- 1Department of Pharmacy, Nanjing First Hospital, Nanjing Medical University, Nanjing, China
- 2Department of Clinical Pharmacy, School of Basic Medicine & Clinical Pharmacy, China Pharmaceutical University, Nanjing, China
- 3Key Laboratory of Drug Metabolism and Pharmacokinetics, State Key Laboratory of Natural Medicines, China Pharmaceutical University, Nanjing, China
- 4Department of Clinical Pharmacology, Nanjing First Hospital, Nanjing Medical University, Nanjing, China
- 5Nanjing Southern Pharmaceutical Technology Co., Ltd., Nanjing, China
Ferroptosis, a newly discovered form of regulatory cell death (RCD), has been demonstrated to be distinct from other types of RCD, such as apoptosis, necroptosis, and autophagy. Ferroptosis is characterized by iron-dependent lipid peroxidation and oxidative perturbation, and is inhibited by iron chelators and lipophilic antioxidants. This process is regulated by specific pathways and is implicated in diverse biological contexts, mainly including iron homeostasis, lipid metabolism, and glutathione metabolism. A large body of evidence suggests that ferroptosis is interrelated with various physiological and pathological processes, including tumor progression (neuro)degenerative diseases, and hepatic and renal failure. There is an urgent need for the discovery of novel effective ferroptosis-modulating compounds, even though some experimental reagents and approved clinical drugs have been well documented to have anti- or pro-ferroptotic properties. This review outlines recent advances in molecular mechanisms of the ferroptotic death process and discusses its multiple roles in diverse pathophysiological contexts. Furthermore, we summarize chemical compounds and natural products, that act as inducers or inhibitors of ferroptosis in the prevention and treatment of various diseases. Herein, it is particularly highlighted that natural products show promising prospects in ferroptosis-associated (adjuvant) therapy with unique advantages of having multiple components, multiple biotargets and slight side effects.
Introduction
Along with other biological processes, cell death is of great significance in various molecular physiological processes of mammalian development, homeostasis and disease (Thompson, 1995; Fuchs and Steller, 2011). Generally, cell death can be divided into “accidental cell death” (ACD) and “regulatory cell death” (RCD). ACD is the actual structural collapse of cells when exposed to extreme physicochemical or mechanical stimuli. However, RCD is initiated by a genetically encoded apparatus and can be altered by pharmacologic or genetic interventions. RCD plays an integral role throughout the normal development and lifespan of humans, eliminating irreversibly impaired, dysfunctional or infected cells to protect the tissue or organ system from environmental injury or infection. Based on different biochemical phenomena, RCD is identified as several subtypes, including apoptosis, necrosis, autophagy and so on (Galluzzi et al., 2015). In 2003, Dolma et al. discovered a non-apoptotic cell death process when exploring the selective toxicity of erastin (a small molecule) in cancer cells (Dolma et al., 2003). In 2007, Yagoda et al. determined mitochondrial voltage-dependent anion channels 2 and 3 (VDAC2/3) as specific targets of erastin and VDAC2/3 also induced a non-apoptotic cell death, which depends on the RAS-RAF-MEK pathway (Yagoda et al., 2007). In 2008, Yang and Stockwell et al. identified two compounds, RSL3 and RSL5, via a high-throughput method that selectively induced iron-dependent, non-apoptotic cell death in cancer cells with mutated oncogenic RAS subtype genes (Yang and Stockwell, 2008). In 2012, Dr. Brent R Stockwell et al. treated the human fibrosarcoma cell line HT-1080 with erastin and found that the subunit of the amino acid cysteine transporter on the cell surface was suppressed. Restriction of cystine import, glutathione (GSH) depletion and phospholipid peroxidase glutathione peroxidase 4 (GPX4) inactivation could result in cell death. Thus, the term “ferroptosis” was formally coined (Dixon et al., 2012). As numerous studies have reported, ferroptosis usually refers to a novel iron-dependent, lipid peroxidation-driven RCD, that is obviously distinct from apoptosis, necrosis and autophagy at the morphological, biochemical, and genetic levels (Xie et al., 2016a; Stockwell et al., 2017). For example, morphological features during ferroptosis include smaller mitochondria, increased membrane density, crest density and decrease/disappearance, plasma membrane blistering, and increased cytoplasmic and lipid peroxidation. Ferroptosis is not limited to neoplastic diseases, and has been mostly linked to other iron- or redox-associated pathophysiological conditions, such as neurodegeneration, ischemia/reperfusion (I/R) injury, diabetes, and immune dysfunction (Skouta et al., 2014). A large number of studies have demonstrated that ferroptosis plays an indispensable role in the occurrence, development and phenotypic shift of a variety of diseases, which may provide new diagnostic and therapeutic strategies to regulate cell survival and death. Hence, it is instructive to discover novel compounds and drugs regulating ferroptosis to expand the development of potential therapeutic approaches. In recent decades, many clinical drugs and experimental compounds have been exploited to modulate ferroptosis by preclinical and clinical studies so as to achieve therapeutic purposes. As studies have progressed, natural products have attracted sufficient attention. Natural products and their analogs, including traditional Chinese medicines (TCMs) are unneglectable and conventional sources for the discovery and development of modern drugs. Due to their natural origin and potential beneficial efficacy, natural products and herbal medicines have been used globally to promote human health concerns for thousands of years, especially for the therapy of many chronic diseases, such as cancer, cardiovascular and (neuro)degenerative diseases (Shu-Feng Zhou et al., 2007; Kibble et al., 2015). Emerging evidence has demonstrated solid ferroptotic bioactivities in natural compounds and herbal medicines. In this review, we discuss emerging molecular mechanisms and biological processes of ferroptosis and its role in various diseases. We summarize synthetic compounds and natural products with anti- or pro-ferroptotic properties, and the great potential of natural products for ferroptosis-associated (adjuvant) therapy is highlighted. Overall, the underlying mechanisms of ferroptosis and corresponding pharmacological agents can provide insights into the discovery and development of promising therapeutic strategies in terms of ferroptosis.
Regulatory Networks and Signaling Pathways Associated With Ferroptosis
In recent years, the regulatory and metabolic mechanisms involved in ferroptosis have been emphatically elucidated and summarized by numerous original articles and reviews in detail. Hence, in this section, we briefly introduce the modulatory mechanisms and biological processes of ferroptosis, mainly including iron homeostasis, lipid metabolism, glutathione metabolism and other pathways, and illustrate them in Figure 1. Understanding the molecular mechanisms and signaling pathways of ferroptosis may open up new therapeutic opportunities to regulate cell fate in human diseases.
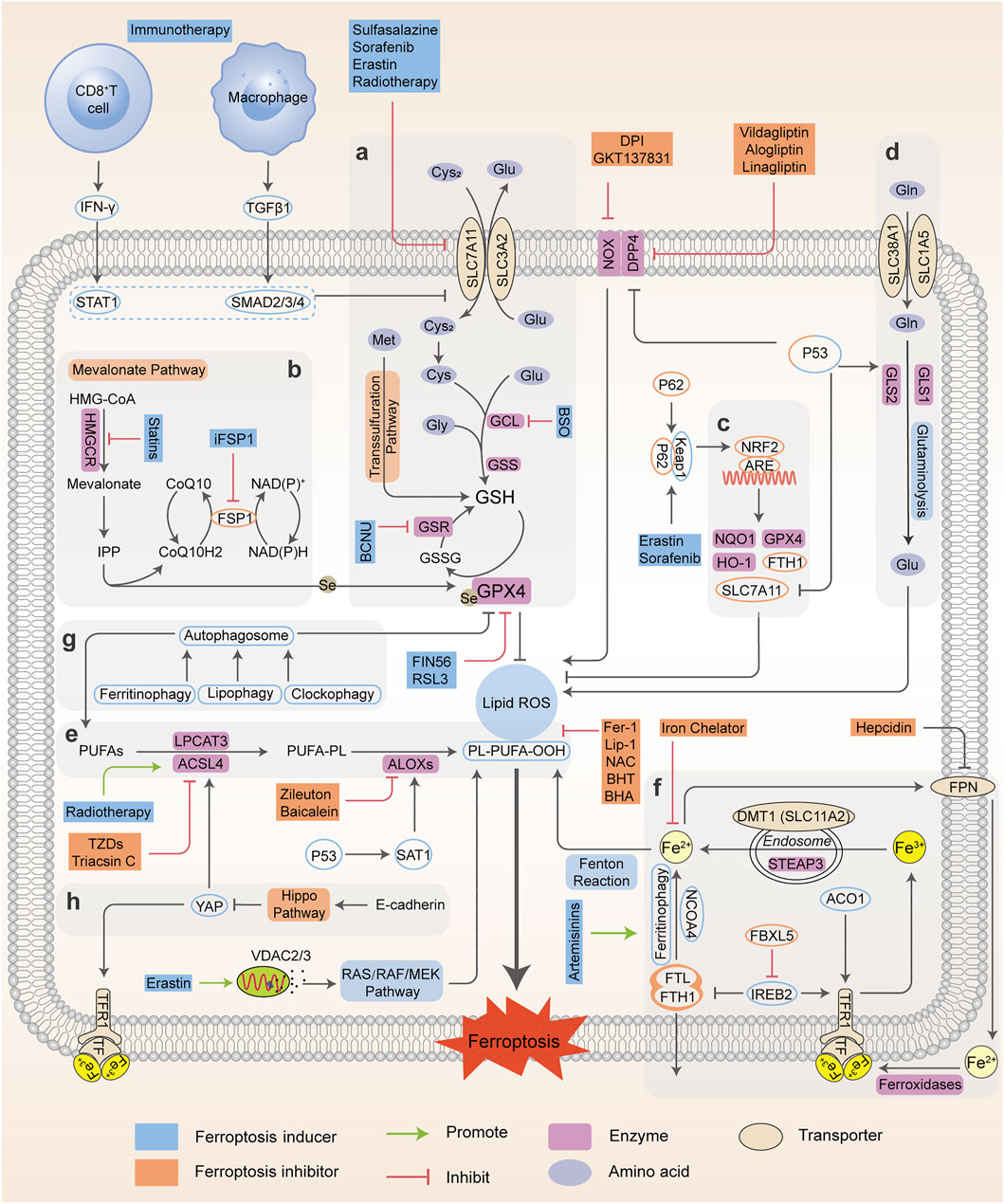
FIGURE 1. Regulatory networks and signaling pathways associated with ferroptosis. Ferroptosis is primarily activated by iron-dependent lipid peroxidation and redox perturbation, which mainly occurs through two major pathways, the extrinsic or transporter-dependent pathway, and the intrinsic or enzyme-regulated pathway. (A) The cyst(e)ine/GSH/GPX4 antioxidative axis. (B) The mevalonate pathway (IPP/FSP1/CoQ10 system). (C) NRF2-regulated ARE defence. (D) The glutaminolysis pathway. (E) The lipid peroxidation process; (F) The absorption, export, storage and utilization of iron. (G) The autophagy cascade. (H) The EMT-related pathway. Processes favoring or counteracting ferroptotic cell death are labeled with red and green arrows, respectively (Cys2, cystine; Cys, cysteine; Glu, glutamate; Gly, glycine Gln, glutamine; BCNU, 1,3-bis-(2-chloroethyl)-1-nitrosourea; CoQ10H2, ubiquinol; HMG-CoA, 3-hydroxy-3-methyl glutaryl coenzyme A; GSR, glutathione-disulfide reductase; BHA, butylated hydroxyanisole; BHT, butylated hydroxytoluene).
Iron Homeostasis
Iron is of great physiological and biological functions such as the transport and storage of oxygen and energy metabolism. Normally, the intracellular iron maintains a balance within a narrow range. However, the disordered distribution and content of iron perturb physiological processes and biological survival. Excessive iron can provoke subsequent lipid peroxidation either by the iron-mediated Fenton reaction to produce reactive oxygen species (ROS, a group of molecules derived from molecular oxygen, mainly including hydrogen peroxide (H2O2), singlet oxygen (1O2), superoxide anion (O2•–) and hydroxyl radicals (•OH)), or the activation of enzymes containing iron (for example, lipoxygenases) (Xie et al., 2016a; Dixon and Stockwell, 2014). In general, the iron atom (Fe3+) binds to transferrin (TF) and binds with transferrin receptor (TFRC) through blood circulation to form the double iron-TF-TFRC complex, which is then endocytosed into the nucleus and reduced to ferrous (Fe2+) by endosomal six-transmembrane epithelial antigen of prostate 3 (STEAP3). Subsequently, Fe2+ is released from the nuclear endosome to the labile iron pool (LIP) in the cytoplasm via divalent metal transporter 1 (DMT1, SLC11A2). Excessive iron is stored in ferritin (the primary iron storage protein) which consists of ferritin light chain (FTL) and ferritin heavy chain 1 (FTH1). Finally, iron is exported from cells to blood through ferroportin (FPN, encoded by SLC40A1, the only known iron-efflux protein) in the cell membrane, and Fe2+ can be reoxidized by ferroxidases (such as ceruloplasmin or hephaestin) to Fe3+ (Torti and Torti, 2013). Alternatively, Fe2+ can be exported as ferritin through exosomes. Variable iron levels on account of interventions at multiple levels (such as iron absorption, storage, utilization, export and iron chelators) affect ferroptosis via an integrated signaling pathway (Figure 1). At the post-transcriptional level, iron regulatory protein 1 and 2 (IRP1; also known as ACO1, IRP2; also known as IREB2) can regulate the expression of DMT1, TFRC, ferritin and FPN (Torti and Torti, 2013) and thereby influence ferroptotic activity. Initiation of IREB2 promotes erastin-induced cytotoxicity, while inhibition of F-box and leucine-rich repeats protein 5 (FBXL5), an endogenous IREB2 antagonist, can sensitize cells to erastin (Reed and Pellecchia, 2012). Iron chelators, such as deferoxamine (DFO) (Dixon et al., 2012; Chen X. et al., 2020), dexrazoxane (DXZ) (Fang et al., 2019) and deferiprone (DFP) (Wu et al., 2020), can directly chelate iron and have been used in clinical treatment to reduce iron availability. As a result, these iron chelators are capable of relieving ferroptosis intrinsically or caused by proferroptotic reagents. Ferroptosis was originally identified as an autophagy-independent RCD (Xie et al., 2016a; Najafov et al., 2017). According to current studies, autophagy plays an important role in ferroptosis through the regulation of cellular iron levels. Autophagy recognizes and degrades ferritin into autophagosomes for lysosome-dependent degradation by using nuclear receptor coactivator 4 (NCOA4) as a selective cargo receptor. This process called “ferritinophagy”, which leads to increased iron accumulation and subsequent oxidative damage and ferroptosis. On the contrary, abrogating ferritinophagy by knockdown of autophagy-related genes or NCOA4 or inhibition of lysosomal function by specific inhibitors suppresses the availability of labile iron and decreases ferroptotic sensitivity (Mancias et al., 2014; Hou et al., 2016). In addition to NCOA4-mediated ferritinophagy, other forms of selective autophagy, including lipophagy, clockophagy, and chaperone-mediated autophagy, have been demonstrated to trigger ferroptosis in cells (Gao et al., 2016). Thus, intracellular iron homeostasis directly or indirectly targets ferroptosis, and the crosstalk of ferroptosis and autophagy provides new perspectives and contexts for the regulation of RCD.
Lipid Peroxidation Metabolism
Lipid peroxidation has been regarded as the hallmark in the context of ferroptosis. As materials of lipid synthesis, polyunsaturated fatty acids (PUFAs), especially arachidonic acid (AA) and adrenic acid (AdA), are vulnerable to the peroxidation process and incorporated into membrane phospholipids (PLs), which destroy the lipid bilayer to trigger the ferroptotic process (Stockwell et al., 2017; Kagan et al., 2017; Conrad and Pratt, 2019). Acyl coenzyme A synthase long-chain member 4 (ACSL4) connects coenzyme A (CoA) to long-chain PUFAs, preferentially AA or AdA, to form arachidonic acid-CoA (AA-CoA) or adrenic acid-CoA (AdA-CoA), respectively. Then PUFA-CoAs are esterified by lysophosphatidylcholine acyltransferase 3 (LPCAT3) to produce PUFA-containing phospholipids (PUFA-PLs). Particularly, phosphatidylethanolamines (PEs) with both AA and AdA (AA/AdA-PEs) are the significant PUFA-PLs for ferroptosis, and are susceptible to free radical trigged oxidation catalyzed by lipoxygenases (ALOXs). ALOXs are nonheme iron-containing dioxygenases that catalyze the oxidation of PUFAs to produce fatty acid hydroperoxides, with a context-dependent role in mediating lipid peroxidation (Kagan et al., 2017; Doll et al., 2017) (Figure 1). Based on this, many reports have uncovered the implications of ACSL4, LPCAT3 and ALOXs during ferroptosis and exploited some candidate compounds with ferroptotic activities (Ou et al., 2016; Colakoglu et al., 2018). ACSL4 knockdown or inhibition can significantly lower the levels of AA-CoA and AdA-CoA to prevent the destruction of membrane structure, and confer ferroptosis resistance. Conversely, supplementation with AA or other PUFAs can block the original ACSL4-mediated reaction, which promotes the occurrence of ferroptosis or sensitizes cells to ferroptosis induced by erastin or RSL3 (Yang et al., 2016; Shintoku et al., 2017). However, the actual driver of lipid peroxidation and subsequent ferroptosis is still controversial, as it has been shown that ALOX15 is not essential for ferroptosis in a mouse model of GPX4-mediated acute renal failure (Friedmann Angeli et al., 2014).
As a master repressor of ferroptosis, selenoenzyme glutathione peroxidase 4, GPX4, consumes two molecules of reductive GSH after being combined with lipids to reduce toxic phospholipid hydroperoxide (LOOH) to nontoxic phospholipid lipid alcohol (LOH), which simultaneously produces oxidative glutathione (GSSG) as a byproduct, and ultimately inhibits phospholipid lipid peroxidation. Once they enter the membrane, PUFAs which are peroxidized and not cleared up by GPX4, eventually drive a large amount of lipid peroxidation and ferroptosis (Yang et al., 2016; Kagan et al., 2017). Further studies have shown that GPX4 inactivation is observed in the ferroptotic death process in vitro and in vivo, whereas deletion of GPX4 with pharmacological or genetic approaches can induce ferroptosis execution (Hangauer et al., 2017; Guo et al., 2018a). Besides western blot or RT-PCR evidence, many studies have confirmed the function and activity of GPX4 in the regulation of ferroptosis using cell or mouse models (e.g. GPX4 knockout or overexpression) (Alvarez et al., 2017; Hangauer et al., 2017; Viswanathan et al., 2017). For example, GSH-depleting reagents (erastin and buthionine sulfoximine (BSO)) inhibits GPX activity to induce ferroptosis and the total activity of GPXs in cells is examined using tert-butylhydroperoxide (tBuOOH) as a substrate. Furthermore, 7a-cholesterol-OOH is a specific substrate for GPX4; no other GPX enzyme can catalyze the reduction of 7a-cholesterol-OOH (1S, 3R)-RSL3, a ferroptosis inducer, has been demonstrated to inhibit enzyme activity of GPX4, indicated by unchanged level of 7a-cholesterol-OOH (Yang et al., 2014). Additionally, it is shown that FINO2 indirectly inhibits GPX4 enzymatic function while FIN56 directly depletes GPX4 enzymatic function using 1H-15N heteronuclear single quantum coherence (HSQC) NMR spectroscopy, ultimately causing widespread lipid peroxidation and ferroptosis (Gaschler et al., 2018a). It should be noted that the GPX4 defense system is also linked to other non-ferroptotic RCDs such as apoptosis and necroptosis, suggesting that lipid peroxidation lies at the crossroads of these pathways with diverse functions and different downstream effectors (Ran et al., 2007; Canli et al., 2016). Additionally, GPX4 is necessary for tumor recurrence, indicating that eradicating drug-resistant cancer cells by targeting GPX4 to induce cellular ferroptosis is a promising clinical strategy (Rennekamp, 2017).
