 2017; Ziętek et al., 2021). SCFAs, especially acetate, propionate, and butyrate, not only provide substrates for lipid synthesis but also act as regulators to regulate lipid metabolism (He et al., 2020). MCT1 as an acetate transporter increases acetate uptake (Jeon et al., 2018). Exogenous acetate is converted to acetyl-CoA by cytosolic acetyl- CoA synthetase for lipid synthesis in acetate-dependent tumors (Lyssiotis and Cantley, 2014). Moreover, the expression of acyl-CoA synthetase 2 and fatty acid synthase, the key enzymes of controlling lipid synthesis, are elevated in the presence of acetate in HepG2 cells (Jeon et al., 2018).
Rapidly proliferating cancer cells usually show an increased dependence on amino acid metabolism (Contreras-Baeza et al., 2019). Tumor cells are involved in various crucial biochemical functions inthe brain and other tissues, such as protein synthesis and energy production, through the uptake of glutamine and the branched-chain amino acids (BCAAs) valine, leucine, and isoleucine (Jain et al., 2012). MCT1 but not MCT4 mediates the effusion of BCAAs and branched-chain ketoacids (BCKAs)-branched-chain amino acid catabolite, which may play a role in tumor immunosuppression (Silva et al., 2017).
Lactate transport across the cell membrane is mainly promoted by passive transporters of the MCT family (Halestrap and Wilson, 2012). Lactate and hydrogen ions produced by glycolytic tumor cells in the hypoxic region are excreted into the tumor microenvironment through MCT4 and then taken up by peripheral oxidized tumor cells expressing MCT1. Due to the inward gradient of lactate and protons, oxidized cancer cells are able to import lactate for signaling, whereas glycolytic cancer cells that produce and export lactate do not take up lactate and show intracellular signaling in response to exogenous lactate (De Saedeleer et al., 2012).
Lactate intake through MCT1 acts as a paracrine signaling agent that generates a pseudohypoxic response in oxidized cancer cells where it not only leads to HIF- 1α but also HIF-2α stabilization and HIF-2 activation after oxidation to pyruvate and pyruvate-mediated inhibition of proguanidin hydroxylase (PHD) (Lu et al., 2005; Perez-Escuredo et al., 2016a). It was reported that HIF-2α mediates c-Myc transactivation, which promotes glutamine uptake and metabolism through enhancing expression of the inward glutamine transporter ASCT2 and the glutamine-metabolizing enzyme glutaminase 1 (GLS1) (Perez-Escuredo et al., 2016b). (Figure 1). Furthemore, lactate-induced glutamine metabolism has been shown to activate mTOR, a key nutrient sensor and master regulator of cell growth, which stimulates protein synthesis (Allen et al., 2016).
The tumor microenvironment (TME) is the internal environment for the generation and growth of tumor cells. It includes not only tumor cells themselves, but also cells and interstitial cells closely related to tumor cells (Arneth, 2019). Poor vascular differentiation in the TME results in inefficient oxygen and nutrient delivery and metabolic waste removal, leading to nutrient limitation, low pH, hypoxia, and metabolic accumulation (Bader et al., 2020).
Rapidly proliferating tumor cells compete for relatively scarce nutrients with immune cells for anti-tumor defense, aking tumor cells themselves create an anti-immune metabolic microenvironment. Lactate produced by tumor cells is excreted by MCT and plays an immunosuppressive role in the tumor microenvironment. Lactate accumulation in the TME inhibits effector T cell function by decreasing T cell proliferation and IFN-γ production, and activates G protein-coupled receptor 81 (GPR81) on immune cells and endothelial cells, promoting angiogenesis and immune escape (Brand et al., 2016; Brown and Ganapathy, 2019). Lactate affects the maturation of dendritic cells (DCs) by reducing the efficiency of NF-κB binding to DNA and significantly promotes the differentiation of monocyte-derived DCs, which reduces glucose consumption, upregulates mitochondrial respiratory genes, and inhibits mTORC1 activity (Puig-Kroger et al., 2003). Lactate can also promote the differentiation of peripheral blood mononuclear cells in myeloid-derived suppressor cells (MDSCs), reducing the activity of NK cells, and promoting immunosuppression (Husain et al., 2013). Molecularly, Lactate can inhibit the expression of NFAT in T cells and NK cells (Puig-Kroger et al., 2003).
Activated cytotoxic T lymphocytes (CTLs) depend on glycolysis and export lactate through MCT1. However, MCT is a passive transporter whose activity is driven by the concentration of lactate and protons across the cell membrane. The accumulation of lactate in the TME prevents the efflux of lactate from these cells and impairs glycolytic activity, proliferation, and function of cytotoxic T lymphocytes and activated monocytes, thereby promoting immune resistance (Dietl et al., 2010; Fischer et al., 2007). Given the importance of MCT1 for CTL proliferation in immune responses, MCT1 inhibitors were initially developed as immunosuppressants to inhibit tissue graft rejection. Extracellular lactic acid inhibits HDACs activity, leading to histone hyperacetylation, reduced chromatin compactness, and altered gene expression, which plays an important role in promoting DNA repair and promoting chemotherapy resistance in cancer cells. Both GPR81 silencing and MCT1 inhibition can interfere with this process (Wagner et al., 2015) (Figure 1).
MCT also has an important effect on the expression of immune checkpoints such as PD- 1 on the surface of immune cells. Rapidly proliferating tumor cells enhance the expression of glucose transporter 1 (GLUT1) and accelerate glucose absorption, while regulatory T cells (Tregs) are forced to ingress lactate through MCT1, which not only maintains its growth and metabolism but also promotes the translocation of activated T nuclear factor 1 (TNF- 1) to the nucleus. Thus, the expression of PD- 1 is enhanced in Tregs but inhibited in effector T cells (Kumagai et al., 2022). PD- 1 blockade activates PD- 1-expressing Treg cells and inhibits effector T cells, including CD8+ T cells, which play a crucial role in killing cancer cells in the host, ultimately leading to treatment failure (Wherry and Kurachi, 2015). Combined treatment with MCT antibody and anti-PD- 1 can effectively inhibit tumor growth (Zhou et al., 2022).
Neutrophils are the most abundant inflammatory cells in peripheral blood. Under the stimulation of chemokines, neutrophils rapidly migrate to TME to play a role. On the one hand, reactive oxygen species (ROS), hydrogen peroxide, and tumor necrosis factor (TNF) related apoptosis-inducing ligands are released to attack tumor cells. On the other hand, neutrophils release inflammatory factors that stimulate angiogenesis, regulate tumor immunity, and promote tumor development and invasion. MCT1 and MCT4 are the major lactate receptors on neutrophils, not GPR81 (Khatib-Massalha et al., 2020). Lactate enters neutrophils through MCT1 and induces the expression of PD-L1 through NF-κB/COX-2 pathway. At the same time, lactate-H + can prolong the life span of neutrophils and partially promote the expression of PD-L1 and reduce T cell cytotoxicity (Deng et al., 2021).
There are several mechanisms identified for the development of MDR in cancer, such as loss of drug targets, increased DNA repair mechanisms, decreased uptake of the drug, and increased drug efflux due to overexpression of ATP binding cassette (ABC) transporters (Gillet and Gottesman, 2010). Apart fromthese, one of the important MDR mechanisms commonly found in solid tumors is the altered metabolism, mainly at the level of cellular glycolytic (Alfarouk et al., 2014).
Generally, solid tumors consist of two heterogeneous cell types, in which normoxic cells are close to the blood vessels and highly oxygenated, and conversely, hypoxic cells are far from the blood vessels and deficient in oxygen (Rockwell et al., 2009). Hypoxic cancer cells with enhanced expression of GLUT1 overcome ATP deficiency by accelerating glucose uptake and thereby increasing the overall ATP production. HIF- 1 also facilitates the conversion of glucose to pyruvate, later converted to lactate by a well-expressed enzyme lactate dehydrogenase (LDH) (Nagao et al., 2019). To avoid cells death caused by lactate-mediated intracellular acidification, lactate is excreted from hypoxic cells by MCT4, and subsequently, these exported lactate molecules are taken up by well-oxygenated cells via MCT1, utilizing these input lactate as an alternative fuel for energy production (Park et al., 2018). Aerobic tumor cells contain low levels of HIF- 1, resulting in inefficient glycolysis. Therefore, to meet the energy demand, these cells utilize lactate produced by hypoxic cells and oxidize it to pyruvate via LDH- 1 while reducing NAD + to NADH. The generated pyruvate and NADH enter the TCA cycle and eventually undergo OXPHOS to generate ATP.
As less oxygen is available in hypoxic regions of the tumor, they develop an alternative method, a high rate of glycolysis, to produce energy. Upon the occurrence of a hypoxic TME, instead of weakening, tumor cells adapt to hypoxic conditions, which leads to more aggressive proliferation and the development of a therapy-resistant phenotype (Rohwer and Cramer, 2011).
