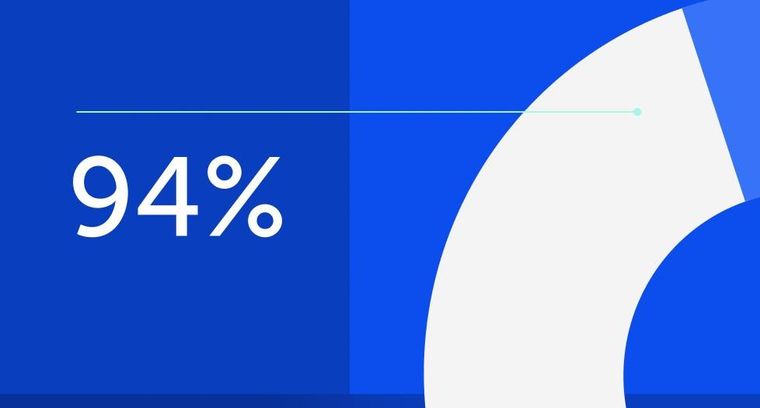
94% of researchers rate our articles as excellent or good
Learn more about the work of our research integrity team to safeguard the quality of each article we publish.
Find out more
REVIEW article
Front. Cell Dev. Biol., 12 November 2021
Sec. Epigenomics and Epigenetics
Volume 9 - 2021 | https://doi.org/10.3389/fcell.2021.772921
Sepsis is a life-threatening organ dysfunction caused by a host’s dysfunctional response to infection. As is known to all, septic heart disease occurs because pathogens invading the blood stimulate the activation of endothelial cells, causing a large number of white blood cells to accumulate and trigger an immune response. However, in severe sepsis, the hematopoietic system is inhibited, and there will also be a decline in white blood cells, at which time the autoimmune system will also be suppressed. During the immune response, a large number of inflammatory factors are released into cells to participate in the inflammatory process, which ultimately damages cardiac myocytes and leads to impaired cardiac function. N6-methyladenosine (m6A) is a common RNA modification in mRNA and non-coding RNA that affects RNA splicing, translation, stability, and epigenetic effects of some non-coding RNAs. A large number of emerging evidences demonstrated m6A modification had been involved in multiple biological processes, especially for sepsis and immune disorders. Unfortunately, there are limited results provided to analyze the association between m6A modification and sepsis-induced cardiovascular dysfunction (SICD). In this review, we firstly summarized current evidences on how m6A mediates the pathophysiological process in cardiac development and cardiomyopathy to emphasize the importance of RNA methylation in maintaining heart biogenesis and homeostasis. Then, we clarified the participants of m6A modification in extended inflammatory responses and immune system activation, which are the dominant and initial changes secondary to sepsis attack. After that, we deeply analyzed the top causes of SICD and identified the activation of inflammatory cytokines, endothelial cell dysfunction, and mitochondrial failure. Thus, the highlight of this review is that we systematically collected all the related potential mechanisms between m6A modification and SICD causes. Although there is lack of direct evidences on SICD, indirect evidences had been demonstrated case by case on every particular molecular mechanism and signal transduction, which require further explorations into the potential links among the listed mechanisms. This provides novel insights into the understanding of SICD.
Sepsis is defined as a highly heterogeneous syndrome that is associated with a dysregulated systemic inflammatory host response to infection and causes organ dysfunction (Singer et al., 2016; Cecconi et al., 2018). Sepsis can be caused by bacterial, viral, and fungal infections and is a major cause of death in critical care patients. Damage-associated molecular patterns (DAMPs), which can be released by necrotic cardiomyocytes, can also induce potent inflammatory responses and cause sepsis-induced injuries. In-hospital mortality among patients with septic shock is reported to reach 40% (Singer et al., 2016). Septic shock—a series of circulatory, metabolic, and cellular abnormalities—is defined by a requirement for vasopressor support and persistent hyperlactatemia in the absence of hypovolemia (Gotts and Matthay, 2016; Hollenberg and Singer, 2021). Epidemiological studies showed that approximately 28.3–41% of all hospitalized patients with sepsis died due to multiple-organ failure (Levy et al., 2012). Furthermore, sepsis-induced cardiovascular dysfunction (SICD) was identified as being closely associated with higher mortality rates (Hochstadt et al., 2011; Romero-Bermejo et al., 2011). Cardiac dysfunction is one of the major complications of sepsis and is therefore predictive of a poor clinical outcome. The pathophysiological changes that occur during sepsis mean cardiac lesions might be induced by a series of factors including myocardial ischemia, myocardial depressant substance, inflammation, deregulation of adrenergic pathways, calcium overload, mitochondrial disorder, coronary microvascular dysfunction, and myocardial damage (Hollenberg and Singer, 2021). Animal and cell experiments with lipopolysaccharide (LPS)-induced sepsis models demonstrated a significantly higher rate of cardiomyocyte apoptosis, accumulation of intracellular reactive oxygen species (ROS), elevated cytoplasmic levels of cytochrome C, and activated inflammatory pathways (Han et al., 2020).
At present, there are more than 100 recognized modifications involved in regulating RNA stability and function. In eukaryotes, 5′ caps and 3′ poly-A modifications play an important role in transcriptional regulation, while mRNA internal modifications are used to maintain mRNA stability (Tong et al., 2018a). Methylation of the sixth nitrogen atom on the RNA molecule adenosine, termed N6-methyladenosine (m6A) modification, contributes to RNA splicing, translation, stability, and epigenetic effects among mRNAs and non-coding RNAs. Environmental exposures can alter the m6A modification levels in several signaling processes, and imbalances in m6A can mediate disease pathogenesis, providing novel insights into the potential application of m6A modifications as biomarkers for numerous diseases, immune responses, and autoimmunity pathogenesis (Li et al., 2017; Tong et al., 2018b). In addition, m6A participates in regulating bacterial infection according to an analysis of m6A-SNP and expression quantitative trait locus (eQTL) data. Sun et al. identified 1,321 genes as locations of m6A-cis-eQTLs (Sun et al., 2020). These genes were enriched in pathways of platelet degranulation and Staphylococcus aureus infection, which are vital for the pathophysiological process of sepsis. Collectively, this emerging evidence indicates a convincing association between m6A modification and sepsis attacks. Moreover, such regulation of RNA methylation has been shown to be involved in several types of cardiovascular diseases. This review summarizes the relative evidences available to support whether m6A modification facilitates the mechanisms between sepsis-induced immune responses and cardiomyopathy or myocardial injuries.
The methylation of m6A is dynamically regulated by three regulatory factors, namely, methyltransferase (writers), demethylase (erasers), and methylated reading protein (readers) (Cao et al., 2016). Methylation of m6A is catalyzed by a multi-component methyltransferase complex consisting primarily of methyltransferase-like 3 (METTL3), methyltransferase-like 14 (METTL14), and WT1-associated protein (WTAP). METTL3 plays a central role and forms a dimer complex with METTL14 at a similar ratio, which is mainly located in the nuclear macular region. M6A-methylated mRNA requires a specific RNA-binding protein, methylated reader, to perform a specific biological function. The discovery of demethylase showed that the modification of m6A methylation was reversible. Currently known demethylation enzymes include FTO and ALKBH5, both of them belong to the ALKB family. The reading proteins found so far include YTH domain proteins (including YTHDF1, YTHDF2, YTHDF3, YTHDC1, and YTHDC2), the heterogeneous nuclear ribonucleoprotein family HNRNP (including HNRNPA2B1 and HNRNPC), and the IGF2BPS family (including IGF2BP1, IGF2BP2, and IGF2BP3), which are involved in mRNA translation, degradation, and processing (Li et al., 2017; Gao et al., 2020; Chen et al., 2021a; Chao et al., 2021; Zhou et al., 2021). The schematic diagram in Figure 1 presents the biological process of m6A modification.
To retrieve evidence on the relationship between m6A and SICD, it is important to identify the essentiality of m6A in cardiovascular diseases, which demonstrates that such RNA modification contributes significantly to the biological functions of heart contraction, coronary artery perfusion, and energy production. This section summarizes the evidence for the involvement of m6A modification in cardiovascular dysfunctions (CVDs) based on clinical research studies of patients with CVDs and basic biology explorations using animal or cell models of CVDs. The essential role of m6A in heart development and the generation of cardiomyopathy was revealed from such studies.
Regulation of heart development is extremely complex. The cardiovascular system consists of cardiomyocytes, fibroblasts, pericytes, endothelial cells, vascular smooth muscle cells, and cardiac-resident macrophages, which are all heterogeneous, and cells of the same type even show heterogeneity in different locations. m6A was found to play an important role in the regulation of heart development. Batista et al. analyzed the embryonic stem cell (ESC) m6A methylome in mouse and human cells and discovered extensive m6A modification of ESC genes, including most key regulators of ESC pluripotency and lineage control (Batista et al., 2014). Genetic inactivation or depletion of mouse and human METTL3 led to m6A erasure on selected target genes, prolonged NANOG expression upon differentiation, and disrupted exit of ESCs from self-renewal towards differentiation into several lineages in vitro and in vivo.
Postnatal cardiomyocyte morphology and functional maturation also involve m6A. Transcriptome-wide mapping of m6A in mRNA allowed m6A targets in human and murine adult hearts to be cataloged. Increased m6A methylation was found in human cardiomyopathy as knockdown and overexpression of the m6A writer enzyme METTL3 affected cell size and cellular remodeling both in vitro and in vivo (Kmietczyk et al., 2019). Furthermore, Dorn et al. found that METTL3-mediated methylation of mRNA on N6-adenosines was a dynamic modification that was enhanced in response to hypertrophic stimuli and was necessary for a normal hypertrophic response in cardiomyocytes (Dorn et al., 2019). Cardiac-specific METTL3 knockout mice also exhibited morphological and functional signs of heart failure with aging and stress. Berulava et al. observed that changes in m6A RNA methylation exceeded changes in gene expression both in mice and humans during progression to heart failure. The RNAs with altered m6A RNA methylation were mainly linked to metabolic and regulatory pathways, while changes in RNA expression level predominantly represented changes in structural plasticity (Berulava et al., 2020). These studies show the role of the regulation of m6A methylation in the occurrence and development of cardiomyopathy, highlighting the critical importance of this stress response mechanism for maintaining normal heart function.
The above information support the idea that m6A modification is involved in many kinds of cardiomyocyte injuries. However, as there is limited direct evidence available for SICD, a general understanding of the cellular and molecular mechanisms of SICD is required to determine whether m6A modification potentially affects SICD based on indirect evidences.
