- 1Département de Pharmacologie-Physiologie, Faculté de Médecine et des Sciences de la Santé, Institut de Pharmacologie de Sherbrooke, Centre de Recherche du Centre Hospitalier Universitaire de Sherbrooke, Université de Sherbrooke, Sherbrooke, QC, Canada
- 2Division of Biological Sciences Christopher S. Bond Life Sciences Center, University of Missouri, Columbia, MO, United States
The extracellular matrix (ECM) is an interconnected macromolecular scaffold occupying the space between cells. Amongst other functions, the ECM provides structural support to tissues and serves as a microenvironmental niche that conveys regulatory signals to cells. Cell-matrix adhesions, which link the ECM to the cytoskeleton, are dynamic multi-protein complexes containing surface receptors and intracellular effectors that control various downstream pathways. In skeletal muscle, the most abundant tissue of the body, each individual muscle fiber and its associated muscle stem cells (MuSCs) are surrounded by a layer of ECM referred to as the basal lamina. The core scaffold of the basal lamina consists of self-assembling polymeric laminins and a network of collagens that tether proteoglycans, which provide lateral crosslinking, establish collateral associations with cell surface receptors, and serve as a sink and reservoir for growth factors. Skeletal muscle also contains the fibrillar collagenous interstitial ECM that plays an important role in determining tissue elasticity, connects the basal laminae to each other, and contains matrix secreting mesenchymal fibroblast-like cell types and blood vessels. During skeletal muscle regeneration fibroblast-like cell populations expand and contribute to the transitional fibronectin-rich regenerative matrix that instructs angiogenesis and MuSC function. Here, we provide a comprehensive overview of the role of the skeletal muscle ECM in health and disease and outline its role in orchestrating tissue regeneration and MuSC function.
Introduction
Multicellularity is thought to have emerged independently over 25 times during the history of life on Earth (Grosberg and Strathmann, 2007). When compared to single-celled organisms, multicellularity has the advantage that cells can specialize and come together to form tissues and organs that have distinct functions. Animals (metazoans) that have developed an astonishing variety of body plans are amongst the most complex organisms in the phylogenetic tree of life. The development of an extracellular matrix (ECM), the non-cellular element of all tissues, was essential in enabling the rapid evolution of the metazoan phyla (Hynes, 2012). ECM consists of a sophisticated and highly organized network of macromolecules occupying the space between cells. The wide range of biological functions of the ECM includes providing structure to cells and tissues, serving as an adhesion substrate, facilitating the communication of neighboring cells, transmitting mechanical signals, regulating cellular growth, and promoting or restricting cell movement. The critical importance of ECM for metazoans is illustrated by the fact that heterotrimeric laminins are already synthesized by 16-cell embryos, while collagen IV is detectable in the inner cell mass of the blastocyst (Leivo et al., 1980; Cooper and MacQueen, 1983). In its basic structure, the majority of ECM is composed of two main classes of biomolecules: glycosaminoglycan (GAGs) polysaccharide chains, which are typically linked to protein in the form of proteoglycans, and proteinaceous ECM components such as collagen, fibronectin, and laminin. Most ECM proteins are composed of multiple, often repeated domains, some of which are highly evolutionarily conserved.
In metazoans, the ECM of the basal lamina is defined by its specialized, flat laminar structure and anatomic location at the interface of parenchymal cells with the extracellular space. The basal lamina, which depending on the tissue is between 40 and 120 nm thick, includes a core network of self-assembling laminins that can form continuous sheets intertwined with a network of collagen and interconnected by linker molecules such as the proteoglycans nidogen and perlecan (Aumailley et al., 1993; Hynes, 2012). The interstitial matrix occupies the extracellular space adjacent to the pericellular basal lamina. The majority of interstitial ECM is made up of proteoglycans, which due to negatively charged sulfates in their glycosaminoglycan chains are typically highly hydrated (Frantz et al., 2010). In contrast to the network collagens found in the basal lamina, the interstitial matrix contains fibrillar collagen, which binds to proteoglycans to form a sturdy gel. Depending on the tissue, the interstitial ECM also contains fibronectin, a high-molecular weight glycoprotein with a wide spectrum of functions ranging from structural organization to cell surface receptor interactions (Singh et al., 2010).
Next to its role during development, the ECM has key functions for tissue regeneration in adult organisms. This includes both regular cellular renewal during tissue homeostasis and reparative regeneration after injury. Several metazoan phyla have a remarkable regenerative capacity that in some cases persists throughout life. For instance, urodele amphibians can regenerate entire limbs through an epimorphic regeneration process mediated by a blastema formed either from dedifferentiation or tissue-resident stem cells involving a unique pro-regenerative ECM (Sandoval-Guzman et al., 2014; Seifert and Muneoka, 2018). In adult mammals, the regenerative capacity of tissues ranges from absent to very low in the central nervous system to highly efficient in the liver, the latter of which can regrow to its original size after losing up to 70% of its original volume within 1 week in rodents (Zhao et al., 2016). By providing interaction sites and through its physical properties, the ECM presents instructive signals to cells during adult tissue regeneration, while its ability to sequester growth factors and generate concentration gradients also has indirect effects on this process (Hynes, 2009).
Many tissues in vertebrates contain adult stem cells that are involved in physiological regeneration, maintaining homeostasis as well as in reparative regeneration following injury or loss of body parts. Arguably, the most prominent example is hematopoietic stem cells that can self-maintain while producing a wide spectrum of circulating cells (Clevers and Watt, 2018). Other examples include stem cells in the skin, certain brain structures, the intestine, and skeletal muscle. While all cell types in vertebrates are exposed to some form of ECM, adult stem cell niches often contain a highly specialized instructive structural microenvironment (Gattazzo et al., 2014). ECM niches involve both autoregulatory matrix that is secreted by stem cells into their own microenvironment and molecules produced by supportive accessory cell types. Here, we discuss the ECM in the stem cell niche in the context of skeletal muscle which, since the first description of a putative myogenic progenitor cell residing on the surface of muscle fibers by electron microscopy in 1961, has become one of the most studied paradigms of adult tissue regeneration (Katz, 1961; Mauro, 1961; Scharner and Zammit, 2011).
Skeletal muscle stem cells
Without considering “connective tissue”, an umbrella term used for ECM spaces and their associated fibroblast-like cells, skeletal muscle represents the most abundant tissue in vertebrates. Adult skeletal muscle contains a population of stem cells that are directly associated with muscle fibers referred to as “satellite cells” or “muscle stem cells” (MuSCs). Under homeostatic conditions adult MuSCs have exited the cell cycle and reside in a quiescent state under the ECM of the basal lamina that surrounds each individual muscle fiber (Mashinchian et al., 2018; Ancel et al., 2021). Following damage to muscle fibers, MuSCs can activate, enter the cell cycle and divide. While a fraction of MuSCs self-renews and maintains its stem cell characteristics, the bulk of cells progresses through the myogenic program and ultimately turns into myocytes that align and fuse to form new muscle fibers replacing the lost cells.
In everyday life, skeletal muscle tissue can be injured by blunt or penetrating traumatic events, for instance following road traffic, workplace, and sports accidents or because of lacerations during surgical interventions. Damage to muscle fibers leads to hypercontraction, necrosis, and subsequent removal of cellular debris by immune cells. In most types of skeletal muscle injury, the majority of ECM initially remains in the wound and MuSCs can migrate along the remaining scaffold to fuse and form new muscle fibers for tissue repair (Garg et al., 2015; Webster et al., 2016). Owing to MuSCs, skeletal muscle has the striking ability to go through multiple rounds of injury and repair without significant loss of functionality. However, efficient adult skeletal muscle regeneration in mammals depends on instructive signals from the existing extracellular matrix (Singhal and Martin, 2011; Webster et al., 2016). If large portions of a skeletal muscle including the ECM are lost, the tissue fails to regenerate efficiently. A well-known example of this kind of injury is volumetric muscle loss (VML) occurring after excision of large portions of skeletal muscle, for instance as a consequence of explosions on the battlefield (Testa et al., 2021). In case of VML the missing parts of damaged muscles are partially replaced by fibrotic scar tissue and revascularization and reinnervation of the small number of disoriented fibers that are forming at the injury site is inefficient. Thus, although extensive remodeling of the ECM takes place during skeletal muscle regeneration, at least in the early stages an existing ECM template is required for efficient MuSC function.
Snake venoms such as cardiotoxin or notexin, a frequently used experimental paradigm for skeletal muscle regeneration, lead to a type of myotoxic injury that induces hypercontraction and degeneration of muscle fibers but is thought to leave ECM and mono-nuclear cell types including MuSCs largely intact (Hardy et al., 2016). Thus, this type of injury induces highly coordinated and efficient skeletal muscle regeneration with MuSCs reaching a peak in proliferation around 3–5 days post injury (Fukada et al., 2022). During the first few days after snake venom injury remnants of the original basal laminae are still present in the tissue, while at later stages newly formed fibers of small diameter and their associated proliferating MuSCs are surrounded by proportionately sized basal laminae containing specialized pro-regenerative ECM molecules (Bentzinger et al., 2013). The latter observation suggests that de novo ECM synthesis and remodeling of the basal lamina, as well as removal of the residual scaffold during the regenerative time-course are highly coordinated processes critical to efficient skeletal muscle regeneration (Yoshimoto et al., 2020). While the cellular sources and mechanisms driving remodeling of the pro-regenerative ECM after myotoxic injury remain only partially understood, evidence points towards immune cells and fibroblast-like cell populations as major contributors to these dynamic processes. MuSCs integrate paracrine and autocrine ECM signals through several different transmembrane proteins that, together with the coordinated activation of cell-cell and growth factor receptors, instruct all stages of adult myogenesis.
Transmembrane extracellular matrix components and receptors in muscle stem cells
The basal lamina of skeletal muscle, which surrounds the muscle fiber plasma membrane and sublaminar MuSCs, is composed of a scaffold of heterotrimeric laminins and an interlaced network of collagen IV (Figure 1). Laminins that bind to integrins and dystroglycan are the predominant macromolecular polymeric ECM component expected to interface directly with MuSCs. Several other ECM components in the basal lamina can bind to cell surface receptors. This includes the non-neuronal isoform of agrin, which links the laminin network to both integrins and dystroglycan (Bezakova and Ruegg, 2003). The heparan sulfate proteoglycan perlecan, a large multidomain protein, connects the collagen IV and laminin networks to dystroglycan (Talts et al., 1999). Biglycan, a small leucine-rich proteoglycan has a role in tethering dystroglycan and some of its associated sarcoglycans to collagens (Bowe et al., 2000; Rafii et al., 2006). The basal lamina is laterally stabilized by proteins such as the nidogens that have multiple interaction partners including laminin, collagen IV, and perlecan (Zhou et al., 2022). Although nidogens can also bind to integrins and promote cell spreading, evidence for direct cell surface receptor interactions in skeletal muscle remains limited (Dong et al., 1995; Wu et al., 1995). Under homeostatic conditions, the basal lamina spatially separates MuSCs from interstitial ECM components. However, the example of the interstitial collagens I and VI that are linked to each other and the C-terminus of collagen IV in the basal lamina through the fibril growth promoting proteoglycan decorin, illustrates that all ECM layers in skeletal muscle are intimately interconnected and spatially distant structures may have indirect effects on MuSC function (Danielson et al., 1997; Kuo et al., 1997; Nareyeck et al., 2004).
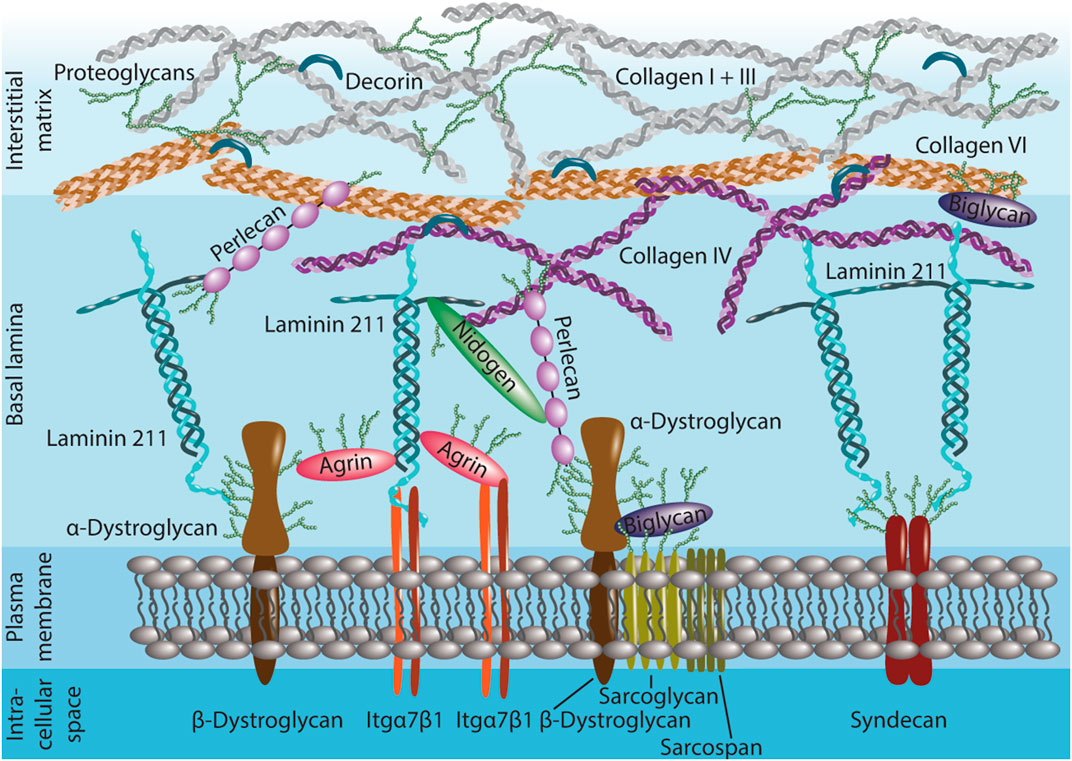
FIGURE 1. Structure of the extracellular matrix (ECM) in the quiescent muscle stem cell (MuSC) niche. MuSCs interface directly with the laminin 211 and collagen IV-rich basal lamina. Laminin 211, agrin, biglycan and perlecan interact with cell surface receptors such as dystroglycan and integrin (Itg) α7β1 and connect to syndecans. Nidogen and perlecan link the collagen IV network in the basal lamina to the plasma membrane and laminin. Perlecan, biglycan and decorin help anchoring the basal lamina to collagen I, III, and VI in the proteoglycan-rich interstitial matrix. Many ECM proteins are extensively glycosylated (green sidechains).
MuSCs contain high levels of the heterodimeric laminin receptor integrin α7β1, which has frequently been used as a marker for flow cytometry isolation (Figures 2A,D) (Blanco-Bose et al., 2001). While the mRNA coding for integrin β1 is readily detectable in quiescent MuSCs, the α7 subunit appears to be expressed at lower levels (Figure 2A). This phenomenon may be due to negative feedback regulation induced by the high abundance of laminin ligand in the quiescent niche, a notion that is supported by the fact that integrin α7 mRNA is upregulated in activated or differentiating cells when the ECM surrounding the cells becomes more heterogenous. The integrin α subunit consists of a short cytoplasmatic tail, followed by a transmembrane domain, two β-sandwich “calf” domains, the genu or “knee” domain, and an Ig-like “thigh” domain supporting the β-propeller that forms the ligand binding head (Figure 2B) (Gahmberg et al., 2009). The integrin β subunit is composed of a cytoplasmatic tail, followed by a transmembrane segment, the β-tail, 4 EGF-like domains, a β I-like domain, and the plexin-semaphorin-integrin (PSI) domain. Both the α and β integrin subunits contain binding sites for the divalent metal ions Mg2+ and Ca2+ that have differential functions in regulating ligand affinity (Zhang and Chen, 2012). However, complete removal of these cations using EDTA leads to an inhibition of integrin-ligand interactions, a mechanism that is commonly exploited when mild enzyme-free dissociation buffers are used to detach primary MuSC derived myoblasts from culture dishes containing ECM coating. Integrins have different ligand affinity states that depend on their structural conformation (Figure 2C) (Takagi and Springer, 2002). In their inactive state the two integrin subunits remain in a bent over, low-affinity conformation. Following intracellular signals or binding to ECM the extracellular portion of the two subunits can extend but remains in a closed low-ligand-affinity conformation. Upon multivalent ligand binding and intracellular stabilization by focal adhesion molecules including kindlin and talin that are anchored to the actin cytoskeleton, integrins will transition to the open high-affinity state in which the cytoplasmic “leg” domains become separated. Pax7-dependent deletion of integrin β1 leads to a depletion of the MuSC pool, suggesting a role in maintaining quiescence (Rozo et al., 2016). Interestingly, aged MuSCs contain less active, high-affinity integrin β1, and treatment with an activating antibody ameliorates age-associated stem cell dysfunction. Integrin α7 can be alternatively spliced to generate the variants α7X1, α7X2 that bind to different forms of laminin (von der Mark et al., 2002). α7X1, which does not contain exon 6 of the ITGA7 gene, appears to be mainly expressed during skeletal muscle development, while α7X2, which does not contain exon 5, is expressed in adult skeletal muscle (Collo et al., 1993; von der Mark et al., 2002). Inclusion of exon 5 leading to α7X1 expression at the expense of α7X2 impairs asymmetric MuSC division and the generation of committed myogenic progenitors (Dominici and Richard, 2022). Following activation, MuSCs have been reported to upregulate integrin α6β1, which binds to certain cell-autonomously secreted laminin isoforms driving planar divisions required for expansion of the stem cell pool (Rayagiri et al., 2018). While single cell sequencing data suggests that MuSCs in vivo do not express high levels of collagen-binding integrin heterodimers, it has been shown that primary MuSC-derived myoblasts cultured on collagen-rich substrates express a variety of α and β subunits including the collagen receptors integrin α1β1 and α2β1 (Figure 2A) (Hynes, 2002; Siegel et al., 2009). Interestingly, integrin α7β1 also has a role in MuSC motility, and antibody mediated blockade of either subunit slowed the movement of cells on cultured single fibers in live-imaging experiments. In contrast, blockade of the RGD specific integrin α5 and laminin specific integrin α6 subunits increased MuSC motility suggesting an inhibitory effect of certain ECM components on cell migration (Siegel et al., 2009).
