- 1Mechanobiology Institute, National University of Singapore, Singapore, Singapore
- 2Department of Biological Sciences, National University of Singapore, Singapore, Singapore
The formation of functional eggs (oocyte) in ovarian follicles is arguably one of the most important events in early mammalian development since the oocytes provide the bulk genetic and cytoplasmic materials for successful reproduction. While past studies have identified many genes that are critical to normal ovarian development and function, recent studies have highlighted the role of mechanical force in shaping folliculogenesis. In this review, we discuss the underlying mechanobiological principles and the force-generating cellular structures and extracellular matrix that control the various stages of follicle development. We also highlight emerging techniques that allow for the quantification of mechanical interactions and follicular dynamics during development, and propose new directions for future studies in the field. We hope this review will provide a timely and useful framework for future understanding of mechano-signalling pathways in reproductive biology and diseases.
1 Introduction
In mammals, the ovarian follicles housing the oocytes are the functional units for female reproduction. The maturation of follicles, or folliculogenesis, is not only essential for supporting oogenesis, but also triggers the production of hormones for female sexual characteristics and early pregnancy. The developmental stages of follicles, loosely defined by the follicle size, the number of cells at the follicular envelope and the morphology, can be classified into the primordial, primary, secondary and antral follicle stage (Hertig and Adams, 1967) (Figure 1A). Folliculogenesis begins with the primordial follicle stage, which consists of an oocyte surrounded by a single layer of somatic granulosa cells (GCs). Upon activation, the primordial follicles develop into the primary follicles, which are characterised by the formation of cuboidal-shaped GCs and the zona pellucida (ZP) that encapsulates the oocyte while maintaining transzonal projections between the oocyte and the GCs. These primary follicles then grow into secondary follicles characterised by multiple layers of GCs and an outer layer of theca cells (TCs). Multiple pockets of fluid-filled lumens form within the GCs as the secondary follicles increase in size and acquire stratified layers of GCs and TCs. These lumen eventually resolve into a single antrum that grows in size. Under the action of luteinizing hormone, the theca wall undergoes extensive extracellular matrix (ECM) remodelling, which, together with antrum expansion, lead to follicle rupture and the release of oocytes out of the ovary, a process known as ovulation.
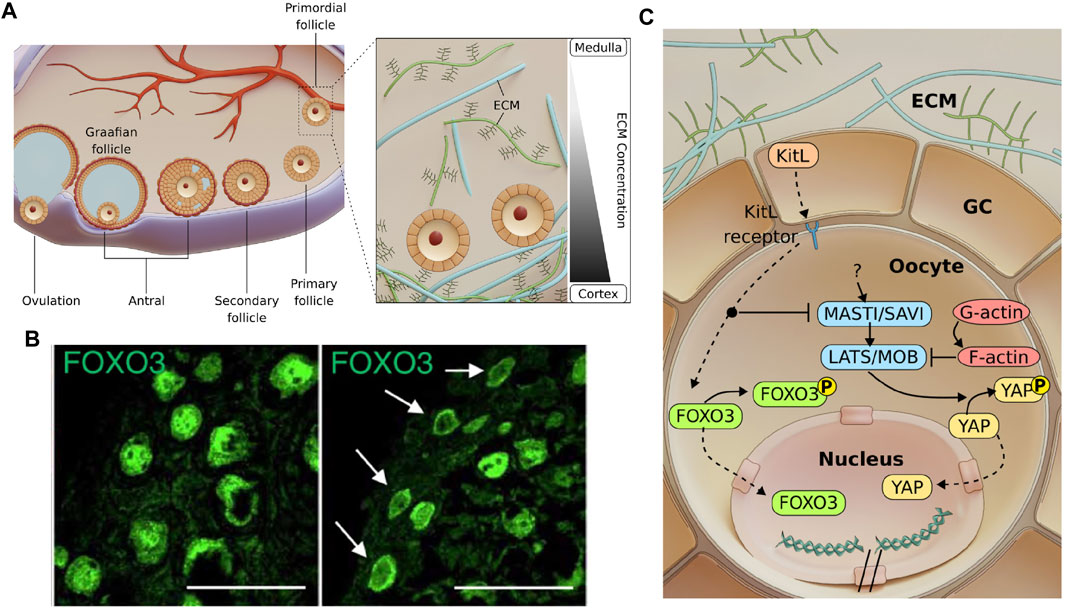
FIGURE 1. Follicle development and possible mechano-signalling pathways involved in primordial follicle activation. (A). Mammalian folliculogenesis is classified into the primordial, primary, secondary and antral follicle stage. Antral follicles undergo ovulation to release the cumulus-oocyte complex before forming a corpus luteum. Inset: A gradient of ECM stiffness was proposed to extend from the soft ovarian medulla to the stiffer cortex where the dormant primordial follicles predominantly reside. (B). Images showing that primordial follicles can be activated via ECM degradation (right), as indicated by FOXO3 cytoplasmic localisation compared to the nuclear localisation of FOXO3 in dormant primordial follicles (left). Scale bar: 50 μm. Images from (Nagamatsu et al., 2019). (C). Mechanosensitive Akt and Hippo pathways govern primordial follicle activation. GCs express Kit ligands (KitL), which are bound to the Kit receptors on the oocyte. Activating the Akt pathway leads to phosphorylation of FOXO3 in the cytoplasm. The Akt pathway also disrupts the Hippo pathway at MST1 to allow for YAP nuclear localisation and downstream activation and growth of follicle. Mechanical stress exerted by the GCs may independently activate mechanosensitive proteins and contribute to dormancy.
Early work on ovarian folliculogenesis was largely descriptive, relying on histology, histochemistry and electron microscopy (Hertig and Adams, 1967). There were extensive functional studies using molecular genetics and transgenics approaches, which revealed key genes and proteins that are required for primary follicle activation and growth (Choi and Rajkovic, 2006; França and Mendonca, 2022). The advancement of immunofluorescence imaging further enables the visualisation of follicular cells and the cytoskeletal proteins during development (Da Silva-Buttkus et al., 2008; Mora et al., 2012), paving the way for a more quantitative approach to understand follicular morphogenesis. Along with these studies, novel approaches were developed to culture follicles ex vivo, such as the seminal work by Eppig et al. on 2D systems and organ culture (Eppig, 1977; Eppig and Schroeder, 1989; Eppig and O'Brien, 1996). In the 2000s, a series of works on culturing follicles in 3D using novel biomaterials were introduced (Pangas et al., 2003; Xu et al., 2006a; West et al., 2007; Hornick et al., 2012), which provide a more native follicle environment with tunable stiffness for optimal follicle growth and survival.
While these studies revealed key paracrine and autocrine signalling patterns in the mammalian ovary, the biomechanics and the roles of mechanical signalling during folliculogenesis have not been investigated as much. It is known that mechanical forces play an integral role in controlling cellular dynamics and functions in development, tissue homeostasis and cancer growth (Heisenberg and Bellaïche, 2013; Hannezo and Heisenberg, 2019). Effective coordination of long-range force transmission and mechanosensing of cells do not only lead to robust tissue morphogenesis, they also impact cell fate specification and tissue patterning via mechanotransduction (Chan et al., 2017). In recent years, new evidence has emerged showing that mechanical cues play a critical role in regulating ovarian folliculogenesis. Ex vivo culture of follicles revealed that the follicle growth is sensitive to the surrounding matrix stiffness, and compressive stress exerted by the matrix can act against follicle expansion and development. Fragmentation of the ovary, which releases tissue mechanical tension and disrupts F-actin, can promote follicle activation and growth via Hippo signalling pathway, a well known mechanotransduction pathway (Kawamura et al., 2013). It has also been hypothesised that the regional difference in ECM stiffness at the outer cortex and the inner medulla may contribute to differential follicle growth or migration (Woodruff and Shea, 2011), which was supported by recent studies on primate (rhesus monkey) and bovine ovaries (Hornick et al., 2012; Henning and Laronda, 2021). These studies highlight the importance of applying tissue mechanics to understand how the follicle size, number and positioning are controlled robustly during ovarian development.
Follicle development is often associated with extensive tissue remodelling (Smith et al., 1999), and changes in tissue mechanical properties have been implicated to influence oocyte quality and fertility. Indeed, ovarian ageing is highly correlated with increased fibrosis and tissue stiffness (Amargant et al., 2020) due to increased expression or altered composition of ECM in the stroma (Briley et al., 2016). Interestingly, ovarian diseases such as polycystic ovarian syndrome (PCOS) (Wood et al., 2015) have densely collagenized and thickened cortex, and are characterised by anovulation, probably due to the lack of ECM degradation or abnormal ECM architecture. Similarly, endometriosis was shown to be associated with abnormal mechanical rigidity in the ECM (Huang et al., 2022). These studies suggest that proper follicle growth requires a biomechanically permissive environment, and calls for a need to better understand the mechanobiological principles governing folliculogenesis.
In this review, we first introduce the various stages of ovarian development and the classical molecular signalling pathways associated with each stage. We then discuss how mechanical stress may be generated by cells within the follicle, and the ECM and stromal cells at the extra-follicular level to impact follicle activation, growth and tissue patterning during ovarian development. We will also introduce the relevant biophysical techniques and reconstitutive biomimetic approaches that allow us to better investigate the underlying mechano-signalling pathways regulating folliculogenesis. Finally, we provide some future perspectives and highlight open questions for the field of ovarian mechanobiology.
2 Primordial follicle dormancy and activation
Prior to the primordial follicle formation, the female primordial germ cells first form cell cysts during embryonic development. In mouse, following birth, cyst breakdown occurs due to the invading GCs, which eventually surround the germ cells to form dormant primordial follicles (Lei and Spradling, 2013; Lei and Spradling, 2016). Bidirectional communication between the oocyte and the GCs has been shown to be essential at this stage, as the absence of either the oocyte or the GCs lead to follicle death (Eppig, 1979). The oocyte controls glycolysis within the GCs via the expression levels of glycolytic genes (Sugiura et al., 2005) and influences the level of luteinizing hormone receptor (LHR) in the GCs (Eppig et al., 1997). The GCs, on the other hand, play an active role in modulating the transcriptional activity and chromatin remodelling of the oocyte (De La Fuente and Eppig, 2001), and express Kit ligand (KitL) which binds to the c-Kit receptors on the oocyte to initiate AKT-mediated follicle activation (Jones and Pepling, 2013). The activation of dormant primordial follicles may also involve protein interactions from within the cell or across cell-cell junctions. In this section, we focus on the PI3K/Akt and Hippo pathways, two signalling pathways that have been identified as major regulators of primordial follicle activation. These pathways may be triggered by extrinsic mechanical stress imposed by the surrounding GCs and the ECM (Figure 1A, inset), which helps to maintain primordial follicle dormancy and ensure sufficient ovarian follicle reserve throughout the fertility period.
The Akt pathway is a well-established signalling pathway that responds to extracellular signals and promotes growth and survival (Hemmings and Restuccia, 2012). In response to a KitL, KIT proto-oncogene receptor tyrosine kinases (c-Kit) induce a kinase cascade, which in turn phosphorylates and activates AKT. Phosphorylated AKT then phosphorylates the transcription factor FOXO3 (Forkhead Box O3), leading to cytoplasmic localisation of FOXO3. It was demonstrated that FOXO3 nuclear export coincides with primordial follicle activation (John et al., 2008) and FOXO3 overexpression leads to increased reproductive capability in mice (Pelosi et al., 2013). Constitutively active FOXO3 was found to cause infertility (Liu et al., 2007), suggesting that nuclear FOXO3 is essential for dormancy regulation but only at a sufficient level. In contrast, the lack of FOXO3 resulted in uncontrolled follicle activation and early infertility (Castrillon et al., 2003), but did not affect follicular growth after activation (John et al., 2007). This suggests that FOXO3 specifically regulates the primordial follicle dormancy, and can be used as a reliable marker for primordial follicle activation.
Primordial follicles are mainly found at the cortex of the ovary, surrounded by abundant ECM. Recent work by Nagamatsu et. al. demonstrated that the cortical ECM of mouse ovaries provides mechanical stress to maintain the primordial follicles in their dormant state (Nagamatsu et al., 2019) (Figure 1B). In this study, they showed that the disruption of collagen leads to FOXO3 translocation to the cytoplasm in primordial follicles, while application of external pressure restores nuclear FOXO3 and dormancy. Notably, primordial follicles that are under mechanical stress exhibit nuclear rotation. However, the mechanosensing mechanism for FOXO3 dynamics and how forces are related to nuclear rotation remains unknown. Of note, the oocytes expanded in size upon ECM abolishment, suggesting that they are physically compressed in their dormant state. The transient change in oocyte volume also implies fluid exchange between the oocyte and the surrounding GCs and possible volume regulation by hydraulic stress.
Apart from the Akt pathway, the Hippo pathway is another well known mechano-signalling pathway. The Hippo pathway can respond to various signals, such as ECM stiffness (Dupont et al., 2011), substrate stiffness (Thomasy et al., 2013), stretching (Aragona et al., 2013), cell geometry (Dupont et al., 2011), cell density (Zhao et al., 2007), cellular tension (Perez Gonzalez et al., 2018) and shear stress (Lee et al., 2017). These factors were integrated by the Hippo pathway to regulate various cellular processes such as growth, development and homeostasis (Wu and Guan, 2021). When the Hippo pathway is activated, the Yes-associated protein (YAP) becomes phosphorylated and localises to the cytoplasm, where it remains non-functional or gets degraded. However, when the Hippo pathway is disrupted, non-phosphorylated YAP translocates to the nucleus to form a transcription activation complex with TEA domain family members (TEAD 1-4), leading to expression of downstream genes. The Hippo pathway was found to play a role in follicle growth. Disruption of the Hippo pathway caused a primary ovarian insufficiency (POI)-like phenotype (St John et al., 1999), indicating that YAP might be necessary for follicle activation. This is further corroborated by a study of Kawamura et al., who demonstrated that ovarian fragmentation can lead to F-actin polymerisation, increased nuclear YAP localisation and primordial follicle activation (Kawamura et al., 2013). Moreover, F-actin polymerisation itself leads to increased nuclear YAP localisation and follicle growth (Cheng et al., 2015), highlighting YAP’s mechanosensing capability and its role in follicle activation.
Given that the dormant oocytes with nuclear FOXO3 are mechanically compressed (Nagamatsu et al., 2019), and that compressive stress is implicated in YAP signalling, this suggests a potential crosstalk between Akt pathway and Hippo pathway. There is evidence that the Hippo pathway functions downstream of the Akt pathway. In Drosophila, Borreguero-Muñoz et al. showed that AKT can directly disrupt the Hippo pathway (Figure 1C) (Borreguero-Muñoz et al., 2019). In mouse ovaries, the use of MK2206 (AKT inhibitor) not only suppressed follicle activation, but also led to an increase in pYAP-to-YAP ratio (Hu et al., 2019). This implies that AKT can disrupt Hippo signalling and trigger YAP nuclear localisation and follicle activation. This evidence points to the existence of a crosstalk between the Akt and Hippo pathways and should be carefully examined together in the context of primordial follicle activation.
The GCs surrounding the oocyte may also directly regulate oocyte functions through mechanotransduction (Figure 1C). Notably, the squamous GCs exhibit contractile stress fibres at the primordial follicle stage (Nagamatsu et al., 2019), and have flat morphology compared to the cuboidal GCs at the primary follicle stage. Is this change in morphology during activation a result of reduced compressive stress from ECM remodelling, or due to intrinsic change in GC contractility? Furthermore, since flat cells are often associated with high surface tension (Lecuit and Lenne, 2007), does it imply that the squamous GCs exert significant forces on the oocyte during dormancy, and the release of mechanical tension leads to follicle activation? Another open question is whether the GC mechanics can directly trigger the expression of KitL and downstream AKT-mediated activation in the oocyte. These questions will likely be addressed in the near future with the application of novel biophysical tools and ex vivo reconstitution approaches (see Section 6).
