- 1Department of Hematology, Tongji Hospital, Tongji Medical College, Huazhong University of Science and Technology, Wuhan, China
- 2Department of Hematology, Wuhan No. 1 Hospital, Wuhan, China
The functional state of chimeric antigen receptor T (CAR T) cells determines their efficacy in vivo. Exhausted CAR T cells exhibit decreased proliferative capacity, impaired anti-tumor activity, and attenuated persistence. CAR T cell exhaustion has been recognized as a vital cause of nonresponse and relapse after CAR T cell therapy. However, the triggers and mechanisms leading to CAR T cell exhaustion remain blurry and complicated. Therefore, it is essential to clear the regulation network of CAR T cell exhaustion and explore potent solutions. Here, we review the diverse inducers of CAR T cell exhaustion in terms of manufacture process and immunosuppressive tumor microenvironment. In addition to the admitted immune checkpoint blockade, we also describe promising strategies that may reverse CAR T cell exhaustion including targeting the tumor microenvironment, epigenetics and transcriptomics.
Introduction
Despite the tremendous efficacy of chimeric antigen receptor T (CAR T) cells in hematological malignancies such as B-cell acute lymphoblastic leukemia (B-ALL), B-cell lymphoma, and multiple myeloma, as well as in solid tumors, CAR T cell exhaustion remained a main obstacle to achieve remission (Fraietta et al., 2018; Holstein and Lunning, 2019; Marofi et al., 2021). T cell exhaustion was a dysfunctional state of T cells that usually observed in chronic infections and tumors (Hashimoto et al., 2018). It was first identified as a population of CD8+ T cells without effector function which appeared during chronic lymphocytic choriomeningitis virus infection in mice, and the persistence of this cell population led to viral immune escape (Zajac et al., 1998). At the same time, Gallimore and colleagues also observed a similar phenomenon in the chronic lymphocytic choriomeningitis virus infection, which they defined as T cell exhaustion (Gallimore et al., 1998). T cell exhaustion was characterized by progressive loss of effector function, co-expression of multiple inhibitory receptors, and exaggerated effector cell differentiation. Besides, the exhausted T cells had distinct transcriptional, epigenetic, and metabolic signatures (Doering et al., 2012; Schietinger and Greenberg, 2014; Philip et al., 2017).
Abundant clinical practice and research revealed that CAR T cell exhaustion ultimately contributed to the failure of CAR T cell therapy. For instance, Fraietta et al. performed transcriptomic sequencing and functional assessment of CAR T cells from 41 patients with chronic lymphocytic leukemia (CLL). They identified that CAR T cells in responders possessed memory-like characteristics, while CAR T cells in non-responders were in a highly exhaustion state (Fraietta et al., 2018). Consistent with this, other clinical studies on B-ALL and B-cell lymphoma also indicated that CAR T cell exhaustion was related to the treatment failure (Schuster et al., 2017; Finney et al., 2019; Deng et al., 2020). Therefore, the explorations focused on the mechanisms of CAR T cell exhaustion may provide potential insights on preventing CAR T cell exhaustion and improving CAR T cell efficacy.
In this article, we reviewed the underlying regulatory factors of CAR T cell exhaustion that existed in design, generation and infusion process. We also discussed the current strategies to combat CAR T cell exhaustion including blocking immune checkpoint, resisting the tumor microenvironment (TME), and regulating epigenetic and transcriptomic profiles.
CAR design affects CAR T cell exhaustion
The second generation of CARs approved by the US Food and Drug Administration contained an antigen binding domain, a hinge region, a transmembrane domain, an intracellular co-stimulatory domain and a signal transduction domain (Zhao et al., 2018). CAR molecules were activated upon stimulation by an antigen, which triggered the effector response (Salter et al., 2021). Nevertheless, undesirable CAR design would lead to suboptimal T cell activation and ligand-independent tonic signaling (Jayaraman et al., 2020). Long and colleagues described that GD2, CD22, or ErbB2 CAR T cells contained a CD28-CD3ζ intracellular domain tended to be exhausted during early expansion in vitro, but CD19.28z CAR T cells did not. This was due to the tonic CAR signaling induced by aggregation of single-chain variable fragments (scFv) on the surface (Figure 1). The co-stimulatory domain was also involved in tonic CAR signaling, with CD28 co-stimulation enhancing while 4-1BB co-stimulation reducing the tonic CAR signaling and exhaustion (Long et al., 2015). Intriguingly, it was proved that introduction of null mutations in the CD28 domain would down-regulate exhaustion-related genes and improve the anti-tumor function of CD19 CAR T cells in vitro and in vivo (Boucher et al., 2021). Similarly, ICOS co-stimulation also had an advantage over CD28 co-stimulation in averting exhaustion. Indeed, CD28 co-stimulation and ICOS co-stimulation had a shared motif but differed only in a single amino acid residue. Guedan et al. observed that this single amino acid residue asparagine in CD28 was related to the exhaustion of CAR T cells, and replacing asparagine with phenylalanine reduced CAR T cell differentiation and exhaustion in xenograft tumor models (Guedan et al., 2020). Moreover, Nunoya and colleagues evaluated the feature of CAR T cells with different co-stimulatory domain in vitro, and they identified that the co-stimulatory domain derived from herpes virus entry mediator (HVEM) was favorable for a low-level of exhaustion (Nunoya et al., 2019). More recently, a report using CAR pooling to evaluate the propensity to resist exhaustion of CAR T cells during repetitive antigen stimulation. It was revealed that B cell-activating factor receptor (BAFF-R) and transmembrane activator and CAML interactor (TACI) delayed the exhaustion (Goodman et al., 2022). In addition to the co-stimulatory domain, signaling of the intracellular domain of CD3ζ also affected CAR T cell exhaustion. Using the xenograft model, Feucht et al. showed CD19 CAR T cells retaining only a single membrane-proximal immunoreceptor tyrosine-based activation motif (ITAM) had lower exhaustion levels and stronger persistence compared with conventional CAR T cells that had 3 ITAMs (Feucht et al., 2019).
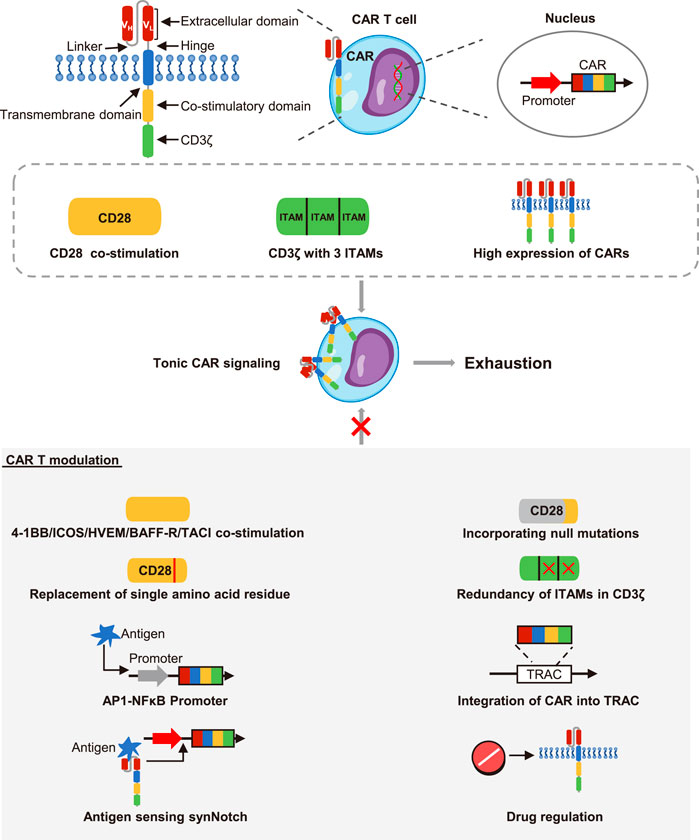
FIGURE 1. The effects of CARs structure on CAR T cell exhaustion. The CARs contained an extracellular domain, a hinge region, a transmembrane domain, a co-stimulatory domain and a CD3ζ stimulatory domain. The extracellular domain was composed of the variable heavy chain (VH) and the variable light chain (VL) connected by a linker. The upstream promoter initiated the strong expression of CARs on the cell surface. However, the CD3ζ with 3 immunoreceptor tyrosine-based activation motifs (ITAMs), CD28 co-stimulation and constitutively high expression of CARs could cause tonic CAR signaling and promote cell exhaustion. However, the 4-1BB, ICOS, HVEM, BAFF-R, and TACI co-stimulation reduced the exhaustion of CAR T cells. Both incorporation of null mutations and replacement of single amino acid residue in CD28 co-stimulation could inhibit exhaustion. Redundancy of ITAMs could also inhibit exhaustion. Besides, the synthetic activator protein 1-nuclear factor κB (AP1-NF-κB) promoter, the T cell receptor alpha constant locus (TRAC) that contained CAR structure, the synNotch-CAR circuits, and drug regulation could all restrain the CARs expression. Then the ligand-independent tonic signaling and exhaustion were thus reduced.
