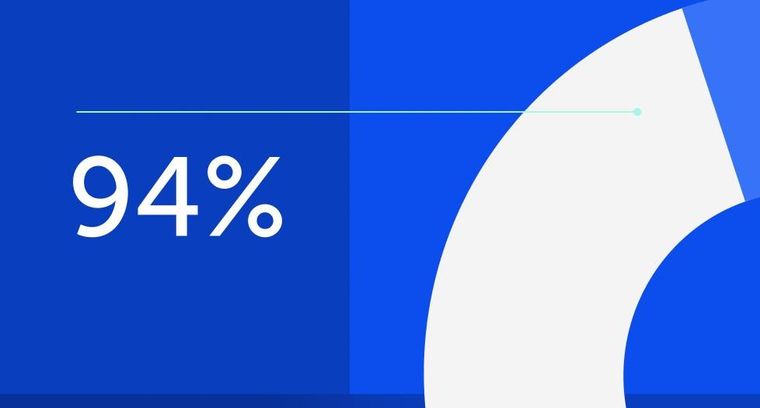
94% of researchers rate our articles as excellent or good
Learn more about the work of our research integrity team to safeguard the quality of each article we publish.
Find out more
REVIEW article
Front. Cell Dev. Biol., 12 September 2022
Sec. Cancer Cell Biology
Volume 10 - 2022 | https://doi.org/10.3389/fcell.2022.1011435
This article is part of the Research TopicPolyphenols in Cancer Prevention and TherapyView all 4 articles
Polyphenols are secondary plant metabolites or organic compounds synthesized by them. In other words, these are molecules that are found in plants. Due to the wide variety of polyphenols and the plants in which they are found, these compounds are divided according to the source of origin, the function of the polyphenols, and their chemical structure; where the main ones are flavonoids. All the beneficial properties of polyphenols have not yet been studied, since this group of substances is very extensive and diverse. However, most polyphenols are known to be powerful antioxidants and have anti-inflammatory effects. Polyphenols help fight cell damage caused by free radicals and immune system components. In particular, polyphenols are credited with a preventive effect that helps protect the body from certain forms of cancer. The onset and progression of tumors may be related directly to oxidative stress, or inflammation. These processes can increase the amount of DNA damage and lead to loss of control over cell division. A number of studies have shown that oxidative stress uncontrolled by antioxidants or an uncontrolled and prolonged inflammatory process increases the risk of developing sarcoma, melanoma, and breast, lung, liver, and prostate cancer. Therefore, a more in-depth study of the effect of polyphenolic compounds on certain signaling pathways that determine the complex cascade of oncogenesis is a promising direction in the search for new methods for the prevention and treatment of tumors.
Polyphenols are naturally occurring compounds found primarily in fruits, vegetables, cereals, beverages, and dry beans. Polyphenols are secondary metabolites of plants and are usually involved in protection against ultraviolet (UV) radiation or against aggression from pathogens (Figure 1) (Singla et al., 2019; Reed and de Freitas, 2020). In food, polyphenols can contribute bitterness, astringency, color, taste, odor, and oxidative stability. Animal, human, and epidemiological studies indicate that various polyphenols have antioxidant and anti-inflammatory properties that may have preventive and/or therapeutic effects in cardiovascular disease, neurodegenerative disorders, fat metabolism disorders, and tumors (Yahfoufi et al., 2018; Potì et al., 2019). In recent years, interest in polyphenols has increased as they are the subject of scientific research due to their possible beneficial effects on human health. Also, the main advantage of polyphenolic compounds is their use as chemoprophylaxis due to low toxicity and high tolerability (Singla et al., 2019; Reed and de Freitas, 2020).
FIGURE 1. Main groups of natural polyphenolic compounds (A–B). (A) Phenolic compounds (polyphenols) constitute one of the most numerous and widespread groups of plant substances. The structure of polyphenols can contain both simple molecules (phenolic acids) and highly polymerized compounds (condensed tannins). (B) It should be noted that the main share here falls on flavonoids. Structural changes in the rings subdivide flavonoids into several families: flavonols, flavones, flavanols, isoflavones, anthocyanins, etc. These families are often found in the form of glycosides. Various variations of flavonoids are linked by a common biosynthesis.
The main indicator in the use of polyphenols is bioavailability. Bioavailability is the proportion of a nutrient that is digested, absorbed, and metabolized through normal pathways. The bioavailability of each polyphenol is different, but their relationship between the amount of polyphenols in food and their bioavailability in the human body is not fully understood. It is important that the chemical structure of polyphenols, and not their concentration, determines the rate and extent of absorption, as well as the nature of the metabolites circulating in plasma. The most abundant polyphenols in the diet are not necessarily those that show the highest concentration of active metabolites in target tissues; therefore, the biological properties of polyphenols differ significantly from one polyphenol to another (Brglez Mojzer et al., 2016; Rogovskii, 2022). Evidence from preclinical and clinical studies indicates that the antioxidant and anti-inflammatory properties of polyphenols may potentially prevent or treat various tumors (Table 1) (Henning et al., 2015; Gee et al., 2017; Silva et al., 2019; Zeng et al., 2019; Basak et al., 2020; Lee et al., 2021; Mallet et al., 2021; Rojas-Ochoa et al., 2021; Shan et al., 2021; Wang et al., 2021). This review focuses on the current understanding of the biological effects of polyphenolic compounds and their importance in health and tumor prevention and therapy.
TABLE 1. Results of some preclinical and clinical experiments on the study of the therapeutic effect of polyphenols in tumors.
In Table 1, various types of tumors are reviewed for which polyphenolic compounds have been used as therapy and have been positive. From the indicators in Table 1, the dominant explanation for these benefits is the neutralization of free radicals, the formation of stabilized chemical complexes, thus preventing further reactions. There is also evidence of an additional mechanism by which polyphenols protect against oxidative stress by producing hydrogen peroxide (H2O2), which may then help regulate immune response actions such as cell growth. It is believed that polyphenols have an anticarcinogenic effect, inhibit cell growth, causing aging or apoptosis of tumor cells, and their differential redox status can selectively affect tumor cells.
The effect of polyphenols on tumor cells is most often protective. To date, many polyphenols, such as quercetin, catechins, isoflavones, lignans, flavanones, ellagic acid, red wine polyphenols, resveratrol, and curcumin, have been studied and tested for effectiveness (Brglez Mojzer et al., 2016; Rogovskii, 2022). All of them showed protective effects in experimental models with tumors, their mechanisms of action turned out to be different.
Oncogenesis is a multistage and microevolutionary process. There are three main stages of oncogenesis: initiation, promotion and progression. Initiated cells thus may have the ability to transform into a malignant tumor if further development follows. Development is influenced by factors that do not alter the DNA sequence and include the selection of initiated cells (Patterson et al., 2018; Peters and Gonzalez, 2018).
Several mechanisms of action have been identified for the chemopreventive action of polyphenols, including estrogenic/antiestrogen activity, antiproliferative activity, apoptosis induction, cell cycle inhibition, oxidation prevention, detoxification enzyme induction, regulation of the host immune system, and anti-inflammatory activity (Singh et al., 2021a; Bahrami et al., 2021; Weh et al., 2022) (Figure 2, Figure 3 and Figure 4). At the same time, polyphenols can affect the metabolism of pro-carcinogens and modulate the expression of cytochrome P450 enzymes involved in their activation into carcinogens. They may also facilitate their clearance by increasing the expression of phase II conjugating enzymes. This induction of phase II enzymes may be due to polyphenol toxicity (Diaz-Gerevini et al., 2016).
FIGURE 2. Schematic representation of cell cycle regulation. The activating and inhibitory effect of some polyphenols [curcumin, resveratrol, apigenin, and epigallocatechin-3-gallate (EGCG)] on the cell cycle of a tumor cell was shown.
FIGURE 3. A scheme of intracellular signal transduction is presented. The inhibitory effect of epigallocatechin-3-gallate (EGCG) on some protein kinase signaling pathways and transcription factors has been shown, as a complex of p65 and p50 subunits. Note: EGFR, Epidermal growth factor receptor; HER 2/3, Human epidermal growth factor receptor 2/3; PI3K, Phosphoinositide 3-kinases; PKC, Protein kinase C; Ras GTP, RAS guanosine triphosphate; NIK, NF-kappa-B-inducing kinase; MKK4, Mitogen-activated protein kinase kinase 4; MEK 1/2, Mitogen-activated protein kinase kinase 1/2; ERK 1/2, Extracellular signal-regulated kinase-1/2; JNK, c-Jun N-terminal Kinase; BAD, BCL2-associated agonist of cell death; PIP 2/3, Prolactin induced protein 2/3; Bcl-xL, B-cell lymphoma-extra large; IKK α, β, γ, Cytokine-responsive IkappaB kinase α, β, γ; IkBα, Nuclear factor of kappa light polypeptide gene enhancer in B-cells inhibitor, alpha; Ub, Ubiquitin B; AP-1, Activator protein 1; MMP-9, Matrix metallopeptidase 9; COX-2, cyclooxygenase 2; Bcl-2, B-cell lymphoma-2; c-FLIP, Cellular FLICE-like inhibitory protein; IAP 1/2, Inhibitor of apoptosis 1/2.
FIGURE 4. Apoptosis induction by some natural polyphenols. There are two main ways of apoptosis: 1. Apoptosis is activated by the interaction of specific ligands on the cell surface with receptor proteins containing “death domains”; and 2. The mitochondrial pathway of apoptosis begins with the collapse of the mitochondrial membrane potential and is accompanied by the release of cytochrome C from the mitochondrial intermembrane space into the cell cytoplasm. The vast experimental material obtained so far indicates that some natural polyphenols (epigallocatechin-3-gallate (EGCG), apigenin, genistein, luteolin, resveratrol, curcumin, and anthocyanin) have an apoptogenic effect using a variety of cellular targets. Note: BAX, Bcl-2 associated X-protein; Bcl-2, B-cell lymphoma-2; ROS, Reactive oxygen species; FADD, Fas-associated death domain; c-FLIP, Cellular FLICE-like inhibitory protein; Apaf-1, Apoptotic protease activating factor 1; ATP, Adenosine triphosphate; BID, BH3 interacting-domain death agonist.
Polyphenols can form potentially toxic quinones in the body, which are themselves substrates for these enzymes. Consumption of polyphenols can then activate these enzymes to detoxify themselves and thus cause an overall increase in the body’s defenses against toxic xenobiotics (Erlank et al., 2011). It has been demonstrated that tea catechins in the form of capsules, when administered to men with high grade intraepithelial neoplasia of the prostate, have a prophylactic activity by inhibiting the transformation of high grade intraepithelial neoplasia lesions into tumors (Bettuzzi et al., 2006).
Theaflavins, thearubigins and black tea polyphenols also had strong antitumor properties. Black tea polyphenols have been found to inhibit proliferation and enhance apoptosis in prostate carcinoma DU 145 cell lines (Klein and Fischer, 2002). Higher levels of insulin like growth factor-1 (IGF-1) have been found to be associated with a higher risk of developing prostate cancer (Cao et al., 2015). The binding of IGF-1 to its receptor is part of the signal transduction pathway that causes cell proliferation. The addition of black tea polyphenols was found to block the progression of IGF-1-induced cell growth into the S-phase of the cell cycle at a dose of 40 mg/ml in prostate cancer.
Polyphenols act as generators of reactive oxygen species (ROS) that act as second messengers in cellular signal transduction. In tumor cells, there are many targets that polyphenolic compounds can act on. However, nuclear factor kappa B (NF-κB) can be considered a central target, since it controls the expression of genes responsible for tumor proliferation, apoptosis, and metastasis (Khan et al., 2020).
Resveratrol prevents all stages of tumor development and has been shown to be effective in most types of tumors, including lung cancer, melanoma, breast cancer, prostate cancer, gastric cancer, and colorectal cancer (Rauf et al., 2018). It has also been shown to inhibit angiogenesis and metastasis. Extensive data in vitro show that resveratrol can modulate multiple signaling pathways involved in cell growth, apoptosis, and inflammation (Wu et al., 2019). The anticarcinogenic effects of resveratrol appear to be closely related to its antioxidant activity, where it inhibits cyclooxygenase, hydroperoxidase, protein kinase C, B-cell lymphoma 2 (Bcl-2) phosphorylation, Akt-kinase with focal adhesion, NF-κB expression, expression of matrix metalloproteases (MMPs) and cell cycle regulators (Yousef et al., 2017; El-Readi et al., 2019; Buhrmann et al., 2020). These and other in vitro and in vivo studies justify the use of polyphenols in tumor chemoprophylaxis in a combinatorial approach with chemotherapeutic drugs or cytotoxic factors for the effective treatment of drug-resistant tumors (Patra et al., 2021).
Based on the literature data on the effects of polyphenolic compounds on tumors, it can be concluded that polyphenols have great potential for use in combination with chemotherapy. A suitable combination of polyphenols with existing chemotherapeutic agents will result in a reduction in side effects without reducing the therapeutic effects of chemotherapy drugs.
Curcumin is the main curcuminoid found in turmeric root. Curcuminoids also include dimethoxycurcumin and bisdimethoxycurcumin. Turmeric has been used for its medicinal properties for thousands of years and is a widely used spice in Asian and Indian foods. Curcumin has a characteristic yellow color, is poorly soluble in water, but readily soluble in alcohol and dimethyl sulfoxide (DMSO). Curcumin has many health benefits, such as relieving inflammation, pain, and symptoms of metabolic syndromes. There is also evidence that curcumin has anti-tumor properties (Gupta et al., 2013; Lestari and Indrayanto, 2014).
Curcumin has been researched to have anticancer properties, but most of these studies have been done in vitro. These studies suggest that curcumin inhibits proliferation, induces cell cycle arrest and apoptosis through different mechanisms, in different types of tumor cell lines. There is evidence that curcumin reduces the expression of many different enzymes, transcription factors, inflammatory cytokines, growth factors, and other cell signaling components that are important for tumor growth and progression (Table 2) (Lee et al., 2011; Wang et al., 2019a; Zhang et al., 2020; Ebrahimi et al., 2021; Liang et al., 2021; Mao et al., 2021; Shi et al., 2021; Sun and Fang, 2021). For instance, in many studies of various tumor cell lines, curcumin has been found to downregulate the expression of the transcription factor NF-κB, which is normally highly expressed in tumor cells and is known to promote development, metastasis and tumor growth. In addition, curcumin stops the cell cycle in the G1/S or G2/M phases by inhibiting various cyclins in tumor cells (Figure 2). Curcumin also induces tumor cell apoptosis through caspase-dependent pathways and reduces the expression of anti-apoptotic proteins. Moreover, to in vitro studies, the properties of curcumin have also been studied in in vivo; overall demonstrating that curcumin has anti-proliferative effects (Lee et al., 2011; Wang et al., 2019a; Zhang et al., 2020; Ebrahimi et al., 2021; Liang et al., 2021; Mao et al., 2021; Shi et al., 2021; Sun and Fang, 2021). For instance, a mouse model of colorectal cancer treated with an intraperitoneal injection of curcumin demonstrated that curcumin inhibits tumor growth with favorable prognosis for overall survival. These data also suggest that curcumin activated miR-130a, which reduced expression of the Wnt/β-catenin signaling pathway and resulted in increased overall survival (Dou et al., 2017).