In the absence of other nutrients, glucose deprivation drives tumor cells to migrate towards serum and glucose, in which MCTI, MCT4, and their chaperone proteins play an important role. It has been shown that MCT1 activates the transcription factor NF-κB, which is a protein complex and gene regulator that controls cell proliferation and cell survival and promotes tumor cell metastasis (Zhao et al., 2014). For renal clear cell carcinoma, MCT1 can drive lipid desaturation through the AMPK- SREBP1-SCD1 pathway to regulate the NF-κB signaling pathway, and promote tumor cell growth and migration (Yang et al., 2018). Silencing MCT1 can inhibit the NF-κB signaling pathway and the migration and metastasis of breast cancer cells while restoring MCT1 expression restore the NF-κB activation-dependent migration of cancer cells (Vegran et al., 2011). MCT1 inhibitors such as AR-C155858 and AZD3965 effectively inhibit the activity of transporters and reduce lactate transport, but they do not inhibit the migration and invasion of cancer cells. This indicates that MCT1 activates the transcription factor NF-κB to promote cancer cell migration independently of its transporter activity (Payen et al., 2017).
Glucose deprivation, which occurs in tumors, reduces essential fuel for mitochondria and promotes oxidative stress (Ferretti et al., 2012). The survival of tumor cells depends on autophagy and the ability to resist excessive ROS production, which induces the overexpression of MCT1 and CD147 after translation in a MTROs-dependent manner (De Saedeleer et al., 2014). CD147 can trigger the migration and metastasis of cancer cells by activating matrix metalloproteinases (MMPs) (Kumar et al., 2019). Thereby, it is providing a functional complex known to be involved in the transport of monocarboxylic acids (through MCT1) and the activation of MMPs (through CD147). Upon glucose starvation, the complex promotes tumor cell migration toward serum and glucose (De Saedeleer et al., 2014).
In humans, high MCT1 and MCT4 expression are usually associated with poor prognosis (Payen et al., 2020). MCT4 knockdown can result in abnormal transport and accumulation of CD147 in lysosomes, increase focal adhesion size, upregulate epithelial markers and downregulate mesenchymal markers (Gallagher et al., 2007; Zhu et al., 2014). Loss of MCT4 activity can locally alter transmembrane pH gradient and integrin signaling pathway and cell adhesion, leading to the migration and invasion of cancer cells (Gallagher et al., 2007).
Most previous studies have relied on silencing or inhibition of MCT1/MCT4 expression or activity in highly metastatic cell lines to investigate the relationship between MCTs and metastasis. However, silencing can be transient or incomplete, and inhibition can also have off-target effects. Whether cancer cells are dependent on both MCT1 and MCT4 remains uncertain. Some studies have used the non-tumorigenic mouse NCTC clone 929 (L929) cell line to express endogenous MCT1 and MCT4 and transfect MCT1 and MCT4, respectively. The results showed that overexpression of MCT4, but not MCT1, promoted the migration and invasion of L929 cells (Li et al., 2021). MCT4 can also promote the proliferation, migration, invasion, and epithelial-mesenchymal transition (EMT) of liver cancer cells by upregulating the transport protein particle complex subunit 5 (TRAPPC5) gene (Niu et al., 2022). Furthermore, MCT4 overexpression enhances cell migration and invasiveness by reorganizing the actin cytoskeleton (Reuss et al., 2021).
In oxidized cancer cells and endothelial cells, lactate is taken up via MCT1 and oxidized to pyruvate, which competes with α-ketoglutarate to inhibit proline hydroxylases (PHDs) and catalyze the hydroxylation of HIF- 1α at two proline residues. This activates HIF- 1 and stimulates the transcription of vascular endothelial growth factor (VEGF-A), VEGF receptor 2 (VEGFR2), and basic fibroblast growth factor (bFGF) (Sonveaux et al., 2012; Payen et al., 2015). In addition, compared with the typical HIF1-dependent angiogenic factor VEGF produced by tumor cells or tumor-associated macrophages, endothelial cells also have an autocrine pathway for angiogenesis. Inhibition of PHD by lactate-derived pyruvate not only stabilizes HIF- 1α, but also regulates the expression and activity of an inhibitor of κB-kinase β (IκKβ), leading to phosphorylation of inhibitor of κBα (IκBα) and subsequent proteasomal degradation, and nuclear translocation of NF-κB and transcription of the proangiogenic factor interleukin-8 (IL-8) (Vegran et al., 2011). VEGF-A, VEGFR2, bFGF, and IL-8 are all pro-angiogenic factors with favorable properties, which activate their receptors respectively, stimulate endothelial cell proliferation and migration, and promote the generation of new blood vessels. Targeting MCT1 in endothelial cells can inhibit lactate-induced HIF- 1 activation and tumor angiogenesis (Sonveaux et al., 2012).
Lactate released from MCT4 by glycolytic tumor cells is absorbed by MCT1, thereby supporting pro-angiogenic signaling through HIF- 1α and autocrine NF-κB/IL-8 pathways (Reuss et al., 2021). At the same time, lactate is transported from tumor cells to the extracellular matrix, which increases the acidity of the extracellular environment and maintains the acidic tumor microenvironment. This transition leads to the activation of many cytokines by matrix metalloproteinases or other proteinases, such as IL-8, VEGF, and angiopoietin 2 (ANGPT2), which promote tumor neovascularization (Pouyssegur et al., 2006; Vegran et al., 2011; Xiao et al., 2020). Co-expression of MCT subtypes with VEGF family members has been demonstrated, such as the association between MCT1 and VEGF-C and between MCT4 and VEGF-A and VEGFR-3 (Pinheiro et al., 2015). In addition, MCT4 and VEGF can be regulated by HIF- 1α. Hypoxic environment is first established in cancer development and growth, then VEGF promotes angiogenesis and proliferation in the early stage of colorectal cancer development, and subsequently MCT4 promotes tumor cell growth through VEGF (Ullah et al., 2006). MCT4 inhibition can down-regulate VEGF expression in colorectal cancer cell lines, thereby inhibiting angiogenesis (Kim et al., 2018).
It is well established that PUFAs and MUFAs are the two major lipids that affect ferroptosis susceptibility in cancer cells. The ferroptosis process is driven by the PUFAs, of which the biosynthesis is catalyzed by the acyl-CoA synthetase long-chain family member 4 (ACSL4) (Das, 2019). It has also been reported that the SCD1-catalyzed MUFAs may replace PUFAs in the lipid membrane and reduce the accumulation of lipid ROS (Tesfay et al., 2019). The relative changes in the SCD1 and ACSL4 levels after MCT1-mediated lactate uptake suggest that the lactate-induced shifting in MUFAs and PUFAs production may act in concert to synergistically enhance the ferroptosis resistance in tumor cells by activating the AMPK-SREBP1-SCD1 pathway (Zhao et al., 2020). It has been reported that MCT4 can promote cell cycle progression and increase cell survival by altering cell cycle regulation and cell death mechanisms, especially late apoptosis/necrosis. At the same time, ferroptosis occurred when MCT4 was overexpressed, but not when MCT4 was expressedat baseline or not (Reuss et al., 2021).
Autophagy plays a complex dual role in the pathogenesis of tumors, which can act as an inhibitory factor or a promoting factor in the process of tumor development (White, 2015). Key proteins involved in autophagy, such as Beclin1, UVRAG, BIF- 1, and ATG, maintain cellular environment homeostasis in normal cells, prevent malignant transformation, and inhibit tumor initiation (Kriel and Loos, 2019). On the contrary, autophagy can also act as a tumor promoter by providing nutrients and energy to maintain the growth of cancer cells under hypoxic and hypotrophic conditions when tumors are formed, usually in the late stage of tumorigenesis (Schaaf et al., 2019; Mulcahy Levy and Thorburn, 2020).
Previous studies have shown that MCT1-mediated lactate uptake can activate the AMPK-SREBP1-SCD1 pathway and play a role in lipid metabolism and iron death (Zhao et al., 2020). MUFA has been reported to increase the fluidity and curvature of lipid bilayers, promote the formation of endoplasmic reticulum autophagosomes and activate autophagy. In turn, autophagy can also clear excess saturated fatty acids and damaged components, thereby counteracting cellular lipotoxicity, which is particularly important for the survival of tumor cells, especially during tumor initiation characterized by increased autophagy and up-regulation of SCD1 (Ascenzi et al., 2021). In hepatocellular carcinoma, inhibition of SCD1 can regulate autophagy by stimulating AMPK signaling (Huang et al., 2015). AMPK is an important energy signal sensor, which can phosphorylate ULK1 to form PI3K complex, promote glycolysis and induce autophagy to maintain ATP level (Chung et al., 2017; Yang et al., 2018). In the treatment of colorectal cancer (CRC), osimertinib (OSI) can up-regulate the protein level of MCT1 and subsequently induce autophagy in CRC cells through LKB1-mediated activation of AMPK, thereby antagonizing the anti-tumor effect of OSI, but the mechanism may be independent of the monocarboxylate transport function of MCT1 (Jin et al., 2019). The role of MCT1-mediated AMPK-SREBP1-SCD1 pathway in lipid metabolism and the induction of autophagy by its key molecules indicate the coupling of MCT1 in lipid metabolism and autophagy. In addition, autophagy can inhibit the phosphorylation and degradation of β-catenin by activating Wnt signaling, and the increased β-catenin is transported to the nucleus by transporters, thereby promoting the metastasis of hepatocellular carcinoma and glycolysis by increasing the transcription of MCT1 (Doherty et al., 2014).
