- Centre for Molecular Biology of Inflammation, Institute of Medical Biochemistry, University of Muenster, Muenster, Germany
Vascular endothelial cells produce and release compounds regulating vascular tone, blood vessel growth and differentiation, plasma composition, coagulation and fibrinolysis, and also engage in interactions with blood cells thereby controlling hemostasis and acute inflammatory reactions. These interactions have to be tightly regulated to guarantee smooth blood flow in normal physiology, but also allow specific and often local responses to blood vessel injury and infectious or inflammatory insults. To cope with these challenges, endothelial cells have the remarkable capability of rapidly changing their surface properties from non-adhesive (supporting unrestricted blood flow) to adhesive (capturing circulating blood cells). This is brought about by the evoked secretion of major adhesion receptors for platelets (von-Willebrand factor, VWF) and leukocytes (P-selectin) which are stored in a ready-to-be-used form in specialized secretory granules, the Weibel-Palade bodies (WPB). WPB are unique, lysosome related organelles that form at the trans-Golgi network and further mature by receiving material from the endolysosomal system. Failure to produce correctly matured VWF and release it through regulated WPB exocytosis results in pathologies, most importantly von-Willebrand disease, the most common inherited blood clotting disorder. The biogenesis of WPB, their intracellular motility and their fusion with the plasma membrane are regulated by a complex interplay of proteins and lipids, involving Rab proteins and their effectors, cytoskeletal components as well as membrane tethering and fusion machineries. This review will discuss aspects of WPB biogenesis, trafficking and exocytosis focussing on recent findings describing factors contributing to WPB maturation, WPB-actin interactions and WPB-plasma membrane tethering and fusion.
Introduction
Endothelial cells comprise the inner lining of blood vessels and thus the first cellular barrier separating blood and tissue. They form single-layered epithelia that differ in morphology, molecular characteristics, physiology and function depending on the type of vascular bed. As such they seal blood vessels and control traffic of nutrients, hormones, growth and differentiation factors, particles and cells (immune cells, metastasizing tumor cells and even pathogens) to and from the vasculature. Moreover, through selective secretion and uptake as well as production and decoding of signaling molecules they regulate blood vessel homeostasis including clotting and coagulation, fibrinolysis and thrombosis as well as vascular tone and local inflammatory reactions.
One striking characteristic of endothelial cells relates to the adhesive properties of their apical cell surface that faces the blood vessel lumen. In the normal physiological state this surface does not interact firmly with leukocytes, erythrocytes and platelets thereby permitting an unrestricted blood flow and blood cell circulation. However, upon insult and endothelial cell activation surface properties change rapidly allowing leukocytes and platelets to adhere to the vessel wall. These cell interactions are vital to ensure proper responses to blood vessel injury (platelet plug formation and initiation of coagulation) and inflammatory or infectious insult (recruitment of leukocytes to sites of tissue damage or infection). Endothelial cells can actively control these surface properties by the regulated presentation of specific adhesion molecules. To do so, vascular endothelial cells are equipped with unique secretory organelles that store among other things leukocyte and platelet adhesion receptors to be released on demand. In honor of their initial discovery by Ewald Weibel and George Palade in electron microscopic analyses of rat and human pulmonary arteries these organelles were termed Weibel-Palade bodies (WPB) (Weibel and Palade, 1964). Only later these peculiar membrane compartments were shown to contain the major platelet adhesion molecule von-Willebrand factor (VWF) and the leukocyte receptor P-selectin (Wagner et al., 1982; Bonfanti et al., 1989). The physiological and also pathophysiological importance of WPB and their principal cargo VWF is emphasized by the fact that failure to produce and release proper VWF results in von-Willebrand disease, the major inherited bleeding disorder (for reviews see Schneppenheim and Budde, 2011; Leebeek and Eikenboom, 2016). On the other hand, vascular occlusion is a consequence of highly elevated vascular VWF levels as for instance observed in thrombotic thrombocytopenic purpura. (for review see Sadler, 2008). Thus, WPB are pivotal components of the precisely tuned machinery that orchestrates blood vessel homeostasis. This mini review will highlight the unique features of WPB particularly emphasizing recent developments in the understanding of their maturation and secretion.
WPB Maturation
WPB are born at the trans-Golgi network (TGN) where they bud off in the form of discernible structures. Their dimensions and unique morphology are dictated by the main cargo VWF, a large glycoprotein synthesized and first processed in the ER (for references and recent crystal structure of the VWF D’D3 domains see Dong et al., 2019). VWF is then transported to the Golgi where it is assembled into defined quanta. A copacking of these quanta occurs in the TGN prior to or concomitant with the actual budding of immature WPB which can maintain connections to the Golgi for 2–4 h (Zenner et al., 2007; Ferraro et al., 2014; Mourik et al., 2015). These connections and the close proximity to the Golgi likely permit the further addition of VWF and possibly other cargo to the immature WPB (Mourik et al., 2015). The early WPB released from the TGN further mature to finally yield the highly elongated cigar-shaped organelles primarily found in the periphery of endothelial cells (for reviews see van Mourik et al., 2002; Michaux and Cutler, 2004; McCormack et al., 2017; Karampini et al., 2020). This maturation is driven on one hand by the continued multimerization and tight packing of VWF into a quasi-crystalline arrangement enwrapped by a membrane, which requires luminal acidification and reflects itself in the condensation of WPB from an electron lucent immature organelle to an electron dense mature structure. On the other hand, post-Golgi maturation is accompanied by acquisition of additional cytosolic and also endosomal/lysosomal components. They include the RabGTPase Rab27a and the tetraspanin CD63 identifying WPB as lysosome-related organelles (LRO) that share molecular features with pigment-storing melanosomes (for reviews see Raposo et al., 2007; Bowman et al., 2019). It is worth noting here that the net size of WPB is primarily determined at the level of the Golgi and that further maturation mainly leads to condensation and tubular elongation. Several aspects of WPB size control and maturation have been addressed recently revealing novel and exciting connections.