TABLE 2. Results of some preclinical experiments on the study of the therapeutic effect of curcumin in tumors.
Curcumin also downregulates NF-κB expression in an animal model of breast cancer, with subsequent inhibition of metastasis and angiogenesis. Studies in an animal model of pancreatic cancer have also shown that curcumin inhibits tumor growth, suppresses proliferation, and inhibits angiogenesis (Bimonte et al., 2016; Coker-Gurkan et al., 2018). These studies on the anticancer effects of curcumin are mostly pilot studies; however, curcumin has been shown to be non-toxic and well tolerated even at high concentrations. A Phase 2 pilot study evaluated the use of curcumin with docetaxel in 26 men with metastatic castration-resistant prostate cancer (mCRPC) (Passildas-Jahanmohan et al., 2021). In this study, the men received 6 g of curcumin daily in addition to docetaxel and prednisone for 6 cycles. There was a prostate-specific antigen (PSA) response among 59% of patients, and 40% experienced a partial response. There were no side effects in 100% of patients. While there appear to be countless therapeutic benefits with curcumin supplementation, most of these benefits are due to its antioxidant and anti-inflammatory effects.
One of the main problems of curcumin is its low bioavailability, which seems to be mainly due to poor absorption, rapid metabolism and rapid elimination. Polyphenols have been tested that will improve the bioavailability of curcumin. Most of them were designed to block the metabolic pathway of curcumin in order to increase its bioavailability (Anand et al., 2007). For instance, piperine, a well-known bioavailability enhancer, is the main active ingredient in black pepper and has been associated with a 2000% increase in the bioavailability of curcumin (Haq et al., 2021). Preclinical and clinical evidence suggests that curcumin has antitumor properties, and these data warrant its further evaluation as a potential treatment for tumors in humans. However, due to its poor bioavailability, ongoing research is developing approaches to improve its delivery to cells, thereby potentially improving dosage and efficacy. Thus, the problem of poor bioavailability seems to be eliminated by the addition of agents such as piperine, which increase bioavailability, thereby creating a curcumin complex.
Curcumin also shows significant interactions with cardiolipin, a lipid found exclusively in the mitochondrial membrane. It has been studied that curcumin influences the structure and dynamics of cardiolipin-containing biomimetic and biological membranes of mitochondria. The application of several biophysical methods reveals cardiolipin-stimulated association and internalization of curcumin into the lipid bilayer. In parallel, curcumin with cardiolipin-containing bilayers increased their fluidity and reduced lipid order (Taylor et al., 2011). These data suggest that membrane modifications mediated by cardiolipin interactions may play a role in the therapeutic functions of curcumin and that the mitochondrial inner membrane as a whole may represent a potential therapeutic target.
One of the polyphenolic compounds of plant origin that attracts the attention of clinicians is carnosic acid. The body is not able to synthesize carnosic acid on its own, so it can only get through food. Carnosic acid is a chemical compound that is found in large quantities in rosemary and sage, has a number of properties that make it possible to include it in some medicines and prophylactic products (Birtić et al., 2015). Rosemary leaves are used as a seasoning and food preservative. These fragrant herbs are not only valued for their unique taste and smell, but also chemical composition of spices has served as an excellent raw material for obtaining a useful medicinal compound - carnosic acid (Moore et al., 2016). Rosemary leaves are included in the British Herbal Pharmacopoeia (BHP). In the United States, India, China, they are the official pharmacological raw materials and are used in herbal medicine and homeopathy. Rosemary leaves are used as a seasoning and food preservative. Rosemary essential oil exhibits pronounced antibacterial, antifungal, anti-inflammatory, cytostatic, and antioxidant properties (Bahri et al., 2016).
In preclinical studies, it was proved that сarnosic acid had anti-inflammatory, antioxidant and antitumor activity (Bahri et al., 2016; Donmez et al., 2020; Hosokawa et al., 2020). The main property of this compound is that сarnosic acid has a high ability to neutralize free radicals, even in the human brain, preventing disorders associated with weakened nerve signal transmission. It is well known that impaired neural signaling, such as low neurotransmitter levels, is associated with mental illness, increased sensitivity to stress, and cognitive impairment. The use of сarnosic acid is possible for the prevention or treatment of conditions associated with reduced transmitter/mediator activity of dopamine, serotonin and norepinephrine (de Oliveira, 2018). ROS can cause inflammation, the development of cancer and simply lead to aging (Brieger et al., 2012). Tumors such as prostate cancer, colorectal cancer and breast cancer acquire metabolically highly active mitochondria with an increased frequency of respiratory complexes and a higher level of mitochondrial membrane potential and calcium retention (Brieger et al., 2012). Therefore, in such oxidative tumor types, the treatment strategy should be aimed at disabling oxidative mitochondrial phosphorylation. It has been investigated that the growth arrest caused by this polyphenol does not limit its protonophoric activity, but also depends on the alteration of mitochondria containing proteins that regulate the cell cycle. Targeting tumor metabolism with carnosic acid represents a promising strategy to overcome drug resistance and tumor sensitization in cancer therapy. Table 3 presents a number of preclinical studies showing the antitumor activity of the сarnosic acid (de Souza et al., 2021; de Oliveira et al., 2019; de Oliveira et al., 2016; Coyne and Narayanan, 2019; Corveloni et al., 2020; Liu et al., 2018a; D'Alesio et al., 2017; Min et al., 2021; Shao et al., 2022; Hasei et al., 2021; Liu et al., 2018b; Park et al., 2014; Petiwala et al., 2016; Kim et al., 2016).
TABLE 3. Results of some preclinical experiments on the study of the therapeutic effect of carnosic acid in tumors.
One of the promising sources of phytopreparations are medicinal plants containing flavonoids, which, due to their wide distribution in plants and great structural diversity, are currently in the focus of attention of researchers in the field of pharmacognosy, pharmacy and medicine. Flavonoids are the most numerous class of natural phenolic compounds, which are characterized by structural diversity, high and versatile activity and low toxicity. The wide range of biological activity of flavonoids is associated with the diversity of their chemical structures and the various physicochemical properties resulting from them (Figure 1). This interest is due to the fact that flavonoids cause antioxidant, angioprotective, hepatoprotective, and antitumor activity (Serafini et al., 2010; Wen et al., 2021). Moreover, it is the antitumor properties that attract scientists to the greatest extent in the field of creating new drugs in the fight against tumor.
Cell signaling systems are involved in the transmission of chemical signals from the cell surface to the cytoplasm, due to which the cell is able to respond to environmental changes. To do this, cells have specialized receptors on the surface of the plasma membrane that can recognize the presence of certain molecules in the environment, called extracellular signaling molecules. Flavonoids are able to influence the functioning of cytokine receptors, tyrosine kinase receptors (RTKs), tumor necrosis factor ligand superfamily member 10 (TRAIL), protein-coupled receptors (GRCRs), and a broad class of transmembrane signaling protein called integrins (Wiseman et al., 2001; Chen et al., 2018). The molecular mechanisms of this influence and signal propagation pathways are not well understood. In recent years, only scattered information has appeared indicating changes in the activity or expression of proteins of a particular signaling system in the presence of certain flavonoids. In this chapter, we will discuss the main mechanisms of action of flavonoids on the signaling pathways of oncogenesis.
It is now known that polyphenolic compounds can affect the functioning of receptors for cytokines such as tumor necrosis factor alpha (TNF-α) or some interleukin receptors, which can be used in tumor therapy. To a large extent, therapy is provided by suppressing the production of inflammatory cytokines, which leads to a decrease in the binding of the nuclear factor NF-kB to DNA and a decrease in the production of a number of cytokines such as interleukin-1β (IL-1β), interleukin-6 (IL-6), interleukin-8 (IL-8) and TNF-α (Leyva-López et al., 2016).
Epigallocatechin-3-gallate (EGCG) is one of the most active green tea flavonoids, has the ability to normalize many cellular processes by neutralizing the damaging effects of high concentrations of cytokines that occur during inflammation (Ohishi et al., 2016). Thus, when acting on insulin-producing pancreatic β-cells, EGCG protected against the action of IL-1β and TNF-α and restored the ability of cells to produce insulin under the influence of glucose (Zhang et al., 2011). At the same time, the content of oxidation products and ROS in the cytoplasm decreased, the potential on the mitochondrial membranes was restored, the release of cytochrome c from mitochondria into the cytoplasm stopped, and the concentration of nitrogen oxide in the cytoplasm decreased due to the suppression of the expression of nitric oxide synthase genes under the action of cytokines (Jeong and Kim, 2021). Which suggests prophylactic properties to prevent the PC development. Hoffman et al. demonstrated that EGCG was able to block constitutive and IL-1-dependent NF-kB activation as well as production of tumorigenic factors in pancreatic cancer (Hoffmann et al., 2011). EGCG inhibited tumor growth, invasion, and metastasis implying an association of the EGCG-mediated downregulation of IL-1 with the reduction in tumor growth and the development of a malignant phenotype that might play a crucial role in oncogenesis of pancreatic cancer. In other study, was demonstrated that EGCG inhibited IL-6-induced vascular endothelial growth factor (VEGF) expression in gastric cancer cells, and this inhibitory effect was at transcriptional level (Zhu et al., 2011). The preclinical studies using flavonoids in tumors therapy through control the expression inflammation mediators are shown in Table 4 (Nicholas et al., 2007; Cai et al., 2011; Ma et al., 2011; Khan et al., 2012; García-Zepeda et al., 2013; Youn et al., 2013; Abaza et al., 2015; Zhang et al., 2015; Erdogan et al., 2016; Zhao et al., 2016; Zhang et al., 2019).
The role of TRAIL has not been sufficiently studied, but it has been shown that this protein plays a role in the formation of T-lymphocyte memory, in the processes of hematopoiesis, in the development of autoimmune diseases, and in many other phenomena (Falschlehner et al., 2009). TRAIL plays a significant role in the antitumor activity of T-lymphocytes and natural killer cells (NK cells) (Falschlehner et al., 2009). Thus, TRAIL regulates the growth and metastasis of tumors, which is an important part of the body’s immune defense against the development of oncogenesis (Cardoso Alves et al., 2021). This protein contains 281 amino acids and is a homotrimer that combines three identical molecules. TRAIL is found on the surface of some immune cells (T cells, NK cells) (Falschlehner et al., 2009). There is also a water-soluble form of the TRAIL protein. The soluble form of TRAIL exhibits less liver toxicity than the membrane-bound form and can be used to initiate tumor cell apoptosis. The TRAIL molecule circulating in the blood binds to the transmembrane cell death receptors DR4 (TRAIL-R1) or DR5 (TRAIL-R2) located on the plasma membrane of cancer cells, which triggers a cascade of chemical processes leading to apoptosis (Yuan et al., 2018). The apoptosis factor TRAIL is synthesized by immune cells (T- and NK-cells), attaches to the DR4/DR5 cell death receptors on the surface of tumor cells, after which the death-inducing signaling complex (DISC) is formed, which also involves the adapter FAS-associating death domain-containing protein (FADD) and procaspase-8 or -10. Subsequently formed caspase-8 or -10 activates caspase-3 (possibly also -6 or -7), which is an effector of apoptosis (Voss et al., 2021). This path is called external. It can be influenced by the apoptosis regulator FADD-like IL-1β-converting enzyme)-inhibitory protein (c-FLIP) protein (also called caspase 8). It is also possible to activate caspase-3 through mitochondria (MTX). In this case, caspase-8 or -10 activates the apoptosis agonist BH3 interacting-domain death agonist (BID) protein (another designation BH3), which acts on mitochondrial membranes through Bax and/or Bak proteins, resulting in the formation of pores in the outer mitochondrial membrane, through which cytochrome c (Cyt C) (Engels et al., 2005; Crowder and El-Deiry, 2012). The latter through caspases is able to initiate apoptosis. The action of the Bax protein is regulated by its associated apoptosis regulatory proteins Bcl-2, BCL-xL and the inducible myeloid leukemia cell differentiation protein induced myeloid leukemia cell differentiation protein (Mcl-1). The action of caspase-9 and caspase-3 can be modulated by the apoptosis inhibitors X-linked inhibitor of apoptosis protein (XIAP), calf intestinal alkaline phosphatase (CIAP) and Survivin, which are regulated by the mitochondrial caspase activator SMAC (also known as Diablo) (Singh et al., 2021b). Mitochondrial damage can also be caused by the tumor suppressor protein p53, which acts in the presence of ROS or Akt protein kinase, which in turn is activated by phosphoinositide 3-kinase (PI3K) (Wendt et al., 2005). For unknown reasons, activation of the TRAIL signaling pathway does not cause toxicity to normal cells, which distinguishes this factor from TNF-a or FasL (Falschlehner et al., 2009). The latter can also trigger apoptosis processes, but their use in medicine is very problematic, since these proteins are highly toxic to healthy cells of various organs, especially to hepatocytes.
Clinical trials using recombinant human TRAIL in combination with conventional chemotherapy have shown encouraging results. However, some tumor cells are resistant to activation of the TRAIL signaling pathway. Overcoming this resistance and increasing the ability of cells to apoptosis can significantly help in the treatment of various types of tumors (Deng and Shah, 2020; Thapa et al., 2020). Many polyphenolic compounds, in most cases flavonoids, show synergistic effects with TRAIL, affecting various proteins involved in the regulation of apoptosis, survival, or the rate of tumor cell division. Thus, Nishikawa et al. was the first to discover that green tea EGCG was able to enhance the effect of TRAIL on human hepatocarcinoma cells, through a negative regulatory effect on Bcl-2α and BCL-xL proteins (Nishikawa et al., 2007). A similar mechanism of action through the proteins Bcl-2, BCL-xL and a number of other proteins was found in the action of EGCG to TRAIL in prostate carcinoma cells (Thapa et al., 2020). Later, the effectiveness of kaempferol against TRAIL in relation to glioblastomas was shown, where the specified flavonoid initiated the degradation of survivin and inhibition of the Akt pathway, which led to the death of tumor cells (Yoshida et al., 2008). Quercetin may enhance the action of TRAIL through dephosphorylation of the Akt pathway and activation of caspases in human adenocarcinoma cells (Moon et al., 2015). However, no cytotoxicity was found in relation to normal cells. The same authors showed that quercetin is able to activate caspases-3, -8 and -9. The ability of quercetin to interact with the survivin promoter and prevent the expression of this protein was also found (Özsoy et al., 2020). Information on the effect of flavonoids on various components of the signaling system of TRAIL-dependent apoptosis is given in Table 5 (Ismail et al., 2015; Han et al., 2016; Park et al., 2016; Li et al., 2017; Xu et al., 2018; Kim et al., 2020; Wu et al., 2020; Huang et al., 2021).