It has also been suggested that inhibition of MCT4 can induce autophagy, thereby enhancing the cytotoxicity of NK cells and the ability to kill tumor cells (Long et al., 2018). Therefore, autophagy plays different roles according to the development stage and tissue type of cancer, which are opposite to some extent. These roles need to be fully elucidated when trying to formulate targeted therapy strategies for MCT and autophagy (Mulcahy Levy and Thorburn, 2020).
MCTs, mainly MCT1 and MCT4, are overexpressed in solid tumors, and their mediated lactate shuttling between tumor cells plays an important role in maintaining the energy and PH balance necessary for tumor cell survival. Inhibition of these lactate transporters proved to be a novel anticancer strategy (Payen et al., 2020).
At present, several MCT inhibitors have been developed, but none of them are specific to MCT subtypes. Instead, a variety of molecular targets exist (Perez-Escuredo et al., 2016a). Early MCTs inhibitors, such as phloderin, quercetin, α-cyano4-hydroxycinnamate (CHC), and 4, 4' -diisocyanate- 2, 2′-disulfonic acid (DIDS), usually have a low affinity, poor specificity, and off-target effects (Wang et al., 2021). Recently, four novel selective MCT1 inhibitors have been reported with clinical potentials, such as AR-C155858, AZD3965, BAY-8002, and 7ACC2. AZD3965 is a small-molecule drug developed by Astrazeneca and is currently in phase I clinical trials. Although AZD3965, a derivative of AR-C155858, can inhibit both MCT1 and MCT2, the inhibitory effect on MCT1 was 6 times than MCT2 (Critchlow et al., 2012). The inhibitory effect of BAY-8002 on MCT1 is 5 times that of MCT2, and there is no off-target effect on MCT4 (Quanz et al., 2018).7ACC2 inhibits lactate inflow, but does not inhibit lactate outflow from cancer cells expressing MCT1/4 (Draoui et al., 2014). This may be related to the different conformational changes of MCT1 when these inhibitors bind to MCT1. It has been reported that MCT1 exhibits an outward-open conformation when combined with BAY-8002 and AZD3965, whereas in the presence of 7ACC2, it exhibits an inward-open conformation (Wang et al., 2021) (Table 3).
The only effective small-molecule selective MCT inhibitors developed to date are for MCT1, while very few for MCT4. Some small molecules, such as AZ93 and acridine flavine, have been reported in the literature, but their role has not been determined (Marchiq and Pouyssegur, 2016; Voss et al., 2017).
When MCT1 blocks the uptake of lactate with tumor cells, oxidative tumor cells can adapt by substrate switching. In other words, they take in more glucose from neighboring vascular regions and switch from lactate-driven OXPHOS to aerobic glycolysis for survival. However, glycolytic tumor cells that rely on metabolic symbiosis cannot perform substrate switch because of glucose deprivation, leading to apoptosis (Sonveaux et al., 2008). Therefore, inhibition of MCT1 overexpression in oxidized tumor cells can indirectly kill glycolytic tumor cells in hypoxic areas, where they are resistant to conventional chemotherapy, and it is the cause of tumor recurrence (Boasquevisque et al., 2018). This provides a new idea for cancer treatment. Once the tumor cells in the hypoxic zone are eliminated, more reactive tumor cells in the normoxic zone are more easily and effectively killed by conventional chemotherapy or radiotherapy.
MCT1 inhibition can also block the uptake of lactate by oxidized cancer cells to stromal cells, impairing the promotion of cancer cell proliferation by CAFs and the angiogenesis induced by lactate on endothelial cells (Vegran et al., 2011). In addition, MCT1 blockade usually results in the termination of lactate intake and accumulation of lactate in the tumor microenvironment. Increased extracellular lactate concentration can lead to reduced pyruvate and/or lactate release from glycolytic cells, intracytoplasmic acidification, and inhibition of glycolysis (Perez-Escuredo et al., 2016b).
Knockdown of MCT1 or blockade of lactate also promoted ferroptosis, but this effect was mainly achieved by regulating intracellular lipid metabolism rather than traditional regulators of ferroptosis, such as fibroblast specific protein 1 (FSP1) and glutathione peroxidase 4 (GPX4), which was not significantly altered in response to MCT1 inhibition (Zhao et al., 2020).
Although no effective small-molecule inhibitor of MCT4 has been reported, it is attractive considering the role of MCT4 in gravity flow metabolism. Inhibition of MCT4 can lead to the accumulation of lactate and H+ and cytoplasmic acidification in glycolytic tumor cells, and ultimately significantly increase cell death (Todenhofer et al., 2018). Secondly, inhibition of MCTs, especially MCT4, can reduce the production of a large number of antioxidants in the hypoxic zone, such as glutathione or lactate, and prevent tumor cells from entering the G0 quiescent phase by affecting the AMPK pathway and the mammalian target of rapamycin (mTOR) pathway, thereby enhancing the effect of radiation therapy (Brandstetter et al., 2021).
AZD3965 is a potential selective MCT1 inhibitor. Its main mechanism of action is the specific inhibition of MCT1, which leads to the accumulation of intracellular lactic acid and reduction of intracellular PH, and feedback inhibition of glycolysis, thereby inhibiting the proliferation of tumor cells (Doherty et al., 2014). One of the challenges of AZD3965 in clinical application is its toxicity to heart and eye tissues, which can increase cardiac troponin levels and change retinal current patterns (Halford et al., 2017). Some studies have loaded AZD3965 into ultra-ph-sensitive nanoparticles to form nanopharmacology (AZD-UPS NP) (Huang et al., 2021). It remains stable at PH 7.4, but rapidly decomposes and releases AZD3965 when exposed to acidic PH, thus effectively inhibiting tumor growth. On the other hand, nanomedicine can significantly reduce the dose of oral AZD3965 and can decrease the accumulation and toxicity of the drug in the heart and liver tissues.
Another challenge with MCT1 inhibitors is that they are ineffective when MCT4 is overexpressed. This is a particularly serious defect because hypoxia leads to MCT4 expression in most tumors. In addition, MCT2 and MCT4 can overcompensate for the loss of MCT1 activity (Benjamin et al., 2018). It has been also reported that MCT4 is a “resistance factor” to MCT1 inhibitors (Polanski et al., 2014). Therefore, inhibition of both MCT1 and MCT4 is desirable.
It has been found that MCT1 inhibitor, at high concentrations of AR-C155858, does not cause cell death, but only reduces cell proliferation, even though MCT1 is the only lactate transporter (Benjamin et al., 2018). These results suggest that inhibition of lactate transport per se is not cytotoxic and that other mechanism may be involved in inducing cell lethality. Mitochondrial complex I (an NADH dehydrogenase) and lactate dehydrogenase (LDH) are the major cellular sources of NAD + regeneration required for glycolysis. In glycolytic tumor cells, LDH reduces pyruvate to lactate and simultaneously generates NAD+, which is beneficial to replenish the NAD + consumed by ATP generation in the glycolytic pathway. Inhibition of MCTs leads to intracellular lactic acid accumulation and feedback inhibition of LDH, thereby losing the ability of NAD + regeneration. In addition, metformin, an inhibitor of mitochondrial NADH dehydrogenase, can inhibit mitochondrial complex I, decrease NAD+/NADH ratio, and block glycolysis, followed by ATP production disorders and cell death (Benjamin et al., 2018). Therefore, pharmacological inhibition of MCT1 and MCT4 in combination with metformin is a potential tumor therapy.
Although AR-C155858 and AZD3965 can effectively inhibit lactate transport, their effects on cancer cell migration and invasion are very limited and cannot reduce tumor progression (Kong et al., 2016; Payen et al., 2017). Especially in HGF/C-Met metastatic tumors, such as breast and prostate cancer cells, pharmacological inhibition alone does not reduce the phosphorylation of liver growth factor (HGF) receptor c-Met and NF-κB activity and reduce the migration of cancer cells without MCT1 knockdown. Therefore, targeting MCT1 expression as well as transporter activity may be more effective than targeting transporter activity alone in anti-tumor metastasis (Gray et al., 2016).
