- 1Department of Molecular and Clinical Medicine, Wallenberg Laboratory, Institute of Medicine, Sahlgrenska Academy, University of Gothenburg, Gothenburg, Sweden
- 2Wallenberg Centre for Molecular and Translational Medicine, University of Gothenburg, Gothenburg, Sweden
- 3Region Vaestra Goetaland, Department of Clinical Physiology, Sahlgrenska University Hospital, Gothenburg, Sweden
- 4The Lundberg Laboratory for Diabetes Research, Department of Molecular and Clinical Medicine, Sahlgrenska Academy, University of Gothenburg, Gothenburg, Sweden
- 5Metabolic Disease, Evotec International GmbH, Göttingen, Germany
- 6Division of Cardiovascular Medicine, Department of Medicine Solna, Karolinska Institutet, Stockholm, Sweden
- 7Center for Molecular Medicine, Karolinska Institutet, Stockholm, Sweden
The prevalence of obesity and metabolic diseases continues to rise, which has led to an increased interest in studying adipose tissue to elucidate underlying disease mechanisms. The use of genetic mouse models has been critical for understanding the role of specific genes for adipose tissue function and the tissue’s impact on other organs. However, mouse adipose tissue displays key differences to human fat, which has led, in some cases, to the emergence of some confounding concepts in the adipose field. Such differences include the depot-specific characteristics of visceral and subcutaneous fat, and divergences in thermogenic fat phenotype between the species. Adipose tissue characteristics may therefore not always be directly compared between species, which is important to consider when setting up new studies or interpreting results. This mini review outlines our current knowledge about the cell biological differences between human and mouse adipocytes and fat depots, highlighting some examples where inadequate knowledge of species-specific differences can lead to confounding results, and presenting plausible anatomic explanations that may underlie the differences. The article thus provides critical insights and guidance for researchers working primarily with only human or mouse fat tissue, and may contribute to new ideas or concepts in the important and evolving field of adipose biology.
Introduction
The mammalian white adipose tissue is essential for whole body lipid metabolism by storing excess meal-derived lipids as triglycerides and mobilizing them as fatty acids between meals. However, upon obesity, these functions are undermined, leading to lipids accumulating in the circulation and in peripheral organs instead, and thereby causing insulin resistance and ultimately metabolic disease (Morigny et al., 2021). The study of obesity and its detrimental consequences requires multiorgan model systems, where this complex inter-organ crosstalk can be studied and tested. The most commonly used model is rodents, and especially mice. Genetically modified mice have for example been key for improving our understanding of how obesity affects adipose tissue cellularity (Wang et al., 2013), how changes in fat cell (adipocyte) number versus size impact systemic metabolism (Kim et al., 2007; Wang et al., 2008), and for discovering genes that affect body weight and/or systemic insulin resistance, with the best-known example being the discovery of the obese or leptin gene (Zhang et al., 1994). However, when the ultimate goal is to translate these findings to human pathophysiology, a clearer understanding of species differences, and the potential limitations of these murine model systems, is needed. The fact remains that many aspects of adipocyte biology differ significantly between mice and humans, which is not always acknowledged. This mini review aims to outline some of the most important aspects of how human and mouse visceral and subcutaneous adipose depots differ, highlighting how inadequate knowledge of species differences easily can lead to confounding concepts, and how to avoid such confusion. We hope it can serve as a guide for researchers new to the adipose field who mostly work solely with either human or mouse material. We also think that acknowledging species differences could bring new ideas about adipose biology and help us identify which adipocyte characteristics drive the development of adipose tissue dysfunction during obesity, and which adipocyte characteristics merely correlating with it.
Divergent characteristics of mouse and human adipose depots
The basis of all confusion—Different fat pad organization
In general, the white adipose tissue (WAT) depots can, for both mice and humans, be divided into two major anatomical regions, subcutaneous and visceral fat. Subcutaneous fat is found just beneath the skin, and visceral fat is located within the central body cavity. Whereas obesity is associated with an overall negative impact on health and increased mortality, the expansion of subcutaneous fat in humans has been shown to be associated with beneficial or neutral effects on metabolism, whereas excess visceral fat correlates with both metabolic and cardiovascular risk factors (Goodpaster et al., 1997; Lotta et al., 2017). The subcutaneous fat is therefore considered the physiological site for lipid storage, that for lean humans typically comprises the majority, around 80%, of the total body fat (Sakers et al., 2022).
These two major fat depots can be further subdivided into specific fat pads, and here confusion may arise. In mice, subcutaneous fat is mainly found in the posterior inguinal WAT (iWAT) depot and in the anterior axillary region (Figure 1). Humans similarly develop subcutaneous fat in the abdominal region (abWAT) but also in the femoral (thigh) and gluteal (bum) region, the latter especially in women (Chusyd et al., 2016). Human abdominal subcutaneous fat can further be divided into a superficial and a deep subcutaneous layer, whereas mice most likely lack this anatomical division (Chusyd et al., 2016). Despite these slight differences, most researchers agree that the majority of results from mouse iWAT and human abWAT are largely comparable. It is important to note that the subcutaneous fat is the major physiological storage location for meal-derived lipids, and only when its expansion capacity is exceeded do lipids start to accumulate in visceral fat. This is clearly illustrated in patients with almost any kind of lipodystrophy, where genetically induced loss of subcutaneous fat, especially of that in the gluteal and femoral depots, recapitulates most features of the metabolic syndrome, whereas their amount of visceral fat is not correlated to disease severity (Mann and Savage, 2019).
