- Multifactorial Disease and Complex Phenotype Research Area, Bambino Gesù Children’s Hospital, IRCCS, Rome, Italy
The bone microenvironment homeostasis is guaranteed by the balanced and fine regulated bone matrix remodeling process. This equilibrium can be disrupted by cancer cells developed in the bone (primary bone cancers) or deriving from other tissues (bone metastatic lesions), through a mechanism by which they interfere with bone cells activities and alter the microenvironment both biochemically and mechanically. Among the factors secreted by cancer cells and by cancer-conditioned bone cells, extracellular vesicles (EVs) are described to exert pivotal roles in the establishment and the progression of bone cancers, by conveying tumorigenic signals targeting and transforming normal cells. Doing this, EVs are also responsible in modulating the production of proteins involved in regulating matrix stiffness and/or mechanotransduction process, thereby altering the bone mechanoenvironment. In turn, bone and cancer cells respond to deregulated matrix stiffness by modifying EV production and content, fueling the vicious cycle established in tumors. Here, we summarized the relationship between EVs and the mechanoenvironment during tumoral progression, with the final aim to provide some innovative perspectives in counteracting bone cancers.
Introduction
Bone primary tumors are a heterogeneous group of rare neoplasms of the skeleton, accounting for approximately 0.2% of all tumors (Franchi, 2012). Their etiology is almost unknown, and an appropriate prognostication of primary bone tumors is complicated by the morphological overlap with other bone lesions of mesenchymal and non-mesenchymal origin. Bone tumors are classified as: chondrogenic, osteogenic and fibrogenic tumors, vascular tumors of bone, osteoclastic giant cell-rich tumors, notochordal tumors, other mesenchymal tumors of bone, hematopoietic neoplasms of bone and undifferentiated small round cell sarcomas of bone (Choi and Ro, 2021). Besides primary tumors, bone metastatic lesions are actually much more common, especially in adults, most of which resulting from breast and prostate primary cancers (Cecchini et al., 2005). Bone metastases are classified as osteolytic (characterized by destruction of bone matrix), osteoblastic (characterized by deposition of aberrant new bone) or mixed in dependance on how cancer cells interfere with physiological bone remodeling (Macedo et al., 2017). The main features of the metaphyseal bone, where metastases usually arise, are the rich vasculature and the constant remodeling of the bone matrix by which a plethora of soluble factors (among which growth factors, cytokines and extracellular vesicles) are released, thus acting as chemoattractant signal for cancer cells from distant sites (Bussard et al., 2008). Bone remodeling is a physiological process deriving from the continuous turnover of the bone matrix guaranteed by the finely regulated activity of the bone cells: osteoblasts (bone forming cells) (Lin et al., 2020) and osteoclasts (bone resorbing cells) (Peruzzi and Teti, 2012) that are responsible in maintaining the structural balance of bone matrix content, and the osteocytes that participate in bone remodeling in response to environmental and mechanical stimuli (Hu and Qin, 2020; Qin et al., 2020). In the bone microenvironment, the osteocytes can perceive and respond coordinately to environmental cues, such as hormones, physical stress, and mechanical loading and unloading. Doing this, osteocytes coordinate bone homeostasis by releasing factors that regulate bone formation or resorption with respect to demands (Bonewald, 2011). Several pathological factors can deregulate bone homeostasis, thereby inducing bone structural defects and macro- and micro-environment alteration, ultimately leading to cancer cells colonization within the tissue (Buenrostro et al., 2016). The mechanisms by which tumor cells metastasize to bone are poorly understood, although the theory of the premetastatic niches organized by primary tumors from near and distal regions of the body is now accepted by the scientific community (Doglioni et al., 2019; Wang et al., 2021). Once established in the bone microenvironment, cancer cells from both bone primary tumors or secondary metastases are responsible of bone remodeling deregulation, by altering bone cell activities (Taube et al., 1994; Guise et al., 2006). This process induces the release by bone cells and bone matrix of growth factors, cytokines and other soluble molecules that fuel tumor cells, leading to a “vicious cycle” in which bone integrity is destroyed and cancer cell growth and migration are promoted (Lamora et al., 2016; Esposito et al., 2018). Among the factors released by the vicious cycle, tumor- and bone-derived extracellular vesicles (EVs) are receiving growing interest because of their involvement in cancer progression and cancer bone tropism (Tamura et al., 2020) (Figure 1). EVs are non-replicable, lipid bilayer nanoscale vesicles virtually released by every cell type into the extracellular space (Yáñez-Mó et al., 2015; Théry et al., 2018). The traditional classification of EVs distinguishes three main sub-populations as microvesicles, exosomes, and apoptotic bodies (Yáñez-Mó et al., 2015; Zaborowski et al., 2015) on the basis of their biogenesis, release mechanisms, size, content and function, and their cargo consists of lipids, nuclear acids as DNAs, mRNAs, miRNAs and long non-coding RNAs, and proteins that reflect the composition of the cell of origin. Emerging evidence suggests that within a tumor microenvironment EVs are crucial factors in the communication between cancer and normal cells, influencing cancer onset, progression and metastatization (Tai et al., 2018; Dai et al., 2020). In turn, cancer cells can modulate EV production by altering the microenvironment in terms of mechanics (Eichholz et al., 2020; Nicastro et al., 2020) and acidity (Parolini et al., 2009; Logozzi et al., 2018). In the bone microenvironment, EVs are known to participate in tissue remodeling process by regulating the fine equilibrium between bone deposition and bone resorption, at both physiological and pathological levels, as well as in the tissue engineering-based bone regeneration (Eichholz et al., 2020; Yan et al., 2020). More in detail, EVs released by monocyte (Ekström et al., 2013) and osteoclasts (Wang et al., 2013; Li et al., 2016; Sun et al., 2016), as well as by mature osteoblasts (Cui et al., 2016) and osteoblast precursors (Furuta et al., 2016), are involved in regulating mesenchymal stromal cell differentiation towards osteogenic lineage. On the other side of the coin, osteoclastogenesis, the process by which bone-resorbing osteoclasts are formed starting from monocyte precursors (Zaidi et al., 2003), is mediated by bone cell-derived EVs (Cui et al., 2016; Huynh et al., 2016; Xie et al., 2017). In this context, transforming EVs derived from cancer cells are described as pivotal factors involved in bone tumor formation and progression (Han et al., 2019; Tamura et al., 2020). Indeed, EVs released by bone primary and/or secondary cancer cells are able to alter bone homeostasis favoring cancer establishment and progression (Li et al., 2019; Chicón-Bosch and Tirado, 2020; Raimondi et al., 2020). The deregulation of bone cell activities mediated by cancer-derived EVs depends on the transfer of transforming factors from cancer to normal bone cells, thus further fueling tumoral transformation and inducing aberrant bone cell activity. Most of the bone microenvironment alterations related to bone cancers are also reflected in the mechanics of the tissue, in terms of extracellular matrix stiffness and architecture. Sekita and colleagues have reported that the bone metastases induced by prostate cancer show impair mechanical functions that might be attributed to disruption of the anisotropic microstructure of bone in multiple phases (Sekita et al., 2017). Moreover, tumor-generated pressure acts to osteocytes and modifies the bone microenvironment promoting the growth of prostate cancer bone metastasis (Sottnik et al., 2015). In view of this, here we summarized the mutual relationship between extracellular vesicles and the mechanoenvironment in the context of bone tumors, both primary and metastatic, with the final aim to provide some novel points of view to counteract cancer by considering the roles exerted by EVs and the tissue biomechanics.