MCTs are upregulated in most types of human tumor cells, related to tumor stage and independent prognostic markers (Payen et al., 2020). Substrates of MCT include pyruvate, l-lactate, ketone bodies aceto-acetate and d- β-hydroxybutyrate, and short-chain fatty acid propionate and butyrate, in which lactate transportplays an important role in tumor metabolism (Wang et al., 2022). MCT1/MCT4-mediated lactate shuttling closely links tumor cells to each other, tumor cells to stromal cells, and tumor cells to immune cells. In the hypoxic and rapidly proliferating tumor cells, glycolysis and lactic acid fermentation are preferentially performed to obtain energy and maintain the growth of tumor cells (Liberti and Locasale, 2016). The lactate produced by the high glycolytic activity of tumor cells is excreted out of the cells by MCT4 to avoid intracellular acidification and death. Lactate transported by MCT1 and GPR81 is oxidatively phosphorylated in cells and affects lipid and amino acid metabolism to maximize the utilization of substrates, though oxidized tumor cells can use substrates such as glucose, lactate, lipid, and glutamine for metabolism (Pérez-Escuredo et al., 2016). In addition, the signal transduction of lactate can promote angiogenesis, immune escape, multidrug resistance, migration, and metastasis, and affect ferroptosis and autophagy, so as to avoid tumor cell death.
MCTs, especially MCT1 and MCT4, are potential targets for anti-tumor treatment. However, anti- MCTs drugs still face many problems, such as whether combined inhibition or highly selective inhibition of MCT is needed, and how to prevent tumor metastasis and reduce the toxic effects of drugs. Further research is needed to develop MCT-targeted drugs.
QD and SZ drafted the manuscript and produced tables and figures. YW and DL contributed to review the literature and produced tables. YS and YW supervised the work and reviewed the final version. All authors revised and edited the manuscript, figures and tables.
This work was supported by the “Natural Science Foundation of Fujian Province, China” (No. 2019J01588) to DL which provided funds for our research.
The authors declare that the research was conducted in the absence of any commercial or financial relationships that could be construed as a potential conflict of interest.
All claims expressed in this article are solely those of the authors and do not necessarily represent those of their affiliated organizations, or those of the publisher, the editors and the reviewers. Any product that may be evaluated in this article, or claim that may be made by its manufacturer, is not guaranteed or endorsed by the publisher.
Adijanto, J., and Philp, N. J. (2012). The SLC16A family of monocarboxylate transporters (MCTs)-- physiology and function in cellular metabolism, pH homeostasis, and fluid transport. Curr. Top. Membr. 70, 275–311. doi:10.1016/B978-0-12-394316-3.00009-0
Alfarouk, K. O., Verduzco, D., Rauch, C., Muddathir, A. K., Adil, H. H., Elhassan, G. O., et al. (2014). Glycolysis, tumour metabolism, cancer growth and dissemination. A new pH-based etiopathogenic perspective and therapeutic approach to an old cancer question. Oncoscience 18, 777–802. doi:10.18632/oncoscience
Allen, E., Mieville, P., Warren, C. M., Saghafinia, S., Li, L., Peng, M. W., et al. (2016). Metabolic symbiosis enables adaptive resistance to anti-angiogenic therapy that is dependent on mTOR signaling. Cell Rep. 15, 1144–1160. doi:10.1016/j.celrep.2016.04.029
Alves, V. A., Pinheiro, C., Morais-Santos, F., Felipe-Silva, A., Longatto-Filho, A., Baltazar, F., et al. (2014). Characterization of monocarboxylate transporter activity in hepatocellular carcinoma. World J. Gastroenterol. 20, 11780–11787. doi:10.3748/wjg.v20.i33.11780
Ascenzi, F., De Vitis, C., Maugeri-Saccà, M., Napoli, C., Ciliberto, G., and Mancini, R. (2021). SCD1, autophagy and cancer: Implications for therapy. J. Exp. Clin. Cancer Res. 40, 265. doi:10.1186/s13046-021-02067-6
Bader, J. E., Voss, K., and Rathmell, J. C. (2020). Targeting metabolism to improve the tumor microenvironment for cancer immunotherapy. Mol. Cell 78, 1019–1033. doi:10.1016/j.molcel.2020.05.034
Beloueche-Babari, M., Casals Galobart ., T., Delgado-Goni, T., Wantuch, S., Parkes, H. G., Tandy, D., et al. (2020). Monocarboxylate transporter 1 blockade with AZD3965 inhibits lipid biosynthesis and increases tumour immune cell infiltration. Br. J. Cancer 122, 895–903. doi:10.1038/s41416-019-0717-x
Benjamin, D., Robay, D., Hindupur, S. K., Pohlmann, J., Colombi, M., El-Shemerly, M. Y., et al. (2018). Dual inhibition of the lactate transporters MCT1 and MCT4 is synthetic lethal with metformin due to NAD+ depletion in cancer cells. Cell Rep. 25, 3047–3058. doi:10.1016/j.celrep.2018.11.043
Benny, S., Mishra, R., Manojkumar, M. K., Aneesh, T. P., et al. (2020). From warburg effect to reverse warburg effect; the new horizons of anti-cancer therapy. Med Hypotheses 144, 110216. doi:10.1016/j.mehy.2020.110216
Beyoğlu, D., Imbeaud, S., Maurhofer, O., Bioulac-Sage, P., Zucman-Rossi, J., Dufour, J. F., et al. (2013). Tissue metabolomics of hepatocellular carcinoma: Tumor energy metabolism and the role of transcriptomic classification. Hepatology 58, 229–238. doi:10.1002/hep.26350
Boasquevisque, P. H., Schoeneberger, V., Caporiccio, L., Vellanki, R., Koritzinsky, M., Wouters, B. G., et al. (2018). Abstract 5476: Inhibiting lactate transporters MCT- 1 and MCT-4 target hypoxic HNSCC cells and sensitize them to metformin. Cancer Res. 78, 5476. doi:10.1158/1538-7445.am2018-5476
Brand, A., Singer, S., Koehl, G. E., Kolitzus, M., Schoenhammer, G., Thiel, A., et al. (2016). LDHA-associated lactic acid production blunts tumor immunosurveillance by T and NK cells. Cell Metab. 24, 657–671. doi:10.1016/j.cmet.2016.08.011
Brandstetter, G., Blatt, S., Goldschmitt, J., Taylor, L., Heymann, P., Al-Nawas, B., et al. (2021). Targeted sensitization of tumor cells for radiation through monocarboxylate transporters 1 and 4 inhibition in vitro. Clin. Oral Investig. 25, 295–310. doi:10.1007/s00784-020-03364-8
Broer, S., Broer, A., Schneider, H. P., Stegen, C., Halestrap, A. P., and Deitmer, J. W. (1999). Characterization of the high-affinity monocarboxylate transporter MCT2 in Xenopus laevis oocytes. Biochem. J. 341, 529–535. doi:10.1042/0264-6021:3410529
Brown, T. P., and Ganapathy, V. (2019). Lactate/GPR81 signaling and proton motive force in cancer: Role in angiogenesis, immune escape, nutrition, and Warburg phenomenon. Pharmacol Ther. 206, 107451. doi:10.1016/j.pharmthera.2019.107451
Chen, Y. J., Mahieu, N. G., Huang, X., Singh, M., Crawford, P. A., Johnson, S. L., et al. (2016). Lactate metabolism is associated with mammalian mitochondria. Nat. Chem. Biol. 12, 937–943. doi:10.1038/nchembio.2172
Choi, J. W., Kim, Y., Lee, J. H., and Kim, Y. S. (2014). Prognostic significance of lactate/proton symporters MCT1, MCT4, and their chaperone CD147 expressions in urothelial carcinoma of the bladder. Urology 84, e9–e15. doi:10.1016/j.urology.2014.03.031
Chung, S. J., Nagaraju, G. P., Nagalingam, A., Muniraj, N., Kuppusamy, P., Walker, A., et al. (2017). ADIPOQ/adiponectin induces cytotoxic autophagy in breast cancer cells through STK11/LKB1-mediated activation of the AMPK-ULK1 axis. Autophagy 13, 1386–1403. doi:10.1080/15548627.2017.1332565
