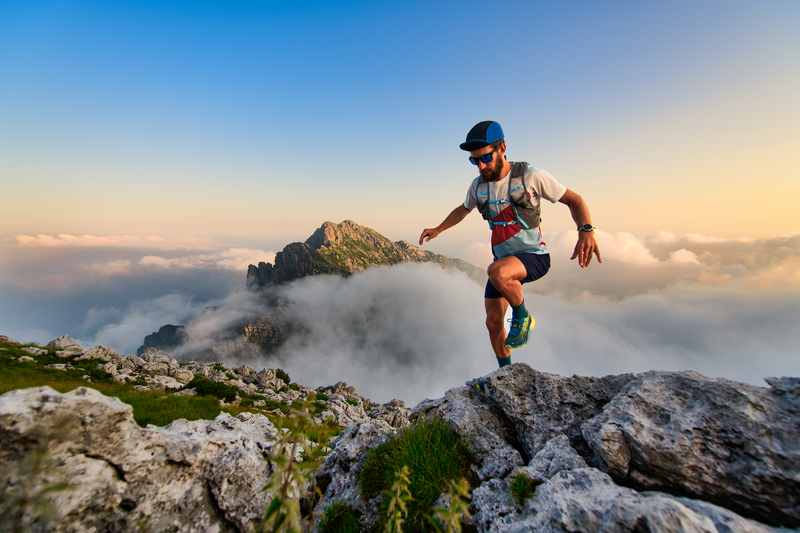
94% of researchers rate our articles as excellent or good
Learn more about the work of our research integrity team to safeguard the quality of each article we publish.
Find out more
REVIEW article
Front. Cell Dev. Biol. , 12 October 2022
Sec. Stem Cell Research
Volume 10 - 2022 | https://doi.org/10.3389/fcell.2022.1006295
This article is part of the Research Topic Current Progress in Mesenchymal Stem/Stromal Cell Research, volume II View all 8 articles
In recent years, mesenchymal stromal cells (MSCs) have generated a lot of attention due to their paracrine and immuno-modulatory properties. mesenchymal stromal cells derived from the umbilical cord (UC) are becoming increasingly recognized as having increased therapeutic potential when compared to mesenchymal stromal cells from other sources. The purpose of this review is to provide an overview of the various compartments of umbilical cord tissue from which mesenchymal stromal cells can be isolated, the differences and similarities with respect to their regenerative and immuno-modulatory properties, as well as the single cell transcriptomic profiles of in vitro expanded and freshly isolated umbilical cord-mesenchymal stromal cells. In addition, we discuss the therapeutic potential and biodistribution of umbilical cord-mesenchymal stromal cells following systemic administration while providing an overview of pre-clinical and clinical trials involving umbilical cord-mesenchymal stromal cells and their associated secretome and extracellular vesicles (EVs). The clinical applications of umbilical cord-mesenchymal stromal cells are also discussed, especially in relation to obstacles and potential solutions for their effective translation from bench to bedside.
Mesenchymal stromal cell (MSC) therapy has enormous potential in regenerative medicine given their paracrine, anti-inflammatory and immunomodulatory capabilities. Although, several studies in early 2000s reported the ability of UC-MSCs to differentiate into various cell types, these claims were primarily based on in vitro experiments with limited characterization (Caplan, 1991; Guadix et al., 2017; Viswanathan et al., 2019). In 2006, the International Society for Cellular Therapy (ISCT) established the minimal criteria required for a cell to be classified as an MSC: 1) They must adhere to the surface in a tissue culture plate, 2) express adhesion markers CD44 (hyaluronan receptor), mesenchymal markers such as CD90 (thy-1) and CD105 (endoglin), and lack expression of hematopoietic markers e.g. CD34, 3) differentiate into multiple lineages in vitro, 4) self-renew and exhibit low immunogenicity (Dominici et al., 2006). Over several years, MSCs have gained much attention as a potential therapeutic for several clinical applications such as osteoarthritis, myocardial infarction, graft-versus-host disease (GVHD), diabetes mellitus, multiple sclerosis, systemic lupus erythematosus, kidney injury, amyotrophic lateral sclerosis, spinal cord injury and several other diseases (Parekkadan and Milwid, 2010; Saeedi et al., 2019; Payandeh et al., 2022; Norooznezhad et al., 2022; Ullah et al., 2019). In addition, MSCs release soluble factors, extracellular vesicles (EVs) such as exosomes, and microvesicles (MVs) that can collectively act in a paracrine and autocrine fashion to repair tissue and modulate the tissue microenvironment (Joerger-Messerli et al., 2018). Stromal cells derived from the embryos, such as those resulting from the inner cell mass, are surrounded by ethical issues related with embryo destruction. While induced pluripotent stem cells circumvent the ethical issues, they introduce risk of tumor formation by transferring pluripotent genes (Lo and Parham, 2009). In contrast, MSCs that can be isolated from adults (bone marrow, peripheral blood, adipose tissue and dental pulp) and birth-related tissues (cord blood, Amnion membrane and amniotic fluid) have low to non-existent tumorigenic potential which, combined with absence of the major histocompatibility complex II and low expression of co-stimulatory factors CD80, CD86 and CD40, allow for allogeneic administration of MSCs across a wide range of clinical applications (Chen et al., 2004; Lazarus et al., 2005; Fouillard et al., 2007; Lee et al., 2008). Therefore, the umbilical cord (UC), which is considered medical waste and retrieved at delivery using a painless, simple and safe approach, has piqued significant interest in recent years as a potential source for MSCs. The existing isolation procedure for MSCs has consistently resulted in effective collection of substantial quantities of MSCs from UC tissues. Furthermore, UC-MSCs also have a greater self-renewal capacity (300 cell divisions) and a shorter doubling time (30–36 h) (Koike et al., 2014). Clinical trials have shown that UC-MSCs can heal nerve, bone, heart and kidney injuries, as well as promote the formation of blood vessels; these finding have been reported in several clinical trials as reported in the ClinicalTrials.gov database–please see Table 3 for full details (Lv et al., 2013; Chang et al., 2014; Kim et al., 2015; Bartolucci et al., 2017; Riordan et al., 2018; Powell and Silvestri, 2019; He et al., 2020; Özmert and Arslan, 2020; Zhang et al., 2021; Kim et al., 2021; Lee et al., 2021; Shi et al., 2021; Monsel et al., 2022; Zang et al., 2022). The main benefit of UC-MSCs over MSCs from other sources is their availability as well as their high proliferation and plasticity (Pipino et al., 2013).
The purpose of this review is to provide an overview of different UC tissue compartments to isolate MSCs, the characteristic differences and similarities in their regeneration and immuno-modulatory factors, as well as the single cell transcriptomic profiles of in vitro grown and tissue extracted UC-MSCs. The review also highlights the therapeutic potential and biodistribution of UC-MSCs after systemic administration in addition to an overview of completed pre-clinical trials using UC-MSCs, and therapeutic interventions using the secretome and extracellular vesicles (EVs). Finally, the banking and socio-ethical issues concerning UC-MSCs for future healthcare needs are discussed.
MSCs isolated from various areas of the UC provide a possible alternative for BM and adipose tissue (AD) derived MSCs, as both involve invasive isolation procedures, and have a low cell recovery and shorter stemness (Baksh et al., 2007). UC is a conduit that connects the mother and baby in the pregnancy and is made of narrow-tube structures that prevents the cord itself from compressing, bending, or twisting. The UC develops between the 4th and 8th week of human embryogenesis, with a mean length of 55 cm, mean diameter of 14.42 mm, and mean weight of 40 g (32). UC consists of two arteries and one vein encased in the proteoglycan-rich gelatinous Wharton’s jelly (WJ) and associated by the amnion layers. A schematic illustration of the UC is shown in Figure 1. The extracellular matrix is rich in fibroblast growth factors 1 and 2, platelet-derived growth factor (PDGF), insulin-like growth factor 1 (IGF-1), epidermal growth factor (EGF) and transforming growth factor (TGF). In contrast to other MSC tissue sources, the colony forming unit-fibroblasts (CFU-F) frequency during collection is higher from the UC [(Davies et al., 2017), (Majore et al., 2009)].
FIGURE 1. Schematic illustration of the umbilical cord (UC) representing the four major compartments: Amnion Membrane (AM), Wharton’s Jelly (WJ), Sub-Amnion (SA), and Peri-Vascular (PV) region along with arteries and veins.
Commonly, MSC isolation from UC is divided into four main sections: the Wharton’s Jelly (WJ), the perivascular area (PV), the sub-amnion (SA), and the Amnion membrane (AM). WJ-MSCs are isolated by dissecting out the arteries and extracting the WJ from the UC using knife or forceps. PV-MSCs are separated by looping the collected blood arteries and enzymatically digesting them to allow cell dissociation for further culture. SA-MSCs are isolated by dissecting the cord lining using scissors or a scalpel and then cultivating the explants to get adherent cells. AM-MSCs are isolated by dissecting the UC and dipping the Amnion membrane side in an enzymatic solution for cell dissociation and subsequent expansion in culture medium (Subramanian et al., 2015). McElreavey et al. isolated fibroblast-like cells that showed features of typical MSCs from the WJ of UC for the first time in 1991 (McElreavey et al., 1991). In 2003, Mitchell et al. extracted MSCs from WJ through explant culture and differentiated them into glial cells and neurons as a possible alternative for regenerative treatments (Mitchell et al., 2003). The first cord blood transplantation was conducted in France in 1988 using MSCs, as they were suggested to be circulating in the pre-term fetus (Gluckman et al., 1989). Romanov et al. developed an effective isolation prototype of MSCs from UC sub-endothelial layers, after which UC became increasingly prevalent as a potential source of MSCs (Romanov et al., 2003).
Cross-sectional histological staining of UC with hematoxylin and eosin reveals six different regions: arteries, veins, PV, WJ, AM, and SA. Among them, WJ takes up a significant amount of the UC and includes a greater number of MSCs with stellate-like shape than the other regions; up to 5.5 × 106 (Subramanian et al., 2015). MSC isolation from PV, SA and AM, requires enzymatic treatment and repeated culture because these areas are closely linked through the extracellular matrix due to which cross contamination of MSCs from these compartments is possible (Zhang et al., 2012). In contrast, isolation of MSCs from WJ is simpler and faster because of their short doubling time, allowing sufficient cell counts to be achieved in a shorter culture period for clinical translation, thereby reducing the risk of culture-induced genetic manipulation and microbial contamination (Liau et al., 2020). The WJ-MSCs have higher viability and proliferation rates than other areas (i.e. AM, SA, PV). Although the telomerase activity of MSCs from WJ, AM, SA, and PV are comparable, the regenerative ability of WJ-MSCs is substantially higher than those from the other areas at higher passages (Pera, 2009). MSCs generated from WJ, AM, SA, and PV, have been shown to easily develop into adipocytes, chondrocytes, and osteocytes (Subramanian et al., 2015). However, the osteogenic differentiation of WJ-MSCs presents greater Von Kossa-stained cells, revealing increased calcium accumulation, whereas the chondrogenic differentiation of WJ- and PV-MSCs shows Alcian blue-stained cells, revealing higher proteoglycan and glycosaminoglycan synthesis by articular chondrocytes (Mitchell et al., 2003). Additionally, gene expression of osteogenic markers (OCN, OPN, ALP, BSP) in WJ-MSCs is considerably greater (5-fold) than other areas (AM, SA, PV). Similarly, chondrogenic marker gene expression (COL2A1, COMP, FMOD, SOX9) is substantially greater in WJ-MSCs (30-fold) (Subramanian et al., 2015).
