- 1Department of Bioengineering, Imperial College London, London, United Kingdom
- 2Department of Biomedical Engineering, The Hong Kong Polytechnic University, Kowloon, Hong Kong, China
- 3AIDS Institute and Department of Microbiology, The University of Hong Kong, Pokfulam, Hong Kong, China
Effective immunotherapy treats cancers by eradicating tumourigenic cells by activated tumour antigen-specific and bystander CD8+ T-cells. However, T-cells can gradually lose cytotoxicity in the tumour microenvironment, known as exhaustion. Recently, DNA methylation, histone modification, and chromatin architecture have provided novel insights into epigenetic regulations of T-cell differentiation/exhaustion, thereby controlling the translational potential of the T-cells. Thus, developing strategies to govern epigenetic switches of T-cells dynamically is critical to maintaining the effector function of antigen-specific T-cells. In this mini-review, we 1) describe the correlation between epigenetic states and T cell phenotypes; 2) discuss the enzymatic factors and intracellular/extracellular microRNA imprinting T-cell epigenomes that drive T-cell exhaustion; 3) highlight recent advances in epigenetic interventions to rescue CD8+ T-cell functions from exhaustion. Finally, we express our perspective that regulating the interplay between epigenetic changes and transcriptional programs provides translational implications of current immunotherapy for cancer treatments.
Introduction
Adaptive immunity is a physiological defensive mechanism, including fighting against cancers. T cell-based adaptive immunity is one of the current immunotherapies that relies on the recognition and lysis of target cancer cells to achieve an effective anti-tumor response (Wong W. K. et al., 2021). In the tumor microenvironment (TME), dendritic cells (DCs) phagocytose apoptotic tumorigenic cell antigens and present the antigen as peptide-major histocompatibility complex (pMHC). The DCs subsequently migrate into the lymph node to prime naïve T cells through engaging the pMHC with T cell receptor (TCR) (Albert et al., 1998; Mempel et al., 2004; Bottcher and Sousa, 2018; Hiam-Galvez et al., 2021). However, TCR is a highly specific biomolecular construct that can vary T cell responses to cognate antigen with minor changes in the peptide sequence of the TCR (Liu Y. et al., 2016). Subsequently, the activated T cells with antigen-specific TCR from a T cell pool in lymph nodes undergo differentiation, migrate and infiltrate into TME to attack target cancer cells (Bousso, 2008; Gattinoni et al., 2012; Ozga et al., 2016; van der Woude et al., 2017). We mainly focus on the physiology of CD8+ T cells against chronic antigen exposure or cancer in this review.
Activated T cells differentiate into T cell subsets with various biological functions in adaptive immunity. Previous studies proposed a progressive T cell differentiation model to describe the T cell lineage relationship depending on signal strength (Henning et al., 2018a). Gattinoni et al. suggested that naïve T cell (TN) differentiated in the following order: memory stem T (TSCM) cell, central memory T (TCM) cell, effector memory T (TEM), effector T (TE) cell as illustrated in Figure 1A (Huster et al., 2006; Gattinoni et al., 2012; Henning et al., 2018a). Each subset has its feature in terms of lifespan and T cell activity. For instance, TSCM is a recently identified subset that possesses stem cell characteristics: self-renewal and the potency of differentiation into TCM, TEM, TE. Also, TSCM showed the highest proliferation capacity among T cell subsets (including TN) and higher anti-tumor activity than TCM, TEM, and TE (Gattinoni et al., 2011). Memory T cells can respond to antigen challenges with robust expansion in vivo and show cytolytic features earlier than TN cells (Croft, 1994; Bruno et al., 1995; Cho et al., 1999; Veiga-Fernandes et al., 2000; Berard and Tough, 2002; Joshi and Kaech, 2008). TCM and TEM are two longer living subsets than TE. TCM is defined as CC-chemokine receptor 7 positive (CCR7+) and able to return into secondary lymphoid organs (e.g., lymph node and spleen (Ruddle and Akirav, 2009)), while TEM is defined as CCR7– and can accumulate in inflamed tissues and shows more prominent effector feature, including higher production of interferon-gamma (IFNγ), compared to TCM (Sallusto et al., 1999; Sallusto et al., 2004; Huster et al., 2006). TE is short-lived and the terminal phenotype of T cells in the differentiation lineage, according to the proposed model. TE is featured by more effector molecules storage such as granzyme B (GZMB) in granules and higher early cytotoxicity (<6 h) in vitro compared to TCM, TEM cells (Wolint et al., 2004; Huster et al., 2006).
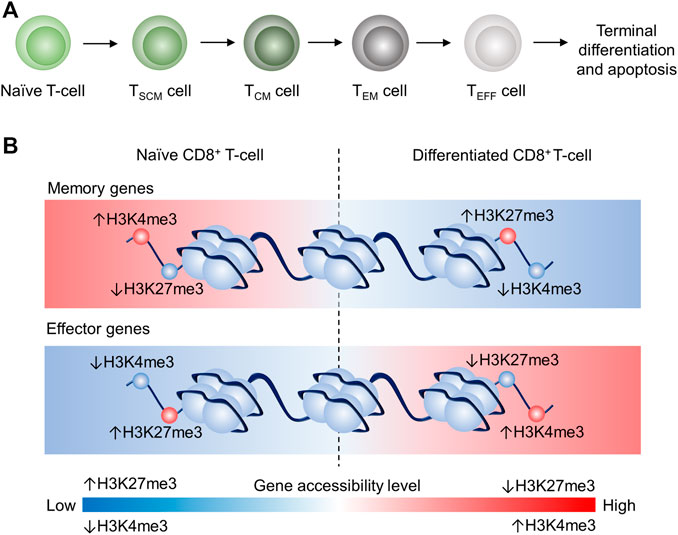
FIGURE 1. Schematic illustration of the proposed linear model and epigenetic remodeling through dynamic histone modification of CD8+ T cell differentiation CD8+ T cell differentiation (Crompton et al., 2016; Henning et al., 2018b). (A) Progressive acquisition of effector function by naïve CD8+ T-cell differentiation: Naïve (TN)→TSCM→TCM→TEM→TEFF, which will eventually terminate differentiation and undergo apoptosis. (B) Schematic illustrating of the epigenetic remodeling dynamics of H3K4me3 and H3K24me3 in T cells. In TN, memory genes are more accessible because of high H3K4me3 tag density and low H3K27me3 tag density, whereas effector genes in TN are less accessible due to low H3K4me3 tag density and high H3K27me3 tag density. In differentiated CD8+ T cells, memory genes are less accessible due to low H3K4me3 tag density and high H3K27me3 tag density, whereas effector genes in CD8+ differentiated T cells are more accessible because of high H3K4me3 tag density and low H3K27me3 tag density. This epigenetic remodeling is associated with the memory gene downregulation and effector gene upregulation in differentiated CD8+ T cells.
However, human adaptive immunity often fails to clear tumors completely, leading to the progressive growth of cancer cells and metastasis without medical intervention. One key challenge to this self-defense is T cell exhaustion, which promotes apoptosis and the upregulation of inhibitory receptors as a state of T cell dysfunction (Wherry, 2011). Inhibitory ligands, such as programmed death-ligand 1 (PD-L1) expressed on cancer cells, bind to the corresponding inhibitory receptor, programmed cell death protein 1 (PD-1), expressed on T cells, leading to inhibition of T cell activation signals (Boussiotis, 2016; Wong S. H. D. et al., 2021). Thus, T cell exhaustion weakens adaptive immunity that causes the failure of self-defense against tumors. To address this issue, researchers have developed immune-checkpoint blockade strategies to disrupt the interaction between inhibitory ligands and receptors, thereby preventing inhibitory signals in T cells (Leach et al., 1996; Freeman et al., 2000; Iwai et al., 2005). This strategy has shown improved therapeutic outcomes for cancers (Hamid et al., 2013; Robert et al., 2014; Tumeh et al., 2014; Ansell et al., 2015).
Therefore, versatile biological signals can regulate CD8+ T cell behaviors. Stimulatory/inhibitory signals initiate intracellular signal cascades through T-cell membrane receptors and alter gene expression profiles that ultimately define T cell functionality and phenotype (Gattinoni et al., 2012; Chen and Flies, 2013; Boussiotis, 2016). More importantly, those signals indirectly modulate gene methylation and histone modification (methylation of histone protein tail). Hence, T cell differentiation and T cell exhaustion are two critical determinants in anti-tumor activities by adaptive immunity. It is highly desirable to explore the mechanistic insights into epigenetic regulation of T cell behaviors for engineering T cell-based immunotherapy strategies to treat cancers (Hiam-Galvez et al., 2021). Several recent reviews have explored the microRNA (miRNA) involved in the regulations of T cell differentiation and malignancy (Liang et al., 2015; Saki et al., 2015; Rodríguez-Galán et al., 2018). However, limited reviews discuss the association between miRNA and the enzymatic factors to govern epigenetics of CD8+ T cells against tumors and pathogens. In this mini-review, we discuss the correlated epigenetic profile of the respective CD8+ T cell phenotype, highlight the significant enzymatic factors and intracellular/extracellular miRNA that regulate T cell response at the epigenetic level, and discuss the possible strategies by manipulating these factors for improved cancer treatments.