Contreras-Baeza, Y., Sandoval, P. Y., Alarcón, R., Galaz, A., Cortés-Molina, F., Alegría, K., et al. (2019). Monocarboxylate transporter 4 (MCT4) is a high affinity transporter capable of exporting lactate in high-lactate microenvironments. J. Biol. Chem. 294, 20135–20147. doi:10.1074/jbc.RA119.009093
Critchlow, S. E., Hopcroft, L., Curtis, L. N., Whalley, N., Zhong, H., Logie, A., et al. (2012). Abstract 3224: Pre-clinical targeting of the metabolic phenotype of lymphoma by AZD3965, a selective inhibitor of monocarboxylate transporter 1 (MCT1). Cancer Res. 72, 3224. doi:10.1158/1538-7445.AM2012-3224
Curry, J. M., Tuluc, M., Whitaker-Menezes, D., Ames, J. A., Anantharaman, A., Butera, A., et al. (2013). Cancer metabolism, stemness and tumor recurrence: MCT1 and MCT4 are functional biomarkers of metabolic symbiosis in head and neck cancer. Cell Cycle 12, 1371–1384. doi:10.4161/cc.24092
Das, U. N. (2019). Saturated fatty acids, MUFAs and PUFAs regulate ferroptosis. Cell Chem. Biol. 6, 309–311. doi:10.1016/j.chembiol.2019.03.001
De Oliveira, A. T., Pinheiro, C., Longatto-Filho, A., Brito, M. J., Martinho, O., Matos, D., et al. (2012). Co-expression of monocarboxylate transporter 1 (MCT1) and its chaperone (CD147) is associated with low survival in patients with gastrointestinal stromal tumors (GISTs). J. Bioenerg. Biomembr. 44, 171–178. doi:10.1007/s10863-012-9408-5
De Saedeleer, C. J., Copetti, T., Porporato, P. E., Verrax, J., Feron, O., and Sonveaux, P. (2012). Lactate activates HIF- 1 in oxidative but not in Warburg-phenotype human tumor cells. Plos One 7, e46571. doi:10.1371/journal.pone.0046571
De Saedeleer, C. J., Porporato, P. E., Copetti, T., Perez-Escuredo, J., Payen, V. L., Brisson, L., et al. (2014). Glucose deprivation increases monocarboxylate transporter 1 (MCT1) expression and MCT1-dependent tumor cell migration. Oncogene 33, 4060–4068. doi:10.1038/onc.2013.454
Deng, H., Kan, A., Lyu, N., He, M., Huang, X., Qiao, S., et al. (2021). Tumor-derived lactate inhibit the efficacy of lenvatinib through regulating PD-L1 expression on neutrophil in hepatocellular carcinoma. J. Immunother. Cancer 9, e002305. doi:10.1136/jitc-2020-002305
Dhup, S., Dadhich, R. K., Porporato, P. E., and Sonveaux, P. (2012). Multiple biological activities of lactic acid in cancer: Influences on tumor growth, angiogenesis and metastasis. Curr. Pharm. Des. 18, 1319–1330. doi:10.2174/138161212799504902
Dietl, K., Renner, K., Dettmer, K., Timischl, B., Eberhart, K., Dorn, C., et al. (2010). Lactic acid and acidification inhibit TNF secretion and glycolysis of human monocytes. J. Immunol. 184, 1200–1209. doi:10.4049/jimmunol.0902584
Doherty, J. R., and Cleveland, J. L. (2013). Targeting lactate metabolism for cancer therapeutics. J. Clin. Invest. 123, 3685–3692. doi:10.1172/JCI69741
Doherty, J. R., Yang, C., Scott, K. E., Cameron, M. D., Fallahi, M., Li, W., et al. (2014). Blocking lactate export by inhibiting the Myc target MCT1 Disables glycolysis and glutathione synthesis. Cancer Res. 74, 908–920. doi:10.1158/0008-5472.CAN-13-2034
Draoui, N., Schicke, O., Seront, E., Bouzin, C., Sonveaux, P., Riant, O., et al. (2014). Antitumor activity of 7-aminocarboxycoumarin derivatives, a new class of potent inhibitors of lactate influx but not efflux. Mol. Cancer Ther. 13, 1410–1418. doi:10.1158/1535-7163.MCT-13-0653
Eskuri, M., Kemi, N., and Kauppila, J. H. (2021). Monocarboxylate transporters 1 and 4 and MTCO1 in gastric cancer. Cancers (Basel) 13, 2142. doi:10.3390/cancers13092142
Faubert, B., Li, K. Y., Cai, L., Hensley, C. T., Kim, J., Zacharias, L. G., et al. (2017). Lactate metabolism in human lung tumors. Cell 171, 358–371. doi:10.1016/j.cell.2017.09.019
Felmlee, M. A., Jones, R. S., Rodriguez-Cruz, V., Follman, K. E., and Morris, M. E. (2020). Monocarboxylate transporters (SLC16): Function, regulation, and role in health and disease. Pharmacol. Rev. 72, 466–485. doi:10.1124/pr.119.018762
Ferretti, A. C., Larocca, M. C., and Favre, C. (2012). Nutritional stress in eukaryotic cells: Oxidative species and regulation of survival in time of scarceness. Mol. Genet. Metab. 105, 186–192. doi:10.1016/j.ymgme.2011.11.007
Fischer, K., Hoffmann, P., Voelkl, S., Meidenbauer, N., Ammer, J., Edinger, M., et al. (2007). Inhibitory effect of tumor cell-derived lactic acid on human T cells. Blood 109, 3812–3819. doi:10.1182/blood-2006-07-035972
Fu, Y., Liu, S., Yin, S., Niu, W., Xiong, W., Tan, M., et al. (2017). The reverse Warburg effect is likely to be an Achilles' heel of cancer that can be exploited for cancer therapy. Oncotarget 8, 57813–57825. doi:10.18632/oncotarget.18175
Gallagher, S. M., Castorino, J. J., Wang, D., and Philp, N. J. (2007). Monocarboxylate transporter 4 regulates maturation and trafficking of CD147 to the plasma membrane in the metastatic breast cancer cell line MDA-MB-231. Cancer Res. 67, 4182–4189. doi:10.1158/0008-5472
Ganapathy-Kanniappan, S., and Geschwind, J. F. (2013). Tumor glycolysis as a target for cancer therapy: Progress and prospects. Mol. Cancer 12, 152. doi:10.1186/1476-4598-12-152
Gillet, J. P., and Gottesman, M. M. (2010). Mechanisms of multidrug resistance in cancer. Methods Mol. Biol. 596, 47–76. doi:10.1007/978-1-60761-416-6_4
Gray, A. L., Coleman, D. T., Shi, R., and Cardelli, J. A. (2016). Monocarboxylate transporter 1 contributes to growth factor-induced tumor cell migration independent of transporter activity. Oncotarget 7, 32695–32706. doi:10.18632/oncotarget.9016
Halestrap, A. P., and Wilson, M. C. (2012). The monocarboxylate transporter family–role and regulation. IUBMB Life 64, 109–119. doi:10.1002/iub.572
Halestrap, A. P. (2012). The monocarboxylate transporter family —Structure and functional characterization. IUBMB Life 64, 1–9. doi:10.1002/iub.573
Halestrap, A. P. (2013). The SLC16 gene family - structure, role and regulation in health and disease. Mol. Asp. Med. 34, 337–349. doi:10.1016/j.mam.2012.05.003
Halford, S. E. R., Jones, P., Wedge, S., Hirschberg, S., Katugampola, S., Veal, G., et al. (2017). A first-in-human first-in-class (FIC) trial of the monocarboxylate transporter 1 (MCT1) inhibitor AZD3965 in patients with advanced solid tumours. J. Clin.Oncol. 35, 2516. doi:10.1200/JCO.2017.35.15_suppl.2516
He, J., Zhang, P., Shen, L., Niu, L., Tan, Y., Chen, L., et al. (2020). Short-chain fatty acids and their association with signalling pathways in inflammation, glucose and lipid metabolism. Int. J. Mol. Sci. 21, 6356. doi:10.3390/ijms21176356
Huang, G. M., Jiang, Q. H., Cai, C., Qu, M., and Shen, W. (2015). SCD1 negatively regulates autophagy- induced cell death in human hepatocellular carcinoma through inactivation of the AMPK signaling pathway. Cancer Lett. 358, 180–190. doi:10.1016/j.canlet.2014.12.036
Huang, T., Feng, Q., Wang, Z., Li, W., Sun, Z., Wilhelm, J., et al. (2021). Tumor-targeted inhibition of monocarboxylate transporter 1 improves T-cell immunotherapy of solid tumors. Adv. Healthc. Mat. 10, e2000549. doi:10.1002/adhm.202000549
Husain, Z., Huang, Y., Seth, P., and Sukhatme, V. P. (2013). Tumor-derived lactate modifies antitumor immune response: Effect on myeloid-derived suppressor cells and NK cells. J. Immunol. 191, 1486–1495. doi:10.4049/jimmunol.1202702