3 Mechano-signalling in pre-antral follicle development
Secondary follicle development is characterised by the development of the spindle shaped TCs overlying the basement membrane (BM) around the GCs (Figure 2A). It takes more than 30 days for primordial follicles to become secondary follicles in rat ovaries, and 120 days in the case of human ovaries (McGee and Hsueh, 2000). Sizes of secondary follicles range from 59 to 303 µm in diameter as measured across multiple species (Griffin et al., 2006). Secondary folliculogenesis is also marked by a rapid increase in oocyte volume, although the oocyte is observed to remain in meiotic arrest (DiLuigi et al., 2008). The ZP surrounding the oocyte becomes thicker and more prominent at this stage for most organisms (Albertini et al., 2001). Here we focus our discussion on three key components in secondary follicles that may potentially regulate their development via mechanical signalling.
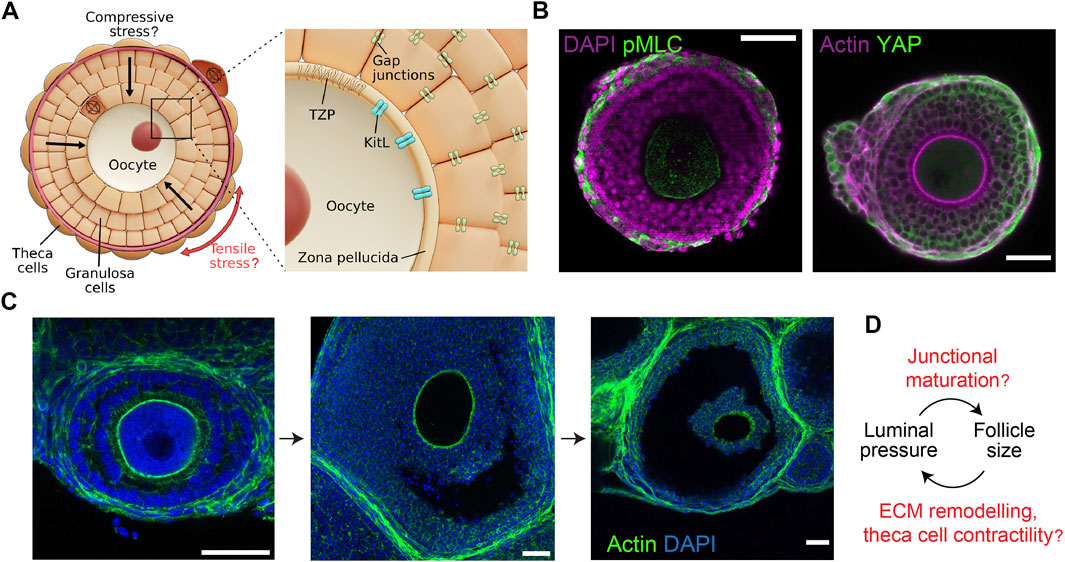
FIGURE 2. Mechano-hydraulic control of secondary and antral follicle development. (A). During secondary follicle development, spindle-shaped TCs form around the basement membrane (dark red) and the GCs, potentially generating compressive stress within the follicle. Anisotropic stress pattern may guide cell division orientation in the TCs (circumferential) and the GCs (radial). Inset: Compressive stress may affect oocyte growth by modifying the oocyte-granulosa interactions mediated by the TZP and Kit ligands. Mechanical stress may also influence the GC proliferation via gap junction activities. (B). Left: Isolated follicle immuno-stained for nuclei (DAPI) and phosphorylated-myosin light chain (pMLC), showing contractile TCs. Right: Follicle immuno-stained for actin and YAP, showing YAP nuclear localisation in the TCs only. Scale bar: 50 µm. (C). Antral follicles immuno-stained for nuclei (DAPI) and actin. Luminal fluid accumulates at the intercellular space, eventually merging into a singly-resolved antrum that continues to expand till ovulation. Scale bar: 40 µm. (D). Potential feedback loop between luminal pressure and follicle size: increased luminal pressure may trigger junctional maturation and follicle expansion through mechanosensing, while TC stiffening may control the final size of the follicles.
3.1 Basement membrane
One of the most crucial internal components of the follicle is the BM. The basal lamina separating the TCs from the GCs is typically composed of isoforms of collagen IV and laminin (O’Shea et al., 1978), but the relative compositions change during folliculogenesis (Rodgers et al., 2003; Berkholtz et al., 2006). Fibronectin is not prominent in the BM of primordial and primary follicles, but its expression becomes stronger at the secondary follicle stage (Akkoyunlu et al., 2003; Heeren et al., 2015). BM is nano-porous and can allow size-dependent passage of molecules across it. Molecules with molecular weights lower than 100 kDa can move freely through the BM, while those larger than 500 kDa cannot (Perloff et al., 1954; Shalgi et al., 1973). There exist reports on the pore size and thickness of BM in non-ovarian models (Abrams et al., 2000; Nicholas and Jacques, 2005; Halfter et al., 2013; Gaiko-Shcherbak et al., 2015), but these parameters have not been characterised in ovarian follicles. It is suggested that the composition, geometry, and crosslinking of the different proteins can dictate the BM mechanical properties (Miller, 2017; Ramos-Lewis and Page-McCaw, 2019), but the physical properties and mechanical functions of BM in folliculogenesis remain poorly characterised.
BM is common in most tissues in the body and is involved in a variety of diseases (Christensen et al., 2015). The thickness of the reticular BM (Saglani et al., 2006) and capillary BM (Ganda et al., 1983) has been implicated in asthma and diabetes respectively. It has been shown in epithelial cancers that cells can generate physical forces to invade the BM, leading to metastasis (Chang and Chaudhuri, 2019). Cancer-associated fibroblasts, myoepithelial cells and immune cells have also been implicated to regulate cell invasion. It is possible that similar conditions may apply to ovarian cancers. Hence, a deeper understanding of BM mechanics may inspire new approaches to modify the BM and help to prevent cancer progression.
The stiffness of the BM provides another key mechanical cue that may impact follicle development. The values of BM stiffness vary, ranging from ∼55 kPa in mouse mesentery to 400-3,000 kPa in mouse renal tubules (Bhave et al., 2017; Glentis et al., 2017). In Drosophila, BM stiffness has been shown to determine the shape of developing egg chambers (Crest et al., 2017; Chen et al., 2019) and in altering cell migration in vivo in the central nervous system (Kim et al., 2014; Sánchez-Sánchez et al., 2017). Softer BM in the Drosophila wing disc allowed the wing disc to expand and flatten (Ramos-Lewis and Page-McCaw, 2019). Increased niche-derived mechanical stress due to increased BM stiffness in aged murine hair follicle stem cells has been observed to repress transcription, silence bivalent promoters and compromise stem cell potential (Koester et al., 2021). Recently it has also been shown that the intricate interplay between cell-cell and cell-BM interactions can contribute to stratified epithelial budding and branching morphogenesis of murine embryonic salivary glands (Wang et al., 2021).
In ovarian development, the BM has been suggested to protect ovarian follicles from physical damage (Figueiredo et al., 1995). It was proposed that the stiff basal lamina acts as a mechanical barrier for the proliferation and inward division of GCs (Da Silva-Buttkus et al., 2008): the GCs become more densely packed when they divide in a radial fashion within the follicles, thereby leading to the emergence of multi-layered GCs at this stage (Da Silva-Buttkus et al., 2008). Here we speculate that as the follicles grow in size, the BM may deform or undergo active remodelling to accommodate for the growing number of GCs contained within. How this changes the BM mechanical properties and regulates TC and GC functions will constitute an exciting topic for future research.
3.2 Granulosa cells
The internal geometry of a follicle loosely resembles that of a multicellular spheroid and it is plausible that mechanical cues affecting spheroid growth could also play a role in folliculogenesis. Increased mechanical stress inside tumour spheroids have been shown to lead to reduced cellular proliferation (Delarue et al., 2014; Dolega et al., 2017). As the spheroids grow, compressive stress can build up and promote cancer cell migration (Tse et al., 2012). It has been shown that the tumour spheroid growth responds to the stiffer mechano-environment through cytoskeletal remodelling and ROCK signalling pathway (Taubenberger et al., 2019). In the developing wing imaginal disc of Drosophila, the varied stress patterns between cells at the periphery (tension) and the cells in the centre (compression) lead to differential cell shape and division orientation (LeGoff et al., 2013). In secondary follicles, a similar mechanism may operate where follicle growth leads to the emergence of anisotropic stress pattern, characterised by radial compressive stress in the GC layer and tangential tensile stress at the theca layer. This may in turn direct cell division pattern and follicle morphogenesis (Figure 2A).
GCs secrete mucopolysaccharides which thicken and rigidify the ZP during follicle growth, thereby changing the mechanical environment around the oocytes (Ouni et al., 2020). Transzonal projections (TZPs) are hair-like projections from the GCs which invade the ZP and maintain direct contact with the oocyte (El-Hayek et al., 2018). The communication between the oocyte and its surrounding GCs is also mediated by connexin 37, a gap junction protein that is present at the tip of the TZPs (Simon et al., 1997; Ackert et al., 2001). Among the GCs, it is known that another type of gap junction proteins, connexin 43, connect the GCs and help with the exchange of nutrients like amino acids, glucose, ions, and cGMP in order to maintain cellular osmolarity and metabolism (Eppig, 1991; Winterhager and Kidder, 2015). Gene knockout studies have shown that connexin 37 are not only essential for oocyte maturation, they are also required for the luteinisation of GCs prior to ovulation (Simon et al., 1997). Loss of connexin 43 in mutant ovaries showed impaired GC proliferation, absence of antrum and developmentally incompetent oocytes (Ackert et al., 2001). Connexins in endothelial cells are known to be affected by shear stress and connexin 43 hemichannels in osteocytes are known to be opened by mechanical loading which helps in bone formation (Islam and Steward, 2019; Riquelme et al., 2020; Zhao et al., 2020). Whether connexins respond to mechanical stimuli and regulate follicle growth requires further investigation.
As the GCs become multi-layered, several signalling molecules–namely, BMP-15 and GDF-9—secreted by the oocyte start to impact this process (Dong et al., 1996; Moore et al., 2003). As described in the previous section, both these factors along with the Kit ligands and their receptors are believed to take part in a feedback loop between the oocyte and the GCs during this developmental stage (Eppig, 2001; Otsuka and Shimasaki, 2002). A similar bidirectional communication also exists between the GCs and TCs. While the Kit ligands and other growth factors from the GCs can prompt TC proliferation (Huang et al., 2001; Matsuura et al., 2002; Field et al., 2014; Shiomi-Sugaya et al., 2015), several BMPs secreted by the TCs are shown to impact GC proliferation (Lee et al., 2001; Glister et al., 2005) through the Wnt (Wang et al., 2010), Notch (Trombly et al., 2009), and Hedgehog (Wijgerde et al., 2005) signalling pathways. Though some of the signalling pathways have been implicated in tissue mechanics in non-ovarian developmental systems (Bonewald and Johnson, 2008; Brunt et al., 2017; Stassen et al., 2020; Yang et al., 2021), definitive evidence on potential mechano-regulation of these signalling pathways in the ovary remains to be established.
3.3 Theca cells
TCs are observed to appear at the periphery of secondary follicles that have at least two layers of GCs (Figure 2A) (Magoffin and Weitsman, 1994), and the process is gonadotropin independent. However, the origin of these cells remains debatable. While some believe that they originate from putative stem cells in ovaries (Honda et al., 2007), others suggest that they are derived from progenitor cells at the embryonic stage (Liu et al., 2015). Though not characterised, it is hypothesised that the cells are recruited from the ovarian stroma mediated by oocyte or GC secreted factors (Orisaka et al., 2009). TCs provide structural integrity to the follicles and produce important endocrine regulatory factors like androgens and other growth regulatory factors (Erickson et al., 1985; Young and McNeilly, 2010). Malfunction of TCs such as overproduction of TC-secreted androgens leads to PCOS (Azziz et al., 2009; Huang et al., 2010), which is a leading cause of female infertility that affects 4-20% of reproductively aged women worldwide (Deswal et al., 2020).
The theca layer is not homogeneous. The layer consists of the inner cells bordering the BM, also known as the theca interna, which secrete steroids that serve as substrates for estrogen production from the GCs (Magoffin et al., 1995). The outermost layers of TCs, the theca externa, are fibroblast-like in nature. They emerge at the later stages of follicle development, and are believed to play a role during ovulation. During follicle expansion in this growth phase, the theca layers are known to provide nutrients by developing vasculature through secreting vascular endothelial growth factor (VEGF) (Jones and Shikanov, 2019), thereby leading to increased oxygenation in the follicle (Shin et al., 2005).
While the role of TCs in steroid production and hormonal regulation is well documented, the structural and mechanical functions of these cells are less well understood. Recently, cancer-associated fibroblasts have been shown to self-organise to form a capsule around tumour cells and initiate mechanotransduction through active compression (Barbazan et al., 2021). The contractile TCs may wrap around the follicles in a similar fashion and provide compressive stress to coordinate signalling and growth within the follicle (Figure 2B). A recent study revealed the presence of mechanical heterogeneity within the follicle, where the TCs were shown to have a higher loss tangent (>20%) than the GCs, particularly during the antral follicle stage (Chan et al., 2021). The loss tangent here can be understood as the ratio between the cell’s effective micro-viscosity and stiffness. A higher loss tangent of TCs therefore indicates greater energy dissipation and a more viscous response when subjected to mechanical stress. This may be associated with the increased hyaluronan production by the TCs (Figure 3B) (Amargant et al., 2020).
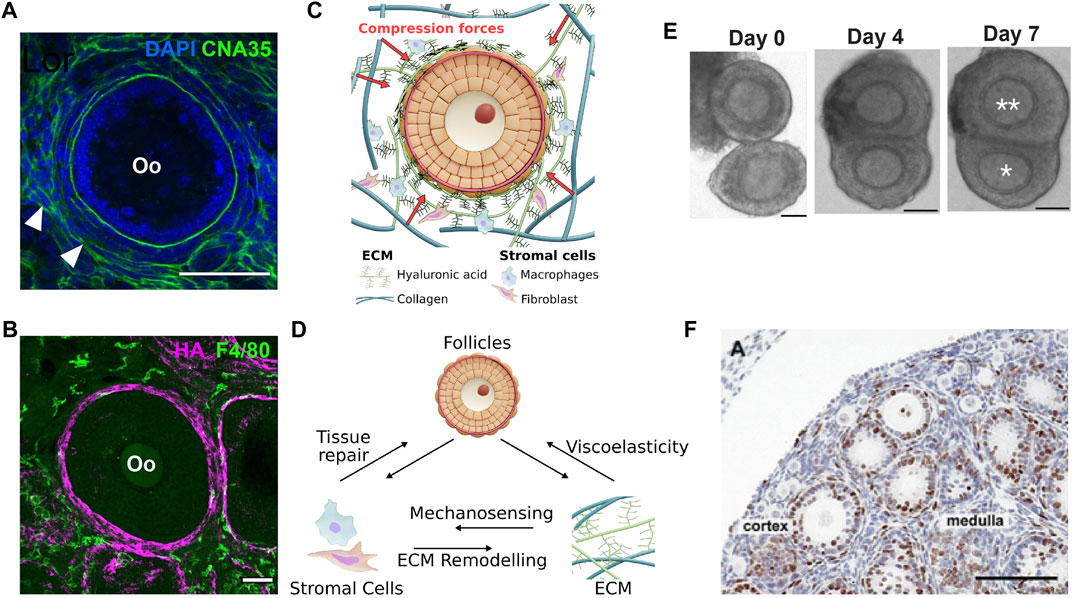
FIGURE 3. Stromal mechanics and inter-follicle dynamics in ovary development. (A). Labelling of CNA35, a collagen-binding protein, shows the presence of basement membrane within the follicle and at the interstitial tissue (white arrowhead). Scale bar: 50 μm. (B). Macrophages labelled with F4/80 were observed at the interstitial matrix, while the hyaluronic acid (HA) was highly expressed at the TC layer. Scale bar: 50 μm. (C). Growing follicles may be mechanically confined by the surrounding ECM that comprises mainly of collagen and HA. (D). Potential feedback interactions between stromal cells, ECM and follicle growth during ovarian development and ageing. Changes in ECM composition and mechanical properties may activate immune response from macrophages via mechanosensing pathways, or the stromal cells may actively remodel the ECM or participate in tissue repair during follicle death or post-ovulation. Changes in ECM viscoelastic properties may directly influence follicle growth. (E–F). Follicle-follicle interactions may provide additional mechanical signals to influence follicle growth. (E). In vitro culture of follicle doublet shows that the follicles can merge over the course of 7 days to generate a dominant, growing follicle (double asterisks) and a ”subordinate” follicle with limited growth (single asterisk). Scale bar: 50 μm. (F). Evidence of close contact among pre-antral follicles in a P12 mouse ovary. Scale bar: 100 μm. Image from (Da Silva-Buttkus et al., 2008).