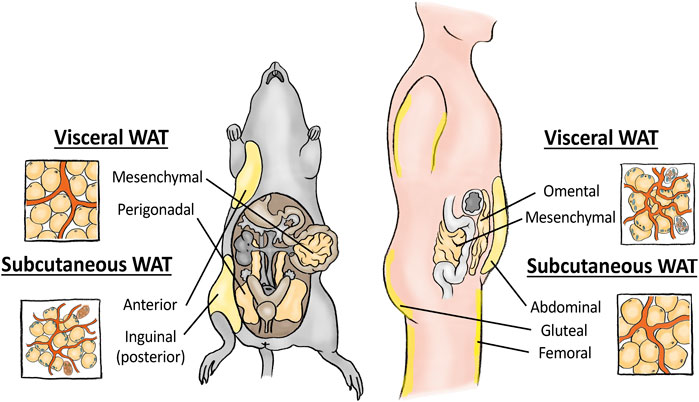
FIGURE 1. Schematic showing the most important visceral and subcutaneous white adipose tissue depots in mice (left) and humans (right), respectively. Enlarged simplified drawings of the respective tissue structures are merely indicative of the relative differences between depots, and do not include all details of the tissue itself.
For visceral fat pads, the direct comparison between species is less correct. In mice, the most well studied visceral fat depot is the peri-gonadal white adipose tissue (gWAT, is also sometimes termed epididymal fat in males, and periovarian fat in females), which dominates their central cavity (Figure 1). Mice also have peri-renal, some mesenteric and some omental visceral WAT, but the latter two mouse fat pads are of negligible size and their impact on systemic metabolism could be questioned (Vitali et al., 2012). The gWAT has therefore remained the best studied fat pad and is in rodent studies often referred to just as visceral fat. Importantly, humans almost completely lack this fat pad, and instead organize most of their visceral fat into the omental WAT (oWAT), with mesenteric and peri-renal fat being the other two dominating human visceral fat pads (Chusyd et al., 2016). Accumulation of human oWAT has in numerous studies been shown to associate with metabolic disease and increased risk for developing comorbidities such as insulin resistance, dyslipidaemia, type-2 diabetes and cardiovascular disease (Zhang et al., 2015; Chait and den Hartigh, 2020). In mice, the expansion of visceral gWAT fat is similarly associated with metabolic dysfunction and whole-body insulin resistance (Gabriely et al., 2002), and these correlations could have given rise to the misguiding notion that these two visceral fat pads are comparable. However, these fat pads exhibit numerous important differences. The human oWAT is a large flat adipose tissue layer hanging down as a “curtain” or an elastic apron from the stomach to the liver, floating on top of the small and large intestine and other visceral organs (Di Nicola, 2019). Despite its tight correlation with metabolic disease, its main physiological role in addition to lipid storage is to control anatomical infection and isolate wounds (Di Nicola, 2019). The tissue in lean, healthy patients therefore contains high levels of immune cells, including macrophages, B- and T-cells, often found concentrated to milky spots within the tissue. The second important human visceral fat region is the mesenteric fat, that wraps around the intestine (Zwick et al., 2018). In both humans and mice, the mesenteric fat is thought to function similarly to the omentum, storing lipids and upholding the intestinal barrier. However, this fat is small in mice, and difficult to extract in humans due to its high degree of vascularization, and thus remains understudied, as does peri-renal white adipose tissue for both species (Wernstedt Asterholm et al., 2014; Chusyd et al., 2016). Instead, when studying visceral fat, mouse gWAT is often considered to correspond to human oWAT due to their abdominal locations. However, gWAT is located adjacent to the mouse sex organs and as such maintains none of the immunomodulatory functions of human omental and mesenteric fat, but is rather thought to function mainly as a cushion for the mouse reproductive organs in addition to storing lipids (Chusyd et al., 2016). Moreover, while the human visceral oWAT drains directly to the portal circulation, mouse gWAT drains to the systemic circulation, which greatly impacts the influence that respective fat depot has on the liver (Rytka et al., 2011). In addition, lipid tracing experiments in humans have shown that while human oWAT directly drains to the liver, lipids released from this fat pad constitute only a small proportion of the total lipid released by WAT, suggesting that oWAT-derived pro-inflammatory adipokines rather than lipid release may mediate the negative effects of having excess visceral fat (Jensen, 2008; Rytka et al., 2011). For more detailed information on the specific anatomical fat pad organizations and their differences between mice and men we refer to two recent excellent reviews (Chusyd et al., 2016; Di Nicola, 2019), whereas the focus below will be on the more cell biological differences that exists between these two major fat pads in mice and humans.
Human and mouse fat pads show opposite patterns of adipocyte size
During weight gain, WAT expands both as a result of resident adipocytes growing in size, leading to a more hypertrophic tissue, but also via the emergence of new fat cells through the differentiation of tissue-resident progenitor cells termed pre-adipocytes, resulting in a more hyperplastic adipose tissue. Whereas adipocyte hypertrophy has been suggested to dominate the initial phase of human weight gain (Salans et al., 1971; Spalding et al., 2008), elegant pulse-chase studies showed new adipocytes being formed within the mouse visceral gWAT fat after approximately 8 weeks of high fat diet feeding (Wang et al., 2013). Mouse subcutaneous iWAT seemed to expand only via hypertrophy, analogous to the human tissue. Importantly, these two modes of tissue expansion, hypertrophy and hyperplasia, associate very differently with insulin resistance and other metabolic risk factors. Adipocyte hypertrophy and increased adipocyte size have by numerous studies been identified as one of the best markers of a dysfunctional WAT, associated with an increased risk for development of insulin resistance, dyslipidemia and metabolic disease even in non-obese individuals (Acosta et al., 2016; Verboven et al., 2018; Rawshani et al., 2020). Transgenic mice that develop hypertrophic obesity, such as the ob/ob and db/db mice, typically develop metabolic dysfunctions, whereas severely obese mice with hyperplastic fat containing many small adipocytes instead are able to remain relatively healthy (Shepherd et al., 1993; Boucher et al., 2002; Kim et al., 2007; Kim et al., 2014). Some notable exceptions to this exist such as when collagen 6 is knocked out, which causes severely hypertrophic obesity without apparent metabolic defects, potentially because the reduced levels of restraining collagen in the adipocytes’ microenvironment allow them to take up and store more lipid (Khan et al., 2009). In addition to large hypertrophic adipocytes most often being an adverse marker of metabolic health, mouse visceral adipocytes are also larger than subcutaneous cells (Johnson and Hirsch, 1972), contributing to the wrongful conception that absolute adipocyte size is a determinant of adipocyte dysfunction. It is therefore important to recognize that in humans the adipocyte size relationship between fat pads is the opposite, with the more beneficial abdominal subcutaneous fat displaying larger fat cell sizes, especially in lean individuals, than the human visceral oWAT (Belligoli et al., 2019; O'Connell et al., 2010). Moreover, human femoral subcutaneous adipocytes, which are considered to possess the most beneficial impact on systemic metabolism, are even larger than abWAT from the same subject, further contradicting the notion of a large absolute size being detrimental for adipocyte functionality (Tchoukalova et al., 2008; Vogel et al., 2019). Interestingly, several studies comparing adipocyte size in humans with a wide range of body weights show that whereas human subcutaneous abWAT display larger cell sizes than that of visceral oWAT in healthy lean subjects, the relative increase in size/volume of visceral oWAT cells is larger during weight gain (Verboven et al., 2018; O'Connell et al., 2010; Suarez-Cuenca et al., 2021). In rodents, this pattern seems to be the same (Gabriely et al., 2002). Thus, the relative growth of adipocytes, as compared to cells from the same anatomical location of controls, may represent a better marker of metabolic dysfunction than absolute adipocyte size per se. However, taking the above differences in account, most generalizations from comparing subcutaneous to visceral adipocyte size between species should on the whole be avoided.
