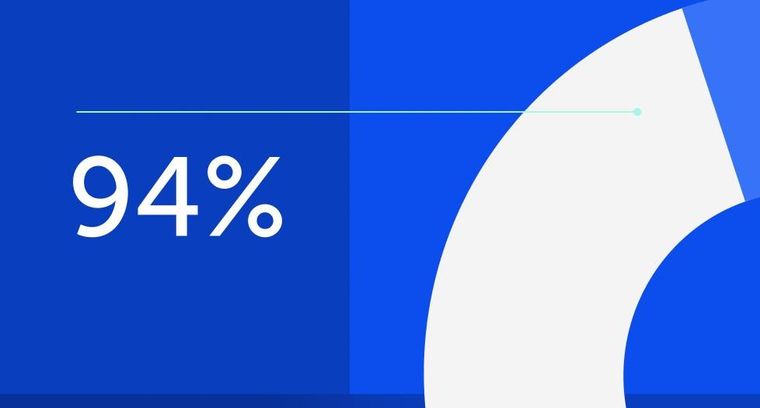
94% of researchers rate our articles as excellent or good
Learn more about the work of our research integrity team to safeguard the quality of each article we publish.
Find out more
REVIEW article
Front. Cell Dev. Biol., 01 March 2023
Sec. Stem Cell Research
Volume 10 - 2022 | https://doi.org/10.3389/fcell.2022.1047094
This article is part of the Research TopicCurrent Progress in Mesenchymal Stem/Stromal Cell Research, volume IIView all 8 articles
Mesenchymal stem cells (MSCs) are crucial for tissue homeostasis and repair, secreting vesicles to the extracellular environment. Isolated exosomes were shown to affect angiogenesis, immunomodulation and tissue regeneration. Numerous efforts have been dedicated to describe the mechanism of action of these extracellular vesicles (EVs) and guarantee their safety, since the final aim is their therapeutic application in the clinic. The major advantage of applying MSC-derived EVs is their low or inexistent immunogenicity, prompting their use as drug delivery or therapeutic agents, as well as wound healing, different cancer types, and inflammatory processes in the neurological and cardiovascular systems. MSC-derived EVs display no vascular obstruction effects or apparent adverse effects. Their nano-size ensures their passage through the blood–brain barrier, demonstrating no cytotoxic or immunogenic effects. Several in vitro tests have been conducted with EVs obtained from different sources to understand their biology, molecular content, signaling pathways, and mechanisms of action. Application of EVs to human therapies has recently become a reality, with clinical trials being conducted to treat Alzheimer’s disease, retina degeneration, and COVID-19 patients. Herein, we describe and compare the different extracellular vesicles isolation methods and therapeutic applications regarding the tissue repair and regeneration process, presenting the latest clinical trial reports.
Regenerative medicine has faced great challenges in the search for alternatives to ensure an effective treatment to accelerate the tissue regeneration process without altering its phases, especially for wound healing, in clinical, morphophysiological, and molecular environments (Lin et al., 2011; Choi et al., 2012; Waycaster et al., 2016). Instead of whole organ/tissue transplantation, a novel approach is cell therapy characterized by the use of cells with immunomodulatory properties as treatment. In order to do that, Mesenchymal/stromal stem cells have been used as an important resource for cell therapy being widely described for several applications, such as the treatment of wounds and ulcers of Diabetes mellitus (Cao et al., 2017; Di et al., 2017) and regeneration of several tissue types (Brett et al., 2017; Thangarajah et al., 2017), as an alternative for cancer treatment, and in studies of cancer biology (Gomes et al., 2017; Yao et al., 2017). Despite the accumulated knowledge about stem cell therapeutic applications, several mechanisms involved in the regenerative process have been unclear for a long time. Currently, some light has been shed on this problem, bringing attention to the extracellular components released by these cells. The discovery of extracellular vesicles (EVs) raised the question of whether these components would be the actual effectors responsible for MSC-mediated cell therapy (Akyurekli et al., 2015).
This recent finding drew the attention of the scientific community with several questions emerging: 1) what are these vesicles released by MSCs?; 2) what are the mechanisms of release of these vesicles?; 3) what are the characteristics of these vesicles and the molecular components of their content; and 4) what are the possible mechanisms of action of these secreted molecules and their therapeutic potential, among several other questions regarding EVs (Pankajakshan and Agrawal, 2014; Marote et al., 2016; Derkus et al., 2017).
EVs were first described in the 1980s as vesicles of endosomal origin, which were observed to be released during the maturation of reticulocytes, as a consequence of multi-vesicular endosome fusion to the plasma membrane (Johnstone et al., 1987). Recent studies report EVs as active participants in biological, regenerative, inflammatory, and pathological processes (De Toro et al., 2015; Wang B et al., 2019). Their molecular content has been the subject of investigation seeking to uncover the basis for their efficacy in several therapies. It is suggested that EVs play a fundamental role in intercellular communication, acting as bioindicators (An et al., 2015) or therapeutic agents, in addition to promoting a better understanding of pre-existent cell therapy (Phinney and Pittenger, 2017). Therefore, this review aims to highlight the most recent studies on EVs derived from different types of MSCs, discussing the different methodological approaches for their isolation and use in tissue regeneration processes.
Mesenchymal/stromal stem cells (MSCs) are multipotent non-hematopoietic stem cells, which are natural residents of adult tissues (Figure 1) and are involved in tissue homeostasis and recovery from injuries (Dominici et al., 2006; Katsuda and Ochiya, 2015). These cells can be obtained from many sources, such as the umbilical cord, peripheral blood, dental tissue, and liver. However, the most common and best characterized have been bone marrow and adipose tissue (Wang et al., 2020). According to Dominici et al. (2006) and the International Society for Stem Cell Therapy (ISSCR), MSCs are characterized by three main criteria: a) plastic adherence; b) expression of CD105, CD73, and CD90 and non-expression of CD45, CD34, CD14, CD19, and HLA class II; and c) differentiation into osteoblasts, chondroblasts, and adipocytes.
FIGURE 1. Mesenchymal stem cells (MSCs) could be isolated from adipose tissue, bone marrow, and different joints (especially to obtain chondrocytes). After the isolation method, they should be able to differentiate into adipocytes, osteoblasts, and chondrocytes. Several characteristics draw attention to studies with MSCs, but the most attractive is their self-renewal potential, as well as other types of stem cells, and the presence of a secretome with different molecules such as cytokines, growth factors, and extracellular vesicles. Image created on BioRender.
Their principal mechanism of action is through immunomodulation of innate and adaptative immune response interacting with T cells, B cells, natural killer (NK) cells, macrophages, and dendritic cells and by paracrine activity via secretome (Song N et al., 2021). Also, immune suppression of MSCs is influenced by the balance of their own secretion with the systemic and local environment inflammatory cytokines (Ankrum et al., 2014). Due to their immune tolerance, application of allogeneic or autologous MSCs has been widespread in clinical trials (Samsonraj et al., 2017). Together with the ability of MSCs to differentiate into other cell types ex vivo, much evidence is available on their therapeutic potential (Toh et al., 2016).
The ability to secrete soluble factors exerting paracrine activity in the microenvironment exemplifies the beneficial effects of MSCs in tissue repair and regeneration (Abreu et al., 2016). Among the mediators released by their secretome are cytokines, microRNAs (miRNA), growth factors, and EVs (Raposo and Stoorvogel, 2013).
MSCs have already been used in different lung diseases, cardiovascular repair, cancer treatment, immunological disease, spinal cord injury, and bone and cartilage replacement. Recent clinical trial application of these cells can be found in Table 3.
EVs include a broad spectrum of vesicles secreted by various cell types, originating from different body fluids (Table 1). This name is also used as a general term which includes: exosomes, ectosomes, oncosomes, release vesicles, apoptotic bodies, and microvesicles (Figure 2) (Choi and Lee, 2016; Elahi et al., 2019). To date, the International Society of Extracellular Vesicles (ISEV) recommends the application of EVs as an operational term and suggests classifications according to size (small, medium, or large EVs), biochemical composition (CD63+ EVs/CD81+ EVs) or conditions, and cell origin (apoptotic bodies and hypoxic EVs) (Théry et al., 2018).
TABLE 1. Different sources used to isolate EVs, methods of isolation, and effectiveness of each project.