Immuno-phenotyping of MSCs isolated from various compartments of UC revealed positive for CD29, CD90, CD44, CD73, HLA-ABC, CD105 and negative for CD34, CD14, CD45, CD19, HLA-DR ABC, CD117. WJ-MSCs have significant levels of CD29, HLA-ABC, CD44, and CD73 markers when compared to cells from AM, SA, and PV (Subramanian et al., 2015). WJ-MSCs also have higher levels of the CD24 and CD108 markers that distinguish MSCs from non-MSCs (Wetzig et al., 2013). The pericyte markers CD146 and CD271 are positive in both WJ and PV-MSCs, indicating that there is no apparent anatomical divide between the two areas (Covas et al., 2008). In contrast, CD140b and CD49 days are markers that are infrequently expressed in AM and SA-MSCs owing to contamination with neighboring fibroblast or stromal cells during isolation (Mafi et al., 2011). The expression of the CD40 marker, which is found in non-MSCs, is reported in MSC cultures primarily isolated from AM, SA, PV (50.8%–70.2%) and least from WJ (26.1%–27.4%); likely due to cross-contamination of smooth muscle, epithelial, endothelial and fibroblast cells (Majore et al., 2011). The immuno-histological labelling and RT-PCR studies of MSCs generated from various compartments of UC are positive for pluripotent markers Tra-1–60, Tra-1–81, SSEA-4, SSEA-3, as well as pluripotent genes OCT4A, SOX2, OCT2, OCT4B, OCT1 and NANOG. Pluripotent genes are highly expressed in WJ-MSCs, while fibroblast-associated genes FAP and FSP, are significantly expressed in AM- and SA-MSCs (Subramanian et al., 2015). Despite high levels of expression of pluripotent markers, researchers have revealed that UC-MSCs do not form teratomas when injected into immunodeficient SCID mice (Wang et al., 2013; Chen et al., 2014).
It has been observed that MSCs isolated from various compartments of UC have distinct morphologies. WJ-MSCs form a confluent monolayer of adherent cells with stellate-like morphology, while MSCs obtained from AM, SA, and PV areas show fibroblast-like morphology in vitro (Subramanian et al., 2015). MSCs generated from the entire UC showed colonies with both epithelioid and fibroblast morphologies. Further differences in the MSCs properties isolated from the different UC compartments are compared in Table 1 (Zhang et al., 2012; Subramanian et al., 2015).
The hypothesis that all UC-MSCs have the same therapeutic qualities and functions ignores biological heterogeneity and may limit their use in disease treatment (Wegmeyer et al., 2013). The standardization of UC-MSC populations is one of the most important criteria influencing their clinical efficacy. Using routine analysis of cell surface antigen expression and differentiation potential, a better understanding of the differences in functional properties of UC-MSC from different donors, tissues, clones and even different cells from the same clonal population can be achieved. However, global transcriptional profiling can clearly reveal expression patterns that identify biological states or compare genes with comparable expression patterns (Wells and Choi, 2019). Bulk transcriptomic profiles, however, lack the spatial and temporal precision to differentiate genetic variability among low colonized groups within the same tissue of origin, unlike single cell transcriptomics which can resolve subtle changes across in vitro expanded clonal UC-MSC populations (Wang et al., 2021).
Regarding molecular heterogeneity within in vitro expanded UC-MSCs, Huang et al. revealed distinct subpopulations of cultured UC-MSCs from the same tissue source, defined by differential expression of genes (Huang et al., 2019). Interestingly, the expression pattern of the sub-populations remained persistent throughout passage numbers, donors, and following both INFγ and TNFα stimulation. Similar conclusions have been drawn by other researchers regarding variability of sub-populations among different MSC sources (AD, BM and UC) (Hou et al., 2021) and genomic heterogeneity across subpopulations of cultured UC-MSCs using gene markers for each sub-population as shown in Table 2 (Hou et al., 2021) (Barrett et al., 2019).
Several studies noted there was a correlation between the weighted percentage of these subpopulations in the clonal line and their immunomodulatory effects (Barrett et al., 2019; Huang et al., 2019; Hou et al., 2021; Wang et al., 2021). For example, HMGB2 and other genes are highly expressed in UMSC1 subpopulations that is believed to contribute to their proliferative capacity, while genes that are highly expressed in UMSC3 subpopulations align towards a committed differentiation status (Wang et al., 2021). On the other hand, high expression of AREG, CSF3, CCL20 and IL6 make UC-MSCs exert a stronger immunomodulatory effect compared to other clonal lines or even other sources of MSC (Hou et al., 2021). In addition, it was also observed that the limited heterogeneity observed among UC-MSCs was related to cell cycle stages, with the CD168 marker being negatively corelated with the immunosuppressive effect of those subpopulations of MSCs (Huang et al., 2019). These results suggest that the dynamics of cell cycle status plays an important role in determining the regenerative and immunomodulatory effects exerted by UC-MSCs.
Molecular heterogeneity within the tissue extracted naïve UC-MSCs raise the intriguing question of whether UC-MSCs expanded in vitro prior to treatment retain the same stemness properties as their original isolates. In fact, single-cell RNA sequencing revealed three different subpopulations of UC-MSCs isolated from WJ, each expressing epithelial, pericyte, and stromal genetic markers, which defined their ligand-receptor crosstalk, as shown in Table 2. The epithelial subpopulation had immuno-modulatory, pro-survival, and differentiation potential, while the stromal subpopulation had properties more useful for regeneration and epithelial–mesenchymal transition (EMT). Molecular heterogeneity was revealed in single cell transcriptomics studies of WJ-MSCs versus in vitro clonally expanded UC-MSCs (Barrett et al., 2019; Zhang et al., 2021). Subpopulation changes affect gene expression patterns, possibly affecting the therapeutic effect of UC-MSCs. EMT genes, perhaps, were significantly expressed in tissue-derived UC-MSCs (Barrett et al., 2019). However, in the clonally expanded UC-MSCs, telomere maintenance associated DNA repair and senescence, required for irradiation-induced damage, was increased (Yang et al., 2016; Wu et al., 2017). This shows that even UC-MSCs from the same tissue have distinct ligand-receptor interactions that affect their biological activity in diverse disease microenvironments.
Recent studies on cell-free therapy have suggested that the MSC secretome can also be used to treat different diseases (Eleuteri and Fierabracci, 2019; Soltani et al., 2021). Like other MSCs, UC-MSCs are reported to secrete molecules such as chemokines, cytokines, growth factors, and insoluble factors including EVs that have therapeutic effects in several diseases. The UC-MSC secretome consists of around 90 cytokines and numerous growth factors, which exhibit anti-inflammatory, pro-inflammatory, angiogenesis, neuroprotective, anti-apoptotic and anti-tumor properties (Bai et al., 2016). To investigate the therapeutic benefit of the UC-MSCs secretome, one study used injured brain extracts and pre-treated them with UC-MSCs; the secretome resulting from these pre-treated cells was then stereotaxically injected in rats with traumatic brain injury (TBI) with result showing a significant improvement in cognitive function when using the secretome from pre-treated UC-MSCs compared to the normal secretome (Liu et al., 2020a). In another study, the UC-MSC secretome was used in mice with diabetic wounds, and the results showed effective wound healing via an increase in angiogenesis as well as macrophage polarization from an inflammatory to an anti-inflammatory phenotype (Zhang et al., 2020).
International society for extracellular vesicles (ISEV) recognizes “EVs” as the general name for particles naturally expelled from the cell that are bordered by a lipid bilayer and cannot multiply (i.e. they do not include a functioning nucleus). EVs are described by 1) physical features such as size [100 nm or 200 nm (small) or >200 nm (big and/or medium)] or density (low, intermediate, high, with each range specified); 2) biochemical content (CD63+/CD81+ EVs, Annexin A5-stained EVs, etc.); and 3) conditions or cell of origin (podocyte EVs, hypoxic EVs, large oncosomes, apoptotic bodies) (Théry et al., 2018; Alcaraz et al., 2020). Haraszti et al. compared the yield of exosomes from three different types of MSCs such as AD-, BM-, and UC-MSCs and showed UC-MSCs to have the highest exosomal yield. Furthermore, modification of the EVs isolation method from differential ultra-centrifugation to tangential flow filtration and culture condition of UC-MSCs from 2D to 3D culture led to an increase in yield (Haraszti et al., 2018). In another study, UC-MSCs improved the cardioprotective effect in a MI model (Xu et al., 2020). Exosomes from UC-MSC have shown promising outcomes in the treatment of colitis (Ma et al., 2019), neurodegenerative diseases (Ding et al., 2018), liver failure (Jiang et al., 2019), diabetes (Zhang et al., 2020), as well as cancer (Ngadiono and Hardiany, 2019). ExoCarta, an online database is available to identify molecular markers of tissue/cell type derived exosomes, has catalogued various published and unpublished exosomal proteins, RNAs, and lipids. There are 100 genes encoding proteins in the ExoCarta, including the top five protein-coding genes (index>90) namely CD9, HSPA8, PDCD6IP, GAPDH, and Actin B.
Exosomes obtained from UC-MSCs have been reported to regulate inflammation and immune responses in acute liver failure (ALF) in mice models via reduction of NLRP3 inflammasome expression and downstream inflammatory cytokine secretion (Jiang et al., 2019). In addition, exosomes from TNF-α pre-treated UC-MSCs have efficiently prevented ALF by decreasing the NLRP3 expression and inflammatory factors (Zhang et al., 2020c). In another study, the delivery of UC-MSC EVs combined with estrogen exhibited a highly effective alternative treatment for endometrial injury (Ebrahim et al., 2018). Further, UC-MSCs exosomal microRNA-17–5P improved ovarian function by regulating SIRT7 expression following chemotherapy-induced damage in human ovarian cells (Ding et al., 2020). Hu et al. evaluated the effect of UC-MSC EVs in bone regeneration in osteoporotic mice and the study reported restoration of trabecular and cortical bone mass and attenuated bone loss in old mice. In another study, exosomes from UC-MSCs after osteogenic induction for 2 days were injected with hydrogel (hydroxylapatite embedded hyaluronic acid and alginate hydrogel) to provide long-term release of exosomes, which improved bone regeneration and angiogenesis (Yang et al., 2020). Jie et al evaluated the effect of UC-MSC exosomes on experimental colitis and showed significant improvement in the DSS-induced colitis in a mouse model (Ma et al., 2019). In the study by Shiue et al, constant intrathecal infusion of UC-MSC exosomes attenuated spinal nerve ligation-induced pain in rats. Moreover, the exosomes inhibited glial cell activation resulting in a decrease in proinflammatory cytokines (TNF-α, IL-1β) and an increase in the anti-inflammatory cytokines (IL-10) and neurotrophic factors (BDNF, GDNF) (Shiue et al., 2019). It has been reported that UC-MSC EVs protect HIE‐induced apoptosis by transferring microRNA (let‐7‐5p) to neuronal cells (Saeedi et al., 2019). Additionally, WJ-MSC EVs have been demonstrated to treat IR-induced kidney injury via regulation of ERK1/2 to suppress CX3CL1 to improve cell proliferation, inhibit apoptosis, and inflammation (Chen et al., 2017; Wu et al., 2018). Zhang et al. showed anti-oxidative regulation of UC-MSC EVs via downregulation of NOX2, and upregulation of NRF2/ARE in ischemia-reperfusion (IR)-induced kidney injured rats (Zhang et al., 2014; Zhang et al., 2016).
MSCs have been shown to perform a regenerative function in damaged or injured tissues such as skin, bone, cartilage, liver, and cornea due to their abilities to secrete factors that directly induce tissue intrinsic progenitors, modulate local immune cells, inhibit apoptosis, prevent scar formation, and stimulate angiogenesis as illustrated in Figure 2 (Han et al., 2019) (Law and Chaudhuri, 2013).
FIGURE 2. Highlights the applications of UC-MSCs for treatment of variety of diseases such as cutaneous, neural, myocardial, renal, hepatic, bone and muscular dysfunction.