Adipocyte characteristics primarily follow adipocyte size, not anatomical location
The species-specific pattern of adipocyte sizes has far more important consequences for adipocyte biology than one realizes at first glance. Several studies have confirmed (directly or indirectly) that the larger subcutaneous adipocyte size in humans is associated with a higher degree of adipocyte insulin resistance (Virtanen et al., 2002; Lundgren et al., 2004; Laviola et al., 2006) and lower mitochondrial content (Kraunsoe et al., 2010) in human abWAT as compared to oWAT. Exceptions do exist and the results may depend on the degree of obesity or measurement method. Human abWAT also takes up glucose less efficiently than oWAT, as evaluated by PET imaging or uptake of radioactive glucose (Stolic et al., 2002; Lundgren et al., 2004; Christen et al., 2010). This is the opposite to what several studies have shown for mouse fat pads (Wueest et al., 2012; Schottl et al., 2015a; Schottl et al., 2015b). Again, notable exceptions do exist, and it is unclear what underlies these discrepancies (Macotela et al., 2009). These species differences imply that adipocyte size might be a more important determinant of some of these characteristics than anatomical location. In addition, some of these adipocyte characteristics may not have adverse effects on adipocyte function in humans, as expansion of the more (insulin resistant) human subcutaneous fat in general is not considered to have adverse effects on systemic metabolic health (Lotta et al., 2017). One of the underlying mechanisms for the altered subcutaneous insulin sensitivity could be that as the adipocytes accumulate increased amounts of triglycerides in their lipid droplets, their plasma membrane becomes somewhat stretched, potentially influencing local concentrations of signalling receptors and thereby influencing insulin receptor dimerization upon ligand binding (Livingston et al., 1984). This may of course be context dependent and thus remains highly debated. It should also be noted that some studies suggest that the various signalling pathways downstream of the insulin receptor could be differentially affected by obesity, leading to some pathways being more efficiently blocked by insulin resistance compared to others (Farnier et al., 2003). Thus, whether adipocyte insulin sensitivity is assessed by measuring insulin-stimulated glucose uptake, or as insulin-induced receptor phosphorylation may lead to different results (Lundgren et al., 2004; Laviola et al., 2006).
Importantly, in adipocytes, insulin signalling does not only regulate glucose uptake, but also functions as the main inhibitor of fatty acid release from adipocytes by limiting intracellular lipolysis. Therefore, adipocyte insulin resistance can also be measured as sensitivity to insulin-mediated inhibition of lipolysis. Again, reports suggest that human visceral oWAT adipocytes are more insulin sensitive than subcutaneous adipocytes in this regard as well, and that subcutaneous adipocytes therefore have a higher basal lipolytic activity compared to oWAT cells from matched individuals (Arner, 1995; Hoffstedt et al., 1997; van Harmelen et al., 2002). Another important example of depot and species differences is the differential regulation of catecholamine-induced adipocyte lipolysis (Tchernof et al., 2006; Lafontan and Langin, 2009). Different adipose tissue depots express varying levels of the pro-lipolytic beta1, beta2 and beta3 adrenergic receptors, as well as the antilipolytic alpha2A adrenergic receptor, and their relative balance is determinant for each depot’s lipolytic activity. Human adipocytes from the subcutaneous (especially gluteal and femoral) depots have a higher expression of antilipolytic alpha2A adrenergic receptors compared to human visceral depots, and thus display lower catecholamine-induced lipolysis (Mauriege et al., 1987; Castan et al., 1993; Lafontan and Berlan, 1995). Rodent adipocytes on the other hand express very few alpha2A adrenergic receptors in any of their depots (Castan et al., 1994), and their catecholamine-induced lipolysis is mainly mediated via activation of the pro-lipolytic beta3 adrenergic receptor (Lafontan, 1994). In human adipocytes the beta3 adrenergic receptor is expressed at very low levels, and instead catecholamine-induced lipolytic activation is mediated via the beta1 and beta2 adrenergic receptors (Tavernier et al., 1996). Taken together, these results highlight key differences between human and mouse adipose tissue depot characteristics with regards to insulin sensitivity and control of lipolysis.