Jain, M., Nilsson, R., Sharma, S., Madhusudhan, N., Kitami, T., Souza, A. L., et al. (2012). Metabolite profiling identifies a key role for glycine in rapid cancer cell proliferation. Science 336, 1040–1044. doi:10.1126/science.1218595
Jeon, J. Y., Lee, M., Whang, S. H., Kim, J. W., Cho, A., and Yun, M. (2018). Regulation of acetate utilization by monocarboxylate transporter 1 (MCT1) in hepatocellular carcinoma (HCC). Oncol. Res. 26, 71–81. doi:10.3727/096504017X14902648894463
Jin, P., Jiang, J., Xie, N., Zhou, L., Huang, Z., Zhang, L., et al. (2019). MCT1 relieves osimertinib- induced CRC suppression by promoting autophagy through the LKB1/AMPK signaling. Cell Death Dis. 10, 615. doi:10.1038/s41419-019-1844-2
Jonnalagadda, S., Jonnalagadda, S. K., Ronayne, C. T., Nelson, G. L., Solano, L. N., Rumbley, J., et al. (2019). Novel N, N-dialkyl cyanocinnamic acids as monocarboxylate transporter 1 and 4 inhibitors. Oncotarget 10, 2355–2368. doi:10.18632/oncotarget.26760
Khatib-Massalha, E., Bhattacharya, S., Massalha, H., Biram, A., Golan, K., Kollet, O., et al. (2020). Lactate released by inflammatory bone marrow neutrophils induces their mobilization via endothelial GPR81 signaling. Nat. Commun. 11, 3547. doi:10.1038/s41467-020-17402-2
Kim, J. W., Tchernyshyov, I., Semenza, G. L., and Dang, C. V. (2006). HIF- 1-mediated expression of pyruvate dehydrogenase kinase: A metabolic switch required for cellular adaptation to hypoxia. Cell Metab. 3, 177–185. doi:10.1016/j.cmet.2006.02.002
Kim, H. K., Lee, I., Bang, H., Kim, H. C., Lee, W. Y., Yun, S. H., et al. (2018). MCT4 expression is a potential therapeutic target in colorectal cancer with peritoneal carcinomatosis. Mol. Cancer Ther. 17, 838–848. doi:10.1158/1535-7163.MCT-17-0535
Kim, Y., Choi, J. W., Zeeberg, K., Lee, J. H., and Kim, Y. S. (2015). Expression of lactate/H+ symporters MCT1 and MCT4 and their chaperone CD147 predicts tumor progression in clear cell renal cell carcinoma: immunohistochemical and The Cancer Genome Atlas data analyses. Hum. Pathol. 46, 104–112. doi:10.1016/j.humpath.2014.09.013
Kong, S. C., Nohr-Nielsen, A., Zeeberg, K., Reshkin, S. J., Hoffmann, E. K., Novak, I., et al. (2016). Monocarboxylate transporters MCT1 and MCT4 regulate migration and invasion of pancreatic ductal adenocarcinoma cells. Pancreas 45, 1036–1047. doi:10.1097/MPA.0000000000000571
Kriel, J., and Loos, B. (2019). The good, the bad and the autophagosome: Exploring unanswered questions of autophagy-dependent cell death. Cell Death Differ. 26, 640–652. doi:10.1038/s41418-018-0267-4
Kumagai, S., Koyama, S., Itahashi, K., Tanegashima, T., Lin, Y. T., Togashi, Y., et al. (2022). Lactic acid promotes PD- 1 expression in regulatory T cells in highly glycolytic tumor microenvironments. Cancer Cell 40, 201–218.e9. doi:10.1016/j.ccell.2022.01.001
Kumar, D., Vetrivel, U., Parameswaran, S., and Subramanian, K. K. (2019). Structural insights ondruggable hotspots in CD147: A bull's eye view. Life Sci. 224, 76–87. doi:10.1016/j.lfs.2019.03.044
Latif, A., Chadwick, A. L., Kitson, S. J, Gregson, H. J., Sivalingam, V. N., Bolton, J., et al. (2017). Monocarboxylate Transporter 1 (MCT1) is an independent prognostic biomarker in endometrial cancer. BMC Clin. Pathol. 17, 27. doi:10.1186/s12907-017-0067-7
Leite, T. C., Coelho, R. G., Da Silva, D., Coelho, W. S., Marinho-Carvalho, M. M., Sola-Penna, M., et al. (2011). Lactate downregulates the glycolytic enzymes hexokinase and phosphofructokinase in diverse tissues from mice. FEBS Lett. 585, 92–98. doi:10.1016/j.febslet.2010.11.009
Li, R., Li, Y., Yang, X., Hu, Y., Yu, H., and Li, Y. (2022). Reducing VEGFB accelerates NAFLD and insulin resistance in mice via inhibiting AMPK signaling pathway. J. Transl Med. 20, 341. doi:10.1186/s12967-022-03540-2
Li, X., Zhou, X., Liu, Y., Fan, J., Huo, H., Yao, J., et al. (2021). Overexpression of monocarboxylate transporter 4 promotes the migration and invasion of non-carcinogenic L929 fibroblast cells. Oncol. Lett. 21, 44. doi:10.3892/ol.2020.12305
Liberti, M. V., and Locasale, J. W. (2016). The warburg effect: How does it benefit cancer cells?Trends. Biochem Sci. 41, 211–218. doi:10.1016/j.tibs.2015.12.001
Long, Y., Gao, Z., Hu, X., Xiang, F., Wu, Z., Zhang, J., et al. (2018). Downregulation of MCT4 for lactate exchange promotes the cytotoxicity of NK cells in breast carcinoma. Cancer Med. 7, 4690–4700. doi:10.1002/cam4.1713
Lu, H., Dalgard, C. L., Mohyeldin, A., McFate, T., Tait, A. S., and Verma, A. (2005). Reversible inactivation of HIF- 1 prolyl hydroxylases allows cell metabolism to control basal HIF- 1. J. Biol. Chem. 280, 41928–41939. doi:10.1074/jbc.M508718200
Lyssiotis, C. A., and Cantley, L. C. (2014). Acetate fuels the cancer engine. Cell 159, 1492–1494. doi:10.1016/j.cell.2014.12.009
Manning Fox, J. E., Meredith, D., and Halestrap, A. P. (2000). Molecular basis of functional voltage-gated K+ channel diversity in the mammalian myocardium. J. Physiol. 529, 285–298. doi:10.1111/j.1469-7793.2000.t01-1-00285.x
Marchiq, I., and Pouyssegur, J. (2016). Hypoxia, cancer metabolism and the therapeutic benefit of targeting lactate/H(+) symporters. J. Mol. Med. Berl. 94, 155–171. doi:10.1007/s00109-015-1307-x
Marchiq, I., Le Floch, R., Roux, D., Simon, M. P., and Pouyssegur, J. (2015). Genetic disruption of lactate/H+ symporters (MCTs) and their subunit CD147/BASIGIN sensitizes glycolytic tumor cells to phenformin. Cancer Res. 75, 171–180. doi:10.1158/0008-5472.CAN-14-2260
Miranda-Gonçalves, V., Honavar, M., Pinheiro, C., Martinho, O., Pires, M. M., Pinheiro, C., et al. (2013). Monocarboxylate transporters (MCTs) in gliomas: Expression and exploitation as therapeutic targets. Neuro. Oncol. 15, 172–188. doi:10.1093/neuonc/nos298
Morais-Santos, F., Granja, S., Miranda-Goncalves, V., Moreira, A. H., Queiros, S., Vilaca, J. L., et al. (2015). Targeting lactate transport suppresses in vivo breast tumour growth. Oncotarget 6, 19177–19189. doi:10.18632/oncotarget.3910
Mulcahy Levy, J. M., and Thorburn, A. (2020). Autophagy in cancer: Moving from understanding mechanism to improving therapy responses in patients. Cell Death Differ. 27, 843–857. doi:10.1038/s41418-019-0474-7
Nagao, A., Kobayashi, M., Koyasu, S., Chow, C. C. T., and Harada, H. (2019). HIF-1-Dependent reprogramming of glucose metabolic pathway of cancer cells and its therapeutic significance. Int. J. Mol. Sci. 20, 238. doi:10.3390/ijms20020238
Niu, Z., Yang, F., Li, H., Wang, J., Ni, Q., Ma, C., et al. (2022). MCT4 promotes hepatocellular carcinoma progression by upregulating TRAPPC5 gene. J. Hepatocell. Carcinoma 9, 289–300. doi:10.2147/JHC.S352948
Ovens, M. J., Davies, A. J., Wilson, M. C., Murray, C. M., and Halestrap, A. P. (2010a). AR-C155858 is a potent inhibitor of monocarboxylate transporters MCT1 and MCT2 that binds to an intracellular site involving transmembrane helices 7- 10. Biochem. J. 425, 523–530. doi:10.1042/BJ20091515
Ovens, M. J., Manoharan, C., Wilson, M. C., Murray, C. M., and Halestrap, A. P. (2010b). The inhibition of monocarboxylate transporter 2 (MCT2) by AR-C155858 is modulated by the associated ancillary protein. Biochem. J. 431, 217–225. doi:10.1042/BJ20100890
Park, S. J., Smith, C. P., Wilbur, R. R., Cain, C. P., Kallu, S. R., Valasapalli, S., et al. (2018). An overview of MCT1 and MCT4 in GBM: Small molecule transporters with large implications. Am. J. Cancer Res. 8, 1967–1976.
