- Institut Necker Enfants Malades (INEM), INSERM UMR-S1151, CNRS UMR-S8253, Université Paris Cité, Paris, France
The maintenance of cellular homeostasis in response to extracellular stimuli, i.e., nutrient and hormone signaling, hypoxia, or mechanical forces by autophagy, is vital for the health of various tissues. The primary cilium (PC) is a microtubule-based sensory organelle that regulates the integration of several extracellular stimuli. Over the past decade, an interconnection between autophagy and PC has begun to be revealed. Indeed, the PC regulates autophagy and in turn, a selective form of autophagy called ciliophagy contributes to the regulation of ciliogenesis. Moreover, the PC regulates both mitochondrial biogenesis and lipophagy to produce free fatty acids. These two pathways converge to activate oxidative phosphorylation and produce ATP, which is mandatory for cell metabolism and membrane transport. The autophagy-dependent production of energy is fully efficient when the PC senses shear stress induced by fluid flow. In this review, we discuss the cross-talk between autophagy, the PC and physical forces in the regulation of cell biology and physiology.
Introduction
The recent years have witnessed the importance of the role of autophagy in cell metabolism (Kaur and Debnath, 2015; Lahiri et al., 2019; Levine and Kroemer, 2019; Poillet-Perez and White, 2019; He, 2022). Beyond the recycling of nutrients from the lysosomal compartment to fuel different metabolic pathways (Ballabio and Bonifacino, 2020), recent research has highlighted the function of selective forms of autophagy, such as lipophagy (degradation of lipid droplets) (Schulze et al., 2017) and mitophagy (partial or total degradation of mitochondria) in metabolism (Onishi et al., 2021). The impact of autophagy on cell metabolism assumes different forms in mammals: Macroautophagy (see next paragraph), microautophagy (uptake of cytoplasmic material at the surface of lysosomes or endosomes) [reviewed in (Oku and Sakai, 2018; Tekirdag and Cuervo, 2018; Schuck, 2020)] and chaperone-mediated autophagy (CMA), which allows the selective transport of proteins containing a KFERQ motif into the lysosomal lumen in a manner dependent on chaperones and on the lysosomal membrane protein LAMP2A [Reviewed in (Kaushik and Cuervo, 2018)]. As mentioned above, macroautophagy (hereafter referred to as autophagy) contributes to metabolism mostly via different aspects: by providing nutrients (amino acids, nucleosides, fatty acids and monosaccharides) for different metabolic pathways (Mortimore and Poso, 1987; Yim and Mizushima, 2020), by generating fatty acids (lipophagy) available for lipid synthesis or for fueling oxidative phosphorylation (Miceli et al., 2020; Xie et al., 2020; Zhang et al., 2022) and finally, by acting in the quality control of mitochondria (mitophagy). Thus, autophagy contributes in adapting cell metabolism to the cell program, e.g., by reorienting a cell from oxidative phosphorylation to glycolysis or by controlling the levels of reactive oxygen species generated by mitochondria (Pickles et al., 2018). In turn, autophagy is controlled by nutrients and metabolites, such as acetyl-CoA and α-ketoglutarate (Pietrocola et al., 2015; Baracco et al., 2019). CMA, which is stimulated by starvation, contributes to glucose metabolism by regulating the levels of many enzymes engaged in glycolysis and the TCA cycle (Schneider et al., 2014). CMA also contributes to lipid metabolism by degrading perilipins at the surface of lipid droplets (Kaushik and Cuervo, 2015), a prerequisite for lipophagy. In addition, several enzymes involved in lipogenesis are substrates for CMA (Tasset and Cuervo, 2016). Although microautophagy was first recognized in hepatocytes under starvation (Mortimore and Poso, 1984; Mortimore et al., 1988), it was characterized in yeast, where bulk and various forms of selective microautophagy were identified [reviewed in (Oku and Sakai, 2018; Schuck, 2020)]. In mammals, endosomal-microautophagy (Tekirdag and Cuervo, 2018) and Mitochondrial-derived Vesicle (MDV)-micromitophagy are the best studied forms of microautophagy (Lemasters, 2014). MDV-microautophagy, along with mitophagy, contribute to the quality control of mitochondria (Pickles et al., 2018).
Many signaling pathways emanate from the primary cilium and among these, several are engaged in the regulation of metabolism (Pala et al., 2017; Anvarian et al., 2019; Avalos et al., 2022; Yang et al., 2022a). For example, an interplay between the Hedgehog and mTORC1 pathways exist (Larsen and Moller, 2020), and both are regulated by the primary cilium. Also, cAMP produced by the type III adenylyl cyclase in neuronal primary cilium contributes to energy balance (Yang et al., 2022b), and mutations in the ciliary melanocortin 4 receptor in paraventricular hypothalamic neurons give rise to obesity (Lee et al., 2022). Moreover, a defective primary cilium impairs adipose-derived mesenchymal stem cell (ASCs) functions (Strong et al., 2015; Louwen et al., 2018) that are responsible for cell renewal, spontaneous repair and immunomodulation in adipose tissue, suggesting that obesity could be considered as a secondary form of ciliopathy (Ritter et al., 2018).
The specific aim of the present review is to present the importance of the cross-talk between autophagy and the primary cilium in the control of metabolism and also to highlight stimuli and signaling pathways that emanate from the primary cilium to regulate autophagy. Readers interested in a deeper discussion on the cross-talk between the primary cilium and autophagy and/or on the role of primary cilium in regulating proteostasis can consult recent reviews on these topics (Orhon et al., 2015; Pampliega and Cuervo, 2016; Boukhalfa et al., 2019; Wiegering et al., 2019; Morel et al., 2021; Morleo et al., 2022; Senatore et al., 2022).
The autophagic pathway
Autophagy is characterized by the formation of a double-membrane-bound autophagosome (Mizushima et al., 2008; Boya et al., 2013). The autophagosome, which originates from a membranous structure named the phagophore, sequesters fractions of the cytoplasm, in a selective or non-selective manner, to deliver them to the lysosome (Khaminets et al., 2016) [Readers interested in the origin of the phagophore can consult recent reviews (Deretic and Lazarou, 2022; Hu and Reggiori, 2022)]. Autophagy is regulated by Autophagy-related (ATG) proteins and associated factors (Nakatogawa et al., 2009; Mizushima et al., 2011). Initiation of autophagosome formation is triggered by changes in cell nutritional levels or by different stress situations (Levine and Kroemer, 2019). Autophagy stimulation is typically dependent on the downregulation of mTORC1 kinase activity leading to stimulation of the ULK complex, which consists of the serine/threonine kinase ULK1 or ULK2 (mammalian homologs of yeast Atg1), ATG101, ATG13 and a 200 kDa focal adhesion kinase family-interacting protein (FIP200, also called RB1CC1) (Kawabata and Yoshimori, 2020; Nishimura and Tooze, 2020). The growth of the autophagosome membrane depends on ATG9A-containing vesicles. Indeed, ATG9A, which harbor a scramblase activity, binds to ATG2, a factor able to transfer phospholipids from the ER to the phagophore in formation (Sawa-Makarska et al., 2020). This interaction is important for expanding the phagophore (Maeda et al., 2020; Chang et al., 2021). The ULK complex stimulates the class III phosphoinositide 3-kinase (PI3KIII) complex. The core PI3KIII complex is composed of the kinase VPS34 (encoded by PIK3C3 in mammals), VPS15, ATG14L1 and Beclin1 (homolog of yeast Atg6) (Kawabata and Yoshimori, 2020; Nishimura and Tooze, 2020). VPS34 allows the formation of phosphatidylinositol 3-phosphate (PI3P) to recruit the PI3P-interacting proteins WIPI2B and ATG16L1, followed by the interaction of the ATG12–ATG5 ubiquitin-like conjugate to form a complex with ATG16L1. The ATG12–ATG5-ATG16L1 complex triggers the conjugation of ATG8 family LC3/GABARAP proteins to phosphatidylethanolamine, which will be integrated in the autophagic membrane. During the different forms of selective autophagy, the ATG8 family proteins can bind to cargo receptors, including p62 (SQSTM1), NDP52 and optineurin, which harbor LC3-interacting region (LIR) motifs (Johansen and Lamark, 2020; Gubas and Dikic, 2022). After closure and maturation, the autophagosome can deliver its content to endosomes or lysosomes, in a process regulated by several proteins, including small Rab GTPases and SNAREs, to induce the degradation of the autophagy cargo (Zhao et al., 2021). The autophagic pathway is also controlled at transcriptional and epigenetic levels (Fullgrabe et al., 2014). Of note, the inhibition of mTORC1 also allows for the nuclear translocation of the transcription factor TFEB, which controls lysosomal biogenesis as well as the expression of several key autophagy genes (SQSTM1, WIPI1, WIPI2, MAP1LC3B, ATG9B) (Napolitano and Ballabio, 2016).