Ryu et al. demonstrated the paracrine potential of WJ-MSCs in promoting neural renewal, neural protection and preventing inflammation at the site of spinal cord injury (SCI), with evidence showing it to be more effective than BM- and AD-MSCs (Ryu et al., 2012). Mohamadi et al. have demonstrated anti‐inflammatory properties of WJ-MSCs following intrathecal administration in an SCI model (Mohamadi et al., 2019). WJ-MSCs have shown reduced expression of IL‐1b and increased expression of neural growth factor (NGF) in the treated spinal cord tissue; this results in renewal of motor function and integrity of spinal cord in a dose‐dependent manner, and over repeated administrations (Li et al., 2016; Chudickova et al., 2019). The therapeutic effects were further improved using conditioned media from WJ-MSCs (Chudickova et al., 2019). Recently, Wang et al. demonstrated enhanced functional retrieval, neural regeneration, and neurotrophic factors in the transected sciatic nerves of mice using WJ-MSCs in contrast to AD-MSCs (Wang et al., 2020). Also, Kim et al. have shown recovery of the behavioral role in hypoxic‐ischemic encephalopathy (HIE)-induced brain infarcted rats via anti-astroglia, anti-apoptotic, and anti-inflammatory factors of UC-MSCs (Kim et al., 2019). In another study, Lee et al. examined whether sRAGE secreting UC-MSCs via CRISPR/Cas9 technique protect neuronal cell death in a Parkinson’s Disease animal model (Lee et al., 2019). The study revealed that decreasing AGE-RAGE binding may be the potential therapeutic approach for curing Parkinson’s Disease by preventing neuronal cell death.
The therapeutic effects of UC-MSCs in treatment of myocardial tissue is demonstrated in several studies. Liu et al. have shown improvement in left ventricular function, perfusion, and rejuvenation in a porcine model (Liu et al., 2016). UC-MSC treatment caused reduced expression of TNF-α and activation of Erk1/2 kinases in the heart tissue of rats cardiomyopathy, thereby preventing interstitial fibrosis and cardiac dysfunction (Zhang et al., 2018). Further, epigenetic modification of UC-MSCs have been shown to enhance cardiomyocyte differentiation through Wnt signaling in mice with cardiac injury (Bhuvanalakshmi et al., 2017). Rabbani et al. have proposed combinational therapy using WJ-MSCs with IGF-1 to regenerate myocardial tissue with enhanced angiogenesis, reduced fibrosis, and improvement in cardiac function in a rabbit model (Rabbani et al., 2017). This study revealed that overexpression of the anti-fibrotic factors in WJ-MSCs play an important role in protecting cardiac fibrosis owing to their greater viability, collagen deposition, and proliferation properties (Nimsanor et al., 2019) (Pu et al., 2017). Cho et al. investigated the cardio-protective benefit of UC-MSCs from rats expressing lymphoid enhancer-binding factor 1 (LEF1) linked using CRISPR/Cas9, and demonstrated increased cell survival and cardio-protective effects in the infarcted myocardium (Cho et al., 2020).
UC-MSCs have been widely explored as a potential alternative to other MSCs for regeneration of cutaneous tissues. Arno et al. have shown improved vascularization, re‐epithelialization, survival, proliferation, and migration of skin fibroblast cells in a skin-excised mouse model (Arno et al., 2014). Additionally, UC-MSCs have been reported to improve wound healing by supporting proliferation, angiogenesis, and regeneration of sebaceous glands in rats with radiation‐induced skin injury (Sun et al., 2019). The anti‐inflammatory potential was further enhanced by priming UC-MSCs with IFN-γ and poly (I:C) in atopic dermatitis (Park et al., 2019). Also, culturing of WJ-MSCs in a silk fibroin scaffold has shown improved re‐epithelialization and a reduction in the formation of fibrotic scars (Millán-Rivero et al., 2019). Recently, Martin‐Piedra et al. proposed that WJ-MSCs are better suited for preparation of bioengineered skin tissue over other types of MSCs such as AD-MSCs, dental pulp and BM-MSCs (Martin-Piedra et al., 2019).
Mortezaee et al. reported retinoic acid as an effective inducer for stimulating the differentiation of WJ-MSCs into hepatocyte-like cells, though the exact mechanism remains incompletely understood. However, given that UC-MSCs do not readily differentiate in vivo, this approach will necessitate the in vitro generation and expansion of WJ-MSCs into hepatocyte-like cells prior to their administration with the additional need to also examine the expression of hepatocyte genes (Mortezaee et al., 2015). Tsai et al. demonstrated a reduction in serum glutamic oxaloacetic transaminase, TGF-β1, glutamic pyruvate transaminase, and collagen deposition, which cause an upregulation of mesenchymal‐epithelial transition factors and HG, leading to a decrease in liver fibrosis (Tsai et al., 2009). WJ-MSCs showed significant reduction of sepsis‐associated liver damage and endothelial dysfunction in a rat model (Cóndor et al., 2016). Interestingly, WJ-MSCs improved liver function and attenuated the d-Galactosamine-induced hepatotoxicity in acute liver injured mice (Ramanathan et al., 2017). Chetty et al. confirmed regeneration of liver in acetaminophen-induced injury via renewal of portal tracts and hepatic arteries after 3 days of WJ-MSCs administration in BALB/c mice (Chetty et al., 2019a). Another study showed paracrine activity of intrauterine transplanted WJ-MSCs in liver injured rabbit fetuses via expression of HNF-4, CYP2B6 messenger RNA, α‐fetoprotein, and albumin (Rezaeian et al., 2018). Hammam et al. observed that the combination of WJ-MSCs and praziquantel (PZQ) increased the therapeutic effects of PZQ on S. mansoni-induced liver fibrosis, as compared to utilizing each alone (Hammam et al., 2016).
WJ-MSCs administration has revealed the role of endocrine effects in the treatment of IR-induced acute and chronic kidney injury through activation of Akt in tubular epithelial cells, resulting in inhibition of apoptosis and upregulation of HGF (Du et al., 2012). Hu et al. showed a reduction in renal fibrosis following administration of UC-MSCs in a decellularized kidney scaffold by inhibiting mesenchymal‐epithelial transition through TGF‐β/Smad pathway in rats (Hu et al., 2020). Several researchers have examined the use of UC-MSCs in kidney transplants from brain or cardiac deceased donors (Assfalg et al., 2016; Sun et al., 2018). These techniques are linked with a greater incidence of early graft malfunction and severe rejection due to prolonged ischemia (Summers et al., 2010). The combinatorial infusion of UC-MSCs before and during surgery in dead donor graft recipients was shown to be safe with no adverse clinical outcomes (Sun et al., 2017). Overall, UC-MSCs greater proliferative capacity, higher immunosuppressive effects, and hypo-immunogenic features make them suitable for large-scale, universal production.
UC-MSCs have been shown to be an appealing source in combination with desired scaffolds for regeneration of cartilage tissue. Paduszyński et al. have shown regulation in the transcriptional profile of WJ‐MSCs following chondrogenic differentiation on a 3D Poly (lactic-co-glycolic acid) (PLGA) scaffold for repairing cartilage (Paduszyński et al., 2016). Similarly, several studies have demonstrated the therapeutic potential of UC-MSCs in combination with 3D HyStem and collagen-built hydrogel for cartilage repair due to elevation in the expression of cartilage‐specific matrix genes (Chen et al., 2013; Aleksander-Konert et al., 2016; Shie et al., 2017). Recently, Zhang et al. have described co-culture of UC-MSCs with articular cartilage cells in an extracellular matrix‐oriented scaffold for developing framework engineered hyaline cartilage in vitro (Zhang et al., 2019). In addition, UC-MSCs have revealed promising results in fabricating tissue‐engineered cartilage without using the scaffold. Saulnier et al. have reported that intra‐articular administration of WJ‐MSCs in the knee joints of 30 rabbits with osteoarthritis (OA) modulated MMP proteins in the synovium and prevented cartilage deprivation (Saulnier et al., 2015). Recently, Cheng et al. proposed a combinational therapy using WJ-MSCs along with shockwaves as an efficient treatment for early OA in rats (Cheng et al., 2019).
Several studies have reported that UC-MSCs are a potential alternative to BM-MSCs for bone regeneration (Lim et al., 2018a; Ansari et al., 2018; Cabrera-Pérez et al., 2019). Todeschi et al. have reported enhanced angiogenesis after subcutaneous implantation of scaffolds loaded UC-MSCs in mice with bone defects (Todeschi et al., 2015). It has been hypothesized that the modulation in RUNX2/p57 expression via JARID1B histone demethylase inhibition plays a role in the osteogenic potential of WJ-MSCs (Bustos et al., 2017). Recently, Liu et al. have shown that overexpression of Wnt10b in UC-MSCs is beneficial for treatment of calvarial defect in rats due to an enhancement of osteogenic potential and VEGF-activated angiogenesis (Liu et al., 2020b). Choi et al. have recently shown that CRISPR-Cpf1 activation of the BMP4 gene promote osteogenic differentiation of UC-MSCs (Choi et al., 2020). Lastly, outcomes of phase I/II randomized control trials using UC-MSCs in patients after intra‐articular administration revealed the therapy to be more effective with repeated doses as compared to single-dose or hyaluronic acid treatment (Matas et al., 2019).
WJ-MSCs and their secreted XCL1 protein have been proposed as a novel approach for treating myopathies due to their anti-apoptotic potential (Kwon et al., 2016). Wang et al. have demonstrated a reduction in sarcopenia following activation of skeletal muscle cell proliferation, and an inhibition of apoptosis and inflammation in aged mice (Wang et al., 2018). Differentiation potential of WJ-MSCs into smooth muscle cells was shown to be accelerated by priming with TGF‐β1 and ascorbic acid (Mesure et al., 2017), and priming with SDF-1 improved the migration potential of UC-MSCs during regeneration of skeletal muscle in vitro (Kowalski et al., 2017). In addition, recovery of sensory and motor functions was enhanced via neuro‐muscular regeneration during neurotmesis injuries using biodegradable scaffolds loaded with UC-MSCs (Caseiro et al., 2017). Recently, Su et al. have demonstrated re-establishment of impaired skeletal muscle functions in mice via downregulation of neutrophil‐associated acute inflammation, attributed to the antifibrotic properties of UC-MSCs (Su et al., 2019).
The most used delivery method for UC-MSCs in pre-clinical and clinical studies is systemic intravenous infusion of cells. In the last few years, labelling techniques have allowed scientists to detect the fate of MSCs after injection in vivo (Laurila et al., 2009; Reinders et al., 2013; Chetty et al., 2019b; Ullah et al., 2019). Lim et al. have reported that UC-MSCs transfected with lentivirus-eGFP were distributed in many organs, especially in the lungs after intravenous administration following acute myocardial infarction in porcine models (Lim et al., 2018b). Violatti et al. confirmed the previous results labelling UC-MSCs with poly (methyl methacrylate) nanoparticles loaded with the nuclear dye Hoechst-33258 for tracking in the healthy and early symptomatic SOD1G93A mice. Interestingly, the authors were able to track UC-MSCs at different time points during the disease progressions. In line with previous data, UC-MSCs, like other MSCs, are rapidly and efficiently captured by the lungs before reaching the reticuloendothelial organs (spleen, liver) for metabolism and clearance (Violatto et al., 2015). An outline of the potential interactions of UC-MSCs following their systemic administration is illustrated in Figure 3. T-cell anergy is caused by the lack of expression of the co-stimulatory molecules CD40, CD86, and CD80 on the surface of UC-MSCs. Allogeneic MSCs have been shown to inhibit the proliferation, activation, and IgG secretion of B cells from BXSB mice, which are used as an experimental model for human systemic lupus erythematosus. In vitro investigation also revealed that CD4+ T-cells in interaction with UC-MSCs are halted in the G0/G1 phase and cease to proliferate, whereas Treg cell proliferation is induced; plasma cell IgG production appears to be impacted as well (Nauta and Fibbe, 2007). Moreover, UC-MSCs express very little MHC-I and apparently no MHC-II (until after interferon therapy), making them resistant to NK cell toxicity in an allogenic/xenogeneic environment by down-regulating interferon expression and up-regulating the anti-inflammatory cytokines IL-4 and IL-10. Intravital microscopy of UC-MSCs in a cremaster muscle mouse model showed that UC-MSCs are likely to be disrupted by the shear force generated by blood flow. This can result in cell fragmentation and formation of extracellular vesicles capable of influencing paracrine secretion of immuno-modulatory factors, or trigger phagocytosis of the cell fragments by macrophages and endothelial cells. Following phagocytosis, immune modulatory factors are secreted, which then act to inhibit inflammation. UC-MSCs that survive the trip through the circulation may engage actively or passively with the endothelium wall, extravasate after engaging with the extracellular matrix (e.g., with MMP-2 and gelatinase), and eventually dwell in a pericyte-like position (Leibacher and Henschler, 2016).
