- 1Department of Biology, University of Toronto Mississauga, Mississauga, ON, Canada
- 2Department of Cell and Systems Biology, University of Toronto, Toronto, ON, Canada
Histone H3 lysine 9 di- and trimethylation are well-established marks of constitutively silenced heterochromatin domains found at repetitive DNA elements including pericentromeres, telomeres, and transposons. Loss of heterochromatin at these sites causes genomic instability in the form of aberrant DNA repair, chromosome segregation defects, replication stress, and transposition. H3K9 di- and trimethylation also regulate cell type-specific gene expression during development and form a barrier to cellular reprogramming. However, the role of H3K9 methyltransferases extends beyond histone methylation. There is a growing list of non-histone targets of H3K9 methyltransferases including transcription factors, steroid hormone receptors, histone modifying enzymes, and other chromatin regulatory proteins. Additionally, two classes of H3K9 methyltransferases modulate their own function through automethylation. Here we summarize the structure and function of mammalian H3K9 methyltransferases, their roles in genome regulation and constitutive heterochromatin, as well as the current repertoire of non-histone methylation targets including cases of automethylation.
1 Introduction
Lysine methylation is a dynamic posttranslational modification (PTM) that regulates protein structure and function in all three domains of life (Manzur and Zhou, 2005; Paik et al., 2007). The human genome is predicted to contain over one hundred protein lysine methyltransferases and almost two dozen demethylases that display a variety of substrate and product specificities (Shi and Tsukada, 2013; Husmann and Gozani, 2019). The first of these lysine methyltransferases to be identified was mammalian suppressor of variegation 3–9 homologue 1 (SUV39H1), Drosophila melanogaster suppressor of variegation 3–9 Su(var)3–9, and its Schizosaccharomyces pombe homolog, cryptic loci regulator 4 (Clr4), which produce histone H3 lysine 9 di- and trimethylation (H3K9me2 and H3K9me3) (Rea et al., 2000; Czermin et al., 2001; Nakayama et al., 2001). Since this discovery, many other methyltransferases have also been shown to methylate histones at various sites, which regulates genome packaging, organization, and functions including gene expression, DNA damage response, and DNA replication (Husmann and Gozani, 2019).
Histone proteins are highly conserved basic proteins that along with other non-histone proteins package and organize the eukaryotic genome into a complex called chromatin. The fundamental unit of chromatin, the nucleosome, is composed of approximately 147 base pairs of DNA wrapped around a histone octamer containing two copies of each of the core histones—H2A, H2B, H3, and H4 (Kornberg, 1974; Luger et al., 1997). Observations made by Emil Heitz in the 1920s established that there are two distinct types of chromatin for which he coined the terms ‘heterochromatin’ and ‘euchromatin,’ referring to condensed darkly stained chromosomal regions and decondensed light staining regions, respectively (Heitz, 1928). Euchromatin is associated with active transcription, whereas heterochromatin is predominantly transcriptionally repressed (Allis and Jenuwein, 2016). Heterochromatin clusters into distinct compartments within the nucleus including the perinucleolar region and at the nuclear periphery in a variety of different species including S. pombe, D. melanogaster, Caenorhabditis elegans, mouse, and humans (Funabiki et al., 1993; Horsley et al., 1996; Minc et al., 1999; Pickersgill et al., 2006; Guelen et al., 2008; Ikegami et al., 2010; Peric-Hupkes et al., 2010). These localizations and clustering are mediated by protein-protein interactions as well as phase separation (Olins et al., 2010; Brachner and Foisner, 2011; Towbin et al., 2012; Poleshko et al., 2013; Solovei et al., 2013; Wong et al., 2014; Larson et al., 2017; Strom et al., 2017). H3K9me2 and H3K9me3 are the hallmarks of heterochromatin conserved from fission yeast to humans (Allshire and Madhani, 2018). Traditionally, H3K9 methylation (H3K9me) is associated with the silencing of repetitive DNA sequences including pericentromere and subtelomere repeats and transposable elements in order to maintain genome stability (Janssen et al., 2018). This is referred to as constitutive heterochromatin because these domains remain methylated and silent throughout the cell cycle and development in most cell types. However, more recently, H3K9me has been reported to silence protein-coding genes in a cell type-specific manner throughout development, which is called facultative heterochromatin (Padeken et al., 2022). In addition to its role in establishing cell type-specific gene expression programs, H3K9me also helps stabilize cell fate decisions and maintain cell identity (Becker et al., 2016; Padeken et al., 2022). H3K9me coats critical genomic binding sites for master regulator proteins in terminally differentiated cells, which reduces binding of these master regulators and hinders cellular reprogramming of induced pluripotent stem (iPS) cells and somatic cell nuclear transfer (SCNT) (Shi et al., 2008; Onder et al., 2012; Soufi et al., 2012; Sridharan et al., 2013; Matoba et al., 2014; Becker et al., 2016; Liu et al., 2018).
Mammals have six well characterized H3K9 methyltransferases including SUV39H1 and SUV39H2, SET domain bifurcated 1 and 2 (SETDB1 and SETDB2), G9a, and G9a-like protein (GLP). A compound mutant eliminating the function of all six of these methyltransferases in mouse embryonic fibroblasts was recently generated (Montavon et al., 2021). In these cells, a complete loss of all H3K9 methylation states, decondensation of heterochromatin, additive derepression of a wide variety of repeat elements, genome instability, and loss of heterochromatin compartmentalization was observed (Montavon et al., 2021). Partial overlapping functions between mammalian H3K9 methyltransferases have been identified in this and other studies (Montavon et al., 2021; Fritsch et al., 2010; Bulut-Karslioglu et al., 2014; García-Cao et al., 2004; Gauchier et al., 2019; Maksakova et al., 2013; Liu et al., 2014; di Giacomo et al., 2014). These redundancies combined with technical challenges involved with mapping genomic H3K9me complicate studies of H3K9me in mammalian systems (Montavon et al., 2021; Fritsch et al., 2010; Bulut-Karslioglu et al., 2014; García-Cao et al., 2004; Gauchier et al., 2019; Maksakova et al., 2013; Liu et al., 2014; di Giacomo et al., 2014; Becker et al., 2017). As a result, much of what we know about the role and regulation of H3K9me has come from studies in simpler model organisms with fewer H3K9 methyltransferases including S. pombe, D. melanogaster, and C. elegans (Elgin and Reuter, 2013; Holoch and Moazed, 2015; Ahringer and Gasser, 2018; Allshire and Madhani, 2018).
However, H3K9 is not the only target of H3K9 methyltransferases. They methylate a variety of other histone and non-histone targets, which have important functional consequences in gene regulation. SUV39H1 and SUV39H2 primarily methylate other chromatin modifying proteins (Zhang et al., 2011; Piao et al., 2015; Kudithipudi et al., 2017). SETDB1 methylates proteins involved in proliferation and cell cycle regulation including AKT and mutant p53, while G9a/GLP regulates various transcription factors (TFs) as well as several hypoxia stress response factors (Feldman et al., 2006; Pless et al., 2008; Lee et al., 2010; Lee et al., 2011; Ling et al., 2012; Choi et al., 2014; Bao et al., 2018). SUV39H2 and G9a/GLP have also been shown to regulate their own functions through automethylation (Chin et al., 2007; Sampath et al., 2007; Piao et al., 2016; Poulard et al., 2017; Iglesias et al., 2018).
In this review, we will provide a synopsis of the structural and biochemical characteristics that define the main classes of H3K9 methyltransferases in mammals. We will describe the canonical role H3K9me plays in silencing constitutive heterochromatin domains and the maintenance of genome stability along with its more recently appreciated function in the regulation of cell type-specific gene expression. Lastly, we will highlight the regulation of non-histone targets by H3K9 methyltransferase-mediated methylation and automethylation.
1.1 Structure and domain architecture of H3K9 methyltransferases
All lysine methyltransferases, except for DOT1-like methyltransferases, contain a catalytic SET domain named after the three founding members Su(var)3–9, Enhancer-of-zeste, and Trithorax (Jones and Gelbart, 1993; Tschiersch et al., 1994; Stassen et al., 1995; Jenuwein et al., 1998; Min et al., 2003). The core SET domain structure is comprised of two non-contiguous regions that span approximately 130 amino acids (Figure 1)(Dillon et al., 2005). The N-terminal and C-terminal regions are both highly conserved and consist of a short helix and three or four short ß-strands that adopts a ß-sheet fold pseudo-knot-type structure (Figure 1) (Dillon et al., 2005). An insert region, referred to as SET-I, joins the two-halves of the SET domain and plays important roles in substrate recognition and enzyme regulation (Figure 1) (Qian and Zhou, 2006; Jiao and Liu, 2015; Ishimoto et al., 2016; Li et al., 2016; Sun and Fang, 2016). The size and structure of SET-I varies greatly depending on the methyltransferase: SUV39H2 SET-I consists of 37 amino acids that form a helix followed by a short loop (Wu et al., 2010); whereas, SETDB1 has a 362 amino acid SET-I that is predicted to contain a mixed structure. The canonical SET domain is flanked by both pre- and post-SET domains in the SUV39-family of methyltransferases, which includes all H3K9 methyltransferases (Figures 1, 2) (Dillon et al., 2005). The pre-SET domain is composed of random coil with nine invariant cysteines that coordinate three zinc ions in a triangular cluster (Figure 1) (Dillon et al., 2005). Six of the cysteines are involved in the coordination of a single zinc atom with the remaining three cysteines coordinating two zinc atoms each (Dillon et al., 2005). The post-SET domain is also composed of random coil (Figure 1) (Dillon et al., 2005). Three conserved cysteines from the post-SET domain and a fourth in the knot-like structure of the SET domain close to the active site coordinate a single zinc atom that is required for methyltransferase activity (Figure 1) (Zhang et al., 2002; Dillon et al., 2005). Proper folding of the post-SET domain is required for methyltransferase activity as mutating these invariant cysteines abolish enzyme function (Rea et al., 2000; Schultz et al., 2002; Zhang et al., 2002). The conserved catalytic tyrosine is found at the C-terminus of SET domain with the methyl donor s-adenosyl-l-methionine (SAM) sandwiched between the core SET domain, SET-I, and the post-SET domain (Figure 1) (Qian and Zhou, 2006). Product specificity is dictated by a phenylalanine/tyrosine switch site located two amino acids N-terminal to the catalytic tyrosine (Collins et al., 2005; Couture et al., 2008). There are four possible lysine methylation states—unmethylated, monomethylated, dimethylated, and trimethylated—each of which represent distinct modifications associated with unique biological consequences (Figure 3) (Black et al., 2012). Enzymes with a phenylalanine in the switch position catalyze di- and tri-methylation, whereas tyrosine in the switch position restricts the enzymes to mono- and dimethylation (Collins et al., 2005; Couture et al., 2008).
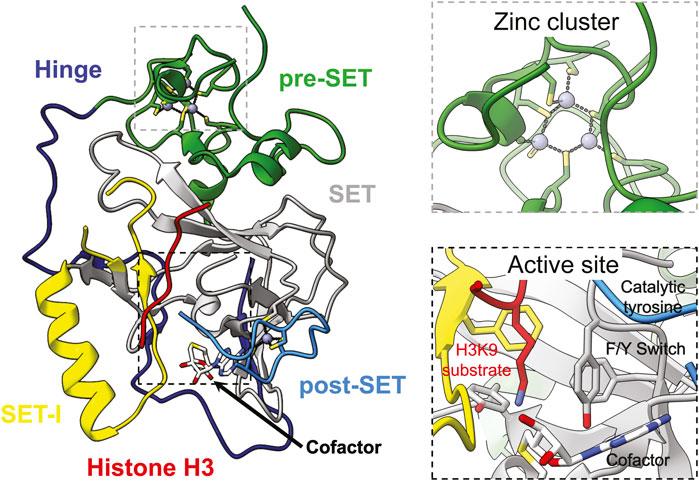
FIGURE 1. Structure of the conserved core catalytic SET domain of H3K9 methyltransferases. Structure of the first SET domain to be determined, Dim-5 an H3K9 methyltransferase from Neurospora crassa, in complex with cofactor (white) and histone peptide substrate (red) (PDB ID 1PEG) (left). Close up of conserved cysteines and triangular zinc cluster of the pre-SET domain (top right). Close up of active site (bottom right). The Hinge, pre-SET, SET, SET-I, and post-SET domains are coloured dark blue, green, grey, yellow, and light blue, respectively. Zinc atoms are depicted as dark grey spheres.
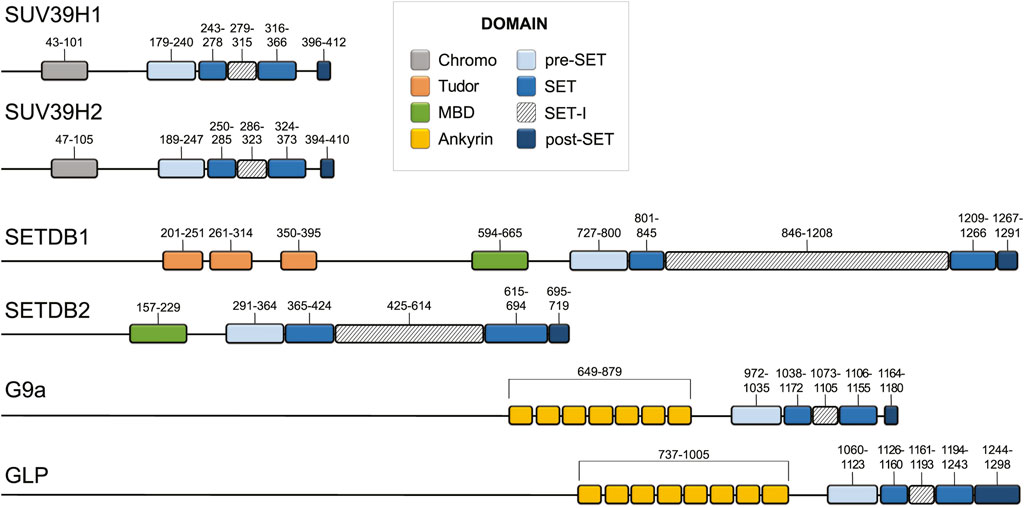
FIGURE 2. Domain architecture of the main human H3K9 methyltransferases. Domain architecture of SUV39H1, SUV39H2, SETDB1, SETDB2, G9a, and GLP (Apweiler et al., 2004; Wu et al., 2010; Torrano et al., 2019; Weirich et al., 2021). Domain boundaries are listed above each domain with chromodomain, pre-SET, SET, SET-I, post-SET, Tudor, MBD, and Ankyrin repeat domains coloured grey, light blue, blue, diagonal hatching, dark blue, orange, green, and yellow, respectively.

FIGURE 3. Methylation states catalyzed by main mammalian H3K9 methyltransferases. SUV39H1, SUV39H2, SETDB1, SETDB2, G9a, and GLP transfer methyl groups (red) from cofactor SAM to the epsilon nitrogen (blue) of lysine residues to create mono-, di-, and trimethyllysine.