The primary cilium
Cilia are microtubule-based structures present at the surface of different cell types. Conventionally, cilia which are motile or non-motile, are classified by their microtubule structure and motility (Satir and Christensen, 2007). Motile cilia (MC) is a beating organelle which generate fluid flow. MC are present on the surface of epithelial cells of the ependyma and in the brain as well as on epithelial cells lining the airways and reproductive tracts. In contrast to MC, primary cilia are solitary organelles found in almost all cell types, with some exceptions such as some cells of the lymphoid and myeloid lineage and other cells like hepatocytes (Finetti et al., 2014; Douanne et al., 2021). From a structural point of view, they consist of an axoneme of nine doublet microtubules growing from a basal body that is coming from one of the centrioles of the centrosome (the mother centriole) (Malicki and Johnson, 2017). Of note, ciliogenesis and cell division are mutually exclusive. A ciliary subdomain highly organized called transition zone is crucial for gating the entry of cytosolic factors into the axoneme (Satir and Christensen, 2007; Garcia-Gonzalo and Reiter, 2012). Primary cilia is a sensory non-motile organelle playing a role during embryonic development, olfaction, vision, and mechanotransduction (Satir and Christensen, 2007; Goetz and Anderson, 2010). They respond to different stimuli such as mechanical stimuli [for example, shear stress that may lead to cilia bending (Ferreira et al., 2019)]and chemical stimuli [e.g., specific ligand, growth factor, hormone or morphogen recognition (Satir and Christensen, 2007; Goetz and Anderson, 2010)]. Various signaling pathways emanate from this antenna, such as the Hedgehog and Wnt pathways, the platelet-derived growth factor (PDGF) pathway (Anvarian et al., 2019; Nachury and Mick, 2019). Intraflagellar transport (IFT) complexes are crucial for the ciliogenesis (Nakayama and Katoh, 2018; Nachury and Mick, 2019; Pigino, 2021). While the IFT-B complex consists of 16 proteins, which together with the motor protein kinesin 2, are involved in the transport of proteins to the tip of the primary cilium, the IFT-A complex, which is composed of six factors, contributes to the protein transport from the tip to the basal body of the primary cilium in a dynein-dependent manner (Nakayama and Katoh, 2018). Abnormalities of cilia structure or/and function lead to human diseases known as ciliopathies (Braun and Hildebrandt, 2017; Anvarian et al., 2019).
Primary cilium and autophagy in cell metabolism regulation
The first evidence of a primary cilium-dependent activation of autophagy was originally described in cells deprived of serum and/or nutrients (Pampliega et al., 2013; Tang et al., 2013). Subsequently, fluid flow (shear stress) has been shown to induce autophagy in a primary cilium-dependent manner in kidney proximal tubule epithelial cells (PTEC), thus highlighting a physiological role for primary cilium-dependent autophagy. Indeed, in PTEC, primary cilia are located at the apical membrane facing the urinary flow and regulates the cell size upon shear stress (Boehlke et al., 2010). This process is dependent on the stimulation of a pool of AMP-activated protein kinase (AMPK) at the base of the cilium (basal body), downstream of liver kinase B1 (LKB1) (Boehlke et al., 2010). Afterwards, we demonstrated the LKB1/AMPK-dependent activation of autophagy plays a crucial role in the regulation of cell size in kidney epithelial cells subjected to shear stress (Orhon et al., 2016). In fact, two signaling pathways dependent on AMPK come from the primary cilium (Miceli et al., 2020). On one hand, the fluid flow-dependent activation of AMPK upregulates the mitochondrial mass by controlling the expression of two master regulators of mitochondrial protein expression: peroxisome proliferator-activated receptor-gamma coactivator alpha (PGC1α) and mitochondrial transcription factor A (TFAM). On the other hand, AMPK induces a form of selective autophagy that degrades lipid droplets, called lipophagy (Singh et al., 2009a; Roberts and Olzmann, 2020), to generate free fatty acids used as substrates in mitochondria (Walch et al., 2015; Ogasawara et al., 2020; Rodriguez et al., 2021). Together, mitochondrial biogenesis and lipophagy in PTEC allow ATP production to supports energy consuming cellular processes, such as glucose reabsorption and gluconeogenesis (Miceli et al., 2020) (Figure 1). It is worth noting that under physiological conditions, PTEC contain numerous mitochondria to produce high levels of ATP in a manner dependent on fatty acid oxidation (FAO) (Bhargava and Schnellmann, 2017), supported by the lack of lipid droplets (Minami et al., 2017; Yamamoto et al., 2017). However, during fasting periods (Minami et al., 2017) or pathological situations such as acute kidney injury (AKI) (Li et al., 2009; Zhang et al., 2018), lipid droplets can be observed. Accordingly, it has been reported that tubular injury upon AKI is associated with the downregulation of PGC1α expression, mitochondrial and FAO dysfunction (Zhan et al., 2013; Zuk and Bonventre, 2016; Zhang et al., 2018). Moreover, in cisplatin-induced injury, the length of primary cilium is shortened and associated with reduced autophagy levels and mitochondrial dysfunction (Fujii et al., 2021; Wang et al., 2021). Defective FAO (Menezes et al., 2016; Warner et al., 2016), altered mitochondrial oxidative phosphorylation (Hajarnis et al., 2017; Ishimoto et al., 2017) and impairment of autophagy (Belibi et al., 2011; Rowe et al., 2013) have also been observed in cells lining renal cysts in a mouse model of autosomal dominant polycystic kidney disease (ADPKD) and in renal tissue from ADPKD patients (Padovano et al., 2018). ADPKD is mainly associated with mutations in the PKD1 or PKD2 genes, encoding the ciliary proteins polycystin-1 and -2 (PC1 and PC2), respectively (Hughes et al., 1995; Mochizuki et al., 1996). It can thus be speculated that a loss of primary cilium-dependent stimulation of autophagy could contribute to the development and/or early stages of these ciliopathies. Moreover, the maintenance of tissue homeostasis dependent on autophagy and mitochondrial biogenesis is not only restricted to the renal tubules. Indeed, in endothelial cells, fluid flow can also support mitochondrial biogenesis and ATP production (Kim et al., 2015; Wu et al., 2018; Yamamoto et al., 2018). However, the primary cilium does not function upstream of autophagy in endothelial cells submitted to high shear stress (Vion et al., 2017) and its role upstream of mitochondrial biogenesis has not been documented.
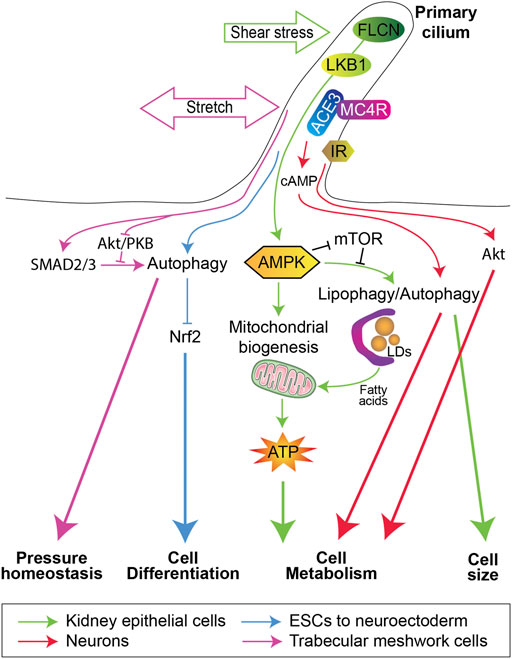
FIGURE 1. Primary cilium and autophagy in the regulation of cell fate. Shear stress in kidney epithelial cells (Green arrows) activates the folliculin (FLCN)/LKB1/AMPK/mTORC1 cascade to induce on one hand mitochondria biogenesis and on the other hand autophagy/lipophagy to regulate cell size and to produce fatty acids from lipid droplets (LDs) degradation, which will be used by mitochondria for ATP synthesis. This energy production favorizes the kidney epithelial cells metabolic reprogramming to control many activities, e.g., glucose reabsorption and gluconeogenesis. Stretch in trabecular meshwork cells (Purple arrows) activates the SMAD2/3 pathway and inhibits the Akt/PKB signaling, to positively regulate autophagy and control intraocular pressure homeostasis. Neuronal primary cilium (Red arrows) can regulate cell metabolism by the melanocortin 4 receptor (MC4R) or Insulin receptor (IR), which control cAMP production via type III adenylyl cyclase (ACE3) and Akt signaling, respectively. In embryonic stem cells (Blue arrows), a primary cilium-dependent inhibition of nuclear factor erythroid-related factor 2 (Nrf2) promotes their differentiation towards a neuroectodermal fate.