An interesting link between WPB size control and cell metabolism was discovered recently following the identification of the Arf guanine nucleotide exchange factor (GEF) GBF1 (a GEF for Arf1 and 4) as a factor promoting ER/Golgi trafficking of VWF. GBF1 can be activated by phosphorylation by AMP-activated protein kinase (AMPK), a key enzyme coupling metabolic changes to cellular signaling, and it was shown that low glucose levels and subsequent AMPK activation lead to GBF1 phosphorylation and a resulting upregulation of anterograde VWF trafficking. This in turn produces smaller WPB and reduces VWF secretion (Lopes-da-Silva et al., 2019) (Figure 1). Arf GTPase activating proteins (GAPs) that inactivate their cognate Arf proteins also appear to regulate WPB size as depletion in endothelial cells of the ArfGAP SMAP1 leads to a size reduction in the WPB that form (Watanabe et al., 2021). The SNARE Sec22b was recently identified as another factor controlling WPB morphology presumably also by affecting the ER/Golgi transport route of VWF. Depletion of Sec22b causes a loss of large, elongated WPB along with a dilation of ER cisternae that accumulate non-processed VWF (Karampini et al., 2020) (Figure 1). Thus, several factors regulating VWF maturation and packing into WPB and thereby affecting WPB size and morphology have been discovered and approaches to exploit these also in pathophysiological settings appear promising. Along these lines, Ferraro and coworkers developed a microscopic screening approach measuring WPB size that led to the identification of first candidate compounds that reduce WPB length. As a consequence, this also reduces the pro-thrombotic activity of secreted VWF as VWF secretion from shorter WPB significantly dampens its platelet adhesion capability (Ferraro et al., 2016, 2020).
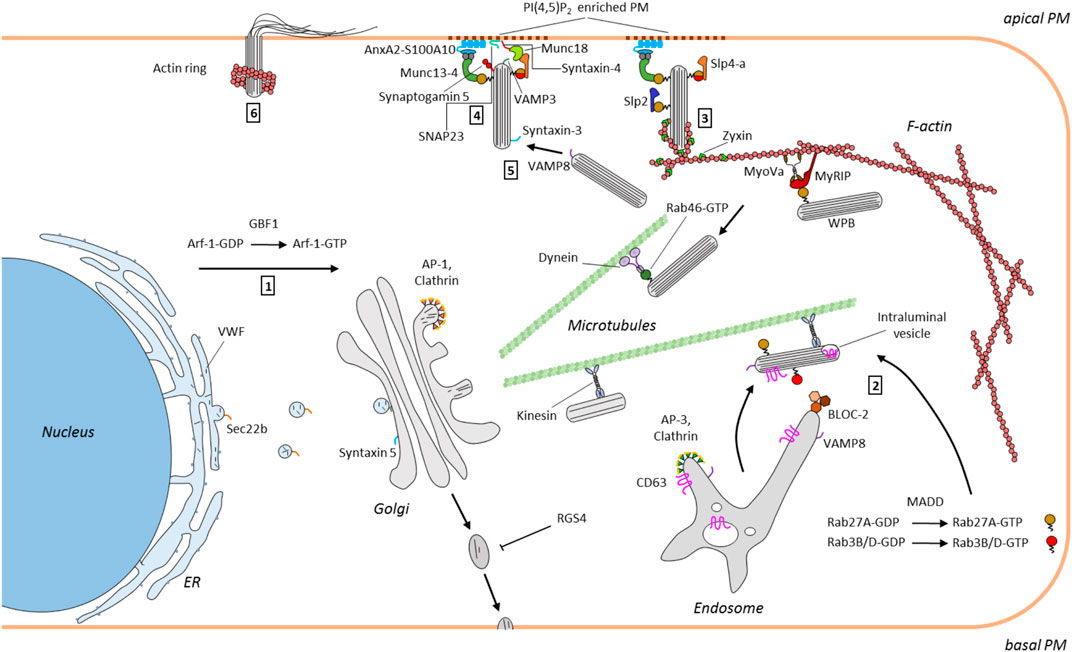
FIGURE 1. Scheme depicting the WPB itinerary in endothelial cells. WPB formation is driven by VWF that is produced at the ER and trafficked to the Golgi (1). WPB that bud from the TGN in an AP-1 and clathrin dependent process are then transported to the cell periphery along microtubules. This is accompanied by the BLOC-2 and annexin A8 dependent transfer of endosomal components such as CD63 and VAMP8 to WPB (2). Maturing WPB acquire certain RabGTPases, e.g. Rab27A and Rab3B/D, the former required for linking WPB at the cortical actin cytoskeleton (via MyRIP/MyoVc) and supporting exocytosis (via Slp4-a) (3). Secretagogue induced tethering at and fusion with the PM requires docking factors, such as the annexin A2/S100A10/Munc13-4 complex and a SNARE-based fusion machinery and can also involve compound and cumulative events (3, 4 and 5). Finally, post fusion actin rings have been observed that support the full release of highly multimeric VWF (6). Mainly factors identified in the recent years have been included.