Of the six mammalian SUV39-family H3K9 methyltransferases: G9a and GLP generate mono- and dimethylation and SUV39H1, SUV39H2, SETDB1, and SETDB2 catalyze di- and trimethylation, with the majority of trimethylation coming from SUV39H1 and SUV39H2 in vivo (Wu et al., 2010; Montavon et al., 2021) (Figure 3). These enzymes display differences in the SET-I region and in their complement of N-terminal domains (Marmorstein, 2003; Xiao et al., 2003) (Figure 2). In addition to a catalytic “writer” domain, some histone methyltransferases also have what are referred to as “reader” domains, which recognize and recruit the enzyme to regions of the genome enriched in a particular modification (Hyun et al., 2017). The “read/write” mechanism refers to a particular situation when the writer domain produces a modification that is recognized by a reader domain on the same protein, which creates a positive feedback loop that reinforces a chromatin state (Zhang et al., 2015). In addition to the “reader” domains, H3K9 methyltransferases are also recruited via TFs, non-coding RNA, the RNAi pathway, and m6A RNA (Schultz et al., 2002; Jia et al., 2004; Porro et al., 2014; Holoch and Moazed, 2015; Scarola et al., 2015; Johnson et al., 2017; Shirai et al., 2017; Velazquez Camacho et al., 2017; Ahringer and Gasser, 2018; Tie et al., 2018; Erdmann and Picard, 2020; Chelmicki et al., 2021; Liu et al., 2021; Xu et al., 2021; Wei et al., 2022). SUV39H1 and SUV39H2, two highly related H3K9 methyltransferases, have an N-terminal chromodomain connected to their catalytic SET domains via a flexible hinge region (Figure 2) (Melcher et al., 2000; O’Carroll et al., 2000). The chromodomain is 40–50 residues composed of a short alpha helix packed against three antiparallel beta strands that recognize and recruit SUV39H1 and SUV39H2 to H3K9me via a conserved aromatic cage (Jacobs and Khorasanizadeh, 2002; Wang et al., 2012). The chromodomain of SUV39H1 and the mouse-specific N-terminal 81 amino acid basic domain of SUV39H2 also bind RNA transcribed from major satellite repeats, which provides a mechanism to recruit SUV39H1 and SUV39H2 to transcribed repeat sequences (Johnson et al., 2017; Shirai et al., 2017; Velazquez Camacho et al., 2017). The chromodomain and linker region between the chromodomain and pre-SET domain of Clr4 has been shown to interact with the nucleosome core (Akoury et al., 2019). G9a reads H3K9me1 and H3K9me2 through N-terminal ankyrin repeat domains (Figure 2) (Collins et al., 2008). GLP is a paralog of G9a which shares 70% sequence similarity. The greatest sequence divergence between G9a and GLP is in the N-terminus of the protein at the glutamic acid rich domain of G9a, which contains a series of repeated aspartic and glutamic acid residues in GLP. Although G9a and GLP are capable of forming both homo and heterodimers via their SET domains, the heterodimer is the more active form of the enzyme with greater reading and writing capabilities in vitro (Sanchez et al., 2021). However, the significance of this observation remains to be tested in vivo. SETDB1 and SETDB2 contain three N-terminal Tudor domains and a methyl-CpG binding domain (MBD) domain (Figure 2) (Jurkowska et al., 2017). The Tudor domains form complexes with other regulators that are vital for transcriptional repression and cooperate to recognize histone tails bearing both H3K14 acetylation and H3K9 methylation (Yang et al., 2003; Li et al., 2006; Jurkowska et al., 2017). The MBD domain possesses two arginine residues that facilitate DNA binding and link H3K9 trimethylation with DNA methylation via MBD interaction with DNA methyltransferase 3 in organisms that have DNA methylation (Li et al., 2006; Chen et al., 2017). However, the MBD domain is conserved in species that lack DNA methylation including D. melanogaster and C. elegans, which suggests that the MBD domain may also possesses DNA methylation-independent functions. The N-terminus of SETDB1 also contains a binding site for activating transcriptional factor 7-interacting protein 1 (ATF7IP), which is a conserved cofactor of SETDB1 that stimulates SETDB1 methyltransferase activity and is required for its nuclear localization and chromatin association (Wang et al., 2003; Timms et al., 2016; Mutlu et al., 2018; Delaney et al., 2019; Osumi et al., 2019; Tsusaka et al., 2019).
2 Constitutive heterochromatin and the regulation of repetitive DNA elements
About half of the human genome is composed of repetitive DNA elements including centromeres and pericentromeric regions, telomeres and subtelomeres, transposons, and ribosomal DNA (rDNA) that are packaged into constitutive heterochromatin (Lander et al., 2001; Venter et al., 2001). H3K9me and silencing of these regions is critical for maintaining genome stability by protecting telomeres and preventing chromosome segregation defects, recombination, and transposition (Ekwall et al., 1996; Nonaka et al., 2002; Slotkin and Martienssen, 2007; Peng and Karpen, 2009). The role of H3K9me and H3K9 methyltransferases is best understood in constitutive heterochromatin. Mammalian H3K9 methyltransferases play both distinct and overlapping functions in the silencing of these repetitive elements. A multimeric complex containing SUV39H1, G9a, GLP, and SETDB1 has been reported, which may help explain some of the redundancies (Fritsch et al., 2010). In SUV39H1 or G9a knockout cells the remaining methyltransferase components of the complex are destabilized, which results in an overall reduction in their protein levels (Fritsch et al., 2010). SUV39H1 and SUV39H2 target pericentromeric repeats, telomeres, class II endogenous retroviruses (ERVs), and long interspersed nuclear elements (LINEs) (García-Cao et al., 2004; Martens et al., 2005; Bulut-Karslioglu et al., 2014). SUV39H1 also represses non-transcribed rDNA repeats (Murayama et al., 2008). However, in the absence of SUV39H1 and SUV39H2, low levels of H3K9me3 persist at telomeres and LINE elements that are SETDB1-dependent (García-Cao et al., 2004; Bulut-Karslioglu et al., 2014; Gauchier et al., 2019). Endoderm-specific conditional SETDB1 knockout mice display only a modest reduction in H3K9me3 levels (Nicetto et al., 2019). The conditional knockout of SETDB1, SUV39H1, and SUV39H2 together causes a substantial decrease in H3K9me3 and marked derepression of nonhepatic genes in mouse livers (Nicetto et al., 2019). The triple knockout results in a significantly different transcriptional profile compared to both the SETDB1 conditional knockout and wild type mouse liver cells (Nicetto et al., 2019). SETDB1 plays non-redundant roles in the silencing of the ERV family class I and class II long terminal repeat (LTR)-containing viruses (Matsui et al., 2010; Karimi et al., 2011; Collins et al., 2015; Fasching et al., 2015; Takikita et al., 2016; Kato et al., 2018; Adoue et al., 2019; Wang et al., 2020; Južnić et al., 2021). Widespread reactivation of ERVs in SETDB1 knockout cells also produces chimeric ERV-initiated transcripts that splice with genic exons and likely interfere with the expression of the native open reading frames (Karimi et al., 2011). G9a is essential for H3K9 methylation outside of pericentric heterochromatin and telomeres/subtelomeric domains along with some repetitive elements including rDNA repeats and class III ERVs (Tachibana et al., 2001; Tachibana et al., 2002; Tachibana et al., 2005; Collins et al., 2008; Maksakova et al., 2013; Jiang et al., 2020; Zhou et al., 2020). G9a and GLP display some overlap in function with SETDB1 when it comes to methylating and silencing intracisternal A-particles (IAPs) (Maksakova et al., 2013; Liu et al., 2014; di Giacomo et al., 2014). The role of SETDB2 in the silencing of repetitive elements is unknown. Further investigation of targets and functions of these methyltransferases in different cell types and contexts is required to better understand how H3K9me contributes to disease and developmental processes. In this section we will focus on the different types of repetitive DNA sequences that form constitutive heterochromatin.
2.1 Centromere and pericentromere
Centromeric chromatin is the site of kinetochore assembly during mitosis (Sullivan et al., 2001; Mellone and Allshire, 2003). Since histone H3 is replaced with the centromere-specific H3 variant CENP-A in centromeric heterochromatin, centromeres themselves lack H3K9 methylation (Yoda et al., 2000; Blower et al., 2002; Sullivan and Karpen, 2004). However, flanking pericentromeric chromatin is highly enriched in H3K9me2, H3K9me3, and heterochromatin protein 1 (HP1), which are all required for de novo CENP-A deposition at centromeres, proper microtubule attachment to kinetochores, sister chromosome cohesion, and chromosome segregation (Ekwall et al., 1995; Ekwall et al., 1996; Bernard et al., 2001; Nonaka et al., 2002; Folco et al., 2008; Yamagishi et al., 2008). Loss of either H3K9 methyltransferases or HP1 proteins cause chromosome segregation defects including micronuclei formation, chromosomal breaks and rearrangements, and aneuploidy (Ekwall et al., 1995; Ekwall et al., 1996; Peters et al., 2001; Peng and Karpen, 2009; Janssen et al., 2011; Crasta et al., 2012).
2.2 Telomere and subtelomere
Telomeres are specialized chromatin structures that cap and safeguard the ends of linear chromosomes (van Steensel et al., 1998; Karlseder et al., 2004; Yang et al., 2005; Bae and Baumann, 2007; Denchi and de Lange, 2007; Sfeir and de Lange, 2012). They are comprised of G-rich short tandem repeats that can reach as long as 50 kb long in mammals (Barral and Déjardin, 2020). These sequences are recognized by the telomere repeat-specific binding proteins of the Shelterin complex, which recruits telomerase and other factors that prevent an aberrant DNA damage response (van Steensel et al., 1998; Karlseder et al., 1999; Li and de Lange, 2003; Karlseder et al., 2004; Ye et al., 2004; Kelleher et al., 2005; Yang et al., 2005; Bae and Baumann, 2007; Denchi and de Lange, 2007; Wang et al., 2007; de Lange, 2018; Barral and Déjardin, 2020). Loss of Shelterin results in catastrophic chromosomal fusion where chromosomes become joined through their telomeres (van Steensel et al., 1998). Telomere lengthening is catalyzed by telomerase and can also occur through the telomerase-independent Alternative Lengthening of Telomeres (ALT) pathway, which is often associated with cancer (Dunham et al., 2000). This pathway enlists components of homologous recombination pathways to lengthen telomeres (Dunham et al., 2000). In S. pombe, both Clr4/Suv39 and the histone deacetylase Snf2/HDAC-containing Repressor Complex (SHREC) are recruited to telomeres by Shelterin, which subsequently establish heterochromatin in the adjacent subtelomeric repeats (Sugiyama et al., 2007; Zhang et al., 2008). The RNAi pathway also recruits Swi6/HP1 and SHREC to the subtelomeres and contributes to heterochromatin formation, however, this only occurs in S. pombe (Volpe et al., 2002; Zofall and Grewal, 2006; Déjardin and Kingston, 2009; Saksouk et al., 2014; Gauchier et al., 2019). In mammals, telomeric and subtelomeric chromatin is heterochromatic and the loss of silencing can cause aberrant recombination and DNA damage, but recent evidence suggests that this may only be true when the ALT pathway is activated (Lovejoy et al., 2012; Arora et al., 2014; Cubiles et al., 2018).
2.3 Transposable elements
Transposable elements (TE) make up at least 45% of the human genome with LINE-1 elements alone accounting for 17% of the genome (Cordaux and Batzer, 2009). Although most TEs lack transposition activity due to acquired inactivating mutations, some remain intact (Cordaux and Batzer, 2009). Silencing of TEs is required to prevent DNA damage caused by deleterious RNA:DNA structures and gene disruption or rearrangements caused by hopping mobile elements (Slotkin and Martienssen, 2007; Zeller et al., 2016). More than 1,200 distinct types of TEs have been identified (Kojima, 2018). For most of these, how they are regulated is unknown. However, several major classes including ERV class I elements, LINE elements, and major satellite repeats (MSRs) are all derepressed in the absence of H3K9me2 and H3K9me3 in mouse embryonic fibroblasts (Montavon et al., 2021).
3 Facultative heterochromatin and the regulation of protein-coding genes
Traditionally, H3K9me3 and H3K27me3 have been associated with constitutive and facultative heterochromatin, respectively. However, a more dynamic role for H3K9me3-mediated gene regulation during development has emerged, which challenges this classical view (Becker et al., 2017; Nicetto et al., 2019). Embryos with tissue-specific triple knockouts of SETDB1, SUV39H1, and SUV39H2 have a loss of cell type-specific gene expression programs in addition to derepression of lineage-inappropriate genes, leading to perturbation of cell identity (Nicetto et al., 2019). Although H3K9me3 levels are diluted over the first two to three cell divisions due to the lack of de novo H3K9me deposition, it becomes enriched at many protein-coding genes and constitutive heterochromatin sites during cell fate determination, which prevents premature activation of cell type-specific genes (Liu et al., 2004; Puschendorf et al., 2008; Nicetto et al., 2019). H3K9me undergoes dramatic reprogramming in the early stages of development with lineage-specific H3K9me3 patterns arising post-implantation (Wang et al., 2018). Upon differentiation, cell type-specific genes are derepressed through loss of H3K9me3 while lineage-inappropriate genes maintain H3K9me3 and transcriptional silencing (Figure 4) (Nicetto et al., 2019). Similar H3K9me dynamics have recently been reported in C. elegans. Genes expressed in embryos tend to gain H3K9me2/3 in differentiated cells, while cell type-specific genes that are expressed in differentiated cells tend to lose H3K9me2/3 (Methot et al., 2021). Together these studies demonstrate that H3K9me mediated gene silencing is specific to both cell type and developmental stage. In this section, we explore the role of H3K9me in facultative heterochromatin and its contribution to development.
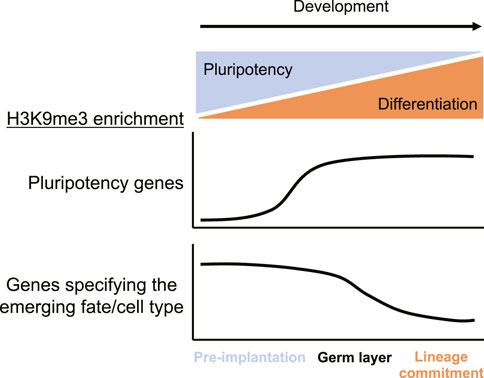
FIGURE 4. H3K9me3 enrichment at pluripotency versus cell type-specific genes throughout development. In early, pre-implantation stages, H3K9me3 is missing from pluripotency genes but highly enriched at cell type-specific genes, which promotes the pluripotency program. As development proceeds into the germ layer stage, H3K9me3 is deposited at pluripotency genes and becomes significantly lost at cell type-specific genes during lineage commitment to promote tissue specificity. H3K9me3 differentially regulates pluripotency and cell type-specific genes to orchestrate the timely progression of developmental processes.
In S. pombe, H3K9me2 plays a critical role in promoting vegetative growth and adaptation to environmental challenges (Zofall et al., 2012; Torres-Garcia et al., 2020). Vegetative growth is achieved through the formation of heterochromatin domains that silence meiotic genes including mei4 and ssm4 that are involved in cell cycle progression and microtubule organization during meiosis, respectively (Zofall et al., 2012). Nitrogen depletion triggers removal of H3K9me2 and derepression of these genes, which results in a shift from vegetative to sexual growth (Zofall et al., 2012). When returned to nitrogen-containing media, H3K9me2 is deposited at these meiotic genes and the cells resume vegetative growth (Zofall et al., 2012). H3K9me2 also mediates phenotypic plasticity in response to unfavourable growth conditions (Torres-Garcia et al., 2020). Caffeine is cytotoxic to S. pombe (Fabre, 1972; Loprieno et al., 1974; Gentner and Werner, 1975; Osman and McCready, 1998). However, exposure to threshold levels of caffeine causes resistance through the formation of epimutations or heritable changes in gene expression that do not affect the underlying DNA sequence (Torres-Garcia et al., 2020). Heterochromatin islands form over and reduce expression of distinct genes, some of which confer caffeine resistance when mutated (Torres-Garcia et al., 2020). The Mst2 histone acetyltransferase and Epe1, a putative H3K9 demethylase, cooperate to prevent the formation of heterochromatin islands (Wang et al., 2015). However, caffeine exposure reduces the levels of Epe1 and produces a shorter and likely functionally impaired isoform of Mst2 (Torres-Garcia et al., 2020). Thus, H3K9me2 heterochromatin is critical for the regulation of sex determination in S. pombe by modulating meiotic gene expression to promote a vegetative state and for phenotypic plasticity by conferring transient resistance to environmental challenges.