FIGURE 3. Schematic illustration of possible interactions of UC-MSCs following their systemic administration.
Numerous recent clinical trials have demonstrated the importance of UC-MSCs in the treatment of numerous diseases, as presented in Table 3 (Lv et al., 2013; Chang et al., 2014; Kim et al., 2015; Bartolucci et al., 2017; Riordan et al., 2018; Powell and Silvestri, 2019; He et al., 2020; Özmert and Arslan, 2020; Zhang et al., 2021; Kim et al., 2021; Lee et al., 2021; Shi et al., 2021; Monsel et al., 2022; Zang et al., 2022). We retrieved 119 studies using research requests citing “UC-MSCs, Umbilical Cord derived Mesenchymal stromal cells” on ClinicalTrials.gov, of which 36 are complete, 54 are recruiting, 13 are not recruiting, and 63 are in unclear inference. In brief, UC-MSCs’ immuno-modulatory, anti-inflammatory, and regenerative characteristics account for most of their therapeutic applications. The conclusions made as a result of these clinical trials are discussed below.
TABLE 3. Clinical Trials on UC-MSCs recruiting patients from ClinicalTrials.gov.
A phase I/II, randomized, controlled study to assess the safety and efficacy of single/repeated intra-articular injection(s) of UC-MSCs in patients with knee osteoarthritis (NCT02580695) held by Universidad de Los Andes, Chile, showed no adverse events with a significant decrease in pain over 12 months and a reduction in cartilage degradation, inflammatory responses and bone sclerosis. Another phase I/II, an open-label dose escalation clinical trial on the safety and efficacy of intratracheal injection of UC-MSCs in premature infants with high risk for bronchopulmonary dysplasia (BPD) (NCT02381366) conducted by Medipost America Inc. Showed these cells to be well tolerated and safe in 12 extremely low birth weight infants (<28 weeks of gestation and <1,000 g at birth at 5–14 days) warranting for larger randomized-controlled blinded study.
UC-MSCs are clinically effective when given by different routes of administration. A randomized clinical trial of intravenously infused UC-MSCs in patients with cardiopathy (NCT01739777) held by Universidad de Los Andes, Chile demonstrated no adverse reactions to the cell infusion with no alloantibody development within the first 90-day post-treatment. Treated patients also showed significant improvement in left ventricular ejection fraction when assessed by transthoracic echocardiography and cardiac MRI. Another study examined the effects of peribulbar injection of UC-MSCs in patients with retinitis pigmentosa (NCT04315025; PT. Prodia StemCell Indonesia) and showed an improvement in the perception of light and vision, due to the UC-MSCs’ ability to regenerate new photoreceptors and retinal pigment epithelial cells.
A prospective, sequential, open-label phase III clinical study on sub-tenon administration of UC-MSCs for treating retinitis pigmentosa (RP) in patients (NCT04224207) was conducted by Ankara Universitesi Teknokent. Follow up of patients 12 months later demonstrated UC-MSCs is effective in the treatment of RP during the first year without any adverse effect by slowing or stopping the disease progression, regardless of the genetic mutation. In another completed trial, “Intravenously infused UC-MSCs treatment for Crohn’s disease” (NCT02445547) that was conducted by Fuzhou General Hospital showed a decrease in the Crohn’s disease activity, Harvey-Bradshaw index, corticosteroid dosage, and colonoscopy presented significant improvement in the mucosa in patients even at 12 months after receiving treatment.
A study called “UC-MSCs gel treatment in difficult healing skin ulcers” (NCT02685722) was conducted by Chinese PLA General Hospital concluded the therapeutic effects of UC-MSCs were due to autophagy via clearance of advanced glycation end products at injured tissues. Another completed trial called “Clinical study of the treatment of infertility caused by recurrent intrauterine adhesions by collagen scaffold loaded with UC-MSCs” (NCT02313415) conducted by Nanjing University Medical School showed no treatment-related adverse effects, increased endometrial thickness due to endometrial proliferation, differentiation, neovascularization and decreased intrauterine adhesion score which raised the pregnancy above 38.4% by the end of a 30 months follow-up period.
Large-scale growth of UC-MSCs in vitro is required since the number of newly separated cells from the cord tissue is so small (Nitkin and Bonfield, 2017). Thus, developing culturing methods that consistently produce clinical-grade UC-MSCs is critical. Apropos to this, bioreactors offer many benefits over regular two-dimensional culture process, including repeatability, simplicity of monitoring, quantity, and uniformity (Sensebé et al., 2013). Aseptic and automated measures, certified bioreactor additives, and tight monitoring are needed for large-scale production of clinical-grade UC-MSCs. Clinical applications benefit from the capacity to acquire UC-MSCs from donors, store them in the clinical-grade banks, and retrieve them as required. Cryopreservation media without serum has been utilized to prevent cells from being damaged by the freezing process (de Lima Prata et al., 2012). The rate of cooling affects MSC viability, and hence controlled rate freezers are often used for cryopreserving and maintaining UC-MSCs (Marquez-Curtis et al., 2015). Some of the companies that have established cutting-edge controlled rate freezers are listed in Table 4.
When acquired safely and with a pre-agreement after delivery, the use of UC-MSCs do not raise ethical issues. These cells are now a crucial component of research and have shown to be effective as well as lifesaving in thousands of allogeneic settings. However, the storage of stromal cells by private, commercially managed banks for future autologous use for the child is a social and ethical issue. The European group on ethics (EGE) addressed this issue in its 16 March 2004: Opinion 19 on “Ethical considerations of umbilical cord blood banking” (https://ec.europa.eu/info/index_en). Only a few autologous applications are made so far for very uncommon illnesses that can be predicted based on the family history. In these situations, the clinic should preserve MSCs from umbilical cord as part of the solidarity system. In certain European countries (e.g., Italy), advertising for private and self-financed storage is prohibited, and lawsuits are being filed against this practice (e.g., in Belgium) (Virt, 2010). At present, it is essentially a matter of consumer protection, which entails safeguarding individuals from misleading expectations due to false advertising (CJT, 2013).
The recent rise in interest in the unique characteristics of UC-MSCs generated from WJ is due to their advantages over BM-MSCs (the “classical” adult source of MSCs), and include their significant in vitro proliferation in addition to their paracrine and immunomodulatory capabilities. These characteristics, along with the ease with which UC-MSCs can be isolated from an endless supply of tissue, have sparked significant interest in their potential use in cell-based therapies supported by both pre-clinical and clinical investigations. However, sufficient comparative studies are needed soon to answer the long-standing issue of whether MSCs from various anatomical sites within the UC are associated with differences in their qualitative and quantitative therapeutic features. The global expression approaches such as multi-omics profiling, CGH microarrays, methylation mapping, CRISPR/Cas and lab-on-Chip technology are expected to significantly enhance the understanding of UC-MSC biology. These results should not only provide theoretical groundwork for future therapeutic applications of UC-MSCs, but also offer a major contribution to stromal cell biology in general.
SC prepared the entire manuscript, RY designed figure 3 and contributed to biodistribution of MSCs in blood stream, GS outlined the review structure, RP reviewed the manuscript, SR contributed the secretome and Extracellular vesicles section, SR contributed to the clinical trials in UC-MSCs, JZ edited the manuscript, AG contributed to single cell transcriptomics and AT reviewed and edited the manuscript.
This work was supported by research grants from the National Institute of Diabetes and Digestive and Kidney Diseases (NIDDK 119293, 129598, and 129343), and the Stanford Maternal and Child Health Research Institute (MCHRI).
The authors declare that the research was conducted in the absence of any commercial or financial relationships that could be construed as a potential conflict of interest.
All claims expressed in this article are solely those of the authors and do not necessarily represent those of their affiliated organizations, or those of the publisher, the editors and the reviewers. Any product that may be evaluated in this article, or claim that may be made by its manufacturer, is not guaranteed or endorsed by the publisher.