Glutathione Metabolism
The cystine-glutamate antiporter system xc--mediated GSH synthesis, representative of the capacity of antioxidation, plays a significant role in the accumulation of lipid peroxides and the occurrence of ferroptosis. The cystine-glutamate antiporter, a transmembrane transport protein, composed of SLC7A11 (xCT) and SLC3A2 (4F2hc), takes up cystine from outside the cell and correspondingly outputs glutamate for exchange at a 1:1 ratio (Dixon et al., 2014). Usually, cysteine under reductive extracellular conditions is transported directly into cells through ASC (a neutral amino acid transport system), while cystine under oxidative extracellular conditions is reduced to cysteine after entering cells through system xc-. Subsequently, cysteine, glutamate, and glycine are catalyzed by γ-glutamylcysteine synthetase (GCLC) and glutathione synthase (GSS) respectively to form GSH, the major intracellular antioxidant (Doll and Conrad, 2017; Liang et al., 2019). The functional activity of GPX4 to reduce lipid hydroperoxides is dependent on GSH biosynthesis, because GSH acts as a cofactor for GPX4. GSH depletion leads to GPX4 inactivation, thereby triggering intracellular lipid peroxidation and ferroptosis. Therefore, system xc- (SLC7A11) is a transportation hub that regulates intracellular redox balance and ferroptotic damage (Yang and Stockwell, 2016; Stockwell et al., 2017). The expression and activity of SLC7A11 is further positively modulated by nuclear factor erythroid 2-related factor 2 (NRF2), and negatively modulated by tumor suppressor genes, such as p53 and BAP1 (Carbone et al., 2013; Zhang Y. et al., 2018). This dual regulation constitutes a fine-tuning mechanism to delicately control and maintain GSH levels in ferroptosis. Consequently, ferroptosis is driven by GSH depletion either by restricting GSH biosynthesis (e.g., BSO inhibits GCLC) or by blocking cystine uptake from extracellular environment (e.g., erastin or sulfasalazine (SAS) inhibits system xc-). As an alternative way apart from system xc-, the transsulfuration pathway can supply cysteine as a compensation system under cystine deprivation, which is negatively regulated by cysteinyl-tRNA synthase (CARS). Loss of CARS has been proven to upregulate the transsulfuration pathway and inhibit erastin-induced ferroptosis (McBean, 2012; Hayano et al., 2016). Taken together, any link of GSH synthesis, representative of antioxidant capacity, can be a mediator of the ferroptotic cascade.
Other Regulatory (co)factors and Signaling Pathways
p53
Emerging evidence suggests the two contradictory functions of the tumor suppressor p53 in the regulation of ferroptosis (Figure 2). On the one hand, p53 transcriptionally suppresses the expression of SLC7A11 in some types of cells (e.g., human breast or lung cancer cells) to trigger ferroptosis, which induces GSH depletion and relieves the specific inhibition of the ALOX12 enzyme to suppress tumor growth (Jiang et al., 2015; Chu et al., 2019). Moreover, p53-mediated transcriptional activation of spermidine/spermine N1-acetyltransferase 1 (SAT1) may indirectly increase ALOX15 expression, thus inducing lipid peroxidation and sensitizing cells to ferroptotic death (Ou et al., 2016). On the other hand, activation of p53 may antagonize ferroptosis in human colorectal cancer (CRC) cells and fibrosarcoma cells through various mechanisms, such as promoting the localization of dipeptidyl peptidase 4 (DPP4) to the nuclear enzyme-free pool in a transcription-independent manner, increasing the expression of CDKN1A (encoding p21) and favoring the preservation of GSH (Jennis et al., 2016; Xie et al., 2017). Considering that the transcription-dependent or independent function of p53 seems to be cell type-specific, it is necessary to evaluate the role of p53 in ferroptosis according to different circumstances.
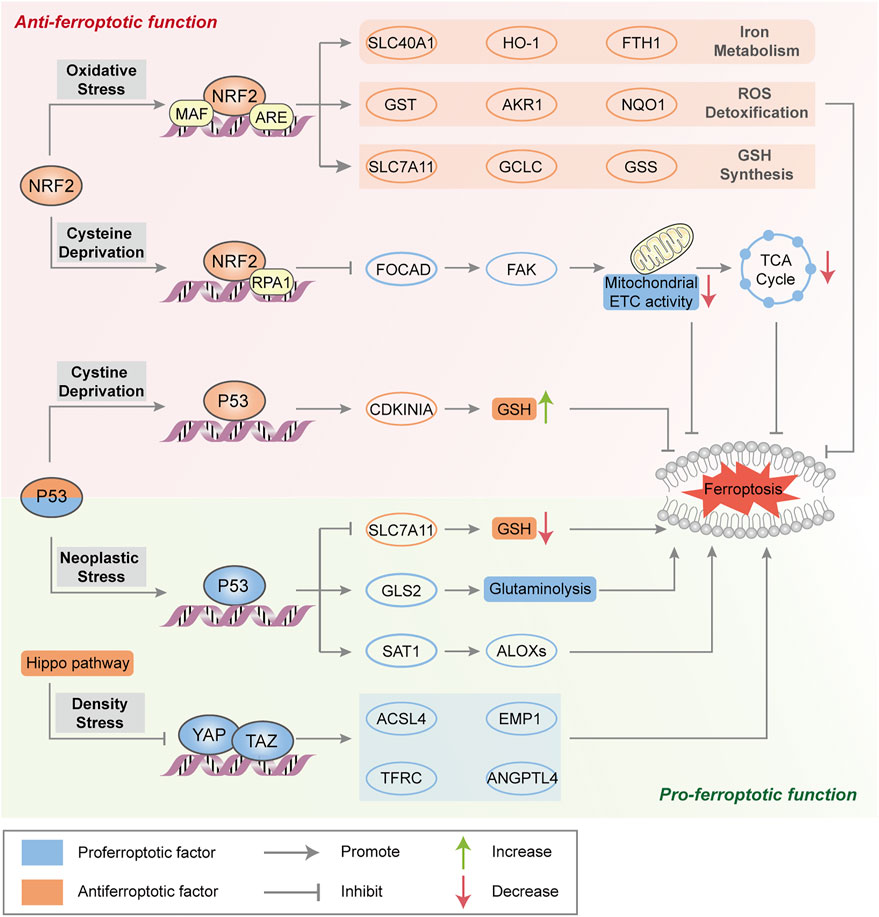
FIGURE 2. Major transcription factors in the regulation of ferroptosis. NRF2 transactivates a range of cytoprotective genes under endogenous oxidative stress to drive antiferroptotic function. Moreover, NRF2 represses FOCAD expression and FAK activity and further reduces sensitivity to cysteine deprivation-induced ferroptosis. Generally, p53 triggers ferroptosis to suppress tumorigenesis by transcriptional regulation of ferroptosis-related genes. In some circumstances, p53 suppresses metabolic stress-induced ferroptosis to preserve cell survival in certain cancer cells. The Hippo pathway negatively regulates a series of proferroptotic genes via YAP/TAZ transcription factors.
NRF2
As a master protective regulator to maintain cellular redox homeostasis, NRF2 is unleashed from its ligand kelch-like ECH-associated protein 1 (Keap1) binding and translocates into the nucleus under oxidative stress. In the nucleus, NRF2 participates in the transcription of a series of antioxidant response element (ARE)-related genes that are implicated in iron metabolism (such as SLC40A1, heme oxygenase‐1 (HO-1) and FTH1), GSH synthesis (such as SLC7A11, GCLC and GSS) and ROS detoxification (such as aldosterone reductase family 1 (AKR1), glutathione thiotransferase (GST) and quinone oxidoreductase‐1 (NQO1)), resulting in the suppression of ROS- and/or iron-related ferroptosis (Sun et al., 2016a; Roh et al., 2017) (Figure 2). The p62-Keap1-NRF2 axis is engaged in oxidative stress-associated ferroptosis via the competitive binding of p62 to Keap1, which leads to the activation of NRF2 and downstream effectors. ARF is a crucial tumor suppressor, that activates the p53 pathway during carcinogenic stress (Sherr, 2006). Studies have shown that ARF blocks CBP (transcription coactivator)-dependent NRF2 acetylation and binds to its cognate transcriptional promoter, which inhibits NRF2-mediated transactivation (including SLC7A11) and leads to ferroptosis (Chen et al., 2017). In addition, NRF2 negatively regulates FOCAD gene expression in human non-small-cell lung cancer (NSCLC) cells, which is dependent on the NRF2-replication protein A1 (RPA1)-ARE complex. FOCAD is essential for focal adhesion kinase (FAK) activity, which further enhances the sensitivity of NSCLC cells to cysteine deprivation-induced ferroptosis via promoting the tricarboxylic acid (TCA) cycle and the activity of Complex I in the mitochondrial electron transport chain (ETC) (Liu P. et al., 2020). The role of NRF2 in ferroptosis sensitiviy and the therapeutic potential of NRF2 inhibitors need further investigation in ferroptosis-associated therapy.
Mitochondrial-Mediated Pathway
The contribution of mitochondria to the ferroptotic pathway is under intense debate. Some studies have shown that erastin alters the permeability of the outer mitochondrial membrane and directly binds to VDAC2/3 after cysteine deprivation (with erastin or cystine-free medium), leading to increased mitochondrial membrane potential (ΔΨ) and lipid peroxides (Bock and Tait, 2019; Gao et al., 2019). Other studies have shown that ROS production by the mitochondrial electron transport chain is not involved in the activation of ferroptosis (Gaschler et al., 2018b). Mitochondrial free iron accumulation can aggravate erastin-mediated ferroptosis and glutaminolysis is required for ferroptosis under cystine deprivation. Glutamine is degraded to glutamate catalyzed by glutaminase 1 and 2 (GLS1 and GLS2) in mitochondria and produces alpha-ketoglutaric acid (α-KG), which can effectively modulate ferroptotic death (Figure 1). Moreover, p53 can favor glutaminolysis by increasing GLS2 expression, which promotes ferroptosis through glutamate accumulation. Excessive extracellular glutamate decreases intracellular cysteine levels and eventually results in ferroptosis via inhibiting system xc-. Correspondingly, upregulation of GLS2 facilitates p53-dependent ferroptosis (Gao et al., 2015).
Mevalonate Pathway
The implication of the mevalonate pathway and ferroptosis has been well established, with two important products, isopentenyl pyrophosphate (IPP) and coenzyme Q10 (CoQ10) (Figure 1). Remarkably, IPP is essential for cholesterol biosynthesis, CoQ10 production and selenocysteine (Se)-tRNA function, which are responsible for the efficient translation of GPX4. Blocking 3-hydroxy-3-methylglutaryl coenzyme A reductase (HMGCR), the rate-limiting enzyme of the mevalonate pathway with statins can downregulate GPX4 activity and consequently induce the occurrence of ferroptosis (Shimada et al., 2016a; Viswanathan et al., 2017). Ferroptosis suppressor protein 1 (FSP1), previously known as ‘‘apoptosis-inducing factor mitochondria associated 2 (AIFM2)’’, promotes the production of CoQ10, an endogenous ferroptosis suppressor to restrain lipid peroxidation, which is independent of GPX4. Indeed, the FSP1-CoQ10-NAD(P)H signaling axis acts as an independent parallel system, that coordinates with GPX4 and GSH to combat oxidative stress and confer ferroptosis resistance (Bersuker et al., 2019; Doll et al., 2019).
Pentose Phosphate Pathway
Nicotinamide adenine dinucleotide phosphate (NADPH) serves as a principal reducing agent for biosynthesis across many biological processes, and is essential for maintaining intracellular GSH levels. Indeed, basal levels of NADPH are considered as a biomarker of ferroptosis susceptibility in several cancer cells, and NADPH depletion facilitates the ferroptotic cascade (Ding et al., 2020). The reduction in NADPH enhances erastin-, RSL3-and FIN56-induced ferroptosis (Shimada et al., 2016b). However, the pentose phosphate pathway (PPP) also produces NADPH as a substrate for NADPH oxidases (NOXs), which promote lipid peroxidation in subsequent ferroptosis. Direct inhibition of PPP or knockdown of related enzymes (glucose-6-phosphate dehydrogenase (G6PD) and phosphoglycerate dehydrogenase (PGD)) can enhance antioxidative activity to inhibit ferroptosis (Dixon et al., 2012; Hassannia et al., 2019). NADPH may play a dual role in ferroptotic regulation, which requires intensive explorations.
Epithelial-Mesenchymal Transition
Epithelial-mesenchymal transition (EMT) is identified that the epithelial phenotype with polarity and intercellular adhesion abilities progressively converts into the mesenchymal phenotype with migratory and invasive properties. EMT is an important cellular program during tumor migration, invasion and metastasis, contributing to drug resistance (Yang J. et al., 2020). EMT-related tumor metastasis and treatment resistance are provoked by transcription factors, such as SNAI1, TWIST1 and ZEB1, which are all potential targets in cancer therapy (van Staalduinen et al., 2018; Wu et al., 2019). Additionally, EMT has been demonstrated to be important for ferroptosis promotion. A highly mesenchymal-like cell state in human cancer cell lines and organoids confers a selective vulnerability to ferroptosis. Ferroptosis inhibitors, including RSL3, and statins have been reported to target the mesenchymal state for cancer therapy (Viswanathan et al., 2017). A CD44-dependent increase in iron endocytosis promotes the activity of iron-dependent demethylases, which increase the expression of genes related to EMT signaling, thereby sensitizing breast cancer cells to ferroptosis (Müller et al., 2020). Collectively, ferroptosis-based treatment may be effective in certain types of cancer, requiring careful consideration of cell type- and differentiation status-related pathways that may determine ferroptosis sensitivity and resistance.
Hippo Pathway
The Hippo pathway is usually believed to control cell number, organ size, tissue development and tumor growth, which negatively regulates the activity of several transcription factors, including transcription coactivators (e.g., yes-related protein 1 (YAP1) and WW domain containing transcription regulator 1 (WWTR1, also known as TAZ). As mentioned above, increased cell adhesion confers resistance to ferroptosis. In epithelial cells, neighboring cells interact through E-cadherin (ECAD) to activate NF2 (still identified as merlin) and the Hippo signaling pathway (Merlin-Hippo signaling) to promote ECAD-dependent cell adhesion, leading to ACSL4 transcriptional downregulation and ferroptosis resistance (Wu et al., 2019). In contrast, the activation of transcription factors involved in the Hippo pathway promotes ferroptosis in human renal cell carcinoma or ovarian cancer cells by regulating the expression of ferroptosis modulators (e.g., YAP-mediated ACSL4 and TFRC expression, TAZ-mediated epithelial membrane protein 1 (EMP1) and angiopoietin-like 4 (ANGPTL4) expression) to increase iron accumulation and lipid peroxidation (Yang et al., 2019; Yang Y. H. et al., 2020) (Figure 2).
Ferroptosis and Its Implication in Diseases
The link between ferroptosis and human diseases has been mainly verified by either the impact of physiopathology on ferroptosis or the impact of ferroptosis on physiopathology. Indicators or hallmarks of ferroptosis (e.g., lipid peroxidation) in some pathological models are obviously altered consistently with the ferroptosis pathway. Alternatively, the pathological state varies with the induction or inhibition of ferroptosis. Either way, molecular mechanisms and signaling pathways involved in ferroptosis help to expand understanding of human health and diseases. Ferroptosis has been interrelated with multiple pathological and physiological environments, with a context-dependent role (Figure 3). On the one hand, ferroptosis, like other forms of RCD, plays a beneficial role in the maintenance of normal physiological functions in the body through the removal of excessively damaged or disordered cells during the response to environmental injury/infection. On the other hand, the death of useful or functional cells aggravates the initiation and progression of diseases, consequently leading to certain pathological states, such as cardiovascular disease and neurodegeneration. Hence, pathologies in diverse tissues or organ systems interrelated with ferroptosis and relevant research models are summarized in Table 1.
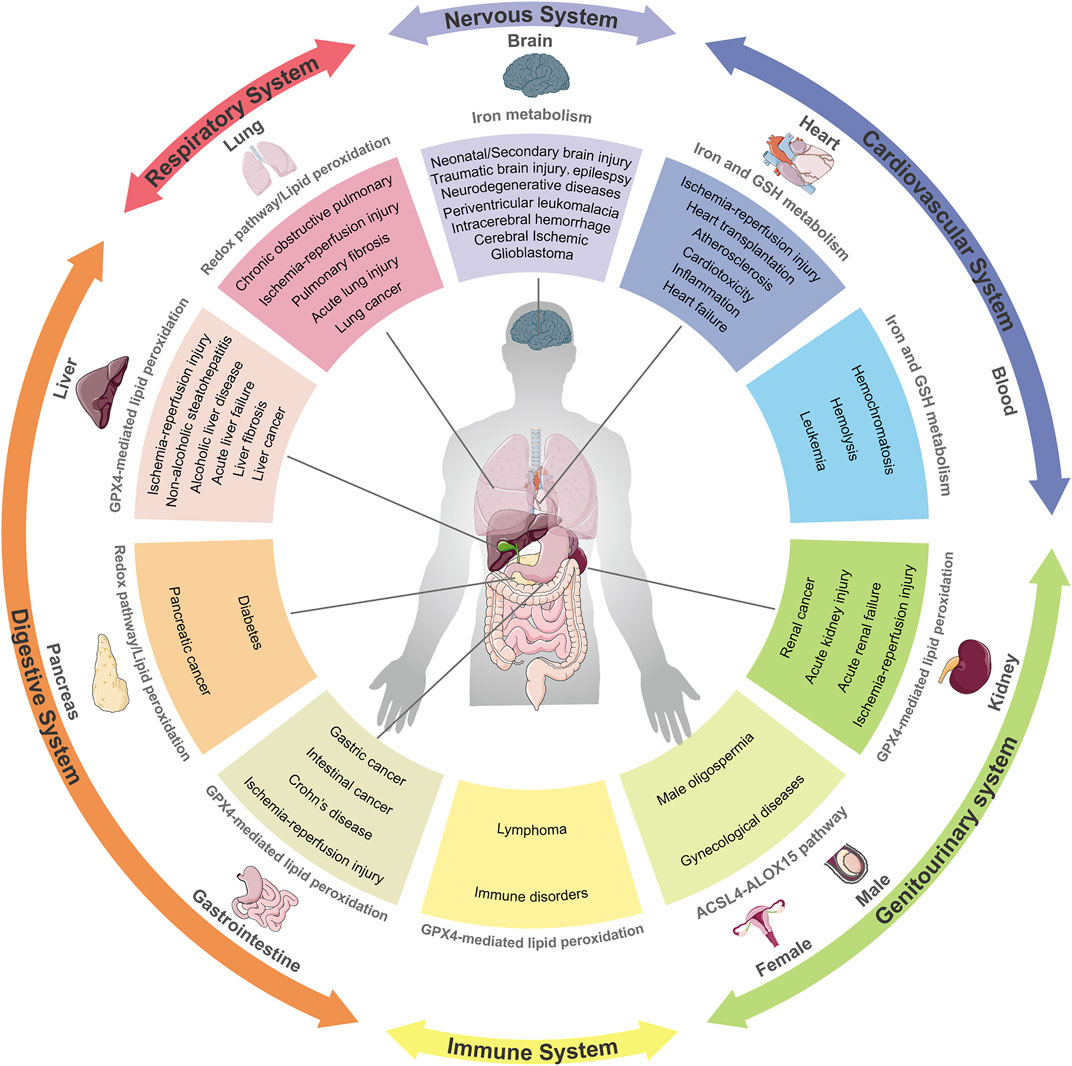
FIGURE 3. Ferroptosis and its implication in different pathophysiological contexts. Ferroptosis has been linked to pathological processes in diverse human body systems, including the nervous system, digestive system, respiratory system, circulatory system and urinary system. Abnormal pathways that contribute to diseases are recapitulatively presented.
Cancer
Cancer is a multifactorial and complicated disease that contributes to a major health burden worldwide, despite substantial efforts and considerable improvement. In general, there are two key challenges in cancer treatment. One is how to kill cancer cells effectively without affecting healthy cells. Second, cancer cells in advanced tumors often exhibit multiple genetic variations with high oxidative stress and enhanced antioxidant capacity, which confer drug resistance (Trachootham et al., 2009). Ferroptosis, as a novel form of cell death, offers unique possibilities efficiently against a variety of cancers. A higher iron requirement than their non-malignant counterparts has been observed to support sustained proliferation and immortalized replication in certain cancer cells, along with FPN downregulation and TFRC upregulation. The strong dependency on iron makes cancer cells more susceptible to iron overload and ROS-mediated lipid peroxidation than noncancerous cells. Epidemiological investigations have also revealed that high dietary iron intake increases the risk of several cancer types (such as hepatocellular carcinoma (HCC) and breast cancer) (Fonseca-Nunes et al., 2014). Ferroptosis inducers have been proven effective in suppressing the occurrence and development of cancers derived from iron-rich tissues, such as HCC, breast cancer and pancreatic ductal adenocarcinoma (PDAC) (Gao et al., 2015; Friedmann Angeli et al., 2019). According to a sensitivity profiling in 177 cancer cell lines, renal cell carcinomas (RCCs) and diffuse large B cell lymphomas (DLBCLs) are particularly susceptible to GPX4-regulated ferroptotic cell death (Yang et al., 2014). From another perspective, it is well acknowledged that the activation of the apoptosis cascade is the most prevalent way to eliminate cancer cells. Unfortunately, the apoptotic pathway of most mutant cancer cells is frequently blocked, which confers robust drug resistance (Amable, 2016). Cancer cells resistant to some forms of death may still be vulnerable to the induction of other forms of cell death, suggesting bypass of apoptotic resistance is a considerable approach to combating resistant cancers. A large amount of evidence has confirmed that ferroptosis induction plays a predominant role against certain chemoresistant cancer cells that are in a high mesenchymal state or escaping drug treatment (Holohan et al., 2013). Recent research has showed that cisplatin can induce both ferroptosis and apoptosis in the NSCLC A549 cells and colon cancer HCT116 cells, while drug resistance is observed in cisplatin-induced apoptosis, rather than ferroptosis (Guo et al., 2018a). Additionally, some drug-resistant cancer cells with activated EMT signaling (upregulation of mesenchymal markers and downregulation of epithelial markers) become specifically sensitive to ferroptosis-based therapies (Viswanathan et al., 2017; Müller et al., 2020). Beyond acting as a monotherapy, ferroptosis induction also participates in combination with conventional therapies. For instance, erastin, a classical ferroptosis inducer, has been shown to enhance the chemotherapeutic efficacy of traditional anticancer drugs, such as temozolomide and cisplatin in specific cancer cell lines (Yamaguchi et al., 2013; Chen et al., 2015). Accordingly, deficiencies in a host of NRF2 targets, including GPX4, SLC7A11 and FTH1/FTL, are also capable of predisposing cells to succumb to proferroptotic agents in many cancer types (Hassannia et al., 2019).