In mammals, H3K9me3 regulates genes involved in pluripotency to terminate stemness and facilitate differentiation (Feldman et al., 2006; Epsztejn-Litman et al., 2008). Pre-implantation, pluripotency factors lack H3K9me3 and are expressed, which facilitates stem-like properties (Figure 4) (Feldman et al., 2006; Epsztejn-Litman et al., 2008). However, following embryonic implantation, both pluripotency genes and lineage-specific genes are methylated at H3K9 and transcriptionally repressed (Figure 4) (Feldman et al., 2006; Epsztejn-Litman et al., 2008). The promoter region of murine Oct-3/4, for instance, is dynamically regulated by H3K9me during the pre- and post-implantation stages (Feldman et al., 2006). During pre-implantation, embryonic stem cells maintain high levels of the active H3K9 acetylation mark at the Oct-3/4 gene, promoting pluripotency (Feldman et al., 2006). Retinoic acid-induced differentiation causes H3K9 deacetylation and G9a-mediated H3K9me3 deposition at the Oct-3/4 locus, which facilitates transcriptional repression (Feldman et al., 2006). Similarly, H3K9me silences genes involved in stemness and memory during terminal differentiation of mouse CD8+ T cells to prevent reprogramming into pluripotent memory cells (Pace et al., 2018). In the nervous system, knocking out SUV39H1 and SUV39H2 in the adult hippocampus increases the proportion of progenitor cells relative to mature neurons and leads to high levels of progenitor proliferation in vitro (Guerra et al., 2021). Lysine-to-methionine mutations have the ability to globally reduce methylation levels at the corresponding lysine by interfering with SET domain methyltransferases (Lewis et al., 2013; Herz et al., 2014; Fang et al., 2016; Jayaram et al., 2016; Lu et al., 2016). Expression of the histone H3.3 variant with lysine 9 mutated to methionine (H3K9M) in mouse embryonic stem cells results in small embryoid bodies with reduced H3K9me3 levels, increased chromatin accessibility, continued expression of several pluripotency markers, and reduced expression of some markers of differentiation (Brumbaugh et al., 2019). Mice expressing H3K9M have increased multipotent progenitors and display a number of additional cell type-specific defects including aberrant lymphopoiesis and thrombocytosis (Brumbaugh et al., 2019). Interestingly, ceasing H3K9M expression reverses differentiation defects, at least in the case of in vitro B cell maturation (Brumbaugh et al., 2019). These studies highlight a role for H3K9me in the dynamic regulation of pluripotency genes at the onset of differentiation (Figure 4).
In addition to regulating pluripotency genes, H3K9me3 also prevents developmental relapse into a more primitive totipotent state by selectively silencing genes associated with the 2-cell stage (Wu et al., 2020). Reducing H3K9me in early development by knocking out SETDB1 leads to peri-implantation lethality, underscoring the importance of this methyltransferase in early embryogenesis (Dodge et al., 2004). The developmental transition from totipotency to pluripotency is marked by the upregulation of stem-cell factors including Oct4 and Nanog, and the downregulation of trophectoderm markers and genes involved in the 2-cell stage (Wu et al., 2020). Knocking out SETDB1 results in aberrant upregulation of Dux, a critical gene associated with 2-cell state totipotency, as well as markers of trophectoderm differentiation including Hand1 and Cdx2 (Bilodeau et al., 2009; Yuan et al., 2009; Lohmann et al., 2010; Wu et al., 2020). Thus, H3K9me deposition by SETDB1 at totipotency genes plays a critical role in regulating stem-like properties following the 2-cell stage. This suggests that H3K9me-mediated gene regulation is not only important for promoting the transition towards pluripotency, but also for preventing relapse into a totipotent state.
In later stages of development during differentiation, H3K9me2/3 regulates cell type-specific genes to promote cell identity and lineage commitment (Figure 4) (Guerra et al., 2021). After the epiblast stage, during germ layer specification in mice, protein-coding genes become significantly enriched in H3K9me3 (Figure 4) (Nicetto et al., 2019). Cell type-specific genes are dynamically derepressed through a loss of H3K9me3 after germ layer specification at the onset of organogenesis (Figure 4) (Nicetto et al., 2019). In endoderm cells, known markers of the hepatic lineage including the Cyp gene cluster are retained in H3K9me3-associated heterochromatin and become derepressed upon differentiation into hepatic progenitors and hepatocytes (Nicetto et al., 2019). However, genes associated with alternate cell fates, such as the pancreas-specific gene Slc30a8, remain H3K9me3-enriched and transcriptionally silent in differentiated hepatic cells (Nicetto et al., 2019). Furthermore, 2-month-old liver cells derived from endoderm-specific conditional triple knockout embryos for SETDB1, SUV39H1, and SUV39H2 have significantly reduced liver-specific gene expression and upregulation of lineage-nonspecific genes involved in various processes such as embryonic morphogenesis, heart development, and RNA processing/translation (Nicetto et al., 2019). Similarly, many genes expressed in C. elegans embryos gain H3K9me2/3 in differentiated cells, while cell type-specific genes that are expressed in differentiated cells lose H3K9me2/3 (Methot et al., 2021). Once established, these methylation patterns require active maintenance by at least the MET-2 SETDB1-like H3K9me1/2 methyltransferase (Methot et al., 2021). Although H3K9me2 blocks transcription factor binding and is necessary for silencing, its loss in MET-2 and SET-25 methyltransferase deficient animals is not sufficient for chromatin decompaction or gene activation (Methot et al., 2021). The presence of specific transcription factors is required for the expression of genes that lack H3K9me2/3, which helps to explain the observed cell type-specific effects of H3K9me2/3 on gene expression in C. elegans (Methot et al., 2021). H3K9me3 is also important for maintaining TH2 lymphocytes since SUV39H1 participates in TH1-specific gene repression and SETDB1 is required for maintaining stable lineage commitment (Allan et al., 2012; Adoue et al., 2019). SETDB1-dependent H3K9me3 also represses adipogenic master regulatory genes until differentiation is required and regulates cell fate decisions in murine neurogenesis, myogenesis, and oligodendrocyte differentiation (Tan et al., 2012; Liu et al., 2015; Matsumura et al., 2015; Beyer et al., 2016; Jiang et al., 2017). Loss of G9a during myogenesis and haematopoiesis results in derepression of lineage inappropriate genes and in the case of myogenesis, cell cycle regulators are also derepressed (Chen et al., 2012; Rao et al., 2016). G9a is also required for neuronal differentiation and the maintenance of the differentiated neurons (Fiszbein et al., 2016). Therefore, H3K9me3 deposition during the germ layer stage is essential for ensuring cell identity in later stages of differentiation and lineage commitment (Nicetto et al., 2019). During differentiation, H3K9me3 is dynamically lost at cell type-specific genes to promote their derepression while lineage-inappropriate genes retain H3K9me3 heterochromatin, which ensures proper cell fate determination (Figure 4) (Nicetto et al., 2019).
Once cells reach a terminally differentiated state, H3K9me3 forms a barrier to cellular reprogramming by maintaining gene expression programs that are critical for cell identity (Becker et al., 2016). Large domains of H3K9me3 coat important pluripotency genes in differentiated cells and hinder the binding of the Yamanaka transcription factors—Oct4, Sox2, Klf4, and c-Myc (Soufi et al., 2012). Suppressing H3K9 methyltransferases improves the efficiency of iPS cell generation by increasing the binding of Oct4 and Sox2 to these sites (Shi et al., 2008; Onder et al., 2012; Soufi et al., 2012; Sridharan et al., 2013). Similarly, SCNT is also enhanced when H3K9 methyltransferases are depleted or an H3K9 demethylase is expressed in conjunction with deacetylase inhibitor treatment (Matoba et al., 2014; Liu et al., 2018). Therefore, H3K9me not only plays important roles in establishing cell type-specific gene expression programs, but also in maintaining the stability of lineage commitment and cell identity.
Selective loss of H3K9me3 at appropriate genes regulates the timely progression of development from the 2-cell stage through lineage commitment. In early murine development, pluripotency genes lack H3K9me3 and are expressed (Figure 4). Pluripotency and cell type-specific genes are repressed by H3K9me3 during the germ layer stage, however the mechanism of cell type-specific gene silencing prior to germ layer specification is still unclear (Nicetto et al., 2019). In later stages of development during differentiation and lineage commitment, H3K9me3 ensures tissue-specific gene expression programs and cell identity through selective loss at cell type-specific genes and retention at lineage-inappropriate genes (Nicetto and Zaret, 2019). Taken together, these studies highlight the importance of H3K9me in development and cell fate determination.
4 Additional methylation targets
Although the best-known target of H3K9 methyltransferases is histone H3K9, they also regulate a growing list of additional histone and non-histone proteins through methylation and in some cases automethylation (for a comprehensive list see Table 1). In this section we provide an overview of automethylation, some key histone and non-histone targets of H3K9 methyltransferases, and outline the known biological consequences of these methylation events.
4.1 Histone targets
G9a has been suggested to methylate other sites on histone H3 including H3K27 and H3K56. Both G9a and GLP methylate H3K27 in vitro and in embryonic stem cells loss of G9a reduces H3K27me1 levels by 30% (Tachibana et al., 2001; Wu et al., 2011). H3K56me1 interacts directly with PCNA in vitro and the two colocalize in the G1 phase of the cell cycle (Yu et al., 2012). Knockout or depletion of G9a or mutating H3K56 impairs DNA replication (Yu et al., 2012). In addition to canonical histone proteins that form the proteinaceous core of the nucleosome, linker histones are another major component of chromatin (Woodcock et al., 2006). Humans have 11 different H1 variants, five of which are methylated by G9a and GLP (Trojer et al., 2009; Weiss et al., 2010; Brockers and Schneider, 2019). G9a and GLP methylate H1.4 on its N-terminus at lysine 26, which can be reversed by the KDM4 demethylase (Trojer et al., 2009; Weiss et al., 2010). H1.4K26me forms a binding site for HP1 proteins, which suggests that this modification may have a role in transcriptional gene silencing and heterochromatin formation (Trojer et al., 2009; Weiss et al., 2010). G9a and GLP methylate H1.0, H1.2, H1.3, and H1.5 on their C-terminus (Weiss et al., 2010). However, unlike H1.4K26me, H1.2K187me does not bind HP1 proteins, which reveals H1 variant-specific functions of G9a and GLP methylation that require further investigation (Trojer et al., 2009; Weiss et al., 2010).
4.2 Non-histone targets
4.2.1 SUV39H2 and Clr4 automethylation
Formation of repressive chromatin domains is tightly regulated to prevent deleterious epigenetic gene silencing. This regulation occurs at many levels and includes pathways that recruit H3K9 methyltransferases, extrinsic antisilencing factors such as H3K9 demethylases, and mechanisms of histone turnover. However, it was recently shown that the activity of Clr4 itself is regulated through a novel intrinsic autoregulatory mechanism (Iglesias et al., 2018). An internal loop, dubbed the autoregulatory loop (ARL) in Clr4, inhibits enzyme activity by blocking the substrate-binding pocket (Figure 5) (Iglesias et al., 2018). Intramolecular automethylation of two lysines within the loop, K455 and K472, promotes a conformational switch in the enzyme that opens the substrate-binding pocket and enhances Clr4 activity (Figure 5) (Iglesias et al., 2018). Mutating Clr4 automethylation sites in vivo disrupts this autoregulation, resulting in aberrant H3K9me2 and H3K9me3, loss of heterochromatin domains, and slow growth in S. pombe (Iglesias et al., 2018). This demonstrates the critical role Clr4 autoregulation plays in both regulating H3K9me2 and H3K9me3 deposition and maintaining epigenetic stability. The second more C-terminal automethylation site, K472, is broadly conserved within the SUV39H family of methyltransferases (Iglesias et al., 2018). However, the first automethylation site, K455, is only found in the mammalian SUV39H2 enzyme and corresponds to K392 (Iglesias et al., 2018). This site is automethylated both in vitro and in vivo and impairs binding to substrates histone H3 and LSD1 in vitro (Piao et al., 2016). However, whether SUV39H2 automethylation plays the same regulatory role as Clr4 in mammalian cells has not been explored.
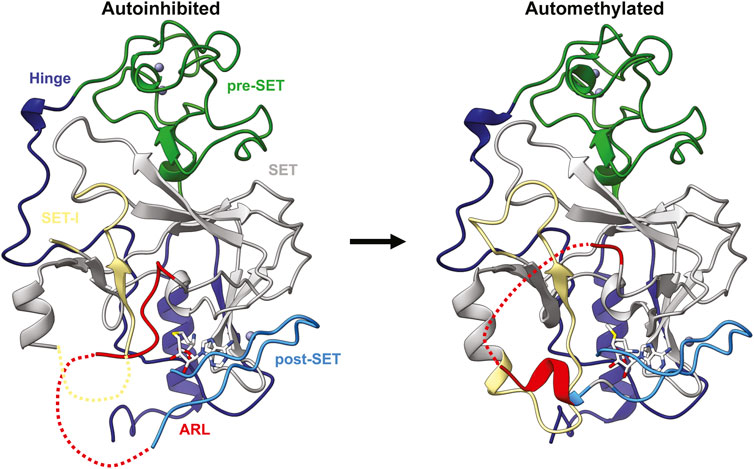
FIGURE 5. Clr4 automethylation-induced conformational switch. Structure of the autoinhibited (left; PDB ID 6BOX) and automethylated (right; PDB ID 6BP4) Clr4 catalytic domains with domains coloured the same as Figure 1 with the autoregulatory loop coloured red.
4.2.2 SUV39H1, SUV39H2, and Clr4 non-histone targets
Clr4 interacts with the RNA-induced transcriptional silencing (RITS) complex, a central component of the RNAi pathway in S. pombe, and the RNA processing and export factor Mlo3 (Zhang et al., 2008; Bayne et al., 2010; Zhang et al., 2011). These interactions bridge heterochromatin formation and RNA processing by channeling antisense and centromeric RNAs into the RNAi pathway. In addition to binding Mlo3, Clr4 also methylates Mlo3 on K167 (Zhang et al., 2011). Abrogating this methylation in vivo, by mutating K167 and adjacent K165 to alanine, impairs Mlo3 function causing a decrease in centromeric siRNA levels and weak upregulation of antisense RNAs (Zhang et al., 2011). Mlo3 methylation may influence the recognition of aberrant RNA by other factors, such as the RITS complex, however, this hypothesis has not been tested (Zhang et al., 2011). Ultimately, how Clr4-mediated methylation of Mlo3 contributes to Mlo3 function remains unclear.
Mammalian SUV39H1 and SUV39H2 also target a variety of non-histone proteins for methylation, specifically chromatin regulatory factors involved in reading, writing, or erasing alternate histone marks. SUV39H1 regulates the subnuclear localization of RAG2, an H3K4me3 reader that functions in variable diversity joining, or VDJ, recombination, via methylation of RAG2 K507 (Kudithipudi et al., 2017). Wild type and K507R mutant RAG2 both display speckled nuclear distribution when expressed in NIH3T3 cells (Kudithipudi et al., 2017). When coexpressed with SUV39H1, wild type RAG2 loses this focal clustering and becomes uniformly distributed throughout the nucleus, while the localization of K507R mutant RAG2 remains unchanged (Kudithipudi et al., 2017). Coexpression of wild type RAG2 with a catalytically inactive version of SUV39H1 also did not alter RAG2 distribution (Kudithipudi et al., 2017). This suggests that SUV39H1 methyltransferase activity may regulate RAG2-mediated VDJ recombination or its other functions.