Epigenetic Landscape Correlates With T Cell Differentiation
The epigenetic landscape represents the gene accessibility profile, which describes the physical occupancy of chromatin-binding molecules (such as transcription factor and polymerase) along with the sequences of interest (Klemm et al., 2019), typically revealed by assay for transposase-accessible chromatin with sequencing (ATAC-Seq). Such assay describes the transcription availability of the chromatin physical openness. Avgustinova et al. reported that the controlled and dynamic epigenetic landscape underlined the stem cell fate of both haematopoietic and mesenchymal lineages (Avgustinova and Benitah, 2016), responsible for replacing damaged or dying cells during tissue homeostasis. In the immune system, CD8+ T cells play a central role in defending against pathogen infection (Harty et al., 2000) and tumors (Yee et al., 2002). The global epigenetic difference lies in the gene loci associated with activated T cell features such as cell division, immune response, and metabolism (Scharer et al., 2017). Specifically, CD8+ TN, TE, and memory T cells showed distinct genome-wide accessibility, suggesting the epigenetic remodeling during T cell differentiation. Scharer et al. showed that the global chromatin accessibility of lymphocytic choriomeningitis virus (LCMV) infection-activated CD8+ TN was dynamically remodeled at the indicated time points (Scharer et al., 2017). Critically, their results indicated that TN progressively/linear changed to memory T cells in terms of chromatin accessibility and most memory-related differentially accessible regions shared with effector cells, with one exceptional small subset that was reprogrammed during differentiation from effector memory. These findings potentially compel the construction of the recently established linear/circular model during T cell differentiation (Henning et al., 2018b). These global views on epigenome indicate the importance of understanding epigenetic mechanisms underlying T cell differentiation and related immune response. These reports motivate us to discuss the effect of individual epigenetic modifications, such as H3K27me3 (trimethylation of lysine 27 on histone H3) and H3K4me3 modifications as the recently investigated candidates, on CD8+ T cell differentiation in this mini-review.
DNA methylation silences gene expressions by restricting accessibility to transcription machinery, including the recruitment of transcription factors and RNA polymerase II, through methylating cytosines without interfering with DNA sequences (epigenetics) (Keshet et al., 1986; Cedar, 1988; Moore et al., 2013; Davies et al., 2014; Yang et al., 2014). Thus, DNA methylation (“OFF”) and demethylation (“ON”) regulate gene expression profiles, thereby predicting T cell phenotypes upon cell differentiation (Cedar, 1988; Robertson, 2005; Moore et al., 2013; Smith and Meissner, 2013). To verify the proposed linear T cell differentiation model, recent research has investigated progressive epigenetic changes across various T cell subtypes from TN. For instance, Yang et al. has recently revealed a methylome analysis on T cells isolated from colorectal cancer patients. The authors showed that DNA methylation levels of signature genes are associated with T cell phenotypes: Tcf7, a naïve characteristic gene, was demethylated in TN, but highly methylated in TEM cells and tumor-infiltrating lymphocytes (TILs); Ifng and Gzmb, known as cytotoxic characteristic genes, were highly methylated in TN, but demethylated in TEM cells (Yang et al., 2019). The “opposing” methylation state features of both TN signature genes and cytotoxic signature genes might facilitate the T cell differentiation. Besides, it is possible to probe T cell subsets by recognizing the respective methylome profile.
Histone modification regulates gene transcription by changing the gene accessibility, including the modulation of physical chromatin compaction (Lawrence et al., 2016). Thus, histone modification enriched at T cell function-associated genes significantly influence gene expression. Acetylation (ac) of histone (e.g., H3 lysine 9/H3K9) is associated with accessible chromatin structure for transcription (Fann et al., 2006). An early report from Fann et al. showed that a higher level of H3K9ac was detected in several cytokine loci (such as IL2 and TNF) in resting memory CD8+ T cells compared to resting TN cells (Fann et al., 2006). Upon stimulation, the transcription activity of these genes was higher in activated memory CD8+ T cells compared to activated TN cells (Fann et al., 2006). These findings suggest that histone modification could control T cell response to stimulation through transcription regulation, and differences in the epigenetic landscape might define the functional difference of distinct T cell subsets.
H3K4me3 (Histone 3 Lysine 4 trimethylation) and H3K27me3 (Histone 3 Lysine 27 trimethylation) are believed in the regulation of stem cell differentiation (Margueron and Reinberg, 2011). H3K4me3 and H3K27me3 are also two well-known examples of CD8+ T cell differentiation (Crompton et al., 2016). Specifically, memory genes (e.g., Tcf7) are downregulated during CD8+ T with reduced H3K4me3 tag and increased H3K27me3 tag at memory gene promoter regions (Figure 1B). However, effector genes (e.g., Tbx21) are upregulated in differentiated CD8+ T cells (e.g., TCM and TEM) with increased H3K4me3 tag and reduced H3K27me3 tag at effector gene promoter regions (Crompton et al., 2016). Therefore, dynamics of methylome profile and histone methylation correlate to the lineage along with the linear model.
T-cell development involves roles for some transcription factors that are products of multigene families, such as the ETS family and the RUNX family that are likely to play important roles as participants in most lymphoid gene expression, based on the extreme enrichment of their binding motifs in enhancers of lymphoid genes with various patterns of activity (Rothenberg et al., 2016). In particular, Russell et al. showed that ETS-1 regulated cytokine production in T cells, and ETS-1 deficient CD8+ T cells significantly reduced the expression of IL-2 and IFNγ (Russell and Garrett-Sinha, 2010). Besides, ETS-1 was shown to be essential for T cell survival (Bories et al., 1995; Muthusamy et al., 1995). In the context of CD8+ T cell differentiation, Grennigingloh et al. revealed that ETS-1 maintained the expression of IL-7 receptor responsible for memory T cell development (Grenningloh et al., 2011). Besides, ETS-1 is also shown to regulate the expression of the IL-12 receptor, responsible for effector T cell development (Li et al., 2010; Liu M. et al., 2016). Therefore, ETS-1 is likely to be an essential transcription factor for facilitating CD8+ T cell differentiation. However, the report of the relation between ETS-1 and overall CD8+ T cell-dependent anti-tumor response in vivo has been rare at this stage.
ETS-1 also mediates the expression of Runt-related transcription factor 3 (Runx3) (Zamisch et al., 2009; Liu M. et al., 2016), a well-known transcription factor involved in CD8 lineage commitment from thymic double-positive (CD4+CD8+) T cells (Sato et al., 2005) and TE cell development (Shan et al., 2017). Recently, Milner and colleagues showed that Runx3 was responsible for generating tissue-resident memory T (TRM) cells (Milner et al., 2017). Interestingly, the authors showed that activated T cells showed increased tbx21 (encoding T-Bet, an immune cell-specific member of the T-box family of transcription factors) accessibility to Runx3 compared to TN cells. The authors also indicated that direct binding of Runx3 on tbx21 loci inhibited the expression of T-bet, which prevented TRM differentiation. In addition, their findings showed Runx3 overexpression promotes TILs tumor residency, which led to improved anti-tumor outcomes.
Epigenetic Remodelling Events During T Cell Exhaustion
The expression of the inhibitory receptor such as PD-1 on T cells suggests that they are vulnerable to respective inhibitory ligands, which are a hallmark of T cell exhaustion (Wherry, 2011). However, exhausted T cell appears to be a functionally heterogeneous population. C-X-C Motif Chemokine Receptor 5 (CXCR5) is a chemokine receptor that is normally present on B cells and CD4+ T follicular helper cells (TFH) (Im et al., 2016). Tim-3 is recently shown the part of a module that contains multiple co-inhibitory receptors (checkpoint receptors), which are co-expressed and co-regulated on exhausted T cells in chronic viral infections and cancer (Wolf et al., 2020). Im and colleagues have recently shown that CXCR5+Tim-3– and CXCR5–Tim-3+ subsets represent the proliferative and non-proliferative population, respectively, within PD-1+ LCMV-specific T cell population (Im et al., 2016). In their study, gene set enrichment analysis (GSEA) showed that CXCR5–CD8+ T cells were related to CD4+ TH1 (T helper type 1) cells and CD8+ terminal effectors, but the CXCR5+ subset was similar to CD4+ TFH cells and CD8 memory precursors. Besides, the GSEA result also indicated a relationship between CXCR5+CD8+ T cells and haematopoietic stem cell progenitors, implying that LCMV-specific CXCR5+CD8+ T cells may function as memory stem cells during chronic infection. Subsequently, the authors confirmed that CXCR5+CD8+ T cells were able to proliferate and give rise to the CXCR5–Tim-3+ subset in vivo. However, CXCR5–CD8+ T cells were terminally differentiated with limited proliferative potential. Specifically, accessibility to Tcf7 (encoding transcription factor 1, TCF1 protein responsible for the generation of CXCR5+CD8+ T cells) was reduced, and accessibilities to Gzmb (encoding granzyme B) and Prf1 (encoding perforin-1) were increased during the transition of CXCR5+Tim-3– to CXCR5–Tim-3+ (Jadhav et al., 2019). The high level of perforin and granzyme expression features in CXCR5–Tim-3+ T cell population suggests their active immune functionality despite poor proliferation (Im et al., 2016). Together, PD-1+ virus-specific CD8+ T-cell population can be characterized by a unique gene signature with similarities to CD4+ TFH cells, CD8+ memory precursor cells, and haematopoietic stem cell progenitors. Thus, the epigenetic landscape is closely related to exhausted T cell functionalities.
TILs are shown to experience two significant epigenetic remodeling events rather than one progressive change in chromatin accessibility. Two distinct T cell dysfunction-associated chromatin accessibility states were identified: (1) dysfunctional plastic state and (2) fixed dysfunctional state (Philip et al., 2017). The former appears in the early tumor exposure and is featured by CD38low, CD101low, CD30Llow, and CD5high whereas the latter appears in long-term tumor exposure and is featured by CD38hi, CD101hi, CD30Lhi, CD5low. Despite the fact that PD-1 is expressed in both states, T cells with state (1) retain the responsiveness to interleukin-15 (IL-15), but T cells with state (2) do not. Therefore, whether the exhausted TILs cells can be rescued to be tumor-reactive again, the functional phenotype of the TILs may depend on the epigenetic landscape rather than the quantity of inhibitory ligand surface expression (Philip et al., 2017). Thus far, biological factors in TME that influence the change of chromatin state from (1) to (2) would be interested in the future direction.
