- 1Laboratory of Immunoregulation and Inflammation, Institut Pasteur de Montevideo, Montevideo, Uruguay
- 2Immunobiology Department, Faculty of Medicine, University of the Republic, Montevideo, Uruguay
- 3Laboratory of Vascular Biology and Drug Development, Institut Pasteur de Montevideo, Montevideo, Uruguay
Characterizing immune regulatory pathways is critical to understand physiological and pathophysiological processes as well as to identify novel immunotherapeutic targets. The cation channel TMEM176B has emerged in the last years as a potential new immunoregulatory player and pharmacological target. Here, we review how expression data, clinical associations of genetic variants and functional studies support a dual role for TMEM176B in regulating immune responses. Thus, TMEM176B can inhibit effector immune responses in some settings whereas it may also promote immunity by supporting antigen presentation in others. We also discuss a potential role for TMEM176B in regulating type 2 and 3 immunity and comment recent data on modulation of DC biology and inflammasome activation as well as CD8+ T cell responses. Understanding the role of TMEM176B in immunity is critical to propose rational pharmacological approaches targeting this channel.
Introduction
The immune system plays a key role in maintaining systemic homeostasis. To do so, immune responses aim at eliminating internal and external threats without damaging healthy tissues and organs. Such a challenging task needs to be controlled by cellular and molecular mechanisms that trigger the right response at the right place and for the right time. Antigens can trigger either effector players aiming at eliminating them or regulatory strategies that rather protect the triggers (Mellor and Munn, 2008). Protective responses result from tight regulation of effector and regulatory arms. In scenarios such as infections and cancer, effector responses are needed whereas regulatory players may be harmful. On the other hand, in pregnancy, transplantation and autoimmunity, regulatory responses will pave the way for homeostasis whereas effector pathways will lead to pathology. Moreover, immunotherapeutic interventions can target immune players to restore the stationary state. Thus, a deep characterization of immune-regulatory strategies is of paramount importance to understand physiology and pathology as well as to rationally develop immunotherapeutic approaches.
The characterization of immune checkpoints such as CTLA-4 and PD-1 has clearly shown that understanding immune regulation at the physiological level can impact on therapy at the clinics. Immune checkpoints can be defined as powerful molecular players that inhibit effector immune responses to prevent damage to healthy tissues. Accordingly, mice deficient in Pdcd1 (Nishimura et al., 1999, 2001; Okazaki et al., 2003) and Ctla4 (Tivol et al., 1995; Waterhouse et al., 1995) suffer from devastating autoimmunity. In humans, blockade of CTLA-4 and PD-1 triggers strong anti-tumoral immune responses in some patients, also increasing the odds of suffering from immune-related adverse events (Ribas and Wolchok, 2018). In complement to immune checkpoints, other immune regulatory strategies, which are also potential therapeutic targets, are needed to achieve homeostasis. In contrast to immune checkpoints, other immune regulators can work as two-way switches and in some circumstances control effector mechanisms at different degrees or even promote them. This dual behavior has been reported for highly relevant immune players such as IL-2 (Pol et al., 2020) and type 1 and 2 interferons (Lee and Ashkar, 2018). In this context, it may be more difficult to anticipate a functional outcome when intervening the pathway. However, its rational modulation may lead to a more nuanced effect and thus, potentially uncoupling protective effector immune responses and immune-mediated damage. Nevertheless, a deep knowledge of the pathway is needed to develop rational immune interventions to avoid unexpected outcomes.
TMEM176B as a new player in the landscape of immune regulation
TMEM176B is an intracellular, acid-sensitive, non-specific cation channel (Segovia et al., 2014) which has been linked to the membrane-spanning 4A (MS4A) family (Louvet et al., 2005; Segovia et al., 2014; Eon Kuek et al., 2016; Mattiola et al., 2021). TMEM176B shares 30% identity with TMEM176A, another member of the MS4A family. These are ubiquitous proteins highly expressed in primary and secondary lymphoid organs, colon, lung and liver (Louvet et al., 2005; Condamine et al., 2010). Within leukocytes, it is highly expressed by the myeloid compartment, although expression by RORγT+ cells such as ILC3, Th17 and γδ T cells has been described (Drujont et al., 2016). Early studies by Cuturi and others have shown that in rat allotransplantation models Tmem176b expression was associated with immunological tolerance, suggesting a potential immunoregulatory role for this protein (Louvet et al., 2005). Accordingly, high TMEM176B expression in the tumor infiltrate in human colon cancer correlates with poor survival (Segovia et al., 2019). TMEM176B expression in tumor biopsies has also been associated with resistance to immune checkpoint blockers in melanoma patients (Segovia et al., 2019). On the other hand, TMEM176B expression in peripheral blood has been associated with clinical responses to anti-TNF therapy in rheumatoid arthritis (Wysocki and Paradowska-Gorycka, 2022). In multiple sclerosis, TMEM176B has been reported as a member of the gene expression signature (Nickles et al., 2013). In particular, the A134T genetic variant of TMEM176B could be associated with multiple sclerosis although this has not been formally proven. (Nickles et al., 2013). Moreover, this genetic variant has been linked to beneficial prognosis in colorectal cancer (Cambui et al., 2022). In the last years we started to get some insight on the potential role of this molecule in immunoregulatory mechanisms. Here we review the literature supporting a potential dual role for TMEM176B in regulating immunity.