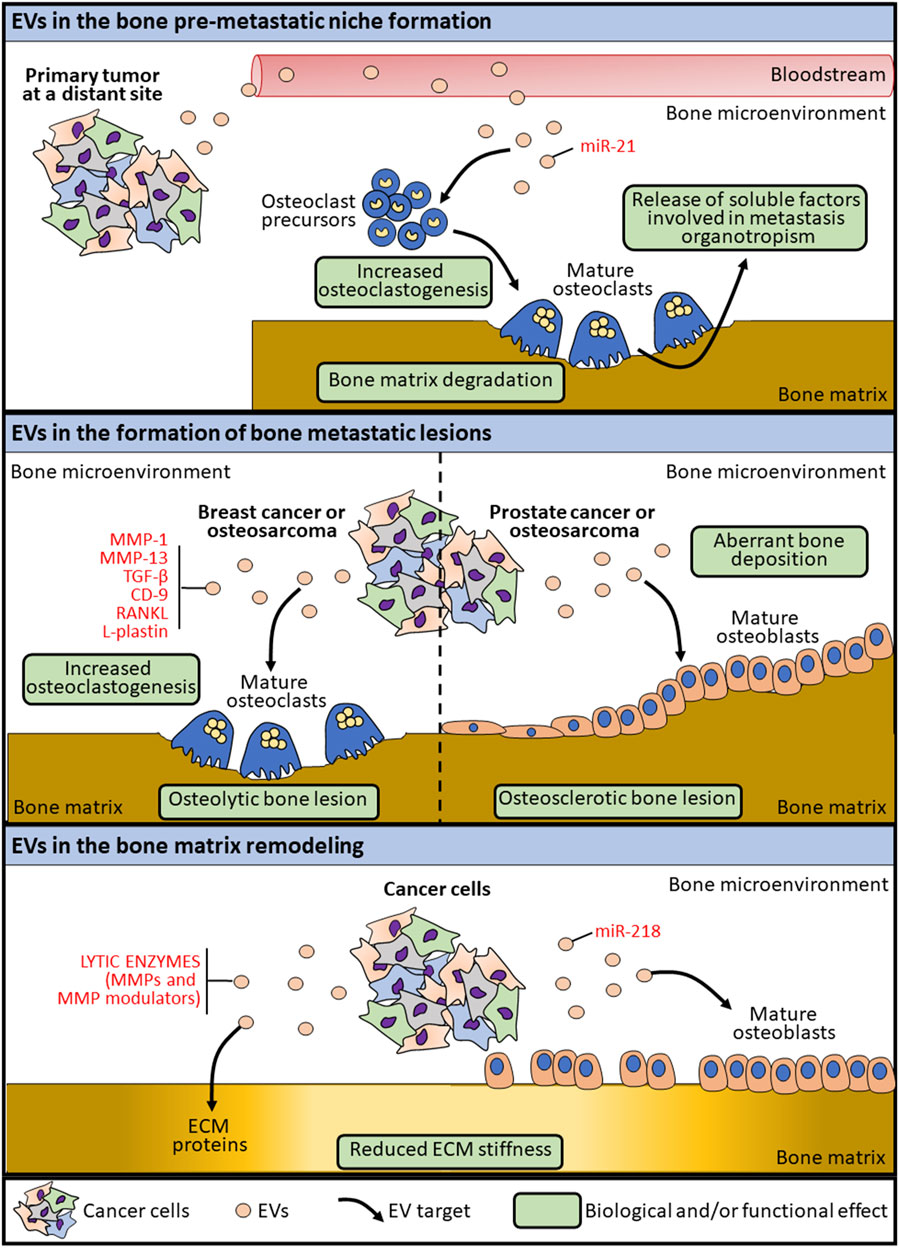
FIGURE 1. Schematic representation of the mechanisms by which EVs are involved in bone lesion formation.