Once early WPB have emerged from the Golgi they acquire additional proteins (and presumably also lipids) in the process of maturation that is accompanied by a microtubule-dependent movement to the cell periphery (for review see McCormack et al., 2017). Many of those additional WPB components have been identified, among other things through proteomic screens (van Breevoort et al., 2012; Holthenrich et al., 2019); however, their delivery to and association with the maturing organelle has only recently been characterized in a few cases. In line with WPB representing LRO, some proteins found on mature WPB are of late endosome/lysosome (LEL) origin (e.g. the tetraspanin and P selectin cofactor CD63) and most likely routed to the organelle by direct transport possibly involving tubular carriers. Whereas earlier studies had identified the Ca2+/phospholipid-binding protein annexin A8 as a LEL-localized component of the machinery facilitating LEL-to-WPB delivery of CD63 (Poeter et al., 2014), Sharda and coworkers (Sharda et al., 2020) recently reported the participation of biogenesis of lysosome related organelle-2 (BLOC-2), a protein that can be mutated in the recessive bleeding disorder Hermansky-Pudlak syndrome. Among other things Hermansky-Pudlak syndrome is associated with platelet aggregation and pigmentation defects, the latter due to compromised maturation of melanosomes, LROs that show several parallels to WPB (for reviews see Raposo et al., 2007; Simons and Raposo, 2009). Depletion of BLOC-2 results in both, compromised LEL-to-WPB transport of CD63 and general WPB maturation defects with the WPB appearing round instead of elongated and clustered in the perinuclear region (Figure 1). As the immature organelles formed under these conditions failed to process VWF into the highly multimeric forms these were absent in the material secreted from BLOC-2 depleted endothelial cells following thrombin stimulation (and intracellular Ca2+ mobilization). Moreover, the exocyst complex was identified as a target of BLOC-2 in endothelial cells and exocyst depletion or inhibition phenocopied the WPB maturation defects seen in BLOC-2 deficient cells. In this study exocyst was also found to serve a second function in impeding WPB exocytosis at the PM (Sharda et al., 2020). The involvement of BLOC-2 in proper WPB maturation was also shown in the respective mutant mice that are characterized by impaired VWF tubulation (Ma et al., 2016). Another gene that can be mutated in Hermansky-Pudlak syndrome is AP3B1 encoding the adaptor complex three β1 subunit. Blood outgrowth endothelial cells from Hermansky-Pudlak syndrome patients carrying the AP3B1 mutation also lack CD63 in their WPB indicative of improper organelle maturation. Moreover, these cells are compromised in their evoked WPB exocytosis, most likely because they fail to recruit the v-SNARE VAMP8 to maturing WPB (Karampini et al., 2019) (Figure 1). While the above-mentioned studies identified maturation factors/pathways involved in the delivery of transmembrane proteins (CD63, VAMP8) to maturing WPB, another hallmark of mature WPB are a specific subset of cytosolically associated RabGTPases, in particular Rab27a and the Rab3 isoforms b and d. Addressing this aspect of the maturation, Kat and coworkers (Kat et al., 2021) could recently identify MAP kinase-activating death domain (MADD) as a crucial component involved. MADD serves as a GEF for these Rabs and silencing of MADD through knockdown approaches markedly reduced the recruitment of Rab27a, Rab3b and Rab3d to maturing WPB (Figure 1). Finally, it should be noted that WPB maturation is not only accompanied by tubulation and tight packing of VWF and the acquisition of additional protein contents, it also generates other morphological characteristics typical for LRO. Specifically, vesicles inside the lumen of the organelle, a hallmark of many LRO, were observed recently in mature WPB of endothelial cells. Following WPB exocytosis these intraluminal vesicles which are positive for CD63 could also be released and possibly function in intercellular communication, again extending the similarity to other LROs (Streetley et al., 2019).
Thus, WPB maturation is a highly complex process involving de novo protein acquisition, LEL-to-WPB protein transport and morphological alterations that eventually generate the unique rod-shaped organelle containing the tubulated highly multimeric VWF.
WPB-Plasma Membrane Tethering and Secretion
The VWF stored in WPB can be released in different ways. Basal secretion, typically of less multimeric VWF, provides the circulation with low levels of these VWF species, and constitutive secretion, preferentially occurring at the basolateral membrane surface of endothelial cells, deposits VWF in the subendothelial matrix. Specific components regulating these secretory events have not been systematically investigated with the exception of a recent screen that identified the regulator of G protein signaling 4 (RGS4) as a negative regulator of the constitutive pathway (Patella and Cutler, 2020). The majority of fully matured WPB, however, is retained inside the cell to await secretagogue stimulation, for example following blood vessel injury or local inflammatory insults, to present highly multimeric VWF and P-selectin on the endothelial cell surface by regulated exocytosis. Retention is achieved by anchorage in the cortical actin cytoskeleton, which is mediated with help of a complex consisting of Rab27a, the Rab27a effector MyRIP and the actin binding myosin Va (Nightingale et al., 2009; Rojo Pulido et al., 2011; Conte et al., 2016) (Figure 1). Endothelial stimulation, which can be elicited by a plethora of agonists (Lowenstein et al., 2005; Schillemans et al., 2019b) and typically results in elevated intracellular Ca2+ or cAMP levels functioning as second messengers, mobilizes the cortically anchored WPB and initiates the tethering/docking at and fusion with the plasma membrane (PM). The detailed molecular mechanisms responsible for releasing WPB from the cortical anchorage and enabling their PM contact are largely unknown, but they are likely to involve WPB associated RabGTPases. A central role for Rab27a in this event has been shown by Bierings and coworkers (Bierings et al., 2012) who reported that the evoked release of mature WPB is regulated by the interaction of Rab27a with either MyRIP (supporting cortical anchorage) or synaptotagmin-like protein 4-a (Slp4-a) (promoting WPB exocytosis) (Figure 1). Rab46 was recently identified as another Rab regulating selective WPB trafficking in the cell cortex and thereby specific cargo release following histamine evoked and Ca2+ mediated exocytosis of WPB. Rab46, which harbors a Ca2+-binding EF hand, localizes to only a subset of the peripheral WPB that are negative for the leukocyte receptor P-selectin but contain angiopoietin-2. It senses the Ca2+ elevation elicited by histamine stimulation and then triggers a retrograde, dynein-dependent transport of the associated peripheral WPB to the cell center. As the Rab46 negative, P-selectin containing WPB exocytose under these conditions, only a fraction of the WPB cargo, e.g. the proinflammatory P-selectin, is released (Miteva et al., 2019) (Figure 1). How and when such WPB diversification, i.e. a sorting of P-selectin to only some organelles, occurs and how Rab46 is recruited to only a subset of WPB is not known but these pose interesting and very central cell biological questions.