Another important characteristic that is influenced by adipocyte size is vascular density when measured per unit volume. Increased adipocyte size is inversely correlated to vascular density, as larger cell sizes lead to resident capillaries being pushed further apart and the inter-capillary distance thereby increasing. Obesity-induced adipocyte enlargement therefore leads to a lower capillary density in all human and mouse fat pads, which subsequently can cause hypoxia and WAT fibrosis (Belligoli et al., 2019; Lempesis et al., 2020). However, due to the intrinsic differences in adipocyte size discussed above, there is already a difference in the basal capillary density between fat pads. Again, due to human abWAT adipocytes being larger than visceral oWAT adipocytes, the human subcutaneous fat has a lower vascular density (Ledoux et al., 2008; Villaret et al., 2010; Belligoli et al., 2019), with the relationship between the mouse depots being the opposite (Song et al., 2016). Decreased oxygen tension, a direct measurement of hypoxia, has been suggested to tightly follow vascular density in mice, whereas the occurrence of WAT hypoxia in human fat has been harder to measure, especially for oWAT (Cifarelli et al., 2020; Lempesis et al., 2020). In summary, by comparing measurements from human fat pads, displaying the opposite pattern of adipocyte sizes than in mice, we could potentially be able to differentiate between adipose tissue characteristics that directly promote metabolic disease, and those that merely correlate with it.
Different thermogenic pattern between the two species
In addition to WAT, rodents also possess brown adipose tissue (BAT), which, as the name alludes to, is more brown-looking and is a thermogenic fat type that specializes in dissipating the energy from lipids and other nutrients as heat instead of solely storing them. BAT is found primarily in the interscapular region in mice, and gets its colour from its high mitochondrial content and vascularization. In addition to bona fide BAT can mouse subcutaneous fat, and to a much lesser extent visceral gWAT, upon chronic stimulation of cold or adrenergic agonists acquire brown-like features, with increased lipid oxidation and thermogenic capacity (Vitali et al., 2012; Herz and Kiefer, 2019). These thermogenic cells can arise both from resident progenitor cells and via transdifferentiation of white adipocytes, and are referred to as brite/beige adipocytes due to their intrinsic differences to bona fide brown adipocytes (Maurer et al., 2021). In more recent years have human adults also been shown to possess thermogenic adipocytes, found predominantly concentrated around larger vessels such as in the supraclavicular, paravertebral, periaortic and axillary regions (Cypess et al., 2009; van Marken Lichtenbelt et al., 2009; Virtanen et al., 2009). In fact, human supraclavicular fat possesses an equally high thermogenic capacity as mouse BAT when expressed per mitochondrion (Porter et al., 2016). However, when measuring the expression of thermogenic and mitochondrial genes within the major human WAT depots, the anatomic expression pattern of these genes is again the opposite from that of mice, with human visceral oWAT and mediastinal fat showing higher thermogenic expression than human abWAT (Cheung et al., 2013; Zuriaga et al., 2017). Although not explicitly shown, this is most likely due to different amounts of beige/brown cells between depots, and not the levels of expression per cell. Whether the browning capacity also is different between the depots remains to be established in humans. Lastly, another significant difference between humans and mice is that human brown adipocytes do not readily express the major murine adrenergic receptor, the beta3 adrenergic receptor, and instead rely on stimulation via only the beta2 adrenergic receptor, and therefore the two species have different responses to such stimulations (Blondin et al., 2020). Taken together, the brown adipose tissue field has recently published a number of interesting papers detailing the species differences between mice and humans, and using this information to better translate knowledge gained from animal models to future clinical applications in humans (Kowaltowski, 2022).
Concluding remarks and potential future developments in the field
How a deeper knowledge of species-specific differences can avoid confounding results and help advance obesity research
Taken together, it is clear that mice are not men, but also that many of our insights in adipose tissue biology come from rodent studies. How can we best utilize these differences to our advantage and avoid confusion? First of all, we should be aware of these differences, and also make others aware by always including the detailed anatomical location of any studied fat pad in articles and abstracts, using for example oWAT and gWAT throughout the text instead of simply visceral WAT. The recent publication of single cell sequencing data of several fat depots from both mice and humans will greatly contribute to the comparison of adipose biology between the species and promises to become an important resource for translational studies (Emont et al., 2022). Secondly, we should more readily start to accept and even embrace adipose tissue species differences, as they can teach us about different aspects of adipose biology and its relationship to metabolic diseases. This includes more readily publishing and discussing results that differ between species, for the field to learn what part of adipose biology is directly translatable between species, and what is not. This may also involve reviewers being more careful when asking for validation of mouse data in human material and vice versa, accepting that not all results can be directly compared, but still can contribute to valuable knowledge. Thirdly, considering the species differences, we should more readily try to use human material in our studies, instead of relying on mouse in vitro and in vivo models. Tools to study human adipocytes have increased dramatically in the past decade, with more human primary or immortalized adipocyte cells becoming available, more commercial vendors of such cells, more fresh adipose biopsies being available, and the development of more sophisticated methods to grow, differentiate and maintain human adipocytes (Dufau et al., 2021). This includes Membrane Mature Adipocyte Aggregate Cultures (MAAC) for long term culturing of mature adipocytes (Harms et al., 2019), and 3D-culturing models for differentiating adipocyte progenitors in vitro using either scaffold-free conditions (Shen et al., 2021), or a scaffold that allows adipocytes to differentiate along vascular sprouts and form human unilocular vascularized adipose spheroids (HUVAS) (Ioannidou et al., 2022). While rodent models remain a vital and unexchangeable part of adipose tissue research, these advances have now opened the door for more labs to do translational research and continue exploring the differences and similarities in metabolism between species.
Author contributions
CEH conceived the idea and wrote the paper. EB and CEH designed the figure. EB and JB discussed the concepts and edited the paper. All authors read and approved the final version of the paper.
Funding
EB is supported by grants from the Wallenberg Centre for Molecular and Translational Medicine at the University of Gothenburg, Knut and Alice Wallenberg Foundation, the Swedish Research Council (#2016/82), SSMF (#S150086), and an ERC StG (#804418). CEH is supported by the Swedish Research Council (#2019-02046) and Karolinska Institutet (#2-1062/2018).
Conflict of interest
JB is an employee and shareholder of Evotec International.
The remaining authors declare that the research was conducted in the absence of any commercial or financial relationships that could be construed as a potential conflict of interest.