H3K79me2 is shown to bind at the Stat5b promoter region and enhance STAT5B transcription that mediates cytokine signallings (such as IL-7 and IL-15) for maintaining CD8+ T cell survival (Kelly et al., 2003; Tripathi et al., 2010; Villarino et al., 2017; Bian et al., 2020). Bian et al. comprehensively investigated the abnormal epigenetic patterns correlation with effector T cell malfunction in tumors (Bian et al., 2020). Mechanistically, their results showed that B16F10 cells consumed and outcompeted CD8+ T cells for methionine via its high expression of SLC43A2, a methionine transporter, causing the T cells to lose H3K79me2 in a co-culture system. Moreover, their findings showed that the deprivation of methionine promoted CD8+ T cell apoptosis and reduced its IFNγ and TNFα production, whereas methionine supplement improved tumor-infiltrating CD8+ T cell cytokines production and anti-tumor responses (Bian et al., 2020). This study indicates that methionine consumption is an immune evasion mechanism, and targeting cancer methionine signaling may provide an immunotherapeutic approach (Kelly et al., 2003; Tripathi et al., 2010; Villarino et al., 2017).
One popularly investigated marker transcription factor, TOX, was found to associate with T cell exhaustion. TOX is induced through the Calcineurin-NFAT2 pathway (Macian, 2005; Martinez et al., 2015; Klein-Hessling et al., 2017), but its maintenance is Calcineurin independent in the late-stage (Khan et al., 2019). Tox is highly accessible epigenetically in exhausted CD8+ T cells compared to naïve, effector, memory phenotypes. Khan et al. showed that knocking out TOX (TOX−) in T cells promoted TE-associated genes upregulation and downregulated exhaustion associated genes. At the epigenetic level, genetic deletion of TOX resulted in decreased exhaustion-related and memory-related chromatin accessibility and increased TE-associated chromatin accessibility.
Enzymatic Factors Regulating T Cell Function Through Epigenetic Remodeling
It is known that DNA methyltransferase (DNMT) mediates DNA methylation, which implies that DNMT participated in the process of T cell differentiation (Pace and Amigorena, 2020; Akbari et al., 2021). Ladle et al. (2016) have shown that the knocking out DNMT3a gene (DNMT3a–) skews early effector CD8+ T cells to differentiate into memory precursor phenotype (CD127+KLRG1–) rather than terminal effector phenotype (CD127–KLRG1+). It is because DNMT3a binds at the Tcf7 promoter region to repress the TCF1 expression, which inhibits the effector CD8+ function. It has also been shown that DNMT3a–CD8+ T cells produce more interleukin-2 (IL-2) and IFNγ in chronic infection settings than those expressing DNMT3a (Ghoneim et al., 2017). Similarly, DNMT1 is critical to TE expansion (Chappell et al., 2006). Knockout of DNMT1 (DNMT1–) resulted in significantly fewer IFNγ+CD8+ T cells than those without knockout upon in vitro stimulation (Table 1). However, the cytotoxicity of DNMT1–CD8+ T cells was enhanced probably because of the demethylation at the perforin enhancer. These findings suggested that the DNA methylome profile of each T cell subset is critical toward T cell functions. The anti-tumor response impacted by intracellular DNMT modulation in CD8+ T cells would be an attractive area of research in immunotherapy.
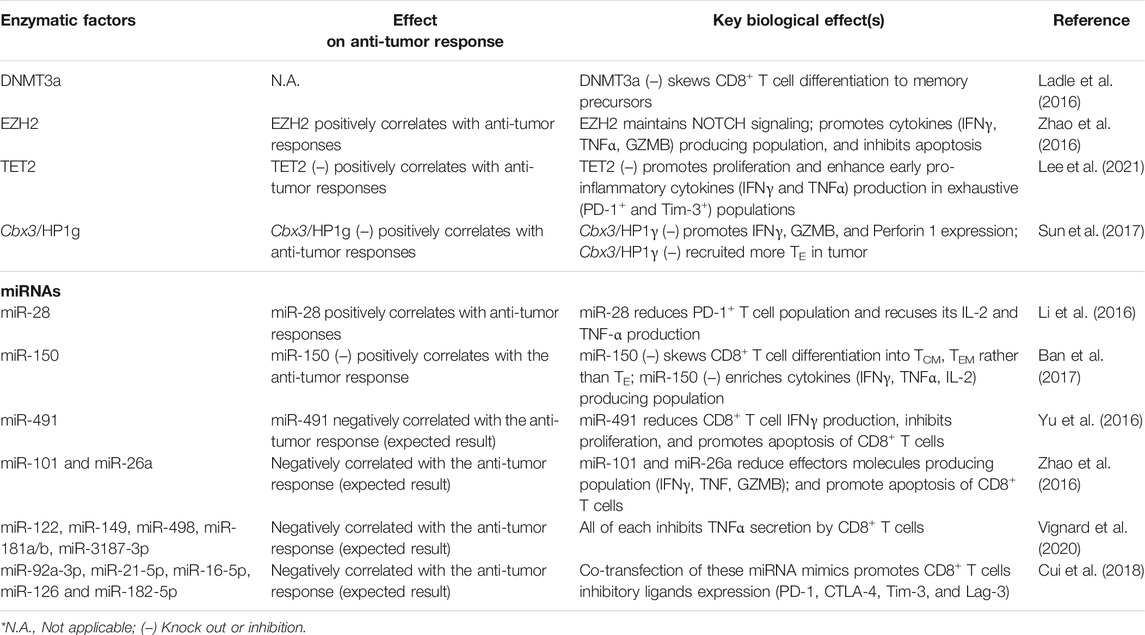
TABLE 1. Summary of enzymatic factors and miRNA regulating epigenetics of CD8+ T cells with corresponding biological outcomes.
Margueron and Reinberg (2011) discussed that polycomb repressive complex 2 (PRC2) silenced gene transcription through H3K27me2 and H3K27me3 modification. H3K27me2 was an intermediate product of H3K27me3 and prevented the acetylation of H3K27. It has been realized that the enhancer of zeste homolog 2 (EZH2), a histone methyltransferase core of PRC2, expresses robustly upon T cell activation (Margueron and Reinberg, 2011; Zhao et al., 2016). Also, EZH2 sustains NOTCH signalings through inhibiting NOTCH suppressor Numb, F-Box, and WD Repeat Domain Containing 7 (FBXW7) through H3K27me3 modification, promoting CD8+ T cell polyfunctionality (expressing 2 or 3 effector molecules: IFNγ, TNFα, and GZMB) and suppressing apoptosis (Öberg et al., 2001; Wirtz-Peitz et al., 2008; Yumimoto et al., 2013; Zhao et al., 2016). In terms of anti-tumor immunity, EZH2+CD8+ T cells are often void of dysfunction markers (KLRG1, Tim-3, CD57), and the EZH2+CD8+ T cell population positively correlates to anti-tumor response (Wherry, 2011; Crespo et al., 2013; Zhao et al., 2016).
Similarly, ten-eleven translocation 2 (TET2), a methylcytosine dioxygenase involved in DNA demethylation, was found to regulate CD8+ T cell functions (Carty et al., 2018; Lee et al., 2021). Upon T cell stimulation, the influx of Ca2+ elicits TET2 mRNA expression (Carty et al., 2018). Adoptive transfer of TET2 knocked out (TET2 KO) CD8+ T cells to murine melanoma tumor model showed significant tumor regression and a significant increase of TET2 KO CD8+ T cell population in the tumor, spleen, and peripheral blood. Functional populations (IFNγ+ and TNFα+) and exhausted populations (PD-1+ and Tim-3+) of TET2 KO CD8+ T cells were enhanced in the early immune response (3 days) rather than late immune response. Short-term (4 h) in vitro cytotoxicity and survival of TET2 KO CD8+ T cells were also promoted. At the epigenetic level, the E26 transformation-specific (ETS) transcription factor family was enriched in the differential chromatin-accessible region of TET2 KO CD8+ TILs, whereas ETS inhibition reduced the cytotoxicity of TET2 KO CD8+ TILs (Lee et al., 2021). These together suggest that the loss of TET2 promotes the accessibility of ETS enriched gene loci which mediate the enhanced anti-tumor function.
H3K9 methylation (e.g., me2 or me3) is an important repressive epigenetic mark on T cell development (Takikita et al., 2016). ESET/SETDB1, one of the major histone methyltransferases, catalyzes H3K9 trimethylation (Schultz et al., 2002; Yang et al., 2002). Takikita et al. (2016) showed that thymocyte-specific deletion of ESET caused impaired T cell development, particularly obvious in CD8 lineage cells that were severely affected. Such deletion led to increased apoptosis and suppressed TCR-induced ERK activation in the CD8+ cells. This study outlined the critical role of H3K9 histone methyltransferases in T cell development. Similarly, Sun et al. reported that histone reader Cbx3/HP1γ occupied the transcription start site of Prf1, Gzmb, and Ifng in activated CD8+ T cells. Cbx3/HP1γ insufficiency caused reduced H3K9me3 deposition at these loci, which were subsequently deposited by Runx3 and polymerase II. Adoptive transfer of Cbx3/HP1γ insufficient CD8+ effector T cells recruited a high population of CD122+CD44+CD8+ effector T cells and NKG2D+ CD8+ T cells in tumors (Sun et al., 2017). In short, the dynamics of epigenetic profiles actively shape the function and feature of T cells during adaptive immunity. Altering enzymatic factors can skew CD8+ T cell effector function. Thus, identifying the mechanism of methylation-associated enzymes remodeling chromatin and DNA methylation in CD8+ T cells provides novel insights into therapeutic design T cell-based therapy (Pace and Amigorena, 2020; Akbari et al., 2021; Frias et al., 2021).