Following cortical release and in preparation of PM fusion, WPB are most likely tethered or docked at the membrane. Here, another Rab27a effector, the mammalian uncoordinated 13–4 (Munc13-4), has been shown to promote WPB exocytosis most likely by providing a link or tether between the organelle surface and a PM-bound complex consisting of annexin A2 (AnxA2) and S100A10 (Zografou et al., 2012; Chehab et al., 2017) (Figure 1). In this configuration the AnxA2/S100A10 complex most likely functions as a module binding Ca2+-dependently to PM phospholipids [e.g. phosphatidylinositol 4,5-bisphosphate, PI(4,5)P2] via its AnxA2 subunit and to WPB-bound Munc13-4 via its S100A10 subunit (Chehab et al., 2017). A special enrichment of certain PM phospholipids is indeed observed at WPB-PM fusion sites and inhibitor and depletion experiments suggest that PI(4,5)P2 and the PI(4,5)P2 producing PI4P 5-kinase are required for efficient histamine-evoked WPB exocytosis (Nguyen et al., 2020). In the course of regulated exocytosis tethered WPB are finally recognized by the membrane fusion machinery consisting of SNAREs and associated proteins. Several of the factors involved at this stage have been described over the years, including a trans-SNARE complex consisting of WPB-localized VAMP3 and PM-localized syntaxin-4 and SNAP23 as well as syntaxin-binding Munc18 proteins (Matsushita et al., 2003; Pulido et al., 2011; van Breevoort et al., 2014) (Figure 1). However, the picture is probably more complex as recent studies employing blood outgrowth endothelial cells which were isolated from a patient suffering from variant microvillus inclusion disease and shown to lack another SNARE, syntaxin-3, showed markedly impaired agonist-evoked VWF secretion. Syntaxin-3 interacts with VAMP8, another WPB-associated v-SNARE, but interestingly, was shown to localize mainly to WPB (Schillemans et al., 2018, 2019). This suggests that syntaxin-3, most likely pairing with VAMP8 on another WPB, supports homotypic fusions of WPB that could occur during compound or cumulative exocytosis (Zupančič et al., 2002; Valentijn et al., 2010; Kiskin et al., 2014; Stevenson et al., 2017) (Figure 1). Thus, several SNARE complexes are likely to support heterotypic and homotypic WPB fusion events that characterize the final steps in regulated exocytosis. Common to these events is their regulation by signaling mediators, in the case of Ca2+-dependent exocytosis the elevated Ca2+ concentrations. Several Ca2+ binding proteins have been implicated in coupling these Ca2+ signals to regulated WPB exocytosis, including the above-mentioned Slp4-a, AnxA2 and Munc13-4 as well as another Munc13 family member, Munc13-2 (Zhou et al., 2016; Holthenrich et al., 2019); however, the actual WPB-associated Ca2+ sensor that could directly activate the SNARE machinery most likely is a member of the synaptotagmin family. Synaptotagmin-5 has recently emerged as an interesting candidate as it localizes to WPB and is required for histamine evoked WPB exocytosis and VWF secretion. Importantly, a mutant synaptotagmin-5 lacking the Ca2+ coordinating asparagine residue in the C2A domain negatively interferes with histamine evoked WPB exocytosis directly showing the importance of synaptotagmin-5 Ca2+ binding (Lenzi et al., 2019). Thus, a complex interplay of Ca2+-regulated proteins, also including the recently identified Slp2-a (Francis et al., 2021), likely transmits the rise in intracellular Ca2+ to WPB-PM docking and fusion in the course of regulated exocytosis.
The Link to Actin
While cargo release in many exocytotic events occurs automatically with completion of the granule-PM fusion, WPB and some other secretory organelles carrying large cargo, e.g. surfactant-loaded lamellar bodies of alveolar epithelial cells (Miklavc et al., 2015), most likely require mechanical forces for efficient cargo expulsion. This can be provided by rearrangements of the cortical actin cytoskeleton that first has to be weakened to allow granule penetration to the PM and then site-specifically repolymerizes to support cargo release. In the case of WPB, it was observed that rings of polymerized actin form at the distal end of WPB several seconds after the actual PM fusion event (Figure 1). Furthermore, it was shown that these structures, in an active myosin motor-dependent process, are required for the efficient release of highly multimeric VWF cargo from the fused WPB (Nightingale et al., 2011). In later studies it was observed that the formation of such actin rings at WPB-PM fusion sites probably is not obligatory for VWF release, at least in case of histamine stimulation and Ca2+-dependent WPB exocytosis (Conte et al., 2015), and that the extent of actin ring formation at these fusion sites appears to depend on the type of stimulus (Nightingale et al., 2018; Mietkowska et al., 2019). Interestingly, a different actomyosin network that is also positive for the focal adhesion protein zyxin has been observed around peripheral WPB of endothelial cells stimulated with cAMP raising agonists. Here, actin framework formation occurs prior to the actual fusion event facilitating WPB exocytosis (Han et al., 2017; Li et al., 2018). Clearly, more work is required to establish a potential link between this zyxin/actomyosin network and the post-fusion actin rings, e.g. by identifying the factor(s) promoting actin polymerisation into the ring/coat-like structures at fused WPB. Moreover, the precise function of the actin structures also needs further attention. They could support exocytotic membrane fusion and VWF expulsion but potentially could also prevent fused WPB from fully collapsing into the PM, for example to permit rapid and spatially restricted compensatory endocytosis that has been shown to occur on the membrane of fused WPB (Stevenson et al., 2017). Another unresolved issue concerns the regulation of the spatially restricted changes in cortical actin architecture, in particular the questions whether certain membrane lipids enriched at WPB fusion sites such as PI(4,5)P2 are involved and which molecular players organize the actin reorganization precisely at the sites where WPB fuse or have fused.