Currently, discovered proferroptotic compounds mainly spotlight on key elements of redox homeostasis (e.g., system xc- and GPX4) to disrupt the existing redox balance and trigger the ferroptotic cascade in cancers whose growth and survival are highly dependent on the uptake of amino acids. Lack of SLC7A11 and knockdown of GPX4 have been demonstrated to increase ROS-mediated lipid peroxidation and induce subsequent ferroptosis in even resistant cancer cells, indicating that deficiency in antiferroptotic function enhances cancer cell death (Yang et al., 2014; Roh et al., 2016). Furthermore, increased expression of SLC7A11, GPX4 or their upstream regulatory gene NRF2 not only promotes ferroptosis resistance, but is also correlated with a poorer prognosis and lower survival rate in many types of cancers, such as liver, lung and ovarian cancers (Fan et al., 2017; Daher et al., 2019; Lim et al., 2019). However, the role of ferroptosis in tumorigenesis and progression remains elusive, as ferroptosis appears to have a dual role. There has been evidence that deficiency in NRF2 favors early tumorigenesis in several cancers (Rojo de la Vega et al., 2018). Another critical mediator of the proferroptotic cascade, ACSL4, is downregulated in bladder, brain, breast, leukemia and lung cancer but upregulated in colorectal, head and neck, kidney, myeloma and liver cancer. Prognostic analysis shows that colorectal cancer patients with high ACSL4 expression have a low survival rate. In contrast, brain, breast and lung cancer patients with low ACSL4 expression have a poor survival, suggesting that ACSL4 plays different roles in different cancer types (Chen et al., 2016).
With a comprehensive understanding, ferroptosis has also been implicated in cancers undergoing immunotherapy and radiotherapy. Immunotherapy with immune checkpoint blockade, which activates an effective cytotoxic T cell-triggered antitumor immune-response, has a revolutionary impact on clinical cancer treatment. Mechanistically, interferon gamma (IFNγ) released from CD8+ T cells can impair cystine uptake by cancer cells upon downregulating the expression of SLC3A2 and SLC7A11 (two subunits of the cystine-glutamate antiporter system xc-) and consequently promote lipid peroxidation and ferroptosis in ovarian cancer, melanoma and fibrosarcoma (Tsoi et al., 2018; Wang W. et al., 2019; Lang et al., 2019). Radiotherapy acts as frontline therapy for approximately half of patients with many cancers. Recently, ferroptosis has also been proven to be interrelated with cancer radiotherapy, which induces ACSL4 expression and enhances oxidative damage in a variety of cancers, including NSCLC, melanoma and esophageal cancer (Lang et al., 2019; Lei et al., 2020). Indeed, immunotherapy and radiotherapy synergistically activate tumoral lipid oxidation and ferroptosis.
However, ferroptosis also triggers severe damage and toxicity in organisms, including bone marrow injury and cisplatin-induced acute kidney injury. How to modulate ferroptosis pathway to obtain the highest clinical benefit in clinical oncology is a critical question that deserves further investigation.
Neurodegeneration and Brain Injury
A large body of evidence suggests that low levels of GSH/GSSG, elevated iron levels and lipid peroxidation are implicated in a variety of neurodegenerative diseases, along with abnormal levels of ferroptosis-associated modulators (Praticò and Sung, 2004; Belaidi and Bush, 2016). Alzheimer’s disease (AD) is the most common neurodegenerative disease and is characterized by synaptic loss and neuronal death. In AD patients, there is abnormally elevated iron in the brain tissue, and ferritin levels are negatively correlated with cognitive ability (Ayton et al., 2015). Excessive ROS generation triggers lipid peroxidation, which contributes to severe oxidative stress damage and is observed in GPX4-deficient AD mouse models, while ferroptosis inhibitors ameliorate neurodegeneration and behavioral dysfunction by blocking iron overload, lipid peroxidation and inflammation (Hambright et al., 2017; Zhang Y.-H. et al., 2018). Parkinson’s disease (PD) is characterized by progressive dopaminergic neuronal loss in the substantia nigra (SN) pars compacta. Epidemiological investigations have shown that high iron levels lead to a high risk of PD, associated with enhanced basal lipid peroxidation and elevated malondialdehyde (MDA) levels, a toxic byproduct of lipid peroxidation (Pyatigorskaya et al., 2015). Given the implication of iron in PD, preclinical experiments and clinical trials have revealed that the U.S. Food and Drug Administration (FDA)-approved iron chelators improve dopamine activity and prevent iron-mediated oxidative damage, by exerting a certain protective effect in PD patients (Devos et al., 2014; Chen X. et al., 2020). Several features of Huntington’s disease (HD) physiopathology are consistent with the ferroptosis pathway, including a lower GSH level, a higher lipid oxidation level and abnormal iron metabolism. Accordingly, administration of DFO significantly improves striatal pathology and motor phenotype in an R6/2 HD mouse model (Klepac et al., 2007; Lee et al., 2011; Chen et al., 2013). Additionally, ferrostatin-1 (Fer-1, classical ferroptosis inhibitor) and its analogs inhibit lipid peroxidation damage and increase the number of healthy spinous process neurons in the HD model. Apart from common neurodegeneration, other neurodegenerative disorders are also characterized by perturbations in iron homeostasis and accumulation, such as periventricular leukomalacia (PVL) (Skouta et al., 2014), amyotrophic lateral sclerosis (ALS) (Southon et al., 2020), multiple sclerosis (MS) (Jhelum et al., 2020), Friedreich’s ataxia (FRDA) (Cotticelli et al., 2019). Affected tissue regions share features of elevated iron, low GSH, decreased GPX4, as well as increased lipid peroxidation, consistent with the salient features of ferroptosis. Although there are few relevant studies, some ferroptosis-associated factors appear to be potential markers and therapeutic targets in these disorders (Hu et al., 2019; Seco-Cervera et al., 2020).
Besides leading to neurodegenerative diseases, the activation of the of ferroptotic cascade has also been determined in a wide variety of neuronal injuries. Neonatal brain injury, as well as traumatic brain injury (TBI), is usually accompanied by a series of biochemical processes, such as dysfunctional iron metabolism, decreased GPX activity, ROS accumulation and disordered ferroptosis-related mediators in vitro and in vivo (Lu et al., 2015; Kenny et al., 2019). Oxidative stress damage induced by specific GPX4 deletion in the brain is observed during the acute phase of intracerebral hemorrhage (ICH) and subsequent secondary brain injury (SBI) and finally leads to hemorrhagic stroke which can be relieved by an increasing GPX4 levels. The application of N-acetylcysteine (NAC, a ROS scavenger) or Fer-1 has been documented to prevent neuronal mortality after ICH and improve the prognosis of patients, by decreasing iron accumulation and lipid ROS (Li et al., 2017; Zhang Z. et al., 2018). Dysfunction of tau protein contributes to age-dependent, iron-mediated neurotoxicity in ischemic stroke tissue. Tau is capable of blocking ferroptosis by promoting iron export against middle cerebral artery occlusion (MCAO)-induced focal cerebral ischemia-reperfusion injury. Ferroptosis has been recognized as a main cause of neuronal death after ischemic stroke, and ferroptosis inhibitors significantly improve the prognosis of patients (Tuo et al., 2017). Actually, ferroptosis inhibitors have been successfully applied in animal models of I/R-associated tissue injuries including heart (Fang et al., 2019), lung (Xu et al., 2020), liver (Yamada et al., 2020a), intestine (Li Y. et al., 2019) and kidney (Friedmann Angeli et al., 2014).
Overall, these studies suggest that ferroptosis irreversibly drives neuronal loss and tissue damage linked with central and/or the peripheral neuronal pathologies. The effectiveness of ferroptosis inhibition as a therapeutic approach has been mostly validated by cellular and animal experiments. However, there seems to be a lack of strong evidence in clinical trials. Providing comprehensive treatments for neurological diseases remains a largely insurmountable challenge.
Cardiovascular Diseases
As “iron-induced heart disease” was first reported, iron-induced ferroptosis has been gradually identified as playing a detrimental role in the occurrence and aggravation of cardiovascular conditions in both animal models and human patients (Sullivan, 1981). Abnormal myocardial iron accumulation plays a pathogenic role in the myocardium which leads to cardiotoxicity and cardiac dysfunction. Studies have shown that ferroptosis mediates myocardial damage caused by doxorubicin (DOX) or I/R mainly by HO-1 upregulation, and subsequently degrading heme to release free iron and generate mitochondrial membrane oxidized lipids. Iron deposition in the myocardium of FPN knockout mice which results in severe damage to cardiac function has been recognized as a risk factor for coronary artery diseases (Bagheri et al., 2013; Lakhal-Littleton et al., 2015). Iron chelators, such as DFO and DFP have been shown to counteract the pathogenic effects of iron overload, indicating a promising therapeutic strategy against ferroptosis-driven cardiac abnormalities (Gao et al., 2015; Fang et al., 2019). Nonetheless, cardiomyocyte iron deficiency can also lead to fatal contractile and metabolic dysregulation as a consequence (Lakhal-Littleton et al., 2016). This findings supports compelling evidence that the influence of ferroptosis on cardiac diseases depends on proper iron homeostasis, rather than absolutely low iron levels. Ferroptosis has also been involved in inflammatory responses after heart transplantation, which promotes the adhesion of neutrophils to coronary endothelial cells and myocardial I/R damage. Fer-1 can alleviate the loss of cardiomyocytes and limit the recruitment of neutrophils by the TLR4/TRIF signaling pathway (Li W. et al., 2019). As the master regulator of systemic iron homeostasis, hepcidin is derived primarily from the liver, and it inhibits the iron exporter FPN to decrease iron availability in blood (Lakhal-Littleton et al., 2016). Due to an inherited genetic disorder or receiving multiple blood transfusions, the depletion of hepcidin induces ferroptosis as a result of iron overload in the body. As an iron overload disease that blocks hepcidin biosynthesis, hemochromatosis can be ameliorated by either iron chelators or genetic regulation of ferroptosis, such as targeting SLC7A11 (Wang H. et al., 2017).
Although the link with cardiovascular diseases still requires further evaluation by clinical evidence, iron-induced ferroptosis has been well-established as a master trigger of several cardiovascular pathologies. Chelation therapy has been considered as an efficient preventative measure and treatment approach and the key is how to maintain relatively precise iron homeostasis.
In addition to common diseases mentioned above, more pathologies associated with ferroptosis are summarized in Table 1 and Figure 3.
Pharmacological Approaches to Ferroptosis Modulation
A more profound understanding of the regulatory and metabolic mechanisms of ferroptosis in a variety of diseases provides attractive approaches to diagnosis and therapy. As mentioned above, the positive or negative role of ferroptosis depends on the context of diseases with different pathological and physiological processes. In cancer therapy, it aims to slow or suppress tumor growth by inducing ferroptosis in cancer cells without affecting non-malignant cells. Thus, the activation of ferroptosis appears to be a promising therapeutic strategy to block pro-carcinogenic alterations, especially those are resistant to other modes of cell death. In degenerative or traumatic diseases, such as neurodegenerative disorders and I/R injury, the therapeutic goal is to protect functional cells from ferroptosis-induced injury. As such, ferroptosis inhibitors are expected to reverse or mitigate oxidative damage and disease progression and even achieve curative effect. Besides individual effects, multiple ferroptosis regulators can also act synergistically with some conventional drugs. Many kinds of synthetic compounds and natural products have been identified as inducers and inhibitors of ferroptosis to overcome diseases by modulating ferroptotic or mixed death.
Synthetic Compounds and Their Modulation on Ferroptosis
Currently, numerous chemical reagents with ferroptotic properties have been continuously exploited and demonstrated in preclinical and clinical experiments. Although the underlying mechanism needs more investigation, ferroptosis inducers are reckoned as a novel therapeutic strategy against various cancers, especially those resistant to conventional drugs. Indeed, ferroptosis was first discovered by studying reaction mechanisms of two small RAS-selective molecule compounds, erastin and RSL3, including their derivatives, that have been confirmed to suppress tumor growth in many types of cancers in vitro and in vivo (Dixon et al., 2012; Yang et al., 2014; Viswanathan et al., 2017; Zhang Y. et al., 2019). Originally, ferroptosis-inducing reagents were divided into two mechanistic classes: 1) inhibitors of cystine via system xc- (e.g., erastin, sorafenib, SAS and glutamate), which subsequently drive GSH depletion, and 2) direct inhibitors of GPX4 (e.g., RSL3 (1S, 3R)-RSL3). More recently, the small molecule FIN56 has been reported to degrade GPX4 and to deplete CoQ10, an endogenous inhibitor of lipid peroxidation, through the mevalonate pathway. Moreover, FINO2 can either oxidize ferrous iron directly, or deactivate GPX4 indirectly (Shimada et al., 2016a; Gaschler et al., 2018a). On the contrary, ferroptosis also participates in other nonneoplastic lesions and a variety of compounds can pharmacologically inhibit ferroptosis, most of which are iron chelators or antioxidants. Iron chelators (e.g., DFO and DXZ) or regulators of iron metabolism suppress ferroptosis through lowering free iron levels (Fang et al., 2019; Chen X. et al., 2020). In addition, inhibitors of lipid peroxidation, including ACSL4 inhibitors (e.g., thiazolidinediones (TZDs)), lipophilic antioxidants (e.g., α-tocopherol), and agonists of the antioxidant system (e.g., ROS scavengers (NAC) and system xc- inducers (β-mercaptoethanol)) inhibit ferroptosis via single or complex mechanisms (Dixon et al., 2012; Karuppagounder et al., 2018).
Fortunately, some of these pro- and anti-ferroptotic compounds are clinical drugs approved by the FDA, and are expected to be novel therapeutic applications based on ferroptotic mechanisms. Mechanistically, SAS, sorafenib and lanperisone have been broadly documented to inhibit cysteine-glutamate antiporter and cystine absorption, leading to the attenuation of GSH, and ultimately resulting in the death of certain types of cancer cells, including HCC, lymphoma and prostate cancer (Lachaier et al., 2014; Sun et al., 2016a; Sun et al., 2016b). Siramesine, lapatinib and salinomycin (ironomycin) can trigger ferroptosis by elevating iron levels in breast cancer cells and leukemia cells (Hamaï et al., 2017; Ma et al., 2017; Mai et al., 2017). Iron chelators are usually used as antidotes for iron poisoning in clinical practice, and new indications seem to be available based on their antiferroptotic properties. TZDs, a class of typical antidiabetic compounds, have been proven to act as ACSL4 inhibitors to restore GPX4 expression and reduce COX2 expression, and finally prevent lipid peroxidation and ferroptosis to ameliorate intestinal and pulmonary I/R injuries in vitro and in vivo (Doll et al., 2017; Li Y. et al., 2019; Xu et al., 2020). Furthermore, chelation therapy targeting iron dyshomeostasis has shown promise against neurodegenerative disorders in preclinical studies and clinical trials. Especially, DXZ is currently the only FDA-approved drug for preventing and treating DOX-induced cardiotoxicity in cancer patients (Fang et al., 2019). DFP has been recently used clinically for systemic iron overload dysfunctions and investigated as a conventional or modified treatment in several clinical trials against PD. It has shown reduced iron deposition in the SN and an improvement in motor function, as well as slowed disease progression and relieved neurological symptoms (Devos et al., 2014). Currently, many studies on ferroptosis relevance in neurodegenerative diseases are attempting to use ferroptosis inhibitors, especially iron chelators, to slow disease progression in preclinical animal models, most of which have achieved an potent efficacy (Guo et al., 2016; Rao et al., 2021). Nevertheless, there are still many restrictions and problems associated with chelation therapy. When applied in human clinical trials, iron chelators appears to be far from satisfactory (Grolez et al., 2015). DFP is well tolerated, achieved target engagement (lowering of iron) in patients with neurodegeneration. It seems to somewhat slow disease progression and improve quality of life, but they have not reach significance (Martin-Bastida et al., 2017; Klopstock et al., 2019). Although some of iron chelators, such as DFO, DFP and deferasirox (DFS) are recommended as iron overloaded first-line treatments, they all have their own definite side effects, such as gastrointestinal disorders, hepatotoxicity, nephrotoxicity and visual and auditory neurotoxicity (Vichinsky et al., 2011; Aydinok et al., 2015; Maggio et al., 2020). Furthermore, poor pharmacokinetic properties of DFO and DFS limit their availabilities in the central nervous system, as they are prevented from crossing the blood-brain barrier (BBB) (Sripetchwandee et al., 2016; Crielaard et al., 2017). More appropriate iron chelators that regulate the ferroptotic process need to be further explored and developed for their potential to treat neurodegenerative diseases in preclinical and clinical studies.
Therefore, it is worth noting that nanotechnology has emerged to achieve anticancer effects by improving the pharmacokinetic properties of existing proferroptotic agents or developing novel nano-inducers of ferroptosis. The FDA-approved iron-supplementing agent ferumoxytol (Feraheme) has been proven to have an anti-leukaemia effect in vitro and in vivo via increasing intracellular iron and ROS production, leading to enhanced oxidative stress and ferroptotic cell death (Trujillo-Alonso et al., 2019). Cisplatin (CDDP)-loaded Fe3O4/Gd2O3 hybrid nanoparticles with the conjugation of lactoferrin and RGD dimer (RGD2) (FeGd-HN@Pt@LF/RGD2), which are able to cross the BBB, have been developed for therapy of orthotopic brain tumors based on ferroptosis mechanisms (Shen et al., 2018). In view of the oxidative stress regulation ability of p53 and inducibility of the metal-organic network (MON) on the Fenton reaction, synthesized MON encapsulated with p53 plasmid (MON-p53) can trigger both ferroptosis and apoptosis pathways to synergistically kill cancer cells (Zheng et al., 2017).
Collectively, we have listed FDA-approved synthetic drugs that have been reported to modulate ferroptosis in Table 2 and experimental chemical compounds in Supplementary Tables S1 and Supplementary Tables S2.
Natural Products and Their Modulation on Ferroptosis
Nowadays, natural medicines are usually used alone or, in most cases, in combination with conventional drugs in clinical in the hope of enhancing the efficacies and/or neutralizing the toxicities of chemical drugs. In addition to clinical (adjuvant) medicines, natural products are widely consumed as supplements in alternative strategies (Shu-Feng Zhou et al., 2007; Kibble et al., 2015). Growing evidence has uncovered the solid regulatory properties of ingredients with bioactive functions on ferroptosis (Figure 4). Hence, we focus on natural compounds that show a pharmacological potential for ferroptosis-associated (adjuvant) therapy.
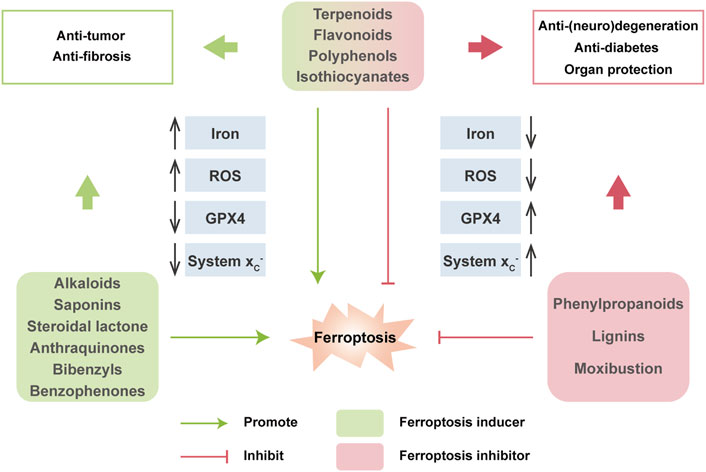
FIGURE 4. Multiple effects of natural compounds regulating ferroptosis. Alkaloids, saponins, steroidal lactone, anthraquinones, bibenzyls and benzophenones can induce ferroptosis with anticancer and antifibrosis functions. In contrast, phenylpropanoids and ligins alleviate (neuro)degeneration and prevent irreparable organic damage via antagonizing ferroptosis. Interestingly, terpenoids, flavonoids, polyphenols and isothiocyanates have dual effects on ferroptosis which depends on different disease contexts.