Publisher’s note
All claims expressed in this article are solely those of the authors and do not necessarily represent those of their affiliated organizations, or those of the publisher, the editors and the reviewers. Any product that may be evaluated in this article, or claim that may be made by its manufacturer, is not guaranteed or endorsed by the publisher.
References
Acosta, J. R., Douagi, I., Andersson, D. P., Backdahl, J., Ryden, M., Arner, P., et al. (2016). Increased fat cell size: A major phenotype of subcutaneous white adipose tissue in non-obese individuals with type 2 diabetes. Diabetologia 59 (3), 560–570. doi:10.1007/s00125-015-3810-6
Arner, P. (1995). Differences in lipolysis between human subcutaneous and omental adipose tissues. Ann. Med. 27 (4), 435–438. doi:10.3109/07853899709002451
Belligoli, A., Compagnin, C., Sanna, M., Favaretto, F., Fabris, R., Busetto, L., et al. (2019). Characterization of subcutaneous and omental adipose tissue in patients with obesity and with different degrees of glucose impairment. Sci. Rep. 9 (1), 11333. doi:10.1038/s41598-019-47719-y
Blondin, D. P., Nielsen, S., Kuipers, E. N., Severinsen, M. C., Jensen, V. H., Miard, S., et al. (2020). Human Brown adipocyte thermogenesis is driven by β2-AR stimulation. Cell Metab. 32 (2), 287–300. doi:10.1016/j.cmet.2020.07.005
Boucher, J., Castan-Laurell, I., Le Lay, S., Grujic, D., Sibrac, D., Krief, S., et al. (2002). Human alpha 2A-adrenergic receptor gene expressed in transgenic mouse adipose tissue under the control of its regulatory elements. J. Mol. Endocrinol. 29 (2), 251–264. doi:10.1677/jme.0.0290251
Castan, I., Valet, P., Larrouy, D., Voisin, T., RemAury, A., DaviauD, D., et al. (1993). Distribution of PYY receptors in human fat cells: An antilipolytic system alongside the alpha 2-adrenergic system. Am. J. Physiol. 265 (1), E74–E80. doi:10.1152/ajpendo.1993.265.1.E74
Castan, I., Valet, P., Quideau, N., Voisin, T., Ambid, L., LaburtheM., , et al. (1994). Antilipolytic effects of alpha 2-adrenergic agonists, neuropeptide Y, adenosine, and PGE1 in mammal adipocytes. Am. J. Physiol. 266 (4), R1141–R1147. doi:10.1152/ajpregu.1994.266.4.R1141
Chait, A., and den Hartigh, L. J. (2020). Adipose tissue distribution, inflammation and its metabolic consequences, including diabetes and cardiovascular disease. Front. Cardiovasc. Med. 7, 22. doi:10.3389/fcvm.2020.00022
Cheung, L., Gertow, J., Werngren, O., FoLkersen, L., PetrovicN., , Nedergaard, J., et al. (2013). Human mediastinal adipose tissue displays certain characteristics of brown fat. Nutr. Diabetes 3, e66. doi:10.1038/nutd.2013.6
Christen, T., Sheikine, Y., Rocha, V. Z., Hurwitz, S., Goldfine, A. B., Di Carli, M., et al. (2010). Increased glucose uptake in visceral versus subcutaneous adipose tissue revealed by PET imaging. JACC. Cardiovasc. Imaging 3 (8), 843–851. doi:10.1016/j.jcmg.2010.06.004
Chusyd, D. E., Wang, D., Huffman, D. M., and Nagy, T. R. (2016). Relationships between rodent white adipose fat pads and human white adipose fat depots. Front. Nutr. 3, 10. doi:10.3389/fnut.2016.00010
Cifarelli, V., Beeman, S. C., Smith, G. I., Yoshino, J., Morozov, D., Beals, J. W., et al. (2020). Decreased adipose tissue oxygenation associates with insulin resistance in individuals with obesity. J. Clin. Invest. 130 (12), 6688–6699. doi:10.1172/JCI141828
Cypess, A. M., Lehman, S., Williams, G., Tal, I., Rodman, D., Goldfine, A. B., et al. (2009). Identification and importance of Brown adipose tissue in adult humans. N. Engl. J. Med. 360 (15), 1509–1517. doi:10.1056/NEJMoa0810780
Di Nicola, V. (2019). Omentum a powerful biological source in regenerative surgery. Regen. Ther. 11, 182–191. doi:10.1016/j.reth.2019.07.008
Dufau, J., Shen, J. X., Couchet, M., De Castro Barbosa, T., Mejhert, N., Massier, L., et al. (2021). In vitro and ex vivo models of adipocytes. Am. J. Physiol. Cell Physiol. 320 (5), C822–C841. doi:10.1152/ajpcell.00519.2020
Emont, M. P., Jacobs, C., Essene, A. L., Pant, D., Tenen, D., Colleluori, G., et al. (2022). A single-cell atlas of human and mouse white adipose tissue. Nature 603 (7903), 926–933. doi:10.1038/s41586-022-04518-2
Farnier, C., Krief, S., Blache, M., Diot-DupuyF., , Mory, G., Ferre, P., et al. (2003). Adipocyte functions are modulated by cell size change: Potential involvement of an integrin/ERK signalling pathway. Int. J. Obes. Relat. Metab. Disord. 27 (10), 1178–1186. doi:10.1038/sj.ijo.0802399
Gabriely, I., Ma, X. H., Yang, X. M., Atzmon, G., Rajala, M. W., Berg, A. H., et al. (2002). Removal of visceral fat prevents insulin resistance and glucose intolerance of aging: An adipokine-mediated process? Diabetes 51 (10), 2951–2958. doi:10.2337/diabetes.51.10.2951
Goodpaster, B. H., Thaete, F. L., Simoneau, J. A., and Kelley, D. E. (1997). Subcutaneous abdominal fat and thigh muscle composition predict insulin sensitivity independently of visceral fat. Diabetes 46 (10), 1579–1585. doi:10.2337/diacare.46.10.1579