Despite growing evidence that mechanical signalling can impact follicle development, questions remain on how these mechanical forces are generated and maintained within the follicles. In particular, it is not known how GC proliferation, which may generate tissue pressure, is coupled to BM remodelling and TC-generated compressive stress during follicle development. Addressing this will require novel methods to quantify cellular dynamics, tissue mechanics and mechanosensing activities in the ovarian follicles (see Section 6).
4 Tissue hydraulics during antral follicle development and ovulation
A key morphological event is the emergence of a fluid-filled cavity (lumen) in the antral follicles (Figure 2C). Fluid cavity can generate hydrostatic pressure that can modulate cell-cell junctional remodelling or ion pump activities that in turn leads to organ or embryo size control and tissue patterning (Chan et al., 2019; Mosaliganti et al., 2019). A similar feedback mechanism may operate during antral follicle morphogenesis (Figure 2D). In addition, the lumen can act as a signalling hub to drive cell differentiation and cell shape changes around the lumen (Durdu et al., 2014; Ryan et al., 2019). In mammalian species, the antrum size varies from species to species: larger species such as human and bovine have larger follicles with the fluid comprising a substantial volume fraction (>95%) of the follicles at ovulation (Rodgers et al., 2001), while follicles of smaller species such as rats and mice contain less follicular fluid (Rodgers and Irving-Rodgers, 2010). Despite previous reports showing a correlation between antrum formation and oocyte maturation (Dumesic et al., 2015), the potential mechano-chemical functions of antrum in folliculogenesis remain enigmatic. Similarly, there remains limited studies on how the micro-lumen first emerge and coalesce into a single antrum, which is essential for late ovulation. One likely mechanism is osmotic regulation, where the antrum fluid could be sourced externally from the blood vessels formed around the TCs that encapsulate the antral follicles. Since the GCs lack functional tight junctions (Mora et al., 2012), it would not be possible to establish an osmotic gradient across the membrane granulosa with small ions like sodium. Instead, larger molecules such as hyaluronan and proteoglycans appeared to be trapped in the antrum which may generate a significant osmotic gradient to draw in fluid from the theca capillary (Clarke et al., 2006; Rodgers and Irving-Rodgers, 2010). However it remains unclear how the GCs actively secrete osmotically active molecules into the antrum, and if this is triggered by some signalling molecules released by the oocyte. One possible hypothesis is that some GCs within the follicle undergo apoptosis and are eliminated, thereby creating a hollow space that is filled with fluid. This process is also known as ‘cavitation’ (Sigurbjörnsdóttir et al., 2014). Cell death releases DNA fragments which, by virtue of their high molecular weights, may generate strong osmotic force to drive lumen expansion and coalescence (Clarke et al., 2006).
As mentioned, the GCs lack functional tight junctions but are connected by the gap junctions (Mora et al., 2012). This suggests that antrum development is not likely mediated by directed fluid transport through apico-basal polarity establishment, a mechanism widely reported in epithelial tissues and in vitro (Roignot et al., 2013). It is however worth noting that fluid accumulation can occur at the basolateral compartment through exocytosis or programmed cell shape changes (Schliffka and Maître, 2019). For example, during mouse blastocyst development, cytoplasmic vesicles are secreted and transported into intercellular space, leading to fluid accumulation at the onset of blastocoel formation (Ryan et al., 2019). During zebrafish gastrulation, mitotic rounding during cell divisions drive cell-cell contact disassembly in the deep cells, leading to increased interstitial fluid that translates to global tissue fluidization at the animal pole (Petridou et al., 2019). Whether these mechanisms operate during antral folliculogenesis constitute exciting topics for future research.
As the follicle matures into the Graafian follicle, it undergoes ovulation, which is characterised by a series of biochemical and morphological events that lead to its ultimate rupture and discharge of the mature oocyte out of the ovary. While it is known that elevated levels of luteinizing and follicle-stimulating hormones are required for ovulation, the precise mechanisms underlying this process remain unknown. Early studies pointed to both mechanical and enzymatic activities in follicle rupture during ovulation. Biophysical studies of follicle wall tension showed a large increase in wall extensibility prior to follicle rupture (Espey and Lipner, 1963), which is associated with the thinning of the theca wall due to ECM degradation by multiple proteolytic enzymes such as matrix metalloproteinases and plasmin (Curry and Smith, 2006). Interestingly, endothelin was proposed as a mechanotransduction gene that may facilitate follicular rupture and ovulation by binding to the smooth muscle at the theca externa, thereby leading to the contractile activity necessary for follicular rupture (Ko et al., 2006). Early studies on antrum fluid mechanics revealed no significant change in the hydrostatic pressure during antrum development (Espey and Lipner, 1963; Rondell, 1964; Bronson et al., 1979), potentially due to species difference and technical limitations. However, recent studies using servo-null micropressure system detects more than 40% increase in luminal pressure from preovulatory to late ovulatory phase in rat ovaries after human chorionic gonadotropin (hCG) stimulation (Matousek et al., 2001). Future studies leveraging on ex vivo culture and live imaging will provide better spatiotemporal dynamics of ovulation and will help to address how coordinated changes in lumen pressure, tissue mechanics and ECM remodelling lead to robust biophysical control of ovulation.
5 Mechanical signalling from extra-follicular environment
In addition to intra-follicular mechanical interactions, extra-follicular mechanical signals from the ovarian stroma also play a significant role in regulating follicle growth. The stroma is highly complex, consisting of nerves, vasculature, ECM, immune and fibroblast-like cells (Kinnear et al., 2020). In this section, we discuss how the ECM, stromal cells and follicle-follicle interactions may dynamically modulate the follicle’s mechanical environment to orchestrate its development.
5.1 Interstitial extracellular matrix
It is well established that the ECM can initiate both mechanical and biochemical signalling cues. Historically, the role of ovarian fibrillar ECM such as collagen, laminin and fibronectin, and their changes during follicular progression were the focus of in-depth characterisation (Berkholtz et al., 2006). Across species, collagen IV is consistently expressed in the basal lamina throughout follicle development (Irving-Rodgers and Rodgers, 2005; Ouni et al., 2020). They were also detected in the BM of atretic follicles (Nakano et al., 2007) and the theca shell (Figure 3A). However, type IV alpha chains 3-6 in the BM decreases after the primary follicle stage (Irving-Rodgers and Rodgers, 2005). Collagen I and VI are expressed throughout the ovary (Woodruff and Shea, 2007; Ouni et al., 2019), with type I mainly located at the ovarian surface epithelium (Woodruff and Shea, 2007). Spatially, decellularized human and bovine ovarian tissues show that the collagen fibres are radially aligned at the cortex and anisotropic at the medulla (Laronda et al., 2015), suggesting a difference in ECM mechanics between the cortex and the medulla, possibly associated with the cortex having a higher stiffness. Recent work on human ovaries also revealed a significant thickening of ECM fibre bundles and increased ECM pore size from prepuberty to reproductive ageing, which is also associated with increased fibre orientation and straightness (Ouni et al., 2021). Similar to collagen, fibronectin was reported to increase in the stroma and theca layer during follicle growth (Woodruff and Shea, 2007). Laminin was also found to increase in the BM along with follicle development (Irving-Rodgers and Rodgers, 2005), with some isoforms present in the ovarian surface epithelium and TC layer (Rodgers et al., 2003).
Apart from fibrillar proteins, a key component of the ECM is hyaluronan or hyaluronic acid (HA), which is an anionic glycosaminoglycan (GAG) matrix with high molecular weight (typically 1,000-8,000 kDa) (Cowman et al., 2015; Monslow et al., 2015). Their ability to trap fluid and swell by osmotic pressure generates mechanical stress to influence the form and function of the tissue microenvironment (Voutouri and Stylianopoulos, 2018). In zebrafish, HA provides anisotropic extracellular stress to guide otic bud morphogenesis (Munjal et al., 2021) or cardiac valve development (Vignes et al., 2022). In murine adenocarcinoma models, administration of hyaluronidase resulted in tumour relaxation, indicating that HA provides tissue compressive resistance (Stylianopoulos et al., 2012; Voutouri and Stylianopoulos, 2018). In bovine and porcine ovaries, HA was localised at the theca layer, stroma, and vasculature (Parkes et al., 2021), similar to our observation in mice (Figure 3B). HA concentrations are known to surge and accumulate in the cumulus cell-oocyte complexes (COC) (Salustri et al., 1999), potentially related to their soft nature (Chen et al., 2016). In contrast to collagen, the roles of HA mechanics in regulating folliculogenesis and ovarian functions are relatively understudied.
There has been recent progress in understanding the spatio-temporal dynamics of interstitial ECM in the context of ovarian ageing, fibrosis, and inflammation. During ovarian ageing, the overall collagen deposition increases while the total HA content decreases, with no significant change in the molecular weights (Amargant et al., 2020). This fibrotic trend was again observed in human samples, indicating that the phenomenon may be conserved across species during ageing (Manuel et al., 2019). A recent study has also shown that this ageing-associated reduction in female reproductive lifespan can be rescued by reversing collagen fibrosis in mouse ovaries (Umehara et al., 2022). It is known that fibrosis mechanically alters the organ by significantly increasing tissue stiffness (Wells, 2013). However, it remains unclear as to how fibrotic ECM remodelling of the ovarian stroma mechanistically modulates follicle development in disease and ageing (Figure 3C).
5.3 Stromal cells
Apart from the ECM, the ovarian stroma is also populated with diverse cell types with myriad functions. Amongst the stromal cells, fibroblasts (Sahai et al., 2020) and macrophages (Kim and Nair, 2019) are known to have the capability to remodel ECM. Indeed, there have been extensive reports of ECM remodelling via matrix metalloproteinases, plasminogens, and associated enzymes in ovaries (Smith et al., 1999; Curry and Smith, 2006). However, which stromal cells are specifically involved during ECM deposition and degradation is not fully understood.
Macrophages make up the largest sub-population of immune cells in the ovaries (Kinnear et al., 2020), and are known to possess ECM remodelling capabilities which can enhance ovarian cancer progression (Busuttil et al., 2014; Cheng et al., 2019). In aged ovaries, it was reported that there was a shift in macrophage polarisation towards pro-tissue regenerative type (M2 polarisation) (Zhang et al., 2020) which is coherent with the observed inflammatory fibrotic ECM structure in aged ovaries (Briley et al., 2016; Rowley et al., 2020; Lliberos et al., 2021), although the underlying mechanotransduction pathways remain unknown. Fibroblasts, in their highly contractile state, are generally associated with ECM deposition and remodelling (Sahai et al., 2020). While there have been several reports linking cancer-associated fibroblasts with ovarian cancer progression and tumour-ECM remodelling (Kenny et al., 2007; Thuwajit et al., 2018), little is known about how the ovarian fibroblast-like cells modulate the ovarian matrix during folliculogenesis. The study of ovarian fibroblasts is challenging due to a lack of suitable experimental platforms (Quiros et al., 2008). This is further compounded by the fact that fibroblasts lack a clear biomarker, and have been shown to be highly heterogeneous between and within organs (Chang et al., 2002).
5.4 Stromal cell-ECM-follicle feedback
While the stromal cells can actively remodel the ECM, ECM biomechanics can also reciprocally impact the stromal cell functions (Figure 3D). It has been shown that macrophage function, polarisation, and migration are influenced by ECM biomechanical and physical properties (McWhorter et al., 2015; Sridharan et al., 2019). For example, macrophages seeded onto stiff polyacrylamide gels were primed towards pro-inflammatory polarisation and exhibit podosome-dependent migration whereas on soft gels, macrophages were anti-inflammatory and showed fast ameboid-like migration (Petersen et al., 2012). Likewise, the ECM remodelling capability of fibroblasts is also dependent on physical cues from the environment–it was observed that high stiffness scaffolds increased fibroblast secretion of matrix metalloproteinase-1 (Petersen et al., 2012). Interestingly, we identified F4/80+ macrophages to be spatially associated with the HA ‘shell’ at the theca layer (Figure 3B), suggesting possible crosstalk between macrophage and HA. Active remodelling of ECM by the stromal cells may lead to changes in the ECM biomechanical properties, which will ultimately impact follicle growth and oocyte quality (Figure 3D). The stromal cells, particularly the macrophages, are known to be associated with atretic follicles across different species (Kasuya, 1995; Best et al., 1996; Petrovská et al., 1996; Gaytán et al., 1998) and in the ovulatory process (Brännström et al., 1993; Takaya et al., 1997), which suggests that the macrophages may play an active role in tissue clearing and wound healing processes during ovarian development and homeostasis. Ultimately, a system-level understanding of the feedback interactions between the stromal cells, ECM, and the follicles will help illuminate the underlying mechanobiological principles regulating ovarian folliculogenesis (Figure 3D).
5.5 Follicle-follicle interactions
Another source of mechanical signalling in vivo could be the direct contact between the follicles. In a seminal work by Spears et al. (Spears et al., 1996), they demonstrated that co-culture of two mouse follicles in contact can lead to follicle dominance, where one follicle invariably grows and becomes dominant while the other one shows arrested growth, as shown in Figure 3E. Notably, the suppressed follicles are not dying, as manual removal of the dominant follicle can induce subsequent growth of the suppressed follicle. In a follow-up paper, the authors further demonstrated that the dominant follicles also render the subordinate follicles more susceptible to lowered FSH concentrations, thereby inducing atresia in these follicles (Baker et al., 2001). These findings on how follicle-follicle contact determines follicle selection and fate may answer the question why only a certain number of follicles undergo size amplification and eventually ovulate during each reproductive cycle (6-7 ovulatory follicles in the case of mouse and only one in human). These findings are physiologically relevant as histological studies revealed that preantral follicles are often found in close contact with each other (Da Silva-Buttkus et al., 2008) (Figure 3F), and preovulatory follicles are found alongside less-developed antral follicles (Baker and SpearsN., 1999). These studies also prompt speculation if differential follicle growth can lead to distinct follicle positioning within the ovary during development. For example, in very young ovaries, the small primordial follicles are often found at the ovarian cortex while the larger growing follicles are found in the inner medulla. Later, as the mice grow past puberty, the ovary is characterised by numerous antral follicles that are found to be close to the ovarian surface, ready for ovulation. Whether such tissue patterning is mediated by follicle-follicle interactions and if these interactions play a similar role in human ovaries are exciting questions for the future.