Other mechanosensors can transduce shear stress, as represented by microvilli-dependent formation of vacuoles in epithelial intestinal cells (Kim et al., 2017), which lack primary cilia. The formation of these vacuoles depends on a subset of ATG (ATG5 and LC3, independent of Beclin 1). This work showed that microvilli transduce shear stress independently of the primary cilium to initiate an ATG-dependent vacuolar process. The authors speculate that these vacuoles could be responsible for the degradation or secretion of macronutrients by intestinal enterocytes.
Stem cell renewal and differentiation are dependent on the functioning of the primary cilium to initiate many signaling pathways (Yanardag and Pugacheva, 2021; Shimada and Kato, 2022; Bodle and Loboa, 2016) and on autophagy, which contributes to proteostasis and metabolic control via mitophagy (Boya et al., 2018). Recently, the coordinated contributions of autophagy and the primary cilium to the differentiation of embryonic stem cells (ESCs) has been shown (Jang et al., 2016). The primary cilium has indeed been demonstrated to emerges from ESCs after induced lineage specification and triggers autophagy. This leads to the inactivation of nuclear factor erythroid-related factor 2 (Nrf2), upregulating the transcriptional stimulation of the pluripotency proteins OCT4 and NANOG and directing ESCs towards a neuroectodermal fate (Jang et al., 2016) (Figure 1). In summary, autophagy is important to fulfil the energy requirements for ESCs remodeling and metabolic changes.
While not directly related to the topic of the review, it is important to keep in mind that a complex crosstalk exist between the primary cilium and the ubiquitin proteasome system (UPS) (Orhon et al., 2015; Pampliega and Cuervo, 2016; Boukhalfa et al., 2019; Wiegering et al., 2019; Morel et al., 2021; Morleo et al., 2022; Senatore et al., 2022) and between autophagy and the UPS (Kwon and Ciechanover, 2017; Pohl and Dikic, 2019). The UPS plays an important role for controlling ciliogenesis and disassembly of the primary cilium (Kim et al., 2010; Wheway et al., 2015). As an example of that, the UPS regulates ciliogenesis by controlling the expression of the centriole and centriolar satellite protein OFD1 (Senatore et al., 2021). Interestingly, the activation of UPS by the SARS-CoV2 protein ORF10 increases the degradation of ciliary proteins, thus impairing ciliogenesis (Wang et al., 2022). In addition, the UPS can turn off signaling pathways initiated by the primary cilium (Gerhardt et al., 2016; Wiegering et al., 2019; Szymanska et al., 2022). Whether the primary cilium-UPS axis operates downstream of mechanosensors to regulate metabolism remains to be investigated.
What is the upstream control of the cross-talk between autophagy and primary cilium
Several signaling pathways could stimulate autophagy in a primary cilium-dependent manner and vary according to cell type and stimuli. For example, it was reported that induction of canonical ciliary Hedgehog signaling (activation of Smo and transcription factor Gli2) was crucial to trigger primary cilium-dependent autophagy upon serum starvation in kidney epithelial cells, fibroblasts and neurons (Pampliega et al., 2013). Recently, it has been reported that a non-canonical hedgehog signaling pathway (without stimulation of Gli transcription factors) initiates ciliogenesis and autophagy (Akhshi and Trimble, 2021). Indeed, on one hand, Smo activates the LKB1-AMPK axis to activate autophagy and early stages of ciliogenesis and on the other hand a heterotrimeric Gi pathway to promote ciliogenesis. Interestingly, the cross-talk between the primary cilium and autophagy via the Gli2 transcription factor modulates cell cycle re-entry (Hsiao et al., 2018).
Upon shear stress, autophagy is induced in kidney epithelial in a manner dependent on the mTORC1/AMPK pathway, as mentioned in the preceding paragraph, but also by PC2, a primary cilium-associated calcium channel (Orhon et al., 2016). In contrast to the mTORC1/AMPK dependent autophagy, PC2-dependent autophagy is not important for cell size regulation. However, based on recent findings, PC2-dependent autophagy could be part of a mechanism acting during kidney repair after structural changes (Dong et al., 2021). Fluid flow senses by the primary cilium triggers LKB1 activation, located in the axoneme, thus allowing the stimulation of the mTORC1/AMPK pathway (Boehlke et al., 2010; Orhon et al., 2016). In this system, folliculin promotes the recruitment of LKB1 at the primary cilium, participates in the regulation of AMPK activity, autophagy and cell size (Zemirli et al., 2019). Moreover, patients suffering from a ciliopathy called Birth-Hogg-Dubbé syndrome present heterozygous mutations in the folliculin gene, suggesting that the activation of autophagy could be an important factor in maintaining cellular homeostasis in a primary cilium-dependent manner (Luijten et al., 2013). Interestingly, LKB1 has been shown to partner with the PC1 mechanosensor in the primary cilium (Viau et al., 2018). PC1, in contrast to PC2, controls kidney epithelial cell size (Viau et al., 2020). Thus, it is tempting to hypothesize that PC1 is the membrane sensor upstream of the LKB1/AMPK axis to control autophagy and cell size.
Similarly, primary cilium-dependent inhibition of mTORC1 stimulates autophagy in chondrocytes in response to cyclic tensile strain (Xiang et al., 2019). Mechanical stretch sensing by the primary cilium can also regulate autophagy in the trabecular meshwork to regulate the intraocular pressure (Shim et al., 2021), in a process dependent on the SMAD2/3 pathway and inhibited by Akt/PKB (Figure 1). The protein RPGRIP1L, encoded by the Rpgrip1l/Ftm/Mks5/Nphp8 (Rpgrip1-like) gene and mutated in patients suffering from deadly ciliopathies (Arts et al., 2007; Delous et al., 2007), is located in the primary cilium transition zone and controls autophagy by inhibiting mTOR signaling (Struchtrup et al., 2018). Interestingly, RPGRIP1L regulates autophagy and the proteasome pathway via two independent signaling pathways (Gerhardt et al., 2015; Struchtrup et al., 2018).
Regarding the molecular events required for autophagosome formation following primary cilium sensing, it has been reported that many ATGs (except ULK1 and Beclin1) can be recruited at the primary cilium upon serum starvation or shear stress (Pampliega et al., 2013; Boukhalfa et al., 2020). In contrast to bulk autophagy initiation, where the ULK and PI3KIII complexes are essential for PI3P formation, autophagy induction in kidney epithelial cells submitted to fluid flow has been shown to be dependent on PI3P synthesis by the class II PI3Kα (PI3K-C2α) (Boukhalfa et al., 2020). Importantly, the PI3K-C2α kinase controls ciliogenesis and is relocated at the primary cilium upon shear stress (Franco et al., 2014; Boukhalfa et al., 2020). Moreover, PI3KC2α ± mice exhibit defects in autophagic stimulation and cell-size regulation in kidney tubular cells (Boukhalfa et al., 2020). Complementary studies are however needed to decipher the molecular signaling necessary to recruit and regulate the activity of PI3K-C2α at the primary-cilium upon shear stress. This would help to understand if the phagophore is directly produced at the vicinity of the primary cilium. How AMPK controls autophagy in the absence of ULK1/2 and Beclin1 is still an open question. An epigenetic regulation of autophagy by AMPK cannot be excluded in this setting (Li et al., 2017).