Another important factor to consider when designing CAR T cells was the expression level of the CARs. In fact, the expression of T cell receptors (TCRs) was strictly controlled by the CD45 molecules and co-inhibitory receptors after initiating activation, but CARs typically contained a strong constitutive promoter to ensure their long-term and stable expression in T cells (Harris and Kranz, 2016; Wu et al., 2020). However, it had been recently reported that high expression of CAR molecules acted as a critical inducer of tonic CAR signaling and exhaustion (Figure 1). To disrupt constitutively expressed CARs, Webster and colleagues constructed the synthetic activator protein 1 (AP1)-nuclear factor κB (NFκB) promoter, which reduced the CAR expression at rest and drove the CAR expression under antigen stimulation. Consequently, AP1-NFκB promoted CAR T cells showed limited ligand-independent tonic signaling and exhaustion in preclinical models (Webster et al., 2021). In another attempt, Eyquem et al. designed CAR T cells through integrating the CD19 CAR into the T cell receptor alpha constant locus. These CAR T cells enabled CAR molecules to be low expressed but dynamically regulated under tumor antigen stimulation, thereby they could control tonic CAR signaling in the absence of antigen and avoid exhaustion in vitro (Eyquem et al., 2017). Recently, T cells with synthetic Notch (synNotch) receptors were developed to improve the specificity of CAR T cells. These CAR T cells with synNotch-CAR circuits activated the CAR expression only when they recognized the synNotch antigen (Srivastava et al., 2019). Impressively, this structure was showed to effectively prevent tonic signaling and enable CAR T cells to maintain memory phenotype and resist exhaustion in mouse models (Hyrenius-Wittsten et al., 2021). Besides, Weber et al. demonstrated that incorporating an FK506 binding protein 12 destabilizing domain into GD2 CAR structure would make the expression of CAR subject to the drug shield-1, this drug-regulatable system reversed the exhausted state of CAR T cells in vitro and in preclinical models. They also described that dasatinib which had been reported to reversibly inhibit TCR and CAR signaling had similar effects in reversing CAR T cells exhaustion (Weber et al., 2021). This study provided an extremely promising example for modulating the activation of CAR T cells to control their efficacy and exhaustion in clinical practice. The pharmacologic on/off switch may become a viable approach to steer the functional state of CAR T cells in vivo. Of note, Huang et al. initiated a clinical research (NCT04603872) to investigate the combination effects of dasatinib on in vitro manufacturing and in vivo infusion of CAR T cells.
Overall, the structure of CAR T cells was closely related to their functional characteristics. The component elements including scFv molecules, costimulatory domains and intracellular signaling domains all had complex regulatory effects on the activation and exhaustion. Optimizing the structure and regulating the expression pattern of CARs were feasible to weaken the tonic CAR signaling and prevent the exhaustion.
The in vitro expansion condition affects CAR T cell exhaustion
In order to obtain sufficient CAR T cell numbers, CAR T cells needed to be expanded in vitro before infused into the patient. There was a growing body of evidence that the culture system also affected the exhaustion of CAR T cells (Figure 2). The cytokine IL2, which was commonly added to the expansion system would cause CAR T cell exhaustion and reduce their in vivo persistence. However, CAR T cells expanded under cytokine IL15 or IL2 plus IL4 and TGF-β maintained a less differentiated phenotype. They exhibited reduced expression of inhibitory receptors and strengthened antitumor efficacy in vitro and in vivo (Gattinoni et al., 2005; Alizadeh et al., 2019; Liu et al., 2020). Consistently, Giuffrida et al. described that IL15 promoted the production of central memory T cells and up-regulated memory-related genes including TCF7. The persistence and response to adjuvant anti-PD1 therapy were thus significantly enhanced in preclinical models (Giuffrida et al., 2020). Similarly, addition of other cytokines such as IL4, IL7 and IL21 also supported the stemness of CAR T cells and restrained the exhaustion (Ptáčková et al., 2018). Based on these, the clinical research to ascertain the efficacy of IL15 plus IL7 and IL15 plus IL21 were initiated (NCT04186520, NCT04715191). Moreover, it was recently reported that the exhaustion level of CAR T cells increased with the prolongation of expansion time in vitro. The “younger” cells with shorter culture time manifested lowered exhaustion markers and enhanced long-term killing function in vivo (Caruso et al., 2019). In summary, the in vitro expansion condition greatly impacted the differentiation and exhaustion transition of CAR T cells. It was extremely important to modulate the cytokines and expansion time to obtain CAR T cells with optimal phenotypes and healthy states.
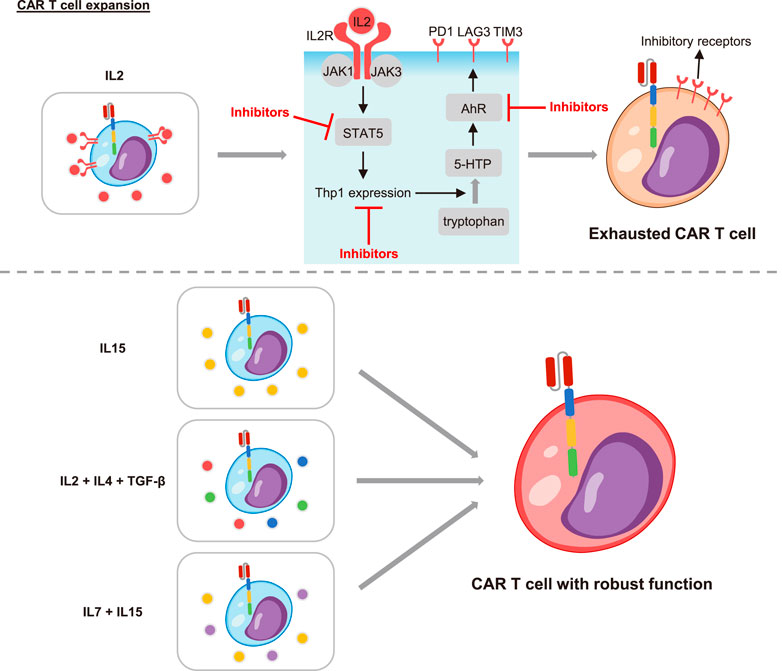
FIGURE 2. The effects of cytokines on CAR T cell exhaustion. The cytokine IL2 promoted CAR T cell exhaustion through the IL2 receptor (IL2R). The combination of IL2 and IL2R led to the recruitment and activation of the Janus family of tyrosine kinases (JAK1 and JAK3). Then the signal transducer and activator of transcription 5 (STAT5) was activated and further induced the expression of tryptophan hydroxylase 1 (Tph1). The Thp1 catalyzed tryptophan to produce 5-hydroxytryptophan (5-HTP), which activated the aryl hydrocarbon receptor (AhR) and led to T cell exhaustion. Inhibitors of STAT5, Thp1 or AhR could all restrain T cell exhaustion. Importantly, the cytokine IL15, IL2 supplemented with IL4/TGF-β, and IL15/IL7 improved the exhaustion. CAR T cells under these culture conditions manifested enhanced persistence and function.