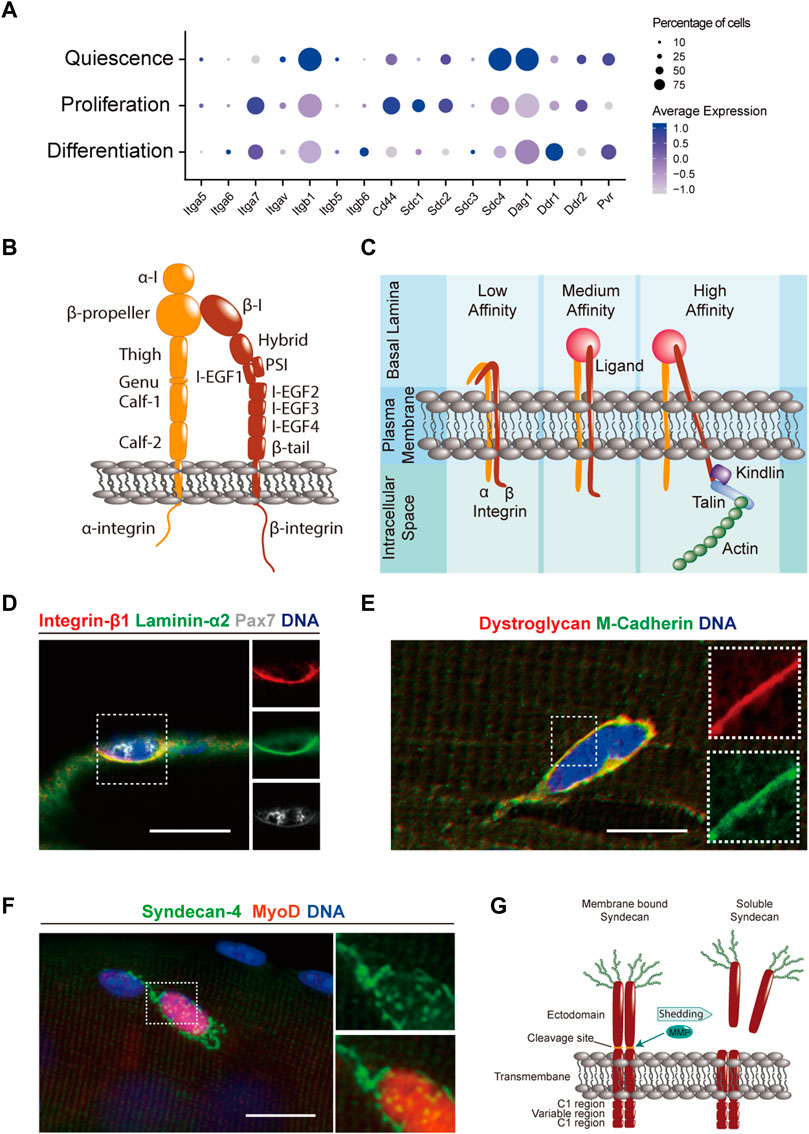
FIGURE 2. Transmembrane ECM components and receptors in MuSCs. (A) Dot plot of single cell RNA-sequencing data (Oprescu et al., 2020) obtained from the Gene Expression Omnibus (GEO) database (Barrett et al., 2013) from uninjured and regenerating skeletal mouse muscle at 5 days post injury that was generated using Seurat 4.0.5. (Hao et al., 2021). Genes coding for ECM interacting transmembrane proteins were selected based on the GO terms “adhesion receptor function” in the adhesome data base and “ECM receptors” in the reactome data base (Zaidel-Bar et al., 2007; Winograd-Katz et al., 2014; Griss et al., 2020). The data for quiescence was obtained from uninjured muscles, while proliferation and differentiation were defined based on the presence or absence of expression of genes such as Pax7, MyoD, MyoG, and MKI67 at 5 days post injury. Only genes significantly expressed by at least 10% of MuSCs are shown. (B) Scheme showing the domain structure of the integrin-α and -β subunits. (C) Scheme illustrating how integrins transition from their low-to high-affinity state upon multivalent ligand binding and intracellular stabilization by focal adhesion molecules. (D) Immunostaining showing integrin β1 (red), laminin α2 (green), Pax7 (white), and DNA (blue) in a MuSC on a manually teased mouse muscle fiber bundle preparation. (E) Immunostaining showing dystroglycan (red), M-cadherin (green), and DNA (blue) in a MuSC on an enzymatically isolated single mouse muscle fiber. (F) Immunostaining showing syndecan-4 (green), MyoD (red) and DNA (blue) in MuSCs on an enzymatically isolated single mouse muscle fiber after 24 h of culture. (G) Scheme showing the domain structure of syndecan dimers in their membrane bound and soluble forms. Matrix metalloproteinases (MMPs) cleave membrane syndecans in a process called “shedding” and release the extracellular domain into the surrounding ECM. Scale bars = 20 μm (D,E), and 10 μm (F).
The second highly expressed ECM receptor in MuSCs is dystroglycan (Figures 2A,E). Dystroglycan is synthesized from a single gene (DAG1) and is post-translationally cleaved into α and β subunits (Figure 1) (Ibraghimov-Beskrovnaya et al., 1992). α-dystroglycan is peripherally linked to β-dystroglycan, which spans the plasma membrane and is directly connected to the inner membrane protein dystrophin (Winder, 2001). Dystroglycan is a core component of the dystrophin glycoprotein complex (DGC), which includes the sarcoglycans and sarcospan as well as several intracellular proteins (Lapidos et al., 2004). The DGC provides a mechanical link to the extracellular matrix, and mutations in genes involved in this multicomponent complex destabilize the muscle fiber plasma membrane and can lead to several different types of muscular dystrophy (Gao and McNally, 2015). α-dystroglycan is highly glycosylated (Ervasti and Campbell, 1991). This includes N- and O-linked modifications, in which oligosaccharides are attached to the amide group of asparagine, or to the hydroxyl group of serine or threonine respectively (Muntoni et al., 2007). O-mannosyl structures on dystroglycan have been proposed to be directly required for laminin, perlecan and agrin binding (Chiba et al., 1997; Sasaki et al., 1998; Michele and Campbell, 2003). Mutations in genes encoding for glycosyltransferases can cause certain forms of congenital muscular dystrophy characterized by abnormal glycosylation of α-dystroglycan (Muntoni et al., 2007). Increasing evidence suggests a direct role of dystroglycan in regulating MuSC function. It has been shown that muscle fiber specific deletion of DAG1 leads to a mild form of muscular dystrophy (Cohn et al., 2002). Supporting the notion that DAG1 is highly expressed in MuSCs, progenitors that fuse to knockout fibers induce its synchronized reexpression during regeneration cycles. Moreover, MuSC-specific deletion of DAG1 has been shown to impair skeletal muscle regeneration (Dumont et al., 2015). Whether these effects are due to a reduced ability to maintain quiescence, or whether dystroglycan has a direct role following activation and proliferation, remains to be determined.
In skeletal muscle, heparan sulfate proteoglycans are present as integral matrix components such as perlecan, decorin, and biglycan. They can be attached to the ECM, as in the case of glypican, or can pass through the cell membrane, like syndecans (Iozzo et al., 1994; Brandan et al., 1996; Couchman and Woods, 1996; Wiberg et al., 2002). In MuSCs, syndecans-3 and -4 have important nonredundant roles in transducing and integrating signals from the ECM as well as from transmembrane signaling proteins (Cornelison et al., 2001). Loss of syndecan-3 leads to diminished proliferation, differentiation defects, and impaired self-renewal due to aberrant niche interactions, while loss of syndecan-4, which is highly expressed by MuSCs (Figures 2A,F), impairs activation, proliferation, migration, and differentiation via multiple mechanisms (Cornelison et al., 2004; Munoz et al., 2006; Pisconti et al., 2010; Shin et al., 2012; Bentzinger et al., 2013; Becsky et al., 2020; Ronning et al., 2020; Szabo et al., 2022). Through their extracellular domain and its associated heparan sulfate or chondroitin sulfate carbohydrate chains, syndecans form ternary structures with growth factors and their receptors including FGFs, transforming growth factor-β family members, Wnts, and small chemokines such as SDF-1/CXCL12 and RANTES/CCL5 (Allen et al., 2001; Slimani et al., 2003; Chen et al., 2004; Charnaux et al., 2005; Munoz et al., 2006). Syndecans also associate with transmembrane and membrane-bound ECM receptors such as integrins and A disintegrin and metalloproteases (ADAMs), and bind to extracellular matrix proteins including laminins, tenascin, collagens, and fibronectin (Couchman and Woods, 1999; Iba et al., 2000; Czarnowski, 2021). While some of these interactions are specific to only one syndecan, as for instance in case of syndecan-4, which is the sole and obligate coreceptor for CXCR4-CXCL12, many protein-protein interactions such as syndecan-integrin binding are common among all four family members, even though the four extracellular domains are each unique in their genomic sequence. Similarly, while with 28–34 amino acids their intracellular domains are comparably small, syndecans mediate interactions with a large number of second messengers, small G proteins, and cytoskeletal proteins (Simons and Horowitz, 2001). Syndecan-4 possesses a unique lysine-rich PIP2 binding domain that is not found in the other family members, which facilitates syndecan-4-specific signaling interactions. All members of the syndecan family form homodimers or multimers, which does not require their carbohydrate chains (Bernfield et al., 1992). In a process known as ectodomain shedding, the extracellular domains of syndecans can be proteolytically cleaved (Figure 2G) (Gopal, 2020). Syndecan shedding yields soluble proteoglycans retaining the binding characteristics of their transmembrane form that can become incorporated into the surrounding matrix and are even found in the systemic circulation (Eustace et al., 2019). Syndecan core protein cleavage by sheddases has been shown to be activated in response to cytokines such as IL-8 (Marshall et al., 2003). Multiple roles for syndecan shedding have been proposed, including a reduction of direct signal transduction in the originating cells, sequestration of growth factors away from cell surfaces, or broader paracrine effects on surrounding cells (Gopal, 2020). The plethora of ECM, cell-surface, and intracellular interactions that syndecans participate in highlights their roles as integrators of multiple signaling inputs and ‘tuners' of the downstream cellular response, although they are not generally considered to be either ECM components or classical transmembrane receptors. Recent single cell expression data further supports a role for syndecans, particularly syndecan-4, as key regulators of multiple signaling pathways in MuSCs (De Micheli et al., 2020). Expression of syndecan-4 is high in quiescent satellite cells, especially those that retain the most stem cell character and is maintained on proliferating and differentiating satellite cell progeny but lost on differentiated myofibers in vivo (Tanaka et al., 2009; Cho and Doles, 2017).
Extracellular matrix in the quiescent muscle stem cell niche
Collagen I and III fibrils are highly abundant in the interstitial space of homeostatic skeletal muscle (Figures 3A,B) (Light and Champion, 1984). Two genes, COL1A1 and COL1A2, are involved in collagen I formation. Collagen I contains homo- or heterotrimers generated from the transcription of these genes, with each subunit comprising over 1000 amino acids that can reach a length of up to 300 nm (Naomi et al., 2021). Collagen III is a homotrimer encoded by the COL3A1 gene that has the same molecular structure and approximate number of amino acids as collagen I (Kuivaniemi and Tromp, 2019). Collagen fibril formation is mainly an entropy-driven self-assembly process (Kadler et al., 1996). Fibril-forming collagens are synthesized in the form of soluble trimeric procollagen (Figure 3C). Through cleavage of N- and C-terminal peptide residues by metalloproteases, procollagen is processed into tropocollagen. Tropocollagen molecules spontaneously self-assemble into striated collagen fibrils that are stabilized by covalent bonds introduced by lysyl oxidase cross-linking.
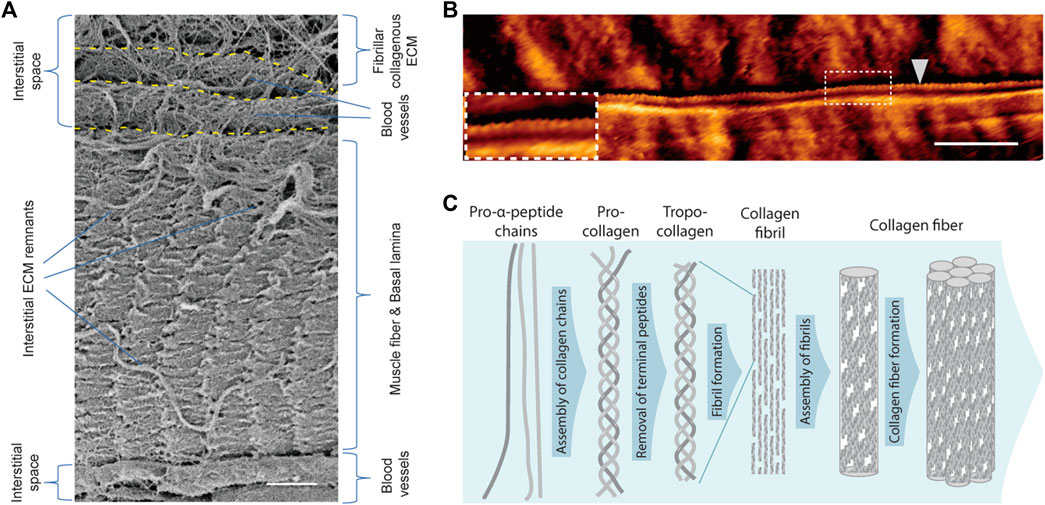
FIGURE 3. Fibrillar collagen and the interstitial space. (A) Scanning electron microscopy image of a manually teased mouse muscle fiber bundle preparation. The fiber is covered by the basal lamina and residues of interstitial ECM proteins. Flanking the fiber several ECM-covered blood vessels embedded in the interstitial space can be discerned. The upper most part of the image shows the extensive collagen I and III-rich ECM network in the interstitial space of skeletal muscle. (B) Atomic force microscopy image (contact mode) of a single collagen fibril (white arrowhead) on the surface of an enzymatically isolated single mouse muscle fiber. The characteristic banding pattern of overlap and gap regions of the collagen fibril can be discerned in the insert. (C) Scheme illustrating the fiber assembly process of collagen I. Scale bars = 2 µm (A), and 1 µm (B).
In the endomysial space around muscle fibers collagen appears to be mainly organized in wavy fibrillar networks, while in the connective tissue of the perimysium surrounding bundles of muscle fibers, collagen can form large “cables” (Gillies and Lieber, 2011). These large collagen cables are oriented from tendon to tendon and run along the surface of muscle fibers. Interestingly, in a mouse model displaying extensive fibrosis, it has been shown that the number and not the size of collagen cables in the perimysium determines muscle stiffness (Gillies et al., 2017). Fibrillar collagens are major determinants of tissue elasticity, which has been demonstrated to be critical for the self-renewal capacity of MuSCs (Gilbert et al., 2010; Moyle et al., 2020; Piersma et al., 2020). MuSCs cultured on hydrogels with an elastic modulus around 12 kPa, corresponding to skeletal muscle tissue, show improved survival, reduced differentiation, and an increased engraftment capacity after transplantation (Gilbert et al., 2010). Notably, MuSC dysfunction in aged skeletal muscle correlates with a 4-fold increase in tissue stiffness, an accumulation of collagen, and a higher hydroxyproline and advanced glycation end-product content (Lacraz et al., 2015). Moreover, it has been shown that aged skeletal muscle is characterized by decreased collagen fibril tortuosity and alignment. (Stearns-Reider et al., 2017). The resulting increase in tissue stiffness leads to altered YAP/TAZ mechanosensing in fibroblasts. Consequently, these fibroblasts release an anti-myogenic ECM that increases the expression of fibrogenic markers in MuSCs.