In the above studies, the authors proposed the existence of a contact-mediated mechanism for follicle dominance and suppression, although the molecular mechanism was not addressed. Others have proposed that the growing follicles may secrete inhibitory signals such as anti-Müllerian hormone (AMH) and activin to maintain primordial or pre-antral follicle dormancy (Durlinger et al., 1999; Mizunuma et al., 1999). Here we propose that in addition to paracrine signalling, mechanical signals, such as the compressive stress exerted by one follicle on another, may provide further inhibitory signals to break the initial size symmetry and trigger differential growth. This corroborates with a recent atomic force microscopy (AFM) study which revealed that the large follicles are the mechanically dominant structures in the ovary that may transmit mechanical stress to the surrounding follicles (Hopkins et al., 2021).
6 Approaches to study ovarian mechanobiology
6.1 Biomechanical characterisation of ovarian mechanics
The concept of mechanobiology in ovarian biology is relatively new, and biophysical studies to quantify tissue mechanical properties in ovaries remain scarce. To date, AFM remains the primary tool to measure the ovarian biomechanical properties, such as the follicle and stromal stiffness during reproductive ageing and menopause (Amargant et al., 2020; Hopkins et al., 2021; Ouni et al., 2021). Here, the sample is indented with a cantilever attached to an AFM tip of known geometry and the applied force is measured from the bending angle of the cantilever. The surface stiffness is calculated by fitting the force indentation curves to known mechanical models. Of note, while these studies were conducted on different layers of tissues, tissue sectioning itself may potentially release tissue stress and perturb the mechanical state of the ovaries (Stylianopoulos et al., 2012). Micropipette aspiration is another technique that can be used to probe the surface tension of a cell or tissue, based on the Laplace Law (Maître et al., 2016; Chan et al., 2019). Furthermore, by tracking the dynamics of the aspirated ‘tongue’ of the tissue, the tissue viscosity can be extracted (Guevorkian et al., 2010). Another well established technique to infer cellular or tissue tension is laser ablation. Here, UV or femtosecond-pulsed near-infrared lasers are used to cut biological tissues locally. Upon disruption, the speed and direction of recoil of the cell-cell junctions or tissue segment can be used to infer the magnitude and orientation of local cell or tissue tension (Sugimura et al., 2016).
The above-mentioned techniques are mostly confined to measuring the surface mechanics of tissues. To quantify mechanical properties within the tissues, other tools such as magnetic tweezer have been developed. Here, magnetic beads or ferrofluid oil droplets are introduced into tissues, which can be manipulated in the presence of an externally applied magnetic field. If the magnetic properties of the beads or droplets is known, the force-displacement profiles can be used to calculate the local stiffness and viscosity of the tissue (Mongera et al., 2018; Zhu et al., 2020; D’Angelo and Solon, 2020). In recent years, label-free, non-invasive approaches have also been developed to directly ‘image’ the mechanical landscape of tissue interior. For example, Brillouin microscopy has been developed to measure micro-viscoelasticity of cells and tissues with high spatial resolution in 3D (Prevedel et al., 2019). It relies on the interaction and inelastic scattering of monochromatic laser light from thermally driven acoustic phonons at high frequencies. The scattered light spectrum is indicative of the material’s local mechanical properties, as shown by recent work on mouse ovaries and living embryos (Chan et al., 2021; Bevilacqua et al., 2022). Another imaging technique is second harmonic generation microscopy, which probes the tissue composition and the molecular structure of collagen with high sensitivity and specificity. It has been used extensively to study alterations in fibrillar collagens in scar tissues of skin, lung, heart and eyes (Mostaço-Guidolin et al., 2017), as well as in mouse ovaries (Watson et al., 2012; Bochner et al., 2015).
Besides measuring tissue material properties, another important parameter that remains poorly characterised is the mechanical stress within ovaries. To evaluate mechanical stress in tissue interior, several techniques have been developed in recent years. For example, hydrogel-based deformable beads have been developed, which allows ones to quantify the local stress fields in living tissues, as demonstrated in zebrafish and in vitro cell aggregates (Dolega et al., 2017; Mohagheghian et al., 2018; Träber et al., 2019; Souchaud et al., 2022). Larger beads have been used to infer the contractile stress exerted by cells. For example, a recent study shows that the compressive stress exerted by contractile cardiomyocytes can be quantified by measuring the change in volume of large gelatin beads of known compressibility, before and after cardiomyocyte enwrapment (He et al., 2021). To quantify tissue pressure in 3D tissues, laser ablation, combined with the tracking of tissue outflow from the abscission site, allows one to compare the mechanical state of compressed tumour aggregates (Barbazan et al., 2021). While many of these techniques have yet to be applied to mammalian ovaries, we foresee that such quantitative studies will yield important insights to advance the field of ovarian biology.
6.2 Quantitative imaging of ovarian dynamics
Recent advancement in deep tissue imaging has allowed us to gain insights into follicle dynamics within the ovary. One such tool is light-sheet microscopy. This technique has been increasingly used in the last few years for rapid visualisation of live specimens, particularly for deep tissues where a single sample plane is excited optically by a light sheet and fluorescent images are captured by a camera placed perpendicular to the excitation (Power and Huisken, 2017). This technique allows an enhanced image quality and does not rely on physical sectioning of the tissues. Combined with tissue clearing, light-sheet microscopy has been used to quantify follicle morphometrics in pig ovaries (Lin et al., 2017) and human ovaries that revealed marked architectural remodelling in certain ECM biomarkers at prepubertal, reproductive-age and menopausal stages (Ouni et al., 2022). Another powerful tool for studying follicle development in vivo is optical coherence tomography, which allows imaging of ovaries in situ and in real time with micron-scale imaging resolution (Wang et al., 2015; Watanabe et al., 2015). Widely used in ophthalmology and endoscopic studies of luminal organ systems (Swanson et al., 1993; Fujimoto et al., 2000), there has been a growing number of work utilising this approach to study reproductive events when combined with intra-vital imaging, such as in mouse ovary and oviduct (Burton et al., 2015), co-operative sperm flow (Wang and Larina, 2018), and oocyte and embryo transport during pre-implantation development (Wang and Larina, 2021).
6.3 Ex vivo reconstitution
Owing to the multifactorial complexity in the tissue microenvironment, it is challenging to dissect the individual contribution of mechanical signals on follicle growth. Here, ex vivo reconstitution of follicles provides a bottom-up approach to understand how the follicle’s mechanical environment impacts its maturation. For example, when cultured in 3D alginate hydrogel (Figure 4B,B′), secondary follicles in the softer matrix showed increased follicle growth, theca development, antrum formation and higher oocyte quality, suggesting that mechanical confinement can regulate follicle development (Xu et al., 2006b; West et al., 2007; West-Farrell et al., 2009). To allow follicle expansion at later stages of development, degradable hydrogel was developed, such as the fibrin-alginate interpenetrating network (Shikanov et al., 2011). Recently, poly(ethylene glycol) (PEG) hydrogels have also been developed to allow better control of matrix stiffness and pore size that promotes optimal follicle growth (Kim et al., 2016). Others have further incorporated mechanical heterogeneities within the gel, where microfluidics was employed to encapsulate follicles within a gel that is made of a soft inner core of collagen and stiff alginate shell (Choi et al., 2014; He, 2017). This was proposed to better mimic the supposedly soft medulla and stiffer cortex of the ovary that are required for follicles to develop to the antrum stage.
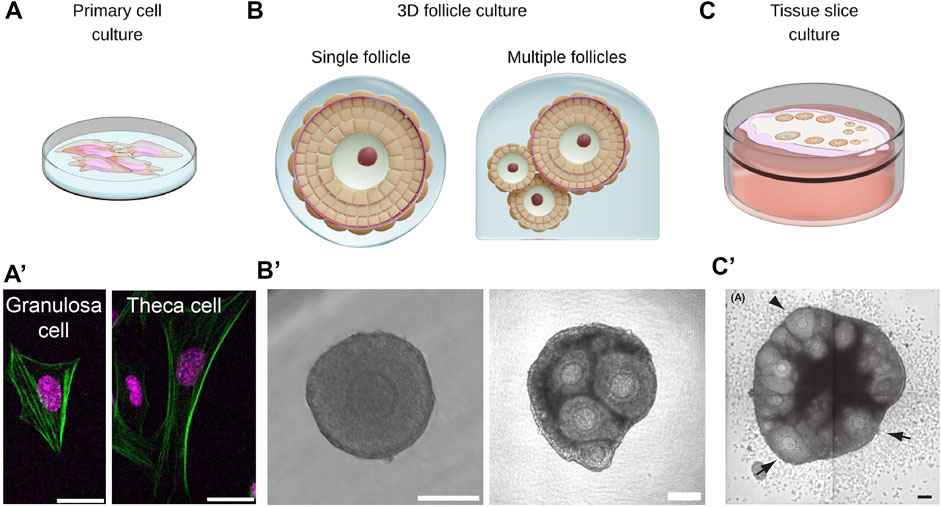
FIGURE 4. Multiscale ex vivo reconstitution approaches are required for integrative understanding of ovarian mechanobiology. (A,A’). Primary cells such as the theca cells and the granulosa cells can be isolated from bulk ovaries for studying single cell mechanics or cell-cell interactions in co-culture systems. Scale bar: 20 μm. (B,B’). Isolated follicles can be cultured in 3D hydrogel for studying morphogenesis and the impact of mechanical confinement on follicle growth. Aggregates of follicles can also be cultured in 3D to study follicle-follicle interactions. Scale bar: 100 μm. (C,C’). Ovarian tissue slices can be cultured ex vivo to study collective dynamics of follicles and ovulation during development. Scale bar: 100 μm. Image from (Komatsu and Masubuchi, 2017).
In addition to stiffness, ovarian tissues may be viscoelastic and exhibit stress relaxation, which measures how fast the material dissipates its internal stress in response to externally applied deformation. Recent studies demonstrated that hydrogels that exhibit slow relaxation provide greater mechanical confinement to hinder spheroid growth and cell proliferation (Nam et al., 2019; Indana et al., 2021). As discussed before (see Section 5), HA is a major component of ovarian ECM that is highly expressed in the ovarian stroma and TCs. Their ability to trap interstitial fluid and resist compression (Irving-Rodgers and Rodgers, 2005) may generate a slow stress relaxation environment to confine follicle growth. In future, it will be instructive to study follicle growth when cultured in gels of different stress relaxation profiles. Also, how HA remodelling impacts follicle growth and oocyte functions during ovarian ageing will be an exciting topic for future research.
Moving beyond single follicle studies, other works have attempted to study follicle dynamics using ovarian tissue slices (Komatsu and Masubuchi, 2017; Komatsu et al., 2018). By growing tissues on culture inserts, the authors were able to image and quantify pre-antral follicle development, ovulation and atresia (Figure 4C,C′). This approach may provide a good platform to study collective dynamics of follicles, such as differential growth and follicle dominance as discussed in previous sections.
To investigate mechanical interactions between the oocyte and somatic cells, it is noteworthy that there exist protocols for isolating and culturing primary GCs and TCs in vitro (Li and Hearn, 2000; Choi et al., 2014; Tian et al., 2015) (Figure 4A,A′). This provides a unique opportunity to probe their biomechanical properties and mechanosensing properties, which is not easily accessible in vivo. By adding complexity to such systems, such as TC-follicle or GC-oocyte co-culture systems, one could also gain new insights into how the follicle components mechanically interact with each other.
7 Conclusion
With the advancements in mechanobiology and experimental tools, we are now at a unique position to address how mechanical signals act in concert with hormonal signalling to control robust mammalian folliculogenesis. While we have discussed new biophysical techniques that allow us to dissect the intricate mechanical interactions at the intra- and extra-follicular level, in vivo perturbations remain challenging given the multiscale complexity of the organ. An attractive approach to address these experimental limitations is theoretical modelling and computational simulations (Johnson et al., 2015; Fischer et al., 2021; Fischer-Holzhausen and Röblitz, 2022), which allow us to tune mechanical parameters and ‘perturb’ the system in silico in order to assess functional sufficiency of these mechanical signals in ovarian growth.
Understanding ovarian mechanobiology has profound implications in understanding ovarian ageing, cancer, and disease, which are often associated with impaired tissue mechanics and misregulated mechano-signalling pathways. From the clinical perspective, a ‘mechanical’ understanding of follicular development has important implications in assisted reproductive technology such as in vitro fertilisation (IVF) and in vitro maturation (IVM). Both approaches generate relatively low success rates, potentially due to the removal of the follicle’s mechanical environment that helps to ensure oocyte health. The design of novel biomaterials to provide appropriate mechanical signals may contribute to future improvement of IVF and IVM and even oocyte rejuvenation. Of note, the ovaries are similar to many organs in terms of tissue architecture and functions, and similar biophysical principles may operate in these diverse systems. Indeed, recent work revealed the role of mechanics in lymphocyte infiltration and stromal remodelling during lymph node expansion (Assen et al., 2022; Horsnell et al., 2022) and mammalian kidney development (Viola et al., 2022).
We end this review by proposing several questions for future research. Does mechanotransduction play a role in regulating oocyte growth? If so, what are the key mechanosensors involved in the process? How are the various mechanical signals integrated across multiple scales to influence follicle maturation? At the tissue level, how are mechanics and hormonal signalling coupled to generate robust follicle patterns in terms of their number, size and positions? Finally, does follicle-follicle interactions play a role in follicle death (atresia) that is known to take place during ovarian development? Recent work revealed that cell-cell fluid exchange can lead to collective cell death and the selection of functional eggs in C. elegans during oogenesis (Chartier et al., 2021), suggesting that germ cell fate can be controlled by environmental factors. Ultimately, it will be exciting to explore if the different spatiotemporal dynamics of follicle development in diverse mammalian species are governed by the same underlying mechanobiological principles.
Author contributions
All authors participated in the writing process. CC conceptualized the review.
Funding
CC. is supported by the Ministry of Education under the Research Centres of Excellence programme through the Mechanobiology Institute and the Department of Biological Sciences at the National University of Singapore (A-0003467-27-00, A-0007085-00-00), and the Bia-Echo Asia Centre for Female Reproductive Longevity & Equality at the National University of Singapore (A-0006323-00-00).
Acknowledgments
We thank members of the Chan laboratory, Tsuyoshi Hirashima and Yuchen Long for helpful comments on the manuscript, and Diego Pitta de Araujo for illustration. We apologise to the authors whose work could not be highlighted due to space limitations.
Conflict of interest
The authors declare that the research was conducted in the absence of any commercial or financial relationships that could be construed as a potential conflict of interest.
Publisher’s note
All claims expressed in this article are solely those of the authors and do not necessarily represent those of their affiliated organizations, or those of the publisher, the editors and the reviewers. Any product that may be evaluated in this article, or claim that may be made by its manufacturer, is not guaranteed or endorsed by the publisher.