The TME promotes CAR T cell exhaustion
Recently, it was reported that the TME promoted by malignant cells played an important role in tumor development and immune regulation (DeBerardinis, 2020). The hallmarks of the TME including diverse cells such as tumor cells, immune cells and stromal cells, as well as soluble factors such as cytokines, metabolites and extracellular vesicles (EVs) (Anderson and Simon, 2020). These complex components exerted intricate regulatory effects on CAR T cells (Figure 3).
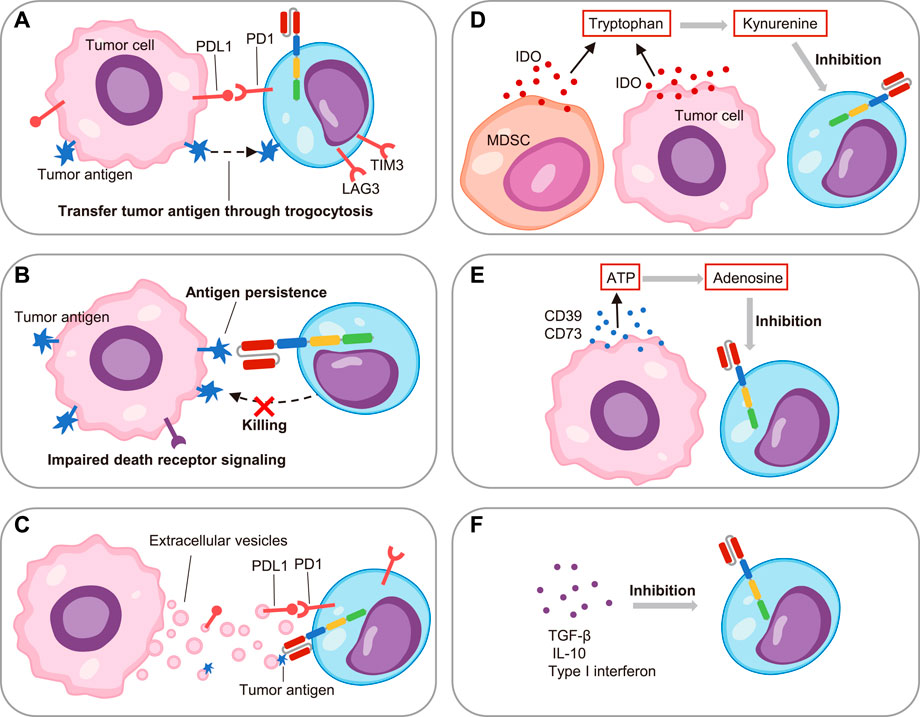
FIGURE 3. The factors promoting CAR T cell exhaustion in the tumor microenvironment.(A) On one hand, the PDL1 on tumor cells induced CAR T cell exhaustion through PD1/PDL1 pathway; on the other hand, the tumor antigen transferred to CAR T cells through trogocytosis and caused exhaustion. (B) The impaired death receptor signaling of leukemia cells led to their resistance to CAR T cells, and the persistent antigen in turn led to CAR T cell exhaustion. (C) The extracellular vesicles secreted by leukemia cells induced CAR T cell exhaustion through PDL1 and tumor antigen. (D) The myeloid-derived suppressor cells (MDSC) and leukemia cells secreted indoleamine 2,3-dioxygenase (IDO), which catalyzed tryptophan to kynurenine to suppress CAR T cell function. (E) The CD39 and CD73 released by tumor cells hydrolyzed ATP to adenosine, which inhibited CAR T cell activity. (F) Soluble factors including TGF-β, IL-10 and type I interferon restrained CAR T cell activity.
As the pivot, tumor cells induced CAR T cell exhaustion versatilely. For example, the multiple immune checkpoint ligands such as PDL1 on tumor cells interacted with their corresponding receptors on CAR T cells and resulted in cell exhaustion (Wang et al., 2019). Additionally, it was reported that the targeted antigen on the ALL cells would be transferred to CAR T cells through trogocytosis in xenograft mouse models, which promoted the expression of PD1, LAG3 and TIM3. Intriguingly, this phenomenon also caused the fratricide of CAR T cells (Hamieh et al., 2019). More recently, Singh et al. showed the impaired death receptor signaling in ALL cells caused the resistance to cytotoxicity of T cells, and thus the persistence of antigens induced functional impairment of CAR T cells in turn. Impressively, they observed that the inherent dysregulation of death receptor signaling in ALL cells directly contributed to CAR T cell exhaustion and correlated with clinical outcomes (Singh et al., 2020). Although CD19 CAR T cells were applied to treat CLL, B-ALL, and B-cell lymphoma, they were less effective in CLL. An explanation for this discrepancy was that the imbalance between the suppressive TME and immune cells (Yoon et al., 2018). Jitschin and colleagues observed that the myeloid-derived suppressor cells (MDSC) were significantly increased in the peripheral blood of CLL patients. On one hand, this MDSC subset promoted the regulatory T cells (Treg). On the other hand, MDSC secreted high levels of indoleamine 2, 3-dioxygenase (IDO) to metabolize tryptophan to limit T cell proliferation and function (Jitschin et al., 2014). All in one, these findings highlighted the significance of the immunosuppressive cell components for inducing CAR T cell exhaustion.
As for immunosuppressive soluble factors in the TME, EVs have attracted the most attention. Cox et al. identified that the number of EVs in patients with CLL was significantly higher than the number in normal individuals, and the same was true for PDL1 positive EVs. They further verified that PDL1 positive EVs induced CD19 CAR T cell exhaustion and attenuated effector function in vitro and in vivo (Cox et al., 2021). Our recent studies also demonstrated that EVs secreted by tumor cells such as B-ALL could induce CD19 CAR T cell exhaustion. Mechanistically, we found that B-ALL cells secreted EVs specifically carrying CD19 antigen, and thus their persistent antigenic stimulation led to exhaustion of CD19 CAR T cells (Zhu et al., 2022). In addition to EVs, tumor cells also secreted masses of metabolites to tame the TME, and consequently to regulate immunity. For instance, the AML cells in bone marrow and B-cell lymphoma cells released IDO to degrade tryptophan, resulting in local nutrient depletion while producing metabolites such as kynurenine to inhibit CAR T cell activity (Teague and Kline, 2013; Ninomiya et al., 2015). Recently, Mussai and colleagues described the AML blast in patients generated Arginase II, which consumed the arginine and led to low-arginine microenvironment. It was proved that the low-arginine conditions would cause the exhaustion and defective proliferation of CAR T cells in vitro (Mussai et al., 2019). Similarly, tumor cells produced ectoenzymes CD39 and CD73, which would hydrolyze ATP to generate adenosine. The adenosine further bound to adenosine 2A receptors on T cells to inhibit their function (Leone et al., 2015; Beavis et al., 2017). In addition, other soluble factors such as transforming growth factor-β (TGF-β), IL-10 and type I interferon were also important regulators of CAR T cell function in the TME (Chang et al., 2018; Stüber et al., 2019). Overall, a great deal of efforts had been devoted to the immune regulation of TME. However, the TME was extremely complex, new drivers of CAR T cell exhaustion were constantly emerging.