FIGURE 2. Extracellular vesicles are a generic name for many kinds of vesicles released by cells; their origin and size are determinants in their classification. Exosomes present size between 30 and 120 nm, and an endosome being initially formed in cell cytosol from the early endosomes until late endosome release by the influence of specific proteins such as ESCRT and Rab family and could be excreted by any type of cells, including MSCs, tumor cells, and, even, immune cells. Microvesicles could present size within the range of 100–1,000 nm and could be released by any cells, whereas apoptotic bodies are the biggest group, excreted only for apoptotic cells. Image created on BioRender.
Since it was noticed that extracellular nanoparticles have clinical potential due to their cargoes, several discoveries occurred in this field. Recently, in addition to the classical EVs, two other nanovesicle groups lacking a lipid bilayer membrane have been reported: exomeres and supermeres. Special attention was given to the supermeres because they appear to be enriched in proteins and other molecules (Zhang et al., 2021).
Small extracellular vesicles (sEVs), which includes the well-known exosomes, are the most studied vesicle group, may be found in body fluids and cell-derived conditioned media, vary between 40 and 150 nm, and arise from the fusion of endosomal multi-vesicular bodies with the plasma membrane released by exocytosis by several cell types (Raposo and Stoorvogel, 2013; Ramirez et al., 2018). The internal composition of sEVs includes lipids; nucleic acids, such as messenger RNAs (mRNA), miRNA, and non-coding RNAs (ncRNA); and proteins (CD9, CD63, and CD81) (Théry et al., 2006; Colombo et al., 2014).
sEVs may be obtained from MSC conditioned culture media or body fluids (Table 1) for studies of their biogenesis, composition, and mechanisms of action. Ultracentrifugation is generally used for sEV concentration in a sucrose density gradient, whereas polymer precipitation is widely used to produce large quantities of EVs (Table 2). Assays that lead to co-precipitation of these vesicles with soluble proteins and supplemental chemical methods are also used to isolate EVs from conditioned media. Immunoaffinity methods and sEV extraction kits have also been employed to enable the isolation of these EVs in a shorter period of time (Raposo and Stoorvogel, 2013; Schageman et al., 2013; Rocco et al., 2016; Sarvar et al., 2016; Silva et al., 2016). Each method presents different advantages that should be evaluated according to application, prioritizing processing time, purity, cost, ease of handling process, or scalability. However, their structure and content should be preserved.
TABLE 2. Different kinds of MSCs used to isolate sEVs with different applications with details of the sEV isolation method and results of each work.
Numerous techniques have been used to describe the morphology and evaluate the molecular content of sEVs. The focus has been to gather the largest amount of information about their biogenesis and mechanism of action. In addittion, protocols need to be standardized to illustrate their interaction with the target cells and their activity in vitro and in vivo. Among the most widely used techniques are transmission electron microscopy (TEM), proteomics, lipidomics, genomics, metabolomics, RNA sequencing, nanoparticle tracking analysis (NTA), immunological labeling, and classification by flow cytometry (Feng et al., 2010; Vallabhaneni et al., 2014; Anderson et al., 2016; Greening et al., 2016; Silva et al., 2016).
In pairwise proteomic and lipidomic comparative studies of EV from normal (bone marrow mesenchymal stem cells) and transformed cells (U87-MG human glioblastoma cells, Huh7 hepatocellular carcinoma cells) identified different expression profile of proteins and lipids between normal and tumor cells (Haraszti et al., 2016). Gene ontology terms related to vesicles and membrane-associated proteins, GTPases, translation, and glycolytic pathways were significantly enriched features of EVs contents. Concerning the lipid content, while sEV were rich in glycolipids and free fatty acids, medium EV displayed larger amounts of ceramides and sphingomyelins (Haraszti et al., 2016).
The specific set of molecules present on the surface and in the interior of the sEV, may enable cell targeting at various levels, mediating intercellular signaling and resulting in regulation of embryonic tissue pattern remodeling and homeostasis, as well as disease progression (Vyas and Dhawan, 2017). Therefore, the molecular composition of sEV has been widely studied, using numerous methodological approaches due to the concrete possibility of using them as diagnostic biomarkers for various diseases and as drug delivery agents and therapeutic RNAs (Lv et al., 2015; Deng and Miller, 2019). Also, studies have shown that MSC-derived EVs display similar composition and function compared to the cells they derive from, being able to exert therapeutic activity, thereby opening new avenues for cell-free therapy (Bollini et al., 2013; Zhang B et al., 2016). sEVs have been successfully employed as a cell-free therapy due to their small size, which facilitates their storage, with no embolus formation concerns, in addittion to being poorly or no immunogenic and reducing the concern of tumor formation upon intravenous application because no cells are involved (Rezakhani et al., 2021).
sEV have been responsible for the exchange of information among cells through various interaction mechanisms. Their bilipid membrane allows greater versatility of extracellular contact by receptor–ligand interactions (van Niel et al., 2018).
These interactions may occur through direct contact between proteins on the surface of the exosomes and signaling receptors on the target cell, as exemplified by the discovery of Purushothaman et al. (2016). Fibronectin on the surface of myosomal cell-derived exosomes facilitated the interaction of target cells in a mechanism where heparan sulfate proteoglycans are present in the exosomes and the target cells, appearing to play a dual role, allowing for fibronectin capture and reception.
A study showed that exosomes display on their surfaces support for binding and activation of bioactive proteins, as described in exosomes derived from human mast cells, which present the active and the latent forms of transforming growth factor-β1 (TGF-β1). TGF-β1 is transferred to MSCs and retained in endosomal compartments, being activated by the action of proteoglycans, heparinase-II, and pH-sensitive elements, resulting in prolonged signaling and migratory phenotype in the receptor cells (Shelke et al., 2019).
sEVs can also fuse with the target cell membrane or be endocytosed, allowing them to deliver all of their intra-vesicular content to the cell, acting as paracrine effectors (Toh et al., 2016). Upon endocytosis, sEVs are internalized by the target cells and then fused to endosomes, which can then undergo transcytosis, moving the sEV through the fused endosomes, where they may mature into lysosomes to be degraded (Mulcahy et al., 2014; Zhang et al., 2015). This mechanism was exemplified by Kita et al. (2019), with sEV from MSCs that migrate into ischemic areas and fuse themselves with neurons, thereby promoting neurogenesis and angiogenesis after stroke damage, resulting in relieving inflammation and lesions. Another example was reported by Feng et al. (2010) in which sEV from K562 human erythroleukemia cells and MT4 human T-lymphotropic virus type-1 (HTLV) transformed leukemia T cells could interact with different cells in two distinct manners: efficient internalization by phagocytic cells and by non-phagocytic cells. However, most sEVs remained anchored to the cell membrane, and the few intracellular sEVs were involved by cell extensions and large phagosomes and also co-localized with phagolysosome markers, indicating that they would be drawn into phagolysosomes.
MSC sEV can load and transfer their charge to parenchyma cells facilitating cerebral plasticity and functional recovery from stroke, acting as the main paracrine effectors, targeting specific modifications in miRNAs, aiming to modulation of therapeutic responses (Xin et al., 2014).
After administration, MSC-derived sEV from induced human pluripotent cells were able to fuse with target hepatocytes and increase the activity of the sphingosine kinase signaling pathway and the levels of sphingosine-1-phosphate, which is directly related to the protective and proliferative effects of these sEV. This mechanism may contribute to hepatic regeneration in a murine model of liver ischemia and reperfusion injury (Du et al., 2017).
Another widely used treatment approach is the application of platelet-rich plasma (PRP), which has been considered effective due to the presence of numerous growth factors and other bioactive molecules responsible for promoting tissue repair (Hara et al., 2016) in processes such as chronic wounds (Ostvar et al., 2015; Spanò et al., 2016) and burns (Marck et al., 2016). However, this technique is not officially standardized, and little is known about the mechanism(s) underlying PRP application (Pavlovic et al., 2016). In this sense, the discovery of platelet-derived sEV that can easily be obtained from the blood has raised great interest because they play key roles in angiogenic and proliferative processes in tissue regeneration, becoming good candidates to replace PRP or even being the “next-generation PRP” (Tao et al., 2017b) with low immunogenic potential. Platelet-derived EVs have also been chosen because they constitute most of the vesicles present in the bloodstream, allowing studies exploring their role in hemostasis, pro-coagulant activity, and their participation as biomarkers in pathologies associated with thromboembolic events, such as atherosclerosis (Goetzl et al., 2017; Tripisciano et al., 2017).