Under normal conditions, a controlled cellular response to bacterial products protects the host from infection. In sepsis, hyperactivation of the immune response leads to excessive production of various proinflammatory cytokines and induces cellular injury. In mammals, the innate immune system is the first line of host defense involved in detecting diverse invading microbial pathogens. Receptors of the innate immune system are activated by microbial components such as LPS, which is a key molecule involved in the initiation of sepsis syndrome. Cardiac dysfunction and cardiovascular collapse during sepsis result from increased levels of several cytokines and from increased production of nitric oxide (NO), which is cytotoxic and can form a complex by combining with cis-aconitase in the respiratory chain, mitochondrial respiratory chain complexes I and II, and the active site of key enzymes in DNA synthesis, thus destroying their activity and causing myocardium apoptosis, and mitochondrial failure in cardiomyocytes (CMs), which leads to further DNA damage and ATP depletion, resulting in secondary energy failure. Cardiomyocyte hypocontractility also occurs, which is related to hibernation to maintain myocyte viability by limiting oxygen consumption, energy requirements, and ATP. Moreover, it is reported that cardiac microvascular dysfunction is associated with cardiac dysfunction in patients with sepsis. Cardiac microvascular endothelial cells (CMVECs), accounting for one-third of all heart cells, exert an important influence on the normal condition of coronary microvessels and adjacent cardiomyocytes. The increased function of microvessels could induce hypoxic stress due to localized microcirculation failure, which might result in ischemic injuries and diffused fibrosis. Thus, maintenance of normal microvessel-based circulation is also an important aspect to preventing SICD.
Mammalian Toll-like receptors (TLRs) are pattern recognition receptors (PRR) that act as CD-14-associated signal transducers to help cells recognize and differentiate pathogens and initiate appropriate signaling cascades (Lackner et al., 2020). TLRs also bridge innate and adaptive immunity by inducing various costimulatory and effector molecules. TLR-1, 2, 4, 5, and 6 are expressed on the cell surface and activate the expression of pro-inflammatory cytokines (such as IL-1, IL-2, IL-6, IL-12, TNF-α, etc.) and cell surface adhesion molecules (such as ICAM-1, VCAM-1, and P-selectin) through nuclear factor kappa B (NF-κB) and interferon (IFN) signaling (Li et al., 2019; Ye et al., 2020).
TLR4 is the most studied TLR family member in SCID research (Chang et al., 2021). Studies on TLR4-deficient mice confirmed the essential role of TLR4 in mediating neutrophil migratory phagocytic functions, attenuating inflammation, reducing ROS generation, and enhancing bacterial clearance. In sepsis, LPS binds to the LPS-binding proteins MD-2 and CD14 and thus binds to TLR4. This interaction can lead to both MyD88-dependent and MyD88-independent signaling events (Feng et al., 2014). MyD88-dependent signaling pathways include activation of TLR4, which leads to recruitment of the adaptor protein MyD88 through its own TIR domain. This association leads to autophosphorylation of IRAK through homophilic interactions in the death domain (Gao et al., 2015). TNF receptor-associated factor 6 (Traf6) is then obtained to signal the activation of the protein kinase NF-κB to induce the kinase, which subsequently activates the IKB kinase to phosphorylate I-kB (Ma et al., 2016; Ren et al., 2016). This phosphorylation step promotes translocation of transcription factor NF-κB to the nucleus. However, TLR2 also increased cardiodepressant cytokine levels in the myocardium and serum and weakened the neutrophil migratory function, which sharpened the SICD (Zou et al., 2011; Zuo et al., 2020). TLR3 was observed to play a deleterious role in mediating cardiac dysfunction in sepsis by increasing cecal ligation and puncture-induced cardiomyocyte apoptosis as well as Fas and Fas ligand expression in the myocardium (Gao et al., 2012; Kalbitz et al., 2016; Fattahi et al., 2017).
Cytokines are known to induce the release of additional inflammatory factors such as prostanoids, NO, and many others, which eventually contribute to myocardial dysfunction. The main inflammatory mediators that might contribute to SICD are TNF-α, IL-1β, and IL-6. These cytokines significantly depressed cardiac contractility activity in vitro (Chen et al., 2021b). Moreover, increased circulating serum levels of IL-6 were associated with the severity of illness and the degree of vasopressor requirement in patients with septic shock (Gamkrelidze et al., 2014).
Sepsis induces endothelial abnormalities, which also lead to SICD. In response to inflammatory cytokines during sepsis, endothelial cells secrete more adhesion molecules, which increase leukocyte–endothelium interactions and facilitate neutrophilic infiltration that is damaging to cardiomyocytes (Lin et al., 2020a; Tu et al., 2020; Cao et al., 2021). The activated monocytes and macrophages are recruited by pro-inflammatory cytokines and are binding to ICAM-a and VCAM-1 molecules (Kang et al., 2012; Franceschelli et al., 2017). Subsequently, these monocytes can migrate into the inter-space among endothelial cells where they differentiate into type I macrophages causing fibrosis and endothelial damage (Li et al., 2012).
The heart is rich in mitochondria, which are involved in both energy provision and intracellular calcium regulation; consequently, the degree of mitochondrial dysfunction is tightly linked to SICD and the prognosis of this condition (Lin et al., 2020a; Shi et al., 2021a; Wang et al., 2021a). The activities of complexes I and II of the mitochondrial respiratory chain were diminished in the hearts from animals with sepsis (Piel et al., 2007; Neviere et al., 2016), and this might be due to the detrimental effects of sepsis mediators such as NO, TNF-α, IL-1β, etc. In addition, ROS accumulation can suppress mitochondrial function by destroying mitochondrial proteins, lipids, and DNA through structural modifications during sepsis in a positive feedback fashion as failed mitochondria led to significantly slower ROS scavenging (Potz et al., 2016). Oxidative stress can cause dynamic m6A modification in the 5′ UTR and 5′ vicinity of coding sequences (CDSs), which might play a vital role in overcoming the stress condition.
In recent years, m6A modification was shown to be involved in several inflammation-induced diseases, such as rheumatoid arthritis and diabetic retinopathy, and was confirmed to be associated with clinical prognosis of such diseases. Zhang et al. analyzed the gene expression datasets of 479 consecutive patients with severe sepsis and found ALKBH5, HNRNPC, KIAA1429, WTAP, and YTHDF2 were significantly correlated with 28-days cumulative mortality (p < 0.05). ALKBH5 and WTAP are risky genes with a hazard ratio (HR) > 1, while HNRNPC, KIAA1429, and YTHDF2 are protective genes with a HR < 1 (Zhang et al., 2020a). Lu et al. demonstrated that METTL3, METTL14, ALKBH5, FTO, and YTHDF2 participated in the inflammation activity in LPS-induced liver injuries (Lu et al., 2018).
m6A is also involved in the immune response in tumor growth and virus infection beyond sepsis attacks. Zhu et al. detected dozens of m6A-SNPs as critical functional polymorphisms and novel genetic biomarkers for ischemic stroke susceptibility and provided a new means of elucidating the biological mechanism underlying vascular inflammation development (Zhu et al., 2021). Chokkalla et al. presented the m6A epitranscriptome of the brain post-stroke, further verifying that m6A modification participated in inflammatory responses (Chokkalla et al., 2019). Recently, Li et al. identified two independent m6A modification patterns with distinct biological functions, immunological characteristics, and prognoses in renal clear cell carcinoma and showed that low-m6A-score groups reflected an inflammatory phenotype, which may be more sensitive to anticancer immunotherapy (Li et al., 2021a). Emerging research indicates that depletion of the host cell m6A methyltransferase METTL3 decreases m6A levels in SARS-CoV-2 and host genes, and the reduction of m6A in viral RNA increases RIG-I binding and subsequently activates downstream innate immune signaling pathways and inflammatory gene expression (Li et al., 2021b; Qu et al., 2021).
Current evidence clearly supports a relationship between m6A modification and inflammation syndrome(s). As sepsis is considered as acute and severe inflammation attacks, it is believed that m6A regulation participates in SICD. Therefore, the following subsections summarize the “writer,” “eraser,” and “reader” in the regulation of cytokines, monocytes/macrophages, and related non-coding RNAs in inflammation.
METTL3 mediated the increased expression of inflammatory cytokines, such as IL-6 and IL-8, and the activation of NF-κB signaling under immune stimulations (Sang et al., 2021). Mechanistically, the promoted expression of cytokines is induced by activation of Traf6 (Wen et al., 2020), and the inflammatory response is suppressed by METTL3 ablation. Knockdown of METTL3 decreased the expression of inflammatory cytokines and phosphorylation of IKKα/β, p65, and IκBα in NF-κB signaling. The depletion of METTL3 attenuated the MAPK pathways involving p38, ERK, and JNK. METTL3 knockdown facilitated the expression of MyD88S, a splice variant of MyD88 that inhibits inflammatory cytokine production, suggesting that METTL3 might inhibit the LPS-induced inflammatory response by regulating alternative splicing of MyD88 (Feng et al., 2018). MyD88 acts as a shared adaptor molecule for most TLRs and can elicit the production of multiple inflammatory cytokine genes and the activation of NF-κB and MAPK involved in neurotoxicity. The depletion of METTL3 decreased the m6A level of Traf6 mRNA, thereby its transcripts were trapped in the nucleus, and the decreased expression of Traf6 led to the suppression of NF-κB and MAPK signaling pathways (Zong et al., 2019). Besides, METTL3 knockdown promoted mRNA expression and stability of negative regulators of Smad signaling, Smad7 and Smurf1 (Zhang et al., 2019). Thus, METTL3 depletion inhibited proinflammatory cytokine expression by alleviating Smad signaling pathways.
METTL14 promoted FOXO1 expression via increased levels of m6A modification and induced endothelial cell inflammatory responses by facilitating TNF-a, IL-1, IL-3, and IL-6 secretion (Jian et al., 2020). Zong et al. revealed that the interplay between FOXO6 and METTL3 regulated the m6A level of GPR161 signaling and the expression of β-defensin during Escherichia coli infection (Zong et al., 2021a). The re-expression of METTL3 facilitated defensin accumulation (Zong et al., 2021b). The dissociation of METTL3 from the METTL3–METTL14–WTAP complex contributed to decreased miR-92b-3p expression in an m6A-dependent post-transcriptional modification manner and increased the protein level of the phosphatase and tensin homolog (PTEN), which subsequently inactivated the PI3K/AKT signaling pathway (Lin et al., 2020b). The PI3K/AKT pathway is known to inhibit FOXO1, which regulates endothelial immune responses.
Monocytes can be activated and recruited by the release of inflammatory cytokines. Activated monocytes adhere to endothelial cells to cause cellular injuries, then the monocytes differentiate into macrophages and migrate into the vascular smooth muscle layer, which leads to fibrotic changes to coronary arteries, as well as damage to myocardial tissues. Therefore, the biological functional status of monocytes or macrophages also greatly contributes to the progress of SICD. Through the use of a pooled CRISPR screening strategy, Tong et al. determined that transcripts of the Irakm gene, encoding a negative regulator of TLR4 signaling, were highly decorated by m6A modification, and congruent with this, Mettl3flox/flox;Lyzm-Cre mice displayed increased susceptibility to bacterial infection (Tong et al., 2021). METTL3 deficiency led to the loss of m6A modification on Irakm mRNA and slowed its degradation, resulting in an increased amount of IRAKM, which ultimately suppressed TLR signaling-mediated macrophage activation (Tong et al., 2021). Moreover, IFN regulatory factor-1 (IRF-1) induced METTL3 expression in macrophages and inhibited the expression of circ_0029589, triggering macrophage pyroptosis (Guo et al., 2020). A controversial opinion was proposed that METTL3 mediated the activities of macrophages in response to LPS stimulation via NF-κB signaling and that the overexpression of METTL3 could significantly attenuate the inflammatory response induced by LPS in macrophages (Wang et al., 2019a).