Conclusion
The past several years have illuminated the cross-talk between the primary cilium and the two major cellular catabolic pathways, i.e., the autophagic pathway and the ubiquitin proteasomesystem (UPS) (Orhon et al., 2015; Pampliega and Cuervo, 2016; Boukhalfa et al., 2019; Wiegering et al., 2019; Morel et al., 2021; Morleo et al., 2022; Senatore et al., 2022). Many studies have demonstrated that autophagy is engaged in the regulation of ciliogenesis by controlling the expression of factors such as IFT20 (Pampliega et al., 2013), OFD1 (Tang et al., 2013), MYH9/myosin 2 (Yamamoto et al., 2021), CP110 (Liu et al., 2021) or Kif19A (Arora et al., 2020). As such, the word “ciliophagy” has been adequately introduced to designate the selective degradation of ciliary proteins by the autophagic pathway (Cloonan et al., 2014). In addition, the ciliary proteins IFT20, OFD1 (Finetti et al., 2021; Morleo et al., 2021) and ATG16L1 (Boukhalfa et al., 2021) are engaged in the regulation of autophagy and ciliogenesis, respectively.
As discussed in this review, a major outcome of the regulation of the cross-talk between the primary cilium and autophagy is the contribution to metabolism, including the fitness of mitochondria. However, it can be surmised that this cross-talk has consequences beyond the regulation of cell metabolism, such as cell differentiation and major physiological processes such as memory acquisition. In line with this assumption, both the primary cilium and autophagy are engaged in white adipocyte differentiation (Singh et al., 2009b; Zhang et al., 2009; Hilgendorf et al., 2019; Zhang et al., 2021) and are known to be involved in hippocampal memory acquisition (Glatigny et al., 2019; Jovasevic et al., 2021; Bashford and Subramanian, 2022). Whether or not the primary cilium and the autophagic pathway communicate during these processes is still an open question. Undoubtedly, future studies will illuminate the importance of the cross-talk between autophagy and the primary cilium in biological and physiological responses to the environment.
Author contributions
AC-T, ND, and PC discussed the topic. PC wrote the ms, AC-T and ND contributed to the writing.
Funding
AC-T, ND, and PC are funded by INSERM, University of Paris Cité and Agence Nationale pour la Recherche (ECLIPSE-18-CE14-0006-02 to PC and MECHANOPHAGY-18-CE14-0026-01 to ND). AC-T is supported by a fellowship from “La Fondation Tourre.”
Acknowledgments
We thank Nicolas Kuperwasser for editing and proofreading.
Conflict of interest
The authors declare that the research was conducted in the absence of any commercial or financial relationships that could be construed as a potential conflict of interest.
Publisher’s note
All claims expressed in this article are solely those of the authors and do not necessarily represent those of their affiliated organizations, or those of the publisher, the editors and the reviewers. Any product that may be evaluated in this article, or claim that may be made by its manufacturer, is not guaranteed or endorsed by the publisher.
References
Akhshi, T., and Trimble, W. S. (2021). A non-canonical Hedgehog pathway initiates ciliogenesis and autophagy. J. Cell Biol. 220, e202004179. doi:10.1083/jcb.202004179
Anvarian, Z., Mykytyn, K., Mukhopadhyay, S., Pedersen, L. B., and Christensen, S. T. (2019). Cellular signalling by primary cilia in development, organ function and disease. Nat. Rev. Nephrol. 15, 199–219. doi:10.1038/s41581-019-0116-9
Arora, K., Lund, J. R., Naren, N. A., Zingarelli, B., and Naren, A. P. (2020). AC6 regulates the microtubule-depolymerizing kinesin KIF19A to control ciliary length in mammals. J. Biol. Chem. 295, 14250–14259. doi:10.1074/jbc.RA120.013703
Arts, H. H., Doherty, D., van Beersum, S. E., Parisi, M. A., Letteboer, S. J., Gorden, N. T., et al. (2007). Mutations in the gene encoding the basal body protein RPGRIP1L, a nephrocystin-4 interactor, cause Joubert syndrome. Nat. Genet. 39, 882–888. doi:10.1038/ng2069
Avalos, Y., Hernandez-Caceres, M. P., Lagos, P., Pinto-Nunez, D., Rivera, P., Burgos, P., et al. (2022). Palmitic acid control of ciliogenesis modulates insulin signaling in hypothalamic neurons through an autophagy-dependent mechanism. Cell Death Dis. 13, 659. doi:10.1038/s41419-022-05109-9
Ballabio, A., and Bonifacino, J. S. (2020). Lysosomes as dynamic regulators of cell and organismal homeostasis. Nat. Rev. Mol. Cell Biol. 21, 101–118. doi:10.1038/s41580-019-0185-4
Baracco, E. E., Castoldi, F., Durand, S., Enot, D. P., Tadic, J., Kainz, K., et al. (2019). α-Ketoglutarate inhibits autophagy. Aging (Albany NY) 11, 3418–3431. doi:10.18632/aging.102001
Bashford, A. L., and Subramanian, V. (2022). Hippocampals neurogenesis is impaired in mice with a deletion in the coiled coil domain of Talpid3-implications for Joubert syndrome. Hum. Mol. Genet. 31, 3245–3265. doi:10.1093/hmg/ddac095
Belibi, F., Zafar, I., Ravichandran, K., Segvic, A. B., Jani, A., Ljubanovic, D. G., et al. (2011). Hypoxia-inducible factor-1α (HIF-1α) and autophagy in polycystic kidney disease (PKD). Am. J. Physiol. Ren. Physiol. 300, F1235–F1243. doi:10.1152/ajprenal.00348.2010
Bhargava, P., and Schnellmann, R. G. (2017). Mitochondrial energetics in the kidney. Nat. Rev. Nephrol. 13, 629–646. doi:10.1038/nrneph.2017.107
Bodle, J. C., and Loboa, E. G. (2016). Concise review: Primary cilia: Control centers for stem cell lineage specification and potential targets for cell-based therapies. Stem Cells 34, 1445–1454. doi:10.1002/stem.2341
Boehlke, C., Kotsis, F., Patel, V., Braeg, S., Voelker, H., Bredt, S., et al. (2010). Primary cilia regulate mTORC1 activity and cell size through Lkb1. Nat. Cell Biol. 12, 1115–1122. doi:10.1038/ncb2117
Boukhalfa, A., Miceli, C., Avalos, Y., Morel, E., and Dupont, N. (2019). Interplay between primary cilia, ubiquitin-proteasome system and autophagy. Biochimie 166, 286–292. doi:10.1016/j.biochi.2019.06.009
Boukhalfa, A., Nascimbeni, A. C., Ramel, D., Dupont, N., Hirsch, E., Gayral, S., et al. (2020). PI3KC2α-dependent and VPS34-independent generation of PI3P controls primary cilium-mediated autophagy in response to shear stress. Nat. Commun. 11, 294. doi:10.1038/s41467-019-14086-1
Boukhalfa, A., Roccio, F., Dupont, N., Codogno, P., and Morel, E. (2021). The autophagy protein ATG16L1 cooperates with IFT20 and INPP5E to regulate the turnover of phosphoinositides at the primary cilium. Cell Rep. 35, 109045. doi:10.1016/j.celrep.2021.109045
