- 1Department of Orthopedics, The First Affiliated Hospital of Zhengzhou University, Zhengzhou, China
- 2Department of Orthopedics, Xiangya Hospital, Central South University, Changsha, China
When suffering from osteoarthritis (OA), articular cartilage homeostasis is out of balance and the living quality declines. The treatment of knee OA has always been an unsolved problem in the world. At present, symptomatic treatment is mainly adopted for OA. Drug therapy is mainly used to relieve pain symptoms, but often accompanied with adverse reactions; surgical treatment involves the problem of poor integration between the repaired or transplanted tissues and the natural cartilage, leading to the failure of repair. Biotherapy which aims to promote cartilage in situ regeneration and to restore endochondral homeostasis is expected to be an effective method for the prevention and treatment of OA. Disease-modifying osteoarthritis drugs (DMOADs) are intended for targeted treatment of OA. The DMOADs prevent excessive destruction of articular cartilage through anti-catabolism and stimulate tissue regeneration via excitoanabolic effects. Sprifermin (recombinant human FGF18, rhFGF18) is an effective DMOAD, which can not only promote the proliferation of articular chondrocyte and the synthesis of extracellular matrix, increase the thickness of cartilage in a dose-dependent manner, but also inhibit the activity of proteolytic enzymes and remarkedly slow down the degeneration of cartilage. This paper reviews the unique advantages of Sprifermin in repairing cartilage injury and improving cartilage homeostasis, aiming to provide an important strategy for the effective prevention and treatment of cartilage injury-related diseases.
Introduction
The pathogenesis of OA mostly involves the whole joint, mainly including cartilage, subchondral bone, joint capsule, synovial membrane and surrounding muscles, most manifested as cartilage injury, subchondral osteosclerosis and synovial inflammation (Karsdal et al., 2016; Mehana et al., 2019; Wu et al., 2020). Cartilage, a kind of connective tissue without nerves and blood vessels, exists in joint, intervertebral disc, ear, nose, etc., and plays an important role in the normal structural composition of human joint and movement (Krishnan and Grodzinsky, 2018; Vincent and Wann, 2019; Żylińska et al., 2021). Articular cartilage is mainly composed of chondrocytes and extracellular matrix (ECM), the ECM mainly contains water, type II collagen (COL2), proteoglycan and glycosaminoglycan (GAG) (Muller et al., 2020). Cartilage is extremely vulnerable to overloading, inflammation, trauma, etc., and articular cartilage damage is one of the major pathological changes of OA.
Articular cartilage has a poor ability to repair itself due to inadequate blood supply and low metabolism. The cartilage in femorotibial joint can relieve pressure and maintain frictionless movement of joint. If the injury of cartilage in OA is not repaired in time, it will further aggravate and involve the surrounding tissues (Martínez-Moreno et al., 2019; Zhao et al., 2019; Zhang S. et al., 2020). Results of microscopy studies showed that the cartilage structure is disorganized, the number of chondrocytes decreased, and the synthesis of COL2, proteoglycan and GAG is obviously reduced during the occurrence of OA, and eventually lead to cartilage tear. The ulcerated surface is easily formed following cartilage tear, which can be covered by connective tissue or fibrocartilage, accompanied by neovascularization, resulting in damage of articular cartilage with the loss of its full-thickness and damage to the biomechanics of articular cartilage (Brody, 2015; McCulloch et al., 2019; Chen et al., 2020). With the further progression of OA, more matrix degradation related enzymes, such as matrix metalloproteinase-13 (MMP-13) and metalloproteinase-5 (ADAMTS-5), inflammatory factors such as tumor necrosis factor α (TNFα) and interleukin-1β (IL-1β) are produced, eventually leading to deteriorative biochemical changes in articular cartilage (van den Berg, 2011; Malemud, 2019; Pu et al., 2021; Zhang et al., 2021). The above biomechanical and biochemical changes ignite each other, forming a “positive feedback” effect similar to a vicious cycle, synergistically disturbing cartilage homeostasis, aggravating the damage of cartilage and its surrounding tissues, eventually accelerating the development of OA (Kapoor et al., 2011; Varady and Grodzinsky, 2016).
Symptomatic treatment is mainly adopted for knee OA, but lacks effective targeted treatment options (Katz et al., 2021). The treatment plan mainly includes: 1. Drug intervention, such as oral acetaminophen, non-steroidal anti-inflammatory drugs, glucosamine, chondroitin sulfate and intraarticular injection of sodium hyaluronate (Alexander et al., 2020; Wolff et al., 2021). 2. Exercise, proper exercise helps strengthen muscle strength and improves joint function (Skou et al., 2018). 3. Weight management, encouraging overweight patients to lose weight, thereby reducing the pressure load and inflammation state in the joints (Springer et al., 2017; Harasymowicz et al., 2020; Oliveira et al., 2020). 4. Traditional Chinese medicine treatment, such as Chinese medicine ointment, massage, acupuncture and moxibustion, which are mainly used to improve blood circulation (Walsh et al., 2017; Zhang Z. et al., 2020; Park et al., 2021). 5. Surgical treatment, such as microfracture surgery, arthroscopic debridement, unicompartmental knee arthroplasty (UKA), total knee arthroplasty, etc. (Deng et al., 2021; Katz et al., 2021) (Table 1). Conservative treatment is mainly used to relieve pain symptoms, but cannot effectively prevent or reverse the progression of OA (Hochberg et al., 2012). There are also some shortcomings in surgical treatment, such as postoperative prosthesis infection, prosthesis loosening, and limited prosthesis life (not suitable for young patients) (Quinn et al., 2018; Xu et al., 2019). Relevant techniques for promoting cartilage repair through cell or cartilage transplantation have once attracted the attention of many scholars (Lv et al., 2020). However, most of these technologies have the problem of poor integration between the repaired or transplanted tissue and the natural cartilage, which changes the stress distribution during the joint load and causes the repaired or transplanted tissues to degrade, ultimately leading to failure of transplantation repair (Li Z. et al., 2016; Ernstbrunner et al., 2018). If biological therapy can effectively promote cartilage repair in situ and restore cartilage homeostasis, it will provide a powerful means for the effective prevention and treatment of OA. Disease-modifying osteoarthritis drugs (DMOADs) are a class of drugs that can be used to treat OA. DMOADs mainly include fibroblast growth factor 18 (FGF18), bone morphogenetic protein-7 (BMP-7), C-type natriuretic peptide (CNP), insulin like growth factor-1 (IGF-1) etc. (Davies et al., 2008; Hunter et al., 2010; Bükülmez et al., 2014; Zhang et al., 2017; Muller et al., 2020; Shah and Mithoefer, 2020b). (Table2). Sprifermin (recombinant human FGF18, rhFGF18) is an effective DMOAD. In the literature Sprifermin is the only DMOAD which can strongly and effectively maintain the chondrocyte phenotype in cell culture models (Gigout et al., 2017; Antunes et al., 2020; Muller et al., 2020). Sprifermin markedly promotes the proliferation of articular chondrocytes and the synthesis of ECM, and thus increases cartilage thickness in a dose-dependent manner (Hochberg et al., 2019). Also, it can efficiently inhibit proteolytic enzyme activity (such as MMP-13 and ADAMTS-5) and significantly reduce articular cartilage degeneration (Hochberg et al., 2018; Hochberg et al., 2019; Muller et al., 2020). Sprifermin is currently in phase III clinical trial, and no local or systemic safety concerns have been reported (Mori et al., 2014; Li et al., 2021; Zeng et al., 2021). In view of this, we reviewed the unique advantages of Sprifermin in promoting chondrocyte proliferation and ECM synthesis, repairing articular cartilage injury and improving cartilage homeostasis, as well as analyzed its possible molecular mechanisms, aiming to provide an important guidance for the effective prevention and treatment of articular cartilage injury related diseases with OA as a typical example.
Basic Structure and Function of Sprifermin
The fibroblast growth factor (FGF) family is a group of proteins with homology in nuclear acid sequences, which plays an important role in many pathophysiological processes such as embryo development, cell growth, tissue repair, tumor growth and invasion etc. (Hung et al., 2016; Khosravi et al., 2021; Liu et al., 2021). There are currently 19 members in the FGF family. In the late 1990s, FGF18 was identified as a new member of the FGF family (Hu et al., 1998). FGF18 was firstly isolated from mouse embryos by Maruoka et al. (Hu et al., 1998; Maruoka et al., 1998). Structurally, it is similar to FGF8 and FGF17 and is a member of the same subfamily, with 70–80% amino acid homology sequence. FGF18 is a highly conserved protein composed of 207 amino acids, and the gene encoding this protein is located on chromosome 5q34 (Whitmore et al., 2000). Yang et al. confirmed that FGF18 overexpression effectively inhibits the epithelial-mesenchymal transformation of renal clear cell carcinoma through PI3K/Akt signaling pathway (Yang et al., 2020). Boylan et al. documented that FGF8, FGF17 and FGF18 are crucial for the formation of the fetal abdominal wall (Boylan et al., 2020). Current studies in the field of OA have reported that FGF18 remarkedly stimulates the proliferation of chondrocytes and synthesis of ECM (Muller et al., 2020). Intraarticular injection of FGF18 attenuates the degradation of GAG, proteoglycan and COL2, inhibits the expression of MMP-13, reduces cartilage degeneration, down-regulates OARSI score, and ultimately promotes the regeneration and repairs of degenerated cartilage (Yao et al., 2019; Muller et al., 2020).