Strategies for combating CAR T cell exhaustion
Immune checkpoint blockade
An important hallmark of CAR T cell exhaustion was the up-regulation of multiple inhibitory receptors such as PD1, CTLA4, and LAG3. Their binding to corresponding ligands on tumor cells promoted the dysfunction of exhausted T cells and led to tumor immune escape. Cherkassky et al. proved that PD1-mediated cell exhaustion significantly inhibited CAR T cell function. Blocking the PD1-PDL1 pathway by PD1 inhibitors or endogenous blockade of PD1 expression could resist exhaustion and enhance function in vitro and in preclinical animal models (Cherkassky et al., 2016). Therefore, PD1 and CTLA4 inhibitors aimed at disrupting the inhibitory receptor pathway had gradually been applied for improving tumor immunity in clinical practice (Kon and Benhar, 2019; Liu, 2019). Cao et al. evaluated the efficacy of combination of CD19 CAR T cells with anti-PD1 antibody (nivolumab) in patients with refractory/relapsed B-cell non-Hodgkin lymphoma (Table 1). Encouragingly, they observed that complete response could be achieved in 45.45% of patients (Cao et al., 2019). Similarly, one recent study reported that the PD1 inhibitor, pembrolizumab, achieved complete remission or partial remission in 25% of patients with B-cell lymphoma refractory to and/or relapsed after CD19 CAR T cells treatment. Immune analysis revealed the patients who achieved remission had mild CAR T cell exhaustion, which indicating that PD1 inhibitors had certain benefits in improving exhaustion and enhancing the efficacy of CD19 CAR T cells (Chong et al., 2022). Meanwhile, administration of CAR T cells with nivolumab and ipilimumab (CTLA-4 inhibitor) was also being tested in clinical trials (NCT04003649).
In addition to PD1 and CTLA4 inhibitors, modifying CAR T cells to block immune checkpoint pathways had also been investigated intensively. For example, Pan and his colleagues introduced a fusion protein consisting of the extracellular domain of PD1 and CH3 from IgG4 into GPC3 CAR T cells to construct CAR T cells secreting a soluble PD1 protein. They found these CAR T cells were protected from exhaustion when stimulated by target cells in vitro and in murine xenogeneic models (Pan et al., 2018). Furthermore, Zou et al. demonstrated simultaneously downregulating three checkpoint receptors PD1, TIM3, and LAG3 could improve the exhaustion of CAR T cells through upregulation of CD56 in xenogenic mouse models (Zou et al., 2019). In line with this, a recent study described that simultaneous down-regulation of PD1 and TIGIT exerted a synergistic anti-tumor effect, the down-regulation of TIGIT was mainly responsible for maintaining the low-differentiation and low-exhaustion state of CAR T cells, while the down-regulation of PD1 enhanced the short-term cytotoxicity of CAR T cells in vitro functional assays (Lee et al., 2022). In summary, immune checkpoint blockade had achieved certain successes in preclinical and clinical practice, which was also the mainstream method for resisting CAR T cell exhaustion currently.
Combating the TME
With the clarification of the complex role of TME, new strategies targeting TME to improve the efficacy of CAR T cells had proliferated recently (Table 1). In terms of the excessive adenosine in the TME, the adenosine 2A receptor on CAR T cells was responsible for mediating its immunosuppressive effects. Accordingly, it was reported that using the adenosine 2A receptor antagonists and knockout of receptors in CAR T cells improved exhaustion and function in preclinical mouse models (Beavis et al., 2017; Li et al., 2020). Besides, to confront adenosine, immune cells could also catabolize it into inosine through adenosine deaminase 1 (ADA). Therefore, Qu et al. engineered ADA-overexpressing CAR T cells and proved that they had an enhanced ability to resist exhaustion in vitro and in vivo (Qu et al., 2022).
As for the arginine, it was recognized that endogenous production of arginine was mainly dependent on argininosuccinate synthetase (ASS) enzymes and ornithine transcarbamylase (OTC). However, the expression of ASS and OTC in T cells was low, so they were dependent on exogenous arginine and were sensitive to low-arginine microenvironment. Encouragingly, inhibiting the arginine metabolism or engineering CAR T cells to express functional ASS and OTC could both significantly enhance the function (Mussai et al., 2019; Fultang et al., 2020).
To overcome the excess TGF-β in the TME, Kloss et al. blocked TGF-β signaling by co-expressing a dominant-negative TGF-β receptor II (TGF-βRII) in PSMA CAR T cells targeting prostate cancer. Tang et al. used CRISPR/Cas9 technology to knockout endogenous TGF-βRII in CAR T cells. Both these modifications prevented CAR T cell exhaustion and enhanced anti-tumor effects in tumor models (Kloss et al., 2018; Tang et al., 2020). And there were abundant agents that were being tested in clinical trials including TGF-β targeted neutralizing antibodies, vaccines, antisense oligonucleotides, and small molecule inhibitors. Of particular interest, a mass of research devoted to developing agents that dual-targeting TGF-β and PDL1 molecules. Especially the bintrafusp alfa, a bifunctional fusion protein targeting TGF-β and PD-L1 (Gulley et al., 2022). More recently, Chen and his team designed CAR T cells with a bispecific trap protein structure that secreted trap proteins, which could simultaneously target TGF-β and PD1. Such CAR T cells significantly attenuated suppressive T cell signaling and resisted exhaustion in vitro and in vivo (Chen et al., 2021).
Furthermore, in order to clear the ability of different types of CAR T cells to resist exhaustion in the TME, one study used CD4 or CD8-targeted lentivirus to generate CD4 CAR T cells or CD8 CAR T cells in vivo, respectively. It was showed that the anti-tumor efficacy of CD4 CAR T cells alone was better than that of CD8 CAR T cells alone or mixture of CD4 and CD8 CAR T cells, because CD8 CAR T cells were more prone to exhaustion under high tumor burden. This report suggested that the phenotype could be adjusted to obtain CAR T cells that were resistant to exhaustion in vivo (Agarwal et al., 2020). In addition, promoting the secretion of cytokines could also resist CAR T cell exhaustion caused by the TME. For example, co-expression of IL7 gene enabled CAR T cells to produce IL7 under antigen stimulation, which promoted CAR T cell proliferation and inhibited CAR T cell exhaustion and apoptosis. These CAR T cells exhibited a less differentiated phenotype with a higher proportion of central memory T cells in preclinical models (He et al., 2020). Therefore, it was feasible to engineer CAR T cells that directly counter the immune suppressors in the TME to resist exhaustion. However, the clinical efficacy and safety of these CAR T cells remained to be further observed.
Epigenetic regulation of CAR T cell exhaustion
It had been established that exhausted T cells had completely different epigenetic characteristics from effector T cells and memory T cells. For instance, exhausted T cells lacked several open chromatin regions presented in the IFNG locus in effector T cells and memory T cells. Because blocking PD1 was unable to fully remodel the epigenetics of exhausted T cells, T cells would be re-exhausted when the antigen persisted (Pauken et al., 2016). Subsequently, Ghoneim et al. performed whole-genome sequencing of effector T cells and exhausted T cells, they identified that the acquired de novo methylation programs limited T cell proliferation and clonal diversity during transition to exhaustion (Ghoneim et al., 2017). In line with this, Zebley and colleagues observed CAR T cells acquired exhaustion-related methylation programs after infusion in patients with relapsed/refractory B-ALL, the genes associated with T cell memory maintenance including TCF and LEF1 were suppressed (Zebley et al., 2021). Consequently, restraining these epigenetic modifications had the potential to reverse exhaustion transition (Table 1). In a recent work, it was described that blocking de novo methylation programs through de novo DNA methyltransferase 3α (DNMT3A) knockout could overcome CAR T cell exhaustion in vitro and in preclinical solid tumor models (Prinzing et al., 2021). Similarly, DNA methyltransferase inhibitor, decitabine, had also been proved to reverse exhaustion-associated DNA-methylation programs. Two research teams successively demonstrated that decitabine enhanced the antitumor activity of CAR T cells both in vitro and in vivo. Through transcriptome and epigenetic sequencing, it was revealed that memory-related genes and immune synapse genes were promoted while exhaustion-related genes were suppressed (You et al., 2020; Wang Y. et al., 2021b). In addition, clinical trials of CD19 CAR T cells in relapsed/refractory CLL (NCT01029366 and NCT01747486) showed the CAR silencing was associated with CAR T treatment failure, this was caused by abnormal methylation programs of the CAR promoter. Kong and colleagues presented that epigenetic modulation by bromodomain and extra-terminal (BET) family protein blockade could reverse CAR silencing and improve CAR T cell exhaustion in vitro (Kong et al., 2021). Overall, these clinical and preclinical data provided evidence for the epigenetic involvement in the regulation of CAR T cell exhaustion and efficacy. In particular, the combination of decitabine with CAR T cell therapy was currently being tested in clinical trials (NCT04697940, NCT04850560 and NCT04553393). Thus, epigenetic regulation had the potential to become an important approach to reverse CAR T cell exhaustion in the future.