Boya, P., Codogno, P., and Rodriguez-Muela, N. (2018). Autophagy in stem cells: Repair, remodelling and metabolic reprogramming. Development 145, dev146506. doi:10.1242/dev.146506
Boya, P., Reggiori, F., and Codogno, P. (2013). Emerging regulation and functions of autophagy. Nat. Cell Biol. 15, 713–720. doi:10.1038/ncb2788
Braun, D. A., and Hildebrandt, F. (2017). Cold Spring Harb. Perspect. Biol. 9, a028191. doi:10.1101/cshperspect.a028191
Chang, C., Shi, X., Jensen, L. E., Yokom, A. L., Fracchiolla, D., Martens, S., et al. (2021). Reconstitution of cargo-induced LC3 lipidation in mammalian selective autophagy. Sci. Adv. 7, eabg4922. doi:10.1126/sciadv.abg4922
Cloonan, S. M., Lam, H. C., Ryter, S. W., and Choi, A. M. (2014). Ciliophagy": The consumption of cilia components by autophagy. Autophagy 10, 532–534. doi:10.4161/auto.27641
Delous, M., Baala, L., Salomon, R., Laclef, C., Vierkotten, J., Tory, K., et al. (2007). The ciliary gene RPGRIP1L is mutated in cerebello-oculo-renal syndrome (Joubert syndrome type B) and Meckel syndrome. Nat. Genet. 39, 875–881. doi:10.1038/ng2039
Deretic, V., and Lazarou, M. (2022). A guide to membrane atg8ylation and autophagy with reflections on immunity. J. Cell Biol. 221, e202203083. doi:10.1083/jcb.202203083
Dong, K., Zhang, C., Tian, X., Coman, D., Hyder, F., Ma, M., et al. (2021). Renal plasticity revealed through reversal of polycystic kidney disease in mice. Nat. Genet. 53, 1649–1663. doi:10.1038/s41588-021-00946-4
Douanne, T., Stinchcombe, J. C., and Griffiths, G. M. (2021). Teasing out function from morphology: Similarities between primary cilia and immune synapses. J. Cell Biol. 220, e202102089. doi:10.1083/jcb.202102089
Ferreira, R. R., Fukui, H., Chow, R., Vilfan, A., and Vermot, J. (2019). The cilium as a force sensor-myth versus reality. J. Cell Sci. 132, jcs213496. doi:10.1242/jcs.213496
Finetti, F., Cassioli, C., Cianfanelli, V., Zevolini, F., Onnis, A., Gesualdo, M., et al. (2021). The intraflagellar transport protein IFT20 recruits ATG16L1 to early endosomes to promote autophagosome formation in T cells. Front. Cell Dev. Biol. 9, 634003. doi:10.3389/fcell.2021.634003
Finetti, F., Patrussi, L., Masi, G., Onnis, A., Galgano, D., Lucherini, O. M., et al. (2014). Specific recycling receptors are targeted to the immune synapse by the intraflagellar transport system. J. Cell Sci. 127, 1924–1937. doi:10.1242/jcs.139337
Franco, I., Gulluni, F., Campa, C. C., Costa, C., Margaria, J. P., Ciraolo, E., et al. (2014). PI3K class II alpha controls spatially restricted endosomal PtdIns3P and Rab11 activation to promote primary cilium function. Dev. Cell 28, 647–658. doi:10.1016/j.devcel.2014.01.022
Fujii, R., Hasegawa, S., Maekawa, H., Inoue, T., Yoshioka, K., Uni, R., et al. (2021). Decreased IFT88 expression with primary cilia shortening causes mitochondrial dysfunction in cisplatin-induced tubular injury. Am. J. Physiol. Ren. Physiol. 321, F278–F292. doi:10.1152/ajprenal.00673.2020
Fullgrabe, J., Klionsky, D. J., and Joseph, B. (2014). The return of the nucleus: Transcriptional and epigenetic control of autophagy. Nat. Rev. Mol. Cell Biol. 15, 65–74. doi:10.1038/nrm3716
Garcia-Gonzalo, F. R., and Reiter, J. F. (2012). Scoring a backstage pass: Mechanisms of ciliogenesis and ciliary access. J. Cell Biol. 197, 697–709. doi:10.1083/jcb.201111146
Gerhardt, C., Lier, J. M., Burmuhl, S., Struchtrup, A., Deutschmann, K., Vetter, M., et al. (2015). The transition zone protein Rpgrip1l regulates proteasomal activity at the primary cilium. J. Cell Biol. 210, 115–133. doi:10.1083/jcb.201408060
Gerhardt, C., Wiegering, A., Leu, T., and Ruther, U. (2016). Control of hedgehog signalling by the cilia-regulated proteasome. J. Dev. Biol. 4, E27. doi:10.3390/jdb4030027
Glatigny, M., Moriceau, S., Rivagorda, M., Ramos-Brossier, M., Nascimbeni, A. C., Lante, F., et al. (2019). Autophagy is required for memory formation and reverses age-related memory decline. Curr. Biol. 29, 435–448. doi:10.1016/j.cub.2018.12.021
Goetz, S. C., and Anderson, K. V. (2010). The primary cilium: A signalling centre during vertebrate development. Nat. Rev. Genet. 11, 331–344. doi:10.1038/nrg2774
Gubas, A., and Dikic, I. (2022). A guide to the regulation of selective autophagy receptors. FEBS J. 289, 75–89. doi:10.1111/febs.15824
Hajarnis, S., Lakhia, R., Yheskel, M., Williams, D., Sorourian, M., Liu, X., et al. (2017). microRNA-17 family promotes polycystic kidney disease progression through modulation of mitochondrial metabolism. Nat. Commun. 8, 14395. doi:10.1038/ncomms14395
He, C. (2022). Balancing nutrient and energy demand and supply via autophagy. Curr. Biol. 32, R684–R696. doi:10.1016/j.cub.2022.04.071
Hilgendorf, K. I., Johnson, C. T., Mezger, A., Rice, S. L., Norris, A. M., Demeter, J., et al. (2019). Omega-3 fatty acids activate ciliary FFAR4 to control adipogenesis. Cell 179, 1289–1305. doi:10.1016/j.cell.2019.11.005
Hsiao, C. J., Chang, C. H., Ibrahim, R. B., Lin, I. H., Wang, C. H., Wang, W. J., et al. (2018). Gli2 modulates cell cycle re-entry through autophagy-mediated regulation of the length of primary cilia. J. Cell Sci. 131, jcs221218. doi:10.1242/jcs.221218
Hu, Y., and Reggiori, F. (2022). Molecular regulation of autophagosome formation. Biochem. Soc. Trans. 50, 55–69. doi:10.1042/bst20210819
Hughes, J., Ward, C. J., Peral, B., Aspinwall, R., Clark, K., San Millan, J. L., et al. (1995). The polycystic kidney disease 1 (PKD1) gene encodes a novel protein with multiple cell recognition domains. Nat. Genet. 10, 151–160. doi:10.1038/ng0695-151
Ishimoto, Y., Inagi, R., Yoshihara, D., Kugita, M., Nagao, S., Shimizu, A., et al. (2017). Mitochondrial abnormality facilitates cyst formation in autosomal dominant polycystic kidney disease. Mol. Cell. Biol. 37, e0033717. doi:10.1128/MCB.00337-17
Jang, J., Wang, Y., Lalli, M. A., Guzman, E., Godshalk, S. E., Zhou, H., et al. (2016). Primary cilium-autophagy-nrf2 (PAN) Axis Activation commits human embryonic stem cells to a neuroectoderm fate. Cell 165, 410–420. doi:10.1016/j.cell.2016.02.014
Johansen, T., and Lamark, T. (2020). Selective autophagy: ATG8 family proteins, LIR motifs and cargo receptors. J. Mol. Biol. 432, 80–103. doi:10.1016/j.jmb.2019.07.016
Jovasevic, V., Zhang, H., Sananbenesi, F., Guedea, A. L., Soman, K. V., Wiktorowicz, J. E., et al. (2021). Primary cilia are required for the persistence of memory and stabilization of perineuronal nets. iScience 24, 102617. doi:10.1016/j.isci.2021.102617
Kaur, J., and Debnath, J. (2015). Autophagy at the crossroads of catabolism and anabolism. Nat. Rev. Mol. Cell Biol. 16, 461–472. doi:10.1038/nrm4024