Sprifermin is a new truncated rhFGF18 which acts through the fibroblast growth factor receptor 3 (FGFR3). Sprifermin is roughly five times more potent in binding to this receptor compared to the natural FGF18 (Reker et al., 2020). Sprifermin exhibits strong capability in stimulating chondrocyte proliferation, stabilizing anabolic chondrocyte phenotype, promoting ECM synthesis, inhibiting proteolytic enzyme activity, increasing cartilage thickness, etc. (Hochberg et al., 2019; Muller et al., 2020; Reker et al., 2020). Sprifermin displays great potential in the treatment of OA and is expected to be marketed soon.
Sprifermin not only stimulates chondrocyte proliferation and chondrogenesis in vitro, but also effectively promotes cartilage repair in vivo (Ellsworth et al., 2002; Moore et al., 2005). Intraarticular administration of Sprifermin boosts chondrocyte proliferation, promotes cartilage anabolism, and improves cartilage biomechanical and histological properties in OA patients who are scheduled to undergo knee arthroplasty (Dahlberg et al., 2016). Through MRI observation, Roemer et al. confirmed that Sprifermin not only promotes chondrocyte proliferation in OA patients, but also plays an effective therapeutic role on bone marrow injury (Roemer et al., 2016). Animal experimental model has demonstrated that intraarticular injection of Sprifermin markedly prevents cartilage degradation and promotes the repair of damaged cartilage in traumatic OA model (Moore et al., 2005). In summary, clinical trials, in vitro experiments and animal experiments all indicate that Sprifermin possesses powerful performance in boosting chondrocyte proliferation and cartilage repair.
Sprifermin has exhibited outstanding prospects in promoting cartilage repair, and intraarticular application of Sprifermin is safe and reliable (Zeng et al., 2021). A comprehensive assessment of the specific role of Sprifermin in promoting cartilage repair and the evaluation of its potential molecular mechanisms are conducive to further improvement of Sprifermin.
Mechanism of Action of Sprifermin
Sprifermin can activate FGFR3 on the surface of chondrocytes and drive chondrocyte proliferation and ECM synthesis (Davidson et al., 2005). In both monolayer and three-dimensional culture models of porcine chondrocytes, it was observed that Sprifermin not only increased the expression of chondrocyte markers, but also decreased COL1 expression level, increased the proportion of COL2:COL1, and maintained the chondrocyte phenotype (Gigout et al., 2017). In addition, Sprifermin does not increase the activity of proteolytic enzymes and expression of hypertrophy markers in three-dimensional culture, indicating that Sprifermin does not exacerbate catabolism and chondrocyte hypertrophy, and is able to safely and effectively maintain endochondral homeostasis (Kapoor et al., 2011; Reker et al., 2017).
The effect of Sprifermin on cartilage repair may be closely related to its ability to promote biphasic ECM remodeling process. Initially, Reker et al. reported that there may be a biphasic ECM remodeling process when Sprifermin acts on bovine articular cartilage explant, which is specifically manifested as the production of proteoglycan degradation enzyme is earlier than that of COL2 synthesis enzyme (Reker et al., 2017). In addition, chondrocytes monolayer and three-dimensional culture both displayed an inverse linear relationship between early chondrocyte proliferation and ECM synthesis, which further corroborated the above point of view (Gigout et al., 2017). In 2020, Reker and colleagues further elaborated the details of Sprifermin-induced biphasic process of ECM remodeling in human knee OA articular cartilage ex vivo (Reker et al., 2020). When Sprifermin promotes chondrogenesis, chondrocyte proliferation occurs in the early-phase and ECM synthesis occurs in the late-phase. The biphasic ECM remodeling process is firstly characterized by increased aggrecanase activity, leading to degradation of proteoglycan and COL2, followed by enlarged compartment and chondrocyte proliferation space, and thus synthesis of a growing amount of proteoglycan and COL2, while the metabolic activity is maintained. Perhaps the biphasic ECM remodeling is because of the expansion of chondrocyte population is limited by the surrounding ECM. When Sprifermin stimulates the resting chondrocytes in the ECM, the chondrocytes will induce ECM degradation to enlarge the compartment and allow chondrocytes to proliferate, which in turn produces more ECM and ultimately promotes chondrogenesis. Reker and colleagues speculated that matrix degradation is a prerequisite for the initiation of chondrocyte proliferation during ECM remodeling, the maintenance of the delicate balance between chondrocyte proliferation and ECM synthesis synergistically promotes cartilage regeneration (Reker et al., 2017; Reker et al., 2020) (Figure 1).
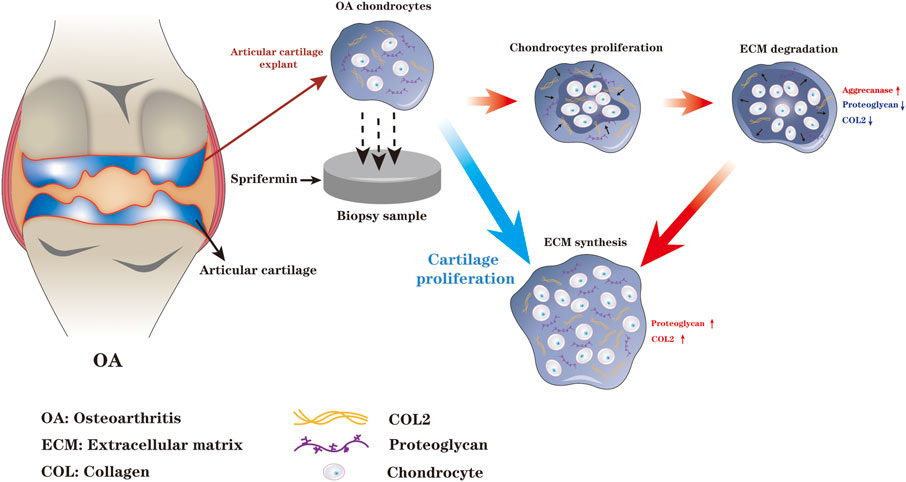
FIGURE 1. When Sprifermin acts on chondrocytes, there is a biphasic ECM remodeling process. The chondrocyte proliferation and ECM degradation occurs in the early-phase and ECM synthesis occurs in the late-phase. The biphasic ECM remodeling ensures that Sprifermin efficiently boosts chondrocyte proliferation, ECM synthesis, and ultimately promotes cartilage regeneration (The figure is made by adobe illustrator 2021).
The retention time of Sprifermin in joint capsule is limited, and the therapeutic effect can be maximized by injection at reasonable time intervals. Studies have demonstrated that the application of Sprifermin for three consecutive weeks with repetition every 6 months exerts the best therapeutic effect on promoting cartilage proliferation (Hochberg et al., 2019; Sieber and Gigout, 2020). As for the long-term continuous medication of Sprifermin, although it promotes the chondrocytes proliferation to a certain extent, the acceleration of chondrogenic anabolism is not as good as intermittent repeated exposure. Similarly, it was reported that different exposure times of Sprifermin can lead to completely different or even opposite effect (Gigout et al., 2017). Long-term exposure to Sprifermin has a weaker down-regulation effect on COL1 than once a week exposure mode, discontinuation after initial exposure is necessary for maximizing Sprifermin’s anabolic potential (Gigout et al., 2017). For example, in the three-dimensional culture of porcine chondrocytes, after exposure to Sprifermin once a week for 4 weeks, the chondrocytes in the culture evidently increased and more GAG and hydroxyproline were synthesized (Sieber and Gigout, 2020); however, when the culture was continuously exposed to Sprifermin, the ECM synthesis was notably reduced (Rozenblatt-Rosen et al., 2002; Gigout et al., 2017). In conclusion, the intermittent exposure of Sprifermin activates the anabolic response instantly, which is beneficial to cartilage repair. However, permanent exposure might act on other signaling pathways and eventually exert a weaker role in promoting cartilage repair. This phenomenon may be attributed to desensitization (or negative feedback loop) of Sprifermin during long term exposure.
FGF18 acts via the FGFR in the cell membrane and regulates the runt-related transcription factor 2 (Runx2) via signaling molecules such as mitogen-activated protein kinase (MAPK) and phosphoinositide 3-kinase (PI3K), thereby regulating cartilage formation at the molecular level (Ellsworth et al., 2002; Jeon et al., 2012; Catheline et al., 2019). Gigout et al. confirmed that extracellular signal-regulated kinases 1 (ERK1) and ERK2 play an important role in Sprifermin signaling, such as promoting the formation of chondrocyte morphology and reducing the expression of COL1 (Gigout et al., 2017). FGF18 plays a crucial role in the development of bone and cartilage, as well as the dynamic balance of bone mineralization. Ohbayashi et al. documented that FGF18 has the highest affinity to FGFR3 and moderate affinity to FGFR2 (Ohbayashi et al., 2002). FGF18 directly regulates cartilage formation by acting on FGFR3. Besides, FGF18 regulates cartilage formation through other signaling pathways indirectly, for instance, FGF18 down-regulates the hedgehog signaling pathway activity in the interarticular region (Barnard et al., 2005; Clarke, 2020).
