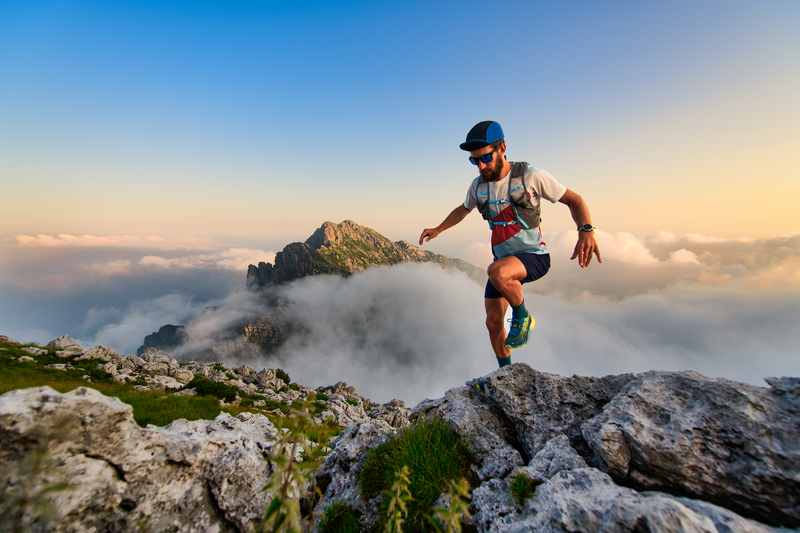
95% of researchers rate our articles as excellent or good
Learn more about the work of our research integrity team to safeguard the quality of each article we publish.
Find out more
REVIEW article
Front. Cell Dev. Biol. , 02 November 2022
Sec. Stem Cell Research
Volume 10 - 2022 | https://doi.org/10.3389/fcell.2022.1005926
Over the past 2 decades, mesenchymal stem cells (MSCs) have attracted a lot of interest as a unique therapeutic approach for a variety of diseases. MSCs are capable of self-renewal and multilineage differentiation capacity, immunomodulatory, and anti-inflammatory properties allowing it to play a role in regenerative medicine. Furthermore, MSCs are low in tumorigenicity and immune privileged, which permits the use of allogeneic MSCs for therapies that eliminate the need to collect MSCs directly from patients. Induced pluripotent stem cells (iPSCs) can be generated from adult cells through gene reprogramming with ectopic expression of specific pluripotency factors. Advancement in iPS technology avoids the destruction of embryos to make pluripotent cells, making it free of ethical concerns. iPSCs can self-renew and develop into a plethora of specialized cells making it a useful resource for regenerative medicine as they may be created from any human source. MSCs have also been used to treat individuals infected with the SARS-CoV-2 virus. MSCs have undergone more clinical trials than iPSCs due to high tumorigenicity, which can trigger oncogenic transformation. In this review, we discussed the overview of mesenchymal stem cells and induced pluripotent stem cells. We briefly present therapeutic approaches and COVID-19-related diseases using MSCs and iPSCs.
Stem cells have the potential to self-renew and can differentiate into a variety of differentiated mature cell types (Weissman, 2000). Embryonic and adult stem cells are both possible sources of stem cells. Their differentiation enables production of differentiated cells that build tissues and organs (Kolios and Moodley, 2013). As a general rule, stem cells possess three main characteristics: First, they are self-renewing, allowing them to reproduce abundantly. Next is clonality, which means cells can be derived from one single cell. Lastly, potency allows cells to differentiate into a variety of cell types (Lin and Talbot, 2014).
Stem cells can be classified according to their differentiation ability into five groups, namely totipotent, pluripotent, multipotent, oligopotent, and unipotent (Łos et al., 2019). Figure 1 summarizes the classification of stem cells according to the differential potential. Totipotent cell can differentiate into any type of cell and develop into a complete organism. In totipotency, a single cell can produce an entire embryo and its extraembryonic components (Posfai et al., 2021). Pluripotent cells can differentiate into three germ layers, mesoderm, endoderm, and ectoderm, as well as into any type of embryonic and adult cells. Multipotent cells consist of progenitor cells that can differentiate into a limited number of cell types. Oligopotency are cells within a tissue that can differentiate into cells of a specific tissue. Unipotent cells can differentiate into cells of the same type (Loya, 2014; Łos et al., 2019).
FIGURE 1. Schematic representation of the differentiation-based stem cells based on differentiation. Created by Biorender.com.
Stem cells can be classified according to their origin, such as embryonic stem cells (ESC), adult stem cells, and fetal stem cells (Ilic and Polak, 2011; Kolios and Moodley, 2013). ESC is pluripotent and is derived from the inner cell mass of the blastocyst, which is the preimplantation embryo 5–6 days after fertilization (Kolios and Moodley, 2013). Although these cells can differentiate into three primary germ layers, they can remain undifferentiated in culture for a long time. Blastocytes have two layers of cells, the inner mass of cells that form the embryo and the outer mass of cells that form the placenta called the trophoblast (Ilic and Polak, 2011). To develop the ESC line, cells obtained from the inner cell are separated from the trophoblast and transferred to culture dish under specific conditions.
Adult stem cells, also known as tissue-specific stem cells, are multipotent cells that are responsible for replacing dead cells in the body. These cells are present in many adult tissues such as bone marrow, gastrointestinal tract, the brain, skin, and nerve cells. They are usually tissue-specific and can only differentiate into cell types where the tissues are located. Adult stem cells have a unique ability to differentiate, so that one type of stem cell can differentiate into another type of tissue under appropriate growth conditions (Loya, 2014).
Fetal stem cells are pluripotent and present in the fetus, which contributes to the early development of all prenatal tissues. They are obtained from fetal tissues or extraembryonic tissues such as umbilical cord blood, amniotic fluid, Wharton’s jelly, and the placenta. These cells have to self-replication and differentiation ability (Loya, 2014).
The central objective of this review is to highlight the therapeutic potential of MSCs and iPSCs in current clinical studies including COVID-19. In addition, animal models used to study the treatment of certain diseases are discussed in more detail. The characteristics, differences, advantages and disadvantages, main concerned issues, mechanism of action between MSCs and iPSCs, and their therapeutic applications in the disease model are also summarized in Figure 2.
FIGURE 2. Summary of mesenchymal stem cells and induced pluripotent stem cells. Created by Biorender.com.
Mesenchymal stem cells (MSCs) are multipotent adult stromal progenitor cells that are heterogeneous, non-hematopoietic, can self-renew and transform into several lineages and cell types (Rastegar et al., 2010). MSCs can be obtained and isolated from a variety of tissues, such as bone marrow, adipose tissue, skin, umbilical cord blood, amniotic fluid, and placenta (Fernandez and Fernandez, 2016). Bone marrow is the main source of MSCs, although MSCs account for only a small proportion of the total number of cells in the bone marrow (Rastegar et al., 2010; Al-Anazi, 2020). MSCs are distinguished by three characteristics, including the ability to differentiate into osteoblasts, adipocytes, and chondrocytes. Second, MSCs should adhere to plastic while maintaining a standard culture condition in a tissue culture flask. The third characteristic is the presence of specific surface markers such as CD105, CD73, and CD90 when measured with flow cytometry (Dominici et al., 2006). Figure 3 shows the schematic diagram of MSC-based cell therapy.
FIGURE 3. Schematic diagram of MSC-based cell therapy. Created by Biorender.com.
In addition to isolation from tissues, MSCs have been generated by differentiation of ESCs and iPSCs. There are three methods involved in the differentiation of ESCs. An embryoid body method (EB) involves growing embryonic stem cells into three-dimensional structures that can differentiate later into ectodermal, mesodermal, and endodermal cells. However, such lineages are often not uniform since they contain multiple cell lines that are present simultaneously. Another method is to use feeder cells to directly differentiate ESCs into specific lineages. For example, OP9 cells are often used to differentiate ESCs into mesodermal cells such as hematopoietic cells (Hematti, 2011a). Initially, MSCs were derived from ESCs using OP9 cells (Trivedi and Hematti, 2007), but later a method was developed to generate MSCs from human ESCs that do not utilize OP9 or feeder cells (Trivedi and Hematti, 2008). An extracellular matrix (ECM) such as Matrigel, collagen, and gelatin is also used for the two-dimensional differentiation culture method along with cytokines and growth factors that are added to the environment (Hematti, 2011b). OP9 had strong clonogenic ability and could induce osteocytes, chondrocytes, and adipocytes. As OP9 cells were genuine MSCs, such a homogeneous cell line will help define the biological characteristics of MSCs at the stem cell level. The MSC-produced ECM largely replicates the composition and structure, thus mimicking the components of stem cell niche components in vitro and maintaining multipotent progenitor cells.
In this study, a one-step method for the generation of from ESC/iPSC derived MSCs was developed, which exhibited typical MSC characteristics as defined by the International Society for Cell Therapy. In culture, these ES-MSCs and iPS-MSCs have normal karyotypes, lack the ability to form teratomas, and show growth and differentiation characteristics similar to those of primary MSCs. This simple EB-free differentiation protocol can be scaled up to allow a wide range of MSC-based therapies due to the ease of harvesting iPSCs and their ability to be grown indefinitely (Y. S. Chen et al., 2012).
In serum-free medium containing the transforming growth factor B pathway inhibitor SB431542, epithelial-like monolayer cells were generated from ESC/iPSC. SB431542 is believed to induce hESC differentiation by inhibiting SMAD2/3 phosphorylation by suppressing activin receptor-like kinase (ALK) receptors 4, 5, and 7. SB431542 inhibits SMAD2/3 binding to a SMAD-responsive element at the NANOG promoter that otherwise maintains OCT4, SOX2, and NANOG expression in pluripotent cells. Using a small molecule-based method, we can generate MSC-like cells in 20 days compared to 30 days in our EB-based protocol and 40 + days with other monolayer-based protocols that require 30 days of differentiation (Boyd et al., 2009). With this method, MSCs can be generated in a rapid and complete manner without immortalization, co-culture with mouse MSCs, epitope selection, or physical selection procedures. In contrast to undifferentiated iPSCs and ESCs, iPS and ES-MSCs did not dependent on attachment factors such as gelatin, fibronectin, or Matrigel, and the feeder layer or growth factors. Following SB431542 treatment for 10 days, MSC genes were not highly expressed in ESCs or iPSCs, however, after one or two passages in MSC medium, cells acquired a MSC phenotype and function (Y. S. Chen et al., 2012). Therefore, SB431542 offers a promising strategy to differentiate human iPSCs into MSCs for use in regenerative medicine.
The isolation techniques were divided into two categories, the explant culture method and the enzymatic culture method. Explant culture is one of the best isolation methods and in vitro cell cultivation techniques (Mushahary et al., 2018). Although explant culture increases the initial culture time, it is an inexpensive process that requires little manipulation and produces a homogeneous cell population, as only those that can be transferred from tissue to plastic will grow (Priya et al., 2014). Enzymatic culturing technique includes an additional step that involves incubation of the cut tissues in an enzyme solution that can degrade the extracellular matrix (ECM) (Mushahary et al., 2018). Enzymatic digestion allows rapid cell separation, but enzymes increase the cost of the process and produce a very high proteolytic stress that can damage the cell membrane adhesion capacity and viability (J. Hua et al., 2013). Additionally, this technique requires multiple steps that lead to an increased risk of biological contamination. Severe tissue degradation causes cultures from enzymatic digestion to become highly heterogeneous with high hematopoietic contamination that results in low growth rates and alterations in morphology (Salehinejad et al., 2012). Besides the explant and enzymatic digestion method, a separation filter device has been utilized in the bone marrow that collects the nucleated cells within a rayon/polyethylene nonwoven fabric. These isolated nucleated bone marrow cells are directly placed into the culture vessels. In the standard ex vivo expansion culture condition, after two passages, about 2-3-fold more MSCs have been generated using a filter device compared to buoyant density centrifugation. Using a filter device is more effective in generating bone marrow MSCs (Mushahary et al., 2018).
Although MSCs have been isolated by explant and enzymatic techniques, some studies have been carried out to compare the main characteristics. One of the studies examined the surface expression of CD markers such as CD34, CD44, CD73, CD105, and HLA-DR in MSCs that have been obtained by the explant and enzyme method (Priya et al., 2014). It shows few differences between the MSCs that have been isolated by both methods. There is also no difference in immunogenic and immunosuppressive responses. Furthermore, MSCs did not enhance peripheral blood mononuclear cell (PBMC) proliferation and strongly inhibit lymphocyte proliferation after mixed lymphocyte reaction (MLR) (Priya et al., 2014). Compared to the enzymatic method, the explant produced more pure and less heterogeneous cells that have a high proliferation rate (Salehinejad et al., 2012). It was suggested that the higher proliferation rate of MSCs derived via the explant method was due to a more homogeneous cell population and less enzymatic damage. High cell viability and number can be obtained through enzymatic methods (Yoon et al., 2013). Furthermore, the use of explant culture required less time compared to the enzymatic digestion method, where collagenase takes more time to break down the tissue (W. Jing et al., 2011).
In transcriptomics, the MSC transcriptome is interrogated using large gene expression-based data (GEO datasets), which reveal significant changes in expression that result from culture expansion, hypoxia preconditioning, stimulus-directed differentiation, trans-differentiation, exposure to biologics, and coculture with other cells. A genome-wide gene expression study can provide insight into the biological nature of MSCs, their expected physiological function, and their probable role in disease pathophysiology. Understanding the data on MSC gene expression can provide insight into native physiological function, improve operational definitions of MSCs, and inform how culture conditions and clinical manufacturing protocols can best characterize their composition and function before they are administered to patients (Pittenger et al., 2019). The original purpose of MSC gene expression studies was to establish a shared identity for bone marrow-derived MSCs (BM-MSCs) in vitro. Serial gene expression (SAGE) was used to analyze the transcriptome of human and mouse BM-MSCs to accomplish this goal. These catalogued transcripts were found to reflect their stem/progenitor properties, as well as paracrine functions related to support and skeletal homeostasis (Tremain et al., 2001). MSCs are widely used for clinical therapies because gene expression data confirm their skeletogenic, angiogenic, anti-inflammatory, and immunomodulatory activities. Studies with RNA sequencing have provided more information on how MSCs respond to differentiation-inducing stimuli at the cellular level (Pittenger et al., 2019). A study of MSC differentiation into an adipogenic or osteogenic line found significant changes in their transcriptomes. ChIP-Seq studies showed that osteoblasts derived from MSCs resembled more closely naive cultured MSCs in terms of epigenome (Meyer et al., 2016).
Before the generation of induced pluripotent stem cells (iPSCs), embryonic stem cells (ESCs) were the only source of pluripotent stem cells that had been studied. Generation of induced pluripotent stem cells (iPSCs) by reprogramming somatic cells to an ESC state with the help of a cocktail of transcription factors was a significant advancement in stem cell research (Bhartiya et al., 2013). ESCs are extracted from the inner cell mass. In 2006, Takahashi and Yamanaka reprogrammed the mouse fibroblast using the retroviral transduction method using 24 genes (Takahashi and Yamanaka, 2006). From the gene pool, four genes have been narrowed down, which are OCT4, SOX2, c-Myc, and KLF4 (Yu et al., 2007). iPSCs are usually like ESCs in morphology, expression of a gene, in vitro differentiation potential, and teratoma formation (Jung et al., 2012). iPS cells can differentiate into primary germ layers invitro, such as ectoderm, mesoderm, and endoderm (Medvedev et al., 2010).
One of the best studied pluripotency regulators is Oct4. Early embryonic cells, germ line cells, and cultured pluripotent stem cells express Oct4, which activates stem gene transcription. The Oct4 protein interacts not only with stemness factors such as Nanog and Sox2, but also with transcriptional repressors such as Polycomb Group proteins (Wang and Dai, 2010). Oct4 is coactivated by Sox2, which is a transcription factor (Chew et al., 2005). Binding of Oct4/Sox2 dimers to Oct4 and Nanog promoter sequences leads to upregulation of their transcription. Nanog is a homeobox-containing transcription factor that stabilizes the stem network (Masui et al., 2007). A zinc finger-containing transcription factor, KLF4, regulates the expression of Oct4, Sox2, and Nanog (Chambers et al., 2003). When Klf4 is overexpressed in ES cells, Oct4 is also induced, further increasing the self-renewal capacity (Y. Li et al., 2005). c-Myc facilitates reprogramming with greater efficiency and speed (Nakagawa et al., 2010). Post-transcriptional expression of Oct4 is stimulated by LIN28 through direct interaction with its mRNA (Qiu et al., 2010). Human protein Glis1 has been recognized as a replacement for c-Myc in recent years (Maekawa et al., 2011). A GLIS1 transactivator activates Wnt ligands, such as Lin28a, Nanog, Mycn, Mycl1, and Foxa2. Figure 4 shows the schematic diagram of iPSC-based cell therapy.
FIGURE 4. Schematic diagram of iPSC-based cell therapy. Created by Biorender.com.
Cell reprogramming efficiency remains low, therefore reprogramming techniques are being intensively researched to produce iPSCs, enhancing the efficiency, process, quality, and safety of cells (Belviso et al., 2020). In general, the enhancement focuses on several elements of the reprogramming method, which are the sources of somatic cells, the reprogramming factor cocktail (Al-Anazi, 2020), culturing and maintaining conditions for iPSCs and most importantly, the method for introducing the reprogramming factors.
A major concern associated with the generation of iPSCs is the use of retrovirus as a reprogramming method to achieve the incorporation of reprogramming factors into the host cell genome. This may lead to the formation of teratomas as a result of oncogene expression such as c-Myc and KLF4 (Riggs et al., 2013). Now, different types of methods are being studied to induce the expression of reprogramming factors. The methods are classified into two major components which are reprogramming via integrating or non-integrating systems using either viral or non-viral methods (Telpalo-Carpio et al., 2013).