METTL14 in myeloid cells exacerbated macrophage responses to acute bacterial infection in mice, which led to higher mortality. METTL14 reduced the rate of Socs1 m6A methylation and decreased YTHDF1 binding. The reduced YTHDF1 m6A modification led to diminished SOCS1 and over-activated TLR4/NF-κB signaling. Overexpressing SOCS1 under METTL14 or YTHDF1 rescued the phenotype of macrophage activation in vitro and in vivo. Accordingly, FTO showed an antagonistic role of METTL14 in regulating macrophage activity (Du et al., 2020). Besides, METTL14 interacted with FOXO1 and acted directly on the promoter regions of VCAM-1 and ICAM-1, which enhanced monocyte binding to endothelial cells during atherosclerosis (Jian et al., 2020). METTL14 also regulated the expression of AKT signaling, and a METTL14 knockout exhibited reduced inflammation and cell survival behavior (Liu et al., 2019a).
Non-coding RNAs, which are involved in essential biological processes, are also subject to methylation by m6A modification. METTL3 has a major catalytic role in promoting miR-21-5p maturation, and miR-21-5p could activate the SPRY1/ERK/NF-kB pathway, which is involved in inflammation responses leading to renal fibrosis (Liu et al., 2021a). METTL3 positively modulated miR-365-3p processing in a microprocessor protein DiGeorge critical region 8-dependent manner, inducing inflammation and pain behavior. miR-365-3p was verified to be involved in cardiac hypertrophy (Zhang et al., 2020b). The activity of m6A modification was also regulated by other mechanisms because SUMOylation of METTL3 regulated its m6A RNA methyltransferase activity without altering its stability, localization, or interaction with METTL14 and WTAP (Du et al., 2018).
Opposite to the writer function, eraser leads to demethylation of RNA in an m6A modification manner. Therefore, understanding the eraser function in controlling inflammation is also necessary. ALKBH5 upregulated the expression of MALAT1 by demethylation, which participates in the production of inflammatory cytokines in HK-2 cells under LPS stimulation (Zhu and Lu, 2020). In addition, dexamethasone inhibited ALKBN5, which decreased related inflammatory cytokines and induced cell apoptosis (Zhu and Lu, 2020). ALKBH5 also participated in demethylating m6A residues in the 3′ untranslated region (UTR) of high-mobility group box 1 (HMGB1) (Chen et al., 2021c). This demethylation resulted in the activation of STING-IRF3 signaling, which mediated the innate immune response in radiation-induced liver disease (Chen et al., 2021c). CircZbtb20 enhanced the interaction of ALKBH5 with Nr4a1 mRNA, leading to ablation of the m6A modification of Nr4a1 mRNA to promote its stability (Liu et al., 2021b). Nr4a1 initiated Notch2 signaling activation, which contributed to the maintenance of type 3 innate lymphoid cell homeostasis. The deletion of ALKBH5 or Nr4a1 also impaired ILC3 homeostasis and increased susceptibility to bacterial infection (Liu et al., 2021b). Zhao et al. found that silencing zinc finger and BTB domain-containing protein 7B (Zbtb7b) resulted in higher radiation-induced IL-6 production in THP1 cells and BEAS-2B lung bronchial epithelial cells. Zbtb7b recruited RNA demethylase ALKBH5 to IL6 mRNA (Zhao et al., 2020). Subsequently, ALKBH5 demethylated the m6A modification of IL6 mRNA and inhibited its nuclear export in endothelial cells.
FTO has increasingly been found in response to inflammation. FTO regulates the integrin signaling pathway and inflammation signaling pathways. An RNA decay assay revealed that TEAD2 mRNA stability was impaired by FTO because FTO stimulated the degradation of TEAD2 and inhibited the formation of the YAP–TEAD complex, a main factor downstream of integrin signaling (Rong et al., 2019). YAP participates in TLR2 and TLR4 regulation, showing an anti-inflammatory function (Gao et al., 2021). In addition, Yu et al. found that an FTO-mediated YTHDF2 epigenetic modification increased the rate of methylation of PPARα, leading to activation of NLRP3 and NF-κB-driven inflammation (Yu et al., 2021). FTO also helped disable the mRNA of pro-inflammatory genes to suppress the activity of inflammatory responses, including IL-1β and TNF-α. In addition, SIRT1 activated RANBP2, which is indispensable for FTO SUMOylation at K-216 sites, leading to destabilization of FTO (Liu et al., 2020a).
YTHDF1 mediated the translation of XPO1 in an m6A-dependent manner by methylating the 5′ UTR of XPO1 RNA. The methylated XPO1 RNA provided greater amounts of XPO1 protein, which resulted in NK-κB activation and subsequent inflammation (Olazagoitia-Garmendia et al., 2021). A report from Zong et al. provided evidence on the function of YTHDF1 during infection of intestinal epithelial cells. The P/Q/N-rich domain in YTHDF1 was found to interact with the DEAD domain in the host factor DDX60, which helped target the Traf6 mRNA and regulated m6A modification near the stop codon of the transcript to initiate the expression of Traf6 (Zong et al., 2021a). The depletion of YTHDF1 alleviated the immune response to bacterial infection as evidenced through an observation that YTHDF1 knockdown macrophages improved the immune paralysis of macrophages, Th1/Th17 cells, and cytotoxic T lymphocytes and reduced the entry of macrophages into the brain to cause endothelial damage in severe sepsis rats with ECMO (Xing et al., 2021). This was through the inhibition of HMGB1/RAGE and YTHDF1 and m6A RNA methylation of JAK2/STAT3 in macrophages (Xing et al., 2021). The overexpression of miR-421-3p inhibited the expression of YTHDF1, which mediated the m6A modification of p65 mRNA. YTHDF1 is known to enhance the expression of p65; thus, miR-421-30/YTHDF1 presented a suppressing role on pro-inflammation factors (Zheng et al., 2020).
Experimentally induced inflammation increased YTHDF2 expression. YTHDF2 ablation increased the absence of multiple m6A-targeted inflammation transcripts, including IFN-γ, TNF-α, IFN-α, IFN regulatory factor 7, STAT1, and TLR4 signaling pathways, which led to the activation of pro-inflammation pathways (Mapperley et al., 2021). Moreover, YTHDF2 processed the decay of m6A containing the LPS-induced IL-1β, IL-6, IL-11, and IL-12 expression and the phosphorylation of p65, p38, and ERK1/2 in NF-κB and MAPK signaling. YTHDF2 depletion increased the expression and stability of MAP2K4 and MAP4K4 mRNAs (Yu et al., 2019). Together, these results suggest that YTHDF2 knockdown increases mRNA expression levels of MAP2K4 and MAP4K4 via stabilization of the mRNA transcripts, which activates MAPK and NF-κB signaling pathways, promoting the expression of proinflammatory cytokines and aggravating the inflammatory response in LPS-stimulated cells.
The loss of YTHDF2 promoted demethylation of H3K27me3, which led to an enhanced production of proinflammatory cytokines. The mRNA of lysine demethylase 6B (KDM6B) was m6A-modified, and its decay was mediated by YTHDF2. YTHDF2 deficiency stabilized KDM6B to promote H3K27me3 demethylation of multiple proinflammatory cytokines and subsequently enhanced their transcription (Wu et al., 2020). Besides, CCR7 stimulation upregulated lnc-Dpf3 via removal of the m6A modification to prevent RNA degradation by inhibiting YTHDF2. Lnc-Dpf3 directly bound to HIF-1α and suppressed HIF-1α-dependent transcription of the glycolytic gene LDHA, thus inhibiting dendritic cell (DC) glycolytic metabolism and migratory capacity. DC-specific lnc-Dpf3 deficiency also increased CCR7-mediated DC migration, leading to exaggerated adaptive immune responses and inflammatory injuries, which indicated that YTHDF2 had the capability to inhibit lnc-Dpf3 expression to enhance inflammatory activity (Liu et al., 2019b). Lysine acetyltransferase 1 (KAT1) activated the transcription activity of YTHDF2 through histone acetylation of the promoter. YTHDF2 then triggered the instability of ITGB1 mRNA to induce mRNA degradation in an m6A-dependent manner and silenced the FAK–PI3K–AKT pathway in endothelial cells, which alleviated the interaction between monocytes and endothelial cells (Qi et al., 2021). Also, SUMOylation of YTHDF2 had little impact on its ubiquitination and localization, but significantly increased its binding affinity to m6A-modified mRNAs and subsequently resulted in deregulated gene expression (Hou et al., 2021).
Besides the YTH domain family, several other readers are involved in m6A modification under inflammatory responses. Molecular studies indicated that IGF2BP2 switched M1 macrophages to M2 activation by targeting tuberous sclerosis 1 via an m6A-dependent process. The depletion of IGF2BP2 also enhanced the M1 phenotype. Additionally, it also has a mediator function on PPARγ involved in the differentiation of M2 macrophages (Wang et al., 2021b). Bechara et al. demonstrated the unexpected involvement of m6A modification in regulating C/EBPβ and C/EBPδ under autoimmune inflammation. The reader “IMP2” facilitated IL-17-mediated Cebpd mRNA stabilization and promoted translation of C/EBPβ/δ. In addition, Imp2 (−/−) mice were resistant to autoantibody-induced glomerulonephritis (AGN), showing impaired renal expression of C/EBPs and LCN2 (Bechara et al., 2021).
The biogenesis of mitochondria is critical for maturation of mitochondrial function. Sufficient amounts of mitochondria help maintain cardiomyocyte morphology and optimal energy production (Liao et al., 2020). METTL3-mediated RNA m6A modification and activation of mitochondrial fusion proteins MFN2 and OPA1, as well as inhibition of the mitophagy BNIP3/NIX receptor pathway, restored mitochondrial function. ALKBH1-deficient HEK293 cells showed increased mtDNA copy numbers and mitochondrial dysfunction (Lv et al., 2021). Meanwhile, Du et al. found that the expression of m6A demethylase FTO was decreased during ischemia–reperfusion injury. FTO contributed to the protective effect via demethylation of the Drp1 mRNA and impairment of the Drp1-mediated mitochondrial fragmentation. LPS-induced sepsis caused a continuous high expression of Drp1 (Du et al., 2021), and Drp1 induced mitochondrial fragmentation leading to mitochondrial dysfunction. In addition, AAV-delivered FTO could ameliorate the ischemia–reperfusion injury, repress the elevated level of m6A-methylated RNA, and alleviate oxidative stress and mitochondrial fragmentation in vivo and in vitro (Liu et al., 2020b). Moreover, a research from Wang et al. demonstrated that downregulation of FTO suppressed mitochondrial biogenesis and energy production, shown as decreased mitochondrial mass and mtDNA content through inhibition of PGC-1α expression. Importantly, this research revealed that this regulation was mTOR signaling-dependent. The suppression of mTOR blocked the enhanced transcription of FTO-induced PGC-1α (Wang et al., 2017). Readers also participate in maintaining mitochondrial biogenesis. The overexpression of either YTHDF1 or YTHDF2 led to the upregulation of the expression of MGMT, NDRG1, PGC1, LONP1, and TFAM, resulting in a more stable mitochondrial homeostasis (Ozkurede et al., 2019).