References
Abrams, G. A., Goodman, S. L., Nealey, P. F., Franco, M., and Murphy, C. J. (2000). Nanoscale topography of the basement membrane underlying the corneal epithelium of the rhesus macaque. Cell Tissue Res. 299 (1), 39–46. doi:10.1007/s004419900074
Ackert, C. L., Gittens, J. E., O’Brien, M. J., Eppig, J. J., and Kidder, G. M. (2001). Intercellular communication via connexin43 gap junctions is required for ovarian folliculogenesis in the mouse. Dev. Biol. 233 (2), 258–270. doi:10.1006/dbio.2001.0216
Akkoyunlu, G., Demir, R., and Üstünel, İ. (2003). Distribution patterns of TGF-α, laminin and fibronectin and their relationship with folliculogenesis in rat ovary. Acta Histochem. 105 (4), 295–301. doi:10.1078/0065-1281-00717
Albertini, D. F., Combelles, C. M., Benecchi, E., and Carabatsos, M. J. (2001). Cellular basis for paracrine regulation of ovarian follicle development. Reproduction 121 (5), 647–653. doi:10.1530/rep.0.1210647
Amargant, F., Manuel, S. L., Tu, Q., Parkes, W. S., Rivas, F., Zhou, L. T., et al. (2020). Ovarian stiffness increases with age in the mammalian ovary and depends on collagen and hyaluronan matrices. Aging Cell 19 (11), e13259. doi:10.1111/acel.13259
Aragona, M., Panciera, T., Manfrin, A., Giulitti, S., Michielin, F., Elvassore, N., et al. (2013). A mechanical checkpoint controls multicellular growth through YAP/TAZ regulation by actin-processing factors. Cell 154 (5), 1047–1059. doi:10.1016/j.cell.2013.07.042
Assen, F. P., Abe, J., Hons, M., Hauschild, R., Shamipour, S., Kaufmann, W. A., et al. (2022). Multitier mechanics control stromal adaptations in the swelling lymph node. Nat. Immunol. 23 (8), 1246–1255. doi:10.1038/s41590-022-01257-4
Azziz, R., Carmina, E., Dewailly, D., Diamanti-Kandarakis, E., Escobar-Morreale, H. F., Futterweit, W., et al. (2009). The androgen excess and PCOS society criteria for the polycystic ovary syndrome: The complete task force report. Fertil. Steril. 91 (2), 456–488. doi:10.1016/j.fertnstert.2008.06.035
Baker, S. J., Sršeň, V., Lapping, R., and Spears, N. (2001). Combined effect of follicle-follicle interactions and declining follicle-stimulating hormone on murine follicle health in vitro. Biol. Reprod. 65 (4), 1304–1310. doi:10.1095/biolreprod65.4.1304
Baker, S., and SpearsN., (1999). The role of intra-ovarian interactions in the regulation of follicle dominance. Hum. Reprod. Update 5 (2), 153–165. doi:10.1093/humupd/5.2.153
Barbazan, J., Pérez-González, C., Gómez-González, M., Dedenon, M., Richon, S., Latorre, E., et al. (2021). Cancer-associated fibroblasts actively compress cancer cells and modulate mechanotransduction. Cell Biol. doi:10.1101/2021.04.05.438443
Berkholtz, C. B., Shea, L. D., and Woodruff, T. K. (2006). Extracellular matrix functions in follicle maturation. Semin. Reprod. Med. 24 (4), 262–269. doi:10.1055/s-2006-948555
Best, C. L., Pudney, J., Welch, W. R., Burger, N., and Hill, J. A. (1996). Localization and characterization of white blood cell populations within the human ovary throughout the menstrual cycle and menopause. Hum. Reprod. 11 (4), 790–797. doi:10.1093/oxfordjournals.humrep.a019256
Bevilacqua, C., Gomez, J. M., Fiuza, U. M., Chan, C. J., Wang, L., Hambura, S., et al. (2022). High-resolution line-scan Brillouin microscopy for live-imaging of mechanical properties during embryo development. Bioengineering. doi:10.1101/2022.04.25.489364
Bhave, G., Colon, S., and Ferrell, N. (2017). The sulfilimine cross-link of collagen IV contributes to kidney tubular basement membrane stiffness. Am. J. Physiol. Ren. Physiol. 313 (3), F596–F602. doi:10.1152/ajprenal.00096.2017
Bochner, F., Fellus-Alyagor, L., Kalchenko, V., Shinar, S., and Neeman, M. (2015). A novel intravital imaging window for longitudinal microscopy of the mouse ovary. Sci. Rep. 5 (1), 12446. doi:10.1038/srep12446
Bonewald, L. F., and Johnson, M. L. (2008). Osteocytes, mechanosensing and Wnt signaling. Bone 42 (4), 606–615. doi:10.1016/j.bone.2007.12.224
Borreguero-Muñoz, N., Fletcher, G. C., Aguilar-Aragon, M., Elbediwy, A., Vincent-Mistiaen, Z. I., and Thompson, B. J. (2019). The Hippo pathway integrates PI3K-Akt signals with mechanical and polarity cues to control tissue growth. PLoS Biol. 17 (10), e3000509. doi:10.1371/journal.pbio.3000509
Brännström, M., Mayrhofer, G., and Robertson, S. A. (1993). Localization of leukocyte subsets in the rat ovary during the periovulatory period. Biol. Reprod. 48 (2), 277–286. doi:10.1095/biolreprod48.2.277
Briley, S. M., Jasti, S., McCracken, J. M., Hornick, J. E., Fegley, B., Pritchard, M. T., et al. (2016). Reproductive age-associated fibrosis in the stroma of the mammalian ovary. Reproduction 152 (3), 245–260. doi:10.1530/REP-16-0129
Bronson, R. A., Bryant, G., Balk, M. W., and Emanuele, N. (1979). Intrafollicular pressure within preovulatory follicles of the pig. Fertil. Steril. 31 (2), 205–213. doi:10.1016/s0015-0282(16)43824-0
Brunt, L. H., Begg, K., Kague, E., Cross, S., and Hammond, C. L. (2017). Wnt signalling controls the response to mechanical loading during Zebrafish joint development. Development 144, 2798–2809. doi:10.1242/dev.153528
Burton, J. C., Wang, S., Stewart, C. A., Behringer, R. R., and Larina, I. V. (2015). High-resolution three-dimensional in vivo imaging of mouse oviduct using optical coherence tomography. Biomed. Opt. Express 6 (7), 2713–2723. doi:10.1364/BOE.6.002713
Busuttil, R. A., George, J., Tothill, R. W., Ioculano, K., Kowalczyk, A., Mitchell, C., et al. (2014). A signature predicting poor prognosis in gastric and ovarian cancer represents a coordinated macrophage and stromal response. Clin. Cancer Res. 20 (10), 2761–2772. doi:10.1158/1078-0432.CCR-13-3049
Castrillon, D. H., Miao, L., Kollipara, R., Horner, J. W., and DePinho, R. A. (2003). Suppression of ovarian follicle activation in mice by the transcription factor Foxo3a. Science 301 (5630), 215–218. doi:10.1126/science.1086336
Chan, C. J., Bevilacqua, C., and Prevedel, R. (2021). Mechanical mapping of mammalian follicle development using Brillouin microscopy. Commun. Biol. 4 (1), 1133. doi:10.1038/s42003-021-02662-5
Chan, C. J., Costanzo, M., Ruiz-Herrero, T., Mönke, G., Petrie, R. J., Bergert, M., et al. (2019). Hydraulic control of mammalian embryo size and cell fate. Nature 571 (7763), 112–116. doi:10.1038/s41586-019-1309-x
Chan, C. J., Heisenberg, C. P., and Hiiragi, T. (2017). Coordination of morphogenesis and cell-fate specification in development. Curr. Biol. 27 (18), R1024–R1035. doi:10.1016/j.cub.2017.07.010
Chang, H. Y., Chi, J. T., Dudoit, S., Bondre, C., van de Rijn, M., Botstein, D., et al. (2002). Diversity, topographic differentiation, and positional memory in human fibroblasts. Proc. Natl. Acad. Sci. U. S. A. 99 (20), 12877–12882. doi:10.1073/pnas.162488599
Chang, J., and Chaudhuri, O. (2019). Beyond proteases: Basement membrane mechanics and cancer invasion. J. Cell Biol. 218 (8), 2456–2469. doi:10.1083/jcb.201903066
Chartier, N. T., Mukherjee, A., Pfanzelter, J., Fürthauer, S., Larson, B. T., Fritsch, A. W., et al. (2021). A hydraulic instability drives the cell death decision in the nematode germline. Nat. Phys. 17 (8), 920–925. doi:10.1038/s41567-021-01235-x
Chen, D. Y., Crest, J., Streichan, S. J., and Bilder, D. (2019). Extracellular matrix stiffness cues junctional remodeling for 3D tissue elongation. Nat. Commun. 10 (1), 3339. doi:10.1038/s41467-019-10874-x
Chen, X., Bonfiglio, R., Banerji, S., Jackson, D. G., Salustri, A., and Richter, R. P. (2016). Micromechanical analysis of the hyaluronan-rich matrix surrounding the oocyte reveals a uniquely soft and elastic composition. Biophys. J. 110 (12), 2779–2789. doi:10.1016/j.bpj.2016.03.023
Cheng, H., Wang, Z., Fu, L., and Xu, T. (2019). Macrophage polarization in the development and progression of ovarian cancers: An overview. Front. Oncol. 9, 421. doi:10.3389/fonc.2019.00421
Cheng, Y., Feng, Y., Jansson, L., Sato, Y., Deguchi, M., Kawamura, K., et al. (2015). Actin polymerization-enhancing drugs promote ovarian follicle growth mediated by the Hippo signaling effector YAP. FASEB J. 29 (6), 2423–2430. doi:10.1096/fj.14-267856
Choi, J. K., Agarwal, P., Huang, H., Zhao, S., and He, X. (2014). The crucial role of mechanical heterogeneity in regulating follicle development and ovulation with engineered ovarian microtissue. Biomaterials 35 (19), 5122–5128. doi:10.1016/j.biomaterials.2014.03.028
Choi, Y., and Rajkovic, A. (2006). Genetics of early mammalian folliculogenesis. Cell. Mol. Life Sci. 63 (5), 579–590. doi:10.1007/s00018-005-5394-7
Christensen, A. P., Patel, S. H., Grasa, P., Christian, H. C., and Williams, S. A. (2015). Oocyte glycoproteins regulate the form and function of the follicle basal lamina and theca cells. Dev. Biol. 401 (2), 287–298. doi:10.1016/j.ydbio.2014.12.024
Clarke, H. G., Hope, S. A., Byers, S., and Rodgers, R. J. (2006). Formation of ovarian follicular fluid may be due to the osmotic potential of large glycosaminoglycans and proteoglycans. Reproduction 132 (1), 119–131. doi:10.1530/rep.1.00960
Cowman, M. K., Schmidt, T. A., Raghavan, P., and Stecco, A. (2015). Viscoelastic properties of hyaluronan in physiological conditions. F1000Res. 4, 622. doi:10.12688/f1000research.6885.1
Crest, J., Diz-Muñoz, A., Chen, D. Y., Fletcher, D. A., and Bilder, D. (2017). Organ sculpting by patterned extracellular matrix stiffness. eLife 6, e24958. doi:10.7554/eLife.24958
Curry, T., and Smith, M. (2006). Impact of extracellular matrix remodeling on ovulation and the folliculo-luteal transition. Semin. Reprod. Med. 24 (4), 228–241. doi:10.1055/s-2006-948552
Da Silva-Buttkus, P., Jayasooriya, G. S., Mora, J. M., Mobberley, M., Ryder, T. A., Baithun, M., et al. (2008). Effect of cell shape and packing density on granulosa cell proliferation and formation of multiple layers during early follicle development in the ovary. J. Cell Sci. 121 (23), 3890–3900. doi:10.1242/jcs.036400
D’Angelo, A., and Solon, J. (2020). Application of mechanical forces on Drosophila embryos by manipulation of microinjected magnetic particles. Bio. Protoc. 10 (9), e3608. doi:10.21769/BioProtoc.3608
De La Fuente, R., and Eppig, J. J. (2001). Transcriptional activity of the mouse oocyte genome: Companion granulosa cells modulate transcription and chromatin remodeling. Dev. Biol. 229 (1), 224–236. doi:10.1006/dbio.2000.9947
Delarue, M., Montel, F., Vignjevic, D., Prost, J., Joanny, J. F., and Cappello, G. (2014). Compressive stress inhibits proliferation in tumor spheroids through a volume limitation. Biophys. J. 107 (8), 1821–1828. doi:10.1016/j.bpj.2014.08.031
Deswal, R., Narwal, V., Dang, A., and Pundir, C. S. (2020). The prevalence of polycystic ovary syndrome: A brief systematic review. J. Hum. Reprod. Sci. 13 (4), 261–271. doi:10.4103/jhrs.JHRS_95_18
DiLuigi, A., Weitzman, V. N., Pace, M. C., Siano, L. J., Maier, D., and Mehlmann, L. M. (2008). Meiotic arrest in human oocytes is maintained by a Gs signaling pathway. Biol. Reprod. 78 (4), 667–672. doi:10.1095/biolreprod.107.066019
Dolega, M. E., Delarue, M., Ingremeau, F., Prost, J., Delon, A., and Cappello, G. (2017). Cell-like pressure sensors reveal increase of mechanical stress towards the core of multicellular spheroids under compression. Nat. Commun. 8 (1), 14056. doi:10.1038/ncomms14056
Dong, J., Albertini, D. F., Nishimori, K., Kumar, T. R., Lu, N., and Matzuk, M. M. (1996). Growth differentiation factor-9 is required during early ovarian folliculogenesis. Nature 383 (6600), 531–535. doi:10.1038/383531a0
Dumesic, D. A., Meldrum, D. R., Katz-Jaffe, M. G., Krisher, R. L., and Schoolcraft, W. B. (2015). Oocyte environment: Follicular fluid and cumulus cells are critical for oocyte health. Fertil. Steril. 103 (2), 303–316. doi:10.1016/j.fertnstert.2014.11.015
Dupont, S., Morsut, L., Aragona, M., Enzo, E., Giulitti, S., Cordenonsi, M., et al. (2011). Role of YAP/TAZ in mechanotransduction. Nature 474 (7350), 179–183. doi:10.1038/nature10137
Durdu, S., Iskar, M., Revenu, C., Schieber, N., Kunze, A., Bork, P., et al. (2014). Luminal signalling links cell communication to tissue architecture during organogenesis. Nature 515 (7525), 120–124. doi:10.1038/nature13852
Durlinger, A. L. L., Kramer, P., Karels, B., Jong, F. H. D., Uilenbroek, J. T. J., Grootegoed, J. A., et al. (1999). Control of primordial follicle recruitment by anti-Müllerian hormone in the mouse ovary. Endocrinology 140 (12), 5789–5796. doi:10.1210/endo.140.12.7204
El-Hayek, S., Yang, Q., Abbassi, L., FitzHarris, G., and Clarke, H. J. (2018). Mammalian oocytes locally remodel follicular architecture to provide the foundation for germline-soma communication. Curr. Biol. 28 (7), 1124–1131. e3. doi:10.1016/j.cub.2018.02.039
Eppig, J. J. (1979). A comparison between oocyte growth in coculture with granulosa cells and oocytes with granulosa cell-oocyte junctional contact maintained in vitro. J. Exp. Zool. 209 (2), 345–353. doi:10.1002/jez.1402090216
Eppig, J. J. (1991). Intercommunication between mammalian oocytes and companion somatic cells. Bioessays 13 (11), 569–574. doi:10.1002/bies.950131105
Eppig, J. J. (1977). Mouse oocyte development in vitro with various culture systems. Dev. Biol. 60 (2), 371–388. doi:10.1016/0012-1606(77)90135-x
Eppig, J. J., and O'Brien, M. J. (1996). Development in vitro of mouse oocytes from primordial follicles. Biol. Reprod. 54 (1), 197–207. doi:10.1095/biolreprod54.1.197
Eppig, J. J. (2001). Oocyte control of ovarian follicular development and function in mammals. Reproduction 122 (6), 829–838. doi:10.1530/rep.0.1220829
Eppig, J. J., and Schroeder, A. C. (1989). Capacity of mouse oocytes from preantral follicles to undergo embryogenesis and development to live young after growth, maturation, and fertilization in vitro. Biol. Reprod. 41 (2), 268–276. doi:10.1095/biolreprod41.2.268
Eppig, J. J., Wigglesworth, K., Pendola, F., and Hirao, Y. (1997). Murine oocytes suppress expression of luteinizing hormone receptor messenger ribonucleic acid by granulosa cells. Biol. Reprod. 56 (4), 976–984. doi:10.1095/biolreprod56.4.976
