- 1The Second Clinical Medical College of Nanchang University, The Second Affiliated Hospital of Nanchang University, Nanchang, China
- 2Department of Pharmacology and Systems Physiology, University of Cincinnati College of Medicine, Cincinnati, OH, United States
- 3Department of Anesthesiology, The Second Affiliated Hospital of Nanchang University, Nanchang, China
- 4Department of Metabolism and Endocrinology, The Second Affiliated Hospital of Nanchang University, Nanchang, China
Diabetes is a widespread metabolic disease with various complications, including diabetic nephropathy, retinopathy, cardiomyopathy, and other cardiovascular or cerebrovascular diseases. As the prevalence of diabetes increases in all age groups worldwide, diabetes and its complications cause an emerging public health burden. NLRP3 inflammasome is a complex of several proteins that play a critical role in inflammatory response and various diseases, including diabetes and its complications. Accumulating evidences indicate that NLRP3 inflammasome contributes to the development of diabetes and diabetic complications and that NLRP3 inflammation inactivation is beneficial in treating these illnesses. Emerging evidences suggest the critical role of long non-coding RNAs (lncRNAs) in regulating NLRP3 inflammasome activity in various diseases. LncRNAs are non-coding RNAs exceeding 200 nucleotides in length. Its dysregulation has been linked to the development of diseases, including diabetes. Recently, growing evidences hint that regulating lncRNAs on NLRP3 inflammasome is critical in developing and progressing diabetes and diabetic complications. Here, we discuss the role of lncRNAs in regulating NLRP3 inflammasome as well as its participation in diabetes and diabetic complications, providing novel insights into developing future therapeutic approaches for diabetes.
Introduction
Diabetes is a chronic metabolic disorder characterized by hyperglycaemia. Persistent hyperglycaemia and long-term metabolic disorders can damage a wide range of organs throughout the body, including diabetic nephropathy, retinopathy, cardiomyopathy, and many other complications (Forbes and Cooper, 2013; Reddy et al., 2015; Vujosevic et al., 2020). As the prevalence for all age groups increases worldwide, diabetes and its related complications not only impair physical and psychological properties of people but also impose a tremendous burden on society, both in economic and well-being terms (Cho et al., 2018). Therefore, a better understanding of the pathogenesis of diabetes and its complications is crucial for identifying therapeutic targets and developing effective medications.
The pathogenesis of diabetes and its complications is complex and encompasses a plethora of distinct pathways. Inflammation plays a vital role in diabetes and its complications, and the underlying mechanisms have been investigated for a prolonged time. An early clinical trial has indicated that high inflammation levels were strongly associated with type 2 diabetes (Bertoni et al., 2010). Chen G. and Goeddel D. V. depicted an authoritative tumor necrosis factor receptor-1 (TNF-R1)-mediated inflammatory signaling pathway that was implicated in the pathogenesis of diabetes (Chen and Goeddel, 2002). Obesity-associated diabetes causes an intensified crisis via numerous fat-derived molecules, such as IkappaB kinase, which seriously provoke inflammation (Lazar, 2005; Olefsky, 2009). In addition, previous research has proved that chronic inflammatory stimulation can cause a surge in plasma glucose levels by inhibiting the rate-limiting enzyme of bile acid biosynthesis, CYP7A1, which is linked to hepatic mevalonate pathway regulation (Okin and Medzhitov, 2016). Recently, several lncRNAs have been implicated in the inflammation associated with diabetes and its complications. Kato and others discovered that a megacluster of nearly 40 microRNAs (miRNAs) hosted by long non-coding RNA-megacluster (lnc-MGC) is coordinately upregulated to induce renal extracellular matrix accumulation and glomerular hypertrophy through cumulative effects in diabetic nephropathy (Kato et al., 2016). A study revealed that long non-coding RNA (lncRNA) and microRNA (miRNA) are correlated with inflammatory response, oxidative stress, apoptosis, hypertrophy, and fibrosis in diabetic cardiomyopathy, implying the development of new therapeutic and preventative strategies in diabetes complications (Jakubik et al., 2021).
Currently, NLRP3 inflammasome activation is a prominent mechanism of inflammation response (Haneklaus et al., 2013; Swanson et al., 2019; Sharma and Kanneganti, 2021). NLRP3 inflammasomes are innate immune system protein complexes composed of NLRP3 (Figure 1); the adaptor protein apoptosis-associated speck-like protein (ASC), proinflammatory caspase, and caspase-1 (Swanson et al., 2019). NLRP3 is an intracellular sensor that detects a broad range of microbial motifs, endogenous danger signals, and environmental irritants. ASC is mainly distributed in the nucleus of human monocytes/macrophages. It quickly transfers to the cytoplasm under stress, connecting NLRP3 and pro-caspase-1. Caspase-1 is the effector protein of NLRP3 inflammasome, cleaved by the precursor molecule pro-caspase-1. Recently, NIMA-related kinase 7 (NEK7) is a serine-threonine kinase that appears to be a component specific to NLRP3 inflammasome (Shi H. et al., 2016; He et al., 2016; Schmid-Burgk et al., 2016). Upon inflammasome activation, NEK7 oligomerizes with NLRP3 into a complex essential for ASC speck formation and caspase-1 activation. Since inflammasome activation is an inflammatory process, it must be strictly regulated. With few exceptions, inflammasome activation is considered a two-step process (Guo et al., 2015; Swanson et al., 2019). First, it must be primed, and then it can be activated. The first step is to promote nuclear factor-κB (NF-κB) into the nucleus and upregulate the expression of NLRP3, caspase-1, and pro-IL-1β (Bauernfeind et al., 2009). This transcriptional upregulation can be induced through pattern recognition receptors (PRRs) such as Toll-like receptors (TLRs) recognizing various pathogen-associated molecular patterns (PAMPs) or damage-associated molecular patterns (DAMPs), or through cytokines such as TNF and IL-1β (Xing et al., 2017). The second step is activated by recognizing NLRP3 activators such as ATP, pore-forming toxins, viral RNA, or particulate matter. This cellular and molecular effect promotes the oligomerization of inflammasomes and leads to caspase-1-dependent release of pro-inflammatory cytokines IL-1β and IL-18, as well as gasdermin D (GSDMD)-mediated pyroptotic cell death (Rathinam and Fitzgerald, 2016; Swanson et al., 2019).
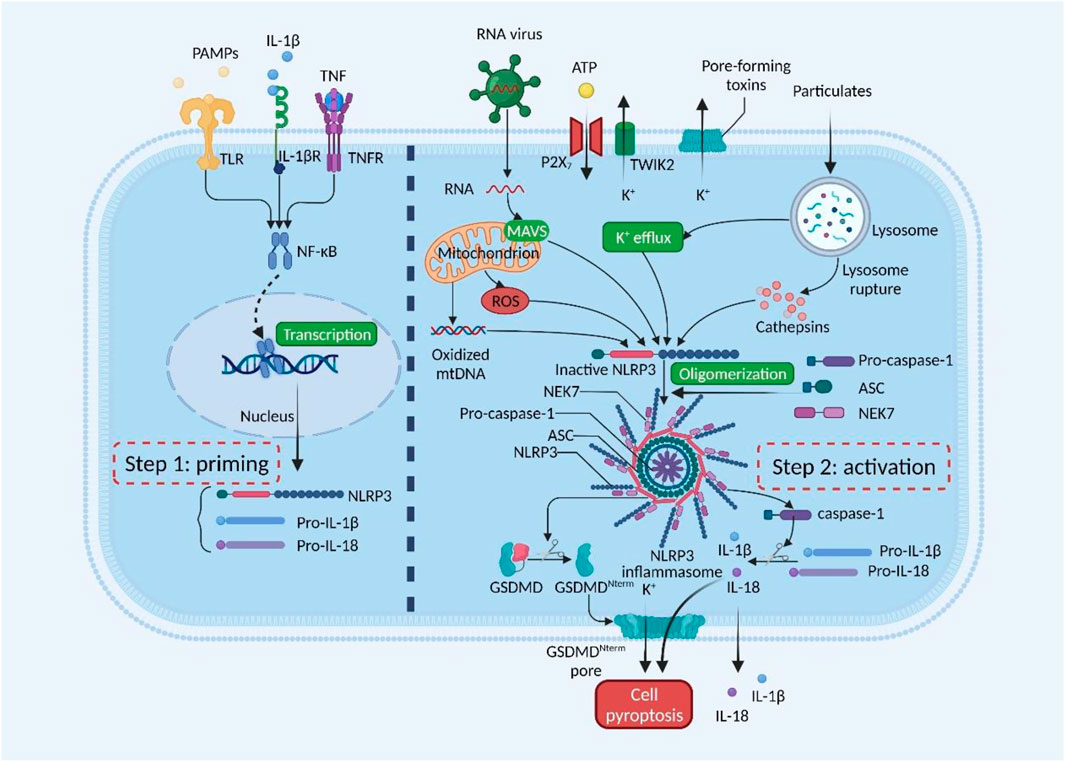
FIGURE 1. Mechanisms of NLRP3 inflammasome activation. NLRP3 inflammasome must be primed, followed by activation. The priming step is activated by pathogen-associated molecular patterns (PAMPs) or cytokines, leading to transcriptional upregulation of NLRP3, pro-IL-1β, and pro-IL-18. The activation step is induced by numerous PAMPs or damage-associated molecular patterns (DAMPs), such as particulates, pore-forming toxins, and ATP. RNA viruses activate NLRP3 through mitochondrial antiviral signaling protein (MAVS) on the mitochondrial outer membrane. NLRP3 inflammasome activates caspase-1, which in turn cleaves pro-IL-1β and pro-IL-18. Gasdermin D (GSDMD) is also cleaved and inserted into the membrane, forming pores and inducing pyroptosis. GSDMDNterm, GSDMD amino-terminal cell death domain; NEK7, NIMA-related kinase 7; NF-κB, nuclear factor-κB; P2X7, P2X purinoceptor 7; ROS, reactive oxygen species; TLR, Toll-like receptor; TNF, tumor necrosis factor; TNFR, tumor necrosis factor receptor; TWIK2, two-pore domain weak inwardly rectifying K+ channel 2. This figure was created with BioRender.com.