Terpenoids
Terpenoids are important sources for the research of natural products and the development of modern drugs with a great deal of pharmacological functions such as inflammation, neurodegeneration and cancers. Artemisinin and its derivatives (Artemisinins, including artesunate, artemether, dihydroartemisinin, etc.) are sesquiterpene lactone ingredients derived from Artemisia annua L., and are well known globally for their antimalarial bioactivity (Dondorp et al., 2005). Beyond the antimalarial efficacy, artemisinins have been well documented to be potently effective in diverse diseases in vitro and in vivo. Artemisinins have been extensively demonstrated to induce ferroptosis for cancer treatment through various mechanisms, including regulating iron-related genes, promoting ROS production, depleting intracellular GSH, and increasing intracellular iron levels and activating ferritinophagy. Artesunate (ART), a water-soluble derivative of artemisinin, has been approved as a ferroptosis inducer to selectively promote ROS- and lysosomal iron-dependent cell death in KRAS-transformed PDAC cells, which are highly resistant to apoptosis. Of note, ART exerts no effect on nonneoplastic human pancreatic ductal epithelial cells (Eling et al., 2015). Surprisingly, ART induces ferroptosis via the accumulation of iron, leading to elevated lipid peroxides and reduced antioxidant capacity in several other cancers, such as ovarian cancer, head and neck cancer and Burkitt’s lymphoma (Greenshields et al., 2017; Roh et al., 2017; Wang N. et al., 2019). ART has undergone several clinical trials against various cancers, including metastatic breast cancer and cervical intraepithelial neoplasia, and has been proven to be safe and well-tolerated in patients (von Hagens et al., 2017). Dihydroartemisinin (DHA), one of the semisynthetic derivatives of artemisinin, can trigger ferroptosis to directly kill cancer cells or synergistically sensitize the efficacy of chemotherapeutic agents via multiple functions, including autophagic degradation of ferritin, direct binding to free iron and disturbing iron homeostasis in a cohort of cancer cells (Chan et al., 2013; Du et al., 2019; Chen G. -Q. et al., 2020). Interestingly, DHA activates the feedback pathway of ferroptosis in glioma cells. That is, under endoplasmic reticulum stress induced by DHA, the subsequent increase in GPX4 expression counteracts lipid peroxidation-mediated ferroptosis (Chen et al., 2019). In addition to antineoplastic activities, artemisinins also exhibit hepatoprotective effects via the execution of ferroptosis. ART and artemether have been proven to trigger p53-dependent ferroptosis by promoting the accumulation of iron and lipid peroxides, which can inactivate hepatic stellate cells (HSCs) and ameliorate hepatic fibrosis (Wang L. et al., 2019; Kong et al., 2019). Danshen (Salvia miltiorrhiza) is a commonly used traditional Chinese medicinal herb for the clinical therapy of many diseases. However, two diterpenoid compounds, dihydroisotanshinone I (DT) and cryptotanshinone isolated from Danshen show opposite functions in cancer cells. DT treatment significantly induces ferroptosis to suppress breast carcinoma through downregulating GPX4 protein expression in cell and xenograft models (Lin et al., 2019). In contrast, cryptotanshinone definitely blocks erastin-induced ferroptosis in PDAC cells upon antagonizing STAT3, a positive modulator of ferroptosis (Gao et al., 2018). Glycyrrhizin (GLY) and magnesium isoglycyrrhizinate (MgIG) are natural ingredients extracted from the root of Glycyrrhiza with neuroprotective activity. Nevertheless, current studies have demonstrated that GLY and MgIG protect hepatic function through different modulations of ferroptosis in different subtypes of hepatic cells. MgIG remarkably induces HSC ferroptosis through iron accumulation and ROS production, and finally ameliorates CCl4-induced hepatic fibrosis where HO-1 plays an important role (Sui et al., 2018). Yet, GLY, a high mobility group protein B1 (HMGB1) inhibitor, has been shown to reduce the degree of ferroptosis of hepatocytes during acute liver failure (ALF) via activating NRF2/HO-1/HMGB1 pathway to antagonize oxidative stress (Wang Y. et al., 2019).
Flavonoids
Flavonoids are extensively distributed in the plant kingdom, with multifaceted pharmacological properties and diversified molecular structures. Due to powerful free radical scavenging and antioxidant action, many flavonoids have been reported to be potential ferroptosis inhibitors. Baicalein (also termed 5,6,7-trihydroxyflavone) is a flavonoid originally isolated from the whole root of Scutellaria baicalensis, and has been shown to be effective against cancer, bacterial infections, organ dysfunctions and oxidative stress diseases. Before ferroptosis was formally coined, solid evidence has revealed that baicalein acted as a strong iron chelating agent, which inhibited the Fenton reaction and lipid peroxidation by forming an iron-baicalein complex (Perez et al., 2009). It is reasonable to speculate that baicalein plays its pharmacological role through the above ferroptosis-associated mechanisms. Emerging studies have shown that baicalein is a selective inhibitor of ALOX12/15, which in turn strongly downregulates prostaglandin-endoperoxide synthase 2 (PTGS2) and upregulates GPX4, and significantly suppresses ferroptosis to exhibit a neuroprotective role in post-traumatic epileptic seizures (Li Q. et al., 2019). Furthermore, as a natural ferroptosis inhibitor, baicalein limits iron production, GSH depletion, GPX4 degradation and lipid peroxidation to reverse erastin- or RSL3-induced ferroptosis in pancreatic cancer cells (Xie et al., 2016b; Probst et al., 2017). Quercetin (3,30,40,5,7-pentahydroxyflavone) is one of the most widely distributed flavonoids in edible and medicinal plants, such as onion, tea and ginkgo biloba, and displays a variety of biological activities, especially antioxidative capacity. A recent study has demonstrated that type 2 diabetes (T2DM) molding depletes GSH, downregulates GPX4, and induces oxidative stress in pancreatic β cells (PBCs), indicating that ferroptosis contributes to the loss and dysfunction of PBCs. Indeed, quercetin protects PBCs from pancreatic iron deposition to alleviate ferroptosis in T2DM (Li D. et al., 2020). Moreover, both quercetin and its metabolite quercetin Diels-Alder anti-dimer (QDAD) prevent bone marrow-derived mesenchymal stem cells (bmMSCs) from erastin-induced ferroptosis possibly through the antioxidant pathway, which in turn converts quercetin into QDAD, an inferior ferroptosis inhibitor and antioxidant (Li X. et al., 2020). Despite that flavonoids discussed above are usually approved as strong antioxidants, there are some other flavonoids functioning as ferroptosis inducers, including amentoflavone (AF), a polyphenolic flavonoid widely found in Selaginella and typhaneoside (TYP), a major flavonoid in the extract of Pollen Typhae. Both AF and TYP trigger ferroptosis in an autophagy-dependent manner via AMPK signaling, contributing to ferritin degradation, ROS accumulation and ultimately ferroptotic cell death in acute myeloid leukemia (AML) and glioma (Zhu et al., 2019; Chen Y. et al., 2020).
Polyphenols
Phenolic compounds isolated from plants are known as a fighter against various diseases related to oxidative stress, such as cancer, inflammation, obesity, organic damage and neuronal disorders (Curti et al., 2017). Curcumin, a polyphenol extracted from the rhizome of turmeric and other plants of the family Zingiberaceae, is a powerful antioxidant compound with a great deal of pharmacological activities. Notably, curcumin has been demonstrated to enhance antioxidative capacity as a ferroptosis inhibitor. In renal tubular cells, curcumin inhibits myoglobin (Mb)-induced ferroptosis and ameliorates renal injury associated with rhabdomyolysis by inhibiting the TLR4/NF-κB axis and activating the cytoprotective enzyme HO-1 (Guerrero-Hue et al., 2019). Proanthocyanidins (PACs), widely found in plants in nature, are potent free radical scavengers. PAC treatment significantly decreases the levels of iron, ACSL4, and ALOX15B, while increasing the levels of GSH, GPX4, NRF2, and HO-1 in the traumatic spinal cord, eventually rescues ferroptosis-induced injuries and improves the locomotive function of spinal cord injury (SCI) mice (Zhou et al., 2020). Similarly, Epigallocatechin gallate (EGCG), the most abundant catechin in tea, is a polyphenol with various pharmacological benefits. Both curcumin and EGCG have been demonstrated to prevent ferroptosis against iron-induced oxidative damage in mouse MIN6 pancreatic β cells through neutralizing iron, reducing GSH consumption as well as preventing GPX4 inactivation (Kose et al., 2019). Beyond the organ protection effect, the anticancer efficacy of polyphenols has been well established by accumulated clinical experience, where ferroptosis is believed to be involved. EGCG is a direct inhibitor of heat shock 70 kDa protein 5 (HSPA5), which has been proven to negatively regulate ferroptosis. Further research shows that EGCG can prevent the formation of the anti-ferroptotic HSPA5-GPX4 complex and subsequent lipid peroxidation, which enhances gemcitabine sensitivity by disinhibiting ferroptosis in PDAC in vitro and in vivo (Zhu et al., 2017). Interestingly, EGCG either prevents ferroptosis in normal pancreatic β cells, or induces ferroptosis in cancerous PDAC cells, implying context-dependent bidirectional regulation and special pancreatic for pancreas protection of EGCG.
Isothiocyanates
Isothiocyanates (ITCs) are abundant in cruciferous vegetables, exhibiting chemopreventive and chemotherapeutic properties to tackle many types of diseases. Two ITCs, phenylethyl isothiocyanate (PEITC) and sulforaphane have been employed in small human clinical trials against various diseases ranging from cancer to autism, either within cruciferous food or as single agents (Palliyaguru et al., 2018). PEITC is a dietary anticancer compound with pharmacokinetic behaviors of rapid absorption and high bioavailability (Ji et al., 2005). It has been documented that PEITC triggers multiple forms of cell death, namely ferroptosis, apoptosis, and autophagy in both murine and human osteosarcoma models. PEITC drives oxidative stress as a consequence of GSH depletion-induced ROS generation and lipid peroxidation. ROS generation is proven to be the major cause of PEITC-induced cell death, accompanied by altered iron metabolism and activation of the MAPK signaling pathway (Lv H. -H. et al., 2020; Lv H. et al., 2020). However, another ITC, sulforaphane, acting as a ferroptosis inhibitor, reduces busulfan-induced damage to prevent oligospermia by activating the upregulation of GPX4 and FPN1 protein expression (Zhao et al., 2020).
Alkaloids
Alkaloids are one type of important active component in Chinese herbal medicine and display a broad range of biological properties, such as neurogenesis promotion, pro-oxidant activities, and anti-infection. Trigonelline is an alkaloid derived from a traditional Chinese herbal, Trigonella foenum-graecum L., present in coffee and fenugreek seeds in considerable amounts (Sun et al., 2016a). Trigonelline has been believed to be of great benefits in the prevention and treatment of diabetes and central nervous system disorders (Zhou et al., 2012). Recent studies have revealed that trigonelline exerts antitumor efficiency as a pharmacological inhibitor of NRF2. The inhibition of NRF2 by trigonelline enhances the anticancer activity of classical ferroptosis inducers, such as erastin, sorafenib and RSL3, and sensitizes chemoresistant cancer cells to ferroptosis both in vitro and in vivo models of HCC and HNC (Sun et al., 2016a; Shin et al., 2018). Solasonine isolated from Solanum melongena has various pharmacological benefits. Solasonine has been proven to inhibit the growth and migration of HCC cells. Detailed studies elucidate that solasonine increases lipid ROS levels via GPX4-induced destruction of the GSH redox system, leading to the promotion of ferroptosis (Jin et al., 2020).
Saponins
Saponins are the major active ingredients of many Chinese herbal medicines, such as ginseng, licorice, and bupleurum, processing valuable biological activities including antipyretic, anticancer properties, as well as immunoregulation. Ruscogenin, is initially extracted from Ruscus aculeatus and is a main component of Radix Ophiopogon japonicas, with a wide range of biological functions (Song et al., 2020). Acting as a promising ferroptosis inducer, ruscogenin is found to increase ferrous iron and aberrant ROS production and regulate the levels of TF and FPN, which triggers ferroptosis against pancreatic cancer in vitro and in vivo. Ardisiacrispin B, a natural oleanane-type tritepene saponin isolated from the fruit of Ardisia kivuensis Taton (Myrsinaceae), displays a significant cytotoxic effect in a panel of 9 cancer cell lines (including various sensitive and drug-resistant phenotypes) depending on ROS-mediated ferroptosis (Mbaveng et al., 2018a).
Others
Actually, many kinds of natural compounds serve as potential modulators of ferroptosis. Withaferin A (WA), a major constituent of Withania somnifera extract, has been well documented to provide pharmacological benefits. WA can obviously induce ferroptosis to suppress high-risk neuroblastoma via a double-edged mechanism. On the one hand, WA decreases the protein level and activity of GPX4, which resembles the canonical ferroptosis-inducing pathway. On the other hand, WA induces excessive lipid peroxidation by increasing the labile Fe (II) pool upon HO-1 activation through direct targeting of Keap1, which represents for a novel noncanonical ferroptosis pathway (Hassannia et al., 2018). Erianin, a natural product isolated from a famous TCM, Dendrobium chrysotoxum Lindl, has been reported to exert anticancer effects on several cancer types. Erianin has shown to inhibit the growth and migration of lung cancer cells via Ca2+/CaM-mediated ferroptosis, accompanied by ROS accumulation, GSH depletion and lipid peroxidation (Chen P. et al., 2020). Cullen corylifolium is a famous TCM known as “Buguzhi” and listed officially in Chinese pharmcopoeia, containing various phytochemical compounds such as flavonoids and coumarines. The extracts have been approved to be effective for its anti-inflammatory, antioxidation, neuroprotection properties. Compound 3 (psoralidin) isolated from Cullen corylifolium displays tremendous inhibitory affinity for ALOX and Keap1-NRF2 protein-protein interactions, two important ferroptosis-related targets, and shows anti-ferroptotic activities on erastin-treated hippocampal HT22 cells (Yaseen et al., 2021). Nordihydroguaiaretic acid (NDGA), a lignin contained in Larrea tridentata, is a potent antioxidant. As a pan-ALOX inhibitor, NDGA effectively prevented lipid peroxidation, ROS generation and ferroptosis induced by proferroptotic reagents in BT-474, AML12 and acute lymphoblastic leukemia (ALL) cells (Hangauer et al., 2017; Probst et al., 2017; Fang et al., 2018).
Cooperation of Multiple Natural Compounds
In addition to single compound, cooperation of multiple (two or more) natural products or direct extracts of herbals and TCM formulations that are rich in various compounds, actually exhibits pro- or anti-ferroptosis pharmaco-activities. Cotylenin A (CN-A, plant growth regulator) combined with PEITC in the treatment of pancreatic cancer can synergistically induce ROS production and lead to cell death mainly due to the induction of ferroptosis, while CN-A or PEITC alone fails to induce ferroptosis (Kasukabe et al., 2016). Betula etnensis Raf (Birch Etna) belongs to the Betulaceae family, which contains multiple antineoplastic ingredients in bark, including polyphenols, terpenoids, betulin, betulinic acid, and ursolic acid. Methanolic extract of B. etnensis Raf. promotes ROS production and an oxidative cellular microenvironment, which results in the death of colon cancer cells dependent on ferroptosis mediated by HO-1 hyper-expression and lipid peroxidation (Malfa et al., 2019). Brown rice is known as an antioxidant-rich food with a high level of vitamin E. Methanol extracts of brown rice have been reported to be a ferroptosis inhibitor and protect cells against lipid peroxidation via compensating for GPX4 loss, suggesting that brown rice is useful for the pathologies of vascular diseases driven by lipid peroxidation (Sakai et al., 2017). Naotaifang is a compound traditional Chinese herbal medicine consisting of four herbs, namely, Radix Astragali (Huangqi), Rhizoma chuanxiong (Chuangxiong), Pheretima (Dilong) and Bombyx batryticatus (Jiangcan). Its extract (NTE) has clinical benefits for the neurological improvement of patients with acute cerebral ischemia. NTE can ameliorate neuronal ferroptosis in MCAO rats through the TFRC/DMT1 and SCL7A11/GPX4 pathways (Lan et al., 2020).
Clinical Therapeutic Potential of Natural Products Targeting Ferroptosis
Iron homeostasis, lipid peroxidation and GSH metabolism are three mainstays in the modulation of ferroptosis, and are supposed to be promising in ferroptosis-associated diseases. Based on physiopathological characteristics, proferroptotic compounds are potent anticancer candidates, whereas anti-ferroptotic agents are organo-protective therapies. Apart from chemical compounds, natural products, including TCM resources, which are rich in nature, provide abundant bioactive ingredients that modulate ferroptosis. Indeed, preclinical and clinical studies have demonstrated that many natural compounds with ferroptotic activity exhibit definite regulatory effects on the cyst(e)ine/GSH/GPX4 antioxidative axis (Figure 4). Of note, many of them, including artemisinin and its derivatives, β-elemene, SFN, baicalein, kaempferol, quercetin, curcumin and EGCG, have been evaluated in extensive clinical trials for various pharmacological effects (Wang Y. et al., 2017; Luo et al., 2019; Parvez and Rishi, 2019). Except for antimalarial effects, artemisinin and its derivatives seem to be good proferroptotic candidates and have been evidenced for their immune surveillance to inhibit carcinogenesis, tumor progression and metastasis (Kiani et al., 2020). For cancer therapy, a large body of studies have indicated that artemisinin and its derivatives trigger ferroptosis through overproducing ROS and regulating the xc-/GPX4 system axis in various cancer cells, which has undergone several clinical trials and is proven to be safe and well-tolerated in patients with metastatic breast cancer or advanced-stage solid tumors (von Hagens et al., 2017). Additionally, β-elemene (a sesquiterpene) injection has been approved recently as adjuvant therapy by China’s State Food and Drug Administration (SFDA) for lung cancer (Wang et al., 2012). Mechanistically, combinative treatment with β-elemene and cetuximab induces ferroptosis and suppresses CRC migration by regulating EMT through both GPX4-dependent and-independent pathways (Chen P. et al., 2020). Two ITCs, PEITC and sulforaphane protect cells from ferroptosis via limiting iron accumulation and preventing GPX4 degradation and have been investigated in clinical trials against various diseases ranging from cancer to autism, either alone or in combination with other drugs (Palliyaguru et al., 2018). In addition to proferroptotic cancer treatment, bioactive ferroptosis inhibitors, which are usually antioxidants that restore GSH/GPX4 levels, have also been clinically investigated to treat other diseases. EGCG, a famous antioxidant has been evaluated in numerous clinical trials for various disorders for decades, such as metabolic syndromes, neurodegenerative diseases and cardiovascular diseases. EGCG has been proven to protect pancreatic β cells against iron toxicity and erastin-induced ferroptosis through neutralizing iron and preventing GPX4 inactivation (Kose et al., 2019). Moreover, EGCG can also act as an iron chelator to regulate the TFRC/FTH expression and to prevent GPX4 activation, resulting in the inhibition of ferroptosis (Md Nesran et al., 2020). In addition to its antioxidative properties, EGCG is evidenced by clinical experience as an anticancer agent that modulates GPX4 levels to promote ferroptosis (Zhu et al., 2017). Owing to the context-dependent regulation of ferroptosis based on GPX4, EGCG has been recognized as a promising effective ferroptosis-modulating compound for clinical application or herbal supplementation.
Many natural compounds that are already in clinical use or have a strong potential for clinical translation are known to promote or block ferroptosis. Compared with classical ferroptosis regulators, TCM has the characteristics of many regulatory targets, stable structure, high safety, low cost and easy availability. However, it remains to be determined whether their biological activities rely on ferroptosis-regulating properties. The lack of specific biomarkers and molecular mechanisms for ferroptosis blocks the clinical transformation of novel ferroptosis-associated treatments mediated by natural product candidates. Due to starting late and accumulating less, more studies should be conducted to avoid side effects and to develop individualized and precise therapy.
Apart from the above discussed representative compounds, more natural products that have been reported to be able to induce/inhibit ferroptosis are classified and summarized in Tables 3, 4.
Conclusion and Perspectives
Nowadays, numerous studies have shown an ever-growing enthusiasm in exploring the role of ferroptosis in the occurrence and progression of various human diseases. A better understanding of the regulatory mechanisms underlying ferroptosis occurrence and susceptibility will facilitate the identification of better targets for the treatment of diversified diseases and the optimization of clinical prevention, diagnostics, prognostics and treatment. Therefore, the activation of ferroptosis-related sites can induce not only the death of ordinary tumor cells, but also the death of drug-resistant tumor cells. In other diseases, inhibiting the occurrence of ferroptosis can effectively alleviate the disease, offering a novel concept for future drug treatment and development. Despite the rapid growth of ferroptosis research, there are some insurmountable and inevitable challenges that remain to be resolved. First, many puzzles lie in ferroptosis-associated complicated mechanisms and signaling pathways that need to be further explored, although they indeed provide some constructive and meaningful answers for a multitude of questions regarding the survival and death of cells. It should be noted that ferroptosis also leads to unexpected toxicity and injury, raising awareness that such ferroptosis-modulating therapies need careful consideration and conduction. Additionally, robust and unique biomarkers of ferroptosis, analogous to active caspase-3 for apoptosis, have not yet been discovered and identified until now to guide the appropriate therapy for human pathologies. Instead, evaluating and validating a whole cascade of biochemical and genetic/proteic changes to facilitate the detection and tracking ferroptosis will be necessary to definitively distinguish between distinct forms of RCD.