The popular single fiber isolation and culture method, which allows one to monitor MuSC dynamics ex vivo, exploits the high fibrillar collagen content in the interstitial space of skeletal muscle. In this technique, muscles are exposed to collagenase type I (ColG) typically isolated from the bacterium Clostridium histolyticum, which cleaves collagen I, II, and III (Rosenblatt et al., 1995; Cornelison and Wold, 1997; Pasut et al., 2013; Zhang et al., 2015). After digestion with this enzyme, single muscle fibers with their associated MuSCs and partially intact basal laminae that still contain most of their laminin and collagen IV core-networks can be isolated and maintained in growth media (Figure 4A). Due to its abundance of over 90% of the dry mass in bone and 60% in cartilage, collagen I from animal sources, either purified or in the form of gelatine, is frequently used as a coating substrate for the culture of MuSC derived primary myoblasts (Von Der Mark, 2006; Kim et al., 2020).
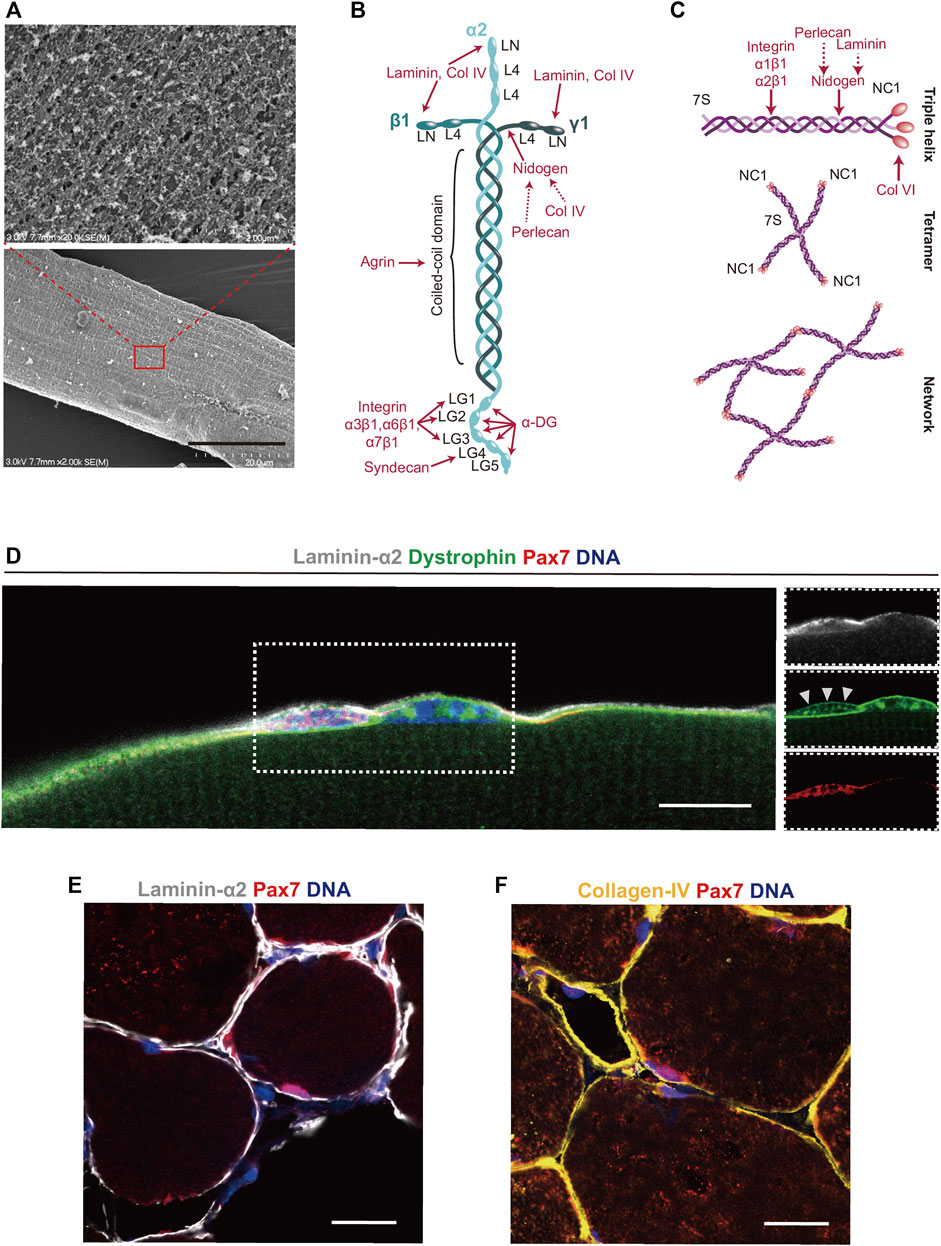
FIGURE 4. Laminin 211 and collagen IV in the basal lamina. (A) Scanning electron microscopy image of a single mouse muscle fiber that was isolated using collagenase type I, which cleaves collagen I, II, and III. The insert shows a higher magnification of the muscle fiber basal lamina as well as residues of interstitial ECM components. (B,C) Schemes showing the structure of laminin 211 and the assembly of the collagen IV network in the basal lamina. Interaction sites of ECM proteins and receptors are shown in red. (D) Immunostaining showing a single Pax7 positive MuSC (red) covered by laminin α2 (white) in the basal lamina, co-stained with the inner membrane marker dystrophin (green) and DNA (blue) on a manually teased mouse muscle fiber bundle. Dystrophin is expressed by both the muscle fiber and MuSCs, albeit levels are lower in quiescent MuSCs (white arrowheads). (E) Immunostaining of mouse skeletal muscle cross sections showing laminin α2 (white), (E), collagen IV (yellow), (F), Pax7 (red) and DNA (blue). Scale bars = 20 µm (Lower image A,D-F), and 2 µm (High magnification insert in A).
Notch signaling, which is for instance activated by proximity to blood vessels, controls enhancers proximal to the COL5A1, COL5A3, COL6A1, and COL6A2 genes in quiescent MuSCs (Baghdadi et al., 2018a; Verma et al., 2018). MuSC-specific conditional deletion of COL5A1 leads to premature cell cycle entry and a loss of quiescent cells from skeletal muscle. The quiescence promoting effects of collagen V appear to be mediated by the calcitonin receptor, a G-protein-coupled receptor that has been shown to have important functions in preventing MuSC activation via the cAMP-PKA pathway (Yamaguchi et al., 2015). Through microRNA-708, Notch signalling also has a role in supressing the expression of the focal adhesion protein tensin 3, which promotes MuSC quiescence and antagonizes cell migration (Baghdadi et al., 2018b). Tensins integrate ECM signals by binding to the intracellular tail of integrin β1, as well as to the actin cytoskeleton (Mouneimne and Brugge, 2007). Interestingly, Pax3-dependent deletion of the Notch effector Rbpj has been shown to lead to decreased autocrine deposition of laminin and collagen XVIII around emerging MuSCs during development (Brohl et al., 2012). Thus, several lines of evidence point towards Notch signaling as a key upstream regulator of cell-autonomous ECM deposition in the MuSC niche.
Over 30 years ago laminin was identified and isolated from Engelbreth-Holm-Swarm (EHS) mouse sarcoma (Chung et al., 1979; Timpl et al., 1979). Laminins are heterotrimeric high molecular weight proteins (∼400–900 kDa) that contain α, β, and γ chains, which depending on the subunit isoforms assemble into cross- or T-like structures (Holmberg and Durbeej, 2013). The five α, four β, and three γ chains in mammals are believed to have evolved from a single archetypical set of genes (Domogatskaya et al., 2012). Even though the laminin genes allow for over 60 possible permutations, only 16 heterotrimer combinations have been identified (Yap et al., 2019). Laminins are named based on the chains they contain. For instance, laminin 111, the isoform most abundantly secreted by EHS sarcoma cells, contains α1β1γ1 chains (Aumailley et al., 2005). αβγ laminin chains assemble through their coiled coil domains that are linked to each other by disulphide bonds (Hohenester, 2019).
During development the skeletal muscle basal lamina contains high amounts of laminin 211, while the 411 and 511 isoforms are detectable at much lower levels (Sanes et al., 1990; Patton et al., 1999). Except for the neuromuscular junction and blood vessels, the basal lamina in homeostatic adult skeletal muscle contains almost exclusively laminin 211. Following regeneration laminin 511 is transiently upregulated, while the 211 and 411 isoforms remain largely unchanged (Patton et al., 1999). The autopolymerisation of laminin 211 depends on the N-terminal globular LN domains of the three short arms (Figure 4B). Isoforms of laminin containing the α4, α3A, and γ2 chains lack the N-terminal globular domains and do not polymerize efficiently (Karamanos et al., 2021). Laminins mostly bind cell-surface receptors through five G domains (LG1–5) on the α chain C-terminus. While LG1–3 are responsible for binding to integrin α7β1, binding to dystroglycan appears to involve all 5 LG domains (Talts et al., 1999; Timpl et al., 2000; Smirnov et al., 2002). Due to its abundance in the niche, laminin 211 likely has a role in maintaining MuSC quiescence (Figures 4D,E). Supporting this notion, it has been shown that primary MuSC derived myoblasts proliferate and differentiate less efficiently on laminin 211 than on laminins containing the α5 chain (Penton et al., 2016).
Several peptide motifs in the LG4 domain of the laminin α2 chain contain heparin-binding sites and interact with syndecans, which could be another mechanism involved in the maintenance of MuSC quiescence (Hoffman et al., 1998; Suzuki et al., 2003). The N-terminal domain (NtA) of agrin binds to laminin near the center of its coiled coil domain and, through some of its C-terminal laminin-like G domains, links the basal lamina to cell surface receptors such as dystroglycan (Gesemann et al., 1996; Denzer et al., 1998). Nidogen-1 and -2 (also known as entactins) are 30–40 nm long sulfated monomeric glycoproteins of about 150 kDa that have a structurally homologous domain organisation (Kohfeldt et al., 1998). Nidogen has two N-terminal and one C-terminal globular domains that are connected by rod-like segments (Fox et al., 1991). By providing a link between the laminin and collagen IV networks, nidogen represents an important central hub in the basal lamina ECM (Mayer et al., 1993; Reinhardt et al., 1993; Kohfeldt et al., 1998). Binding of nidogen to the triple helical rod domain of collagen IV occurs via its G2 domain (Aumailley et al., 1989; Aumailley et al., 1993). Next to the collagen IV network, nidogen also connects the large proteoglycan perlecan to laminin (Hopf et al., 1999). The perlecan core protein has a molecular weight of approximately 500 kDa, which is increased by the addition of multiple heparan sulfate chains (Farach-Carson and Carson, 2007). Perlecan knockout mice show changes in the number of fast contracting fibers and display muscle hypertrophy accompanied by decreased myostatin expression, but direct effects on MuSCs remain to be assessed (Xu et al., 2010). Notably, perlecan binds and enhances the activity of several growth factors including vascular endothelial growth factor (VEGF) and fibroblast growth factors (FGFs) (Jiang and Couchman, 2003). Since VEGF is involved in maintaining quiescence and FGFs inhibit differentiation, it is plausible that perlecan mediated tethering of these molecules in the basal lamina plays a role in regulating MuSC function (Pawlikowski et al., 2017; Verma et al., 2018).
Collagen IV, which next to laminin forms the second core network of the basal lamina, originates from up to six different α-chains named α1(IV) to α6(IV) that can assemble into α1α1α2, α3α4α5, and α5α5α6 heterotrimers (Khoshnoodi et al., 2008). The α1(IV) and α2(IV) chains are present in most adult tissues, while the other four chains appear to largely play a role during development. Collagen IV chains contain an N-terminal 7S domain (named based on its sedimentation coefficient) that is followed by a long collagenous domain, and a non-collagenous C-terminal domain (NC1) (Figures 4C,F) (Hudson et al., 2003). By binding of two NC1 domains or by uniting four triple helical 7S domains, collagen IV can form irregular polygonal networks (Yurchenco and Ruben, 1987). Mutations in COL4A1 have been shown to correlate with myopathic changes in skeletal muscles of a human patient and mice with a mutation in this gene develop a progressive neuromuscular phenotype (Labelle-Dumais et al., 2019). However, a direct role of collagen IV in regulating MuSC function remains to be uncovered. In vitro experiments using blocking antibodies suggest that MuSC binding to collagen IV is largely indirect and mediated by perlecan (Villar et al., 1999). Cross-linking of NC1 domains in collagen IV is established through sulfilimine bonds (between methionine sulfur and lysine nitrogen), whose loss has been shown to influence tissue elasticity in the kidney (Bhave et al., 2017). Thus, it is conceivable that alongside interstitial fibrillar collagens, the collagen IV network influences MuSC function by finetuning the biomechanical properties of the basal lamina and the tissue in general (Gilbert et al., 2010).
Extracellular matrix regulation of activated muscle stem cells
Following damage to muscle fibers, MuSCs exit quiescence and enter the cell cycle to subsequently initiate the regenerative response that involves a multitude of highly complex spatiotemporally controlled niche interactions with a wide range of different supportive cell types (Mashinchian et al., 2018). In earliest stages of MuSC activation cellular protrusions stabilized by Rac GTPases are retracted through an upregulation of Rho/ROCK signaling, which is correlates with an increase in the expression of immediate early gene products such as c-jun and c-fos (Machado et al., 2017; van Velthoven et al., 2017; Almada et al., 2021; Kann et al., 2022). In order to overcome inhibitory signals by pro-inflammatory immune cells following skeletal muscle injury, the histone H3 lysine 27 demethylase JMJD3 epigenetically modifies the Has2 locus in MuSCs, which initiates hyaluronic acid synthesis and creates a permissive autoregulatory niche allowing the cells to exit quiescence (Nakka et al., 2022). Intravital imaging of mouse skeletal muscle has revealed that MuSC division and migration following injury are mostly oriented bi-directionally along the longitudinal axis of remnant basal lamina sheets that were termed “ghost fibers” (Webster et al., 2016). Reorientation of these ghost fibers lead to disorganization of newly formed muscle fibers, which emphasizes the importance of an instructive ECM template during the early stages of the regenerative response.
Within 8–9 h after activation, MuSCs begin to secrete copious amounts of the ECM glycoprotein fibronectin into their microenvironment (Figures 5A,B) (Bentzinger et al., 2013). Fibronectin has a molecular weight of about 230–270 kDa and is typically found as a dimer connected by C-terminal disulfide bonds (Singh et al., 2010). The fibronectin molecule consists of 12 FNI, 2 FNII, and 15–17 FNIII domains and, depending on alternative splicing, three main variable domains, the EDA and EDB FNIII extra domains, and the IIICS region can be included or excluded. Fibronectin is either found as soluble plasma fibronectin (pFN) devoid of EDA and EDB domains, or as cellular fibronectin (cFN) that may contain one or both of the EDA and EDB extra domains. Next to its ability to bind to laminin and collagen, fibronectin can engage a variety of integrins as well as syndecan-4 (Mao and Schwarzbauer, 2005). The RGD sequence (Arg–Gly–Asp) of fibronectin, which binds to α5β1 and αVβ3 integrins, is found in the 10th FNIII domain. Fibronectin is required for normal skeletal muscle regeneration and genetic ablation in mice leads to reduced MuSC numbers (Bentzinger et al., 2013; Lukjanenko et al., 2016). Whether specific splice variants of fibronectin have differential roles during skeletal muscle regeneration has not yet been explored. The expression of connective tissue growth factor (CTGF/CCN2), which is implicated in modulating TGF-β activity, has been shown to be an autoregulatory upstream regulator of fibronectin expression in activated myogenic cells (Vial et al., 2008). CTGF signalling is deregulated in pathologic conditions that are accompanied by excessive fibrotic deposition of ECM. Next to cell-autonomous secretion by activated MuSCs, fibronectin is secreted by fibro–adipogenic progenitors (FAPs) and cells of the hematopoietic lineage in regenerating skeletal muscle (Lukjanenko et al., 2016). Fibronectin binds to the syndecan-4/frizzled7 co-receptor complex and, together with Wnt7a, expands the stem cell pool during muscle regeneration (Bentzinger et al., 2013). As a consequence of aging, fibronectin levels are significantly reduced in regenerating skeletal muscle and exogenous supply of this protein improves MuSC function (Lukjanenko et al., 2016). Reduced levels of fibronectin in aged muscles goes along with a decreased activation of integrin β1 in MuSCs, which has been discussed in more detail above (Rozo et al., 2016).