At the cellular and molecular level, apoptosis is closely related to the occurrence and development of OA (Hwang and Kim, 2015). Mitochondria are organelles within the cytoplasm, bound by a double membrane. The membrane space and mitochondrial matrix jointly formed by outer membrane and inner membrane are capable of sensing and responding to external stressors. Generally speaking, mitochondria maintain cell homeostasis by generating energy and activating intracellular signaling pathways (Eisner et al., 2018). Reduced mitochondrial membrane potential and leakage of cytochrome C from mitochondria are landmark events of apoptosis. Under the stress environment, mitochondrial dysfunction may occur, which results in the inability to maintain enough protons to power oxidative phosphorylation (Shen et al., 2021). The PI3K/Akt pathway is a classical anti-apoptotic signal transduction pathway, which is regulated by a variety of upstream inflammatory mediators and could inhibit apoptosis and autophagy of chondrocytes (Xue et al., 2017). The activated Akt depolymerizes tuberous sclerosis complex 1 (TSC1)/TSC2 dimer, thereby abolishing the inhibitory effect on Rheb, and eventually activates mammalian target of rapamycin (mTOR) and downstream transcription factor eIF4E, regulates the expression of apoptosis-related genes (Xu et al., 2015). Phosphorylation of PI3K and Akt down-regulates the levels of various apoptotic factors such as Bax, Bim (Bcl-2-interacting mediator of cell death) and FoxO1 induced by IL-1β, thereby inhibiting cell apoptosis, restoring mitochondrial membrane potential and reducing the generation of ROS, finally promoting the proliferation and migration of chondrocytes. In most cases, the activity of PI3K/Akt signaling pathway is remarkedly blocked during the progression of OA (Wang et al., 2018; Zhang Y. et al., 2020). The balance of mitochondrial fusion and fission (MFF) can timely and effectively remove damaged mitochondria and restore the normal mechanism or function of mitochondria (Youle and van der Bliek, 2012). By maintaining MFF balance, FGF18 evidently restores the function and morphology of mitochondria, enhances the phosphorylation of PI3K and Akt, and exerts an efficient anti-osteoarthritis efficacy. Excessive activation of autophagy will exacerbate chondrocytes damage (Wang J. et al., 2020). The mTOR, an important downstream signaling molecule of PI3K/Akt, is involved in the negative regulation of autophagy (Li Y.-S. et al., 2016). After cytochrome C enters the cytoplasm, it will activate caspase-3 and induce apoptosis through cascading amplification reaction (Zhou et al., 2015). Bcl-2 is an important anti-apoptotic molecule, which can reduce the permeability of mitochondrial membrane to cytochrome C and inhibit the release of cytochrome C from mitochondria into the cytoplasm (Karaliotas et al., 2015). From the literature it is known that FGF18 activates the PI3/Akt signaling pathway to inhibit IL-1β-induced caspase-3 activation and Bcl-2 expression, thus playing an anti-apoptotic effect (Li Z. et al., 2016; Koundouros and Poulogiannis, 2018; Yao et al., 2019) (Figure 2).
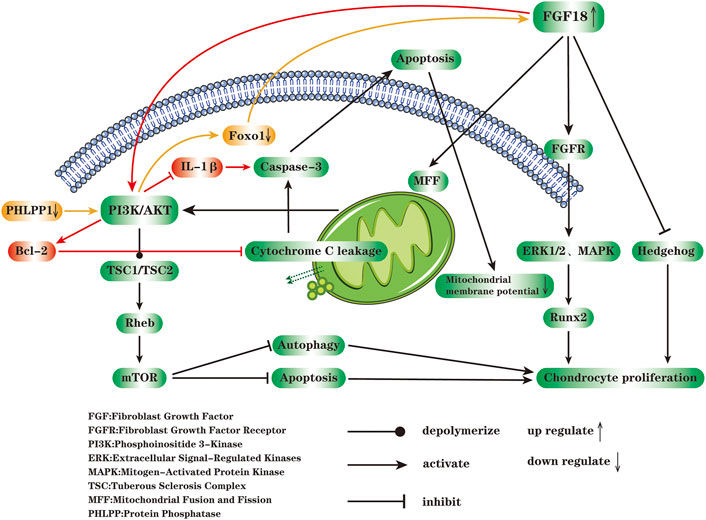
FIGURE 2. FGF18 acts on FGFR on the cell membrane and regulates chondrocyte proliferation through MAPK, ERK1/2 and hedgehog signaling pathways. The reduction of mitochondrial membrane potential and the leakage of cytochrome C into cytoplasm are closely related to cell apoptosis. FGF18 can maintain the balance of mitochondrial fusion and fission (MFF), restore the function and morphology of mitochondria, enhance the phosphorylation of PI3K and AKT, thereby depolymerizing TSC1/TSC2 and activating mTOR, ultimately inhibit apoptosis and autophagy of chondrocytes. After cytochrome C is released from mitochondria into the cytoplasm, it activates caspase-3 and causes apoptosis through a cascaded amplification reaction. FGF18 inhibits IL-1β-induced caspase3 activation through PI3K/AKT signaling pathway, enhances the expression of Bcl-2, and exerts an anti-apoptotic effect. Protein phosphatase1 (PHLPP1) is widely presented in chondrocytes during OA, and inhibits the proliferation of chondrocytes, differentiation, and matrix production. The decrease of PHLPP1 enhances the activity of Akt, and then down-regulates the transcription factor FoxO1 expression, followed by the increased expression of FGF18, and the enhanced phosphorylation of ERK1 or ERK2 through FGFR, and finally accelerates the proliferation of chondrocytes (The figure is made by adobe illustrator 2021).
Cartilage Homeostasis
Cartilage is mainly composed of chondrocytes and gelatinous ECM. The natural ECM consists of COL2, COL1, GAG, etc. (Peng et al., 2021). The unique structure and composition of the cartilage ECM are important factors in maintaining cartilage homeostasis, providing a smooth joint articulation and enabling articular cartilage to withstand loads several times the body weight (Bailey et al., 2021; Peng et al., 2021). COL2 is the most abundant component in the ECM of cartilage, where it forms a fibrous network structure; while GAG absorbs a large amount of water to form gel, and thus maintaining the dilatability and elasticity of cartilage (Sherwood et al., 2014). Cartilage lacks blood vessels and nerves, and its self-repair ability is limited. Chondrocytes are the singular cell type of cartilage which maintain and regulate the osmotic pressure of cartilage. Death, abnormal activation and differentiation of chondrocytes, increased degradation of ECM, and excessive generation of proteolytic enzymes or inflammatory mediators can destruct cartilage homeostasis and induce or aggravate OA (Sandya et al., 2007; Muntyanu et al., 2016; Rim et al., 2020).
The imbalance of cartilage homeostasis is mainly the result of cartilage degeneration and inflammation (Ritter et al., 2013; Redondo et al., 2018; Xie et al., 2021). During OA, the initial stage of cartilage degeneration is manifested as superficial cartilage defect or fibrosis, followed by the formation of ulcers and cracks, and gradually expands to subchondral bone, making the cartilage thinner and thinner, leading to full-thickness cartilage defect (McCulloch et al., 2019; Chen et al., 2020). As the above events worsen, it can lead to subchondral bone exposure and intensified degeneration. Chondrodegeneration is an important event in the early stage of OA, and the articular inflammatory environment caused by degradation and destruction of cartilage in the middle and late stage ultimately lead to synovial hyperplasia and angiogenesis (Yu et al., 2016). Inflammatory factors produced in the pathological process directly act on chondrocytes and ECM, which can further destroy the cartilage homeostasis. Mechanical stress and acute injury up-regulate the gene expression of inflammatory factors IL-1, IL-6 and TNFα, thereby promoting the expression of ADAMTs, MMP-3, MMP-9 and MMP-13, leading to the degradation of COL2A1 and GAG (Yang et al., 2017). Proteolytic enzymes are involved in the synthesis, recombination, and repair of connective tissue. The external or internal injury, genetic abnormalities and irregular mechanical loading of cartilage could result in the imbalance of metabolic activity by overly enhancing the activity of proteolytic enzymes, and ultimately accelerate cartilage degradation (Choi et al., 2019). IL-1 has a strong ability to induce aggrecanolysis and up-regulate the synthesis of chondrodegrading enzymes, such as MMP-3, ADAMTS-4, and ADAMTS-5 (Kapoor et al., 2011; Na et al., 2020). IL-6 can efficiently reduce proteoglycan synthesis in normal cartilage in vitro (Wang W. et al., 2020). TNFα induces the synthesis of MMPs and other proteases in chondrocytes, meanwhile increases prostaglandin E2 (PGE2) level by stimulating the synthesis of cyclooxygenase-2, microsomal PGE synthase-1 and soluble phospholipase A2 (Fitzgerald et al., 2004). The cartilage degeneration and inflammatory microenvironment of cartilage can activate each other and jointly aggravate the destruction of cartilage homeostasis.
Research Progress of Sprifermin in the Treatment of Cartilage Related Diseases
OA is a heterogeneous disease involving the whole joint, and cartilage degeneration is the main feature (Shah and Mithoefer, 2020a). Under the microscope, articular cartilage damage can be assessed by analysis of tissue sections. OA cartilage can be characterized by the appearance of clusters of chondrocytes near the superficial layer, chondrocyte apoptosis in the deep and calcified layer, and disruption of ECM due to the degradation of collagen and proteoglycan. Although the proliferative activity of chondrocytes is also activated, the catabolic activity is much higher than the proliferative activity (Sandell and Aigner, 2001; Carlo and Loeser, 2008). As the disease progresses, the degrading enzymes produced by articular chondrocytes gradually increase, further aggravating the destruction of articular biomechanics and biochemistry (Dreier, 2010) (Figure 3).