Increasing evidence demonstrates that NLRP3 inflammasome is implicated in developing diabetes and associated complications (Schroder et al., 2010; Wada and Makino, 2016; Tang and Yiu, 2020; Li et al., 2021). Diabetes and its complications activate NLRP3 inflammasome through hyperglycemia, hypercholesterolemia, and hyperuricemia, resulting in a rise of IL-1β and IL-18 levels and inducing inflammatory response (Schroder et al., 2010). The current study revealed that reducing NLRP3 inflammasome activation can prevent and reduce diabetic complications (Ashrafizadeh et al., 2021; Li et al., 2021). As an upstream regulator of NLRP3 inflammasome, lncRNA can exert control over diabetes and its complications (Li et al., 2017b). Understanding the mechanism by which lncRNA regulates NLRP3 inflammasome is significant to discover novel therapeutic targets for diabetes and its complications. This review summarizes the mechanism by which lncRNAs contribute to the development of diabetes and its complications by regulating NLRP3 inflammasome.
The Mechanism of LncRNA Involved in NLRP3 Inflammasome Regulation
LncRNAs are linear non-coding RNAs having a length of more than 200 nucleotides (Hon et al., 2017). According to their relative protein-coding gene location in the genome, lncRNAs can be classified into five types (Table 1) (Ponting et al., 2009): (A) sense lncRNAs: their transcriptional direction is the same as that of neighboring protein-coding gene; (B) antisense lncRNAs: their transcriptional direction is opposite to that of neighboring protein-coding genes; (C) bidirectional lncRNAs: they can be simultaneously transcribed from the same and opposite direction with neighboring protein-coding genes; transcription occurs in the opposite two directions; (D) intronic lncRNAs: they can be transcribed from the intronic regions of genes; and (E) intergenic lncRNAs: they are derived from intergenic transcription of two genes. In addition, lncRNAs can be classified into four categories based on their biological functions (Table 1) (Guo et al., 2019): (A) signals lncRNAs: act as molecular signals or indicators of transcriptional activity; (B) decoy lncRNAs: bind to and sequester other regulatory RNAs or proteins; (C) guide lncRNAs: direct the localization of ribonucleoprotein complexes to specific targets; (D): scaffold lncRNAs: act as platforms for the assembly of relevant molecular elements (proteins and/or RNAs).
Recent evidence indicates that lncRNAs play essential functions in many biological processes, such as X chromosome inactivation, dosage compensation, genomic imprinting, chromatin modification and remodeling, cellular proliferation, differentiation, and apoptosis (Kopp and Mendell, 2018; Nair et al., 2020). The mechanisms involved are mainly regulation at gene expression levels. In general, lncRNAs regulate gene expression through multiple pathways and molecular mechanisms at three levels: epigenetic, transcriptional, and post-transcriptional (Kopp and Mendell, 2018). On the one hand, lncRNAs can regulate gene expression at the epigenetic level (dosage compensating effects, chromatin modifications, and genomic imprinting). On the other hand, lncRNAs can be regulated at the transcriptional level by interfering with the transcription of messenger RNA (mRNA) or other non-coding RNAs, complexing with proteins, or acting through cis-acting elements (Gil and Ulitsky, 2020). Moreover, lncRNAs can be regulated at the post-transcriptional level by participating in mRNA degradation, regulating mRNA translation, and competitively binding miRNAs (Thomson and Dinger, 2016).
As known, lncRNAs not only regulate cell proliferation, differentiation, and metabolism but also participate in the pathological processes of various diseases, including cancer, diabetes, and neurodegenerative diseases (Boon et al., 2016; Leung and Natarajan, 2018; Feng et al., 2019; Guo et al., 2019). Diabetes-induced inflammation has been linked to the development of a variety of illnesses. As long-term hyperglycemia induces inflammatory response, vascular and target organs damage occurs (Nolan et al., 2011), ultimately increasing the incidence of tumors (Shikata et al., 2013; Singh et al., 2021), cardiovascular and cerebrovascular diseases (Kozakova et al., 2019; Eckel et al., 2021), and other infections (D'Elia et al., 1991; Knapp, 2013; Fang et al., 2021). NLRP3 inflammasome regulation by lncRNAs is a current research hotspot and has been widely documented in many diseases, such as inflammatory bowel diseases (Samoilă et al., 2020), Parkinson’s disease (Haque et al., 2020; Cao et al., 2021), and cancer (Farooqi et al., 2020; Tang et al., 2020). Studies indicate that lncRNAs and NLRP3 inflammasome are overexpressed in diabetes complications, implying that lncRNAs could cause inflammatory responses by activating NLRP3 inflammasome (Hu et al., 2019; Farooqi et al., 2020; Liu et al., 2020).
Notably, a vast number of studies have demonstrated that upregulation or downregulation of lncRNAs can inhibit NLRP3 inflammasome activation and reduce inflammatory response, hence improving diabetic complications (Xie et al., 2019; Liu et al., 2021). LncRNAs indirectly regulate NLRP3 inflammasome by acting as competing endogenous RNAs (ceRNAs) and sponging miRNAs (Figure 2). miRNAs could directly regulate downstream protein NLRP3 expression, ultimately affecting NLRP3/IL-1β pathway. Numerous investigations have demonstrated this lncRNA/miRNA-mediated NLRP3 inflammasome regulation mechanism in diabetic complications (Che et al., 2020c; Du et al., 2020; Liu et al., 2020; Xu et al., 2020; Wang and Zhao, 2021).
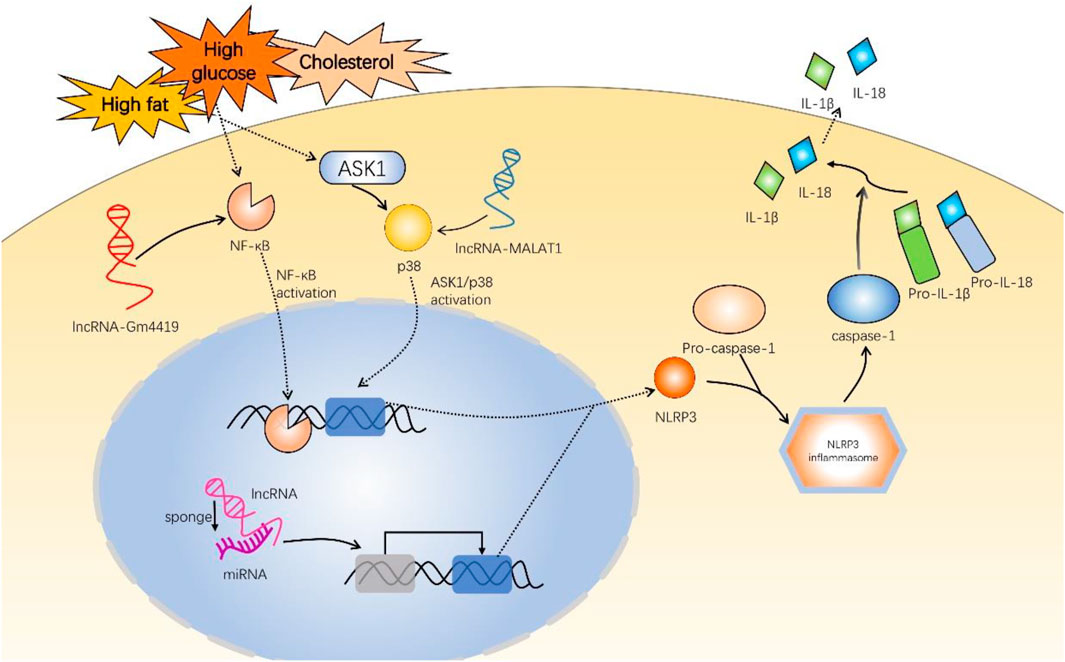
FIGURE 2. Mechanisms of long non-coding RNAs involved in inflammatory responses of diabetic complications via NLRP3 inflammasome. These experiments used high-glucose induction to establish diabetic mouse models. LncRNA-Gm4419 activates NF-κB pathway to upregulate NLRP3 expression in DN. LncRNA-MALAT1 activates ASK1/p38 pathway to upregulate NLRP3 in DR. In addition, most lncRNAs, such as ANRIL, Kcnq1ot1, NEAT1, HCP5, SNHG16, H19, and HCG18 promote or inhibit NLRP3 expression by sponging miRNA and regulating downstream target genes. NLRP3 and pro-caspase-1 are indispensable to NLRP3 inflammasome assembly. NLRP3 inflammasome activates pro-caspase-1 into caspase-1, promoting IL-1β and IL-18 generation. NF-κB, nuclear factor-κB; ASK1, apoptosis signal-regulating kinase 1; MALAT1, metastasis-associated lung adenocarcinoma transcript 1; lncRNA, long non-coding RNA; miRNA, microRNA; ANRIL, antisense noncoding RNA in the INK4 locus; Kcnq1ot1, Kcnq1 overlapping transcript 1; NEAT1, nuclear-enriched abundant transcript 1; HCP5, HLA complex P5; SNHG16, small nucleolar RNA host gene 16; HCG18, HLA complex group 18.