Alcaraz, M. J., Compañ, A., and Guillén, M. I. (2020). Extracellular vesicles from mesenchymal stem cells as novel treatments for musculoskeletal diseases. Cells 9 (1), 98. doi:10.3390/cells9010098
Aleksander-Konert, E., Paduszyński, P., Zajdel, A., Dzierżewicz, Z., and Wilczok, A. (2016). In vitro chondrogenesis of Wharton’s jelly mesenchymal stem cells in hyaluronic acid-based hydrogels. Cell. Mol. Biol. Lett. 21 (1), 11. doi:10.1186/s11658-016-0016-y
Ansari, A. S., Yazid, M. D., Sainik, N. Q. A. V., Razali, R. A., Saim, A. B., and Idrus, R. B. H. (2018). Osteogenic induction of wharton’s jelly-derived mesenchymal stem cell for bone regeneration: A systematic review. Stem Cells Int. 2018, 2406462. doi:10.1155/2018/2406462
Arno, A. I., Amini-Nik, S., Blit, P. H., Al-Shehab, M., Belo, C., Herer, E., et al. (2014). Human Wharton’s jelly mesenchymal stem cells promote skin wound healing through paracrine signaling. Stem Cell Res. Ther. 5 (1), 28–13. doi:10.1186/scrt417
Assfalg, V., Hüser, N., Van Meel, M., Haller, B., Rahmel, A., De Boer, J., et al. (2016). High-urgency kidney transplantation in the eurotransplant kidney allocation system: Success or waste of organs? The eurotransplant 15-year all-centre survey. Nephrol. Dial. Transpl. 31 (9), 1515–1522. doi:10.1093/ndt/gfv446
Bai, L., Li, D., Li, J., Luo, Z., Yu, S., Cao, S., et al. (2016). Bioactive molecules derived from umbilical cord mesenchymal stem cells. Acta Histochem. 118 (8), 761–769. doi:10.1016/j.acthis.2016.09.006
Baksh, D., Yao, R., and Tuan, R. S. (2007). Comparison of proliferative and multilineage differentiation potential of human mesenchymal stem cells derived from umbilical cord and bone marrow. Stem Cells 25 (6), 1384–1392. doi:10.1634/stemcells.2006-0709
Barrett, A. N., Fong, C-Y., Subramanian, A., Liu, W., Feng, Y., Choolani, M., et al. (2019). Human Wharton's jelly mesenchymal stem cells show unique gene expression compared with bone marrow mesenchymal stem cells using single-cell RNA-sequencing. Stem Cells Dev. 28 (3), 196–211. doi:10.1089/scd.2018.0132
Bartolucci, J., Verdugo, F. J., González, P. L., Larrea, R. E., Abarzua, E., Goset, C., et al. (2017). Safety and efficacy of the intravenous infusion of umbilical cord mesenchymal stem cells in patients with heart failure: A phase 1/2 randomized controlled trial (RIMECARD trial [randomized clinical trial of intravenous infusion umbilical cord mesenchymal stem cells on cardiopathy]). Circ. Res. 121 (10), 1192–1204. doi:10.1161/CIRCRESAHA.117.310712
Bhuvanalakshmi, G., Arfuso, F., Kumar, A. P., Dharmarajan, A., and Warrier, S. (2017). Epigenetic reprogramming converts human Wharton’s jelly mesenchymal stem cells into functional cardiomyocytes by differential regulation of Wnt mediators. Stem Cell Res. Ther. 8 (1), 1–15. doi:10.1186/s13287-017-0638-7
Bustos, F., Sepulveda, H., Prieto, C., Carrasco, M., Diaz, L., Palma, J., et al. (2017). Runt-related transcription factor 2 induction during differentiation of wharton's jelly mesenchymal stem cells to osteoblasts is regulated by jumonji AT-rich interactive domain 1B histone demethylase. Stem Cells 35, 2430–2441. doi:10.1002/stem.2704
Cabrera-Pérez, R., Monguió-Tortajada, M., Gámez-Valero, A., Rojas-Márquez, R., Borràs, F. E., Roura, S., et al. (2019). Osteogenic commitment of wharton’s jelly mesenchymal stromal cells: Mechanisms and implications for bioprocess development and clinical application. Stem Cell Res. Ther. 10 (1), 1–11. doi:10.1186/s13287-019-1450-3
Caplan, A. I. (1991). Mesenchymal stem cells. J. Orthop. Res. 9 (5), 641–650. doi:10.1002/jor.1100090504
Caseiro, A., Pereira, T., Ribeiro, J., Santos, J., Amorim, I., Luis, A., et al. (2017). Neuro-muscular regeneration using scaffolds with mesenchymal stem cells (MSCs) isolated from human umbilical cord Wharton's jelly. Ciencia Tecnologia dos Materiais 29 (1), e135–e139. doi:10.1016/j.ctmat.2016.04.003
Chang, Y. S., Ahn, S. Y., Yoo, H. S., Sung, S. I., Choi, S. J., Oh, W. I., et al. (2014). Mesenchymal stem cells for bronchopulmonary dysplasia: Phase 1 dose-escalation clinical trial. J. Pediatr. 164 (5), 966–972. e6. doi:10.1016/j.jpeds.2013.12.011
Chen, G., Yue, A., Ruan, Z., Yin, Y., Wang, R., Ren, Y., et al. (2014). Human umbilical cord-derived mesenchymal stem cells do not undergo malignant transformation during long-term culturing in serum-free medium. PloS one 9 (6), e98565. doi:10.1371/journal.pone.0098565
Chen, S. L., Fang, W. W., Ye, F., Liu, Y. H., Qian, J., Shan, S. J., et al. (2004). Effect on left ventricular function of intracoronary transplantation of autologous bone marrow mesenchymal stem cell in patients with acute myocardial infarction. Am. J. Cardiol. 94 (1), 92–95. doi:10.1016/j.amjcard.2004.03.034
Chen, W., Yan, Y., Song, C., Ding, Y., and Du, T. (2017). Microvesicles derived from human Wharton's Jelly mesenchymal stem cells ameliorate ischemia–reperfusion-induced renal fibrosis by releasing from G2/M cell cycle arrest. Biochem. J. 474 (24), 4207–4218. doi:10.1042/BCJ20170682
Chen, X., Zhang, F., He, X., Xu, Y., Yang, Z., Chen, L., et al. (2013). Chondrogenic differentiation of umbilical cord-derived mesenchymal stem cells in type I collagen-hydrogel for cartilage engineering. Injury 44 (4), 540–549. doi:10.1016/j.injury.2012.09.024
Cheng, J-H., Wang, C-J., Chou, W-Y., Hsu, S-L., Chen, J-H., and Hsu, T-C. (2019). Comparison efficacy of ESWT and Wharton’s jelly mesenchymal stem cell in early osteoarthritis of rat knee. Am. J. Transl. Res. 11 (2), 586–598.
Chetty, S. S., Praneetha, S., Govarthanan, K., Verma, R. S., and Vadivel Murugan, A. (2019). Noninvasive tracking and regenerative capabilities of transplanted human umbilical cord-derived mesenchymal stem cells labeled with I-III-IV semiconducting nanocrystals in liver-injured living mice. ACS Appl. Mat. Interfaces 11 (9), 8763–8778. doi:10.1021/acsami.8b19953
Chetty, S. S., Praneetha, S., Vadivel Murugan, A., Govarthanan, K., and Verma, R. S. (2019). Human umbilical cord wharton’s jelly-derived mesenchymal stem cells labeled with Mn2+ and Gd3+ Co-doped CuInS2–ZnS nanocrystals for multimodality imaging in a tumor mice model. ACS Appl. Mat. Interfaces 12 (3), 3415–3429. doi:10.1021/acsami.9b19054
Cho, H-M., Lee, K-H., Shen, Y-m., Shin, T-J., Ryu, P-D., Choi, M-C., et al. (2020). Transplantation of hMSCs genome edited with LEF1 improves cardio-protective effects in myocardial infarction. Mol. Ther. Nucleic Acids 19, 1186–1197. doi:10.1016/j.omtn.2020.01.007
Choi, J., Bae, T., Byambasuren, N., Park, S-H., Jo, C. H., Kim, D., et al. (2020). CRISPR-Cpf1 activation of endogenous BMP4 gene for osteogenic differentiation of umbilical-cord-derived mesenchymal stem cells. Mol. Ther. Methods Clin. Dev. 17, 309–316. doi:10.1016/j.omtm.2019.12.010
Chudickova, M., Vackova, I., Machova Urdzikova, L., Jancova, P., Kekulova, K., Rehorova, M., et al. (2019). The effect of wharton jelly-derived mesenchymal stromal cells and their conditioned media in the treatment of a rat spinal cord injury. Int. J. Mol. Sci. 20 (18), 4516. doi:10.3390/ijms20184516
Cjt, Petrini. Ethical issues in umbilical cord blood banking: A comparative analysis of documents from national and international institutions2013;53(4):902–910.
Cóndor, J. M., Rodrigues, C. E., de Sousa Moreira, R., Canale, D., Volpini, R. A., Shimizu, M. H., et al. (2016). Treatment with human Wharton's jelly‐derived mesenchymal stem cells attenuates sepsis‐induced kidney injury, liver injury, and endothelial dysfunction. Stem Cells Transl. Med. 5 (8), 1048–1057. doi:10.5966/sctm.2015-0138
Covas, D. T., Panepucci, R. A., Fontes, A. M., Silva, W. A., Orellana, M. D., Freitas, M. C., et al. (2008). Multipotent mesenchymal stromal cells obtained from diverse human tissues share functional properties and gene-expression profile with CD146+ perivascular cells and fibroblasts. Exp. Hematol. 36 (5), 642–654. doi:10.1016/j.exphem.2007.12.015
Davies, J. E., Walker, J. T., and Keating, A. (2017). Concise review: Wharton's jelly: The rich, but enigmatic, source of mesenchymal stromal cells. Stem Cells Transl. Med. 6 (7), 1620–1630. doi:10.1002/sctm.16-0492
de Lima Prata, K., de Santis, G. C., Orellana, M. D., Palma, P. V. B., Brassesco, M. S., and Covas, D. T. (2012). Cryopreservation of umbilical cord mesenchymal cells in xenofree conditions. Cytotherapy 14 (6), 694–700. doi:10.3109/14653249.2012.677820
Ding, C., Zhu, L., Shen, H., Lu, J., Zou, Q., Huang, C., et al. (2020). Exosomal miRNA-17-5p derived from human umbilical cord mesenchymal stem cells improves ovarian function in premature ovarian insufficiency by regulating SIRT7. Stem Cells 38 (9), 1137–1148. doi:10.1002/stem.3204
Ding, M., Shen, Y., Wang, P., Xie, Z., Xu, S., Zhu, Z., et al. (2018). Exosomes isolated from human umbilical cord mesenchymal stem cells alleviate neuroinflammation and reduce amyloid-beta deposition by modulating microglial activation in alzheimer’s disease. Neurochem. Res. 43 (11), 2165–2177. doi:10.1007/s11064-018-2641-5
Dominici, M., Le Blanc, K., Mueller, I., Slaper-Cortenbach, I., Marini, F., Krause, D., et al. (2006). Minimal criteria for defining multipotent mesenchymal stromal cells. The International Society for Cellular Therapy position statement. Cytotherapy 8 (4), 315–317. doi:10.1080/14653240600855905
Du, T., Cheng, J., Zhong, L., Zhao, X-F., Zhu, J., Zhu, Y-J., et al. (2012). The alleviation of acute and chronic kidney injury by human Wharton's jelly-derived mesenchymal stromal cells triggered by ischemia-reperfusion injury via an endocrine mechanism. Cytotherapy 14 (10), 1215–1227. doi:10.3109/14653249.2012.711471
Ebrahim, N., Mostafa, O., El Dosoky, R. E., Ahmed, I. A., Saad, A. S., Mostafa, A., et al. (2018). Human mesenchymal stem cell-derived extracellular vesicles/estrogen combined therapy safely ameliorates experimentally induced intrauterine adhesions in a female rat model. Stem Cell Res. Ther. 9 (1), 175. doi:10.1186/s13287-018-0924-z
Eleuteri, S., and Fierabracci, A. (2019). Insights into the secretome of mesenchymal stem cells and its potential applications. Int. J. Mol. Sci. 20 (18), 4597. doi:10.3390/ijms20184597
Fouillard, L., Chapel, A., Bories, D., Bouchet, S., Costa, J. M., Rouard, H., et al. (2007). Infusion of allogeneic-related HLA mismatched mesenchymal stem cells for the treatment of incomplete engraftment following autologous haematopoietic stem cell transplantation. Leukemia 21 (3), 568–570. doi:10.1038/sj.leu.2404550
Gluckman, E., Broxmeyer, H. A., Auerbach, A. D., Friedman, H. S., Douglas, G. W., Devergie, A., et al. (1989). Hematopoietic reconstitution in a patient with Fanconi's anemia by means of umbilical-cord blood from an HLA-identical sibling. N. Engl. J. Med. 321 (17), 1174–1178. doi:10.1056/NEJM198910263211707
Guadix, J. A., Zugaza, J. L., and Gálvez-Martín, P. (2017). Characteristics, applications and prospects of mesenchymal stem cells in cell therapy. Med. Clin. 148 (9), 408–414. doi:10.1016/j.medcli.2016.11.033
Hammam, O. A., Elkhafif, N., Attia, Y. M., Mansour, M. T., Elmazar, M. M., Abdelsalam, R. M., et al. (2016). Wharton’s jelly-derived mesenchymal stem cells combined with praziquantel as a potential therapy for Schistosoma mansoni-induced liver fibrosis. Sci. Rep. 6 (1), 21005–21014. doi:10.1038/srep21005
Han, Y., Li, X., Zhang, Y., Han, Y., Chang, F., and Ding, J. (2019). Mesenchymal stem cells for regenerative medicine. Cells 8 (8), 886. doi:10.3390/cells8080886
Haraszti, R. A., Miller, R., Stoppato, M., Sere, Y. Y., Coles, A., Didiot, M-C., et al. (2018). Exosomes produced from 3D cultures of MSCs by tangential flow filtration show higher yield and improved activity. Mol. Ther. 26 (12), 2838–2847. doi:10.1016/j.ymthe.2018.09.015
He, X., Wang, Q., Zhao, Y., Zhang, H., Wang, B., Pan, J., et al. (2020). Effect of intramyocardial grafting collagen scaffold with mesenchymal stromal cells in patients with chronic ischemic heart disease: A randomized clinical trial. JAMA Netw. Open 3 (9), e2016236–e. doi:10.1001/jamanetworkopen.2020.16236
Hou, W., Duan, L., Huang, C., Li, X., Xu, X., Qin, P., et al. (2021). Mesenchymal stem cell subpopulations and their heterogeneity of response to inductions revealed by single-cell RNA-seq. New York, United States: bioRxiv.