Several types of integrated viral vectors, such as retrovirus and lentivirus, have been widely used in the generation of iPSC cells. Figure 5 shows the different methods of integrating and non-integrating viral vectors in cell reprogramming. Retroviral vectors were the first type of vector to be used to generate iPSCs, and the site of viral incorporation has been extensively researched (Han and Yoon, 2011). The Moloney murine leukemia virus (MMLV) was the first retrovirus that was used to deliver specific transcription factors to human and mouse fibroblasts. MMLV has the ability to infect actively dividing cells and remains silent in immature cells like ESCs (Han and Yoon, 2011). Retroviruses are more likely to cause malignant formation when they integrate near transcription sites.
FIGURE 5. Different methods of integrating and non-integrating viral vectors are used in cell reprogramming to generate iPSCs. Created by Biorender.com.
Compared to fibroblasts, human ectodermal keratinocytes can be reprogrammed to pluripotency at least 100 times more efficiently and two-fold faster (Aasen et al., 2008). As a result, keratinocyte-derived iPS (KiPS) cells are less likely to integrate retroviruses than fibroblast-derived iPS cells, highlighting the importance of intrinsic differences between these two types of somatic cells. The presence of keratinocyte stem cells may contribute to a higher efficiency of keratinocyte reprogramming (Fuchs, 2007). Keratinocytes are more similar to hES cells and KiPS cells than fibroblasts are to hES cells and KiPS cells based on the expression of stem cell-related genes. CD24, a putative stem cell marker, was also detected in primary keratinocytes but not in fibroblasts by flow cytometry (Aasen et al., 2008).
In addition to retrovirus, the lentiviral vector (LV) is known to be more suitable due to its broad tropism. LVs are used to reprogram a wide range of somatic cells such as mice, rats, and humans (Al Abbar et al., 2020). HIV is the source of the most common lentivirus used as a delivery vector. Lentiviruses have higher cloning capacity and infection efficiency compared to retroviruses. LVs can replicate in both dividing and non-dividing cells, unlike MMLV-based. Lentivirus and retrovirus vectors carry a severe risk of insertion mutagenesis during transfection due to their genomic integration. These vectors can have a high risk of tumorigenicity when reactivated during the differentiation of iPSCs even though they have been silenced (Belviso et al., 2020). In integrating viral vectors, lentiviral is known to be better than retroviral vector. In a study researchers found lentiviral vectors did not cause higher tumor incidence or earlier tumor onset in mice compared to retroviral vectors (Montini et al., 2006). Lentivectors can overcome the limitations of retroviruses, such as their inability to transduce quiescent cells (Lewis and Emerman, 1994). As a result of their development from complex retroviruses, lentiviral vectors have several advantages over simple retroviral vectors, including higher virion stability and titer, and a reduced frequency of insertional mutagenesis (Dufait et al., 2012).
Furthermore, the next method of reprogramming involves non-integrating viral vectors such as Adenoviruses and Sendai viruses. Human and mouse iPSCs have been successfully generated using adenovirus (W. Zhou and Freed, 2009). The iPSCs showed no evidence of insertion of exogenous DNA insertion into the host genome. Human iPSCs produced from adenovirus have been shown to be pluripotent and capable of differentiation into three germ layers (Stadtfeld et al., 2008). Another study uses adenoviral vectors expressing c-Myc, KLF4, OCT4, and SOX2 to produce iPSCs from human embryonic fibroblasts, and the resulting iPSCs express ESC-specific markers and undergo significant differentiation. It showed the ability, and viral or transgenic integration was absent (W. Zhou and Freed, 2009). However, this method eliminate the risk of malignant transformation associated with retroviruses or lentiviruses and reduces the efficiency of repeated transduction to maintain an appropriate level of transgene expression. One of the factors is the time taken for stem cells to be cultured prior to transduction, where a longer period may show altered transduction of the AAV vector compared to stem cells cultured for a short period of time. Furthermore, the number of vector genomes per cell used to transduce stem cells is inconsistent. As for Sendai virus, the replication cycle is localized in the cytoplasm and it only takes a shorter time for the virus to be taken up by the cells.
An alternative to adenovirus, Sendai virus (SeV) can be used where it is an RNA virus that could infect a variety of proliferating or inactive cell and does not penetrate the host cell nucleus (Fusaki et al., 2009). They have a low risk of genomic insertion and are used to reprogram neonatal and adult fibroblasts, which makes them suitable for vehicle Yamanaka factors (Takahashi and Yamanaka, 2006). After infection, the virus replicates in the cytoplasm, and it can be washed out of the host cells through several passages. Using the Sendai virus in reprogramming can generate iPSCs without altering the genome. Sendai virus vectors are deficient in replication and their copy becomes diluted after cell division, resulting in obtaining virus-free iPSCs after 10 passages (Belviso et al., 2020). As for adenovirus, they are highly transducible, express transgenes efficiently, and have a wide range of viral tropisms. It is possible for them to infect both dividing and nondividing cells (Ura et al., 2014). There are several unique characteristics of SeV that make it suitable for immunotherapy, including the fact that its replication cycle is localized in the cytoplasm and that it takes only a short time for the virus to be taken up by the cells (Hosoya et al., 2008). SeV has a low transgene capacity compared to other viral vectors, which limits its use.
Additionally, peripheral blood contains terminally differentiated circulating T cells (hTDCTC). It was demonstrated that hiPSCs can be generated easily, efficiently, and safely within a 1-month time frame by combining activated T cell culture with temperature-sensitive mutated Sendai virus (SeV) (Seki et al., 2010). The SeV vector was used for the generation in order to prevent transgene integration (H. O. Li et al., 2000). To reduce the expression and SeV residue in the generated lines, a mutated SeV vector was used, which was temperature sensitive. At standard culture temperatures, this type of SeV vector produces weaker transgene expression and cannot proliferate. SeV has been shown to efficiently transduce and express exogenous genes in human T cells (Okano et al., 2003). Using SeV, multiple transcription factors were delivered to cells to generate iPSC from hTDCTCs, including OCT3/4, SOX2, KLF4, and c-MYC.
Generation of iPS cells using Sendai virus was carried out using two different conditions, feeder-free condition and xeno-free condition. A feeder-free condition, using Matrigel and a defined culture medium instead of a feeder layer, minimizes exposure risks from unknown exogenous factors (Kishino et al., 2015). It is possible for the xenogeneic and chemically undefined components of culture systems to pose pathogenic risks and cause immune rejection (Y. Hua et al., 2022). Matrigel adhesive proteins have become the focus of research, along with recombinant ECM proteins synthesized from human cells. During embryogenesis, laminin is a key ECM protein, and laminin-based substrates are more effective than Matrigel in adhesion, survival, and self-renewal of hiPSCs (Miyazaki et al., 2012). Gelatin nanofibers (GNFs) have recently been developed as a low-attachment substrate, similar to Matrigel for the long-term expansion of hiPSCs (L. Liu et al., 2014). In the presence of AscleStem PSC medium, ON2, GNF fully maintained hiPSCs over 30 passages without any morphological or chromosomal abnormalities. GNF-grown hiPSCs showed a higher proliferation rate (Y. Hua et al., 2022). There are several possible reasons for the spontaneous differentiation of iPS cells in culture. The main cause is the over-confluency of cultures. Therefore, colonies must be passed before reaching full confluence to avoid differentiation. The second problem is the poor quality of the ECM coating. This can be prevented by performing quality control tests on each batch of coated vessels before they are allowed to proceed with production or adding more coating solution might reduce the risk of coating failure. For all pluripotent stem cell cultures, oxygen tension should be adjusted to 3%–5% O2. When cell cultures are kept outside of an incubator for prolonged periods of time, they can be exposed to high fluctuations in temperature. It is also recommended to add prewarmed reagents into culture, but to prevent growth factors from losing their activity, prolonged exposure to 37°C should be limited. Differentiated cells can be removed during dissociation with EDTA-based dissociation reagents, since iPSCs will be harvested preferentially, while differentiated cells will remain attached to the current culture surface (Rivera et al., 2020).
In addition to using viral vectors, non-viral vectors are alternatives for reprogramming to produce iPSCs. Due to the limitations in integrating viral vectors, non-viral vectors are actively used that are safer for therapeutic approaches. Figure 6 shows the different methods of integrating and non-integrating non-viral vectors in cell reprogramming. Integrated non-viral vectors such as plasmids were used to generate iPSCs from mature embryonic fibroblast cells. Two plasmid constructs were used, where one plasmid encoded for c-Myc and another polycistronic vector ended the four reprogramming factors (Han and Yoon, 2011; Al Abbar et al., 2020). Furthermore, it is extremely efficient to deliver exogenous pluripotency genes using a mobile genetic element such as PiggyBac (PB) transposons. The most important and distinctive feature of this approach is that transient transposase expression can completely remove the remnants of this element from reprogrammed cells (Han and Yoon, 2011). However, the PB-like transposon elements may present in the human genome and contain endogenous, which may result in nonspecific genomic alterations following transgene excision. Using this plasmid-based protocol, plasmid-iPS cells are established at a lower rate than those induced by viruses (Okita et al., 2008). Therefore, PiggyBac transposons can be an alternative in the host genome, they can be eliminated without leaving a “footprint” of mutations. Using piggyBac transposons, it is possible to permanently eliminate reprogramming factors from iPSCs without affecting their genetic makeup (Yusa et al., 2009).
FIGURE 6. Different methods involved in reprogramming using integrating and non-integrating non-viral vectors. Created by Biorender.com.
The last method used in reprogramming involves non-integrating non-viral vectors such as episomal vectors, mRNAs, minicircle vectors, recombinant proteins, microRNAs, and exosomes. These methods have been developed to generate iPSCs that are completely free of viral contamination. The use of non-replicating or replicating episomal vectors was one of the first integration-free cell reprogramming techniques. This method is very simple and does not require any manpower to perform the experiment. Furthermore, this method has a low transfection power, which requires multiple transfections to achieve a stable expression level of the desired genes. Episomes work best in reprogramming blood cells, but to generate iPSCs from fibroblasts, they need several modifications in a standard culture condition (Okita et al., 2008).
Minicircle vectors are alternatives to episomal vectors that have higher transfection efficiency. Minicircle coding genes are highly efficient in both dividing and nondividing cells and they result in a high level of desired protein expression due to being less likely to be silenced and inactivated by a cellular mechanism that targets foreign nucleic acids (Jia et al., 2010). Due to its low activation of exogenous silencing mechanisms, minicircle DNA can offer higher transfection efficiency and longer ectopic expression, making it ideal for generating iPS cells (Z.-Y. Chen et al., 2005). To examine the reprogramming capacity of the minicircle vector, we induced pluripotency in human adipose stem cells (hASCs). Compared to other types of somatic cells such as fibroblasts, hASCs can be isolated in large quantities with minimal morbidity (Fraser et al., 2006). Photon counts and qPCR confirm that minicircle DNA with the same reporter gene maintains higher expression in hASCs for a longer time than standard plasmids (Jia et al., 2010). Despite the differences in donor cell types (neonatal fibroblasts vs. hASCs) and reprogramming factors used, minicircle DNA still had higher efficiency than previous plasmid-based transfection reprogramming methods (Yu et al., 2009).
Furthermore, the use of vectors, synthetic RNA, or messenger RNA (mRNA) encoding reprogramming factors could provide a significant opportunity for the generation of integrase-free pluripotent cells. Although it requires multiple sets of transfections, this method is suitable for producing iPSCs with improved safety profiles (Warren et al., 2010). In addition, reprosomes are exosomes that contain a cocktail of reprogramming factors for a specific purpose. To generate iPSCs, exosomes appear to be suitable for clinical application, as they only need fewer procedures and carry a lower risk of tumor formation and mutation.
Mesenchymal stem cells have become the most widely used types of stem cell in therapeutic applications because of their multiple advantages. The first advantage of MSCs is being easily accessible. This can be proved by obtaining MSCs from different sources of the human body. For example, MSCs can be obtained from bone marrow, adipose tissue, human umbilical cord, amniotic fluid, dental pulp, and skin. The techniques used to isolate MSCs are minimally invasive and do not damage the patient’s organs.
Second, MSCs can be produced on larger scales, which can be used for clinical purposes. Producing therapeutic cells with good manufacturing practice (GMP) requires a scalable and controllable bioprocess that can be run in a closed system. The most widely used cell culture bioreactor on a laboratory scale, especially for anchorage-dependent cells, is the T-flask with several surface areas such as 25, 75, 150, and 225 cm2. T-flasks are simple to use, inexpensive, and disposable. Various types of bioreactors have been developed and utilized to scale up the expansion of hMSCs, which are multilayered cell factories, roller bottles, fixed bed bioreactors, and hollow fiber (Jung et al., 2012).
The third advantage is that MSCs have multilineage differentiation potential. MSCs can differentiate into other cell types such as chondrocytes, osteoblasts, myocytes, adipocytes, and neurons. The next advantage is that it has pleiotropic effects such as trophic properties, anti-inflammatory and immunomodulatory properties, antiapoptotic properties, and antimicrobial properties (Merimi et al., 2021). Lastly, MSCs have been widely used in clinical applications such as the treatment of neurodegenerative diseases (Boika et al., 2020), skin problems (Shin et al., 2017), diabetes wound healing (Nasiri et al., 2019), liver disorders (X. Yang et al., 2020), and cardiac ischemia (Quevedo et al., 2009; Poomani et al., 2022). Table 1 summarizes the advantages and disadvantages of MSCs.
In addition to these advantages, MSCs also have several drawbacks in which they experience replicative senescence, which is a senescence-related growth (Kassem et al., 1997). Replicative senescence is a common feature of the growth of diploid cells in vitro, limiting their ability to generate the large number of cells that require therapy. A factor that contributes to replicative senescence in MSC cells is the persistent shortening of telomeres after continuous culturing in vitro due to a lack of telomerase activity (Stenderup et al., 2003). This can be overcome by forced expression of the human telomerase reverse transcriptase gene (hTERT) in cultured human MSCs that can reverse the senescence phenotype and thus restore telomerase activity (Estrada et al., 2013).
Furthermore, after stem cell transplantation, the chance of getting cancer is higher. Due to their proliferative abilities, high survival rates, and resistance to apoptosis, stem cells can associate with tumor cells (Musiał-Wysocka et al., 2019). Patients who receive stem cell transplants frequently receive long-term chemotherapy, causing their immune system to malfunction, leading to an increase in the risk of cancer. Modification of MSCs and long-term in vitro culture can result in chromosomal aberrations and genetic instability (Barkholt et al., 2013).
In addition, tumor growth is aided by MSCs. Excessive production of cytokines such as chemokines and growth factors acts directly on the receptors on the surface of cancer cells, which controls tumor growth. The ability of MSCs to suppress the immune system contributes to the growth and spread of tumor cells (Karnoub et al., 2007).
iPSCs are well established and developed, as they have several advantages in that cell sources are easily accessible for reprogramming. iPSCs can be produced by various types of somatic cells such as fibroblasts, myoblasts, keratinocytes, hepatocytes, and adult stem cells. At first, mouse embryonic or adult fibroblasts were used to produce embryonic-like pluripotent stem cells using reprogramming factors (Takahashi and Yamanaka, 2006). The use of fibroblasts as a source to produce iPSC has the advantages of being low cost, easy to use, and well established in various fields of research. Human fibroblasts can be obtained from numerous organs and tissues in the body, such as the dermal, cardiac, and lungs. Isogenic disease modelling with iPSCs can be done through two strategies. The first strategy involves repairing a pre-existing, suspected causative allele in iPSCs taken from patients who have the disease. It establishes whether this particular genetic change results in a disease phenotype, but it does not provide information on whether it is sufficient to cause the disease. In addition, patient-derived cell lines will be expected to express whatever cellular or molecular phenotypes are responsible for causing the disease, and therefore reversion of those phenotypes in the edited line can be used as an indication of the disease’s etiology. The second strategy involves taking an iPSC line from a healthy individual and then introducing a lesion that might be of significance. Using this method, we can determine whether the genetic mutation causes the disease phenotype, as it removes it from the genetic background of the diseased person (Bassett, 2017).
Next, patient and disease-specific human cells are now readily available due to recent developments in iPSCs technology (Gomes et al., 2020). Patient-specific iPSCs have been developed as a resource for drug testing, toxicity, cell replacement treatment, and disease modelling (Chun et al., 2011). For example, liver transplantation is the only therapy option for people with end-stage liver disease. Hepatocyte or hepatocyte-like cell transplantation could be a viable alternative to liver transplantation in cases of acute liver failure. Adult human hepatocytes ex vivo is a good choice for cellular treatment and drug testing.
In addition, human-induced pluripotent stem cells (hiPSCs) can be made from somatic cells instead of human embryos, avoiding the ethical issues associated with embryo destruction. Informed consent, health, and safety concerns of donating eggs to produce embryos by in vitro fertilization also influence the use of ESC (Singh et al., 2015). Since iPSCs are made from the patient’s own body somatic cells, there will be no immunorejection (Z. Jiang et al., 2014). One of those studies showed that iPSCs have been differentiated into embryoid bodies (EB). There was no evidence of increased T cell proliferation or antigen-specific secondary immunological responses after transplantation of EB derived from iPSCs (Guha et al., 2013). Furthermore, iPSCs can reduce the overall cost of clinical trials. This is because around 5,000–10,000 chemicals must be examined in preclinical trials for each drug that reaches to the market. Using iPSCs to provide toxicity information about drugs through several cytotoxicity assays could minimize the cost of clinical studies. Animal models in clinical trials do not fully match the milieu of human cells, where the use of iPSCs reduces the expenditures involved with animal models (Singh et al., 2015).
There are also some drawbacks that need more attention when generating iPSCs. Table 2 summarizes the advantages and disadvantages of generating iPSCs. First, reprogramming methods can cause tumorigenesis (Bhartiya et al., 2013). Because iPSCs are produced through retroviral or lentiviral vectors, the viral system might integrate with the host DNA. The genetic material introduced through retroviral may randomly integrate into the host genome, which can cause genetic abnormalities and the formation of teratomas (Singh et al., 2015).