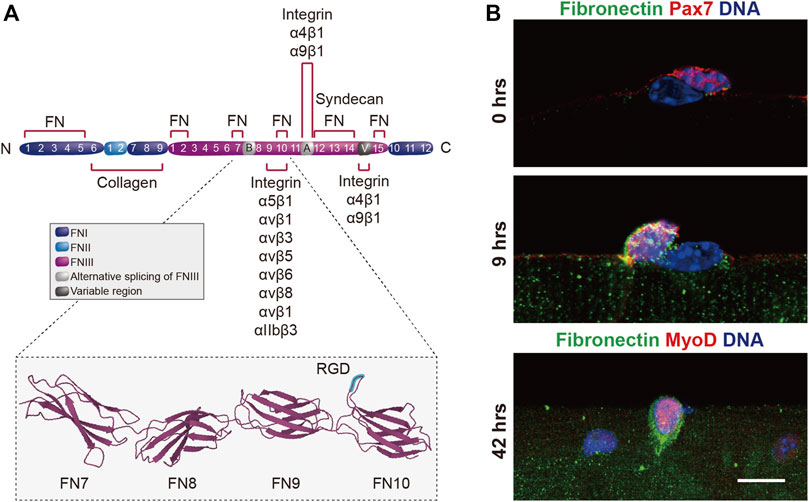
FIGURE 5. Autologous expression of fibronectin by MuSCs. (A) Scheme showing the domain structure of fibronectin and the binding sites for collagen, integrin and syndecan. The insert shows the seventh through the RGD-containing 10th type III repeats of fibronectin (Leahy et al., 1996) obtained from the RCSB Protein Data Bank (Berman et al., 2000). The RGD motif is highlighted in blue. (B) Immunostaining showing the endogenous expression of fibronectin (green), Pax7 or MyoD (red) and DNA (blue) in muscle stem cells on enzymatically isolated mouse single muscle fibers after 0, 9, and 42 h (hrs) in culture. Scale bar = 20 µm.
Laminin α1 and α5 have been shown to be enriched in the microenvironment of activated MuSCs (Rayagiri et al., 2018). Sox2-dependent ablation of laminin α1 in mice leads to a reduction in sublaminal MuSCs and a shift towards smaller muscle fibers in a multiple injury paradigm. MuSCs also express collagen VI and have been shown to reside in a microenvironment enriched in this ECM component (Urciuolo et al., 2013). Collagen VI is implicated in the pathogenesis of some forms of muscular dystrophy and is required for MuSC self-renewal, maintenance and survival. During development fetal myogenic progenitors have been shown to express elevated levels of tenascin-C, fibronectin, and collagen VI. In transplantation experiments it was observed that all three of these ECM components are critical for the function of adult MuSCs, while only knockdown of tenascin-c and collagen VI reduced the ability of fetal myogenic progenitors to participate in skeletal muscle repair (Tierney et al., 2016). Next to autoregulatory ECM deposition, activated MuSCs also remodel their structural microenvironment enzymatically. This includes expression of matrix metalloproteinase (MMP) 2 and 9 (Rayagiri et al., 2018). Pharmacologic inhibition of MMPs impairs MuSC proliferation in single fiber culture and genetic ablation of MMP9, which is pathologically increased in a mouse model of Duchenne muscular dystrophy, improves muscle regeneration (Li et al., 2009). Human myogenic progenitors express MMP14, which has been shown to be required for invasion of collagen I matrices (Lund et al., 2014). In summary, these results support the idea that autocrine deposition and modulation of different ECM components has stage-specific regulatory roles in the developmental and adult MuSC niche.
Amongst all mononuclear cell types contributing to skeletal muscle regeneration, FAPs appear to secrete the most significant amounts of ECM into the tissue (Figures 6A,B) (De Micheli et al., 2020; Oprescu et al., 2020). Large-scale integration of single-cell transcriptomic data sets has revealed that FAPs have a predicted interaction strength with myogenic cells that is magnitudes higher than with any other mononuclear cell type in regenerating muscle (McKellar et al., 2021). Moreover, 58% of the interactions between FAPs and MuSCs fall into the “Secreted Signaling” category, while 36% involve “ECM-Receptor” interactions. In agreement with their classification as mesenchymal stromal cells and coinciding with their anatomic localization, FAPs in homeostatic adult skeletal muscle express high levels of the interstitial ECM components collagen III and decorin (De Micheli et al., 2020). Close to the peak of MuSC proliferation around day 5 post injury, FAPs show strong expression of collagen I alongside periostin, biglycan and SPARC. Moreover, it has been demonstrated that a population of connective tissue resident mesenchymal cells are the main cell type secreting collagen VI in skeletal muscle (Figure 6C) (Braghetta et al., 2008). During development myogenic cells are required to activate a COL6A1 gene enhancer region in connective tissue resident mesenchymal cells. Notably, it has been shown that diphtheria-toxin mediated ablation of MuSCs in adult skeletal muscles leads to an expansion of mesenchymal fibroblast-like cell types and substantial fibrosis (Murphy et al., 2011). These observations suggest that MuSCs and ECM secreting mesenchymal cell populations have an intimate reciprocal relationship during skeletal muscle regeneration. FAPs have also been shown to be affected by the aging process and produce lower levels of the ECM-associated matricellular signaling protein WISP1, which influences MuSC self-renewal and differentiation. Transplantation of young FAPs or treatment with WISP1 restores the myogenic capacity of MuSCs in aged mice and improves skeletal muscle regeneration (Lukjanenko et al., 2019). Aged FAPs also secrete higher levels of SPARC Related modular calcium binding 2 (Smoc2), another matricellular protein, which induces aberrant integrin and MAP kinase signaling in aged MuSCs (Schüler et al., 2021).
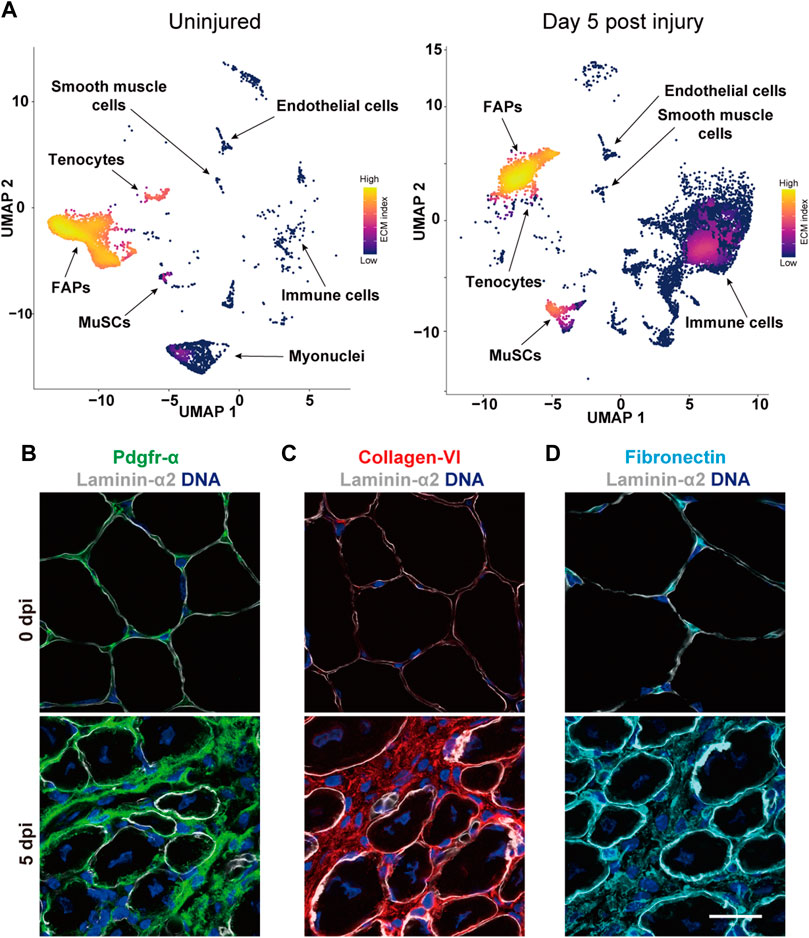
FIGURE 6. The MuSC niche in regenerating skeletal muscle. (A) The images show the joint gene expression density (Alquicira-Hernandez and Powell, 2021) of an index of ∼100 ECM genes obtained from the Matrisome database (Shao et al., 2020) in the UMAP projection (McInnes et al., 2018) of single cell RNA-sequencing data (Oprescu et al., 2020) deposited in the Gene Expression Omnibus (GEO) database (Barrett et al., 2013) from uninjured and regenerating mouse skeletal muscle at 5 days post injury. FAPs is short for fibro–adipogenic progenitors. (B) Immunostaining of mouse skeletal muscle cross sections under uninjured conditions (0 dpi) or 5 days post injury (5 dpi) visualizing FAPs based on Pdgfrα (green) co-stained with laminin α2 (white) and DNA (blue). (C,D) Immunostaining of mouse skeletal muscle cross sections at 0 or 5 dpi showing collagen IV (red), fibronectin (turquoise), laminin α2 (white) and DNA (blue). Scale bar = 20 µm.
During the expansion phase of the MuSC pool following injury, skeletal muscle becomes permeated with a transitional pro-regenerative ECM environment that is fundamentally different from its homeostatic composition (Goetsch et al., 2003; Bentzinger et al., 2013; Ceafalan et al., 2020). By day 5 after snake venom injury around 50% of transcripts that are at least two-fold increased in regenerating skeletal muscle fall into the gene ontology category of “ECM signaling” (Goetsch et al., 2003). This pro-regenerative ECM involves high levels of biglycan, fibronectin, collagen I and III-VIII, tenascin C, agrin, laminin α2, α5, β1, and β2, nidogen, perlecan, periostin, and others. Interestingly, during the remarkably efficient limb regeneration process in urodele amphibians a transitional regenerative ECM containing hyaluronic acid, fibronectin, and tenascin C has been suggested to instruct cell cycle entry and proliferation of myogenic progenitors (Calve et al., 2010). Under homeostatic conditions, these ECM molecules are thought to localize mainly in the interstitial space (Frantz et al., 2010). However, during the appendage regeneration process some of them can be detected in the basal lamina of muscle fibers, which suggests that they have a direct role in regulating the function of sublaminal myogenic progenitors. In regenerating mouse skeletal muscles, a similar phenomenon can be observed. While certain typically interstitial ECM components such as collagen VI do appear to overlap to a significant degree with the basal lamina during skeletal muscle regeneration, others such as fibronectin permeate it extensively (Figures 6C,D). Together with the observation that activated sublaminal MuSCs express several typically interstitial molecules with autoregulatory function, these observations suggest that the traditional compartmentalization of ECM components applies only partially during skeletal muscle regeneration.
Several ECM components have been implicated in the regulation of MuSC differentiation. For instance, the small proteoglycan fibromodulin antagonizes the inhibitory effects of myostatin and promotes myoblast differentiation (Lee et al., 2016). Insulin-like growth factor 1 stimulates myoblast fusion in synergy with collagen IV (Ito et al., 2015). The large glycoprotein fibrillin-1 is yet another example of an ECM component that influences myogenesis by modulating growth factor activity. Mutations in fibrillin-1 cause the connective tissue disease Marfan syndrome and influence the bioavailability of TGFβ1 (Chaudhry et al., 2007). Mice with a mutation in fibrilin-1 analogous to that found in humans with Marfan syndrome, display chronically increased TGFβ1 signaling, which impairs muscle regeneration by inhibiting MuSC proliferation and differentiation (Cohn et al., 2007). The heparan sulfate proteoglycan glypican-1 is expressed by MuSC-derived myoblasts and sequesters FGF-2 into lipid rafts, allowing the cells to efficiently differentiate. Loss of glypican-1 leads to reduced myotube formation and supresses the expression of myogenin (Gutierrez and Brandan, 2010). An analogous pro-differentiative mechanism seems to involve perlecan, which is also expressed by myoblasts and binds FGF-2 (Larrain et al., 1997). Activated MuSCs also express reversion-inducing cysteine-rich protein with Kazal motifs (RECK), an extracellular GPI-anchored glycoprotein with inhibitory functions for matrix metalloproteinases (Gutierrez et al., 2021). Reduced levels of RECK increase differentiation both in vitro and in knockout mice. These observations suggest a role of this protein in fine-tuning of the differentiation process by ECM remodeling. Altogether, multiple ECM proteins are involved in controlling MuSC differentiation and, in particular, the regulation of growth factors and matrix remodelling enzymes appears to be of central importance during this process.
Extracellular matrix-related muscular dystrophies and muscle stem cells
Muscular dystrophies are genetic disorders characterized by skeletal muscle weakening and degeneration that can lead to severe disability and premature death. Increasing evidence suggests that MuSC dysfunction plays a role in driving the progressive regenerative failure that characterizes many of these diseases (Le Moal et al., 2022). Recently, the term “satellite cell-opathies” has been proposed to describe conditions in which MuSC defects are the principal driver of disease progression (Ganassi et al., 2022). For instance, mutations in the MuSC master regulator Pax7 have been shown to lead to a progressive congenital myopathy in humans (Feichtinger et al., 2019; Marg et al., 2019). In case of muscular dystrophies caused by mutations that lead to an instability of muscle fibers, regenerative failure and MuSC dysfunction can be concomitant or arise subsequent to disease-initiating events. Consequently, such conditions may be considered secondary satellite cell-opathies. An example is Duchenne muscular dystrophy, a condition characterized by mutations in the DGC protein dystrophin, an inner membrane protein that is expressed by both muscle fibers and MuSCs. In preclinical models of Duchenne muscular dystrophy, it has been shown that in addition to the typical chronic multifocal muscle fiber degeneration, asymmetric satellite cell division is impaired and leads to a decreased pool of committed progenitors (Dumont et al., 2015).
Several other mutations causing forms of muscular dystrophy affect gene products involved in linking the interstitial space and the basal lamina to the muscle fiber plasma membrane (Henry and Campbell, 1999; Guiraud et al., 2015; Gawlik, 2018). In particular, mutations in the LAMA2 gene coding for the laminin α2 chain cause LAMA2-related muscular dystrophy, while mutations in the COL6A1, COL6A2, and COL6A3 genes lead to collagen VI-related myopathies, including Bethlem myopathy and Ullrich congenital muscular dystrophy (Pegoraro et al., 2000; Allamand and Guicheney, 2002; Lampe and Bushby, 2005; Bonnemann, 2011). The critical role of both laminin α2 and collagen VI in regulating MuSCs, supports the notion that stem cell dysfunction contributes to disease pathogenesis in both types of muscular dystrophy (Le Moal et al., 2022).
LAMA2-related muscular dystrophy shows a spectrum of clinical manifestations ranging from comparably mild late onset forms in adulthood to conditions that manifest in neonates, leading to severe muscle wasting accompanied by a broad spectrum of secondary effects such as spinal deformities (Sarkozy et al., 2020). Representative of the spectrum of disease severity in humans, several mouse models of LAMA2-related muscular dystrophy have been generated that express varying levels of mutated LAMA2 gene products or are full knockouts (Gawlik and Durbeej, 2020). These animal models reproduce the human skeletal muscle pathology well and present with features such as a wide variation in fiber size, extensive regeneration evidenced by centralized myonuclei, and fibrosis (Figures 7A,B). Interestingly, in the dyW model, which expresses reduced levels of a truncated LAMA2 gene product, or in dy3K mice which are complete knockouts, levels of the laminin receptor α-dystroglycan are significantly reduced (Moll et al., 2001; Bentzinger et al., 2005). In agreement with an important role of the laminin-dystroglycan interaction in MuSCs, dyW, and dy3K mice show incomplete skeletal muscle regeneration (Kuang et al., 1999; Bentzinger et al., 2005; Le Moal et al., 2022). Loss of the laminin α2 chain in LAMA2-related muscular dystrophy patients and mutant mice leads to a compensatory upregulation of laminin α4, which binds only weakly to dystroglycan and integrin α7β1, and does not effectively autopolymerize (Patton et al., 1997; Ringelmann et al., 1999; Colognato and Yurchenco, 2000; Talts et al., 2000; von der Mark et al., 2002; Reinhard et al., 2017). During fetal development in dyW mice laminins 411 and 511 are both present in close proximity to myogenic progenitors (Nunes et al., 2017). However, these laminins do not seem to be able to compensate for laminin α2, and the mice display reduced fetal muscle growth accompanied by a lower number of Pax7 and myogenin positive cells. Notably, restoration of basal lamina polymerization and cell surface receptor binding by transgenic expression chimeric linker-molecules composed of domains of agrin, nidogen, and laminin α1 leads to a dramatic rescue of the dystrophic pathology in dyW mice (Moll et al., 2001; Reinhard et al., 2017). One of these transgenes, a miniaturized form of agrin containing binding sites for laminins and α-dystroglycan, significantly improves skeletal muscle regeneration in dy3K mice (Bentzinger et al., 2005). Interestingly, it has been shown that laminin 111 is able to reduce pathology in mouse models of Duchenne and LAMA2-related muscular dystrophy (Rooney et al., 2009; Rooney et al., 2012). Presumably laminin 111 stabilizes dystrophin deficient muscle fibers via integrin α7, while it compensates for multiple processes in laminin α2 deficient muscles. In light of its important function for MuSCs, Laminin 111 likely also improves the regenerative capacity of LAMA2 deficient muscles (Rayagiri et al., 2018).