Erickson, G. F., Magoffin, D. A., Dyer, C. A., and Hofeditz, C. (1985). The ovarian androgen producing cells: A review of structure/function relationships. Endocr. Rev. 6 (3), 371–399. doi:10.1210/edrv-6-3-371
Espey, L. L., and Lipner, H. (1963). Measurements of intrafollicular pressures in the rabbit ovary. Am. J. Physiol. 205 (6), 1067–1072. doi:10.1152/ajplegacy.1963.205.6.1067
Field, S. L., Dasgupta, T., Cummings, M., and Orsi, N. M. (2014). Cytokines in ovarian folliculogenesis, oocyte maturation and luteinisation: Cytokines in folliculogenesis. Mol. Reprod. Dev. 81 (4), 284–314. doi:10.1002/mrd.22285
Figueiredo, J. R., Hulshof, S. C., Thiry, M., Van den Hurk, R., Bevers, M. M., Nusgens, B., et al. (1995). Extracellular matrix proteins and basement membrane: Their identification in bovine ovaries and significance for the attachment of cultured preantral follicles. Theriogenology 43 (5), 845–858. doi:10.1016/0093-691x(95)00036-8
Fischer, S., Ehrig, R., Schäfer, S., Tronci, E., Mancini, T., Egli, M., et al. (2021). Mathematical modeling and simulation provides evidence for new strategies of ovarian stimulation. Front. Endocrinol. 12, 613048. doi:10.3389/fendo.2021.613048
Fischer-Holzhausen, S., and Röblitz, S. (2022). Hormonal regulation of ovarian follicle growth in humans: Model-based exploration of cycle variability and parameter sensitivities. J. Theor. Biol. 547, 111150. doi:10.1016/j.jtbi.2022.111150
França, M. M., and Mendonca, B. B. (2022). Genetics of ovarian insufficiency and defects of folliculogenesis. Best. Pract. Res. Clin. Endocrinol. Metab. 36 (1), 101594. doi:10.1016/j.beem.2021.101594
Fujimoto, J. G., Pitris, C., Boppart, S. A., and Brezinski, M. E. (2000). Optical coherence tomography: An emerging technology for biomedical imaging and optical biopsy. Neoplasia 2 (1–2), 9–25. doi:10.1038/sj.neo.7900071
Gaiko-Shcherbak, A., Fabris, G., Dreissen, G., Merkel, R., Hoffmann, B., and Noetzel, E. (2015). The acinar cage: Basement membranes determine molecule exchange and mechanical stability of human breast cell acini. PLoS One 10 (12), e0145174. doi:10.1371/journal.pone.0145174
Ganda, O. P., Williamson, J. R., Soeldner, J. S., Gleason, R. E., Kilo, C., Kaldany, A., et al. (1983). Muscle capillary basement membrane width and its relationship to diabetes mellitus in monozygotic twins. Diabetes 32 (6), 549–556. doi:10.2337/diab.32.6.549
Gaytán, F., Morales, C., Bellido, C., Aguilar, E., and Sánchez-Criado, J. E. (1998). Ovarian follicle macrophages: Is follicular atresia in the immature rat a macrophage-mediated Event?1. Biol. Reprod. 58 (1), 52–59. doi:10.1095/biolreprod58.1.52
Glentis, A., Oertle, P., Mariani, P., Chikina, A., El Marjou, F., Attieh, Y., et al. (2017). Author Correction: Cancer-associated fibroblasts induce metalloprotease-independent cancer cell invasion of the basement membrane. Nat. Commun. 8 (1), 1036. doi:10.1038/s41467-018-03304-x
Glister, C., Richards, S. L., and Knight, P. G. (2005). Bone morphogenetic proteins (BMP) -4, -6, and -7 potently suppress basal and luteinizing hormone-induced androgen production by bovine theca interna cells in primary culture: Could ovarian hyperandrogenic dysfunction be caused by a defect in thecal BMP signaling? Endocrinology 146 (4), 1883–1892. doi:10.1210/en.2004-1303
Griffin, J., Emery, B. R., Huang, I., Peterson, C. M., and Carrell, D. T. (2006). Comparative analysis of follicle morphology and oocyte diameter in four mammalian species (mouse, hamster, pig, and human). J. Exp. Clin. Assist. Reprod. 3, 2. doi:10.1186/1743-1050-3-2
Guevorkian, K., Colbert, M. J., Durth, M., Dufour, S., and Brochard-Wyart, F. (2010). Aspiration of biological viscoelastic drops. Phys. Rev. Lett. 104 (21), 218101. doi:10.1103/PhysRevLett.104.218101
Halfter, W., Candiello, J., Hu, H., Zhang, P., Schreiber, E., and Balasubramani, M. (2013). Protein composition and biomechanical properties of in vivo-derived basement membranes. Cell adh. Migr. 7 (1), 64–71. doi:10.4161/cam.22479
Hannezo, E., and Heisenberg, C. P. (2019). Mechanochemical feedback loops in development and disease. Cell 178 (1), 12–25. doi:10.1016/j.cell.2019.05.052
He, C., Wei, X., Liang, T., Liu, M., Jiang, D., Zhuang, L., et al. (2021). Quantifying the compressive force of 3D cardiac tissues via calculating the volumetric deformation of built-in elastic gelatin microspheres. Adv. Healthc. Mat. 10 (16), 2001716. doi:10.1002/adhm.202001716
He, X. (2017). Microfluidic encapsulation of ovarian follicles for 3D culture. Ann. Biomed. Eng. 45 (7), 1676–1684. doi:10.1007/s10439-017-1823-7
Heeren, A. M., van Iperen, L., Klootwijk, D. B., de Melo Bernardo, A., Roost, M. S., Gomes Fernandes, M. M., et al. (2015). Development of the follicular basement membrane during human gametogenesis and early folliculogenesis. BMC Dev. Biol. 15 (1), 4. doi:10.1186/s12861-015-0054-0
Heisenberg, C. P., and Bellaïche, Y. (2013). Forces in tissue morphogenesis and patterning. Cell 153 (5), 948–962. doi:10.1016/j.cell.2013.05.008
Hemmings, B. A., and Restuccia, D. F. (2012). PI3K-PKB/Akt pathway. Cold Spring Harb. Perspect. Biol. 4 (9), a011189. doi:10.1101/cshperspect.a011189
Henning, N. F. C., and Laronda, M. (2021). The matrisome contributes to the increased rigidity of the bovine ovarian cortex and provides a source of new bioengineering tools to investigate ovarian biology. SSRN J. doi:10.2139/ssrn.3943652
Hertig, A. T., and Adams, E. C. (1967). Studies on the human oocyte and its follicle. I. Ultrastructural and histochemical observations on the primordial follicle stage. J. Cell Biol. 34 (2), 647–675. doi:10.1083/jcb.34.2.647
Honda, A., Hirose, M., Hara, K., Matoba, S., Inoue, K., Miki, H., et al. (2007). Isolation, characterization, and in vitro and in vivo differentiation of putative thecal stem cells. Proc. Natl. Acad. Sci. U. S. A. 104 (30), 12389–12394. doi:10.1073/pnas.0703787104
Hopkins, T. I. R., Bemmer, V. L., Franks, S., Dunlop, C., Hardy, K., and Dunlop, I. E. (2021). Micromechanical mapping of the intact ovary interior reveals contrasting mechanical roles for follicles and stroma. Biomaterials 277, 121099. doi:10.1016/j.biomaterials.2021.121099
Hornick, J. E., Duncan, F. E., Shea, L. D., and Woodruff, T. K. (2012). Isolated primate primordial follicles require a rigid physical environment to survive and grow in vitro. Hum. Reprod. 27 (6), 1801–1810. doi:10.1093/humrep/der468
Horsnell, H. L., Tetley, R. J., De Belly, H., Makris, S., Millward, L. J., Benjamin, A. C., et al. (2022). Lymph node homeostasis and adaptation to immune challenge resolved by fibroblast network mechanics. Nat. Immunol. 23 (8), 1169–1182. doi:10.1038/s41590-022-01272-5
Hu, L., Su, T., Luo, R., Zheng, Y., Huang, J., Zhong, Z., et al. (2019). Hippo pathway functions as a downstream effector of AKT signaling to regulate the activation of primordial follicles in mice. J. Cell. Physiol. 234 (2), 1578–1587. doi:10.1002/jcp.27024
Huang, A., Brennan, K., and Azziz, R. (2010). Prevalence of hyperandrogenemia in the polycystic ovary syndrome diagnosed by the National Institutes of Health 1990 criteria. Fertil. Steril. 93 (6), 1938–1941. doi:10.1016/j.fertnstert.2008.12.138
Huang, C. T. F., Weitsman, S. R., Dykes, B. N., and Magoffin, D. A. (2001). Stem cell factor and insulin-like growth factor-I stimulate luteinizing hormone-independent differentiation of rat ovarian theca cells. Biol. Reprod. 64 (2), 451–456. doi:10.1095/biolreprod64.2.451
Huang, Q., Liu, X., and Guo, S. (2022). Higher fibrotic content of endometriotic lesions is associated with diminished prostaglandin E2 signaling. Reprod. Med. Biol. 21 (1), e12423. doi:10.1002/rmb2.12423
Indana, D., Agarwal, P., Bhutani, N., and Chaudhuri, O. (2021). Viscoelasticity and adhesion signaling in biomaterials control human pluripotent stem cell morphogenesis in 3D culture. Adv. Mat. 33 (43), 2101966. doi:10.1002/adma.202101966
Irving-Rodgers, H. F., and Rodgers, R. J. (2005). Extracellular matrix in ovarian follicular development and disease. Cell Tissue Res. 322 (1), 89–98. doi:10.1007/s00441-005-0042-y
Islam, M. M., and Steward, R. L. (2019). Probing endothelial cell mechanics through connexin 43 disruption. Exp. Mech. 59 (3), 327–336. doi:10.1007/s11340-018-00445-4
John, G. B., Gallardo, T. D., Shirley, L. J., and Castrillon, D. H. (2008). Foxo3 is a PI3K-dependent molecular switch controlling the initiation of oocyte growth. Dev. Biol. 321 (1), 197–204. doi:10.1016/j.ydbio.2008.06.017
John, G. B., Shirley, L. J., Gallardo, T. D., and Castrillon, D. H. (2007). Specificity of the requirement for Foxo3 in primordial follicle activation. Reproduction 133 (5), 855–863. doi:10.1530/REP-06-0051
Johnson, J., Chen, X., Xu, X., and Emerson, J. W. (2015). ŌvSim: A simulation of the population dynamics of mammalian ovarian follicles. Physiology. [Internet][cited 2022 Jun 26]. doi:10.1101/034249
Jones, A. S. K., and Shikanov, A. (2019). Follicle development as an orchestrated signaling network in a 3D organoid. J. Biol. Eng. 13, 2. doi:10.1186/s13036-018-0134-3
Jones, R. L., and Pepling, M. E. (2013). KIT signaling regulates primordial follicle formation in the neonatal mouse ovary. Dev. Biol. 382 (1), 186–197. doi:10.1016/j.ydbio.2013.06.030
Kasuya, K. (1995). The process of apoptosis in follicular epithelial cells in the rabbit ovary, with special reference to involvement by macrophages. Arch. Histol. Cytol. 58 (2), 257–264. doi:10.1679/aohc.58.257
Kawamura, K., Cheng, Y., Suzuki, N., Deguchi, M., Sato, Y., Takae, S., et al. (2013). Hippo signaling disruption and Akt stimulation of ovarian follicles for infertility treatment. Proc. Natl. Acad. Sci. U. S. A. 110 (43), 17474–17479. doi:10.1073/pnas.1312830110
Kenny, H. A., Krausz, T., Yamada, S. D., and Lengyel, E. (2007). Use of a novel 3D culture model to elucidate the role of mesothelial cells, fibroblasts and extra-cellular matrices on adhesion and invasion of ovarian cancer cells to the omentum. Int. J. Cancer 121 (7), 1463–1472. doi:10.1002/ijc.22874
Kim, J., Perez, A. S., Claflin, J., David, A., Zhou, H., and Shikanov, A. (2016). Synthetic hydrogel supports the function and regeneration of artificial ovarian tissue in mice. NPJ Regen. Med. 1 (1), 16010. doi:10.1038/npjregenmed.2016.10
Kim, S. N., Jeibmann, A., Halama, K., Witte, H. T., Wälte, M., Matzat, T., et al. (2014). ECM stiffness regulates glial migration in Drosophila and mammalian glioma models. Development 141 (16), 3233–3242. doi:10.1242/dev.106039
Kim, S. Y., and Nair, M. G. (2019). Macrophages in wound healing: Activation and plasticity. Immunol. Cell Biol. 97 (3), 258–267. doi:10.1111/imcb.12236
Kinnear, H. M., Tomaszewski, C. E., Chang, F. L., Moravek, M. B., Xu, M., Padmanabhan, V., et al. (2020). The ovarian stroma as a new frontier. Reproduction 160 (3), R25–R39. doi:10.1530/REP-19-0501
Ko, C., Gieske, M. C., Al-Alem, L., Hahn, Y., Su, W., Gong, M. C., et al. (2006). Endothelin-2 in ovarian follicle rupture. Endocrinology 147 (4), 1770–1779. doi:10.1210/en.2005-1228
Koester, J., Miroshnikova, Y. A., Ghatak, S., Chacón-Martínez, C. A., Morgner, J., Li, X., et al. (2021). Niche stiffening compromises hair follicle stem cell potential during ageing by reducing bivalent promoter accessibility. Nat. Cell Biol. 23 (7), 771–781. doi:10.1038/s41556-021-00705-x
Komatsu, K., Iwase, A., Murase, T., and Masubuchi, S. (2018). Ovarian tissue culture to visualize phenomena in mouse ovary. J. Vis. Exp. (136), 57794. doi:10.3791/57794
Komatsu, K., and Masubuchi, S. (2017). Observation of the dynamics of follicular development in the ovary. Reprod. Med. Biol. 16 (1), 21–27. doi:10.1002/rmb2.12010
Laronda, M. M., Jakus, A. E., Whelan, K. A., Wertheim, J. A., Shah, R. N., and Woodruff, T. K. (2015). Initiation of puberty in mice following decellularized ovary transplant. Biomaterials 50, 20–29. doi:10.1016/j.biomaterials.2015.01.051
Lecuit, T., and Lenne, P. F. (2007). Cell surface mechanics and the control of cell shape, tissue patterns and morphogenesis. Nat. Rev. Mol. Cell Biol. 8 (8), 633–644. doi:10.1038/nrm2222
Lee, H. J., Diaz, M. F., Price, K. M., Ozuna, J. A., Zhang, S., Sevick-Muraca, E. M., et al. (2017). Fluid shear stress activates YAP1 to promote cancer cell motility. Nat. Commun. 8 (1), 14122. doi:10.1038/ncomms14122
Lee, W. S., Otsuka, F., Moore, R. K., and Shimasaki, S. (2001). Effect of bone morphogenetic protein-7 on folliculogenesis and ovulation in the rat. Biol. Reprod. 65 (4), 994–999. doi:10.1095/biolreprod65.4.994
LeGoff, L., Rouault, H., and Lecuit, T. (2013). A global pattern of mechanical stress polarizes cell divisions and cell shape in the growing Drosophila wing disc. Development 140 (19), 4051–4059. doi:10.1242/dev.090878
Lei, L., and Spradling, A. C. (2016). Mouse oocytes differentiate through organelle enrichment from sister cyst germ cells. Science 352, 95–99. doi:10.1126/science.aad2156
Lei, L., and Spradling, A. C. (2013). Mouse primordial germ cells produce cysts that partially fragment prior to meiosis. Development 140 (10), 2075–2081. doi:10.1242/dev.093864
Li, S. K. B., and Hearn, M. T. W. (2000). Isolation of thecal cells: An assessment of purity and steroidogenic potential. J. Biochem. Biophys. Methods 45 (2), 169–181. doi:10.1016/s0165-022x(00)00107-x
Lin, H. C. A., Dutta, R., Mandal, S., Kind, A., Schnieke, A., and Razansky, D. (2017). “Light-sheet microscopy for quantitative ovarian folliculometry,” in San francisco. Editors M. C. Skala, and P. J. Campagnola (California: United States), 100430K. doi:10.1117/12.2252242
Liu, C., Peng, J., Matzuk, M. M., and Yao, H. H. C. (2015). Lineage specification of ovarian theca cells requires multicellular interactions via oocyte and granulosa cells. Nat. Commun. 6, 6934. doi:10.1038/ncomms7934
Liu, L., Rajareddy, S., Reddy, P., Du, C., Jagarlamudi, K., Shen, Y., et al. (2007). Infertility caused by retardation of follicular development in mice with oocyte-specific expression of Foxo3a. Development 134 (1), 199–209. doi:10.1242/dev.02667