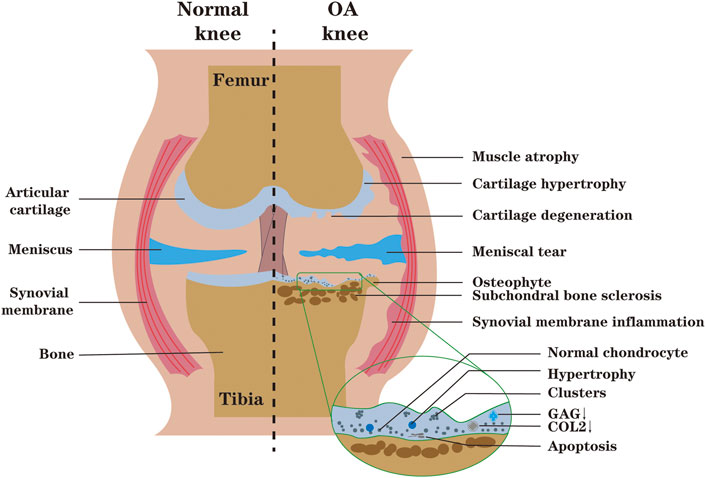
FIGURE 3. During OA, the synthesis of ECM (GAG, COL2) decreases, and cartilage degeneration occurs. Microscopically, the spatial arrangement of chondrocytes is changed from single to double strings, then to clusters. With the development of OA, chondrocytes undergo abnormal activation and even death, which intensifies the degradation and destruction of cartilage. The inflammatory environment of the joint may lead to synovial proliferation, biochemical and biomechanical changes in the articular cartilage, compensatory osteophyte formation, muscle atrophy and meniscus tears of the knee (The figure is made by adobe illustrator 2021).
A first-in-human study showed that intraarticular injection of Sprifermin efficiently promotes chondrocytes proliferation and has a positive effect on histological and biomechanical cartilage properties (Dahlberg et al., 2016). Quantitative measurement and ultrastructural analysis indicated that treatment with Sprifermin increases the synthesis of GAG and COL2 and promotes the formation of ECM connections across the cartilage, which helps damaged cartilage to complete the repair process (Gigout et al., 2017). In the in vitro repair model, mechanical and biochemical analysis displayed that the adhesion strength between cartilage surfaces is stronger and the contact area between core cartilage and cartilage rings is larger in the Sprifermin treatment group, indicating that Sprifermin effectively promotes the healing of articular cartilage defects and the integration between lateral cartilage (Sennett et al., 2018). In a sheep model, Power and colleagues compared the efficacy between Microfracture (MFX) and MFX with rhFGF18 in the treatment of articular cartilage defects, and they observed significant statistical differences in International Cartilage Repair Society (ICRS) tissue repair scores, tissue fill scores, and improved O'Driscoll scores at 6 months between the two groups. MFX combined with rhFGF18 promotes the formation of hyaline chondroid tissue compared to the MFX group (Power et al., 2014). Sennett et al. also reported that, compared with the control group, the Sprifermin treatment group has stronger cartilage-to-cartilage interface adhesion strength, more COL2 content, and larger contact area between core cartilage and annular cartilage, which indicates that Sprifermin can effectively promote the repair of damaged cartilage (Sennett et al., 2018).
The current literature shows that, compared with placebo treatment, administration of Sprifermin not only increases cartilage thickness in some locations of the joint (adds cartilage volume and thickness globally, or at some regions where cartilage thickness is expected to be static), but also notably reduces cartilage loss (Lohmander et al., 2014; Eckstein et al., 2015). Similarly, following Sprifermin treatment, the percentage of cartilage thickness of medial central tibia, lateral central tibia and medial central femoral condylar has the largest changes, indicating a strong sensitivity to Sprifermin, and the most obvious difference between Sprifermin and placebo group is in medial central tibia (Hochberg et al., 2019). This indicates that the high bearing area of the joint and the area where cartilage is prone to damage are more sensitive to Sprifermin (Hochberg et al., 2018; Eckstein et al., 2020). Roemer et al. confirmed that Sprifermin exerts a positive effect on cartilage morphology and improvement of bone marrow-induced lesions (BML), and no negative effects associated with Sprifermin are reported in other joint tissues (Roemer et al., 2016).
Sprifermin can specifically target FGFR3 of chondrocytes. Since FGFR3 is expressed in meniscus, Sprifermin is expected to achieve a therapeutical effect when meniscus is injured (Roemer et al., 2020). Studies by Lohmander et al. showed that after intra-articular injection of Sprifermin, cartilage thickness significantly increases in a dose-dependent manner (Lohmander et al., 2014). Gigout and colleagues confirmed that in monolayer culture of porcine chondrocytes and 3D culture of human and porcine chondrocytes, Sprifermin could promote chondrocyte proliferation dose-dependently (Gigout et al., 2017). (Lohmander et al., 2014). Intra-articular injection of FGF18 can activate FGFR3C, alleviate the cartilage degradation of rat post-traumatic osteoarthritis (PTOA), increase the deposition of COL2, and inhibit the expression of MMP-13 (Karuppaiah et al., 2016; Onuora, 2021). When OA occurs, the expression of FGFR3 in chondrocytes is down-regulated, usually accompanied by increased FGFR1 level, while FGF18 can up-regulate the expression of FGFR3 and down-regulate FGFR1 level in chondrocytes (Yao et al., 2019). In addition, FGF18 expression and FGFR3 activation in growth plate chondrocytes inhibit proliferation and hypertrophy of chondrocytes (Karuppaiah et al., 2016). Ohbayashi et al. reported that FGF18 is required for both osteogenesis and chondrogenesis in bone development, it promotes both proliferation of osteogenic mesenchymal cells and terminal differentiation to mature osteoblasts (Ohbayashi et al., 2002).
There are many studies which focused on the anti-OA effect of FGF18 in vivo. Power et al. found that, through animal experiments, microfracture surgery combined with intraarticular injection of rhFGF18 effectively can promote the repair of articular cartilage defects of the medial femoral condyle (Power et al., 2014). Moore et al. also demonstrated that intraarticular injection of FGF18 stimulates cartilage repair in a rat meniscus tear model, giving rise to a dose-dependent increase in cartilage thickness and a significant reduction in cartilage degeneration scores (Moore et al., 2005). Similarly, in another OA rat model, administration of rhFGF18 could prevent cartilage degeneration (Mori et al., 2014). RhFGF18 is expected to protect articular cartilage from injury. In a five-year phase II clinical trial, Eckstein et al. have proved that intra-articular injection of Sprifermin can promote knee cartilage regeneration in OA patients. Sprifermin is the first DMOAD candidate drug that can promote the regeneration of damaged articular cartilage (Hochberg et al., 2019; Eckstein et al., 2020; Eckstein et al., 2021). Initially, Hochberg et al. reported that intra-articular injection of Sprifermin efficiently increases the cartilage thickness of femorotibial joint, compared with other doses and frequencies, the intra-articular administration of 100 μg every 6 months is most effective (Hochberg et al., 2019). Through a large sample study, they further confirmed that Sprifermin increases the thickness of the cartilage in all parts of the femorotibial joint in OA patients, and the effect is more obvious in high load-bearing areas such as the central medial tibia (Eckstein et al., 2020). Based on above, Eckstein et al. further evaluated the effectiveness and safety of Sprifermin in patients with OA. Following treatment with Sprifermin, the WOMAC pain score improved by approximately 50% in all groups with different doses and frequencies. Sprifermin treatment can maintain long-term structural modification of articular cartilage, hereby they confirmed that Sprifermin can not only modify the structure of articular cartilage, but also effectively alleviate clinical symptoms (Eckstein et al., 2021).
The promotion effect of Sprifermin on chondrogenesis depends on the inflammatory environment to some extent. Under inflammatory state, the regulative effect of Sprifermin on articular cartilage anabolism is obviously attenuated (Reker et al., 2017). For instance, when explants are co-cultured with pro-inflammatory cytokines prior to Sprifermin, almost no changes in COL2 synthesis and ECM degradation markers are observed following Sprifermin treatment. Other studies have confirmed that continuous co-culture of oncostatin M + TNFα almost eliminates Sprifermin-stimulated COL2 synthesis during the whole culture period (Reker et al., 2017). In clinical work, matching the appropriate treatment method with the corresponding patient is very important for the effective treatment of OA, due to that the cartilage regeneration effect of Sprifermin may be largely affected by the inflammation of the joint cavity. In view of the above studies, Sprifermin may be a more effective DMOAD for OA patients with mild synovitis or low levels of pro-inflammatory factors. Sprifermin injection in the knee cavity in patients with early OA (Kellgren-Lawrence grade 1 or 2) combined with other oral agents can delay or even reverse the progression of OA. For severe OA patients (Kellgren-Lawrence grade 3 or 4), Sprifermin is expected to delay the time to total knee replacement (Table 3).
Limitation
At present, most of the experiments on Sprifermin are still in the animal stage, and the application of Sprifermin in human body is rare. The effect of Sprifermin may be different between human and animal cartilage. Although Sprifermin can promote cartilage regeneration and increase cartilage thickness, it remains unclear whether cartilage can regenerate according to the natural cartilage structure. Additionally, the dose and method of administration of Sprifermin are still controversial. Due to the limited volume of articular cavity, there is no consensus on how to achieve the maximum therapeutic effect with the minimum dose of Sprifermin. For patients with symptomatic knee OA, the follow up time after intraarticular injection of Sprifermin is yet not long enough, and its clinical effectiveness remains uncertain. The long-term effect of Sprifermin in clinical patients requires longer follow-up time and more clinical samples, and more clinical trials are needed to prove that articular cartilage structural modification in OA patients can be translated into symptomatic benefit.
Conclusion
Thus far, there is no satisfactory drug or method for the treatment of OA. Sprifermin is a promising drug of DMOAD, displaying unique advantage in maintaining cartilage homeostasis. Sprifermin can effectively promote chondrocyte proliferation and ECM synthesis, maintain chondrocyte phenotype. Following Sprifermin treatment, the enlarged chondrocyte population produce transparent ECM, and there is a biphasic ECM remodeling process, which remarkably amplifies the efficiency of cartilage regeneration. The functions of Sprifermin might be closely related to its anti-inflammatory and maintenance of mitochondrial performance. Clinical trials have confirmed that intermittent injections of Sprifermin in the knee joint once a week can maximize the effect of anti-OA. Therefore, Sprifermin is expected to become a safe and effective drug for delaying or even reversing cartilage damage-related diseases. Although some progress has been made, the specific mechanism of its action is still unclear. Before Sprifermin can be used on a large scale in clinical treatment of cartilage injury-related diseases, its long-term safety and effectiveness still need to be evaluated by further studies.