Transcriptomic regulation of CAR T cell exhaustion
Multiple genes and transcription factors were involved in the regulatory network of CAR T cell exhaustion. One of the most important was the transcription factor TCF1, it was a key regulator of T cell differentiation. TCF1-positive CAR T cells exhibited a less exhaustion level and enhanced persistence in vitro and in vivo (Wu et al., 2016; Zheng et al., 2021). The other key transcription factors were the nuclear factor of activated T cells (NFAT) family and NR4A nuclear receptor family (NR4A1, NR4A2 and NR4A3). They played an important role in the immune homeostasis, including maintaining the development of regulatory T cells, regulating the activation of T cells, controlling the survival of B cells and T cells when encountered antigen (Macian, 2005; Sekiya et al., 2013; Hiwa et al., 2022). However, their strong activity and redundant effects were thought to be related to T cell exhaustion. It was reported that the NFAT/NR4A axis cooperatively controlled the expression of inhibitory receptors including PD1, TIM3 and LAG3. Deleting all three NR4A transcription factors could enhance the anti-tumor effect in solid tumor models (Table 1) (Chen et al., 2019). And meanwhile, Seo and colleagues observed that the transcription factors TOX and TOX2 were also downstream targets of NFAT to prompt CAR T cell exhaustion. Disruption the expression and activity of TOX and TOX2 in CAR T cells promoted tumor regression in tumor-bearing mouse models (Seo et al., 2019). Another NFAT partner, transcription factor AP1, was also involved in the regulation of CAR T cell exhaustion. Lynn et al. described that there was an epigenetic dysregulation of AP1 in exhausted CAR T cells using an exhaustion cell model with tonic CAR signaling, they proposed that this may be associated with the immunoregulatory AP-1/IRF transcriptional complexes (Lynn et al., 2019). In addition, some genes that may regulate CAR T cell exhaustion had been gradually uncovered through genetic screening. For example, one study identified that MAP4K1 gene (encoding HPK1) was highly correlated with the expression of inhibitory receptors in a variety of cancer through database analysis, the HPK1-NFκB-Blimp1 axis may mediate CAR T cell exhaustion. (Si et al., 2020). More recently, Wang et al. screened the whole genome of glioblastoma stem cells and CAR T cells to obtain the key molecules that determine the cytotoxicity of CAR T cells. They found that genes TLE2 and IKZF2 had a suppressive effect on CAR T cells. Intervention to down-regulate the expression of these two genes could inhibit CAR T cell exhaustion and enhance function in glioblastoma mouse models (Wang D. et al., 2021a). Therefore, with the clarity of genetic changes, the intricate regulation network behind CAR T cell exhaustion gradually emerged. Although the intervention of these transcription factors and genes was still confined to in vitro and preclinical stage currently, these regulators provided attractive drug targets for inhibiting CAR T cell exhaustion and improving immunotherapy response. Interventions against these key factors were expected to enter clinical applications soon.
Conclusion
The mechanisms of CAR T cell exhaustion are extraordinarily complex and need to be explored in depth. First, inappropriate CAR T cell structure could induce ligand-independent tonic signaling and thus leading to CAR T cell exhaustion. Then, the cytokines and the duration of in vitro expansion also affect CAR T cell exhaustion. More importantly, there are abundant immunosuppressive factors in the TME. Hence, these multifaceted attacks make it difficult to reverse CAR T cell exhaustion. At present, PD1 and CTLA4 inhibitors are the dominant agents to combat CAR T cell exhaustion in clinical practice. However, the experience using immune checkpoint inhibitors with CAR T cells is limited. Besides, exhausted CAR T cells have epigenetic remodeling as well as transcriptomic abnormalities that increase the difficulty to intervene. Therefore, designing CAR T cells that can resist exhaustion or targeting these exhaustion-inducing factors may offer the potential to improve the efficacy of CAR T cells.
Author contributions
XyZ wrote the first draft of this manuscript. QL and XjZ revised the manuscript. All authors have read and approved the submitted version.
Conflict of interest
The authors declare that the research was conducted in the absence of any commercial or financial relationships that could be construed as a potential conflict of interest.
Publisher’s note
All claims expressed in this article are solely those of the authors and do not necessarily represent those of their affiliated organizations, or those of the publisher, the editors and the reviewers. Any product that may be evaluated in this article, or claim that may be made by its manufacturer, is not guaranteed or endorsed by the publisher.
References
Agarwal, S., Hanauer, J. D. S., Frank, A. M., Riechert, V., Thalheimer, F. B., and Buchholz, C. J. (2020). In vivo generation of CAR T cells selectively in human CD4+ lymphocytes. Mol. Ther. 28, 1783–1794. doi:10.1016/j.ymthe.2020.05.005
Alizadeh, D., Wong, R. A., Yang, X., Wang, D., Pecoraro, J. R., Kuo, C.-F., et al. (2019). IL15 enhances CAR-T cell antitumor activity by reducing mTORC1 activity and preserving their stem cell memory phenotype. Cancer Immunol. Res. 7, 759–772. doi:10.1158/2326-6066.CIR-18-0466
Anderson, N. M., and Simon, M. C. (2020). The tumor microenvironment. Curr. Biol. 30, R921–R925. doi:10.1016/j.cub.2020.06.081
Beavis, P. A., Henderson, M. A., Giuffrida, L., Mills, J. K., Sek, K., Cross, R. S., et al. (2017). Targeting the adenosine 2A receptor enhances chimeric antigen receptor T cell efficacy. J. Clin. Invest. 127, 929–941. doi:10.1172/JCI89455
Boucher, J. C., Li, G., Kotani, H., Cabral, M. L., Morrissey, D., Lee, S. B., et al. (2021). CD28 costimulatory domain-targeted mutations enhance chimeric antigen receptor T-cell function. Cancer Immunol. Res. 9, 62–74. doi:10.1158/2326-6066.CIR-20-0253
Cao, Y., Lu, W., Sun, R., Jin, X., Cheng, L., He, X., et al. (2019). Anti-CD19 chimeric antigen receptor T cells in combination with nivolumab are safe and effective against relapsed/refractory B-cell non-hodgkin lymphoma. Front. Oncol. 9, 767. doi:10.3389/fonc.2019.00767
Caruso, H. G., Tanaka, R., Liang, J., Ling, X., Sabbagh, A., Henry, V. K., et al. (2019). Shortened ex vivo manufacturing time of EGFRvIII-specific chimeric antigen receptor (CAR) T cells reduces immune exhaustion and enhances antiglioma therapeutic function. J. Neurooncol. 145, 429–439. doi:10.1007/s11060-019-03311-y