MicroRNA Modulates CD8+ T Cell Anti-Tumor Responses
MicroRNA (miRNA) is a non-coding RNA sequence with 18–22 nucleotide units. It is reported that miRNA hybridizes with mRNA to form miRNA/mRNA duplex to restrict mRNA translation and cause mRNA degradation (John et al., 2004; Bushati and Cohen, 2007; Cai et al., 2009; Ha and Kim, 2014). miRNAs are critical to regulating embryonic development and stem cell differentiation. An impaired miRNA profile is often associated with diseases, such as cancers (Yao et al., 2019). Hence, the detection of miRNA expression has recently been an active area of research (Wong et al., 2020; Dai et al., 2021). Moreover, previous reports have shown that miRNAs are differentially expressed in different T cell phenotypes upon T cell activation, implying that miRNA dynamics involves in T cell differentiation. Several excellent reviews have explored the relationship between miRNA dynamics and T cell differentiation (Liang et al., 2015; Emming et al., 2018; Rodríguez-Galán et al., 2018). In this section, we discuss and summarize the latest research progress on how miRNA regulates T cell behaviors (Table 1).
miR-28 targets mRNA of inhibitory receptors PD-1 (Li et al., 2016). Transfecting miR-28 mimetic to exhausted-CD8+ T cells reduces PD-1+ T cell population and recuses lymphocytes to produce IL-2 and TNF-α. Therefore, miR-28 can be a nucleic acid-based therapeutic approach to shifting T cells from exhausted to non-exhausted phenotype. Also, miR-150 inhibits memory T cell differentiation by targeting 3’ UTR of fork-head box O1 (Foxo1) and therefore suppressing TCF1 (Ban et al., 2017). Ban and colleagues reported that miR-150 deficiency skewed CD8+ T cells into TCM and TEM rather than effector T cells (TE) phenotype in an acute infection model and enriched the multiple cytokines producing population (e.g., IFNγ, TNFα and IL-2). Moreover, miR-150 deficient memory T cells proliferated more robustly than WT TM cells.
Besides intrinsic miRNA in T cells that cause their exhaustion, tumor cells also secrete biomolecules to suppress anti-tumor activities of T cells and promote their survival. For instance, TGF-β1 secreted by cancer cells upregulates miR-491 in CD8+ T cells, subsequently reducing their IFNγ production. miR-491 also limits T cell proliferation by targeting TCF1 and Cyclin-dependent kinase 4 (CDK4) mRNA (Jeannet et al., 2010; Zhou et al., 2010; Lee et al., 2013; Xu et al., 2014; Yu et al., 2016). miR-491 is reported to inhibit B-cell lymphoma-extra large (Bcl-xL) expression, and this downregulation promotes CD8+ T cell apoptosis (Yu et al., 2016). Thus, miR-491 is a negative regulator in CD8+ T cells for anti-tumor immunity.
miR-101 (Varambally et al., 2008) and miR-26a (Sander et al., 2008) can restrict the aforementioned EZH2 signaling pathway via targeting 3’ UTR of EZH2 coding genes and hence suppress NOTCH signalings (Zhao et al., 2016). In TME, the amount of glucose is limited that impaired the glycolysis of TIL, leading to upregulation of miR-101 (Varambally et al., 2008) and miR-26a in TILs (Sander et al., 2008). The transfection of miR-101 or miR-26a mimetic RNA to CD8+ T cells reduces stimulating cytokines producing T cell population and promotes T cells apoptosis. Recall that the EZH2+CD8+ T cell population highly associates with the enhanced anti-tumor response, miR-101 and miR-26a inhibit the expression of EZH2 in CD8+ T cells (Zhao et al., 2016). Thus, suppressing both miR-101 and miR-26a in CD8+ T cells could be a potential strategy to boost immunotherapy efficiency.
Tumor cells can also deliver miRNA-containing extracellular vesicles (EVs) to inhibit CD8+ T cell activities (Ye et al., 2014; Yi et al., 2020). It has been reported that human melanoma cell line-derived EVs contain hsa-miR-122, hsa-miR-149, hsa-miR-498, hsa-miR-181a/b, and hsa-miR-3187-3p. After TILs uptaking the EVs with these miRNAs, their TNFα secretion amount is reduced (Xiao et al., 2012; Vignard et al., 2020). Besides, these EVs can also regulate the expression of miRNA in TILs, which eventually become exhausted. For example, Cui et al. have reported that human leukemia cell line K562-derived EVs contains miR-92a-3p, miR-21-5p, miR-16-5p, miR-126, and miR-182-5p. Co-transfecting with mentioned miRNA mimics to CD8+ T cells promotes their inhibitory ligands expression (PD-1, CTLA-4, Tim-3, and Lag-3) (Cui et al., 2018). Hence, inhibiting tumor cells from expressing inhibitory miRNAs that refrain T cell anti-tumor activities can also be one of the future therapeutic strategies in cancer immunotherapy.
Future Perspective
Traditional clinical trials target single proteins or nodes in biological pathways (e.g., protein inhibitors) but often fail to resolve the diseased phenotypes (Hopkins, 2008). Currently, both miRNA mimics and repressors for therapies are in clinical development or in phase 1–2 clinical trials, although they have not yet been translated into FDA-approved candidates (Hanna et al., 2019). Nevertheless, both traditional and miRNA therapeutics provoke off-target biological effects by the pleiotropic nature. Similarly, enzymatic factor-based therapeutics, such as DNMT inhibitors, have been recognized as novel strategies for cancer therapy via epimutations (on cancer cells or Tregs), yet they are still in the developmental stage (Hu et al., 2021). Intriguingly, highly proliferative cells, including cancer cells, are shown to be very sensitive to hypomethylating agents, i.e., DNMT inhibitors (e.g., azacytidine, decitabine, and zebularine), which also potentiate the effects of radiotherapy for cancers by exposing nucleoside analogues as radiosensitizers (Gravina et al., 2010). These findings imply that these inhibitors are on-target and can overcome melanoma resistance to immunotherapy (e.g., PD-1/PD-L1 interactions). However, the report of the effect of these inhibitors on T cells has been limited despite the intensive studies of overexpressing/knocking out the related genes responsible for DNA methylation/demethylation via biological means. Also, exhausted T cells are much less proliferative, suggesting a low targeting effect by DNMT inhibitors in the TME (Gravina et al., 2010). In addition, literature indicated that DNMT inhibitors caused toxicity and possibly provoked mutagenic/carcinogenic potentials to non-cancerous cells in the long term (Eden et al., 2003). Together, selective delivery of enzymatic drugs or miRNAs is crucial to limit off-target effects and optimize the outcome of cancer immunotherapy.
To facilitate on-target delivery of miRNA for reversing T cell exhaustion, we believe that cytocompatible nanomaterials such as liposomes and synthetic polymers are effective miRNA vehicles, and their surfaces can be modified with T cell-targeting ligands (e.g., CD8 binding ligands) to increase intracellular controlled released, thereby enhancing the therapeutic efficacy (Li et al., 2019; Hou et al., 2021). However, studies employing nanomaterials for intracellular delivery of miRNA in T cells have been rare to increase tumor response rates to immunotherapy. Similarly, these nanocarriers are also suitable to encapsulate proteins for controlled releasing the enzymatic factors/inhibitors. For instance, a recent study fabricated FDA-approved polymers poly(lactic-co-glycolic acid) (PLGA) and polyethylene glycol (PEG) hybrid nanoparticles bearing CD8a and PD-1 antibodies to target CD8+/PD-1+ T cells for specifically delivering TGFβ (an immunosuppressor) inhibitors extending the survival and sensitivity to PD-1 antibodies in TILs (Schmid et al., 2017). Thus, we suggest that the recent advances in nanotechnology provide a highly sound footing platform to foster the integration of epigenetic regulation in cancer immunotherapy.
Conclusion
CD8+ T cells recognize and can potentially eradicate cancer cells in adaptive immunity. Upon T cell activation, phenotypical and functional change dramatically and progressively. T cell differentiation is accompanied by changes in the epigenetic profile. Enzymes and miRNAs are the two discussed factors that control the change in T cell phenotype and function acquisition in this review. Besides, the interactions between TILs and tumor cells in TME can cause sophisticated functional changes in TILs through epigenetic remodeling. Therefore, understanding the mechanisms of T cell dysfunction at the epigenetic level can potentially explore the conceptual gaps in knowledge for the failure of conventional T cell-based cancer immunotherapy. With the recent advances in nanotechnology, the efficacy of such therapy can potentially be optimized.
Author Contributions
WKW and BY contributed equally. All authors wrote the paper. WKW and BY conceptualize the manuscript. SW and ZT supervised and revised the papers.
Funding
This work was supported by start-up funding (0033912) from the Department of Biomedical Engineering and Start-up Fund for RAPs under the Strategic Hiring Scheme (0035876), the Hong Kong Polytechnic University (University Grant Council). ZT would like to acknowledge the support of Health and Medical Research Fund (#05162326) and Collaborative Research Fund (C1134-20GF).
Conflict of Interest
The authors declare that the research was conducted in the absence of any commercial or financial relationships that could be construed as a potential conflict of interest.
Publisher’s Note
All claims expressed in this article are solely those of the authors and do not necessarily represent those of their affiliated organizations, or those of the publisher, the editors and the reviewers. Any product that may be evaluated in this article, or claim that may be made by its manufacturer, is not guaranteed or endorsed by the publisher.