In addition to H3K9me, other histone modifications are enriched in heterochromatin in mammals including H4K20me2, H4K20me3, H3K23me3, H3K56me3, and H3K64me3 (Schotta et al., 2004; Jack et al., 2013; Lange et al., 2013; Papazyan et al., 2014; Schwartz-Orbach et al., 2020). H4K20me2 and H4K20me3 are both produced by the methyltransferase SUV420H (Schotta et al., 2004). However, SUV420H requires the methyltransferase SET8 to deposit H4K20me1 first in order for the SUV420H enzymes to generate the two higher order methylation states, H4K20me2 and H4K20me3 (Schotta et al., 2004; Karachentsev et al., 2005; Schotta et al., 2008). SUV39H1 also contributes to H4K20 methylation by regulating SET8 activity (Kudithipudi et al., 2017). SUV39H1 methylates K210 of SET8 in vitro, which enhances SET8 methyltransferase activity (Kudithipudi et al., 2017). Thus, methylation of SET8 may represent another important contribution of SUV39H1 to heterochromatin formation in addition to producing H3K9me2 and H3K9me3.
LSD1 is a histone demethylase that promotes gene silencing by erasing the transcriptionally active H3K4me1 and H3K4me2 marks (Piao et al., 2015). SUV39H2 regulates this function by methylating LSD1 K322 (Piao et al., 2015). However, the mechanism of regulation differs from that of SET8 regulation by SUV39H1 methylation. Instead of enhancing enzyme activity, methylation of LSD1 by SUV39H2 increases its stability by inhibiting polyubiquitination and subsequent degradation by the proteasome (Piao et al., 2015). This is another example of non-histone target methylation by a SUV39-family enzyme that complements its role in heterochromatin function. In this case, SUV39H2 both deposits the silencing marks H3K9me2 and H3K9me3 and facilitate the removal of active marks, H3K4me1 and H3K4me2, by stabilizing the LSD1 demethylase (Piao et al., 2015).
4.2.3 G9a and GLP automethylation
Like SUV39H2 and Clr4, G9a and GLP also possess automethylation activity and automethylate motifs that resemble histone H3 at K185 and K239, for G9a, and K205, for GLP (Chin et al., 2007; Sampath et al., 2007; Poulard et al., 2017). Automethylation of these histone mimics creates a binding site for HP1 family proteins, which can be blocked by phosphorylation of the subsequent threonine, T186 in G9a and T206 in GLP, by the Aurora B kinase (Chin et al., 2007; Sampath et al., 2007; Poulard et al., 2017). This regulation is reminiscent of HP1 eviction from their H3K9me marked binding sites during mitosis by Aurora B mediated phosphorylation of H3S10 (Fischle et al., 2005). In addition to their roles in heterochromatin formation and gene silencing, G9a and GLP also form complexes that are responsible for gene activation (Poulard et al., 2018). The ternary complex of G9a or GLP with HP1γ and Glucocorticoid Receptor (GR) upregulates a subset of GR target genes (Poulard et al., 2018). Inhibition of JmjC family lysine demethylases, using the small molecule inhibitor JIB-04, increases HP1γ and GR complex formation and expression of GR target genes that are upregulated by G9a, GLP, and HP1γ likely due to enhanced G9a methylation stabilizing HP1γ binding (Poulard et al., 2018). A screen of lysine demethylases demonstrated that G9a automethylation can be removed by KDM4 in vitro (Poulard et al., 2018). Therefore G9a-HP1γ complex formation and its role in transcriptional regulation of GR target genes can be regulated dynamically by reversible methylation and phosphorylation.
4.2.4 G9a and GLP non-histone targets
Just like G9a automethylation produces a new binding site to recruit additional chromatin factors, so does G9a-mediated methylation of other non-histone targets. G9a methylates oestrogen receptor α (ERα), a nuclear hormone receptor that mediates the cells response to oestrogen, at K235 (Zhang et al., 2016). This methylation is recognized by the tandem tudor domain of PHF20, which recruits the MOF histone acetyltransferase complex to ERα target genes where it promotes transcription by depositing H4K16 acetylation (Zhang et al., 2016). DNA ligase 1 (LIG1) is methylated by G9a and GLP on a sequence that resembles histone H3K9 (Ferry et al., 2017). This creates a binding site for UHRF1, which recruits UHRF1 to replication sites (Ferry et al., 2017). Disruption of this interaction results in a significant reduction of DNA methylation in mouse embryonic stem cells (Ferry et al., 2017). ATF7IP is also methylated by G9a and GLP on a histone H3K9 mimic, which forms a binding site for the chromodomain of M-phase phosphoprotein 8 (MMP8), a component of the human silencing hub (HUSH) complex (Tsusaka et al., 2018). Expression of an unmethylatable mutant version of ATF7IP impairs SETDB11/MPP8-dependent silencing in a provirus reporter silencing assay (Tsusaka et al., 2018).
G9a also regulates several TFs through methylation. G9a methylates a conserved lysine (K39) in the transactivation domain of C/EBPβ, a basic leucine zipper TF that regulates tissue-specific gene expression, cell proliferation, and differentiation (Pless et al., 2008). C/EBPβ methylation suppresses its transcription activity (Pless et al., 2008). G9a also regulates a number of TFs involved in skeletal muscle differentiation. Myogenesis-promoting TF MyoD is methylated on K104, which inhibits transcription activity and suppresses myogenic differentiation (Ling et al., 2012). G9a mono- and dimethylates MEF2 on K267, which suppresses MEF2 transcription activity and downregulates genes important for myogenesis (Choi et al., 2014). G9a-mediated methylation of MEF2 has also been suggested to inhibit p38α-mediated phosphorylation of MEF2 at residues adjacent to K267 (Choi et al., 2014). Since phosphorylation of MEF2 by p38α promotes its transcription activity, G9a-mediated methylation may represent an inhibitory mechanism that impedes MEF2 phosphorylation to prevent aberrant gene activation.
Additionally, G9a methylates a variety of non-histone proteins that are involved in the hypoxia stress response pathway. Pontin is a chromatin remodeling factor involved in regulating the hypoxia response, which is methylated by G9a and GLP at several sites (Lee et al., 2011). Under normal conditions, Pontin displays low basal levels of methylation (Lee et al., 2011). Hypoxic conditions strongly induce methylation of Pontin in vivo and promote the expression of Pontin-dependent hypoxia target genes (Lee et al., 2011). A mutant version of Pontin with all lysine methylation sites replaced with alanine (K265A, K267A, K268A, K274A, K281A, and K285A) no longer displays hypoxia-induced methylation and fails to activate a subset of Pontin target genes (Lee et al., 2011). This Pontin mutant also impairs proliferation and migration within the MCF7 breast cancer cell line (Lee et al., 2011). G9a regulates another chromatin remodeling factor involved in the hypoxia stress response, called Reptin (Lee et al., 2010). G9a methylates Reptin at K67 under hypoxic conditions, which negatively regulates a subset of hypoxia response genes by suppressing HIF-1α transcriptional activity (Lee et al., 2010). HIF-1α, the master transcription regulator of the hypoxia response pathway, is also methylated by G9a at K674, which reduces downstream target gene activation (Bao et al., 2018). Combined, this reveals an important role for G9a and GLP non-histone target methylation in both positive and negative regulation of the hypoxia stress response pathway.
4.2.5 SETDB1 non-histone targets
SETDB1-mediated non-histone methylation regulates several important pathways that contribute to disease. AKT kinase regulates metabolism, cell proliferation, and survival and its hyperactivation plays an important role in tumorigenesis (Manning and Toker, 2017). K63-linked ubiquitination of AKT is essential for both activation and relocation of the enzyme to the cell membrane (Yang et al., 2009; Chan et al., 2012). SETDB1 di- and trimethylates AKT on a lysine that is adjacent to this ubiquitination site, K64 (Wang et al., 2019). This forms a binding site for JMJD2A, which in turn recruits E3 ligase resulting in ubiquitinated AKT (Wang et al., 2019). K64 methylation stimulates AKT activity, localization to the cell membrane, and its prosurvival function, which promotes tumorigenesis and correlates with poor prognosis in non-small cell lung cancer patients (Wang et al., 2019). SETDB1 also trimethylates K140 and K142 of AKT (Guo et al., 2019). These additional methylation sites bind the tudor domain of SETDB1 reinforcing its interaction with AKT, enhancing K64 methylation, and ultimately AKT activity (Guo et al., 2019).
The tumour suppressor, p53, regulates the cell cycle, apoptosis, and genome stability (Oren, 2003). It is frequently mutated in cancer where a number of gain-of-function (GOF) mutations have been identified (Brosh and Rotter, 2009). SETDB1 methylates wild type p53 and at least one of its GOF mutants, R249S, on K370 (Fei et al., 2015). P53R249S displays increased stability relative to wild type p53, which is partially dependent on SETDB1 (Fei et al., 2015). However, whether this increased stability is caused by methylated K370 or SETDB1 is unclear. SETDB1 binds more tightly to the GOF mutant relative to wild type p53 (Fei et al., 2015). Therefore, the increased stability of GOF p53 could be attributed to SETDB1 binding instead of its methyltransferase activity or potentially both contribute.
SETDB1 may also regulate HIV viral pathogenesis. SETDB1 binds and methylates the HIV-1 Tat protein on K50 and K51 (van Duyne et al., 2008). The Tat protein forms a ternary complex with Cdk9/cyclin T and the TAR RNA molecule, which is disrupted by K50 and K51 methylation (van Duyne et al., 2008). Knockdown of SETDB1 increases activation of HIV-1 LTRs in two reporter systems and enhanced reverse transcriptase activity in vivo (van Duyne et al., 2008). However, the in vivo role K50 and K51 methylation plays in HIV pathogenesis remains to be tested.
5 Conclusion
The studies discussed here highlight the growing repertoire of genomic, histone, and non-histone targets of H3K9 methyltransferases. This has expanded the role of these methyltransferases beyond the maintenance of genome stability and the formation of constitutive heterochromatin. However, further investigation is needed to understand how these novel methylation events are regulated, when they are used by the cell, and how they integrate with the other functions of these enzymes. How H3K9 methyltransferases are recruited to sites of constitutive heterochromatin has been studied extensively. However, how H3K9me is selectively deposited and removed from protein-coding genes at different stages of development is not fully understood. Moreover, the molecular mechanisms that trigger and turn over non-histone methylation and automethylation also remain largely unknown. What we have summarized here likely only scratches the surface. A complete methylproteome for these methyltransferases will be required to appreciate the full scope of cellular, developmental, and pathological processes regulated by H3K9 methyltransferases.
Author contributions
AJL, GM, and NS contributed equally to this work. AJL, GM, and MAC made the table. All authors participated in the writing, editing, and preparation of figures for the manuscript.
Funding
This work was supported by the Canadian Foundation for Innovation - John R. Evans Leaders Fund, Ontario Research Fund (CFI/ORF project no. 40684), Research and Scholarly Activity Fund, and Connaught New Researcher Award funding to MAC. GM is supported by an Ontario Graduate Scholarship.
Acknowledgments
The authors apologize to all of those whose articles could not be cited due to space limitations. The authors would like to thank Antonis Tatarakis (Harvard Medical School) for reviewing and commenting on the manuscript.
Conflict of interest
The authors declare that the research was conducted in the absence of any commercial or financial relationships that could be construed as a potential conflict of interest.
Publisher’s note
All claims expressed in this article are solely those of the authors and do not necessarily represent those of their affiliated organizations, or those of the publisher, the editors and the reviewers. Any product that may be evaluated in this article, or claim that may be made by its manufacturer, is not guaranteed or endorsed by the publisher.