Experimental Critical Issues in Studying EVs and Mechanoenvironment
From an experimental point of view, studying the extracellular vesicles is a complex challenge due to the heterogeneity of these particles (Beltraminelli et al., 2021). Furthermore, several revisions of the nomenclature referring to EVs have occurred in recent years, trying to identify a specific signature for each EV sub-populations and to standardize isolation and characterization procedures (Théry et al., 2018). The traditional classification into exosomes, microvesicles and apoptotic bodies based on biogenesis and surface protein markers can no longer be considered specific due to the overlap of size range, density and endosome-associated protein expression among EV categories. For this reason, a more recent and comprehensive classification of EVs is based on differential ultracentrifugation, the most used technique for EV isolation from cell culture media and biological fluids. By this, exosomes and microvesicles <150 nm and pelleted by 100 000g/200 000 g ultracentrifugation are collectively referred to as “small” EVs, in comparison to “large” EVs obtained by slower centrifugation (Théry et al., 2006). Therefore, given the heterogeneity of EVs and the lack of standardized techniques to isolate, purify and characterize them, it is important to keep in mind these critical issues when comparing biological results achieved by different studies. Other than any experimental bias derived by EV sample collection, purification and analysis, another level of complexity is given by the observation that EV metabolic signature depends on cell culture conditions (Palviainen et al., 2019). As deepened below, it is worth noting that the conditions in which a cell culture is maintained can modulate per se the production and the molecular signature of EVs, thus suggesting the importance in choosing the proper mechanoenvironment, in terms of both matrix stiffness and mechanical stimuli, for each cell type when the aim is studying EV production and cargo.
Role of Tumor-Derived EVs in Regulating Bone Mechanoenvironment
Extracellular vesicles secreted by cancer cells can transform the surrounding environment as well as distant tissues, thus triggering the metastatic process, by creating favorable conditions for tumor progression (Tamura et al., 2020; Mo et al., 2021). Unlike cancers that develops in other soft organs, the establishment of a tumor lesion in the bone microenvironment implies the remodeling of the bone matrix to physically create the space for tumor growth. By a mechanism known as “the vicious cycle”, cancer cells interfere with the physiological crosstalk among bone cells leading to the bone lesion (Mundy, 2002). During this process, EVs released by cancer cells or by transformed bone cells are involved in altering the bone microenvironment and the bone matrix, thereby causing the deregulation of the stiffness and the mechanics properties of the tissue (Figure 1). The crucial processes involving EVs in bone primary tumor progression, dissemination and transformation of surrounding normal cells have been well characterized (Urciuoli et al., 2018; Chicón-Bosch and Tirado, 2020). Among these, one of the main processes by which EVs modulate the bone microenvironment is through the conveying of lytic enzymes involved in extracellular matrix remodeling, as matrix metalloproteinases (MMPs) and MMP modulators (Nawaz et al., 2018). Beside the transport of ECM-remodeling factors as cargo, osteosarcoma-derived EVs can modulate the MSC and osteoblast epigenetics by inducing the expression of MMP1 and VEGF, both involved in the bone matrix remodeling (Mannerström et al., 2019). At the same time, EVs produced by cancer cells are known to interfere with the physiological bone remodeling process, by altering the functions of both osteoblasts and osteoclasts (Figure 1). Indeed, osteosarcoma-derived extracellular membrane vesicles (EMVs) convey bioactive pro-osteoclastogenesis factors (as MMP-1 and -13, TGF-β, CD-9 and RANKL) responsible in stimulating osteoclast formation and bone resorption (Garimella et al., 2014). Raimondi et al. also described the packaging of pro-osteoclastogenesis miRNAs (miR-148a-3p and miR-21-5p) in osteosarcoma-derived exosomes that are responsible of promoting osteoclast differentiation and bone resorption activity (Raimondi et al., 2020), further demonstrating a specific role for cancer-derived exosome cargo in the alteration of bone matrix remodeling (Table 1). In the context of bone lesions induced by cancer cells developed in distant organs, EVs exploit the same pathways mentioned above to alter, directly or by transforming the surrounding cells, the bone mechanoenvironment. Prostate cancer-derived EVs are capable to affect both osteoblasts and osteoclasts, leading to osteogenic or osteolytic metastases, respectively. Exosomes collected from a prostate cancer cell line (TRAMP-C1) impair osteoclast precursor differentiation into mature osteoclasts by decreasing the expression of differentiation markers, among which cathepsin K and MMP-9 (Karlsson et al., 2016). At the same time, prostate-cancer derived exosomes overexpressing hsa-miR-940 can stimulate an aberrant osteogenic differentiation of mesenchymal cells and the activation of osteoblasts, thereby favoring the formation of an osteosclerotic lesion (Hashimoto et al., 2018) (Table 1). Breast cancer-derived EVs are known to participate in the formation of osteolytic bone lesions usually related to this type of cancer. Exosomes collected from the conditioned medium of breast cancer cells MDA-MB-231 and conveying L-Plastin are responsible in osteoclast activation, thus inducing an osteolytic bone microenvironment favoring cancer growth (Tiedemann et al., 2019). Moreover, miR-218 conveyed by MDA-MB-231-derived EVs has been described to reduced osteoblast differentiation and type 1 collagen deposition (Liu et al., 2018), thereby altering bone matrix composition (Table 1). MiRNAs conveyed by cancer cell-derived EVs can also appease the metastatic progression to bone. MiR-192 conveyed by lung adenocarcinoma-derived exosome-like vesicles is responsible alone in eliciting a multimodal mechanism by which reducing bone metastasis by acting on tumor-induced osteoclastogenesis and interfering with metastatic angiogenesis (Valencia et al., 2014) (Table 1). It is worth noting that, in the case of metastatic bone lesions, cancer-derived EVs are involved in supporting a pre-metastatic niche formation to prime successful tumor growth and survival in an otherwise hostile environment for circulating tumor cells (Guo et al., 2019) (Figure 1). Exosomes collected from conditioned medium of SCP28 cells, a bone-seeking subpopulation of MDA-MB-231 breast cancer cell line, promote the differentiation and the resorbing activity of osteoclasts, thus favoring the formation of a pre-metastatic niche via transferring miR-21 to bone cells (Yuan et al., 2021) (Table 1). All together, these findings demonstrate that EVs represent an important factor in the crosstalk between tumor and the host tissue and in the modulation of the bone extracellular matrix composition by interfering with bone cell activities.