Besides the morphology of mitochondria, the metabolic function or status is related to the prognosis of cardiovascular diseases. Thus, deciphering the relationship between m6A modification and mitochondrial function is critical to reveal the potential mechanisms of m6A in SICD. METTL3 regulated NRIP1 (encoding RIP140) expression in an m6A-dependent manner, and METTL3 knockdown reduced the m6A modification of NRIP1 mRNA and increased its expression. The decay rate of NRIP1 mRNA was significantly reduced in METTL3 knockdown cells but increased in METTL3-overexpressing cells. RIP140 has a role in inflammation since it acts as a coactivator for NF-κB/RelA-dependent cytokine gene expression. Lack of RIP140 leads to inhibition of proinflammatory pathways in macrophages. RIP140 is also instrumental in regulating lipid and glucose metabolism and regulates gene expression in metabolic tissues including the heart and skeletal muscle (Shi et al., 2021b). NRIP1 affects oxidative metabolism and mitochondrial biogenesis by negatively controlling mitochondrial pathways regulated by PGC-1α. The downregulation of NRIP1 would thus attenuate the dysfunction of mitochondria. However, a study by Zhuang et al. reported that FTO enhanced the expression of PGC-1α, which helped restore mitochondrial function (Zhuang et al., 2019).
Adenylate kinase 4 (AK4) participates in the regulation of the ADP/ATP production cycle of mitochondria. However, AK4 was identified as being downregulated in sepsis, which lowered the cellular ATP level (Liu et al., 2020c). Liu et al. demonstrated that METTL3 controlled the m6A modification of AK4 mRNA and maintained the expression of the protein. A higher level of expression of AK4 promoted inflammatory gene expression via Hif-1α and AMPK signaling in macrophages and induced ROS accumulation (Liu et al., 2020c). Moreover, the transcription factor Hif-2α was mediated by METTL3 methylation, and this enhanced its expression. Hif-2α then targeted MTHFD2 and induced MTHFD2 gene transcription. MTHDF2 is involved in the serine metabolism circle and supported IL-1β production in macrophages (Li et al., 2020).
m6A modification marks mitochondrial stress response genes and promotes their transcription to alleviate mitochondrial stress in progeny. MiR-600 inhibited the expression of METTL3 and increased the Bax/Bcl-2 ratio, indicating activation of the mitochondrial apoptotic pathway (Wei et al., 2019). Jia et al. revealed that YTHDF1 promoted the translation of methylated HINT2 mRNA, which worked as a mitochondrial apoptotic sensitizer to induce cell death (Jia et al., 2019). Furthermore, Shen et al. demonstrated that FTO suppressed the mitochondrial unfolded protein response (UPRmt) and inhibited the activation of caspase-3 via the JAK2/STAT3 signaling pathway. UPRmt is associated with mitochondrial function. In septic rats, UPRmt is known to be downregulated under sepsis attacks (Shen et al., 2021). Enhancement of endogenous UPRmt through oligomycin administration reduced sepsis-mediated mitochondrial injury and myocardial dysfunction through mitophagy (Wang et al., 2021a).
m6A methylation is the most prevalent post-transcriptional modification on mRNAs. Post-transcriptional regulation has several advantages, such as prompt stimuli responses, fine-tuning of protein amounts, and localized regulation control. In coronary heart disease, endothelial dysfunction caused by various factors and the repair after hypoxia stimulation play an important role in pathological changes.
Firstly, METTL3 was shown to be involved in plaque formation by stimulating recruitment of monocytes and macrophages. Altered m6A levels were confirmed in leukocytes and the endothelium in western diet-induced atherosclerosis mice and in oxidized-LDL (ox-LDL)-treated human endothelium and monocyte cells (Wu et al., 2019). The upregulation of METTL3 expression increased NF-κB and p65 phosphorylation and enhanced monocyte adhesion to endothelial cells (Chien et al., 2021). In addition, METTL3-mediated RNA hypermethylation upregulated NLRP1 transcripts and downregulated KLF4 transcripts through YTHDF1 and YTHDF2 m6A reader proteins, which trigger the atherogenesis (Chien et al., 2021). Another m6A writer, METTL14, has been shown to interact with FOXO1 and act directly on the promoter regions of VCAM-1 and ICAM-1, which enhanced the binding of monocytes to endothelial cells during atherosclerosis (Jian et al., 2020). Moreover, METTL14 increased the m6A modification of pri-miR-19a and promoted the processing of mature miR-19a, thus enhancing the proliferation and invasion of CMVECs (Zhang et al., 2020c). Moreover, miR-19a was shown to improve prognosis and clinical cardiac function after myocardial infarction (Gao et al., 2019).
Secondly, the demethylase (“eraser”) contributed to coronary artery diseases in a deteriorative role. ALKBH1 decreased the m6A modification of the myocardial infarction associated transcript (MIAT) promoter region, which allowed HIF-1α binding to this site to upregulate the transcripts of lnc-MIAT. In turn, lnc-MIAT promoted the inflammation response to sepsis in endothelial cells, driving endothelial dysfunction (Wu et al., 2019). Furthermore, Zhao et al. demonstrated that ALKBH5 was elevated during hypoxic stimulation of CMVECs and caused a general reduction in m6A modification. ALKBH5 attenuated the stability of the mRNA of WNT5A and incited the process of angiogenesis, which exacerbated the hypoxia of CMs during sepsis attacks. An AAV strategy has been used to knockdown ALKBH5 to promote angiogenic phenotypes (Zhao et al., 2021). Therefore, METTL3 and METTL14 worked in a protective role to maintain microvascular circulation and myocardial function during sepsis. Controversially, ALKBH1 presented a negative role in maintaining the normal biological function of endothelial cells.
METTL3 predominantly participates in the regulation of vascular endothelial growth factor (VEGF)-A, which is regulated by mTOR-AKT, NOTCH, and LRP6 signaling, as well as ubiquitylation modification. Studies from Tian et al. (Tian et al., 2019) and Chen et al. (Chen et al., 2019) demonstrated that knockdown of METTL3 also restrained the expression of VEGF-A. Mechanistically, deletion of METTL3 leads to the suppression of endothelial tyrosine kinase and VEGF-A through reduced abundance of m6A peaks on a specific region (Wang et al., 2021c). Parial et al. observed that knockdown of METTL3 in human endothelial cells inhibited cell proliferation, migration, and capillary tube formation. METTL3 was found to activate the phosphorylation of PHLPP2-mTOR-AKT signaling to enhance angiogenesis (Parial et al., 2021). Yao et al. studied the function of METTL3 in endothelial cells under hypoxia stress and found that METTL3 promoted neovascularization by methylating the mRNA of LRP6 and DVL1 in a YTHDF1-dependent manner. LRP6 and DVL1 were initiated in LPS-induced endothelial cells (Yao et al., 2020). METTL3 also increased splicing of precursor miR-143-3p to facilitate its biogenesis, and miR-143-3p bound to sites in the 3′ UTR of vasohibin-1 (VASH1) to inhibit its expression. A lower expression of VASH1 was presumed to reduce ubiquitylation of VEGF-A and increase VEGF-A expression (Wang et al., 2019b).
Two pioneering studies in 2017 and 2018 provided the initial understanding of how m6A regulates endothelial fate and function in angiogenesis (Zhang et al., 2017; Lv et al., 2018). Endothelial expression of METTL3 could methylate Notch1 mRNA, and this led to repression of Notch activity. Moreover, YTHDF2-mediated mRNA decay of the arterial endothelial genes Notch1a and rhoca also contributed to this maintenance of normal angiogenesis under METTL3 methylation (Zhang et al., 2017). In addition, METTL3 could reduce the level of heterodimeric Notch E3 ubiquitin ligase formed by DTX1 and DTX3L and continuously activate the Notch signaling pathway, which affected the angiogenesis of endothelial cells (Wang et al., 2020a).
Similarly, high levels of METTL14 expression on vascular endothelial cells (CD31+) suggested that angiogenesis was accompanied by differential modification of m6A RNA. However, miR-4729 inhibited the expression of METTL14 by targeting the 3′ UTR of METTL14 mRNA and reducing the m6A modification of TIE1 mRNA and downstream VEGF-A signaling (Liu et al., 2021c). A subsequent study confirmed that IGF2BP3 could bind to VEGF mRNA and read the m6A modification, thus regulating both expression and stability of VEGF mRNA. Knockdown of IGF2BP3 repressed angiogenesis (Yang et al., 2020). VEGF has also been reported to be increased in response to septic inflammation, which provides a reasonable explanation for the regulation of METTL3, METTL14, and IGF2BP3 in sepsis. In addition, WTAP maintains desmoplakin (DSP) expression through m6A modification in an IGF2BPS-dependent manner and enhances angiogenesis of endothelial cells in vitro by regulating WNT signaling activity (Wang et al., 2020b).
Sepsis is a life-threatening organ dysfunction caused by a host’s dysfunctional response to infection. Pathogenic bacteria invade the human heart, lungs, liver, spleen, and other organs and produce inflammatory substances in these tissues. This can result in various cardiovascular diseases. Septic heart disease occurs because pathogens invading the blood stimulate the activation of endothelial cells, cause accumulation of vast quantities of white blood cells, and trigger an immune response. During the immune response, numerous inflammatory factors are released into cells to participate in the inflammatory process, which ultimately damages cardiac myocytes and leads to impaired cardiac function. Mitochondrial dysfunction is one of the main manifestations of myocardial cell injury. Increasing evidence has demonstrated the role of m6A modification in multiple biological processes, particularly for sepsis and immune disorders. In addition, a series of studies have confirmed the role of m6A modification in the regulation of cardiovascular diseases. Unfortunately, there is limited evidence available to analyze the association between m6A modification and SICD. In this review, we firstly summarized current evidence on m6A mediation of the pathophysiological processes involved in cardiac development and cardiomyopathy to emphasize the importance of RNA methylation in maintaining heart biogenesis and homeostasis. Next, we clarified the participants of m6A modification in extended inflammation responses and immune system activation, which are the dominant and initial changes secondary to sepsis attack. Finally, the predominant causes of SICD were analyzed in detail, identifying activation of TLRs and inflammatory cytokines, endothelial cell dysfunction, and mitochondrial failure. Thus, this review systematically collates all related potential mechanisms between m6A modification and causes of SICD and provides novel insights into the understanding of SICD. Although there is lack of direct evidence available for SICD, the indirect evidences have been presented, case by case, for each particular molecular mechanism and signaling transduction process (Figure 2). Further explorations are required into the potential links among the listed mechanisms to obtain direct evidence for the roles of m6A modification in SICD.
