- 1Department of Surgery/Otolaryngology, University of California, San Diego, San Diego, CA, United States
- 2Department of Otolaryngology, St. Elisabeth-Hospital, Ruhr University Bochum, Bochum, Germany
- 3Department of Biology, University of California, San Diego, San Diego, CA, United States
- 4Department of Otolaryngology, University Medical Center Schleswig-Holstein, Lübeck, Germany
- 5Department of Neurosciences, University of California, San Diego, San Diego, CA, United States
- 6VA San Diego Healthcare System, San Diego, CA, United States
Introduction: Autophagy is a degradative pathway to safely break down and recycle dysfunctional cellular components. There is prior evidence of autophagy participation during hair cell (HC) damage. Our goal was to screen compounds targeting different aspects of autophagy for their effects on HC loss due to an ototoxic aminoglycoside, gentamicin (GM).
Methods: The SELLECKChem autophagy compound library, consisting of 154 compounds with defined autophagy inducing or inhibitory activity, was used for targeted screening in vitro model of ototoxicity. Organ of Corti from postnatal days 3–5 pou4f3/GFP transgenic mice (HCs express green fluorescent protein) were utilized. The organs were micro-dissected, and basal and middle turns divided into micro-explants individually placed into the single wells of a 96-well plate. Samples were treated with 200 μM of GM plus three dosages of tested compound and cultured for 72 h. Negative controls were treated with media only; positive ototoxicity controls were treated with GM only.
Results: The majority of the library compounds had no effect on GM-induced HC loss. However, 18 compounds exhibited a significant, protective effect, two compounds were protective at low dosage but showed enhanced GM toxicity at higher doses and one compound was toxic to HCs in the absence of GM.
Conclusions: This study evaluated many autophagy compounds that have not been tested previously on HCs. The disparate results obtained underscore the complexity of autophagy events that can influence HC responses to aminoglycosides, but also implicate the proteosome as an important damage mechanism. The screening results can serve as basis for further studies with protective compounds as potential drug targets.
Introduction
Ototoxicity is a significant side effect of some valuable medications that are used to treat life-threatening diseases. Ototoxic drugs can cause irreversible damage to the inner ear, leading to loss of hearing and balance function. A major class of ototoxic drugs are the aminoglycoside antibiotics. Aminoglycoside-induced ototoxicity occurs in as many as 50% of patients with multi-drug resistance tuberculosis and in up to 20% of children treated for cystic fibrosis (Al-Malky et al., 2015; Sagwa et al., 2015).
The aminoglycoside antibiotic, gentamicin (GM) was discovered in 1963 as an extract of Micromonospora purpurea. It is recommended as an effective, safe and essential medicine (Abou-Zeid et al., 1978; Obregon et al., 1994), widely used to treat endocarditis, meningitis, pelvic inflammatory disease, urinary tract infections, bone infections, pneumonia and other bacterial infections, including sepsis (Hamilton, 2001). Although GM is an extremely effective antimicrobial, ototoxicity is its major side effect (Kim et al., 2017; Sapizhak et al., 2017). The sensory hair cells (HCs) of the inner ear are major targets of GM toxicity. As terminally differentiated cells, HCs are unable to regenerate. Loss of HCs due to ototoxicity causes permanently reduced hearing sensitivity, with complete deafness in severe cases (Xing et al., 2017).