Kaushik, S., and Cuervo, A. M. (2015). Degradation of lipid droplet-associated proteins by chaperone-mediated autophagy facilitates lipolysis. Nat. Cell Biol. 17, 759–770. doi:10.1038/ncb3166
Kaushik, S., and Cuervo, A. M. (2018). The coming of age of chaperone-mediated autophagy. Nat. Rev. Mol. Cell Biol. 19, 365–381. doi:10.1038/s41580-018-0001-6
Kawabata, T., and Yoshimori, T. (2020). Autophagosome biogenesis and human health. Cell Discov. 6, 33. doi:10.1038/s41421-020-0166-y
Khaminets, A., Behl, C., and Dikic, I. (2016). Ubiquitin-dependent and independent signals in selective autophagy. Trends Cell Biol. 26, 6–16. doi:10.1016/j.tcb.2015.08.010
Kim, J., Lee, J. E., Heynen-Genel, S., Suyama, E., Ono, K., Lee, K., et al. (2010). Functional genomic screen for modulators of ciliogenesis and cilium length. Nature 464, 1048–1051. doi:10.1038/nature08895
Kim, J. S., Kim, B., Lee, H., Thakkar, S., Babbitt, D. M., Eguchi, S., et al. (2015). Shear stress-induced mitochondrial biogenesis decreases the release of microparticles from endothelial cells. Am. J. Physiol. Heart Circ. Physiol. 309, H425–H433. doi:10.1152/ajpheart.00438.2014
Kim, S. W., Ehrman, J., Ahn, M. R., Kondo, J., Lopez, A. A. M., Oh, Y. S., et al. (2017). Shear stress induces noncanonical autophagy in intestinal epithelial monolayers. Mol. Biol. Cell 28, 3043–3056. doi:10.1091/mbc.E17-01-0021
Kwon, Y. T., and Ciechanover, A. (2017). The ubiquitin code in the ubiquitin-proteasome system and autophagy. Trends biochem. Sci. 42, 873–886. doi:10.1016/j.tibs.2017.09.002
Lahiri, V., Hawkins, W. D., and Klionsky, D. J. (2019). Watch what you (self-) eat: Autophagic mechanisms that modulate metabolism. Cell Metab. 29, 803–826. doi:10.1016/j.cmet.2019.03.003
Larsen, L. J., and Moller, L. B. (2020). Crosstalk of hedgehog and mTORC1 pathways. Cells 9, E2316. doi:10.3390/cells9102316
Lee, C. H., Kang, G. M., and Kim, M. S. (2022). Mechanisms of weight control by primary cilia. Mol. Cells 45, 169–176. doi:10.14348/molcells.2022.2046
Lemasters, J. J. (2014). Variants of mitochondrial autophagy: Types 1 and 2 mitophagy and micromitophagy (Type 3). Redox Biol. 2, 749–754. doi:10.1016/j.redox.2014.06.004
Levine, B., and Kroemer, G. (2019). Biological functions of autophagy genes: A disease perspective. Cell 176, 11–42. doi:10.1016/j.cell.2018.09.048
Li, S., Nagothu, K. K., Desai, V., Lee, T., Branham, W., Moland, C., et al. (2009). Transgenic expression of proximal tubule peroxisome proliferator-activated receptor-alpha in mice confers protection during acute kidney injury. Kidney Int. 76, 1049–1062. doi:10.1038/ki.2009.330
Li, X., Yu, W., Qian, X., Xia, Y., Zheng, Y., Lee, J. H., et al. (2017). Nucleus-translocated ACSS2 promotes gene transcription for lysosomal biogenesis and autophagy. Mol. Cell 66, 684–697. doi:10.1016/j.molcel.2017.04.026
Liu, M., Zhang, W., Li, M., Feng, J., Kuang, W., Chen, X., et al. (2021). NudCL2 is an autophagy receptor that mediates selective autophagic degradation of CP110 at mother centrioles to promote ciliogenesis. Cell Res. 31, 1199–1211. doi:10.1038/s41422-021-00560-3
Louwen, F., Ritter, A., Kreis, N. N., and Yuan, J. (2018). Insight into the development of obesity: Functional alterations of adipose-derived mesenchymal stem cells. Obes. Rev. 19, 888–904. doi:10.1111/obr.12679
Luijten, M. N., Basten, S. G., Claessens, T., Vernooij, M., Scott, C. L., Janssen, R., et al. (2013). Birt-Hogg-Dube syndrome is a novel ciliopathy. Hum. Mol. Genet. 22, 4383–4397. doi:10.1093/hmg/ddt288
Maeda, S., Yamamoto, H., Kinch, L. N., Garza, C. M., Takahashi, S., Otomo, C., et al. (2020). Structure, lipid scrambling activity and role in autophagosome formation of ATG9A. Nat. Struct. Mol. Biol. 27, 1194–1201. doi:10.1038/s41594-020-00520-2
Malicki, J. J., and Johnson, C. A. (2017). The cilium: Cellular antenna and central processing unit. Trends Cell Biol. 27, 126–140. doi:10.1016/j.tcb.2016.08.002
Menezes, L. F., Lin, C. C., Zhou, F., and Germino, G. G. (2016). Fatty acid oxidation is impaired in an orthologous mouse model of autosomal dominant polycystic kidney disease. EBioMedicine 5, 183–192. doi:10.1016/j.ebiom.2016.01.027
Miceli, C., Roccio, F., Penalva-Mousset, L., Burtin, M., Leroy, C., Nemazanyy, I., et al. (2020). The primary cilium and lipophagy translate mechanical forces to direct metabolic adaptation of kidney epithelial cells. Nat. Cell Biol. 22, 1091–1102. doi:10.1038/s41556-020-0566-0
Minami, S., Yamamoto, T., Takabatake, Y., Takahashi, A., Namba, T., Matsuda, J., et al. (2017). Lipophagy maintains energy homeostasis in the kidney proximal tubule during prolonged starvation. Autophagy 13, 1629–1647. doi:10.1080/15548627.2017.1341464
Mizushima, N., Levine, B., Cuervo, A. M., and Klionsky, D. J. (2008). Autophagy fights disease through cellular self-digestion. Nature 451, 1069–1075. doi:10.1038/nature06639
Mizushima, N., Yoshimori, T., and Ohsumi, Y. (2011). The role of Atg proteins in autophagosome formation. Annu. Rev. Cell Dev. Biol. 27, 107–132. doi:10.1146/annurev-cellbio-092910-154005
Mochizuki, T., Wu, G., Hayashi, T., Xenophontos, S. L., Veldhuisen, B., Saris, J. J., et al. (1996). PKD2, a gene for polycystic kidney disease that encodes an integral membrane protein. Science 272, 1339–1342. doi:10.1126/science.272.5266.1339
Morel, E., Dupont, N., and Codogno, P. (2021). Primary cilium-dependent autophagy in the response to shear stress. Biochem. Soc. Trans. 49, 2831–2839. doi:10.1042/BST20210810
Morleo, M., Brillante, S., Formisano, U., Ferrante, L., Carbone, F., Iaconis, D., et al. (2021). Regulation of autophagosome biogenesis by OFD1-mediated selective autophagy. EMBO J. 40, e105120. doi:10.15252/embj.2020105120
Morleo, M., Vieira, H. L. A., Pennekamp, P., Palma, A., Bento-Lopes, L., Omran, H., et al. (2022). Crosstalk between cilia and autophagy: Implication for human diseases. Autophagy 25, 1–20. doi:10.1080/15548627.2022.2067383
Mortimore, G. E., Lardeux, B. R., and Adams, C. E. (1988). Regulation of microautophagy and basal protein turnover in rat liver. Effects of short-term starvation. J. Biol. Chem. 263, 2506–2512. doi:10.1016/s0021-9258(18)69235-x
Mortimore, G. E., and Poso, A. R. (1987). Intracellular protein catabolism and its control during nutrient deprivation and supply. Annu. Rev. Nutr. 7, 539–564. doi:10.1146/annurev.nu.07.070187.002543
Mortimore, G. E., and Poso, A. R. (1984). Lysosomal pathways in hepatic protein degradation: Regulatory role of amino acids. Fed. Proc. 43, 1289–1294.