Lliberos, C., Liew, S. H., Zareie, P., La Gruta, N. L., Mansell, A., and Hutt, K. (2021). Evaluation of inflammation and follicle depletion during ovarian ageing in mice. Sci. Rep. 11 (1), 278. doi:10.1038/s41598-020-79488-4
Magoffin, D. A., Hubert-Leslie, D., and Zachow, R. J. (1995). Estradiol-17 beta, insulin-like growth factor-I, and luteinizing hormone inhibit secretion of transforming growth factor beta by rat ovarian theca-interstitial cells. Biol. Reprod. 53 (3), 627–635. doi:10.1095/biolreprod53.3.627
Magoffin, D. A., and Weitsman, S. R. (1994). Insulin-like growth factor-I regulation of luteinizing hormone (LH) receptor messenger ribonucleic acid expression and LH-stimulated signal transduction in rat ovarian theca-interstitial cells. Biol. Reprod. 51 (4), 766–775. doi:10.1095/biolreprod51.4.766
Maître, J. L., Turlier, H., Illukkumbura, R., Eismann, B., Niwayama, R., Nédélec, F., et al. (2016). Asymmetric division of contractile domains couples cell positioning and fate specification. Nature 536 (7616), 344–348. doi:10.1038/nature18958
Manuel, S. L., Antonova, E., Hornick, J. E., Riera, F. A., Wei, J. J., Pavone, M. E., et al. (2019). Collagen and hyaluronan matrices undergo age-related changes in the human ovary. Fertil. Steril. 112 (3), e252–e253. doi:10.1016/j.fertnstert.2019.07.1406
Matousek, M., Carati, C., Gannon, B., and Brännström, M. (2001). Novel method for intrafollicular pressure measurements in the rat ovary: Increased intrafollicular pressure after hCG stimulation. Reproduction 8, 307–314. doi:10.1530/rep.0.1210307
Matsuura, T., Sugimura, M., Iwaki, T., Ohashi, R., Kanayama, N., and Nishihira, J. (2002). Anti-macrophage inhibitory factor antibody inhibits PMSG-hCG-induced follicular growth and ovulation in mice. J. Assist. Reprod. Genet. 19 (12), 591–595. doi:10.1023/a:1021219317155
McGee, E. A., and Hsueh, A. J. (2000). Initial and cyclic recruitment of ovarian follicles. Endocr. Rev. 21 (2), 200–214. doi:10.1210/edrv.21.2.0394
McWhorter, F. Y., Davis, C. T., and Liu, W. F. (2015). Physical and mechanical regulation of macrophage phenotype and function. Cell. Mol. Life Sci. 72 (7), 1303–1316. doi:10.1007/s00018-014-1796-8
Miller, R. T. (2017). Mechanical properties of basement membrane in health and disease. Matrix Biol. 57 (58), 366–373. doi:10.1016/j.matbio.2016.07.001
Mizunuma, H., Liu, X., Andoh, K., Abe, Y., Kobayashi, J., Yamada, K., et al. (1999). Activin from secondary follicles causes small preantral follicles to remain dormant at the resting stage. Endocrinology 140 (1), 37–42. doi:10.1210/endo.140.1.6409
Mohagheghian, E., Luo, J., Chen, J., Chaudhary, G., Chen, J., Sun, J., et al. (2018). Quantifying compressive forces between living cell layers and within tissues using elastic round microgels. Nat. Commun. 9 (1), 1878. doi:10.1038/s41467-018-04245-1
Mongera, A., Rowghanian, P., Gustafson, H. J., Shelton, E., Kealhofer, D. A., Carn, E. K., et al. (2018). A fluid-to-solid jamming transition underlies vertebrate body axis elongation. Nature 561 (7723), 401–405. doi:10.1038/s41586-018-0479-2
Monslow, J., Govindaraju, P., and Puré, E. (2015). Hyaluronan - a functional and structural sweet spot in the tissue microenvironment. Front. Immunol. 6, 231. doi:10.3389/fimmu.2015.00231
Moore, R. K., Otsuka, F., and Shimasaki, S. (2003). Molecular basis of bone morphogenetic protein-15 signaling in granulosa cells. J. Biol. Chem. 278 (1), 304–310. doi:10.1074/jbc.M207362200
Mora, J. M., Fenwick, M. A., Castle, L., Baithun, M., Ryder, T. A., Mobberley, M., et al. (2012). Characterization and significance of adhesion and junction-related proteins in mouse ovarian follicles. Biol. Reprod. 86 (5153), 1–14. doi:10.1095/biolreprod.111.096156
Mosaliganti, K. R., Swinburne, I. A., Chan, C. U., Obholzer, N. D., Green, A. A., Tanksale, S., et al. (2019). Size control of the inner ear via hydraulic feedback. eLife 8, e39596. doi:10.7554/eLife.39596
Mostaço-Guidolin, L., Rosin, N., and Hackett, T. L. (2017). Imaging collagen in scar tissue: Developments in second harmonic generation microscopy for biomedical applications. Int. J. Mol. Sci. 18 (8), 1772. doi:10.3390/ijms18081772
Munjal, A., Hannezo, E., Tsai, T. Y. C., Mitchison, T. J., and Megason, S. G. (2021). Extracellular hyaluronate pressure shaped by cellular tethers drives tissue morphogenesis. Cell 184 (26), 6313–6325.e18. e18. doi:10.1016/j.cell.2021.11.025
Nagamatsu, G., Shimamoto, S., Hamazaki, N., Nishimura, Y., and Hayashi, K. (2019). Mechanical stress accompanied with nuclear rotation is involved in the dormant state of mouse oocytes. Sci. Adv. 5 (6), eaav9960. doi:10.1126/sciadv.aav9960
Nakano, K., Naito, I., Momota, R., Sado, Y., Hasegawa, H., Ninomiya, Y., et al. (2007). The distribution of type IV collagen alpha chains in the mouse ovary and its correlation with follicular development. Arch. Histol. Cytol. 70 (4), 243–253. doi:10.1679/aohc.70.243
Nam, S., Gupta, V. K., Leepyo, H., Lee, J. Y., Wisdom, K. M., Varma, S., et al. (2019). Cell cycle progression in confining microenvironments is regulated by a growth-responsive TRPV4-PI3K/Akt-p27 Kip1 signaling axis. Sci. Adv. 5 (8), eaaw6171. doi:10.1126/sciadv.aaw6171
Nicholas, A. K., and Jacques, P. B. (2005). “Morphology and ultrastructure of basement membranes,” in Current topics in membranes (Elsevier), 19–42.
Orisaka, M., Jiang, J. Y., Orisaka, S., Kotsuji, F., and Tsang, B. K. (2009). Growth differentiation factor 9 promotes rat preantral follicle growth by up-regulating follicular androgen biosynthesis. Endocrinology 150 (6), 2740–2748. doi:10.1210/en.2008-1536
O’Shea, J. D., Cran, D. G., Hay, M. F., and Moor, R. M. (1978). Ultrastructure of the theca interna of ovarian follicles in sheep. Cell Tissue Res. 187 (3), 457–472. doi:10.1007/BF00229610
Otsuka, F., and Shimasaki, S. (2002). A negative feedback system between oocyte bone morphogenetic protein 15 and granulosa cell kit ligand: Its role in regulating granulosa cell mitosis. Proc. Natl. Acad. Sci. U. S. A. 99 (12), 8060–8065. doi:10.1073/pnas.122066899
Ouni, E., Bouzin, C., Dolmans, M. M., Marbaix, E., Pyr Dit Ruys, S., Vertommen, D., et al. (2020). Spatiotemporal changes in mechanical matrisome components of the human ovary from prepuberty to menopause. Hum. Reprod. 35 (6), 1391–1410. doi:10.1093/humrep/deaa100
Ouni, E., Nedbal, V., Da Pian, M., Cao, H., Haas, K. T., Peaucelle, A., et al. (2022). Proteome-wide and matrisome-specific atlas of the human ovary computes fertility biomarker candidates and open the way for precision oncofertility. Matrix Biol. 109, 91–120. doi:10.1016/j.matbio.2022.03.005
Ouni, E., Peaucelle, A., Haas, K. T., Van Kerk, O., Dolmans, M. M., Tuuri, T., et al. (2021). A blueprint of the topology and mechanics of the human ovary for next-generation bioengineering and diagnosis. Nat. Commun. 12 (1), 5603. doi:10.1038/s41467-021-25934-4
Ouni, E., Vertommen, D., Chiti, M. C., Dolmans, M. M., and Amorim, C. A. (2019). A draft map of the human ovarian proteome for tissue engineering and clinical applications. Mol. Cell. Proteomics 18, S159–S173. doi:10.1074/mcp.RA117.000469
Pangas, S. A., Saudye, H., Shea, L. D., and Woodruff, T. K. (2003). Novel approach for the three-dimensional culture of granulosa cell–oocyte complexes. Tissue Eng. 9 (5), 1013–1021. doi:10.1089/107632703322495655
Parkes, W. S., Amargant, F., Zhou, L. T., Villanueva, C. E., Duncan, F. E., and Pritchard, M. T. (2021). Hyaluronan and collagen are prominent extracellular matrix components in bovine and porcine ovaries. Genes 12 (8), 1186. doi:10.3390/genes12081186
Pelosi, E., Omari, S., Michel, M., Ding, J., Amano, T., Forabosco, A., et al. (2013). Constitutively active Foxo3 in oocytes preserves ovarian reserve in mice. Nat. Commun. 4 (1), 1843. doi:10.1038/ncomms2861
Perez Gonzalez, N., Tao, J., Rochman, N. D., Vig, D., Chiu, E., Wirtz, D., et al. (2018). Cell tension and mechanical regulation of cell volume. Mol. Biol. Cell 29 (21), 0. doi:10.1091/mbc.E18-04-0213
Perloff, W. H., Schultz, J., Farris, E. J., and Balin, H. (1954). Some aspects of the chemical nature of human ovarian follicular fluid. Fertil. Steril. 6 (1), 11–17. doi:10.1016/s0015-0282(16)31861-1
Petersen, A., Joly, P., Bergmann, C., Korus, G., and Duda, G. N. (2012). The impact of substrate stiffness and mechanical loading on fibroblast-induced scaffold remodeling. Tissue Eng. Part A 18 (17–18), 1804–1817. doi:10.1089/ten.TEA.2011.0514
Petridou, N. I., Grigolon, S., Salbreux, G., Hannezo, E., and Heisenberg, C. P. (2019). Fluidization-mediated tissue spreading by mitotic cell rounding and non-canonical Wnt signalling. Nat. Cell Biol. 21 (2), 169–178. doi:10.1038/s41556-018-0247-4
Petrovská, M., Dimitrov, D. G., and Michael, S. D. (1996). Quantitative changes in macrophage distribution in normal mouse ovary over the course of the estrous cycle examined with an image analysis system. Am. J. Reprod. Immunol. 36 (3), 175–183. doi:10.1111/j.1600-0897.1996.tb00159.x
Power, R. M., and Huisken, J. (2017). A guide to light-sheet fluorescence microscopy for multiscale imaging. Nat. Methods 14 (4), 360–373. doi:10.1038/nmeth.4224
Prevedel, R., Diz-Muñoz, A., Ruocco, G., and Antonacci, G. (2019). Brillouin microscopy: An emerging tool for mechanobiology. Nat. Methods 16 (10), 969–977. doi:10.1038/s41592-019-0543-3
Quiros, R. M., Valianou, M., Kwon, Y., Brown, K. M., Godwin, A. K., and Cukierman, E. (2008). Ovarian normal and tumor-associated fibroblasts retain in vivo stromal characteristics in a 3-D matrix-dependent manner. Gynecol. Oncol. 110 (1), 99–109. doi:10.1016/j.ygyno.2008.03.006
Ramos-Lewis, W., and Page-McCaw, A. (2019). Basement membrane mechanics shape development: Lessons from the fly. Matrix Biol. 75–76, 72–81. doi:10.1016/j.matbio.2018.04.004
Riquelme, M. A., Cardenas, E. R., Xu, H., and Jiang, J. X. (2020). The role of connexin channels in the response of mechanical loading and unloading of bone. Int. J. Mol. Sci. 21 (3), E1146. doi:10.3390/ijms21031146
Rodgers, R. J., and Irving-Rodgers, H. F. (2010). formation of the ovarian follicular antrum and follicular fluid. Biol. Reprod. 82 (6), 1021–1029. doi:10.1095/biolreprod.109.082941
Rodgers, R. J., Irving-Rodgers, H. F., and Russell, D. L. (2003). Extracellular matrix of the developing ovarian follicle. Reproduction 126 (4), 415–424. doi:10.1530/rep.0.1260415
Rodgers, R. J., Irving-Rodgers, H. F., van Wezel, I. L., Krupa, M., and Lavranos, T. C. (2001). Dynamics of the membrana granulosa during expansion of the ovarian follicular antrum. Mol. Cell. Endocrinol. 171 (1–2), 41–48. doi:10.1016/s0303-7207(00)00430-5
Roignot, J., Peng, X., and Mostov, K. (2013). Polarity in mammalian epithelial morphogenesis. Cold Spring Harb. Perspect. Biol. 5 (2), a013789. doi:10.1101/cshperspect.a013789
Rowley, J. E., Amargant, F., Zhou, L. T., Galligos, A., Simon, L. E., Pritchard, M. T., et al. (2020). Low molecular weight hyaluronan induces an inflammatory response in ovarian stromal cells and impairs gamete development in vitro. Int. J. Mol. Sci. 21 (3), 1036. doi:10.3390/ijms21031036
Ryan, A. Q., Chan, C. J., Graner, F., and Hiiragi, T. (2019). Lumen expansion facilitates epiblast-primitive endoderm fate specification during mouse blastocyst formation. Dev. Cell 51 (6), 684–697. e4. doi:10.1016/j.devcel.2019.10.011
Saglani, S., Molyneux, C., Gong, H., Rogers, A., Malmström, K., Pelkonen, A., et al. (2006). Ultrastructure of the reticular basement membrane in asthmatic adults, children and infants. Eur. Respir. J. 28 (3), 505–512. doi:10.1183/09031936.06.00056405
Sahai, E., Astsaturov, I., Cukierman, E., DeNardo, D. G., Egeblad, M., Evans, R. M., et al. (2020). A framework for advancing our understanding of cancer-associated fibroblasts. Nat. Rev. Cancer 20 (3), 174–186. doi:10.1038/s41568-019-0238-1
Salustri, A., CamAioni, A., Fulop, C., and Hascall, V. C. (1999). Hyaluronan and proteoglycans in ovarian follicles. Hum. Reprod. Update 5 (4), 293–301. doi:10.1093/humupd/5.4.293
Sánchez-Sánchez, B. J., Urbano, J. M., Comber, K., Dragu, A., Wood, W., Stramer, B., et al. (2017). Drosophila embryonic hemocytes produce laminins to strengthen migratory response. Cell Rep. 21 (6), 1461–1470. doi:10.1016/j.celrep.2017.10.047
Schliffka, M. F., and Maître, J. L. (2019). Stay hydrated: Basolateral fluids shaping tissues. Curr. Opin. Genet. Dev. 57, 70–77. doi:10.1016/j.gde.2019.06.015
Shalgi, R., Kraicer, P., Rimon, A., Pinto, M., and Soferman, N. (1973). Proteins of human follicular fluid: The blood-follicle barrier. Fertil. Steril. 24 (6), 429–434. doi:10.1016/s0015-0282(16)39730-8
Shikanov, A., Xu, M., Woodruff, T. K., and Shea, L. D. (2011). A method for ovarian follicle encapsulation and culture in a proteolytically degradable 3 dimensional system. J. Vis. Exp. (49), 2695. doi:10.3791/2695
Shin, S. Y., Lee, H. J., Ko, D. S., Lee, H. C., and Park, W. I. (2005). The regulators of VEGF expression in mouse ovaries. Yonsei Med. J. 46 (5), 679–686. doi:10.3349/ymj.2005.46.5.679
Shiomi-Sugaya, N., Komatsu, K., Wang, J., Yamashita, M., Kikkawa, F., and Iwase, A. (2015). Regulation of secondary follicle growth by theca cells and insulin-like growth factor 1. J. Reprod. Dev. 61 (3), 161–168. doi:10.1262/jrd.2014-107
Sigurbjörnsdóttir, S., Mathew, R., and Leptin, M. (2014). Molecular mechanisms of de novo lumen formation. Nat. Rev. Mol. Cell Biol. 15 (10), 665–676. doi:10.1038/nrm3871
Simon, A. M., Goodenough, D. A., Li, E., and Paul, D. L. (1997). Female infertility in mice lacking connexin 37. Nature 385 (6616), 525–529. doi:10.1038/385525a0
Smith, M. F., McIntush, E. W., Ricke, W. A., Kojima, F. N., and Smith, G. W. (1999). Regulation of ovarian extracellular matrix remodelling by metalloproteinases and their tissue inhibitors: Effects on follicular development, ovulation and luteal function. J. Reprod. Fertil. Suppl. 54, 367–381.