Several stimuli need to accompany tissue regeneration that is subdivided into three phases: inflammation, repair, and remodeling (Stroncek and Reichert, 2008). All these steps involve four molecular processes: a) attenuation of apoptosis, helping to prevent extensive cell loss; b) inflammation controlled by modulation of the immune system; c) migration of endothelial cells to promote angiogenesis; and d) repopulation of lost cell types through cell proliferation and differentiation. Generally, the injury process activates tissue cells, including epithelial, stromal, and resident immune cells, which induce the recruitment of other circulating immune cells, stimulating cytokine and growth factor secretion and initiating the inflammatory response. Then, phagocytosis and the removal of foreign and pathogenic bodies from the site of injury will occur. After the cleansing of dead and infected cells, the proliferative phase is reached. This phase is characterized by cell proliferation and migration to the lesion site, new deposition of the extracellular matrix, angiogenesis, formation of granular tissue, and re-epithelization. Subsequently, the remodeling phase starts, being characterized by extracellular matrix remodelling and scar maturation. The effectiveness of MSC-derived EVs has been reported at each one of these phases (Clark et al., 2007; Gurtner et al., 2008; Choi et al., 2012; Silva et al., 2016; Sorg et al., 2017; Wu et al., 2018). Also, utilization of the mesenchymal stem-cell secretome can positively influence the injured tissue by modulating the local microenvironment, providing cytoprotective, anti-inflammatory, and angiogenic effects during the acute regenerative phase, either by attenuating or by inducing the action of pro-inflammatory cytokines (Zhang et al., 2014), boosting resident progenitors/stem cells in place to achieve a more tissue-specific programmed repair and instructing the stimulation of resident endogenous progenitors (Bollini et al., 2013). In addition, cleansing enzymes are biochemically active in the proteome of MSC sEV associated with the restoration of homeostasis for the most important activities in the tissue environment, constituting an interesting mechanism to be targeted for future development of paracrine pharmacological therapy (Toh et al., 2016). Despite the need for further studies, the advantage of low or no immunogenicity displayed by MSC-derived sEV influences their use as drug delivery, therapeutic agents in vaccination, cancer biomarkers and in inflammatory disorders (Robbins and Morelli, 2014) of both the nervous (Lv et al., 2015) or cardiovascular system (Pankajakshan and Agrawal, 2014), and in wound healing (Geiger et al., 2015; Elahi et al., 2019). Considering that, 19 studies were found at the Clinicaltrials.gov (Table 3) website upon searching for “MSC+exosome” (https://clinicaltrials.gov/ct2/results?cond=&term=MSC+exosomes&cntry=&state=&city=&dist=), several focused on exploring their efficiency in tissue regeneration in diseases such as epidermolysis bullosa, acute ischemic stroke, and degenerative meniscal injury. Others aim to test the therapeutic effects of MSC-derived sEV in Alzheimer’s disease and COVID-19-associated pneumonia (Accessed on 20 July 2022, at 15:20 p.m.).
TABLE 3. Active clinical trials undertaken with mesenchymal stem cell-derived Extracellular vesicles in different disease treatments.
A study with sEV derived from human placental MSCs pointed out that they can stimulate the transcriptional activity of Oct4 and Nanog in dermal fibroblasts. Then, by their incorporation, stem cells’ plasticity for differentiation into adipogenic and osteogenic lineages was considerably increased (Tooi et al., 2015). The pro-angiogenic capacity of sEV from the human placenta was also described in vitro using the ischemic lesion model of the murine atria, in which increased levels and migration of endothelial tubes were observed along with increased expression of genes related to angiogenesis (Komaki et al., 2017). Upon evaluating the therapeutic potential of human fibrocyte-derived sEV, a population of bone marrow-derived mesenchymal progenitors’ cells, sequentially stimulated with platelet-derived growth factor-BB (PDGF-BB) and TGF-β1 growth factors and in the presence of fibroblast growth factor (FGF), presented pro-angiogenic properties in vitro, induction of keratinocytes migration and proliferation, and an accelerated healing process in type 2 diabetic mice (Geiger et al., 2015).
Another interesting property of these vesicles is their ability to be internalized by human umbilical vein endothelial cells (HUVECs) in vitro, increasing their proliferation and migration, increasing their proloferation and migration accelerating the healing process of cutaneous wounds on the dorsal region of diabetic rats by stimulating angiogenesis through activation of the extracellular signal-regulated kinases 1/2 (Erk-1/2) signaling pathway, contributing to the discovery of specific pathways, which are activated during cutaneous regeneration (Zhang J et al., 2016).
Acellular derivatives from bone marrow MSCs were described to accelerate wound closure by re-epithelialization, dermo-epidermal junction, regeneration of skin appendages, inflammatory infiltration, vascularization, and formation of granular tissue and higher density collagen fibers in diabetic mice, in addition to concentrate factors and proteins relevant to regeneration, compared to only bone marrow MSCs (de Mayo et al., 2017). Therefore, the ability to migrate to the site of injury and promote fibroblast migration and proliferation, together with collagen synthesis, has been described for human adipose tissue-derived MSC sEVs, contributing to the skin healing process in mice (Hu et al., 2016), as well as skin flap transplants through neovascularization and protection against ischemia/reperfusion, in addition to stimulating lesion recovery by increasing the interleukin- (IL-) 6 levels (Pu et al., 2017). Moreover, MSC-derived EVs from induced human pluripotent cells (iPSCs) could accelerate the healing process of cutaneous wounds in rats by stimulating the proliferation and migration of human fibroblasts motility, leading to increased collagen and elastin synthesis and stimulating blood capillary networks formation in vitro (Zhang et al., 2015b). This process could be regulated by different mechanisms. For example, fetal dermal MSC-derived EVs could promote cutaneous wound healing in vivo and in vitro by activating fibroblast motility and secretion ability via the activation of the Notch signaling pathway (Wang X et al., 2019). sEVs derived from synovial MSCs presented the potential to stimulate cell proliferation and migration and maintain the synthesis of extracellular matrix proteins, in addition to delaying the progression of osteoarthritis in an animal model of knee joint cartilage injury through miR-140-5p (Tao et al., 2017a).
The wound healing process requires oxygen to promote cytokine interactions and activation of cell proliferation (Han and Ceilley, 2017). sEVs derived from bone marrow MSCs displayed rapid degradation of the hypoxia-inducible factor 1α gene (HIF-1α) under normoxic conditions. This effect is responsible for attenuating avascular necrosis of the steroid-induced femoral head in rabbits by promoting angiogenesis and accelerating bone regeneration, indicated by trabecular reconstruction and microvascular density (Li H. et al., 2017). The regeneration potential of EVs goes beyond wound repair. sEVs derived from human embryonic MSC demonstrated significant potential to reduce apoptotic rates and promote the proliferation of cells together with osteochondral differentiation (Zhang et al., 2018). Similar results were obtained using sEVs derived from human umbilical cord MSCs in an Alzheimer’s disease culture model. Results showed a high level of neuronal apoptosis via exosomal miR-223 targeting the PTEN-PI3K/Akt pathway, and this study demonstrated that EV derived from Alzheimer’s patients was capable of stimulating morphological changes, cell number reduction, and shortened synapses (Wei H et al., 2020).
Different studies have searched for a faster lung injury therapy aiming to relieve the idiopathic pulmonary fibrosis (IPF) condition, and during the COVID-19 pandemic, a race against time started aiming for alternative therapies for acute pneumonia from coronavirus infection. Thus, the inhalation of lung spheroid secretome derived from hypoxic sEV could attenuate the fibrosis scenario, decreasing collagen accumulation and myofibroblast proliferation (Dinh et al., 2020).
In injury, resident skin cells are exposed to multiple danger signals known as damage-associated molecular patterns (DAMPs) and pathogen-associated molecular patterns (PAMPs) that will be recognized by the immune system, starting an inflammatory process (Landén et al., 2016). An interesting recently evaluated mechanism was that mouse adipose mesenchymal stem cells (ASCs) display the potential to polarize macrophages from the M1 to M2 phenotype via miR-21 secretion targeting the PI3K/Akt pathway, directly influencing the pro-angiogenic effects of these EVs (Zhu et al., 2020). Thus, sEVs derived from bone marrow MSCs induced macrophage M2 polarization via transfer of miR-223, accelerating the wound healing process (He et al., 2019). This mechanism was recently reported by Zhang et al., (2018), describing that MSC sEV treatment enhances the M2-macrophage infiltration and maintains this effect in cartilage. In contrast, M1 macrophage levels and inflammation-associated cytokines, such as IL-6 and tumor necrosis factor-α (TNF-α), were lower after EV treatment (Zhang et al., 2018). Such evidence highlight sEV microRNAs as a possible therapeutic target for tissue repair. In addition, human bone marrow MSC-derived sEVs could educate macrophages, resulting in an M2-like phenotype, which was then used to promote tendon healing in an Achilles tendon injury in vivo model via modulation of tissue repair and inflammation (Chamberlain et al., 2019). Additionally, accelerating wound healing without scar formation is the target of many studies aiming at specific components that sEVs could present. By analyzing sEVs from umbilical cord MSCs, specific miRNAs were observed, such as miR21, miR23a, miR125b, and miR145. Evidence has shown that they can play a key role in suppressing the formation of myofibroblasts and scars in in vitro and in vivo models by inhibiting the transforming growth factor-β2 (TGF-β2) and the SMAD2 pathway in mice (Fang et al., 2016). This suggests an alternative pathway to cell therapy by administering modified EVs with transfected miRNAs in wounds, preventing scar formation.