Regulation of LncRNA on NLRP3 Inflammation in Diabetes and Its Complications
Nephropathy
Diabetic nephropathy (DN) is a microvascular complication caused by diabetes-induced glomerular capillary damage. The main pathogenesis comprises glucose metabolism disorder, abnormal renal hemodynamics, extracellular matrix accumulation, abnormal expression of cytokines, genetic factors, and reactive oxygen species (ROS) formation (Wolf, 2004).
Numerous studies have demonstrated that inflammation plays a key role in DN pathogenesis, proving possible regulatory mechanisms (Matoba et al., 2019). NF-κB signal pathway is not only one of the principal inflammatory signal pathways in DN progression but also the signal pathway that governs DNA transcription in vivo. One recent study revealed that long intergenic noncoding RNA (lincRNA)-Gm4419 expression is elevated in mesangial cells (MCs) under a high glucose medium. Gm4419 can directly interact with P50, a functional subunit of NF-κB, activating NF-κB pathway. Meanwhile, P50 promotes transcription of NLRP3 inflammasome and inflammatory cytokines. These results indicate that Gm4419 may contribute to inflammation, fibrosis, and proliferation in MCs exposed to high glucose via NF-κB/NLRP3 inflammasome signaling pathway (Yi et al., 2017). NLRP3 inflammasome activation causes podocyte pyroptosis, proliferation of MCs, and renal tubular injury (Xiong et al., 2021). Interestingly, P50 can form a positive synergistic Gm4419 regulation in MCs (Yi et al., 2017).
Studies have indicated that lncRNAs can act as regulators by interacting with particular miRNAs in DN. Zhang C et al. found that lncRNAs promote podocyte pyroptosis by NLRP3 upregulation through interaction with microRNA (miR)-486a-3p. Additionally, podocytes were induced by sublytic complement C5b-9 (sC5b-9) in vitro (Zhang et al., 2021). This means that lncRNA/miRNA/NLRP3 signal pathway can be activated under specific conditions. Another experiment revealed that DN serum samples and high-glucose (HG)-treated MCs increased lncRNA-HLA complex P5 (HCP5) and high mobility group AT-hook 2 (HMGA2) expression and decreased miR-93-5p expression. Experiments have verified that lncRNA-HCP5 upregulates HMGA2 expression via miR-93-5p sponging. Additionally, HMGA2 can promote the release of inflammatory cytokines, such as TNF-α, IL-1β, and IL-6. Therefore, targeting lncRNA-HCP5/miR-93-5p/HMGA2 axis can inhibit hyperproliferation, fibrosis, and inflammation of HG-treated MCs (Wang X. et al., 2021). In addition, there are additional similar signaling pathways that have a similar function in DN (Li et al., 2017b; Liu et al., 2020; Zhan et al., 2020; Zhu et al., 2020). Specifically, long noncoding RNA-growth arrest-specific 5 (lncRNA-GAS5) expression was downregulated in HG-induced human renal tubular (HK-2) cells. Meanwhile, GAS5 overexpression could downregulate the expression of NLRP3, cleaved-caspase1, IL-1β, and GSDMD-N by directly targeting miR-452-5p (Xie et al., 2019). However, the specific inhibitory mechanism remains unclear. It may competitively inhibit NLRP3 expression by promoting the expression of specific downstream genes.
Thioredoxin-interacting protein (TXNIP) as a mediator of OS (oxidative stress) was implicated in activating NLRP3 inflammasome in DN progression (Samra et al., 2016). Both lncRNA-antisense noncoding RNA in the INK4 locus (ANRIL) and TXNIP expressions were significantly increased in DN kidney tissues and HG-treated HK-2 cells, whereas miR-497 was reduced. ANRIL has been proved to promote pyroptosis in DN, most likely via miR-497/TXNIP/NLRP3 pathway transmission (Wang and Zhao, 2021). Another research revealed that maternally expressed 3 (MEG3) knockdown resisted hyperoxia-induced lung cell pyroptosis by promoting miR-18a expression, whereas miR-18a inhibited TXNIP (Zou et al., 2020).
Overall, these outcomes reveal that lncRNA plays a critical part in DN pathogenesis (Table 2). In recent years, DN-specific processes, such as podocyte loss, glomerulosclerosis, and tubulointerstitial fibrosis, mediated by lncRNA through acting on NLRP3 inflammasome, have garnered considerable attention. However, the critical regulatory targets remain unknown. Further mechanism research is required to provide new ideas for DN treatment.
Retinopathy
Diabetic retinopathy (DR) is a significant consequence of diabetes caused by diabetic microvascular disease. Approximately one-third of diabetic patients have DR (Wong et al., 2016). The pathophysiological processes of DR mainly include abnormal proliferation, migration, and neovascularization in the retina (Cai et al., 2021).
Although the mechanisms by which lncRNAs contribute to DR remain largely unclear, an increasing number of studies has demonstrated critical regulatory functions of various lncRNAs in microvascular dysfunction. For instance, Yan et al. has explored the mechanism of retinal microvascular dysfunction caused by diabetes and found that lncRNA-myocardial infarction-associated transcript (MIAT) expression increased in diabetic retinopathy. MIAT can act as a competing endogenous RNA, forming a feedback loop with vascular endothelial growth factor and miR-150-5p to regulate endothelial cell function, thereby contributing to pathological angiogenesis (Yan et al., 2015).
DR is a major cause of blindness in middle-aged and elderly patients. Visual function is a significant function of the body. If it can be protected early, it improves health-related quality of life in patients. Recently, one research has demonstrated that lncRNA small nucleolar RNA host gene 16 (SNHG16) upregulation in HG-stimulated human retinal microvascular endothelial cells (hRMECs) reduces proliferative DR-related abnormalities in cell proliferation, migration, and angiogenesis via regulating miR-146a-5p/interleukin-1 receptor-associated kinase 1 (IRAK1) and miR-7-5p/insulin receptor substrate 1 (IRS1) to activate NF-κB and phosphatidylinositol 3-kinase (PI3K)/AKT signaling pathways (Cai et al., 2021). It was also confirmed that SNHG16 exerts its function by isolating miR-146a-5p and miR-7-5p (Cai et al., 2021). Therefore, SNHG16 can guide individual therapy in DR. Another experiment revealed that in retinal pigment epithelial (ARPE-19) cells with HG conditions, lncRNA H19 and silence information regulator factor-related enzymes 1 (SIRT1) decreased while miR-19b increased. Besides, SIRT1 suppresses the expression of inflammatory cytokines, such as TNF-α, IL-1β, and IL-6 (Luo et al., 2021).
LncRNA metastasis-associated lung adenocarcinoma transcript 1 (MALAT1) knockdown prevents hyper-proliferation of retinal endothelial cells through p38 mitogen-activated protein kinase (MAPK) signaling (Liu et al., 2014). This year, another research has demonstrated that NLRP3 promoted tube formation and angiogenesis of retinal microvascular endothelial cells (Zou et al., 2021). Also, the findings indicated that NLRP3-mediated aberrant retinal angiogenesis in DR was regulated via apoptosis signal-regulating kinase 1 (ASK1)/p38 axis (Zou et al., 2021). Obviously, MALAT1 regulates diabetes-related retinal vessel function by activating ASK1/p38/NLRP3 signaling pathway.
Cardiomyopathy
Diabetic cardiomyopathy (DCM) is a serious end-stage complication related to diabetes. Nowadays, it is recognized that DCM pathogenesis includes hyperglycemia, protein non-enzymatic glycosylation, oxidative stress, myocardial fibrosis, abnormal calcium ion transport, increased fatty acid oxidation, neuroendocrine function activation, etc. (Yilmaz et al., 2015).
Myocardial fibrosis is one of the main causes of DCM. One study revealed that lncRNA-MALAT1 is elevated in diabetic mice and cardiac fibroblasts (CFs) treated with high glucose. Melatonin has the function of reducing collagen production in CFs treated with high glucose. It suppresses lncRNA-MALAT1/miR-141-mediated inflammatory activation of NLRP3 inflammasome and transforming growth factor (TGF)-β1/Smads signaling to produce anti-myocardial fibrosis effects (Che et al., 2020b). Other researchers discovered that lncRNA-Kcnq1ot1 was highly upregulated in diabetic myocardial tissues and CFs cultured under high glucose. After silencing lncRNA-Kcnq1 overlapping transcript 1 (Kcnq1ot1), miR-214-3p can inhibit caspase-1 due to the competitive binding between Kcnq1ot1 and miR-214-3p turns into loosening. Additionally, its downstream inflammatory cytokine IL-1β reduces collagen deposition and myocardial fibrosis (Yang et al., 2018). Therefore, Kcnq1ot1/miR-214-3p/caspase-1 regulatory signaling pathway is critical for regulating DCM myocardial fibrosis.