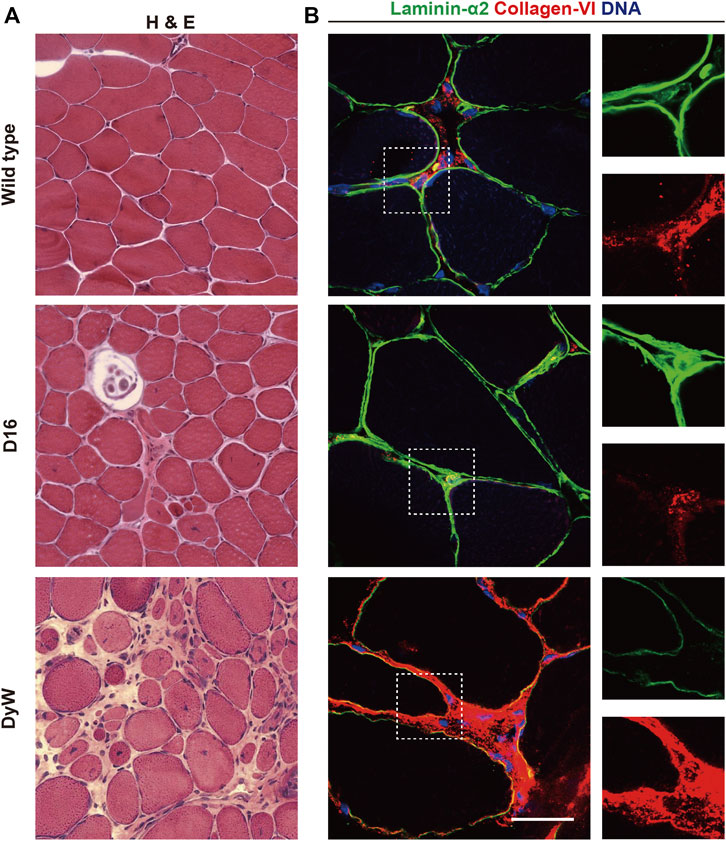
FIGURE 7. ECM and muscular dystrophy. (A) Hematoxylin and eosin (H&E) staining of skeletal muscle sections from wild type mice, COL6A3 mutant D16 mice, and LAMA2 mutant dyW mice (Kuang et al., 1998; Pan et al., 2014). (B) Immunostaining of skeletal muscle cross sections from wild type, D16 and dyW mice showing laminin α2 (green), collagen VI (red) and DNA (blue).
Similar to LAMA2-related diseases, collagen VI-related myopathies show a continuum of phenotypes ranging from comparably mild Bethlem myopathy to severe Ullrich congenital muscular dystrophy (Allamand et al., 2011; Bonnemann, 2011). A spectrum of different mutations in the COL6A1, COL6A2 and COL6A3 genes have been shown to cause effects ranging from dominant negative mechanisms to complete loss of function (Allamand et al., 2011; Bonnemann, 2011). The most common clinical presentation of collagen VI-related myopathies is muscle weakness, contractures, and hyperlaxity (Allamand et al., 2011). In Ullrich congenital muscular dystrophy these symptoms can be very severe and lead to an arrest of motor milestones. Skeletal muscle in Ullrich muscular dystrophy patients displays dystrophic features including fiber size variation, fibrosis, and centralized myonuclei (Nonaka et al., 1981). Mouse models of collagen VI-related myopathies show comparably mild phenotypes, which have paradoxically made them highly useful in exploring the underlying disease mechanisms (Figure 7A) (Bonaldo et al., 1998; Pan et al., 2014). In both COL6A1 and COL6A3 mutant mice, mild myopathic changes are associated with ultrastructural changes in mitochondria and the sarcoplasmic reticulum (Irwin et al., 2003; Pan et al., 2014). In addition, defects in autophagic flux in COL6A1-deficient mice have been linked to apoptotic processes in skeletal muscle (Grumati et al., 2010). COL6A1-deficient mice show smaller fibers and lower numbers of MuSCs after snake venom injury, and have a reduced ability to maintain quiescent MyoD negative cells (Urciuolo et al., 2013). In COL6A1 deficient mice, wild-type MuSCs have been shown to engraft better than COL6A1-deficient cells, suggesting that this ECM component acts through a cell-autonomous mechanism. Interestingly, the authors also demonstrated that COL6A1-deficient muscles display an increase in tissue elasticity whose partial rescue by transplantation of wild-type fibroblasts ameliorates the MuSC defect. Similarly, MuSC pathology in COL6A1 deficient mice is reduced upon transplantation of collagen VI secreting human adipose-derived stem cells and mesenchymal stromal cells (Alexeev et al., 2014; Takenaka-Ninagawa et al., 2021). Thus, collagen VI-related myopathies are another example highlighting the importance of tissue elasticity in the pathogenesis of MuSC defects.
Concluding remarks
Recent advances in single cell RNA sequencing have considerably improved our understanding of the contribution of different cell types to ECM synthesis in skeletal muscle and the MuSC niche. One interesting problem associated with single cell sequencing is the fact that muscle fibers are multinucleated, and therefore difficult to analyze using this technique. Thus, except for insights obtained from single nucleus RNA sequencing experiments, which only cover a fraction of all transcripts, the proportional contribution of muscle fibers to ECM synthesis in the niche remains somewhat enigmatic. In particular, newly formed multinucleated muscle fibers are highly transcriptionally active and may well contribute significantly to the pro-regenerative transitional ECM that instructs MuSC function following skeletal muscle injury. Emerging technologies such as spatial RNA-sequencing, multiplexed proteomics, and single-cell protein analysis, will ideally further advance our understanding of the complex niche regulation of MuSCs (Pham et al., 2021; McKellar et al., 2022; Wang et al., 2022). Importantly, compared to other relatively static stem cell niches, the microenvironment of MuSCs is highly dynamic and depends on the respective stage of muscle regeneration. Many aspects of the intricate ECM biology involved in these processes, for instance the return of MuSCs to quiescence, remain underexplored and future research regarding these topics will undoubtedly open interesting new avenues for stem cell dysfunction in aging and disease.
Author contributions
All authors listed have made a substantial, direct, and intellectual contribution to the work and approved it for publication.
Funding
CFB is supported by the Canadian Institutes of Health Research (PJT-162442), the Natural Sciences and Engineering Research Council of Canada (NSERC) (RGPIN-2017-05490), the Fonds de recherche du Québec - Santé (FRQS) (Dossiers 34813 and 296357), the ThéCell Network (supported by the FRQS), the Canadian Stem Cell Network, and a research chair of the Centre de Recherche Médicale de l’Université de Sherbrooke (CRMUS). MG is supported by NSERC (RGPIN-2019-06609). SS and YL are supported by a postdoctoral fellowship by the Centre de recherche du centre hospitalier universitaire de Sherbrooke (CRCHUS). SD is supported by a PhD fellowship by the FRQS (Dossier 305231). SS is supported by the Deutsche Forschungsgemeinschaft (DFG, German Research Foundation) – Projektnummer (505064275). DDWC is supported by the National Institutes of Health (NIH) (AR078045).
Acknowledgments
We are grateful to the laboratory of Shihuan Kuang (Purdue University) whose publicly deposited single-cell sequencing data we reanalyzed for Figures 2A,6A of this review (Oprescu et al., 2020). We are also grateful for the dyW and D16 mouse strains available from the Jackson Laboratory that were deposited by Eva Engvall (Sanford-Burnham Medical Research Institute) and Mon-Li Chu (Thomas Jefferson University), and which were used to generate Figure 7A of this review (Kuang et al., 1998; Pan et al., 2014). Lastly, we also thank Harold P. Erickson and colleagues (Duke University Medical Center), who made the structure encompassing of the seventh through the RGD-containing 10th type III repeats of fibronectin, which we used for the graphic in Figure 5A of this review, publicly available (Leahy et al., 1996).
Conflict of interest
The authors declare that the research was conducted in the absence of any commercial or financial relationships that could be construed as a potential conflict of interest.
Publisher’s note
All claims expressed in this article are solely those of the authors and do not necessarily represent those of their affiliated organizations, or those of the publisher, the editors and the reviewers. Any product that may be evaluated in this article, or claim that may be made by its manufacturer, is not guaranteed or endorsed by the publisher.
References
Alexeev, V., Arita, M., Donahue, A., Bonaldo, P., Chu, M. L., and Igoucheva, O. (2014). Human adipose-derived stem cell transplantation as a potential therapy for collagen VI-related congenital muscular dystrophy. Stem Cell Res. Ther. 5, 21. doi:10.1186/scrt411
Allamand, V., Brinas, L., Richard, P., Stojkovic, T., Quijano-Roy, S., and Bonne, G. (2011). ColVI myopathies: Where do we stand, where do we go? Skelet. Muscle 1, 30. doi:10.1186/2044-5040-1-30
Allamand, V., and Guicheney, P. (2002). Merosin-deficient congenital muscular dystrophy, autosomal recessive (MDC1A, MIM#156225, LAMA2 gene coding for alpha2 chain of laminin). Eur. J. Hum. Genet. 10, 91–94. doi:10.1038/sj.ejhg.5200743
Allen, B. L., Filla, M. S., and Rapraeger, A. C. (2001). Role of heparan sulfate as a tissue-specific regulator of FGF-4 and FGF receptor recognition. J. Cell Biol. 155, 845–858. doi:10.1083/jcb.200106075
Almada, A. E., Horwitz, N., Price, F. D., Gonzalez, A. E., Ko, M., Bolukbasi, O. V., et al. (2021). FOS licenses early events in stem cell activation driving skeletal muscle regeneration. Cell Rep. 34, 108656. doi:10.1016/j.celrep.2020.108656
Alquicira-Hernandez, J., and Powell, J. E. (2021). Nebulosa recovers single cell gene expression signals by kernel density estimation. Bioinformatics 37, 2485–2487. doi:10.1093/bioinformatics/btab003
Ancel, S., Stuelsatz, P., and Feige, J. N. (2021). Muscle stem cell quiescence: Controlling stemness by staying asleep. Trends Cell Biol. 31, 556–568. doi:10.1016/j.tcb.2021.02.006
Aumailley, M., Battaglia, C., Mayer, U., Reinhardt, D., Nischt, R., Timpl, R., et al. (1993). Nidogen mediates the formation of ternary complexes of basement membrane components. Kidney Int. 43, 7–12. doi:10.1038/ki.1993.3
Aumailley, M., Bruckner-Tuderman, L., Carter, W. G., Deutzmann, R., Edgar, D., Ekblom, P., et al. (2005). A simplified laminin nomenclature. Matrix Biol. 24, 326–332. doi:10.1016/j.matbio.2005.05.006
Aumailley, M., Wiedemann, H., Mann, K., and Timpl, R. (1989). Binding of nidogen and the laminin-nidogen complex to basement membrane collagen type IV. Eur. J. Biochem. 184, 241–248. doi:10.1111/j.1432-1033.1989.tb15013.x
Baghdadi, M. B., Castel, D., Machado, L., Fukada, S. I., Birk, D. E., Relaix, F., et al. (2018a). Reciprocal signalling by Notch-Collagen V-CALCR retains muscle stem cells in their niche. Nature 557, 714–718. doi:10.1038/s41586-018-0144-9
Baghdadi, M. B., Firmino, J., Soni, K., Evano, B., Di Girolamo, D., Mourikis, P., et al. (2018b). Notch-induced miR-708 antagonizes satellite cell migration and maintains quiescence. Cell Stem Cell 23, 859–868. doi:10.1016/j.stem.2018.09.017
Barrett, T., Wilhite, S. E., Ledoux, P., Evangelista, C., Kim, I. F., Tomashevsky, M., et al. (2013). NCBI GEO: Archive for functional genomics data sets-update. Nucleic Acids Res. 41, D991–D995. doi:10.1093/nar/gks1193
Becsky, D., Szabo, K., Gyulai-Nagy, S., Gajdos, T., Bartos, Z., Balind, A., et al. (2020). Syndecan-4 modulates cell polarity and migration by influencing centrosome positioning and intracellular calcium distribution. Front. Cell Dev. Biol. 8, 575227. doi:10.3389/fcell.2020.575227
Bentzinger, C. F., Barzaghi, P., Lin, S., and Ruegg, M. A. (2005). Overexpression of mini-agrin in skeletal muscle increases muscle integrity and regenerative capacity in laminin-alpha2-deficient mice. FASEB J. 19, 934–942. doi:10.1096/fj.04-3376com
Bentzinger, C. F., Wang, Y. X., Von Maltzahn, J., Soleimani, V. D., Yin, H., and Rudnicki, M. A. (2013). Fibronectin regulates Wnt7a signaling and satellite cell expansion. Cell Stem Cell 12, 75–87. doi:10.1016/j.stem.2012.09.015
Berman, H. M., Westbrook, J., Feng, Z., Gilliland, G., Bhat, T. N., Weissig, H., et al. (2000). The protein Data Bank. Nucleic Acids Res. 28, 235–242. doi:10.1093/nar/28.1.235
Bernfield, M., Kokenyesi, R., Kato, M., Hinkes, M. T., Spring, J., Gallo, R. L., et al. (1992). Biology of the syndecans: A family of transmembrane heparan sulfate proteoglycans. Annu. Rev. Cell Biol. 8, 365–393. doi:10.1146/annurev.cb.08.110192.002053
Bezakova, G., and Ruegg, M. A. (2003). New insights into the roles of agrin. Nat. Rev. Mol. Cell Biol. 4, 295–308. doi:10.1038/nrm1074
Bhave, G., Colon, S., and Ferrell, N. (2017). The sulfilimine cross-link of collagen IV contributes to kidney tubular basement membrane stiffness. Am. J. Physiol. Ren. Physiol. 313, F596-F602–F602. doi:10.1152/ajprenal.00096.2017
Blanco-Bose, W. E., Yao, C. C., Kramer, R. H., and Blau, H. M. (2001). Purification of mouse primary myoblasts based on alpha 7 integrin expression. Exp. Cell Res. 265, 212–220. doi:10.1006/excr.2001.5191
Bonaldo, P., Braghetta, P., Zanetti, M., Piccolo, S., Volpin, D., and Bressan, G. M. (1998). Collagen VI deficiency induces early onset myopathy in the mouse: An animal model for Bethlem myopathy. Hum. Mol. Genet. 7, 2135–2140. doi:10.1093/hmg/7.13.2135
Bonnemann, C. G. (2011). The collagen VI-related myopathies: Muscle meets its matrix. Nat. Rev. Neurol. 7, 379–390. doi:10.1038/nrneurol.2011.81
Bowe, M. A., Mendis, D. B., and Fallon, J. R. (2000). The small leucine-rich repeat proteoglycan biglycan binds to alpha-dystroglycan and is upregulated in dystrophic muscle. J. Cell Biol. 148, 801–810. doi:10.1083/jcb.148.4.801
Braghetta, P., Ferrari, A., Fabbro, C., Bizzotto, D., Volpin, D., Bonaldo, P., et al. (2008). An enhancer required for transcription of the Col6a1 gene in muscle connective tissue is induced by signals released from muscle cells. Exp. Cell Res. 314, 3508–3518. doi:10.1016/j.yexcr.2008.08.006
Brandan, E., Carey, D. J., Larrain, J., Melo, F., and Campos, A. (1996). Synthesis and processing of glypican during differentiation of skeletal muscle cells. Eur. J. Cell Biol. 71, 170–176.