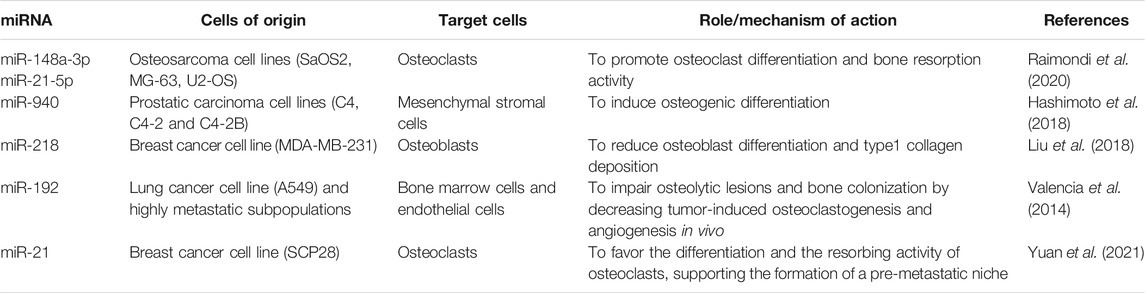
TABLE 1. List of cited miRNAs conveyed as EV cargo and involved in the crosstalk between EVs and mechanoenvironment in bone tumors.
How Mechanical Stimuli Influence the EV-Mediated Effects in the Bone Tumor Microenvironment
The tumor microenvironment is commonly defined as desmoplastic, namely a condition characterized by chronic inflammatory status, hyperactivation of fibroblasts and pro-fibrotic pathways, elevated angiogenesis and increased production of ECM proteins responsible in stiffening the surrounding stroma, thereby altering the physiological mechanoenvironment (Pickup et al., 2014). The desmoplastic response of tissue stroma to the presence of cancer cells has been described to be driven, at least in part, by cancer-derived EVs which act on resident cells to increase ECM protein production (Jiang et al., 2017; Saber et al., 2020). In turn, matrix stiffness in the tumor microenvironment can modulate the effects mediated by cancer-derived EVs, as well as mechanical stimuli impact the EV biological properties (Piffoux et al., 2018). By using synthetic substrates mimicking the stiffness of the tumor and tumor stroma, Schwager and co-authors demonstrated that microvesicles collected from high aggressive breast cancer cell line MDA-MB-231 cultured on stiff matrix (20 kPa Polyacrylamide gels) were more able to induce a transforming phenotype in normal NIH-3t3 fibroblasts than by using softer matrices (Schwager et al., 2019). These data suggest that matrix stiffness in the tumor microenvironment can prime normal cells for response to cancer-derived EVs. Moreover, the mechanical nature of the bone matrix can influence the EV cargo. Indeed, a proteomic study on EVs produced by mineralizing vs. non-mineralizing osteoblasts revealed several differences in terms of conveyed proteins and of effects on prostate cancer cell growth (Morhayim et al., 2015), leading to the speculation that an altered mineralization status likely occurring in bone metastasis can modulate the production and the cargo of osteoblast-derived EVs. Furthermore, Morrell and co-authors showed that mechanical stimulation of osteocytes induced Ca2+ oscillations influencing EV release and regulating bone formation, both in vitro and in vivo experimentations (Morrell et al., 2018). Starting from these observations, a speculation can be made on an existing loop between EV cargo and release and the peculiar mechanoenvironment associated to a bone tumor lesion.
Clinical Relevance of EV-Mechanoenvironment Crosstalk in Bone Tumors
Although the feasibility to interfere with EV production has not been already explored in the clinical approach to cancers, these vesicles are good biomarkers for cancer diagnosis and prognosis, as well as a feasible tool for drug delivery in pre-clinical and clinical applications (Ciferri et al., 2021). Similarly, as a consequence of the altered ECM processing and degradation in cancer, the protein-specific fragments deriving from ECM turnover and released in the bloodstream can be potentially used as circulating biomarkers useful for clinical diagnosis and monitoring of cancer patients (Leeming et al., 2011; Petersen et al., 2020). The relevance in considering the interplay between EV production and the bone mechanoenvironment can be instrumental in the therapeutic approaches to bone cancers. Indeed, therapies aim at reducing tissue stiffness have been exploited to decrease tumor aggressiveness and potentiate cancer treatment response. Anti-fibrotic drugs used to reduce ECM stiffening in solid cancers can also act on ECM-derived EV production by cells within the primary tumor, thereby impacting metastatization by preventing the EV-mediated conditioning of the premetastatic niche (Guo et al., 2019). Moreover, the altered ECM stiffness associated to bone tumor progression leads to changes in ECM-derived EV release, and the coordinated modulation of these two biomarkers can be monitored simultaneously, providing a new diagnostic/prognostic tool specific for the management of bone tumors. Another relevant aspect that is worth to be considered is the in vitro experimental conditions used in the pre-clinical studies on cancer cell culture models. The procedures historically developed for cancer cell in vitro experimentation involved utilizing a flat layer of cells attaching to plastic/glass substrates. Now, the scientific community has fully accepted that two-dimensional (2D) culture conditions is not physiologically relevant, and studies achieved in such conditions may difficulty be translated in vivo (Lovitt et al., 2014). The tissue-specific mechanoenvironment is an essential component of a tumor and must be recapitulated as much as possible in cell culture models. This can be achieved by using three-dimensional conditions and substrates with the stiffness of the tissue of origin. Notably, EV production in cell culture is strictly related to the experimental condition (Villasante et al., 2016; Palviainen et al., 2019), and this is another key aspect to be considered when cancer-derived EVs are studies in cell culture models.