Although some progress has been made, inconsistent findings are frequently observed in cells, organoids, animals, and even patients (Conrad and Pratt, 2019). As mentioned above, most clinical trials on administering iron chelators and antioxidants have showed only moderate treatment effect, compared to corresponding preclinical studies (Grolez et al., 2015). DFP seems to somewhat slow disease progression and improve quality of life, but they have not reach significance (Martin-Bastida et al., 2017; Klopstock et al., 2019). Unlike mice with a deadly global GPX4-deficiency, SLC7A11-deficient mice have a normal lifespan and do not display any major phenotype develop (Sato et al., 2005; Conrad and Pratt, 2019). However, almost all cells isolated from these mice die rapidly on account of cysteine starvation, GSH depletion, lipid peroxidation and ferroptotic effects that be induced by erastin or other system xc--inhibitors. Actully, cysteine is mainly present in the culture medium in its oxidized dimeric form, cystine which can be transported into cells only by system xc-. Whereas, cysteine is still available in its reduced monomeric form in plasma and other extracellular body fluids in a whole organism, which can be uptaken by cells via neutral amino acid transporters instead of system xc- (Conrad and Pratt, 2019). Collectively, the relevance of results from various models require for careful evaluation, owing to the physiological, histological and enzymological differences on specific conditions.
As listed and discussed, some synthetic drugs and natural products have suggested to overcome and treat diseases by regulating ferroptosis or mixed death mainly in preclinical models. However, a general lack of animal experiments and clinical trials limit their further development as future targeted drugs with ferroptotic properties. Particularly, we have shed light on the potential of natural compounds with multiple targets, multiple mechanisms and relatively safe profiles as ferroptosis inducers or inhibitors for (adjuvant) therapy of diseases from the perspective of integration. Although there is growing evidence that natural products are ideal templates for both single ingredient and multiple-component modern drugs, they are faced with many dilemmas and challenges, including productivity problems, poor pharmacokinetics, and unclear pharmacology. Further studies should be conducted to elaborate the pharmacokinetic properties both in vitro and in vivo to optimize the best functional ingredients and dosage for clinical therapy.
Author Contributions
YZ, DF, and JA designed the framework and content of the review. CG, SZ and HM wrote the original review draft. SZ, ZT, XH, CX and JZ revised the article with feedback from all the co-authors. All authors approved this work for publication.
Funding
This work was supported by the National Natural Science Foundation of China (No.81803624, 81803622), China Postdoctoral Science Foundation (No. 2018M640498) and the Jiangsu postdoctoral grant program (No. 2018Z092).
Conflict of Interest
DF was employed by Nanjing Southern Pharmaceutical Technology Co., Ltd.
The remaining authors declare that the research was conducted in the absence of any commercial or financial relationships that could be construed as a potential conflict of interest.
Publisher’s Note
All claims expressed in this article are solely those of the authors and do not necessarily represent those of their affiliated organizations, or those of the publisher, the editors and the reviewers. Any product that may be evaluated in this article, or claim that may be made by its manufacturer, is not guaranteed or endorsed by the publisher.
Supplementary Material
The Supplementary Material for this article can be found online at: https://www.frontiersin.org/articles/10.3389/fcell.2021.774957/full#supplementary-material
References
Alvarez, S. W., Sviderskiy, V. O., Terzi, E. M., Papagiannakopoulos, T., Moreira, A. L., Adams, S., et al. (2017). NFS1 Undergoes Positive Selection in Lung Tumours and Protects Cells from Ferroptosis. Nature 551, 639–643. doi:10.1038/nature24637
Amable, L. (2016). Cisplatin Resistance and Opportunities for Precision Medicine. Pharmacol. Res. 106, 27–36. doi:10.1016/j.phrs.2016.01.001
Aydinok, Y., Kattamis, A., Cappellini, M. D., El-Beshlawy, A., Origa, R., Elalfy, M., et al. (2015). Effects of Deferasirox-Deferoxamine on Myocardial and Liver Iron in Patients with Severe Transfusional Iron Overload. Blood 125, 3868–3877. doi:10.1182/blood-2014-07-586677
Ayton, S., Faux, N. G., Faux, N. G., and Bush, A. I. (2015). Ferritin Levels in the Cerebrospinal Fluid Predict Alzheimer's Disease Outcomes and Are Regulated by APOE. Nat. Commun. 6, 6760. doi:10.1038/ncomms7760
Baba, Y., Higa, J. K., Shimada, B. K., Horiuchi, K. M., Suhara, T., Kobayashi, M., et al. (2018). Protective Effects of the Mechanistic Target of Rapamycin against Excess Iron and Ferroptosis in Cardiomyocytes. Am. J. Physiology-Heart Circulatory Physiol. 314, H659–H668. doi:10.1152/ajpheart.00452.2017
Bagheri, B., Shokrzadeh, M., Mokhberi, V., Azizi, S., Khalilian, A., Akbari, N., et al. (2013). Association between Serum Iron and the Severity of Coronary Artery Disease. Int. Cardiovasc. Res. J. 7, 95–98.
Bai, T., Lei, P., Zhou, H., Liang, R., Zhu, R., Wang, W., et al. (2019). Sigma‐1 Receptor Protects against Ferroptosis in Hepatocellular Carcinoma Cells. J. Cel Mol Med 23, 7349–7359. doi:10.1111/jcmm.14594
Bai, T., Wang, S., Zhao, Y., Zhu, R., Wang, W., and Sun, Y. (2017). Haloperidol, a Sigma Receptor 1 Antagonist, Promotes Ferroptosis in Hepatocellular Carcinoma Cells. Biochem. biophysical Res. Commun. 491, 919–925. doi:10.1016/j.bbrc.2017.07.136
Basit, F., van Oppen, L. M., Schöckel, L., Bossenbroek, H. M., van Emst-de Vries, S. E., Hermeling, J. C., et al. (2017). Mitochondrial Complex I Inhibition Triggers a Mitophagy-dependent ROS Increase Leading to Necroptosis and Ferroptosis in Melanoma Cells. Cell Death Dis 8, e2716. doi:10.1038/cddis.2017.133
Belaidi, A. A., and Bush, A. I. (2016). Iron Neurochemistry in Alzheimer's Disease and Parkinson's Disease: Targets for Therapeutics. J. Neurochem. 139 (Suppl. 1), 179–197. doi:10.1111/jnc.13425
Belavgeni, A., Bornstein, S. R., von Mässenhausen, A., Tonnus, W., Stumpf, J., Meyer, C., et al. (2019). Exquisite Sensitivity of Adrenocortical Carcinomas to Induction of Ferroptosis. Proc. Natl. Acad. Sci. USA 116, 22269–22274. doi:10.1073/pnas.1912700116
Bersuker, K., Hendricks, J. M., Li, Z., Magtanong, L., Ford, B., Tang, P. H., et al. (2019). The CoQ Oxidoreductase FSP1 Acts Parallel to GPX4 to Inhibit Ferroptosis. Nature 575, 688–692. doi:10.1038/s41586-019-1705-2
Bock, F. J., and Tait, S. W. G. (2019). Mitochondria as Multifaceted Regulators of Cell Death. Nat. Rev. Mol. Cel Biol. 21, 85–100. doi:10.1038/s41580-019-0173-8
Bueno, D. C., Canto, R. F. S., de Souza, V., Andreguetti, R. R., Barbosa, F. A. R., Naime, A. A., et al. (2020). New Probucol Analogues Inhibit Ferroptosis, Improve Mitochondrial Parameters, and Induce Glutathione Peroxidase in HT22 Cells. Mol. Neurobiol. 57, 3273–3290. doi:10.1007/s12035-020-01956-9
Canli, Ö., Alankuş, Y. B., Grootjans, S., Vegi, N., Hültner, L., Hoppe, P. S., et al. (2016). Glutathione Peroxidase 4 Prevents Necroptosis in Mouse Erythroid Precursors. Blood 127, 139–148. doi:10.1182/blood-2015-06-654194
Carbone, M., Yang, H., Pass, H. I., Krausz, T., Testa, J. R., and Gaudino, G. (2013). BAP1 and Cancer. Nat. Rev. Cancer 13, 153–159. doi:10.1038/nrc3459
Chan, H. W., Singh, N. P., and Lai, H. C. (2013). Cytotoxicity of Dihydroartemisinin toward Molt-4 Cells Attenuated by N-Tert-Butyl-Alpha-Phenylnitrone and Deferoxamine. Anticancer Res. 33, 4389–4393.
Chen, D., Tavana, O., Chu, B., Erber, L., Chen, Y., Baer, R., et al. (2017). NRF2 Is a Major Target of ARF in P53-independent Tumor Suppression. Mol. Cel. 68, 224–232. e4. doi:10.1016/j.molcel.2017.09.009
Chen, X., Li, D., Sun, H. Y., Wang, W. W., Wu, H., Kong, W., et al. (2020). Relieving Ferroptosis May Partially Reverse Neurodegeneration of the Auditory Cortex. Febs J. 287, 4747–4766. doi:10.1111/febs.15266
Chen, G.-Q., Benthani, F. A., Wu, J., Liang, D., Bian, Z.-X., and Jiang, X. (2020). Artemisinin Compounds Sensitize Cancer Cells to Ferroptosis by Regulating Iron Homeostasis. Cell Death Differ 27, 242–254. doi:10.1038/s41418-019-0352-3
Chen, Y., Li, N., Wang, H., Wang, N., Peng, H., Wang, J., et al. (2020). Amentoflavone Suppresses Cell Proliferation and Induces Cell Death through Triggering Autophagy-dependent Ferroptosis in Human Glioma. Life Sci. 247, 117425. doi:10.1016/j.lfs.2020.117425
Chen, P., Wu, Q., Feng, J., Yan, L., Sun, Y., Liu, S., et al. (2020). Erianin, a Novel Dibenzyl Compound in Dendrobium Extract, Inhibits Lung Cancer Cell Growth and Migration via Calcium/calmodulin-dependent Ferroptosis. Sig Transduct Target. Ther. 5, 51. doi:10.1038/s41392-020-0149-3
Chen, P., Li, X., Zhang, R., Liu, S., Xiang, Y., Zhang, M., et al. (2020). Combinative Treatment of β-elemene and Cetuximab Is Sensitive to KRAS Mutant Colorectal Cancer Cells by Inducing Ferroptosis and Inhibiting Epithelial-Mesenchymal Transformation. Theranostics 10, 5107–5119. doi:10.7150/thno.44705
Chen, J., Marks, E., Lai, B., Zhang, Z., Duce, J. A., Lam, L. Q., et al. (2013). Iron Accumulates in Huntington's Disease Neurons: protection by Deferoxamine. PLoS One 8, e77023. doi:10.1371/journal.pone.0077023
Chen, L., Li, X., Liu, L., Yu, B., Xue, Y., and Liu, Y. (2015). Erastin Sensitizes Glioblastoma Cells to Temozolomide by Restraining xCT and Cystathionine-γ-Lyase Function. Oncol. Rep. 33, 1465–1474. doi:10.3892/or.2015.3712
Chen, W.-C., Wang, C.-Y., Hung, Y.-H., Weng, T.-Y., Yen, M.-C., and Lai, M.-D. (2016). Systematic Analysis of Gene Expression Alterations and Clinical Outcomes for Long-Chain Acyl-Coenzyme A Synthetase Family in Cancer. PLoS One 11, e0155660. doi:10.1371/journal.pone.0155660
Chen, Y., Mi, Y., Zhang, X., Ma, Q., Song, Y., Zhang, L., et al. (2019). Dihydroartemisinin-induced Unfolded Protein Response Feedback Attenuates Ferroptosis via PERK/ATF4/HSPA5 Pathway in Glioma Cells. J. Exp. Clin. Cancer Res. 38, 402. doi:10.1186/s13046-019-1413-7
Chu, B., Kon, N., Chen, D., Li, T., Liu, T., Jiang, L., et al. (2019). ALOX12 Is Required for P53-Mediated Tumour Suppression through a Distinct Ferroptosis Pathway. Nat. Cel Biol 21, 579–591. doi:10.1038/s41556-019-0305-6
Colakoglu, M., Tuncer, S., and Banerjee, S. (2018). Emerging Cellular Functions of the Lipid Metabolizing Enzyme 15-Lipoxygenase-1. Cel Prolif. 51, e12472. doi:10.1111/cpr.12472
Conrad, M., and Pratt, D. A. (2019). The Chemical Basis of Ferroptosis. Nat. Chem. Biol. 15, 1137–1147. doi:10.1038/s41589-019-0408-1
Cotticelli, M. G., Xia, S., Lin, D., Lee, T., Terrab, L., Wipf, P., et al. (2019). Ferroptosis as a Novel Therapeutic Target for Friedreich's Ataxia. J. Pharmacol. Exp. Ther. 369, 47–54. doi:10.1124/jpet.118.252759
Crielaard, B. J., Lammers, T., and Rivella, S. (2017). Targeting Iron Metabolism in Drug Discovery and Delivery. Nat. Rev. Drug Discov. 16, 400–423. doi:10.1038/nrd.2016.248
Cui, Z., Zhong, Z., Yang, Y., Wang, B., Sun, Y., Sun, Q., et al. (2016). Ferrous Iron Induces Nrf2 Expression in Mouse Brain Astrocytes to Prevent Neurotoxicity. J. Biochem. Mol. Toxicol. 30, 396–403. doi:10.1002/jbt.21803
Curti, V., Di Lorenzo, A., Dacrema, M., Xiao, J., Nabavi, S. M., and Daglia, M. (2017). In Vitro polyphenol Effects on Apoptosis: An Update of Literature Data. Semin. Cancer Biol. 46, 119–131. doi:10.1016/j.semcancer.2017.08.005
Daher, B., Parks, S. K., Durivault, J., Cormerais, Y., Baidarjad, H., Tambutte, E., et al. (2019). Genetic Ablation of the Cystine Transporter xCT in PDAC Cells Inhibits mTORC1, Growth, Survival, and Tumor Formation via Nutrient and Oxidative Stresses. Cancer Res. 79, 3877–3890. doi:10.1158/0008-5472.can-18-3855
Devos, D., Moreau, C., Devedjian, J. C., Kluza, J., Petrault, M., Laloux, C., et al. (2014). Targeting Chelatable Iron as a Therapeutic Modality in Parkinson's Disease. Antioxid. Redox Signaling 21, 195–210. doi:10.1089/ars.2013.5593
Ding, C.-K. C., Rose, J., Sun, T., Wu, J., Chen, P.-H., Lin, C.-C., et al. (2020). MESH1 Is a Cytosolic NADPH Phosphatase that Regulates Ferroptosis. Nat. Metab. 2, 270–277. doi:10.1038/s42255-020-0181-1
Dixon, S. J., Patel, D. N., Welsch, M., Skouta, R., Lee, E. D., Hayano, M., et al. (2014). Pharmacological Inhibition of Cystine-Glutamate Exchange Induces Endoplasmic Reticulum Stress and Ferroptosis. Elife 3, e02523. doi:10.7554/eLife.02523
Dixon, S. J., Lemberg, K. M., Lamprecht, M. R., Skouta, R., Zaitsev, E. M., Gleason, C. E., et al. (2012). Ferroptosis: an Iron-dependent Form of Nonapoptotic Cell Death. Cell 149, 1060–1072. doi:10.1016/j.cell.2012.03.042
Dixon, S. J., and Stockwell, B. R. (2014). The Role of Iron and Reactive Oxygen Species in Cell Death. Nat. Chem. Biol. 10, 9–17. doi:10.1038/nchembio.1416
Do Van, B., Gouel, F., Jonneaux, A., Timmerman, K., Gelé, P., Pétrault, M., et al. (2016). Ferroptosis, a Newly Characterized Form of Cell Death in Parkinson's Disease that Is Regulated by PKC. Neurobiol. Dis. 94, 169–178. doi:10.1016/j.nbd.2016.05.011
Doll, S., Freitas, F. P., Shah, R., Aldrovandi, M., da Silva, M. C., Ingold, I., et al. (2019). FSP1 Is a Glutathione-independent Ferroptosis Suppressor. Nature 575, 693–698. doi:10.1038/s41586-019-1707-0
Doll, S., and Conrad, M. (2017). Iron and Ferroptosis: A Still Ill‐defined Liaison. IUBMB Life 69, 423–434. doi:10.1002/iub.1616
Doll, S., Proneth, B., Tyurina, Y. Y., Panzilius, E., Kobayashi, S., Ingold, I., et al. (2017). ACSL4 Dictates Ferroptosis Sensitivity by Shaping Cellular Lipid Composition. Nat. Chem. Biol. 13, 91–98. doi:10.1038/nchembio.2239
Dolma, S., Lessnick, S. L., Hahn, W. C., and Stockwell, B. R. (2003). Identification of Genotype-Selective Antitumor Agents Using Synthetic Lethal Chemical Screening in Engineered Human Tumor Cells. Cancer cell 3, 285–296. doi:10.1016/s1535-6108(03)00050-3
Dondorp, A., Nosten, F., Stepniewska, K., Day, N., and White, N. (2005). Artesunate versus Quinine for Treatment of Severe Falciparum Malaria: a Randomised Trial. Lancet 366, 717–725. doi:10.1016/S0140-6736(05)67176-0