Hu, D., Zhang, D., Liu, B., Liu, Y., Zhou, Y., Yu, Y., et al. (2020). Human ucMSCs seeded in a decellularized kidney scaffold attenuate renal fibrosis by reducing epithelial–mesenchymal transition via the TGF-β/Smad signaling pathway. Pediatr. Res. 88 (2), 192–201. doi:10.1038/s41390-019-0736-6
Huang, Y., Li, Q., Zhang, K., Hu, M., Wang, Y., Du, L., et al. (2019). Single cell transcriptomic analysis of human mesenchymal stem cells reveals limited heterogeneity. Cell Death Dis. 10 (5), 1–12. doi:10.1038/s41419-019-1583-4
Jiang, L., Zhang, S., Hu, H., Yang, J., Wang, X., Ma, Y., et al. (2019). Exosomes derived from human umbilical cord mesenchymal stem cells alleviate acute liver failure by reducing the activity of the NLRP3 inflammasome in macrophages. Biochem. Biophys. Res. Commun. 508 (3), 735–741. doi:10.1016/j.bbrc.2018.11.189
Joerger-Messerli, M. S., Oppliger, B., Spinelli, M., Thomi, G., Di Salvo, I., Schneider, P., et al. (2018). Extracellular vesicles derived from Wharton’s jelly mesenchymal stem cells prevent and resolve programmed cell death mediated by perinatal hypoxia-ischemia in neuronal cells. Cell Transpl. 27 (1), 168–180. doi:10.1177/0963689717738256
Kim, H. J., Cho, K. R., Jang, H., Lee, N. K., Jung, Y. H., Kim, J. P., et al. (2021). Intracerebroventricular injection of human umbilical cord blood mesenchymal stem cells in patients with alzheimer’s disease dementia: A phase I clinical trial Alzheimers Res. Ther. 13 (1), 1–11. doi:10.1186/s13195-021-00897-2
Kim, H. J., Seo, S. W., Chang, J. W., Lee, J. I., Kim, C. H., Chin, J., et al. (2015). Stereotactic brain injection of human umbilical cord blood mesenchymal stem cells in patients with alzheimer's disease dementia: A phase 1 clinical trial.. Alzheimers Dement. 1 (2), 95–102. doi:10.1016/j.trci.2015.06.007
Kim, Y. E., Sung, S. I., Chang, Y. S., Ahn, S. Y., Sung, D. K., and Park, W. S. (2019). Thrombin preconditioning enhances therapeutic efficacy of human wharton’s jelly–derived mesenchymal stem cells in severe neonatal hypoxic ischemic encephalopathy. Int. J. Mol. Sci. 20 (10), 2477. doi:10.3390/ijms20102477
Koike, C., Zhou, K., Takeda, Y., Fathy, M., Okabe, M., Yoshida, T., et al. (2014). Characterization of amniotic stem cells. Cell. Reprogr. 16 (4), 298–305. doi:10.1089/cell.2013.0090
Kowalski, K., Kołodziejczyk, A., Sikorska, M., Płaczkiewicz, J., Cichosz, P., Kowalewska, M., et al. (2017). Stem cells migration during skeletal muscle regeneration-the role of Sdf-1/Cxcr4 and Sdf-1/Cxcr7 axis. Cell adh. Migr. 11 (4), 384–398. doi:10.1080/19336918.2016.1227911
Kwon, S., Ki, S. M., Park, S. E., Kim, M-J., Hyung, B., Lee, N. K., et al. (2016). Anti-apoptotic effects of human Wharton's jelly-derived mesenchymal stem cells on skeletal muscle cells mediated via secretion of XCL1. Mol. Ther. 24 (9), 1550–1560. doi:10.1038/mt.2016.125
Laurila, J. P., Laatikainen, L., Castellone, M. D., Trivedi, P., Heikkila, J., Hinkkanen, A., et al. (2009). Human embryonic stem cell-derived mesenchymal stromal cell transplantation in a rat hind limb injury model. Taylor & Francis.
Law, S., and Chaudhuri, S. (2013). Mesenchymal stem cell and regenerative medicine: Regeneration versus immunomodulatory challenges. Am. J. Stem Cells 2 (1), 22–38.
Lazarus, H. M., Koc, O. N., Devine, S. M., Curtin, P., Maziarz, R. T., Holland, H. K., et al. (2005). Cotransplantation of HLA-identical sibling culture-expanded mesenchymal stem cells and hematopoietic stem cells in hematologic malignancy patients. Biol. Blood Marrow Transpl. 11 (5), 389–398. doi:10.1016/j.bbmt.2005.02.001
Lee, J., Bayarsaikhan, D., Arivazhagan, R., Park, H., Lim, B., Gwak, P., et al. (2019). CRISPR/Cas9 edited sRAGE-MSCs protect neuronal death in Parkinson’s disease model. Int. J. Stem Cells 12 (1), 114–124. doi:10.15283/ijsc18110
Lee, P. H., Kim, J. W., Bang, O. Y., Ahn, Y. H., Joo, I. S., and Huh, K. (2008). Autologous mesenchymal stem cell therapy delays the progression of neurological deficits in patients with multiple system atrophy. Clin. Pharmacol. Ther. 83 (5), 723–730. doi:10.1038/sj.clpt.6100386
Lee, S. E., Lee, S-J., Kim, S-E., Kim, K., Cho, B., Roh, K., et al. (2021). Intravenous allogeneic umbilical cord blood–derived mesenchymal stem cell therapy in recessive dystrophic epidermolysis bullosa patients. JCI insight 6 (2), 143606. doi:10.1172/jci.insight.143606
Leibacher, J., and Henschler, R. (2016). Biodistribution, migration and homing of systemically applied mesenchymal stem/stromal cells. Stem Cell Res. Ther. 7 (1), 7–12. doi:10.1186/s13287-015-0271-2
Li, C., Chen, X., Qiao, S., Liu, X., Liu, C., Zhu, D., et al. (2016). Effects of Wharton's jelly cells of the human umbilical cord on acute spinal cord injury in rats, and expression of interleukin-1β and nerve growth factor in spinal cord tissues. Artif. Cells Nanomed. Biotechnol. 44 (5), 1254–1258. doi:10.3109/21691401.2015.1019671
Liau, L., Ruszymah, B., Ng, M., and Law, J. (2020). Characteristics and clinical applications of Wharton’s jelly-derived mesenchymal stromal cells. Curr. Res. Transl. Med. 68 (1), 5–16. doi:10.1016/j.retram.2019.09.001
Lim, J., Razi, Z. R. M., Law, J. X., Nawi, A. M., Idrus, R. B. H., Chin, T. G., et al. (2018). Mesenchymal stromal cells from the maternal segment of human umbilical cord is ideal for bone regeneration in allogenic setting. Tissue Eng. Regen. Med. 15 (1), 75–87. doi:10.1007/s13770-017-0086-6
Lim, M., Wang, W., Liang, L., Han, Z-b., Li, Z., Geng, J., et al. (2018). Intravenous injection of allogeneic umbilical cord-derived multipotent mesenchymal stromal cells reduces the infarct area and ameliorates cardiac function in a porcine model of acute myocardial infarction. Stem Cell Res. Ther. 9 (1), 129. doi:10.1186/s13287-018-0888-z
Liu, C-B., Huang, H., Sun, P., Ma, S-Z., Liu, A-H., Xue, J., et al. (2016). Human umbilical cord‐derived mesenchymal stromal cells improve left ventricular function, perfusion, and remodeling in a porcine model of chronic myocardial ischemia. Stem Cells Transl. Med. 5 (8), 1004–1013. doi:10.5966/sctm.2015-0298
Liu, X. Y., Wei, M. G., Liang, J., Xu, H. H., Wang, J. J., Wang, J., et al. (2020). Injury-preconditioning secretome of umbilical cord mesenchymal stem cells amplified the neurogenesis and cognitive recovery after severe traumatic brain injury in rats. J. Neurochem. 153 (2), 230–251. doi:10.1111/jnc.14859
Liu, Y., Fang, J., Zhang, Q., Zhang, X., Cao, Y., Chen, W., et al. (2020). Wnt10b-overexpressing umbilical cord mesenchymal stem cells promote critical size rat calvarial defect healing by enhanced osteogenesis and VEGF-mediated angiogenesis. J. Orthop. Transl. 23, 29–37. doi:10.1016/j.jot.2020.02.009
Lo, B., and Parham, L. (2009). Ethical issues in stem cell research. Endocr. Rev. 30 (3), 204–213. doi:10.1210/er.2008-0031
Lv, Y-T., Zhang, Y., Liu, M., Ashwood, P., Cho, S. C., Huan, Y., et al. (2013). Transplantation of human cord blood mononuclear cells and umbilical cord-derived mesenchymal stem cells in autism. J. Transl. Med. 11 (1), 1–10. doi:10.1186/1479-5876-11-196
Ma, Z. J., Wang, Y. H., Li, Z. G., Wang, Y., Li, B. Y., Kang, H. Y., et al. (2019). Immunosuppressive effect of exosomes from mesenchymal stromal cells in defined medium on experimental colitis. Int. J. Stem Cells 12 (3), 440–448. doi:10.15283/ijsc18139
Mafi, P., Hindocha, S., Mafi, R., Griffin, M., and Khan, W. (2011). Suppl 2: Adult mesenchymal stem cells and cell surface characterization-A systematic review of the literature. Open Orthop. J. 5, 253–260. doi:10.2174/1874325001105010253
Majore, I., Moretti, P., Hass, R., and Kasper, C. (2009). Identification of subpopulations in mesenchymal stem cell-like cultures from human umbilical cord. Cell Commun. Signal. 7 (1), 6–8. doi:10.1186/1478-811X-7-6
Majore, I., Moretti, P., Stahl, F., Hass, R., and Kasper, C. (2011). Growth and differentiation properties of mesenchymal stromal cell populations derived from whole human umbilical cord. Stem Cell Rev. Rep. 7 (1), 17–31. doi:10.1007/s12015-010-9165-y
Marquez-Curtis, L. A., Janowska-Wieczorek, A., McGann, L. E., and Elliott, J. A. (2015). Mesenchymal stromal cells derived from various tissues: Biological, clinical and cryopreservation aspects. Cryobiology 71 (2), 181–197. doi:10.1016/j.cryobiol.2015.07.003
Martin-Piedra, M. Á., Alfonso-Rodríguez, C. A., Zapater Latorre, A., Durand-Herrera, D., Chato-Astrain, J., Campos, F., et al. (2019). Effective use of mesenchymal stem cells in human skin substitutes generated by tissue engineering. Eur. Cell. Mat. 37, 233–249. doi:10.22203/eCM.v037a14
Matas, J., Orrego, M., Amenabar, D., Infante, C., Tapia‐Limonchi, R., Cadiz, M. I., et al. (2019). Umbilical cord‐derived mesenchymal stromal cells (MSCs) for knee osteoarthritis: Repeated MSC dosing is superior to a single MSC dose and to hyaluronic acid in a controlled randomized phase I/II trial. Stem Cells Transl. Med. 8 (3), 215–224. doi:10.1002/sctm.18-0053
McElreavey, K. D., Irvine, A. I., Ennis, K. T., and McLean, W. H. (1991). Isolation, culture and characterisation of fibroblast-like cells derived from the Wharton's jelly portion of human umbilical cord. Biochem. Soc. Trans. 19 (1), 29s. doi:10.1042/bst019029s
Mesure, B., Huber-Villaume, S., Menu, P., and Velot, E. (2017). Transforming growth factor-beta 1 or ascorbic acid are able to differentiate Wharton’s jelly mesenchymal stem cells towards a smooth muscle phenotype. Biomed. Mat. Eng. 28, S101–S5. doi:10.3233/BME-171630
Millán-Rivero, J. E., Martínez, C. M., Romecín, P. A., Aznar-Cervantes, S. D., Carpes-Ruiz, M., Cenis, J. L., et al. (2019). Silk fibroin scaffolds seeded with Wharton’s jelly mesenchymal stem cells enhance re-epithelialization and reduce formation of scar tissue after cutaneous wound healing. Stem Cell Res. Ther. 10 (1), 1–14. doi:10.1186/s13287-019-1229-6
Mitchell, K. E., Weiss, M. L., Mitchell, B. M., Martin, P., Davis, D., Morales, L., et al. (2003). Matrix cells from Wharton's jelly form neurons and glia. Stem Cells 21 (1), 50–60. doi:10.1634/stemcells.21-1-50
Mohamadi, Y., Moghahi, S. M. H. N., Mousavi, M., Borhani-Haghighi, M., Abolhassani, F., Kashani, I. R., et al. (2019). Intrathecal transplantation of Wharton’s jelly mesenchymal stem cells suppresses the NLRP1 inflammasome in the rat model of spinal cord injury. J. Chem. Neuroanat. 97, 1–8. doi:10.1016/j.jchemneu.2019.01.011
Monsel, A., Hauw-Berlemont, C., Mebarki, M., Heming, N., Mayaux, J., Nguekap Tchoumba, O., et al. (2022). Treatment of COVID-19-associated ARDS with mesenchymal stromal cells: A multicenter randomized double-blind trial. Crit. Care 26 (1), 48–14. doi:10.1186/s13054-022-03930-4
Mortezaee, K., Minaii, B., Sabbaghziarani, F., Kashani, I. R., Hassanzadeh, G., Pasbakhsh, P., et al. (2015). Retinoic acid as the stimulating factor for differentiation of Wharton's Jelly-Mesenchymal stem cells into hepatocyte-like cells. Avicenna J. Med. Biotechnol. 7 (3), 106–112.