The most common COVID-19 symptom was lung injury produced by high levels of pro-inflammatory cytokines, such as TNF-α, granulocyte colony-stimulating factor (G-CSF), monocyte chemoattractant protein 1 (MCP-1), interferon- γ inducible protein 10 (IP10), IL-6, and IL-7 (Gupta et al., 2020; Jayaramayya et al., 2020). MSCs’ potential to stimulate the immune system by cytokine release and differentiation into other cell phenotypes called attention during the COVID-19 pandemic. Considering all that was previously discussed, human blood samples were analyzed, demonstrating elevated ACE2+ EV levels in COVID-19 patients with an even-higher rate in acute-inflammatory phase patients. Together, the infection inhibition potentials of ACE2+-EVs and ACE2−-EVs were compared, concluding that virus infection was blocked in the presence of ACE2−-EVs (El-Shennawy et al., 2022). Considering the variants tested, ACE2+EV can neutralize their infection, supporting their potential as an antiviral mechanism (El-Shennawy et al., 2022). Similar effects were demonstrated by comparing EV-ACE2 with soluble ACE (Cocozza et al., 2020).
Also, the potential of EVs was shown in neurological pathologies. Human exfoliated deciduous teeth (SHED) derived-sEVs were used as an alternative for the treatment of rat traumatic brain injury, reducing the neuro-inflammation resulting from the change in polarization of the microglia. In the end, sEV could improve motor function and reduce the cortical lesion in the in vivo test (Li Y. et al., 2017).
This review highlights the high therapeutic potential of MSC-derived extracellular vesicles, which have been of growing interest as a promising tool for cell-free therapy. The standardization of extracellular vesicle isolation is already a reality with great improvements in recent years. However, the literature is very poor regarding fundamental information, such as the initial and final number of cells used to obtain EV, the exact content of EV and their respective protein amount. Moreover, most of the time, there is a lack of details about the methodology used for analysis. These drawbacks result in standardization issues and the impossibility of conducting comparative tests among different applications, hampering trials/ reprodutibility worldwide.
The action of these vesicles is demonstrated at all stages of the regenerative process, mediating cell migration and proliferation and stimulating angiogenesis in newly formed tissues until the recruitment of new cells. Despite that and their low immunogenic potential, compared to their host cells, further studies are needed to validate their efficacy and safety before employing them in clinical applications, as already occurring in different clinical trials shown previously. Issues such as the composition of EVs still need to be evaluated: what frequency should be used and the exact amount of EVs required in each application still require a rigorous investigation.
Moreover, considering their punctual action as an immunomodulatory molecule, the mechanism involved in EVs on tissue regeneration must be deeply investigated to avoid any adverse reaction that could harm the patients’ health after treatment. Taking this into account, a wide spectrum of questions is open to investigate and map which EV components act in each step of the regenerative process. The future perspective lies in strategically step into explore specific components of EVs cargo to modulate the body’s response for efficient tissue regeneration through target cell-free therapy.
MSC EVs have shown great potential since they appear to have the same beneficial characteristics of cells application, such as the release of cytokines and growth factors. They are particularly used in tissue regeneration tests due to a vast number of reports in the last year uncovering a great deal of information on their biogenesis, isolation, and characterization processes. Currently, with the COVID-19 pandemic and the requirement for faster therapies, their application has been widely explored for coronavirus pneumonia and other diseases, such as tissue repair. This can be noted in the increasing number of clinical trials demonstrating that MSC-sEV present potential for tissue regeneration. Despite this progress, further studies in the field are needed to clarify misunderstood questions such as mechanisms of action and exactly which molecules participate in the regenerative process to provide a safe therapy approach.
The study was designed by AC and written by BA and LF, who performed the literature analysis. PF, MS, and AC provided conceptual and technical guidance for all aspects of the study, and all authors reviewed and commented on the manuscript.
This study was funded by the following Brazilian Federal and State research agencies: Coordination for Improvement of Higher Education Personnel (CAPES) (Grant no. 88882.327814/2019-01 (Ph.D. fellowship to BA)); Brazilian Bank for Social and Economic Development (BNDES) (Grant no. 09.2.1066.1); Coordination for Improvement of Higher Education Personnel (CAPES) (Grant nos. 88881.068070/2014-01 and 88882.315613/2019-01 (postdoctoral fellowship to AC)); Sāo Paulo State Research Foundation (FAPESP)–Grant no. 2016/05311-2 (Thematic Project); National Council for Scientific and Technological Development (CNPq) (Grant no. 304068/2019-5 (Productivity Award to MS) and 401430/2013-8, 457601/2013-2, 409960/2013-6, 426896/2016-5, and 465656/2014-5); Brazilian Federal Agency for Studies and Projects (FINEP) (Grant nos. 01.08.0622.05, 51634-1AD, and 01.08.0484.00); Brazilian Science and Technology Ministry (MCTI); and the Health Ministry (MS-DECIT).
We thank the Faculty of Veterinary Medicine and Animal Science (FMVZ) and the Cell and Molecular Therapy Group—NUCEL (https://www.usp.br/nucel) of the School of Medicine of the University of São Paulo for providing the infrastructure to generate this review article.
The authors declare that the research was conducted in the absence of any commercial or financial relationships that could be construed as a potential conflict of interest.
All claims expressed in this article are solely those of the authors and do not necessarily represent those of their affiliated organizations or those of the publisher, the editors, and the reviewers. Any product that may be evaluated in this article, or claim that may be made by its manufacturer, is not guaranteed or endorsed by the publisher.
MSCs, Mesenchymal/stromal stem cells; EVs, extracellular vesicles; mRNA, messenger RNA; miRNA, microRNA; ncRNA, non-coding RNA; TEM, transmission electron microscopy; NTA, nanoparticle tracking analysis; TGF-β1, transforming growth factor-β1; HTLV1, human T-lymphotropic virus type-1; TNF-α, tumor necrosis factor-α; IL-6, interleukin-6; IF-1α, hypoxia factor-1α; PDGF-BB, platelet-derived growth factor-BB; FGF, fibroblast growth factor; ASCs, adipose mesenchymal stem cells; TGF-β2, transforming growth factor; GCSF, granulocyte colony-stimulating factor; MCP1, monocyte chemoattractant protein-1; IP10, interferon- γ inducible protein 10; IL-7, interleukin-7; Erk-1, extracellular signal-regulated kinases-1; SHED, human exfoliated deciduous teeth; SHED-Exo, human exfoliated deciduous teeth-derived exosomes; PRP, platelet-rich plasma; P-exos, platelet-derived exosomes; sEVs, small extracellular vesicles.