TABLE 5. The antitumor effects of flavonoids by regulation the expression of TRAIL-induced apoptosis.
RTKs play an essential role in the regulation of processes associated with cell proliferation or death. In addition, this receptor is a therapeutic target for many drugs used in the treatment of tumors (Trenker and Jura, 2020). The receptor is a transmembrane protein with which various growth factors, cell division and some hormones interact. Accordingly, about 20 different types of RTK are distinguished. These include the insulin receptor (IR), epidermal growth factor receptor (EGFR), the ephrin (Eph) receptor, a protein that regulates intracellular interactions and cell migration, and the angiopoietin receptor responsible for angiogenesis (Choura and Rebaï, 2011).
Catechins may have a therapeutic effect on many types of scrotum cells, as well as on the development and progression of tumors in vivo, due to the suppression of RTK signals (Larsen et al., 2010). Being located in the plasma membrane, this receptor is sensitive to changes in the physical properties of lipids, which can be influenced by flavonoids. Among them, catechins are perhaps one of the most effective anticarcinogenic agents among plant polyphenols. One possible explanation for their activity suggests that flavonoids are mimetics of the adenine portion of the adenosine triphosphate (ATP) molecule and are able to block the ATP-binding sites of protein kinase receptors (Baranwal et al., 2022). In addition, attention is drawn to their ability to influence the lateral segregation of plasma membrane lipids and the formation of lipid rafts, which disrupts the functioning of membrane receptors, such as RTK or the epithelial growth factor receptor EGFR (Choura and Rebaï, 2011; Talukdar et al., 2020). Thus, EGCG prevents the binding of the epithelial growth factor to the corresponding receptor and inhibits the functioning of other RTKs, which determines the anticarcinogenic effect of this flavonoid (Shimizu et al., 2011). The flavonoid silibinin probably also has a similar effect on RTK (Rho et al., 2010). Table 6 presents preclinical studies on the inhibitory effect of some flavonoids on RTK activity in various tumors (Peterson and Barnes, 1993; Lee et al., 2004; Shimizu et al., 2010; Kim et al., 2014; Suh et al., 2015; Lee et al., 2017; Namiki et al., 2020; Kim et al., 2021).
TABLE 6. The antitumor effects of flavonoids by regulation of receptor tyrosine kinases (RTKs) and integrins.
Integrins are surface cellular receptors that transmit signals to the cytoplasm about changes in the chemical composition of the matrix surrounding cells. Integrins are present on the cell surface of most multicellular organisms, and usually consist of two α and β subunits that form 24 different dimeric molecules. Each subunit has a transmembrane segment, extracellular and cytoplasmic domains (Desgrosellier and Cheresh, 2010). Integrins are of great importance in the regulation of intercellular interaction, cell adhesion and migration. Integrins are involved in various diseases, including tumor development and metastasis processes (Sonnenberg, 1993). Accordingly, integrins serve as targets for the therapeutic effects of various drugs in tumors. EGCG is able to influence the activity of monocytes due to a decrease in the expression of α5β3 integrins, which is essential in the regulation of tumor growth and metastasis (Sen and Chatterjee, 2011). EGCG can inhibit the migration and adhesion of B-lymphocytes, which are also involved in the development of the immune response by blocking the expression of CD11b integrin (Kawai et al., 2004). EGCG is also able to suppress the expression of the EGFR through its action on the α5β1 integrin, which is of great importance in the development of human carcinoma (Ye et al., 2017). There are also data on the effect of EGCG on the motility and migration of fibroblasts due to the suppression of the expression of the α2β1 integrin, which may be important in the antitumor activity of this catechin (Hung et al., 2005). Apigenin, present in many medicinal herbs (chamomile, adonis, lemon balm, etc.) can block p5 integrin in breast cancer cells (Imran et al., 2020). Kaempferol, a flavonoid from cumin, tea, viburnum, and others, suppresses TNF-α-induced β2 integrin expression of eosinophils, which prevents them from infiltrating the airway epithelium in vivo, suggesting preventive properties for lung cancer (Kuo et al., 2015). Glabridin, a licorice flavonoid, suppresses the expression of αnuβ3 integrin, which, along with the suppression of the activity of some other components of the signaling system (FAC/Src, Akt, and Ras homolog family member A (RhoA)), prevents migration, invasion, and angiogenesis of lung cancer cells (Tsai et al., 2011). Table 7 presents preclinical studies on the inhibitory effect of some flavonoids on integrins activity in various tumors (Pilorget et al., 2003; Dastpeyman et al., 2012; Ruan et al., 2012; Deep et al., 2014; Li et al., 2018; Min et al., 2018; Liu et al., 2021; Qiao et al., 2022).
In order to assess the potential of various polyphenolic compounds to have an effect on tumors of the central nervous system (CNS), it is first necessary to consider the ability of these substances to crossing the blood-brain barrier (BBB). The bioavailability of polyphenolic compounds for the CNS is very low. For example, direct administration of large amounts of EGCG into the stomach during the day made it possible to obtain very high concentrations of this substance in the blood plasma, but its concentration in the brain was 5–10% of the concentration in the blood in vivo (Ferri et al., 2015). Thus, in order to achieve therapeutic concentrations of EGCG in the brain, it was necessary to increase its concentration in the blood to excessively high values. The study of other flavonoids showed that quercetin penetrates poorly through the BBB, but after penetrating, it accumulates in such parts of the brain as the hippocampus, striatum, cerebellum, where its concentration can reach 1 mg per gram of brain tissue (Pervin et al., 2019). Kaempferol and isorhamnetin penetrate better, and the average concentration of these substances in the brain can reach several hundred nanograms per gram of healthy tissue (Rangel-Ordóñez et al., 2010). At present, doubts have arisen about the adequacy of estimates of the degree of penetration of polyphenolic compounds into the CNS, as well as the effectiveness of the action of low concentrations of these substances, since, despite the apparent low content of these substances in the tissues of the CNS, there is a lot of experimental evidence of their effective effect on behavioral reactions and cognitive functions of animals and humans. In addition, it was found that after penetration into the brain tissue, flavonoids can undergo significant modification. Thus, catechins are conjugated with glycosides and are present in the form of glucuronides, which also have the ability to protect cells from oxidative stress. Moreover, chemical modification of flavonoids and other plant polyphenols can be used to deliver these substances to the brain, where they can be highly active (Rudrapal et al., 2021; Zhou et al., 2022). Thus, it is proposed to use a fully acetylated form of EGCG as a drug precursor. It was shown that active EGCG is released in the cytoplasm of cells due to the action of intracellular esterases (Lambert et al., 2006). The use of flavonoids as building blocks for the creation of substances capable of penetrating the BBB and exhibiting drug activity is one of the most promising research strategies in the therapy of CNS tumors.
Prolonged intense exposure to UV radiation on the surface of the skin leads to the development of oxidative stress, damage to DNA molecules and the development of inflammatory processes (Aphalo et al., 2015). Exposure to ultraviolet light can cause various skin diseases, not only premature aging, but also serious diseases such as melanoma and non-melanoma skin cancers (Watson et al., 2016). Most polyphenolic compounds of plant origin are able to absorb radiation in the UV range and, therefore, can act as a screen. Indeed, it has been experimentally shown that when extracts from plants are applied to the surface of the skin, the reaction of the skin to ultraviolet light irradiation is significantly reduced (Cavinato et al., 2017). However, the protective effect of these substances is not limited to shielding tissues from the action of the ultraviolet part of the spectrum. Protection is also carried out due to the action on the regulatory systems of the cell.
It was found that the appearance of ROS is associated with the activation of the factor NF-kB and the subsequent expression of NADPH oxidase and cyclooxygenase-2 (COX-2), the activity of which is the cause of the accumulation of ROS in keratinocytes (Wang et al., 2019b). The activity of cyclooxygenase in the cytoplasm and the accumulation of products of lipid peroxidation (LPO) are associated with an increase in the concentration of calcium cations in the cytoplasm during ultraviolet irradiation. Antioxidants do not appear to be able to protect the skin from the effects of UV radiation (Sourivong et al., 2007). Thus, it was experimentally shown that the antioxidant ionol was not effective in protecting cells exposed to UV radiation, while the blocker of COX-2 cyclooxygenase aspirin reduced the concentration of lipid peroxidation in keratinocytes (Ponomareva et al., 1985). Due to the fact that UV radiation induces the activity of COX-2 cyclooxygenase, the concentration of prostaglandins formed from arachidonic acid increases in skin cells (De Leo et al., 1984). As a result, inflammation processes develop, edema is observed, keratinocyte proliferation accelerates, epidermal hyperplasia accelerates, oxidation products accumulate, which leads to oxidative damage to DNA. Therefore, as a result of chronic irradiation, mutations accumulate, which leads to malignant degeneration of keratinocytes and the development of oncogenesis (Leonardi et al., 2018). On the contrary, the action of COX-2 inhibitors or agents that interfere with the expression of this enzyme can significantly prevent the carcinogenic degeneration of epidermal cells (Leonardi et al., 2018).
The ability of some flavonoids to suppress the expression of COX-2 may underlie the mechanisms of the protective action of these substances against the action of UV radiation, as was shown for baicalein and wogonin, hesperetin, magniferin and tangeritin (Fischer et al., 2018; Farjadmand et al., 2021). It is possible that this regulation is carried out through the mitogen-activated protein kinase (MAPK) regulatory pathway, as was shown for luteolin (Aziz et al., 2018). Using the methylated flavonol 5,7-dimethoxyflavone as an example, it was shown that not only COX, but also other components of the regulatory chain, such as peroxisome proliferator-activated receptor (PPAR), NF-kB, can be regulated, resulting in a decrease in the concentration of IL-6 and IL-8 (Walle and Walle, 2007). In addition, there is a decrease in the expression of MMPs, a decrease in the concentration of oxidative stress components, and suppression of the activity of inflammation components through the regulatory pathways NF-kB and MAPK (Walle and Walle, 2007). As a result, damage to the skin and the subsequent possible development of oncology associated with the action of UV radiation are prevented.
Flavonoids may also affect other regulatory systems. Silibinin has recently been found to prevent damage to the epidermis after UV radiation by activating the tumor suppressor protein p53, resulting in the activation of the GADD45α protein, which contributes to the protection of cells under conditions of stress and DNA damage (Rigby et al., 2017). Chrysin protects epidermal keratinocytes from damage by UV irradiation, primarily by restoring the expression of aquaporin 3 (AQP-3), which ensures the normalization of the osmotic and salt balance of the cell, disturbed by irradiation. In a study on the protective effects of eriodictyol on keratinocytes, this flavanoid was found to act through activation of the p38/MAPK/Akt signaling pathway (Wu et al., 2011). Blackberry anthocyanins protect keratinocytes from UV radiation by significantly increasing the expression of antioxidant enzymes such as catalase, mitochondrial superoxide dismutase and glutathione peroxidase, thus preventing the development of oxidative stress (Murapa et al., 2012). Grape procyanidins also prevent the production of reactive oxygen species in cells, but using a different mechanism - suppression of p38 (MAPK14) and c-Jun NH1-terminal kinase/c-Jun NH2-terminal kinase (JNK1/2) (MAPK8) expression (Matito et al., 2011). The soy isoflavone metabolite daidzein, 7,3',4'-trihydroxy-isoflavone, prevents the development of melanoma induced by UV irradiation and by acting on the ATP-binding sites of protein kinases Cot and mitogen-activated protein (MAP) kinase kinase 4 (MKK4) (Ravindranath et al., 2004). It is noteworthy that the original daidzein molecule is not able to interact with these proteins and does not exhibit anticarcinogenic activity under these conditions.
According to current data, viruses are the etiological agents of about 15% of human tumors. These viruses include: human T-leukemia/lymphoma virus, human immunodeficiency virus (HIV), human papillomavirus (HPV), hepatitis B and C viruses, Epstein-Barr virus (EBV), and other (Cao and Li, 2018). It is important to note that some viruses are associated with tumors of only one localization, while others are associated with various malignant neoplasms, which is probably due to the tropism of viruses for certain types of cell systems. The virus-genetic theory of the occurrence of tumors, proposed back in the 40 s of the 20th century by Zilber, has received numerous confirmations over the years (Cao and Li, 2018; Haley et al., 2019). At present, it is clear that although viruses are not the only cause of tumors, they play a large role in the occurrence of malignant neoplasms (for instance, the presence of hepatitis B virus increases the risk of developing hepatocellular carcinoma) in both humans and animals. A characteristic feature of tumor diseases associated with viruses is a long latent period, years and even decades can pass from the moment of infection to the manifestation of the disease (Mui et al., 2019; Blackard and Sherman, 2021).
The creation of antiviral drugs based on natural compounds is undoubtedly one of the promising real directions. The expansion of the number of compounds used and the use of the synergism of their biological action provide a large reserve of therapeutic antiviral action and, along with the prevention of oncological diseases. Along with a number of different natural substances, polyphenolic compounds with antioxidant activity can also be used, in particular flavanoids, which occupies one of the leading positions in antioxidant activity. The mechanism of the antiviral action of polyphenolic compounds includes both direct interference with the mechanism of viral replication and suppression of cellular signaling pathways necessary for replication (Di Petrillo et al., 2022) (Figure 5). Numerous studies have shown that some polyphenols have an effect against influenza A, herpes virus, hepatitis B and C, HPV and others. Table 8 lists the polyphenols for which their antiviral activity has been confirmed (Docherty et al., 2004; Docherty et al., 2005; Yiu et al., 2010; Ciesek et al., 2011; Fatima et al., 2014; Pang et al., 2014; Rastogi et al., 2015; Sun et al., 2021).
FIGURE 5. Targets and processes affected by some natural polyphenolic compounds in viral infections. This scheme shows the possible mechanisms of the antiviral action of the main classes of natural polyphenolic compounds. Flavonoids have been found to inhibit merger, integration, and reverse transcription. Inhibition of protease, reverse transcriptase, replication and maturation are among the anti-HIV mechanisms of some terpenoids. Coumarins inhibit transcriptase and activation of nuclear factor-kappa B (NF-kB). Note: PGC-1α, Peroxisome proliferator-activated receptor gamma coactivator 1-alpha; TLR-3, Toll-like receptor 3; TLR-7, Toll-like receptor 7; ErbB1, Receptor tyrosine-protein kinase; CCR5, C-C chemokine receptor type 5; CXCR4, C-X-C chemokine receptor type 4.
Significant progress has been made in the use of plant polyphenolic compounds for the treatment of HIV infection. Flavonoids have been found to inhibit viral fusion, integration, and reverse transcription. Inhibition of protease, reverse transcriptase, replication, and maturation are among the anti-HIV mechanisms of some polyphenols (Yu and Zhao, 2012; Andrae-Marobela et al., 2013). Flavonoids, alkaloids, anthocyanins, chalcones, xanthones and homoisoflavonoids, which inhibit neuraminidase, are proposed to be used as anti-influenza agents (Bahramsoltani et al., 2016). Polyphenol, isochlorogenic acid, dehydrocheilantifoline and some other amide alkaloids have an effect against hepatitis B virus (Man et al., 2021). Curcumin inhibits the replication and expression of the hepatitis B virus gene (Thongsri et al., 2021). In cell model studies, it was found that quercetin inhibits the entry of the influenza virus at an early stage of infection, due to its pronounced anti-inflammatory properties, reduces the effects of pro-inflammatory cytokines and the risk of developing lung inflammation (Mehrbod et al., 2020; Nile et al., 2020). In addition, it enhances the effect of other drugs.