Inflammation has a key role in DCM development and progression. A study in 2020 revealed that lncRNA-GAS5 expression was upregulated in AC16 cardiomyocytes induced by high glucose. Additional studies indicated that lncRNA-GAS5 could competitively bind to miR-21-5p. Because miR-21-5p targets Toll-like receptor 4 (TLR4), silencing GAS5 can partially inhibit miR-21-5p-mediated TLR4/NF-κB signaling pathway, hence reducing inflammatory response triggered by high glucose (Zhao et al., 2020). However, another study concluded the opposite result. Xu, Y. et al. induced cardiac muscle cell line (HL-1) cardiomyocytes by high glucose and found that GAS5 was severely downregulated in DCM mice. Further experiments revealed that GAS5 overexpression could inhibit NLRP3 activation by regulating miR-34b-3p/aryl hydrocarbon receptor (AHR) signaling pathway, thereby reducing cardiomyocytes pyroptosis (Xu et al., 2020). The different results may be due to differences between cell lines or because multiple regulatory pathways coexist in the cell. As DCM is a complication of diabetes, the principal Frontier of its research is self-evident. To summarize, these results imply that lncRNA might be an underlying therapeutic target for DCM by alleviating NLRP3 inflammasome activation, fibrosis, and apoptosis.
Peripheral Neuropathy
Diabetic peripheral neuropathy (DPN) is a ubiquitous complication of diabetes. Redox-sensitive transcription factors such as NF-кB play a critical role in triggering the cascade of cytokine and chemokine production, including proinflammatory cytokines IL-1β, IL-6, TNF-α, etc. (Zhou and Zhou, 2014). These are key inflammatory factors downstream of NLRP3 inflammasome and are involved in inflammatory response of DPN, which can not only enhance inflammation and immune response but also promote activation of various downstream cell oxidative stress pathways. Wang, C. et al. employed whole-transcriptome sequencing technology to systematically analyze the differential expression of lncRNAs, mRNAs, and miRNAs in Schwann cells (SCs) of DPN and control rats and constructed lncRNA–miRNA–mRNA competing endogenous RNA (ceRNA) network of SCs. This network has identified the inhibited relationship of lncRNA, miRNA, and mRNA and underlined that they function as key mediators in the pathophysiological process of SCs in DPN (Wang et al., 2020). This ceRNA regulatory network has a particular clinical application value in DPN. Another recent array study demonstrated that four lncRNAs, namely XR_353891, XR_600244, XR_595664, and XR_598132, can regulate inflammation signaling pathways by competitively binding with miR-146a-5p in DPN rats using qRT-PCR (Feng et al., 2020). LncRNA HLA complex group 18 (HCG18) promotes the polarization of M1 macrophages and DPN progression by regulating miR-146a/TNF receptor-associated factor 6 (TRAF6) axis. Additionally, in DPN model, inflammatory factors, such as TNF-α, IL-1β, and IL-6, are upregulated (Ren et al., 2021).
Other Diabetes Complications
Diabetic foot is a serious complication of diabetes, mainly caused by DPN, peripheral vascular diseases, or infection. As known, lncRNAs usually act as sponges for microRNAs to exert their regulatory effects. However, some ceRNAs in diabetic feet require additional investigation. For instance, inhibiting miR-217 can upregulate hypoxia-inducible factor-1 (HIF-1α)/vascular endothelial growth factor (VEGF) pathway to promote angiogenesis in diabetic foot ulcer rats and effectively improve inflammatory response by decreasing inflammatory factors (IL-1β, TNF-α, and IL-6) (Lin et al., 2019). Furthermore, emodin has been demonstrated to protect diabetic foot through miR-9 upregulation and modulation of PI3K/AKT and NF-κB signaling pathways in neuron-like PC-12 cells (Fan et al., 2018). Consequently, it is imperative to further investigate the interaction and contrast relationship between lncRNA and miRNA to better cure diabetic foot.
The incidence of diabetic osteoarthritis (OA) increases, making it critical to identify an exact therapy. One research has verified that lncRNA plasmacytoma variant translocation 1 (PVT1) promoted cartilage degradation in diabetic OA mice by downregulating miR-146a and activating TGF-β/mothers against decapentaplegic homolog 4 (SMAD4) signaling pathway. It has been demonstrated that silencing PVT1 decreases the expression of proinflammatory mediators such as TNF-α, IL-1, IL-6, and TGF-β1, hence alleviating joint inflammation (Wang Y.-Z. et al., 2021). Interestingly, another experiment confirmed that PVT1 silencing could act as a sponge for miR-149 to combat metabolic imbalance to catabolism and inflammation after IL-1β exposure (Zhao et al., 2018). It provided a new direction for diabetic OA treatment.
Potential Drugs Targeting LncRNA-Regulated NLRP3 Inflammasome in Diabetes and Its Complications
Melatonin can be produced by the pineal gland and is also present in various plants. It possesses pharmacological activities, including antioxidant, anti-inflammatory, liver protection, heart protection, and neuroprotection properties. In recent years, the effect of treating cancer and diabetes has been demonstrated (Ashrafizadeh et al., 2021). The clinical use of melatonin is controversial (Garaulet et al., 2020), but there are now some basic studies on its mechanism for different diabetes complications. For instance, melatonin has been demonstrated to have a protective effect of alleviating cardiac fibrosis on DCM by inhibiting lncRNA MALAT1. miR-141-5p, which acts as a sponge of MALAT1, inhibits the expression of NLRP3 inflammasome and TGF-β1/Smads signaling (Table 3) (Che et al., 2020c). TGF-β1 can initiate cardiac fibrosis via regulating extracellular matrix proteins in cardiac fibroblasts through activating Smads-mediated signal pathways in diabetic mice (Chen et al., 2015). In another work, melatonin can inhibit OS and inflammation by enhancing the activity of long non-coding RNA MEG3/miR-204/Sirt1 axis in experimental DR rats (Tu et al., 2020). Sirt1 can deacetylate the target gene forkhead box o1 (Foxo1) and the subunit p65 of NF-κB, leading to downregulation of inflammatory factors (Tu et al., 2021). Therefore, melatonin may be implemented as a potential agent for treating diabetic neuropathy (Che et al., 2020a).
Metformin has become a ‘foundation therapy’ for treating diabetes due to its excellent efficacy and safety. As a first-line treatment option for diabetes, metformin effectively controls the amount of glycogen when used alone or in combination with other drugs, such as sulfonylurea, thiazolidinedione, DPP-4 inhibitor, SGLT2 inhibitor, and GLP-1 receptor agonist or insulin (Sanchez-Rangel and Inzucchi, 2017). Recently, metformin has been confirmed to regulate lncRNA-mediated NLRP3 inflammasome in diabetes complications. Diabetic periodontitis is caused by diabetes leading to excessive inflammatory response of periodontal microbiome, and it subsequently increases insulin resistance (Lalla and Papapanou, 2011). NIMA-related kinases 7 (NEK7) is an essential mediator of NLRP3 activation downstream of potassium efflux (He et al., 2016). One research has revealed that metformin suppresses NEK7 expression in diabetic periodontitis to improve NLPP3 inflammasome-mediated pyroptosis (Zhou et al., 2020). Therefore, with additional research into the mechanism of action, metformin has a good clinical treatment prospect in diabetes and its complications, which warrants much attention.
Atorvastatin (AT) has lipid-decreasing, anticoagulant, antioxidative and anti-inflammatory functions (Shi M.-M. et al., 2016). Clinically, AT is often universally applied to treat lipid abnormalities and related angiopathies. Nuclear factor erythroid-2-related factor 2 (NRF2) plays a tremendous role in regulating OS and is lowly expressed under HG environments (Uruno et al., 2015). For example, one study has indicated that NRF2 hyperactivation can induce nephrogenic diabetes insipidus in early renal tube development (Suzuki et al., 2017). LncRNA MALAT1 is thought to be intimately linked to pyroptosis in diabetes complications (Li et al., 2017a). Interestingly, MALAT1 can stabilize and activate NRF2 in human umbilical vein endothelial cells under H2O2 disposed (Zeng et al., 2018). miR-200c overexpression can promote OS in endothelial cells and interact with MALAT1 structurally (Li et al., 2016; Carlomosti et al., 2017). In addition, Zuo Y et al. demonstrated that AT suppresses caspase-1, GSDMD, and NLRP3 expressions by regulating MALAT1/miR-200c/NRF2 activation to prevent podocyte pyrolysis and OS induced by high glucose (Zuo et al., 2021). It opens a new door to AT-induced therapy for diabetes complications.
Sinapic acid, a small naturally occurring hydroxycinnamic acid, contains 3,5-dimethoxyl and 4-hydroxyl substitutions in the phenyl ring of cinnamic acid. Sinapic acid is well known to show antioxidant, anti-inflammatory, anticancer, antiglycemic, and neuroprotective activities (Chen, 2016). Numerous regulation mechanisms for sinapic acid in diabetes and its complications have been revealed (Zych et al., 2019; Alaofi, 2020; Altındağ et al., 2021). Diabetic atherosclerosis is caused by chronic inflammation, dyslipidemia, and vascular endothelial injury under high blood sugar levels and is also an important cause of death and disability in diabetic patients (Giacco and Brownlee, 2010). It was stated that sinapic acid could alleviate inflammatory responses via inhibiting NLRP3 inflammasome activation (Lee et al., 2021). Recently, a study revealed that low-dose sinapic acid inhibits lncRNA-MALAT1 to downregulate NLRP3 expression, thereby alleviating macrophage pyroptosis in diabetic atherosclerosis rats (Han et al., 2018). These outcomes have revealed that sinapic acid has potential therapeutic value for diabetes complications.