TMEM176B expression in DCs and RORγT+ cells
To understand the functional role played by TMEM176B in the immune system, characterizing its cellular expression and subcellular localization is critical. Within rat leukocytes, Tmem176b was shown to be highly expressed by the myeloid cell compartment (Louvet et al., 2005). Specifically, high Tmem176b expression at the mRNA level was detected in rat peritoneal macrophages and in splenic CD103+ (OX62) dendritic cells. Within those cells, Tmem176b was expressed at higher levels in the CD4+ subset (rat DCs thought to resemble type 2 conventional DCs) versus CD4− cells. Accordingly, immunofluorescence studies in the spleen showed that TMEM176B staining co-localized with MHC class II and CD172 as well as with CD68 (Louvet et al., 2005). In mice, Tmem176b was expressed by both type 1 and type 2 cDCs, although expression levels were higher in the latter (Condamine et al., 2010; Crozat et al., 2011; Segovia et al., 2014). In tumor-draining lymph nodes, Tmem176b expression in cDC2 was shown to be supported by regulatory T (Treg) CD4+ cells (Binnewies et al., 2019), suggesting an immunoregulatory role for this cation channels in cDC2. Moreover, Tmem176b expression was associated with regulation of immune responses by migratory DCs (Anandasabapathy et al., 2014).
Recently, transcriptomic studies at the single-cell level have revealed an increasing complexity of leukocyte subsets. Particularly, although cDC1 (XCR1+) are thought to represent a homogeneous population, cDC2 (CD1C+) have been shown to gather different subsets in mice and humans. In human healthy blood, Villani et al. have identified two subpopulations within CD14− CD1C+ DCs, DC2 and DC3 (Villani et al., 2017). DC3 are CD11Clo, CD1Clo, CD163+, CD36+ whereas DC2 are CD11C+, CD1C+, CD32B+. DC3 differentially expressed inflammatory genes such as inflammasome-related genes as well as TMEM176B. Functionally, both subsets stimulated the proliferation of allogeneic CD4+ and CD8+ T cell proliferation in vitro (Villani et al., 2017). Dutertre et al. then added more phenotypic, functional and molecular heterogeneity to cDC2s (Dutertre et al., 2019). They showed that human cDC2 comprise CD5+ DC2 and CD5− DC3. Within the DC3 compartment, they described three clusters: CD163-, CD163- CD14+ and CD163+ CD14+. CD163+ CD14+ DC3 expressed high levels of inflammasome-related genes (Dutertre et al., 2019). CD1C+ CD163+ DC3s develop in a GM-CSF-dependent pathway and have been identified as immediate precursors of CD14+ CD1C+ CD163+ FcεRI+ inflammatory DCs (Bourdely et al., 2020). In cells stimulated with Toll-like receptors agonists (TLRs), TMEM176B was differentially expressed in DC3 versus cDC2 (Bourdely et al., 2020). Functionally, stimulated DC3 secreted higher levels of TNF and IL-1β as compared to cDC2. Furthermore, DC3 were able to prime naïve CD4+ and CD8+ T cells in vitro. Moreover, DC3 efficiently trigger CD103 expression in CD8+ T cells, which is a hallmark of tissue resident memory (TRM) cells. Inflammatory DC3 were expanded in the blood of systemic lupus erythematosus patients and correlated with disease activity (Dutertre et al., 2019). Accordingly, TMEM176B emerges as a potential target to control the inflammatory activity of DC3 cells in autoimmunity settings. Thus, unraveling the role of TMEM176B in DC3 is critical to understand its physiological and pathological impact.
It has been classically accepted that subsets of cDC specialize in the activation of particular T cell populations. Thus, cDC1 initiate CD8+ T cell responses whereas cDC2 prime CD4+ T cells (Dudziak et al., 2007). Nevertheless, the DC3 subset of cDC2 has been shown to activate CD4+ and CD8+ T cells (Villani et al., 2017; Bourdely et al., 2020). Human CD14+ CD163+ DC3 have been shown to specifically trigger Th2 and Th17 differentiation in comparison to CD5+ DC2, CD14− CD163- and CD14+ CD163- DC3. Moreover, lung cDC2 have been shown to promote differentiation of Th2 or Th17 cells depending on their maturation stage (Izumi et al., 2021). Thus, TMEM176B which is associated with the immature stage of DCs may regulate Th2 and/or Th17 differentiation in these subsets. In fact, Tmem176b was also found to be expressed in a Th2-driving CD11c+ PD-L2+ IRF-4+ CD301b+ DC subset in murine skin-draining lymph nodes (Gao et al., 2013). However, the functional role of Tmem176b in that subset and in type 2 immune responses remains to be elucidated.
In the tumor-draining lymph node of mice, Tmem176b deletion was associated with increased Caspase-1 activation in CD11b+ cDCs (cDC2) as well as with increased CD4+ RORγT+ cells versus WT animals (Segovia et al., 2019). In vitro restimulation of lymph node cells with tumoral antigens triggered IL-17 production in cells from Tmem176b−/− but not WT mice (Segovia et al., 2019). Thus, Tmem176b expression in cDC2/DC3 may control differentiation of Th17 cells.
TMEM176B was also shown to be expressed in RORγT+ cells such as human and mouse ILC3, Th17 and γδ T cells (Drujont et al., 2016). In human and mouse cDC2, TMEM176B expression has been associated with a subset of proinflammatory RORγT+ cells (Brown et al., 2019). Strikingly, Tmem176b was identified as one of a few genes that were dependent on RORγT in Th17 cells (Ciofani et al., 2012). Thus, Tmem176b may help to determine the differentiation program in Th17 cells. However, the biological role of intrinsic TMEM176B in RORγT+ cells remain elusive. In certain circumstances, Th17 cells can ensemble inflammasomes leading to Caspase-8 activation (Martin et al., 2016; Zhang et al., 2022). Further work is needed to determine whether Tmem176b may control inflammasome activation and/or other relevant processes in Th17 cells.