FIGURE 2. The mechanisms involved in SICD and the potential m6A modification process that would contribute to SICD. Some important m6A-mediating targets or signaling pathways are also listed in the right panel.
JT and YL conceived of the presented idea. YW, MX, and PY summarized the reference and draft the manuscript. DZ organized the figure with online free material. DZ, JT, and YL supervised the project and contributed equally to the final version of the manuscript.
All phase of this study was supported by a Hubei Province Science Fund for Distinguished Young Scholars (2019CFA092), Key R and D Program of Sichuan Province of China (2020YFS0102) and Natural Science Foundation of China (81700360, 31871496). The funders had no role in study design, data collection and analysis, decision to publish, or preparation of the manuscript.
The authors declare that the research was conducted in the absence of any commercial or financial relationships that could be construed as a potential conflict of interest.
All claims expressed in this article are solely those of the authors and do not necessarily represent those of their affiliated organizations, or those of the publisher, the editors, and the reviewers. Any product that may be evaluated in this article, or claim that may be made by its manufacturer, is not guaranteed or endorsed by the publisher.
AK4, adenylate kinase 4; CMs, cardiomyocytes; CMVES, cardiac microvascular endothelial cells; CVDs, cardiovascular dysfunctions; DC, dendritic cell; DSP, desmoplakin; eQTLs, expression quantitative trait loci; ESC, embryonic stem cell; HMGB1, high-mobility group box 1; IFN, interferon; IRF-1, IFN regulatory factor-1; KAT1, lysine acetyltransferase 1; LPS, lipopolysaccharide; m6A, N6-methyladenosine; NF-κB, nuclear factor kappa B; PRR, pattern recognition receptors; PTEN, phosphatase and tensin homolog; ROS, reactive oxygen species; SICD, sepsis-induced cardiovascular dysfunction; Traf6, TNF receptor-associated factor 6; UPRmt, mitochondrial unfolded protein response; UTR, untranslated region; VASH1, vasohibin-1; VEGF, vascular endothelial growth factor; Zbtb7b, zinc finger and BTB domain-containing protein 7B.
Batista, P. J., Molinie, B., Wang, J., Qu, K., Zhang, J., Li, L., et al. (2014). m6A RNA Modification Controls Cell Fate Transition in Mammalian Embryonic Stem CellsA RNA Modification Controls Cell Fate Transition in Mammalian Embryonic Stem Cells. Cell Stem Cell 15 (6), 707–719. doi:10.1016/j.stem.2014.09.019
Bechara, R., Amatya, N., Bailey, R. D., Li, Y., Aggor, F. E. Y., Li, D.-D., et al. (2021). The M 6 A Reader IMP2 Directs Autoimmune Inflammation through an IL-17- and TNFα-dependent C/EBP Transcription Factor axis. Sci. Immunol. 6 (61), 6. doi:10.1126/sciimmunol.abd1287
Berulava, T., Buchholz, E., Elerdashvili, V., Pena, T., Islam, M. R., Lbik, D., et al. (2020). Changes in m6A RNA Methylation Contribute to Heart Failure Progression by Modulating Translation. Eur. J. Heart Fail. 22 (1), 54–66. doi:10.1002/ejhf.1672
Cao, G., Li, H.-B., Yin, Z., and Flavell, R. A. (2016). Recent Advances in Dynamic M 6 A RNA Modification. Open Biol. 6 (4), 160003. doi:10.1098/rsob.160003
Cao, Y., Wang, Y., Xiao, L., Xu, J. Y., Liu, Y., Jiang, R., et al. (2021). Endothelial-derived Exosomes Induced by Lipopolysaccharide Alleviate Rat Cardiomyocytes Injury and Apoptosis. Am. J. Transl Res. 13 (3), 1432–1444.
Cecconi, M., Evans, L., Levy, M., and Rhodes, A. (2018). Sepsis and Septic Shock. The Lancet 392 (10141), 75–87. doi:10.1016/s0140-6736(18)30696-2
Chang, C., Hu, L., Sun, S., Song, Y., Liu, S., Wang, J., et al. (2021). Regulatory Role of the TLR4/JNK Signaling Pathway in Sepsis Myocardial Dysfunction. Mol. Med. Rep. 23 (5). doi:10.3892/mmr.2021.11973
Chao, Y., Li, H.-B., and Zhou, J. (2021). Multiple Functions of RNA Methylation in T Cells: A Review. Front. Immunol. 12, 627455. doi:10.3389/fimmu.2021.627455
Chen, G., Zhao, Q., Yuan, B., Wang, B., Zhang, Y., Li, Z., et al. (2021). ALKBH5-modified HMGB1-STING Activation Contributes to Radiation Induced Liver Disease via Innate Immune Response. Int. J. Radiat. Oncology*Biology*Physics 111, 491–501. doi:10.1016/j.ijrobp.2021.05.115
Chen, H., Yao, J., Bao, R., Dong, Y., Zhang, T., Du, Y., et al. (2021). Cross-talk of Four Types of RNA Modification Writers Defines Tumor Microenvironment and Pharmacogenomic Landscape in Colorectal Cancer. Mol. Cancer 20 (1), 29. doi:10.1186/s12943-021-01322-w
Chen, X., Hua, W., Huang, X., Chen, Y., Zhang, J., and Li, G. (2019). Regulatory Role of RNA N6-Methyladenosine Modification in Bone Biology and Osteoporosis. Front. Endocrinol. 10, 911. doi:10.3389/fendo.2019.00911
Chen, Y.-H., Teng, X., Hu, Z.-J., Tian, D.-Y., Jin, S., and Wu, Y.-M. (2021). Hydrogen Sulfide Attenuated Sepsis-Induced Myocardial Dysfunction through TLR4 Pathway and Endoplasmic Reticulum Stress. Front. Physiol. 12, 653601. doi:10.3389/fphys.2021.653601
Chien, C.-S., Li, J. Y.-S., Chien, Y., Wang, M.-L., Yarmishyn, A. A., Tsai, P.-H., et al. (2021). METTL3-dependent N6-Methyladenosine RNA Modification Mediates the Atherogenic Inflammatory Cascades in Vascular Endothelium. Proc. Natl. Acad. Sci. USA 118 (7), e2025070118. doi:10.1073/pnas.2025070118
Chokkalla, A. K., Mehta, S. L., Kim, T., Chelluboina, B., Kim, J., and Vemuganti, R. (2019). Transient Focal Ischemia Significantly Alters the M 6 A Epitranscriptomic Tagging of RNAs in the Brain. Stroke 50 (10), 2912–2921. doi:10.1161/strokeaha.119.026433
Dorn, L. E., Lasman, L., Chen, J., Xu, X., Hund, T. J., Medvedovic, M., et al. (2019). The N 6 -Methyladenosine mRNA Methylase METTL3 Controls Cardiac Homeostasis and Hypertrophy. Circulation 139 (4), 533–545. doi:10.1161/circulationaha.118.036146
Du, J., Liao, W., Liu, W., Deb, D. K., He, L., Hsu, P. J., et al. (2020). N6-Adenosine Methylation of Socs1 mRNA Is Required to Sustain the Negative Feedback Control of Macrophage Activation. Develop. Cel. 55 (6), 737–753. e7. doi:10.1016/j.devcel.2020.10.023
Du, Y. D., Guo, W. Y., Han, C. H., Wang, Y., Chen, X. S., Li, D. W., et al. (2021). N6-methyladenosine Demethylase FTO Impairs Hepatic Ischemia-Reperfusion Injury via Inhibiting Drp1-Mediated Mitochondrial Fragmentation. Cell Death Dis 12 (5), 442. doi:10.1038/s41419-021-03622-x
Du, Y., Hou, G., Zhang, H., Dou, J., He, J., Guo, Y., et al. (2018). SUMOylation of the m6A-RNA Methyltransferase METTL3 Modulates its Function. Nucleic Acids Res. 46 (10), 5195–5208. doi:10.1093/nar/gky156
Fattahi, F., Kalbitz, M., Malan, E. A., Abe, E., Jajou, L., Huber‐Lang, M. S., et al. (2017). Complement‐induced Activation of MAPKs and Akt during Sepsis: Role in Cardiac Dysfunction. FASEB j. 31 (9), 4129–4139. doi:10.1096/fj.201700140R
Feng, Y., Zou, L., Chen, C., Li, D., and Chao, W. (2014). Role of Cardiac- and Myeloid-MyD88 Signaling in Endotoxin Shock. Anesthesiology 121 (6), 1258–1269. doi:10.1097/aln.0000000000000398
Feng, Z., Li, Q., Meng, R., Yi, B., and Xu, Q. (2018). METTL 3 Regulates Alternative Splicing of MyD88 upon the Lipopolysaccharide‐induced Inflammatory Response in Human Dental Pulp Cells. J. Cel Mol Med 22 (5), 2558–2568. doi:10.1111/jcmm.13491
Franceschelli, S., Pesce, M., Ferrone, A., Gatta, D. M. P., Patruno, A., Lutiis, M. A. D., et al. (2017). Biological Effect of Licochalcone C on the Regulation of PI3K/Akt/eNOS and NF-κB/iNOS/NO Signaling Pathways in H9c2 Cells in Response to LPS Stimulation. Ijms 18 (4), 690. doi:10.3390/ijms18040690
Gamkrelidze, M., Intskirveli, N., Vardosanidze, K., Goliadze, L., Chikhladze, K. H., and Ratiani, L. (2014). Myocardial Dysfunction during Septic Shock (Review). Georgian Med. News 237, 40–46.