In short, NLRP3 inflammasome is required for the inflammatory signaling pathway. IL-1β, IL-18, caspase-1, caspase-11, and NF-κB are important inflammatory factors in the inflammatory signaling pathway, whose expression indicates the role of NLRP3 inflammasome (Al Mamun et al., 2021). Simultaneously, the function of NLRP3 inflammasome provides a vital theoretical basis for using lncRNA as a therapeutic target to treat diabetes complications.
Conclusions and Future Perspectives
This review discussed the role and potential regulatory mechanism of lncRNAs on NLRP3 inflammasome, presented recent progress on the functional role of lncRNA-linked NLRP3 inflammasome regulation for developing and progressing various diabetes complications, and illustrated potential medications that might be useful in preventing and treating diabetes and its complications. This opens up potential new avenues to treat diabetes and its complications by targeting lncRNA-linked NLRP3 inflammasome.
At present, with lncRNA as the target, research on the role of drugs to interfere with diabetic complications is in its infancy. LncRNA intervention has been demonstrated to affect initiation and activation of NLRP3 inflammasome, as well as the expression of its downstream genes, consequently inhibiting the occurrence and development of diabetic complications. However, the specific mechanisms still require in-depth studies.
As a key role in developing and progressing diabetes complications, NLRP3 inflammasome brings new research directions for preventing and treating diabetes complications in the future. The treatment of NLRP3 inflammasome inhibition by targeting lncRNA of specific inflammatory signaling pathways may become a novel strategy for delaying the progression of diabetic complications in the future. Given the complexity of diabetes pathogenesis and its complications, lncRNA regulation on NLRP3 inflammasome has been investigated.
Additional research is required to elucidate the role and mechanism of lncRNA-linked NLRP3-inflammasome regulation in diabetes complications and other inflammatory diseases.
Author Contributions
Conceptualization, JZ and PY; writing—original draft preparation, XL, QT, and PY; writing—review and editing, PY and JM; project administration, PY; funding acquisition, JZ and PY. All authors have read and agreed to the published version of the manuscript.
Funding
This research was supported by grants from the Key Science and Technology Research Project of Education Department of Jiangxi Province (GJJ200115).
Conflict of Interest
The authors declare that the research was conducted in the absence of any commercial or financial relationships that could be construed as a potential conflict of interest.
Publisher’s Note
All claims expressed in this article are solely those of the authors and do not necessarily represent those of their affiliated organizations, or those of the publisher, the editors and the reviewers. Any product that may be evaluated in this article, or claim that may be made by its manufacturer, is not guaranteed or endorsed by the publisher.
Acknowledgments
We would like to express our deepest gratitude to tutor Jing Zhang and Peng Yu, who helped us during the writing and modification of this thesis. Without their consistent and illuminating instruction, this thesis could not have reached its present form.
References
Al Mamun, A., Wu, Y., Monalisa, I., Jia, C., Zhou, K., Munir, F., et al. (2021). Role of Pyroptosis in Spinal Cord Injury and its Therapeutic Implications. J. Adv. Res. 28, 97–109. doi:10.1016/j.jare.2020.08.004
Alaofi, A. L. (2020). Sinapic Acid Ameliorates the Progression of Streptozotocin (STZ)-Induced Diabetic Nephropathy in Rats via NRF2/HO-1 Mediated Pathways. Front. Pharmacol. 11, 1119. doi:10.3389/fphar.2020.01119
Altındağ, F., Rağbetli, M. Ç., Özdek, U., Koyun, N., Ismael Alhalboosi, J. K., and Elasan, S. (2021). Combined Treatment of Sinapic Acid and Ellagic Acid Attenuates Hyperglycemia in Streptozotocin-Induced Diabetic Rats. Food Chem. Toxicol. 156, 112443. doi:10.1016/j.fct.2021.112443
Ashrafizadeh, M., Najafi, M., Kavyiani, N., Mohammadinejad, R., Farkhondeh, T., and Samarghandian, S. (2021). Anti-Inflammatory Activity of Melatonin: a Focus on the Role of NLRP3 Inflammasome. Inflammation 44 (4), 1207–1222. doi:10.1007/s10753-021-01428-9
Bauernfeind, F. G., Horvath, G., Stutz, A., Alnemri, E. S., MacDonald, K., Speert, D., et al. (2009). Cutting Edge: NF-Κb Activating Pattern Recognition and Cytokine Receptors License NLRP3 Inflammasome Activation by Regulating NLRP3 Expression. J. Immunol. 183 (2), 787–791. doi:10.4049/jimmunol.0901363
Bertoni, A. G., Burke, G. L., Owusu, J. A., Carnethon, M. R., Vaidya, D., Barr, R. G., et al. (2010). Inflammation and the Incidence of Type 2 Diabetes: the Multi-Ethnic Study of Atherosclerosis (MESA). Diabetes care 33 (4), 804–810. doi:10.2337/dc09-1679
Boon, R. A., Jaé, N., Holdt, L., and Dimmeler, S. (2016). Long Noncoding RNAs. J. Am. Coll. Cardiol. 67 (10), 1214–1226. doi:10.1016/j.jacc.2015.12.051
Cai, F., Jiang, H., Li, Y., Li, Q., and Yang, C. (2021). Upregulation of Long Non-coding RNA SNHG16 Promotes Diabetes-Related RMEC Dysfunction via Activating NF-Κb and PI3K/AKT Pathways. Mol. Ther. - Nucleic Acids 24, 512–527. doi:10.1016/j.omtn.2021.01.035
Cao, H., Han, X., Jia, Y., and Zhang, B. (2021). Inhibition of Long Non-coding RNA HOXA11-AS against Neuroinflammation in Parkinson's Disease Model via Targeting miR-124-3p Mediated FSTL1/NF-Κb axis. Aging 13 (8), 11455–11469. doi:10.18632/aging.202837
Carlomosti, F., D'Agostino, M., Beji, S., Torcinaro, A., Rizzi, R., Zaccagnini, G., et al. (2017). Oxidative Stress-Induced miR-200c Disrupts the Regulatory Loop Among SIRT1, FOXO1, and eNOS. Antioxid. Redox Signaling 27 (6), 328–344. doi:10.1089/ars.2016.6643
Che, H., Li, H., Li, Y., Wang, Y. Q., Yang, Z. Y., Wang, R. L., et al. (2020a). Melatonin Exerts Neuroprotective Effects by Inhibiting Neuronal Pyroptosis and Autophagy in STZ‐induced Diabetic Mice. FASEB J. 34 (10), 14042–14054. doi:10.1096/fj.202001328R
Che, H., Wang, Y., Li, H., Li, Y., Sahil, A., Lv, J., et al. (2020b). Melatonin Alleviates Cardiac Fibrosis via Inhibiting lncRNA MALAT1/miR‐141‐mediated NLRP3 Inflammasome and TGF‐β1/Smads Signaling in Diabetic Cardiomyopathy. FASEB j. 34 (4), 5282–5298. doi:10.1096/fj.201902692R
Chen, C. (2016). Sinapic Acid and its Derivatives as Medicine in Oxidative Stress-Induced Diseases and Aging. Oxidative Med. Cell. longevity 2016, 1–10. doi:10.1155/2016/3571614
Chen, G., and Goeddel, D. V. (2002). TNF-R1 Signaling: a Beautiful Pathway. Science 296 (5573), 1634–1635. doi:10.1126/science.1071924
Chen, X., Liu, G., Zhang, W., Zhang, J., Yan, Y., Dong, W., et al. (2015). Inhibition of MEF2A Prevents Hyperglycemia-Induced Extracellular Matrix Accumulation by Blocking Akt and TGF-β1/Smad Activation in Cardiac Fibroblasts. Int. J. Biochem. Cel Biol. 69, 52–61. doi:10.1016/j.biocel.2015.10.012
Cho, N. H., Shaw, J. E., Karuranga, S., Huang, Y., da Rocha Fernandes, J. D., Ohlrogge, A. W., et al. (2018). IDF Diabetes Atlas: Global Estimates of Diabetes Prevalence for 2017 and Projections for 2045. Diabetes Res. Clin. Pract. 138, 271–281. doi:10.1016/j.diabres.2018.02.023
D'Elia, J. A., Weinrauch, L. A., Paine, D. F., Domey, P. E., Smith-Ossman, S. L., Williams, M. E., et al. (1991). Increased Infection Rate in Diabetic Dialysis Patients Exposed to Cocaine. Am. J. Kidney Dis. 18 (3), 349–352. doi:10.1016/s0272-6386(12)80094-1
Du, P., Wang, J., Han, Y., and Feng, J. (2020). Blocking the LncRNA MALAT1/miR-224-5p/NLRP3 Axis Inhibits the Hippocampal Inflammatory Response in T2DM with OSA. Front. Cel. Neurosci. 14, 97. doi:10.3389/fncel.2020.00097
Eckel, R. H., Bornfeldt, K. E., and Goldberg, I. J. (2021). Cardiovascular Disease in Diabetes, beyond Glucose. Cel Metab. 33 (8), 1519–1545. doi:10.1016/j.cmet.2021.07.001
Fan, L., Zhang, H., Li, X., Yang, G., Ru, J., and Liu, T. (2018). Emodin Protects Hyperglycemia-Induced Injury in PC-12 Cells by Up-Regulation of miR-9. Mol. Cell. Endocrinol. 474, 194–200. doi:10.1016/j.mce.2018.03.009
Fang, M., Ishigami, J., Echouffo-Tcheugui, J. B., Lutsey, P. L., Pankow, J. S., and Selvin, E. (2021). Diabetes and the Risk of Hospitalisation for Infection: the Atherosclerosis Risk in Communities (ARIC) Study. Diabetologia 64, 2458–2465. doi:10.1007/s00125-021-05522-3
Farooqi, A. A., Attar, R., Tanriover, G., Sabitaliyevich, U. Y., Zhailganov, A., and Rabandiyarov, M. (2020). Regulation of NLRP3 by Non-coding RNAs in Different Cancers: Interplay between Non-coding RNAs and NLRP3 in Carcinogenesis and Metastasis. Cel Mol Biol (Noisy-le-grand) 66 (8), 47–51.