References
Adoue, V., Binet, B., Malbec, A., Fourquet, J., Romagnoli, P., van Meerwijk, J. P. M., et al. (2019). The histone methyltransferase SETDB1 controls T helper cell lineage integrity by repressing endogenous retroviruses. Immunity 50, 629–644. doi:10.1016/j.immuni.2019.01.003
Ahringer, J., and Gasser, S. M. (2018). Repressive chromatin in Caenorhabditis elegans: Establishment, composition, and function. Genetics 208, 491–511. doi:10.1534/genetics.117.300386
Akoury, E., Ma, G., Demolin, S., Bronner, C., Zocco, M., Cirilo, A., et al. (2019). Disordered region of H3K9 methyltransferase Clr4 binds the nucleosome and contributes to its activity. Nucleic Acids Res. 47, 6726–6736. doi:10.1093/nar/gkz480
Allan, R. S., Zueva, E., Cammas, F., Schreiber, H. A., Masson, V., Belz, G. T., et al. (2012). An epigenetic silencing pathway controlling T helper 2 cell lineage commitment. Nature 487, 249–253. doi:10.1038/nature11173
Allis, C. D., and Jenuwein, T. (2016). The molecular hallmarks of epigenetic control. Nat. Rev. Genet. 17, 487–500. doi:10.1038/nrg.2016.59
Allshire, R. C., and Madhani, H. D. (2018). Ten principles of heterochromatin formation and function. Nat. Rev. Mol. Cell Biol. 19, 229–244. doi:10.1038/nrm.2017.119
Apweiler, R., Bairoch, A., Wu, C. H., Barker, W. C., Boeckmann, B., Ferro, S., et al. (2004). UniProt: The universal protein knowledgebase. Nucleic Acids Res. 32, D115–D119. doi:10.1093/nar/gkh131
Arora, R., Lee, Y., Wischnewski, H., Brun, C. M., Schwarz, T., and Azzalin, C. M. (2014). RNaseH1 regulates TERRA-telomeric DNA hybrids and telomere maintenance in ALT tumour cells. Nat. Commun. 5, 5220. doi:10.1038/ncomms6220
Bae, N. S., and Baumann, P. (2007). A RAP1/TRF2 complex inhibits nonhomologous end-joining at human telomeric DNA ends. Mol. Cell 26, 323–334. doi:10.1016/j.molcel.2007.03.023
Bao, L., Chen, Y., Lai, H. T., Wu, S. Y., Wang, J. E., Hatanpaa, K. J., et al. (2018). Methylation of hypoxia-inducible factor (HIF)-1α by G9a/GLP inhibits HIF-1 transcriptional activity and cell migration. Nucleic Acids Res. 46, 6576–6591. doi:10.1093/nar/gky449
Barral, A., and Déjardin, J. (2020). Telomeric chromatin and TERRA. J. Mol. Biol. 432, 4244–4256. doi:10.1016/j.jmb.2020.03.003
Bayne, E. H., White, S. A., Kagansky, A., Bijos, D. A., Sanchez-Pulido, L., Hoe, K. L., et al. (2010). Stc1: A critical link between RNAi and chromatin modification required for heterochromatin integrity. Cell 140, 666–677. doi:10.1016/j.cell.2010.01.038
Becker, J. S., McCarthy, R. L., Sidoli, S., Donahue, G., Kaeding, K. E., He, Z., et al. (2017). Genomic and proteomic resolution of heterochromatin and its restriction of alternate fate genes. Mol. Cell 68, 1023–1037. doi:10.1016/j.molcel.2017.11.030
Becker, J. S., Nicetto, D., and Zaret, K. S. (2016). H3K9me3-Dependent heterochromatin: Barrier to cell fate changes. Trends Genet. 32, 29–41. Preprint at . doi:10.1016/j.tig.2015.11.001
Bernard, P., Maure, J. F., Partridge, J. F., Genier, S., Javerzat, J. P., and Allshire, R. C. (2001). Requirement of heterochromatin for cohesion at centromeres. Science 294, 2539–2542. doi:10.1126/science.1064027
Beyer, S., Pontis, J., Schirwis, E., Battisti, V., Rudolf, A., Le Grand, F., et al. (2016). Erratum: Canonical Wnt signalling regulates nuclear export of Setdb1 during skeletal muscle terminal differentiation. Cell Discov. 2, 16043. doi:10.1038/celldisc.2016.43
Bilodeau, S., Kagey, M. H., Frampton, G. M., Rahl, P. B., and Young, R. A. (2009). SetDB1 contributes to repression of genes encoding developmental regulators and maintenance of ES cell state. Genes Dev. 23, 2484–2489. doi:10.1101/gad.1837309
Black, J. C., van Rechem, C., and Whetstine, J. R. (2012). Histone lysine methylation dynamics: Establishment, regulation, and biological impact. Mol. Cell 48, 491–507. doi:10.1016/j.molcel.2012.11.006
Blower, M. D., Sullivan, B. A., and Karpen, G. H. (2002). Conserved organization of centromeric chromatin in flies and humans. Dev. Cell 2, 319–330. doi:10.1016/s1534-5807(02)00135-1
Brachner, A., and Foisner, R. (2011). Evolvement of LEM proteins as chromatin tethers at the nuclear periphery. Biochem. Soc. Trans. 39, 1735–1741. doi:10.1042/BST20110724
Brockers, K., and Schneider, R. (2019). Histone H1, the forgotten histone. Epigenomics 11 363, 363–366. –366 Preprint at. doi:10.2217/epi-2019-0018
Brosh, R., and Rotter, V. (2009). When mutants gain new powers: News from the mutant p53 field. Nat. Rev. Cancer 9, 701–713. doi:10.1038/nrc2693
Brumbaugh, J., Kim, I. S., Ji, F., Huebner, A. J., Di Stefano, B., Schwarz, B. A., et al. (2019). Inducible histone K-to-M mutations are dynamic tools to probe the physiological role of site-specific histone methylation in vitro and in vivo. Nat. Cell Biol. 21, 1449–1461. doi:10.1038/s41556-019-0403-5
Bulut-Karslioglu, A., De La Rosa-Velazquez, I. A., Ramirez, F., Barenboim, M., Onishi-Seebacher, M., Arand, J., et al. (2014). Suv39h-dependent H3K9me3 marks intact retrotransposons and silences LINE elements in mouse embryonic stem cells. Mol. Cell 55, 277–290. doi:10.1016/j.molcel.2014.05.029
Chan, C.-H., Li, C. F., Yang, W. L., Gao, Y., Lee, S. W., Feng, Z., et al. (2012). The Skp2-SCF E3 ligase regulates Akt ubiquitination, glycolysis, herceptin sensitivity, and tumorigenesis. Cell 149, 1098–1111. doi:10.1016/j.cell.2012.02.065
Chelmicki, T., Roger, E., Teissandier, A., Dura, M., Bonneville, L., Rucli, S., et al. (2021). m(6 A RNA methylation regulates the fate of endogenous retroviruses. Nature 591, 312–316. doi:10.1038/s41586-020-03135-1
Chen, K., Zhang, F., Ding, J., Liang, Y., Zhan, Z., Zhan, Y., et al. (2017). Histone methyltransferase SETDB1 promotes the progression of colorectal cancer by inhibiting the expression of TP53. J. Cancer 8, 3318–3330. doi:10.7150/jca.20482
Chen, X., Skutt-Kakaria, K., Davison, J., Ou, Y. L., Choi, E., Malik, P., et al. (2012). G9a/GLP-dependent histone H3K9me2 patterning during human hematopoietic stem cell lineage commitment. Genes Dev. 26, 2499–2511. doi:10.1101/gad.200329.112
Chin, H. G., Esteve, P. O., Pradhan, M., Benner, J., Patnaik, D., Carey, M. F., et al. (2007). Automethylation of G9a and its implication in wider substrate specificity and HP1 binding. Nucleic Acids Res. 35, 7313–7323. doi:10.1093/nar/gkm726
Choi, J., Jang, H., Kim, H., Lee, J. H., Kim, S. T., Cho, E. J., et al. (2014). Modulation of lysine methylation in myocyte enhancer factor 2 during skeletal muscle cell differentiation. Nucleic Acids Res. 42, 224–234. doi:10.1093/nar/gkt873
Collins, P. L., Kyle, K. E., Egawa, T., Shinkai, Y., and Oltz, E. M. (2015). The histone methyltransferase SETDB1 represses endogenous and exogenous retroviruses in B lymphocytes. Proc. Natl. Acad. Sci. U. S. A. 112, 8367–8372. doi:10.1073/pnas.1422187112
Collins, R. E., Northrop, J. P., Horton, J. R., Lee, D. Y., Zhang, X., Stallcup, M. R., et al. (2008). The ankyrin repeats of G9a and GLP histone methyltransferases are mono- and dimethyllysine binding modules. Nat. Struct. Mol. Biol. 15, 245–250. doi:10.1038/nsmb.1384
Collins, R. E., Tachibana, M., Tamaru, H., Smith, K. M., Jia, D., Zhang, X., et al. (2005). In vitro and in vivo analyses of a Phe/Tyr switch controlling product specificity of histone lysine methyltransferases. J. Biol. Chem. 280, 5563–5570. doi:10.1074/jbc.M410483200
Cordaux, R., and Batzer, M. A. (2009). The impact of retrotransposons on human genome evolution. Nat. Rev. Genet. 10, 691–703. doi:10.1038/nrg2640
Couture, J.-F., Dirk, L. M. A., Brunzelle, J. S., Houtz, R. L., and Trievel, R. C. (2008). Structural origins for the product specificity of SET domain protein methyltransferases. Proc. Natl. Acad. Sci. U. S. A. 105, 20659–20664. doi:10.1073/pnas.0806712105
Crasta, K., Ganem, N. J., Dagher, R., Lantermann, A. B., Ivanova, E. V., Pan, Y., et al. (2012). DNA breaks and chromosome pulverization from errors in mitosis. Nature 482, 53–58. doi:10.1038/nature10802
Cubiles, M. D., Barroso, S., Vaquero-Sedas, M. I., Enguix, A., Aguilera, A., and Vega-Palas, M. A. (2018). Epigenetic features of human telomeres. Nucleic Acids Res. 46, 2347–2355. doi:10.1093/nar/gky006
Czermin, B., Schotta, G., Hulsmann, B. B., Brehm, A., Becker, P. B., Reuter, G., et al. (2001). Physical and functional association of SU(VAR)3-9 and HDAC1 in Drosophila. EMBO Rep. 2, 915–919. doi:10.1093/embo-reports/kve210
de Lange, T. (2018). Shelterin-mediated telomere protection. Annu. Rev. Genet. 52, 223–247. doi:10.1146/annurev-genet-032918-021921
Déjardin, J., and Kingston, R. E. (2009). Purification of proteins associated with specific genomic Loci. Cell 136, 175–186. doi:10.1016/j.cell.2008.11.045
Delaney, C. E., Methot, S. P., Guidi, M., Katic, I., Gasser, S. M., and Padeken, J. (2019). Heterochromatic foci and transcriptional repression by an unstructured MET-2/SETDB1 co-factor LIN-65. J. Cell Biol. 218, 820–838. doi:10.1083/jcb.201811038
Denchi, E. L., and de Lange, T. (2007). Protection of telomeres through independent control of ATM and ATR by TRF2 and POT1. Nature 448, 1068–1071. doi:10.1038/nature06065
di Giacomo, M., Comazzetto, S., Sampath, S. C., Sampath, S. C., and O’Carroll, D. (2014). G9a co-suppresses LINE1 elements in spermatogonia. Epigenetics Chromatin 7, 24. doi:10.1186/1756-8935-7-24
Dillon, S. C., Zhang, X., Trievel, R. C., and Cheng, X. (2005). The SET-domain protein superfamily: Protein lysine methyltransferases. Genome Biol. 6, 227. doi:10.1186/gb-2005-6-8-227
Dodge, J. E., Kang, Y.-K., Beppu, H., Lei, H., and Li, E. (2004). Histone H3-K9 methyltransferase ESET is essential for early development. Mol. Cell. Biol. 24, 2478–2486. doi:10.1128/mcb.24.6.2478-2486.2004
Dunham, M. A., Neumann, A. A., Fasching, C. L., and Reddel, R. R. (2000). Telomere maintenance by recombination in human cells. Nat. Genet. 26, 447–450. doi:10.1038/82586
Ekwall, K., Javerzat, J. P., Lorentz, A., ScHmidt, H., Cranston, G., and AllshiRe, R. (1995). The chromodomain protein Swi6: A key component at fission yeast centromeres. Science 269, 1429–1431. doi:10.1126/science.7660126
Ekwall, K., Nimmo, E. R., Javerzat, J. P., Borgstrom, B., Egel, R., Cranston, G., et al. (1996). Mutations in the fission yeast silencing factors clr4+ and rik1+ disrupt the localisation of the chromo domain protein Swi6p and impair centromere function. J. Cell Sci. 109 (1), 2637–2648. doi:10.1242/jcs.109.11.2637
Elgin, S. C. R., and Reuter, G. (2013). Position-effect variegation, heterochromatin formation, and gene silencing in Drosophila. Cold Spring Harb. Perspect. Biol. 5, a017780. doi:10.1101/cshperspect.a017780
Epsztejn-Litman, S., Feldman, N., Abu-Remaileh, M., Shufaro, Y., Gerson, A., Ueda, J., et al. (2008). De novo DNA methylation promoted by G9a prevents reprogramming of embryonically silenced genes. Nat. Struct. Mol. Biol. 15, 1176–1183. doi:10.1038/nsmb.1476
Erdmann, R. M., and Picard, C. L. (2020). RNA-Directed DNA methylation. PLoS Genet. 16, e1009034. doi:10.1371/journal.pgen.1009034
Fabre, F. (1972). Relation between repair mechanisms and induced mitotic recombination after UV irradiation, in the yeast Schizosaccharomyces pombe. Effects of caffeine. Mol. Gen. Genet. 117, 153–166. doi:10.1007/BF00267612
Fang, D., Gan, H., Lee, J. H., Han, J., Wang, Z., Riester, S. M., et al. (2016). The histone H3.3K36M mutation reprograms the epigenome of chondroblastomas. Science 352, 1344–1348. doi:10.1126/science.aae0065
Fasching, L., Kapopoulou, A., Sachdeva, R., Petri, R., Jonsson, M. E., Manne, C., et al. (2015). TRIM28 represses transcription of endogenous retroviruses in neural progenitor cells. Cell Rep. 10, 20–28. doi:10.1016/j.celrep.2014.12.004
Fei, Q., Shang, K., Zhang, J., Chuai, S., Kong, D., Zhou, T., et al. (2015). Histone methyltransferase SETDB1 regulates liver cancer cell growth through methylation of p53. Nat. Commun. 6, 8651. doi:10.1038/ncomms9651
Feldman, N., Gerson, A., Fang, J., Li, E., Zhang, Y., Shinkai, Y., et al. (2006). G9a-mediated irreversible epigenetic inactivation of Oct-3/4 during early embryogenesis. Nat. Cell Biol. 8, 188–194. doi:10.1038/ncb1353
Ferry, L., Fournier, A., Tsusaka, T., Adelmant, G., Shimazu, T., Matano, S., et al. (2017). Methylation of DNA ligase 1 by G9a/GLP recruits UHRF1 to replicating DNA and regulates DNA methylation. Mol. Cell 67, 550e5–565. doi:10.1016/j.molcel.2017.07.012
Fischle, W., Tseng, B. S., Dormann, H. L., Ueberheide, B. M., Garcia, B. A., Shabanowitz, J., et al. (2005). Regulation of HP1-chromatin binding by histone H3 methylation and phosphorylation. Nature 438, 1116–1122. doi:10.1038/nature04219
Fiszbein, A., Giono, L. E., Quaglino, A., Berardino, B. G., Sigaut, L., von Bilderling, C., et al. (2016). Alternative splicing of G9a regulates neuronal differentiation. Cell Rep. 14, 2797–2808. doi:10.1016/j.celrep.2016.02.063
Folco, H. D., Pidoux, A. L., Urano, T., and Allshire, R. C. (2008). Heterochromatin and RNAi are required to establish CENP-A chromatin at centromeres. Science 319, 94–97. doi:10.1126/science.1150944
Fritsch, L., Robin, P., Mathieu, J. R. R., Souidi, M., Hinaux, H., Rougeulle, C., et al. (2010). A subset of the histone H3 lysine 9 methyltransferases Suv39h1, G9a, GLP, and SETDB1 participate in a multimeric complex. Mol. Cell 37, 46–56. doi:10.1016/j.molcel.2009.12.017
Funabiki, H., Hagan, I., Uzawa, S., and Yanagida, M. (1993). Cell cycle-dependent specific positioning and clustering of centromeres and telomeres in fission yeast. J. Cell Biol. 121, 961–976. doi:10.1083/jcb.121.5.961
García-Cao, M., O’Sullivan, R., Peters, A. H. F. M., Jenuwein, T., and Blasco, M. A. (2004). Epigenetic regulation of telomere length in mammalian cells by the Suv39h1 and Suv39h2 histone methyltransferases. Nat. Genet. 36, 94–99. doi:10.1038/ng1278