Chang, Z. L., Lorenzini, M. H., Chen, X., Tran, U., Bangayan, N. J., and Chen, Y. Y. (2018). Rewiring T-cell responses to soluble factors with chimeric antigen receptors. Nat. Chem. Biol. 14, 317–324. doi:10.1038/nchembio.2565
Chen, J., López-Moyado, I. F., Seo, H., Lio, C. J., Hempleman, L. J., Sekiya, T., et al. (2019). NR4A transcription factors limit CAR T cell function in solid tumours. Nature 567, 530–534. doi:10.1038/s41586-019-0985-x
Chen, X., Yang, S., Li, S., Qu, Y., Wang, H.-Y., Liu, J., et al. (2021). Secretion of bispecific protein of anti-PD-1 fused with TGF-β trap enhances antitumor efficacy of CAR-T cell therapy. Mol. Ther. Oncolytics 21, 144–157. doi:10.1016/j.omto.2021.03.014
Cherkassky, L., Morello, A., Villena-Vargas, J., Feng, Y., Dimitrov, D. S., Jones, D. R., et al. (2016). Human CAR T cells with cell-intrinsic PD-1 checkpoint blockade resist tumor-mediated inhibition. J. Clin. Invest. 126, 3130–3144. doi:10.1172/JCI83092
Chong, E. A., Alanio, C., Svoboda, J., Nasta, S. D., Landsburg, D. J., Lacey, S. F., et al. (2022). Pembrolizumab for B-cell lymphomas relapsing after or refractory to CD19-directed CAR T-cell therapy. Blood 139, 1026–1038. doi:10.1182/blood.2021012634
Cox, M. J., Lucien, F., Sakemura, R., Boysen, J. C., Kim, Y., Horvei, P., et al. (2021). Leukemic extracellular vesicles induce chimeric antigen receptor T cell dysfunction in chronic lymphocytic leukemia. Mol. Ther. 29, 1529–1540. doi:10.1016/j.ymthe.2020.12.033
DeBerardinis, R. J. (2020). Tumor microenvironment, metabolism, and immunotherapy. N. Engl. J. Med. 382, 869–871. doi:10.1056/NEJMcibr1914890
Deng, Q., Han, G., Puebla-Osorio, N., Ma, M. C. J., Strati, P., Chasen, B., et al. (2020). Characteristics of anti-CD19 CAR T cell infusion products associated with efficacy and toxicity in patients with large B cell lymphomas. Nat. Med. 26, 1878–1887. doi:10.1038/s41591-020-1061-7
Doering, T. A., Crawford, A., Angelosanto, J. M., Paley, M. A., Ziegler, C. G., and Wherry, E. J. (2012). Network analysis reveals centrally connected genes and pathways involved in CD8+ T cell exhaustion versus memory. Immunity 37, 1130–1144. doi:10.1016/j.immuni.2012.08.021
Eyquem, J., Mansilla-Soto, J., Giavridis, T., van der Stegen, S. J., Hamieh, M., Cunanan, K. M., et al. (2017). Targeting a CAR to the TRAC locus with CRISPR/Cas9 enhances tumour rejection. Nature 543, 113–117. doi:10.1038/nature21405
Feucht, J., Sun, J., Eyquem, J., Ho, Y.-J., Zhao, Z., Leibold, J., et al. (2019). Calibration of CAR activation potential directs alternative T cell fates and therapeutic potency. Nat. Med. 25, 82–88. doi:10.1038/s41591-018-0290-5
Finney, O. C., Brakke, H. M., Rawlings-Rhea, S., Hicks, R., Doolittle, D., Lopez, M., et al. (2019). CD19 CAR T cell product and disease attributes predict leukemia remission durability. J. Clin. Invest. 129, 2123–2132. doi:10.1172/JCI125423
Fraietta, J. A., Lacey, S. F., Orlando, E. J., Pruteanu-Malinici, I., Gohil, M., Lundh, S., et al. (2018). Determinants of response and resistance to CD19 chimeric antigen receptor (CAR) T cell therapy of chronic lymphocytic leukemia. Nat. Med. 24, 563–571. doi:10.1038/s41591-018-0010-1
Fultang, L., Booth, S., Yogev, O., Martins da Costa, B., Tubb, V., Panetti, S., et al. (2020). Metabolic engineering against the arginine microenvironment enhances CAR-T cell proliferation and therapeutic activity. Blood 136, 1155–1160. doi:10.1182/blood.2019004500
Gallimore, A., Glithero, A., Godkin, A., Tissot, A. C., Plückthun, A., Elliott, T., et al. (1998). Induction and exhaustion of lymphocytic choriomeningitis virus–specific cytotoxic T lymphocytes visualized using soluble tetrameric major histocompatibility complex class I–peptide complexes. J. Exp. Med. 187, 1383–1393. doi:10.1084/jem.187.9.1383
Gattinoni, L., Klebanoff, C. A., Palmer, D. C., Wrzesinski, C., Kerstann, K., Yu, Z., et al. (2005). Acquisition of full effector function in vitro paradoxically impairs the in vivo antitumor efficacy of adoptively transferred CD8+ T cells. J. Clin. Invest. 115, 1616–1626. doi:10.1172/JCI24480
Ghoneim, H. E., Fan, Y., Moustaki, A., Abdelsamed, H. A., Dash, P., Dogra, P., et al. (2017). De novo epigenetic programs inhibit PD-1 blockade-mediated T cell rejuvenation. Cell 170, 142–157. e19. doi:10.1016/j.cell.2017.06.007
Giuffrida, L., Sek, K., Henderson, M. A., House, I. G., Lai, J., Chen, A. X. Y., et al. (2020). IL-15 preconditioning augments CAR T cell responses to checkpoint blockade for improved treatment of solid tumors. Mol. Ther. 28, 2379–2393. doi:10.1016/j.ymthe.2020.07.018
Goodman, D. B., Azimi, C. S., Kearns, K., Talbot, A., Garakani, K., Garcia, J., et al. (2022). Pooled screening of CAR T cells identifies diverse immune signaling domains for next-generation immunotherapies. Sci. Transl. Med. 14, eabm1463. doi:10.1126/scitranslmed.abm1463
Guedan, S., Madar, A., Casado-Medrano, V., Shaw, C., Wing, A., Liu, F., et al. (2020). Single residue in CD28-costimulated CAR-T cells limits long-term persistence and antitumor durability. J. Clin. Invest. 130, 3087–3097. doi:10.1172/JCI133215
Gulley, J. L., Schlom, J., Barcellos-Hoff, M. H., Wang, X.-J., Seoane, J., Audhuy, F., et al. (2022). Dual inhibition of TGF-β and PD-L1: A novel approach to cancer treatment. Mol. Oncol. 16, 2117–2134. doi:10.1002/1878-0261.13146
Hamieh, M., Dobrin, A., Cabriolu, A., van der Stegen, S. J. C., Giavridis, T., Mansilla-Soto, J., et al. (2019). CAR T cell trogocytosis and cooperative killing regulate tumour antigen escape. Nature 568, 112–116. doi:10.1038/s41586-019-1054-1
Harris, D. T., and Kranz, D. M. (2016). Adoptive T cell therapies: A comparison of T cell receptors and chimeric antigen receptors. Trends Pharmacol. Sci. 37, 220–230. doi:10.1016/j.tips.2015.11.004
Hashimoto, M., Kamphorst, A. O., Im, S. J., Kissick, H. T., Pillai, R. N., Ramalingam, S. S., et al. (2018). CD8 T cell exhaustion in chronic infection and cancer: Opportunities for interventions. Annu. Rev. Med. 69, 301–318. doi:10.1146/annurev-med-012017-043208
He, C., Zhou, Y., Li, Z., Farooq, M. A., Ajmal, I., Zhang, H., et al. (2020). Co-expression of IL-7 improves nkg2d-based CAR T cell therapy on prostate cancer by enhancing the expansion and inhibiting the apoptosis and exhaustion. Cancers 12, 1969. doi:10.3390/cancers12071969
Hiwa, R., Brooks, J. F., Mueller, J. L., Nielsen, H. V., and Zikherman, J. (2022). NR4A nuclear receptors in T and B lymphocytes: Gatekeepers of immune tolerance. Immunol. Rev. 307, 116–133. doi:10.1111/imr.13072