The next drawback is quality assessment and variability. In iPSC research, evaluation tools for evaluation are essential for the reprogramming method and the final quality of cells. There must be some experiments to ensure that all pluripotent cells have developed and are not genetically altered during the reprogramming process. When cells are derived from sick patients for autologous treatment, there is always a concern that the disease underlying mechanism may remain in the generated iPSCs and express itself in the same way. Analyzing this variation could help to determine possible somatic tissues for the production of iPSC (Singh et al., 2015). Although genome editing is extremely effective in reducing variability between patients, the process itself can introduce artefacts from both off-target mutations and clonal variability. Controlling the variability introduced by genome editing can be achieved by reintroducing disease mutations in genetically corrected patients. The introduction of mutations into a consistent WT line can also reduce the variability between individuals and during the iPSC derivation process (Bassett, 2017).
In iPSC, epigenetic memory may not necessarily influence gene expression patterns due to missing transcription factors, but is expected to affect differentiation capacity (Cahan and Daley, 2013). iPSCs might be limited in some applications, but they may also be prone to enhanced differentiation into the parental cell type, thus enabling them to generate specific cells. According to Kim et al., iPSCs derived from adult umbilical cord blood and neonatal keratinocytes have different DNA methylation profiles and differentiation potentials (K. Kim et al., 2011). iPSCs derived from each cell type showed different DNA methylation patterns, resulting in incomplete erasure of tissue-specific methylation and aberrant denovo methylation. Extensive passages did not eradicate these differences (K. Kim et al., 2011).
Another significant issue is the set of genes used to generate iPSCs. Because the expression of the OCT4, SOX2, KLF4, and c-Myc genes is related to the development of many malignancies. Ectopic transcription of these genes can lead to neoplastic growth of cells produced from iPSCs. Overexpression of OCT4 induces murine epithelial cell dysplasia, while expression of SOX2 causes serrated polyps and mucinous colon carcinomas (Hochedlinger et al., 2005; Park et al., 2008). Excess KLF4 expression can be found in breast tumors and c-Myc overexpression is found in 70% of human cancers (Ghaleb et al., 2005; Kuttler and Mai, 2006). Tumor development has been observed by injecting retroviral system-generated iPSCs into blastocytes, most likely due to reactivation of c-Myc.
There are some concerns that should be considered when using MSCs for therapeutic purposes, such as the way MSCs are administered inside the body. In the case of tissue defects, local implantation with direct application to the wounded area is very desirable. However, because most MSCs target systemic diseases, intravenous injection of MSCs is essential. Therefore, this is related to concerns about cell biodistribution and the number of cells that reach the target wound site. Most intravenously delivered MSCs are believed to remain in the lungs, they are the first organ to be encountered before other tissues (Mastrolia et al., 2019).
Second, there is not enough clinical evidence to support the long-term safety of MSCs. Although the use of MSCs is believed to be safe, the long-term safety of the procedure is still unknown. Large-scale, controlled, and double-blind clinical trials are needed to assess cell safety before MSC transplantation becomes a common therapeutic option (Si et al., 2011).
In addition to that, MSCs have a limited expansion rate. Theoretically, MSCs can be grown in vitro in a standard culture flask of any size essential for the experiment. MSCs, on the other hand, hit the Hayflick limit, where a phenomenon cell can divide several times before it reaches cell death or apoptosis. After a lengthy culture period and an increase in passage numbers, the result is a significant decrease in proliferation and morphological transition from a thin spindle shape to a flattened square shape (T. Zhao et al., 2019). The properties of MSCs are also influenced by the larger-scale growth in 2D plates over a longer time. Multiple passages have caused mutation spectra to function and cells at high passages had a reduced therapeutic impact in an animal model (Q. Zhao et al., 2019).
However, the generation of iPSCs for therapeutic use also shows some concern that needs attention. The first concern is genetic material and confidential personal information (Moradi et al., 2019). iPSCs formed from any individual carry a tremendous amount of private information (DNA) that, if misused, may violate the law and privacy of individuals. Although the donor of the cell is dead, iPSCs still contain the information of its close relatives, which can be an ethical and legal concern about personal privacy. Currently, with the development of human genome sequencing data, genetic information can determine close relatives (Lin et al., 2004).
Second, it is necessary to obtain informed and voluntary consent from participants whenever an individual or their cells are used in the research (Jefford and Moore, 2008). The type of informed consent form used and the information contained inside is crucial. If patient-derived iPSCs are only used for laboratory research, they must be included in the consent form and notified to the cell donor. Researchers must take responsibility for explaining all research purposes and then discuss the possible adverse effects of treatment in patients (Moradi et al., 2019).
The next concern is the possibility of the potential tumorigenicity of undifferentiated iPSCs in the cell population. It is possible for iPSC transplanted in an undifferentiated condition to develop teratomas, as well as malignant tumors such as neuroblastoma and follicular carcinoma (Okita et al., 2008). As a result, if transplanted cells are contaminated with undifferentiated iPSC, there will be a possible risk of tumorigenicity to patient health. Different methods such as purification using Fluorescence-activated cell sorting (FACS)/magnetic bead-based sorting (MACS) have been identified to eliminate the potential risk of tumorigenicity (Sutermaster and Darling, 2019). Monoclonal antibody (mAb) raised against hESCs, designated SSEA-5, which binds to the glycan H -1 expressed at higher levels in hPSCs (Tang et al., 2011). A significant reduction in teratoma formation was observed when high cells were separated by fluorescence-activated cell sorting (FACS). In partially differentiated cultures, depletion with SSEA-5 alone greatly reduced teratoma-initiating activity (Tang et al., 2011). The only way to achieve complete removal was to combine SSEA-5 with two additional pluripotent surface markers (PSM), such as (SSEA-5, CD9, CD90 and SSEA-5, CD50, CD200).
Paracrine activity, which secretes a range of soluble molecules to exert immunomodulatory, angiogenic, antiapoptotic, and antioxidative effects, is the main mechanism behind MSC-based therapy. MSCs can adjust their immunosuppressive effect and enhance cell survival through cell-to-cell interaction (Liang et al., 2014). MSCs can be used in allogeneic transplants because of their minimal immunogenicity. MCS secrete transforming growth factor-β (TGF-β), hepatocyte growth factor (HGF), inducible indoleamine 2,3-dioxygenase (IDO), class I human leukocyte antigen (HLA) -G5, prostaglandin E2 (PGE2), interleukin (IL) -6, IL-10 and TNFα-stimulated gene protein (TSG)-6 to inhibit CD4+ cells CD8+ T cells, and dendritic cell maturation and suppress plasma cell immunoglobulin production (Fan et al., 2020). In a study, MSCs were found to decrease lymphocyte proliferation, modify lymphocyte response to allogenic target cells, and prolong skin graft survival in MHC-mismatched baboons after being delivered intravenously (Bartholomew et al., 2002). Another study showed that soluble substances produced by MSCs play an immunomodulatory role in a coculture of MSCs and T-lymphocytes in a Transwell method that eliminates cell-to-cell contact. The addition of monoclonal antibodies such as TGF-β and HGF inhibits T cell proliferation (Di Nicola et al., 2002; Liang et al., 2014).
MSCs offer novel and interesting ways to improve bone regeneration, where bone is one of the tissues in the body that can generate without leaving scars. Following bone injury, a complicated bone healing process occurs to restore bone structure and function. Locally and systemically infused MSCs traveling to the damage site at earlier stages of bone fracture repair were attracted by strong chemokines that were generated at the fracture site. The ligand of the chemokine C-X-C motif ligand 12 (CXCL12) has been found to be upregulated in this setting, stimulating MSC migration that expresses the chemokine receptor 4 (Yellowley, 2013). In an experimental femoral bone defect model, adenoviral-induced adipose tissue grafts that express CXCL12 and bone morphogenic protein 2 (BMP-2) have been used at the damage sites. The MSCs were then administered systemically after 24 h and migrated for 42 days at various intervals, and a significant increase in bone volume fraction and bone healing was found compared to the negative control. CXCL12 and BMP2 increased the recruitment of MSCs to the injury site, and osteoblasts were found to outnumber osteoclasts, indicating a proclivity to improve bone remodeling (Zwingenberger et al., 2014).
On the other hand, Alzheimer’s disease (AD) is a progressive neurodegenerative brain disease marked by memory loss and cognitive impairment. Due to the immense potential of MSCs, such as differentiation potential, immunoregulatory function, and lack of immunological rejection, MSC transplantation is becoming a popular therapy in AD. Primary immune cells in the brain, astrocytes, and microglia play a key role in neuroinflammation. The effect of hUCB-MSCs on reducing Aβ accumulation is probably due to immunomodulatory inhibition of β-site APP-cleaving enzyme 1 (BACE1) at the site. Placenta-derived MSCs (PDMSCs) reduce BACE1 expression and γ-secretase activity and improve cognitive impairment in an Aβ1-42-infused mouse model (Yun et al., 2013). hUCB-MSC transplantation in APP/PS1 double transgenic mice significantly reduced the levels of Aβ, BACE1, and tau hyperphosphorylation levels and improved spatial learning and memory impairment by targeting anti-inflammatory cytokines (IL-4, IL -10, TGF- β) and down-regulation of pro-inflammatory cytokines (IL-1, TNF- α) through activation of microglia (Lee et al., 2012). MSC therapy increased IL-4 production, which may lead microglia to produce insulin-like growth factor (IGF)-1 (Butovsky et al., 2006), reducing Aβ toxicity and enhancing Aβ phagocytosis. Systemic transplantation of hUC-MSCs into APP/PS1 transgenic mice reduced interferon-γ (IFN-γ) and increased IL-10 and transforming growth factor (TGF-1) in peripheral plasma, significantly improved cognition defects and reduced Aβ plaque deposition (H. Yang et al., 2013).
Astrocytes have many chemokines and inflammatory cytokine receptors, including IL-1β and TNF-α (Morales et al., 2014). Astrocytes are critical for Aβ removal and breakdown when stimulated by IL-1β and TNF-α. MSC transplantation was found to help neonatal rats with hypoxic ischemic brain injury recover their learning and memory function by reducing reactive astrocyte growth (He et al., 2019). Based on the findings, exogenous MSCs play a restorative role in AD by decreasing astrocyte activation. When MSCs are placed in the microenvironment of wounded tissues, they can release different substances such as TGF- β and IGF-1 that cause activation (L. Zhang et al., 2020). Subsequently, activated astrocytes can remove Aβ plaque deposition and secrete TGF-β and IGF-1 as neuroprotective agents. MSCs are linked to Toll-like receptors (TLRs), which allow MSCs to secrete IL-10 and TGF- β1 (DelaRosa and Lombardo, 2010). When inflammatory cytokines released by MSCs engage TLRs, such as TLR3, they induce a comprehensive neuroprotective response by increasing the production of anti-inflammatory cytokines such as IL-9, IL-10, and IL-11 while downregulating proinflammatory cytokines such as IL-12 (Bsibsi et al., 2006).
Mesenchymal stem cells have been exposed to stresses mainly due to oxidative stress. In the context of oxidative stress, reactive oxygen, and nitrogen species (ROS and RNS) are produced unregulated and/or scavenged. In MSC differentiation, ROS are predominantly generated by mitochondrial complexes I and III and NADPH oxidase isoform NOX4 (Schröder et al., 2009). All biomolecules can be damaged by free radical accumulation, including DNA, proteins, and lipids. To maintain cellular proliferation, differentiation, and survival, ROS levels must be kept at a low level to prevent cell damage and dysfunction (Atashi et al., 2015). Initially, MSCs exhibit little ROS and high glutathione levels, but other studies indicate that they lack antioxidant activity and are much more susceptible to oxidative stress than differentiated cells (Ko et al., 2012). A high level of ROS or exogenous H2O2 can inhibit the self-renewal, differentiation, and proliferation of MSCs. However, antioxidants stimulate MSC proliferation (Zou et al., 2004).
In most studies, ROS have been found to inhibit osteogenic differentiation. The addition of exogenous H2O2 reduces osteogenic differentiation in human and murine MSCs, as well as osteoblast precursors (C.-T. Chen et al., 2008). Furthermore, the osteogenic potential is decreased in older donors’ MSCs (de Girolamo et al., 2009). Human MSCs that are induced to osteogenesis in vitro have been shown to upregulate the number of copies of mtDNA, respiratory enzyme proteins, superoxide dismutase 2 (SOD2, alias MnSOD), the consumption of catalase oxygen and antioxidant enzymes, but a decrease in ROS. Furthermore, undifferentiated MSCs produce greater amounts of glycolytic enzymes and lactate. Compared to MSC-differentiated osteoblasts, which rely more on mitochondrial oxidative metabolism for energy, MSCs use more glycolysis for energy.
An increase in ROS occurs during adipogenesis as MSCs differentiate into adipocytes. Human MSCs upregulate antioxidant enzymes such as SOD, catalase, and GPX during adipogenesis (Higuchi et al., 2013). The addition of exogenous H2O2 or ROS induces adipogenesis in human and murine MSCs and adipocyte precursor cells, supporting the idea that ROS contribute to adipogenesis (Schröder et al., 2009). Furthermore, H2O2 increases adipogenesis at higher doses, as adipogenesis is dose dependent. Consequently, N-acetylcysteine (NAC), a ROS scavenger, inhibited adipogenesis in the mouse MSC cell line 10T1/2 (Kanda et al., 2011). Furthermore, ROS generated by mitochondrial complex III are essential for activating adipogenic transcription factors. Adipogenesis increases mitochondrial biogenesis and oxygen consumption similarly to osteogenic differentiation (Wilson-Fritch et al., 2003).
Primary murine chondrocytes and the ATDC5 cell line require ROS generated by NADPH oxidases two and four for chondrogenic differentiation (K. S. Kim et al., 2010). Chondrogenesis was associated with a reduction in SOD3 levels (Nightingale et al., 2012). SOD3 is known to help inhibit ROS production in the extracellular matrix. Furthermore, ROS removal with NAC blocked the differentiation of chondrogenic cells. The increase in ROS levels stimulated chondrocyte hypertrophy, which was inhibited by NAC (Morita et al., 2007).
As with MSCs, iPSCs have effects on immunomodulation where they have greater immune privilege, they have a higher survival rate, and the graft was better following transplantation. This suggests that iPSCs have a greater advantage in allogeneic transplantation without immunological rejection. As growth factors, hormones, culture environment, and 3D structure affect pluripotent stem cells, various stress-inducible mechanisms have been discovered to increase pluripotent stem cells into specific lineages. To preserve the normal function of cells, they respond to a range of stimuli posed by the environment, known as cellular homeostasis that includes hypoxic, oxidative, thermal, mechanical, physical, and metabolic.
Hypoxia is caused by a reduction in cellular oxygen. Hypoxic stress is defined as the occurrence of a molecular event in response to hypoxia in less than 5% (Sridharan et al., 2017). When a cell is exposed to hypoxia, transcription factors known as hypoxia-inducible factors (HIFs), such as HIF-1α, HIF-2α, and HIF-1β, launch a cascade of hypoxic signaling (Sridharan et al., 2017). HIFs play a key role in pluripotency and proliferation of pluripotent stem cells. Maintaining human ESCs at low oxygen tension of 2%–5% helps reduce spontaneous differentiation, improve proliferation, and promote the expression of critical pluripotent markers (H-F. Chen et al., 2010). HIF-2α is seemed to be more prevalent than HIF-1α in signaling. HIF-2α has been shown to influence the expression of OCT4 and NANOG (Forristal et al., 2010; Petruzzelli et al., 2014).
Next, there is the thermal stress for directed differentiation. An elevated temperature greater than 40°C promotes protein denaturation and aggregation, resulting in cell toxicity and cell death (Y. E. Kim et al., 2013). Heat shock factor (HSF1) is activated in response to cell stress and increases the transcription of the gene that encodes molecular chaperons. The heat shock response (HSR) is normal in pluripotent stem cells, and it has been proven that human and mouse ESCs are resistant to high temperatures compared to differentiated cells (Prinsloo et al., 2009). Furthermore, temperature stress at 42°C was discovered to alter gene expression by activating the decommissioning of their enhancers, which is mediated by pluripotent factors such as KLF4, NANOG, OCT 4, HSF1 and AP-1 (Lyu et al., 2018). Heat shock treatment at 46°C promoted the differentiation of human ESCs through HSF1-mediated suppression of OCT4 expression. HSF1 inhibits OCT4 expression, and SAPK/JNK mediates this impact through phosphorylation (Byun et al., 2013).
In addition to that, mechanical forces have been discovered to modulate a variety of physiological processes. Cell growth, differentiation, shape changes, and cell death are all known to be regulated by reaction to mechanical forces (Weng et al., 2016). Fluid shear stress and a signal of stiffness are the two mechanical forces involved in stem cell activity and differentiation (Kaitsuka and Hakim, 2021). Pluripotent stem cells use the mechanosensitive ion channel Piezo1 and its primary cilium that regulates mechanotransduction. It was discovered that a stiff substrate stimulates focal adhesion via the activation of focal adhesion kinase (FAK) and steroid receptor coactivator (SRC). Then FAK phosphorylates and activates YAP, resulting in activation of the YAP/TAZ transcription factor and nuclear translocation, which is known to be involved in cellular mechanoresponses (Dupont et al., 2011). It was discovered that YAP binds to the promoters of pluripotent genes in pluripotent stem cells and is essential for the pluripotency of mouse ESCs.
MSCs were first used in tissue injury research due to their multipotent capability. MSCs have been studied and have found to have several properties that can help them for therapeutic purposes. Examples of diseases that have used MSCs as therapeutic potential include bronchiolitis obliterans, Alzheimer’s disease, retinal ischemia, Type 2 diabetes mellitus, schizophrenia, etc. Table 3 summarizes the list of diseases that have been studied using MSCs.