Abreu, S. C., Weiss, D. J., and Rocco, P. R. M. (2016). Extracellular vesicles derived from mesenchymal stromal cells: a therapeutic option in respiratory diseases? Stem Cell Res. Ther. 7, 53. doi:10.1186/s13287-016-0317-0
Akyurekli, C., Le, Y., Richardson, R. B., Fergusson, D., Tay, J., and Allan, D. S. (2015). A systematic review of preclinical studies on the therapeutic potential of mesenchymal stromal cell-derived microvesicles. Stem Cell Rev. Rep. 11, 150–160. doi:10.1007/s12015-014-9545-9
An, T., Qin, S., Xu, Y., Tang, Y., Huang, Y., Situ, B., et al. (2015). Exosomes serve as tumour markers for personalized diagnostics owing to their important role in cancer metastasis. J. Extracell. Vesicles 4, 27522–27615. doi:10.3402/jev.v4.27522
Anderson, J. D., Johansson, H. J., Graham, C. S., Vesterlund, M., Pham, M. T., Bramlett, C. S., et al. (2016). Comprehensive proteomic analysis of mesenchymal stem cell exosomes reveals modulation of angiogenesis via nuclear factor-kappaB signaling. Stem Cells 34, 601–613. doi:10.1002/stem.2298
Ankrum, J. A., Ong, J. F., and Karp, J. M. (2014). Mesenchymal stem cells: immune evasive, not immune privileged. Nat. Biotechnol. 32, 252–260. doi:10.1038/NBT.2816
Baranyai, T., Herczeg, K., Onódi, Z., Voszka, I., Módos, K., Marton, N., et al. (2015). Isolation of exosomes from blood plasma: Qualitative and quantitative comparison of ultracentrifugation and size exclusion chromatography methods. PLoS One 10, e0145686. doi:10.1371/journal.pone.0145686
Böing, A. N., Pol, E., Grootemaat, A. E., Coumans, F. a., Sturk, A., and Nieuwland, R. (2014). Single-step isolation of extracellular vesicles from plasma by size-exclusion chromatography. Int. Meet. ISEV Rotterdam 3, 118. doi:10.3402/jev.v3.23430
Bollini, S., Gentili, C., Tasso, R., and Cancedda, R. (2013). The regenerative role of the fetal and adult stem cell secretome. J. Clin. Med. 2, 302–327. doi:10.3390/jcm2040302
Brett, E., Chung, N., Leavitt, W. T., Momeni, A., Longaker, M. T., and Wan, D. C. (2017). A review of cell-based strategies for soft tissue reconstruction. Tissue Eng. Part B Rev. 23, 336–346. doi:10.1089/ten.teb.2016.0455
Cao, Y., Gang, X., Sun, C., and Wang, G. (2017). Mesenchymal stem cells improve healing of diabetic foot ulcer. J. Diabetes Res. 2017, 9328347–9328410. doi:10.1155/2017/9328347
Cao, J., Wang, B., Tang, T., Lv, L., Ding, Z., Li, Z., et al. (2020). Three-dimensional culture of MSCs produces exosomes with improved yield and enhanced therapeutic efficacy for cisplatin-induced acute kidney injury. Stem Cell Res. Ther. 11, 206. doi:10.1186/S13287-020-01719-2
Chamberlain, C. S., Clements, A. E. B., Kink, J. A., Choi, U., Baer, G. S., Halanski, M. A., et al. (2019). Extracellular vesicle-educated macrophages promote early Achilles tendon healing. Stem Cells 37, 652–662. doi:10.1002/stem.2988
Choi, H., and Lee, D. S. (2016). Illuminating the physiology of extracellular vesicles. Stem Cell Res. Ther. 7, 55–57. doi:10.1186/s13287-016-0316-1
Choi, J. S., Kim, J. D., Yoon, H. S., and Cho, Y. W. (2012). Full-thickness skin wound healing using human placenta-derived extracellular matrix containing bioactive molecules. Tissue Eng. Part A 19, 329–339. doi:10.1089/ten.tea.2011.0738
Clark, R. A. F., Ghosh, K., and Tonnesen, M. G. (2007). Tissue engineering for cutaneous wounds. J. Invest. Dermatol. 127, 1018–1029. doi:10.1038/sj.jid.5700715
Cocozza, F., Névo, N., Piovesana, E., Lahaye, X., Buchrieser, J., Schwartz, O., et al. (2020). Extracellular vesicles containing ACE2 efficiently prevent infection by SARS-CoV-2 Spike protein-containing virus. J. Extracell. Vesicles 10, e12050. doi:10.1002/JEV2.12050
Colombo, M., Raposo, G., and Théry, C. (2014). Biogenesis, secretion, and intercellular interactions of exosomes and other extracellular vesicles. Annu. Rev. Cell Dev. Biol. 30, 255–289. doi:10.1146/annurev-cellbio-101512-122326
Corso, G., Mäger, I., Lee, Y., Görgens, A., Bultema, J., Giebel, B., et al. (2017). Reproducible and scalable purification of extracellular vesicles using combined bind-elute and size exclusion chromatography. Sci. Rep. 7, 11561–11610. doi:10.1038/s41598-017-10646-x
de Mayo, T., Conget, P., Becerra-Bayona, S., Sossa, C. L., Galvis, V., and Arango-Rodríguez, M. L. (2017). The role of bone marrow mesenchymal stromal cell derivatives in skin wound healing in diabetic mice. PLoS One 12, e0177533. doi:10.1371/journal.pone.0177533
De Toro, J., Herschlik, L., Waldner, C., and Mongini, C. (2015). Emerging roles of exosomes in normal and pathological conditions: New insights for diagnosis and therapeutic applications. Front. Immunol. 6, 203. doi:10.3389/fimmu.2015.00203
Deng, F., and Miller, J. (2019). A review on protein markers of exosome from different bio-resources and the antibodies used for characterization. J. Histotechnol. 42, 226–239. doi:10.1080/01478885.2019.1646984
Derkus, B., Emregul, K. C., and Emregul, E. (2017). A new approach in stem cell research — exosomes : Their mechanism of action via cellular pathways. Cell Biol. Int. 41, 466–475. doi:10.1002/cbin.10742
Di, G., Du, X., Qi, X., Zhao, X., Duan, H., Li, S., et al. (2017). Mesenchymal stem cells promote diabetic corneal epithelial wound healing through TSG-6–dependent stem cell activation and macrophage switch. Invest. Ophthalmol. Vis. Sci. 58, 4344–4354. doi:10.1167/iovs.17-21506
Dinh, P. U. C., Paudel, D., Brochu, H., Popowski, K. D., Gracieux, M. C., Cores, J., et al. (2020). Inhalation of lung spheroid cell secretome and exosomes promotes lung repair in pulmonary fibrosis. Nat. Commun. 11, 1064. doi:10.1038/S41467-020-14344-7
Dominici, M., Le Blanc, K., Mueller, I., Slaper-Cortenbach, I., Marini, F., Krause, D., et al. (2006). Minimal criteria for defining multipotent mesenchymal stromal cells. The International Society for Cellular Therapy position statement. Cytotherapy 8, 315–317. doi:10.1080/14653240600855905
Du, Y., Li, D., Han, C., Wu, H., Xu, L., Zhang, M., et al. (2017). Exosomes from human-induced pluripotent stem cell-derived mesenchymal stromal cells (hiPSC-MSCs) protect liver against hepatic ischemia/reperfusion injury via activating sphingosine kinase and sphingosine-1-phosphate signaling pathway. Cell. Physiol. biochem. 43, 611–625. doi:10.1159/000480533
Elahi, F. M., Farwell, D. G., Nolta, J. A., and Anderson, J. D. (2019). Preclinical translation of exosomes derived from mesenchymal stem/stromal cells. Stem Cells 38, 15–21. doi:10.1002/stem.3061
El-Shennawy, L., Hoffmann, A. D., Dashzeveg, N. K., McAndrews, K. M., Mehl, P. J., Cornish, D., et al. (2022). Circulating ACE2-expressing extracellular vesicles block broad strains of SARS-CoV-2. Nat. Commun. 13, 405. doi:10.1038/S41467-021-27893-2
Fang, S., Xu, C., Zhang, Y., Xue, C., Yang, C., Bi, H., et al. (2016). Umbilical cord-derived mesenchymal stem cell-derived exosomal MicroRNAs suppress myofibroblast differentiation by inhibiting the transforming growth factor-β/SMAD2 pathway during wound healing. Stem Cells Transl. Med. 5, 1425–1439. doi:10.5966/SCTM.2015-0367
Feng, D., Zhao, W., Ye, Y., Bai, X., Liu, R., Chang, L., et al. (2010). Cellular internalization of exosomes occurs through phagocytosis. Traffic 11, 675–687. doi:10.1111/j.1600-0854.2010.01041.x
Gámez-Valero, A., Monguió-Tortajada, M., Carreras-Planella, L., Franquesa, M., Beyer, K., and Borràs, F. E. (2016). Size-Exclusion Chromatography-based isolation minimally alters Extracellular Vesicles’ characteristics compared to precipitating agents OPEN. Berlin: Nature Publishing Group. doi:10.1038/srep33641
Geiger, A., Walker, A., and Nissen, E. (2015). Human fibrocyte-derived exosomes accelerate wound healing in genetically diabetic mice. Biochem. Biophys. Res. Commun. 467, 303–309. doi:10.1016/j.bbrc.2015.09.166
Goetzl, E. J., Schwartz, J. B., Mustapic, M., Lobach, I. V., Daneman, R., Abner, E. L., et al. (2017). Altered cargo proteins of human plasma endothelial cell–derived exosomes in atherosclerotic cerebrovascular disease. FASEB J. 31, 3689–3694. doi:10.1096/fj.201700149
Gomes, J. P. A., Assoni, A. F., Pelatti, M., Coatti, G., Okamoto, O. K., and Zatz, M. (2017). Deepening a simple question: Can MSCs Be used to treat cancer? Anticancer Res. 37, 4747–4758. doi:10.21873/anticanres.11881
Greening, D. W., Xu, R., Gopal, S., Rai, A., and Simpson, R. J. (2016). Proteomic insights into extracellular vesicle biology - defining exosomes and shed microvesicles. Expert Rev. Proteomics 00, 69–95. doi:10.1080/14789450.2017.1260450
Gupta, A., Kashte, S., Gupta, M., Rodriguez, H. C., Gautam, S. S., and Kadam, S. (2020). Mesenchymal stem cells and exosome therapy for COVID-19: current status and future perspective. Hum. Cell 33, 907–918. doi:10.1007/S13577-020-00407-W
Gurtner, G. C., Werner, S., Barrandon, Y., and Longaker, M. T. (2008). Wound repair and regeneration. Nature 453, 314–321. doi:10.1038/nature07039
Gutierrez, S., Strug, I., Cappione, A., Smith, J., Mabuchi, M., and Nadler, T. (2013). Methods for the isolation and analysis of biomarkers from exosomes in cell culture media. USA: Merk Milipore, 3661.