Holstein, S. A., and Lunning, M. A. (2019). CAR T-cell therapy in hematologic malignancies: A voyage in progress. Clin. Pharmacol. Ther. 107, 112–122. doi:10.1002/cpt.1674
Hyrenius-Wittsten, A., Su, Y., Park, M., Garcia, J. M., Alavi, J., Perry, N., et al. (2021). SynNotch CAR circuits enhance solid tumor recognition and promote persistent antitumor activity in mouse models. Sci. Transl. Med. 13, eabd8836. doi:10.1126/scitranslmed.abd8836
Jayaraman, J., Mellody, M. P., Hou, A. J., Desai, R. P., Fung, A. W., Pham, A. H. T., et al. (2020). CAR-T design: Elements and their synergistic function. EBioMedicine 58, 102931. doi:10.1016/j.ebiom.2020.102931
Jitschin, R., Braun, M., Büttner, M., Dettmer-Wilde, K., Bricks, J., Berger, J., et al. (2014). CLL-cells induce IDOhi CD14+HLA-DRlo myeloid-derived suppressor cells that inhibit T-cell responses and promote TRegs. Blood 124, 750–760. doi:10.1182/blood-2013-12-546416
Kloss, C. C., Lee, J., Zhang, A., Chen, F., Melenhorst, J. J., Lacey, S. F., et al. (2018). Dominant-negative TGF-β receptor enhances PSMA-targeted human CAR T cell proliferation and augments prostate cancer eradication. Mol. Ther. 26, 1855–1866. doi:10.1016/j.ymthe.2018.05.003
Kon, E., and Benhar, I. (2019). Immune checkpoint inhibitor combinations: Current efforts and important aspects for success. Drug resist. updat. 45, 13–29. doi:10.1016/j.drup.2019.07.004
Kong, W., Dimitri, A., Wang, W., Jung, I.-Y., Ott, C. J., Fasolino, M., et al. (2021). BET bromodomain protein inhibition reverses chimeric antigen receptor extinction and reinvigorates exhausted T cells in chronic lymphocytic leukemia. J. Clin. Invest. 131, e145459. doi:10.1172/JCI145459
Lee, Y.-H., Lee, H. J., Kim, H. C., Lee, Y., Nam, S. K., Hupperetz, C., et al. (2022). PD-1 and TIGIT downregulation distinctly affect the effector and early memory phenotypes of CD19-targeting CAR T cells. Mol. Ther. 30, 579–592. doi:10.1016/j.ymthe.2021.10.004
Leone, R. D., Lo, Y.-C., and Powell, J. D. (2015). A2aR antagonists: Next generation checkpoint blockade for cancer immunotherapy. Comput. Struct. Biotechnol. J. 13, 265–272. doi:10.1016/j.csbj.2015.03.008
Li, N., Tang, N., Cheng, C., Hu, T., Wei, X., Han, W., et al. (2020). Improving the anti-solid tumor efficacy of CAR-T cells by inhibiting adenosine signaling pathway. Oncoimmunology 9, 1824643. doi:10.1080/2162402X.2020.1824643
Liu, D. (2019). CAR-T "the living drugs", immune checkpoint inhibitors, and precision medicine: A new era of cancer therapy. J. Hematol. Oncol. 12, 113. doi:10.1186/s13045-019-0819-1
Liu, L., Bi, E., Ma, X., Xiong, W., Qian, J., Ye, L., et al. (2020). Enhanced CAR-T activity against established tumors by polarizing human T cells to secrete interleukin-9. Nat. Commun. 11, 5902. doi:10.1038/s41467-020-19672-2
Long, A. H., Haso, W. M., Shern, J. F., Wanhainen, K. M., Murgai, M., Ingaramo, M., et al. (2015). 4-1BB costimulation ameliorates T cell exhaustion induced by tonic signaling of chimeric antigen receptors. Nat. Med. 21, 581–590. doi:10.1038/nm.3838
Lynn, R. C., Weber, E. W., Sotillo, E., Gennert, D., Xu, P., Good, Z., et al. (2019). c-Jun overexpression in CAR T cells induces exhaustion resistance. Nature 576, 293–300. doi:10.1038/s41586-019-1805-z
Macian, F. (2005). NFAT proteins: Key regulators of T-cell development and function. Nat. Rev. Immunol. 5, 472–484. doi:10.1038/nri1632
Marofi, F., Motavalli, R., Safonov, V. A., Thangavelu, L., Yumashev, A. V., Alexander, M., et al. (2021). CAR T cells in solid tumors: Challenges and opportunities. Stem Cell Res. Ther. 12, 81. doi:10.1186/s13287-020-02128-1
Mussai, F., Wheat, R., Sarrou, E., Booth, S., Stavrou, V., Fultang, L., et al. (2019). Targeting the arginine metabolic brake enhances immunotherapy for leukaemia. Int. J. Cancer 145, 2201–2208. doi:10.1002/ijc.32028
Ninomiya, S., Narala, N., Huye, L., Yagyu, S., Savoldo, B., Dotti, G., et al. (2015). Tumor indoleamine 2, 3-dioxygenase (Ido) inhibits CD19-CAR T cells and is downregulated by lymphodepleting drugs. Blood 125, 3905–3916. doi:10.1182/blood-2015-01-621474
Nunoya, J.-I., Masuda, M., Ye, C., and Su, L. (2019). Chimeric antigen receptor T cell bearing herpes virus entry mediator Co-stimulatory signal domain exhibits high functional potency. Mol. Ther. Oncolytics 14, 27–37. doi:10.1016/j.omto.2019.03.002
Pan, Z., Di, S., Shi, B., Jiang, H., Shi, Z., Liu, Y., et al. (2018). Increased antitumor activities of glypican-3-specific chimeric antigen receptor-modified T cells by coexpression of a soluble PD1–CH3 fusion protein. Cancer Immunol. Immunother. 67, 1621–1634. doi:10.1007/s00262-018-2221-1
Pauken, K. E., Sammons, M. A., Odorizzi, P. M., Manne, S., Godec, J., Khan, O., et al. (2016). Epigenetic stability of exhausted T cells limits durability of reinvigoration by PD-1 blockade. Science 354, 1160–1165. doi:10.1126/science.aaf2807
Philip, M., Fairchild, L., Sun, L., Horste, E. L., Camara, S., Shakiba, M., et al. (2017). Chromatin states define tumour-specific T cell dysfunction and reprogramming. Nature 545, 452–456. doi:10.1038/nature22367
Prinzing, B., Zebley, C. C., Petersen, C. T., Fan, Y., Anido, A. A., Yi, Z., et al. (2021). Deleting DNMT3A in CAR T cells prevents exhaustion and enhances antitumor activity. Sci. Transl. Med. 13, eabh0272. doi:10.1126/scitranslmed.abh0272
Ptáčková, P., Musil, J., Štach, M., Lesný, P., Němečková, Š., Král, V., et al. (2018). A new approach to CAR T-cell gene engineering and cultivation using piggyBac transposon in the presence of IL-4, IL-7 and IL-21. Cytotherapy 20, 507–520. doi:10.1016/j.jcyt.2017.10.001
Qu, Y., Dunn, Z. S., Chen, X., MacMullan, M., Cinay, G., Wang, H.-Y., et al. (2022). Adenosine deaminase 1 overexpression enhances the antitumor efficacy of chimeric antigen receptor-engineered T cells. Hum. Gene Ther. 33, 223–236. doi:10.1089/hum.2021.050
Salter, A. I., Rajan, A., Kennedy, J. J., Ivey, R. G., Shelby, S. A., Leung, I., et al. (2021). Phosphoproteomic analysis of chimeric antigen receptor signaling reveals kinetic and quantitative differences that affect cell function. Sci. Signal. 14, eaat6753. doi:10.1126/scisignal.aat6753
Schietinger, A., and Greenberg, P. D. (2014). Tolerance and exhaustion: Defining mechanisms of T cell dysfunction. Trends Immunol. 35, 51–60. doi:10.1016/j.it.2013.10.001
Schuster, S. J., Svoboda, J., Chong, E. A., Nasta, S. D., Mato, A. R., Anak, Ö., et al. (2017). Chimeric antigen receptor T cells in refractory B-cell lymphomas. N. Engl. J. Med. 377, 2545–2554. doi:10.1056/NEJMoa1708566