Payen, V. L., Brisson, L., Dewhirst, M. W., and Sonveaux, P. (2015). Common responses of tumors and wounds to hypoxia. Cancer J. 21, 75–87. doi:10.1097/PPO.0000000000000098
Payen, V. L., Hsu, M. Y., Rädecke, K. S., Wyart, E., Vazeille, T., Bouzin, C., et al. (2017). Monocarboxylate transporter MCT1 promotes tumor metastasis independently of its activity as a lactate transporter. Cancer Res. 77, 5591–5601. doi:10.1158/0008-5472.CAN-17-0764
Payen, V. L., Mina, E., Van Hée, V. F., Porporato, P. E., and Sonveaux, P. (2020). Monocarboxylate transporters in cancer. Mol. Metab. 33, 48–66. doi:10.1016/j.molmet.2019.07.006
Perez-Escuredo, J., Dadhich, R. K., Dhup, S., Cacace, A., Van Hee, V. F., De Saedeleer, C. J., et al. (2016a). Lactate promotes glutamine uptake and metabolism in oxidative cancer cells. Cell Cycle 15, 72–83. doi:10.1080/15384101.2015.1120930
Perez-Escuredo, J., Van Hee, V. F., Sboarina, M., Falces, J., Payen, V. L., Pellerin, L., et al. (2016b). Monocarboxylate transporters in the brain and in cancer. Biochim. Biophys. Acta 1863, 1863 2481–2497. doi:10.1016/j.bbamcr.2016.03.013
Pertega-Gomes, N., Vizcaíno, J. R., Miranda-Gonçalves, V., Pinheiro, C., Silva, J., Pereira, H., et al. (2011). Monocarboxylate transporter 4 (MCT4) and CD147 overexpression is associated with poor prognosis in prostate cancer. BMC Cancer 11, 312. doi:10.1186/1471-2407-11-312
Pinheiro, C., Longatto-Filho, A., Scapulatempo, C., Ferreira, L., Martins, S., Pellerin, L., et al. (2008). Increased expression of monocarboxylate transporters 1, 2, and 4 in colorectal carcinomas. Virchows Arch. 452, 139–146. doi:10.1007/s00428-007-0558-5
Pinheiro, C., Longatto-Filho, A., Pereira, S. M., Etlinger, D., Moreira, M. A., Jubé, L. F., et al. (2009). Monocarboxylate transporters 1 and 4 are associated with CD147 in cervical carcinoma. Dis. Markers 26, 97–103. doi:10.3233/DMA-2009-0596
Pinheiro, C., Albergaria, A., Paredes, J., Sousa, B., Dufloth, R., Vieira, D., et al. (2010). Monocarboxylate transporter 1 is up-regulated in basal-like breast carcinoma. Histopathology 56, 860–867. doi:10.1111/j.1365-2559.2010.03560.x
Pinheiro, C., Penna, V., Morais-Santos, F., Abrahão-Machado, L. F., Ribeiro, G., Curcelli, E. C., et al. (2014). Characterization of monocarboxylate transporters (MCTs) expression in soft tissue sarcomas: Distinct prognostic impact of MCT1 sub-cellular localization. J. Transl. Med. 12, 118. doi:10.1186/1479-5876-12-118
Pinheiro, C., Garcia, E. A., Morais-Santos, F., Moreira, M. A., Almeida, F. M., Jube, L. F., et al. (2015). Reprogramming energy metabolism and inducing angiogenesis: Co-expression of monocarboxylate transporters with VEGF family members in cervical adenocarcinomas. BMC Cancer 15, 835. doi:10.1186/s12885-015-1842-4
Pinheiro, C., Miranda-Gonçalves, V., Longatto-Filho, A., Vicente, A. L. S. A., Berardinelli, G. N., Scapulatempo-Neto, C., et al. (2016). The metabolic microenvironment of melanomas: Prognostic value of MCT1 and MCT4. Cell Cycle 15, 1462–1470. doi:10.1080/15384101.2016.1175258
Polanski, R., Hodgkinson, C. L., Fusi, A., Nonaka, D., Priest, L., Kelly, P., et al. (2014). Activity of the monocarboxylate transporter 1 inhibitor AZD3965 in small cell lung cancer. Clin. Cancer Res. 20, 926–937. doi:10.1158/1078-0432.CCR-13-2270
Potter, M., Newport, E., and Morten, K. J. (2016). The Warburg effect: 80 years on. Biochem. Soc. Trans. 44, 14991505–1505. doi:10.1042/BST20160094
Pouyssegur, J., Dayan, F., and Mazure, N. M. (2006). Hypoxia signalling in cancer and approaches to enforce tumour regression. Nature 441, 437–443. doi:10.1038/nature04871
Puig-Kroger, A., Pello, O. M., Selgas, R., Criado, G., Bajo, M. A., Sanchez-Tomero, J. A., et al. (2003). Peritoneal dialysis solutions inhibit the differentiation and maturation of human monocyte-derived dendritic cells: Effect of lactate and glucose-degradation products. J. Leukoc. Biol. 73, 482–492. doi:10.1189/jlb.0902451
Puri, S., and Juvale, K. (2020). Monocarboxylate transporter 1 and 4 inhibitors as potential therapeutics for treating solid tumours: A review with structure-activity relationship insights. Eur. J. Med. Chem. 199, 112393. doi:10.1016/j.ejmech.2020.112393
Quanz, M., Bender, E., Kopitz, C., Grunewald, S., Schlicker, A., Schwede, W., et al. (2018). Preclinical efficacy of the novel monocarboxylate transporter 1 inhibitor BAY-8002 andassociated markers of resistance. Mol. Cancer Ther. 17, 2285–2296. doi:10.1158/1535-7163.MCT-17-1253
Reuss, A. M., Groos, D., Ghoochani, A., Buchfelder, M., and Savaskan, N. (2021). MCT4 promotes tumor malignancy in F98 glioma cells. J. Oncol. 2021, 6655529. doi:10.1155/2021/6655529
Rockwell, S., Dobrucki, I. T., Kim, E. Y., Marrison, S. T., and Vu, V. T. (2009). Hypoxia and radiation therapy: Past history, ongoing research, and future promise. Curr. Mol. Med. 9, 442–458. doi:10.2174/156652409788167087
Rohwer, N., and Cramer, T. (2011). Hypoxia-mediated drug resistance: Novel insights on the functional interaction of HIFs and cell death pathways. Drug resist. updat. 14, 191–201. doi:10.1016/j.drup.2011.03.001
Schaaf, M. B., Houbaert, D., Meçe, O., and Agostinis, P. (2019). Autophagy in endothelial cells and tumor angiogenesis. Cell Death Differ. 26, 665–679. doi:10.1038/s41418-019-0287-8
Silva, L. S., Poschet, G., Nonnenmacher, Y., Becker, H. M., Sapcariu, S., Gaupel, A. C., et al. (2017). Branched-chain ketoacids secreted by glioblastoma cells via MCT1 modulate macrophage phenotype. EMBO Rep. 18, 2172–2185. doi:10.15252/embr.201744154
Silva, E. C. A., Carcano, F. M., Bonatelli, M., Zaia, M. G., Morais-Santos, F., Baltazar, F., et al. (2018). The clinicopathological significance of monocarboxylate transporters in testicular germ cell tumors. Oncotarget 9, 20386–20398. doi:10.18632/oncotarget.24910
Sonveaux, P., Vegran, F., Schroeder, T., Wergin, M. C., Verrax, J., Rabbani, Z. N., et al. (2008). Targeting lactate-fueled respiration selectively kills hypoxic tumor cells in mice. J. Clin. Invest. 118, 3930–3942. doi:10.1172/JCI36843
Sonveaux, P., Copetti, T., De Saedeleer, C. J., Vegran, F., Verrax, J., Kennedy, K. M., et al. (2012). Targeting the lactate transporter MCT1 in endothelial cells inhibits lactate-induced HIF- 1activation and tumor angiogenesis. Plos One 7, e33418. doi:10.1371/journal.pone.0033418
Sweeny, L., Dean, N. R., Frederick, J. W., Magnuson, J. S., Carroll, W. R., Desmond, R. A., et al. (2012). CD147 expression in advanced cutaneous squamous cell carcinoma. J. Cutan. Pathol. 39, 603–609. doi:10.1111/j.1600-0560.2012.01912.x
Tesfay, L., Paul, B. T., Konstorum, A., Deng, Z. Y., Cox, A. O., Lee, J. Y., et al. (2019). Stearoyl-CoA desaturase 1 protects ovarian cancer cells from ferroptotic cell death. Cancer Res. 79, 5355–5366. doi:10.1158/0008-5472.CAN-19-0369
Todenhofer, T., Seiler, R., Stewart, C., Moskalev, I., Gao, J., Ladhar, S., et al. (2018). Selective inhibition of the lactate transporter MCT4 reduces growth of invasive bladder cancer. Mol. Cancer Ther. 17, 2746–2755. doi:10.1158/1535-7163.MCT-18-0107
Tong, Y. H., Hu, X. P., Xiang, X. P., and Fang, L. (2021). High expression of monocarboxylate transporter 4 (MCT 4), but not MCT 1, predicts poor prognosis in patients with non-small cell lung cancer. Transl. Cancer Res. 10, 1336–1345. doi:10.21037/tcr-20-3117
Ullah, M. S., Davies, A. J., and Halestrap, A. P. (2006). The plasma membrane lactate transporter MCT4, but not MCT1, is up-regulated by hypoxia through a HIF-1alpha-dependent mechanism. J. Biol. Chem. 281, 9030–9037. doi:10.1074/jbc.M511397200