Conclusion
The tumor microenvironment is a complex structure containing cells (malignant and not), soluble factors and extracellular matrix. Cancer cells can alter the surrounding environment directly or by conditioning the normal cells, by processes involving, among others, the stiffening of the ECM and the production of transforming EVs. These two phenomena are interconnected and modulate each other, participating in a coordinated way in the onset, progression and metastasis of virtually any tumor. In the bone microenvironment, dysregulated mechanical stimuli, both in terms of matrix stiffness and of perceived mechanical forces, can act firstly on mechanosensing osteocytes, then on bone and/or cancer cells which in turn respond by releasing EVs. These can target cells in the microenvironment, interfering with the physiological bone homeostasis processes and fueling the formation and the progression of a bone tumor lesion by regulating the bone ECM composition and stiffness. Here, we recapitulated the functional crosstalk between EVs and the mechanoenvironment in bone tumors, also emphasizing the relevance to consider these aspects in the in vitro cell culture models for cancer drug development and personalized therapy.
Author Contributions
EU and BP contributed equally, conceptualized the paper, wrote the manuscript, read, edited and approved the submitted version.
Funding
This work was supported by a grant from the Italian Ministry of Health (“Ricerca corrente 2020”) to BP.
Conflict of Interest
The authors declare that the research was conducted in the absence of any commercial or financial relationships that could be construed as a potential conflict of interest.
Publisher’s Note
All claims expressed in this article are solely those of the authors and do not necessarily represent those of their affiliated organizations, or those of the publisher, the editors and the reviewers. Any product that may be evaluated in this article, orclaim that may be made by its manufacturer, is not guaranteed or endorsed by the publisher.
References
Beltraminelli, T., Perez, C. R., and De Palma, M. (2021). Disentangling the Complexity of Tumor-Derived Extracellular Vesicles. Cel Rep. 35, 108960. doi:10.1016/j.celrep.2021.108960
Bonewald, L. F. (2011). The Amazing Osteocyte. J. Bone Miner Res. 26 (2), 229–238. doi:10.1002/jbmr.320
Buenrostro, D., Mulcrone, P. L., Owens, P., and Sterling, J. A. (2016). The Bone Microenvironment: a Fertile Soil for Tumor Growth. Curr. Osteoporos. Rep. 14, 151–158. doi:10.1007/s11914-016-0315-2
Bussard, K. M., Gay, C. V., and Mastro, A. M. (2008). The Bone Microenvironment in Metastasis; what Is Special about Bone. Cancer Metastasis Rev. 27, 41–55. doi:10.1007/s10555-007-9109-4
Cecchini, M. G., Wetterwald, A., Pluijm, G. v. d., and Thalmann, G. N. (2005). Molecular and Biological Mechanisms of Bone Metastasis. EAU Update Ser. 3 (4), 214–226. doi:10.1016/j.euus.2005.09.006
Chicón-Bosch, M., and Tirado, O. M. (2020). Exosomes in Bone Sarcomas: Key Players in Metastasis. Cells 9, 241. doi:10.3390/cells9010241
Choi, J. H., and Ro, J. Y. (2021). The 2020 WHO Classification of Tumors of Bone: An Updated Review. Adv. Anat. Pathol. 28, 119–138. doi:10.1097/PAP.0000000000000293
Ciferri, M. C., Quarto, R., and Tasso, R. (2021). Extracellular Vesicles as Biomarkers and Therapeutic Tools: From Pre-clinical to Clinical Applications. Biology 10, 359. doi:10.3390/biology10050359
Cook, L. M., Shay, G., Aruajo, A., and Lynch, C. C. (2014). Integrating New Discoveries into the "vicious Cycle" Paradigm of Prostate to Bone Metastases. Cancer Metastasis Rev. 33, 511–525. doi:10.1007/s10555-014-9494-4
Cui, Y., Luan, J., Li, H., Zhou, X., and Han, J. (2016). Exosomes Derived from Mineralizing Osteoblasts Promote ST2 Cell Osteogenic Differentiation by Alteration of microRNA Expression. FEBS Lett. 590 (1), 185–192. doi:10.1002/1873-3468.12024
Dai, J., Su, Y., Zhong, S., Cong, L., Liu, B., Yang, J., et al. (2020). Exosomes: Key Players in Cancer and Potential Therapeutic Strategy. Sig Transduct Target. Ther. 5, 1–10. doi:10.1038/s41392-020-00261-0
Doglioni, G., Parik, S., and Fendt, S.-M. (2019). Interactions in the (Pre)metastatic Niche Support Metastasis Formation. Front. Oncol. 9, 219. doi:10.3389/fonc.2019.00219
Eichholz, K. F., Woods, I., Riffault, M., Johnson, G. P., Corrigan, M., Lowry, M. C., et al. (2020). Human Bone Marrow Stem/stromal Cell Osteogenesis Is Regulated via Mechanically Activated Osteocyte‐derived Extracellular Vesicles. STEM CELLS Transl Med. 9 (11), 1431–1447. doi:10.1002/sctm.19-0405
Ekström, K., Omar, O., Granéli, C., Wang, X., Vazirisani, F., and Thomsen, P. (2013). Monocyte Exosomes Stimulate the Osteogenic Gene Expression of Mesenchymal Stem Cells. PLoS ONE 8 (9), e75227. doi:10.1371/journal.pone.0075227
Esposito, M., Guise, T., and Kang, Y. (2018). The Biology of Bone Metastasis. Cold Spring Harb Perspect. Med. 8 (6), a031252. doi:10.1101/cshperspect.a031252
Franchi, A. (2012). Epidemiology and Classification of Bone Tumors. Clin. Cases Miner Bone Metab. 9, 92–95.