Harms, M. J., Li, Q., Lee, S., Zhang, C., Kull, B., Hallen, S., et al. (2019). Mature human white adipocytes cultured under membranes maintain identity, function, and can transdifferentiate into Brown-like adipocytes. Cell Rep. 27 (1), 213–225. doi:10.1016/j.celrep.2019.03.026
Herz, C. T., and Kiefer, F. W. (2019). Adipose tissue browning in mice and humans. J. Endocrinol. 241 (3), R97–R109. doi:10.1530/joe-18-0598
Hoffstedt, J., Arner, P., Hellers, G., and Lonnqvist, F. (1997). Variation in adrenergic regulation of lipolysis between omental and subcutaneous adipocytes from obese and non-obese men. J. Lipid Res. 38 (4), 795–804. doi:10.1016/s0022-2275(20)37246-1
Ioannidou, A., Alatar, S., Schipper, R., Baganha, F., Ahlander, M., Hornell, A., et al. (2022). Hypertrophied human adipocyte spheroids as in vitro model of weight gain and adipose tissue dysfunction. J. Physiol. 600 (4), 869–883. doi:10.1113/JP281445
Jensen, M. D. (2008). Role of body fat distribution and the metabolic complications of obesity. J. Clin. Endocrinol. Metab. 93 (11), S57–S63. doi:10.1210/jc.2008-1585
Johnson, P. R., and Hirsch, J. (1972). Cellularity of adipose depots in six strains of genetically obese mice. J. Lipid Res. 13 (1), 2–11. doi:10.1016/s0022-2275(20)39428-1
Khan, T., Muise, E. S., Iyengar, P., Wang, Z. V., Chandalia, M., Abate, N., et al. (2009). Metabolic dysregulation and adipose tissue fibrosis: Role of collagen VI. Mol. Cell. Biol. 29 (6), 1575–1591. doi:10.1128/MCB.01300-08
Kim, J. Y., van de Wall, E., Laplante, M., Azzara, A., Trujillo, M. E., Hofmann, S. M., et al. (2007). Obesity-associated improvements in metabolic profile through expansion of adipose tissue. J. Clin. Invest. 117 (9), 2621–2637. doi:10.1172/JCI31021
Kim, S. M., Lun, M., Wang, M., Senyo, S. E., Guillermier, C., Patwari, P., et al. (2014). Loss of white adipose hyperplastic potential is associated with enhanced susceptibility to insulin resistance. Cell Metab. 20 (6), 1049–1058. doi:10.1016/j.cmet.2014.10.010
Kowaltowski, A. J. (2022). Cold exposure and the metabolism of mice, men, and other wonderful creatures. Physiol. (Bethesda) 37 (5), 0. doi:10.1152/physiol.00002.2022
Kraunsoe, R., Boushel, R., Hansen, C. N., Schjerling, P., Qvortrup, K., Stockel, M., et al. (2010). Mitochondrial respiration in subcutaneous and visceral adipose tissue from patients with morbid obesity. J. Physiol. 588 (12), 2023–2032. doi:10.1113/jphysiol.2009.184754
Lafontan, M., and Berlan, M. (1995). Fat cell alpha 2-adrenoceptors: The regulation of fat cell function and lipolysis. Endocr. Rev. 16 (6), 716–738. doi:10.1210/edrv-16-6-716
Lafontan, M. (1994). Differential recruitment and differential regulation by physiological amines of fat cell beta-1, beta-2 and beta-3 adrenergic receptors expressed in native fat cells and in transfected cell lines. Cell. Signal. 6 (4), 363–392. doi:10.1016/0898-6568(94)90085-x
Lafontan, M., and Langin, D. (2009). Lipolysis and lipid mobilization in human adipose tissue. Prog. Lipid Res. 48 (5), 275–297. doi:10.1016/j.plipres.2009.05.001
Laviola, L., Perrini, S., Cignarelli, A., Natalicchio, A., Leonardini, A., De Stefano, F., et al. (2006). Insulin signaling in human visceral and subcutaneous adipose tissue in vivo. Diabetes 55 (4), 952–961. doi:10.2337/diabetes.55.04.06.db05-1414
Ledoux, S., Queguiner, I., Msika, S., Calderari, S., Rufat, P., Gasc, J. M., et al. (2008). Angiogenesis associated with visceral and subcutaneous adipose tissue in severe human obesity. Diabetes 57 (12), 3247–3257. doi:10.2337/db07-1812
Lempesis, I. G., van Meijel, R. L. J., Manolopoulos, K. N., and Goossens, G. H. (2020). Oxygenation of adipose tissue: A human perspective. Acta Physiol. 228 (1), e13298. doi:10.1111/apha.13298
Livingston, J. N., Lerea, K. M., Bolinder, J., Kager, L., Backman, L., and Arner, P. (1984). Binding and molecular weight properties of the insulin receptor from omental and subcutaneous adipocytes in human obesity. Diabetologia 27 (4), 447–453. doi:10.1007/BF00273909
Lotta, L. A., Gulati, P., Day, F. R., Payne, F., Ongen, H., van de Bunt, M., et al. (2017). Integrative genomic analysis implicates limited peripheral adipose storage capacity in the pathogenesis of human insulin resistance. Nat. Genet. 49 (1), 17–26. doi:10.1038/ng.3714
Lundgren, M., Buren, J., Ruge, T., Myrnas, T., and Eriksson, J. W. (2004). Glucocorticoids down-regulate glucose uptake capacity and insulin-signaling proteins in omental but not subcutaneous human adipocytes. J. Clin. Endocrinol. Metab. 89 (6), 2989–2997. doi:10.1210/jc.2003-031157
Macotela, Y., Boucher, J., Tran, T. T., and Kahn, C. R. (2009). Sex and depot differences in adipocyte insulin sensitivity and glucose metabolism. Diabetes 58 (4), 803–812. doi:10.2337/db08-1054
Mann, J. P., and Savage, D. B. (2019). What lipodystrophies teach us about the metabolic syndrome. J. Clin. Invest. 129 (10), 4009–4021. doi:10.1172/JCI129190