Gauchier, M., Kan, S., Barral, A., Sauzet, S., Agirre, E., Bonnell, E., et al. (2019). SETDB1-dependent heterochromatin stimulates alternative lengthening of telomeres. Sci. Adv. 5, eaav3673. doi:10.1126/sciadv.aav3673
Gentner, N. E., and Werner, M. M. (1975). Repair in Schizosaccharomyces pombe as measured by recovery from caffeine enhancement of radiation-induced lethality. Mol. Gen. Genet. 142, 171–183. doi:10.1007/BF00425643
Guelen, L., Pagie, L., Brasset, E., Meuleman, W., Faza, M. B., Talhout, W., et al. (2008). Domain organization of human chromosomes revealed by mapping of nuclear lamina interactions. Nature 453, 948–951. doi:10.1038/nature06947
Guerra, M. v., Caceres, M. I., Herrera-Soto, A., Arredondo, S. B., Varas-Godoy, M., van Zundert, B., et al. (2021). H3K9 methyltransferases Suv39h1 and Suv39h2 control the differentiation of neural progenitor cells in the adult Hippocampus. Front. Cell Dev. Biol. 9, 778345. doi:10.3389/fcell.2021.778345
Guo, J., Dai, X., Laurent, B., Zheng, N., Gan, W., Zhang, J., et al. (2019). AKT methylation by SETDB1 promotes AKT kinase activity and oncogenic functions. Nat. Cell Biol. 21, 226–237. doi:10.1038/s41556-018-0261-6
Herz, H.-M., Morgan, M., Gao, X., Jackson, J., Rickels, R., Swanson, S. K., et al. (2014). Histone H3 lysine-to-methionine mutants as a paradigm to study chromatin signaling. Science 345, 1065–1070. doi:10.1126/science.1255104
Holoch, D., and Moazed, D. (2015). RNA-mediated epigenetic regulation of gene expression. Nat. Rev. Genet. 16, 71–84. doi:10.1038/nrg3863
Horsley, D., Hutchings, A., Butcher, G. W., and Singh, P. B. (1996). M32, a murine homologue of Drosophila heterochromatin protein 1 (HP1), localises to euchromatin within interphase nuclei and is largely excluded from constitutive heterochromatin. Cytogenet. Cell Genet. 73, 308–311. doi:10.1159/000134363
Husmann, D., and Gozani, O. (2019). Histone lysine methyltransferases in biology and disease. Nat. Struct. Mol. Biol. 26, 880–889. doi:10.1038/s41594-019-0298-7
Hyun, K., Jeon, J., Park, K., and Kim, J. Writing (2017). Writing, erasing and reading histone lysine methylations. Exp. Mol. Med. 49, e324. doi:10.1038/emm.2017.11
Iglesias, N., Currie, M. A., Jih, G., Paulo, J. A., Siuti, N., Kalocsay, M., et al. (2018). Automethylation-induced conformational switch in Clr4 (Suv39h) maintains epigenetic stability. Nature 560, 504–508. doi:10.1038/s41586-018-0398-2
Ikegami, K., Egelhofer, T. A., Strome, S., and Lieb, J. D. (2010). Caenorhabditis elegans chromosome arms are anchored to the nuclear membrane via discontinuous association with LEM-2. Genome Biol. 11, R120. doi:10.1186/gb-2010-11-12-r120
Ishimoto, K., Kawamata, N., Uchihara, Y., Okubo, M., Fujimoto, R., Gotoh, E., et al. (2016). Ubiquitination of lysine 867 of the human SETDB1 protein upregulates its histone H3 lysine 9 (H3K9) methyltransferase activity. PLoS One 11, e0165766. doi:10.1371/journal.pone.0165766
Jack, A. P. M., Bussemer, S., Hahn, M., Punzeler, S., Snyder, M., Wells, M., et al. (2013). H3K56me3 is a novel, conserved heterochromatic mark that largely but not completely overlaps with H3K9me3 in both regulation and localization. PLoS One 8, e51765. doi:10.1371/journal.pone.0051765
Jacobs, S. A., and Khorasanizadeh, S. (2002). Structure of HP1 chromodomain bound to a lysine 9-methylated histone H3 tail. Science 295, 2080–2083. doi:10.1126/science.1069473
Janssen, A., Colmenares, S. U., and Karpen, G. H. (2018). Heterochromatin: Guardian of the genome. Annu. Rev. Cell Dev. Biol. 34, 265–288. Preprint at. doi:10.1146/annurev-cellbio-100617-062653
Janssen, A., van der Burg, M., Szuhai, K., Kops, G. J. P. L., and Medema, R. H. (2011). Chromosome segregation errors as a cause of DNA damage and structural chromosome aberrations. Science 333, 1895–1898. doi:10.1126/science.1210214
Jayaram, H., Hoelper, D., Jain, S. U., Cantone, N., Lundgren, S. M., Poy, F., et al. (2016). S-adenosyl methionine is necessary for inhibition of the methyltransferase G9a by the lysine 9 to methionine mutation on histone H3. Proc. Natl. Acad. Sci. U. S. A. 113, 6182–6187. doi:10.1073/pnas.1605523113
Jenuwein, T., Laible, G., Dorn, R., and Reuter, G. (1998). SET domain proteins modulate chromatin domains in eu- and heterochromatin. Cell. Mol. Life Sci. 54, 80–93. doi:10.1007/s000180050127
Jia, S., Noma, K., and Grewal, S. I. S. (2004). RNAi-independent heterochromatin nucleation by the stress-activated ATF/CREB family proteins. Science 304, 1971–1976. doi:10.1126/science.1099035
Jiang, Q., Lee, A. Y., Cao, Q., Li, K. Y., Yip, K. Y., and Leung, D. C. Y. (2020). G9a plays distinct roles in maintaining DNA methylation, retrotransposon silencing, and chromatin looping. Cell Rep. 33, 108315. doi:10.1016/j.celrep.2020.108315
Jiang, Y., Loh, Y. H. E., Rajarajan, P., Hirayama, T., Liao, W., Kassim, B. S., et al. (2017). The methyltransferase SETDB1 regulates a large neuron-specific topological chromatin domain. Nat. Genet. 49, 1239–1250. doi:10.1038/ng.3906
Jiao, L., and Liu, X. (2015). Structural basis of histone H3K27 trimethylation by an active polycomb repressive complex 2. Science 350, aac4383. doi:10.1126/science.aac4383
Johnson, W. L., Yewdell, W. T., Bell, J. C., McNulty, S. M., Duda, Z., O'Neill, R. J., et al. (2017). RNA-dependent stabilization of SUV39H1 at constitutive heterochromatin. Elife 6, e25299. doi:10.7554/eLife.25299
Jones, R. S., and Gelbart, W. M. (1993). The Drosophila Polycomb-group gene Enhancer of zeste contains a region with sequence similarity to trithorax. Mol. Cell. Biol. 13, 6357–6366. doi:10.1128/mcb.13.10.6357
Jurkowska, R. Z., Qin, S., Kungulovski, G., Tempel, W., Liu, Y., Bashtrykov, P., et al. (2017). H3K14ac is linked to methylation of H3K9 by the triple Tudor domain of SETDB1. Nat. Commun. 8, 2057. doi:10.1038/s41467-017-02259-9
Južnić, L., Peuker, K., Strigli, A., Brosch, M., Herrmann, A., Hasler, R., et al. (2021). SETDB1 is required for intestinal epithelial differentiation and the prevention of intestinal inflammation. Gut 70, 485–498. doi:10.1136/gutjnl-2020-321339
Karachentsev, D., Sarma, K., Reinberg, D., and Steward, R. (2005). PR-Set7-dependent methylation of histone H4 Lys 20 functions in repression of gene expression and is essential for mitosis. Genes Dev. 19, 431–435. doi:10.1101/gad.1263005
Karimi, M. M., Goyal, P., Maksakova, I. A., Bilenky, M., Leung, D., Tang, J. X., et al. (2011). DNA methylation and SETDB1/H3K9me3 regulate predominantly distinct sets of genes, retroelements, and chimeric transcripts in mESCs. Cell Stem Cell 8, 676–687. doi:10.1016/j.stem.2011.04.004
Karlseder, J., Broccoli, D., Dai, Y., Hardy, S., and de Lange, T. (1999). p53- and ATM-dependent apoptosis induced by telomeres lacking TRF2. Science 283, 1321–1325. doi:10.1126/science.283.5406.1321
Karlseder, J., Hoke, K., Mirzoeva, O. K., Bakkenist, C., Kastan, M. B., Petrini, J. H. J., et al. (2004). The telomeric protein TRF2 binds the ATM kinase and can inhibit the ATM-dependent DNA damage response. PLoS Biol. 2, E240. doi:10.1371/journal.pbio.0020240
Kato, M., Takemoto, K., and Shinkai, Y. (2018). A somatic role for the histone methyltransferase Setdb1 in endogenous retrovirus silencing. Nat. Commun. 9, 1683. doi:10.1038/s41467-018-04132-9
Kelleher, C., Kurth, I., and Lingner, J. (2005). Human protection of telomeres 1 (POT1) is a negative regulator of telomerase activity in vitro. Mol. Cell. Biol. 25, 808–818. doi:10.1128/MCB.25.2.808-818.2005
Kojima, K. K. (2018). Human transposable elements in repbase: Genomic footprints from fish to humans. Mob. DNA 9, 2. doi:10.1186/s13100-017-0107-y
Kornberg, R. D. (1974). Chromatin structure: A repeating unit of histones and DNA. Science 184, 868–871. doi:10.1126/science.184.4139.868
Kudithipudi, S., Schuhmacher, M. K., Kebede, A. F., and Jeltsch, A. (2017). The SUV39H1 protein lysine methyltransferase methylates chromatin proteins involved in heterochromatin formation and VDJ recombination. ACS Chem. Biol. 12, 958–968. doi:10.1021/acschembio.6b01076
Lander, E. S., Linton, L. M., Birren, B., Nusbaum, C., Zody, M. C., Baldwin, J., et al. (2001). Initial sequencing and analysis of the human genome. Nature 409, 860–921. doi:10.1038/35057062
Lange, U. C., Siebert, S., Wossidlo, M., Weiss, T., Ziegler-Birling, C., Walter, J., et al. (2013). Dissecting the role of H3K64me3 in mouse pericentromeric heterochromatin. Nat. Commun. 4, 2233. doi:10.1038/ncomms3233
Larson, A. G., Elnatan, D., Keenen, M. M., Trnka, M. J., Johnston, J. B., Burlingame, A. L., et al. (2017). Liquid droplet formation by HP1α suggests a role for phase separation in heterochromatin. Nature 547, 236–240. doi:10.1038/nature22822
Lee, J. S., Kim, Y., Bhin, J., Shin, H. J. R., Nam, H. J., Lee, S. H., et al. (2011). Hypoxia-induced methylation of a pontin chromatin remodeling factor. Proc. Natl. Acad. Sci. U. S. A. 108, 13510–13515. doi:10.1073/pnas.1106106108
Lee, J. S., Kim, Y., Kim, I. S., Kim, B., Choi, H. J., Lee, J. M., et al. (2010). Negative regulation of hypoxic responses via induced Reptin methylation. Mol. Cell 39, 71–85. doi:10.1016/j.molcel.2010.06.008
Lewis, P. W., Muller, M. M., Koletsky, M. S., Cordero, F., Lin, S., Banaszynski, L. A., et al. (2013). Inhibition of PRC2 activity by a gain-of-function H3 mutation found in pediatric glioblastoma. Science 340, 857–861. doi:10.1126/science.1232245
Li, B., and de Lange, T. (2003). Rap1 affects the length and heterogeneity of human telomeres. Mol. Biol. Cell 14, 5060–5068. doi:10.1091/mbc.e03-06-0403
Li, H., Rauch, T., Chen, Z. X., Szabo, P. E., Riggs, A. D., and Pfeifer, G. P. (2006). The histone methyltransferase SETDB1 and the DNA methyltransferase DNMT3A interact directly and localize to promoters silenced in cancer cells. J. Biol. Chem. 281, 19489–19500. doi:10.1074/jbc.M513249200
Li, Y., Han, J., Zhang, Y., Cao, F., Liu, Z., Li, S., et al. (2016). Structural basis for activity regulation of MLL family methyltransferases. Nature 530, 447–452. doi:10.1038/nature16952
Ling, B. M. T., Bharathy, N., Chung, T. K., Kok, W. K., Li, S., Tan, Y. H., et al. (2012). Lysine methyltransferase G9a methylates the transcription factor MyoD and regulates skeletal muscle differentiation. Proc. Natl. Acad. Sci. U. S. A. 109, 841–846. doi:10.1073/pnas.1111628109
Liu, H., Kim, J.-M., and Aoki, F. (2004). Regulation of histone H3 lysine 9 methylation in oocytes and early pre-implantation embryos. Development 131, 2269–2280. doi:10.1242/dev.01116
Liu, J., Gao, M., He, J., Wu, K., Lin, S., Jin, L., et al. (2021). The RNA m(6)A reader YTHDC1 silences retrotransposons and guards ES cell identity. Nature 591, 322–326. doi:10.1038/s41586-021-03313-9
Liu, J., Magri, L., Zhang, F., Marsh, N. O., Albrecht, S., Huynh, J. L., et al. (2015). Chromatin landscape defined by repressive histone methylation during oligodendrocyte differentiation. J. Neurosci. 35, 352–365. doi:10.1523/JNEUROSCI.2606-14.2015
Liu, S., Brind'Amour, J., Karimi, M. M., Shirane, K., Bogutz, A., Lefebvre, L., et al. (2014). Setdb1 is required for germline development and silencing of H3K9me3-marked endogenous retroviruses in primordial germ cells. Genes Dev. 28, 2041–2055. doi:10.1101/gad.244848.114
Liu, Z., Cai, Y., Wang, Y., Nie, Y., Zhang, C., Xu, Y., et al. (2018). Cloning of macaque monkeys by somatic cell nuclear transfer. Cell 172, 881e7–887. doi:10.1016/j.cell.2018.01.020
Lohmann, F., Loureiro, J., Su, H., Fang, Q., Lei, H., Lewis, T., et al. (2010). KMT1E mediated H3K9 methylation is required for the maintenance of embryonic stem cells by repressing trophectoderm differentiation. Stem Cells 28, 201–212. doi:10.1002/stem.278
Loprieno, N., Barale, R., and Baroncelli, S. (1974). Genetic effects of caffeine. Mutat. Res. 26, 83–87. doi:10.1016/s0027-5107(74)80038-2
Lovejoy, C. A., Li, W., Reisenweber, S., Thongthip, S., Bruno, J., de Lange, T., et al. (2012). Loss of ATRX, genome instability, and an altered DNA damage response are hallmarks of the alternative lengthening of telomeres pathway. PLoS Genet. 8, e1002772. doi:10.1371/journal.pgen.1002772
Lu, C., Jain, S. U., Hoelper, D., Bechet, D., Molden, R. C., Ran, L., et al. (2016). Histone H3K36 mutations promote sarcomagenesis through altered histone methylation landscape. Science 352, 844–849. doi:10.1126/science.aac7272
Luger, K., Mäder, A. W., Richmond, R. K., Sargent, D. F., and Richmond, T. J. (1997). Crystal structure of the nucleosome core particle at 2.8 Å resolution. Nature 389, 251–260. doi:10.1038/38444
Maksakova, I. A., Thompson, P. J., Goyal, P., Jones, S. J., Singh, P. B., Karimi, M. M., et al. (2013). Distinct roles of KAP1, HP1 and G9a/GLP in silencing of the two-cell-specific retrotransposon MERVL in mouse ES cells. Epigenetics Chromatin 6, 15. doi:10.1186/1756-8935-6-15
Manning, B. D., and Toker, A. (2017). AKT/PKB signaling: Navigating the network. Cell 169, 381–405. doi:10.1016/j.cell.2017.04.001
Manzur, K. L., and Zhou, M.-M. (2005). An archaeal SET domain protein exhibits distinct lysine methyltransferase activity towards DNA-associated protein MC1-alpha. FEBS Lett. 579, 3859–3865. doi:10.1016/j.febslet.2005.05.026
Marmorstein, R. (2003). Structure of SET domain proteins: A new twist on histone methylation. Trends biochem. Sci. 28, 59–62. doi:10.1016/S0968-0004(03)00007-0
Martens, J. H. A., O'Sullivan, R. J., Braunschweig, U., Opravil, S., Radolf, M., Steinlein, P., et al. (2005). The profile of repeat-associated histone lysine methylation states in the mouse epigenome. EMBO J. 24, 800–812. doi:10.1038/sj.emboj.7600545
Matoba, S., Liu, Y., Lu, F., Iwabuchi, K. A., Shen, L., Inoue, A., et al. (2014). Embryonic development following somatic cell nuclear transfer impeded by persisting histone methylation. Cell 159, 884–895. doi:10.1016/j.cell.2014.09.055