Gao, F., Kataoka, M., Liu, N., Liang, T., Huang, Z.-P., Gu, F., et al. (2019). Therapeutic Role of miR-19a/19b in Cardiac Regeneration and protection from Myocardial Infarction. Nat. Commun. 10 (1), 1802. doi:10.1038/s41467-019-09530-1
Gao, M., Ha, T., Zhang, X., Liu, L., Wang, X., Kelley, J., et al. (2012). Toll-like Receptor 3 Plays a central Role in Cardiac Dysfunction during Polymicrobial Sepsis*. Crit. Care Med. 40 (8), 2390–2399. doi:10.1097/CCM.0b013e3182535aeb
Gao, M., Wang, X., Zhang, X., Ha, T., Ma, H., Liu, L., et al. (2015). Attenuation of Cardiac Dysfunction in Polymicrobial Sepsis by MicroRNA-146a Is Mediated via Targeting of IRAK1 and TRAF6 Expression. J.I. 195 (2), 672–682. doi:10.4049/jimmunol.1403155
Gao, Y., Sun, Y., Ercan-Sencicek, A. G., King, J. S., Akerberg, B. N., Ma, Q., et al. (2021). YAP/TEAD1 Complex Is a Default Repressor of Cardiac Toll-like Receptor Genes. Ijms 22 (13), 6649. doi:10.3390/ijms22136649
Gao, Y., Vasic, R., Song, Y., Teng, R., Liu, C., Gbyli, R., et al. (2020). m6A Modification Prevents Formation of Endogenous Double-Stranded RNAs and Deleterious Innate Immune Responses during Hematopoietic DevelopmentA Modification Prevents Formation of Endogenous Double-Stranded RNAs and Deleterious Innate Immune Responses during Hematopoietic Development. Immunity 52 (6), 1007–1021. e8. doi:10.1016/j.immuni.2020.05.003
Gotts, J. E., and Matthay, M. A. (2016). Sepsis: Pathophysiology and Clinical Management. Bmj 353, i1585. doi:10.1136/bmj.i1585
Guo, M., Yan, R., Ji, Q., Yao, H., Sun, M., Duan, L., et al. (2020). IFN Regulatory Factor-1 Induced Macrophage Pyroptosis by Modulating m6A Modification of Circ_0029589 in Patients with Acute Coronary Syndrome. Int. immunopharmacology 86, 106800. doi:10.1016/j.intimp.2020.106800
Han, Y., Cai, Y., Lai, X., Wang, Z., Wei, S., Tan, K., et al. (2020). lncRNA RMRP Prevents Mitochondrial Dysfunction and Cardiomyocyte Apoptosis via the miR-1-5p/hsp70 Axis in LPS-Induced Sepsis Mice. Inflammation 43 (2), 605–618. doi:10.1007/s10753-019-01141-8
Hochstadt, A., Meroz, Y., and Landesberg, G. (2011). Myocardial Dysfunction in Severe Sepsis and Septic Shock: More Questions Than Answers? J. Cardiothorac. Vasc. Anesth. 25 (3), 526–535. doi:10.1053/j.jvca.2010.11.026
Hollenberg, S. M., and Singer, M. (2021). Pathophysiology of Sepsis-Induced Cardiomyopathy. Nat. Rev. Cardiol. 18, 424–434. doi:10.1038/s41569-020-00492-2
Hou, G., Zhao, X., Li, L., Yang, Q., Liu, X., Huang, C., et al. (2021). SUMOylation of YTHDF2 Promotes mRNA Degradation and Cancer Progression by Increasing its Binding Affinity with m6A-Modified mRNAs. Nucleic Acids Res. 49 (5), 2859–2877. doi:10.1093/nar/gkab065
Jia, R., Chai, P., Wang, S., Sun, B., Xu, Y., Yang, Y., et al. (2019). m6A Modification Suppresses Ocular Melanoma through Modulating HINT2 mRNA Translation. Mol. Cancer 18 (1), 161. doi:10.1186/s12943-019-1088-x
Jian, D., Wang, Y., Jian, L., Tang, H., Rao, L., Chen, K., et al. (2020). METTL14 Aggravates Endothelial Inflammation and Atherosclerosis by Increasing FOXO1 N6-Methyladeosine Modifications. Theranostics 10 (20), 8939–8956. doi:10.7150/thno.45178
Kalbitz, M., Fattahi, F., Grailer, J. J., Jajou, L., Malan, E. A., Zetoune, F. S., et al. (2016). Complement‐induced Activation of the Cardiac NLRP3 Inflammasome in Sepsis. FASEB j. 30 (12), 3997–4006. doi:10.1096/fj.201600728R
Kang, K., Lee, A. S., Kim, D. H., Jung, Y. J., Lee, S., Park, B. H., et al. (2012). Janex-1, a JAK3 Inhibitor, Ameliorates Tumor Necrosis Factor α induced Expression of Cell Adhesion Molecules and Improves Myocardial Vascular Permeability in Endotoxemic Mice. Int. J. Mol. Med. 29 (5), 864–870. doi:10.3892/ijmm.2012.920
Kmietczyk, V., Riechert, E., Kalinski, L., Boileau, E., Malovrh, E., Malone, B., et al. (2019). m6A-mRNA Methylation Regulates Cardiac Gene Expression and Cellular growthA-mRNA Methylation Regulates Cardiac Gene Expression and Cellular Growth. Life Sci. Alliance 2 (2), e201800233. doi:10.26508/lsa.201800233
Lackner, I., Weber, B., Chakraborty, S., Braumüller, S., Huber-Lang, M., Gebhard, F., et al. (2020). Toll-Like Receptor-Mediated Cardiac Injury during Experimental Sepsis. Mediators Inflamm. 2020, 6051983. doi:10.1155/2020/6051983
Levy, M. M., Artigas, A., Phillips, G. S., Rhodes, A., Beale, R., Osborn, T., et al. (2012). Outcomes of the Surviving Sepsis Campaign in Intensive Care Units in the USA and Europe: a Prospective Cohort Study. Lancet Infect. Dis. 12 (12), 919–924. doi:10.1016/s1473-3099(12)70239-6
Li, H.-B., Tong, J., Zhu, S., Batista, P. J., Duffy, E. E., Zhao, J., et al. (2017). m6A mRNA Methylation Controls T Cell Homeostasis by Targeting the IL-7/STAT5/SOCS pathwaysA mRNA Methylation Controls T Cell Homeostasis by Targeting the IL-7/STAT5/SOCS Pathways. Nature 548 (7667), 338–342. doi:10.1038/nature23450
Li, H., Hu, J., Yu, A., Othmane, B., Guo, T., Liu, J., et al. (2021). RNA Modification of N6-Methyladenosine Predicts Immune Phenotypes and Therapeutic Opportunities in Kidney Renal Clear Cell Carcinoma. Front. Oncol. 11, 642159. doi:10.3389/fonc.2021.642159
Li, H., Zhang, Z., Feng, D., Xu, L., Li, F., Liu, J., et al. (2020). PGRN Exerts Inflammatory Effects via SIRT1-NF-Κb in Adipose Insulin Resistance. J. Mol. Endocrinol. 64 (3), 181–193. doi:10.1530/jme-19-0211
Li, N., Hui, H., Bray, B., Gonzalez, G. M., Zeller, M., Anderson, K. G., et al. (2021). METTL3 Regulates Viral m6A RNA Modification and Host Cell Innate Immune Responses during SARS-CoV-2 Infection. Cel Rep. 35 (6), 109091. doi:10.1016/j.celrep.2021.109091
Li, N., Zhou, H., Wu, H., Wu, Q., Duan, M., Deng, W., et al. (2019). STING-IRF3 Contributes to Lipopolysaccharide-Induced Cardiac Dysfunction, Inflammation, Apoptosis and Pyroptosis by Activating NLRP3. Redox Biol. 24, 101215. doi:10.1016/j.redox.2019.101215
Li, Z., Bryant, A. E., Parimon, T., and Stevens, D. L. (2012). Cardiac Dysfunction in StrepTSS: Group A streptococcus Disrupts the Directional Cardiomyocyte-To-Macrophage Crosstalk that Maintains Macrophage Quiescence. Cytokine 59 (1), 191–194. doi:10.1016/j.cyto.2012.03.023
Liao, H., Qi, Y., Ye, Y., Yue, P., Zhang, D., and Li, Y. (2020). Mechanotranduction Pathways in the Regulation of Mitochondrial Homeostasis in Cardiomyocytes. Front. Cel Dev. Biol. 8, 625089. doi:10.3389/fcell.2020.625089
Lin, R., Zhan, M., Yang, L., Wang, H., Shen, H., Huang, S., et al. (2020). Deoxycholic Acid Modulates the Progression of Gallbladder Cancer through N6-methyladenosine-dependent microRNA Maturation. Oncogene 39 (26), 4983–5000. doi:10.1038/s41388-020-1349-6
Lin, Y., Xu, Y., and Zhang, Z. (2020). Sepsis-Induced Myocardial Dysfunction (SIMD): the Pathophysiological Mechanisms and Therapeutic Strategies Targeting Mitochondria. Inflammation 43 (4), 1184–1200. doi:10.1007/s10753-020-01233-w
Liu, B., Liu, N., Zhu, X., Yang, L., Ye, B., Li, H., et al. (2021). Circular RNA circZbtb20 Maintains ILC3 Homeostasis and Function via Alkbh5-dependent m6A Demethylation of Nr4a1 mRNA. Cell Mol Immunol 18 (6), 1412–1424. doi:10.1038/s41423-021-00680-1
Liu, E., Lv, L., Zhan, Y., Ma, Y., Feng, J., He, Y., et al. (2021). METTL3/N6‐methyladenosine/miR‐21‐5p Promotes Obstructive Renal Fibrosis by Regulating Inflammation through SPRY1/ERK/NF‐κB Pathway Activation. J. Cel Mol Med 25, 7660–7674. doi:10.1111/jcmm.16603
Liu, J., Luo, G., Sun, J., Men, L., Ye, H., He, C., et al. (2019). METTL14 Is Essential for β-cell Survival and Insulin Secretion. Biochim. Biophys. Acta (Bba) - Mol. Basis Dis. 1865 (9), 2138–2148. doi:10.1016/j.bbadis.2019.04.011
Liu, J., Zhang, X., Chen, K., Cheng, Y., Liu, S., Xia, M., et al. (2019). CCR7 Chemokine Receptor-Inducible Lnc-Dpf3 Restrains Dendritic Cell Migration by Inhibiting HIF-1α-Mediated Glycolysis. Immunity 50 (3), 600–615. e15. doi:10.1016/j.immuni.2019.01.021
Liu, R., Wang, S. C., Li, M., Ma, X. H., Jia, X. N., Bu, Y., et al. (2020). An Inhibitor of DRP1 (Mdivi-1) Alleviates LPS-Induced Septic AKI by Inhibiting NLRP3 Inflammasome Activation. Biomed. Res. Int. 2020, 2398420. doi:10.1155/2020/2398420
Liu, T., Zhou, H., Lu, H., Luo, C., Wang, Q., Peng, Y., et al. (2021). MiR-4729 Regulates TIE1 mRNA m6A Modification and Angiogenesis in Hemorrhoids by Targeting METTL14. Ann. Transl Med. 9 (3), 232. doi:10.21037/atm-20-3399
Liu, X., Gonzalez, G., Dai, X., Miao, W., Yuan, J., Huang, M., et al. (2020). Adenylate Kinase 4 Modulates the Resistance of Breast Cancer Cells to Tamoxifen through an m6A-Based Epitranscriptomic Mechanism. Mol. Ther. 28 (12), 2593–2604. doi:10.1016/j.ymthe.2020.09.007
Liu, X., Liu, J., Xiao, W., Zeng, Q., Bo, H., Zhu, Y., et al. (2020). SIRT1 Regulates N 6 ‐Methyladenosine RNA Modification in Hepatocarcinogenesis by Inducing RANBP2‐Dependent FTO SUMOylation. Hepatology 72 (6), 2029–2050. doi:10.1002/hep.31222
Lu, N., Li, X., Yu, J., Li, Y., Wang, C., Zhang, L., et al. (2018). Curcumin Attenuates Lipopolysaccharide-Induced Hepatic Lipid Metabolism Disorder by Modification of M6 A RNA Methylation in Piglets. Lipids 53 (1), 53–63. doi:10.1002/lipd.12023
Lv, J., Zhang, Y., Gao, S., Zhang, C., Chen, Y., Li, W., et al. (2018). Endothelial-specific m6A Modulates Mouse Hematopoietic Stem and Progenitor Cell Development via Notch Signaling. Cell Res 28 (2), 249–252. doi:10.1038/cr.2017.143
Lv, Y., Li, T., Yang, M., Su, L., Zhu, Z., Zhao, S., et al. (2021). Melatonin Attenuates Chromium (VI)-Induced Spermatogonial Stem Cell/Progenitor Mitophagy by Restoration of METTL3-Mediated RNA N6-Methyladenosine Modification. Front. Cel Dev. Biol. 9, 684398. doi:10.3389/fcell.2021.684398