Human umbilical cord-derived mesenchymal stem cell exosomes (hucMSC-ex) have been studied for their therapeutic effect on type 2 diabetes mellitus (T2DM). T2DM is mainly caused by peripheral insulin resistance, loss of pancreatic β-cell mass, and cell malfunction. This may lead to an uncontrolled glucose level in the body. T2DM is currently treated with daily insulin injection and with drugs such as metformin and thiazolidinediones (Nyenwe et al., 2011). In this study, exosomes have some active contents that can mediate their therapeutic effect on diabetes. They hypothesized that, like hucMSC, hucMSC-ex could reduce hyperglycemia in T2DM. This study tested the feasibility and effectiveness of using hucMSC-ex to reduce T2DM in a rat model. This rat model has been induced by a high-fat diet (HFD) and streptozotocin (Reed et al., 2000). The result suggests that intravenous injection of hucMSC-ex can lower the blood glucose level in rats with T2DM (Sun et al., 2018). This is due to the improved insulin sensitivity in peripheral organs and decreased islet destruction. In summary, hucMSC-ex has been found to successfully treat hyperglycemia in HFD/STZ-induced T2DM rats by improving insulin sensitivity, increasing glucose uptake, and metabolism in peripheral tissues.
Furthermore, in T2DM models induced by high-fat diet (HFD) and streptozotocin (STZ) administration, human umbilical cord MSCs (UCMSC) and Wharton jelly MSCs (WJ-MSC) have been shown to improve islet cell function (J. Chen et al., 2021). It also helps restore the islet structure and prevents pancreatic cell hypertrophy and cell death. As a result, the blood level was normalized and enhanced glucose levels and insulin levels. Systemic transplantation of human adipose-derived MSCs (ADMSCs) decreased the expression of proinflammatory markers.
In another recent study, small extracellular vesicles derived from mesenchymal stem cells (MSC-sEV) improve pancreatic cell function, while, after transplantation, MSC-sEVs can chemoattract and migrate to the damaged site (F.-X.-Z. Li et al., 2021). This is due to the proliferation of cells in damaged islets. Sabry et al. discovered that injecting MSC-sEVs into STZ-induced diabetic rats caused the blood glucose level to decrease, plasma insulin levels increased, and the number and size increased (Sabry et al., 2020). MSC-sEVs improve the survival rate and function of islet cells as well as parental MSCs.
Furthermore, MSCs have the potential to treat bronchiolitis obliterans (BO), which is a fibroproliferative disease that causes inflammation in the submucosal cavity and fibrosis of the bronchiolar wall that blocks the lumen (King, 1989). Due to immunomodulatory properties, MSCs locally and systematically MSCs can decrease the progression of BO in different animal models. This study was carried out to investigate the effect of allogeneic adult bone marrow-derived mesenchymal stem cells (BM-MSC) with different ways of administration in a rat model of BO.
The route of administration of BM-MSCs to rat models includes endotracheal, intravenous, and lung injection. On day 7, rats that received BM-MSC through the endotracheal route exhibited no signs of inflammation. MSCs exhibit immunomodulatory capabilities that prevent inflammatory responses. Histopathological changes were observed in the early stages corresponding to inflammatory activity (Gómez de Antonio et al., 2020). The reparative effects of MSCs are activated by endothelial cells and fibroblasts to repair the injured site by releasing immunoregulation and growth factors.
BM-MSCs mediate the response to the injured, which develop alveolar tissues that resemble normal tissue. In summary, BM-MSCs can recognize the injury site and alter the histopathology of BO lesions and restore structure. Although intravenous and locally injected BM-MSCs have an impact on the treatment of BO lesions, endotracheal injection has the most expected results (Gómez de Antonio et al., 2020).
In another study, MSCs were used as an alternative after treating patients with BOS with allogeneic hematopoietic stem cell transplantation (allo-HSCT). Compared to non-MSC treatment, MSC infusion was associated with a considerably improved response, including an improvement in forced expiratory volume in 1 s (FEV1) and steroid reduction at 3 months. Multiple infusions of MSCs were well tolerated, with no increased risk of infection or relapse of leukemia. The efficacy of steroids and azithromycin combined with MSC was much higher than that of steroids and azithromycin alone, with 71% of MSC patients responding compared to 44% of non-MSC patients (S. Chen et al., 2019).
Furthermore, placenta-derived human mesenchymal stem cells (PMSC) and PMSC-conditioned medium (PMSCCM) slow the progression of BO by protecting epithelial integrity at the cellular level. A single injection of PMSCCM could lower proinflammatory cytokines, resulting in decreased infiltration of inflammatory and immune cell infiltration. On the third day, PMSCs were injected intratracheally, which is an important moment for epithelial regeneration. On the 14th day after tracheal transplantation, intratracheal injection of PMSCs and PMSCCM dramatically reduced CD3 + T cell infiltration. These findings imply that PMSCs and PMSCCM can have immunosuppressive properties during the cellular infiltration phase of BO formation (Y. Zhao et al., 2014). Table 4 highlights current clinical trials of the use of MSCs in cell therapy applications.
MSCs isolated from sources such as bone marrow, adipose tissue (ASC), and Wharton’s jelly possess different surface expression markers, chemokines, proinflammatory proteins, and growth factors that aid in cell therapy applications. Considering their high potential in regenerative medicine, WJ-MSCs may exhibit relatively high expression of CD54 and CD146. In response to proinflammatory mediators, CD54 (ICAM-1) stimulates the mobilization and transendothelial migration of circulating cells into injured tissues (L. Yang et al., 2005). In MSCs, CD146 (MCAM, MUC18) is a cell adhesion molecule with a higher differentiation potential (Russell et al., 2010). Both BM-MSCs and AD-MSCs express a high cell marker such as CD73, CD90, CD105, while less expression of hematopoietic markers such as CD34, CD45, and HLA-DR (Mohamed-Ahmed et al., 2018). Both BMSCs and ASCs express Stro-1 (De Ugarte et al., 2003; Strem et al., 2005), a marker of multilineage potential (Dennis et al., 2002). The study confirmed that BMSCs expressed Stro-1 more than ASCs (Mohamed-Ahmed et al., 2018). In this study, ASCs were shown to continue to increase over the next 21 days in this study. In MSCs, CD34 is believed to play an important role in their long-term proliferation. Therefore, the continued proliferation of ASCs may be related to the expression of CD34 by ASCs (Sidney et al., 2014).
As part of the IL-1 family of cytokines, IL1-RA is a receptor antagonist that belongs to at least 11 inflammatory mediators (Amable et al., 2014). Since IL1-RA has protective effects, it has already been proposed as a therapeutic candidate for the treatment of diabetes mellitus II treatment. A model of induced diabetes mellitus showed that IL1-RA prevented pancreatic mononuclear cell infiltration, islet destruction, and hyperglycemia (Sandberg et al., 1994). Ortiz et al., 2007, demonstrated in preclinical studies in mice that BM-MSCs were more effective than recombinant IL1-RA in reducing inflammation and inhibiting fibrosis (Ortiz et al., 2007). However, BM-MSC secreted detectable amounts of VEGF-D as part of the angiogenic process. As the strongest angiogenic and lymphangiogenic VEGF isoform, VEGF-D has been tested in phase I clinical trials for myocardial infarction (Rissanen et al., 2003). Genetically engineered MSCs that overexpress VEGF have been shown to overexpress VEGF are more effective in treating acute myocardial infarction than MSCs alone because VEGF extends the lifespan of MSCs, protects them from apoptosis, and improves their ability to recover heart function (Augustin et al., 2013). The highest concentrations of thrombospondin-2 were found in WJ-MSC supernatants, the most abundant angiogenic factor (Amable et al., 2014). According to Jeong and others, WJ-MSC was able to regenerate cartilage and this effect was mediated by thrombospondin-2 because thrombospondin-2 alone can exert similar effects, and knockdown of thrombospondin-2 with siRNA abolished the ability to regenerate cartilage (Jeong et al., 2013). AT-MSCs also secrete a high concentration of thrombospondin-2, making them a good candidate for cartilage regeneration, especially if patients did not cryopreserve their own umbilical cord cells and could use autologous adipose tissue (Amable et al., 2014). Compared to MSCs alone, genetically modified MSCs expressing angiopoietin-1 were more efficient in regenerating myocardial tissue after infarction (Paul et al., 2012).
MiRNAs are one of the components that were secreted out of the exosomes by stem cells. Studies have indicated that miRNAs can act on gene enhancers within the nucleus to activate genes and promote their expression (Xiao et al., 2016). This shows that miRNAs in MSC exosomes could be used as a future therapeutic target to treat diseases. There are different types of miRNAs that target different genes and can have an impact on different diseases. Using miRNAs, inflammation can be reduced and fibrosis can be prevented (W. Zhang et al., 2019). MiRNAs can be used by stem cell-derived exosomes to correct immune disorders in organ tissues, including allergic airways, Duchenne muscular dystrophy, and myocardial ischemia-reperfusion injury (Bier et al., 2018; Fang et al., 2020; J. Zhao et al., 2019). MiR-146a-5p regulates allergic airway diseases by decreasing the expression of interleukin-9 (IL-9) and interleukin-13 (IL-13) in innate lymphoid cells of Group 2. Therefore, miR-146a-5p expression in MSC exosomes is associated with allergic diseases. An ischemia-reperfusion injury causes inflammation in the heart due to the process of ischemia and reperfusion. According to these findings, miRNAs in exosomes can regulate the immune response by changing macrophages from M1 to M2 anti-inflammatory phenotypes (J. Zhao et al., 2019). In sepsis, miR-233 in MSC exosomes may protect the heart from inflammatory cytokines and reduce heart stimulation and damage (Wang et al., 2015).
MSCs are the most established stem cells that have been used to study disease models compared to iPSCs for many decades. Human iPSCs are currently emerging in research fields and being studied in disease models. Examples of diseases that have used iPSC are Parkinson’s disease, myocardial infarction, mitochondrial DNA depletion syndrome, and Post-traumatic stress disorder (PTSD). Table 5 summarizes the list of diseases that have been studied using iPSCs.
First and foremost, Parkinson’s disease (PD) is the second most prevalent progressive neurodegenerative disorder in the world that affects 0.3% of the global population over the age of 70 years (Lee and Gilbert, 2016). The development of Lewy bodies (LBs) or Lewy neurites that are positive for α-synuclein (α-syn) is the key pathological hallmark of PD. DA neurons derived from the midbrain iPSC are greatly desired for the in vitro PD model (Spathopoulou et al., 2022). This model could aid in studying the α-syn-driven which occurs mostly in dopaminergic (DA) neurons in the SNpc in Parkinson’s disease. This reveals that α-synuclein pathology has adequate seed quality in neurons of patients with PD induced by genetic abnormalities (Hu et al., 2020).
In the latest study, a primate received neurotoxic MPTP, which causes a PD-like condition in the host. This is due to the study on IPSC graft on the effect of the DA neuron IPSC graft (Jarbæk Nielsen et al., 2020). The primate showed a significant increase in spontaneous movement after transplantation, measured by score-based and video-based studies. For more than 2 years, no malignancies grew and only a small immune response was evoked. The capacity of patients to use iPSC from their bodies reduces the risk of immune-mediated graft rejection and eliminates the requirement for immunosuppressive therapy after transplantation (Baniadam et al., 2021).
Furthermore, purified human-induced pluripotent stem cell-derived cardiomyocytes (iPS-CM) were injected directly into the myocardium after MI. There is an improvement after 4 weeks of transplantation. The infarct area was significantly less than in the control group. Through paracrine action, transplanted cells increased angiogenesis and maintained the survival rate of surrounding residential CMs, perhaps leading to functional improvement. Because CMs are a terminal stage of iPS and have limited proliferating ability, no teratomas were observed in any part of the heart (X. Jiang et al., 2020).
The release of exosomes from iPSC-CMs provides a novel type of iPSC-based cardiomyogenesis treatment for heart regeneration as an alternative to direct cell replacement (Ong et al., 2018). Exosomes have antiapoptotic properties, increase angiogenesis, reduce infarct size, and aid in heart recovery. Exosomes obtained from MSCs co-cultured with iPSC-CM boosted prolonged survival and better therapeutic effects in cells after transplantation compared to those derived from iPSC-CMs alone (Yoshida et al., 2018).
In addition, the next study involves the first human trial using clinical-grade hiPSC-CM patches to treat ischemic cardiomyopathy in a patient (Miyagawa et al., 2022). Ischemic cardiomyopathy develops when a patient has a previous myocardial infarction. In the clinical case, no severe arrhythmias or tumors were detected after transplantation. It showed that clinical grade hiPSC-CM patches are non-tumorigenic and non-arrhythmogenic, suggesting that they could be a safe approach to the delivery of cardiac cells (Chow et al., 2017). In the recipient heart, transplanted cardiomyocyte patches have been observed to undergo synchronous contraction/relaxation. After transplantation, the injured myocardium demonstrated time-course healing. Table 6 highlights the current clinical trials carried out using iPSC in cell therapy applications.
The new coronavirus disease 2019 (COVID-19) has drawn the attention of researchers from numerous sectors. The severe acute respiratory syndrome coronavirus 2 (SARS-CoV-2) causes COVID-19, which is a viral acute respiratory disease. COVID-19 mostly harms the lungs and other organs and systems like the heart, immunological system, and so on. Researchers have tried several experiments to develop an appropriate therapeutic option to combat the COVID-19 virus in patients. There are significant concerns about COVID-19 rapid proliferation, although several efforts to create therapeutic platforms have begun, but there is no proper treatment. Cell-based treatment, particularly stem cells, has both therapeutic and preventive potentials.
Stem cells can self-renew and differentiate into multiple types, making them a good option for cell treatment in COVID-19. Stem cell therapy is one of the first treatments to develop for diseases that had not been adequately cured (Du et al., 2020). In this pandemic, stem cell therapies, particularly MSC- and iPSC-related therapies, have demonstrated their therapeutic potential for newly emerging diseases. MSCs have been widely used to study COVID-19-associated diseases, as they release molecules that have potential against the disease. Examples of COVID-19 associated diseases are SARS-CoV-2 pneumonia, COVID-19 pneumonia, acute respiratory distress syndrome (ARDS), and so on. Table 7 summarizes the data of using MSCs in treated patients with COVID-19-associated diseases.
Mesenchymal stem cells have the potential to treat SARS-CoV2 pneumonia by secreting exosomes. These cells are commonly considered the most effective regenerative medicine tool, as they can repair damaged tissues and organs. They also release a variety of chemokines, cytokines, growth factors, and extracellular vesicles (T. Zhao et al., 2019). It also helps stimulate regeneration through the production of soluble substances and exosomes to reduce inflammation and promote tissue regeneration (Ullah et al., 2019). MSC transplantation significantly improved the lung function of individuals with 2019-nCov pneumonia in 2 days (Leng et al., 2020). Intravenous infusion of MSCs could minimize immune system overactivation and assist in healing by altering the pulmonary microenvironment after SARS-CoV2 infection.
In the latest study, BM-MSC exosomes were administered to 24 patients aged 18–85 to determine safety and efficacy within 14 days after treatment. There was no adverse reaction after infusion. This study has a high survival rate and cure rate compared to the mortality rate (Sengupta et al., 2020).
Furthermore, human umbilical cord mesenchymal stem cells have the potential to reduce and heal inflammation-induced lung damage caused by COVID-19 (Wei et al., 2021). In patients with COVID-19 who were treated with hUC-MSC, it improved the oxygenation index and decreased IgM levels. Furthermore, the lung imaging of treated patients improved drastically. The infusion of MSCs did not show any allergic response in patients. hUC-MSCs have shown the ability to move into the wounded lung region and differentiate into alveolar cells (Fu et al., 2019). These cells prevent further lung injury and promote regeneration of wounded lung tissues. The severity of lung inflammation was dramatically reduced. Intravenously injected MSCs in patients with COVID-19 were effective and tolerable.
Induced pluripotent stem cells (iPSCs) are human cells that can self-renew indefinitely and develop into a variety of somatic cells (Sharma et al., 2020). Furthermore, iPSCs are well established and widely discovered by researchers to combat COVID-19. Human iPSCs can be used as an in vitro predictive model for SARS-CoV-2 infection and as a screening platform. iPSCs could be one of the best sources for creating patient-specific organs that have fewer complications after implantation. Since the targeted tissues in COVID-19 patients are the lung, it shows a significant impact on the patient’s prognosis (Carsana et al., 2020). Therefore, lung transplantation employing iPSC technology has positive results and does not present immunological rejection (Basiri et al., 2021). SARS-CoV-2 infections have recently been simulated in various organs using organoid and cellular models derived from iPSC, including the heart, brain, liver, intestines, and pancreas. Table 8 summarizes the data of using iPSCs in the study of patients with diseases associated with COVID-19.
iPSCs have been used currently by researchers to study and design a protocol to overcome SARS-CoV-2 in patients. COVID-19 causes gastrointestinal symptoms such as diarrhea, nausea, and vomiting, as well as respiratory failure. In this study, human-induced pluripotent stem cell (iPSC)-derived small intestinal epithelial cells (iPSC-SIEC) could be employed as a SARS-CoV-2 infection model (Yamada et al., 2022). SARS-CoV-2 infection was found in absorptive and Paneth cells from iPSC-SIECs. Infection with SARS-CoV-2 also reduced transepithelial electrical resistance (TEER) and increased proinflammatory gene expression. Various intestinal disorders, such as inflammatory bowel diseases, which induce nausea, vomiting, and diarrhea, are more likely to be caused by epithelial barrier damage. COVID-19-induced gastrointestinal symptoms could be caused by barrier injury of the intestinal mucosa caused by viral infection. In SIECs, infection with SARS-CoV-2 increased the expression of proinflammatory genes. Increased cytokine levels have often been observed in patients with severe COVID-19 (L. Zhou et al., 2020, p. 2). The gastrointestinal tract was also involved in broad systemic inflammation in deceased COVID-19 individuals.