Matsui, T., Leung, D., Miyashita, H., Maksakova, I. A., Miyachi, H., Kimura, H., et al. (2010). Proviral silencing in embryonic stem cells requires the histone methyltransferase ESET. Nature 464, 927–931. doi:10.1038/nature08858
Matsumura, Y., Nakaki, R., Inagaki, T., Yoshida, A., Kano, Y., Kimura, H., et al. (2015). H3K4/H3K9me3 bivalent chromatin domains targeted by lineage-specific DNA methylation pauses adipocyte differentiation. Mol. Cell 60, 584–596. doi:10.1016/j.molcel.2015.10.025
Melcher, M., SchMidM., , Aagaard, L., Selenko, P., Laible, G., and Jenuwein, T. (2000). Structure-function analysis of SUV39H1 reveals a dominant role in heterochromatin organization, chromosome segregation, and mitotic progression. Mol. Cell. Biol. 20, 3728–3741. doi:10.1128/mcb.20.10.3728-3741.2000
Mellone, B. G., and Allshire, R. C. (2003). Stretching it: Putting the CEN(P-A) in centromere. Curr. Opin. Genet. Dev. 13, 191–198. doi:10.1016/s0959-437x(03)00019-4
Methot, S. P., Padeken, J., Brancati, G., Zeller, P., Delaney, C. E., Gaidatzis, D., et al. (2021). H3K9me selectively blocks transcription factor activity and ensures differentiated tissue integrity. Nat. Cell Biol. 23, 1163–1175. doi:10.1038/s41556-021-00776-w
Min, J., Feng, Q., Li, Z., Zhang, Y., and Xu, R.-M. (2003). Structure of the catalytic domain of human DOT1L, a non-SET domain nucleosomal histone methyltransferase. Cell 112, 711–723. doi:10.1016/s0092-8674(03)00114-4
Minc, E., Allory, Y., Worman, H. J., Courvalin, J. C., and Buendia, B. (1999). Localization and phosphorylation of HP1 proteins during the cell cycle in mammalian cells. Chromosoma 108, 220–234. doi:10.1007/s004120050372
Montavon, T., Shukeir, N., Erikson, G., Engist, B., Onishi-Seebacher, M., Ryan, D., et al. (2021). Complete loss of H3K9 methylation dissolves mouse heterochromatin organization. Nat. Commun. 12, 4359. doi:10.1038/s41467-021-24532-8
Murayama, A., Ohmori, K., Fujimura, A., Minami, H., Yasuzawa-Tanaka, K., Kuroda, T., et al. (2008). Epigenetic control of rDNA loci in response to intracellular energy status. Cell 133, 627–639. doi:10.1016/j.cell.2008.03.030
Mutlu, B., Chen, H. M., Moresco, J. J., Orelo, B. D., Yang, B., Gaspar, J. M., et al. (2018). Regulated nuclear accumulation of a histone methyltransferase times the onset of heterochromatin formation in C. elegans embryos. Sci. Adv. 4, eaat6224. doi:10.1126/sciadv.aat6224
Nakayama, J., Rice, J. C., Strahl, B. D., Allis, C. D., and Grewal, S. I. (2001). Role of histone H3 lysine 9 methylation in epigenetic control of heterochromatin assembly. Science 292, 110–113. doi:10.1126/science.1060118
Nicetto, D., Donahue, G., Jain, T., Peng, T., Sidoli, S., Sheng, L., et al. (2019). H3K9me3-heterochromatin loss at protein-coding genes enables developmental lineage specification. Science 363, 294–297. doi:10.1126/science.aau0583
Nicetto, D., and Zaret, K. S. (2019). Role of H3K9me3 heterochromatin in cell identity establishment and maintenance. Curr. Opin. Genet. Dev. 55, 1–10. doi:10.1016/j.gde.2019.04.013
Nonaka, N., Kitajima, T., Yokobayashi, S., Xiao, G., Yamamoto, M., Grewal, S. I. S., et al. (2002). Recruitment of cohesin to heterochromatic regions by Swi6/HP1 in fission yeast. Nat. Cell Biol. 4, 89–93. doi:10.1038/ncb739
O’Carroll, D., ScHertHan, H., Peters, A. H., Opravil, S., Haynes, A. R., Laible, G., et al. (2000). Isolation and characterization of Suv39h2, a second histone H3 methyltransferase gene that displays testis-specific expression. Mol. Cell. Biol. 20, 9423–9433. doi:10.1128/mcb.20.24.9423-9433.2000
Olins, A. L., Rhodes, G., Welch, D. B. M., Zwerger, M., and Olins, D. E. (2010). Lamin B receptor: Multi-tasking at the nuclear envelope. Nucleus 1, 53–70. doi:10.4161/nucl.1.1.10515
Onder, T. T., Kara, N., Cherry, A., Sinha, A. U., Zhu, N., Bernt, K. M., et al. (2012). Chromatin-modifying enzymes as modulators of reprogramming. Nature 483, 598–602. doi:10.1038/nature10953
Oren, M. (2003). Decision making by p53: Life, death and cancer. Cell Death Differ. 10, 431–442. doi:10.1038/sj.cdd.4401183
Osman, F., and McCready, S. (1998). Differential effects of caffeine on DNA damage and replication cell cycle checkpoints in the fission yeast Schizosaccharomyces pombe. Mol. Gen. Genet. 260, 319–334. doi:10.1007/s004380050901
Osumi, K., Sato, K., Murano, K., Siomi, H., and Siomi, M. C. (2019). Essential roles of Windei and nuclear monoubiquitination of Eggless/SETDB1 in transposon silencing. EMBO Rep. 20, e48296. doi:10.15252/embr.201948296
Pace, L., Goudot, C., Zueva, E., Gueguen, P., Burgdorf, N., Waterfall, J. J., et al. (2018). The epigenetic control of stemness in CD8(+) T cell fate commitment. Science 359, 177–186. doi:10.1126/science.aah6499
Padeken, J., Methot, S. P., and Gasser, S. M. (2022). Establishment of H3K9-methylated heterochromatin and its functions in tissue differentiation and maintenance. Nat. Rev. Mol. Cell Biol. 23, 623–640. doi:10.1038/s41580-022-00483-w
Paik, W. K., Paik, D. C., and Kim, S. (2007). Historical review: The field of protein methylation. Trends biochem. Sci. 32, 146–152. doi:10.1016/j.tibs.2007.01.006
Papazyan, R., Voronina, E., Chapman, J. R., Luperchio, T. R., Gilbert, T. M., Meier, E., et al. (2014). Methylation of histone H3K23 blocks DNA damage in pericentric heterochromatin during meiosis. Elife 3, e02996. doi:10.7554/eLife.02996
Peng, J. C., and Karpen, G. H. (2009). Heterochromatic genome stability requires regulators of histone H3 K9 methylation. PLoS Genet. 5, e1000435. doi:10.1371/journal.pgen.1000435
Peric-Hupkes, D., Meuleman, W., Pagie, L., Bruggeman, S. W. M., Solovei, I., Brugman, W., et al. (2010). Molecular maps of the reorganization of genome-nuclear lamina interactions during differentiation. Mol. Cell 38, 603–613. doi:10.1016/j.molcel.2010.03.016
Peters, A. H., O'Carroll, D., ScHertHan, H., Mechtler, K., Sauer, S., Schofer, C., et al. (2001). Loss of the Suv39h histone methyltransferases impairs mammalian heterochromatin and genome stability. Cell 107, 323–337. doi:10.1016/s0092-8674(01)00542-6
Piao, L., Nakakido, M., Suzuki, T., Dohmae, N., Nakamura, Y., and Hamamoto, R. (2016). Automethylation of SUV39H2, an oncogenic histone lysine methyltransferase, regulates its binding affinity to substrate proteins. Oncotarget 7, 22846–22856. doi:10.18632/oncotarget.8072
Piao, L., Suzuki, T., Dohmae, N., Nakamura, Y., and Hamamoto, R. (2015). SUV39H2 methylates and stabilizes LSD1 by inhibiting polyubiquitination in human cancer cells. Oncotarget 6, 16939–16950. doi:10.18632/oncotarget.4760
Pickersgill, H., Kalverda, B., de Wit, E., Talhout, W., Fornerod, M., and van Steensel, B. (2006). Characterization of the Drosophila melanogaster genome at the nuclear lamina. Nat. Genet. 38, 1005–1014. doi:10.1038/ng1852
Pless, O., Kowenz-Leutz, E., Knoblich, M., Lausen, J., Beyermann, M., Walsh, M. J., et al. (2008). G9a-mediated lysine methylation alters the function of CCAAT/enhancer-binding protein-beta. J. Biol. Chem. 283, 26357–26363. doi:10.1074/jbc.M802132200
Poleshko, A., Mansfield, K. M., Burlingame, C. C., Andrake, M. D., Shah, N. R., and Katz, R. A. (2013). The human protein PRR14 tethers heterochromatin to the nuclear lamina during interphase and mitotic exit. Cell Rep. 5, 292–301. doi:10.1016/j.celrep.2013.09.024
Porro, A., Feuerhahn, S., Delafontaine, J., Riethman, H., Rougemont, J., and Lingner, J. (2014). Functional characterization of the TERRA transcriptome at damaged telomeres. Nat. Commun. 5, 5379. doi:10.1038/ncomms6379
Poulard, C., Baulu, E., Lee, B. H., Pufall, M. A., and Stallcup, M. R. (2018). Increasing G9a automethylation sensitizes B acute lymphoblastic leukemia cells to glucocorticoid-induced death. Cell Death Dis. 9, 1038. doi:10.1038/s41419-018-1110-z
Poulard, C., Bittencourt, D., Wu, D. Y., Hu, Y., Gerke, D. S., and Stallcup, M. R. (2017). A post-translational modification switch controls coactivator function of histone methyltransferases G9a and GLP. EMBO Rep. 18, 1442–1459. doi:10.15252/embr.201744060
Puschendorf, M., Terranova, R., Boutsma, E., Mao, X., Isono, K. i., Brykczynska, U., et al. (2008). PRC1 and Suv39h specify parental asymmetry at constitutive heterochromatin in early mouse embryos. Nat. Genet. 40, 411–420. doi:10.1038/ng.99
Qian, C., and Zhou, M.-M. (2006). SET domain protein lysine methyltransferases: Structure, specificity and catalysis. Cell. Mol. Life Sci. 63, 2755–2763. doi:10.1007/s00018-006-6274-5
Rao, V. K., Ow, J. R., Shankar, S. R., Bharathy, N., Manikandan, J., Wang, Y., et al. (2016). G9a promotes proliferation and inhibits cell cycle exit during myogenic differentiation. Nucleic Acids Res. 44, 8129–8143. doi:10.1093/nar/gkw483
Rea, S., EisenhaberF., , O'Carroll, D., Strahl, B. D., Sun, Z. W., SchMidM., , et al. (2000). Regulation of chromatin structure by site-specific histone H3 methyltransferases. Nature 406, 593–599. doi:10.1038/35020506
Saksouk, N., Barth, T. K., Ziegler-Birling, C., Olova, N., Nowak, A., Rey, E., et al. (2014). Redundant mechanisms to form silent chromatin at pericentromeric regions rely on BEND3 and DNA methylation. Mol. Cell 56, 580–594. doi:10.1016/j.molcel.2014.10.001
Sampath, S. C., Marazzi, I., Yap, K. L., Krutchinsky, A. N., Mecklenbrauker, I., Viale, A., et al. (2007). Methylation of a histone mimic within the histone methyltransferase G9a regulates protein complex assembly. Mol. Cell 27, 596–608. doi:10.1016/j.molcel.2007.06.026
Sanchez, N. A., Kallweit, L. M., Trnka, M. J., Clemmer, C. L., and Al-Sady, B. (2021). Heterodimerization of H3K9 histone methyltransferases G9a and GLP activates methyl reading and writing capabilities. J. Biol. Chem. 297, 101276. doi:10.1016/j.jbc.2021.101276
Scarola, M., Comisso, E., Pascolo, R., Chiaradia, R., Marion, R. M., Schneider, C., et al. (2015). Epigenetic silencing of Oct4 by a complex containing SUV39H1 and Oct4 pseudogene lncRNA. Nat. Commun. 6, 7631. doi:10.1038/ncomms8631
Schotta, G., Lachner, M., Sarma, K., Ebert, A., Sengupta, R., Reuter, G., et al. (2004). A silencing pathway to induce H3-K9 and H4-K20 trimethylation at constitutive heterochromatin. Genes Dev. 18, 1251–1262. doi:10.1101/gad.300704
Schotta, G., Sengupta, R., Kubicek, S., Malin, S., Kauer, M., Callen, E., et al. (2008). A chromatin-wide transition to H4K20 monomethylation impairs genome integrity and programmed DNA rearrangements in the mouse. Genes Dev. 22, 2048–2061. doi:10.1101/gad.476008
Schultz, D. C., Ayyanathan, K., Negorev, D., Maul, G. G., and Rauscher, F. J. (2002). SETDB1: A novel KAP-1-associated histone H3, lysine 9-specific methyltransferase that contributes to HP1-mediated silencing of euchromatic genes by KRAB zinc-finger proteins. Genes Dev. 16, 919–932. doi:10.1101/gad.973302
Schwartz-Orbach, L., Zhang, C., Sidoli, S., Amin, R., Kaur, D., Zhebrun, A., et al. (2020). Caenorhabditis elegans nuclear RNAi factor SET-32 deposits the transgenerational histone modification, H3K23me3. Elife 9, e54309. doi:10.7554/eLife.54309
Sfeir, A., and de Lange, T. (2012). Removal of shelterin reveals the telomere end-protection problem. Science 336, 593–597. doi:10.1126/science.1218498
Shi, Y., Do, J. T., Desponts, C., Hahm, H. S., Scholer, H. R., and Ding, S. (2008). A combined chemical and genetic approach for the generation of induced pluripotent stem cells. Cell stem Cell 2, 525–528. –528 Preprint at. doi:10.1016/j.stem.2008.05.011
Shi, Y. G., and Tsukada, Y. (2013). The discovery of histone demethylases. Cold Spring Harb. Perspect. Biol. 5, a017947. doi:10.1101/cshperspect.a017947
Shirai, A., Kawaguchi, T., Shimojo, H., Muramatsu, D., Ishida-Yonetani, M., Nishimura, Y., et al. (2017). Impact of nucleic acid and methylated H3K9 binding activities of Suv39h1 on its heterochromatin assembly. Elife 6, e25317. doi:10.7554/eLife.25317
Slotkin, R. K., and Martienssen, R. (2007). Transposable elements and the epigenetic regulation of the genome. Nat. Rev. Genet. 8, 272–285. doi:10.1038/nrg2072
Solovei, I., Wang, A. S., Thanisch, K., Schmidt, C. S., Krebs, S., Zwerger, M., et al. (2013). LBR and lamin A/C sequentially tether peripheral heterochromatin and inversely regulate differentiation. Cell 152, 584–598. doi:10.1016/j.cell.2013.01.009
Soufi, A., Donahue, G., and Zaret, K. S. (2012). Facilitators and impediments of the pluripotency reprogramming factors’ initial engagement with the genome. Cell 151, 994–1004. doi:10.1016/j.cell.2012.09.045
Sridharan, R., Gonzales-Cope, M., Chronis, C., Bonora, G., McKee, R., Huang, C., et al. (2013). Proteomic and genomic approaches reveal critical functions of H3K9 methylation and heterochromatin protein-1γ in reprogramming to pluripotency. Nat. Cell Biol. 15, 872–882. doi:10.1038/ncb2768
Stassen, M. J., Bailey, D., Nelson, S., Chinwalla, V., and Harte, P. J. (1995). The Drosophila trithorax proteins contain a novel variant of the nuclear receptor type DNA binding domain and an ancient conserved motif found in other chromosomal proteins. Mech. Dev. 52, 209–223. doi:10.1016/0925-4773(95)00402-m
Strom, A. R., Emelyanov, A. V., Mir, M., Fyodorov, D. V., Darzacq, X., and Karpen, G. H. (2017). Phase separation drives heterochromatin domain formation. Nature 547, 241–245. doi:10.1038/nature22989
Sugiyama, T., Cam, H. P., Sugiyama, R., Noma, K. i., Zofall, M., Kobayashi, R., et al. (2007). SHREC, an effector complex for heterochromatic transcriptional silencing. Cell 128, 491–504. doi:10.1016/j.cell.2006.12.035
Sullivan, B. A., Blower, M. D., and Karpen, G. H. (2001). Determining centromere identity: Cyclical stories and forking paths. Nat. Rev. Genet. 2, 584–596. doi:10.1038/35084512