Control of DC biology by TMEM176B
TMEM176B is highly expressed by mouse bone marrow-derived DCs (BMDCs) and by human monocyte-derived DCs (MoDCs) (Louvet et al., 2005). Treatment with CD40L and TNF plus poly I: C down-regulated TMEM176B expression in rat splenic DCs and human Mo-DCs respectively (Louvet et al., 2005). Functionally, Tmem176b over-expression in BMDCs inhibited basal expression of MHC class II and CD86 and after LPS treatment (Louvet et al., 2005). In mouse BMDCs, Tmem176a and Tmem176b expression was down-regulated by LPS or poly I:C treatment (Condamine et al., 2010). Moreover, knock-down of TMEM176B or TMEM176A was associated with increased CD40, CD80, and CD86 expression as well as with increased allostimulatory capacity on lymph node cells (Condamine et al., 2010). In contrast, phenotypic analysis of Tmem176b−/− BMDCs showed no differences with WT cells (Segovia et al., 2014).
Determining the subcellular localization of TMEM176B has been critical to add functional data on the role of this protein in DCs. TMEM176B has been shown to be localized at the Golgi apparatus (Drujont et al., 2016; Lancien et al., 2021) and at endosomes and phagosomes in human and mouse DCs (Segovia et al., 2014). Given that TMEM176B belongs to a family (MS4A) that includes ion channels, we speculated that this protein may regulate endophagosomal pH. Indeed, we have shown that TMEM176B can transport cation channels across biological membranes. Moreover, endophagosomal pH is tightly regulated in DCs through the activity of vacuolar ATPase (V-ATPase) and NADPH oxidase 2 (NOX2) (Savina et al., 2006). We showed that Tmem176b−/− BMDCs display a transitory alkalinized phagosomal pH in comparison to WT cells (Segovia et al., 2014). Moreover, Na+ substitution with the membrane-impermeable molecule NMDG in the culture media, alkalinized phagosomes in WT but not in Tmem176b−/− BMDCs. These results suggested that TMEM176B might be responsible for a counterion conductance that may support V-ATPase activity. Accordingly, V-ATPase inhibition with Bafilomycin-1 alkalinized phagosomes in WT but not in Tmem176b−/− BMDCs (Segovia et al., 2014). This result, together with the alkalinized phagosomal pH, suggest that V-ATPase is inhibited in Tmem176b−/− BMDCs. Thus, we proposed that a Tmem176b-dependent counterion conductance supports V-ATPase activity leading to phagosomal pH regulation (Figure 1).
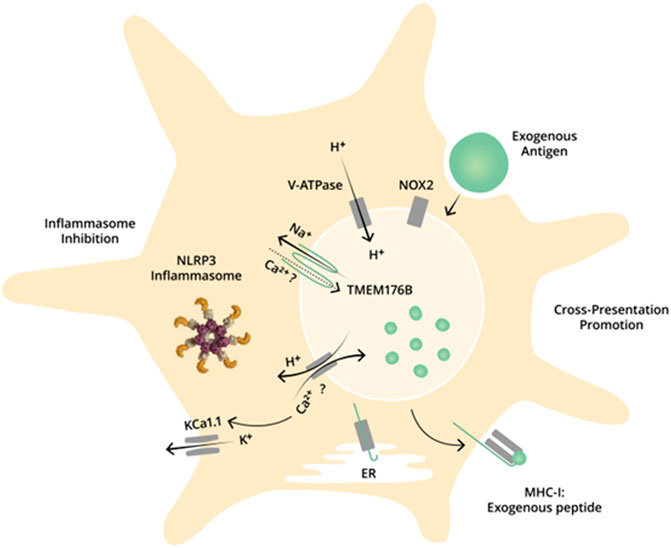
FIGURE 1. Dual role of TMEM176B in DCs: promotion of antigen cross-presentation and inhibition of inflammasome activation. TMEM176B at the endophagosmal membrane in DCs. TMEM176B exports Na+ from the endophagosomal lumen to the cytosol. This conductance alleviates the electrochemical gradient to V-ATPase, promoting the pumping of H+ into the lumen. A neutral pH in the endophagosomal lumen in DCs is critical to process antigens through the cross-presentation pathway. Accordingly, deletion of Tmem176b is associated with diminished V-ATPase activity, alkalinized phagosomal pH and impaired antigen cross-presentation. Moreover, TMEM176B inhibits NLRP3 inflammasome activation through ionic mechanisms. Tmem176b deletion is associated with KCa1.1-dependent inflammasome activation. TMEM176B controls cytosolic Ca2+ levels although the mechanisms of this effect remain unknown. Ca2+ might be internalized via TMEM176B into the phagosomal lumen and/or TMEM176B activity may promote H+/Ca2+ interchange, leading to decreased cytosolic Ca2+.
Antigen presentation to CD8+ T cells through the cross-presentation pathway strongly depends on neutral phagosomal pH within DCs. In fact, processing of different antigens was altered in Tmem176b−/− DCs, leading to deficient cross-presentation ability of those cells in comparison to WT ones. In vivo, the diminished antigen processing capacity of Tmem176b−/− tolerogenic BMDCs was associated with reduced numbers of CD8+ Treg cells and shorter allograft survival in comparison to animals injected with WT tolerogenic BMDCs. It has also been suggested that genetic deletion of both Tmem176b and Tmem176a may affect antigen processing through the MHC class II pathway in cDC2 (Lancien et al., 2021). Thus, antagonism of both molecules may inhibit antigen presentation and effector immune responses in some circumstances although Lancien et al. did not succeed in finding significant differences in EAE severity when comparing WT and Tmem176a−/−Tmem176b−/− animals (Lancien et al., 2021).