Souchaud, A., Boutillon, A., Charron, G., Asnacios, A., Noûs, C., David, N. B., et al. (2022). Live 3D imaging and mapping of shear stresses within tissues using incompressible elastic beads. Development 149 (4), dev199765. doi:10.1242/dev.199765
Spears, N., de Bruin, J. P., and Gosden, R. G. (1996). The establishment of follicular dominance in co-cultured mouse ovarian follicles. J. Reprod. Fertil. 106 (1), 1–6. doi:10.1530/jrf.0.1060001
Sridharan, R., Cavanagh, B., Cameron, A. R., Kelly, D. J., and O’Brien, F. J. (2019). Material stiffness influences the polarization state, function and migration mode of macrophages. Acta Biomater. 89, 47–59. doi:10.1016/j.actbio.2019.02.048
St John, M. A. R., Tao, W., Fei, X., Fukumoto, R., Carcangiu, M. L., Brownstein, D. G., et al. (1999). Mice deficient of Lats1 develop soft-tissue sarcomas, ovarian tumours and pituitary dysfunction. Nat. Genet. 21 (2), 182–186. doi:10.1038/5965
Stassen, O. M. J. A., Ristori, T., and Sahlgren, C. M. (2020). Notch in mechanotransduction - from molecular mechanosensitivity to tissue mechanostasis. J. Cell Sci. 133 (24), jcs250738. doi:10.1242/jcs.250738
Stylianopoulos, T., Martin, J. D., Chauhan, V. P., Jain, S. R., Diop-Frimpong, B., Bardeesy, N., et al. (2012). Causes, consequences, and remedies for growth-induced solid stress in murine and human tumors. Proc. Natl. Acad. Sci. U. S. A. 109 (38), 15101–15108. doi:10.1073/pnas.1213353109
Sugimura, K., Lenne, P. F., and Graner, F. (2016). Measuring forces and stresses in situ in living tissues. Development 143 (2), 186–196. doi:10.1242/dev.119776
Sugiura, K., Pendola, F. L., and Eppig, J. J. (2005). Oocyte control of metabolic cooperativity between oocytes and companion granulosa cells: Energy metabolism. Dev. Biol. 279 (1), 20–30. doi:10.1016/j.ydbio.2004.11.027
Swanson, E. A., Izatt, J. A., Lin, C. P., Fujimoto, J. G., Schuman, J. S., Hee, M. R., et al. (1993). In vivo retinal imaging by optical coherence tomography. Opt. Lett. 18 (21), 1864–1866. doi:10.1364/ol.18.001864
Takaya, R., Fukaya, T., Sasano, H., Suzuki, T., Tamura, M., and Yajima, A. (1997). Macrophages in normal cycling human ovaries; immunohistochemical localization and characterization. Hum. Reprod. 12 (7), 1508–1512. doi:10.1093/humrep/12.7.1508
Taubenberger, A. V., Girardo, S., Träber, N., Fischer-Friedrich, E., Kräter, M., Wagner, K., et al. (2019). 3D microenvironment stiffness regulates tumor spheroid growth and mechanics via p21 and ROCK. Adv. Biosyst. 3 (9), e1900128. doi:10.1002/adbi.201900128
Thomasy, S. M., Morgan, J. T., Wood, J. A., Murphy, C. J., and Russell, P. (2013). Substratum stiffness and latrunculin B modulate the gene expression of the mechanotransducers YAP and TAZ in human trabecular meshwork cells. Exp. Eye Res. 113, 66–73. doi:10.1016/j.exer.2013.05.014
Thuwajit, C., Ferraresi, A., Titone, R., Thuwajit, P., and Isidoro, C. (2018). The metabolic cross-talk between epithelial cancer cells and stromal fibroblasts in ovarian cancer progression: Autophagy plays a role. Med. Res. Rev. 38 (4), 1235–1254. doi:10.1002/med.21473
Tian, Y., Shen, W., Lai, Z., Shi, L., Yang, S., Ding, T., et al. (2015). Isolation and identification of ovarian theca-interstitial cells and granulose cells of immature female mice: Isolation of theca-interstitial cells and granulosa cells. Cell Biol. Int. 39 (5), 584–590. doi:10.1002/cbin.10426
Träber, N., Uhlmann, K., Girardo, S., Kesavan, G., Wagner, K., Friedrichs, J., et al. (2019). Polyacrylamide bead sensors for in vivo quantification of cell-scale stress in zebrafish development. Sci. Rep. 9 (1), 17031. doi:10.1038/s41598-019-53425-6
Trombly, D. J., Woodruff, T. K., and Mayo, K. E. (2009). Suppression of Notch signaling in the neonatal mouse ovary decreases primordial follicle formation. Endocrinology 150 (2), 1014–1024. doi:10.1210/en.2008-0213
Tse, J. M., Cheng, G., Tyrrell, J. A., Wilcox-Adelman, S. A., Boucher, Y., Jain, R. K., et al. (2012). Mechanical compression drives cancer cells toward invasive phenotype. Proc. Natl. Acad. Sci. U. S. A. 109 (3), 911–916. doi:10.1073/pnas.1118910109
Umehara, T., Winstanley, Y. E., Andreas, E., Morimoto, A., Williams, E. J., Smith, K. M., et al. (2022). Female reproductive life span is extended by targeted removal of fibrotic collagen from the mouse ovary. Sci. Adv. 8 (24), eabn4564. doi:10.1126/sciadv.abn4564
Vignes, H., Vagena-Pantoula, C., Prakash, M., Fukui, H., Norden, C., Mochizuki, N., et al. (2022). Extracellular mechanical forces drive endocardial cell volume decrease during zebrafish cardiac valve morphogenesis. Dev. Cell 57 (5), 598–609.e5. e5. doi:10.1016/j.devcel.2022.02.011
Viola, J. M., Liu, J., Prahl, L. S., Huang, A., Trevor, J., Chan, G., et al. (2022). Tubule jamming in the developing kidney creates cyclical mechanical stresses instructive to nephron formation. doi:10.1101/2022.06.03.494718
Voutouri, C., and Stylianopoulos, T. (2018). “Accumulation of mechanical forces in tumors is related to hyaluronan content and tissue stiffness,PLoS One. 13, e0193801. doi:10.1371/journal.pone.0193801
Wang, H. X., Li, T. Y., and Kidder, G. M. (2010). WNT2 regulates DNA synthesis in mouse granulosa cells through beta-catenin. Biol. Reprod. 82 (5), 865–875. doi:10.1095/biolreprod.109.080903
Wang, S., and Larina, I. V. (2021). In vivo dynamic 3D imaging of oocytes and embryos in the mouse oviduct. Cell Rep. 36 (2), 109382. doi:10.1016/j.celrep.2021.109382
Wang, S., and Larina, I. V. (2018). In vivo three-dimensional tracking of sperm behaviors in the mouse oviduct. Development 145, 157685. doi:10.1242/dev.157685
Wang, S., Matsumoto, K., Lish, S. R., Cartagena-Rivera, A. X., and Yamada, K. M. (2021). Budding epithelial morphogenesis driven by cell-matrix versus cell-cell adhesion. Cell 184 (14), 3702–3716.e30. e30. doi:10.1016/j.cell.2021.05.015
Wang, T., Brewer, M., and Zhu, Q. (2015). An overview of optical coherence tomography for ovarian tissue imaging and characterization: An overview of optical coherence tomography. Wiley Interdiscip. Rev. Nanomed. Nanobiotechnol. 7 (1), 1–16. doi:10.1002/wnan.1306
Watanabe, Y., Takakura, K., Kurotani, R., and Abe, H. (2015). Optical coherence tomography imaging for analysis of follicular development in ovarian tissue. Appl. Opt. 54 (19), 6111–6115. doi:10.1364/AO.54.006111
Watson, J. M., Rice, P. F., Marion, S. L., Brewer, M. A., Davis, J. R., Rodriguez, J. J., et al. (2012). Analysis of second-harmonic-generation microscopy in a mouse model of ovarian carcinoma. J. Biomed. Opt. 17 (7), 076002. doi:10.1117/1.JBO.17.7.076002
Wells, R. G. (2013). Tissue mechanics and fibrosis. Biochim. Biophys. Acta 1832 (7), 884–890. doi:10.1016/j.bbadis.2013.02.007
West, E., Xu, M., Woodruff, T., and Shea, L. (2007). Physical properties of alginate hydrogels and their effects on in vitro follicle development. Biomaterials 28 (30), 4439–4448. doi:10.1016/j.biomaterials.2007.07.001
West-Farrell, E. R., Xu, M., Gomberg, M. A., Chow, Y. H., Woodruff, T. K., and Shea, L. D. (2009). The mouse follicle microenvironment regulates antrum formation and steroid production: Alterations in gene expression profiles. Biol. Reprod. 80 (3), 432–439. doi:10.1095/biolreprod.108.071142
Wijgerde, M., Ooms, M., Hoogerbrugge, J. W., and Grootegoed, J. A. (2005). Hedgehog signaling in mouse ovary: Indian hedgehog and desert hedgehog from granulosa cells induce target gene expression in developing theca cells. Endocrinology 146 (8), 3558–3566. doi:10.1210/en.2005-0311
Winterhager, E., and Kidder, G. M. (2015). Gap junction connexins in female reproductive organs: Implications for women’s reproductive health. Hum. Reprod. Update 21 (3), 340–352. doi:10.1093/humupd/dmv007
Wood, C. D., Vijayvergia, M., Miller, F. H., Carroll, T., Fasanati, C., Shea, L. D., et al. (2015). Multi-modal magnetic resonance elastography for noninvasive assessment of ovarian tissue rigidity in vivo. Acta Biomater. 13, 295–300. doi:10.1016/j.actbio.2014.11.022
Woodruff, T. K., and Shea, L. D. (2011). A new hypothesis regarding ovarian follicle development: Ovarian rigidity as a regulator of selection and health. J. Assist. Reprod. Genet. 28 (1), 3–6. doi:10.1007/s10815-010-9478-4
Woodruff, T. K., and Shea, L. D. (2007). The role of the extracellular matrix in ovarian follicle development. Reprod. Sci. 14, 6–10. doi:10.1177/1933719107309818
Wu, Z., and Guan, K. L. (2021). Hippo signaling in embryogenesis and development. Trends biochem. Sci. 46 (1), 51–63. doi:10.1016/j.tibs.2020.08.008
Xu, M., Kreeger, P. K., Shea, L. D., and Woodruff, T. K. (2006). Tissue-Engineered follicles produce live, fertile offspring. Tissue Eng. 12 (10), 2739–2746. doi:10.1089/ten.2006.12.2739
Xu, M., West, E., Shea, L. D., and Woodruff, T. K. (2006). Identification of a stage-specific permissive in vitro culture environment for follicle growth and oocyte development. Biol. Reprod. 75 (6), 916–923. doi:10.1095/biolreprod.106.054833
Yang, Y., Paivinen, P., Xie, C., Krup, A. L., Makela, T. P., Mostov, K. E., et al. (2021). Ciliary Hedgehog signaling patterns the digestive system to generate mechanical forces driving elongation. Nat. Commun. 12 (1), 7186. doi:10.1038/s41467-021-27319-z
Young, J. M., and McNeilly, A. S. (2010). Theca: The forgotten cell of the ovarian follicle. Reproduction 140 (4), 489–504. doi:10.1530/REP-10-0094
Zhang, Z., Schlamp, F., Huang, L., Clark, H., and Brayboy, L. (2020). Inflammaging is associated with shifted macrophage ontogeny and polarization in the aging mouse ovary. Reproduction 159 (3), 325–337. doi:10.1530/REP-19-0330
Zhao, B., Wei, X., Li, W., Udan, R. S., Yang, Q., Kim, J., et al. (2007). Inactivation of YAP oncoprotein by the Hippo pathway is involved in cell contact inhibition and tissue growth control. Genes Dev. 21 (21), 2747–2761. doi:10.1101/gad.1602907
Zhao, D., Liu, R., Li, G., Chen, M., Shang, P., Yang, H., et al. (2020). Connexin 43 channels in osteocytes regulate bone responses to mechanical unloading. Front. Physiol. 11, 299. doi:10.3389/fphys.2020.00299
Keywords: folliculogenesis, mechanobiology, oocyte, mechanotransduction, mammalian reproduction, theca cell
Citation: Biswas A, Ng BH, Prabhakaran VS and Chan CJ (2022) Squeezing the eggs to grow: The mechanobiology of mammalian folliculogenesis. Front. Cell Dev. Biol. 10:1038107. doi: 10.3389/fcell.2022.1038107
Received: 06 September 2022; Accepted: 16 November 2022;
Published: 02 December 2022.
Edited by:
Naotaka Nakazawa, Kindai University, JapanReviewed by:
Ariella Shikanov, University of Michigan, United StatesChristiani A. Amorim, Université Catholique de Louvain, Belgium
Copyright © 2022 Biswas, Ng, Prabhakaran and Chan. This is an open-access article distributed under the terms of the Creative Commons Attribution License (CC BY). The use, distribution or reproduction in other forums is permitted, provided the original author(s) and the copyright owner(s) are credited and that the original publication in this journal is cited, in accordance with accepted academic practice. No use, distribution or reproduction is permitted which does not comply with these terms.
*Correspondence: Chii Jou Chan, ZGJzY2hpaUBudXMuZWR1LnNn