Dostalikova-Cimburova, M., Balusikova, K., Kratka, K., Chmelikova, J., Hejda, V., Hnanicek, J., et al. (2014). Role of Duodenal Iron Transporters and Hepcidin in Patients with Alcoholic Liver Disease. J. Cel Mol Med 18, 1840–1850. doi:10.1111/jcmm.12310
Du, J., Wang, T., Li, Y., Zhou, Y., Wang, X., Yu, X., et al. (2019). DHA Inhibits Proliferation and Induces Ferroptosis of Leukemia Cells through Autophagy Dependent Degradation of Ferritin. Free Radic. Biol. Med. 131, 356–369. doi:10.1016/j.freeradbiomed.2018.12.011
Eling, N., Reuter, L., Hazin, J., Hamacher-Brady, A., and Brady, N. R. (2015). Identification of Artesunate as a Specific Activator of Ferroptosis in Pancreatic Cancer Cells. Oncoscience 2, 517–532. doi:10.18632/oncoscience.160
Fan, Z., Wirth, A.-K., Chen, D., Wruck, C. J., Rauh, M., Buchfelder, M., et al. (2017). Nrf2-Keap1 Pathway Promotes Cell Proliferation and Diminishes Ferroptosis. Oncogenesis 6, e371. doi:10.1038/oncsis.2017.65
Fang, S., Yu, X., Ding, H., Han, J., and Feng, J. (2018). Effects of Intracellular Iron Overload on Cell Death and Identification of Potent Cell Death Inhibitors. Biochem. biophysical Res. Commun. 503, 297–303. doi:10.1016/j.bbrc.2018.06.019
Fang, X., Cai, Z., Wang, H., Han, D., Cheng, Q., Zhang, P., et al. (2020). Loss of Cardiac Ferritin H Facilitates Cardiomyopathy via Slc7a11-Mediated Ferroptosis. Circ. Res. 127, 486–501. doi:10.1161/circresaha.120.316509
Fang, X., Wang, H., Han, D., Xie, E., Yang, X., Wei, J., et al. (2019). Ferroptosis as a Target for protection against Cardiomyopathy. Proc. Natl. Acad. Sci. USA 116, 2672–2680. doi:10.1073/pnas.1821022116
Fischer, W., Currais, A., Liang, Z., Pinto, A., and Maher, P. (2019). Old Age-Associated Phenotypic Screening for Alzheimer's Disease Drug Candidates Identifies Sterubin as a Potent Neuroprotective Compound from Yerba Santa. Redox Biol. 21, 101089. doi:10.1016/j.redox.2018.101089
Fonseca-Nunes, A., Jakszyn, P., and Agudo, A. (2014). Iron and Cancer Risk-A Systematic Review and Meta-Analysis of the Epidemiological Evidence : a Publication of the American Association for Cancer Research, Cosponsored by the American Society of Preventive. Cancer Epidemiol. Biomarkers Prev. 23, 12–31. doi:10.1158/1055-9965.epi-13-0733
Friedmann Angeli, J. P., Krysko, D. V., and Conrad, M. (2019). Ferroptosis at the Crossroads of Cancer-Acquired Drug Resistance and Immune Evasion. Nat. Rev. Cancer 19, 405–414. doi:10.1038/s41568-019-0149-1
Friedmann Angeli, J. P., Schneider, M., Proneth, B., Tyurina, Y. Y., Tyurin, V. A., Hammond, V. J., et al. (2014). Inactivation of the Ferroptosis Regulator Gpx4 Triggers Acute Renal Failure in Mice. Nat. Cel Biol 16, 1180–1191. doi:10.1038/ncb3064
Fuchs, Y., and Steller, H. (2011). Programmed Cell Death in Animal Development and Disease. Cell 147, 742–758. doi:10.1016/j.cell.2011.10.033
Galluzzi, L., Bravo-San Pedro, J. M., Vitale, I., Aaronson, S. A., Abrams, J. M., Adam, D., et al. (2015). Essential versus Accessory Aspects of Cell Death: Recommendations of the NCCD 2015. Cel Death Differ 22, 58–73. doi:10.1038/cdd.2014.137
Gao, H., Bai, Y., Jia, Y., Zhao, Y., Kang, R., Tang, D., et al. (2018). Ferroptosis Is a Lysosomal Cell Death Process. Biochem. biophysical Res. Commun. 503, 1550–1556. doi:10.1016/j.bbrc.2018.07.078
Gao, M., Monian, P., Pan, Q., Zhang, W., Xiang, J., and Jiang, X. (2016). Ferroptosis Is an Autophagic Cell Death Process. Cell Res 26, 1021–1032. doi:10.1038/cr.2016.95
Gao, M., Monian, P., Quadri, N., Ramasamy, R., and Jiang, X. (2015). Glutaminolysis and Transferrin Regulate Ferroptosis. Mol. Cel. 59, 298–308. doi:10.1016/j.molcel.2015.06.011
Gao, M., Yi, J., Zhu, J., Minikes, A. M., Monian, P., Thompson, C. B., et al. (2019). Role of Mitochondria in Ferroptosis. Mol. Cel. 73, 354–363. e3. doi:10.1016/j.molcel.2018.10.042
Gao, Z., Deng, G., Li, Y., Huang, H., Sun, X., Shi, H., et al. (2020). Actinidia Chinensis Planch Prevents Proliferation and Migration of Gastric Cancer Associated with Apoptosis, Ferroptosis Activation and Mesenchymal Phenotype Suppression. Biomed. Pharmacother. 126, 110092. doi:10.1016/j.biopha.2020.110092
Gaschler, M. M., Andia, A. A., Liu, H., Csuka, J. M., Hurlocker, B., Vaiana, C. A., et al. (2018a). FINO2 Initiates Ferroptosis through GPX4 Inactivation and Iron Oxidation. Nat. Chem. Biol. 14, 507–515. doi:10.1038/s41589-018-0031-6
Gaschler, M. M., Hu, F., Feng, H., Linkermann, A., Min, W., and Stockwell, B. R. (2018b). Determination of the Subcellular Localization and Mechanism of Action of Ferrostatins in Suppressing Ferroptosis. ACS Chem. Biol. 13, 1013–1020. doi:10.1021/acschembio.8b00199
Greenshields, A. L., Shepherd, T. G., and Hoskin, D. W. (2017). Contribution of Reactive Oxygen Species to Ovarian Cancer Cell Growth Arrest and Killing by the Anti-malarial Drug Artesunate. Mol. Carcinog. 56, 75–93. doi:10.1002/mc.22474
Grolez, G., Moreau, C., Sablonnière, B., Garçon, G., Devedjian, J.-C., Meguig, S., et al. (2015). Ceruloplasmin Activity and Iron Chelation Treatment of Patients with Parkinson's Disease. BMC Neurol. 15, 74. doi:10.1186/s12883-015-0331-3
Guerrero-Hue, M., García-Caballero, C., Palomino-Antolín, A., Rubio-Navarro, A., Vázquez-Carballo, C., Herencia, C., et al. (2019). Curcumin Reduces Renal Damage Associated with Rhabdomyolysis by Decreasing Ferroptosis-Mediated Cell Death. Faseb j 33, 8961–8975. doi:10.1096/fj.201900077R
Gunesch, S., Hoffmann, M., Kiermeier, C., Fischer, W., Pinto, A. F. M., Maurice, T., et al. (2020). 7-O-Esters of Taxifolin with Pronounced and Overadditive Effects in Neuroprotection, Anti-neuroinflammation, and Amelioration of Short-Term Memory Impairment In Vivo. Redox Biol. 29, 101378. doi:10.1016/j.redox.2019.101378
Guo, C., Hao, L.-J., Yang, Z.-H., Chai, R., Zhang, S., Gu, Y., et al. (2016). Deferoxamine-mediated Up-Regulation of HIF-1α Prevents Dopaminergic Neuronal Death via the Activation of MAPK Family Proteins in MPTP-Treated Mice. Exp. Neurol. 280, 13–23. doi:10.1016/j.expneurol.2016.03.016
Guo, J., Xu, B., Han, Q., Zhou, H., Xia, Y., Gong, C., et al. (2018a). Ferroptosis: A Novel Anti-tumor Action for Cisplatin. Cancer Res. Treat. 50, 445–460. doi:10.4143/crt.2016.572
Guo, J., Xu, B., Han, Q., Zhou, H., Xia, Y., Gong, C., et al. (2018b). Ferroptosis: A Novel Anti-tumor Action for Cisplatin. Cancer Res. Treat. 50, 445–460. doi:10.4143/crt.2016.572
Guo, Z., Ran, Q., Roberts, L. J., Zhou, L., Richardson, A., Sharan, C., et al. (2008). Suppression of Atherogenesis by Overexpression of Glutathione Peroxidase-4 in Apolipoprotein E-Deficient Mice. Free Radic. Biol. Med. 44, 343–352. doi:10.1016/j.freeradbiomed.2007.09.009
Habashy, H. O., Powe, D. G., Staka, C. M., Rakha, E. A., Ball, G., Green, A. R., et al. (2010). Transferrin Receptor (CD71) Is a Marker of Poor Prognosis in Breast Cancer and Can Predict Response to Tamoxifen. Breast Cancer Res. Treat. 119, 283–293. doi:10.1007/s10549-009-0345-x
Hamaï, A., Cañeque, T., Müller, S., Mai, T. T., Hienzsch, A., Ginestier, C., et al. (2017). An Iron Hand over Cancer Stem Cells. Autophagy 13, 1465–1466. doi:10.1080/15548627.2017.1327104
Hambright, W. S., Fonseca, R. S., Chen, L., Na, R., and Ran, Q. (2017). Ablation of Ferroptosis Regulator Glutathione Peroxidase 4 in Forebrain Neurons Promotes Cognitive Impairment and Neurodegeneration. Redox Biol. 12, 8–17. doi:10.1016/j.redox.2017.01.021
Hangauer, M. J., Viswanathan, V. S., Ryan, M. J., Bole, D., Eaton, J. K., Matov, A., et al. (2017). Drug-tolerant Persister Cancer Cells Are Vulnerable to GPX4 Inhibition. Nature 551, 247–250. doi:10.1038/nature24297
Hao, S., Yu, J., He, W., Huang, Q., Zhao, Y., Liang, B., et al. (2017). Cysteine Dioxygenase 1 Mediates Erastin-Induced Ferroptosis in Human Gastric Cancer Cells. Neoplasia 19, 1022–1032. doi:10.1016/j.neo.2017.10.005
Hassannia, B., Vandenabeele, P., and Vanden Berghe, T. (2019). Targeting Ferroptosis to Iron Out Cancer. Cancer cell 35, 830–849. doi:10.1016/j.ccell.2019.04.002
Hassannia, B., Wiernicki, B., Ingold, I., Qu, F., Van Herck, S., Tyurina, Y. Y., et al. (2018). Nano-targeted Induction of Dual Ferroptotic Mechanisms Eradicates High-Risk Neuroblastoma. J. Clin. Invest. 128, 3341–3355. doi:10.1172/jci99032
Hayano, M., Yang, W. S., Corn, C. K., Pagano, N. C., and Stockwell, B. R. (2016). Loss of Cysteinyl-tRNA Synthetase (CARS) Induces the Transsulfuration Pathway and Inhibits Ferroptosis Induced by Cystine Deprivation. Cel Death Differ 23, 270–278. doi:10.1038/cdd.2015.93
Holohan, C., Van Schaeybroeck, S., Longley, D. B., and Johnston, P. G. (2013). Cancer Drug Resistance: an Evolving Paradigm. Nat. Rev. Cancer 13, 714–726. doi:10.1038/nrc3599
Hou, W., Xie, Y., Song, X., Sun, X., Lotze, M. T., Zeh, H. J., et al. (2016). Autophagy Promotes Ferroptosis by Degradation of Ferritin. Autophagy 12, 1425–1428. doi:10.1080/15548627.2016.1187366
Houessinon, A., François, C., Sauzay, C., Louandre, C., Mongelard, G., Godin, C., et al. (2016). Metallothionein-1 as a Biomarker of Altered Redox Metabolism in Hepatocellular Carcinoma Cells Exposed to Sorafenib. Mol. Cancer 15, 38. doi:10.1186/s12943-016-0526-2
Hu, C. L., Nydes, M., Shanley, K. L., Morales Pantoja, I. E., Howard, T. A., and Bizzozero, O. A. (2019). Reduced Expression of the Ferroptosis Inhibitor Glutathione Peroxidase‐4 in Multiple Sclerosis and Experimental Autoimmune Encephalomyelitis. J. Neurochem. 148, 426–439. doi:10.1111/jnc.14604
Isani, G., Bertocchi, M., Andreani, G., Farruggia, G., Cappadone, C., Salaroli, R., et al. (2019). Cytotoxic Effects of Artemisia Annua L. And Pure Artemisinin on the D-17 Canine Osteosarcoma Cell Line. Oxid Med. Cel Longev 2019, 1615758. doi:10.1155/2019/1615758
Jennis, M., Kung, C.-P., Basu, S., Budina-Kolomets, A., Leu, J. I.-J., Khaku, S., et al. (2016). An African-specific Polymorphism in the TP53 Gene Impairs P53 Tumor Suppressor Function in a Mouse Model. Genes Dev. 30, 918–930. doi:10.1101/gad.275891.115
Jhelum, P., Santos-Nogueira, E., Teo, W., Haumont, A., Lenoël, I., Stys, P. K., et al. (2020). Ferroptosis Mediates Cuprizone-Induced Loss of Oligodendrocytes and Demyelination. J. Neurosci. 40, 9327–9341. doi:10.1523/jneurosci.1749-20.2020
Ji, Y., Kuo, Y., and Morris, M. E. (2005). Pharmacokinetics of Dietary Phenethyl Isothiocyanate in Rats. Pharm. Res. 22, 1658–1666. doi:10.1007/s11095-005-7097-z
Jiang, L., Kon, N., Li, T., Wang, S.-J., Su, T., Hibshoosh, H., et al. (2015). Ferroptosis as a P53-Mediated Activity during Tumour Suppression. Nature 520, 57–62. doi:10.1038/nature14344
Jin, M., Shi, C., Li, T., Wu, Y., Hu, C., and Huang, G. (2020). Solasonine Promotes Ferroptosis of Hepatoma Carcinoma Cells via Glutathione Peroxidase 4-induced Destruction of the Glutathione Redox System. Biomed. Pharmacother. 129, 110282. doi:10.1016/j.biopha.2020.110282
Kagan, V. E., Mao, G., Qu, F., Angeli, J. P. F., Doll, S., Croix, C. S., et al. (2017). Oxidized Arachidonic and Adrenic PEs Navigate Cells to Ferroptosis. Nat. Chem. Biol. 13, 81–90. doi:10.1038/nchembio.2238
Karuppagounder, S. S., Alin, L., Chen, Y., Brand, D., Bourassa, M. W., Dietrich, K., et al. (2018). N-acetylcysteine Targets 5 Lipoxygenase-Derived, Toxic Lipids and Can Synergize with Prostaglandin E2to Inhibit Ferroptosis and Improve Outcomes Following Hemorrhagic Stroke in Mice. Ann. Neurol. 84, 854–872. doi:10.1002/ana.25356
Kasukabe, T., Honma, Y., Okabe-Kado, J., Higuchi, Y., Kato, N., and Kumakura, S. (2016). Combined Treatment with Cotylenin A and Phenethyl Isothiocyanate Induces strong Antitumor Activity Mainly through the Induction of Ferroptotic Cell Death in Human Pancreatic Cancer Cells. Oncol. Rep. 36, 968–976. doi:10.3892/or.2016.4867
Kenny, E. M., Fidan, E., Yang, Q., Anthonymuthu, T. S., New, L. A., Meyer, E. A., et al. (2019). Ferroptosis Contributes to Neuronal Death and Functional Outcome after Traumatic Brain Injury*. Crit. Care Med. 47, 410–418. doi:10.1097/ccm.0000000000003555
Khorsandi, K., Kianmehr, Z., Hosseinmardi, Z., and Hosseinzadeh, R. (2020). Anti-cancer Effect of Gallic Acid in Presence of Low Level Laser Irradiation: ROS Production and Induction of Apoptosis and Ferroptosis. Cancer Cel Int 20, 18. doi:10.1186/s12935-020-1100-y
Kiani, B. H., Kayani, W. K., Khayam, A. U., Dilshad, E., Ismail, H., and Mirza, B. (2020). Artemisinin and its Derivatives: a Promising Cancer Therapy. Mol. Biol. Rep. 47, 6321–6336. doi:10.1007/s11033-020-05669-z
Kibble, M., Saarinen, N., Tang, J., Wennerberg, K., Mäkelä, S., and Aittokallio, T. (2015). Network Pharmacology Applications to Map the Unexplored Target Space and Therapeutic Potential of Natural Products. Nat. Prod. Rep. 32, 1249–1266. doi:10.1039/c5np00005j
Kim, E. H., Shin, D., Lee, J., Jung, A. R., and Roh, J.-L. (2018). CISD2 Inhibition Overcomes Resistance to Sulfasalazine-Induced Ferroptotic Cell Death in Head and Neck Cancer. Cancer Lett. 432, 180–190. doi:10.1016/j.canlet.2018.06.018
Kinoshita, H., Okabe, H., Beppu, T., Chikamoto, A., Hayashi, H., Imai, K., et al. (2013). Cystine/glutamic Acid Transporter Is a Novel Marker for Predicting Poor Survival in Patients with Hepatocellular Carcinoma. Oncol. Rep. 29, 685–689. doi:10.3892/or.2012.2162
Klepac, N., Relja, M., Klepac, R., Hećimović, S., Babić, T., and Trkulja, V. (2007). Oxidative Stress Parameters in Plasma of Huntington's Disease Patients, Asymptomatic Huntington's Disease Gene Carriers and Healthy Subjects. J. Neurol. 254, 1676–1683. doi:10.1007/s00415-007-0611-y
Klopstock, T., Tricta, F., Neumayr, L., Karin, I., Zorzi, G., Fradette, C., et al. (2019). Safety and Efficacy of Deferiprone for Pantothenate Kinase-Associated Neurodegeneration: a Randomised, Double-Blind, Controlled Trial and an Open-Label Extension Study. Lancet Neurol. 18, 631–642. doi:10.1016/s1474-4422(19)30142-5
Kong, Z., Liu, R., and Cheng, Y. (2019). Artesunate Alleviates Liver Fibrosis by Regulating Ferroptosis Signaling Pathway. Biomed. Pharmacother. 109, 2043–2053. doi:10.1016/j.biopha.2018.11.030
Kose, T., Vera-Aviles, M., Sharp, P. A., and Latunde-Dada, G. O. (2019). Curcumin and (-)- Epigallocatechin-3-Gallate Protect Murine MIN6 Pancreatic Beta-Cells against Iron Toxicity and Erastin-Induced Ferroptosis. Pharmaceuticals (Basel) 12, 26. doi:10.3390/ph12010026
Lachaier, E., Louandre, C., Godin, C., Saidak, Z., Baert, M., Diouf, M., et al. (2014). Sorafenib Induces Ferroptosis in Human Cancer Cell Lines Originating from Different Solid Tumors. Anticancer Res. 34, 6417–6422.