References
Akbari, B., Ghahri-Saremi, N., Soltantoyeh, T., Hadjati, J., Ghassemi, S., and Mirzaei, H. R. (2021). Epigenetic Strategies to Boost CAR T Cell Therapy. Mol. Ther. 29 (9), 2640–2659. doi:10.1016/j.ymthe.2021.08.003
Albert, M. L., Sauter, B., and Bhardwaj, N. (1998). Dendritic Cells Acquire Antigen from Apoptotic Cells and Induce Class I-Restricted CTLs. Nature 392 (6671), 86–89. doi:10.1038/32183
Ansell, S. M., Lesokhin, A. M., Borrello, I., Halwani, A., Scott, E. C., Gutierrez, M., et al. (2015). PD-1 Blockade with Nivolumab in Relapsed or Refractory Hodgkin’s Lymphoma. N. Engl. J. Med. 372 (4), 311–319. doi:10.1056/NEJMoa1411087
Avgustinova, A., and Benitah, S. A. (2016). Epigenetic Control of Adult Stem Cell Function. Nat. Rev. Mol. Cell Biol. 17 (10), 643–658. doi:10.1038/nrm.2016.76
Ban, Y. H., Oh, S.-C., Seo, S.-H., Kim, S.-M., Choi, I.-P., Greenberg, P. D., et al. (2017). miR-150-Mediated Foxo1 Regulation Programs CD8 + T Cell Differentiation. Cell Rep. 20 (11), 2598–2611. doi:10.1016/j.celrep.2017.08.065
Berard, M., and Tough, D. F. (2002). Qualitative Differences between Naive and Memory T Cells. Immunology 106 (2), 127–138. doi:10.1046/j.1365-2567.2002.01447.x
Bian, Y., Li, W., Kremer, D. M., Sajjakulnukit, P., Li, S., Crespo, J., et al. (2020). Cancer SLC43A2 Alters T Cell Methionine Metabolism and Histone Methylation. Nature 585 (7824), 277–282. doi:10.1038/s41586-020-2682-1
Bories, J.-C., Willerford, D. M., Grévin, D., Davidson, L., Camus, A., Martin, P., et al. (1995). Increased T-Cell Apoptosis and Terminal B-Cell Differentiation Induced by Inactivation of the Ets-1 Proto-Oncogene. Nature 377 (6550), 635–638. doi:10.1038/377635a0
Böttcher, J. P., and Reis e Sousa, C. (2018). The Role of Type 1 Conventional Dendritic Cells in Cancer Immunity. Trends Cancer 4 (11), 784–792. doi:10.1016/j.trecan.2018.09.001
Boussiotis, V. A. (2016). Molecular and Biochemical Aspects of the PD-1 Checkpoint Pathway. N. Engl. J. Med. 375 (18), 1767–1778. doi:10.1056/NEJMra1514296
Bousso, P. (2008). T-Cell Activation by Dendritic Cells in the Lymph Node: Lessons from the Movies. Nat. Rev. Immunol. 8 (9), 675–684. doi:10.1038/nri2379
Bruno, L., Kirberg, J., and von Boehmer, H. (1995). On the Cellular Basis of Immunological T Cell Memory. Immunity 2 (1), 37–43. doi:10.1016/1074-7613(95)90077-2
Bushati, N., and Cohen, S. M. (2007). MicroRNA Functions. Annu. Rev. Cell Dev. Biol. 23, 175–205. doi:10.1146/annurev.cellbio.23.090506.123406
Cai, Y., Yu, X., Hu, S., and Yu, J. (2009). A Brief Review on the Mechanisms of miRNA Regulation. Genomics Proteomics Bioinformatics 7 (4), 147–154. doi:10.1016/S1672-0229(08)60044-3
Carty, S. A., Gohil, M., Banks, L. B., Cotton, R. M., Johnson, M. E., Stelekati, E., et al. (2018). The Loss of TET2 Promotes CD8+ T Cell Memory Differentiation. J. Immunol. 200 (1), 82–91. doi:10.4049/jimmunol.1700559
Cedar, H. (1988). DNA Methylation and Gene Activity. Cell 53 (1), 3–4. doi:10.1016/0092-8674(88)90479-5
Chappell, C., Beard, C., Altman, J., Jaenisch, R., and Jacob, J. (2006). DNA Methylation by DNA Methyltransferase 1 Is Critical for Effector CD8 T Cell Expansion. J. Immunol. 176 (8), 4562–4572. doi:10.4049/jimmunol.176.8.4562
Chen, L., and Flies, D. B. (2013). Molecular Mechanisms of T Cell Co-Stimulation and Co-inhibition. Nat. Rev. Immunol. 13 (4), 227–242. doi:10.1038/nri3405
Cho, B. K., Wang, C., Sugawa, S., Eisen, H. N., and Chen, J. (1999). Functional Differences between Memory and Naive CD8 T Cells. Proc. Natl. Acad. Sci. 96 (6), 2976–2981. doi:10.1073/pnas.96.6.2976
Crespo, J., Sun, H., Welling, T. H., Tian, Z., and Zou, W. (2013). T Cell Anergy, Exhaustion, Senescence, and Stemness in the Tumor Microenvironment. Curr. Opin. Immunol. 25 (2), 214–221. doi:10.1016/j.coi.2012.12.003
Croft, M. (1994). Activation of Naive, Memory and Effector T Cells. Curr. Opin. Immunol. 6 (3), 431–437. doi:10.1016/0952-7915(94)90123-6
Crompton, J. G., Narayanan, M., Cuddapah, S., Roychoudhuri, R., Ji, Y., Yang, W., et al. (2016). Lineage Relationship of CD8+ T Cell Subsets is Revealed by Progressive Changes in the Epigenetic Landscape. Cell. Mol. Immunol. 13 (4), 502–513. doi:10.1038/cmi.2015.32
Cui, J., Li, Q., Luo, M., Zhong, Z., Zhou, S., Jiang, L., et al. (2018). Leukemia Cell-Derived Microvesicles Induce T Cell Exhaustion via miRNA Delivery. Oncoimmunology 7 (7), e1448330. doi:10.1080/2162402X.2018.1448330
Dai, G., Choi, C. K. K., Zhou, Y., Bai, Q., Xiao, Y., Yang, C., et al. (2021). Immobilising Hairpin DNA-Conjugated Distyryl boron Dipyrromethene on Gold@polydopamine Core-Shell Nanorods for microRNA Detection and microRNA-Mediated Photodynamic Therapy. Nanoscale 13 (13), 6499–6512. doi:10.1039/D0NR09135A
Davies, P. F., Manduchi, E., Stoeckert, C. J., Jiménez, J. M., and Jiang, Y.-Z. (2014). Emerging Topic: Flow-Related Epigenetic Regulation of Endothelial Phenotype through DNA Methylation. Vasc. Pharmacol. 62 (2), 88–93. doi:10.1016/j.vph.2014.05.007
Eden, A., Gaudet, F., Waghmare, A., and Jaenisch, R. (2003). Chromosomal Instability and Tumors Promoted by DNA Hypomethylation. Science 300 (5618), 455. doi:10.1126/science.1083557
Emming, S., Chirichella, M., and Monticelli, S. (2018). MicroRNAs as Modulators of T Cell Functions in Cancer. Cancer Lett. 430, 172–178. doi:10.1016/j.canlet.2018.05.019
Fann, M., Godlove, J. M., Catalfamo, M., Wood, W. H., Chrest, F. J., Chun, N., et al. (2006). Histone Acetylation is Associated with Differential Gene Expression in the Rapid and Robust Memory CD8+ T-Cell Response. Blood 108 (10), 3363–3370. doi:10.1182/blood-2006-02-005520
Freeman, G. J., Long, A. J., Iwai, Y., Bourque, K., Chernova, T., Nishimura, H., et al. (2000). Engagement of the PD-1 Immunoinhibitory Receptor by a Novel B7 Family Member Leads to Negative Regulation of Lymphocyte Activation. J. Exp. Med. 192 (7), 1027–1034. doi:10.1084/jem.192.7.1027
Frias, A. B., Boi, S. K., Lan, X., and Youngblood, B. (2021). Epigenetic Regulation of T Cell Adaptive Immunity. Immunol. Rev. 300 (1), 9–21. doi:10.1111/imr.12943
Gattinoni, L., Klebanoff, C. A., and Restifo, N. P. (2012). Paths to Stemness: Building the Ultimate Antitumour T Cell. Nat. Rev. Cancer 12 (10), 671–684. doi:10.1038/nrc3322
Gattinoni, L., Lugli, E., Ji, Y., Pos, Z., Paulos, C. M., Quigley, M. F., et al. (2011). A Human Memory T Cell Subset with Stem Cell-Like Properties. Nat. Med. 17 (10), 1290–1297. doi:10.1038/nm.2446
Ghoneim, H. E., Fan, Y., Moustaki, A., Abdelsamed, H. A., Dash, P., Dogra, P., et al. (2017). De Novo Epigenetic Programs Inhibit PD-1 Blockade-Mediated T Cell Rejuvenation. Cell 170 (1), 142–157.e19. doi:10.1016/j.cell.2017.06.007
Gravina, G. L., Festuccia, C., Marampon, F., Popov, V. M., Pestell, R. G., Zani, B. M., et al. (2010). Biological Rationale for the Use of DNA Methyltransferase Inhibitors as New Strategy for Modulation of Tumor Response to Chemotherapy and Radiation. Mol. Cancer 9, 305. doi:10.1186/1476-4598-9-305
Grenningloh, R., Tai, T.-S., Frahm, N., Hongo, T. C., Chicoine, A. T., Brander, C., et al. (2011). Ets-1 Maintains IL-7 Receptor Expression in Peripheral T Cells. J. Immunol. 186 (2), 969–976. doi:10.4049/jimmunol.1002099
Ha, M., and Kim, V. N. (2014). Regulation of microRNA Biogenesis. Nat. Rev. Mol. Cel Biol. 15 (8), 509–524. doi:10.1038/nrm3838