Han, G., and Ceilley, R. (2017). Chronic wound healing: A review of current management and treatments. Adv. Ther. 34, 599–610. doi:10.1007/S12325-017-0478-Y
Hara, T., Kakudo, N., Morimoto, N., Ogawa, T., Lai, F., and Kusumoto, K. (2016). Platelet-rich plasma stimulates human dermal fibroblast proliferation via a Ras-dependent extracellular signal-regulated kinase 1/2 pathway. J. Artif. Organs 19, 372–377. doi:10.1007/s10047-016-0913-x
Haraszti, R. A., Didiot, M., Sapp, E., Leszyk, J., Shaffer, S. A., Rockwell, H. E., et al. (2016). High-resolution proteomic and lipidomic analysis of exosomes and microvesicles from different cell sources. J. Extracell. Vesicles 5, 32570. doi:10.3402/jev.v5.32570
He, X., Dong, Z., Cao, Y., Wang, H., Liu, S., Liao, L., et al. (2019). MSC-derived exosome promotes M2 polarization and enhances cutaneous wound healing. Stem Cells Int. 2019, 7132708–7132716. doi:10.1155/2019/7132708
Hu, L., Wang, J., Zhou, X., Xiong, Z., Zhao, J., Yu, R., et al. (2016). Exosomes derived from human adipose mensenchymal stem cells accelerates cutaneous wound healing via optimizing the characteristics of fibroblasts. Sci. Rep. 6, 32993. doi:10.1038/srep32993
Jayaramayya, K., Mahalaxmi, I., Subramaniam, M. D., Raj, N., Dayem, A. A., Lim, K. M., et al. (2020). Immunomodulatory effect of mesenchymal stem cells and mesenchymal stem-cell-derived exosomes for COVID-19 treatment. BMB Rep. 53, 400–412. doi:10.5483/BMBREP.2020.53.8.121
Johnstone, R. M., Adam, M., Hammond, J. R., Orr, L., and Turbide, C. (1987). Vesicle formation during reticulocyte maturation. Association of plasma membrane activities with released vesicles (exosomes). J. Biol. Chem. 262, 9412–9420. doi:10.1016/s0021-9258(18)48095-7
Katsuda, T., and Ochiya, T. (2015). Molecular signatures of mesenchymal stem cell-derived extracellular vesicle-mediated tissue repair. Stem Cell Res. Ther. 6, 212–218. doi:10.1186/s13287-015-0214-y
Kita, S., Maeda, N., and Shimomura, I. (2019). Interorgan communication by exosomes, adipose tissue, and adiponectin in metabolic syndrome. J. Clin. Invest. 129, 4041–4049. doi:10.1172/JCI129193
Komaki, M., Numata, Y., Morioka, C., Honda, I., Tooi, M., Yokoyama, N., et al. (2017). Exosomes of human placenta-derived mesenchymal stem cells stimulate angiogenesis. Stem Cell Res. Ther. 8, 219. doi:10.1186/S13287-017-0660-9
Landén, N. X., Li, D., and Ståhle, M. (2016). Transition from inflammation to proliferation: a critical step during wound healing. Cell. Mol. Life Sci. 73, 3861–3885. doi:10.1007/S00018-016-2268-0
Li, H., Liu, D., Li, C., Zhou, S., Tian, D., Xiao, D., et al. (2017). Exosomes secreted from mutant-HIF-1α-modified bone-marrow-derived mesenchymal stem cells attenuate early steroid-induced avascular necrosis of femoral head in rabbit. Cell Biol. Int. 41, 1379–1390. doi:10.1002/cbin.10869
Li, Y., Yang, Y., Ren, J., Xu, F., Chen, F., and Li, A. (2017). Exosomes secreted by stem cells from human exfoliated deciduous teeth contribute to functional recovery after traumatic brain injury by shifting microglia M1/M2 polarization in rats. Stem Cell Res. Ther. 9, 198–211. doi:10.1186/s13287-017-0648-5
Lin, Q., Fang, D., Fang, J., Ren, X., Yang, X., Wen, F., et al. (2011). Impaired wound healing with defective expression of chemokines and recruitment of myeloid cells in TLR3-deficient mice. J. Immunol. 186, 3710–3717. doi:10.4049/jimmunol.1003007
Lou, G., Chen, L., Xia, C., Wang, W., Qi, J., Li, A., et al. (2020). MiR-199a-modified exosomes from adipose tissue-derived mesenchymal stem cells improve hepatocellular carcinoma chemosensitivity through mTOR pathway. J. Exp. Clin. Cancer Res. 39, 4. doi:10.1186/S13046-019-1512-5
Lv, L., Zeng, Q., Wu, S., Xie, H., Chen, J., Jiang Guo, X., et al. (2015). “Exosome-Based translational nanomedicine: The therapeutic potential for drug delivery,” in Mesenchymal stem cell derived exosomes. Editors Y. Tang, and B. Dawn (USA: Elsevier), 161–176. doi:10.1016/B978-0-12-800164-6.00008-3
Macías, M., Rebmann, V., Mateos, B., Varo, N., Perez-Gracia, J. L., Alegre, E., et al. (2019). Comparison of six commercial serum exosome isolation methods suitable for clinical laboratories. Effect in cytokine analysis. Clin. Chem. Lab. Med. 57, 1539–1545. doi:10.1515/CCLM-2018-1297
Marck, R. E., Gardien, K. L. M., Stekelenburg, C. M., Vehmeijer, M., Baas, D., Tuinebreijer, W. E., et al. (2016). The application of platelet-rich plasma in the treatment of deep dermal burns: A randomized, double-blind, intra-patient controlled study. Wound Repair Regen. 24, 712–720. doi:10.1111/wrr.12443
Marote, A., Teixeira, F. G., Mendes-Pinheiro, B., and Salgado, A. J. (2016). MSCs-derived exosomes: Cell-secreted nanovesicles with regenerative potential. Front. Pharmacol. 7, 231–238. doi:10.3389/fphar.2016.00231
Martin, C., Patel, M., Williams, S., Arora, H., and Sims, B. (2018). Human breast milk-derived exosomes attenuate cell death in intestinal epithelial cells. Innate Immun. 24, 278–284. doi:10.1177/1753425918785715
Mulcahy, L. A., Pink, R. C., and Carter, D. R. F. (2014). Routes and mechanisms of extracellular vesicle uptake. J. Extracell. Vesicles 3, 24641. doi:10.3402/jev.v3.24641
Ostvar, O., Shadvar, S., Yahaghi, E., Azma, K., Fayyaz, A. F., Ahmadi, K., et al. (2015). Effect of platelet-rich plasma on the healing of cutaneous defects exposed to acute to chronic wounds: a clinico-histopathologic study in rabbits. Diagn. Pathol. 10, 85. doi:10.1186/s13000-015-0327-8
Pankajakshan, D., and Agrawal, D. K. (2014). Mesenchymal stem cell paracrine factors in vascular repair and regeneration. J. Biomed. Tech. Res. 1, 1–9. doi:10.19104/jbtr.2014.107
Pavlovic, V., Ciric, M., Jovanovic, V., and Stojanovic, P. (2016). Platelet rich plasma: a short overview of certain bioactive components. Open Med. 11, 242–247. doi:10.1515/med-2016-0048
Phinney, D. G., and Pittenger, M. F. (2017). Concise review: MSC-derived exosomes for cel-free therapy. Stem Cells 26, 591–599. doi:10.1634/stemcells.2007-0439
Pu, C. M., Liu, C. W., Liang, C. J., Yen, Y. H., Chen, S. H., Jiang-Shieh, Y. F., et al. (2017). Adipose-derived stem cells protect skin flaps against ischemia/reperfusion injury via IL-6 expression. J. Invest. Dermatol. 137, 1353–1362. doi:10.1016/J.JID.2016.12.030
Purushothaman, A., Bandari, S. K., Liu, J., Mobley, J. A., Brown, E. E., and Sanderson, R. D. (2016). Fibronectin on the surface of myeloma cell-derived exosomes mediates exosome-cell interactions. J. Biol. Chem. 291, 1652–1663. doi:10.1074/jbc.M115.686295