Vallee, A., Lecarpentier, Y., Guillevin, R., and Vallee, J. N. (2017). Thermodynamics in gliomas: Interactions between the canonical WNT/Beta-Catenin pathway and PPAR gamma. Front. Physiol. 8, 352. doi:10.3389/fphys.2017.00352
Van Hee, V. F., Perez-Escuredo, J., Cacace, A., Copetti, T., and Sonveaux, P. (2015). Lactate does not activate NF-κB in oxidative tumor cells. Front. Pharmacol. 6 228, 228. doi:10.3389/fphar.2015.00228
Van Hée, V. F., Labar, D., Dehon, G., Grasso, D., Grégoire, V., Muccioli, G. G., et al. (2017). Radiosynthesis and validation of (±)-[18F]-3-fluoro-2-hydroxypropionate ([18F]-FLac) as a PET tracer of lactate to monitor MCT1-dependent lactate uptake in tumors. Oncotarget 8, 24415–24428. doi:10.18632/oncotarget.14705
Van Rymenant, E., Abranko, L., Tumova, S., Grootaert, C., Van Camp, J., Williamson, G., et al. (2017). Chronic exposure to short-chain fatty acids modulates transport and metabolism of microbiome-derived phenolics in human intestinal cells. J. Nutr. Biochem. 39, 156–168. doi:10.1016/j.jnutbio.2016.09.009
Vander Heiden, M. G., Cantley, L. C., and Thompson, C. B. (2017). Understanding the Warburg effect: The metabolic requirements of cell proliferation. Science 324, 1029–1033. doi:10.1126/science.1160809
Vaupel, P., and Multhoff, G. (2021). Revisiting the Warburg effect: Historical dogma versus current understanding. J Physiol. 599, 1745–1757. doi:10.1113/JP278810
Vaupel, P., Schmidberger, H., and Mayer, A. (2019). The Warburg effect: Essential part of metabolic reprogramming and central contributor to cancer progression. Int. J. Radiat. Biol. 95, 912–919. doi:10.1080/09553002.2019.1589653
Vegran, F., Boidot, R., Michiels, C., Sonveaux, P., and Feron, O. (2011). Lactate influx through the endothelial cell monocarboxylate transporter MCT1 supports an NF-κB/IL-8 pathway that drives tumor angiogenesis. Cancer Res. 71, 2550–2560. doi:10.1158/0008-5472.CAN-10-2828
Voss, D. M., Spina, R., Carter, D. L., Lim, K. S., Jeffery, C. J., and Bar, E. E. (2017). Disruption of the monocarboxylate transporter-4-basigin interaction inhibits the hypoxic response, proliferation, and tumor progression. Sci. Rep. 7, 4292. doi:10.1038/s41598-017-04612-w
Wagner, W., Ciszewski, W. M., and Kania, K. D. (2015). L- and D-lactate enhance DNA repair and modulate the resistance of cervical carcinoma cells to anticancer drugs via histone deacetylase inhibition and hydroxycarboxylic acid receptor 1 activation. Cell Commun. Signal. 13, 36. doi:10.1186/s12964-015-0114-x
Wang, N., Jiang, X., Zhang, S., Zhu, A., Yuan, Y., Xu, H., et al. (2021). Structural basis of human monocarboxylate transporter 1 inhibition by anti-cancer drug candidates. Cell 184, 370. doi:10.1016/j.cell.2020.11.043
Wang, X., Liu, H., Ni, Y., Shen, P., and Han, X. (2022). Lactate shuttle: From substance exchange to regulatory mechanism. Hum Cell 35, 1–14. doi:10.1007/s13577-021-00622-z
Wherry, E. J., and Kurachi, M. (2015). Molecular and cellular insights into T cell exhaustion. Nat. Rev. Immunol. 15, 486–499. doi:10.1038/nri3862
White, E. (2015). The role for autophagy in cancer. J. Clin. Invest. 125, 42–46. doi:10.1172/JCI73941
Wilde, L., Roche, M., Domingo-Vidal, M., Tanson, K., Philp, N., Curry, J., et al. (2017). Metabolic coupling and the reverse warburg effect in cancer: Implications for novel biomarker and anticancer agent development. Semin Oncol. 44, 198–203. doi:10.1053/j.seminoncol.2017.10.004
Xiao, S., Zhu, H., Shi, Y., Wu, Z., Wu, H., and Xie, M. (2020). Prognostic and predictive value of monocarboxylate transporter 4 in patients with breast cancer. Oncol. Lett. 20, 2143–2152. doi:10.3892/ol.2020.11776
Yan, Y., Mukherjee, S., Harikumar, K. G., Strutzenberg, T. S., Zhou, X. E., Suino-Powell, K., et al. (2021). Structure of an AMPK complex in an inactive, ATP-bound state. Science 373, 413–419. doi:10.1126/science.abe7565
Yang, H., Zhang, X., Liu, F., Fan, J., Wang, B., and Dong, C. (2018). SREBP1-driven lipid desaturation supports clear cell renal cell carcinoma growth through regulation of NF- κB signaling. Biochem. Biophys. Res. Commun. 495, 1383–1388. doi:10.1016/j.bbrc.2017.11.163
Yuan, C., Zhang, J., Lou, J., Wang, S., Jiang, Y., Wu, F., et al. (2021). Comprehensive Analysis of Monocarboxylate Transporter 4 (MCT4) expression in breast cancer prognosis and immune infiltration via integrated bioinformatics analysis. Bioengineered 12, 3850–3863. doi:10.1080/21655979.2021.1951928
Zhao, Z., Wu, M. S., Zou, C., Tang, Q., Lu, J., Liu, D., et al. (2014). Downregulation of MCT1 inhibits tumor growth, metastasis and enhances chemotherapeutic efficacy in osteosarcoma through regulation of the NF-κB pathway. Cancer Lett. 342, 150–158. doi:10.1016/j.canlet.2013.08.042
Zhao, Y., Li, M., Yao, X., Fei, Y., LinCai, Z. K., Li, Z., et al. (2020). HCAR1/MCT1 regulates tumor ferroptosis through the lactate-mediated AMPK-SCD1 activity and its therapeutic implications. Cell Rep. 33, 108487. doi:10.1016/j.celrep.2020.108487
Zhao, H., Chen, Y., Liao, Y. P., Chen, H. M., Yang, Q. H., Xiao, Y., et al. (2022). Immunohistochemical evaluation and prognostic value of monocarboxylate transporter 1 (MCT1) and 4 (MCT4) in T-cell non-Hodgkin lymphoma. Clin. Exp. Med. doi:10.1007/s10238-022-00805-4
Zhou, J., Shao, Q., Lu, Y., Li, Y., Xu, Z., Zhou, B., et al. (2022). Monocarboxylate transporter upregulation in induced regulatory T cells promotes resistance to anti-PD- 1 therapy in hepatocellular carcinoma patients. Front. Oncol. 12, 960066. doi:10.3389/fonc.2022.960066
Zhu, J., Wu, Y. N., Zhang, W., Zhang, X. M., Ding, X., Li, H. Q., et al. (2014). Monocarboxylate transporter 4 facilitates cell proliferation and migration and is associated with poor prognosis in oral squamous cell carcinoma patients. Plos One 9, e87904. doi:10.1371/journal.pone.0087904
Keywords: monocarboxylate transporters, lactate, tumor metabolism, metastasis, angiogenesis, tumor microenvironment, autophagy, MCT inhibitors
Citation: Duan Q, Zhang S, Wang Y, Lu D, Sun Y and Wu Y (2022) Proton-coupled monocarboxylate transporters in cancer: From metabolic crosstalk, immunosuppression and anti-apoptosis to clinical applications. Front. Cell Dev. Biol. 10:1069555. doi: 10.3389/fcell.2022.1069555
Received: 14 October 2022; Accepted: 02 November 2022;
Published: 23 November 2022.
Edited by:
Chunming Cheng, The Ohio State University, United StatesReviewed by:
Rajesh Kumar Gandhirajan, Sri Ramachandra Institute of Higher Education and Research, IndiaCopyright © 2022 Duan, Zhang, Wang, Lu, Sun and Wu. This is an open-access article distributed under the terms of the Creative Commons Attribution License (CC BY). The use, distribution or reproduction in other forums is permitted, provided the original author(s) and the copyright owner(s) are credited and that the original publication in this journal is cited, in accordance with accepted academic practice. No use, distribution or reproduction is permitted which does not comply with these terms.
*Correspondence: Yongyang Wu, d3V5eWZqQGZqbXUuZWR1LmNu; Yingming Sun, eWluZ21pbmdzdW5AZmptdS5lZHUuY24=
†These authors have contributed equally to this work and share first authorship
Disclaimer: All claims expressed in this article are solely those of the authors and do not necessarily represent those of their affiliated organizations, or those of the publisher, the editors and the reviewers. Any product that may be evaluated in this article or claim that may be made by its manufacturer is not guaranteed or endorsed by the publisher.
Research integrity at Frontiers
Learn more about the work of our research integrity team to safeguard the quality of each article we publish.