Hamid, O., Robert, C., Daud, A., Hodi, F. S., Hwu, W.-J., Kefford, R., et al. (2013). Safety and Tumor Responses with Lambrolizumab (Anti-PD-1) in Melanoma. N. Engl. J. Med. 369 (2), 134–144. doi:10.1056/NEJMoa1305133
Hanna, J., Hossain, G. S., and Kocerha, J. (2019). The Potential for microRNA Therapeutics and Clinical Research. Front. Genet. 10, 478. doi:10.3389/fgene.2019.00478
Harty, J. T., Tvinnereim, A. R., and White, D. W. (2000). CD8+ T Cell Effector Mechanisms in Resistance to Infection. Annu. Rev. Immunol. 18 (1), 275–308. doi:10.1146/annurev.immunol.18.1.275
Henning, A. N., Klebanoff, C. A., and Restifo, N. P. (2018a). Silencing Stemness in T Cell Differentiation. Science 359 (6372), 163–164. doi:10.1126/science.aar5541
Henning, A. N., Roychoudhuri, R., and Restifo, N. P. (2018b). Epigenetic Control of CD8+ T Cell Differentiation. Nat. Rev. Immunol. 18 (5), 340–356. doi:10.1038/nri.2017.146
Hiam-Galvez, K. J., Allen, B. M., and Spitzer, M. H. (2021). Systemic Immunity in Cancer. Nat. Rev. Cancer 21 (6), 345–359. doi:10.1038/s41568-021-00347-z
Hopkins, A. L. (2008). Network Pharmacology: The Next Paradigm in Drug Discovery. Nat. Chem. Biol. 4 (11), 682–690. doi:10.1038/nchembio.118
Hou, X., Zaks, T., Langer, R., and Dong, Y. (2021). Lipid Nanoparticles for mRNA Delivery. Nat. Rev. Mater. 6, 1078–1094. doi:10.1038/s41578-021-00358-0
Hu, C., Liu, X., Zeng, Y., Liu, J., and Wu, F. (2021). DNA Methyltransferase Inhibitors Combination Therapy for the Treatment of Solid Tumor: Mechanism and Clinical Application. Clin. Epigenet 13, 166. doi:10.1186/s13148-021-01154-x
Huster, K. M., Koffler, M., Stemberger, C., Schiemann, M., Wagner, H., and Busch, D. H. (2006). Unidirectional Development of CD8+ central Memory T Cells into protectiveListeria-specific Effector Memory T Cells. Eur. J. Immunol. 36 (6), 1453–1464. doi:10.1002/eji.200635874
Im, S. J., Hashimoto, M., Gerner, M. Y., Lee, J., Kissick, H. T., Burger, M. C., et al. (2016). Defining CD8+ T Cells that Provide the Proliferative Burst after PD-1 Therapy. Nature 537 (7620), 417–421. doi:10.1038/nature19330
Iwai, Y., Terawaki, S., and Honjo, T. (2005). PD-1 Blockade Inhibits Hematogenous Spread of Poorly Immunogenic Tumor Cells by Enhanced Recruitment of Effector T Cells. Int. Immunol. 17 (2), 133–144. doi:10.1093/intimm/dxh194
Jadhav, R. R., Im, S. J., Hu, B., Hashimoto, M., Li, P., Lin, J.-X., et al. (2019). Epigenetic Signature of PD-1+ TCF1+ CD8 T Cells that Act as Resource Cells during Chronic Viral Infection and Respond to PD-1 Blockade. Proc. Natl. Acad. Sci. U.S.A. 116 (28), 14113–14118. doi:10.1073/pnas.1903520116
Jeannet, G., Boudousquie, C., Gardiol, N., Kang, J., Huelsken, J., and Held, W. (2010). Essential Role of the Wnt Pathway Effector Tcf-1 for the Establishment of Functional CD8 T Cell Memory. Proc. Natl. Acad. Sci. 107 (21), 9777–9782. doi:10.1073/pnas.0914127107
John, B., Enright, A. J., Aravin, A., Tuschl, T., Sander, C., and Marks, D. S. (2004). Human MicroRNA Targets. PLoS Biol. 2 (11), e363. doi:10.1371/journal.pbio.0020363
Joshi, N. S., and Kaech, S. M. (2008). Effector CD8 T Cell Development: A Balancing Act between Memory Cell Potential and Terminal Differentiation. J. Immunol. 180 (3), 1309–1315. doi:10.4049/jimmunol.180.3.1309
Kelly, J., Spolski, R., Imada, K., Bollenbacher, J., Lee, S., and Leonard, W. J. (2003). A Role for Stat5 in CD8+ T Cell Homeostasis. J. Immunol. 170 (1), 210–217. doi:10.4049/jimmunol.170.1.210
Keshet, I., Lieman-Hurwitz, J., and Cedar, H. (1986). DNA Methylation Affects the Formation of Active Chromatin. Cell 44 (4), 535–543. doi:10.1016/0092-8674(86)90263-1
Khan, O., Giles, J. R., McDonald, S., Manne, S., Ngiow, S. F., Patel, K. P., et al. (2019). TOX Transcriptionally and Epigenetically Programs CD8+ T Cell Exhaustion. Nature 571 (7764), 211–218. doi:10.1038/s41586-019-1325-x
Klein-Hessling, S., Muhammad, K., Klein, M., Pusch, T., Rudolf, R., Flöter, J., et al. (2017). NFATc1 Controls the Cytotoxicity of CD8+ T Cells. Nat. Commun. 8 (1), 511. doi:10.1038/s41467-017-00612-6
Klemm, S. L., Shipony, Z., and Greenleaf, W. J. (2019). Chromatin Accessibility and the Regulatory Epigenome. Nat. Rev. Genet. 20 (4), 207–220. doi:10.1038/s41576-018-0089-8
Ladle, B. H., Li, K.-P., Phillips, M. J., Pucsek, A. B., Haile, A., Powell, J. D., et al. (2016). De Novo DNA Methylation by DNA Methyltransferase 3a Controls Early Effector CD8+ T-Cell Fate Decisions Following Activation. Proc. Natl. Acad. Sci. U.S.A. 113 (38), 10631–10636. doi:10.1073/pnas.1524490113
Lawrence, M., Daujat, S., and Schneider, R. (2016). Lateral Thinking: How Histone Modifications Regulate Gene Expression. Trends Genet. 32 (1), 42–56. doi:10.1016/j.tig.2015.10.007
Leach, D. R., Krummel, M. F., and Allison, J. P. (1996). Enhancement of Antitumor Immunity by CTLA-4 Blockade. Science 271 (5256), 1734–1736. doi:10.1126/science.271.5256.1734
Lee, D. Y., Choi, B. K., Lee, D. G., Kim, Y. H., Kim, C. H., Lee, S. J., et al. (2013). 4-1BB Signaling Activates the T Cell Factor 1 Effector/β-Catenin Pathway with Delayed Kinetics via ERK Signaling and Delayed PI3K/AKT Activation to Promote the Proliferation of CD8+ T Cells. PLoS One 8 (7), e69677. doi:10.1371/journal.pone.0069677
Lee, M., Li, J., Li, J., Fang, S., Zhang, J., Vo, A. T. T., et al. (2021). Tet2 Inactivation Enhances the Antitumor Activity of Tumor-Infiltrating Lymphocytes. Cancer Res. 81 (8), 1965–1976. doi:10.1158/0008-5472.Can-20-3213
Li, J., Jiang, X., and Wang, K. (2019). Exosomal miRNA: An Alternative Mediator of Cell-to-Cell Communication. ExRNA 1 (1), 31. doi:10.1186/s41544-019-0025-x
Li, Q., Eppolito, C., Odunsi, K., and Shrikant, P. A. (2010). Antigen-induced Erk1/2 Activation Regulates Ets-1-Mediated Sensitization of CD8+ T Cells for IL-12 Responses. J. Leukoc. Biol. 87 (2), 257–263. doi:10.1189/jlb.0409221
Li, Q., Johnston, N., Zheng, X., Wang, H., Zhang, X., Gao, D., et al. (2016). miR-28 Modulates Exhaustive Differentiation of T Cells Through Silencing Programmed Cell Death-1 and Regulating Cytokine Secretion. Oncotarget 7 (33), 53735–53750. doi:10.18632/oncotarget.10731
Liang, Y., Pan, H.-F., and Ye, D.-Q. (2015). microRNAs Function in CD8+ T Cell Biology. J. Leukoc. Biol. 97 (3), 487–497. doi:10.1189/jlb.1ru0814-369r
Liu, M., Gao, W., van Velkinburgh, J. C., Wu, Y., Ni, B., and Tian, Y. (2016). Role of Ets Proteins in Development, Differentiation, and Function of T-Cell Subsets. Med. Res. Rev. 36 (2), 193–220. doi:10.1002/med.21361
Liu, Y., Blanchfield, L., Ma, V. P.-Y., Andargachew, R., Galior, K., Liu, Z., et al. (2016). DNA-Based Nanoparticle Tension Sensors Reveal that T-Cell Receptors Transmit Defined pN Forces to Their Antigens for Enhanced Fidelity. Proc. Natl. Acad. Sci. U.S.A. 113 (20), 5610–5615. doi:10.1073/pnas.1600163113
Macian, F. (2005). NFAT Proteins: Key Regulators of T-Cell Development and Function. Nat. Rev. Immunol. 5 (6), 472–484. doi:10.1038/nri1632
Margueron, R., and Reinberg, D. (2011). The Polycomb Complex PRC2 and its Mark in Life. Nature 469 (7330), 343–349. doi:10.1038/nature09784
Martinez, G. J., Pereira, R. M., Äijö, T., Kim, E. Y., Marangoni, F., Pipkin, M. E., et al. (2015). The Transcription Factor NFAT Promotes Exhaustion of Activated CD8 + T Cells. Immunity 42 (2), 265–278. doi:10.1016/j.immuni.2015.01.006
Mempel, T. R., Henrickson, S. E., and von Andrian, U. H. (2004). T-Cell Priming by Dendritic Cells in Lymph Nodes Occurs in Three Distinct Phases. Nature 427 (6970), 154–159. doi:10.1038/nature02238