Remdesivir also decreased SARS-CoV-2 infection and reversed SARS-CoV-2-induced barrier degradation and inflammatory responses. The disruption of the mucosal barrier and inflammatory reactions after SARS-CoV-2 infection could have caused these gut injuries. Remdesivir suppresses the cytokine storm in patients with COVID-19, implying that remdesivir reduces patients’ gastrointestinal problems (Yamada et al., 2022).
Additionally, SARS-CoV-2 infection affects other organs than the lungs. Neurological tissues and eyes have been observed to be affected in some patients with COVID-19. Different studies showed that ACE2 expression was found in the human cornea and conjunctiva (L. Zhou et al., 2020). Furthermore, SARS-CoV-2 was discovered in postmortem retinal samples from COVID-19 patients (Casagrande et al., 2020). This study was to check whether the expression of ACE2 in human retinal neurons has a function during SARS-CoV-2 infection. Thus, hiPSC retinal organoids and monolayer cultures from dissociated retinal organoids were created (Ahmad Mulyadi Lai et al., 2021).
SARS-CoV-2 pseudovirus was used, which is based on the lentiviral system and contains the S protein on the surface of the capsid. Dissociated retinal organoids in monolayer culture were sensitive to low MOI of the SARS-CoV-2 pseudovirus. In retinal organoids, ACE2 acts as a virus entry channel. RNA sequence analysis and bioinformatic analysis were performed to identify genes expressed differently in infected organoids and monolayer cultures (Ahmad Mulyadi Lai et al., 2021). The RNA sequence shows that the retinal organoids and monolayer cultures regulated epithelial cell apoptosis and the inflammatory response. Inflammatory responses caused by SARS-CoV-2 are the leading cause of disease development in COVID-19 patients (García, 2020). The genes in the inflammatory response, such as F3 and SEMA7A, appear higher in SARS-CoV-2 pseudovirus infected retinal organoids and monolayer cultures than in uninfected controls.
This can be concluded that both retinal organoids and monolayer cultures express ACE2 and are likely to be infected by the SARS-CoV-2 pseudovirus. This shows that human iPSC-derived retinal organoid and monolayer cultures are the best disease platform to study viral entry.
In summary, after decades of research, stem cell therapy is proving to be a tremendous game changer in medicine. The potential of stem cells develops with each experiment, yet there are still numerous challenges to overcome. The use of MSCs for therapeutic purposes is significant because they are easily available from different sources, they can produce in large quantities, and they have a simple isolation procedure. Currently, MSCs are one of the most vital cells widely utilized in clinical and preclinical trials due to their valuable advantages. The challenges in MSCs involve using autologous and allogeneic MSCs for clinical application. Allogeneic MSCs appear to be used more frequently in preclinical studies and clinical trials. Transplantation of autologous MSCs has some limitations. The first problem is the high cost of preparing cells for one recipient alone. It is also challenging to obtain a clinical dose of MSCs from some patients. MSCs isolated from older donors have decreased proliferation, differentiation, and regenerative potential, resulting in ineffective therapies (Marędziak et al., 2016). On the contrary, it is evident that the use of allogeneic versus autologous MSCs for cell therapy has clear advantages. To solve this problem, allogeneic MSCs from healthy young donors are the best option. Furthermore, autologous MSCs expand at a slow rate, making this therapeutic approach difficult to use for early treatment of acute diseases. The benefit of allogeneic MSCs is that once obtained, they can be frozen and stored, then rapidly thawed and administered immediately to the patient (García-Bernal et al., 2021).
On the other hand, iPSCs are crucial, as MSCs are limited in number and differentiation potential. Human-induced pluripotent stem cells (hiPSCs) are a promising alternative to embryonic cells, eliminating ethical concerns. Clinical trials using iPSCs are less expensive and reduce immunorejection rates. Despite the advances made so far, more intense research is required on human iPSCs to understand the basic biology of pluripotency and cellular differentiation to address all challenges related to therapeutic applications. To generate and expand iPS cells, it requires a high cost, including the necessary experiments to validate the safety and pluripotency. It also requires a longer period of time for production. To overcome this issue, allogeneic therapies, which are more economical and widely available for clinical use. Regarding clinical trials involving MSCs and iPSCs, more studies have been recorded using MSCs compared to iPSCs from 2018 to 2021. This is because MSCs are well established and commonly used in therapeutic applications, whereas iPSCs are relatively new and need to be improved to obtain clinical-grade human iPSCs for safe cell treatment. We project that hiPSCs would further dominate stem cell-based therapy due to their availability if most of their limitations can be addressed.
Conceptualization, FN, GT, WW, SD; validation, GT, WW, and SD; writing -original draft preparation, KT, AJ; drawing of figures, KT, AJ; writing-review and editing, GT, WW, FN, SD; visualization and supervision, GT, WW, and FN; project administration, FN; funding acquisition, FN. All authors have read and agreed to the published version of the manuscript.
The study was supported by the Ministry of Higher Education Malaysia (MOHE) through the award grant FRGS/1/2020/SKK06/UKM/03/4 and Universiti Kebangsaan Malaysia through a Faculty of Medicine grant (FF-2020-327). This funding body accepted the study as proposed.
We would like to thank the members of Centre for Tissue Engineering and Regenerative Medicine (CTERM), UKM Medical Centre (UKMMC), for their support and assistance. Additionally, we thank Universiti Kebangsaan Malaysia (UKM) for the support and approval to conduct this study (Ethics approval code UKM PPI/111/8/JEP-2021-347).
The authors declare that the research was conducted in the absence of any commercial or financial relationships that could be construed as a potential conflict of interest.
All claims expressed in this article are solely those of the authors and do not necessarily represent those of their affiliated organizations, or those of the publisher, the editors and the reviewers. Any product that may be evaluated in this article, or claim that may be made by its manufacturer, is not guaranteed or endorsed by the publisher.
Aasen, T., Raya, A., Barrero, M. J., Garreta, E., Consiglio, A., Gonzalez, F., et al. (2008). Efficient and rapid generation of induced pluripotent stem cells from human keratinocytes. Nat. Biotechnol. 26 (11), 1276–1284. doi:10.1038/nbt.1503
Ahmad Mulyadi Lai, H. I., Chou, S.-J., Chien, Y., Tsai, P.-H., Chien, C.-S., Hsu, C.-C., et al. (2021). Expression of endogenous angiotensin-converting enzyme 2 in human induced pluripotent stem cell-derived retinal organoids. Int. J. Mol. Sci. 22 (3), 1320. doi:10.3390/ijms22031320
Akbari, A., and Rezaie, J. (2020). Potential therapeutic application of mesenchymal stem cell-derived exosomes in SARS-CoV-2 pneumonia. Stem Cell Res. Ther. 11 (1), 356. doi:10.1186/s13287-020-01866-6
Al Abbar, A., Ngai, S. C., Nograles, N., Alhaji, S. Y., and Abdullah, S. (2020). Induced pluripotent stem cells: Reprogramming platforms and applications in cell replacement therapy. Biores. Open Access 9 (1), 121–136. doi:10.1089/biores.2019.0046
Al-Anazi, K. A. (2020). “Introductory chapter,” in Update on mesenchymal and induced pluripotent stem cells. London: IntechOpen. doi:10.5772/intechopen.90236
Amable, P. R., Teixeira, M. V. T., Carias, R. B. V., Granjeiro, J. M., and Borojevic, R. (2014). Protein synthesis and secretion in human mesenchymal cells derived from bone marrow, adipose tissue and Wharton’s jelly. Stem Cell Res. Ther. 5 (2), 53. doi:10.1186/scrt442
Atashi, F., Modarressi, A., and Pepper, M. S. (2015). The role of reactive oxygen species in mesenchymal stem cell adipogenic and osteogenic differentiation: A review. Stem Cells Dev. 24 (10), 1150–1163. doi:10.1089/scd.2014.0484
Augustin, M., Mahar, M. A. A., Lakkisto, P., Tikkanen, I., Vento, A., Pätilä, T., et al. (2013). VEGF overexpression improves mesenchymal stem cell sheet transplantation therapy for acute myocardial infarction. J. Tissue Eng. Regen. Med. 7 (9), 742–750. doi:10.1002/term.1471
Baniadam, K., Tranchina, S., Lawlor, M.-K., and Kakazu, J. (2021). Therapeutic applications of induced pluripotent stem cell use in Parkinson’s disease models. Georget. Med. Rev. 5 (1), 29784. doi:10.52504/001c.29784
Barkholt, L., Flory, E., Jekerle, V., Lucas-Samuel, S., Ahnert, P., Bisset, L., et al. (2013). Risk of tumorigenicity in mesenchymal stromal cell-based therapies—bridging scientific observations and regulatory viewpoints. Cytotherapy 15 (7), 753–759. doi:10.1016/j.jcyt.2013.03.005
Bartholomew, A., Sturgeon, C., Siatskas, M., Ferrer, K., McIntosh, K., Patil, S., et al. (2002). Mesenchymal stem cells suppress lymphocyte proliferation in vitro and prolong skin graft survival in vivo. Exp. Hematol. 30 (1), 42–48. doi:10.1016/s0301-472x(01)00769-x
Basiri, A., Mansouri, F., Azari, A., Ranjbarvan, P., Zarein, F., Heidari, A., et al. (2021). Stem cell therapy potency in personalizing severe COVID-19 treatment. Stem Cell Rev. Rep. 17 (1), 193–213. doi:10.1007/s12015-020-10110-w
Bassett, A. R. (2017). Editing the genome of hiPSC with CRISPR/Cas9: Disease models. Mamm. Genome 28 (7), 348–364. doi:10.1007/s00335-017-9684-9
Belviso, I., Romano, V., Nurzynska, D., Castaldo, C., and Meglio, F. D. (2020). “Non-integrating methods to produce induced pluripotent stem cells for regenerative medicine: An overview,” in Biomechanics and functional tissue engineering. London: IntechOpen. doi:10.5772/intechopen.95070
Bhartiya, D., Nagvenkar, P., Sriraman, K., and Shaikh, A. (2013). “An overview of pluripotent stem cells,” in Pluripotent stem cells. London: IntechOpen. doi:10.5772/55130
Bier, A., Berenstein, P., Kronfeld, N., Morgoulis, D., Ziv-Av, A., Goldstein, H., et al. (2018). Placenta-derived mesenchymal stromal cells and their exosomes exert therapeutic effects in Duchenne muscular dystrophy. Biomaterials 174, 67–78. doi:10.1016/j.biomaterials.2018.04.055
Boika, A., Aleinikava, N., Chyzhyk, V., Zafranskaya, M., Nizheharodava, D., and Ponomarev, V. (2020). Mesenchymal stem cells in Parkinson’s disease: Motor and nonmotor symptoms in the early posttransplant period. Surg. Neurol. Int. 11, 380. doi:10.25259/SNI_233_2020
Bojkova, D., Wagner, J. U. G., Shumliakivska, M., Aslan, G. S., Saleem, U., Hansen, A., et al. (2020). SARS-CoV-2 infects and induces cytotoxic effects in human cardiomyocytes. Cardiovasc. Res. 116, 2207–2215. cvaa267. doi:10.1093/cvr/cvaa267
Boyd, N. L., Robbins, K. R., Dhara, S. K., West, F. D., and Stice, S. L. (2009). Human embryonic stem cell–derived mesoderm-like epithelium transitions to mesenchymal progenitor cells. Tissue Eng. Part A 15 (8), 1897–1907. doi:10.1089/ten.tea.2008.0351
Bsibsi, M., Persoon-Deen, C., Verwer, R. W. H., Meeuwsen, S., Ravid, R., and Van Noort, J. M. (2006). Toll-like receptor 3 on adult human astrocytes triggers production of neuroprotective mediators. Glia 53 (7), 688–695. doi:10.1002/glia.20328
Butovsky, O., Koronyo-Hamaoui, M., Kunis, G., Ophir, E., Landa, G., Cohen, H., et al. (2006). Glatiramer acetate fights against Alzheimer’s disease by inducing dendritic-like microglia expressing insulin-like growth factor 1. Proc. Natl. Acad. Sci. U. S. A. 103 (31), 11784–11789. doi:10.1073/pnas.0604681103
Byun, K., Kim, T.-K., Oh, J., Bayarsaikhan, E., Kim, D., Lee, M. Y., et al. (2013). Heat shock instructs hESCs to exit from the self-renewal program through negative regulation of OCT4 by SAPK/JNK and HSF1 pathway. Stem Cell Res. 11 (3), 1323–1334. doi:10.1016/j.scr.2013.08.014
Cahan, P., and Daley, G. Q. (2013). Origins and implications of pluripotent stem cell variability and heterogeneity. Nat. Rev. Mol. Cell Biol. 14 (6), 357–368. doi:10.1038/nrm3584
Carsana, L., Sonzogni, A., Nasr, A., Rossi, R. S., Pellegrinelli, A., Zerbi, P., et al. (2020). Pulmonary post-mortem findings in a series of COVID-19 cases from northern Italy: A two-centre descriptive study. Lancet. Infect. Dis. 20 (10), 1135–1140. doi:10.1016/S1473-3099(20)30434-5
Casagrande, M., Fitzek, A., Püschel, K., Aleshcheva, G., Schultheiss, H.-P., Berneking, L., et al. (2020). Detection of SARS-CoV-2 in human retinal biopsies of deceased COVID-19 patients. Ocul. Immunol. Inflamm. 28 (5), 721–725. doi:10.1080/09273948.2020.1770301
Chambers, I., Colby, D., Robertson, M., Nichols, J., Lee, S., Tweedie, S., et al. (2003). Functional expression cloning of Nanog, a pluripotency sustaining factor in embryonic stem cells. Cell 113 (5), 643–655. doi:10.1016/s0092-8674(03)00392-1
Chen, C.-T., Shih, Y.-R. V., Kuo, T. K., Lee, O. K., and Wei, Y.-H. (2008). Coordinated changes of mitochondrial biogenesis and antioxidant enzymes during osteogenic differentiation of human mesenchymal stem cells. Stem Cells Dayt. Ohio) 26 (4), 960–968. doi:10.1634/stemcells.2007-0509
Chen, H.-F., Kuo, H.-C., Lin, S.-P., Chien, C.-L., Chiang, M.-S., and Ho, H.-N. (2010). Hypoxic culture maintains self-renewal and enhances embryoid body formation of human embryonic stem cells. Tissue Eng. Part A 16 (9), 2901–2913. doi:10.1089/ten.tea.2009.0722
Chen, J., Zheng, C.-X., Jin, Y., and Hu, C.-H. (2021). Mesenchymal stromal cell-mediated immune regulation: A promising remedy in the therapy of type 2 diabetes mellitus. STEM CELLS 39 (7), 838–852. doi:10.1002/stem.3357
Chen, S., Zhao, K., Lin, R., Wang, S., Fan, Z., Huang, F., et al. (2019). The efficacy of mesenchymal stem cells in bronchiolitis obliterans syndrome after allogeneic hsct: A multicenter prospective cohort study. EBioMedicine 49, 213–222. doi:10.1016/j.ebiom.2019.09.039
Chen, Y. S., Pelekanos, R. A., Ellis, R. L., Horne, R., Wolvetang, E. J., and Fisk, N. M. (2012). Small molecule mesengenic induction of human induced pluripotent stem cells to generate mesenchymal stem/stromal cells. Stem Cells Transl. Med. 1 (2), 83–95. doi:10.5966/sctm.2011-0022
Chen, Z.-Y., He, C.-Y., and Kay, M. A. (2005). Improved production and purification of minicircle DNA vector free of plasmid bacterial sequences and capable of persistent transgene expression in vivo. Hum. Gene Ther. 16 (1), 126–131. doi:10.1089/hum.2005.16.126
Chew, J.-L., Loh, Y.-H., Zhang, W., Chen, X., Tam, W.-L., Yeap, L.-S., et al. (2005). Reciprocal transcriptional regulation of Pou5f1 and Sox2 via the oct4/sox2 complex in embryonic stem cells. Mol. Cell. Biol. 25 (14), 6031–6046. doi:10.1128/MCB.25.14.6031-6046.2005