Concluding Remarks
WPB are unique secretory organelles that allow vascular endothelial cells to respond rapidly to environmental changes by the secretion of factors that control hemostasis and inflammation. Marked progress in understanding their biogenesis, intracellular transport and secretion has been made in the last decade revealing fascinating cell biological phenomena that drive the formation of the organelle and its many modes of exocytosis. However, our picture of the organelle is far from complete and important questions, e.g. concerning unique maturation steps, cargo selection and Rab recruitment and the involvement of different actin structures in VWF release, remain to be answered. Future research in this exciting topic of cell biology has to tell and will likely also benefit pharmacological interventions of the pathway that could help controlling vascular VWF (and P-selectin) levels in pathophysiological scenarios (Karampini et al., 2020; El-Mansi and Nightingale, 2021).
Author Contributions
JN, JT, and VG contributed to writing of the manuscript and generating/editing the figure.
Funding
The authors research is supported by the 290 German Research Foundation (SFB1348/A04, SFB1009/A06, and GE514/6-3). JN is member of the joint graduate school Cells-in-Motion 292 (CiM)/IMPRS, Münster, Germany.
Conflict of Interest
The authors declare that the research was conducted in the absence of any commercial or financial relationships that could be construed as a potential conflict of interest.
Publisher’s Note
All claims expressed in this article are solely those of the authors and do not necessarily represent those of their affiliated organizations, or those of the publisher, the editors and the reviewers. Any product that may be evaluated in this article, or claim that may be made by its manufacturer, is not guaranteed or endorsed by the publisher.
References
Bierings, R., Hellen, N., Kiskin, N., Knipe, L., Fonseca, A.-V., Patel, B., et al. (2012). The Interplay between the Rab27A Effectors Slp4-A and MyRIP Controls Hormone-Evoked Weibel-Palade Body Exocytosis. Blood 120, 2757–2767. doi:10.1182/blood-2012-05-429936
Bonfanti, R., Furie, B., Furie, B., and Wagner, D. (1989). PADGEM (GMP140) Is a Component of Weibel-Palade Bodies of Human Endothelial Cells. Blood 73, 1109–1112. doi:10.1182/blood.v73.5.1109.bloodjournal7351109
Bowman, S. L., Bi‐Karchin, J., Le, L., and Marks, M. S. (2019). The Road to Lysosome‐related Organelles: Insights from Hermansky‐Pudlak Syndrome and Other Rare Diseases. Traffic 20, 404–435. doi:10.1111/tra.12646
Chehab, T., Santos, N. C., Holthenrich, A., Koerdt, S. N., Disse, J., Schuberth, C., et al. (2017). A Novel Munc13-4/S100A10/annexin A2 Complex Promotes Weibel-Palade Body Exocytosis in Endothelial Cells. MBoC 28, 1688–1700. doi:10.1091/mbc.e17-02-0128
Conte, I. L., Cookson, E., Hellen, N., Bierings, R., Mashanov, G., and Carter, T. (2015). Is There More Than One Way to Unpack a Weibel-Palade Body? Blood 126, 2165–2167. doi:10.1182/blood-2015-08-664961
Conte, I. L., Hellen, N., Bierings, R., Mashanov, G. I., Manneville, J.-B., Kiskin, N. I., et al. (2016). Interaction between MyRIP and the Actin Cytoskeleton Regulates Weibel-Palade Body Trafficking and Exocytosis. J. Cel Sci. 129, 592–603. doi:10.1242/jcs.178285
Dong, X., Leksa, N. C., Chhabra, E. S., Arndt, J. W., Lu, Q., Knockenhauer, K. E., et al. (2019). The von Willebrand factor D′D3 assembly and structural principles for factor VIII binding and concatemer biogenesis. Blood 133, 1523–1533. doi:10.1182/blood-2018-10-876300
El-Mansi, S., and Nightingale, T. D. (2021). Emerging Mechanisms to Modulate VWF Release from Endothelial Cells. Int. J. Biochem. Cel Biol. 131, 105900. doi:10.1016/j.biocel.2020.105900
Ferraro, F., da Silva, M. L., Grimes, W., Lee, H. K., Ketteler, R., Kriston-Vizi, J., et al. (2016). Weibel-Palade body size modulates the adhesive activity of its von Willebrand Factor cargo in cultured endothelial cells. Sci. Rep. 6, 32473. doi:10.1038/srep32473
Ferraro, F., Kriston-Vizi, J., Metcalf, D. J., Martin-Martin, B., Freeman, J., Burden, J. J., et al. (2014). A Two-Tier Golgi-Based Control of Organelle Size Underpins the Functional Plasticity of Endothelial Cells. Develop. Cel 29, 292–304. doi:10.1016/j.devcel.2014.03.021