In general, polyphenols are able to interfere with different stages of the life cycle of viruses, which characterizes them as multipurpose drugs that act on vital proteins of the pathogen (Table 8). At the same time, many researchers pay attention to the fact that for the development of drugs based on polyphenols, it is necessary to overcome quite a lot of difficulties, since these compounds are characterized by complex structures, low bioavailability, and rapid excretion from the body. In addition, in-depth studies in vitro (ex vivo), in vivo, as well as multicenter clinical studies are required. Despite all the difficulties, polyphenols should eventually find their place as candidates for creating on their basis not only antiviral drugs, but also for the prevention of cancer.
The search for effective methods of treatment and prevention of tumors, despite the successes achieved in recent decades, remains one of the most urgent tasks in medicine. Antitumor therapies (e.g., chemotherapy) use approaches based on induction of cell death by increasing the intracellular concentration of ROS (Wang et al., 2017). Since ROS are formed in cells not only as a result of the action of external physicochemical factors, but also in the processes of cellular metabolism, pharmacological correction of the redox properties of tumor cells is a promising approach to improve the effectiveness of antitumor therapy. In chemotherapy, drugs that enhance the production of ROS by cells are widely used. In recent decades, it has been shown that ROS generation is an important step in the process of induction of apoptosis of tumor cells by such widely used chemotherapeutic agents as cisplatin and doxorubicin (Li et al., 2020; Xia et al., 2020).
To increase the effectiveness of chemotherapeutic agents, approaches are proposed aimed at inducing oxidative stress in tumor cells. Therefore, pharmacological correction of the redox properties of tumor cells is a promising approach to improve the effectiveness of antitumor therapy. Currently, natural polyphenolic compounds are considered as preparations for the development of selective chemosensitizers. Due to the large number and variety of phenolic compounds, the antitumor properties of many of them have not been studied. In recent years, the regulatory properties of EGCG, the main catechin in green tea, resveratrol, one of the main polyphenols contained in the skin of grapes and red wines, and curcumin, the main curcuminoid that is part of the turmeric root, have been most actively studied (NavaneethaKrishnan et al., 2019). Polyphenols at low micromolar concentrations cause a protective antioxidant effect. At high concentrations, polyphenolic compounds exhibit pro-oxidant and cytotoxic properties. At the same concentrations in tumor and normal cells, polyphenols can induce oppositely directed effects (Oalđe Pavlović et al., 2021).
EGCG inhibits ROS generation in normal epithelial cells but induces ROS generation in tumor cells. In transformed cells, EGCG activates a mitochondria-mediated pathway of cell death, accompanied by the generation of ROS, a decrease in the transmembrane mitochondrial potential, and the release of apoptotic proteins (Min and Kwon, 2014). Similar results have been obtained in pancreatic cancer, lung cancer, colon cancer, and melanoma cell lines, as well as in breast cancer xenograft animal models (Gan et al., 2018; Khan and Mukhtar, 2018; Ravindran Menon et al., 2021; Romano and Martel, 2021). At concentrations of 5–20 μM, EGCG induced apoptosis only in melanoma cells, without any toxic effect on normal melanocytes (Ravindran Menon et al., 2021). At concentrations of 10–80 mg/ml, EGCG induced apoptosis in hepatocellular carcinoma cells, but not in the normal liver cells (Sojoodi et al., 2020). Sensitization of EGCG tumor cells to the action of a number of antitumor drugs has been shown in both in vitro and in vivo studies. EGCG enhances the effects of doxorubicin, 5-fluorouracil, cisplatin, trizenox (As2O3), bortezomib, and etoposide (Lecumberri et al., 2013; Almatroodi et al., 2020; Huang et al., 2020). Among the proposed mechanisms of tumor cell chemosensitization, the key role is played by redox modulation as a result of increased intracellular ROS production. For example, in ovarian cancer cells, EGCG increased cisplatin toxicity three to six fold, including in cisplatin-resistant cells (Zhou et al., 2014). However, the selectivity of the action of the compound in relation to tumor cells is not justified.
Numerous in vitro and in vivo studies have shown high antitumor activity of curcumin (see section “THE EFFECTIVENESS OF POLYPHENOLS”). It has been shown that the antitumor properties of curcumin are realized with the participation of ROS (Wan et al., 2019). It is assumed that curcumin causes ROS-induced decrease in transmembrane mitochondrial potential, resulting in activation of apoptosis (Wan et al., 2019). A number of studies have shown the ability of curcumin to activate autophagy through increased intracellular production of ROS (Tang et al., 2021). When autophagy is activated, mitochondria are the main source of ROS. At the same time, it is known that the functional relationship between apoptosis and autophagy is complex, that is, in some cases, autophagy is part of the cellular adaptation mechanism that protects cells from apoptosis, while in other conditions, autophagy can cause cell death or initiate apoptosis (Maiuri et al., 2007). It has recently been found that the molecular mechanism of sensitization of MCF-7 cells to the action of paclitaxel and adrenomycin by curcumin involves inhibition of the flap structure-specific endonuclease 1 (Fen1) endonuclease with the participation of ROS and NF-E2–related factor 2 (Nrf2) (Chen et al., 2014).
During therapy, resveratrol sensitizes tumor cells to the action of radiation therapy, cisplatin, doxorubicin, paclitaxel, and bortezamib (Fu et al., 2021; Ren et al., 2021). Synergy of action has been observed with the combined use of resveratrol and curcumin in relation to breast cancer, colon cancer, lung cancer and hepatocellular cancer (Niedzwiecki et al., 2016; Pavan et al., 2016; Arena et al., 2021). An increase in intracellular ROS production also plays a key role in the mechanism of cell sensitization under the action of resveratrol. It has been shown that resveratrol increases the sensitivity of pancreatic cancer cells as a result of Nrf2 activation and increased ROS production (Cheng et al., 2018). It is important to note that during radio- and chemotherapy, resveratrol protects normal cells from radiation damage and the toxic effects of chemotherapy drugs.
Man consumes polyphenolic compounds throughout the evolutionary process, and these substances have been and remain a constantly present component of the internal environment of the body. Once in the body, they are involved in numerous processes of cell signaling, gene expression and various metabolic functions. Polyphenolic compounds, in particular flavonoids, are sometimes an inconspicuous but necessary link in the assembly and functioning of proteins, in the formation of biological membranes, and in the transmission of information in the cell. Being always available, they serve as helpers in many processes. It can be hoped that further research on polyphenols will make it possible to make many interesting discoveries, and the creation of artificial derivatives of flavonoids will make it possible to obtain new effective medicinal substances.
Targeting the stage of oncogenesis and development of tumors with polyphenolic compounds is a promising strategy for their use in both prevention and treatment (including increasing sensitivity to chemotherapy) of tumors. Data published in the literature that meet the requirements of evidence-based medicine confirm the beneficial effect of turmeric and rosemary with a high content of polyphenols, such as curcumin and carnazole acid, in pathological disorders in the body’s division processes, in particular, in oncological processes. Studies of the action of flavonoids show their ability to influence various life processes, both individual cells and the body as a whole. Although epidemiological studies of the relationship between the spread of cancer and the consumption of flavonoids have not yielded unambiguous results, in experimental conditions in vitro and in vivo, as well as in studies of volunteers, quite convincing evidence has been obtained of the promise of the use of certain flavonoids in the prevention and even in the treatment of tumors.
IG: conceptualization, writing–original draft, and project administration. OB: writing–review and editing, investigation, and project administration. GS: formal analysis, methodology, and original draft. AS: resources and data curation. AS: validation and data curation. JW and XC: validation and visualization. AS and SZ: supervision and funding acquisition. All authors have read and agreed to the published version of the manuscript.
The authors declare that the research was conducted in the absence of any commercial or financial relationships that could be construed as a potential conflict of interest.
All claims expressed in this article are solely those of the authors and do not necessarily represent those of their affiliated organizations, or those of the publisher, the editors and the reviewers. Any product that may be evaluated in this article, or claim that may be made by its manufacturer, is not guaranteed or endorsed by the publisher.
Abaza, M. S., Orabi, K. Y., Al-Quattan, E., and Al-Attiyah, R. J. (2015). Growth inhibitory and chemo-sensitization effects of naringenin, a natural flavanone purified from Thymus vulgaris, on human breast and colorectal cancer. Cancer Cell Int. 15, 46. doi:10.1186/s12935-015-0194-0
Almatroodi, S. A., Almatroudi, A., Khan, A. A., Alhumaydhi, F. A., Alsahli, M. A., and Rahmani, A. H. (2020). Potential therapeutic targets of epigallocatechin gallate (EGCG), the most abundant catechin in green tea, and its role in the therapy of various types of cancer. Molecules 25 (14), 3146. doi:10.3390/molecules25143146
Anand, P., Kunnumakkara, A. B., Newman, R. A., and Aggarwal, B. B. (2007). Bioavailability of curcumin: Problems and promises. Mol. Pharm. 4 (6), 807–818. doi:10.1021/mp700113r
Andrae-Marobela, K., Ghislain, F. W., Okatch, H., and Majinda, R. R. (2013). Polyphenols: A diverse class of multi-target anti-HIV-1 agents. Curr. Drug Metab. 14 (4), 392–413. doi:10.2174/13892002113149990095
Aphalo, P. J., Jansen, M. A., McLeod, A. R., and Urban, O. (2015). Ultraviolet radiation research: From the field to the laboratory and back. Plant Cell Environ. 38 (5), 853–855. doi:10.1111/pce.12537
Arena, A., Romeo, M. A., Benedetti, R., Masuelli, L., Bei, R., Gilardini Montani, M. S., et al. (2021). New insights into curcumin- and resveratrol-mediated anti-cancer effects. Pharm. (Basel) 14 (11), 1068. doi:10.3390/ph14111068
Aziz, N., Kim, M. Y., and Cho, J. Y. (2018). Anti-inflammatory effects of luteolin: A review of in vitro, in vivo, and in silico studies. J. Ethnopharmacol. 225, 342–358. doi:10.1016/j.jep.2018.05.019
Bahrami, A., Makiabadi, E., Jalali, S., Heidari, Z., Assadi, M., and Rashidkhani, B. (2021). Dietary intake of polyphenols and the risk of breast cancer: A case-control study. Clin. Nutr. Res. 10 (4), 330–340. doi:10.7762/cnr.2021.10.4.330
Bahramsoltani, R., Sodagari, H. R., Farzaei, M. H., Abdolghaffari, A. H., Gooshe, M., and Rezaei, N. (2016). The preventive and therapeutic potential of natural polyphenols on influenza. Expert Rev. anti. Infect. Ther. 14 (1), 57–80. doi:10.1586/14787210.2016.1120670
Bahri, S., Jameleddine, S., and Shlyonsky, V. (2016). Relevance of carnosic acid to the treatment of several health disorders: Molecular targets and mechanisms. Biomed. Pharmacother. 84, 569–582. doi:10.1016/j.biopha.2016.09.067
Baranwal, A., Aggarwal, P., Rai, A., and Kumar, N. (2022). Pharmacological actions and underlying mechanisms of catechin: A review. Mini Rev. Med. Chem. 22 (5), 821–833. doi:10.2174/1389557521666210902162120
Basak, S. K., Bera, A., Yoon, A. J., Morselli, M., Jeong, C., Tosevska, A., et al. (2020). A randomized, phase 1, placebo-controlled trial of APG-157 in oral cancer demonstrates systemic absorption and an inhibitory effect on cytokines and tumor-associated microbes. Cancer 126 (8), 1668–1682. doi:10.1002/cncr.32644
Bettuzzi, S., Brausi, M., Rizzi, F., Castagnetti, G., Peracchia, G., and Corti, A. (2006). Chemoprevention of human prostate cancer by oral administration of green tea catechins in volunteers with high-grade prostate intraepithelial neoplasia: A preliminary report from a one-year proof-of-principle study. Cancer Res. 66 (2), 1234–1240. doi:10.1158/0008-5472.CAN-05-1145
Bimonte, S., Barbieri, A., Leongito, M., Piccirillo, M., Giudice, A., Pivonello, C., et al. (2016). Curcumin AntiCancer studies in pancreatic cancer. Nutrients 8 (7), 433. doi:10.3390/nu8070433
Birtić, S., Dussort, P., Pierre, F. X., Bily, A. C., and Roller, M. (2015). Carnosic acid. Phytochemistry 115, 9–19. doi:10.1016/j.phytochem.2014.12.026
Blackard, J. T., and Sherman, K. E. (2021). Drugs of abuse and their impact on viral pathogenesis. Viruses 13 (12), 2387. doi:10.3390/v13122387
Brglez Mojzer, E., Knez Hrnčič, M., Škerget, M., Knez, Ž., and Bren, U. (2016). Polyphenols: Extraction methods, antioxidative action, bioavailability and anticarcinogenic effects. Molecules 21 (7), 901. doi:10.3390/molecules21070901
Brieger, K., Schiavone, S., Miller, F. J., and Krause, K. H. (2012). Reactive oxygen species: From health to disease. Swiss Med. Wkly. 142, w13659. doi:10.4414/smw.2012.13659
Buhrmann, C., Shayan, P., Brockmueller, A., and Shakibaei, M. (2020). Resveratrol suppresses cross-talk between colorectal cancer cells and stromal cells in multicellular tumor microenvironment: A bridge between in vitro and in vivo tumor microenvironment study. Molecules 25 (18), 4292. doi:10.3390/molecules25184292
Cai, X., Ye, T., Liu, C., Lu, W., Lu, M., Zhang, J., et al. (2011). Luteolin induced G2 phase cell cycle arrest and apoptosis on non-small cell lung cancer cells. Toxicol. Vitro 25 (7), 1385–1391. doi:10.1016/j.tiv.2011.05.009
Cao, J., and Li, D. (2018). Searching for human oncoviruses: Histories, challenges, and opportunities. J. Cell. Biochem. 119 (6), 4897–4906. doi:10.1002/jcb.26717
Cao, Y., Nimptsch, K., Shui, I. M., Platz, E. A., Wu, K., Pollak, M. N., et al. (2015). Prediagnostic plasma IGFBP-1, IGF-1 and risk of prostate cancer. Int. J. Cancer 136 (10), 2418–2426. doi:10.1002/ijc.29295
Cardoso Alves, L., Corazza, N., Micheau, O., and Krebs, P. (2021). The multifaceted role of TRAIL signaling in cancer and immunity. FEBS J. 288 (19), 5530–5554. doi:10.1111/febs.15637
Cavinato, M., Waltenberger, B., Baraldo, G., Grade, C. V. C., Stuppner, H., and Jansen-Dürr, P. (2017). Plant extracts and natural compounds used against UVB-induced photoaging. Biogerontology 18 (4), 499–516. doi:10.1007/s10522-017-9715-7
Chen, B., Zhang, Y., Wang, Y., Rao, J., Jiang, X., and Xu, Z. (2014). Curcumin inhibits proliferation of breast cancer cells through Nrf2-mediated down-regulation of Fen1 expression. J. Steroid Biochem. Mol. Biol. 143, 11–18. doi:10.1016/j.jsbmb.2014.01.009
Chen, L., Teng, H., Jia, Z., Battino, M., Miron, A., Yu, Z., et al. (2018). Intracellular signaling pathways of inflammation modulated by dietary flavonoids: The most recent evidence. Crit. Rev. Food Sci. Nutr. 58 (17), 2908–2924. doi:10.1080/10408398.2017.1345853
Cheng, L., Yan, B., Chen, K., Jiang, Z., Zhou, C., Cao, J., et al. (2018). Resveratrol-induced downregulation of NAF-1 enhances the sensitivity of pancreatic cancer cells to gemcitabine via the ROS/Nrf2 signaling pathways. Oxid. Med. Cell. Longev. 2018, 9482018. doi:10.1155/2018/9482018
Choura, M., and Rebaï, A. (2011). Receptor tyrosine kinases: From biology to pathology. J. Recept. Signal Transduct. Res. 31 (6), 387–394. doi:10.3109/10799893.2011.625425
Ciesek, S., von Hahn, T., Colpitts, C. C., Schang, L. M., Friesland, M., Steinmann, J., et al. (2011). The green tea polyphenol, epigallocatechin-3-gallate, inhibits hepatitis C virus entry. Hepatology 54 (6), 1947–1955. doi:10.1002/hep.24610
Coker-Gurkan, A., Celik, M., Ugur, M., Arisan, E. D., Obakan-Yerlikaya, P., Durdu, Z. B., et al. (2018). Curcumin inhibits autocrine growth hormone-mediated invasion and metastasis by targeting NF-κB signaling and polyamine metabolism in breast cancer cells. Amino Acids 50 (8), 1045–1069. doi:10.1007/s00726-018-2581-z
Corveloni, A. C., Semprebon, S. C., Baranoski, A., Biazi, B. I., Zanetti, T. A., and Mantovani, M. S. (2020). Carnosic acid exhibits antiproliferative and proapoptotic effects in tumoral NCI-H460 and nontumoral IMR-90 lung cells. J. Toxicol. Environ. Health. A 83 (10), 412–421. doi:10.1080/15287394.2020.1767741
Coyne, C. P., and Narayanan, L. (2019). Carnosic acid, tangeretin, and ginkgolide-B anti-neoplastic cytotoxicity in dual combination with dexamethasone-[anti-EGFR] in pulmonary adenocarcinoma (A549). Anticancer. Agents Med. Chem. 19 (6), 802–819. doi:10.2174/1871520619666181204100226
Crowder, R. N., and El-Deiry, W. S. (2012). Caspase-8 regulation of TRAIL-mediated cell death. Exp. Oncol. 34 (3), 160–164.