Author Contributions
ZS, SC, and HL designed the Review. ZS, YL, CS, JL, and SC contributed to writing of the manuscript. GS and HK participated in revising the manuscript. Finally, all authors have reviewed and approved the final submitted manuscript. The integrity of this work is guaranteed by SC and HL.
Funding
We received support from the National Natural Science Foundation of China (Grant Nos. 82172484, 81902253).
Conflict of Interest
The authors declare that the research was conducted in the absence of any commercial or financial relationships that could be construed as a potential conflict of interest.
Publisher’s Note
All claims expressed in this article are solely those of the authors and do not necessarily represent those of their affiliated organizations, or those of the publisher, the editors and the reviewers. Any product that may be evaluated in this article, or claim that may be made by its manufacturer, is not guaranteed or endorsed by the publisher.
References
Alexander, L. A. M., Ln, D., Eg, Z., Is, D., Ay, K., Ss, R., et al. (2020). Pharmacological Management of Osteoarthritis with a Focus on Symptomatic Slow-Acting Drugs. J. Clin. Rheumatol. 27, e533–e539. doi:10.1097/rhu.0000000000001507
Antunes, B. P., Vainieri, M. L., Alini, M., Monsonego-Ornan, E., Grad, S., and Yayon, A. (2020). Enhanced Chondrogenic Phenotype of Primary Bovine Articular Chondrocytes in Fibrin-Hyaluronan Hydrogel by Multi-Axial Mechanical Loading and FGF18. Acta Biomater. 105, 170–179. doi:10.1016/j.actbio.2020.01.032
Bailey, K. N., Nguyen, J., Yee, C. S., Dole, N. S., Dang, A., Alliston, T., et al. (2021). Mechanosensitive Control of Articular Cartilage and Subchondral Bone Homeostasis in Mice Requires Osteocytic Transforming Growth Factor β Signaling. Arthritis Rheumatol. 73, 414–425. doi:10.1002/art.41548
Barnard, J. C., Williams, A. J., Rabier, B., Chassande, O., Samarut, J., Cheng, S.-y., et al. (2005). Thyroid Hormones Regulate Fibroblast Growth Factor Receptor Signaling during Chondrogenesis. Endocrinology 146, 5568–5580. doi:10.1210/en.2005-0762
Boylan, M., Anderson, M. J., Ornitz, D. M., and Lewandoski, M. (2020). The Fgf8 Subfamily (Fgf8, Fgf17 and Fgf18) Is Required for Closure of the Embryonic Ventral Body wall. Development 147, dev189506. doi:10.1242/dev.189506
Brody, L. T. (2015). Knee Osteoarthritis: Clinical Connections to Articular Cartilage Structure and Function. Phys. Ther. Sport 16, 301–316. doi:10.1016/j.ptsp.2014.12.001
Bükülmez, H., Khan, F., Bartels, C. F., Murakami, S., Ortiz‐Lopez, A., Sattar, A., et al. (2014). Protective Effects of C‐Type Natriuretic Peptide on Linear Growth and Articular Cartilage Integrity in a Mouse Model of Inflammatory Arthritis. Arthritis Rheumatol. 66, 78–89. doi:10.1002/art.38199
Carlo, M. D., and Loeser, R. F. (2008). Cell Death in Osteoarthritis. Curr. Rheumatol. Rep. 10, 37–42. doi:10.1007/s11926-008-0007-8
Catheline, S. E., Hoak, D., Chang, M., Ketz, J. P., Hilton, M. J., Zuscik, M. J., et al. (2019). Chondrocyte‐Specific RUNX2 Overexpression Accelerates Post‐traumatic Osteoarthritis Progression in Adult Mice. J. Bone Miner Res. 34, 1676–1689. doi:10.1002/jbmr.3737
Chen, L., Zheng, J. J. Y., Li, G., Yuan, J., Ebert, J. R., Li, H., et al. (2020). Pathogenesis and Clinical Management of Obesity-Related Knee Osteoarthritis: Impact of Mechanical Loading. J. Orthopaedic Translation 24, 66–75. doi:10.1016/j.jot.2020.05.001
Choi, M., Jo, J., Park, J., Kang, H. K., and Park, Y. (2019). NF-B Signaling Pathways in Osteoarthritic Cartilage Destruction. Cells 8, 734. doi:10.3390/cells8070734
Clarke, J. (2020). Opioid and Hedgehog Signalling Pathways Converge to Modulate OA. Nat. Rev. Rheumatol. 16, 297. doi:10.1038/s41584-020-0429-x
Dahlberg, L. E., Aydemir, A., Muurahainen, N., Gühring, H., Fredberg Edebo, H., Krarup-Jensen, N., et al. (2016). A First-In-Human, Double-Blind, Randomised, Placebo-Controlled, Dose Ascending Study of Intra-articular rhFGF18 (Sprifermin) in Patients with Advanced Knee Osteoarthritis. Clin. Exp. Rheumatol. 34, 445–450.
Davidson, D., Blanc, A., Filion, D., Wang, H., Plut, P., Pfeffer, G., et al. (2005). Fibroblast Growth Factor (FGF) 18 Signals through FGF Receptor 3 to Promote Chondrogenesis. J. Biol. Chem. 280, 20509–20515. doi:10.1074/jbc.M410148200
Davies, L. C., Blain, E. J., Gilbert, S. J., Caterson, B., and Duance, V. C. (2008). The Potential of IGF-1 and TGFβ1 for Promoting "Adult" Articular Cartilage Repair: AnIn VitroStudy. Tissue Eng. A 14, 1251–1261. doi:10.1089/ten.tea.2007.0211
Deng, M., Hu, Y., Zhang, Z., Zhang, H., Qu, Y., Shao, G., et al. (2021). Unicondylar Knee Replacement versus Total Knee Replacement for the Treatment of Medial Knee Osteoarthritis: a Systematic Review and Meta-Analysis. Arch. Orthop. Trauma Surg. 141, 1361–1372. doi:10.1007/s00402-021-03790-7
Dreier, R. (2010). Hypertrophic Differentiation of Chondrocytes in Osteoarthritis: the Developmental Aspect of Degenerative Joint Disorders. Arthritis Res. Ther. 12, 216. doi:10.1186/ar3117
Eckstein, F., Hochberg, M. C., Guehring, H., Moreau, F., Ona, V., Bihlet, A. R., et al. (2021). Long-term Structural and Symptomatic Effects of Intra-articular Sprifermin in Patients with Knee Osteoarthritis: 5-year Results from the FORWARD Study. Ann. Rheum. Dis. 80, 1062–1069. doi:10.1136/annrheumdis-2020-219181
Eckstein, F., Kraines, J. L., Aydemir, A., Wirth, W., Maschek, S., and Hochberg, M. C. (2020). Intra-articular Sprifermin Reduces Cartilage Loss in Addition to Increasing Cartilage Gain Independent of Location in the Femorotibial Joint: post-hoc Analysis of a Randomised, Placebo-Controlled Phase II Clinical Trial. Ann. Rheum. Dis. 79, 525–528. doi:10.1136/annrheumdis-2019-216453
Eckstein, F., Wirth, W., Guermazi, A., Maschek, S., and Aydemir, A. (2015). Brief Report: Intraarticular Sprifermin Not Only Increases Cartilage Thickness, but Also Reduces Cartilage Loss: Location‐Independent Post Hoc Analysis Using Magnetic Resonance Imaging. Arthritis Rheumatol. 67, 2916–2922. doi:10.1002/art.39265
Eisner, V., Picard, M., and Hajnóczky, G. (2018). Mitochondrial Dynamics in Adaptive and Maladaptive Cellular Stress Responses. Nat. Cel Biol 20, 755–765. doi:10.1038/s41556-018-0133-0
Ellsworth, J. L., Berry, J., Bukowski, T., Claus, J., Feldhaus, A., Holderman, S., et al. (2002). Fibroblast Growth Factor-18 Is a Trophic Factor for Mature Chondrocytes and Their Progenitors. Osteoarthritis and Cartilage 10, 308–320. doi:10.1053/joca.2002.0514
Ernstbrunner, L., Imam, M. A., Andronic, O., Perz, T., Wieser, K., and Fucentese, S. F. (2018). Lateral Unicompartmental Knee Replacement: a Systematic Review of Reasons for Failure. Int. Orthopaedics (Sicot) 42, 1827–1833. doi:10.1007/s00264-017-3662-4
Fitzgerald, J. B., Jin, M., Dean, D., Wood, D. J., Zheng, M. H., and Grodzinsky, A. J. (2004). Mechanical Compression of Cartilage Explants Induces Multiple Time-dependent Gene Expression Patterns and Involves Intracellular Calcium and Cyclic AMP. J. Biol. Chem. 279, 19502–19511. doi:10.1074/jbc.M400437200
Gigout, A., Guehring, H., Froemel, D., Meurer, A., Ladel, C., Reker, D., et al. (2017). Sprifermin (rhFGF18) Enables Proliferation of Chondrocytes Producing a Hyaline Cartilage Matrix. Osteoarthritis and Cartilage 25, 1858–1867. doi:10.1016/j.joca.2017.08.004
Harasymowicz, N. S., Choi, Y. R., Wu, C. L., Iannucci, L., Tang, R., Guilak, F., et al. (2020). Intergenerational Transmission of Diet‐Induced Obesity, Metabolic Imbalance, and Osteoarthritis in Mice. Arthritis Rheumatol. 72, 632–644. doi:10.1002/art.41147