Ferraro, F., Patella, F., Costa, J. R., Ketteler, R., Kriston‐Vizi, J., and Cutler, D. F. (2020). Modulation of Endothelial Organelle Size as an Antithrombotic Strategy. J. Thromb. Haemost. 18, 3296–3308. doi:10.1111/jth.15084
Francis, C. R., Claflin, S., and Kushner, E. J. (2021).Synaptotagmin-Like Protein 2a Regulates Angiogenic Lumen Formation via Weibel-Palade Body Apical Secretion of Angiopoietin-2, Atvb. Arteriosclerosis, Thrombosis, and Vascular Biology, 41121.ATVBAHA, Dallas, USA, 1972–1986. doi:10.1161/ATVBAHA.121.316113
Han, X., Li, P., Yang, Z., Huang, X., Wei, G., Sun, Y., et al. (2017). Zyxin regulates endothelial von Willebrand factor secretion by reorganizing actin filaments around exocytic granules. Nat. Commun. 8, 14639. doi:10.1038/ncomms14639
Holthenrich, A., Drexler, H. C. A., Chehab, T., Naß, J., and Gerke, V. (2019). Proximity proteomics of endothelial Weibel-Palade bodies identifies novel regulator of von Willebrand factor secretion. Blood 134, 979–982. doi:10.1182/blood.2019000786
Karampini, E., Bierings, R., and Voorberg, J. (2020a). Orchestration of Primary Hemostasis by Platelet and Endothelial Lysosome-Related Organelles. ATVB 40, 1441–1453. doi:10.1161/ATVBAHA.120.314245
Karampini, E., Petra E. Bürgisser, P. E., Jenny Olins, J., Aat A. Mulder, A. A., Carolina R. Jost, C. R., Dirk Geerts, D., et al. (2020b). Sec22b Determines Weibel-Palade Body Length by Controlling Anterograde ER-Golgi Transport. haematol 106, 1138–1147. doi:10.3324/haematol.2019.242727
Karampini, E., Schillemans, M., Hofman, M., van Alphen, F., de Boer, M., Kuijpers, T. W., et al. (2019). Defective AP-3-dependent VAMP8 Trafficking Impairs Weibel-Palade Body Exocytosis in Hermansky-Pudlak Syndrome Type 2 Blood Outgrowth Endothelial Cells. Haematologica 104, 2091–2099. doi:10.3324/haematol.2018.207787
Kat, M., Bürgisser, P. E., Janssen, H., De Cuyper, I. M., Conte, I. L., Hume, A. N., et al. (2021). GDP/GTP Exchange Factor MADD Drives Activation and Recruitment of Secretory Rab GTPases to Weibel-Palade Bodies. Blood Adv. Bloodadvances. 2021. 202100482. doi:10.1182/bloodadvances.2021004827
Kiskin, N. I., Babich, V., Knipe, L., Hannah, M. J., and Carter, T. (2014). Differential Cargo Mobilisation within Weibel-Palade Bodies after Transient Fusion with the Plasma Membrane. PLoS ONE 9, e108093. doi:10.1371/journal.pone.0108093
Leebeek, F. W. G., and Eikenboom, J. C. J. (2016). Von Willebrand's Disease. N. Engl. J. Med. 375, 2067–2080. doi:10.1056/NEJMra1601561
Lenzi, C., Stevens, J., Osborn, D., Hannah, M. J., Bierings, R., and Carter, T. (2019). Synaptotagmin 5 Regulates Calcium-dependent Weibel-Palade Body Exocytosis in Human Endothelial Cells. J. Cel Sci., 221952. doi:10.1242/jcs.221952
Li, P., Wei, G., Cao, Y., Deng, Q., Han, X., Huang, X., et al. (2018). Myosin IIa is critical for cAMP-mediated endothelial secretion of von Willebrand factor. Blood 131, 686–698. doi:10.1182/blood-2017-08-802140
Lopes-da-Silva, M., McCormack, J. J., Burden, J. J., Harrison-Lavoie, K. J., Ferraro, F., and Cutler, D. F. (2019). A GBF1-dependent Mechanism for Environmentally Responsive Regulation of ER-Golgi Transport. Develop. Cel 49, 786–801. e6. doi:10.1016/j.devcel.2019.04.006
Lowenstein, C. J., Morrell, C. N., and Yamakuchi, M. (2005). Regulation of Weibel-Palade Body Exocytosis. Trends Cardiovasc. Med. 15, 302–308. doi:10.1016/j.tcm.2005.09.005
Ma, J., Zhang, Z., Yang, L., Kriston-Vizi, J., Cutler, D. F., and Li, W. (2016). BLOC-2 subunit HPS6 deficiency affects the tubulation and secretion of von Willebrand factor from mouse endothelial cells. J. Genet. Genomics 43, 686–693. doi:10.1016/j.jgg.2016.09.007
Matsushita, K., Morrell, C. N., Cambien, B., Yang, S.-X., Yamakuchi, M., Bao, C., et al. (2003). Nitric Oxide Regulates Exocytosis by S-Nitrosylation of N-Ethylmaleimide-Sensitive Factor. Cell 115, 139–150. doi:10.1016/S0092-8674(03)00803-1
McCormack, J. J., Lopes da Silva, M., Ferraro, F., Patella, F., and Cutler, D. F. (2017). Weibel−Palade Bodies at a Glance. J. Cel Sci. 130, 3611–3617. doi:10.1242/jcs.208033
Michaux, G., and Cutler, D. F. (2004). How to Roll an Endothelial Cigar: The Biogenesis of Weibel-Palade Bodies. Traffic 5, 69–78. doi:10.1111/j.1600-0854.2004.00157.x