Nachury, M. V., and Mick, D. U. (2019). Establishing and regulating the composition of cilia for signal transduction. Nat. Rev. Mol. Cell Biol. 20, 389–405. doi:10.1038/s41580-019-0116-4
Nakatogawa, H., Suzuki, K., Kamada, Y., and Ohsumi, Y. (2009). Dynamics and diversity in autophagy mechanisms: Lessons from yeast. Nat. Rev. Mol. Cell Biol. 10, 458–467. doi:10.1038/nrm2708
Nakayama, K., and Katoh, Y. (2018). Ciliary protein trafficking mediated by IFT and BBSome complexes with the aid of kinesin-2 and dynein-2 motors. J. Biochem. 163, 155–164. doi:10.1093/jb/mvx087
Napolitano, G., and Ballabio, A. (2016). TFEB at a glance. J. Cell Sci. 129, 2475–2481. doi:10.1242/jcs.146365
Nishimura, T., and Tooze, S. A. (2020). Emerging roles of ATG proteins and membrane lipids in autophagosome formation. Cell Discov. 6, 32. doi:10.1038/s41421-020-0161-3
Ogasawara, Y., Tsuji, T., and Fujimoto, T. (2020). Multifarious roles of lipid droplets in autophagy - target, product, and what else? Semin. Cell Dev. Biol. 108, 47–54. doi:10.1016/j.semcdb.2020.02.013
Oku, M., and Sakai, Y. (2018). Three distinct types of microautophagy based on membrane dynamics and molecular machineries. Bioessays 40, e1800008. doi:10.1002/bies.201800008
Onishi, M., Yamano, K., Sato, M., Matsuda, N., and Okamoto, K. (2021). Molecular mechanisms and physiological functions of mitophagy. EMBO J. 40, e104705. doi:10.15252/embj.2020104705
Orhon, I., Dupont, N., Pampliega, O., Cuervo, A. M., and Codogno, P. (2015). Autophagy and regulation of cilia function and assembly. Cell Death Differ. 22, 389–397. doi:10.1038/cdd.2014.171
Orhon, I., Dupont, N., Zaidan, M., Boitez, V., Burtin, M., Schmitt, A., et al. (2016). Primary-cilium-dependent autophagy controls epithelial cell volume in response to fluid flow. Nat. Cell Biol. 18, 657–667. doi:10.1038/ncb3360
Padovano, V., Podrini, C., Boletta, A., and Caplan, M. J. (2018). Metabolism and mitochondria in polycystic kidney disease research and therapy. Nat. Rev. Nephrol. 14, 678–687. doi:10.1038/s41581-018-0051-1
Pala, R., Alomari, N., and Nauli, S. M. (2017). Primary cilium-dependent signaling mechanisms. Int. J. Mol. Sci. 18, E2272. doi:10.3390/ijms18112272
Pampliega, O., and Cuervo, A. M. (2016). Autophagy and primary cilia: Dual interplay. Curr. Opin. Cell Biol. 39, 1–7. doi:10.1016/j.ceb.2016.01.008
Pampliega, O., Orhon, I., Patel, B., Sridhar, S., Diaz-Carretero, A., Beau, I., et al. (2013). Functional interaction between autophagy and ciliogenesis. Nature 502, 194–200. doi:10.1038/nature12639
Pickles, S., Vigie, P., and Youle, R. J. (2018). Mitophagy and quality control mechanisms in mitochondrial maintenance. Curr. Biol. 28, R170–R185. doi:10.1016/j.cub.2018.01.004
Pietrocola, F., Galluzzi, L., Bravo-San Pedro, J. M., Madeo, F., and Kroemer, G. (2015). Acetyl coenzyme A: A central metabolite and second messenger. Cell Metab. 21, 805–821. doi:10.1016/j.cmet.2015.05.014
Pigino, G. (2021). Intraflagellar transport. Curr. Biol. 31, R530–R536. doi:10.1016/j.cub.2021.03.081
Pohl, C., and Dikic, I. (2019). Cellular quality control by the ubiquitin-proteasome system and autophagy. Science 366, 818–822. doi:10.1126/science.aax3769
Poillet-Perez, L., and White, E. (2019). Role of tumor and host autophagy in cancer metabolism. Genes Dev. 33, 610–619. doi:10.1101/gad.325514.119
Ritter, A., Louwen, F., and Yuan, J. (2018). Deficient primary cilia in obese adipose-derived mesenchymal stem cells: Obesity, a secondary ciliopathy? Obes. Rev. 19, 1317–1328. doi:10.1111/obr.12716
Roberts, M. A., and Olzmann, J. A. (2020). Protein quality control and lipid droplet metabolism. Annu. Rev. Cell Dev. Biol. 36, 115–139. doi:10.1146/annurev-cellbio-031320-101827
Rodriguez, C., Munoz, M., Contreras, C., and Prieto, D. (2021). AMPK, metabolism, and vascular function. FEBS J. 288, 3746–3771. doi:10.1111/febs.15863
Rowe, I., Chiaravalli, M., Mannella, V., Ulisse, V., Quilici, G., Pema, M., et al. (2013). Defective glucose metabolism in polycystic kidney disease identifies a new therapeutic strategy. Nat. Med. 19, 488–493. doi:10.1038/nm.3092
Satir, P., and Christensen, S. T. (2007). Overview of structure and function of mammalian cilia. Annu. Rev. Physiol. 69, 377–400. doi:10.1146/annurev.physiol.69.040705.141236
Sawa-Makarska, J., Baumann, V., Coudevylle, N., von Bulow, S., Nogellova, V., Abert, C., et al. (2020). Reconstitution of autophagosome nucleation defines Atg9 vesicles as seeds for membrane formation. Science 369, eaaz7714. doi:10.1126/science.aaz7714
Schneider, J. L., Suh, Y., and Cuervo, A. M. (2014). Deficient chaperone-mediated autophagy in liver leads to metabolic dysregulation. Cell Metab. 20, 417–432. doi:10.1016/j.cmet.2014.06.009
Schuck, S. (2020). Microautophagy - distinct molecular mechanisms handle cargoes of many sizes. J. Cell Sci. 133, jcs246322. doi:10.1242/jcs.246322
Schulze, R. J., Sathyanarayan, A., and Mashek, D. G. (2017). Breaking fat: The regulation and mechanisms of lipophagy. Biochim. Biophys. Acta. Mol. Cell Biol. Lipids 1862, 1178–1187. doi:10.1016/j.bbalip.2017.06.008
Senatore, E., Chiuso, F., Rinaldi, L., Intartaglia, D., Delle Donne, R., Pedone, E., et al. (2021). The TBC1D31/praja2 complex controls primary ciliogenesis through PKA-directed OFD1 ubiquitylation. EMBO J. 40, e106503. doi:10.15252/embj.2020106503
Senatore, E., Iannucci, R., Chiuso, F., Delle Donne, R., Rinaldi, L., and Feliciello, A. (2022). Pathophysiology of primary cilia: Signaling and proteostasis regulation. Front. Cell Dev. Biol. 10, 833086. doi:10.3389/fcell.2022.833086
Shim, M. S., Nettesheim, A., Dixon, A., and Liton, P. B. (2021). Primary cilia and the reciprocal activation of AKT and SMAD2/3 regulate stretch-induced autophagy in trabecular meshwork cells. Proc. Natl. Acad. Sci. U. S. A. 118, e2021942118. doi:10.1073/pnas.2021942118
Shimada, I. S., and Kato, Y. (2022). Ciliary signaling in stem cells in health and disease: Hedgehog pathway and beyond. Semin. Cell Dev. Biol. 129, 115–125. doi:10.1016/j.semcdb.2022.04.011
Singh, R., Kaushik, S., Wang, Y., Xiang, Y., Novak, I., Komatsu, M., et al. (2009). Autophagy regulates lipid metabolism. Nature 458, 1131–1135. doi:10.1038/nature07976
Singh, R., Xiang, Y., Wang, Y., Baikati, K., Cuervo, A. M., Luu, Y. K., et al. (2009). Autophagy regulates adipose mass and differentiation in mice. J. Clin. Invest. 119, 3329–3339. doi:10.1172/JCI39228
Strong, A. L., Burow, M. E., Gimble, J. M., and Bunnell, B. A. (2015). Concise review: The obesity cancer paradigm: Exploration of the interactions and crosstalk with adipose stem cells. Stem Cells 33, 318–326. doi:10.1002/stem.1857
Struchtrup, A., Wiegering, A., Stork, B., Ruther, U., and Gerhardt, C. (2018). The ciliary protein RPGRIP1L governs autophagy independently of its proteasome-regulating function at the ciliary base in mouse embryonic fibroblasts. Autophagy 14, 567–583. doi:10.1080/15548627.2018.1429874
Szymanska, K., Boldt, K., Logan, C. V., Adams, M., Robinson, P. A., Ueffing, M., et al. (2022). Regulation of canonical Wnt signalling by the ciliopathy protein MKS1 and the E2 ubiquitin-conjugating enzyme UBE2E1. Elife 11, e57593. doi:10.7554/eLife.57593
Tang, Z., Lin, M. G., Stowe, T. R., Chen, S., Zhu, M., Stearns, T., et al. (2013). Autophagy promotes primary ciliogenesis by removing OFD1 from centriolar satellites. Nature 502, 254–257. doi:10.1038/nature12606