Furuta, T., Miyaki, S., Ishitobi, H., Ogura, T., Kato, Y., Kamei, N., et al. (2016). Mesenchymal Stem Cell-Derived Exosomes Promote Fracture Healing in a Mouse Model. STEM CELLS Translational Med. 5 (12), 1620–1630. doi:10.5966/sctm.2015-0285
Garimella, R., Washington, L., Isaacson, J., Vallejo, J., Spence, M., Tawfik, O., et al. (2014). Extracellular Membrane Vesicles Derived from 143B Osteosarcoma Cells Contain Pro-osteoclastogenic Cargo: A Novel Communication Mechanism in Osteosarcoma Bone Microenvironment. Translational Oncol. 7 (3), 331–340. doi:10.1016/j.tranon.2014.04.011
Guise, T. A., Mohammad, K. S., Clines, G., Stebbins, E. G., Wong, D. H., Higgins, L. S., et al. (2006). Basic Mechanisms Responsible for Osteolytic and Osteoblastic Bone Metastases: Fig. 1. Clin. Cancer Res. 12, 6213s–6216s. doi:10.1158/1078-0432.CCR-06-1007
Guo, Y., Ji, X., Liu, J., Fan, D., Zhou, Q., Chen, C., et al. (2019). Effects of Exosomes on Pre-metastatic Niche Formation in Tumors. Mol. Cancer 18. doi:10.1186/s12943-019-0995-1
Han, L., Lam, E. W.-F., and Sun, Y. (2019). Extracellular Vesicles in the Tumor Microenvironment: Old Stories, but New Tales, 18. Chennai: Molecular Cancer. BioMed Central Ltd., 1–14. doi:10.1186/s12943-019-0980-8
Hashimoto, K., Ochi, H., Sunamura, S., Kosaka, N., Mabuchi, Y., Fukuda, T., et al. (2018). Cancer-secreted Hsa-miR-940 Induces an Osteoblastic Phenotype in the Bone Metastatic Microenvironment via Targeting ARHGAP1 and FAM134A. Proc. Natl. Acad. Sci. USA 115 (9), 2204–2209. doi:10.1073/pnas.1717363115
Hu, C., Qin, Q.-H., and Qin, Q.-H. (2020). Bone Remodeling and Biological Effects of Mechanical Stimulus. AIMS Bioeng. 7 (1), 12–28. doi:10.3934/bioeng.2020002
Huynh, N., VonMoss, L., Smith, D., Rahman, I., Felemban, M. F., Zuo, J., et al. (2016). Characterization of Regulatory Extracellular Vesicles from Osteoclasts. J. Dent Res. 95 (6), 673–679. doi:10.1177/0022034516633189
Jiang, X., Hu, S., Liu, Q., Qian, C., Liu, Z., and Luo, D. (2017). Exosomal microRNA Remodels the Tumor Microenvironment. PeerJ 5 (12), e4196. doi:10.7717/peerj.4196
Karlsson, T., Lundholm, M., Widmark, A., and Persson, E. (2016). Tumor Cell-Derived Exosomes from the Prostate Cancer Cell Line TRAMP-C1 Impair Osteoclast Formation and Differentiation. PLoS ONE 11 (11), e0166284. doi:10.1371/journal.pone.0166284
Lamora, A., Talbot, J., Mullard, M., Brounais-Le Royer, B., Redini, F., and Verrecchia, F. (2016). TGF-β Signaling in Bone Remodeling and Osteosarcoma Progression. Jcm 5 (11), 96. doi:10.3390/jcm5110096
Leeming, D. J., Bay-Jensen, A. C., Vassiliadis, E., Larsen, M. R., Henriksen, K., and Karsdal, M. A. (2011). Post-translational Modifications of the Extracellular Matrix Are Key Events in Cancer Progression: Opportunities for Biochemical Marker Development. Biomarkers 16 (3), 193–205. doi:10.3109/1354750X.2011.557440
Li, D., Liu, J., Guo, B., Liang, C., Dang, L., Lu, C., et al. (2016). Osteoclast-derived Exosomal miR-214-3p Inhibits Osteoblastic Bone Formation. Nat. Commun. 7. doi:10.1038/ncomms10872
Li, F. X. Z., Liu, J. J., Xu, F., Lin, X., Zhong, J. Y., Wu, F., et al. (2019). Role of Tumor-derived E-xosomes in B-one M-etastasis (Review). Oncol. Lett., 3935–3945. doi:10.3892/ol.2019.10776
Lin, X., Patil, S., Gao, Y.-G., and Qian, A. (2020). The Bone Extracellular Matrix in Bone Formation and Regeneration. Front. Pharmacol. 11. doi:10.3389/fphar.2020.00757
Liu, X., Cao, M., Palomares, M., Wu, X., Li, A., Yan, W., et al. (2018). Metastatic Breast Cancer Cells Overexpress and Secrete miR-218 to Regulate Type I Collagen Deposition by Osteoblasts. Breast Cancer Res. 20 (1). doi:10.1186/s13058-018-1059-y
Logozzi, M., Mizzoni, D., Angelini, D., Di Raimo, R., Falchi, M., Battistini, L., et al. (2018). Microenvironmental pH and Exosome Levels Interplay in Human Cancer Cell Lines of Different Histotypes. Cancers 10 (10), 370. doi:10.3390/cancers10100370
Lovitt, C., Shelper, T., and Avery, V. (2014). Advanced Cell Culture Techniques for Cancer Drug Discovery. Biology 3, 345–367. doi:10.3390/biology3020345
Macedo, F., Ladeira, K., Pinho, F., Saraiva, N., Bonito, N., Pinto, L., et al. (2017). Bone Metastases: An Overview. Oncol. Rev. 11 (1). doi:10.4081/oncol.2017.321
Mannerström, B., Kornilov, R., Abu-Shahba, A. G., Chowdhury, I. M., Sinha, S., Seppänen-Kaijansinkko, R., et al. (2019). Epigenetic Alterations in Mesenchymal Stem Cells by Osteosarcoma-Derived Extracellular Vesicles. Epigenetics 14 (4), 352–364. doi:10.1080/15592294.2019.1585177
Mo, Z., Cheong, J. Y. A., Xiang, L., Le, M. T. N., Grimson, A., and Zhang, D. X. (2021). Extracellular Vesicle‐associated Organotropic Metastasis. Cell Prolif 54, e12948. doi:10.1111/cpr.12948