Lakhal-Littleton, S., Wolna, M., Chung, Y. J., Christian, H. C., Heather, L. C., Brescia, M., et al. (2016). An Essential Cell-Autonomous Role for Hepcidin in Cardiac Iron Homeostasis. Elife 5. doi:10.7554/eLife.19804
Lakhal-Littleton, S., Wolna, M., Carr, C. A., Miller, J. J. J., Christian, H. C., Ball, V., et al. (2015). Cardiac Ferroportin Regulates Cellular Iron Homeostasis and Is Important for Cardiac Function. Proc. Natl. Acad. Sci. USA 112, 3164–3169. doi:10.1073/pnas.1422373112
Lan, B., Ge, J. W., Cheng, S. W., Zheng, X. L., Liao, J., He, C., et al. (2020). Extract of Naotaifang, a Compound Chinese Herbal Medicine, Protects Neuron Ferroptosis Induced by Acute Cerebral Ischemia in Rats. J. Integr. Med. 18, 344–350. doi:10.1016/j.joim.2020.01.008
Lang, X., Green, M. D., Wang, W., Yu, J., Choi, J. E., Jiang, L., et al. (2019). Radiotherapy and Immunotherapy Promote Tumoral Lipid Oxidation and Ferroptosis via Synergistic Repression of SLC7A11. Cancer Discov. 9, 1673–1685. doi:10.1158/2159-8290.cd-19-0338
Lanzardo, S., Conti, L., Rooke, R., Ruiu, R., Accart, N., Bolli, E., et al. (2016). Immunotargeting of Antigen xCT Attenuates Stem-like Cell Behavior and Metastatic Progression in Breast Cancer. Cancer Res. 76, 62–72. doi:10.1158/0008-5472.can-15-1208
Lee, J., Kosaras, B., Del Signore, S. J., Cormier, K., McKee, A., Ratan, R. R., et al. (2011). Modulation of Lipid Peroxidation and Mitochondrial Function Improves Neuropathology in Huntington's Disease Mice. Acta Neuropathol. 121, 487–498. doi:10.1007/s00401-010-0788-5
Lei, G., Zhang, Y., Koppula, P., Liu, X., Zhang, J., Lin, S. H., et al. (2020). The Role of Ferroptosis in Ionizing Radiation-Induced Cell Death and Tumor Suppression. Cel Res 30, 146–162. doi:10.1038/s41422-019-0263-3
Li, Q., Han, X., Lan, X., Gao, Y., Wan, J., Durham, F., et al. (2017). Inhibition of Neuronal Ferroptosis Protects Hemorrhagic Brain. JCI insight 2, e90777. doi:10.1172/jci.insight.90777
Li, Y., Feng, D., Wang, Z., Zhao, Y., Sun, R., Tian, D., et al. (2019). Ischemia-induced ACSL4 Activation Contributes to Ferroptosis-Mediated Tissue Injury in Intestinal Ischemia/reperfusion. Cel Death Differ 26, 2284–2299. doi:10.1038/s41418-019-0299-4
Li, W., Feng, G., Gauthier, J. M., Lokshina, I., Higashikubo, R., Evans, S., et al. (2019). Ferroptotic Cell Death and TLR4/Trif Signaling Initiate Neutrophil Recruitment after Heart Transplantation. J. Clin. Invest. 129, 2293–2304. doi:10.1172/jci126428
Li, Q., Li, Q.-Q., Jia, J.-N., Sun, Q.-Y., Zhou, H.-H., Jin, W.-L., et al. (2019). Baicalein Exerts Neuroprotective Effects in FeCl3-Induced Posttraumatic Epileptic Seizures via Suppressing Ferroptosis. Front. Pharmacol. 10, 638. doi:10.3389/fphar.2019.00638
Li, X., Wang, T. X., Huang, X., Li, Y., Sun, T., Zang, S., et al. (2020). Targeting Ferroptosis Alleviates Methionine‐choline Deficient (MCD)‐diet Induced NASH by Suppressing Liver Lipotoxicity. Liver Int. 40, 1378–1394. doi:10.1111/liv.14428
Li, D., Jiang, C., Mei, G., Zhao, Y., Chen, L., Liu, J., et al. (2020). Quercetin Alleviates Ferroptosis of Pancreatic β Cells in Type 2 Diabetes. Nutrients 12. doi:10.3390/nu12102954
Li, X., Zeng, J., Liu, Y., Liang, M., Liu, Q., Li, Z., et al. (2020). Inhibitory Effect and Mechanism of Action of Quercetin and Quercetin Diels-Alder Anti-dimer on Erastin-Induced Ferroptosis in Bone Marrow-Derived Mesenchymal Stem Cells. Antioxidants (Basel) 9, 205. doi:10.3390/antiox9030205
Liang, C., Zhang, X., Yang, M., and Dong, X. (2019). Recent Progress in Ferroptosis Inducers for Cancer Therapy. Adv. Mater. 31, e1904197. doi:10.1002/adma.201904197
Lim, J. K. M., Delaidelli, A., Minaker, S. W., Zhang, H.-F., Colovic, M., Yang, H., et al. (2019). Cystine/glutamate Antiporter xCT (SLC7A11) Facilitates Oncogenic RAS Transformation by Preserving Intracellular Redox Balance. Proc. Natl. Acad. Sci. USA 116, 9433–9442. doi:10.1073/pnas.1821323116
Lin, R., Zhang, Z., Chen, L., Zhou, Y., Zou, P., Feng, C., et al. (2016). Dihydroartemisinin (DHA) Induces Ferroptosis and Causes Cell Cycle Arrest in Head and Neck Carcinoma Cells. Cancer Lett. 381, 165–175. doi:10.1016/j.canlet.2016.07.033
Lin, Y.-S., Shen, Y.-C., Wu, C.-Y., Tsai, Y.-Y., Yang, Y.-H., Lin, Y.-Y., et al. (2019). Danshen Improves Survival of Patients with Breast Cancer and Dihydroisotanshinone I Induces Ferroptosis and Apoptosis of Breast Cancer Cells. Front. Pharmacol. 10, 1226. doi:10.3389/fphar.2019.01226
Liu, B., Zhao, C., Li, H., Chen, X., Ding, Y., and Xu, S. (2018). Puerarin Protects against Heart Failure Induced by Pressure Overload through Mitigation of Ferroptosis. Biochem. Biophysical Res. Commun. 497, 233–240. doi:10.1016/j.bbrc.2018.02.061
Liu, P., Wu, D., Duan, J., Xiao, H., Zhou, Y., Zhao, L., et al. (2020). NRF2 Regulates the Sensitivity of Human NSCLC Cells to Cystine Deprivation-Induced Ferroptosis via FOCAD-FAK Signaling Pathway. Redox Biol. 37, 101702. doi:10.1016/j.redox.2020.101702
Liu, J., Li, X., Cai, R., Ren, Z., Zhang, A., Deng, F., et al. (2020). Simultaneous Study of Anti-ferroptosis and Antioxidant Mechanisms of Butein and (S)-Butin. Molecules 25, 674. doi:10.3390/molecules25030674
Liu, Y., Wang, W., Li, Y., Xiao, Y., Cheng, J., and Jia, J. (2015). The 5-Lipoxygenase Inhibitor Zileuton Confers Neuroprotection against Glutamate Oxidative Damage by Inhibiting Ferroptosis. Biol. Pharm. Bull. 38, 1234–1239. doi:10.1248/bpb.b15-00048
Llabani, E., Hicklin, R. W., Lee, H. Y., Motika, S. E., Crawford, L. A., Weerapana, E., et al. (2019). Diverse Compounds from Pleuromutilin lead to a Thioredoxin Inhibitor and Inducer of Ferroptosis. Nat. Chem. 11, 521–532. doi:10.1038/s41557-019-0261-6
Lu, J., Liu, X., Tian, Y., Li, H., Ren, Z., Liang, S., et al. (2019). Moxibustion Exerts a Neuroprotective Effect through Antiferroptosis in Parkinson's Disease. Evid. Based Complement. Alternat Med. 2019, 2735492. doi:10.1155/2019/2735492
Lu, Q., Harris, V. A., Rafikov, R., Sun, X., Kumar, S., and Black, S. M. (2015). Nitric Oxide Induces Hypoxia Ischemic Injury in the Neonatal Brain via the Disruption of Neuronal Iron Metabolism. Redox Biol. 6, 112–121. doi:10.1016/j.redox.2015.06.007
Luo, H., Vong, C. T., Chen, H., Gao, Y., Lyu, P., Qiu, L., et al. (2019). Naturally Occurring Anti-cancer Compounds: Shining from Chinese Herbal Medicine. Chin. Med. 14, 48. doi:10.1186/s13020-019-0270-9
Luo, M., Wu, L., Zhang, K., Wang, H., Zhang, T., Gutierrez, L., et al. (2018). miR-137 Regulates Ferroptosis by Targeting Glutamine Transporter SLC1A5 in Melanoma. Cel Death Differ 25, 1457–1472. doi:10.1038/s41418-017-0053-8
Lv, H.-H., Zhen, C.-x., Liu, J.-y., and Shang, P. (2020). PEITC Triggers Multiple Forms of Cell Death by GSH-Iron-ROS Regulation in K7M2 Murine Osteosarcoma Cells. Acta Pharmacol. Sin 41, 1119–1132. doi:10.1038/s41401-020-0376-8
Lv, H., Zhen, C., Liu, J., and Shang, P. (2020). β-Phenethyl Isothiocyanate Induces Cell Death in Human Osteosarcoma through Altering Iron Metabolism, Disturbing the Redox Balance, and Activating the MAPK Signaling Pathway. Oxid Med. Cel Longev 2020, 5021983. doi:10.1155/2020/5021983
Ma, S., Dielschneider, R. F., Henson, E. S., Xiao, W., Choquette, T. R., Blankstein, A. R., et al. (2017). Ferroptosis and Autophagy Induced Cell Death Occur Independently after Siramesine and Lapatinib Treatment in Breast Cancer Cells. PLoS One 12, e0182921. doi:10.1371/journal.pone.0182921
Maggio, A., Kattamis, A., Felisi, M., Reggiardo, G., El-Beshlawy, A., Bejaoui, M., et al. (2020). Evaluation of the Efficacy and Safety of Deferiprone Compared with Deferasirox in Paediatric Patients with Transfusion-dependent Haemoglobinopathies (DEEP-2): a Multicentre, Randomised, Open-Label, Non-inferiority, Phase 3 Trial. Lancet Haematol. 7, e469–e478. doi:10.1016/s2352-3026(20)30100-9
Maher, P., Fischer, W., Liang, Z., Soriano-Castell, D., Pinto, A. F. M., Rebman, J., et al. (2020). The Value of Herbarium Collections to the Discovery of Novel Treatments for Alzheimer's Disease, a Case Made with the Genus Eriodictyon. Front. Pharmacol. 11, 208. doi:10.3389/fphar.2020.00208
Mai, T. T., Hamaï, A., Hienzsch, A., Cañeque, T., Müller, S., Wicinski, J., et al. (2017). Salinomycin Kills Cancer Stem Cells by Sequestering Iron in Lysosomes. Nat. Chem 9, 1025–1033. doi:10.1038/nchem.2778
Malfa, G. A., Tomasello, B., Acquaviva, R., Genovese, C., La Mantia, A., Cammarata, F. P., et al. (2019). Betula Etnensis Raf. (Betulaceae) Extract Induced HO-1 Expression and Ferroptosis Cell Death in Human Colon Cancer Cells. Int. J. Mol. Sci. 20, 2723. doi:10.3390/ijms20112723
Mancias, J. D., Wang, X., Gygi, S. P., Harper, J. W., and Kimmelman, A. C. (2014). Quantitative Proteomics Identifies NCOA4 as the Cargo Receptor Mediating Ferritinophagy. Nature 509, 105–109. doi:10.1038/nature13148
Martin-Bastida, A., Ward, R. J., Newbould, R., Piccini, P., Sharp, D., Kabba, C., et al. (2017). Brain Iron Chelation by Deferiprone in a Phase 2 Randomised Double-Blinded Placebo Controlled Clinical Trial in Parkinson's Disease. Sci. Rep. 7, 1398. doi:10.1038/s41598-017-01402-2
Matsushita, M., Freigang, S., Schneider, C., Conrad, M., Bornkamm, G. W., and Kopf, M. (2015). T Cell Lipid Peroxidation Induces Ferroptosis and Prevents Immunity to Infection. J. Exp. Med. 212, 555–568. doi:10.1084/jem.20140857
Mayr, L., Grabherr, F., Schwärzler, J., Reitmeier, I., Sommer, F., Gehmacher, T., et al. (2020). Dietary Lipids Fuel GPX4-Restricted Enteritis Resembling Crohn's Disease. Nat. Commun. 11, 1775. doi:10.1038/s41467-020-15646-6
Mbaveng, A. T., Bitchagno, G. T. M., Kuete, V., Tane, P., and Efferth, T. (2019). Cytotoxicity of Ungeremine towards Multi-Factorial Drug Resistant Cancer Cells and Induction of Apoptosis, Ferroptosis, Necroptosis and Autophagy. Phytomedicine 60, 152832. doi:10.1016/j.phymed.2019.152832
Mbaveng, A. T., Chi, G. F., Bonsou, I. N., Abdelfatah, S., Tamfu, A. N., Yeboah, E. M. O., et al. (2020). N-acetylglycoside of Oleanolic Acid (Aridanin) Displays Promising Cytotoxicity towards Human and Animal Cancer Cells, Inducing Apoptotic, Ferroptotic and Necroptotic Cell Death. Phytomedicine 76, 153261. doi:10.1016/j.phymed.2020.153261
Mbaveng, A. T., Ndontsa, B. L., Kuete, V., Nguekeu, Y. M. M., Çelik, İ., Mbouangouere, R., et al. (2018a). A Naturally Occuring Triterpene Saponin Ardisiacrispin B Displayed Cytotoxic Effects in Multi-Factorial Drug Resistant Cancer Cells via Ferroptotic and Apoptotic Cell Death. Phytomedicine 43, 78–85. doi:10.1016/j.phymed.2018.03.035
Mbaveng, A. T., Fotso, G. W., Ngnintedo, D., Kuete, V., Ngadjui, B. T., Keumedjio, F., et al. (2018b). Cytotoxicity of Epunctanone and Four Other Phytochemicals Isolated from the Medicinal Plants Garcinia Epunctata and Ptycholobium Contortum towards Multi-Factorial Drug Resistant Cancer Cells. Phytomedicine 48, 112–119. doi:10.1016/j.phymed.2017.12.016
McBean, G. J. (2012). The Transsulfuration Pathway: a Source of Cysteine for Glutathione in Astrocytes. Amino Acids 42, 199–205. doi:10.1007/s00726-011-0864-8
Md Nesran, Z. N., Shafie, N. H., Md Tohid, S. F., Norhaizan, M. E., and Ismail, A. (2020). Iron Chelation Properties of Green Tea Epigallocatechin-3-Gallate (EGCG) in Colorectal Cancer Cells: Analysis on Tfr/Fth Regulations and Molecular Docking. Evid. Based Complement. Alternat Med. 2020, 7958041. doi:10.1155/2020/7958041
Müller, S., Sindikubwabo, F., Cañeque, T., Lafon, A., Versini, A., Lombard, B., et al. (2020). CD44 Regulates Epigenetic Plasticity by Mediating Iron Endocytosis. Nat. Chem. 12, 929–938. doi:10.1038/s41557-020-0513-5
Najafov, A., Chen, H., and Yuan, J. (2017). Necroptosis and Cancer. Trends Cancer 3, 294–301. doi:10.1016/j.trecan.2017.03.002
Niu, Y., Zhang, J., Tong, Y., Li, J., and Liu, B. (2019). Physcion 8-O-β-Glucopyranoside Induced Ferroptosis via Regulating miR-103a-3p/GLS2 axis in Gastric Cancer. Life Sci. 237, 116893. doi:10.1016/j.lfs.2019.116893
Ou, Y., Wang, S.-J., Li, D., Chu, B., and Gu, W. (2016). Activation of SAT1 Engages Polyamine Metabolism with P53-Mediated Ferroptotic Responses. Proc. Natl. Acad. Sci. USA 113, E6806–E6812. doi:10.1073/pnas.1607152113
Palliyaguru, D. L., Yuan, J. M., Kensler, T. W., and Fahey, J. W. (2018). Isothiocyanates: Translating the Power of Plants to People. Mol. Nutr. Food Res. 62, e1700965. doi:10.1002/mnfr.201700965
Park, S., Oh, J., Kim, M., and Jin, E.-J. (2018). Bromelain Effectively Suppresses Kras-Mutant Colorectal Cancer by Stimulating Ferroptosis. Anim. Cell Syst. 22, 334–340. doi:10.1080/19768354.2018.1512521
Park, U. J., Lee, Y. A., Won, S. M., Lee, J. H., Kang, S.-H., Springer, J. E., et al. (2011). Blood-derived Iron Mediates Free Radical Production and Neuronal Death in the Hippocampal CA1 Area Following Transient Forebrain Ischemia in Rat. Acta Neuropathol. 121, 459–473. doi:10.1007/s00401-010-0785-8
Parvez, M. K., and Rishi, V. (2019). Herb-Drug Interactions and Hepatotoxicity. Cdm 20, 275–282. doi:10.2174/1389200220666190325141422
Perez, C. A., Wei, Y., and Guo, M. (2009). Iron-binding and Anti-Fenton Properties of Baicalein and Baicalin. J. Inorg. Biochem. 103, 326–332. doi:10.1016/j.jinorgbio.2008.11.003
Praticò, D., and Sung, S. (2004). Lipid Peroxidation and Oxidative Imbalance: Early Functional Events in Alzheimer's Disease. Jad 6, 171–175. doi:10.3233/jad-2004-6209
Probst, L., Dächert, J., Schenk, B., and Fulda, S. (2017). Lipoxygenase Inhibitors Protect Acute Lymphoblastic Leukemia Cells from Ferroptotic Cell Death. Biochem. Pharmacol. 140, 41–52. doi:10.1016/j.bcp.2017.06.112
Pyatigorskaya, N., Sharman, M., Corvol, J.-C., Valabregue, R., Yahia-Cherif, L., Poupon, F., et al. (2015). High Nigral Iron Deposition in LRRK2 and Parkin Mutation Carriers Using R2* Relaxometry. Mov Disord. 30, 1077–1084. doi:10.1002/mds.26218
Ran, Q., Liang, H., Ikeno, Y., Qi, W., Prolla, T. A., Roberts, L. J., et al. (2007). Reduction in Glutathione Peroxidase 4 Increases Life Span through Increased Sensitivity to Apoptosis. Journals Gerontol. Ser. A: Biol. Sci. Med. Sci. 62, 932–942. doi:10.1093/gerona/62.9.932
Rao, S. S., Lago, L., Volitakis, I., Shukla, J. J., McColl, G., Finkelstein, D. I., et al. (2021). Deferiprone Treatment in Aged Transgenic Tau Mice Improves Y-Maze Performance and Alters Tau Pathology. Neurotherapeutics 18, 1081–1094. doi:10.1007/s13311-020-00972-w
Reed, J. C., and Pellecchia, M. (2012). Ironing Out Cell Death Mechanisms. Cell 149, 963–965. doi:10.1016/j.cell.2012.05.009
Roh, J.-L., Kim, E. H., Jang, H. J., Park, J. Y., and Shin, D. (2016). Induction of Ferroptotic Cell Death for Overcoming Cisplatin Resistance of Head and Neck Cancer. Cancer Lett. 381, 96–103. doi:10.1016/j.canlet.2016.07.035
Roh, J.-L., Kim, E. H., Jang, H., and Shin, D. (2017). Nrf2 Inhibition Reverses the Resistance of Cisplatin-Resistant Head and Neck Cancer Cells to Artesunate-Induced Ferroptosis. Redox Biol. 11, 254–262. doi:10.1016/j.redox.2016.12.010
Rojo de la Vega, M., Chapman, E., and Zhang, D. D. (2018). NRF2 and the Hallmarks of Cancer. Cancer cell 34, 21–43. doi:10.1016/j.ccell.2018.03.022
Sakai, O., Yasuzawa, T., Sumikawa, Y., Ueta, T., Imai, H., Sawabe, A., et al. (2017). Role of GPx4 in Human Vascular Endothelial Cells, and the Compensatory Activity of Brown rice on GPx4 Ablation Condition. Pathophysiology 24, 9–15. doi:10.1016/j.pathophys.2016.11.002
Sato, H., Shiiya, A., Kimata, M., Maebara, K., Tamba, M., Sakakura, Y., et al. (2005). Redox Imbalance in Cystine/glutamate Transporter-Deficient Mice. J. Biol. Chem. 280, 37423–37429. doi:10.1074/jbc.m506439200
Seco-Cervera, M., Gonzalez-Cabo, P., Pallardo, F. V., Roma-Mateo, C., and Garcia-Gimenez, J. L. (2020). Thioredoxin and Glutaredoxin Systems as Potential Targets for the Development of New Treatments in Friedreich's Ataxia. Antioxidants (Basel) 9, 1257. doi:10.3390/antiox9121257
Shaw, A. T., Winslow, M. M., Magendantz, M., Ouyang, C., Dowdle, J., Subramanian, A., et al. (2011). Selective Killing of K-Ras Mutant Cancer Cells by Small Molecule Inducers of Oxidative Stress. Proc. Natl. Acad. Sci. 108, 8773–8778. doi:10.1073/pnas.1105941108
Shen, Z., Liu, T., Li, Y., Lau, J., Yang, Z., Fan, W., et al. (2018). Fenton-Reaction-Acceleratable Magnetic Nanoparticles for Ferroptosis Therapy of Orthotopic Brain Tumors. ACS nano 12, 11355–11365. doi:10.1021/acsnano.8b06201
Sherr, C. J. (2006). Divorcing ARF and P53: an Unsettled Case. Nat. Rev. Cancer 6, 663–673. doi:10.1038/nrc1954
Shimada, K., Skouta, R., Kaplan, A., Yang, W. S., Hayano, M., Dixon, S. J., et al. (2016a). Global Survey of Cell Death Mechanisms Reveals Metabolic Regulation of Ferroptosis. Nat. Chem. Biol. 12, 497–503. doi:10.1038/nchembio.2079
Shimada, K., Hayano, M., Pagano, N. C., and Stockwell, B. R. (2016b). Cell-Line Selectivity Improves the Predictive Power of Pharmacogenomic Analyses and Helps Identify NADPH as Biomarker for Ferroptosis Sensitivity. Cel Chem. Biol. 23, 225–235. doi:10.1016/j.chembiol.2015.11.016
Shin, D., Kim, E. H., Lee, J., and Roh, J.-L. (2018). Nrf2 Inhibition Reverses Resistance to GPX4 Inhibitor-Induced Ferroptosis in Head and Neck Cancer. Free Radic. Biol. Med. 129, 454–462. doi:10.1016/j.freeradbiomed.2018.10.426
Shintoku, R., Takigawa, Y., Yamada, K., Kubota, C., Yoshimoto, Y., Takeuchi, T., et al. (2017). Lipoxygenase‐mediated Generation of Lipid Peroxides Enhances Ferroptosis Induced by Erastin and RSL3. Cancer Sci. 108, 2187–2194. doi:10.1111/cas.13380
Shu-Feng Zhou, S. F., Charlie Changli Xue, C. C., Xue-Qing Yu, X. Q., and Guangji Wang, G. (2007). Metabolic Activation of Herbal and Dietary Constituents and its Clinical and Toxicological Implications: an Update. Cdm 8, 526–553. doi:10.2174/138920007781368863
Skouta, R., Dixon, S. J., Wang, J., Dunn, D. E., Orman, M., Shimada, K., et al. (2014). Ferrostatins Inhibit Oxidative Lipid Damage and Cell Death in Diverse Disease Models. J. Am. Chem. Soc. 136, 4551–4556. doi:10.1021/ja411006a
Song, Z., Xiang, X., Li, J., Deng, J., Fang, Z., Zhang, L., et al. (2020). Ruscogenin Induces Ferroptosis in Pancreatic Cancer Cells. Oncol. Rep. 43, 516–524. doi:10.3892/or.2019.7425
Southon, A., Szostak, K., Acevedo, K. M., Dent, K. A., Volitakis, I., Belaidi, A. A., et al. (2020). Cu II (Atsm) Inhibits Ferroptosis: Implications for Treatment of Neurodegenerative Disease. Br. J. Pharmacol. 177, 656–667. doi:10.1111/bph.14881