Feng, Y., Ge, Y., Wu, M., Xie, Y., Wang, M., Chen, Y., et al. (2020). Long Non-coding RNAs Regulate Inflammation in Diabetic Peripheral Neuropathy by Acting as ceRNAs Targeting miR-146a-5p. Dmso Vol. 13, 413–422. doi:10.2147/DMSO.S242789
Feng, Y., Xu, W., Zhang, W., Wang, W., Liu, T., and Zhou, X. (2019). LncRNA DCRF Regulates Cardiomyocyte Autophagy by Targeting miR-551b-5p in Diabetic Cardiomyopathy. Theranostics 9 (15), 4558–4566. doi:10.7150/thno.31052
Forbes, J. M., and Cooper, M. E. (2013). Mechanisms of Diabetic Complications. Physiol. Rev. 93 (1), 137–188. doi:10.1152/physrev.00045.2011
Garaulet, M., Qian, J., Florez, J. C., Arendt, J., Saxena, R., and Scheer, F. A. J. L. (2020). Melatonin Effects on Glucose Metabolism: Time to Unlock the Controversy. Trends Endocrinol. Metab. 31 (3), 192–204. doi:10.1016/j.tem.2019.11.011
Giacco, F., and Brownlee, M. (2010). Oxidative Stress and Diabetic Complications. Circ. Res. 107 (9), 1058–1070. doi:10.1161/CIRCRESAHA.110.223545
Gil, N., and Ulitsky, I. (2020). Regulation of Gene Expression by Cis-Acting Long Non-coding RNAs. Nat. Rev. Genet. 21 (2), 102–117. doi:10.1038/s41576-019-0184-5
Guo, H., Callaway, J. B., and Ting, J. P.-Y. (2015). Inflammasomes: Mechanism of Action, Role in Disease, and Therapeutics. Nat. Med. 21 (7), 677–687. doi:10.1038/nm.3893
Guo, J., Liu, Z., and Gong, R. (2019). Long Noncoding RNA: an Emerging Player in Diabetes and Diabetic Kidney Disease. Clin. Sci. (Lond) 133 (12), 1321–1339. doi:10.1042/cs20190372
Han, Y., Qiu, H., Pei, X., Fan, Y., Tian, H., and Geng, J. (2018). Low-dose Sinapic Acid Abates the Pyroptosis of Macrophages by Downregulation of lncRNA-MALAT1 in Rats with Diabetic Atherosclerosis. J. Cardiovasc. Pharmacol. 71 (2), 104–112. doi:10.1097/fjc.0000000000000550
Haneklaus, M., O’Neill, L. A., and Coll, R. C. (2013). Modulatory Mechanisms Controlling the NLRP3 Inflammasome in Inflammation: Recent Developments. Curr. Opin. Immunol. 25 (1), 40–45. doi:10.1016/j.coi.2012.12.004
Haque, M. E., Akther, M., Jakaria, M., Kim, I. S., Azam, S., and Choi, D. K. (2020). Targeting the Microglial NLRP3 Inflammasome and its Role in Parkinson's Disease. Mov Disord. 35 (1), 20–33. doi:10.1002/mds.27874
He, Y., Zeng, M. Y., Yang, D., Motro, B., and Núñez, G. (2016). NEK7 Is an Essential Mediator of NLRP3 Activation Downstream of Potassium Efflux. Nature 530 (7590), 354–357. doi:10.1038/nature16959
Hon, C.-C., Ramilowski, J. A., Harshbarger, J., Bertin, N., Rackham, O. J. L., Gough, J., et al. (2017). An Atlas of Human Long Non-coding RNAs with Accurate 5′ Ends. Nature 543 (7644), 199–204. doi:10.1038/nature21374
Hu, J., Wu, H., Wang, D., Yang, Z., and Dong, J. (2019). LncRNA ANRIL Promotes NLRP3 Inflammasome Activation in Uric Acid Nephropathy through miR-122-5p/BRCC3 axis. Biochimie 157, 102–110. doi:10.1016/j.biochi.2018.10.011
Jakubik, D., Fitas, A., Eyileten, C., Jarosz-Popek, J., Nowak, A., Czajka, P., et al. (2021). MicroRNAs and Long Non-coding RNAs in the Pathophysiological Processes of Diabetic Cardiomyopathy: Emerging Biomarkers and Potential Therapeutics. Cardiovasc. Diabetol. 20 (1), 55. doi:10.1186/s12933-021-01245-2
Kato, M., Wang, M., Chen, Z., Bhatt, K., Oh, H. J., Lanting, L., et al. (2016). An Endoplasmic Reticulum Stress-Regulated lncRNA Hosting a microRNA Megacluster Induces Early Features of Diabetic Nephropathy. Nat. Commun. 7, 12864. doi:10.1038/ncomms12864
Knapp, S. (2013). Diabetes and Infection: Is There a Link? - A Mini-Review. Gerontology 59 (2), 99–104. doi:10.1159/000345107
Kopp, F., and Mendell, J. T. (2018). Functional Classification and Experimental Dissection of Long Noncoding RNAs. Cell 172 (3), 393–407. doi:10.1016/j.cell.2018.01.011
Kozakova, M., Morizzo, C., Goncalves, I., Natali, A., Nilsson, J., and Palombo, C. (2019). Cardiovascular Organ Damage in Type 2 Diabetes Mellitus: the Role of Lipids and Inflammation. Cardiovasc. Diabetol. 18 (1), 61. doi:10.1186/s12933-019-0865-6
Lalla, E., and Papapanou, P. N. (2011). Diabetes Mellitus and Periodontitis: a Tale of Two Common Interrelated Diseases. Nat. Rev. Endocrinol. 7 (12), 738–748. doi:10.1038/nrendo.2011.106
Lazar, M. A. (2005). How Obesity Causes Diabetes: Not a Tall Tale. Science 307 (5708), 373–375. doi:10.1126/science.1104342
Lee, E. H., Shin, J. H., Kim, S. S., and Seo, S. R. (2021). Sinapic Acid Controls Inflammation by Suppressing NLRP3 Inflammasome Activation. Cells 10 (9), 2327. doi:10.3390/cells10092327
Leung, A., and Natarajan, R. (2018). Long Noncoding RNAs in Diabetes and Diabetic Complications. Antioxid. Redox Signaling 29 (11), 1064–1073. doi:10.1089/ars.2017.7315
Li, Q., Zhang, C., Chen, R., Xiong, H., Qiu, F., Liu, S., et al. (2016). Disrupting MALAT1/miR-200c Sponge Decreases Invasion and Migration in Endometrioid Endometrial Carcinoma. Cancer Lett. 383 (1), 28–40. doi:10.1016/j.canlet.2016.09.019
Li, X., Zeng, L., Cao, C., Lu, C., Lian, W., Han, J., et al. (2017a). Long Noncoding RNA MALAT1 Regulates Renal Tubular Epithelial Pyroptosis by Modulated miR-23c Targeting of ELAVL1 in Diabetic Nephropathy. Exp. Cel. Res. 350 (2), 327–335. doi:10.1016/j.yexcr.2016.12.006
Li, Y., Huang, H., Liu, B., Zhang, Y., Pan, X., Yu, X.-Y., et al. (2021). Inflammasomes as Therapeutic Targets in Human Diseases. Sig Transduct Target. Ther. 6 (1), 247. doi:10.1038/s41392-021-00650-z
Lin, C.-J., Lan, Y.-M., Ou, M.-Q., Ji, L.-Q., and Lin, S.-D. (2019). Expression of miR-217 and HIF-1α/VEGF Pathway in Patients with Diabetic Foot Ulcer and its Effect on Angiogenesis of Diabetic Foot Ulcer Rats. J. Endocrinol. Invest. 42 (11), 1307–1317. doi:10.1007/s40618-019-01053-2
Liu, C., Zhuo, H., Ye, M. Y., Huang, G. X., Fan, M., and Huang, X. Z. (2020). LncRNA MALAT1 Promoted High Glucose‐induced Pyroptosis of Renal Tubular Epithelial Cell by Sponging miR ‐30c Targeting for NLRP3. Kaohsiung J. Med. Sci. 36 (9), 682–691. doi:10.1002/kjm2.12226
Liu, J.-Y., Yao, J., Li, X.-M., Song, Y.-C., Wang, X.-Q., Li, Y.-J., et al. (2014). Pathogenic Role of lncRNA-MALAT1 in Endothelial Cell Dysfunction in Diabetes Mellitus. Cell Death Dis 5, e1506. doi:10.1038/cddis.2014.466
Liu, X., Song, W., Zhang, X., Long, F., Yin, J., He, X., et al. (2021). Downregulating LncRNA XIST Attenuated Contrast-Induced Nephropathy Injury via Regulating miR-133a-3p/NLRP3 axis. J. Thromb. Thrombolysis 52, 440–453. doi:10.1007/s11239-020-02369-0
Luo, R., Li, L., Hu, Y. X., and Xiao, F. (2021). LncRNA H19 Inhibits High Glucose‐induced Inflammatory Responses of Human Retinal Epithelial Cells by Targeting miR ‐19b to Increase SIRT1 Expression. Kaohsiung J. Med. Sci. 37 (2), 101–110. doi:10.1002/kjm2.12302