Morhayim, J., Peppel, J., Demmers, J. A. A., Kocer, G., Nigg, A. L., Driel, M., et al. (2015). Proteomic Signatures of Extracellular Vesicles Secreted by Nonmineralizing and Mineralizing Human Osteoblasts and Stimulation of Tumor Cell Growth. FASEB j. 29 (1), 274–285. doi:10.1096/fj.14-261404
Morrell, A. E., Brown, G. N., Robinson, S. T., Sattler, R. L., Baik, A. D., Zhen, G., et al. (2018). Mechanically Induced Ca2+ Oscillations in Osteocytes Release Extracellular Vesicles and Enhance Bone Formation. Bone Res. 6 (1). doi:10.1038/s41413-018-0007-x
Mundy, G. R. (2002). Metastasis to Bone: Causes, Consequences and Therapeutic Opportunities. Nat. Rev. Cancer 2, 584–593. doi:10.1038/nrc867
Nawaz, M., Shah, N., Zanetti, B., Maugeri, M., Silvestre, R., Fatima, F., et al. (2018). Extracellular Vesicles and Matrix Remodeling Enzymes: The Emerging Roles in Extracellular Matrix Remodeling, Progression of Diseases and Tissue Repair. Progression Dis. Tissue Repair’Cells 7 (10), 167. doi:10.3390/cells7100167
Nicastro, L., Catapano, F., Nunez Toldra, R., Floriano, J., Oliveira, C. C., Emanueli, C., et al. (2020). Mechanical Load Modulates the Cargo of Secreted Extracellular Vesicles (EVs) from Living Myocardial Slices. Eur. Heart J. 41 (Suppl. ment_2). doi:10.1093/ehjci/ehaa946.3614
Palviainen, M., Saari, H., Kärkkäinen, O., Pekkinen, J., Auriola, S., Yliperttula, M., et al. (2019). Metabolic Signature of Extracellular Vesicles Depends on the Cell Culture Conditions. J. Extracellular Vesicles 8 (1), 1596669. doi:10.1080/20013078.2019.1596669
Parolini, I., Federici, C., Raggi, C., Lugini, L., Palleschi, S., De Milito, A., et al. (2009). Microenvironmental pH Is a Key Factor for Exosome Traffic in Tumor Cells. J. Biol. Chem. 284 (49), 34211–34222. doi:10.1074/jbc.M109.041152
Peruzzi, B., and Teti, A. (2012). The Physiology and Pathophysiology of the Osteoclast. Clinic Rev. Bone Miner Metab. 10 (2), 71–97. doi:10.1007/s12018-011-9086-6
Petersen, E. V., Chudakova, D. A., Skorova, E. Y., Anikin, V., Reshetov, I. V., and Mynbaev, O. A. (2020). The Extracellular Matrix-Derived Biomarkers for Diagnosis, Prognosis, and Personalized Therapy of Malignant Tumors. Front. Oncol. 10, 2792. doi:10.3389/fonc.2020.575569
Pickup, M. W., Mouw, J. K., and Weaver, V. M. (2014). The Extracellular Matrix Modulates the Hallmarks of Cancer. EMBO Rep. 15 (12), 1243–1253. doi:10.15252/embr.201439246
Piffoux, M., Nicolás-Boluda, A., Mulens-Arias, V., Richard, S., Rahmi, G., Gazeau, F., et al. (2018). Extracellular Vesicles for Personalized Medicine: The Input of Physically Triggered Production, Loading and Theranostic Properties. Adv Drug Deliv Rev. 138, 247–258. doi:10.1016/j.addr.2018.12.009
Qin, L., Liu, W., Cao, H., and Xiao, G. (2020). Molecular Mechanosensors in Osteocytes. Bone Res. 8, 1–24. doi:10.1038/s41413-020-0099-y
Raimondi, L., De Luca, A., Gallo, A., Costa, V., Russelli, G., Cuscino, N., et al. (2020). Osteosarcoma Cell-Derived Exosomes Affect Tumor Microenvironment by Specific Packaging of microRNAs. Carcinogenesis 41 (5), 666–677. doi:10.1093/carcin/bgz130
Ren, G., Esposito, M., and Kang, Y. (2015). Bone Metastasis and the Metastatic Niche. J. Mol. Med. 93, 1203–1212. doi:10.1007/s00109-015-1329-4
Saber, S. H., Ali, H. E. A., Gaballa, R., Gaballah, M., Ali, H. I., Zerfaoui, M., et al. (2020). Exosomes Are the Driving Force in Preparing the Soil for the Metastatic Seeds: Lessons from the Prostate Cancer. Cells 9, 564. doi:10.3390/cells9030564
Schwager, S. C., Bordeleau, F., Zhang, J., Antonyak, M. A., Cerione, R. A., and Reinhart-King, C. A. (2019). Matrix Stiffness Regulates Microvesicle-Induced Fibroblast Activation. Am. J. Physiology-Cell Physiol. 317 (1), C82–C92. doi:10.1152/ajpcell.00418.2018
Sekita, A., Matsugaki, A., and Nakano, T. (2017). Disruption of Collagen/apatite Alignment Impairs Bone Mechanical Function in Osteoblastic Metastasis Induced by Prostate Cancer. Bone 97, 83–93. doi:10.1016/j.bone.2017.01.004
Sottnik, J. L., Dai, J., Zhang, H., Campbell, B., and Keller, E. T. (2015). Tumor-induced Pressure in the Bone Microenvironment Causes Osteocytes to Promote the Growth of Prostate Cancer Bone Metastases. Cancer Res. 75 (11), 2151–2158. doi:10.1158/0008-5472.CAN-14-2493
Sun, W., Zhao, C., Li, Y., Wang, L., Nie, G., Peng, J., et al. (2016). Osteoclast-derived microRNA-Containing Exosomes Selectively Inhibit Osteoblast Activity. Cell Discov 2. doi:10.1038/celldisc.2016.15
Tai, Y. L., Chen, K. C., Hsieh, J. T., and Shen, T. L. (2018). ‘Exosomes in Cancer Development and Clinical Applications’, Cancer Science, 109. United States: Wiley-Blackwell, 2364–2374. doi:10.1111/cas.13697