Chow, A., Stuckey, D. J., Kidher, E., Rocco, M., Jabbour, R. J., Mansfield, C. A., et al. (2017). Human induced pluripotent stem cell-derived cardiomyocyte encapsulating bioactive hydrogels improve rat heart function post myocardial infarction. Stem Cell Rep. 9 (5), 1415–1422. doi:10.1016/j.stemcr.2017.09.003
Chun, Y. S., Byun, K., and Lee, B. (2011). Induced pluripotent stem cells and personalized medicine: Current progress and future perspectives. Anat. Cell Biol. 44 (4), 245–255. doi:10.5115/acb.2011.44.4.245
de Girolamo, L., Lopa, S., Arrigoni, E., Sartori, M. F., Baruffaldi Preis, F. W., and Brini, A. T. (2009). Human adipose-derived stem cells isolated from young and elderly women: Their differentiation potential and scaffold interaction during in vitro osteoblastic differentiation. Cytotherapy 11 (6), 793–803. doi:10.3109/14653240903079393
De Ugarte, D. A., Alfonso, Z., Zuk, P. A., Elbarbary, A., Zhu, M., Ashjian, P., et al. (2003). Differential expression of stem cell mobilization-associated molecules on multi-lineage cells from adipose tissue and bone marrow. Immunol. Lett. 89 (2–3), 267–270. doi:10.1016/s0165-2478(03)00108-1
DelaRosa, O., and Lombardo, E. (2010). Modulation of adult mesenchymal stem cells activity by toll-like receptors: Implications on therapeutic potential. Mediat. Inflamm. 2010, e865601. doi:10.1155/2010/865601
Dennis, J. E., Carbillet, J.-P., Caplan, A. I., and Charbord, P. (2002). The STRO-1+ marrow cell population is multipotential. Cells, Tissues, Organs 170 (2–3), 73–82. doi:10.1159/000046182
Di Nicola, M., Carlo-Stella, C., Magni, M., Milanesi, M., Longoni, P. D., Matteucci, P., et al. (2002). Human bone marrow stromal cells suppress T-lymphocyte proliferation induced by cellular or nonspecific mitogenic stimuli. Blood 99 (10), 3838–3843. doi:10.1182/blood.v99.10.3838
Doi, D., Magotani, H., Kikuchi, T., Ikeda, M., Hiramatsu, S., Yoshida, K., et al. (2020). Pre-clinical study of induced pluripotent stem cell-derived dopaminergic progenitor cells for Parkinson’s disease. Nat. Commun. 11, 3369. doi:10.1038/s41467-020-17165-w
Dominici, M., Le Blanc, K., Mueller, I., Slaper-Cortenbach, I., Marini, F., Krause, D., et al. (2006). Minimal criteria for defining multipotent mesenchymal stromal cells. The International Society for Cellular Therapy position statement. Cytotherapy 8 (4), 315–317. doi:10.1080/14653240600855905
Domnina, A., Novikova, P., Obidina, J., Fridlyanskaya, I., Alekseenko, L., Kozhukharova, I., et al. (2018). Human mesenchymal stem cells in spheroids improve fertility in model animals with damaged endometrium. Stem Cell Res. Ther. 9, 50. doi:10.1186/s13287-018-0801-9
Du, J., Li, H., Lian, J., Zhu, X., Qiao, L., and Lin, J. (2020). Stem cell therapy: A potential approach for treatment of influenza virus and coronavirus-induced acute lung injury. Stem Cell Res. Ther. 11, 192. doi:10.1186/s13287-020-01699-3
Dufait, I., Liechtenstein, T., Lanna, A., Bricogne, C., Laranga, R., Padella, A., et al. (2012). Retroviral and lentiviral vectors for the induction of immunological tolerance. Scientifica 2012, 694137. doi:10.6064/2012/694137
Dupont, S., Morsut, L., Aragona, M., Enzo, E., Giulitti, S., Cordenonsi, M., et al. (2011). Role of YAP/TAZ in mechanotransduction. Nature 474 (7350), 179–183. doi:10.1038/nature10137
Estrada, J. C., Torres, Y., Benguría, A., Dopazo, A., Roche, E., Carrera-Quintanar, L., et al. (2013). Human mesenchymal stem cell-replicative senescence and oxidative stress are closely linked to aneuploidy. Cell Death Dis. 4 (6), e691. doi:10.1038/cddis.2013.211
Fan, X.-L., Zhang, Y., Li, X., and Fu, Q.-L. (2020). Mechanisms underlying the protective effects of mesenchymal stem cell-based therapy. Cell. Mol. Life Sci. 77 (14), 2771–2794. doi:10.1007/s00018-020-03454-6
Fang, S.-B., Zhang, H.-Y., Wang, C., He, B.-X., Liu, X.-Q., Meng, X.-C., et al. (2020). Small extracellular vesicles derived from human mesenchymal stromal cells prevent group 2 innate lymphoid cell-dominant allergic airway inflammation through delivery of miR-146a-5p. J. Extracell. Vesicles 9 (1), 1723260. doi:10.1080/20013078.2020.1723260
Fernandez, T. de S., and Fernandez, C. de S. (2016). “Mesenchymal stem cells: Biological characteristics and potential clinical applications for haematopoietic stem cell transplantation,” in Pluripotent stem cells—from the bench to the clinic. London: IntechOpen. doi:10.5772/63772
Ferrer, L., Kimbrel, E. A., Lam, A., Falk, E. B., Zewe, C., Juopperi, T., et al. (2016). Treatment of perianal fistulas with human embryonic stem cell-derived mesenchymal stem cells: A canine model of human fistulizing crohn’s disease. Regen. Med. 11 (1), 33–43. doi:10.2217/rme.15.69
Forristal, C. E., Wright, K. L., Hanley, N. A., Oreffo, R. O. C., and Houghton, F. D. (2010). Hypoxia inducible factors regulate pluripotency and proliferation in human embryonic stem cells cultured at reduced oxygen tensions. Reprod. Camb. Engl. 139 (1), 85–97. doi:10.1530/REP-09-0300
Fraser, J. K., Wulur, I., Alfonso, Z., and Hedrick, M. H. (2006). Fat tissue: An underappreciated source of stem cells for biotechnology. Trends Biotechnol. 24 (4), 150–154. doi:10.1016/j.tibtech.2006.01.010
Fu, X., Liu, G., Halim, A., Ju, Y., Luo, Q., and Song, A. G. (2019). Mesenchymal stem cell migration and tissue repair. Cells 8 (8), E784. doi:10.3390/cells8080784
Fuchs, E. (2007). Scratching the surface of skin development. Nature 445 (7130), 834–842. doi:10.1038/nature05659
Fusaki, N., Ban, H., Nishiyama, A., Saeki, K., and Hasegawa, M. (2009). Efficient induction of transgene-free human pluripotent stem cells using a vector based on Sendai virus, an RNA virus that does not integrate into the host genome. Proc. Jpn. Acad. Ser. B Phys. Biol. Sci. 85 (8), 348–362. doi:10.2183/pjab.85.348
Gao, L., Gregorich, Z. R., Zhu, W., Mattapally, S., Oduk, Y., Lou, X., et al. (2018). Large cardiac-muscle patches engineered from human induced-pluripotent stem-cell–derived cardiac cells improve recovery from myocardial infarction in swine. Circulation 137 (16), 1712–1730. doi:10.1161/CIRCULATIONAHA.117.030785
García, L. F. (2020). Immune response, inflammation, and the clinical spectrum of COVID-19. Front. Immunol. 11, 1441. doi:10.3389/fimmu.2020.01441
García-Bernal, D., García-Arranz, M., Yáñez, R. M., Hervás-Salcedo, R., Cortés, A., Fernández-García, M., et al. (2021). The current status of mesenchymal stromal cells: Controversies, unresolved issues and some promising solutions to improve their therapeutic efficacy. Front. Cell Dev. Biol. 9, 650664. doi:10.3389/fcell.2021.650664
Ghaleb, A. M., Nandan, M. O., Chanchevalap, S., Dalton, W. B., Hisamuddin, I. M., and Yang, V. W. (2005). Krüppel-like factors 4 and 5: The yin and yang regulators of cellular proliferation. Cell Res. 15 (2), 92–96. doi:10.1038/sj.cr.7290271
Gomes, A. R., Fernandes, T. G., Vaz, S. H., Silva, T. P., Bekman, E. P., Xapelli, S., et al. (2020). Modeling rett syndrome with human patient-specific forebrain organoids. Front. Cell Dev. Biol. 8, 610427. doi:10.3389/fcell.2020.610427
Gómez de Antonio, D., Campo-Cañaveral de la Cruz, J. L., Zurita, M., Santos, M., González Lois, C., Varela de Ugarte, A., et al. (2020). Bone marrow-derived mesenchymal stem cells and chronic allograft disease in a bronchiolitis obliterans animal model. Arch. Bronconeumol. 56 (3), 149–156. doi:10.1016/j.arbres.2019.05.016
Guha, P., Morgan, J. W., Mostoslavsky, G., Rodrigues, N. P., and Boyd, A. S. (2013). Lack of immune response to differentiated cells derived from syngeneic induced pluripotent stem cells. Cell Stem Cell 12 (4), 407–412. doi:10.1016/j.stem.2013.01.006
Han, J. W., and Yoon, Y. (2011). Induced pluripotent stem cells: Emerging techniques for nuclear reprogramming. Antioxid. Redox Signal. 15 (7), 1799–1820. doi:10.1089/ars.2010.3814
He, M., Shi, X., Yang, M., Yang, T., Li, T., and Chen, J. (2019). Mesenchymal stem cells-derived IL-6 activates AMPK/mTOR signaling to inhibit the proliferation of reactive astrocytes induced by hypoxic-ischemic brain damage. Exp. Neurol. 311, 15–32. doi:10.1016/j.expneurol.2018.09.006
Hematti, P. (2011b). Human embryonic stem cell derived mesenchymal stromal cells. Transfusion 51 (4), 138S–144S. doi:10.1111/j.1537-2995.2011.03376.x
Hematti, P. (2011a). Human embryonic stem cell-derived mesenchymal progenitors: An overview. Methods Mol. Biol. 690, 163–174. doi:10.1007/978-1-60761-962-8_11
Higuchi, M., Dusting, G. J., Peshavariya, H., Jiang, F., Hsiao, S. T.-F., Chan, E. C., et al. (2013). Differentiation of human adipose-derived stem cells into fat involves reactive oxygen species and forkhead box O1 mediated upregulation of antioxidant enzymes. Stem Cells Dev. 22 (6), 878–888. doi:10.1089/scd.2012.0306
Hochedlinger, K., Yamada, Y., Beard, C., and Jaenisch, R. (2005). Ectopic expression of oct-4 blocks progenitor-cell differentiation and causes dysplasia in epithelial tissues. Cell 121 (3), 465–477. doi:10.1016/j.cell.2005.02.018
Hosoya, N., Miura, T., Kawana-Tachikawa, A., Koibuchi, T., Shioda, T., Odawara, T., et al. (2008). Comparison between Sendai virus and adenovirus vectors to transduce HIV-1 genes into human dendritic cells. J. Med. Virol. 80 (3), 373–382. doi:10.1002/jmv.21052
Hu, X., Mao, C., Fan, L., Luo, H., Hu, Z., Zhang, S., et al. (2020). Modeling Parkinson’s disease using induced pluripotent stem cells. Stem Cells Int. 2020, 1061470. doi:10.1155/2020/1061470
Hua, J., Gong, J., Meng, H., Xu, B., Yao, L., Qian, M., et al. (2013). Comparison of different methods for the isolation of mesenchymal stem cells from umbilical cord matrix: Proliferation and multilineage differentiation as compared to mesenchymal stem cells from umbilical cord blood and bone marrow. Cell Biol. Int. 38, 198–210. doi:10.1002/cbin.10188
Hua, Y., Yoshimochi, K., Li, J., Takekita, K., Shimotsuma, M., Li, L., et al. (2022). Development and evaluation of a novel xeno-free culture medium for human-induced pluripotent stem cells. Stem Cell Res. Ther. 13 (1), 223. doi:10.1186/s13287-022-02879-z
Ilic, D., and Polak, J. M. (2011). Stem cells in regenerative medicine: Introduction. Br. Med. Bull. 98, 117–126. doi:10.1093/bmb/ldr012
Jacob, F., Pather, S. R., Huang, W.-K., Zhang, F., Wong, S. Z. H., Zhou, H., et al. (2020). Human pluripotent stem cell-derived neural cells and brain organoids reveal SARS-CoV-2 neurotropism predominates in choroid plexus epithelium. Cell Stem Cell 27 (6), 937–950. e9. doi:10.1016/j.stem.2020.09.016
Jansen, J., Reimer, K. C., Nagai, J. S., Varghese, F. S., Overheul, G. J., de Beer, M., et al. (2022). SARS-CoV-2 infects the human kidney and drives fibrosis in kidney organoids. Cell Stem Cell 29 (2), 217–231. e8. doi:10.1016/j.stem.2021.12.010
Jarbæk Nielsen, J. J., Lillethorup, T. P., Glud, A. N., Hedemann Sørensen, J. C., and Orlowski, D. (2020). The application of iPSCs in Parkinson’s disease. Acta Neurobiol. Exp. (Wars). 80 (3), 273–285. doi:10.21307/ane-2020-024
Jefford, M., and Moore, R. (2008). Improvement of informed consent and the quality of consent documents. Lancet. Oncol. 9 (5), 485–493. doi:10.1016/S1470-2045(08)70128-1
Jeong, S. Y., Kim, D. H., Ha, J., Jin, H. J., Kwon, S.-J., Chang, J. W., et al. (2013). Thrombospondin-2 secreted by human umbilical cord blood-derived mesenchymal stem cells promotes chondrogenic differentiation. Stem Cells Dayt. Ohio) 31 (10), 2136–2148. doi:10.1002/stem.1471
Jia, F., Wilson, K. D., Sun, N., Gupta, D. M., Huang, M., Li, Z., et al. (2010). A nonviral minicircle vector for deriving human iPS cells. Nat. Methods 7 (3), 197–199. doi:10.1038/nmeth.1426
Jiang, X., Yang, Z., and Dong, M. (2020). Cardiac repair in a murine model of myocardial infarction with human induced pluripotent stem cell-derived cardiomyocytes. Stem Cell Res. Ther. 11 (1), 297. doi:10.1186/s13287-020-01811-7
Jiang, Z., Han, Y., and Cao, X. (2014). Induced pluripotent stem cell (iPSCs) and their application in immunotherapy. Cell. Mol. Immunol. 11 (1), 17–24. doi:10.1038/cmi.2013.62
Jing, R., Corbett, J. L., Cai, J., Beeson, G. C., Beeson, C. C., Chan, S. S., et al. (2018). A screen using iPSC-derived hepatocytes reveals NAD+ as a potential treatment for mtDNA depletion syndrome. Cell Rep. 25 (6), 1469–1484. e5. doi:10.1016/j.celrep.2018.10.036
Jing, W., Xiao, J., Xiong, Z., Yang, X., Huang, Y., Zhou, M., et al. (2011). Explant culture: An efficient method to isolate adipose-derived stromal cells for tissue engineering. Artif. Organs 35 (2), 105–112. doi:10.1111/j.1525-1594.2010.01054.x
Jung, Y., Bauer, G., and Nolta, J. A. (2012). Concise review: Induced pluripotent stem cell-derived mesenchymal stem cells: Progress toward safe clinical products. Stem Cells Dayt. Ohio) 30 (1), 42–47. doi:10.1002/stem.727
Kaitsuka, T., and Hakim, F. (2021). Response of pluripotent stem cells to environmental stress and its application for directed differentiation. Biology 10 (2), 84. doi:10.3390/biology10020084
Kanda, Y., Hinata, T., Kang, S. W., and Watanabe, Y. (2011). Reactive oxygen species mediate adipocyte differentiation in mesenchymal stem cells. Life Sci. 89 (7–8), 250–258. doi:10.1016/j.lfs.2011.06.007
Karnoub, A. E., Dash, A. B., Vo, A. P., Sullivan, A., Brooks, M. W., Bell, G. W., et al. (2007). Mesenchymal stem cells within tumour stroma promote breast cancer metastasis. Nature 449 (7162), 557–563. doi:10.1038/nature06188
Kase, Y., and Okano, H. (2020). Expression of ACE2 and a viral virulence-regulating factor CCN family member 1 in human iPSC-derived neural cells: Implications for COVID-19-related CNS disorders. Inflamm. Regen. 40 (1), 32. doi:10.1186/s41232-020-00143-6
Kassem, M., Ankersen, L., Eriksen, E. F., Clark, B. F., and Rattan, S. I. (1997). Demonstration of cellular aging and senescence in serially passaged long-term cultures of human trabecular osteoblasts. Osteoporos. Int. 7 (6), 514–524. doi:10.1007/BF02652556
Kim, K. S., Choi, H. W., Yoon, H. E., and Kim, I. Y. (2010). Reactive oxygen species generated by NADPH oxidase 2 and 4 are required for chondrogenic differentiation. J. Biol. Chem. 285 (51), 40294–40302. doi:10.1074/jbc.M110.126821
Kim, K., Zhao, R., Doi, A., Ng, K., Unternaehrer, J., Cahan, P., et al. (2011). Donor cell type can influence the epigenome and differentiation potential of human induced pluripotent stem cells. Nat. Biotechnol. 29 (12), 1117–1119. doi:10.1038/nbt.2052
Kim, Y. E., Hipp, M. S., Bracher, A., Hayer-Hartl, M., and Hartl, F. U. (2013). Molecular chaperone functions in protein folding and proteostasis. Annu. Rev. Biochem. 82, 323–355. doi:10.1146/annurev-biochem-060208-092442
Kishino, Y., Seki, T., Yuasa, S., Fujita, J., and Fukuda, K. (2015). Generation of induced pluripotent stem cells from human peripheral T cells using Sendai virus in feeder-free conditions. J. Vis. Exp. 105, e53225. doi:10.3791/53225
Ko, E., Lee, K. Y., and Hwang, D. S. (2012). Human umbilical cord blood–derived mesenchymal stem cells undergo cellular senescence in response to oxidative stress. Stem Cells Dev. 21 (11), 1877–1886. doi:10.1089/scd.2011.0284
Kolios, G., and Moodley, Y. (2013). Introduction to stem cells and regenerative medicine. Respiration. 85 (1), 3–10. doi:10.1159/000345615