Hochberg, M. C., Altman, R. D., April, K. T., Benkhalti, M., Guyatt, G., McGowan, J., et al. (2012). American College of Rheumatology 2012 Recommendations for the Use of Nonpharmacologic and Pharmacologic Therapies in Osteoarthritis of the Hand, Hip, and Knee. Arthritis Care Res. 64, 465–474. doi:10.1002/acr.21596
Hochberg, M. C., Guermazi, A., Guehring, H., Aydemir, A., Wax, S., Fleuranceau-Morel, P., et al. (2019). Effect of Intra-articular Sprifermin vs Placebo on Femorotibial Joint Cartilage Thickness in Patients with Osteoarthritis. JAMA 322, 1360–1370. doi:10.1001/jama.2019.14735
Hochberg, M., Guermazi, A., Guehring, H., Aydemir, A., Wax, S., Fleuranceau-Morel, P., et al. (2018). Efficacy and Safety of Intra-articular Sprifermin in Symptomatic Radiographic Knee Osteoarthritis: Pre-specified Analysis of 3-year Data from a 5-year Randomized, Placebo-Controlled, Phase II Study. Osteoarthritis and Cartilage 26, S26–S27. doi:10.1016/j.joca.2018.02.069
Hu, M. C.-T., Qiu, W. R., Wang, Y.-p., Hill, D., Ring, B. D., Scully, S., et al. (1998). FGF-18, a Novel Member of the Fibroblast Growth Factor Family, Stimulates Hepatic and Intestinal Proliferation. Mol. Cel Biol 18, 6063–6074. doi:10.1128/mcb.18.10.6063
Hung, I. H., Schoenwolf, G. C., Lewandoski, M., and Ornitz, D. M. (2016). A Combined Series of Fgf9 and Fgf18 Mutant Alleles Identifies Unique and Redundant Roles in Skeletal Development. Develop. Biol. 411, 72–84. doi:10.1016/j.ydbio.2016.01.008
Hunter, D. J., Pike, M. C., Jonas, B. L., Kissin, E., Krop, J., and McAlindon, T. (2010). Phase 1 Safety and Tolerability Study of BMP-7 in Symptomatic Knee Osteoarthritis. BMC Musculoskelet. Disord. 11, 232. doi:10.1186/1471-2474-11-232
Hwang, H., and Kim, H. (2015). Chondrocyte Apoptosis in the Pathogenesis of Osteoarthritis. Ijms 16, 26035–26054. doi:10.3390/ijms161125943
Jeon, E., Yun, Y.-R., Kang, W., Lee, S., Koh, Y.-H., Kim, H.-W., et al. (2012). Investigating the Role of FGF18 in the Cultivation and Osteogenic Differentiation of Mesenchymal Stem Cells. PLoS One 7, e43982. doi:10.1371/journal.pone.0043982
Kapoor, M., Martel-Pelletier, J., Lajeunesse, D., Pelletier, J.-P., and Fahmi, H. (2011). Role of Proinflammatory Cytokines in the Pathophysiology of Osteoarthritis. Nat. Rev. Rheumatol. 7, 33–42. doi:10.1038/nrrheum.2010.196
Karaliotas, G. I., Mavridis, K., Scorilas, A., and Babis, G. C. (2015). Quantitative Analysis of the mRNA Expression Levels of BCL2 and BAX Genes in Human Osteoarthritis and normal Articular Cartilage: An Investigation into Their Differential Expression. Mol. Med. Rep. 12, 4514–4521. doi:10.3892/mmr.2015.3939
Karsdal, M. A., Michaelis, M., Ladel, C., Siebuhr, A. S., Bihlet, A. R., Andersen, J. R., et al. (2016). Disease-modifying Treatments for Osteoarthritis (DMOADs) of the Knee and Hip: Lessons Learned from Failures and Opportunities for the Future. Osteoarthritis and Cartilage 24, 2013–2021. doi:10.1016/j.joca.2016.07.017
Karuppaiah, K., Yu, K., Lim, J., Chen, J., Smith, C., Long, F., et al. (2016). FGF Signaling in the Osteoprogenitor Lineage Non-autonomously Regulates Postnatal Chondrocyte Proliferation and Skeletal Growth. Development 143, 1811–1822. doi:10.1242/dev.131722
Katz, J. N., Arant, K. R., and Loeser, R. F. (2021). Diagnosis and Treatment of Hip and Knee Osteoarthritis. JAMA 325, 568–578. doi:10.1001/jama.2020.22171
Khosravi, F., Ahmadvand, N., Bellusci, S., and Sauer, H. (2021). The Multifunctional Contribution of FGF Signaling to Cardiac Development, Homeostasis, Disease and Repair. Front. Cel Dev. Biol. 9, 672935. doi:10.3389/fcell.2021.672935
Koundouros, N., and Poulogiannis, G. (2018). Phosphoinositide 3-Kinase/Akt Signaling and Redox Metabolism in Cancer. Front. Oncol. 8, 160. doi:10.3389/fonc.2018.00160
Krishnan, Y., and Grodzinsky, A. J. (2018). Cartilage Diseases. Matrix Biol. 71-72, 51–69. doi:10.1016/j.matbio.2018.05.005
Li, J., Wang, X., Ruan, G., Zhu, Z., and Ding, C. (2021). Sprifermin: a Recombinant Human Fibroblast Growth Factor 18 for the Treatment of Knee Osteoarthritis. Expert Opin. Investig. Drugs 30, 923–930. doi:10.1080/13543784.2021.1972970
Li, Y.-S., Zhang, F.-J., Zeng, C., Luo, W., Xiao, W.-F., Gao, S.-G., et al. (2016a). Autophagy in Osteoarthritis. Jt. Bone Spine 83, 143–148. doi:10.1016/j.jbspin.2015.06.009
Li, Z., Zhu, T., and Fan, W. (2016b). Osteochondral Autograft Transplantation or Autologous Chondrocyte Implantation for Large Cartilage Defects of the Knee: a Meta-Analysis. Cell Tissue Bank 17, 59–67. doi:10.1007/s10561-015-9515-8
Liu, G., Chen, T., Ding, Z., Wang, Y., Wei, Y., and Wei, X. (2021). Inhibition of FGF‐FGFR and VEGF‐VEGFR Signalling in Cancer Treatment. Cell Prolif 54, e13009. doi:10.1111/cpr.13009
Lohmander, L. S., Hellot, S., Dreher, D., Krantz, E. F. W., Kruger, D. S., Guermazi, A., et al. (2014). Intraarticular Sprifermin (Recombinant Human Fibroblast Growth Factor 18) in Knee Osteoarthritis: a Randomized, Double-Blind, Placebo-Controlled Trial. Arthritis Rheumatol. 66, 1820–1831. doi:10.1002/art.38614
Lv, X., Sun, C., Hu, B., Chen, S., Wang, Z., Wu, Q., et al. (2020). Simultaneous Recruitment of Stem Cells and Chondrocytes Induced by a Functionalized Self-Assembling Peptide Hydrogel Improves Endogenous Cartilage Regeneration. Front. Cel Dev. Biol. 8, 864. doi:10.3389/fcell.2020.00864
Malemud, C. J. (2019). Inhibition of MMPs and ADAM/ADAMTS. Biochem. Pharmacol. 165, 33–40. doi:10.1016/j.bcp.2019.02.033
Martínez-Moreno, D., Jiménez, G., Gálvez-Martín, P., Rus, G., and Marchal, J. A. (2019). Cartilage Biomechanics: A Key Factor for Osteoarthritis Regenerative Medicine. Biochim. Biophys. Acta (Bba) - Mol. Basis Dis. 1865, 1067–1075. doi:10.1016/j.bbadis.2019.03.011
Maruoka, Y., Ohbayashi, N., Hoshikawa, M., Itoh, N., Hogan, B. L. M., and Furuta, Y. (1998). Comparison of the Expression of Three Highly Related Genes, Fgf8, Fgf17 and Fgf18, in the Mouse Embryo. Mech. Develop. 74, 175–177. doi:10.1016/s0925-4773(98)00061-6
McCulloch, K., Huesa, C., Dunning, L., Litherland, G. J., Van ‘T Hof, R. J., Lockhart, J. C., et al. (2019). Accelerated post Traumatic Osteoarthritis in a Dual Injury Murine Model. Osteoarthritis and Cartilage 27, 1800–1810. doi:10.1016/j.joca.2019.05.027
Mehana, E.-S. E., Khafaga, A. F., and El-Blehi, S. S. (2019). The Role of Matrix Metalloproteinases in Osteoarthritis Pathogenesis: An Updated Review. Life Sci. 234, 116786. doi:10.1016/j.lfs.2019.116786
Moore, E. E., Bendele, A. M., Thompson, D. L., Littau, A., Waggie, K. S., Reardon, B., et al. (2005). Fibroblast Growth Factor-18 Stimulates Chondrogenesis and Cartilage Repair in a Rat Model of Injury-Induced Osteoarthritis. Osteoarthritis and Cartilage 13, 623–631. doi:10.1016/j.joca.2005.03.003
Mori, Y., Saito, T., Chang, S. H., Kobayashi, H., Ladel, C. H., Guehring, H., et al. (2014). Identification of Fibroblast Growth Factor-18 as a Molecule to Protect Adult Articular Cartilage by Gene Expression Profiling. J. Biol. Chem. 289, 10192–10200. doi:10.1074/jbc.M113.524090
Müller, S., Lindemann, S., and Gigout, A. (2020). Effects of Sprifermin, IGF1, IGF2, BMP7, or CNP on Bovine Chondrocytes in Monolayer and 3D Culture. J. Orthop. Res. 38, 653–662. doi:10.1002/jor.24491