Mietkowska, M., Schuberth, C., Wedlich-Söldner, R., and Gerke, V. (20191866). Actin Dynamics during Ca2+-dependent Exocytosis of Endothelial Weibel-Palade Bodies. Biochim. Biophys. Acta (Bba) - Mol. Cel Res. 1866, 1218–1229. doi:10.1016/j.bbamcr.2018.11.010
Miklavc, P., Ehinger, K., Sultan, A., Felder, T., Paul, P., Gottschalk, K.-E., et al. (2015). Actin Depolymerisation and Crosslinking Join Forces with Myosin II to Contract Actin coats on Fused Secretory Vesicles. J. Cel Sci. 128, 1193–1203. doi:10.1242/jcs.165571
Miteva, K. T., Pedicini, L., Wilson, L. A., Jayasinghe, I., Slip, R. G., Marszalek, K., et al. (2019). Rab46 Integrates Ca2+ and Histamine Signaling to Regulate Selective Cargo Release from Weibel-Palade Bodies. J. Cel Biol. 218, 2232–2246. doi:10.1083/jcb.201810118
Mourik, M. J., Faas, F. G. A., Zimmermann, H., Voorberg, J., Koster, A. J., and Eikenboom, J. (2015). Content Delivery to Newly Forming Weibel-Palade Bodies Is Facilitated by Multiple Connections with the Golgi Apparatus. Blood 125, 3509–3516. doi:10.1182/blood-2014-10-608596
Nguyen, T. T. N., Koerdt, S. N., and Gerke, V. (2020). Plasma Membrane Phosphatidylinositol (4,5)-bisphosphate Promotes Weibel-Palade Body Exocytosis. Life Sci. Alliance 3, e202000788. doi:10.26508/lsa.202000788
Nightingale, T. D., McCormack, J. J., Grimes, W., Robinson, C., Lopes da Silva, M., White, I. J., et al. (2018). Tuning the Endothelial Response: Differential Release of Exocytic Cargos from Weibel-Palade Bodies. J. Thromb. Haemost. 16, 1873–1886. doi:10.1111/jth.14218
Nightingale, T. D., Pattni, K., Hume, A. N., Seabra, M. C., and Cutler, D. F. (2009). Rab27a and MyRIP Regulate the Amount and Multimeric State of VWF Released from Endothelial Cells. Blood 113, 5010–5018. doi:10.1182/blood-2008-09-181206
Nightingale, T. D., White, I. J., Doyle, E. L., Turmaine, M., Harrison-Lavoie, K. J., Webb, K. F., et al. (2011). Actomyosin II contractility expels von Willebrand factor from Weibel-Palade bodies during exocytosis. J. Cel Biol. 194, 613–629. doi:10.1083/jcb.201011119
Patella, F., and Cutler, D. F. (2020). Regulator of G protein signalling 4 controls secretion of von Willebrand factor to the subendothelial matrix. J. Cel Sci. 133. doi:10.1242/jcs.247312
Poeter, M., Brandherm, I., Rossaint, J., Rosso, G., Shahin, V., Skryabin, B. V., et al. (2014). Annexin A8 Controls Leukocyte Recruitment to Activated Endothelial Cells via Cell Surface Delivery of CD63. Nat. Commun. 5, 3738. doi:10.1038/ncomms4738
Pulido, I. R., Jahn, R., and Gerke, V. (2011). VAMP3 Is Associated with Endothelial Weibel-Palade Bodies and Participates in Their Ca2+-dependent Exocytosis. Biochim. Biophys. Acta (Bba) - Mol. Cel Res. 1813, 1038–1044. doi:10.1016/j.bbamcr.2010.11.007
Raposo, G., Marks, M. S., and Cutler, D. F. (2007). Lysosome-Related Organelles: Driving post-Golgi Compartments into Specialisation. Curr. Opin. Cel Biol. 19, 394–401. doi:10.1016/j.ceb.2007.05.001
Rojo Pulido, I., Nightingale, T. D., Darchen, F., Seabra, M. C., Cutler, D. F., and Gerke, V. (2011). Myosin Va Acts in Concert with Rab27a and MyRIP to Regulate Acute Von-Willebrand Factor Release from Endothelial Cells. Traffic 12, 1371–1382. doi:10.1111/j.1600-0854.2011.01248.x
Sadler, J. E. (2008). Von Willebrand Factor, ADAMTS13, and Thrombotic Thrombocytopenic Purpura. Blood 112, 11–18. doi:10.1182/blood-2008-02-078170
Schillemans, M., Karampini, E., Hoogendijk, A. J., Wahedi, M., van Alphen, F. P. J., van den Biggelaar, M., et al. (2019a). Interaction Networks of Weibel-Palade Body Regulators Syntaxin-3 and Syntaxin Binding Protein 5 in Endothelial Cells. J. Proteomics 205, 103417. doi:10.1016/j.jprot.2019.103417
Schillemans, M., Karampini, E., Kat, M., and Bierings, R. (2019b). Exocytosis of Weibel-Palade Bodies: How to Unpack a Vascular Emergency Kit. J. Thromb. Haemost. 17, 6–18. doi:10.1111/jth.14322
Schillemans, M., Karampini, E., van den Eshof, B. L., Gangaev, A., Hofman, M., van Breevoort, D., et al. (2018). Weibel-Palade Body Localized Syntaxin-3 Modulates Von Willebrand Factor Secretion from Endothelial Cells. ATVB 38, 1549–1561. doi:10.1161/ATVBAHA.117.310701
Schneppenheim, R., and Budde, U. (2011). von Willebrand factor: the complex molecular genetics of a multidomain and multifunctional protein. J. Thromb. Haemost. 9, 209–215. doi:10.1111/j.1538-7836.2011.04324.x