Matoba, K., Takeda, Y., Nagai, Y., Kawanami, D., Utsunomiya, K., and Nishimura, R. (2019). Unraveling the Role of Inflammation in the Pathogenesis of Diabetic Kidney Disease. Int. J. Mol. Sci. 20 (14), 3393. doi:10.3390/ijms20143393
Nair, L., Chung, H., and Basu, U. (2020). Regulation of Long Non-coding RNAs and Genome Dynamics by the RNA Surveillance Machinery. Nat. Rev. Mol. Cel Biol 21 (3), 123–136. doi:10.1038/s41580-019-0209-0
Nolan, C. J., Damm, P., and Prentki, M. (2011). Type 2 Diabetes across Generations: from Pathophysiology to Prevention and Management. The Lancet 378 (9786), 169–181. doi:10.1016/s0140-6736(11)60614-4
Okin, D., and Medzhitov, R. (2016). The Effect of Sustained Inflammation on Hepatic Mevalonate Pathway Results in Hyperglycemia. Cell 165 (2), 343–356. doi:10.1016/j.cell.2016.02.023
Olefsky, J. M. (2009). IKKɛ: A Bridge between Obesity and Inflammation. Cell 138 (5), 834–836. doi:10.1016/j.cell.2009.08.018
Ponting, C. P., Oliver, P. L., and Reik, W. (2009). Evolution and Functions of Long Noncoding RNAs. Cell 136 (4), 629–641. doi:10.1016/j.cell.2009.02.006
Rathinam, V. A. K., and Fitzgerald, K. A. (2016). Inflammasome Complexes: Emerging Mechanisms and Effector Functions. Cell 165 (4), 792–800. doi:10.1016/j.cell.2016.03.046
Reddy, M. A., Zhang, E., and Natarajan, R. (2015). Epigenetic Mechanisms in Diabetic Complications and Metabolic Memory. Diabetologia 58 (3), 443–455. doi:10.1007/s00125-014-3462-y
Ren, W., Xi, G., Li, X., Zhao, L., Yang, K., Fan, X., et al. (2021). Long Non-coding RNA HCG18 Promotes M1 Macrophage Polarization through Regulating the miR-146a/TRAF6 axis, Facilitating the Progression of Diabetic Peripheral Neuropathy. Mol. Cel Biochem 476 (1), 471–482. doi:10.1007/s11010-020-03923-3
Samoilă, I., Dinescu, S., and Costache, M. (2020). Interplay between Cellular and Molecular Mechanisms Underlying Inflammatory Bowel Diseases Development-A Focus on Ulcerative Colitis. Cells 9 (7), 1647. doi:10.3390/cells9071647
Samra, Y. A., Said, H. S., Elsherbiny, N. M., Liou, G. I., El-Shishtawy, M. M., and Eissa, L. A. (2016). Cepharanthine and Piperine Ameliorate Diabetic Nephropathy in Rats: Role of NF-Κb and NLRP3 Inflammasome. Life Sci. 157, 187–199. doi:10.1016/j.lfs.2016.06.002
Sanchez-Rangel, E., and Inzucchi, S. E. (2017). Metformin: Clinical Use in Type 2 Diabetes. Diabetologia 60 (9), 1586–1593. doi:10.1007/s00125-017-4336-x
Schmid-Burgk, J. L., Chauhan, D., Schmidt, T., Ebert, T. S., Reinhardt, J., Endl, E., et al. (2016). A Genome-wide CRISPR (Clustered Regularly Interspaced Short Palindromic Repeats) Screen Identifies NEK7 as an Essential Component of NLRP3 Inflammasome Activation. J. Biol. Chem. 291 (1), 103–109. doi:10.1074/jbc.C115.700492
Schroder, K., Zhou, R., and Tschopp, J. (2010). The NLRP3 Inflammasome: a Sensor for Metabolic Danger. Science 327 (5963), 296–300. doi:10.1126/science.1184003
Sharma, B. R., and Kanneganti, T.-D. (2021). NLRP3 Inflammasome in Cancer and Metabolic Diseases. Nat. Immunol. 22 (5), 550–559. doi:10.1038/s41590-021-00886-5
Shi, H., Wang, Y., Li, X., Zhan, X., Tang, M., Fina, M., et al. (2016a). NLRP3 Activation and Mitosis Are Mutually Exclusive Events Coordinated by NEK7, a New Inflammasome Component. Nat. Immunol. 17 (3), 250–258. doi:10.1038/ni.3333
Shi, M.-M., Kong, Y., Song, Y., Sun, Y.-Q., Wang, Y., Zhang, X.-H., et al. (2016b). Atorvastatin Enhances Endothelial Cell Function in Posttransplant Poor Graft Function. Blood 128 (25), 2988–2999. doi:10.1182/blood-2016-03-702803
Shikata, K., Ninomiya, T., and Kiyohara, Y. (2013). Diabetes Mellitus and Cancer Risk: Review of the Epidemiological Evidence. Cancer Sci. 104 (1), 9–14. doi:10.1111/cas.12043
Singh, S. K., Apata, T., Singh, S., McFadden, M., and Singh, R. (2021). Clinical Implication of Metformin in Relation to Diabetes Mellitus and Ovarian Cancer. Biomedicines 9 (8), 1020. doi:10.3390/biomedicines9081020
Suzuki, T., Seki, S., Hiramoto, K., Naganuma, E., Kobayashi, E. H., Yamaoka, A., et al. (2017). Hyperactivation of Nrf2 in Early Tubular Development Induces Nephrogenic Diabetes Insipidus. Nat. Commun. 8, 14577. doi:10.1038/ncomms14577
Swanson, K. V., Deng, M., and Ting, J. P.-Y. (2019). The NLRP3 Inflammasome: Molecular Activation and Regulation to Therapeutics. Nat. Rev. Immunol. 19 (8), 477–489. doi:10.1038/s41577-019-0165-0
Tang, S. C. W., and Yiu, W. H. (2020). Innate Immunity in Diabetic Kidney Disease. Nat. Rev. Nephrol. 16 (4), 206–222. doi:10.1038/s41581-019-0234-4
Tang, Y., Cao, G., Zhao, G., Wang, C., and Qin, Q. (2020). LncRNA Differentiation Antagonizing Non-protein Coding RNA Promotes Proliferation and Invasion through Regulating miR-135a/NLRP37 axis in Pancreatic Cancer. Invest. New Drugs 38 (3), 714–721. doi:10.1007/s10637-019-00798-0
Thomson, D. W., and Dinger, M. E. (2016). Endogenous microRNA Sponges: Evidence and Controversy. Nat. Rev. Genet. 17 (5), 272–283. doi:10.1038/nrg.2016.20
Tu, Y., Song, E., Wang, Z., Ji, N., Zhu, L., Wang, K., et al. (2021). Melatonin Attenuates Oxidative Stress and Inflammation of Müller Cells in Diabetic Retinopathy via Activating the Sirt1 Pathway. Biomed. Pharmacother. 137, 111274. doi:10.1016/j.biopha.2021.111274
Tu, Y., Zhu, M., Wang, Z., Wang, K., Chen, L., Liu, W., et al. (2020). Melatonin Inhibits Müller Cell Activation and Pro‐inflammatory Cytokine Production via Upregulating the MEG3/miR‐204/Sirt1 axis in Experimental Diabetic Retinopathy. J. Cel Physiol 235 (11), 8724–8735. doi:10.1002/jcp.29716
Uruno, A., Yagishita, Y., and Yamamoto, M. (2015). The Keap1-Nrf2 System and Diabetes Mellitus. Arch. Biochem. Biophys. 566, 76–84. doi:10.1016/j.abb.2014.12.012
Vujosevic, S., Aldington, S. J., Silva, P., Hernández, C., Scanlon, P., Peto, T., et al. (2020). Screening for Diabetic Retinopathy: New Perspectives and Challenges. Lancet Diabetes Endocrinol. 8 (4), 337–347. doi:10.1016/S2213-8587(19)30411-5
Wada, J., and Makino, H. (2016). Innate Immunity in Diabetes and Diabetic Nephropathy. Nat. Rev. Nephrol. 12 (1), 13–26. doi:10.1038/nrneph.2015.175
Wang, C., Xu, X., Chen, J., Kang, Y., Guo, J., Duscher, D., et al. (2020). The Construction and Analysis of lncRNA-miRNA-mRNA Competing Endogenous RNA Network of Schwann Cells in Diabetic Peripheral Neuropathy. Front. Bioeng. Biotechnol. 8, 490. doi:10.3389/fbioe.2020.00490
Wang, J., and Zhao, S.-M. (2021). LncRNA-antisense Non-coding RNA in the INK4 Locus Promotes Pyroptosis via miR-497/thioredoxin-Interacting Protein axis in Diabetic Nephropathy. Life Sci. 264, 118728. doi:10.1016/j.lfs.2020.118728