Brohl, D., Vasyutina, E., Czajkowski, M. T., Griger, J., Rassek, C., Rahn, H. P., et al. (2012). Colonization of the satellite cell niche by skeletal muscle progenitor cells depends on Notch signals. Dev. Cell 23, 469–481. doi:10.1016/j.devcel.2012.07.014
Calve, S., Odelberg, S. J., and Simon, H. G. (2010). A transitional extracellular matrix instructs cell behavior during muscle regeneration. Dev. Biol. 344, 259–271. doi:10.1016/j.ydbio.2010.05.007
Ceafalan, L. C., Dobre, M., Milanesi, E., Niculae, A. M., Manole, E., Gherghiceanu, M., et al. (2020). Gene expression profile of adhesion and extracellular matrix molecules during early stages of skeletal muscle regeneration. J. Cell. Mol. Med. 24, 10140–10150. doi:10.1111/jcmm.15624
Charnaux, N., Brule, S., Hamon, M., Chaigneau, T., Saffar, L., Prost, C., et al. (2005). Syndecan-4 is a signaling molecule for stromal cell-derived factor-1 (SDF-1)/CXCL12. FEBS J. 272, 1937–1951. doi:10.1111/j.1742-4658.2005.04624.x
Chaudhry, S. S., Cain, S. A., Morgan, A., Dallas, S. L., Shuttleworth, C. A., and Kielty, C. M. (2007). Fibrillin-1 regulates the bioavailability of TGFbeta1. J. Cell Biol. 176, 355–367. doi:10.1083/jcb.200608167
Chen, L., Klass, C., and Woods, A. (2004). Syndecan-2 regulates transforming growth factor-beta signaling. J. Biol. Chem. 279, 15715–15718. doi:10.1074/jbc.C300430200
Chiba, A., Matsumura, K., Yamada, H., Inazu, T., Shimizu, T., Kusunoki, S., et al. (1997). Structures of sialylated O-linked oligosaccharides of bovine peripheral nerve alpha-dystroglycan. The role of a novel O-mannosyl-type oligosaccharide in the binding of alpha-dystroglycan with laminin. J. Biol. Chem. 272, 2156–2162. doi:10.1074/jbc.272.4.2156
Cho, D. S., and Doles, J. D. (2017). Single cell transcriptome analysis of muscle satellite cells reveals widespread transcriptional heterogeneity. Gene 636, 54–63. doi:10.1016/j.gene.2017.09.014
Chung, A. E., Jaffe, R., Freeman, I. L., Vergnes, J. P., Braginski, J. E., and Carlin, B. (1979). Properties of a basement membrane-related glycoprotein synthesized in culture by a mouse embryonal carcinoma-derived cell line. Cell 16, 277–287. doi:10.1016/0092-8674(79)90005-9
Clevers, H., and Watt, F. M. (2018). Defining adult stem cells by function, not by phenotype. Annu. Rev. Biochem. 87, 1015–1027. doi:10.1146/annurev-biochem-062917-012341
Cohn, R. D., Henry, M. D., Michele, D. E., Barresi, R., Saito, F., Moore, S. A., et al. (2002). Disruption of DAG1 in differentiated skeletal muscle reveals a role for dystroglycan in muscle regeneration. Cell 110, 639–648. doi:10.1016/s0092-8674(02)00907-8
Cohn, R. D., Van Erp, C., Habashi, J. P., Soleimani, A. A., Klein, E. C., Lisi, M. T., et al. (2007). Angiotensin II type 1 receptor blockade attenuates TGF-beta-induced failure of muscle regeneration in multiple myopathic states. Nat. Med. 13, 204–210. doi:10.1038/nm1536
Collo, G., Starr, L., and Quaranta, V. (1993). A new isoform of the laminin receptor integrin alpha 7 beta 1 is developmentally regulated in skeletal muscle. J. Biol. Chem. 268, 19019–19024. doi:10.1016/s0021-9258(17)46729-9
Colognato, H., and Yurchenco, P. D. (2000). Form and function: The laminin family of heterotrimers. Dev. Dyn. 218, 213–234. doi:10.1002/(SICI)1097-0177(200006)218:2<213:AID-DVDY1>3.0.CO;2-R
Cooper, A. R., and Macqueen, H. A. (1983). Subunits of laminin are differentially synthesized in mouse eggs and early embryos. Dev. Biol. 96, 467–471. doi:10.1016/0012-1606(83)90183-5
Cornelison, D. D., Filla, M. S., Stanley, H. M., Rapraeger, A. C., and Olwin, B. B. (2001). Syndecan-3 and syndecan-4 specifically mark skeletal muscle satellite cells and are implicated in satellite cell maintenance and muscle regeneration. Dev. Biol. 239, 79–94. doi:10.1006/dbio.2001.0416
Cornelison, D. D., Wilcox-Adelman, S. A., Goetinck, P. F., Rauvala, H., Rapraeger, A. C., and Olwin, B. B. (2004). Essential and separable roles for Syndecan-3 and Syndecan-4 in skeletal muscle development and regeneration. Genes Dev. 18, 2231–2236. doi:10.1101/gad.1214204
Cornelison, D. D., and Wold, B. J. (1997). Single-cell analysis of regulatory gene expression in quiescent and activated mouse skeletal muscle satellite cells. Dev. Biol. 191, 270–283. doi:10.1006/dbio.1997.8721
Couchman, J. R., and Woods, A. (1999). Syndecan-4 and integrins: Combinatorial signaling in cell adhesion. J. Cell Sci. 112 (20), 3415–3420. doi:10.1242/jcs.112.20.3415
Couchman, J. R., and Woods, A. (1996). Syndecans, signaling, and cell adhesion. J. Cell. Biochem. 61, 578–584. doi:10.1002/(sici)1097-4644(19960616)61:4<578:aid-jcb11>3.0.co;2-c
Czarnowski, D. (2021). Syndecans in cancer: A review of function, expression, prognostic value, and therapeutic significance. Cancer Treat. Res. Commun. 27, 100312. doi:10.1016/j.ctarc.2021.100312
Danielson, K. G., Baribault, H., Holmes, D. F., Graham, H., Kadler, K. E., and Iozzo, R. V. (1997). Targeted disruption of decorin leads to abnormal collagen fibril morphology and skin fragility. J. Cell Biol. 136, 729–743. doi:10.1083/jcb.136.3.729
De Micheli, A. J., Laurilliard, E. J., Heinke, C. L., Ravichandran, H., Fraczek, P., Soueid-Baumgarten, S., et al. (2020). Single-cell analysis of the muscle stem cell hierarchy identifies heterotypic communication signals involved in skeletal muscle regeneration. Cell Rep. 30, 3583–3595. doi:10.1016/j.celrep.2020.02.067
Denzer, A. J., Schulthess, T., Fauser, C., Schumacher, B., Kammerer, R. A., Engel, J., et al. (1998). Electron microscopic structure of agrin and mapping of its binding site in laminin-1. EMBO J. 17, 335–343. doi:10.1093/emboj/17.2.335
Dominici, C., and Richard, S. (2022). Muscle stem cell polarity requires QKI-mediated alternative splicing of Integrin Alpha-7 (Itga7). Life Sci. Alliance 5, e202101192. doi:10.26508/lsa.202101192
Domogatskaya, A., Rodin, S., and Tryggvason, K. (2012). Functional diversity of laminins. Annu. Rev. Cell Dev. Biol. 28, 523–553. doi:10.1146/annurev-cellbio-101011-155750
Dong, L. J., Hsieh, J. C., and Chung, A. E. (1995). Two distinct cell attachment sites in entactin are revealed by amino acid substitutions and deletion of the RGD sequence in the cysteine-rich epidermal growth factor repeat 2. J. Biol. Chem. 270, 15838–15843. doi:10.1074/jbc.270.26.15838
Dumont, N. A., Wang, Y. X., Von Maltzahn, J., Pasut, A., Bentzinger, C. F., Brun, C. E., et al. (2015). Dystrophin expression in muscle stem cells regulates their polarity and asymmetric division. Nat. Med. 21, 1455–1463. doi:10.1038/nm.3990
Ervasti, J. M., and Campbell, K. P. (1991). Membrane organization of the dystrophin-glycoprotein complex. Cell 66, 1121–1131. doi:10.1016/0092-8674(91)90035-w
Eustace, A. D., Mcnaughton, E. F., King, S., Kehoe, O., Kungl, A., Mattey, D., et al. (2019). Soluble syndecan-3 binds chemokines, reduces leukocyte migration in vitro and ameliorates disease severity in models of rheumatoid arthritis. Arthritis Res. Ther. 21, 172. doi:10.1186/s13075-019-1939-2
Farach-Carson, M. C., and Carson, D. D. (2007). Perlecan-a multifunctional extracellular proteoglycan scaffold. Glycobiology 17, 897–905. doi:10.1093/glycob/cwm043
Feichtinger, R. G., Mucha, B. E., Hengel, H., Orfi, Z., Makowski, C., Dort, J., et al. (2019). Biallelic variants in the transcription factor PAX7 are a new genetic cause of myopathy. Genet. Med. 21, 2521–2531. doi:10.1038/s41436-019-0532-z
Fox, J. W., Mayer, U., Nischt, R., Aumailley, M., Reinhardt, D., Wiedemann, H., et al. (1991). Recombinant nidogen consists of three globular domains and mediates binding of laminin to collagen type IV. EMBO J. 10, 3137–3146. doi:10.1002/j.1460-2075.1991.tb04875.x
Frantz, C., Stewart, K. M., and Weaver, V. M. (2010). The extracellular matrix at a glance. J. Cell Sci. 123, 4195–4200. doi:10.1242/jcs.023820
Fukada, S. I., Higashimoto, T., and Kaneshige, A. (2022). Differences in muscle satellite cell dynamics during muscle hypertrophy and regeneration. Skelet. Muscle 12, 17. doi:10.1186/s13395-022-00300-0
Gahmberg, C. G., Fagerholm, S. C., Nurmi, S. M., Chavakis, T., Marchesan, S., and Gronholm, M. (2009). Regulation of integrin activity and signalling. Biochim. Biophys. Acta 1790, 431–444. doi:10.1016/j.bbagen.2009.03.007
Ganassi, M., Muntoni, F., and Zammit, P. S. (2022). Defining and identifying satellite cell-opathies within muscular dystrophies and myopathies. Exp. Cell Res. 411, 112906. doi:10.1016/j.yexcr.2021.112906
Gao, Q. Q., and Mcnally, E. M. (2015). The dystrophin complex: Structure, function, and implications for therapy. Compr. Physiol. 5, 1223–1239. doi:10.1002/cphy.c140048
Garg, K., Corona, B. T., and Walters, T. J. (2015). Therapeutic strategies for preventing skeletal muscle fibrosis after injury. Front. Pharmacol. 6, 87. doi:10.3389/fphar.2015.00087
Gattazzo, F., Urciuolo, A., and Bonaldo, P. (2014). Extracellular matrix: A dynamic microenvironment for stem cell niche. Biochim. Biophys. Acta 1840, 2506–2519. doi:10.1016/j.bbagen.2014.01.010
Gawlik, K. I., and Durbeej, M. (2020). A family of laminin α2 chain-deficient mouse mutants: Advancing the research on LAMA2-CMD Front. Mol. Neurosci. 13, 59. doi:10.3389/fnmol.2020.00059
Gawlik, K. I. (2018). At the crossroads of clinical and preclinical research for muscular dystrophy-are we closer to effective treatment for patients? Int. J. Mol. Sci. 19, E1490. doi:10.3390/ijms19051490
Gesemann, M., Cavalli, V., Denzer, A. J., Brancaccio, A., Schumacher, B., and Ruegg, M. A. (1996). Alternative splicing of agrin alters its binding to heparin, dystroglycan, and the putative agrin receptor. Neuron 16, 755–767. doi:10.1016/s0896-6273(00)80096-3
Gilbert, P. M., Havenstrite, K. L., Magnusson, K. E., Sacco, A., Leonardi, N. A., Kraft, P., et al. (2010). Substrate elasticity regulates skeletal muscle stem cell self-renewal in culture. Science 329, 1078–1081. doi:10.1126/science.1191035
Gillies, A. R., Chapman, M. A., Bushong, E. A., Deerinck, T. J., Ellisman, M. H., and Lieber, R. L. (2017). High resolution three-dimensional reconstruction of fibrotic skeletal muscle extracellular matrix. J. Physiol. 595, 1159–1171. doi:10.1113/JP273376
Gillies, A. R., and Lieber, R. L. (2011). Structure and function of the skeletal muscle extracellular matrix. Muscle Nerve 44, 318–331. doi:10.1002/mus.22094
Goetsch, S. C., Hawke, T. J., Gallardo, T. D., Richardson, J. A., and Garry, D. J. (2003). Transcriptional profiling and regulation of the extracellular matrix during muscle regeneration. Physiol. Genomics 14, 261–271. doi:10.1152/physiolgenomics.00056.2003
Gopal, S. (2020). Syndecans in inflammation at a glance. Front. Immunol. 11, 227. doi:10.3389/fimmu.2020.00227
Griss, J., Viteri, G., Sidiropoulos, K., Nguyen, V., Fabregat, A., and Hermjakob, H. (2020). ReactomeGSA - efficient multi-omics comparative pathway analysis. Mol. Cell. Proteomics 19, 2115–2124. doi:10.1074/mcp.TIR120.002155
Grosberg, R. K., and Strathmann, R. R. (2007). The evolution of multicellularity: A minor major transition? Annu. Rev. Ecol. Evol. Syst. 38, 621–654. doi:10.1146/annurev.ecolsys.36.102403.114735
Grumati, P., Coletto, L., Sabatelli, P., Cescon, M., Angelin, A., Bertaggia, E., et al. (2010). Autophagy is defective in collagen VI muscular dystrophies, and its reactivation rescues myofiber degeneration. Nat. Med. 16, 1313–1320. doi:10.1038/nm.2247
Guiraud, S., Aartsma-Rus, A., Vieira, N. M., Davies, K. E., Van Ommen, G. J., and Kunkel, L. M. (2015). The pathogenesis and therapy of muscular dystrophies. Annu. Rev. Genomics Hum. Genet. 16, 281–308. doi:10.1146/annurev-genom-090314-025003
Gutierrez, J., and Brandan, E. (2010). A novel mechanism of sequestering fibroblast growth factor 2 by glypican in lipid rafts, allowing skeletal muscle differentiation. Mol. Cell. Biol. 30, 1634–1649. doi:10.1128/MCB.01164-09
Gutierrez, J., Gonzalez, D., Escalona-Rivano, R., Takahashi, C., and Brandan, E. (2021). Reduced RECK levels accelerate skeletal muscle differentiation, improve muscle regeneration, and decrease fibrosis. FASEB J. 35, e21503. doi:10.1096/fj.202001646RR
Hao, Y., Hao, S., Andersen-Nissen, E., Mauck, W. M., Zheng, S., Butler, A., et al. (2021). Integrated analysis of multimodal single-cell data. Cell 184, 3573–3587.e29. doi:10.1016/j.cell.2021.04.048
Hardy, D., Besnard, A., Latil, M., Jouvion, G., Briand, D., Thepenier, C., et al. (2016). Comparative study of injury models for studying muscle regeneration in mice. PLoS One 11, e0147198. doi:10.1371/journal.pone.0147198
Henry, M. D., and Campbell, K. P. (1999). Dystroglycan inside and out. Curr. Opin. Cell Biol. 11, 602–607. doi:10.1016/s0955-0674(99)00024-1
Hoffman, M. P., Nomizu, M., Roque, E., Lee, S., Jung, D. W., Yamada, Y., et al. (1998). Laminin-1 and laminin-2 G-domain synthetic peptides bind syndecan-1 and are involved in acinar formation of a human submandibular gland cell line. J. Biol. Chem. 273, 28633–28641. doi:10.1074/jbc.273.44.28633
Hohenester, E. (2019). Structural biology of laminins. Essays Biochem. 63, 285–295. doi:10.1042/EBC20180075
Holmberg, J., and Durbeej, M. (2013). Laminin-211 in skeletal muscle function. Cell adh. Migr. 7, 111–121. doi:10.4161/cam.22618
Hopf, M., Gohring, W., Kohfeldt, E., Yamada, Y., and Timpl, R. (1999). Recombinant domain IV of perlecan binds to nidogens, laminin-nidogen complex, fibronectin, fibulin-2 and heparin. Eur. J. Biochem. 259, 917–925. doi:10.1046/j.1432-1327.1999.00127.x
Hudson, B. G., Tryggvason, K., Sundaramoorthy, M., and Neilson, E. G. (2003). Alport's syndrome, Goodpasture's syndrome, and type IV collagen. N. Engl. J. Med. 348, 2543–2556. doi:10.1056/NEJMra022296
Hynes, R. O. (2002). Integrins: Bidirectional, allosteric signaling machines. Cell 110, 673–687. doi:10.1016/s0092-8674(02)00971-6
Hynes, R. O. (2012). The evolution of metazoan extracellular matrix. J. Cell Biol. 196, 671–679. doi:10.1083/jcb.201109041
Hynes, R. O. (2009). The extracellular matrix: Not just pretty fibrils. Science 326, 1216–1219. doi:10.1126/science.1176009
Iba, K., Albrechtsen, R., Gilpin, B., Frohlich, C., Loechel, F., Zolkiewska, A., et al. (2000). The cysteine-rich domain of human ADAM 12 supports cell adhesion through syndecans and triggers signaling events that lead to beta1 integrin-dependent cell spreading. J. Cell Biol. 149, 1143–1156. doi:10.1083/jcb.149.5.1143
Ibraghimov-Beskrovnaya, O., Ervasti, J. M., Leveille, C. J., Slaughter, C. A., Sernett, S. W., and Campbell, K. P. (1992). Primary structure of dystrophin-associated glycoproteins linking dystrophin to the extracellular matrix. Nature 355, 696–702. doi:10.1038/355696a0
Iozzo, R. V., Cohen, I. R., Grassel, S., and Murdoch, A. D. (1994). The biology of perlecan: The multifaceted heparan sulphate proteoglycan of basement membranes and pericellular matrices. Biochem. J. 302 (3), 625–639. doi:10.1042/bj3020625
Irwin, W. A., Bergamin, N., Sabatelli, P., Reggiani, C., Megighian, A., Merlini, L., et al. (2003). Mitochondrial dysfunction and apoptosis in myopathic mice with collagen VI deficiency. Nat. Genet. 35, 367–371. doi:10.1038/ng1270
Ito, A., Yamamoto, M., Ikeda, K., Sato, M., Kawabe, Y., and Kamihira, M. (2015). Effects of type IV collagen on myogenic characteristics of IGF-I gene-engineered myoblasts. J. Biosci. Bioeng. 119, 596–603. doi:10.1016/j.jbiosc.2014.10.008
Jiang, X., and Couchman, J. R. (2003). Perlecan and tumor angiogenesis. J. Histochem. Cytochem. 51, 1393–1410. doi:10.1177/002215540305101101
Kadler, K. E., Holmes, D. F., Trotter, J. A., and Chapman, J. A. (1996). Collagen fibril formation. Biochem. J. 316 (1), 1–11. doi:10.1042/bj3160001
Kann, A. P., Hung, M., Wang, W., Nguyen, J., Gilbert, P. M., Wu, Z., et al. (2022). An injury-responsive Rac-to-Rho GTPase switch drives activation of muscle stem cells through rapid cytoskeletal remodeling. Cell Stem Cell 29, 933–947.e6. doi:10.1016/j.stem.2022.04.016
Karamanos, N. K., Theocharis, A. D., Piperigkou, Z., Manou, D., Passi, A., Skandalis, S. S., et al. (2021). A guide to the composition and functions of the extracellular matrix. FEBS J. 288, 6850–6912. doi:10.1111/febs.15776
Katz, B. (1961). The terminations of the afferent nerve fibre in the muscle spindle of the frog. Philosophical Trans. R. Soc. Lond. Ser. B, Biol. Sci. 243, 221–240.