Ma, H., Wang, X., Ha, T., Gao, M., Liu, L., Wang, R., et al. (2016). MicroRNA-125b Prevents Cardiac Dysfunction in Polymicrobial Sepsis by Targeting TRAF6-Mediated Nuclear Factor κB Activation and P53-Mediated Apoptotic Signaling. J. Infect. Dis. 214 (11), 1773–1783. doi:10.1093/infdis/jiw449
Mapperley, C., van de Lagemaat, L. N., Lawson, H., Tavosanis, A., Paris, J., Campos, J., et al. (2021). The mRNA m6A Reader YTHDF2 Suppresses Proinflammatory Pathways and Sustains Hematopoietic Stem Cell Function. J. Exp. Med. 218 (3), 218. doi:10.1084/jem.20200829
Neviere, R., Delguste, F., Durand, A., Inamo, J., Boulanger, E., and Preau, S. (2016). Abnormal Mitochondrial cAMP/PKA Signaling Is Involved in Sepsis-Induced Mitochondrial and Myocardial Dysfunction. Ijms 17 (12), 2075. doi:10.3390/ijms17122075
Olazagoitia-Garmendia, A., Zhang, L., Mera, P., Godbout, J. K., Sebastian-DelaCruz, M., Garcia-Santisteban, I., et al. (2021). Gluten-induced RNA Methylation Changes Regulate Intestinal Inflammation via Allele-specific XPO1 Translation in Epithelial Cells. Gut. doi:10.1136/gutjnl-2020-322566
Ozkurede, U., Kala, R., Johnson, C., Shen, Z., Miller, R. A., and Garcia, G. G. (2019). Cap-independent mRNA Translation Is Upregulated in Long-Lived Endocrine Mutant Mice. J. Mol. Endocrinol. 63 (2), 123–138. doi:10.1530/jme-19-0021
Parial, R., Li, H., Li, J., Archacki, S., Yang, Z., Wang, I. Z., et al. (2021). Role of Epigenetic M 6 A RNA Methylation in Vascular Development: Mettl3 Regulates Vascular Development through PHLPP2/mTOR‐AKT Signaling. FASEB j. 35 (5), e21465. doi:10.1096/fj.202000516RR
Piel, D. A., Gruber, P. J., Weinheimer, C. J., Courtois, M. R., Robertson, C. M., Coopersmith, C. M., et al. (2007). Mitochondrial Resuscitation with Exogenous Cytochrome C in the Septic Heart*. Crit. Care Med. 35 (9), 2120–2127. doi:10.1097/01.ccm.0000278914.85340.fe
Potz, B. A., Sellke, F. W., and Abid, M. R. (2016). Endothelial ROS and Impaired Myocardial Oxygen Consumption in Sepsis-Induced Cardiac Dysfunction. J. Intensive Crit. Care 2 (1). doi:10.21767/2471-8505.100020
Qi, Y., Yao, R., Zhang, W., and Cui, Q. (2021). KAT1 Triggers YTHDF2-Mediated ITGB1 mRNA Instability to Alleviate the Progression of Diabetic Retinopathy. Pharmacol. Res. 170, 105713. doi:10.1016/j.phrs.2021.105713
Qu, J., Cai, Z., Liu, Y., Duan, X., Han, S., Liu, J., et al. (2021). Persistent Bacterial Coinfection of a COVID-19 Patient Caused by a Genetically Adapted Pseudomonas aeruginosa Chronic Colonizer. Front. Cel. Infect. Microbiol. 11, 641920. doi:10.3389/fcimb.2021.641920
Ren, J., Xu, X., Wang, Q., Ren, S. Y., Dong, M., and Zhang, Y. (2016). Permissive Role of AMPK and Autophagy in Adiponectin Deficiency-Accentuated Myocardial Injury and Inflammation in Endotoxemia. J. Mol. Cell Cardiol. 93, 18–31. doi:10.1016/j.yjmcc.2016.02.002
Romero-Bermejo, F. J., Ruiz-Bailen, M., Gil-Cebrian, J., and J. Huertos-Ranchal, M. (2011). Sepsis-induced Cardiomyopathy. Ccr 7 (3), 163–183. doi:10.2174/157340311798220494
Rong, Z.-X., Li, Z., He, J.-J., Liu, L.-Y., Ren, X.-X., Gao, J., et al. (2019). Downregulation of Fat Mass and Obesity Associated (FTO) Promotes the Progression of Intrahepatic Cholangiocarcinoma. Front. Oncol. 9, 369. doi:10.3389/fonc.2019.00369
Sang, W., Xue, S., Jiang, Y., Lu, H., Zhu, L., Wang, C., et al. (2021). METTL3 Involves the Progression of Osteoarthritis Probably by Affecting ECM Degradation and Regulating the Inflammatory Response. Life Sci. 278, 119528. doi:10.1016/j.lfs.2021.119528
Shen, Z., Liu, P., Sun, Q., Li, Y., Acharya, R., Li, X., et al. (2021). FTO Inhibits UPRmt-Induced Apoptosis by Activating JAK2/STAT3 Pathway and Reducing m6A Level in Adipocytes. Apoptosis 26, 474–487. doi:10.1007/s10495-021-01683-z
Shi, W., Yang, F., Dai, R., Sun, Y., Chu, Y., Liao, S., et al. (2021). METTL3-Mediated N6-Methyladenosine Modification Is Involved in the Dysregulation of NRIP1 Expression in Down Syndrome. Front. Cel Dev. Biol. 9, 621374. doi:10.3389/fcell.2021.621374
Shi, Y., Zheng, X., Zheng, M., Wang, L., Chen, Y., and Shen, Y. (2021). Identification of Mitochondrial Function‐associated lncRNAs in Septic Mice Myocardium. J. Cel Biochem 122 (1), 53–68. doi:10.1002/jcb.29831
Singer, M., Deutschman, C. S., Seymour, C. W., Shankar-Hari, M., Annane, D., Bauer, M., et al. (2016). The Third International Consensus Definitions for Sepsis and Septic Shock (Sepsis-3). Jama 315 (8), 801–810. doi:10.1001/jama.2016.0287
Sun, X., Dai, Y., Tan, G., Liu, Y., and Li, N. (2020). Integration Analysis of m6A-SNPs and eQTLs Associated with Sepsis Reveals Platelet Degranulation and Staphylococcus aureus Infection Are Mediated by m6A mRNA Methylation. Front. Genet. 11, 7. doi:10.3389/fgene.2020.00007
Tian, C., Huang, Y., Li, Q., Feng, Z., and Xu, Q. (2019). Mettl3 Regulates Osteogenic Differentiation and Alternative Splicing of Vegfa in Bone Marrow Mesenchymal Stem Cells. Ijms 20 (3), 551. doi:10.3390/ijms20030551
Tong, J., Cao, G., Zhang, T., Sefik, E., Amezcua Vesely, M. C., Broughton, J. P., et al. (2018). m6A mRNA Methylation Sustains Treg Suppressive functionsA mRNA Methylation Sustains Treg Suppressive Functions. Cel Res 28 (2), 253–256. doi:10.1038/cr.2018.7
Tong, J., Flavell, R. A., and Li, H.-B. (2018). RNA m6A Modification and its Function in Diseases. Front. Med. 12 (4), 481–489. doi:10.1007/s11684-018-0654-8
Tong, J., Wang, X., Liu, Y., Ren, X., Wang, A., Chen, Z., et al. (2021). Pooled CRISPR Screening Identifies M 6 A as a Positive Regulator of Macrophage Activation. Sci. Adv. 7 (18). doi:10.1126/sciadv.abd4742
Tu, F., Wang, X., Zhang, X., Ha, T., Wang, Y., Fan, M., et al. (2020). Novel Role of Endothelial Derived Exosomal HSPA12B in Regulating Macrophage Inflammatory Responses in Polymicrobial Sepsis. Front. Immunol. 11, 825. doi:10.3389/fimmu.2020.00825
Wang, G., Dai, Y., Li, K., Cheng, M., Xiong, G., Wang, X., et al. (2021). Deficiency of Mettl3 in Bladder Cancer Stem Cells Inhibits Bladder Cancer Progression and Angiogenesis. Front. Cel Dev. Biol. 9, 627706. doi:10.3389/fcell.2021.627706
Wang, H., Deng, Q., Lv, Z., Ling, Y., Hou, X., Chen, Z., et al. (2019). N6-methyladenosine Induced miR-143-3p Promotes the Brain Metastasis of Lung Cancer via Regulation of VASH1. Mol. Cancer 18 (1), 181. doi:10.1186/s12943-019-1108-x
Wang, J., Yan, S., Lu, H., Wang, S., and Xu, D. (2019). METTL3 Attenuates LPS-Induced Inflammatory Response in Macrophages via NF-Κb Signaling Pathway. Mediators Inflamm. 2019, 1–8. doi:10.1155/2019/3120391
Wang, L.-j., Xue, Y., Huo, R., Yan, Z., Xu, H., Li, H., et al. (2020). N6-methyladenosine Methyltransferase METTL3 Affects the Phenotype of Cerebral Arteriovenous Malformation via Modulating Notch Signaling Pathway. J. Biomed. Sci. 27 (1), 62. doi:10.1186/s12929-020-00655-w
Wang, L. j., Xue, Y., Li, H., Huo, R., Yan, Z., Wang, J., et al. (2020). Wilms' Tumour 1‐associating Protein Inhibits Endothelial Cell Angiogenesis by m6A‐dependent Epigenetic Silencing of Desmoplakin in Brain Arteriovenous Malformation. J. Cel Mol Med 24 (9), 4981–4991. doi:10.1111/jcmm.15101
Wang, X., Huang, N., Yang, M., Wei, D., Tai, H., Han, X., et al. (2017). FTO Is Required for Myogenesis by Positively Regulating mTOR-PGC-1α Pathway-Mediated Mitochondria Biogenesis. Cel Death Dis 8 (3)–e2702. doi:10.1038/cddis.2017.122
Wang, X., Ji, Y., Feng, P., Liu, R., Li, G., Zheng, J., et al. (2021). The m6A Reader IGF2BP2 Regulates Macrophage Phenotypic Activation and Inflammatory Diseases by Stabilizing TSC1 and PPAR γ. Adv. Sci. 8 (13), 2100209. doi:10.1002/advs.202100209
Wang, Y., Jasper, H., Toan, S., Muid, D., Chang, X., and Zhou, H. (2021). Mitophagy Coordinates the Mitochondrial Unfolded Protein Response to Attenuate Inflammation-Mediated Myocardial Injury. Redox Biol. 45, 102049. doi:10.1016/j.redox.2021.102049
Wei, W., Huo, B., and Shi, X. (2019). miR-600 Inhibits Lung Cancer via Downregulating the Expression of METTL3. Cmar Vol. 11, 1177–1187. doi:10.2147/cmar.s181058
Wen, L., Sun, W., Xia, D., Wang, Y., Li, J., and Yang, S. (2020). The m6A Methyltransferase METTL3 Promotes LPS-Induced Microglia Inflammation through TRAF6/NF-Κb Pathway. Neuroreport. doi:10.1097/wnr.0000000000001550
Wu, C., Chen, W., He, J., Jin, S., Liu, Y., Yi, Y., et al. (2020). Interplay of M 6 A and H3K27 Trimethylation Restrains Inflammation during Bacterial Infection. Sci. Adv. 6 (34), eaba0647. doi:10.1126/sciadv.aba0647
Wu, L., Pei, Y., Zhu, Y., Jiang, M., Wang, C., Cui, W., et al. (2019). Association of N6-Methyladenine DNA with Plaque Progression in Atherosclerosis via Myocardial Infarction-Associated Transcripts. Cel Death Dis 10 (12), 909. doi:10.1038/s41419-019-2152-6
Xing, Y., Cheng, D., Shi, C., and Shen, Z. (2021). The Protective Role of YTHDF1-Knock Down Macrophages on the Immune Paralysis of Severe Sepsis Rats with ECMO. Microvasc. Res. 137, 104178. doi:10.1016/j.mvr.2021.104178
Yang, Z., Wang, T., Wu, D., Min, Z., Tan, J., and Yu, B. (2020). RNA N6-Methyladenosine Reader IGF2BP3 Regulates Cell Cycle and Angiogenesis in colon Cancer. J. Exp. Clin. Cancer Res. 39 (1), 203. doi:10.1186/s13046-020-01714-8
Yao, M.-D., Jiang, Q., Ma, Y., Liu, C., Zhu, C.-Y., Sun, Y.-N., et al. (2020). Role of METTL3-dependent N6-Methyladenosine mRNA Modification in the Promotion of Angiogenesis. Mol. Ther. 28 (10), 2191–2202. doi:10.1016/j.ymthe.2020.07.022
Ye, G., Wang, M., Liu, D., Cheng, L., Yin, X., Zhang, Q., et al. (2020). Mechanism of Naringenin Blocking the Protection of LTB4/BLT1 Receptor against Septic Cardiac Dysfunction. Ann. Clin. Lab. Sci. 50 (6), 769–774.