Kuttler, F., and Mai, S. (2006). C-myc, genomic instability and disease. Genome Dyn. 1, 171–190. doi:10.1159/000092507
Lee, A., and Gilbert, R. M. (2016). Epidemiology of Parkinson Disease. Neurologic Clinics 34 (4), 955–965. doi:10.1016/j.ncl.2016.06.012
Lee, H. J., Lee, J. K., Lee, H., Carter, J. E., Chang, J. W., Oh, W., et al. (2012). Human umbilical cord blood-derived mesenchymal stem cells improve neuropathology and cognitive impairment in an Alzheimer’s disease mouse model through modulation of neuroinflammation. Neurobiol. Aging 33 (3), 588–602. doi:10.1016/j.neurobiolaging.2010.03.024
Leng, Z., Zhu, R., Hou, W., Feng, Y., Yang, Y., Han, Q., et al. (2020). Transplantation of ACE2- mesenchymal stem cells improves the outcome of patients with COVID-19 pneumonia. Aging Dis. 11 (2), 216–228. doi:10.14336/AD.2020.0228
Lewis, P. F., and Emerman, M. (1994). Passage through mitosis is required for oncoretroviruses but not for the human immunodeficiency virus. J. Virol. 68 (1), 510–516. doi:10.1128/JVI.68.1.510-516.1994
Li FXZ, F.-X.-Z., Lin, X., Xu, F., Shan, S.-K., Guo, B., Lei, L.-M., et al. (2021). The role of mesenchymal stromal cells-derived small extracellular vesicles in diabetes and its chronic complications. Front. Endocrinol. 12, 780974. doi:10.3389/fendo.2021.780974
Li, H. O., Zhu, Y. F., Asakawa, M., Kuma, H., Hirata, T., Ueda, Y., et al. (2000). A cytoplasmic RNA vector derived from nontransmissible Sendai virus with efficient gene transfer and expression. J. Virol. 74 (14), 6564–6569. doi:10.1128/jvi.74.14.6564-6569.2000
Li Y, Y., Shi, G., Han, Y., Shang, H., Li, H., Liang, W., et al. (2021). Therapeutic potential of human umbilical cord mesenchymal stem cells on aortic atherosclerotic plaque in a high-fat diet rabbit model. Stem Cell Res. Ther. 12 (1), 407. doi:10.1186/s13287-021-02490-8
Li, Y., McClintick, J., Zhong, L., Edenberg, H. J., Yoder, M. C., and Chan, R. J. (2005). Murine embryonic stem cell differentiation is promoted by SOCS-3 and inhibited by the zinc finger transcription factor Klf4. Blood 105 (2), 635–637. doi:10.1182/blood-2004-07-2681
Liang, X., Ding, Y., Zhang, Y., Tse, H.-F., and Lian, Q. (2014). Paracrine mechanisms of mesenchymal stem cell-based therapy: Current status and perspectives. Cell Transpl. 23 (9), 1045–1059. doi:10.3727/096368913X667709
Lin, S. C., and Talbot, P. (2014). “Stem Cells,” in Encyclopedia of Toxicology (Third Edition). Editor P. Wexler (Academic Press), 390–394. doi:10.1016/B978-0-12-386454-3.00088-9
Lin, Z., Owen, A. B., and Altman, R. B. (2004). Genetics. Genomic research and human subject privacy. Sci. (New York, N.Y.) 305 (5681), 183. doi:10.1126/science.1095019
Liu, L., Yoshioka, M., Nakajima, M., Ogasawara, A., Liu, J., Hasegawa, K., et al. (2014). Nanofibrous gelatin substrates for long-term expansion of human pluripotent stem cells. Biomaterials 35 (24), 6259–6267. doi:10.1016/j.biomaterials.2014.04.024
Liu, Q., Zhang, L., and Zhang, J. (2021). Induced pluripotent stem cell-derived neural progenitor cell transplantation promotes regeneration and functional recovery after post-traumatic stress disorder in rats. Biomed. Pharmacother. 133, 110981. doi:10.1016/j.biopha.2020.110981
Łos, M. J., Skubis, A., and Ghavami, S. (2019). “Chapter 2—stem cells,” in Stem cells and biomaterials for regenerative medicine. Editors M. J. Łos, A. Hudecki, and E. Wiecheć (Academic Press), 5–16. doi:10.1016/B978-0-12-812258-7.00002-2
Loya, K. (2014). “Chapter 11—stem cells,” in Handbook of pharmacogenomics and stratified medicine. Editor S. Padmanabhan (Academic Press), 207–231. doi:10.1016/B978-0-12-386882-4.00011-6
Lyu, X., Rowley, M. J., and Corces, V. G. (2018). Architectural proteins and pluripotency factors cooperate to orchestrate the transcriptional response of hESCs to temperature stress. Mol. Cell 71 (6), 940–955. e7. doi:10.1016/j.molcel.2018.07.012
Maekawa, M., Yamaguchi, K., Nakamura, T., Shibukawa, R., Kodanaka, I., Ichisaka, T., et al. (2011). Direct reprogramming of somatic cells is promoted by maternal transcription factor Glis1. Nature 474 (7350), 225–229. doi:10.1038/nature10106
Marędziak, M., Marycz, K., Tomaszewski, K. A., Kornicka, K., and Henry, B. M. (2016). The influence of aging on the regenerative potential of human adipose derived mesenchymal stem cells. Stem Cells Int. 2016, 2152435. doi:10.1155/2016/2152435
Mastrolia, I., Foppiani, E. M., Murgia, A., Candini, O., Samarelli, A. V., Grisendi, G., et al. (2019). Challenges in clinical development of mesenchymal stromal/stem cells: Concise review. Stem Cells Transl. Med. 8 (11), 1135–1148. doi:10.1002/sctm.19-0044
Masui, S., Nakatake, Y., Toyooka, Y., Shimosato, D., Yagi, R., Takahashi, K., et al. (2007). Pluripotency governed by Sox2 via regulation of Oct3/4 expression in mouse embryonic stem cells. Nat. Cell Biol. 9 (6), 625–635. doi:10.1038/ncb1589
Mathew, B., Ravindran, S., Liu, X., Torres, L., Chennakesavalu, M., Huang, C.-C., et al. (2019). Mesenchymal stem cell-derived extracellular vesicles and retinal ischemia-reperfusion. Biomaterials 197, 146–160. doi:10.1016/j.biomaterials.2019.01.016
Medvedev, S. P., Shevchenko, A. I., and Zakian, S. M. (2010). Induced pluripotent stem cells: Problems and advantages when applying them in regenerative medicine. Acta Naturae 2 (2), 18–27. doi:10.32607/20758251-2010-2-2-18-27
Meng, F., Xu, R., Wang, S., Xu, Z., Zhang, C., Li, Y., et al. (2020). Human umbilical cord-derived mesenchymal stem cell therapy in patients with covid-19: A phase 1 clinical trial. Signal Transduct. Target. Ther. 5 (1), 172. doi:10.1038/s41392-020-00286-5
Merimi, M., El-Majzoub, R., Lagneaux, L., Moussa Agha, D., Bouhtit, F., Meuleman, N., et al. (2021). The therapeutic potential of mesenchymal stromal cells for regenerative medicine: Current knowledge and future understandings. Front. Cell Dev. Biol. 9, 661532. doi:10.3389/fcell.2021.661532
Meyer, M. B., Benkusky, N. A., Sen, B., Rubin, J., and Pike, J. W. (2016). Epigenetic plasticity drives adipogenic and osteogenic differentiation of marrow-derived mesenchymal stem cells. J. Biol. Chem. 291 (34), 17829–17847. doi:10.1074/jbc.M116.736538
Miyagawa, S., Kainuma, S., Kawamura, T., Suzuki, K., Ito, Y., Iseoka, H., et al. (2022). Transplantation of IPSC-derived cardiomyocyte patches for ischemic cardiomyopathy. Japan: medRxiv, 12. doi:10.1101/2021.12.27.21268295
Miyazaki, T., Futaki, S., Suemori, H., Taniguchi, Y., Yamada, M., Kawasaki, M., et al. (2012). Laminin E8 fragments support efficient adhesion and expansion of dissociated human pluripotent stem cells. Nat. Commun. 3, 1236. doi:10.1038/ncomms2231
Mohamed-Ahmed, S., Fristad, I., Lie, S. A., Suliman, S., Mustafa, K., Vindenes, H., et al. (2018). Adipose-derived and bone marrow mesenchymal stem cells: A donor-matched comparison. Stem Cell Res. Ther. 9 (1), 168. doi:10.1186/s13287-018-0914-1
Montini, E., Cesana, D., Schmidt, M., Sanvito, F., Ponzoni, M., Bartholomae, C., et al. (2006). Hematopoietic stem cell gene transfer in a tumor-prone mouse model uncovers low genotoxicity of lentiviral vector integration. Nat. Biotechnol. 24 (6), 687–696. doi:10.1038/nbt1216
Moradi, S., Mahdizadeh, H., Šarić, T., Kim, J., Harati, J., Shahsavarani, H., et al. (2019). Research and therapy with induced pluripotent stem cells (iPSCs): Social, legal, and ethical considerations. Stem Cell Res. Ther. 10, 341. doi:10.1186/s13287-019-1455-y
Morales, I., Guzmán-Martínez, L., Cerda-Troncoso, C., Farías, G. A., and Maccioni, R. B. (2014). Neuroinflammation in the pathogenesis of Alzheimer’s disease. A rational framework for the search of novel therapeutic approaches. Front. Cell. Neurosci. 8, 112. doi:10.3389/fncel.2014.00112
Morita, K., Miyamoto, T., Fujita, N., Kubota, Y., Ito, K., Takubo, K., et al. (2007). Reactive oxygen species induce chondrocyte hypertrophy in endochondral ossification. J. Exp. Med. 204 (7), 1613–1623. doi:10.1084/jem.20062525
Mushahary, D., Spittler, A., Kasper, C., Weber, V., and Charwat, V. (2018). Isolation, cultivation, and characterization of human mesenchymal stem cells. Cytom. A 93 (1), 19–31. doi:10.1002/cyto.a.23242
Musiał-Wysocka, A., Kot, M., and Majka, M. (2019). The pros and cons of mesenchymal stem cell-based therapies. Cell Transpl. 28 (7), 801–812. doi:10.1177/0963689719837897
Nakagawa, M., Takizawa, N., Narita, M., Ichisaka, T., and Yamanaka, S. (2010). Promotion of direct reprogramming by transformation-deficient Myc. Proc. Natl. Acad. Sci. U. S. A. 107 (32), 14152–14157. doi:10.1073/pnas.1009374107
Nasiri, E., Alizadeh, A., Roushandeh, A. M., Gazor, R., Hashemi-Firouzi, N., and Golipoor, Z. (2019). Melatonin-pretreated adipose-derived mesenchymal stem cells efficeintly improved learning, memory, and cognition in an animal model of Alzheimer’s disease. Metab. Brain Dis. 34 (4), 1131–1143. doi:10.1007/s11011-019-00421-4
Nightingale, H., Kemp, K., Gray, E., Hares, K., Mallam, E., Scolding, N., et al. (2012). Changes in expression of the antioxidant enzyme SOD3 occur upon differentiation of human bone marrow-derived mesenchymal stem cells in vitro. Stem Cells Dev. 21 (11), 2026–2035. doi:10.1089/scd.2011.0516
Nyenwe, E. A., Jerkins, T. W., Umpierrez, G. E., and Kitabchi, A. E. (2011). Management of type 2 diabetes: Evolving strategies for the treatment of patients with type 2 diabetes. Metabolism. 60 (1), 1–23. doi:10.1016/j.metabol.2010.09.010
Okano, S., Yonemitsu, Y., Nagata, S., Sata, S., Onimaru, M., Nakagawa, K., et al. (2003). Recombinant Sendai virus vectors for activated T lymphocytes. Gene Ther. 10 (16), 1381–1391. doi:10.1038/sj.gt.3301998
Okita, K., Nakagawa, M., Hyenjong, H., Ichisaka, T., and Yamanaka, S. (2008). Generation of mouse induced pluripotent stem cells without viral vectors. Sci. (New York, N.Y.) 322 (5903), 949–953. doi:10.1126/science.1164270
Ong, S.-G., Lee, W. H., Zhou, Y., and Wu, J. C. (2018). Mining exosomal MicroRNAs from human-induced pluripotent stem cells-derived cardiomyocytes for cardiac regeneration. Methods Mol. Biol. 1733, 127–136. doi:10.1007/978-1-4939-7601-0_10
Ortiz, L. A., DuTreil, M., Fattman, C., Pandey, A. C., Torres, G., Go, K., et al. (2007). Interleukin 1 receptor antagonist mediates the antiinflammatory and antifibrotic effect of mesenchymal stem cells during lung injury. Proc. Natl. Acad. Sci. U. S. A. 104 (26), 11002–11007. doi:10.1073/pnas.0704421104
Park, E. T., Gum, J. R., Kakar, S., Kwon, S. W., Deng, G., and Kim, Y. S. (2008). Aberrant expression of SOX2 upregulates MUC5AC gastric foveolar mucin in mucinous cancers of the colorectum and related lesions. Int. J. Cancer 122 (6), 1253–1260. doi:10.1002/ijc.23225
Paul, A., Nayan, M., Khan, A. A., Shum-Tim, D., and Prakash, S. (2012). Angiopoietin-1-expressing adipose stem cells genetically modified with baculovirus nanocomplex: Investigation in rat heart with acute infarction. Int. J. Nanomedicine 7, 663–682. doi:10.2147/IJN.S26882
Pei, R., Feng, J., Zhang, Y., Sun, H., Li, L., Yang, X., et al. (2021). Host metabolism dysregulation and cell tropism identification in human airway and alveolar organoids upon SARS-CoV-2 infection. Protein Cell 12 (9), 717–733. doi:10.1007/s13238-020-00811-w
Petruzzelli, R., Christensen, D. R., Parry, K. L., Sanchez-Elsner, T., and Houghton, F. D. (2014). HIF-2α regulates NANOG expression in human embryonic stem cells following hypoxia and reoxygenation through the interaction with an oct-sox cis regulatory element. PLoS ONE 9 (10), e108309. doi:10.1371/journal.pone.0108309
Pittenger, M. F., Discher, D. E., Péault, B. M., Phinney, D. G., Hare, J. M., and Caplan, A. I. (2019). Mesenchymal stem cell perspective: Cell biology to clinical progress. NPJ Regen. Med. 4 (1), 22. doi:10.1038/s41536-019-0083-6
Poomani, M. S., Mariappan, I., Perumal, R., Regurajan, R., Muthan, K., and Subramanian, V. (2022). Mesenchymal stem cell (MSCs) therapy for ischemic heart disease: A promising frontier. Glob. Heart 17 (1), 19. doi:10.5334/gh.1098
Posfai, E., Schell, J. P., Janiszewski, A., Rovic, I., Murray, A., Bradshaw, B., et al. (2021). Evaluating totipotency using criteria of increasing stringency. Nat. Cell Biol. 23 (1), 49–60. doi:10.1038/s41556-020-00609-2
Prinsloo, E., Setati, M. M., Longshaw, V. M., and Blatch, G. L. (2009). Chaperoning stem cells: A role for heat shock proteins in the modulation of stem cell self-renewal and differentiation? Bioessays 31 (4), 370–377. doi:10.1002/bies.200800158
Priya, N., Sarcar, S., Majumdar, A. S., and SundarRaj, S. (2014). Explant culture: A simple, reproducible, efficient and economic technique for isolation of mesenchymal stromal cells from human adipose tissue and lipoaspirate. J. Tissue Eng. Regen. Med. 8 (9), 706–716. doi:10.1002/term.1569
Qiu, C., Ma, Y., Wang, J., Peng, S., and Huang, Y. (2010). Lin28-mediated post-transcriptional regulation of Oct4 expression in human embryonic stem cells. Nucleic Acids Res. 38 (4), 1240–1248. doi:10.1093/nar/gkp1071
Quevedo, H. C., Hatzistergos, K. E., Oskouei, B. N., Feigenbaum, G. S., Rodriguez, J. E., Valdes, D., et al. (2009). Allogeneic mesenchymal stem cells restore cardiac function in chronic ischemic cardiomyopathy via trilineage differentiating capacity. Proc. Natl. Acad. Sci. U. S. A. 106 (33), 14022–14027. doi:10.1073/pnas.0903201106
Rastegar, F., Shenaq, D., Huang, J., Zhang, W., Zhang, B.-Q., He, B.-C., et al. (2010). Mesenchymal stem cells: Molecular characteristics and clinical applications. World J. Stem Cells 2 (4), 67–80. doi:10.4252/wjsc.v2.i4.67
Reed, M. J., Meszaros, K., Entes, L. J., Claypool, M. D., Pinkett, J. G., Gadbois, T. M., et al. (2000). A new rat model of type 2 diabetes: The fat-fed, streptozotocin-treated rat. Metabolism. 49 (11), 1390–1394. doi:10.1053/meta.2000.17721
Riggs, J. W., Barrilleaux, B. L., Varlakhanova, N., Bush, K. M., Chan, V., and Knoepfler, P. S. (2013). Induced pluripotency and oncogenic transformation are related processes. Stem Cells Dev. 22 (1), 37–50. doi:10.1089/scd.2012.0375
Rissanen, T. T., Markkanen, J. E., Gruchala, M., Heikura, T., Puranen, A., Kettunen, M. I., et al. (2003). VEGF-D is the strongest angiogenic and lymphangiogenic effector among VEGFs delivered into skeletal muscle via adenoviruses. Circ. Res. 92 (10), 1098–1106. doi:10.1161/01.RES.0000073584.46059.E3
Rivera, T., Zhao, Y., Ni, Y., and Wang, J. (2020). Human-induced pluripotent stem cell culture methods under cGMP conditions. Curr. Protoc. Stem Cell Biol. 54 (1), e117. doi:10.1002/cpsc.117
Russell, K. C., Phinney, D. G., Lacey, M. R., Barrilleaux, B. L., Meyertholen, K. E., and O’Connor, K. C. (2010). In vitro high-capacity assay to quantify the clonal heterogeneity in trilineage potential of mesenchymal stem cells reveals a complex hierarchy of lineage commitment. Stem Cells Dayt. Ohio) 28 (4), 788–798. doi:10.1002/stem.312
Sabry, D., Marzouk, S., Zakaria, R., Ibrahim, H. A., and Samir, M. (2020). The effect of exosomes derived from mesenchymal stem cells in the treatment of induced type 1 diabetes mellitus in rats. Biotechnol. Lett. 42 (8), 1597–1610. doi:10.1007/s10529-020-02908-y