Khoshnoodi, J., Pedchenko, V., and Hudson, B. G. (2008). Mammalian collagen IV. Microsc. Res. Tech. 71, 357–370. doi:10.1002/jemt.20564
Kim, K. H., Qiu, J., and Kuang, S. (2020). Isolation, culture, and differentiation of primary myoblasts derived from muscle satellite cells. Bio. Protoc. 10, e3686. doi:10.21769/BioProtoc.3686
Kohfeldt, E., Sasaki, T., Gohring, W., and Timpl, R. (1998). Nidogen-2: A new basement membrane protein with diverse binding properties. J. Mol. Biol. 282, 99–109. doi:10.1006/jmbi.1998.2004
Kuang, W., Xu, H., Vachon, P. H., Liu, L., Loechel, F., Wewer, U. M., et al. (1998). Merosin-deficient congenital muscular dystrophy Partial genetic correction in two mouse models. J. Clin. Invest. 102, 844–852. doi:10.1172/JCI3705
Kuang, W., Xu, H., Vilquin, J. T., and Engvall, E. (1999). Activation of the lama2 gene in muscle regeneration: Abortive regeneration in laminin alpha2-deficiency. Lab. Invest. 79, 1601–1613.
Kuivaniemi, H., and Tromp, G. (2019). Type III collagen (COL3A1): Gene and protein structure, tissue distribution, and associated diseases. Gene 707, 151–171. doi:10.1016/j.gene.2019.05.003
Kuo, H. J., Maslen, C. L., Keene, D. R., and Glanville, R. W. (1997). Type VI collagen anchors endothelial basement membranes by interacting with type IV collagen. J. Biol. Chem. 272, 26522–26529. doi:10.1074/jbc.272.42.26522
Labelle-Dumais, C., Schuitema, V., Hayashi, G., Hoff, K., Gong, W., Dao, D. Q., et al. (2019). COL4A1 mutations cause neuromuscular disease with tissue-specific mechanistic heterogeneity. Am. J. Hum. Genet. 104, 847–860. doi:10.1016/j.ajhg.2019.03.007
Lacraz, G., Rouleau, A. J., Couture, V., Sollrald, T., Drouin, G., Veillette, N., et al. (2015). Increased stiffness in aged skeletal muscle impairs muscle progenitor cell proliferative activity. PLoS One 10, e0136217. doi:10.1371/journal.pone.0136217
Lampe, A. K., and Bushby, K. M. (2005). Collagen VI related muscle disorders. J. Med. Genet. 42, 673–685. doi:10.1136/jmg.2002.002311
Lapidos, K. A., Kakkar, R., and Mcnally, E. M. (2004). The dystrophin glycoprotein complex: Signaling strength and integrity for the sarcolemma. Circ. Res. 94, 1023–1031. doi:10.1161/01.RES.0000126574.61061.25
Larrain, J., Alvarez, J., Hassell, J. R., and Brandan, E. (1997). Expression of perlecan, a proteoglycan that binds myogenic inhibitory basic fibroblast growth factor, is down regulated during skeletal muscle differentiation. Exp. Cell Res. 234, 405–412. doi:10.1006/excr.1997.3648
Le Moal, E., Liu, Y., Collerette-Tremblay, J., Dumontier, S., Boutin, J., Dort, J., et al. (2022). Apelin stimulation of the perivascular MuSC niche enhances endogenous repair in muscular dystrophy. bioRxiv.
Leahy, D. J., Aukhil, I., and Erickson, H. P. (1996). 2.0 A crystal structure of a four-domain segment of human fibronectin encompassing the RGD loop and synergy region. Cell 84, 155–164. doi:10.1016/s0092-8674(00)81002-8
Lee, E. J., Jan, A. T., Baig, M. H., Ashraf, J. M., Nahm, S. S., Kim, Y. W., et al. (2016). Fibromodulin: A master regulator of myostatin controlling progression of satellite cells through a myogenic program. FASEB J. 30, 2708–2719. doi:10.1096/fj.201500133R
Leivo, I., Vaheri, A., Timpl, R., and Wartiovaara, J. (1980). Appearance and distribution of collagens and laminin in the early mouse embryo. Dev. Biol. 76, 100–114. doi:10.1016/0012-1606(80)90365-6
Li, H., Mittal, A., Makonchuk, D. Y., Bhatnagar, S., and Kumar, A. (2009). Matrix metalloproteinase-9 inhibition ameliorates pathogenesis and improves skeletal muscle regeneration in muscular dystrophy. Hum. Mol. Genet. 18, 2584–2598. doi:10.1093/hmg/ddp191
Light, N., and Champion, A. E. (1984). Characterization of muscle epimysium, perimysium and endomysium collagens. Biochem. J. 219, 1017–1026. doi:10.1042/bj2191017
Lukjanenko, L., Jung, M. J., Hegde, N., Perruisseau-Carrier, C., Migliavacca, E., Rozo, M., et al. (2016). Loss of fibronectin from the aged stem cell niche affects the regenerative capacity of skeletal muscle in mice. Nat. Med. 22, 897–905. doi:10.1038/nm.4126
Lukjanenko, L., Karaz, S., Stuelsatz, P., Gurriaran-Rodriguez, U., Michaud, J., Dammone, G., et al. (2019). Aging disrupts muscle stem cell function by impairing matricellular WISP1 secretion from fibro-adipogenic progenitors. Cell Stem Cell 24, 433–446. doi:10.1016/j.stem.2018.12.014
Lund, D. K., Mouly, V., and Cornelison, D. D. (2014). MMP-14 is necessary but not sufficient for invasion of three-dimensional collagen by human muscle satellite cells. Am. J. Physiol. Cell Physiol. 307, C140–149.
Machado, L., Esteves De Lima, J., Fabre, O., Proux, C., Legendre, R., Szegedi, A., et al. (2017). In situ fixation redefines quiescence and early activation of skeletal muscle stem cells. Cell Rep. 21, 1982–1993. doi:10.1016/j.celrep.2017.10.080
Mao, Y., and Schwarzbauer, J. E. (2005). Fibronectin fibrillogenesis, a cell-mediated matrix assembly process. Matrix Biol. 24, 389–399. doi:10.1016/j.matbio.2005.06.008
Marg, A., Escobar, H., Karaiskos, N., Grunwald, S. A., Metzler, E., Kieshauer, J., et al. (2019). Human muscle-derived CLEC14A-positive cells regenerate muscle independent of PAX7. Nat. Commun. 10, 5776. doi:10.1038/s41467-019-13650-z
Marshall, L. J., Ramdin, L. S., Brooks, T., Pc, D. P., and Shute, J. K. (2003). Plasminogen activator inhibitor-1 supports IL-8-mediated neutrophil transendothelial migration by inhibition of the constitutive shedding of endothelial IL-8/heparan sulfate/syndecan-1 complexes. J. Immunol. 171, 2057–2065. doi:10.4049/jimmunol.171.4.2057
Mashinchian, O., Pisconti, A., Le Moal, E., and Bentzinger, C. F. (2018). The muscle stem cell niche in health and disease. Curr. Top. Dev. Biol. 126, 23–65. doi:10.1016/bs.ctdb.2017.08.003
Mauro, A. (1961). Satellite cell of skeletal muscle fibers. J. Biophys. Biochem. Cytol. 9, 493–495. doi:10.1083/jcb.9.2.493
Mayer, U., Nischt, R., Poschl, E., Mann, K., Fukuda, K., Gerl, M., et al. (1993). A single EGF-like motif of laminin is responsible for high affinity nidogen binding. EMBO J. 12, 1879–1885. doi:10.1002/j.1460-2075.1993.tb05836.x
Mcinnes, L., Healy, J., and Melville, J. (2018). Umap: Uniform manifold approximation and projection for dimension reduction. arXiv preprint arXiv:1802.03426.
Mckellar, D. W., Mantri, M., Hinchman, M., Parker, J. S. L., Sethupathy, P., Cosgrove, B. D., et al. (2022). In situ polyadenylation enables spatial mapping of the total transcriptome. bioRxiv 2004. doi:10.1101/2022.04.20.488964
Mckellar, D. W., Walter, L. D., Song, L. T., Mantri, M., Wang, M. F. Z., De Vlaminck, I., et al. (2021). Large-scale integration of single-cell transcriptomic data captures transitional progenitor states in mouse skeletal muscle regeneration. Commun. Biol. 4, 1280. doi:10.1038/s42003-021-02810-x
Michele, D. E., and Campbell, K. P. (2003). Dystrophin-glycoprotein complex: Post-translational processing and dystroglycan function. J. Biol. Chem. 278, 15457–15460. doi:10.1074/jbc.R200031200
Moll, J., Barzaghi, P., Lin, S., Bezakova, G., Lochmuller, H., Engvall, E., et al. (2001). An agrin minigene rescues dystrophic symptoms in a mouse model for congenital muscular dystrophy. Nature 413, 302–307. doi:10.1038/35095054
Mouneimne, G., and Brugge, J. S. (2007). Tensins: A new switch in cell migration. Dev. Cell 13, 317–319. doi:10.1016/j.devcel.2007.08.010
Moyle, L. A., Cheng, R. Y., Liu, H., Davoudi, S., Ferreira, S. A., Nissar, A. A., et al. (2020). Three-dimensional niche stiffness synergizes with Wnt7a to modulate the extent of satellite cell symmetric self-renewal divisions. Mol. Biol. Cell 31, 1703–1713. doi:10.1091/mbc.E20-01-0078
Munoz, R., Moreno, M., Oliva, C., Orbenes, C., and Larrain, J. (2006). Syndecan-4 regulates non-canonical Wnt signalling and is essential for convergent and extension movements in Xenopus embryos. Nat. Cell Biol. 8, 492–500. doi:10.1038/ncb1399
Muntoni, F., Brockington, M., Godfrey, C., Ackroyd, M., Robb, S., Manzur, A., et al. (2007). Muscular dystrophies due to defective glycosylation of dystroglycan. Acta Myol. 26, 129–135.
Murphy, M. M., Lawson, J. A., Mathew, S. J., Hutcheson, D. A., and Kardon, G. (2011). Satellite cells, connective tissue fibroblasts and their interactions are crucial for muscle regeneration. Development 138, 3625–3637. doi:10.1242/dev.064162
Nakka, K., Hachmer, S., Mokhtari, Z., Kovac, R., Bandukwala, H., Bernard, C., et al. (2022). JMJD3 activated hyaluronan synthesis drives muscle regeneration in an inflammatory environment. Science 377, 666–669. doi:10.1126/science.abm9735
Naomi, R., Ridzuan, P. M., and Bahari, H. (2021). Current insights into collagen type I. Polym. (Basel) 13.
Nareyeck, G., Seidler, D. G., Troyer, D., Rauterberg, J., Kresse, H., and Schonherr, E. (2004). Differential interactions of decorin and decorin mutants with type I and type VI collagens. Eur. J. Biochem. 271, 3389–3398. doi:10.1111/j.1432-1033.2004.04273.x
Nonaka, I., Une, Y., Ishihara, T., Miyoshino, S., Nakashima, T., and Sugita, H. (1981). A clinical and histological study of Ullrich's disease (congenital atonic-sclerotic muscular dystrophy). Neuropediatrics 12, 197–208. doi:10.1055/s-2008-1059651
Nunes, A. M., Wuebbles, R. D., Sarathy, A., Fontelonga, T. M., Deries, M., Burkin, D. J., et al. (2017). Impaired fetal muscle development and JAK-STAT activation mark disease onset and progression in a mouse model for merosin-deficient congenital muscular dystrophy. Hum. Mol. Genet. 26, 2018–2033. doi:10.1093/hmg/ddx083
Oprescu, S. N., Yue, F., Qiu, J., Brito, L. F., and Kuang, S. (2020). Temporal dynamics and heterogeneity of cell populations during skeletal muscle regeneration. iScience 23, 100993. doi:10.1016/j.isci.2020.100993
Pan, T. C., Zhang, R. Z., Arita, M., Bogdanovich, S., Adams, S. M., Gara, S. K., et al. (2014). A mouse model for dominant collagen VI disorders: Heterozygous deletion of Col6a3 exon 16. J. Biol. Chem. 289, 10293–10307. doi:10.1074/jbc.M114.549311
Pasut, A., Jones, A. E., and Rudnicki, M. A. (2013). Isolation and culture of individual myofibers and their satellite cells from adult skeletal muscle. J. Vis. Exp., e50074. doi:10.3791/50074
Patton, B. L., Connoll, A. M., Martin, P. T., Cunningham, J. M., Mehta, S., Pestronk, A., et al. (1999). Distribution of ten laminin chains in dystrophic and regenerating muscles. Neuromuscul. Disord. 9, 423–433. doi:10.1016/s0960-8966(99)00033-4
Patton, B. L., Miner, J. H., Chiu, A. Y., and Sanes, J. R. (1997). Distribution and function of laminins in the neuromuscular system of developing, adult, and mutant mice. J. Cell Biol. 139, 1507–1521. doi:10.1083/jcb.139.6.1507
Pawlikowski, B., Vogler, T. O., Gadek, K., and Olwin, B. B. (2017). Regulation of skeletal muscle stem cells by fibroblast growth factors. Dev. Dyn. 246, 359–367. doi:10.1002/dvdy.24495
Pegoraro, E., Fanin, M., Trevisan, C. P., Angelini, C., and Hoffman, E. P. (2000). A novel laminin alpha2 isoform in severe laminin alpha2 deficient congenital muscular dystrophy. Neurology 55, 1128–1134. doi:10.1212/wnl.55.8.1128
Penton, C. M., Badarinarayana, V., Prisco, J., Powers, E., Pincus, M., Allen, R. E., et al. (2016). Laminin 521 maintains differentiation potential of mouse and human satellite cell-derived myoblasts during long-term culture expansion. Skelet. Muscle 6, 44. doi:10.1186/s13395-016-0116-4
Pham, T., Tyagi, A., Wang, Y. S., and Guo, J. (2021). Single-cell proteomic analysis. WIREs Mech. Dis. 13, e1503. doi:10.1002/wsbm.1503
Piersma, B., Hayward, M. K., and Weaver, V. M. (2020). Fibrosis and cancer: A strained relationship. Biochim. Biophys. Acta. Rev. Cancer 1873, 188356. doi:10.1016/j.bbcan.2020.188356
Pisconti, A., Cornelison, D. D., Olguin, H. C., Antwine, T. L., and Olwin, B. B. (2010). Syndecan-3 and Notch cooperate in regulating adult myogenesis. J. Cell Biol. 190, 427–441. doi:10.1083/jcb.201003081
Rafii, M. S., Hagiwara, H., Mercado, M. L., Seo, N. S., Xu, T., Dugan, T., et al. (2006). Biglycan binds to alpha- and gamma-sarcoglycan and regulates their expression during development. J. Cell. Physiol. 209, 439–447. doi:10.1002/jcp.20740
Rayagiri, S. S., Ranaldi, D., Raven, A., Mohamad Azhar, N. I. F., Lefebvre, O., Zammit, P. S., et al. (2018). Basal lamina remodeling at the skeletal muscle stem cell niche mediates stem cell self-renewal. Nat. Commun. 9, 1075. doi:10.1038/s41467-018-03425-3
Reinhard, J. R., Lin, S., Mckee, K. K., Meinen, S., Crosson, S. C., Sury, M., et al. (2017). Linker proteins restore basement membrane and correct LAMA2-related muscular dystrophy in mice. Sci. Transl. Med. 9, eaal4649. doi:10.1126/scitranslmed.aal4649
Reinhardt, D., Mann, K., Nischt, R., Fox, J. W., Chu, M. L., Krieg, T., et al. (1993). Mapping of nidogen binding sites for collagen type IV, heparan sulfate proteoglycan, and zinc. J. Biol. Chem. 268, 10881–10887. doi:10.1016/s0021-9258(18)82067-1
Ringelmann, B., Roder, C., Hallmann, R., Maley, M., Davies, M., Grounds, M., et al. (1999). Expression of laminin alpha1, alpha2, alpha4, and alpha5 chains, fibronectin, and tenascin-C in skeletal muscle of dystrophic 129ReJ dy/dy mice. Exp. Cell Res. 246, 165–182. doi:10.1006/excr.1998.4244
Ronning, S. B., Carlson, C. R., Aronsen, J. M., Pisconti, A., Host, V., Lunde, M., et al. (2020). Syndecan-4(-/-) mice have smaller muscle fibers, increased akt/mTOR/S6K1 and notch/HES-1 pathways, and alterations in extracellular matrix components. Front. Cell Dev. Biol. 8, 730. doi:10.3389/fcell.2020.00730