Sekiya, T., Kashiwagi, I., Yoshida, R., Fukaya, T., Morita, R., Kimura, A., et al. (2013). Nr4a receptors are essential for thymic regulatory T cell development and immune homeostasis. Nat. Immunol. 14, 230–237. doi:10.1038/ni.2520
Seo, H., Chen, J., González-Avalos, E., Samaniego-Castruita, D., Das, A., Wang, Y. H., et al. (2019). TOX and TOX2 transcription factors cooperate with NR4A transcription factors to impose CD8+ T cell exhaustion. Proc. Natl. Acad. Sci. U. S. A. 116, 12410–12415. doi:10.1073/pnas.1905675116
Si, J., Shi, X., Sun, S., Zou, B., Li, Y., An, D., et al. (2020). Hematopoietic progenitor Kinase1 (HPK1) mediates T cell dysfunction and is a druggable target for T cell-based immunotherapies. Cancer Cell 38, 551–566. e11. doi:10.1016/j.ccell.2020.08.001
Singh, N., Lee, Y. G., Shestova, O., Ravikumar, P., Hayer, K. E., Hong, S. J., et al. (2020). Impaired death receptor signaling in leukemia causes antigen-independent resistance by inducing CAR T-cell dysfunction. Cancer Discov. 10, 552–567. doi:10.1158/2159-8290.CD-19-0813
Srivastava, S., Salter, A. I., Liggitt, D., Yechan-Gunja, S., Sarvothama, M., Cooper, K., et al. (2019). Logic-gated ROR1 chimeric antigen receptor expression rescues T cell-mediated toxicity to normal tissues and enables selective tumor targeting. Cancer Cell 35, 489–503. doi:10.1016/j.ccell.2019.02.003
Stüber, T., Monjezi, R., Wallstabe, L., Kühnemundt, J., Nietzer, S. L., Dandekar, G., et al. (2019). Inhibition of TGF-β-receptor signaling augments the antitumor function of ROR1-specific CAR T-cells against triple-negative breast cancer. J. Immunother. Cancer 8, e000676. doi:10.1136/jitc-2020-000676
Tang, N., Cheng, C., Zhang, X., Qiao, M., Li, N., Mu, W., et al. (2020). TGF-β inhibition via CRISPR promotes the long-term efficacy of CAR T cells against solid tumors. JCI Insight 5, e133977. doi:10.1172/jci.insight.133977
Teague, R. M., and Kline, J. (2013). Immune evasion in acute myeloid leukemia: Current concepts and future directions. J. Immunother. Cancer 1, 1. doi:10.1186/2051-1426-1-13
Wang, D., Prager, B. C., Gimple, R. C., Aguilar, B., Alizadeh, D., Tang, H., et al. (2021a). CRISPR screening of CAR T cells and cancer stem cells reveals critical dependencies for cell-based therapies. Cancer Discov. 11, 1192–1211. doi:10.1158/2159-8290.Cd-20-1243
Wang, H., Kaur, G., Sankin, A. I., Chen, F., Guan, F., and Zang, X. (2019). Immune checkpoint blockade and CAR-T cell therapy in hematologic malignancies. J. Hematol. Oncol. 12, 59. doi:10.1186/s13045-019-0746-1
Wang, Y., Tong, C., Dai, H., Wu, Z., Han, X., Guo, Y., et al. (2021b). Low-dose decitabine priming endows CAR T cells with enhanced and persistent antitumour potential via epigenetic reprogramming. Nat. Commun. 12, 409. doi:10.1038/s41467-020-20696-x
Weber, E. W., Parker, K. R., Sotillo, E., Lynn, R. C., Anbunathan, H., Lattin, J., et al. (2021). Transient rest restores functionality in exhausted CAR-T cells through epigenetic remodeling. Science 372, eaba1786. doi:10.1126/science.aba1786
Webster, B., Xiong, Y., Hu, P., Wu, D., Alabanza, L., Orentas, R. J., et al. (2021). Self-driving armored CAR-T cells overcome a suppressive milieu and eradicate CD19+ Raji lymphoma in preclinical models. Mol. Ther. 29, 2691–2706. doi:10.1016/j.ymthe.2021.05.006
Wu, L., Wei, Q., Brzostek, J., and Gascoigne, N. R. J. (2020). Signaling from T cell receptors (TCRs) and chimeric antigen receptors (CARs) on T cells. Cell. Mol. Immunol. 17, 600–612. doi:10.1038/s41423-020-0470-3
Wu, T., Ji, Y., Moseman, E. A., Xu, H. C., Manglani, M., Kirby, M., et al. (2016). The TCF1-Bcl6 axis counteracts type I interferon to repress exhaustion and maintain T cell stemness. Sci. Immunol. 1, eaai8593. doi:10.1126/sciimmunol.aai8593
Yoon, D., Osborn, M., Tolar, J., and Kim, C. (2018). Incorporation of immune checkpoint blockade into chimeric antigen receptor T cells (CAR-Ts): Combination or built-in CAR-T. Int. J. Mol. Sci. 19, 340. doi:10.3390/ijms19020340
You, L., Han, Q., Zhu, L., Zhu, Y., Bao, C., Yang, C., et al. (2020). Decitabine-mediated epigenetic reprograming enhances anti-leukemia efficacy of cd123-targeted chimeric antigen receptor T-cells. Front. Immunol. 11, 1787. doi:10.3389/fimmu.2020.01787
Zajac, A. J., Blattman, J. N., Murali-Krishna, K., Sourdive, D. J. D., Suresh, M., Altman, J. D., et al. (1998). Viral immune evasion due to persistence of activated T cells without effector function. J. Exp. Med. 188, 2205–2213. doi:10.1084/jem.188.12.2205
Zebley, C. C., Brown, C., Mi, T., Fan, Y., Alli, S., Boi, S., et al. (2021). CD19-CAR T cells undergo exhaustion DNA methylation programming in patients with acute lymphoblastic leukemia. Cell Rep. 37, 110079. doi:10.1016/j.celrep.2021.110079
Zhao, J., Lin, Q., Song, Y., and Liu, D. (2018). Universal CARs, universal T cells, and universal CAR T cells. J. Hematol. Oncol. 11, 132. doi:10.1186/s13045-018-0677-2
Zheng, W., Wei, J., Zebley, C. C., Jones, L. L., Dhungana, Y., Wang, Y. D., et al. (2021). Regnase-1 suppresses TCF-1+ precursor exhausted T-cell formation to limit CAR-T-cell responses against ALL. Blood 138, 122–135. doi:10.1182/blood.2020009309
Zhu, X., Hu, H., Xiao, Y., Li, Q., Zhong, Z., Yang, J., et al. (2022). Tumor-derived extracellular vesicles induce invalid cytokine release and exhaustion of CD19 CAR-T Cells. Cancer Lett. 536, 215668. doi:10.1016/j.canlet.2022.215668
Keywords: CAR T cell exhaustion, tonic signaling, cytokines, tumor microenvironment, immune checkpoint blockade, epigenetics, transcriptomics
Citation: Zhu X, Li Q and Zhu X (2022) Mechanisms of CAR T cell exhaustion and current counteraction strategies. Front. Cell Dev. Biol. 10:1034257. doi: 10.3389/fcell.2022.1034257
Received: 01 September 2022; Accepted: 24 November 2022;
Published: 08 December 2022.
Edited by:
Kristian Michael Hargadon, Hampden–Sydney College, United StatesReviewed by:
Antonio Di Stasi, University of Alabama at Birmingham, United StatesParesh Vishwasrao, City of Hope National Medical Center, United States
Copyright © 2022 Zhu, Li and Zhu. This is an open-access article distributed under the terms of the Creative Commons Attribution License (CC BY). The use, distribution or reproduction in other forums is permitted, provided the original author(s) and the copyright owner(s) are credited and that the original publication in this journal is cited, in accordance with accepted academic practice. No use, distribution or reproduction is permitted which does not comply with these terms.
*Correspondence: Qing Li, bGlxaW5nNTQwNzA5MDdAMTYzLmNvbQ==; Xiaojian Zhu, emh1eGlhb2ppYW5AaHVzdC5lZHUuY24=