TMEM176B-dependent intracellular ion transport
Characterizing TMEM176B as an ion channel has been a milestone in the research targeting this molecule. Understanding its molecular function has been key to identify inhibitory and activating compounds (Segovia et al., 2019; Duhalde Vega et al., 2022) and has the potential to help understand the functional impact (gain or loss of function) of clinically relevant genetic variants. Different lines of evidence led us to propose the hypothesis that TMEM176B may be an ion channel. Its localization in endophagosomal membranes (where pH is tightly regulated) together with significant impacts on excitable tissues supported this possibility. Specifically, transgenic rats over-expressing TMEM176B showed a prominent vacuolar disease in skeletal muscle (Cuturi and others, unpublished). Moreover, a line of Tmem176b−/− mice was shown to suffer from cerebellar ataxia in two-thirds of animals (Maeda et al., 2006). This defect was associated with a severe loss of granule neurons in the cerebellum. Moreover, other members of the MS4A family such as CD20 and MS4A2 are bona fide ion channels (Mattiola et al., 2021). Thus, in collaboration with the electrophysiologist Pierre Charnet we showed that TMEM176B overexpression in Xenopus oocytes was associated with an acid-sensitive, slow inward conductance (Segovia et al., 2014). Substitution of extracellular Na+ with NMDG as well as a reversal potential near 0V helped us to determine that TMEM 176B was a non-selective cation channel (Segovia et al., 2014). TMEM176B physically interacts with TMEM176A (Condamine et al., 2010; Cuajungco et al., 2012) and over-expression of both proteins triggers a conductance with greater amplitude than the ones obtained with each molecule separately (Drujont et al., 2016). However, we lack information on the stoichiometry as well as potential functional differences of homo and hetero channels. TMEM176A may also interact with other channels such as TRPML2 (Cuajungco et al., 2014).
TMEM176B-dependent ion transport in phagosomes certainly impacts on cytosolic processes. Inflammasome activation critically depends on the cytosolic content of cations and anions (Gong et al., 2018). Inflammasomes are cytosolic multiprotein complexes that sense cellular stress and lead to Caspase-1-dependent activation of IL-1β and IL-18 (Rathinam and Fitzgerald, 2016). Tmem176b−/− DCs secrete larger quantities of IL-1β and IL-18 than WT cell when stimulated with NLRP3 triggers such as ATP, nigericin and alumina particles. Accordingly, TMEM176B over-expression significantly blocked IL-1βsecretion triggered by nigericin in human THP-1 macrophages. In vivo, i. p ATP injection triggered enhanced recruitment of peritoneal neutrophils in Tmem176b−/− versus WT animals. Casp1 deletion in Tmem176b−/− mice completely blocked neutrophils recruitment, showing that the observed enhanced recruitment in Tmem176b−/− mice was inflammasome-dependent. In human primary monocytes, pharmacological activation of TMEM176B with the flavonoid isoquercetin inhibited IL-1β secretion and Caspase-1 activation triggered by SARS-CoV-2 (Duhalde Vega et al., 2022). Moreover, TMEM176B overexpression completely blocked IL-1β secretion and Caspase-1 activation triggered by the ion channel SARS-CoV-2 envelope (E) protein (Duhalde Vega et al., 2022). TMEM176B therefore inhibits inflammasome activation, probably through ionic mechanisms (Figure 1). In fact, Tmem176b−/− BMDCs showed enhanced cytosolic Ca2+ versus WT cells when stimulated with ATP. Accordingly, cytosolic Ca++ chelation with BAPTA-AM completely blocked IL-1β secretion in WT and Tmem176b−/− BMDCs (Segovia et al., 2019). Furthermore, blockade of K+ efflux also inhibited IL-1βsecretion in WT and Tmem176b−/− BMDCs. Accordingly, IL-1β secretion in Tmem176b−/− BMDCs was highly sensitive to blockade of the Ca2+-activated K+ channels KCa3.1 (Segovia et al., 2019), which are involved in inflammasome activation (Eugenia Schroeder et al., 2017). Thus, TMEM176B may inhibit inflammasome activation by controlling cytosolic Ca++. Nevertheless, the intimate mechanisms of this effect remain unknown.