Milner, J. J., Toma, C., Yu, B., Zhang, K., Omilusik, K., Phan, A. T., et al. (2017). Runx3 Programs CD8+ T Cell Residency in Non-Lymphoid Tissues and Tumours. Nature 552 (7684), 253–257. doi:10.1038/nature24993
Moore, L. D., Le, T., and Fan, G. (2013). DNA Methylation and its Basic Function. Neuropsychopharmacol 38, 23–38. doi:10.1038/npp.2012.112
Muthusamy, N., Barton, K., and Leiden, J. M. (1995). Defective Activation and Survival of T Cells Lacking the Ets-1 Transcription Factor. Nature 377 (6550), 639–642. doi:10.1038/377639a0
Öberg, C., Li, J., Pauley, A., Wolf, E., Gurney, M., and Lendahl, U. (2001). The Notch Intracellular Domain is Ubiquitinated and Negatively Regulated by the Mammalian Sel-10 Homolog. J. Biol. Chem. 276 (38), 35847–35853. doi:10.1074/jbc.M103992200
Ozga, A. J., Moalli, F., Abe, J., Swoger, J., Sharpe, J., Zehn, D., et al. (2016). pMHC Affinity Controls Duration of CD8+ T Cell-DC Interactions and Imprints Timing of Effector Differentiation Versus Expansion. J. Exp. Med. 213 (12), 2811–2829. doi:10.1084/jem.20160206
Pace, L., and Amigorena, S. (2020). Epigenetics of T Cell Fate Decision. Curr. Opin. Immunol. 63, 43–50. doi:10.1016/j.coi.2020.01.002
Philip, M., Fairchild, L., Sun, L., Horste, E. L., Camara, S., Shakiba, M., et al. (2017). Chromatin States Define Tumour-specific T Cell Dysfunction and Reprogramming. Nature 545 (7655), 452–456. doi:10.1038/nature22367
Robert, C., Ribas, A., Wolchok, J. D., Hodi, F. S., Hamid, O., Kefford, R., et al. (2014). Anti-Programmed-Death-Receptor-1 Treatment with Pembrolizumab in Ipilimumab-Refractory Advanced Melanoma: a Randomised Dose-Comparison Cohort of a Phase 1 Trial. The Lancet 384 (9948), 1109–1117. doi:10.1016/S0140-6736(14)60958-2
Robertson, K. D. (2005). DNA Methylation and Human Disease. Nat. Rev. Genet. 6 (8), 597–610. doi:10.1038/nrg1655
Rodríguez-Galán, A., Fernández-Messina, L., and Sánchez-Madrid, F. (2018). Control of Immunoregulatory Molecules by miRNAs in T Cell Activation. Front. Immunol. 9, 2148. doi:10.3389/fimmu.2018.02148
Rothenberg, E. V., Ungerbäck, J., and Champhekar, A. (2016). Forging T-Lymphocyte Identity. Adv. Immunol. 129, 109–174. doi:10.1016/bs.ai.2015.09.002
Ruddle, N. H., and Akirav, E. M. (2009). Secondary Lymphoid Organs: Responding to Genetic and Environmental Cues in Ontogeny and the Immune Response. J. Immunol. 183 (4), 2205–2212. doi:10.4049/jimmunol.0804324
Russell, L., and Garrett-Sinha, L. A. (2010). Transcription Factor Ets-1 in Cytokine and Chemokine Gene Regulation. Cytokine 51 (3), 217–226. doi:10.1016/j.cyto.2010.03.006
Saki, N., Abroun, S., Soleimani, M., Hajizamani, S., Shahjahani, M., Kast, R. E., et al. (2015). Involvement of MicroRNA in T-Cell Differentiation and Malignancy. Int. J. Hematol. Oncol. Stem Cell Res. 9 (1), 33–49.
Sallusto, F., Geginat, J., and Lanzavecchia, A. (2004). Central Memory and Effector Memory T Cell Subsets: Function, Generation, and Maintenance. Annu. Rev. Immunol. 22 (1), 745–763. doi:10.1146/annurev.immunol.22.012703.104702
Sallusto, F., Lenig, D., Förster, R., Lipp, M., and Lanzavecchia, A. (1999). Two Subsets of Memory T Lymphocytes with Distinct Homing Potentials and Effector Functions. Nature 401 (6754), 708–712. doi:10.1038/44385
Sander, S., Bullinger, L., Klapproth, K., Fiedler, K., Kestler, H. A., Barth, T. F. E., et al. (2008). MYC Stimulates EZH2 Expression by Repression of its Negative Regulator miR-26a. Blood 112 (10), 4202–4212. doi:10.1182/blood-2008-03-147645
Sato, T., Ohno, S.-i., Hayashi, T., Sato, C., Kohu, K., Satake, M., et al. (2005). Dual Functions of Runx Proteins for Reactivating CD8 and Silencing CD4 at the Commitment Process into CD8 Thymocytes. Immunity 22 (3), 317–328. doi:10.1016/j.immuni.2005.01.012
Scharer, C. D., Bally, A. P. R., Gandham, B., and Boss, J. M. (2017). Cutting Edge: Chromatin Accessibility Programs CD8 T Cell Memory. J. Immunol. 198 (6), 2238–2243. doi:10.4049/jimmunol.1602086
Schmid, D., Park, C. G., Hartl, C. A., Subedi, N., Cartwright, A. N., Puerto, R. B., et al. (2017). T Cell-Targeting Nanoparticles Focus Delivery of Immunotherapy to Improve Antitumor Immunity. Nat. Commun. 8 (1), 1747. doi:10.1038/s41467-017-01830-8
Schultz, D. C., Ayyanathan, K., Negorev, D., Maul, G. G., and Rauscher, F. J. (2002). SETDB1: a Novel KAP-1-Associated Histone H3, Lysine 9-Specific Methyltransferase that Contributes to HP1-Mediated Silencing of Euchromatic Genes by KRAB Zinc-finger Proteins. Genes Dev. 16 (8), 919–932. doi:10.1101/gad.973302
Shan, Q., Zeng, Z., Xing, S., Li, F., Hartwig, S. M., Gullicksrud, J. A., et al. (2017). The Transcription Factor Runx3 Guards Cytotoxic CD8+ Effector T Cells against Deviation Towards Follicular Helper T Cell Lineage. Nat. Immunol. 18 (8), 931–939. doi:10.1038/ni.3773
Smith, Z. D., and Meissner, A. (2013). DNA Methylation: Roles in Mammalian Development. Nat. Rev. Genet. 14 (3), 204–220. doi:10.1038/nrg3354
Sun, M., Ha, N., Pham, D.-H., Frederick, M., Sharma, B., Naruse, C., et al. (2017). Cbx3/HP1γ Deficiency Confers Enhanced Tumor-Killing Capacity on CD8+ T Cells. Sci. Rep. 7 (1), 42888. doi:10.1038/srep42888
Takikita, S., Muro, R., Takai, T., Otsubo, T., Kawamura, Y. I., Dohi, T., et al. (2016). A Histone Methyltransferase ESET is Critical for T Cell Development. J. Immunol. 197 (6), 2269–2279. doi:10.4049/jimmunol.1502486
Tripathi, P., Kurtulus, S., Wojciechowski, S., Sholl, A., Hoebe, K., Morris, S. C., et al. (2010). STAT5 is Critical to Maintain Effector CD8+T Cell Responses. J. Immunol. 185 (4), 2116–2124. doi:10.4049/jimmunol.1000842
Tumeh, P. C., Harview, C. L., Yearley, J. H., Shintaku, I. P., Taylor, E. J. M., Robert, L., et al. (2014). PD-1 Blockade Induces Responses by Inhibiting Adaptive Immune Resistance. Nature 515 (7528), 568–571. doi:10.1038/nature13954
van der Woude, L. L., Gorris, M. A. J., Halilovic, A., Figdor, C. G., and de Vries, I. J. M. (2017). Migrating into the Tumor: A Roadmap for T Cells. Trends Cancer 3 (11), 797–808. doi:10.1016/j.trecan.2017.09.006
Varambally, S., Cao, Q., Mani, R.-S., Shankar, S., Wang, X., Ateeq, B., et al. (2008). Genomic Loss of microRNA-101 Leads to Overexpression of Histone Methyltransferase EZH2 in Cancer. Science 322 (5908), 1695–1699. doi:10.1126/science.1165395
Veiga-Fernandes, H., Walter, U., Bourgeois, C., McLean, A., and Rocha, B. (2000). Response of Naïve and Memory CD8+ T Cells to Antigen Stimulation In Vivo. Nat. Immunol. 1 (1), 47–53. doi:10.1038/76907
Vignard, V., Labbé, M., Marec, N., André-Grégoire, G., Jouand, N., Fonteneau, J.-F., et al. (2020). MicroRNAs in Tumor Exosomes Drive Immune Escape in Melanoma. Cancer Immunol. Res. 8 (2), 255–267. doi:10.1158/2326-6066.CIR-19-0522
Villarino, A. V., Kanno, Y., and O’Shea, J. J. (2017). Mechanisms and Consequences of Jak-STAT Signaling in the Immune System. Nat. Immunol. 18 (4), 374–384. doi:10.1038/ni.3691
Wirtz-Peitz, F., Nishimura, T., and Knoblich, J. A. (2008). Linking Cell Cycle to Asymmetric Division: Aurora-A Phosphorylates the Par Complex to Regulate Numb Localization. Cell 135 (1), 161–173. doi:10.1016/j.cell.2008.07.049
Wolf, Y., Anderson, A. C., and Kuchroo, V. K. (2020). TIM3 Comes of Age as an Inhibitory Receptor. Nat. Rev. Immunol. 20 (3), 173–185. doi:10.1038/s41577-019-0224-6
Wolint, P., Betts, M. R., Koup, R. A., and Oxenius, A. (2004). Immediate Cytotoxicity but Not Degranulation Distinguishes Effector and Memory Subsets of CD8+ T Cells. J. Exp. Med. 199 (7), 925–936. doi:10.1084/jem.20031799