Muntyanu, A., Abji, F., Liang, K., Pollock, R. A., Chandran, V., Gladman, D. D., et al. (2016). Differential Gene and Protein Expression of Chemokines and Cytokines in Synovial Fluid of Patients with Arthritis. Arthritis Res. Ther. 18, 296. doi:10.1186/s13075-016-1196-6
Na, H. S., Park, J.-S., Cho, K.-H., Kwon, J. Y., Choi, J., Jhun, J., et al. (2020). Interleukin-1-Interleukin-17 Signaling Axis Induces Cartilage Destruction and Promotes Experimental Osteoarthritis. Front. Immunol. 11, 730. doi:10.3389/fimmu.2020.00730
Ohbayashi, N., Shibayama, M., Kurotaki, Y., Imanishi, M., Fujimori, T., Itoh, N., et al. (2002). FGF18 Is Required for normal Cell Proliferation and Differentiation during Osteogenesis and Chondrogenesis. Genes Dev. 16, 870–879. doi:10.1101/gad.965702
Oliveira, M. C., Vullings, J., and van de Loo, F. A. J. (2020). Osteoporosis and Osteoarthritis Are Two Sides of the Same coin Paid for Obesity. Nutrition 70, 110486. doi:10.1016/j.nut.2019.04.001
Onuora, S. (2021). Sprifermin Benefits Maintained at 5 Years. Nat. Rev. Rheumatol. 17, 378. doi:10.1038/s41584-021-00643-w
Park, Y.-C., Goo, B.-H., Park, K.-J., Kim, J.-Y., and Baek, Y.-H. (2021). Traditional Korean Medicine as Collaborating Treatments with Conventional Treatments for Knee Osteoarthritis: A Protocol for a Systematic Review and Meta-Analysis. Jpr Vol. 14, 1345–1351. doi:10.2147/jpr.S311557
Peng, Z., Sun, H., Bunpetch, V., Koh, Y., Wen, Y., Wu, D., et al. (2021). The Regulation of Cartilage Extracellular Matrix Homeostasis in Joint Cartilage Degeneration and Regeneration. Biomaterials 268, 120555. doi:10.1016/j.biomaterials.2020.120555
Power, J., Hernandez, P., Guehring, H., Getgood, A., and Henson, F. (2014). Intra-articular Injection of rhFGF-18 Improves the Healing in Microfracture Treated Chondral Defects in an Ovine Model. J. Orthop. Res. 32, 669–676. doi:10.1002/jor.22580
Pu, P., Qingyuan, M., Weishan, W., Fei, H., Tengyang, M., Weiping, Z., et al. (2021). Protein-Degrading Enzymes in Osteoarthritis. Z. Orthop. Unfall 159, 54–66. doi:10.1055/a-1019-8117
Quinn, R. H., Murray, J. N., Pezold, R., and Sevarino, K. S. (2018). Surgical Management of Osteoarthritis of the Knee. J. Am. Acad. Orthopaedic Surgeons 26, e191–e193. doi:10.5435/jaaos-d-17-00424
Redondo, M., Beer, A., and Yanke, A. (2018). Cartilage Restoration: Microfracture and Osteochondral Autograft Transplantation. J. Knee Surg. 31, 231–238. doi:10.1055/s-0037-1618592
Reker, D., Kjelgaard-Petersen, C. F., Siebuhr, A. S., Michaelis, M., Gigout, A., Karsdal, M. A., et al. (2017). Sprifermin (rhFGF18) Modulates Extracellular Matrix Turnover in Cartilage Explants Ex Vivo. J. Transl Med. 15, 250. doi:10.1186/s12967-017-1356-8
Reker, D., Siebuhr, A. S., Thudium, C. S., Gantzel, T., Ladel, C., Michaelis, M., et al. (2020). Sprifermin (rhFGF18) versus Vehicle Induces a Biphasic Process of Extracellular Matrix Remodeling in Human Knee OA Articular Cartilage Ex Vivo. Sci. Rep. 10, 6011. doi:10.1038/s41598-020-63216-z
Rim, Y. A., Nam, Y., and Ju, J. H. (2020). The Role of Chondrocyte Hypertrophy and Senescence in Osteoarthritis Initiation and Progression. Ijms 21, 2358. doi:10.3390/ijms21072358
Ritter, S. Y., Subbaiah, R., Bebek, G., Crish, J., Scanzello, C. R., Krastins, B., et al. (2013). Proteomic Analysis of Synovial Fluid from the Osteoarthritic Knee: Comparison with Transcriptome Analyses of Joint Tissues. Arthritis Rheum. 65, 981–992. doi:10.1002/art.37823
Roemer, F. W., Aydemir, A., Lohmander, S., Crema, M. D., Marra, M. D., Muurahainen, N., et al. (2016). Structural Effects of Sprifermin in Knee Osteoarthritis: a post-hoc Analysis on Cartilage and Non-cartilaginous Tissue Alterations in a Randomized Controlled Trial. BMC Musculoskelet. Disord. 17, 267. doi:10.1186/s12891-016-1128-2
Roemer, F. W., Kraines, J., Aydemir, A., Wax, S., Hochberg, M. C., Crema, M. D., et al. (2020). Evaluating the Structural Effects of Intra-articular Sprifermin on Cartilage and Non-cartilaginous Tissue Alterations, Based on sqMRI Assessment over 2 Years. Osteoarthritis and Cartilage 28, 1229–1234. doi:10.1016/j.joca.2020.05.015
Rozenblatt-Rosen, O., Mosonego-Ornan, E., Sadot, E., Madar-Shapiro, L., Sheinin, Y., Ginsberg, D., et al. (2002). Induction of Chondrocyte Growth Arrest by FGF: Transcriptional and Cytoskeletal Alterations. J. Cel Sci 115, 553–562. doi:10.1242/jcs.115.3.553
Sandell, L. J., and Aigner, T. (2001). Articular Cartilage and Changes in Arthritis: Cell Biology of Osteoarthritis. Arthritis Res. Ther. 3, 107–113. doi:10.1186/ar148
Sandya, S., Achan, M. A., and Sudhakaran, P. R. (2007). Parallel Changes in Fibronectin and Alpha5beta1 Integrin in Articular Cartilage in Type II Collagen-Induced Arthritis. Indian J. Biochem. Biophys. 44, 14–18.
Sennett, M. L., Meloni, G. R., Farran, A. J. E., Guehring, H., Mauck, R. L., and Dodge, G. R. (2018). Sprifermin Treatment Enhances Cartilage Integration in an In Vitro Repair Model. J. Orthop. Res. 36, 2648–2656. doi:10.1002/jor.24048
Shah, S. S., and Mithoefer, K. (2020b). Current Applications of Growth Factors for Knee Cartilage Repair and Osteoarthritis Treatment. Curr. Rev. Musculoskelet. Med. 13, 641–650. doi:10.1007/s12178-020-09664-6
Shah, S. S., and Mithoefer, K. (2020a). Scientific Developments and Clinical Applications Utilizing Chondrons and Chondrocytes with Matrix for Cartilage Repair. Cartilage 6, 194760352096888. doi:10.1177/1947603520968884
Shen, L., Zhou, L., Xia, M., Lin, N., Ma, J., Dong, D., et al. (2021). PGC1α Regulates Mitochondrial Oxidative Phosphorylation Involved in Cisplatin Resistance in Ovarian Cancer Cells via Nucleo-Mitochondrial Transcriptional Feedback. Exp. Cel Res. 398, 112369. doi:10.1016/j.yexcr.2020.112369
Sherwood, J. C., Bertrand, J., Eldridge, S. E., and Dell’Accio, F. (2014). Cellular and Molecular Mechanisms of Cartilage Damage and Repair. Drug Discov. Today 19, 1172–1177. doi:10.1016/j.drudis.2014.05.014
Sieber, S., and Gigout, A. (2020). Sprifermin (Recombinant Human FGF18) Is Internalized through Clathrin- and Dynamin-independent Pathways and Degraded in Primary Chondrocytes. Exp. Cel Res. 395, 112236. doi:10.1016/j.yexcr.2020.112236
Skou, S. T., Pedersen, B. K., Abbott, J. H., Patterson, B., and Barton, C. (2018). Physical Activity and Exercise Therapy Benefit More Than Just Symptoms and Impairments in People with Hip and Knee Osteoarthritis. J. Orthop. Sports Phys. Ther. 48, 439–447. doi:10.2519/jospt.2018.7877
Springer, B. D., Carter, J. T., McLawhorn, A. S., Scharf, K., Roslin, M., Kallies, K. J., et al. (2017). Obesity and the Role of Bariatric Surgery in the Surgical Management of Osteoarthritis of the Hip and Knee: a Review of the Literature. Surg. Obes. Relat. Dis. 13, 111–118. doi:10.1016/j.soard.2016.09.011
van den Berg, W. B. (2011). Osteoarthritis Year 2010 in Review: Pathomechanisms. Osteoarthritis and Cartilage 19, 338–341. doi:10.1016/j.joca.2011.01.022
Varady, N. H., and Grodzinsky, A. J. (2016). Osteoarthritis Year in Review 2015: Mechanics. Osteoarthritis and Cartilage 24, 27–35. doi:10.1016/j.joca.2015.08.018
Vincent, T. L., and Wann, A. K. T. (2019). Mechanoadaptation: Articular Cartilage through Thick and Thin. J. Physiol. 597, 1271–1281. doi:10.1113/jp275451
Walsh, N. E., Pearson, J., and Healey, E. L. (2017). Physiotherapy Management of Lower Limb Osteoarthritis. Br. Med. Bull. 122, 151–161. doi:10.1093/bmb/ldx012
Wang, J., Li, J., Song, D., Ni, J., Ding, M., Huang, J., et al. (2020a). AMPK: Implications in Osteoarthritis and Therapeutic Targets. Am. J. Transl Res. 12, 7670–7681.