D'Alesio, C., Bellese, G., Gagliani, M. C., Aiello, C., Grasselli, E., Marcocci, G., et al. (2017). Cooperative antitumor activities of carnosic acid and Trastuzumab in ERBB2+ breast cancer cells. J. Exp. Clin. Cancer Res. 36 (1), 154. doi:10.1186/s13046-017-0615-0
Dastpeyman, M., Motamed, N., Azadmanesh, K., Mostafavi, E., Kia, V., Jahanian-Najafabadi, A., et al. (2012). Inhibition of silibinin on migration and adhesion capacity of human highly metastatic breast cancer cell line, MDA-MB-231, by evaluation of β1-integrin and downstream molecules, Cdc42, Raf-1 and D4GDI. Med. Oncol. 29 (4), 2512–2518. doi:10.1007/s12032-011-0113-8
De Leo, V. A., Horlick, H., Hanson, D., Eisinger, M., and Harber, L. C. (1984). Ultraviolet radiation induces changes in membrane metabolism of human keratinocytes in culture. J. Invest. Dermatol. 83 (5), 323–326. doi:10.1111/1523-1747.ep12264114
de Oliveira, M. R. (2018). Carnosic acid as a promising agent in protecting mitochondria of brain cells. Mol. Neurobiol. 55 (8), 6687–6699. doi:10.1007/s12035-017-0842-6
de Oliveira, M. R., Duarte, A. R., Chenet, A. L., de Almeida, F. J. S., and Andrade, C. M. B. (2019). Carnosic acid pretreatment attenuates mitochondrial dysfunction in SH-SY5Y cells in an experimental model of glutamate-induced excitotoxicity. Neurotox. Res. 36 (3), 551–562. doi:10.1007/s12640-019-00044-8
de Oliveira, M. R., Peres, A., Ferreira, G. C., Schuck, P. F., and Bosco, S. M. (2016). Carnosic acid affords mitochondrial protection in chlorpyrifos-treated sh-sy5y cells. Neurotox. Res. 30 (3), 367–379. doi:10.1007/s12640-016-9620-x
de Souza, I. C. C., Gobbo, R. C. B., de Almeida, F. J. S., Luckachaki, M. D., and de Oliveira, M. R. (2021). Carnosic acid depends on glutathione to promote mitochondrial protection in methylglyoxal-exposed SH-SY5Y cells. Metab. Brain Dis. 36 (3), 471–481. doi:10.1007/s11011-020-00651-x
Deep, G., Kumar, R., Jain, A. K., Agarwal, C., and Agarwal, R. (2014). Silibinin inhibits fibronectin induced motility, invasiveness and survival in human prostate carcinoma PC3 cells via targeting integrin signaling. Mutat. Res. 768, 35–46. doi:10.1016/j.mrfmmm.2014.05.002
Deng, D., and Shah, K. (2020). TRAIL of hope meeting resistance in cancer. Trends Cancer 6 (12), 989–1001. doi:10.1016/j.trecan.2020.06.006
Desgrosellier, J. S., and Cheresh, D. A. (2010). Integrins in cancer: Biological implications and therapeutic opportunities. Nat. Rev. Cancer 10 (1), 9–22. doi:10.1038/nrc2748
Di Petrillo, A., Orrù, G., Fais, A., and Fantini, M. C. (2022). Quercetin and its derivates as antiviral potentials: A comprehensive review. Phytother. Res. 36 (1), 266–278. doi:10.1002/ptr.7309
Diaz-Gerevini, G. T., Repossi, G., Dain, A., Tarres, M. C., Das, U. N., and Eynard, A. R. (2016). Beneficial action of resveratrol: How and why? Nutrition 32 (2), 174–178. doi:10.1016/j.nut.2015.08.017
Docherty, J. J., Fu, M. M., Hah, J. M., Sweet, T. J., Faith, S. A., and Booth, T. (2005). Effect of resveratrol on herpes simplex virus vaginal infection in the mouse. Antivir. Res. 67 (3), 155–162. doi:10.1016/j.antiviral.2005.06.008
Docherty, J. J., Smith, J. S., Fu, M. M., Stoner, T., and Booth, T. (2004). Effect of topically applied resveratrol on cutaneous herpes simplex virus infections in hairless mice. Antivir. Res. 61 (1), 19–26. doi:10.1016/j.antiviral.2003.07.001
Donmez, D. B., Kacar, S., Bagci, R., and Sahinturk, V. (2020). Protective effect of carnosic acid on acrylamide-induced liver toxicity in rats: Mechanistic approach over Nrf2-Keap1 pathway. J. Biochem. Mol. Toxicol. 34, e22524. doi:10.1002/jbt.22524
Dou, H., Shen, R., Tao, J., Huang, L., Shi, H., Chen, H., et al. (2017). Curcumin suppresses the colon cancer proliferation by inhibiting wnt/β-catenin pathways via miR-130a. Front. Pharmacol. 8, 877. doi:10.3389/fphar.2017.00877
Ebrahimi, M., Babaei, E., Neri, F., and Feizi, M. A. H. (2021). Anti-proliferative and apoptotic effect of gemini curcumin in p53-wild type and p53-mutant colorectal cancer cell lines. Int. J. Pharm. 601, 120592. doi:10.1016/j.ijpharm.2021.120592
El-Readi, M. Z., Eid, S., Abdelghany, A. A., Al-Amoudi, H. S., Efferth, T., and Wink, M. (2019). Resveratrol mediated cancer cell apoptosis, and modulation of multidrug resistance proteins and metabolic enzymes. Phytomedicine 55, 269–281. doi:10.1016/j.phymed.2018.06.046
Engels, I. H., Totzke, G., Fischer, U., Schulze-Osthoff, K., and Jänicke, R. U. (2005). Caspase-10 sensitizes breast carcinoma cells to TRAIL-induced but not tumor necrosis factor-induced apoptosis in a caspase-3-dependent manner. Mol. Cell. Biol. 25 (7), 2808–2818. doi:10.1128/MCB.25.7.2808-2818.2005
Erdogan, S., Doganlar, O., Doganlar, Z. B., Serttas, R., Turkekul, K., Dibirdik, I., et al. (2016). The flavonoid apigenin reduces prostate cancer CD44(+) stem cell survival and migration through PI3K/Akt/NF-κB signaling. Life Sci. 162, 77–86. doi:10.1016/j.lfs.2016.08.019
Erlank, H., Elmann, A., Kohen, R., and Kanner, J. (2011). Polyphenols activate Nrf2 in astrocytes via H2O2, semiquinones, and quinones. Free Radic. Biol. Med. 51 (12), 2319–2327. doi:10.1016/j.freeradbiomed.2011.09.033
Falschlehner, C., Schaefer, U., and Walczak, H. (2009). Following TRAIL's path in the immune system. Immunology 127 (2), 145–154. doi:10.1111/j.1365-2567.2009.03058.x
Farjadmand, F., Karimpour-Razkenari, E., Nabavi, S. M., Ardekani, M. R. S., and Saeedi, M. (2021). Plant polyphenols: Natural and potent UV-protective agents for the prevention and treatment of skin disorders. Mini Rev. Med. Chem. 21 (5), 576–585. doi:10.2174/1389557520666201109121246
Fatima, K., Mathew, S., Suhail, M., Ali, A., Damanhouri, G., Azhar, E., et al. (2014). Docking studies of Pakistani HCV NS3 helicase: A possible antiviral drug target. PLoS One 9 (9), e106339. doi:10.1371/journal.pone.0106339
Ferri, P., Angelino, D., Gennari, L., Benedetti, S., Ambrogini, P., Del Grande, P., et al. (2015). Enhancement of flavonoid ability to cross the blood-brain barrier of rats by co-administration with α-tocopherol. Food Funct. 6 (2), 394–400. doi:10.1039/c4fo00817k
Fischer, N., Seo, E. J., and Efferth, T. (2018). Prevention from radiation damage by natural products. Phytomedicine 47, 192–200. doi:10.1016/j.phymed.2017.11.005
Fu, X., Li, M., Tang, C., Huang, Z., and Najafi, M. (2021). Targeting of cancer cell death mechanisms by resveratrol: A review. Apoptosis. 26 (11-12), 561–573. doi:10.1007/s10495-021-01689-7
Gan, R. Y., Li, H. B., Sui, Z. Q., and Corke, H. (2018). Absorption, metabolism, anti-cancer effect and molecular targets of epigallocatechin gallate (EGCG): An updated review. Crit. Rev. Food Sci. Nutr. 58 (6), 924–941. doi:10.1080/10408398.2016.1231168
García-Zepeda, S. P., García-Villa, E., Díaz-Chávez, J., Hernández-Pando, R., and Gariglio, P. (2013). Resveratrol induces cell death in cervical cancer cells through apoptosis and autophagy. Eur. J. Cancer Prev. 22 (6), 577–584. doi:10.1097/CEJ.0b013e328360345f
Gee, J. R., Saltzstein, D. R., Kim, K., Kolesar, J., Huang, W., Havighurst, T. C., et al. (2017). A phase II randomized, double-blind, presurgical trial of polyphenon E in bladder cancer patients to evaluate pharmacodynamics and bladder tissue biomarkers. Cancer Prev. Res. 10 (5), 298–307. doi:10.1158/1940-6207.CAPR-16-0167
Gupta, S. C., Patchva, S., and Aggarwal, B. B. (2013). Therapeutic roles of curcumin: Lessons learned from clinical trials. AAPS J. 15 (1), 195–218. doi:10.1208/s12248-012-9432-8
Haley, C. T., Mui, U. N., Vangipuram, R., Rady, P. L., and Tyring, S. K. (2019). Human oncoviruses: Mucocutaneous manifestations, pathogenesis, therapeutics, and prevention: Papillomaviruses and Merkel cell polyomavirus. J. Am. Acad. Dermatol. 81 (1), 1–21. doi:10.1016/j.jaad.2018.09.062
Han, M. A., Lee, D. H., Woo, S. M., Seo, B. R., Min, K. J., Kim, S., et al. (2016). Galangin sensitizes TRAIL-induced apoptosis through down-regulation of anti-apoptotic proteins in renal carcinoma Caki cells. Sci. Rep. 6, 18642. doi:10.1038/srep18642
Haq, I. U., Imran, M., Nadeem, M., Tufail, T., Gondal, T. A., and Mubarak, M. S. (2021). Piperine: A review of its biological effects. Phytother. Res. 35 (2), 680–700. doi:10.1002/ptr.6855
Hasei, S., Yamamotoya, T., Nakatsu, Y., Ohata, Y., Itoga, S., Nonaka, Y., et al. (2021). Carnosic acid and carnosol activate AMPK, suppress expressions of gluconeogenic and lipogenic genes, and inhibit proliferation of HepG2 cells. Int. J. Mol. Sci. 22 (8), 4040. doi:10.3390/ijms22084040
Henning, S. M., Wang, P., Said, J. W., Huang, M., Grogan, T., Elashoff, D., et al. (2015). Randomized clinical trial of brewed green and black tea in men with prostate cancer prior to prostatectomy. Prostate 75 (5), 550–559. doi:10.1002/pros.22943
Hoffmann, J., Junker, H., Schmieder, A., Venz, S., Brandt, R., Multhoff, G., et al. (2011). EGCG downregulates IL-1RI expression and suppresses IL-1-induced tumorigenic factors in human pancreatic adenocarcinoma cells. Biochem. Pharmacol. 82 (9), 1153–1162. doi:10.1016/j.bcp.2011.07.063
Hosokawa, I., Hosokawa, Y., Ozaki, K., and Matsuo, T. (2020). Carnosic acid inhibits inflammatory cytokines production in human periodontal ligament cells. Immunopharmacol. Immunotoxicol. 42 (4), 373–378. doi:10.1080/08923973.2020.1782427
Huang, C. C., Cheng, Y. C., Lin, Y. C., Chou, C. H., Ho, C. T., Wang, H. K., et al. (2021). CSC-3436 sensitizes triple negative breast cancer cells to TRAIL-induced apoptosis through ROS-mediated p38/CHOP/death receptor 5 signaling pathways. Environ. Toxicol. 36 (12), 2578–2588. doi:10.1002/tox.23372
Huang, Y. J., Wang, K. L., Chen, H. Y., Chiang, Y. F., and Hsia, S. M. (2020). Protective effects of epigallocatechin gallate (EGCG) on endometrial, breast, and ovarian cancers. Biomolecules 10 (11), 1481. doi:10.3390/biom10111481
Hung, C. F., Huang, T. F., Chiang, H. S., and Wu, W. B. (2005). (-)-Epigallocatechin-3-gallate, a polyphenolic compound from green tea, inhibits fibroblast adhesion and migration through multiple mechanisms. J. Cell. Biochem. 96 (1), 183–197. doi:10.1002/jcb.20509
Imran, M., Aslam Gondal, T., Atif, M., Shahbaz, M., Batool Qaisarani, T., Hanif Mughal, M., et al. (2020). Apigenin as an anticancer agent. Phytother. Res. 34 (8), 1812–1828. doi:10.1002/ptr.6647
Ismail, B., Fagnere, C., Limami, Y., Ghezali, L., Pouget, C., Fidanzi, C., et al. (2015). 2'-Hydroxy-4-methylsulfonylchalcone enhances TRAIL-induced apoptosis in prostate cancer cells. Anticancer. Drugs 26 (1), 74–84. doi:10.1097/CAD.0000000000000163
Jeong, G. H., and Kim, T. H. (2021). Plasma-induced oxidation products of (-)-Epigallocatechin gallate with digestive enzymes inhibitory effects. Molecules 26 (19), 5799. doi:10.3390/molecules26195799
Kawai, K., Tsuno, N. H., Kitayama, J., Okaji, Y., Yazawa, K., Asakage, M., et al. (2004). Epigallocatechin gallate attenuates adhesion and migration of CD8+ T cells by binding to CD11b. J. Allergy Clin. Immunol. 113 (6), 1211–1217. doi:10.1016/j.jaci.2004.02.044
Khan, A. Q., Khan, R., Rehman, M. U., Lateef, A., Tahir, M., Ali, F., et al. (2012). Soy isoflavones (daidzein & genistein) inhibit 12-O-tetradecanoylphorbol-13-acetate (TPA)-induced cutaneous inflammation via modulation of COX-2 and NF-κB in Swiss albino mice. Toxicology 302 (2-3), 266–274. doi:10.1016/j.tox.2012.08.008