Tamura, T., Yoshioka, Y., Sakamoto, S., Ichikawa, T., and Ochiya, T. (2020). Extracellular Vesicles in Bone Metastasis: Key Players in the Tumor Microenvironment and Promising Therapeutic Targets. Ijms 21 (18), 1–17. doi:10.3390/ijms21186680
Taube, T., Elomaa, I., Blomqvist, C., Beneton, M. N. C., and Kanis, J. A. (1994). Histomorphometric Evidence for Osteoclast-Mediated Bone Resorption in Metastatic Breast Cancer. Bone 15 (2), 161–166. doi:10.1016/8756-3282(94)90703-X
Théry, C., Amigorena, S., Raposo, G., and Clayton, A. (2006). Isolation and Characterization of Exosomes from Cell Culture Supernatants and Biological Fluids. Curr. Protoc. Cel Biol. 30 (1), 31–223. doi:10.1002/0471143030.cb0322s30
Théry, C., Kenneth, W. W., Elena, A., Maria, J. A., Johnathon, D. A., and Ramaroson, A. (2018). Minimal Information for Studies of Extracellular Vesicles 2018 (MISEV2018): a Position Statement of the International Society for Extracellular Vesicles and Update of the MISEV2014 Guidelines. J. Extracellular Vesicles 7 (1). doi:10.1080/20013078.2018.1535750
Tiedemann, K., Sadvakassova, G., Mikolajewicz, N., Juhas, M., Sabirova, Z., Tabariès, S., et al. (2019). Exosomal Release of L-Plastin by Breast Cancer Cells Facilitates Metastatic Bone Osteolysis. Translational Oncol. 12 (3), 462–474. doi:10.1016/j.tranon.2018.11.014
Urciuoli, E., Giorda, E., Scarsella, M., Petrini, S., and Peruzzi, B. (2018). Osteosarcoma‐derived Extracellular Vesicles Induce a Tumor‐like Phenotype in normal Recipient Cells. J. Cel Physiol 233 (8), 6158–6172. doi:10.1002/jcp.26464
Valencia, K., Luis-Ravelo, D., Bovy, N., Antón, I., Martínez-Canarias, S., Zandueta, C., et al. (2014). MiRNA Cargo within Exosome-like Vesicle Transfer Influences Metastatic Bone Colonization. Mol. Oncol. 8 (3), 689–703. doi:10.1016/j.molonc.2014.01.012
Villasante, A., Marturano-Kruik, A., Ambati, S. R., Liu, Z., Godier-Furnemont, A., Parsa, H., et al. (2016). Recapitulating the Size and Cargo of Tumor Exosomes in a Tissue-Engineered Model. Theranostics 6 (8), 1119–1130. doi:10.7150/thno.13944
Wang, H., Pan, J., Barsky, L., Jacob, J. C., Zheng, Y., Gao, C., et al. (2021). Characteristics of Pre-metastatic Niche: the Landscape of Molecular and Cellular Pathways. Mol. Biomed. 2 (1), 1–32. doi:10.1186/s43556-020-00022-z
Wang, X., Guo, B., Li, Q., Peng, J., Yang, Z., Wang, A., et al. (2013). MiR-214 Targets ATF4 to Inhibit Bone Formation. Nat. Med. 19 (1), 93–100. doi:10.1038/nm.3026
Xie, Y., Chen, Y., Zhang, L., Ge, W., and Tang, P. (2017). The Roles of Bone-Derived Exosomes and Exosomal microRNAs in Regulating Bone Remodelling. J. Cel. Mol. Med. 21, 1033–1041. doi:10.1111/jcmm.13039
Yan, H.-C., Yu, T.-T., Li, J., Qiao, Y.-Q., Wang, L.-C., Zhang, T., et al. (2020). The Delivery of Extracellular Vesicles Loaded in Biomaterial Scaffolds for Bone Regeneration. Front. Bioeng. Biotechnol. 8, 1015. doi:10.3389/fbioe.2020.01015
Yáñez-Mó, M., Pia, R-M. S., Zoraida, A., Apolonija, B. Z., Francesc, E. B., and Edit, I. B. (2015). Journal of Extracellular Vesicles 4 (1), 27066. doi:10.3402/jev.v4.27066
Yuan, X., Qian, N., Ling, S., Li, Y., Sun, W., Li, J., et al. (2021). Breast Cancer Exosomes Contribute to Pre-metastatic Niche Formation and Promote Bone Metastasis of Tumor Cells. Theranostics 11 (3), 1429–1445. doi:10.7150/thno.45351
Zaborowski, M. P., Balaj, L., Breakefield, X. O., and Lai, C. P. (2015). Extracellular Vesicles: Composition, Biological Relevance, and Methods of Study, 65. BioScience: Oxford University Press, 783–797. doi:10.1093/biosci/biv084
Keywords: mechanoenvironment, tumor microenvironment, extracellular vesicles, bone tumors, metastatization
Citation: Urciuoli E and Peruzzi B (2021) Mutual Modulation Between Extracellular Vesicles and Mechanoenvironment in Bone Tumors. Front. Cell Dev. Biol. 9:789674. doi: 10.3389/fcell.2021.789674
Received: 05 October 2021; Accepted: 22 November 2021;
Published: 07 December 2021.
Edited by:
Roberta Tasso, University of Genoa, ItalyReviewed by:
Margherita Cortini, Rizzoli Orthopedic Institute (IRCCS), ItalyWolfgang Holnthoner, Ludwig Boltzmann Institute for Experimental and Clinical Traumatology, Austria
Copyright © 2021 Urciuoli and Peruzzi. This is an open-access article distributed under the terms of the Creative Commons Attribution License (CC BY). The use, distribution or reproduction in other forums is permitted, provided the original author(s) and the copyright owner(s) are credited and that the original publication in this journal is cited, in accordance with accepted academic practice. No use, distribution or reproduction is permitted which does not comply with these terms.
*Correspondence: Barbara Peruzzi, YmFyYmFyYS5wZXJ1enppQG9wYmcubmV0