Nauta, A. J., and Fibbe, W. E. (2007). Immunomodulatory properties of mesenchymal stromal cells. Blood 110 (10), 3499–3506. doi:10.1182/blood-2007-02-069716
Ngadiono, E., and Hardiany, N. S. (2019). Advancing towards effective glioma therapy: MicroRNA derived from umbilical cord mesenchymal stem cells' extracellular vesicles. Malays. J. Med. Sci. 26 (4), 5–16. doi:10.21315/mjms2019.26.4.2
Nimsanor, N., Phetfong, J., Kitiyanant, N., Kamprom, W., and Supokawej, A. (2019). Overexpression of anti-fibrotic factors ameliorates anti-fibrotic properties of Wharton's jelly derived mesenchymal stem cells under oxidative damage. Biosci. Trends 13 (5), 411–422. doi:10.5582/bst.2019.01191
Nitkin, C. R., and Bonfield, T. L. (2017). Concise review: Mesenchymal stem cell therapy for pediatric disease: Perspectives on success and potential improvements. Stem Cells Transl. Med. 6 (2), 539–565. doi:10.5966/sctm.2015-0427
Norooznezhad, A. H., Yarani, R., Payandeh, M., Hoseinkhani, Z., Kiani, S., Taghizadeh, E., et al. (2022). Human placental mesenchymal stromal cell‐derived exosome‐enriched extracellular vesicles for chronic cutaneous graft‐versus‐host disease: A case report. J. Cell. Mol. Med. 26 (2), 588–592. doi:10.1111/jcmm.17114
Özmert, E., and Arslan, U. (2020). Management of retinitis pigmentosa by wharton’s jelly-derived mesenchymal stem cells: Prospective analysis of 1-year results. Stem Cell Res. Ther. 11 (1), 1–17. doi:10.1186/s13287-020-01870-w
Paduszyński, P., Aleksander-Konert, E., Zajdel, A., Wilczok, A., Jelonek, K., Witek, A., et al. (2016). Changes in expression of cartilaginous genes during chondrogenesis of Wharton’s jelly mesenchymal stem cells on three-dimensional biodegradable poly (L-lactide-co-glycolide) scaffolds. Cell. Mol. Biol. Lett. 21 (1), 14–15. doi:10.1186/s11658-016-0012-2
Parekkadan, B., and Milwid, J. M. (2010). Mesenchymal stem cells as therapeutics. Annu. Rev. Biomed. Eng. 12, 87–117. doi:10.1146/annurev-bioeng-070909-105309
Park, A., Park, H., Yoon, J., Kang, D., Kang, M-H., Park, Y., et al. (2019). Priming with Toll-like receptor 3 agonist or interferon-gamma enhances the therapeutic effects of human mesenchymal stem cells in a murine model of atopic dermatitis. Stem Cell Res. Ther. 10 (1), 66–11. doi:10.1186/s13287-019-1164-6
Payandeh, M., Habibi, R., Norooznezhad, A. H., Hoseinkhani, Z., Mansouri, F., Yarani, R., et al. (2022). Human placenta-derived mesenchymal stromal cells transfusion in a critically ill infant diagnosed with coronavirus disease 2019 (COVID-19): A case report. Transfus. Apher. Sci., 103454. doi:10.1016/j.transci.2022.103454
Pera, M. F. (2009). Stem cells: Low-risk reprogramming. Nature 458 (7239), 715–716. doi:10.1038/458715a
Pipino, C., Shangaris, P., Resca, E., Zia, S., Deprest, J., Sebire, N. J., et al. (2013). Placenta as a reservoir of stem cells: An underutilized resource? Br. Med. Bull. 105, 43–68. doi:10.1093/bmb/lds033
Powell, S. B., and Silvestri, J. M. (2019). Safety of intratracheal administration of human umbilical cord blood derived mesenchymal stromal cells in extremely low birth weight preterm infants. J. Pediatr. 210, 209–213. doi:10.1016/j.jpeds.2019.02.029
Pu, L., Meng, M., Wu, J., Zhang, J., Hou, Z., Gao, H., et al. (2017). Compared to the amniotic membrane, Wharton’s jelly may be a more suitable source of mesenchymal stem cells for cardiovascular tissue engineering and clinical regeneration. Stem Cell Res. Ther. 8 (1), 72–16. doi:10.1186/s13287-017-0501-x
Rabbani, S., Soleimani, M., Imani, M., Sahebjam, M., Ghiaseddin, A., Nassiri, S. M., et al. (2017). Regenerating heart using a novel compound and human wharton jelly mesenchymal stem cells. Arch. Med. Res. 48 (3), 228–237. doi:10.1016/j.arcmed.2017.03.019
Ramanathan, R., Rupert, S., Selvaraj, S., Satyanesan, J., Vennila, R., and Rajagopal, S. (2017). Role of human Wharton's jelly derived Mesenchymal stem cells (WJ-MSCs) for rescue of d-Galactosamine induced acute liver injury in mice. J. Clin. Exp. Hepatol. 7 (3), 205–214. doi:10.1016/j.jceh.2017.03.010
Reinders, M. E., de Fijter, J. W., Roelofs, H., Bajema, I. M., de Vries, D. K., Schaapherder, A. F., et al. (2013). Autologous bone marrow‐derived mesenchymal stromal cells for the treatment of allograft rejection after renal transplantation: Results of a phase I study. Stem Cells Transl. Med. 2 (2), 107–111. doi:10.5966/sctm.2012-0114
Rezaeian, L., Hosseini, S. E., Dianatpour, M., Edalatmanesh, M. A., Tanideh, N., Mogheiseh, A., et al. (2018). Intrauterine xenotransplantation of human wharton jelly-derived mesenchymal stem cells into the liver of rabbit fetuses: A preliminary study for in vivo expression of the human liver genes. Iran. J. Basic Med. Sci. 21 (1), 89–96. doi:10.22038/IJBMS.2017.24501.6098
Riordan, N. H., Morales, I., Fernández, G., Allen, N., Fearnot, N. E., Leckrone, M. E., et al. (2018). Clinical feasibility of umbilical cord tissue-derived mesenchymal stem cells in the treatment of multiple sclerosis. J. Transl. Med. 16 (1), 57–12. doi:10.1186/s12967-018-1433-7
Romanov, Y. A., Svintsitskaya, V. A., and Smirnov, V. N. (2003). Searching for alternative sources of postnatal human mesenchymal stem cells: Candidate MSC-like cells from umbilical cord. Stem Cells 21 (1), 105–110. doi:10.1634/stemcells.21-1-105
Ryu, H., Kang, B., Park, S., Kim, Y., Sung, G., Woo, H., et al. (2012). Comparison of mesenchymal stem cells derived from fat, bone marrow, Wharton’s jelly, and umbilical cord blood for treating spinal cord injuries in dogs. J. Vet. Med. Sci. 74, 1617–1630. doi:10.1292/jvms.12-0065
Saeedi, P., Halabian, R., and Fooladi, A. A. I. (2019). A revealing review of mesenchymal stem cells therapy, clinical perspectives and Modification strategies. Stem Cell Investig. 6, 34. doi:10.21037/sci.2019.08.11
Saulnier, N., Viguier, E., Perrier-Groult, E., Chenu, C., Pillet, E., Roger, T., et al. (2015). Intra-articular administration of xenogeneic neonatal mesenchymal stromal cells early after meniscal injury down-regulates metalloproteinase gene expression in synovium and prevents cartilage degradation in a rabbit model of osteoarthritis. Osteoarthr. Cartil. 23 (1), 122–133. doi:10.1016/j.joca.2014.09.007
Sensebé, L., Gadelorge, M., and Fleury-Cappellesso, S. (2013). Production of mesenchymal stromal/stem cells according to good manufacturing practices: A review. Stem Cell Res. Ther. 4 (3), 66–6. doi:10.1186/scrt217
Shi, L., Huang, H., Lu, X., Yan, X., Jiang, X., Xu, R., et al. (2021). Effect of human umbilical cord-derived mesenchymal stem cells on lung damage in severe COVID-19 patients: A randomized, double-blind, placebo-controlled phase 2 trial. Signal Transduct. Target. Ther. 6 (1), 58–59. doi:10.1038/s41392-021-00488-5
Shie, M-Y., Chang, W-C., Wei, L-J., Huang, Y-H., Chen, C-H., Shih, C-T., et al. (2017). 3D printing of cytocompatible water-based light-cured polyurethane with hyaluronic acid for cartilage tissue engineering applications. Materials 10 (2), 136. doi:10.3390/ma10020136
Shiue, S-J., Rau, R-H., Shiue, H-S., Hung, Y-W., Li, Z-X., Yang, K. D., et al. (2019). Mesenchymal stem cell exosomes as a cell-free therapy for nerve injury–induced pain in rats. Pain 160 (1), 210–223. doi:10.1097/j.pain.0000000000001395
Soltani, S., Mansouri, K., Parvaneh, S., Thakor, A. S., Pociot, F., and Yarani, R. (2021). Diabetes complications and extracellular vesicle therapy. Rev. Endocr. Metabolic Disord. 23, 1–29. doi:10.1007/s11154-021-09680-y
Su, W-H., Wang, C-J., Fu, H-C., Sheng, C-M., Tsai, C-C., Cheng, J-H., et al. (2019). Human umbilical cord mesenchymal stem cells extricate bupivacaine-impaired skeletal muscle function via mitigating neutrophil-mediated acute inflammation and protecting against fibrosis. Int. J. Mol. Sci. 20 (17), 4312. doi:10.3390/ijms20174312
Subramanian, A., Fong, C-Y., Biswas, A., and Bongso, A. (2015). Comparative characterization of cells from the various compartments of the human umbilical cord shows that the Wharton’s jelly compartment provides the best source of clinically utilizable mesenchymal stem cells. PloS one 10 (6), e0127992. doi:10.1371/journal.pone.0127992
Summers, D. M., Johnson, R. J., Allen, J., Fuggle, S. V., Collett, D., Watson, C. J., et al. (2010). Analysis of factors that affect outcome after transplantation of kidneys donated after cardiac death in the UK: A cohort study. Lancet 376 (9749), 1303–1311. doi:10.1016/S0140-6736(10)60827-6
Sun, J., Zhang, Y., Song, X., Zhu, J., and Zhu, Q. (2019). The healing effects of conditioned medium derived from mesenchymal stem cells on radiation-induced skin wounds in rats. Cell Transpl. 28 (1), 105–115. doi:10.1177/0963689718807410
Sun, Q., Hong, L., Huang, Z., Na, N., Hua, X., Peng, Y., et al. (2017). Allogeneic mesenchymal stem cell as induction therapy to prevent both delayed graft function and acute rejection in deceased donor renal transplantation: Study protocol for a randomized controlled trial. Trials 18 (1), 545–548. doi:10.1186/s13063-017-2291-y
Sun, Q., Huang, Z., Han, F., Zhao, M., Cao, R., Zhao, D., et al. (2018). Allogeneic mesenchymal stem cells as induction therapy are safe and feasible in renal allografts: Pilot results of a multicenter randomized controlled trial. J. Transl. Med. 16 (1), 52–10. doi:10.1186/s12967-018-1422-x
Théry, C., Witwer, K. W., Aikawa, E., Alcaraz, M. J., Anderson, J. D., Andriantsitohaina, R., et al. (2018). Minimal information for studies of extracellular vesicles 2018 (MISEV2018): A position statement of the international society for extracellular vesicles and update of the MISEV2014 guidelines. J. Extracell. Vesicles 7 (1), 1535750. doi:10.1080/20013078.2018.1535750
Todeschi, M. R., El Backly, R., Capelli, C., Daga, A., Patrone, E., Introna, M., et al. (2015). Transplanted umbilical cord mesenchymal stem cells modify the in vivo microenvironment enhancing angiogenesis and leading to bone regeneration. Stem Cells Dev. 24 (13), 1570–1581. doi:10.1089/scd.2014.0490
Tsai, P. C., Fu, T. W., Chen, Y. M. A., Ko, T. L., Chen, T. H., Shih, Y. H., et al. (2009). The therapeutic potential of human umbilical mesenchymal stem cells from Wharton's jelly in the treatment of rat liver fibrosis. Liver Transpl. 15 (5), 484–495. doi:10.1002/lt.21715
Ullah, M., Liu, D. D., and Thakor, A. S. J. I. (2019). Mesenchymal stromal cell homing: Mechanisms and strategies for improvement2019. iScience 15, 421–438. doi:10.1016/j.isci.2019.05.004
Violatto, M. B., Santangelo, C., Capelli, C., Frapolli, R., Ferrari, R., Sitia, L., et al. (2015). Longitudinal tracking of triple labeled umbilical cord derived mesenchymal stromal cells in a mouse model of amyotrophic lateral sclerosis. Stem Cell Res. 15 (1), 243–253. doi:10.1016/j.scr.2015.06.010
Virt, G. (2010). Ethical aspects of human embryonic stem cell use and commercial umbilical cord blood stem cell banking. Ethical reflections on the occasion of the regulation of the European Council and Parliament on advanced therapy medicinal products. Bundesgesundheitsblatt Gesundheitsforsch. Gesundheitsschutz 53 (1), 63–67. doi:10.1007/s00103-009-0994-2
Viswanathan, S., Shi, Y., Galipeau, J., Krampera, M., Leblanc, K., and Martin, I. Mesenchymal stem versus stromal cells: International society for cell & gene therapy (ISCT®) mesenchymal stromal cell committee position statement on nomenclature2019;21(10):1019–1024.