Wang, X., Liu, Y., Rong, J., and Wang, K. (2021a). LncRNA HCP5 Knockdown Inhibits High Glucose-Induced Excessive Proliferation, Fibrosis and Inflammation of Human Glomerular Mesangial Cells by Regulating the miR-93-5p/HMGA2 axis. BMC Endocr. Disord. 21 (1), 134. doi:10.1186/s12902-021-00781-y
Wang, Y.-Z., Yao-Li, L., Liang, S.-K., Ding, L.-B., Feng-Li, L., Guan, J., et al. (2021b). LncPVT1 Promotes Cartilage Degradation in Diabetic OA Mice by Downregulating miR-146a and Activating TGF-Β/smad4 Signaling. J. Bone Miner Metab. 39 (4), 534–546. doi:10.1007/s00774-020-01199-7
Wolf, G. (2004). New Insights into the Pathophysiology of Diabetic Nephropathy: from Haemodynamics to Molecular Pathology. Eur. J. Clin. Invest. 34 (12), 785–796. doi:10.1111/j.1365-2362.2004.01429.x
Wong, T. Y., Cheung, C. M. G., Larsen, M., Sharma, S., and Simó, R. (2016). Diabetic Retinopathy. Nat. Rev. Dis. Primers 2, 16012. doi:10.1038/nrdp.2016.12
Xie, C., Wu, W., Tang, A., Luo, N., and Tan, Y. (2019). lncRNA GAS5/miR-452-5p Reduces Oxidative Stress and Pyroptosis of High-Glucose-Stimulated Renal Tubular Cells. Dmso Vol. 12, 2609–2617. doi:10.2147/dmso.S228654
Xing, Y., Yao, X., Li, H., Xue, G., Guo, Q., Yang, G., et al. (2017). Cutting Edge: TRAF6 Mediates TLR/IL-1R Signaling-Induced Nontranscriptional Priming of the NLRP3 Inflammasome. J.Immunol. 199, 1561–1566. doi:10.4049/jimmunol.1700175
Xiong, W., Meng, X.-F., and Zhang, C. (2021). NLRP3 Inflammasome in Metabolic-Associated Kidney Diseases: An Update. Front. Immunol. 12, 714340. doi:10.3389/fimmu.2021.714340
Xu, Y., Fang, H., Xu, Q., Xu, C., Yang, L., and Huang, C. (2020). LncRNA GAS5 Inhibits NLRP3 Inflammasome Activation-Mediated Pyroptosis in Diabetic Cardiomyopathy by Targeting miR-34b-3p/AHR. Cell Cycle 19 (22), 3054–3065. doi:10.1080/15384101.2020.1831245
Yan, B., Yao, J., Liu, J.-Y., Li, X.-M., Wang, X.-Q., Li, Y.-J., et al. (2015). lncRNA-MIAT Regulates Microvascular Dysfunction by Functioning as a Competing Endogenous RNA. Circ. Res. 116 (7), 1143–1156. doi:10.1161/CIRCRESAHA.116.305510
Yang, F., Qin, Y., Lv, J., Wang, Y., Che, H., Chen, X., et al. (2018). Silencing Long Non-coding RNA Kcnq1ot1 Alleviates Pyroptosis and Fibrosis in Diabetic Cardiomyopathy. Cel Death Dis 9 (10), 1000. doi:10.1038/s41419-018-1029-4
Yi, H., Peng, R., Zhang, L.-y., Sun, Y., Peng, H.-m., Liu, H.-d., et al. (2017). LincRNA-Gm4419 Knockdown Ameliorates NF-Κb/nlrp3 Inflammasome-Mediated Inflammation in Diabetic Nephropathy. Cel Death Dis 8 (2), e2583. doi:10.1038/cddis.2016.451
Yilmaz, S., Canpolat, U., Aydogdu, S., and Abboud, H. E. (2015). Diabetic Cardiomyopathy; Summary of 41 Years. Korean Circ. J. 45 (4), 266–272. doi:10.4070/kcj.2015.45.4.266
Zeng, R., Zhang, R., Song, X., Ni, L., Lai, Z., Liu, C., et al. (2018). The Long Non-coding RNA MALAT1 Activates Nrf2 Signaling to Protect Human Umbilical Vein Endothelial Cells from Hydrogen Peroxide. Biochem. biophysical Res. Commun. 495 (4), 2532–2538. doi:10.1016/j.bbrc.2017.12.105
Zhan, J.-F., Huang, H.-W., Huang, C., Hu, L.-L., and Xu, W.-W. (2020). Long Non-coding RNA NEAT1 Regulates Pyroptosis in Diabetic Nephropathy via Mediating the miR-34c/NLRP3 Axis. Kidney Blood Press. Res. 45 (4), 589–602. doi:10.1159/000508372
Zhang, C., Gong, Y., Li, N., Liu, X., Zhang, Y., Ye, F., et al. (2021). Long Noncoding RNA Kcnq1ot1 Promotes sC5b-9-Induced Podocyte Pyroptosis by Inhibiting miR-486a-3p and Upregulating NLRP3. Am. J. Physiology-Cell Physiol. 320 (3), C355–c364. doi:10.1152/ajpcell.00403.2020
Zhao, J., Liu, B., and Li, C. (2020). Knockdown of Long Noncoding RNA GAS5 Protects Human Cardiomyocyte-like AC16 Cells against High Glucose-Induced Inflammation by Inhibiting miR-21-5p-Mediated TLR4/NF-Κb Signaling. Naunyn-schmiedeberg's Arch. Pharmacol. 393 (8), 1541–1547. doi:10.1007/s00210-019-01795-z
Zhao, Y., Zhao, J., Guo, X., She, J., and Liu, Y. (2018). Long Non-coding RNA PVT1, a Molecular Sponge for miR-149, Contributes Aberrant Metabolic Dysfunction and Inflammation in IL-1β-simulated Osteoarthritic Chondrocytes. Biosci. Rep. 38 (5). doi:10.1042/BSR20180576
Zhou, J., and Zhou, S. (2014). Inflammation: Therapeutic Targets for Diabetic Neuropathy. Mol. Neurobiol. 49 (1), 536–546. doi:10.1007/s12035-013-8537-0
Zhou, X., Wang, Q., Nie, L., Zhang, P., Zhao, P., Yuan, Q., et al. (2020). Metformin Ameliorates the NLPP3 Inflammasome Mediated Pyroptosis by Inhibiting the Expression of NEK7 in Diabetic Periodontitis. Arch. Oral Biol. 116, 104763. doi:10.1016/j.archoralbio.2020.104763
Zhu, B., Cheng, X., Jiang, Y., Cheng, M., Chen, L., Bao, J., et al. (2020). Silencing of KCNQ1OT1 Decreases Oxidative Stress and Pyroptosis of Renal Tubular Epithelial Cells. Dmso Vol. 13, 365–375. doi:10.2147/dmso.S225791
Zou, D.-M., Zhou, S.-M., Li, L.-H., Zhou, J.-L., Tang, Z.-M., and Wang, S.-H. (2020). Knockdown of Long Noncoding RNAs of Maternally Expressed 3 Alleviates Hyperoxia-Induced Lung Injury via Inhibiting Thioredoxin-Interacting Protein-Mediated Pyroptosis by Binding to miR-18a. Am. J. Pathol. 190 (5), 994–1005. doi:10.1016/j.ajpath.2019.12.013
Zou, W., Luo, S., Zhang, Z., Cheng, L., Huang, X., Ding, N., et al. (2021). ASK1/p38mediated NLRP3 Inflammasome Signaling Pathway Contributes to Aberrant Retinal Angiogenesis in Diabetic Retinopathy. Int. J. Mol. Med. 47 (2), 732–740. doi:10.3892/ijmm.2020.4833
Zuo, Y., Chen, L., He, X., Ye, Z., Li, L., Liu, Z., et al. (2021). Atorvastatin Regulates MALAT1/miR-200c/NRF2 Activity to Protect against Podocyte Pyroptosis Induced by High Glucose. Dmso Vol. 14, 1631–1645. doi:10.2147/dmso.S298950
Keywords: NLRP3, inflammasomes, lncRNA, diabetes complications, antidiabetics
Citation: Lu X, Tan Q, Ma J, Zhang J and Yu P (2022) Emerging Role of LncRNA Regulation for NLRP3 Inflammasome in Diabetes Complications. Front. Cell Dev. Biol. 9:792401. doi: 10.3389/fcell.2021.792401
Received: 11 October 2021; Accepted: 01 December 2021;
Published: 12 January 2022.
Edited by:
Cheng Yang, Fudan University, ChinaReviewed by:
Catriona Kelly, University of Ulster, United KingdomCaroline Volpe, Institute of Education and Research Santa Casa BH, Brazil
Copyright © 2022 Lu, Tan, Ma, Zhang and Yu. This is an open-access article distributed under the terms of the Creative Commons Attribution License (CC BY). The use, distribution or reproduction in other forums is permitted, provided the original author(s) and the copyright owner(s) are credited and that the original publication in this journal is cited, in accordance with accepted academic practice. No use, distribution or reproduction is permitted which does not comply with these terms.
*Correspondence: Jing Zhang, emhhbmdqaW5nNjY2ZG9jQDE2My5jb20=; Peng Yu, eXU4MjIwMTgyQDE2My5jb20=