Wang, S., Wang, L., Wu, C., Sun, S., and Pan, J.-h. (2018). E2F2 Directly Regulates the STAT1 and PI3K/AKT/NF-κB Pathways to Exacerbate the Inflammatory Phenotype in Rheumatoid Arthritis Synovial Fibroblasts and Mouse Embryonic Fibroblasts. Arthritis Res. Ther. 20, 225. doi:10.1186/s13075-018-1713-x
Wang, W., Han, X., Zhao, T., Zhang, X., Qu, P., Zhao, H., et al. (2020b). AGT, Targeted by miR-149-5p, Promotes IL-6-induced Inflammatory Responses of Chondrocytes in Osteoarthritis via Activating JAK2/STAT3 Pathway. Clin. Exp. Rheumatol. 38, 1088–1095.
Whitmore, T. E., Maurer, M. F., Sexson, S., Raymond, F., Conklin, D., Deisher, T. A., et al. (2000). Assignment1 of Fibroblast Growth Factor 18 (FGF18) to Human Chromosome 5q34 by Use of Radiation Hybrid Mapping and Fluorescence In Situ Hybridization. Cytogenet. Genome Res. 90, 231–233. doi:10.1159/000056775
Wolff, D. G., Christophersen, C., Brown, S. M., and Mulcahey, M. K. (2021). Topical Nonsteroidal Anti-inflammatory Drugs in the Treatment of Knee Osteoarthritis: a Systematic Review and Meta-Analysis. The Physician and Sportsmedicine 49, 381–391. doi:10.1080/00913847.2021.1886573
Wu, H., Xu, T., Chen, Z., Wang, Y., Li, K., Chen, P. S., et al. (2020). Specific Inhibition of FAK Signaling Attenuates Subchondral Bone Deterioration and Articular Cartilage Degeneration during Osteoarthritis Pathogenesis. J. Cel Physiol 235, 8653–8666. doi:10.1002/jcp.29709
Xie, W.-q., Chen, S.-f., Tao, X.-h., Zhang, L.-y., Hu, P.-w., Pan, W.-l., et al. (2021). Melatonin: Effects on Cartilage Homeostasis and Therapeutic Prospects in Cartilage-Related Diseases. Aging Dis. 12, 297–307. doi:10.14336/ad.2020.0519
Xu, C., Qu, P., Deng, T., Bell, K., and Chen, J. (2019). Does Simultaneous Bilateral Total Joint Arthroplasty Increase Deep Infection Risk Compared to Staged Surgeries? A Meta-Analysis. J. Hosp. Infect. 101, 214–221. doi:10.1016/j.jhin.2018.08.019
Xu, J., Yi, Y., Li, L., Zhang, W., and Wang, J. (2015). Osteopontin Induces Vascular Endothelial Growth Factor Expression in Articular Cartilage through PI3K/AKT and ERK1/2 Signaling. Mol. Med. Rep. 12, 4708–4712. doi:10.3892/mmr.2015.3975
Xue, J.-F., Shi, Z.-M., Zou, J., and Li, X.-L. (2017). Inhibition of PI3K/AKT/mTOR Signaling Pathway Promotes Autophagy of Articular Chondrocytes and Attenuates Inflammatory Response in Rats with Osteoarthritis. Biomed. Pharmacother. 89, 1252–1261. doi:10.1016/j.biopha.2017.01.130
Yang, C.-Y., Chanalaris, A., and Troeberg, L. (2017). ADAMTS and ADAM Metalloproteinases in Osteoarthritis - Looking beyond the 'usual Suspects'. Osteoarthritis and Cartilage 25, 1000–1009. doi:10.1016/j.joca.2017.02.791
Yang, C., Zhang, Z., Ye, F., Mou, Z., Chen, X., Ou, Y., et al. (2020). FGF18 Inhibits Clear Cell Renal Cell Carcinoma Proliferation and Invasion via Regulating Epithelial-Mesenchymal Transition. Front. Oncol. 10, 1685. doi:10.3389/fonc.2020.01685
Yao, X., Zhang, J., Jing, X., Ye, Y., Guo, J., Sun, K., et al. (2019). Fibroblast Growth Factor 18 Exerts Anti-osteoarthritic Effects through PI3K-AKT Signaling and Mitochondrial Fusion and Fission. Pharmacol. Res. 139, 314–324. doi:10.1016/j.phrs.2018.09.026
Youle, R. J., and van der Bliek, A. M. (2012). Mitochondrial Fission, Fusion, and Stress. Science 337, 1062–1065. doi:10.1126/science.1219855
Yu, D., Xu, J., Liu, F., Wang, X., Mao, Y., Zhu, Z., et al. (2016). Subchondral Bone Changes and the Impacts on Joint Pain and Articular Cartilage Degeneration in Osteoarthritis. Clin. Exp. Rheumatol. 34, 929–934.
Zeng, N., Chen, X.-Y., Yan, Z.-P., Li, J.-T., Liao, T., Ni, G.-X., et al. (2021). Efficacy and Safety of Sprifermin Injection for Knee Osteoarthritis Treatment: a Meta-Analysis. Arthritis Res. Ther. 23, 107. doi:10.1186/s13075-021-02488-w
Zhang, S., Hu, B., Liu, W., Wang, P., Lv, X., Chen, S., et al. (2020a). Articular Cartilage Regeneration: The Role of Endogenous Mesenchymal Stem/progenitor Cell Recruitment and Migration. Semin. Arthritis Rheum. 50, 198–208. doi:10.1016/j.semarthrit.2019.11.001
Zhang, S., Hu, B., Liu, W., Wang, P., Lv, X., Chen, S., et al. (2021). The Role of Structure and Function Changes of Sensory Nervous System in Intervertebral Disc-Related Low Back Pain. Osteoarthritis and Cartilage 29, 17–27. doi:10.1016/j.joca.2020.09.002
Zhang, Y., Cai, W., Han, G., Zhou, S., Li, J., Chen, M., et al. (2020b). Panax Notoginseng Saponins Prevent Senescence and Inhibit Apoptosis by Regulating the PI3K-AKT-mTOR P-athway in O-steoarthritic C-hondrocytes. Int. J. Mol. Med. 45, 1225–1236. doi:10.3892/ijmm.2020.4491
Zhang, Z., Huang, C., Jiang, Q., Zheng, Y., Liu, Y., Liu, S., et al. (2020c). Guidelines for the Diagnosis and Treatment of Osteoarthritis in China (2019 Edition). Ann. Transl Med. 8, 1213. doi:10.21037/atm-20-4665
Zhang, Z., Li, L., Yang, W., Cao, Y., Shi, Y., Li, X., et al. (2017). The Effects of Different Doses of IGF-1 on Cartilage and Subchondral Bone during the Repair of Full-Thickness Articular Cartilage Defects in Rabbits. Osteoarthritis and Cartilage 25, 309–320. doi:10.1016/j.joca.2016.09.010
Zhao, Z., Dai, X.-S., Wang, Z.-Y., Bao, Z.-Q., and Guan, J.-Z. (2019). MicroRNA-26a Reduces Synovial Inflammation and Cartilage Injury in Osteoarthritis of Knee Joints through Impairing the NF-Κb Signaling Pathway. Biosci. Rep. 39, BSR20182025. doi:10.1042/bsr20182025
Zhou, Y., Liu, S.-q., Peng, H., Yu, L., He, B., and Zhao, Q. (2015). In Vivo anti-apoptosis Activity of Novel Berberine-Loaded Chitosan Nanoparticles Effectively Ameliorates Osteoarthritis. Int. Immunopharmacology 28, 34–43. doi:10.1016/j.intimp.2015.05.014
Keywords: sprifermin, FGF18, cartilage homeostasis, disease modifying osteoarthritis drugs, osteoarthritis, cartilage-related diseases
Citation: Song Z, Li Y, Shang C, Shang G, Kou H, Li J, Chen S and Liu H (2021) Sprifermin: Effects on Cartilage Homeostasis and Therapeutic Prospects in Cartilage-Related Diseases. Front. Cell Dev. Biol. 9:786546. doi: 10.3389/fcell.2021.786546
Received: 30 September 2021; Accepted: 23 November 2021;
Published: 14 December 2021.
Edited by:
Phillip Trevor Newton, Karolinska Institutet (KI), SwedenReviewed by:
Hong Ouyang, Sun Yat-sen University, ChinaKazuo Yudoh, St. Marianna University School of Medicine, Japan
Lukas G. Weigl, Medical University of Vienna, Austria
Copyright © 2021 Song, Li, Shang, Shang, Kou, Li, Chen and Liu. This is an open-access article distributed under the terms of the Creative Commons Attribution License (CC BY). The use, distribution or reproduction in other forums is permitted, provided the original author(s) and the copyright owner(s) are credited and that the original publication in this journal is cited, in accordance with accepted academic practice. No use, distribution or reproduction is permitted which does not comply with these terms.
*Correspondence: Hongjian Liu, ZmNjbGl1aGpAenp1LmVkdS5jbg==; Songfeng Chen, ZmNjY2hlbnNmQHp6dS5lZHUuY24=
†These authors have contributed equally to this work and share first authorship