Yu, J.-T., Hu, X.-W., Chen, H.-Y., Yang, Q., Li, H.-D., Dong, Y.-H., et al. (2021). DNA Methylation of FTO Promotes Renal Inflammation by Enhancing m6A of PPAR-α in Alcohol-Induced Kidney Injury. Pharmacol. Res. 163, 105286. doi:10.1016/j.phrs.2020.105286
Yu, R., Li, Q., Feng, Z., Cai, L., and Xu, Q. (2019). m6A Reader YTHDF2 Regulates LPS-Induced Inflammatory Response. Ijms 20 (6), 1323. doi:10.3390/ijms20061323
Zhang, B. Y., Han, L., Tang, Y. F., Zhang, G. X., Fan, X. L., Zhang, J. J., et al. (2020). METTL14 Regulates M6A Methylation-Modified Primary miR-19a to Promote Cardiovascular Endothelial Cell Proliferation and Invasion. Eur. Rev. Med. Pharmacol. Sci. 24 (12), 7015–7023. doi:10.26355/eurrev_202006_21694
Zhang, C., Chen, Y., Sun, B., Wang, L., Yang, Y., Ma, D., et al. (2017). m6A Modulates Haematopoietic Stem and Progenitor Cell Specification. Nature 549 (7671), 273–276. doi:10.1038/nature23883
Zhang, C., Wang, Y., Peng, Y., Xu, H., and Zhou, X. (2020). METTL3 Regulates Inflammatory Pain by Modulating M 6 A‐dependent pri‐miR‐365‐3p Processing. FASEB j. 34 (1), 122–132. doi:10.1096/fj.201901555R
Zhang, S., Liu, F., Wu, Z., Xie, J., Yang, Y., and Qiu, H. (2020). Contribution of m6A Subtype Classification on Heterogeneity of Sepsis. Ann. Transl Med. 8 (6), 306. doi:10.21037/atm.2020.03.07
Zhang, Y., Gu, X., Li, D., Cai, L., and Xu, Q. (2019). METTL3 Regulates Osteoblast Differentiation and Inflammatory Response via Smad Signaling and MAPK Signaling. Ijms 21 (1), 199. doi:10.3390/ijms21010199
Zhao, J., Han, D.-X., Wang, C.-B., and Wang, X.-L. (2020). Zbtb7b Suppresses Aseptic Inflammation by Regulating m6A Modification of IL6 mRNA. Biochem. biophysical Res. Commun. 530 (1), 336–341. doi:10.1016/j.bbrc.2020.07.011
Zhao, Y., Hu, J., Sun, X., Yang, K., Yang, L., Kong, L., et al. (2021). Loss of m6A Demethylase ALKBH5 Promotes post-ischemic Angiogenesis via post-transcriptional Stabilization of WNT5A. Clin. translational Med. 11 (5), e402. doi:10.1002/ctm2.402
Zheng, L., Tang, X., Lu, M., Sun, S., Xie, S., Cai, J., et al. (2020). microRNA-421-3p Prevents Inflammatory Response in Cerebral Ischemia/reperfusion Injury through Targeting m6A Reader YTHDF1 to Inhibit P65 mRNA Translation. Int. immunopharmacology 88, 106937. doi:10.1016/j.intimp.2020.106937
Zhou, J., Zhang, X., Hu, J., Qu, R., Yu, Z., Xu, H., et al. (2021). m 6 A Demethylase ALKBH5 Controls CD4 + T Cell Pathogenicity and Promotes Autoimmunity. Sci. Adv. 7 (25), eabg0470. doi:10.1126/sciadv.abg0470
Zhu, R., Tian, D., Zhao, Y., Zhang, C., and Liu, X. (2021). Genome-Wide Detection of m6A-Associated Genetic Polymorphisms Associated with Ischemic Stroke. J. Mol. Neurosci. 71, 2107–2115. doi:10.1007/s12031-021-01805-x
Zhu, S., and Lu, Y. (2020). Dexmedetomidine Suppressed the Biological Behavior of HK-2 Cells Treated with LPS by Down-Regulating ALKBH5. Inflammation 43 (6), 2256–2263. doi:10.1007/s10753-020-01293-y
Zhuang, C., Zhuang, C., Luo, X., Huang, X., Yao, L., Li, J., et al. (2019). N6-methyladenosine Demethylase FTO Suppresses clear Cell Renal Cell Carcinoma through a Novel FTO-PGC-1α Signalling axis. J. Cel Mol Med 23 (3), 2163–2173. doi:10.1111/jcmm.14128
Zong, X., Wang, H., Xiao, X., Zhang, Y., Hu, Y., Wang, F., et al. (2021). Enterotoxigenic Escherichia coli Infection Promotes Enteric Defensin Expression via FOXO6-METTL3-m6A-Gpr161 Signalling axis. RNA Biol. 18 (4), 576–586. doi:10.1080/15476286.2020.1820193
Zong, X., Xiao, X., Shen, B., Jiang, Q., Wang, H., Lu, Z., et al. (2021). The N 6-methyladenosine RNA-Binding Protein YTHDF1 Modulates the Translation of TRAF6 to Mediate the Intestinal Immune Response. Nucleic Acids Res. 49 (10), 5537–5552. doi:10.1093/nar/gkab343
Zong, X., Zhao, J., Wang, H., Lu, Z., Wang, F., Du, H., et al. (2019). Mettl3 Deficiency Sustains Long-Chain Fatty Acid Absorption through Suppressing Traf6-dependent Inflammation Response. J.I. 202 (2), 567–578. doi:10.4049/jimmunol.1801151
Zou, L., Feng, Y., Zhang, M., Li, Y., and Chao, W. (2011). Nonhematopoietic Toll-like Receptor 2 Contributes to Neutrophil and Cardiac Function Impairment during Polymicrobial Sepsis. Shock 36 (4), 370–380. doi:10.1097/SHK.0b013e3182279868
Keywords: m6A modification, sepsis, myocardial injuries, mitochondrial damages, inflammation response
Citation: Wang Y, Xu M, Yue P, Zhang D, Tong J and Li Y (2021) Novel Insights Into the Potential Mechanisms of N6-Methyladenosine RNA Modification on Sepsis-Induced Cardiovascular Dysfunction: An Update Summary on Direct and Indirect Evidences. Front. Cell Dev. Biol. 9:772921. doi: 10.3389/fcell.2021.772921
Received: 09 September 2021; Accepted: 18 October 2021;
Published: 12 November 2021.
Edited by:
Shafiul Alam, Louisiana State University Health Shreveport, United StatesReviewed by:
Mohammad Abdul Hai Siddique, National Institutes of Health (NIH), United StatesCopyright © 2021 Wang, Xu, Yue, Zhang, Tong and Li. This is an open-access article distributed under the terms of the Creative Commons Attribution License (CC BY). The use, distribution or reproduction in other forums is permitted, provided the original author(s) and the copyright owner(s) are credited and that the original publication in this journal is cited, in accordance with accepted academic practice. No use, distribution or reproduction is permitted which does not comply with these terms.
*Correspondence: Yifei Li, bGl5Zndjc2hAc2N1LmVkdS5jbg==; Jiyu Tong, aml5dS50b25nQHNjdS5lZHUuY24=; Donghui Zhang, ZG9uZ2guemhhbmdAaHVidS5lZHUuY24=
†These authors have contributed equally to this work
Disclaimer: All claims expressed in this article are solely those of the authors and do not necessarily represent those of their affiliated organizations, or those of the publisher, the editors and the reviewers. Any product that may be evaluated in this article or claim that may be made by its manufacturer is not guaranteed or endorsed by the publisher.
Research integrity at Frontiers
Learn more about the work of our research integrity team to safeguard the quality of each article we publish.