Khan, H., Ullah, H., Castilho, P. C. M. F., Gomila, A. S., D'Onofrio, G., Filosa, R., et al. (2020). Targeting NF-κB signaling pathway in cancer by dietary polyphenols. Crit. Rev. Food Sci. Nutr. 60 (16), 2790–2800. doi:10.1080/10408398.2019.1661827
Khan, N., and Mukhtar, H. (2018). Tea polyphenols in promotion of human health. Nutrients 11 (1), 39. doi:10.3390/nu11010039
Kim, B., Seo, J. H., Lee, K. Y., and Park, B. (2020). Icariin sensitizes human colon cancer cells to TRAIL-induced apoptosis via ERK-mediated upregulation of death receptors. Int. J. Oncol. 56 (3), 821–834. doi:10.3892/ijo.2020.4970
Kim, D. H., Park, K. W., Chae, I. G., Kundu, J., Kim, E. H., Kundu, J. K., et al. (2016). Carnosic acid inhibits STAT3 signaling and induces apoptosis through generation of ROS in human colon cancer HCT116 cells. Mol. Carcinog. 55 (6), 1096–1110. doi:10.1002/mc.22353
Kim, H. I., Lee, S. J., Choi, Y. J., Kim, M. J., Kim, T. Y., and Ko, S. G. (2021). Quercetin induces apoptosis in glioblastoma cells by suppressing axl/IL-6/STAT3 signaling pathway. Am. J. Chin. Med. 49 (3), 767–784. doi:10.1142/S0192415X21500361
Kim, K. C., Choi, E. H., and Lee, C. (2014). Axl receptor tyrosine kinase is a novel target of apigenin for the inhibition of cell proliferation. Int. J. Mol. Med. 34 (2), 592–598. doi:10.3892/ijmm.2014.1804
Klein, R. D., and Fischer, S. M. (2002). Black tea polyphenols inhibit IGF-I-induced signaling through Akt in normal prostate epithelial cells and Du145 prostate carcinoma cells. Carcinogenesis 23 (1), 217–221. doi:10.1093/carcin/23.1.217
Kuo, W. T., Tsai, Y. C., Wu, H. C., Ho, Y. J., Chen, Y. S., Yao, C. H., et al. (2015). Radiosensitization of non-small cell lung cancer by kaempferol. Oncol. Rep. 34 (5), 2351–2356. doi:10.3892/or.2015.4204
Lambert, J. D., Sang, S., Hong, J., Kwon, S. J., Lee, M. J., Ho, C. T., et al. (2006). Peracetylation as a means of enhancing in vitro bioactivity and bioavailability of epigallocatechin-3-gallate. Drug Metab. Dispos. 34 (12), 2111–2116. doi:10.1124/dmd.106.011460
Larsen, C. A., Dashwood, R. H., and Bisson, W. H. (2010). Tea catechins as inhibitors of receptor tyrosine kinases: Mechanistic insights and human relevance. Pharmacol. Res. 62 (6), 457–464. doi:10.1016/j.phrs.2010.07.010
Lecumberri, E., Dupertuis, Y. M., Miralbell, R., and Pichard, C. (2013). Green tea polyphenol epigallocatechin-3-gallate (EGCG) as adjuvant in cancer therapy. Clin. Nutr. 32 (6), 894–903. doi:10.1016/j.clnu.2013.03.008
Lee, L. T., Huang, Y. T., Hwang, J. J., Lee, A. Y., Ke, F. C., Huang, C. J., et al. (2004). Transinactivation of the epidermal growth factor receptor tyrosine kinase and focal adhesion kinase phosphorylation by dietary flavonoids: Effect on invasive potential of human carcinoma cells. Biochem. Pharmacol. 67 (11), 2103–2114. doi:10.1016/j.bcp.2004.02.023
Lee, S. J., Krauthauser, C., Maduskuie, V., Fawcett, P. T., Olson, J. M., and Rajasekaran, S. A. (2011). Curcumin-induced HDAC inhibition and attenuation of medulloblastoma growth in vitro and in vivo. BMC Cancer 11, 144. doi:10.1186/1471-2407-11-144
Lee, W. J., Cheng, T. C., Yen, Y., Fang, C. L., Liao, Y. C., Kuo, C. C., et al. (2021). Tea polyphenol epigallocatechin-3-gallate inhibits cell proliferation in a patient-derived triple-negative breast cancer xenograft mouse model via inhibition of proline-dehydrogenase-induced effects. J. Food Drug Anal. 29 (1), 113–127. doi:10.38212/2224-6614.3230
Lee, Y. J., Lim, T., Han, M. S., Lee, S. H., Baek, S. H., Nan, H. Y., et al. (2017). Anticancer effect of luteolin is mediated by downregulation of TAM receptor tyrosine kinases, but not interleukin-8, in non-small cell lung cancer cells. Oncol. Rep. 37 (2), 1219–1226. doi:10.3892/or.2016.5336
Leonardi, G. C., Falzone, L., Salemi, R., Zanghì, A., Spandidos, D. A., Mccubrey, J. A., et al. (2018). Cutaneous melanoma: From pathogenesis to therapy (Review). Int. J. Oncol. 52 (4), 1071–1080. doi:10.3892/ijo.2018.4287
Lestari, M. L., and Indrayanto, G. (2014). Curcumin. Profiles Drug Subst. Excip. Relat. Methodol. 39, 113–204. doi:10.1016/B978-0-12-800173-8.00003-9
Leyva-López, N., Gutierrez-Grijalva, E. P., Ambriz-Perez, D. L., and Heredia, J. B. (2016). Flavonoids as cytokine modulators: A possible therapy for inflammation-related diseases. Int. J. Mol. Sci. 17 (6), 921. doi:10.3390/ijms17060921
Li, P., Li, S., Yin, D., Li, J., Wang, L., Huang, C., et al. (2017). EGCG sensitizes human nasopharyngeal carcinoma cells to TRAIL-mediated apoptosis by activation NF-κB. Neoplasma 64 (1), 74–80. doi:10.4149/neo_2017_109
Li, W., Yu, X., Ma, X., Xie, L., Xia, Z., Liu, L., et al. (2018). Deguelin attenuates non-small cell lung cancer cell metastasis through inhibiting the CtsZ/FAK signaling pathway. Cell. Signal. 50, 131–141. doi:10.1016/j.cellsig.2018.07.001
Li, Y., Chen, F., Chen, J., Chan, S., He, Y., Liu, W., et al. (2020). Disulfiram/copper induces antitumor activity against both nasopharyngeal cancer cells and cancer-associated fibroblasts through ROS/MAPK and ferroptosis pathways. Cancers (Basel) 12 (1), 138. doi:10.3390/cancers12010138
Liang, Y., Kong, D., Zhang, Y., Li, S., Li, Y., Dong, L., et al. (2021). Curcumin inhibits the viability, migration and invasion of papillary thyroid cancer cells by regulating the miR-301a-3p/STAT3 axis. Exp. Ther. Med. 22 (2), 875. doi:10.3892/etm.2021.10307
Liu, D., Wang, B., Zhu, Y., Yan, F., and Dong, W. (2018). Carnosic acid regulates cell proliferation and invasion in chronic myeloid leukemia cancer cells via suppressing microRNA-708. J. BUON 23 (3), 741–746.
Liu, S., Dong, Y., Wang, Y., Hu, P., Wang, J., and Wang, R. Y. (2021). Pristimerin exerts antitumor activity against MDA-MB-231 triple-negative breast cancer cells by reversing of epithelial-mesenchymal transition via downregulation of integrin β3. Biomed. J. 44 (6), S84–S92. doi:10.1016/j.bj.2020.07.004
Liu, W., Wu, T. C., Hong, D. M., Hu, Y., Fan, T., Guo, W. J., et al. (2018). Carnosic acid enhances the anti-lung cancer effect of cisplatin by inhibiting myeloid-derived suppressor cells. Chin. J. Nat. Med. 16 (12), 907–915. doi:10.1016/S1875-5364(18)30132-8
Ma, Y., Wang, J., Liu, L., Zhu, H., Chen, X., Pan, S., et al. (2011). Genistein potentiates the effect of arsenic trioxide against human hepatocellular carcinoma: Role of Akt and nuclear factor-κB. Cancer Lett. 301 (1), 75–84. doi:10.1016/j.canlet.2010.10.022
Maiuri, M. C., Zalckvar, E., Kimchi, A., and Kroemer, G. (2007). Self-eating and self-killing: Crosstalk between autophagy and apoptosis. Nat. Rev. Mol. Cell Biol. 8 (9), 741–752. doi:10.1038/nrm2239
Mallet, J. F., Shahbazi, R., Alsadi, N., and Matar, C. (2021). Polyphenol-enriched blueberry preparation controls breast cancer stem cells by targeting FOXO1 and miR-145. Molecules 26 (14), 4330. doi:10.3390/molecules26144330
Man, S., Luo, C., Yan, M., Zhao, G., Ma, L., and Gao, W. (2021). Treatment for liver cancer: From sorafenib to natural products. Eur. J. Med. Chem. 224, 113690. doi:10.1016/j.ejmech.2021.113690
Mao, X., Zhang, X., Zheng, X., Chen, Y., Xuan, Z., and Huang, P. (2021). Curcumin suppresses LGR5(+) colorectal cancer stem cells by inducing autophagy and via repressing TFAP2A-mediated ECM pathway. J. Nat. Med. 75 (3), 590–601. doi:10.1007/s11418-021-01505-1
Matito, C., Agell, N., Sanchez-Tena, S., Torres, J. L., and Cascante, M. (2011). Protective effect of structurally diverse grape procyanidin fractions against UV-induced cell damage and death. J. Agric. Food Chem. 59 (9), 4489–4495. doi:10.1021/jf103692a
Mehrbod, P., Hudy, D., Shyntum, D., Markowski, J., Łos, M. J., and Ghavami, S. (2020). Quercetin as a natural therapeutic candidate for the treatment of influenza virus. Biomolecules 11 (1), 10. doi:10.3390/biom11010010
Min, F., Liu, X., Li, Y., Dong, M., Qu, Y., and Liu, W. (2021). Carnosic acid suppresses the development of oral squamous cell carcinoma via mitochondrial-mediated apoptosis. Front. Oncol. 11, 760861. doi:10.3389/fonc.2021.760861
Min, H. Y., Jung, Y., Park, K. H., Oh, W. K., and Lee, H. Y. (2018). Erybraedin A is a potential Src inhibitor that blocks the adhesion and viability of non-small-cell lung cancer cells. Biochem. Biophys. Res. Commun. 502 (1), 145–151. doi:10.1016/j.bbrc.2018.05.137
Min, K. J., and Kwon, T. K. (2014). Anticancer effects and molecular mechanisms of epigallocatechin-3-gallate. Integr. Med. Res. 3 (1), 16–24. doi:10.1016/j.imr.2013.12.001
Moon, J. H., Eo, S. K., Lee, J. H., and Park, S. Y. (2015). Quercetin-induced autophagy flux enhances TRAIL-mediated tumor cell death. Oncol. Rep. 34 (1), 375–381. doi:10.3892/or.2015.3991
Moore, J., Yousef, M., and Tsiani, E. (2016). Anticancer effects of rosemary (rosmarinus officinalis L.) extract and rosemary extract polyphenols. Nutrients 8 (11), 731. doi:10.3390/nu8110731
Mui, U. N., Haley, C. T., Vangipuram, R., and Tyring, S. K. (2019). Human oncoviruses: Mucocutaneous manifestations, pathogenesis, therapeutics, and prevention: Hepatitis viruses, human T-cell leukemia viruses, herpesviruses, and Epstein-Barr virus. J. Am. Acad. Dermatol. 81 (1), 23–41. doi:10.1016/j.jaad.2018.10.072
Murapa, P., Dai, J., Chung, M., Mumper, R. J., and D'Orazio, J. (2012). Anthocyanin-rich fractions of blackberry extracts reduce UV-induced free radicals and oxidative damage in keratinocytes. Phytother. Res. 26 (1), 106–112. doi:10.1002/ptr.3510
Namiki, K., Wongsirisin, P., Yokoyama, S., Sato, M., Rawangkan, A., Sakai, R., et al. (2020). (-)-Epigallocatechin gallate inhibits stemness and tumourigenicity stimulated by AXL receptor tyrosine kinase in human lung cancer cells. Sci. Rep. 10 (1), 2444. doi:10.1038/s41598-020-59281-z
NavaneethaKrishnan, S., Rosales, J. L., and Lee, K. Y. (2019). ROS-mediated cancer cell killing through dietary phytochemicals. Oxid. Med. Cell. Longev. 2019, 9051542. doi:10.1155/2019/9051542
Nicholas, C., Batra, S., Vargo, M. A., Voss, O. H., Gavrilin, M. A., Wewers, M. D., et al. (2007). Apigenin blocks lipopolysaccharide-induced lethality in vivo and proinflammatory cytokines expression by inactivating NF-kappaB through the suppression of p65 phosphorylation. J. Immunol. 179 (10), 7121–7127. doi:10.4049/jimmunol.179.10.7121
Niedzwiecki, A., Roomi, M. W., Kalinovsky, T., and Rath, M. (2016). Anticancer efficacy of polyphenols and their combinations. Nutrients 8 (9), 552. doi:10.3390/nu8090552
Nile, S. H., Kim, D. H., Nile, A., Park, G. S., Gansukh, E., and Kai, G. (2020). Probing the effect of quercetin 3-glucoside from Dianthus superbus L against influenza virus infection- in vitro and in silico biochemical and toxicological screening. Food Chem. Toxicol. 135, 110985. doi:10.1016/j.fct.2019.110985
Nishikawa, H., Wakano, K., and Kitani, S. (2007). Inhibition of NADPH oxidase subunits translocation by tea catechin EGCG in mast cell. Biochem. Biophys. Res. Commun. 362 (2), 504–509. doi:10.1016/j.bbrc.2007.08.015
Oalđe Pavlović, M., Kolarević, S., Đorđević, J., Jovanović Marić, J., Lunić, T., Mandić, M., et al. (2021). A study of phytochemistry, genoprotective activity, and antitumor effects of extracts of the selected lamiaceae species. Plants (Basel) 10 (11), 2306. doi:10.3390/plants10112306