Oxidative stress and apoptosis are major drivers of GM induced ototoxicity, which implies that autophagy is also involved (He et al., 2018). Autophagy is an intracellular, lysosomal-mediated process in which dysfunctional cell material is broken down and recycled (Mizushima, 2011; He et al., 2018). This results in a dynamic relationship between autophagy, oxidative stress, damage to organelles and proteins, and apoptosis (Lee et al., 2012; Benkafadar et al., 2019). Autophagy is an important component of apoptosis, packaging damaged cellular constituents prior to cell dissolution. Autophagy can also play a role in the survival of cells in stress conditions, such as starvation and oxidative stress, by preventing the intracellular and extracellular spread of damage (Mizushima et al., 2008; Esclatine et al., 2009; Rabinowitz and White, 2010). Autophagy participates in regulating other physiological processes in non-stress situations, such as cell proliferation and differentiation, where cellular constituents must be destroyed or recycled (Esclatine et al., 2009; Oh et al., 2012; Magarinos et al., 2017). However, when autophagy is over-enhanced, cellular components essential for cell survival can be degraded, leading to cell death (Chen et al., 2010). Given its multifunctional nature, it is not surprising that autophagy dysfunction has been suggested to be involved in several pathological changes, such as cancer, inflammation, neurodegenerative disease, and metabolic disorders (Levine and Kroemer, 2008; Arroyo et al., 2014; Ryter et al., 2014).
Autophagy (Figure 1) is initiated by the formation of double membrane cytosolic vesicles, called the phagophore or isolation membrane, that contains damaged cellular constituents (Mizushima and Komatsu, 2011). The phagophore matures into a closed double-membrane-bound structure and then fuses with lysosomes, called the autophagosome, for disposal of its contents (Ohsumi, 2001). Autophagy involves the coordinated, and sequential activation of specific sets of autophagy-related genes (Ohsumi, 2001).
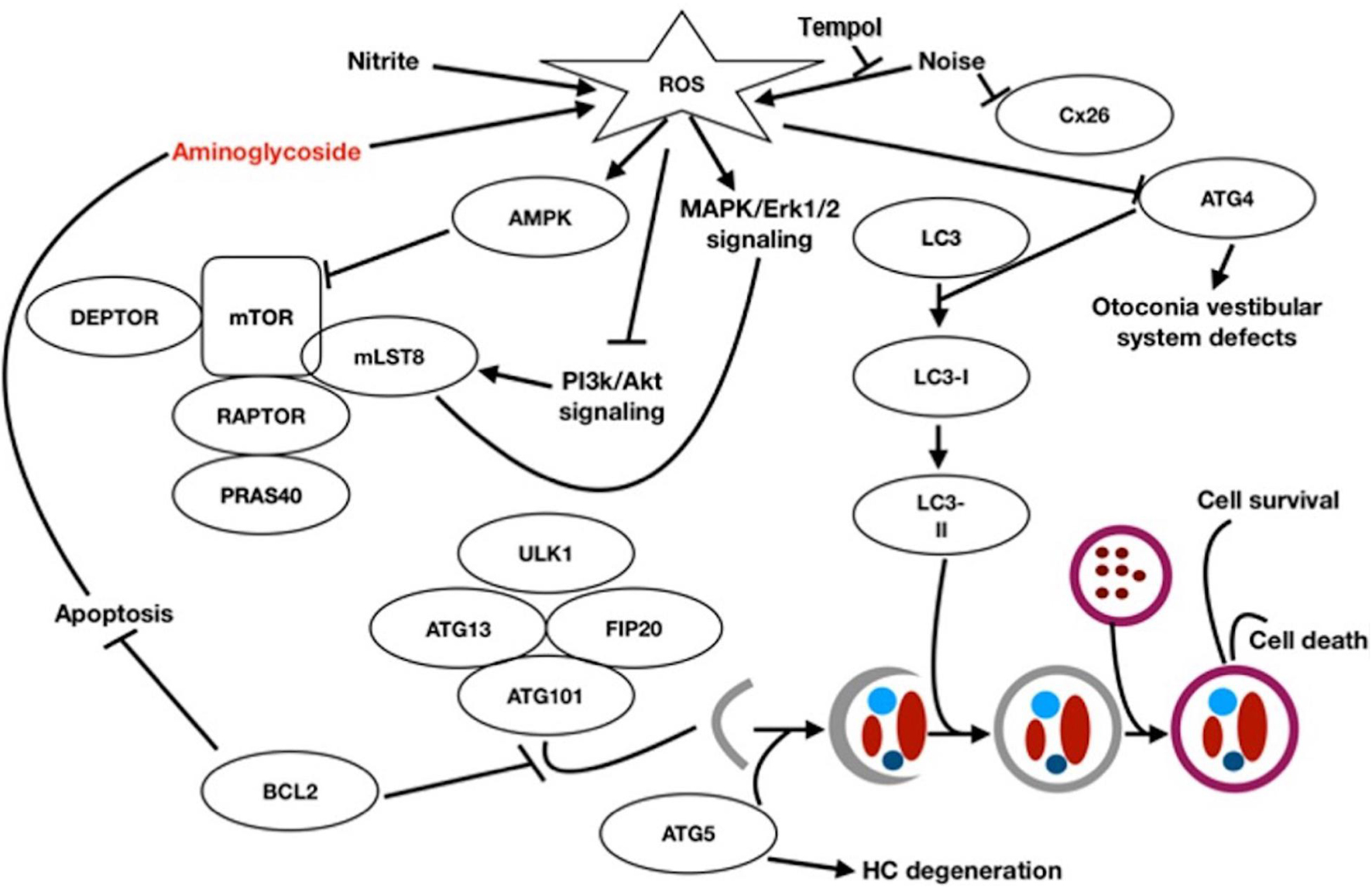
Figure 1. Autophagy signal pathway and relationship with the auditory system [Figure modified after He et al. (2018)] Macroautophagy: A part of the endoplasmic reticulum encloses the structures to be degraded and isolates them with a double membrane (autophagosome). The autophagosome binds to a lysosome under hydrofusion. This results in the degradation of the autophagosomal components.