Tasset, I., and Cuervo, A. M. (2016). Role of chaperone-mediated autophagy in metabolism. FEBS J. 283, 2403–2413. doi:10.1111/febs.13677
Tekirdag, K., and Cuervo, A. M. (2018). Chaperone-mediated autophagy and endosomal microautophagy: Joint by a chaperone. J. Biol. Chem. 293, 5414–5424. doi:10.1074/jbc.R117.818237
Viau, A., Bienaime, F., Lukas, K., Todkar, A. P., Knoll, M., Yakulov, T. A., et al. (2018). Cilia-localized LKB1 regulates chemokine signaling, macrophage recruitment, and tissue homeostasis in the kidney. EMBO J. 37, e98615. doi:10.15252/embj.201798615
Viau, A., Kotsis, F., Boehlke, C., Braeg, S., Klein, M., Nitschke, R., et al. (2020). Divergent function of polycystin 1 and polycystin 2 in cell size regulation. Biochem. Biophys. Res. Commun. 521, 290–295. doi:10.1016/j.bbrc.2019.10.074
Vion, A. C., Kheloufi, M., Hammoutene, A., Poisson, J., Lasselin, J., Devue, C., et al. (2017). Autophagy is required for endothelial cell alignment and atheroprotection under physiological blood flow. Proc. Natl. Acad. Sci. U. S. A. 114, E8675–E8684. doi:10.1073/pnas.1702223114
Walch, L., Copic, A., and Jackson, C. L. (2015). Fatty acid metabolism meets organelle dynamics. Dev. Cell 32, 657–658. doi:10.1016/j.devcel.2015.03.008
Wang, L., Liu, C., Yang, B., Zhang, H., Jiao, J., Zhang, R., et al. (2022). SARS-CoV-2 ORF10 impairs cilia by enhancing CUL2ZYG11B activity. J. Cell Biol. 221, e202108015. doi:10.1083/jcb.202108015
Wang, S., Zhuang, S., and Dong, Z. (2021). IFT88 deficiency in proximal tubular cells exaggerates cisplatin-induced injury by suppressing autophagy. Am. J. Physiol. Ren. Physiol. 321, F269–F277. doi:10.1152/ajprenal.00672.2020
Warner, G., Hein, K. Z., Nin, V., Edwards, M., Chini, C. C., Hopp, K., et al. (2016). Food restriction ameliorates the development of polycystic kidney disease. J. Am. Soc. Nephrol. 27, 1437–1447. doi:10.1681/ASN.2015020132
Wheway, G., Schmidts, M., Mans, D. A., Szymanska, K., Nguyen, T. T., Racher, H., et al. (2015). An siRNA-based functional genomics screen for the identification of regulators of ciliogenesis and ciliopathy genes. Nat. Cell Biol. 17, 1074–1087. doi:10.1038/ncb3201
Wiegering, A., Ruther, U., and Gerhardt, C. (2019). The role of primary cilia in the crosstalk between the Ubiquitin(-)Proteasome system and autophagy. Cells 8, E241. doi:10.3390/cells8030241
Wu, L. H., Chang, H. C., Ting, P. C., and Wang, D. L. (2018). Laminar shear stress promotes mitochondrial homeostasis in endothelial cells. J. Cell. Physiol. 233, 5058–5069. doi:10.1002/jcp.26375
Xiang, W., Jiang, T., Hao, X., Wang, R., Yao, X., Sun, K., et al. (2019). Primary cilia and autophagy interaction is involved in mechanical stress mediated cartilage development via ERK/mTOR axis. Life Sci. 218, 308–313. doi:10.1016/j.lfs.2019.01.001
Xie, Y., Li, J., Kang, R., and Tang, D. (2020). Interplay between lipid metabolism and autophagy. Front. Cell Dev. Biol. 8, 431. doi:10.3389/fcell.2020.00431
Yamamoto, K., Imamura, H., and Ando, J. (2018). Shear stress augments mitochondrial ATP generation that triggers ATP release and Ca(2+) signaling in vascular endothelial cells. Am. J. Physiol. Heart Circ. Physiol. 315, H1477–H1485. doi:10.1152/ajpheart.00204.2018
Yamamoto, T., Takabatake, Y., Takahashi, A., Kimura, T., Namba, T., Matsuda, J., et al. (2017). High-Fat diet-induced lysosomal dysfunction and impaired autophagic flux contribute to lipotoxicity in the kidney. J. Am. Soc. Nephrol. 28, 1534–1551. doi:10.1681/ASN.2016070731
Yamamoto, Y., Chino, H., Tsukamoto, S., Ode, K. L., Ueda, H. R., and Mizushima, N. (2021). NEK9 regulates primary cilia formation by acting as a selective autophagy adaptor for MYH9/myosin IIA. Nat. Commun. 12, 3292. doi:10.1038/s41467-021-23599-7
Yanardag, S., and Pugacheva, E. N. (2021). Primary cilium is involved in stem cell differentiation and renewal through the regulation of multiple signaling pathways. Cells 10, 1428. doi:10.3390/cells10061428
Yang, D. J., Tran, L. T., Yoon, S. G., Seong, J. K., Shin, D. M., Choi, Y. H., et al. (2022). Primary cilia regulate adaptive responses to fasting. Metabolism 135, 155273. doi:10.1016/j.metabol.2022.155273
Yang, D., Wu, X., Wang, W., Zhou, Y., and Wang, Z. (2022). Ciliary type III adenylyl cyclase in the VMH is crucial for high-fat diet-induced obesity mediated by autophagy. Adv. Sci. 9, e2102568. doi:10.1002/advs.202102568
Yim, W. W., and Mizushima, N. (2020). Lysosome biology in autophagy. Cell Discov. 6, 6. doi:10.1038/s41421-020-0141-7
Zemirli, N., Boukhalfa, A., Dupont, N., Botti, J., Codogno, P., and Morel, E. (2019). The primary cilium protein folliculin is part of the autophagy signaling pathway to regulate epithelial cell size in response to fluid flow. Cell Stress 3, 100–109. doi:10.15698/cst2019.03.180
Zhan, M., Brooks, C., Liu, F., Sun, L., and Dong, Z. (2013). Mitochondrial dynamics: Regulatory mechanisms and emerging role in renal pathophysiology. Kidney Int. 83, 568–581. doi:10.1038/ki.2012.441
Zhang, D., Xing, Y., Li, W., Yang, F., Lang, Y., Yang, J., et al. (2018). Renal tubules transcriptome reveals metabolic maladaption during the progression of ischemia-induced acute kidney injury. Biochem. Biophys. Res. Commun. 505, 432–438. doi:10.1016/j.bbrc.2018.08.111
Zhang, S., Peng, X., Yang, S., Li, X., Huang, M., Wei, S., et al. (2022). The regulation, function, and role of lipophagy, a form of selective autophagy, in metabolic disorders. Cell Death Dis. 13, 132. doi:10.1038/s41419-022-04593-3
Zhang, Y., Goldman, S., Baerga, R., Zhao, Y., Komatsu, M., and Jin, S. (2009). Adipose-specific deletion of autophagy-related gene 7 (atg7) in mice reveals a role in adipogenesis. Proc. Natl. Acad. Sci. U. S. A. 106, 19860–19865. doi:10.1073/pnas.0906048106
Zhang, Y., Hao, J., Tarrago, M. G., Warner, G. M., Giorgadze, N., Wei, Q., et al. (2021). FBF1 deficiency promotes beiging and healthy expansion of white adipose tissue. Cell Rep. 36, 109481. doi:10.1016/j.celrep.2021.109481
Zhao, Y. G., Codogno, P., and Zhang, H. (2021). Machinery, regulation and pathophysiological implications of autophagosome maturation. Nat. Rev. Mol. Cell Biol. 22, 733–750. doi:10.1038/s41580-021-00392-4
Keywords: cilium, cell signaling, macroautophagy, mechanical forces, mitochondria
Citation: Claude-Taupin A, Dupont N and Codogno P (2022) Autophagy and the primary cilium in cell metabolism: What’s upstream?. Front. Cell Dev. Biol. 10:1046248. doi: 10.3389/fcell.2022.1046248
Received: 16 September 2022; Accepted: 25 October 2022;
Published: 09 November 2022.
Edited by:
David A. Tumbarello, University of Southampton, United KingdomReviewed by:
Eugenia Morselli, Universidad de San Sebastián, ChilePaolo Grumati, Telethon Institute of Genetics and Medicine (TIGEM), Italy
Copyright © 2022 Claude-Taupin, Dupont and Codogno. This is an open-access article distributed under the terms of the Creative Commons Attribution License (CC BY). The use, distribution or reproduction in other forums is permitted, provided the original author(s) and the copyright owner(s) are credited and that the original publication in this journal is cited, in accordance with accepted academic practice. No use, distribution or reproduction is permitted which does not comply with these terms.
*Correspondence: Aurore Claude-Taupin, YXVyb3JlLmNsYXVkZS10YXVwaW5AaW5zZXJtLmZy; Nicolas Dupont, bmljb2xhcy5kdXBvbnRAaW5zZXJtLmZy; Patrice Codogno, cGF0cmljZS5jb2RvZ25vQGluc2VybS5mcg==