Ohishi, T., Goto, S., Monira, P., Isemura, M., and Nakamura, Y. (2016). Anti-inflammatory action of green tea. Antiinflamm. Antiallergy. Agents Med. Chem. 15 (2), 74–90. doi:10.2174/1871523015666160915154443
Özsoy, S., Becer, E., Kabadayı, H., Vatansever, H. S., and Yücecan, S. (2020). Quercetin-mediated apoptosis and cellular senescence in human colon cancer. Anticancer. Agents Med. Chem. 20 (11), 1387–1396. doi:10.2174/1871520620666200408082026
Pang, J. Y., Zhao, K. J., Wang, J. B., Ma, Z. J., and Xiao, X. H. (2014). Green tea polyphenol, epigallocatechin-3-gallate, possesses the antiviral activity necessary to fight against the Hepatitis B virus replication in vitro. J. Zhejiang Univ. Sci. B 15 (6), 533–539. doi:10.1631/jzus.B1300307
Park, D., Ha, I. J., Park, S. Y., Choi, M., Lim, S. L., Kim, S. H., et al. (2016). Morusin induces TRAIL sensitization by regulating EGFR and DR5 in human glioblastoma cells. J. Nat. Prod. 79 (2), 317–323. doi:10.1021/acs.jnatprod.5b00919
Park, S. Y., Song, H., Sung, M. K., Kang, Y. H., Lee, K. W., and Park, J. H. (2014). Carnosic acid inhibits the epithelial-mesenchymal transition in B16F10 melanoma cells: A possible mechanism for the inhibition of cell migration. Int. J. Mol. Sci. 15 (7), 12698–12713. doi:10.3390/ijms150712698
Passildas-Jahanmohan, J., Eymard, J. C., Pouget, M., Kwiatkowski, F., Van Praagh, I., Savareux, L., et al. (2021). Multicenter randomized phase II study comparing docetaxel plus curcumin versus docetaxel plus placebo in first-line treatment of metastatic castration-resistant prostate cancer. Cancer Med. 10 (7), 2332–2340. doi:10.1002/cam4.3806
Patra, S., Pradhan, B., Nayak, R., Behera, C., Rout, L., Jena, M., et al. (2021). Chemotherapeutic efficacy of curcumin and resveratrol against cancer: Chemoprevention, chemoprotection, drug synergism and clinical pharmacokinetics. Semin. Cancer Biol. 73, 310–320. doi:10.1016/j.semcancer.2020.10.010
Patterson, A. D., Gonzalez, F. J., Perdew, G. H., and Peters, J. M. (2018). Molecular regulation of carcinogenesis: Friend and foe. Toxicol. Sci. 165 (2), 277–283. doi:10.1093/toxsci/kfy185
Pavan, A. R., Silva, G. D., Jornada, D. H., Chiba, D. E., Fernandes, G. F., Man Chin, C., et al. (2016). Unraveling the anticancer effect of curcumin and resveratrol. Nutrients 8 (11), 628. doi:10.3390/nu8110628
Pervin, M., Unno, K., Takagaki, A., Isemura, M., and Nakamura, Y. (2019). Function of green tea catechins in the brain: Epigallocatechin gallate and its metabolites. Int. J. Mol. Sci. 20 (15), 3630. doi:10.3390/ijms20153630
Peters, J. M., and Gonzalez, F. J. (2018). The evolution of carcinogenesis. Toxicol. Sci. 165 (2), 272–276. doi:10.1093/toxsci/kfy184
Peterson, G., and Barnes, S. (1993). Genistein and biochanin A inhibit the growth of human prostate cancer cells but not epidermal growth factor receptor tyrosine autophosphorylation. Prostate 22 (4), 335–345. doi:10.1002/pros.2990220408
Petiwala, S. M., Li, G., Bosland, M. C., Lantvit, D. D., Petukhov, P. A., and Johnson, J. J. (2016). Carnosic acid promotes degradation of the androgen receptor and is regulated by the unfolded protein response pathway in vitro and in vivo. Carcinogenesis 37 (8), 827–838. doi:10.1093/carcin/bgw052
Pilorget, A., Berthet, V., Luis, J., Moghrabi, A., Annabi, B., and Béliveau, R. (2003). Medulloblastoma cell invasion is inhibited by green tea (-)epigallocatechin-3-gallate. J. Cell. Biochem. 90 (4), 745–755. doi:10.1002/jcb.10667
Ponomareva, T., Merkushev, G., Ivanov, E., Bisenieks, E., and Dubur, G. (1985). Features of the intracellular repair of irradiated keratinocytes. Arkh. Anat. Gistol. Embriol. 88 (5), 66–71.
Potì, F., Santi, D., Spaggiari, G., Zimetti, F., and Zanotti, I. (2019). Polyphenol health effects on cardiovascular and neurodegenerative disorders: A review and meta-analysis. Int. J. Mol. Sci. 20 (2), 351. doi:10.3390/ijms20020351
Qiao, D., Xing, J., Duan, Y., Wang, S., Yao, G., Zhang, S., et al. (2022). The molecular mechanism of baicalein repressing progression of gastric cancer mediating miR-7/FAK/AKT signaling pathway. Phytomedicine. 100, 154046. doi:10.1016/j.phymed.2022.154046
Rangel-Ordóñez, L., Nöldner, M., Schubert-Zsilavecz, M., and Wurglics, M. (2010). Plasma levels and distribution of flavonoids in rat brain after single and repeated doses of standardized Ginkgo biloba extract EGb 761®. Planta Med. 76 (15), 1683–1690. doi:10.1055/s-0030-1249962
Rastogi, N., Duggal, S., Singh, S. K., Porwal, K., Srivastava, V. K., Maurya, R., et al. (2015). Proteasome inhibition mediates p53 reactivation and anti-cancer activity of 6-gingerol in cervical cancer cells. Oncotarget 6 (41), 43310–43325. doi:10.18632/oncotarget.6383
Rauf, A., Imran, M., Butt, M. S., Nadeem, M., Peters, D. G., and Mubarak, M. S. (2018). Resveratrol as an anti-cancer agent: A review. Crit. Rev. Food Sci. Nutr. 58 (9), 1428–1447. doi:10.1080/10408398.2016.1263597
Ravindran Menon, D., Li, Y., Yamauchi, T., Osborne, D. G., Vaddi, P. K., Wempe, M. F., et al. (2021). EGCG inhibits tumor growth in melanoma by targeting JAK-STAT signaling and its downstream PD-L1/PD-L2-PD1 Axis in tumors and enhancing cytotoxic T-cell responses. Pharm. (Basel) 14 (11), 1081. doi:10.3390/ph14111081
Ravindranath, M. H., Muthugounder, S., Presser, N., and Viswanathan, S. (2004). Anticancer therapeutic potential of soy isoflavone, genistein. Adv. Exp. Med. Biol. 546, 121–165. doi:10.1007/978-1-4757-4820-8_11
Reed, J. D., and de Freitas, V. (2020). Polyphenol chemistry: Implications for nutrition, health, and the environment. J. Agric. Food Chem. 68 (10), 2833–2835. doi:10.1021/acs.jafc.9b07948
Ren, B., Kwah, M. X., Liu, C., Ma, Z., Shanmugam, M. K., Ding, L., et al. (2021). Resveratrol for cancer therapy: Challenges and future perspectives. Cancer Lett. 515, 63–72. doi:10.1016/j.canlet.2021.05.001
Rho, J. K., Choi, Y. J., Jeon, B. S., Choi, S. J., Cheon, G. J., Woo, S. K., et al. (2010). Combined treatment with silibinin and epidermal growth factor receptor tyrosine kinase inhibitors overcomes drug resistance caused by T790M mutation. Mol. Cancer Ther. 9 (12), 3233–3243. doi:10.1158/1535-7163.MCT-10-0625
Rigby, C. M., Roy, S., Deep, G., Guillermo-Lagae, R., Jain, A. K., Dhar, D., et al. (2017). Role of p53 in silibinin-mediated inhibition of ultraviolet B radiation-induced DNA damage, inflammation and skin carcinogenesis. Carcinogenesis 38 (1), 40–50. doi:10.1093/carcin/bgw106
Rogovskii, V. (2022). Polyphenols as the potential disease-modifying therapy in cancer. Anticancer. Agents Med. Chem. 22 (13), 2385–2392. doi:10.2174/1871520622666220201105204
Rojas-Ochoa, A., Córdova, E. J., Carrillo-García, A., Lizano, M., Pedraza-Chaverri, J., Patiño, N., et al. (2021). The polyphenols α-mangostin and nordihydroguaiaretic acid induce oxidative stress, cell cycle arrest, and apoptosis in a cellular model of medulloblastoma. Molecules 26 (23), 7230. doi:10.3390/molecules26237230
Romano, A., and Martel, F. (2021). The role of EGCG in breast cancer prevention and therapy. Mini Rev. Med. Chem. 21 (7), 883–898. doi:10.2174/1389557520999201211194445
Ruan, J., Zhang, L., Yan, L., Liu, Y., Yue, Z., Chen, L., et al. (2012). Inhibition of hypoxia-induced epithelial mesenchymal transition by luteolin in non-small cell lung cancer cells. Mol. Med. Rep. 6 (1), 232–238. doi:10.3892/mmr.2012.884
Rudrapal, M., Khan, J., Dukhyil, A. A. B., Alarousy, R. M. I. I., Attah, E. I., Sharma, T., et al. (2021). Chalcone scaffolds, bioprecursors of flavonoids: Chemistry, bioactivities, and pharmacokinetics. Molecules 26 (23), 7177. doi:10.3390/molecules26237177
Sen, T., and Chatterjee, A. (2011). Epigallocatechin-3-gallate (EGCG) downregulates EGF-induced MMP-9 in breast cancer cells: Involvement of integrin receptor α5β1 in the process. Eur. J. Nutr. 50 (6), 465–478. doi:10.1007/s00394-010-0158-z
Serafini, M., Peluso, I., and Raguzzini, A. (2010). Flavonoids as anti-inflammatory agents. Proc. Nutr. Soc. 69 (3), 273–278. doi:10.1017/S002966511000162X
Shan, S., Lu, Y., Zhang, X., Shi, J., Li, H., and Li, Z. (2021). Inhibitory effect of bound polyphenol from foxtail millet bran on miR-149 methylation increases the chemosensitivity of human colorectal cancer HCT-8/Fu cells. Mol. Cell. Biochem. 476 (2), 513–523. doi:10.1007/s11010-020-03906-4
Shao, N., Mao, J., Xue, L., Wang, R., Zhi, F., and Lan, Q. (2022). Correction to: Carnosic acid potentiates the anticancer effect of temozolomide by inducing apoptosis and autophagy in glioma. J. Neurooncol. 158 (1), 129. doi:10.1007/s11060-022-04006-7
Shi, G., Zhang, Z., Fang, Y., Bian, D., and Bai, Z. (2021). Curcumin combined with low-intensity ultrasound suppresses the growth of glioma cells via inhibition of the AKT pathway. Neoplasma 68 (2), 290–297. doi:10.4149/neo_2020_200605N604
Shimizu, M., Adachi, S., Masuda, M., Kozawa, O., and Moriwaki, H. (2011). Cancer chemoprevention with green tea catechins by targeting receptor tyrosine kinases. Mol. Nutr. Food Res. 55 (6), 832–843. doi:10.1002/mnfr.201000622
Shimizu, M., Shirakami, Y., Sakai, H., Yasuda, Y., Kubota, M., Adachi, S., et al. (2010). (-)-Epigallocatechin gallate inhibits growth and activation of the VEGF/VEGFR axis in human colorectal cancer cells. Chem. Biol. Interact. 185 (3), 247–252. doi:10.1016/j.cbi.2010.03.036
Silva, C., Correia-Branco, A., Andrade, N., Ferreira, A. C., Soares, M. L., Sonveaux, P., et al. (2019). Selective pro-apoptotic and antimigratory effects of polyphenol complex catechin:lysine 1:2 in breast, pancreatic and colorectal cancer cell lines. Eur. J. Pharmacol. 859, 172533. doi:10.1016/j.ejphar.2019.172533
Singh, D., Tewari, M., Singh, S., and Narayan, G. (2021). Revisiting the role of TRAIL/TRAIL-R in cancer biology and therapy. Future Oncol. 17 (5), 581–596. doi:10.2217/fon-2020-0727
Singh, K., Tarapcsák, S., Gyöngy, Z., Ritter, Z., Batta, G., Bosire, R., et al. (2021). Effects of polyphenols on P-glycoprotein (ABCB1) activity. Pharmaceutics 13 (12), 2062. doi:10.3390/pharmaceutics13122062
Singla, R. K., Dubey, A. K., Garg, A., Sharma, R. K., Fiorino, M., Ameen, S. M., et al. (2019). Natural polyphenols: Chemical classification, definition of classes, subcategories, and structures. J. AOAC Int. 102 (5), 1397–1400. doi:10.5740/jaoacint.19-0133
Sojoodi, M., Wei, L., Erstad, D. J., Yamada, S., Fujii, T., Hirschfield, H., et al. (2020). Epigallocatechin gallate induces hepatic stellate cell senescence and attenuates development of hepatocellular carcinoma. Cancer Prev. Res. 13 (6), 497–508. doi:10.1158/1940-6207.CAPR-19-0383
Sonnenberg, A. (1993). Integrins and their ligands. Curr. Top. Microbiol. Immunol. 184, 7–35. doi:10.1007/978-3-642-78253-4_2
Sourivong, P., Schronerová, K., and Babincová, M. (2007). Scoparone inhibits ultraviolet radiation-induced lipid peroxidation. Z. Naturforsch. C J. Biosci. 62 (1-2), 61–64. doi:10.1515/znc-2007-1-211
Suh, Y. A., Jo, S. Y., Lee, H. Y., and Lee, C. (2015). Inhibition of IL-6/STAT3 axis and targeting Axl and Tyro3 receptor tyrosine kinases by apigenin circumvent taxol resistance in ovarian cancer cells. Int. J. Oncol. 46 (3), 1405–1411. doi:10.3892/ijo.2014.2808
Sun, S., and Fang, H. (2021). Curcumin inhibits ovarian cancer progression by regulating circ-PLEKHM3/miR-320a/SMG1 axis. J. Ovarian Res. 14 (1), 158. doi:10.1186/s13048-021-00916-8