Qiu, X., Liu, J., Zheng, C., Su, Y., Bao, L., Zhu, B., et al. (2020). Exosomes released from educated mesenchymal stem cells accelerate cutaneous wound healing via promoting angiogenesis. Cell Prolif. 53, e12830. doi:10.1111/CPR.12830
Ramirez, M. I., Amorim, M. G., Gadelha, C., Milic, I., Welsh, J. A., Freitas, V. M., et al. (2018). Technical challenges of working with extracellular vesicles. Nanoscale 10, 881–906. doi:10.1039/C7NR08360B
Raposo, G., and Stoorvogel, W. (2013). Extracellular vesicles: Exosomes, microvesicles, and friends. J. Cell Biol. 200, 373–383. doi:10.1083/jcb.201211138
Rezakhani, L., Kelishadrokhi, A. F., Soleimanizadeh, A., and Rahmati, S. (2021). Mesenchymal stem cell (MSC)-derived exosomes as a cell-free therapy for patients Infected with COVID-19: Real opportunities and range of promises. Chem. Phys. Lipids 234, 105009. doi:10.1016/J.CHEMPHYSLIP.2020.105009
Robbins, P. D., and Morelli, A. E. (2014). Regulation of immune responses by extracellular vesicules. Nat. Immunol. 14, 195–208. doi:10.1038/nri3622
Rocco, G. D., Baldari, S., and Toietta, G. (2016). Towards therapeutic delivery of extracellular vesicles : Strategies for in vivo tracking and biodistribution analysis. Stem Cells Int. 2016, 5029619. doi:10.1155/2016/5029619
Samsonraj, R. M., Raghunath, M., Nurcombe, V., Hui, J. H., van Wijnen, A. J., and Cool, S. M. (2017). Concise review: multifaceted characterization of human mesenchymal stem cells for use in regenerative medicine. Stem Cells Transl. Med. 6, 2173–2185. doi:10.1002/SCTM.17-0129
Sarvar, D. P., Shamsasenjan, K., and Akbarzadehlaleh, P. (2016). Mesenchymal stem cell-derived exosomes : New opportunity in cell- free therapy. Adv. Pharm. Bull. 6, 293–299. doi:10.15171/apb.2016.041
Schageman, J., Zeringer, E., Li, M., Barta, T., Lea, K., Gu, J., et al. (2013). The complete exosome workflow solution : From isolation to characterization of RNA cargo. Biomed. Res. Int. 2013, 253957. doi:10.1155/2013/253957
Shelke, G. V., Yin, Y., Jang, S. C., Lässer, C., Wennmalm, S., Hoffmann, H. J., et al. (2019). Endosomal signalling via exosome surface TGFβ-1. J. Extracell. Vesicles 8, 1650458–1650520. doi:10.1080/20013078.2019.1650458
Silva, A. M., Teixeira, J. H., Almeida, M. I., Goncalves, R. M., Barbosa, M. A., and Santos, S. G. (2016). Extracellular Vesicles: Immunomodulatory messengers in the context of tissue repair/regeneration. Eur. J. Pharm. Sci. 98, 86. doi:10.1016/j.ejps.2016.09.017
Song, N., Wakimoto, H., Rossignoli, F., Bhere, D., Ciccocioppo, R., Chen, K. S., et al. (2021). Mesenchymal stem cell immunomodulation: In pursuit of controlling COVID-19 related cytokine storm. Stem Cells 39, 707–722. doi:10.1002/STEM.3354
Song, Y., Wang, B., Zhu, X., Hu, J., Sun, J., Xuan, J., et al. (2021). Human umbilical cord blood-derived MSCs exosome attenuate myocardial injury by inhibiting ferroptosis in acute myocardial infarction mice. Cell Biol. Toxicol. 37, 51–64. doi:10.1007/S10565-020-09530-8
Sorg, H., Tilkorn, D. J., Hager, S., Hauser, J., and Mirastschijski, U. (2017). Skin wound healing: An update on the current knowledge and concepts. Eur. Surg. Res. 58, 81–94. doi:10.1159/000454919
Spanò, R., Muraglia, A., Todeschi, M. R., Nardini, M., Strada, P., Cancedda, R., et al. (2016). Platelet rich plasma-based bioactive membrane as a new advanced wound care tool. J. Tissue Eng. Regen. Med. 12, e82–e96. doi:10.1002/term.2357
Stroncek, J., and Reichert, W. (2008). “Overview of wound healing in different tissue types,” in Indwelling neural implants: Strategies for contending with the in vivo environment. Editor W. Reichert (Boca Raton FL: CRC Press/Taylor & Francis).
Tao, S. C., Yuan, T., Zhang, Y. L., Yin, W. J., Guo, S. C., and Zhang, C. Q. (2017a). Exosomes derived from miR-140-5p-overexpressing human synovial mesenchymal stem cells enhance cartilage tissue regeneration and prevent osteoarthritis of the knee in a rat model. Theranostics 7, 180–195. doi:10.7150/THNO.17133
Tao, S. C., Guo, S.-C., and Zhang, C.-Q. (2017b). Platelet-derived extracellular vesicles: An emerging therapeutic approach. Int. J. Biol. Sci. 13, 828–834. doi:10.7150/ijbs.19776
Thangarajah, T., Sanghani-Kerai, A., Henshaw, F., Lambert, S. M., Pendegrass, C. J., and Blunn, G. W. (2017). Application of a demineralized cortical bone matrix and bone marrow–derived mesenchymal stem cells in a model of chronic rotator cuff degeneration. Am. J. Sports Med. 46, 98–108. doi:10.1177/0363546517727512
Théry, C., Amigorena, S., Raposo, G., and Clayton, A. (2006). Isolation and characterization of exosomes from cell culture supernatants. Curr. Protoc. Cell Biol., 1–29. doi:10.1002/0471143030.cb0322s30
Théry, C., Witwer, K. W., Aikawa, E., Alcaraz, M. J., Anderson, J. D., Andriantsitohaina, R., et al. (2018). Minimal information for studies of extracellular vesicles 2018 (MISEV2018): a position statement of the international society for extracellular vesicles and update of the MISEV2014 guidelines. J. Extracell. Vesicles 7. doi:10.1080/20013078.2018.1535750
Toh, W. S., Lai, R. C., Hui, J. H. P., and Lim, S. K. (2016). MSC exosome as a cell-free MSC therapy for cartilage regeneration: Implications for osteoarthritis treatment. Semin. Cell Dev. Biol. 67, 56–64. doi:10.1016/j.semcdb.2016.11.008
Tooi, M., Komaki, M., Morioka, C., Honda, I., Iwasaki, K., Yokoyama, N., et al. (2015). Placenta mesenchymal stem cell derived exosomes confer plasticity on fibroblasts. J. Cell. Biochem. 13, 1658–1670. doi:10.1002/jcb.25459
Tripisciano, C., Weiss, R., Eichhorn, T., Spittler, A., Heuser, T., Fischer, M. B., et al. (2017). Different potential of extracellular vesicles to support thrombin generation: contributions of phosphatidylserine, tissue factor, and cellular origin. Sci. Rep. 7, 6522. doi:10.1038/s41598-017-03262-2
Vallabhaneni, K. C., Penfornis, P., Dhule, S., Adams, K. V., Mo, Y. Y., Xu, R., et al. (2014). Extracellular vesicles from bone marrow mesenchymal stem/stromal cells transport tumor regulatory microRNA , proteins , and metabolites. Oncotarget 6, 4953–4967. doi:10.18632/oncotarget.3211
van Niel, G., D’Angelo, G., and Raposo, G. (2018). Shedding light on the cell biology of extracellular vesicles. Nat. Rev. Mol. Cell Biol. 19, 213–228. doi:10.1038/nrm.2017.125
Vyas, N., and Dhawan, J. (2017). Exosomes: mobile platforms for targeted and synergistic signaling across cell boundaries. Cell. Mol. Life Sci. 74, 1567–1576. doi:10.1007/s00018-016-2413-9
Wang, B., Xing, D., Zhu, Y., Dong, S., and Zhao, B. (2019). The state of exosomes research: A global visualized analysis. Biomed. Res. Int. 2019, 1495130. doi:10.1155/2019/1495130