Rooney, J. E., Gurpur, P. B., and Burkin, D. J. (2009). Laminin-111 protein therapy prevents muscle disease in the mdx mouse model for Duchenne muscular dystrophy. Proc. Natl. Acad. Sci. U. S. A. 106, 7991–7996. doi:10.1073/pnas.0811599106
Rooney, J. E., Knapp, J. R., Hodges, B. L., Wuebbles, R. D., and Burkin, D. J. (2012). Laminin-111 protein therapy reduces muscle pathology and improves viability of a mouse model of merosin-deficient congenital muscular dystrophy. Am. J. Pathol. 180, 1593–1602. doi:10.1016/j.ajpath.2011.12.019
Rosenblatt, J. D., Lunt, A. I., Parry, D. J., and Partridge, T. A. (1995). Culturing satellite cells from living single muscle fiber explants. Vitro Cell. Dev. Biol. Anim. 31, 773–779. doi:10.1007/BF02634119
Rozo, M., Li, L., and Fan, C. M. (2016). Targeting β1-integrin signaling enhances regeneration in aged and dystrophic muscle in mice Nat. Med. 22, 889–896. doi:10.1038/nm.4116
Sandoval-Guzman, T., Wang, H., Khattak, S., Schuez, M., Roensch, K., Nacu, E., et al. (2014). Fundamental differences in dedifferentiation and stem cell recruitment during skeletal muscle regeneration in two salamander species. Cell Stem Cell 14, 174–187. doi:10.1016/j.stem.2013.11.007
Sanes, J. R., Engvall, E., Butkowski, R., and Hunter, D. D. (1990). Molecular heterogeneity of basal laminae: Isoforms of laminin and collagen IV at the neuromuscular junction and elsewhere. J. Cell Biol. 111, 1685–1699. doi:10.1083/jcb.111.4.1685
Sarkozy, A., Foley, A. R., Zambon, A. A., Bonnemann, C. G., and Muntoni, F. (2020). LAMA2-Related dystrophies: Clinical phenotypes, disease biomarkers, and clinical trial readiness. Front. Mol. Neurosci. 13, 123. doi:10.3389/fnmol.2020.00123
Sasaki, T., Yamada, H., Matsumura, K., Shimizu, T., Kobata, A., and Endo, T. (1998). Detection of O-mannosyl glycans in rabbit skeletal muscle alpha-dystroglycan. Biochim. Biophys. Acta 1425, 599–606. doi:10.1016/s0304-4165(98)00114-7
Scharner, J., and Zammit, P. S. (2011). The muscle satellite cell at 50: The formative years. Skelet. Muscle 1, 28. doi:10.1186/2044-5040-1-28
Schüler, S. C., Kirkpatrick, J. M., Schmidt, M., Santinha, D., Koch, P., Di Sanzo, S., et al. (2021). Extensive remodeling of the extracellular matrix during aging contributes to age-dependent impairments of muscle stem cell functionality. Cell Rep. 35, 109223. doi:10.1016/j.celrep.2021.109223
Seifert, A. W., and Muneoka, K. (2018). The blastema and epimorphic regeneration in mammals. Dev. Biol. 433, 190–199. doi:10.1016/j.ydbio.2017.08.007
Shao, X., Taha, I. N., Clauser, K. R., Gao, Y. T., and Naba, A. (2020). MatrisomeDB: The ECM-protein knowledge database. Nucleic Acids Res. 48, D1136-D1144–D1144. doi:10.1093/nar/gkz849
Shin, J., Mcfarland, D. C., and Velleman, S. G. (2012). Heparan sulfate proteoglycans, syndecan-4 and glypican-1, differentially regulate myogenic regulatory transcription factors and paired box 7 expression during Turkey satellite cell myogenesis: Implications for muscle growth. Poult. Sci. 91, 201–207. doi:10.3382/ps.2011-01695
Siegel, A. L., Atchison, K., Fisher, K. E., Davis, G. E., and Cornelison, D. D. (2009). 3D timelapse analysis of muscle satellite cell motility. Stem Cells 27, 2527–2538. doi:10.1002/stem.178
Simons, M., and Horowitz, A. (2001). Syndecan-4-mediated signalling. Cell. Signal. 13, 855–862. doi:10.1016/s0898-6568(01)00190-5
Singh, P., Carraher, C., and Schwarzbauer, J. E. (2010). Assembly of fibronectin extracellular matrix. Annu. Rev. Cell Dev. Biol. 26, 397–419. doi:10.1146/annurev-cellbio-100109-104020
Singhal, N., and Martin, P. T. (2011). Role of extracellular matrix proteins and their receptors in the development of the vertebrate neuromuscular junction. Dev. Neurobiol. 71, 982–1005. doi:10.1002/dneu.20953
Slimani, H., Charnaux, N., Mbemba, E., Saffar, L., Vassy, R., Vita, C., et al. (2003). Interaction of RANTES with syndecan-1 and syndecan-4 expressed by human primary macrophages. Biochim. Biophys. Acta 1617, 80–88. doi:10.1016/j.bbamem.2003.09.006
Smirnov, S. P., Mcdearmon, E. L., Li, S., Ervasti, J. M., Tryggvason, K., and Yurchenco, P. D. (2002). Contributions of the LG modules and furin processing to laminin-2 functions. J. Biol. Chem. 277, 18928–18937. doi:10.1074/jbc.M201880200
Stearns-Reider, K. M., D'amore, A., Beezhold, K., Rothrauff, B., Cavalli, L., Wagner, W. R., et al. (2017). Aging of the skeletal muscle extracellular matrix drives a stem cell fibrogenic conversion. Aging Cell 16, 518–528. doi:10.1111/acel.12578
Suzuki, N., Nakatsuka, H., Mochizuki, M., Nishi, N., Kadoya, Y., Utani, A., et al. (2003). Biological activities of homologous loop regions in the laminin alpha chain G domains. J. Biol. Chem. 278, 45697–45705. doi:10.1074/jbc.M304667200
Szabo, K., Varga, D., Vegh, A. G., Liu, N., Xiao, X., Xu, L., et al. (2022). Syndecan-4 affects myogenesis via Rac1-mediated actin remodeling and exhibits copy-number amplification and increased expression in human rhabdomyosarcoma tumors. Cell. Mol. Life Sci. 79, 122. doi:10.1007/s00018-021-04121-0
Takagi, J., and Springer, T. A. (2002). Integrin activation and structural rearrangement. Immunol. Rev. 186, 141–163. doi:10.1034/j.1600-065x.2002.18613.x
Takenaka-Ninagawa, N., Kim, J., Zhao, M., Sato, M., Jonouchi, T., Goto, M., et al. (2021). Collagen-VI supplementation by cell transplantation improves muscle regeneration in Ullrich congenital muscular dystrophy model mice. Stem Cell Res. Ther. 12, 446. doi:10.1186/s13287-021-02514-3
Talts, J. F., Andac, Z., Gohring, W., Brancaccio, A., and Timpl, R. (1999). Binding of the G domains of laminin alpha1 and alpha2 chains and perlecan to heparin, sulfatides, alpha-dystroglycan and several extracellular matrix proteins. EMBO J. 18, 863–870. doi:10.1093/emboj/18.4.863
Talts, J. F., Sasaki, T., Miosge, N., Gohring, W., Mann, K., Mayne, R., et al. (2000). Structural and functional analysis of the recombinant G domain of the laminin alpha4 chain and its proteolytic processing in tissues. J. Biol. Chem. 275, 35192–35199. doi:10.1074/jbc.M003261200
Tanaka, K. K., Hall, J. K., Troy, A. A., Cornelison, D. D., Majka, S. M., and Olwin, B. B. (2009). Syndecan-4-expressing muscle progenitor cells in the SP engraft as satellite cells during muscle regeneration. Cell Stem Cell 4, 217–225. doi:10.1016/j.stem.2009.01.016
Testa, S., Fornetti, E., Fuoco, C., Sanchez-Riera, C., Rizzo, F., Ciccotti, M., et al. (2021). The war after war: Volumetric muscle loss incidence, implication, current therapies and emerging reconstructive strategies, a comprehensive review. Biomedicines 9, 564. doi:10.3390/biomedicines9050564
Tierney, M. T., Gromova, A., Sesillo, F. B., Sala, D., Spenle, C., Orend, G., et al. (2016). Autonomous extracellular matrix remodeling controls a progressive adaptation in muscle stem cell regenerative capacity during development. Cell Rep. 14, 1940–1952. doi:10.1016/j.celrep.2016.01.072
Timpl, R., Rohde, H., Robey, P. G., Rennard, S. I., Foidart, J. M., and Martin, G. R. (1979). Laminin-a glycoprotein from basement membranes. J. Biol. Chem. 254, 9933–9937. doi:10.1016/s0021-9258(19)83607-4
Timpl, R., Tisi, D., Talts, J. F., Andac, Z., Sasaki, T., and Hohenester, E. (2000). Structure and function of laminin LG modules. Matrix Biol. 19, 309–317. doi:10.1016/s0945-053x(00)00072-x
Urciuolo, A., Quarta, M., Morbidoni, V., Gattazzo, F., Molon, S., Grumati, P., et al. (2013). Collagen VI regulates satellite cell self-renewal and muscle regeneration. Nat. Commun. 4, 1964. doi:10.1038/ncomms2964
Van Velthoven, C. T. J., De Morree, A., Egner, I. M., Brett, J. O., and Rando, T. A. (2017). Transcriptional profiling of quiescent muscle stem cells in vivo. Cell Rep. 21, 1994–2004. doi:10.1016/j.celrep.2017.10.037
Verma, M., Asakura, Y., Murakonda, B. S. R., Pengo, T., Latroche, C., Chazaud, B., et al. (2018). Muscle satellite cell cross-talk with a vascular niche maintains quiescence via VEGF and Notch signaling. Cell Stem Cell 23, 530–543. e539. doi:10.1016/j.stem.2018.09.007
Vial, C., Zuniga, L. M., Cabello-Verrugio, C., Canon, P., Fadic, R., and Brandan, E. (2008). Skeletal muscle cells express the profibrotic cytokine connective tissue growth factor (CTGF/CCN2), which induces their dedifferentiation. J. Cell. Physiol. 215, 410–421. doi:10.1002/jcp.21324
Villar, M. J., Hassell, J. R., and Brandan, E. (1999). Interaction of skeletal muscle cells with collagen type IV is mediated by perlecan associated with the cell surface. J. Cell. Biochem. 75, 665–674. doi:10.1002/(sici)1097-4644(19991215)75:4<665:aid-jcb12>3.0.co;2-s
Von Der Mark, H., Williams, I., Wendler, O., Sorokin, L., Von Der Mark, K., and Poschl, E. (2002). Alternative splice variants of alpha 7 beta 1 integrin selectively recognize different laminin isoforms. J. Biol. Chem. 277, 6012–6016. doi:10.1074/jbc.M102188200
Von Der Mark, K. (2006). “Chapter 1 - structure, biosynthesis and gene regulation of collagens in cartilage and bone,” in Dynamics of bone and cartilage metabolism. Editors M. J. Seibel, S. P. Robins, and J. P. Bilezikian. Second Edition (Burlington: Academic Press), 3–40.
Wang, Y. X., Holbrook, C. A., Hamilton, J. N., Garoussian, J., Afshar, M., Su, S., et al. (2022). A single cell spatial temporal atlas of skeletal muscle reveals cellular neighborhoods that orchestrate regeneration and become disrupted in aging. bioRxiv 2006. doi:10.1101/2022.06.10.494732
Webster, M. T., Manor, U., Lippincott-Schwartz, J., and Fan, C. M. (2016). Intravital imaging reveals ghost fibers as architectural units guiding myogenic progenitors during regeneration. Cell Stem Cell 18, 243–252. doi:10.1016/j.stem.2015.11.005
Wiberg, C., Heinegard, D., Wenglen, C., Timpl, R., and Morgelin, M. (2002). Biglycan organizes collagen VI into hexagonal-like networks resembling tissue structures. J. Biol. Chem. 277, 49120–49126. doi:10.1074/jbc.M206891200
Winder, S. J. (2001). The complexities of dystroglycan. Trends biochem. Sci. 26, 118–124. doi:10.1016/s0968-0004(00)01731-x
Winograd-Katz, S. E., Fassler, R., Geiger, B., and Legate, K. R. (2014). The integrin adhesome: From genes and proteins to human disease. Nat. Rev. Mol. Cell Biol. 15, 273–288. doi:10.1038/nrm3769
Wu, C., Chung, A. E., and Mcdonald, J. A. (1995). A novel role for alpha 3 beta 1 integrins in extracellular matrix assembly. J. Cell Sci. 108 (6), 2511–2523. doi:10.1242/jcs.108.6.2511
Xu, Z., Ichikawa, N., Kosaki, K., Yamada, Y., Sasaki, T., Sakai, L. Y., et al. (2010). Perlecan deficiency causes muscle hypertrophy, a decrease in myostatin expression, and changes in muscle fiber composition. Matrix Biol. 29, 461–470. doi:10.1016/j.matbio.2010.06.001
Yamaguchi, M., Watanabe, Y., Ohtani, T., Uezumi, A., Mikami, N., Nakamura, M., et al. (2015). Calcitonin receptor signaling inhibits muscle stem cells from escaping the quiescent state and the niche. Cell Rep. 13, 302–314. doi:10.1016/j.celrep.2015.08.083
Yap, L., Tay, H. G., Nguyen, M. T. X., Tjin, M. S., and Tryggvason, K. (2019). Laminins in cellular differentiation. Trends Cell Biol. 29, 987–1000. doi:10.1016/j.tcb.2019.10.001
Yoshimoto, Y., Ikemoto-Uezumi, M., Hitachi, K., Fukada, S. I., and Uezumi, A. (2020). Methods for accurate assessment of myofiber maturity during skeletal muscle regeneration. Front. Cell Dev. Biol. 8, 267. doi:10.3389/fcell.2020.00267
Yurchenco, P. D., and Ruben, G. C. (1987). Basement membrane structure in situ: Evidence for lateral associations in the type IV collagen network. J. Cell Biol. 105, 2559–2568. doi:10.1083/jcb.105.6.2559
Zaidel-Bar, R., Itzkovitz, S., Ma'ayan, A., Iyengar, R., and Geiger, B. (2007). Functional atlas of the integrin adhesome. Nat. Cell Biol. 9, 858–867. doi:10.1038/ncb0807-858
Zhang, K., and Chen, J. (2012). The regulation of integrin function by divalent cations. Cell adh. Migr. 6, 20–29. doi:10.4161/cam.18702
Zhang, Y. Z., Ran, L. Y., Li, C. Y., and Chen, X. L. (2015). Diversity, structures, and collagen-degrading mechanisms of bacterial collagenolytic proteases. Appl. Environ. Microbiol. 81, 6098–6107. doi:10.1128/AEM.00883-15
Zhao, A., Qin, H., and Fu, X. (2016). What determines the regenerative capacity in animals? BioScience 66, 735–746. doi:10.1093/biosci/biw079
Keywords: muscle stem cells, satellite cells, extracellular matrix, stem cell niche, skeletal muscle, MuSCs
Citation: Schüler SC, Liu Y, Dumontier S, Grandbois M, Le Moal E, Cornelison D and Bentzinger CF (2022) Extracellular matrix: Brick and mortar in the skeletal muscle stem cell niche. Front. Cell Dev. Biol. 10:1056523. doi: 10.3389/fcell.2022.1056523
Received: 28 September 2022; Accepted: 03 November 2022;
Published: 29 November 2022.
Edited by:
Yu Xin Wang, Stanford University, United StatesReviewed by:
Atsushi Asakura, University of Minnesota Twin Cities, United StatesChristopher M. Madl, Stanford University, United States
Copyright © 2022 Schüler, Liu, Dumontier, Grandbois, Le Moal, Cornelison and Bentzinger. This is an open-access article distributed under the terms of the Creative Commons Attribution License (CC BY). The use, distribution or reproduction in other forums is permitted, provided the original author(s) and the copyright owner(s) are credited and that the original publication in this journal is cited, in accordance with accepted academic practice. No use, distribution or reproduction is permitted which does not comply with these terms.
*Correspondence: C. Florian Bentzinger, Y2YuYmVudHppbmdlckB1c2hlcmJyb29rZS5jYQ==
†These authors have contributed equally to this work