Sun, X., Fu, P., Xie, L., Chai, S., Xu, Q., Zeng, L., et al. (2021). Resveratrol inhibits the progression of cervical cancer by suppressing the transcription and expression of HPV E6 and E7 genes. Int. J. Mol. Med. 47 (1), 335–345. doi:10.3892/ijmm.2020.4789
Talukdar, S., Emdad, L., Das, S. K., and Fisher, P. B. (2020). Egfr: An essential receptor tyrosine kinase-regulator of cancer stem cells. Adv. Cancer Res. 147, 161–188. doi:10.1016/bs.acr.2020.04.003
Tang, X., Ding, H., Liang, M., Chen, X., Yan, Y., Wan, N., et al. (2021). Curcumin induces ferroptosis in non-small-cell lung cancer via activating autophagy. Thorac. Cancer 12 (8), 1219–1230. doi:10.1111/1759-7714.13904
Taylor, M., Moore, S., Mourtas, S., Niarakis, A., Re, F., Zona, C., et al. (2011). Effect of curcumin-associated and lipid ligand-functionalized nanoliposomes on aggregation of the Alzheimer's Aβ peptide. Nanomedicine 7 (5), 541–550. doi:10.1016/j.nano.2011.06.015
Thapa, B., Kc, R., and Uludağ, H. (2020). TRAIL therapy and prospective developments for cancer treatment. J. Control. Release 326, 335–349. doi:10.1016/j.jconrel.2020.07.013
Thongsri, P., Pewkliang, Y., Borwornpinyo, S., Wongkajornsilp, A., Hongeng, S., and Sa-Ngiamsuntorn, K. (2021). Curcumin inhibited Hepatitis B viral entry through NTCP binding. Sci. Rep. 11 (1), 19125. doi:10.1038/s41598-021-98243-x
Trenker, R., and Jura, N. (2020). Receptor tyrosine kinase activation: From the ligand perspective. Curr. Opin. Cell Biol. 63, 174–185. doi:10.1016/j.ceb.2020.01.016
Tsai, Y. M., Yang, C. J., Hsu, Y. L., Wu, L. Y., Tsai, Y. C., Hung, J. Y., et al. (2011). Glabridin inhibits migration, invasion, and angiogenesis of human non-small cell lung cancer A549 cells by inhibiting the FAK/rho signaling pathway. Integr. Cancer Ther. 10 (4), 341–349. doi:10.1177/1534735410384860
Voss, O. H., Arango, D., Tossey, J. C., Villalona Calero, M. A., and Doseff, A. I. (2021). Splicing reprogramming of TRAIL/DISC-components sensitizes lung cancer cells to TRAIL-mediated apoptosis. Cell Death Dis. 12 (4), 287. doi:10.1038/s41419-021-03567-1
Walle, U. K., and Walle, T. (2007). Bioavailable flavonoids: Cytochrome P450-mediated metabolism of methoxyflavones. Drug Metab. Dispos. 35 (11), 1985–1989. doi:10.1124/dmd.107.016782
Wan, M., Tajuddin, W., Lajis, N., Abas, F., Othman, I., and Naidu, R. (2019). Mechanistic understanding of curcumin's therapeutic effects in lung cancer. Nutrients 11 (12), 2989. doi:10.3390/nu11122989
Wang, L., Wang, C., Tao, Z., Zhao, L., Zhu, Z., Wu, W., et al. (2019). Curcumin derivative WZ35 inhibits tumor cell growth via ROS-YAP-JNK signaling pathway in breast cancer. J. Exp. Clin. Cancer Res. 38 (1), 460. doi:10.1186/s13046-019-1424-4
Wang, N., Wu, Y., Bian, J., Qian, X., Lin, H., Sun, H., et al. (2017). Current development of ROS-modulating agents as novel antitumor therapy. Curr. Cancer Drug Targets 17 (2), 122–136. doi:10.2174/1568009616666160216125833
Wang, P., Hao, X., Li, X., Yan, Y., Tian, W., Xiao, L., et al. (2021). Curcumin inhibits adverse psychological stress-induced proliferation and invasion of glioma cells via down-regulating the ERK/MAPK pathway. J. Cell. Mol. Med. 25 (15), 7190–7203. doi:10.1111/jcmm.16749
Wang, Y., Huo, J., Zhang, D., Hu, G., and Zhang, Y. (2019). Chemerin/ChemR23 axis triggers an inflammatory response in keratinocytes through ROS-sirt1-NF-κB signaling. J. Cell. Biochem. 120 (4), 6459–6470. doi:10.1002/jcb.27936
Watson, M., Holman, D. M., and Maguire-Eisen, M. (2016). Ultraviolet radiation exposure and its impact on skin cancer risk. Semin. Oncol. Nurs. 32 (3), 241–254. doi:10.1016/j.soncn.2016.05.005
Weh, K. M., Zhang, Y., Howard, C. L., Howell, A. B., Clarke, J. L., and Kresty, L. A. (2022). Cranberry polyphenols in esophageal cancer inhibition: New insights. Nutrients 14 (5), 969. doi:10.3390/nu14050969
Wen, K., Fang, X., Yang, J., Yao, Y., Nandakumar, K. S., Salem, M. L., et al. (2021). Recent research on flavonoids and their biomedical applications. Curr. Med. Chem. 28 (5), 1042–1066. doi:10.2174/0929867327666200713184138
Wendt, J., von Haefen, C., Hemmati, P., Belka, C., Dörken, B., and Daniel, P. T. (2005). TRAIL sensitizes for ionizing irradiation-induced apoptosis through an entirely Bax-dependent mitochondrial cell death pathway. Oncogene 24 (25), 4052–4064. doi:10.1038/sj.onc.1208580
Wiseman, S., Mulder, T., and Rietveld, A. (2001). Tea flavonoids: Bioavailability in vivo and effects on cell signaling pathways in vitro. Antioxid. Redox Signal. 3 (6), 1009–1021. doi:10.1089/152308601317203549
Wu, B., Xiong, J., Zhou, Y., Wu, Y., Song, Y., Wang, N., et al. (2020). Luteolin enhances TRAIL sensitivity in non-small cell lung cancer cells through increasing DR5 expression and Drp1-mediated mitochondrial fission. Arch. Biochem. Biophys. 692, 108539. doi:10.1016/j.abb.2020.108539
Wu, H., Chen, L., Zhu, F., Han, X., Sun, L., and Chen, K. (2019). The cytotoxicity effect of resveratrol: Cell cycle arrest and induced apoptosis of breast cancer 4T1 cells. Toxins (Basel) 11 (12), 731. doi:10.3390/toxins11120731
Wu, N. L., Fang, J. Y., Chen, M., Wu, C. J., Huang, C. C., and Hung, C. F. (2011). Chrysin protects epidermal keratinocytes from UVA- and UVB-induced damage. J. Agric. Food Chem. 59 (15), 8391–8400. doi:10.1021/jf200931t
Xia, C., Zeng, H., and Zheng, Y. (2020). Low-intensity ultrasound enhances the antitumor effects of doxorubicin on hepatocellular carcinoma cells through the ROS-miR-21-PTEN axis. Mol. Med. Rep. 21 (3), 989–998. doi:10.3892/mmr.2020.10936
Xu, Y., Gao, C. C., Pan, Z. G., and Zhou, C. W. (2018). Irigenin sensitizes TRAIL-induced apoptosis via enhancing pro-apoptotic molecules in gastric cancer cells. Biochem. Biophys. Res. Commun. 496 (3), 998–1005. doi:10.1016/j.bbrc.2018.01.003
Yahfoufi, N., Alsadi, N., Jambi, M., and Matar, C. (2018). The immunomodulatory and anti-inflammatory role of polyphenols. Nutrients 10 (11), 1618. doi:10.3390/nu10111618
Ye, F., Yang, C., Kim, J., MacNevin, C. J., Hahn, K. M., Park, D., et al. (2017). Epigallocatechin gallate has pleiotropic effects on transmembrane signaling by altering the embedding of transmembrane domains. J. Biol. Chem. 292 (24), 9858–9864. doi:10.1074/jbc.C117.787309
Yiu, C. Y., Chen, S. Y., Chang, L. K., Chiu, Y. F., and Lin, T. P. (2010). Inhibitory effects of resveratrol on the Epstein-Barr virus lytic cycle. Molecules 15 (10), 7115–7124. doi:10.3390/molecules15107115
Yoshida, T., Konishi, M., Horinaka, M., Yasuda, T., Goda, A. E., Taniguchi, H., et al. (2008). Kaempferol sensitizes colon cancer cells to TRAIL-induced apoptosis. Biochem. Biophys. Res. Commun. 375 (1), 129–133. doi:10.1016/j.bbrc.2008.07.131
Youn, H., Jeong, J. C., Jeong, Y. S., Kim, E. J., and Um, S. J. (2013). Quercetin potentiates apoptosis by inhibiting nuclear factor-kappaB signaling in H460 lung cancer cells. Biol. Pharm. Bull. 36 (6), 944–951. doi:10.1248/bpb.b12-01004
Yousef, M., Vlachogiannis, I. A., and Tsiani, E. (2017). Effects of resveratrol against lung cancer: In vitro and in vivo studies. Nutrients 9 (11), 1231. doi:10.3390/nu9111231
Yu, S., and Zhao, G. (2012). Development of polyphenols as HIV-1 integrase inhibitors: A summary and perspective. Curr. Med. Chem. 19 (32), 5536–5561. doi:10.2174/092986712803833236
Yuan, X., Gajan, A., Chu, Q., Xiong, H., Wu, K., and Wu, G. S. (2018). Developing TRAIL/TRAIL death receptor-based cancer therapies. Cancer Metastasis Rev. 37 (4), 733–748. doi:10.1007/s10555-018-9728-y
Zeng, S., Zhao, X., Xu, L. S., Yang, D., Chen, L., and Xu, M. H. (2019). Apoptosis induction effect of Apocynum venetum polyphenol on human U87 glioma cells via NF-κB pathway. Future Oncol. 15 (32), 3723–3738. doi:10.2217/fon-2019-0381
Zhang, L., Xie, J., Gan, R., Wu, Z., Luo, H., Chen, X., et al. (2019). Synergistic inhibition of lung cancer cells by EGCG and NF-κB inhibitor BAY11-7082. J. Cancer 10 (26), 6543–6556. doi:10.7150/jca.34285
Zhang, X., Zhang, C., Ren, Z., Zhang, F., Xu, J., Zhang, X., et al. (2020). Curcumin affects gastric cancer cell migration, invasion and cytoskeletal remodeling through gli1-β-catenin. Cancer Manag. Res. 12, 3795–3806. doi:10.2147/CMAR.S244384
Zhang, X. A., Zhang, S., Yin, Q., and Zhang, J. (2015). Quercetin induces human colon cancer cells apoptosis by inhibiting the nuclear factor-kappa B Pathway. Pharmacogn. Mag. 11 (42), 404–409. doi:10.4103/0973-1296.153096
Zhang, Z., Ding, Y., Dai, X., Wang, J., and Li, Y. (2011). Epigallocatechin-3-gallate protects pro-inflammatory cytokine induced injuries in insulin-producing cells through the mitochondrial pathway. Eur. J. Pharmacol. 670 (1), 311–316. doi:10.1016/j.ejphar.2011.08.033
Zhao, X., Jiang, K., Liang, B., and Huang, X. (2016). Anticancer effect of xanthohumol induces growth inhibition and apoptosis of human liver cancer through NF-κB/p53-apoptosis signaling pathway. Oncol. Rep. 35 (2), 669–675. doi:10.3892/or.2015.4455
Zhou, D. H., Wang, X., and Feng, Q. (2014). EGCG enhances the efficacy of cisplatin by downregulating hsa-miR-98-5p in NSCLC A549 cells. Nutr. Cancer 66 (4), 636–644. doi:10.1080/01635581.2014.894101
Zhou, Z. G., Li, D. D., Chen, Y., Chen, X., and Man, R. J. (2022). Discussion on the structural modification and anti-tumor activity of flavonoids. Curr. Top. Med. Chem. 22 (7), 561–577. doi:10.2174/1568026622666220308162049
Keywords: polyphenols, flavonoids, anti-tumor therapy, prevention, signal pathways, oncogenesis
Citation: Sufianova G, Gareev I, Beylerli O, Wu J, Shumadalova A, Sufianov A, Chen X and Zhao S (2022) Modern aspects of the use of natural polyphenols in tumor prevention and therapy. Front. Cell Dev. Biol. 10:1011435. doi: 10.3389/fcell.2022.1011435
Received: 04 August 2022; Accepted: 26 August 2022;
Published: 12 September 2022.
Edited by:
Mohd Farhan, King Faisal University, Saudi ArabiaReviewed by:
Mithun Rudrapal, Rasiklal M. Dhariwal Institute of Pharmaceutical Education and Research, IndiaCopyright © 2022 Sufianova, Gareev, Beylerli, Wu, Shumadalova, Sufianov, Chen and Zhao. This is an open-access article distributed under the terms of the Creative Commons Attribution License (CC BY). The use, distribution or reproduction in other forums is permitted, provided the original author(s) and the copyright owner(s) are credited and that the original publication in this journal is cited, in accordance with accepted academic practice. No use, distribution or reproduction is permitted which does not comply with these terms.
*Correspondence: Albert Sufianov, c3VmaWFub3ZAZ21haWwuY29t; Xin Chen, Y2hlbnhpbl90cmFjeUB5ZWFoLm5ldA==; Shiguang Zhao, Z3VhbmdzekBob3RtYWlsLmNvbQ==
†These authors have contributed equally to this work
Disclaimer: All claims expressed in this article are solely those of the authors and do not necessarily represent those of their affiliated organizations, or those of the publisher, the editors and the reviewers. Any product that may be evaluated in this article or claim that may be made by its manufacturer is not guaranteed or endorsed by the publisher.
Research integrity at Frontiers
Learn more about the work of our research integrity team to safeguard the quality of each article we publish.