Maurer, S., Harms, M., and Boucher, J. (2021). The colorful versatility of adipocytes: white-to-brown transdifferentiation and its therapeutic potential in humans. FEBS J. 288 (12), 3628–3646. doi:10.1111/febs.15470
Mauriege, P., Galitzky, J., Berlan, M., and Lafontan, M. (1987). Heterogeneous distribution of beta and alpha-2 adrenoceptor binding sites in human fat cells from various fat deposits: Functional consequences. Eur. J. Clin. Invest. 17 (2), 156–165. doi:10.1111/j.1365-2362.1987.tb02395.x
Morigny, P., Boucher, J., Arner, P., and Langin, D. (2021). Lipid and glucose metabolism in white adipocytes: Pathways, dysfunction and therapeutics. Nat. Rev. Endocrinol. 17 (5), 276–295. doi:10.1038/s41574-021-00471-8
O'Connell, J., Lynch, L., Cawood, T. J., Kwasnik, A., Nolan, N., Geoghegan, J., et al. (2010). The relationship of omental and subcutaneous adipocyte size to metabolic disease in severe obesity. PLoS One 5 (4), e9997. doi:10.1371/journal.pone.0009997
Porter, C., Herndon, D. N., Chondronikola, M., Chao, T., Annamalai, P., Bhattarai, N., et al. (2016). Human and mouse Brown adipose tissue mitochondria have comparable UCP1 function. Cell Metab. 24 (2), 246–255. doi:10.1016/j.cmet.2016.07.004
Rawshani, A., Eliasson, B., Rawshani, A., Henninger, J., Mardinoglu, A., Carlsson, A., et al. (2020). Adipose tissue morphology, imaging and metabolomics predicting cardiometabolic risk and family history of type 2 diabetes in non-obese men. Sci. Rep. 10 (1), 9973. doi:10.1038/s41598-020-66199-z
Rytka, J. M., Wueest, S., Schoenle, E. J., and Konrad, D. (2011). The portal theory supported by venous drainage-selective fat transplantation. Diabetes 60 (1), 56–63. doi:10.2337/db10-0697
Sakers, A., De Siqueira, M. K., Seale, P., and Villanueva, C. J. (2022). Adipose-tissue plasticity in health and disease. Cell 185 (3), 419–446. doi:10.1016/j.cell.2021.12.016
Salans, L. B., Horton, E. S., and Sims, E. A. (1971). Experimental obesity in man: Cellular character of the adipose tissue. J. Clin. Invest. 50 (5), 1005–1011. doi:10.1172/JCI106570
Schottl, T., Kappler, L., Braun, K., Fromme, T., and Klingenspor, M. (2015). Limited mitochondrial capacity of visceral versus subcutaneous white adipocytes in male C57BL/6N mice. Endocrinology 156 (3), 923–933. doi:10.1210/en.2014-1689
Schottl, T., Kappler, L., Fromme, T., and Klingenspor, M. (2015). Limited OXPHOS capacity in white adipocytes is a hallmark of obesity in laboratory mice irrespective of the glucose tolerance status. Mol. Metab. 4 (9), 631–642. doi:10.1016/j.molmet.2015.07.001
Shen, J. X., Couchet, M., Dufau, J., de Castro Barbosa, T., Ulbrich, M. H., Helmstadter, M., et al. (2021). 3D adipose tissue culture links the organotypic microenvironment to improved adipogenesis. Adv. Sci. 8 (16), e2100106. doi:10.1002/advs.202100106
Shepherd, P. R., Gnudi, L., Tozzo, E., Yang, H., LeachF., , and Kahn, B. B. (1993). Adipose cell hyperplasia and enhanced glucose disposal in transgenic mice overexpressing GLUT4 selectively in adipose tissue. J. Biol. Chem. 268 (30), 22243–22246. doi:10.1016/s0021-9258(18)41516-5
Song, M. G., Lee, H. J., Jin, B. Y., Gutierrez-Aguilar, R., Shin, K. H., Choi, S. H., et al. (2016). Depot-specific differences in angiogenic capacity of adipose tissue in differential susceptibility to diet-induced obesity. Mol. Metab. 5 (11), 1113–1120. doi:10.1016/j.molmet.2016.09.001
Spalding, K. L., Arner, E., Westermark, P. O., Bernard, S., Buchholz, B. A., Bergmann, O., et al. (2008). Dynamics of fat cell turnover in humans. Nature 453 (7196), 783–787. doi:10.1038/nature06902
Stolic, M., Russell, A., Hutley, L., Hay, J., MacDonald, G., Whitehead, J., et al. (2002). Glucose uptake and insulin action in human adipose tissue--influence of BMI, anatomical depot and body fat distribution. Int. J. Obes. Relat. Metab. Disord. 26 (1), 17–23. doi:10.1038/sj.ijo.0801850
Suarez-Cuenca, J. A., De La Pena-Sosa, G., De La Vega-Moreno, K., Banderas-Lares, D. Z., Salamanca-Garcia, M., Martinez-Hernandez, J. E., et al. (2021). Enlarged adipocytes from subcutaneous vs. visceral adipose tissue differentially contribute to metabolic dysfunction and atherogenic risk of patients with obesity. Sci. Rep. 11 (1), 1831. doi:10.1038/s41598-021-81289-2
Tavernier, G., Barbe, P., Galitzky, J., BerlanM., , Caput, D., LafontanM., , et al. (1996). Expression of beta3-adrenoceptors with low lipolytic action in human subcutaneous white adipocytes. J. Lipid Res. 37 (1), 87–97. doi:10.1016/s0022-2275(20)37638-0
Tchernof, A., Belanger, C., Morisset, A. S., Richard, C., Mailloux, J., Laberge, P., et al. (2006). Regional differences in adipose tissue metabolism in women: Minor effect of obesity and body fat distribution. Diabetes 55 (5), 1353–1360. doi:10.2337/db05-1439
Tchoukalova, Y. D., Koutsari, C., Karpyak, M. V., Votruba, S. B., Wendland, E., and Jensen, M. D. (2008). Subcutaneous adipocyte size and body fat distribution. Am. J. Clin. Nutr. 87 (1), 56–63. doi:10.1093/ajcn/87.1.56