TMEM176B and CD8+ T cell responses: Je t’aime, moi non plus
Inflammasome activation can impact on adaptive immune responses and anti-tumoral immunity (Segovia et al., 2019; Hill et al., 2020; Segovia et al., 2020). In mouse experimental models, genetic deletion of Tmem176b was associated with controlled tumor progression in EG7 lymphoma, MC38 colon and LL2 lung cancer. Moreover, anti-tumoral CD8+ T cell responses were strongly reinforced in Tmem176b−/− versus WT animals. In fact, depletion of the CD8 compartment abolished the protective effect observed in Tmem176b−/− mice. Mechanistically, both tumor control and anti-tumoral CD8+ T cell responses depended on enhanced inflammasome activation in Tmem176b−/− mice since they were reversed by IL-1β blockade and Casp1 deletion. Furthermore, the TMEM176B inhibitor BayK8644 controlled tumor growth in a Tmem176b, Casp1/11 and CD8-dependent manner. BayK8644 also improved the anti-tumor efficacy of immune checkpoint blockers in EG7 lymphoma, 5,555 melanoma and MC38 colon cancer. Nevertheless, BayK8644 monotherapy failed to control the growth of established tumors. The dual role played by TMEM176B, promoting antigen cross-presentation while inhibiting inflammasome activation, may help to understand the limited therapeutic efficacy of TMEM176B blockers as well as controversial results recently reported on the role of TMEM176B in regulating anti-tumoral CD8+ T cells. In fact, CD8+ T cells recognize tumoral antigens in the context of MHC I molecules through the cross-presentation pathway (Alloatti et al., 2017) and, as commented above, we have shown that Tmem176b promotes antigen cross-presentation by controlling phagosomal acidification in DCs (Segovia et al., 2014). Louvet and others have failed to observe differences in tumor progression when comparing EG7 growth in WT and Tmem176b−/− mice (Lancien et al., 2021). However, since only 40% of the injected WT mice developed tumors, these results should be taken with caution. In contrast, Jiang et al. reported that in B16 melanoma, tumor growth was accelerated in Tmem176b−/− mice in association with diminished tumor infiltration by CD8+ T cells in comparison to WT animals (Jiang et al., 2022). Although further work is needed to understand this controversy, decreased antigen cross-presentation capacity in Tmem176b−/− mice may explain these observations. Thus, in different models, the impact of inflammasome activation on CD8+ T cell responses may be different (Segovia et al., 2019; Tengesdal et al., 2021). In tumors where CD8+ T cell responses are reinforced by enhanced inflammasome activation, Tmem176b deletion may generate anti-tumoral immune responses despite compromised cross-presentation. In contrast, in tumors where inflammasome activation does not play a relevant role in anti-tumoral immunity, deficient antigen cross-presentation in Tmem176b−/− animals may lead to poor tumor control. However, formulation of TMEM176B blockers may help to uncouple its dual roles in innate and adaptive anti-tumoral immunity. Moreover, the limited therapeutic efficacy of TMEM176B blockers may be linked to its potential capacity to inhibit antigen cross-presentation. We observed that BayK8644 does inhibit the processing of antigens through the cross-presentation pathway in mouse splenic DCs in vitro (Victoria et al., 2022). In cross-presentation, phagosomal antigen processing occurs through a fast kinetic. In fact, a time window of 25 min has been proposed within which antigens must be processed (Howland and Wittrup, 2008). Thus, exogenous antigens that were not processed before that time, would not be cross-presented (Howland and Wittrup, 2008). We therefore speculated that formulating BayK8644 through a strategy that allows a slow release kinetic in endosomes may prevent inhibition of cross-presentation by the compound while potentially maintaining its capacity to induce inflammasome activation. We recently showed that formulating BayK8644 in slow-releasing nanoparticles (NPs) triggered inflammasome activation while preventing the inhibition of antigen cross-presentation and led to improved anti-tumoral efficacy of the compound (Victoria et al., 2022). The therapeutic effect was associated with reinforced tumor infiltration by total and tumor-specific CD8+ T cells.
Furthermore, in coronavirus disease TMEM176B was reported to play a protective role by controlling inflammasome-dependent T cell dysfunction. In COVID-19 patients, TMEM176B expression in peripheral blood and bronchioalveolar lavages was associated with mild disease (Duhalde Vega et al., 2022; Pekayvaz et al., 2022). In mice infected with the β-coronavirus Murine Hepatitis Virus (MHV)-A59, Tmem176b−/− mice showed significantly higher viral loads and diminished survival versus WT animals in an inflammasome-dependent manner. Enhanced inflammasome activation in Tmem176b−/− mice also led to undermined anti-viral CD8+ T cell responses due to exhaustion. Exhausted T cells are a specific lineage of dysfunctional cells characterized by impaired effector mechanisms and transcriptional, epigenetic and metabolic programs as well as the expression of inhibitory receptors such as programmed cell death 1 (PD-1) (Blank et al., 2019; McLane et al., 2019). Therapeutically, T cell exhaustion can be modulated by blocking PD-1 and its cognate ligand PD-L1 (Barber et al., 2006). Accordingly, anti-PD-1 therapy significantly improved viral load and survival in Tmem176b−/− but not in WT animals. In critical COVID-19 patients, low monocytic expression of TMEM176B was associated with increased plasmatic active Caspase-1 which also correlated with CD8+ T cell exhaustion. Moreover, TMEM176B-dependent cross-presentation may also protect the host from critical coronavirus disease. Differential links between inflammasome activation and subsets of exhausted CD8+ T cells in cancer and coronavirus disease may also help to understand the complex relationship between TMEM176B and CD8+ T cell responses.
Concluding remarks
TMEM176B arises as an emergent immunoregulatory player. It is possible that this ion channel inhibits immune effector cells in some circumstances whereas in other settings may promote them, particularly by enhancing antigen presentation to T lymphocytes. We therefore need to answer different questions to increase the odds of targeting this molecule in clinical settings. Particularly, characterization of regulatory partners controlling TMEM176B expression/activity as well as immune regulators controlled by TMEM176B may add critical information. Finally, the role played by TMEM176B in type 2 and type 3 immunity as well as in different inflammatory settings will certainly be unraveled in the next years.
Author contributions
MH, SR, DO, MM, GG, and MS have participated in the design and writing of this minireview.
Conflict of interest
MH is founder and CSO of ARDAN Pharma.
The remaining authors declare that the research was conducted in the absence of any commercial or financial relationships that could be construed as a potential conflict of interest.