Wang, A. Y. L., Loh, C. Y. Y., Shen, H-H., Hsieh, S-Y., Wang, I-K., Lee, C-M., et al. (2020). Human Wharton’s jelly mesenchymal stem cell-mediated sciatic nerve recovery is associated with the upregulation of regulatory T cells. Int. J. Mol. Sci. 21 (17), 6310. doi:10.3390/ijms21176310
Wang, Q., Li, J., Wang, S., Deng, Q., Wang, K., Dai, X., et al. (2021). Single‐cell transcriptome profiling reveals molecular heterogeneity in human umbilical cord tissue and culture‐expanded mesenchymal stem cells. FEBS J. 288 (18), 5311–5330. doi:10.1111/febs.15834
Wang, Q-Q., Jing, X-M., Bi, Y-Z., Cao, X-F., Wang, Y-Z., Li, Y-X., et al. (2018). Human umbilical cord Wharton’s jelly derived mesenchymal stromal cells may attenuate sarcopenia in aged mice induced by hindlimb suspension. Med. Sci. Monit. 24, 9272–9281. doi:10.12659/MSM.913362
Wang, Y., Zhang, Z., Chi, Y., Zhang, Q., Xu, F., Yang, Z., et al. (2013). Long-term cultured mesenchymal stem cells frequently develop genomic mutations but do not undergo malignant transformation. Cell Death Dis. 4 (12), e950–e. doi:10.1038/cddis.2013.480
Wegmeyer, H., Bröske, A-M., Leddin, M., Kuentzer, K., Nisslbeck, A. K., Hupfeld, J., et al. (2013). Mesenchymal stromal cell characteristics vary depending on their origin. Stem Cells Dev. 22 (19), 2606–2618. doi:10.1089/scd.2013.0016
Wells, C. A., and Choi, J. (2019). Transcriptional profiling of stem cells: Moving from descriptive to predictive paradigms. Stem Cell Rep. 13 (2), 237–246. doi:10.1016/j.stemcr.2019.07.008
Wetzig, A., Alaiya, A., Al-Alwan, M., Pradez, C. B., Pulicat, M. S., Al-Mazrou, A., et al. (2013). Differential marker expression by cultures rich in mesenchymal stem cells. BMC Cell Biol. 14, 54. doi:10.1186/1471-2121-14-54
Wu, P. K., Wang, J. Y., Chen, C. F., Chao, K. Y., Chang, M. C., Chen, W. M., et al. (2017). Early passage mesenchymal stem cells display decreased radiosensitivity and increased DNA repair activity. Stem Cells Transl. Med. 6 (6), 1504–1514. doi:10.1002/sctm.15-0394
Wu, X., Yan, T., Wang, Z., Wu, X., Cao, G., Zhang, C., et al. (2018). Micro‐vesicles derived from human Wharton's Jelly mesenchymal stromal cells mitigate renal ischemia‐reperfusion injury in rats after cardiac death renal transplantation. J. Cell. Biochem. 119 (2), 1879–1888. doi:10.1002/jcb.26348
Xu, H., Wang, Z., Liu, L., Zhang, B., and Li, B. (2020). Exosomes derived from adipose tissue, bone marrow, and umbilical cord blood for cardioprotection after myocardial infarction. J. Cell. Biochem. 121 (3), 2089–2102. doi:10.1002/jcb.27399
Yang, D., Scavuzzo, M. A., Chmielowiec, J., Sharp, R., Bajic, A., and Borowiak, M. (2016). Enrichment of G2/M cell cycle phase in human pluripotent stem cells enhances HDR-mediated gene repair with customizable endonucleases. Sci. Rep. 6 (1), 1–15. doi:10.1038/srep21264
Yang, S., Zhu, B., Yin, P., Zhao, L., Wang, Y., Fu, Z., et al. (2020). Integration of human umbilical cord mesenchymal stem cells-derived exosomes with hydroxyapatite-embedded hyaluronic acid-alginate hydrogel for bone regeneration. ACS Biomater. Sci. Eng. 6 (3), 1590–1602. doi:10.1021/acsbiomaterials.9b01363
Zang, L., Li, Y., Hao, H., Liu, J., Cheng, Y., Li, B., et al. (2022). Efficacy and safety of umbilical cord-derived mesenchymal stem cells in Chinese adults with type 2 diabetes: A single-center, double-blinded, randomized, placebo-controlled phase II trial. Stem Cell Res. Ther. 13 (1), 1–10. doi:10.1186/s13287-022-02848-6
Zhang, C., Zhou, G., Chen, Y., Liu, S., Chen, F., Xie, L., et al. (2018). Human umbilical cord mesenchymal stem cells alleviate interstitial fibrosis and cardiac dysfunction in a dilated cardiomyopathy rat model by inhibiting TNF-α and TGF-β1/ERK1/2 signaling pathways. Mol. Med. Rep. 17 (1), 71–78. doi:10.3892/mmr.2017.7882
Zhang, G., Zou, X., Huang, Y., Wang, F., Miao, S., Liu, G., et al. (2016). Mesenchymal stromal cell-derived extracellular vesicles protect against acute kidney injury through anti-oxidation by enhancing Nrf2/ARE activation in rats. Kidney Blood Press. Res. 41 (2), 119–128. doi:10.1159/000443413
Zhang, G., Zou, X., Miao, S., Chen, J., Du, T., Zhong, L., et al. (2014). The anti-oxidative role of micro-vesicles derived from human Wharton-Jelly mesenchymal stromal cells through NOX2/gp91 (phox) suppression in alleviating renal ischemia-reperfusion injury in rats. PloS one 9 (3), e92129. doi:10.1371/journal.pone.0092129
Zhang, H., Zhang, B., Tao, Y., Cheng, M., Hu, J., Xu, M., et al. (2012). Isolation and characterization of mesenchymal stem cells from whole human umbilical cord applying a single enzyme approach. Cell biochem. Funct. 30 (8), 643–649. doi:10.1002/cbf.2843
Zhang, S., Chen, L., Zhang, G., and Zhang, B. (2020). Umbilical cord-matrix stem cells induce the functional restoration of vascular endothelial cells and enhance skin wound healing in diabetic mice via the polarized macrophages. Stem Cell Res. Ther. 11 (1), 39. doi:10.1186/s13287-020-1561-x
Zhang, S., Jiang, L., Hu, H., Wang, H., Wang, X., Jiang, J., et al. (2020). Pretreatment of exosomes derived from hUCMSCs with TNF-alpha ameliorates acute liver failure by inhibiting the activation of NLRP3 in macrophage. Life Sci. 246, 117401. doi:10.1016/j.lfs.2020.117401
Zhang, Y., Liu, S., Guo, W., Hao, C., Wang, M., Li, X., et al. (2019). Coculture of hWJMSCs and pACs in oriented scaffold enhances hyaline cartilage regeneration in vitro. Stem Cells Int. 2019, 5130152. doi:10.1155/2019/5130152
Keywords: umbilical cord (UC), mesenchymal stem cells, extracellular vesicles (EVs), clinical trials, regenerative therapy, single cell transcriptomics
Citation: Chetty S, Yarani R, Swaminathan G, Primavera R, Regmi S, Rai S, Zhong J, Ganguly A and Thakor AS (2022) Umbilical cord mesenchymal stromal cells—from bench to bedside. Front. Cell Dev. Biol. 10:1006295. doi: 10.3389/fcell.2022.1006295
Received: 29 July 2022; Accepted: 27 September 2022;
Published: 12 October 2022.
Edited by:
Lindolfo da Silva Meirelles, Universidade Luterana do Brasil, BrazilReviewed by:
Marcelo Coutinho De Miranda, Albert Einstein College of Medicine, United StatesCopyright © 2022 Chetty, Yarani, Swaminathan, Primavera, Regmi, Rai, Zhong, Ganguly and Thakor. This is an open-access article distributed under the terms of the Creative Commons Attribution License (CC BY). The use, distribution or reproduction in other forums is permitted, provided the original author(s) and the copyright owner(s) are credited and that the original publication in this journal is cited, in accordance with accepted academic practice. No use, distribution or reproduction is permitted which does not comply with these terms.
*Correspondence: Avnesh S Thakor, YXN0aGFrb3JAc3RhbmZvcmQuZWR1
Disclaimer: All claims expressed in this article are solely those of the authors and do not necessarily represent those of their affiliated organizations, or those of the publisher, the editors and the reviewers. Any product that may be evaluated in this article or claim that may be made by its manufacturer is not guaranteed or endorsed by the publisher.
Research integrity at Frontiers
Learn more about the work of our research integrity team to safeguard the quality of each article we publish.