Sharda, A. V., Barr, A. M., Harrison, J. A., Wilkie, A. R., Fang, C., Mendez, L. M., et al. (2020). VWF Maturation and Release Are Controlled by 2 Regulators of Weibel-Palade Body Biogenesis: Exocyst and BLOC-2. Blood 136, 2824–2837. doi:10.1182/blood.2020005300
Simons, M., and Raposo, G. (2009). Exosomes - Vesicular Carriers for Intercellular Communication. Curr. Opin. Cel Biol. 21, 575–581. doi:10.1016/j.ceb.2009.03.007
Stevenson, N. L., White, I. J., McCormack, J. J., Robinson, C., Cutler, D. F., and Nightingale, T. D. (2017). Clathrin-mediated post-fusion Membrane Retrieval Influences the Exocytic Mode of Endothelial Weibel-Palade Bodies. J. Cel Sci. 130, 2591–2605. doi:10.1242/jcs.200840
Streetley, J., Fonseca, A.-V., Turner, J., Kiskin, N. I., Knipe, L., Rosenthal, P. B., et al. (2019). Stimulated Release of Intraluminal Vesicles from Weibel-Palade Bodies. Blood 133, 2707–2717. doi:10.1182/blood-2018-09-874552
Valentijn, K. M., van Driel, L. F., Mourik, M. J., Hendriks, G.-J., Arends, T. J., Koster, A. J., et al. (2010). Multigranular Exocytosis of Weibel-Palade Bodies in Vascular Endothelial Cells. Blood 116, 1807–1816. doi:10.1182/blood-2010-03-274209
van Breevoort, D., Snijders, A. P., Hellen, N., Weckhuysen, S., van Hooren, K. W. E. M., Eikenboom, J., et al. (2014). STXBP1 Promotes Weibel-Palade Body Exocytosis through its Interaction with the Rab27A Effector Slp4-A. Blood 123, 3185–3194. doi:10.1182/blood-2013-10-535831
van Breevoort, D., van Agtmaal, E. L., Dragt, B. S., Gebbinck, J. K., Dienava-Verdoold, I., Kragt, A., et al. (2012). Proteomic Screen Identifies IGFBP7 as a Novel Component of Endothelial Cell-specific Weibel-Palade Bodies. J. Proteome Res. 11, 2925–2936. doi:10.1021/pr300010r
van Mourik, J. A., Romani de Wit, T., and Voorberg, J. (2002). Biogenesis and Exocytosis of Weibel-Palade Bodies. Histochem. Cel Biol 117, 113–122. doi:10.1007/s00418-001-0368-9
Wagner, D. D., Olmsted, J. B., and Marder, V. J. (1982). Immunolocalization of von Willebrand protein in Weibel-Palade bodies of human endothelial cells. J. Cel Biol. 95, 355–360. doi:10.1083/jcb.95.1.355
Watanabe, A., Hataida, H., Inoue, N., Kamon, K., Baba, K., Sasaki, K., et al. (2021). Arf GTPase-activating proteins SMAP1 and AGFG2 regulate the size of Weibel-Palade bodies and exocytosis of von Willebrand factor. Biol. Open 10. doi:10.1242/bio.058789
Weibel, E. R., and Palade, G. E. (1964). New Cytoplasmic Components in Arterial Endothelia. J. Cel Biol. 23, 101–112. doi:10.1083/jcb.23.1.101
Zenner, H. L., Collinson, L. M., Michaux, G., and Cutler, D. F. (2007). High-pressure Freezing Provides Insights into Weibel-Palade Body Biogenesis. J. Cel Sci. 120, 2117–2125. doi:10.1242/jcs.007781
Zhou, H. J., Qin, L., Zhang, H., Tang, W., Ji, W., He, Y., et al. (2016). Endothelial Exocytosis of Angiopoietin-2 Resulting from CCM3 Deficiency Contributes to Cerebral Cavernous Malformation. Nat. Med. 22, 1033–1042. doi:10.1038/nm.4169
Zografou, S., Basagiannis, D., Papafotika, A., Shirakawa, R., Horiuchi, H., Auerbach, D., et al. (2012). Rab-genome Analysis Reveals Novel Insights in Weibel-Palade Body Exocytosis. J. Cel Sci. 125, 4780–4790. doi:10.1242/jcs.104174
Keywords: calcium, exocytosis, lysosome-related organelle, secretory organelles, hemostasis
Citation: Naß J, Terglane J and Gerke V (2021) Weibel Palade Bodies: Unique Secretory Organelles of Endothelial Cells that Control Blood Vessel Homeostasis. Front. Cell Dev. Biol. 9:813995. doi: 10.3389/fcell.2021.813995
Received: 12 November 2021; Accepted: 30 November 2021;
Published: 16 December 2021.
Edited by:
Mitsuo Tagaya, Tokyo University of Pharmacy and Life Sciences, JapanReviewed by:
Ruben Bierings, Erasmus Medical Center, NetherlandsThomas Daniel Nightingale, Queen Mary University of London, United Kingdom
Copyright © 2021 Naß, Terglane and Gerke. This is an open-access article distributed under the terms of the Creative Commons Attribution License (CC BY). The use, distribution or reproduction in other forums is permitted, provided the original author(s) and the copyright owner(s) are credited and that the original publication in this journal is cited, in accordance with accepted academic practice. No use, distribution or reproduction is permitted which does not comply with these terms.
*Correspondence: Volker Gerke, Z2Vya2VAdW5pLW11ZW5zdGVyLmRl
†These authors have contributed equally to this work and share first authorship