Wong, S. H. D., Xu, X., Chen, X., Xin, Y., Xu, L., Lai, C. H. N., et al. (2021). Manipulation of the Nanoscale Presentation of Integrin Ligand Produces Cancer Cells with Enhanced Stemness and Robust Tumorigenicity. Nano Lett. 21 (7), 3225–3236. doi:10.1021/acs.nanolett.1c00501
Wong, W. K., Wong, S. H. D., and Bian, L. (2020). Long-Term Detection of Oncogenic MicroRNA in Living Human Cancer Cells by Gold@ Polydopamine-Shell Nanoprobe. ACS Biomater. Sci. Eng. 6 (7), 3778–3783. doi:10.1021/acsbiomaterials.0c00633
Wong, W. K., Yin, B., Rakhmatullina, A., Zhou, J., and Wong, S. H. D. (2021). Engineering Advanced Dynamic Biomaterials to Optimize Adoptive T-Cell Immunotherapy. Engineered Regen. 2, 70–81. doi:10.1016/j.engreg.2021.06.001
Xiao, D., Ohlendorf, J., Chen, Y., Taylor, D. D., Rai, S. N., Waigel, S., et al. (2012). Identifying mRNA, MicroRNA and Protein Profiles of Melanoma Exosomes. PLoS One 7 (10), e46874. doi:10.1371/journal.pone.0046874
Xu, F., Liu, J., Liu, D., Liu, B., Wang, M., Hu, Z., et al. (2014). LSECtin Expressed on Melanoma Cells Promotes Tumor Progression by Inhibiting Antitumor T-Cell Responses. Cancer Res. 74 (13), 3418–3428. doi:10.1158/0008-5472.CAN-13-2690
Yang, L., Xia, L., Wu, D. Y., Wang, H., Chansky, H. A., Schubach, W. H., et al. (2002). Molecular Cloning of ESET, a Novel Histone H3-specific Methyltransferase that Interacts with ERG Transcription Factor. Oncogene 21 (1), 148–152. doi:10.1038/sj.onc.1204998
Yang, R., Cheng, S., Luo, N., Gao, R., Yu, K., Kang, B., et al. (2019). Distinct Epigenetic Features of Tumor-Reactive CD8+ T Cells in Colorectal Cancer Patients Revealed by Genome-wide DNA Methylation Analysis. Genome Biol. 21, 2. doi:10.1186/s13059-019-1921-y
Yang, X., Han, H., De Carvalho, D. D., Lay, F. D., Jones, P. A., and Liang, G. (2014). Gene Body Methylation Can Alter Gene Expression and is a Therapeutic Target in Cancer. Cancer Cell 26 (4), 577–590. doi:10.1016/j.ccr.2014.07.028
Yao, Q., Chen, Y., and Zhou, X. (2019). The Roles of microRNAs in Epigenetic Regulation. Curr. Opin. Chem. Biol. 51, 11–17. doi:10.1016/j.cbpa.2019.01.024
Ye, S.-b., Li, Z.-L., Luo, D.-h., Huang, B.-j., Chen, Y.-S., Zhang, X.-s., et al. (2014). Tumor-Derived Exosomes Promote Tumor Progression and T-Cell Dysfunction through the Regulation of Enriched Exosomal microRNAs in Human Nasopharyngeal Carcinoma. Oncotarget 5 (14), 5439–5452. doi:10.18632/oncotarget.2118
Yee, C., Thompson, J. A., Byrd, D., Riddell, S. R., Roche, P., Celis, E., et al. (2002). Adoptive T Cell Therapy Using Antigen-specific CD8+ T Cell Clones for the Treatment of Patients with Metastatic Melanoma: In Vivo Persistence, Migration, and Antitumor Effect of Transferred T Cells. Proc. Natl. Acad. Sci. 99 (25), 16168–16173. doi:10.1073/pnas.242600099
Yi, M., Xu, L., Jiao, Y., Luo, S., Li, A., and Wu, K. (2020). The Role of Cancer-Derived microRNAs in Cancer Immune Escape. J. Hematol. Oncol. 13, 25. doi:10.1186/s13045-020-00848-8
Yu, T., Zuo, Q.-F., Gong, L., Wang, L.-N., Zou, Q.-M., and Xiao, B. (2016). MicroRNA-491 Regulates the Proliferation and Apoptosis of CD8+ T Cells. Sci. Rep. 6, 30923. doi:10.1038/srep30923
Yumimoto, K., Matsumoto, M., Onoyama, I., Imaizumi, K., and Nakayama, K. I. (2013). F-box and WD Repeat Domain-Containing-7 (Fbxw7) Protein Targets Endoplasmic Reticulum-Anchored Osteogenic and Chondrogenic Transcriptional Factors for Degradation. J. Biol. Chem. 288 (40), 28488–28502. doi:10.1074/jbc.M113.465179
Zamisch, M., Tian, L., Grenningloh, R., Xiong, Y., Wildt, K. F., Ehlers, M., et al. (2009). The Transcription Factor Ets1 is Important for CD4 Repression and Runx3 Up-Regulation during CD8 T Cell Differentiation in the Thymus. J. Exp. Med. 206 (12), 2685–2699. doi:10.1084/jem.20092024
Zhao, E., Maj, T., Kryczek, I., Li, W., Wu, K., Zhao, L., et al. (2016). Cancer Mediates Effector T Cell Dysfunction by Targeting microRNAs and EZH2 via Glycolysis Restriction. Nat. Immunol. 17 (1), 95–103. doi:10.1038/ni.3313
Zhou, X., Yu, S., Zhao, D.-M., Harty, J. T., Badovinac, V. P., and Xue, H.-H. (2010). Differentiation and Persistence of Memory CD8+ T Cells Depend on T Cell Factor 1. Immunity 33 (2), 229–240. doi:10.1016/j.immuni.2010.08.002
Glossary
Bcl-xL B-cell lymphoma-extra large
Cbx3/HP1γ Chromobox homolog 3/heterochromatin protein 1 gamma
CCR7 CC-chemokine receptor 7
CDK4 Cyclin-dependent kinase 4
CTLA-4 Cytotoxic T-lymphocyte-associated antigen 4
CXCR5 C-X-C Motif Chemokine Receptor 5
DCs Dendritic cells
DNMT DNA methyltransferase
ETS E26 transformation-specific
EVs Extracellular vesicles
EZH2 Enhancer of zeste homolog 2
FBXW7 F-Box and WD Repeat Domain Containing 7
Foxo1 Fork-head box O1
GZMB Granzyme B (encoded by Gzmb)
H3K4me3 Trimethylation of lysine 4 on histone H3
H3K9ac Acetylation of lysine 9 on histone H3
H3K9me2 Dimethylation of lysine 9 on histone H3
H3K9me3 Trimethylation of lysine 9 on histone H3
H3K27me2 Dimethylation of lysine 27 on histone H3
H3K27me3 Trimethylation of lysine 27 on histone H3
H3K79me2 Dimethylation of lysine 79 on histone H3
IFNγ Interferon-gamma (encoded by Ifng)
IL-2 Interleukin-2 (encoded by IL2)
IL-15 Interleukin-15
LAG-3 Lymphocyte-activation gene 3
LCMV Lymphocytic choriomeningitis virus
NFAT Nuclear factor of activated T-cells (NFAT2 is encoded by Nfatc1)
NKG2D Natural killer group 2 member D
KLRG1 Killer Cell Lectin Like Receptor G1
PD-1 Programmed Cell Death Protein 1
PD-L1 Programmed death-ligand 1
pMHC Peptide-major histocompatibility complex
PRC2 Polycomb repressive complex 2
Runx3 Runt-related transcription factor 3TM cells: Memory T cells
TN cells Naïve T cells
TSCM cells Memory stem T cells
TCM cells Central memory T cells
TEM cells Effector memory T cells
TE cells Effector T cells
Tbx21 T-box transcription factor 21 coding gene
TCF1 T cell factor 1 (encoded by Tcf7)
TCR T cell receptor
TET2 Ten-eleven translocation-2
TGFβ Transforming growth factor beta
TILs Tumor infiltrating lymphocytes
Tim-3 T cell immunoglobulin and mucin domain-containing protein-3
TME Tumor microenvironment
TNFα Tumor necrosis factor-alpha
TOX Thymocyte Selection Associated High Mobility Group Box (encoded by Tox)
Keywords: T-cell differentiation, T-cell exhaustion, epigenetic regulation, adoptive immunotherapy, T-cell activation
Citation: Wong WK, Yin B, Lam CYK, Huang Y, Yan J, Tan Z and Wong SHD (2022) The Interplay Between Epigenetic Regulation and CD8+ T Cell Differentiation/Exhaustion for T Cell Immunotherapy. Front. Cell Dev. Biol. 9:783227. doi: 10.3389/fcell.2021.783227
Received: 25 September 2021; Accepted: 20 December 2021;
Published: 11 January 2022.
Edited by:
Jorg Tost, Commissariat à l’Energie Atomique et aux Energies Alternatives, FranceCopyright © 2022 Wong, Yin, Lam, Huang, Yan, Tan and Wong. This is an open-access article distributed under the terms of the Creative Commons Attribution License (CC BY). The use, distribution or reproduction in other forums is permitted, provided the original author(s) and the copyright owner(s) are credited and that the original publication in this journal is cited, in accordance with accepted academic practice. No use, distribution or reproduction is permitted which does not comply with these terms.
*Correspondence: Zhiwu Tan, end0YW5AaGt1Lmhr; Siu Hong Dexter Wong, c2hvbmd3b25nQHBvbHl1LmVkdS5oaw==
†These authors have contributed equally to this work