Sripetchwandee, J., Wongjaikam, S., Krintratun, W., Chattipakorn, N., and Chattipakorn, S. C. (2016). A Combination of an Iron Chelator with an Antioxidant Effectively Diminishes the Dendritic Loss, Tau-Hyperphosphorylation, Amyloids-β Accumulation and Brain Mitochondrial Dynamic Disruption in Rats with Chronic Iron-Overload. Neuroscience 332, 191–202. doi:10.1016/j.neuroscience.2016.07.003
Stockwell, B. R., Friedmann Angeli, J. P., Bayir, H., Bush, A. I., Conrad, M., Dixon, S. J., et al. (2017). Ferroptosis: A Regulated Cell Death Nexus Linking Metabolism, Redox Biology, and Disease. Cell 171, 273–285. doi:10.1016/j.cell.2017.09.021
Sui, M., Jiang, X., Chen, J., Yang, H., and Zhu, Y. (2018). Magnesium Isoglycyrrhizinate Ameliorates Liver Fibrosis and Hepatic Stellate Cell Activation by Regulating Ferroptosis Signaling Pathway. Biomed. Pharmacother. 106, 125–133. doi:10.1016/j.biopha.2018.06.060
Sullivan, J. (1981). Iron and the Sex Difference in Heart Disease Risk. The Lancet 317, 1293–1294. doi:10.1016/s0140-6736(81)92463-6
Sun, X., Ou, Z., Chen, R., Niu, X., Chen, D., Kang, R., et al. (2016a). Activation of the P62-Keap1-NRF2 Pathway Protects against Ferroptosis in Hepatocellular Carcinoma Cells. Hepatology 63, 173–184. doi:10.1002/hep.28251
Sun, X., Niu, X., Chen, R., He, W., Chen, D., Kang, R., et al. (2016b). Metallothionein-1G Facilitates Sorafenib Resistance through Inhibition of Ferroptosis. Hepatology 64, 488–500. doi:10.1002/hep.28574
Sun, Y., Zheng, Y., Wang, C., and Liu, Y. (2018). Glutathione Depletion Induces Ferroptosis, Autophagy, and Premature Cell Senescence in Retinal Pigment Epithelial Cells. Cel Death Dis 9, 753. doi:10.1038/s41419-018-0794-4
Takashima, M., Ichihara, K., and Hirata, Y. (2019). Neuroprotective Effects of Brazilian green Propolis on Oxytosis/ferroptosis in Mouse Hippocampal HT22 Cells. Food Chem. Toxicol. 132, 110669. doi:10.1016/j.fct.2019.110669
Tang, H. M., and Cheung, P. C. K. (2019). Gallic Acid Triggers Iron-dependent Cell Death with Apoptotic, Ferroptotic, and Necroptotic Features. Toxins (Basel) 11, 492. doi:10.3390/toxins11090492
Tang, H. M., and Tang, H. L. (2019). Cell Recovery by Reversal of Ferroptosis. Biol. Open 8, bio043182. doi:10.1242/bio.043182
Thompson, C. B. (1995). Apoptosis in the Pathogenesis and Treatment of Disease. Science 267, 1456–1462. doi:10.1126/science.7878464
Tonik, S. E., Shindelman, J. E., and Sussman, H. H. (1986). Transferrin Receptor Is Inversely Correlated with Estrogen Receptor in Breast Cancer. Breast Cancer Res. Tr 7, 71–76. doi:10.1007/bf01806791
Torti, S. V., and Torti, F. M. (2013). Iron and Cancer: More Ore to Be Mined. Nat. Rev. Cancer 13, 342–355. doi:10.1038/nrc3495
Trachootham, D., Alexandre, J., and Huang, P. (2009). Targeting Cancer Cells by ROS-Mediated Mechanisms: a Radical Therapeutic Approach. Nat. Rev. Drug Discov. 8, 579–591. doi:10.1038/nrd2803
Trujillo-Alonso, V., Pratt, E. C., Zong, H., Lara-Martinez, A., Kaittanis, C., Rabie, M. O., et al. (2019). FDA-approved Ferumoxytol Displays Anti-leukaemia Efficacy against Cells with Low Ferroportin Levels. Nat. Nanotechnol. 14, 616–622. doi:10.1038/s41565-019-0406-1
Tsoi, J., Robert, L., Paraiso, K., Galvan, C., Sheu, K. M., Lay, J., et al. (2018). Multi-stage Differentiation Defines Melanoma Subtypes with Differential Vulnerability to Drug-Induced Iron-dependent Oxidative Stress. Cancer cell 33, 890–904. doi:10.1016/j.ccell.2018.03.017
Tsurusaki, S., Tsuchiya, Y., Koumura, T., Nakasone, M., Sakamoto, T., Matsuoka, M., et al. (2019). Hepatic Ferroptosis Plays an Important Role as the Trigger for Initiating Inflammation in Nonalcoholic Steatohepatitis. Cel Death Dis 10, 449. doi:10.1038/s41419-019-1678-y
Tuo, Q.-z., Lei, P., Jackman, K. A., Li, X.-l., Xiong, H., Li, X.-l., et al. (2017). Tau-mediated Iron export Prevents Ferroptotic Damage after Ischemic Stroke. Mol. Psychiatry 22, 1520–1530. doi:10.1038/mp.2017.171
van Staalduinen, J., Baker, D., Ten Dijke, P., and van Dam, H. (2018). Epithelial-mesenchymal-transition-inducing Transcription Factors: New Targets for Tackling Chemoresistance in Cancer. Oncogene 37, 6195–6211. doi:10.1038/s41388-018-0378-x
Vichinsky, E., Bernaudin, F., Forni, G. L., Gardner, R., Hassell, K., Heeney, M. M., et al. (2011). Long-term Safety and Efficacy of Deferasirox (Exjade) for up to 5 Years in Transfusional Iron-Overloaded Patients with Sickle Cell Disease. Br. J. Haematol. 154, 387–397. doi:10.1111/j.1365-2141.2011.08720.x
Viswanathan, V. S., Ryan, M. J., Dhruv, H. D., Gill, S., Eichhoff, O. M., Seashore-Ludlow, B., et al. (2017). Dependency of a Therapy-Resistant State of Cancer Cells on a Lipid Peroxidase Pathway. Nature 547, 453–457. doi:10.1038/nature23007
von Hagens, C., Walter-Sack, I., Goeckenjan, M., Osburg, J., Storch-Hagenlocher, B., Sertel, S., et al. (2017). Prospective Open Uncontrolled Phase I Study to Define a Well-Tolerated Dose of Oral Artesunate as Add-On Therapy in Patients with Metastatic Breast Cancer (ARTIC M33/2). Breast Cancer Res. Treat. 164, 359–369. doi:10.1007/s10549-017-4261-1
Wang, B., Peng, X.-x., Sun, R., Li, J., Zhan, X.-r., Wu, L.-j., et al. (2012). Systematic Review of β-Elemene Injection as Adjunctive Treatment for Lung Cancer. Chin. J. Integr. Med. 18, 813–823. doi:10.1007/s11655-012-1271-9
Wang, S.-J., Li, D., Ou, Y., Jiang, L., Chen, Y., Zhao, Y., et al. (2016). Acetylation Is Crucial for P53-Mediated Ferroptosis and Tumor Suppression. Cel Rep. 17, 366–373. doi:10.1016/j.celrep.2016.09.022
Wang, D., Peng, Y., Xie, Y., Zhou, B., Sun, X., Kang, R., et al. (2016). Antiferroptotic Activity of Non-oxidative Dopamine. Biochem. biophysical Res. Commun. 480, 602–607. doi:10.1016/j.bbrc.2016.10.099
Wang, H., An, P., Xie, E., Wu, Q., Fang, X., Gao, H., et al. (2017). Characterization of Ferroptosis in Murine Models of Hemochromatosis. Hepatology 66, 449–465. doi:10.1002/hep.29117
Wang, Y., Wang, Q., Li, C., Lu, L., Zhang, Q., Zhu, R., et al. (2017). A Review of Chinese Herbal Medicine for the Treatment of Chronic Heart Failure. Curr. Pharm. Des. 23, 5115–5124. doi:10.2174/1381612823666170925163427
Wang, D., Xie, N., Gao, W., Kang, R., and Tang, D. (2018). The Ferroptosis Inducer Erastin Promotes Proliferation and Differentiation in Human Peripheral Blood Mononuclear Cells. Biochem. biophysical Res. Commun. 503, 1689–1695. doi:10.1016/j.bbrc.2018.07.100
Wang, Z., Ding, Y., Wang, X., Lu, S., Wang, C., He, C., et al. (2018). Pseudolaric Acid B Triggers Ferroptosis in Glioma Cells via Activation of Nox4 and Inhibition of xCT. Cancer Lett. 428, 21–33. doi:10.1016/j.canlet.2018.04.021
Wang, W., Green, M., Choi, J. E., Gijón, M., Kennedy, P. D., Johnson, J. K., et al. (2019). CD8+ T Cells Regulate Tumour Ferroptosis during Cancer Immunotherapy. Nature 569, 270–274. doi:10.1038/s41586-019-1170-y
Wang, N., Zeng, G.-Z., Yin, J.-L., and Bian, Z.-X. (2019). Artesunate Activates the ATF4-CHOP-CHAC1 Pathway and Affects Ferroptosis in Burkitt's Lymphoma. Biochem. biophysical Res. Commun. 519, 533–539. doi:10.1016/j.bbrc.2019.09.023
Wang, L., Zhang, Z., Li, M., Wang, F., Jia, Y., Zhang, F., et al. (2019). P53-dependent Induction of Ferroptosis Is Required for Artemether to Alleviate Carbon Tetrachloride-Induced Liver Fibrosis and Hepatic Stellate Cell Activation. IUBMB Life 71, 45–56. doi:10.1002/iub.1895
Wang, Y., Chen, Q., Shi, C., Jiao, F., and Gong, Z. (2019). Mechanism of Glycyrrhizin on Ferroptosis during Acute Liver Failure by Inhibiting Oxidative Stress. Mol. Med. Rep. 20, 4081–4090. doi:10.3892/mmr.2019.10660
Wang, K., Zhang, Z., Wang, M., Cao, X., Qi, J., Wang, D., et al. (2019). Role of GRP78 Inhibiting Artesunate-Induced Ferroptosis in KRAS Mutant Pancreatic Cancer Cells. Dddt 13, 2135–2144. doi:10.2147/dddt.s199459
Wang, Y., Bi, R., Quan, F., Cao, Q., Lin, Y., Yue, C., et al. (2020). Ferroptosis Involves in Renal Tubular Cell Death in Diabetic Nephropathy. Eur. J. Pharmacol. 888, 173574. doi:10.1016/j.ejphar.2020.173574
Wei, G., Sun, J., Hou, Z., Luan, W., Wang, S., Cui, S., et al. (2018). Novel Antitumor Compound Optimized from Natural Saponin Albiziabioside A Induced Caspase-dependent Apoptosis and Ferroptosis as a P53 Activator through the Mitochondrial Pathway. Eur. J. Med. Chem. 157, 759–772. doi:10.1016/j.ejmech.2018.08.036
Wen, L., Shi, D., Zhou, T., Tu, J., He, M., Jiang, Y., et al. (2020). Identification of Two Novel Prenylated Flavonoids in mulberry Leaf and Their Bioactivities. Food Chem. 315, 126236. doi:10.1016/j.foodchem.2020.126236
Woo, J. H., Shimoni, Y., Yang, W. S., Subramaniam, P., Iyer, A., Nicoletti, P., et al. (2015). Elucidating Compound Mechanism of Action by Network Perturbation Analysis. Cell 162, 441–451. doi:10.1016/j.cell.2015.05.056
Wu, J., Minikes, A. M., Gao, M., Bian, H., Li, Y., Stockwell, B. R., et al. (2019). Intercellular Interaction Dictates Cancer Cell Ferroptosis via NF2-YAP Signalling. Nature 572, 402–406. doi:10.1038/s41586-019-1426-6
Wu, J., Yang, J.-J., Cao, Y., Li, H., Zhao, H., Yang, S., et al. (2020). Iron Overload Contributes to General Anaesthesia-Induced Neurotoxicity and Cognitive Deficits. J. Neuroinflammation 17, 110. doi:10.1186/s12974-020-01777-6
Xie, Y., Hou, W., Song, X., Yu, Y., Huang, J., Sun, X., et al. (2016a). Ferroptosis: Process and Function. Cel Death Differ 23, 369–379. doi:10.1038/cdd.2015.158
Xie, Y., Song, X., Sun, X., Huang, J., Zhong, M., Lotze, M. T., et al. (2016b). Identification of Baicalein as a Ferroptosis Inhibitor by Natural Product Library Screening. Biochem. biophysical Res. Commun. 473, 775–780. doi:10.1016/j.bbrc.2016.03.052
Xie, Y., Zhu, S., Song, X., Sun, X., Fan, Y., Liu, J., et al. (2017). The Tumor Suppressor P53 Limits Ferroptosis by Blocking DPP4 Activity. Cel Rep. 20, 1692–1704. doi:10.1016/j.celrep.2017.07.055
Xu, Y., Li, X., Cheng, Y., Yang, M., and Wang, R. (2020). Inhibition of ACSL4 Attenuates Ferroptotic Damage after Pulmonary Ischemia‐reperfusion. FASEB j. 34, 16262–16275. doi:10.1096/fj.202001758r
Yagoda, N., von Rechenberg, M., Zaganjor, E., Bauer, A. J., Yang, W. S., Fridman, D. J., et al. (2007). RAS-RAF-MEK-dependent Oxidative Cell Death Involving Voltage-dependent Anion Channels. Nature 447, 864–868. doi:10.1038/nature05859
Yamada, N., Karasawa, T., Wakiya, T., Sadatomo, A., Ito, H., Kamata, R., et al. (2020a). Iron Overload as a Risk Factor for Hepatic Ischemia‐reperfusion Injury in Liver Transplantation: Potential Role of Ferroptosis. Am. J. Transpl. 20, 1606–1618. doi:10.1111/ajt.15773
Yamada, N., Komada, T., Ohno, N., and Takahashi, M. (2020b). Acetaminophen-induced Hepatotoxicity: Different Mechanisms of Acetaminophen-Induced Ferroptosis and Mitochondrial Damage. Arch. Toxicol. 94, 2255–2257. doi:10.1007/s00204-020-02722-5
Yamada, N., Karasawa, T., Kimura, H., Watanabe, S., Komada, T., Kamata, R., et al. (2020c). Ferroptosis Driven by Radical Oxidation of N-6 Polyunsaturated Fatty Acids Mediates Acetaminophen-Induced Acute Liver Failure. Cel Death Dis 11, 144. doi:10.1038/s41419-020-2334-2
Yamaguchi, H., Hsu, J. L., Chen, C.-T., Wang, Y.-N., Hsu, M.-C., Chang, S.-S., et al. (2013). Caspase-independent Cell Death Is Involved in the Negative Effect of EGF Receptor Inhibitors on Cisplatin in Non-small Cell Lung Cancer Cells. Clin. Cancer Res. 19, 845–854. doi:10.1158/1078-0432.ccr-12-2621
Yamaguchi, Y., Kasukabe, T., and Kumakura, S. (2018). Piperlongumine Rapidly Induces the Death of Human Pancreatic Cancer Cells Mainly through the Induction of Ferroptosis. Int. J. Oncol. 52, 1011–1022. doi:10.3892/ijo.2018.4259
Yang, W.-H., Ding, C.-K. C., Sun, T., Rupprecht, G., Lin, C.-C., Hsu, D., et al. (2019). The Hippo Pathway Effector TAZ Regulates Ferroptosis in Renal Cell Carcinoma. Cel Rep. 28, 2501–2508. doi:10.1016/j.celrep.2019.07.107
Yang, J., Antin, P., Berx, G., Blanpain, C., Brabletz, T., Bronner, M., et al. (2020). Guidelines and Definitions for Research on Epithelial-Mesenchymal Transition. Nat. Rev. Mol. Cel Biol 21, 341–352. doi:10.1038/s41580-020-0237-9
Yang, W.-H., Huang, Z., Wu, J., Ding, C.-K. C., Murphy, S. K., and Chi, J.-T. (2020). A TAZ-ANGPTL4-NOX2 Axis Regulates Ferroptotic Cell Death and Chemoresistance in Epithelial Ovarian Cancer. Mol. Cancer Res. 18, 79–90. doi:10.1158/1541-7786.mcr-19-0691
Yang, W. S., Kim, K. J., Gaschler, M. M., Patel, M., Shchepinov, M. S., and Stockwell, B. R. (2016). Peroxidation of Polyunsaturated Fatty Acids by Lipoxygenases Drives Ferroptosis. Proc. Natl. Acad. Sci. USA 113, E4966–E4975. doi:10.1073/pnas.1603244113
Yang, W. S., SriRamaratnam, R., Welsch, M. E., Shimada, K., Skouta, R., Viswanathan, V. S., et al. (2014). Regulation of Ferroptotic Cancer Cell Death by GPX4. Cell 156, 317–331. doi:10.1016/j.cell.2013.12.010
Yang, W. S., and Stockwell, B. R. (2016). Ferroptosis: Death by Lipid Peroxidation. Trends Cell Biology 26, 165–176. doi:10.1016/j.tcb.2015.10.014
Yang, W. S., and Stockwell, B. R. (2008). Synthetic Lethal Screening Identifies Compounds Activating Iron-dependent, Nonapoptotic Cell Death in Oncogenic-RAS-Harboring Cancer Cells. Chem. Biol. 15, 234–245. doi:10.1016/j.chembiol.2008.02.010
Yaseen, A., Yang, F., Zhang, X., Li, F., Chen, B., Faraag, A. H. I., et al. (2021). Ferroptosis Inhibitory Constituents from the Fruits of Cullen Corylifolium. Nat. Prod. Res. 35, 5364–5368. doi:10.1080/14786419.2020.1762188
Yoshida, M., Minagawa, S., Araya, J., Sakamoto, T., Hara, H., Tsubouchi, K., et al. (2019). Involvement of Cigarette Smoke-Induced Epithelial Cell Ferroptosis in COPD Pathogenesis. Nat. Commun. 10, 3145. doi:10.1038/s41467-019-10991-7
Youssef, L. A., Rebbaa, A., Pampou, S., Weisberg, S. P., Stockwell, B. R., Hod, E. A., et al. (2018). Increased Erythrophagocytosis Induces Ferroptosis in Red Pulp Macrophages in a Mouse Model of Transfusion. Blood 131, 2581–2593. doi:10.1182/blood-2017-12-822619
Yu, H., Yang, C., Jian, L., Guo, S., Chen, R., Li, K., et al. (2019). Sulfasalazine-induced F-erroptosis in B-reast C-ancer C-ells I-s R-educed by the I-nhibitory E-ffect of E-strogen R-eceptor on the T-ransferrin R-eceptor. Oncol. Rep. 42, 826–838. doi:10.3892/or.2019.7189
Yuan, H., Li, X., Zhang, X., Kang, R., and Tang, D. (2016). Identification of ACSL4 as a Biomarker and Contributor of Ferroptosis. Biochem. biophysical Res. Commun. 478, 1338–1343. doi:10.1016/j.bbrc.2016.08.124
Zhang, Y., Shi, J., Liu, X., Feng, L., Gong, Z., Koppula, P., et al. (2018). BAP1 Links Metabolic Regulation of Ferroptosis to Tumour Suppression. Nat. Cel Biol 20, 1181–1192. doi:10.1038/s41556-018-0178-0
Zhang, Y.-H., Wang, D.-W., Xu, S.-F., Zhang, S., Fan, Y.-G., Yang, Y.-Y., et al. (2018). α-Lipoic Acid Improves Abnormal Behavior by Mitigation of Oxidative Stress, Inflammation, Ferroptosis, and Tauopathy in P301S Tau Transgenic Mice. Redox Biol. 14, 535–548. doi:10.1016/j.redox.2017.11.001
Zhang, Z., Wu, Y., Yuan, S., Zhang, P., Zhang, J., Li, H., et al. (2018). Glutathione Peroxidase 4 Participates in Secondary Brain Injury through Mediating Ferroptosis in a Rat Model of Intracerebral Hemorrhage. Brain Res. 1701, 112–125. doi:10.1016/j.brainres.2018.09.012
Zhang, X., Du, L., Qiao, Y., Zhang, X., Zheng, W., Wu, Q., et al. (2019). Ferroptosis Is Governed by Differential Regulation of Transcription in Liver Cancer. Redox Biol. 24, 101211. doi:10.1016/j.redox.2019.101211
Zhang, Y., Tan, H., Daniels, J. D., Zandkarimi, F., Liu, H., Brown, L. M., et al. (2019). Imidazole Ketone Erastin Induces Ferroptosis and Slows Tumor Growth in a Mouse Lymphoma Model. Cel Chem. Biol. 26, 623–633. e9. doi:10.1016/j.chembiol.2019.01.008
Zhao, X., Liu, Z., Gao, J., Li, H., Wang, X., Li, Y., et al. (2020). Inhibition of Ferroptosis Attenuates Busulfan-Induced Oligospermia in Mice. Toxicology 440, 152489. doi:10.1016/j.tox.2020.152489
Zheng, D.-W., Lei, Q., Zhu, J.-Y., Fan, J.-X., Li, C.-X., Li, C., et al. (2017). Switching Apoptosis to Ferroptosis: Metal-Organic Network for High-Efficiency Anticancer Therapy. Nano Lett. 17, 284–291. doi:10.1021/acs.nanolett.6b04060
Zhou, H., Yin, C., Zhang, Z., Tang, H., Shen, W., Zha, X., et al. (2020). Proanthocyanidin Promotes Functional Recovery of Spinal Cord Injury via Inhibiting Ferroptosis. J. Chem. Neuroanat. 107, 101807. doi:10.1016/j.jchemneu.2020.101807
Zhou, J., Chan, L., and Zhou, S. (2012). Trigonelline: a Plant Alkaloid with Therapeutic Potential for Diabetes and central Nervous System Disease. Cmc 19, 3523–3531. doi:10.2174/092986712801323171
Zhu, H.-Y., Huang, Z.-X., Chen, G.-Q., Sheng, F., and Zheng, Y.-S. (2019). Typhaneoside Prevents Acute Myeloid Leukemia (AML) through Suppressing Proliferation and Inducing Ferroptosis Associated with Autophagy. Biochem. biophysical Res. Commun. 516, 1265–1271. doi:10.1016/j.bbrc.2019.06.070
Keywords: iron homeostasis, lipid peroxidation, redox signaling, cancer, neurodegenerative diseases, natural products
Citation: Ge C, Zhang S, Mu H, Zheng S, Tan Z, Huang X, Xu C, Zou J, Zhu Y, Feng D and Aa J (2022) Emerging Mechanisms and Disease Implications of Ferroptosis: Potential Applications of Natural Products. Front. Cell Dev. Biol. 9:774957. doi: 10.3389/fcell.2021.774957
Received: 13 September 2021; Accepted: 09 December 2021;
Published: 18 January 2022.
Edited by:
Markus Ritter, Paracelsus Medical University, AustriaReviewed by:
Debia Wakhloo, Stanford University, United StatesFeng He, Shanghai University of Traditional Chinese Medicine, China
Copyright © 2022 Ge, Zhang, Mu, Zheng, Tan, Huang, Xu, Zou, Zhu, Feng and Aa. This is an open-access article distributed under the terms of the Creative Commons Attribution License (CC BY). The use, distribution or reproduction in other forums is permitted, provided the original author(s) and the copyright owner(s) are credited and that the original publication in this journal is cited, in accordance with accepted academic practice. No use, distribution or reproduction is permitted which does not comply with these terms.
*Correspondence: Yubing Zhu, NTcyMDE5MDAwMkBjcHUuZWR1LmNu; Dong Feng, d2RsanN5eDg2MTI4QDEyNi5jb20=; Jiye Aa, aml5ZWFAY3B1LmVkdS5jbg==
†These authors have contributed equally to this work and share first authorship