Publisher’s note
All claims expressed in this article are solely those of the authors and do not necessarily represent those of their affiliated organizations, or those of the publisher, the editors and the reviewers. Any product that may be evaluated in this article, or claim that may be made by its manufacturer, is not guaranteed or endorsed by the publisher.
References
Alloatti, A., Rookhuizen, D. C., Joannas, L., Carpier, J.-M., Iborra, S., Magalhaes, J. G., et al. (2017). Critical role for Sec22b-dependent antigen cross-presentation in antitumor immunity. J. Exp. Med. 214, 2231–2241. doi:10.1084/jem.20170229
Anandasabapathy, N., Feder, R., Mollah, S., Tse, S.-W., Longhi, M. P., Mehandru, S., et al. (2014). Classical Flt3L-dependent dendritic cells control immunity to protein vaccine. J. Exp. Med. 211, 1875–1891. doi:10.1084/jem.20131397
Barber, D. L., Wherry, E. J., Masopust, D., Zhu, B., Allison, J. P., Sharpe, A. H., et al. (2006). Restoring function in exhausted CD8 T cells during chronic viral infection. Nature 439, 682–687. doi:10.1038/nature04444
Binnewies, M., Mujal, A. M., Pollack, J. L., Combes, A. J., Hardison, E. A., Barry, K. C., et al. (2019). Unleashing type-2 dendritic cells to drive protective antitumor CD4+ T cell immunity. Cell 177, 556–571. e16. doi:10.1016/j.cell.2019.02.005
Blank, C. U., Haining, W. N., Held, W., Hogan, P. G., Kallies, A., Lugli, E., et al. (2019). Defining ‘T cell exhaustion. Nat. Rev. Immunol. 19, 665–674. doi:10.1038/s41577-019-0221-9
Bourdely, P., Anselmi, G., Vaivode, K., Ramos, R. N., Missolo-Koussou, Y., Hidalgo, S., et al. (2020). Transcriptional and functional analysis of CD1c+ human dendritic cells identifies a CD163+ subset priming CD8+CD103+ T cells. Immunity 53, 335–352. e8. doi:10.1016/j.immuni.2020.06.002
Brown, C. C., Gudjonson, H., Pritykin, Y., Deep, D., Lavallée, V.-P., Mendoza, A., et al. (2019). Transcriptional basis of mouse and human dendritic cell heterogeneity. Cell 179, 846–863. e24. doi:10.1016/j.cell.2019.09.035
Cambui, R. A. G., Fernandes, F. P., Leal, V. N. C., Reis, E. C., de Lima, D. S., do Espírito Santo, G. F., et al. (2022). The Ala134Thr variant in TMEM176B exerts a beneficial role in colorectal cancer prognosis by increasing NLRP3 inflammasome activation. J. Cancer Res. Clin. Oncol. doi:10.1007/s00432-022-04284-8
Ciofani, M., Madar, A., Galan, C., Sellars, M., Mace, K., Pauli, F., et al. (2012). A validated regulatory network for Th17 cell specification. Cell 151, 289–303. doi:10.1016/j.cell.2012.09.016
Condamine, T., Le Texier, L., Howie, D., Lavault, A., Hill, M., Halary, F., et al. (2010). Tmem176B and Tmem176A are associated with the immature state of dendritic cells. J. Leukoc. Biol. 88, 507–515. doi:10.1189/jlb.1109738
Crozat, K., Tamoutounour, S., Vu Manh, T.-P., Fossum, E., Luche, H., Ardouin, L., et al. (2011). Cutting edge: Expression of XCR1 defines mouse lymphoid-tissue resident and migratory dendritic cells of the CD8α + type. J. Immunol. 187, 4411–4415. doi:10.4049/jimmunol.1101717
Cuajungco, M. P., Basilio, L. C., Silva, J., Hart, T., Tringali, J., Chen, C.-C., et al. (2014). Cellular zinc levels are modulated by TRPML1-tmem163 interaction. Traffic 15, 1247–1265. doi:10.1111/tra.12205
Cuajungco, M. P., Podevin, W., Valluri, V. K., Bui, Q., Nguyen, V. H., and Taylor, K. (2012). Abnormal accumulation of human transmembrane (TMEM)-176A and 176B proteins is associated with cancer pathology. Acta Histochem. 114, 705–712. doi:10.1016/j.acthis.2011.12.006
Drujont, L., Lemoine, A., Moreau, A., Bienvenu, G., Lancien, M., Cens, T., et al. (2016). RORγt+ cells selectively express redundant cation channels linked to the Golgi apparatus. Sci. Rep. 6, 23682. doi:10.1038/srep23682
Dudziak, D., Kamphorst, A. O., Heidkamp, G. F., Buchholz, V. R., Trumpfheller, C., Yamazaki, S., et al. (2007). Differential antigen processing by dendritic cell subsets in vivo. Science 315, 107–111. doi:10.1126/science.1136080
Duhalde Vega, M., Olivera, D., Gastão Davanzo, G., Bertullo, M., Noya, V., Fabiano de Souza, G., et al. (2022). PD-1/PD-L1 blockade abrogates a dysfunctional innate-adaptive immune axis in critical β-coronavirus disease. Sci. Adv. 8, eabn6545. doi:10.1126/sciadv.abn6545
Dutertre, C.-A., Becht, E., Irac, S. E., Khalilnezhad, A., Narang, V., Khalilnezhad, S., et al. (2019). Single-cell analysis of human mononuclear phagocytes reveals subset-defining markers and identifies circulating inflammatory dendritic cells. Immunity 51, 573–589. e8. doi:10.1016/j.immuni.2019.08.008