Salehinejad, P., Alitheen, N. B., Ali, A. M., Omar, A. R., Mohit, M., Janzamin, E., et al. (2012). Comparison of different methods for the isolation of mesenchymal stem cells from human umbilical cord Wharton’s jelly. Vitro Cell. Dev. Biol. Anim. 48 (2), 75–83. doi:10.1007/s11626-011-9480-x
Sandberg, J. O., Andersson, A., Eizirik, D. L., and Sandler, S. (1994). Interleukin-1 receptor antagonist prevents low dose streptozotocin induced diabetes in mice. Biochem. Biophys. Res. Commun. 202 (1), 543–548. doi:10.1006/bbrc.1994.1962
Schröder, K., Wandzioch, K., Helmcke, I., and Brandes, R. P. (2009). Nox4 acts as a switch between differentiation and proliferation in preadipocytes. Arterioscler. Thromb. Vasc. Biol. 29 (2), 239–245. doi:10.1161/ATVBAHA.108.174219
Seki, T., Yuasa, S., Oda, M., Egashira, T., Yae, K., Kusumoto, D., et al. (2010). Generation of induced pluripotent stem cells from human terminally differentiated circulating T cells. Cell Stem Cell 7 (1), 11–14. doi:10.1016/j.stem.2010.06.003
Sengupta, V., Sengupta, S., Lazo, A., Woods, P., Nolan, A., and Bremer, N. (2020). Exosomes derived from bone marrow mesenchymal stem cells as treatment for severe COVID-19. Stem Cells Dev. 29 (12), 747–754. doi:10.1089/scd.2020.0080
Sharma, A., Sances, S., Workman, M. J., and Svendsen, C. N. (2020). Multi-Lineage human iPSC-derived platforms for disease modeling and drug discovery. Cell Stem Cell 26 (3), 309–329. doi:10.1016/j.stem.2020.02.011
Shin, T.-H., Kim, H.-S., Choi, S. W., and Kang, K.-S. (2017). Mesenchymal stem cell therapy for inflammatory skin diseases: Clinical potential and mode of action. Int. J. Mol. Sci. 18 (2), 244. doi:10.3390/ijms18020244
Si, Y.-L., Zhao, Y.-L., Hao, H.-J., Fu, X.-B., and Han, W.-D. (2011). MSCs: Biological characteristics, clinical applications and their outstanding concerns. Ageing Res. Rev. 10 (1), 93–103. doi:10.1016/j.arr.2010.08.005
Sidney, L. E., Branch, M. J., Dunphy, S. E., Dua, H. S., and Hopkinson, A. (2014). Concise review: Evidence for CD34 as a common marker for diverse progenitors. Stem Cells Dayt. Ohio) 32 (6), 1380–1389. doi:10.1002/stem.1661
Singh, V. K., Kalsan, M., Kumar, N., Saini, A., and Chandra, R. (2015). Induced pluripotent stem cells: Applications in regenerative medicine, disease modeling, and drug discovery. Front. Cell Dev. Biol. 3, 2. doi:10.3389/fcell.2015.00002
Spathopoulou, A., Edenhofer, F., and Fellner, L. (2022). Targeting α-synuclein in Parkinson’s disease by induced pluripotent stem cell models. Front. Neurol. 12, 786835. doi:10.3389/fneur.2021.786835
Sridharan, S., Varghese, R., Venkatraj, V., and Datta, A. (2017). Hypoxia stress response pathways: Modeling and targeted therapy. IEEE J. Biomed. Health Inf. 21 (3), 875–885. doi:10.1109/JBHI.2016.2559460
Stadtfeld, M., Nagaya, M., Utikal, J., Weir, G., and Hochedlinger, K. (2008). Induced pluripotent stem cells generated without viral integration. Sci. (New York, N.Y.) 322 (5903), 945–949. doi:10.1126/science.1162494
Stenderup, K., Justesen, J., Clausen, C., and Kassem, M. (2003). Aging is associated with decreased maximal life span and accelerated senescence of bone marrow stromal cells. Bone 33 (6), 919–926. doi:10.1016/j.bone.2003.07.005
Strem, B. M., Hicok, K. C., Zhu, M., Wulur, I., Alfonso, Z., Schreiber, R. E., et al. (2005). Multipotential differentiation of adipose tissue-derived stem cells. Keio J. Med. 54 (3), 132–141. doi:10.2302/kjm.54.132
Sun, Y., Shi, H., Yin, S., Ji, C., Zhang, X., Zhang, B., et al. (2018). Human mesenchymal stem cell derived exosomes alleviate type 2 diabetes mellitus by reversing peripheral insulin resistance and relieving β-cell destruction. ACS Nano 12 (8), 7613–7628. doi:10.1021/acsnano.7b07643
Sutermaster, B. A., and Darling, E. M. (2019). Considerations for high-yield, high-throughput cell enrichment: Fluorescence versus magnetic sorting. Sci. Rep. 9, 227. doi:10.1038/s41598-018-36698-1
Takahashi, K., and Yamanaka, S. (2006). Induction of pluripotent stem cells from mouse embryonic and adult fibroblast cultures by defined factors. Cell 126 (4), 663–676. doi:10.1016/j.cell.2006.07.024
Takeuchi, R., Katagiri, W., Endo, S., and Kobayashi, T. (2019). Exosomes from conditioned media of bone marrow-derived mesenchymal stem cells promote bone regeneration by enhancing angiogenesis. PLoS ONE 14 (11), e0225472. doi:10.1371/journal.pone.0225472
Tang, C., Lee, A. S., Volkmer, J.-P., Sahoo, D., Nag, D., Mosley, A. R., et al. (2011). An antibody against SSEA-5 glycan on human pluripotent stem cells enables removal of teratoma-forming cells. Nat. Biotechnol. 29 (9), 829–834. doi:10.1038/nbt.1947
Telpalo-Carpio, S., Aguilar-Yañez, J., Gonzalez-Garza, M., Cruz-Vega, D., and Moreno-Cuevas, J. (2013). iPS cells generation: An overview of techniques and methods. J. Stem Cells Regen. Med. 9 (1), 2–8.
Tremain, N., Korkko, J., Ibberson, D., Kopen, G. C., DiGirolamo, C., and Phinney, D. G. (2001). MicroSAGE analysis of 2, 353 expressed genes in a single cell-derived colony of undifferentiated human mesenchymal stem cells reveals mRNAs of multiple cell lineages. Stem Cells Dayt. Ohio) 19 (5), 408–418. doi:10.1634/stemcells.19-5-408
Trivedi, P., and Hematti, P. (2008). Derivation and immunological characterization of mesenchymal stromal cells from human embryonic stem cells. Exp. Hematol. 36 (3), 350–359. doi:10.1016/j.exphem.2007.10.007
Trivedi, P., and Hematti, P. (2007). Simultaneous generation of CD34+ primitive hematopoietic cells and CD73+ mesenchymal stem cells from human embryonic stem cells cocultured with murine OP9 stromal cells. Exp. Hematol. 35 (1), 146–154. doi:10.1016/j.exphem.2006.09.003
Tsivion-Visbord, H., Perets, N., Sofer, T., Bikovski, L., Goldshmit, Y., Ruban, A., et al. (2020). Mesenchymal stem cells derived extracellular vesicles improve behavioral and biochemical deficits in a phencyclidine model of schizophrenia. Transl. Psychiatry 10, 305. doi:10.1038/s41398-020-00988-y
Ullah, M., Liu, D. D., and Thakor, A. S. (2019). Mesenchymal stromal cell homing: Mechanisms and strategies for improvement. IScience 15, 421–438. doi:10.1016/j.isci.2019.05.004
Ura, T., Okuda, K., and Shimada, M. (2014). Developments in viral vector-based vaccines. Vaccines 2 (3), 624–641. doi:10.3390/vaccines2030624
Wang, X., and Dai, J. (2010). Concise review: Isoforms of OCT4 contribute to the confusing diversity in stem cell biology. Stem Cells Dayt. Ohio) 28 (5), 885–893. doi:10.1002/stem.419
Wang, X., Gu, H., Qin, D., Yang, L., Huang, W., Essandoh, K., et al. (2015). Exosomal miR-223 contributes to mesenchymal stem cell-elicited cardioprotection in polymicrobial sepsis. Sci. Rep. 5, 13721. doi:10.1038/srep13721
Warren, L., Manos, P. D., Ahfeldt, T., Loh, Y.-H., Li, H., Lau, F., et al. (2010). Highly efficient reprogramming to pluripotency and directed differentiation of human cells with synthetic modified mRNA. Cell Stem Cell 7 (5), 618–630. doi:10.1016/j.stem.2010.08.012
Wei, F., Kong, D., Li, T., Li, A., Tan, Y., Fang, J., et al. (2021). Efficacy and safety of umbilical cord mesenchymal stem cells for the treatment of patients with COVID-19. Clinics 76, e2604. doi:10.6061/clinics/2021/e2604
Weissman, I. L. (2000). Stem cells: Units of development, units of regeneration, and units in evolution. Cell 100 (1), 157–168. doi:10.1016/s0092-8674(00)81692-x
Weng, S., Shao, Y., Chen, W., and Fu, J. (2016). Mechanosensitive subcellular rheostasis drives emergent single-cell mechanical homeostasis. Nat. Mat. 15 (9), 961–967. doi:10.1038/nmat4654
Wilson-Fritch, L., Burkart, A., Bell, G., Mendelson, K., Leszyk, J., Nicoloro, S., et al. (2003). Mitochondrial biogenesis and remodeling during adipogenesis and in response to the insulin sensitizer rosiglitazone. Mol. Cell. Biol. 23 (3), 1085–1094. doi:10.1128/MCB.23.3.1085-1094.2003
Xiao, M., Li, J., Li, W., Wang, Y., Wu, F., Xi, Y., et al. (2016). MicroRNAs activate gene transcription epigenetically as an enhancer trigger. RNA Biol. 14 (10), 1326–1334. doi:10.1080/15476286.2015.1112487
Xing, D., Wang, K., Wu, J., Zhao, Y., Liu, W., Li, J. J., et al. (2021). Clinical-grade human embryonic stem cell-derived mesenchymal stromal cells ameliorate the progression of osteoarthritis in a rat model. Molecules 26 (3), 604. doi:10.3390/molecules26030604
Yamada, S., Noda, T., Okabe, K., Yanagida, S., Nishida, M., and Kanda, Y. (2022). SARS-CoV-2 induces barrier damage and inflammatory responses in the human iPSC-derived intestinal epithelium. J. Pharmacol. Sci. 149 (3), 139–146. doi:10.1016/j.jphs.2022.04.010
Yang, H., Yang, H., Xie, Z., Wei, L., and Bi, J. (2013). Systemic transplantation of human umbilical cord derived mesenchymal stem cells-educated T regulatory cells improved the impaired cognition in AβPPswe/PS1dE9 transgenic mice. PLOS ONE 8 (7), e69129. doi:10.1371/journal.pone.0069129
Yang L, L., Han, Y., Nilsson-Payant, B. E., Gupta, V., Wang, P., Duan, X., et al. (2020). A human pluripotent stem cell-based platform to study SARS-CoV-2 tropism and model virus infection in human cells and organoids. Cell Stem Cell 27 (1), 125–136. e7. doi:10.1016/j.stem.2020.06.015
Yang, L., Froio, R. M., Sciuto, T. E., Dvorak, A. M., Alon, R., and Luscinskas, F. W. (2005). ICAM-1 regulates neutrophil adhesion and transcellular migration of TNF-α-activated vascular endothelium under flow. Blood 106 (2), 584–592. doi:10.1182/blood-2004-12-4942
Yang X, X., Meng, Y., Han, Z., Ye, F., Wei, L., and Zong, C. (2020). Mesenchymal stem cell therapy for liver disease: Full of chances and challenges. Cell Biosci. 10 (1), 123. doi:10.1186/s13578-020-00480-6
Yellowley, C. (2013). CXCL12/CXCR4 signaling and other recruitment and homing pathways in fracture repair. Bonekey Rep. 2, 300. doi:10.1038/bonekey.2013.34
Yoon, J. H., Roh, E. Y., Shin, S., Jung, N. H., Song, E. Y., Chang, J. Y., et al. (2013). Comparison of explant-derived and enzymatic digestion-derived MSCs and the growth factors from Wharton’s jelly. Biomed. Res. Int. 2013, 428726. doi:10.1155/2013/428726
Yoshida, S., Miyagawa, S., Fukushima, S., Kawamura, T., Kashiyama, N., Ohashi, F., et al. (2018). Maturation of human induced pluripotent stem cell-derived cardiomyocytes by soluble factors from human mesenchymal stem cells. Mol. Ther. 26 (11), 2681–2695. doi:10.1016/j.ymthe.2018.08.012
Yu, J., Hu, K., Smuga-Otto, K., Tian, S., Stewart, R., Slukvin, I. I., et al. (2009). Human induced pluripotent stem cells free of vector and transgene sequences. Sci. (New York, N.Y.) 324 (5928), 797–801. doi:10.1126/science.1172482
Yu, J., Vodyanik, M. A., Smuga-Otto, K., Antosiewicz-Bourget, J., Frane, J. L., Tian, S., et al. (2007). Induced pluripotent stem cell lines derived from human somatic cells. Science 318 (5858), 1917–1920. doi:10.1126/science.1151526
Yun, H.-M., Kim, H. S., Park, K.-R., Shin, J. M., Kang, A. R., ll Lee, K., et al. (2013). Placenta-derived mesenchymal stem cells improve memory dysfunction in an Aβ1–42-infused mouse model of Alzheimer’s disease. Cell Death Dis. 4 (12), e958. doi:10.1038/cddis.2013.490
Yusa, K., Rad, R., Takeda, J., and Bradley, A. (2009). Generation of transgene-free induced pluripotent mouse stem cells by the piggyBac transposon. Nat. Methods 6 (5), 363–369. doi:10.1038/nmeth.1323
Zhang, L., Dong, Z., and Zhang, J. (2020). Immunomodulatory role of mesenchymal stem cells in Alzheimer’s disease. Life Sci. 246, 117405. doi:10.1016/j.lfs.2020.117405
Zhang, W., Wang, Y., and Kong, Y. (2019). Exosomes derived from mesenchymal stem cells modulate miR-126 to ameliorate hyperglycemia-induced retinal inflammation via targeting HMGB1. Invest. Ophthalmol. Vis. Sci. 60 (1), 294–303. doi:10.1167/iovs.18-25617
Zhao J, J., Li, X., Hu, J., Chen, F., Qiao, S., Sun, X., et al. (2019). Mesenchymal stromal cell-derived exosomes attenuate myocardial ischaemia-reperfusion injury through miR-182-regulated macrophage polarization. Cardiovasc. Res. 115 (7), 1205–1216. doi:10.1093/cvr/cvz040
Zhao Q, Q., Zhang, L., Wei, Y., Yu, H., Zou, L., Huo, J., et al. (2019). Systematic comparison of hUC-MSCs at various passages reveals the variations of signatures and therapeutic effect on acute graft-versus-host disease. Stem Cell Res. Ther. 10 (1), 354. doi:10.1186/s13287-019-1478-4
Zhao T, T., Sun, F., Liu, J., Ding, T., She, J., Mao, F., et al. (2019). Emerging role of mesenchymal stem cell-derived exosomes in regenerative medicine. Curr. Stem Cell Res. Ther. 14 (6), 482–494. doi:10.2174/1574888X14666190228103230
Zhao, Y., Gillen, J. R., Harris, D. A., Kron, I. L., Murphy, M. P., and Lau, C. L. (2014). Treatment with placenta-derived mesenchymal stem cells mitigates development of bronchiolitis obliterans in a murine model. J. Thorac. Cardiovasc. Surg. 147 (5), 1668–1677. e5. doi:10.1016/j.jtcvs.2013.09.041
Zhou, L., Xu, Z., Castiglione, G. M., Soiberman, U. S., Eberhart, C. G., and Duh, E. J. (2020). ACE2 and TMPRSS2 are expressed on the human ocular surface, suggesting susceptibility to SARS-CoV-2 infection. Ocul. Surf. 18 (4), 537–544. doi:10.1016/j.jtos.2020.06.007
Zhou, W., and Freed, C. R. (2009). Adenoviral gene delivery can reprogram human fibroblasts to induced pluripotent stem cells. Stem Cells 27 (11), 2667–2674. doi:10.1002/stem.201
Zou, X., Li, H., Chen, L., Baatrup, A., Bünger, C., and Lind, M. (2004). Stimulation of porcine bone marrow stromal cells by hyaluronan, dexamethasone and rhBMP-2. Biomaterials 25 (23), 5375–5385. doi:10.1016/j.biomaterials.2003.12.041
Keywords: mesenchymal stem cells, induced pluripotent stem cells, therapeutic applications, SARS-CoV-2, COVID-19
Citation: Thanaskody K, Jusop AS, Tye GJ, Wan Kamarul Zaman WS, Dass SA and Nordin F (2022) MSCs vs. iPSCs: Potential in therapeutic applications. Front. Cell Dev. Biol. 10:1005926. doi: 10.3389/fcell.2022.1005926
Received: 28 July 2022; Accepted: 21 October 2022;
Published: 02 November 2022.
Edited by:
Prasad S. Koka, Biomedical Research Institute of Southern California, United StatesReviewed by:
Rohan Bhattacharya, Duke University, United StatesCopyright © 2022 Thanaskody, Jusop, Tye, Wan Kamarul Zaman, Dass and Nordin. This is an open-access article distributed under the terms of the Creative Commons Attribution License (CC BY). The use, distribution or reproduction in other forums is permitted, provided the original author(s) and the copyright owner(s) are credited and that the original publication in this journal is cited, in accordance with accepted academic practice. No use, distribution or reproduction is permitted which does not comply with these terms.
*Correspondence: Fazlina Nordin, bm9yZGluZkBwcHVrbS51a20uZWR1Lm15
Disclaimer: All claims expressed in this article are solely those of the authors and do not necessarily represent those of their affiliated organizations, or those of the publisher, the editors and the reviewers. Any product that may be evaluated in this article or claim that may be made by its manufacturer is not guaranteed or endorsed by the publisher.
Research integrity at Frontiers
Learn more about the work of our research integrity team to safeguard the quality of each article we publish.