Sullivan, B. A., and Karpen, G. H. (2004). Centromeric chromatin exhibits a histone modification pattern that is distinct from both euchromatin and heterochromatin. Nat. Struct. Mol. Biol. 11, 1076–1083. doi:10.1038/nsmb845
Sun, L., and Fang, J. (2016). E3-Independent constitutive monoubiquitination complements histone methyltransferase activity of SETDB1. Mol. Cell 62, 958–966. doi:10.1016/j.molcel.2016.04.022
Tachibana, M., Sugimoto, K., Fukushima, T., and Shinkai, Y. (2001). Set domain-containing protein, G9a, is a novel lysine-preferring mammalian histone methyltransferase with hyperactivity and specific selectivity to lysines 9 and 27 of histone H3. J. Biol. Chem. 276, 25309–25317. doi:10.1074/jbc.M101914200
Tachibana, M., Sugimoto, K., Nozaki, M., Ueda, J., Ohta, T., Ohki, M., et al. (2002). G9a histone methyltransferase plays a dominant role in euchromatic histone H3 lysine 9 methylation and is essential for early embryogenesis. Genes Dev. 16, 1779–1791. doi:10.1101/gad.989402
Tachibana, M., Ueda, J., Fukuda, M., Takeda, N., Ohta, T., Iwanari, H., et al. (2005). Histone methyltransferases G9a and GLP form heteromeric complexes and are both crucial for methylation of euchromatin at H3-K9. Genes Dev. 19, 815–826. doi:10.1101/gad.1284005
Takikita, S., Muro, R., Takai, T., Otsubo, T., Kawamura, Y. I., Dohi, T., et al. (2016). A histone methyltransferase ESET is critical for T cell development. J. Immunol. 197, 2269–2279. doi:10.4049/jimmunol.1502486
Tan, S.-L., Nishi, M., Ohtsuka, T., Matsui, T., Takemoto, K., Kamio-Miura, A., et al. (2012). Essential roles of the histone methyltransferase ESET in the epigenetic control of neural progenitor cells during development. Development 139, 3806–3816. doi:10.1242/dev.082198
Tie, C. H., Fernandes, L., Conde, L., Robbez-Masson, L., Sumner, R. P., Peacock, T., et al. (2018). KAP1 regulates endogenous retroviruses in adult human cells and contributes to innate immune control. EMBO Rep. 19, e45000. doi:10.15252/embr.201745000
Timms, R. T., Tchasovnikarova, I. A., Antrobus, R., Dougan, G., and Lehner, P. J. (2016). ATF7IP-Mediated stabilization of the histone methyltransferase SETDB1 is essential for heterochromatin formation by the HUSH complex. Cell Rep. 17, 653–659. doi:10.1016/j.celrep.2016.09.050
Torrano, J., al Emran, A., Hammerlindl, H., and Schaider, H. (2019). Emerging roles of H3K9me3, SETDB1 and SETDB2 in therapy-induced cellular reprogramming. Clin. Epigenetics 11, 43. doi:10.1186/s13148-019-0644-y
Torres-Garcia, S., Yaseen, I., Shukla, M., Audergon, P. N. C. B., White, S. A., Pidoux, A. L., et al. (2020). Epigenetic gene silencing by heterochromatin primes fungal resistance. Nature 585, 453–458. doi:10.1038/s41586-020-2706-x
Towbin, B. D., Gonzalez-Aguilera, C., Sack, R., Gaidatzis, D., Kalck, V., Meister, P., et al. (2012). Step-wise methylation of histone H3K9 positions heterochromatin at the nuclear periphery. Cell 150, 934–947. doi:10.1016/j.cell.2012.06.051
Trojer, P., Zhang, J., Yonezawa, M., Schmidt, A., Zheng, H., Jenuwein, T., et al. (2009). Dynamic histone H1 Isotype 4 methylation and demethylation by histone lysine methyltransferase g9a/KMT1C and the Jumonji domain-containing JMJD2/KDM4 proteins. J. Biol. Chem. 284, 8395–8405. doi:10.1074/jbc.M807818200
Tschiersch, B., HofmAnn, A., Krauss, V., DoRn, R., KorGe, G., and Reuter, G. (1994). The protein encoded by the Drosophila position-effect variegation suppressor gene Su(var)3-9 combines domains of antagonistic regulators of homeotic gene complexes. EMBO J. 13, 3822–3831. doi:10.1002/j.1460-2075.1994.tb06693.x
Tsusaka, T., Kikuchi, M., Shimazu, T., Suzuki, T., Sohtome, Y., Akakabe, M., et al. (2018). Tri-methylation of ATF7IP by G9a/GLP recruits the chromodomain protein MPP8. Epigenetics Chromatin 11, 56. doi:10.1186/s13072-018-0231-z
Tsusaka, T., Shimura, C., and Shinkai, Y. (2019). ATF7IP regulates SETDB1 nuclear localization and increases its ubiquitination. EMBO Rep. 20, e48297. doi:10.15252/embr.201948297
van Duyne, R., Easley, R., Wu, W., Berro, R., Pedati, C., Klase, Z., et al. (2008). Lysine methylation of HIV-1 Tat regulates transcriptional activity of the viral LTR. Retrovirology 5, 40. doi:10.1186/1742-4690-5-40
van Steensel, B., Smogorzewska, A., and de Lange, T. (1998). TRF2 protects human telomeres from end-to-end fusions. Cell 92, 401–413. doi:10.1016/s0092-8674(00)80932-0
Velazquez Camacho, O., Galan, C., Swist-Rosowska, K., Ching, R., Gamalinda, M., Karabiber, F., et al. (2017). Major satellite repeat RNA stabilize heterochromatin retention of Suv39h enzymes by RNA-nucleosome association and RNA:DNA hybrid formation. Elife 6, e25293. doi:10.7554/eLife.25293
Venter, J. C., Adams, M. D., Myers, E. W., Li, P. W., Mural, R. J., Sutton, G. G., et al. (2001). The sequence of the human genome. Science 291, 1304–1351. doi:10.1126/science.1058040
Volpe, T. A., Kidner, C., Hall, I. M., Teng, G., Grewal, S. I. S., and Martienssen, R. A. (2002). Regulation of heterochromatic silencing and histone H3 lysine-9 methylation by RNAi. Science 297, 1833–1837. doi:10.1126/science.1074973
Wang, C., Liu, X., Gao, Y., Yang, L., Li, C., Liu, W., et al. (2018). Reprogramming of H3K9me3-dependent heterochromatin during mammalian embryo development. Nat. Cell Biol. 20, 620–631. doi:10.1038/s41556-018-0093-4
Wang, F., Podell, E. R., Zaug, A. J., Yang, Y., Baciu, P., Cech, T. R., et al. (2007). The POT1-TPP1 telomere complex is a telomerase processivity factor. Nature 445, 506–510. doi:10.1038/nature05454
Wang, G., Long, J., Gao, Y., Zhang, W., Han, F., Xu, C., et al. (2019). SETDB1-mediated methylation of Akt promotes its K63-linked ubiquitination and activation leading to tumorigenesis. Nat. Cell Biol. 21, 214–225. doi:10.1038/s41556-018-0266-1
Wang, H., Cao, R., Xia, L., Erdjument-Bromage, H., Chatton, B., Tempst, P., et al. (2003). mAM facilitates conversion by ESET of dimethyl to trimethyl lysine 9 of histone H3 to cause transcriptional repression. Mol. Cell 12, 475–487. doi:10.1016/j.molcel.2003.08.007
Wang, J., Reddy, B. D., and Jia, S. (2015). Rapid epigenetic adaptation to uncontrolled heterochromatin spreading. Elife 4. doi:10.7554/eLife.06179
Wang, R., Li, H., Wu, J., Cai, Z. Y., Ni, H., Qiu, X., et al. (2020). Gut stem cell necroptosis by genome instability triggers bowel inflammation. Nature 580, 386–390. doi:10.1038/s41586-020-2127-x
Wang, T., Xu, C., Liu, Y., Fan, K., Li, Z., Sun, X., et al. (2012). Crystal structure of the human SUV39H1 chromodomain and its recognition of histone H3K9me2/3. PLoS One 7, e52977. doi:10.1371/journal.pone.0052977
Wei, J., Yu, X., Yang, L., Liu, X., Gao, B., Huang, B., et al. (2022). FTO mediates LINE1 m(6)A demethylation and chromatin regulation in mESCs and mouse development. Science 376, 968–973. doi:10.1126/science.abe9582
Weirich, S., Khella, M. S., and Jeltsch, A. (2021). Structure, activity and function of the Suv39h1 and Suv39h2 protein lysine methyltransferases. Life (Basel) 11, 703. doi:10.3390/life11070703
Weiss, T., Hergeth, S., Zeissler, U., Izzo, A., Tropberger, P., Zee, B. M., et al. (2010). Histone H1 variant-specific lysine methylation by G9a/KMT1C and Glp1/KMT1D. Epigenetics Chromatin 3, 7. doi:10.1186/1756-8935-3-7
Wong, X., Luperchio, T. R., and Reddy, K. L. (2014). NET gains and losses: The role of changing nuclear envelope proteomes in genome regulation. Curr. Opin. Cell Biol. 28, 105–120. doi:10.1016/j.ceb.2014.04.005
Woodcock, C. L., Skoultchi, A. I., and Fan, Y. (2006). Role of linker histone in chromatin structure and function: H1 stoichiometry and nucleosome repeat length. Chromosome Res. 14, 17–25. doi:10.1007/s10577-005-1024-3
Woodhouse, R. M., Buchmann, G., Hoe, M., Harney, D. J., Low, J. K. K., Larance, M., et al. (2018). Chromatin modifiers SET-25 and SET-32 are required for establishment but not long-term maintenance of transgenerational epigenetic inheritance. Cell Rep. 25, 2259–2272. doi:10.1016/j.celrep.2018.10.085
Wu, H., Chen, X., Xiong, J., Li, Y., Li, H., Ding, X., et al. (2011). Histone methyltransferase G9a contributes to H3K27 methylation in vivo. Cell Res. 21, 365–367. Preprint at. doi:10.1038/cr.2010.157
Wu, H., Min, J., Lunin, V. V., Antoshenko, T., Dombrovski, L., Zeng, H., et al. (2010). Structural biology of human H3K9 methyltransferases. PLoS One 5, e8570. doi:10.1371/journal.pone.0008570
Wu, K., Liu, H., Wang, Y., He, J., Xu, S., Chen, Y., et al. (2020). SETDB1-Mediated cell fate transition between 2C-like and pluripotent states. Cell Rep. 30, 25–36. doi:10.1016/j.celrep.2019.12.010
Xiao, B., Wilson, J. R., and Gamblin, S. J. (2003). SET domains and histone methylation. Curr. Opin. Struct. Biol. 13, 699–705. doi:10.1016/j.sbi.2003.10.003
Xu, W., Li, J., He, C., Wen, J., Rong, B., Diao, J., et al. (2021). METTL3 regulates heterochromatin in mouse embryonic stem cells. Nature 591, 317–321. doi:10.1038/s41586-021-03210-1
Yamagishi, Y., Sakuno, T., Shimura, M., and Watanabe, Y. (2008). Heterochromatin links to centromeric protection by recruiting shugoshin. Nature 455, 251–255. doi:10.1038/nature07217
Yang, L., Mei, Q., Zielinska-Kwiatkowska, A., Matsui, Y., Blackburn, M. L., Benedetti, D., et al. (2003). An ERG (ets-related gene)-associated histone methyltransferase interacts with histone deacetylases 1/2 and transcription co-repressors mSin3A/B. Biochem. J. 369, 651–657. doi:10.1042/BJ20020854
Yang, Q., Zheng, Y.-L., and Harris, C. C. (2005). POT1 and TRF2 cooperate to maintain telomeric integrity. Mol. Cell. Biol. 25, 1070–1080. doi:10.1128/MCB.25.3.1070-1080.2005
Yang, W.-L., Wang, J., Chan, C. H., Lee, S. W., Campos, A. D., Lamothe, B., et al. (2009). The E3 ligase TRAF6 regulates Akt ubiquitination and activation. Science 325, 1134–1138. doi:10.1126/science.1175065
Ye, J. Z.-S., Hockemeyer, D., Krutchinsky, A. N., Loayza, D., Hooper, S. M., Chait, B. T., et al. (2004). POT1-interacting protein PIP1: A telomere length regulator that recruits POT1 to the TIN2/TRF1 complex. Genes Dev. 18, 1649–1654. doi:10.1101/gad.1215404
Yoda, K., Ando, S., MoriShita, S., Houmura, K., Hashimoto, K., TaKeyasu, K., et al. (2000). Human centromere protein A (CENP-A) can replace histone H3 in nucleosome reconstitution in vitro. Proc. Natl. Acad. Sci. U. S. A. 97, 7266–7271. doi:10.1073/pnas.130189697
Yu, Y., Song, C., Zhang, Q., DiMaggio, P. A., Garcia, B. A., York, A., et al. (2012). Histone H3 lysine 56 methylation regulates DNA replication through its interaction with PCNA. Mol. Cell 46, 7–17. doi:10.1016/j.molcel.2012.01.019
Yuan, P., Han, J., Guo, G., Orlov, Y. L., Huss, M., Loh, Y. H., et al. (2009). Eset partners with Oct4 to restrict extraembryonic trophoblast lineage potential in embryonic stem cells. Genes Dev. 23, 2507–2520. doi:10.1101/gad.1831909
Zeller, P., Padeken, J., van Schendel, R., Kalck, V., Tijsterman, M., and Gasser, S. M. (2016). Histone H3K9 methylation is dispensable for Caenorhabditis elegans development but suppresses RNA:DNA hybrid-associated repeat instability. Nat. Genet. 48, 1385–1395. doi:10.1038/ng.3672
Zhang, K., Fischer, T., Porter, R. L., Dhakshnamoorthy, J., Zofall, M., Zhou, M., et al. (2011). Clr4/Suv39 and RNA quality control factors cooperate to trigger RNAi and suppress antisense RNA. Science 331, 1624–1627. doi:10.1126/science.1198712
Zhang, K., Mosch, K., Fischle, W., and Grewal, S. I. S. (2008). Roles of the Clr4 methyltransferase complex in nucleation, spreading and maintenance of heterochromatin. Nat. Struct. Mol. Biol. 15, 381–388. doi:10.1038/nsmb.1406
Zhang, T., Cooper, S., and Brockdorff, N. (2015). The interplay of histone modifications – writers that read. EMBO Rep. 16, 1467–1481. doi:10.15252/embr.201540945
Zhang, X., Peng, D., Xi, Y., Yuan, C., Sagum, C. A., Klein, B. J., et al. (2016). G9a-mediated methylation of ERα links the PHF20/MOF histone acetyltransferase complex to hormonal gene expression. Nat. Commun. 7, 10810. doi:10.1038/ncomms10810
Zhang, X., Tamaru, H., Khan, S. I., Horton, J. R., Keefe, L. J., Selker, E. U., et al. (2002). Structure of the Neurospora SET domain protein DIM-5, a histone H3 lysine methyltransferase. Cell 111, 117–127. doi:10.1016/s0092-8674(02)00999-6
Zhou, H., Wang, Q., Hu, Y., Zhao, W., Gautam, M., and Li, L. (2020). H3K9 demethylation-induced R-loop accumulation is linked to disorganized nucleoli. Front. Genet. 11, 43. doi:10.3389/fgene.2020.00043
Zofall, M., and Grewal, S. I. S. (2006). RNAi-mediated heterochromatin assembly in fission yeast. Cold Spring Harb. Symp. Quant. Biol. 71, 487–496. doi:10.1101/sqb.2006.71.059
Keywords: methyltransferase, methylation, histone, chromatin, heterochromatin
Citation: Levinsky AJ, McEdwards G, Sethna N and Currie MA (2022) Targets of histone H3 lysine 9 methyltransferases. Front. Cell Dev. Biol. 10:1026406. doi: 10.3389/fcell.2022.1026406
Received: 23 August 2022; Accepted: 05 October 2022;
Published: 06 December 2022.
Edited by:
Beisi Xu, St. Jude Children’s Research Hospital, United StatesReviewed by:
Akira Nifuji, Tsurumi Univesity, JapanJan Padeken, Friedrich Miescher Institute for Biomedical Research (FMI), Switzerland
Bassem Al-Sady, University of California, San Francisco, United States
Copyright © 2022 Levinsky, McEdwards, Sethna and Currie. This is an open-access article distributed under the terms of the Creative Commons Attribution License (CC BY). The use, distribution or reproduction in other forums is permitted, provided the original author(s) and the copyright owner(s) are credited and that the original publication in this journal is cited, in accordance with accepted academic practice. No use, distribution or reproduction is permitted which does not comply with these terms.
*Correspondence: Mark A. Currie, mark.currie@utoronto.ca
†These authors have contributed equally to this work