Eon Kuek, L., Leffler, M., Mackay, G. A., and Hulett, M. D. (2016). The MS4A family: Counting past 1, 2 and 3. Immunol. Cell Biol. 94, 11–23. doi:10.1038/icb.2015.48
Eugenia Schroeder, M., Russo, S., Costa, C., Hori, J., Tiscornia, I., Bollati-Fogolín, M., et al. (2017). Pro-inflammatory Ca++-activated K+ channels are inhibited by hydroxychloroquine. Sci. Rep. 7, 1892. doi:10.1038/s41598-017-01836-8
Gao, Y., Nish, S. A., Jiang, R., Hou, L., Licona-Limón, P., Weinstein, J. S., et al. (2013). Control of T Helper 2 responses by transcription factor IRF4-dependent dendritic cells. Immunity 39, 722–732. doi:10.1016/j.immuni.2013.08.028
Gong, T., Yang, Y., Jin, T., Jiang, W., and Zhou, R. (2018). Orchestration of NLRP3 inflammasome activation by ion fluxes. Trends Immunol. 39, 393–406. doi:10.1016/j.it.2018.01.009
Hill, M., Segovia, M., Russo, S., Girotti, M. R., and Rabinovich, G. A. (2020). The paradoxical roles of inflammation during PD-1 blockade in cancer. Trends Immunol. 41, 982–993. doi:10.1016/j.it.2020.09.003
Howland, S. W., and Wittrup, K. D. (2008). Antigen release kinetics in the phagosome are critical to cross-presentation efficiency. J. Immunol. 180, 1576–1583. doi:10.4049/jimmunol.180.3.1576
Izumi, G., Nakano, H., Nakano, K., Whitehead, G. S., Grimm, S. A., Fessler, M. B., et al. (2021). CD11b+ lung dendritic cells at different stages of maturation induce Th17 or Th2 differentiation. Nat. Commun. 12, 5029. doi:10.1038/s41467-021-25307-x
Jiang, L., Yang, Y., Liu, F., Ma, M., Gao, J., Sun, L., et al. (2022). A potential diagnostic and prognostic biomarker TMEM176B and its relationship with immune infiltration in skin cutaneous melanoma. Front. Cell Dev. Biol. 10, 859958. doi:10.3389/fcell.2022.859958
Lancien, M., Bienvenu, G., Salle, S., Gueno, L., Feyeux, M., Merieau, E., et al. (2021). Dendritic cells require TMEM176A/B ion channels for optimal MHC class II antigen presentation to naive CD4 + T cells. J. Immunol. 207, 421–435. doi:10.4049/jimmunol.2000498
Lee, A. J., and Ashkar, A. A. (2018). The dual nature of type I and type II interferons. Front. Immunol. 9, 2061. doi:10.3389/fimmu.2018.02061
Louvet, C., Chiffoleau, E., Heslan, M., Tesson, L., Heslan, J.-M., Brion, R., et al. (2005). Identification of a new member of the CD20/FcepsilonRIbeta family overexpressed in tolerated allografts. Am. J. Transpl. 5, 2143–2153. doi:10.1111/j.1600-6143.2005.01007.x
Maeda, Y., Fujimura, L., O-Wang, J., Hatano, M., Sakamoto, A., Arima, M., et al. (2006). Role of Clast1 in development of cerebellar granule cells. Brain Res. 1104, 18–26. doi:10.1016/j.brainres.2006.05.068
Martin, B. N., Wang, C., Zhang, C., Kang, Z., Gulen, M. F., Zepp, J. A., et al. (2016). T cell–intrinsic ASC critically promotes TH17-mediated experimental autoimmune encephalomyelitis. Nat. Immunol. 17, 583–592. doi:10.1038/ni.3389
Mattiola, I., Mantovani, A., and Locati, M. (2021). The tetraspan MS4A family in homeostasis, immunity, and disease. Trends Immunol. 42, 764–781. doi:10.1016/j.it.2021.07.002
McLane, L. M., Abdel-Hakeem, M. S., and Wherry, E. J. (2019). CD8 T cell exhaustion during chronic viral infection and cancer. Annu. Rev. Immunol. 37, 457–495. doi:10.1146/annurev-immunol-041015-055318
Mellor, A. L., and Munn, D. H. (2008). Creating immune privilege: Active local suppression that benefits friends, but protects foes. Nat. Rev. Immunol. 8, 74–80. doi:10.1038/nri2233
Nickles, D., Chen, H. P., Li, M. M., Khankhanian, P., Madireddy, L., Caillier, S. J., et al. (2013). Blood RNA profiling in a large cohort of multiple sclerosis patients and healthy controls. Hum. Mol. Genet. 22, 4194–4205. doi:10.1093/hmg/ddt267
Nishimura, H., Nose, M., Hiai, H., Minato, N., and Honjo, T. (1999). Development of lupus-like autoimmune diseases by disruption of the PD-1 gene encoding an ITIM motif-carrying immunoreceptor. Immunity 11, 141–151. doi:10.1016/S1074-7613(00)80089-8
Nishimura, H., Okazaki, T., Tanaka, Y., Nakatani, K., Hara, M., Matsumori, A., et al. (2001). Autoimmune dilated cardiomyopathy in PD-1 receptor-deficient mice. Science 291, 319–322. doi:10.1126/science.291.5502.319
Okazaki, T., Tanaka, Y., Nishio, R., Mitsuiye, T., Mizoguchi, A., Wang, J., et al. (2003). Autoantibodies against cardiac troponin I are responsible for dilated cardiomyopathy in PD-1-deficient mice. Nat. Med. 9, 1477–1483. doi:10.1038/nm955