In the inner ear, autophagy has been shown to be an essential catabolic mechanism which is necessary for normal embryonic development (Taylor and Kirkegaard, 2008). The mechanism also responds to otic injury in the adult mouse, for autophagic recycling of intracellular components and elimination of deleterious molecules and organelles (Taylor and Kirkegaard, 2008). Inhibition of the autophagic pathway has been shown to provoke HC degeneration, the damage of neurogenesis and the aberrant axonal outgrowth, leading to hearing loss (Aburto et al., 2012). Conversely, activation of autophagy in HCs and oC-derived cell lines reduces ROS levels and also promotes cell survival (He et al., 2018). With respect to aminoglycoside ototoxicity, a relatively small number of autophagy studies have been performed. However, inhibition of autophagy has been shown to enhance Neomycin damage to HCs (He et al., 2018), and impaired autophagy has been proposed as a mechanism of delayed HC death in GM ototoxicity (Kim et al., 2017).
Given the complexity of the autophagy process and its diverse roles in cellular function and damage, the objective of this study was to screen compounds targeting different aspects of autophagy for their effects on HC loss due to GM. The goal was to enhance understanding of the role of autophagy in ototoxicity, and to identify and compare potentially protective compounds.
Materials and Methods
Animals
Transgenic neonatal mice (3–5 days) in which eGFP was selectively expressed in HCs under the control of a pou4f3 promoter construct (Masuda et al., 2011) were used. All experiments were performed to National Institutes of Health guidelines and approved by the Institutional Animal Care and Use Committee of the VA San Diego Medical Center.
Microdissection
The organ of Corti (oC) was dissected from the cochlea of 3–5 postnatal day pou4f3/eGFP mouse neonates. The apical region of each epithelium, which is relatively insensitive to aminoglycoside toxicity, was discarded. The basal and middle regions of the epithelium were divided using a diamond scalpel into micro-explants consisting of approximately 20 inner HCs and the 60 associated outer HCs. Micro-explants were individually plated in each well of a flat-bottom 96 well plate in media consisting of DMEM F-12(Gibco) plus 30 U/ml Penicillin and 5% FBS, and maintained in a humidified tissue culture incubator (37°C, 5% CO2). Methods workflow is depicted in Figure 2. Mature hair cells at later times are more difficult to micro-dissect and also do not survive well in culture.