Wang, J., Chen, Z., Sun, M., Xu, H., Gao, Y., Liu, J., et al. (2020). Characterization and therapeutic applications of mesenchymal stem cells for regenerative medicine. Tissue Cell 64, 101330. doi:10.1016/J.TICE.2020.101330
Wang, X., Jiao, Y., Pan, Y., Zhang, L., Gong, H., Qi, Y., et al. (2019). Fetal dermal mesenchymal stem cell-derived exosomes accelerate cutaneous wound healing by activating Notch signaling. Stem Cells Int. 2019, 2402916–2403011. doi:10.1155/2019/2402916
Waycaster, C. R., Gilligan, A. M., and Motley, T. A. (2016). Cost-effectiveness of becaplermin gel on wound healing of diabetic foot ulcers. Wound Repair Regen. 106, 353–360. doi:10.1111/wrr.12285
Wei, H., Xu, Y., Chen, Q., Chen, H., Zhu, X., and Li, Y. (2020). Mesenchymal stem cell-derived exosomal miR-223 regulates neuronal cell apoptosis. Cell Death Dis. 11, 290. doi:10.1038/S41419-020-2490-4
Wei, M., Gao, X., Liu, L., Li, Z., Wan, Z., Dong, Y., et al. (2020). Visceral adipose tissue derived exosomes exacerbate colitis severity via pro-inflammatory MiRNAs in high fat diet fed mice. ACS Nano 14, 5099–5110. doi:10.1021/ACSNANO.0C01860
Wu, P., Zhang, B., Shi, H., Qian, H., and Xu, W. (2018). MSC-exosome: a novel cell-free therapy for cutaneous regeneration. Cytotherapy 20, 291–301. doi:10.1016/j.jcyt.2017.11.002
Xie, M. Y., Hou, L. J., Sun, J. J., Zeng, B., Xi, Q. Y., Luo, J. Y., et al. (2019). Porcine milk exosome MiRNAs attenuate LPS-induced apoptosis through inhibiting TLR4/NF-κB and p53 pathways in intestinal epithelial cells. J. Agric. Food Chem. 67, 9477–9491. doi:10.1021/ACS.JAFC.9B02925
Xin, H., Li, Y., and Chopp, M. (2014). Exosomes/miRNAs as mediating cell-based therapy of stroke. Front. Cell. Neurosci. 8, 377. doi:10.3389/fncel.2014.00377
Yan, W., Li, T., Yin, T., Hou, Z., Qu, K., Wang, N., et al. (2020). M2 macrophage-derived exosomes promote the c-KIT phenotype of vascular smooth muscle cells during vascular tissue repair after intravascular stent implantation. Theranostics 10, 10712–10728. doi:10.7150/THNO.46143
Yao, S., Li, X., Liu, J., Sun, Y., Wang, Z., and Jiang, Y. (2017). Maximized nanodrug-loaded mesenchymal stem cells by a dual drug-loaded mode for the systemic treatment of metastatic lung cancer. Drug Deliv. 24, 1372–1383. doi:10.1080/10717544.2017.1375580
Yasui, T., Yanagida, T., Ito, S., Konakade, Y., Takeshita, D., Naganawa, T., et al. (2017). Unveiling massive numbers of cancer-related urinary-microRNA candidates via nanowires. Sci. Adv. 3, 1701133. doi:10.1126/sciadv.1701133
Yu, M., Liu, W., Li, J., Lu, J., Lu, H., Jia, W., et al. (2020). Exosomes derived from atorvastatin-pretreated MSC accelerate diabetic wound repair by enhancing angiogenesis via AKT/eNOS pathway. Stem Cell Res. Ther. 11, 350. doi:10.1186/S13287-020-01824-2
Zhang, B., Yeo, R. W. Y., Tan, K. H., and Lim, S. K. (2016). Focus on extracellular vesicles: Therapeutic potential of stem cell-derived extracellular vesicles. Int. J. Mol. Sci. 17, 174. doi:10.3390/ijms17020174
Zhang, B., Yin, Y., Lai, R. C., Tan, S. S., Choo, A. B. H., and Lim, S. K. (2014). Mesenchymal stem cells secrete immunologically active exosomes. Stem Cells Dev. 23, 1233–1244. doi:10.1089/scd.2013.0479
Zhang, B., Wang, M., Gong, A., Zhang, X., Wu, X., Zhu, Y., et al. (2015). HucMSC-exosome mediated-wnt4 signaling is required for cutaneous wound healing. Stem Cells 33, 2158–2168. doi:10.1002/STEM.1771
Zhang, J., Guan, J., Niu, X., Hu, G., Guo, S., Li, Q., et al. (2015a). Exosomes released from human induced pluripotent stem cells-derived MSCs facilitate cutaneous wound healing by promoting collagen synthesis and angiogenesis. J. Transl. Med. 13, 49. doi:10.1186/s12967-015-0417-0
Zhang, J., Li, S., Li, L., Li, M., Guo, C., Yao, J., et al. (2015b). Exosome and exosomal microRNA: Trafficking, sorting, and function. Genomics Proteomics Bioinforma. 13, 17–24. doi:10.1016/j.gpb.2015.02.001
Zhang, S., Chuah, S. J., Lai, R. C., Hui, J. H. P., Lim, S. K., and Toh, W. S. (2018). MSC exosomes mediate cartilage repair by enhancing proliferation, attenuating apoptosis and modulating immune reactivity. Biomaterials 156, 16–27. doi:10.1016/J.BIOMATERIALS.2017.11.028
Zhang, Q., Jeppesen, D. K., Higginbotham, J. N., Graves-Deal, R., Trinh, V. Q., Ramirez, M. A., et al. (2021). Supermeres are functional extracellular nanoparticles replete with disease biomarkers and therapeutic targets. Nat. Cell Biol. 23, 1240–1254. doi:10.1038/S41556-021-00805-8
Zhang, J., Chen, C., Hu, B., Niu, X., Liu, X., Zhang, G., et al. (2016). Exosomes derived from human endothelial progenitor cells accelerate cutaneous wound healing by promoting angiogenesis through Erk1/2 signaling. Int. J. Biol. Sci. 12, 1472–1487. doi:10.7150/ijbs.15514
Zhao, Y., Gan, Y., Xu, G., Hua, K., and Liu, D. (2020). Exosomes from MSCs overexpressing microRNA-223-3p attenuate cerebral ischemia through inhibiting microglial M1 polarization mediated inflammation. Life Sci. 260, 118403. doi:10.1016/J.LFS.2020.118403
Keywords: extracellular microvesicles, exosomes, tissue regeneration, regenerative medicine, immunomodulation
Citation: Aguiar Koga BA, Fernandes LA, Fratini P, Sogayar MC and Carreira ACO (2023) Role of MSC-derived small extracellular vesicles in tissue repair and regeneration. Front. Cell Dev. Biol. 10:1047094. doi: 10.3389/fcell.2022.1047094
Received: 17 September 2022; Accepted: 07 December 2022;
Published: 01 March 2023.
Edited by:
Lindolfo da Silva Meirelles, Universidade Luterana do Brasil, BrazilReviewed by:
Federica Collino, University of Milan, ItalyCopyright © 2023 Aguiar Koga, Fernandes, Fratini, Sogayar and Carreira. This is an open-access article distributed under the terms of the Creative Commons Attribution License (CC BY). The use, distribution or reproduction in other forums is permitted, provided the original author(s) and the copyright owner(s) are credited and that the original publication in this journal is cited, in accordance with accepted academic practice. No use, distribution or reproduction is permitted which does not comply with these terms.
*Correspondence: Ana Claudia Oliveira Carreira, YW5jb2NAaXEudXNwLmJy, YW5hLmNhcnJlaXJhQHVmYWJjLmVkdS5icg==
Disclaimer: All claims expressed in this article are solely those of the authors and do not necessarily represent those of their affiliated organizations, or those of the publisher, the editors and the reviewers. Any product that may be evaluated in this article or claim that may be made by its manufacturer is not guaranteed or endorsed by the publisher.
Research integrity at Frontiers
Learn more about the work of our research integrity team to safeguard the quality of each article we publish.