Pekayvaz, K., Leunig, A., Kaiser, R., Joppich, M., Brambs, S., Janjic, A., et al. (2022). Protective immune trajectories in early viral containment of non-pneumonic SARS-CoV-2 infection. Nat. Commun. 13, 1018. doi:10.1038/s41467-022-28508-0
Pol, J. G., Caudana, P., Paillet, J., Piaggio, E., and Kroemer, G. (2020). Effects of interleukin-2 in immunostimulation and immunosuppression. J. Exp. Med. 217, e20191247. doi:10.1084/jem.20191247
Rathinam, V. A. K., and Fitzgerald, K. A. (2016). Inflammasome complexes: Emerging mechanisms and effector functions. Cell 165, 792–800. doi:10.1016/j.cell.2016.03.046
Ribas, A., and Wolchok, J. D. (2018). Cancer immunotherapy using checkpoint blockade. Science 359, 1350–1355. doi:10.1126/science.aar4060
Savina, A., Jancic, C., Hugues, S., Guermonprez, P., Vargas, P., Moura, I. C., et al. (2006). NOX2 controls phagosomal pH to regulate antigen processing during crosspresentation by dendritic cells. Cell 126, 205–218. doi:10.1016/j.cell.2006.05.035
Segovia, M., Louvet, C., Charnet, P., Savina, A., Tilly, G., Gautreau, L., et al. (2014). Autologous dendritic cells prolong allograft survival through Tmem176b -dependent antigen cross-presentation: Immunoregulatory mechanisms of autologous DCs. Am. J. Transpl. 14, 1021–1031. doi:10.1111/ajt.12708
Segovia, M., Russo, S., Girotti, M. R., Rabinovich, G. A., and Hill, M. (2020). Role of inflammasome activation in tumor immunity triggered by immune checkpoint blockers. Clin. Exp. Immunol. 200, 155–162. doi:10.1111/cei.13433
Segovia, M., Russo, S., Jeldres, M., Mahmoud, Y. D., Perez, V., Duhalde, M., et al. (2019). Targeting TMEM176B enhances antitumor immunity and augments the efficacy of immune checkpoint blockers by unleashing inflammasome activation. Cancer Cell 35, 767–781. e6. doi:10.1016/j.ccell.2019.04.003
Tengesdal, I. W., Menon, D. R., Osborne, D. G., Neff, C. P., Powers, N. E., Gamboni, F., et al. (2021). Targeting tumor-derived NLRP3 reduces melanoma progression by limiting MDSCs expansion. Proc. Natl. Acad. Sci. U. S. A. 118, e2000915118. doi:10.1073/pnas.2000915118
Tivol, E. A., Borriello, F., Schweitzer, A. N., Lynch, W. P., Bluestone, J. A., and Sharpe, A. H. (1995). Loss of CTLA-4 leads to massive lymphoproliferation and fatal multiorgan tissue destruction, revealing a critical negative regulatory role of CTLA-4. Immunity 3, 541–547. doi:10.1016/1074-7613(95)90125-6
Victoria, S., Castro, A., Pittini, A., Olivera, D., Russo, S., Cebrian, I., et al. (2022). Formulating a TMEM176B blocker in nanoparticles uncouples its paradoxical roles in innate and adaptive antitumoral immunity. Biorxive. doi:10.1101/2022.09.02.506404
Villani, A.-C., Satija, R., Reynolds, G., Sarkizova, S., Shekhar, K., Fletcher, J., et al. (2017). Single-cell RNA-seq reveals new types of human blood dendritic cells, monocytes, and progenitors. Science 356, eaah4573. doi:10.1126/science.aah4573
Waterhouse, P., Penninger, J. M., Timms, E., Wakeham, A., Shahinian, A., Lee, K. P., et al. (1995). Lymphoproliferative disorders with early lethality in mice deficient in ctla-4. Science 270, 985–988. doi:10.1126/science.270.5238.985
Wysocki, T., and Paradowska-Gorycka, A. (2022). Pharmacogenomics of anti-TNF treatment response marks a new era of tailored rheumatoid arthritis therapy. Int. J. Mol. Sci. 23, 2366. doi:10.3390/ijms23042366
Keywords: immunoregulation, antigen presentation, inflammasomes, Tmem176b, ion channels
Citation: Hill M, Russo S, Olivera D, Malcuori M, Galliussi G and Segovia M (2022) The intracellular cation channel TMEM176B as a dual immunoregulator. Front. Cell Dev. Biol. 10:1038429. doi: 10.3389/fcell.2022.1038429
Received: 07 September 2022; Accepted: 27 September 2022;
Published: 20 October 2022.
Edited by:
Luis Mayorga, CONICET Mendoza, ArgentinaReviewed by:
Gaofeng Fan, ShanghaiTech University, ChinaCopyright © 2022 Hill, Russo, Olivera, Malcuori, Galliussi and Segovia. This is an open-access article distributed under the terms of the Creative Commons Attribution License (CC BY). The use, distribution or reproduction in other forums is permitted, provided the original author(s) and the copyright owner(s) are credited and that the original publication in this journal is cited, in accordance with accepted academic practice. No use, distribution or reproduction is permitted which does not comply with these terms.
*Correspondence: Marcelo Hill, bWhpbGxAcGFzdGV1ci5lZHUudXk=; Mercedes Segovia, bXNlZ292aWFAcGFzdGV1ci5lZHUudXk=