van Harmelen, V., Dicker, A., Ryden, M., Hauner, H., Lonnqvist, F., Naslund, E., et al. (2002). Increased lipolysis and decreased leptin production by human omental as compared with subcutaneous preadipocytes. Diabetes 51 (7), 2029–2036. doi:10.2337/diabetes.51.7.2029
van Marken Lichtenbelt, W. D., Vanhommerig, J. W., Smulders, N. M., Drossaerts, J. M. A. F. L., Kemerink, G. J., Bouvy, N. D., et al. (2009). Cold-activated Brown adipose tissue in healthy men. N. Engl. J. Med. 360 (15), 1500–1508. doi:10.1056/NEJMoa0808718
Verboven, K., Wouters, K., Gaens, K., Hansen, D., BijnenM., , WetzelS, S., et al. (2018). Abdominal subcutaneous and visceral adipocyte size, lipolysis and inflammation relate to insulin resistance in male obese humans. Sci. Rep. 8 (1), 4677. doi:10.1038/s41598-018-22962-x
Villaret, A., Galitzky, J., Decaunes, P., Esteve, D., Marques, M. A., Sengenes, C., et al. (2010). Adipose tissue endothelial cells from obese human subjects: Differences among depots in angiogenic, metabolic, and inflammatory gene expression and cellular senescence. Diabetes 59 (11), 2755–2763. doi:10.2337/db10-0398
Virtanen, K. A., Lidell, M. E., Orava, J., Heglind, M., Westergren, R., Niemi, T., et al. (2009). Functional Brown adipose tissue in healthy adults. N. Engl. J. Med. 360 (15), 1518–1525. doi:10.1056/NEJMoa0808949
Virtanen, K. A., Lonnroth, P., Parkkola, R., Peltoniemi, P., Asola, M., Viljanen, T., et al. (2002). Glucose uptake and perfusion in subcutaneous and visceral adipose tissue during insulin stimulation in nonobese and obese humans. J. Clin. Endocrinol. Metab. 87 (8), 3902–3910. doi:10.1210/jcem.87.8.8761
Vitali, A., Murano, I., Zingaretti, M. C., Frontini, A., and Cinti, S. (2012). The adipose organ of obesity-prone C57BL/6J mice is composed of mixed white and Brown adipocytes. J. Lipid Res. 53 (4), 619–629. doi:10.1194/jlr.M018846
Vogel, M. A. A., Wang, P., Bouwman, F. G., HoebersN., , Blaak, E. E., Renes, J., et al. (2019). A comparison between the abdominal and femoral adipose tissue proteome of overweight and obese women. Sci. Rep. 9 (1), 4202. doi:10.1038/s41598-019-40992-x
Wang, M. Y., Grayburn, P., Chen, S., Ravazzola, M., Orci, L., and Unger, R. H. (2008). Adipogenic capacity and the susceptibility to type 2 diabetes and metabolic syndrome. Proc. Natl. Acad. Sci. U. S. A. 105 (16), 6139–6144. doi:10.1073/pnas.0801981105
Wang, Q. A., Tao, C., Gupta, R. K., and Scherer, P. E. (2013). Tracking adipogenesis during white adipose tissue development, expansion and regeneration. Nat. Med. 19 (10), 1338–1344. doi:10.1038/nm.3324
Wernstedt Asterholm, I., Tao, C., Morley, T. S., Wang, Q. A., Delgado-Lopez, F., Wang, Z. V., et al. (2014). Adipocyte inflammation is essential for healthy adipose tissue expansion and remodeling. Cell Metab. 20 (1), 103–118. doi:10.1016/j.cmet.2014.05.005
Wueest, S., Schoenle, E. J., and Konrad, D. (2012). Depot-specific differences in adipocyte insulin sensitivity in mice are diet- and function-dependent. Adipocyte 1 (3), 153–156. doi:10.4161/adip.19910
Zhang, M., Hu, T., Zhang, S., and Zhou, L. (2015). Associations of different adipose tissue depots with insulin resistance: A systematic review and meta-analysis of observational studies. Sci. Rep. 5, 18495. doi:10.1038/srep18495
Zhang, Y., Proenca, R., Maffei, M., BaroneM., , LeopoLd, L., and Friedman, J. M. (1994). Positional cloning of the mouse obese gene and its human homologue. Nature 372 (6505), 425–432. doi:10.1038/372425a0
Zuriaga, M. A., Fuster, J. J., Gokce, N., and Walsh, K. (2017). Humans and mice display opposing patterns of "browning" gene expression in visceral and subcutaneous white adipose tissue depots. Front. Cardiovasc. Med. 4, 27. doi:10.3389/fcvm.2017.00027
Keywords: obesity, adipose tissue, species differences, hypertrophy, metabolism
Citation: Börgeson E, Boucher J and Hagberg CE (2022) Of mice and men: Pinpointing species differences in adipose tissue biology. Front. Cell Dev. Biol. 10:1003118. doi: 10.3389/fcell.2022.1003118
Received: 25 July 2022; Accepted: 29 August 2022;
Published: 15 September 2022.
Edited by:
Siegfried Ussar, Helmholtz Association of German Research Centres (HZ), GermanyReviewed by:
Vijay Karkal Hegde, Texas Tech University, United StatesDorit John, Leipzig University, Germany
Copyright © 2022 Börgeson, Boucher and Hagberg. This is an open-access article distributed under the terms of the Creative Commons Attribution License (CC BY). The use, distribution or reproduction in other forums is permitted, provided the original author(s) and the copyright owner(s) are credited and that the original publication in this journal is cited, in accordance with accepted academic practice. No use, distribution or reproduction is permitted which does not comply with these terms.
*Correspondence: Carolina E. Hagberg, Y2Fyb2xpbmEuaGFnYmVyZ0BraS5zZQ==