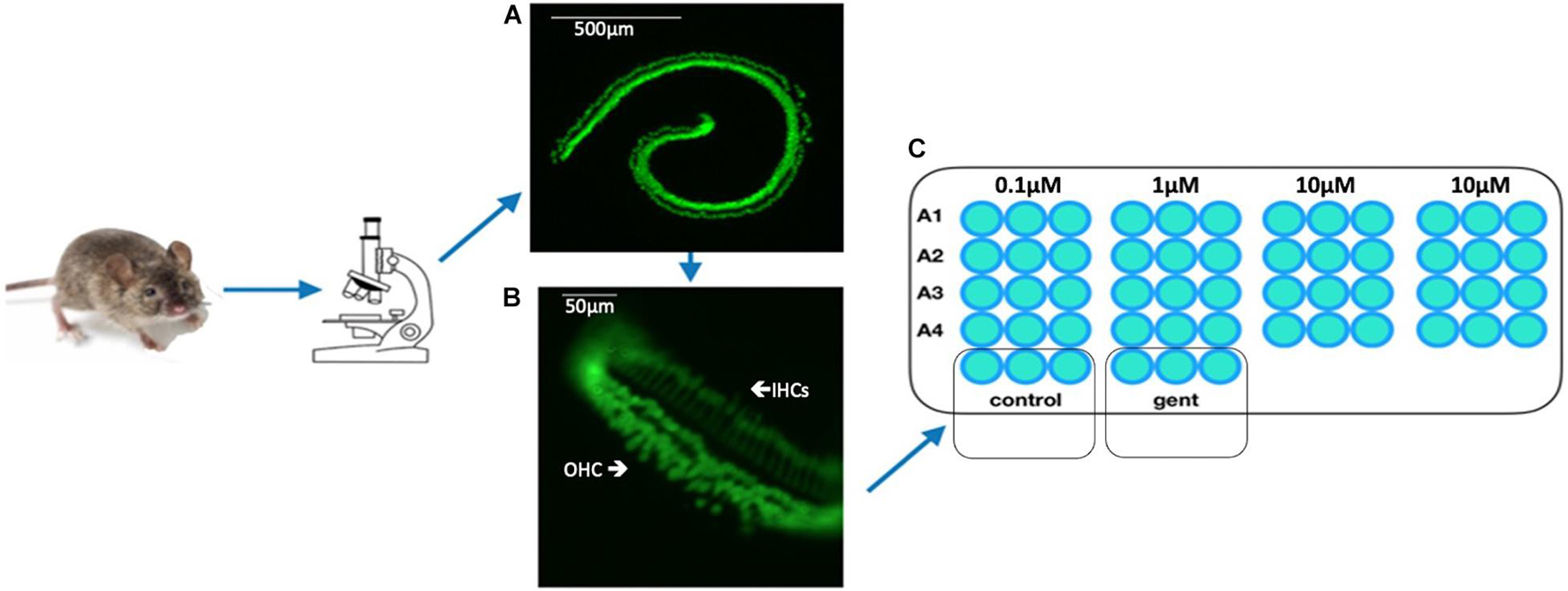
Figure 2. Schematic of workflow from the organ of Corti (A) of the pou4f3/GFP transgenic mouse to microexplants (B) into the 96-well plate which contained nutrient medium, autophagy compound (A1–A4) and GM (C). The microexplant (B) at the beginning of the treatment. The typical structure of one row of IHCs and 3 rows of OHCs is visible.
Screening
Targeted screening was performed using the SelleckChem Autophagy Compound Library (Z167594, Selleck Chem, Houston, TX, United States), consisting of 154 autophagy-related compounds targeting the: PI3K/Akt/mTOR (15%), DNA damage and sirtuin (15%), cell cycle (15%), proteasome and ubiquitin (10%), transmembrane transporters (10%), apoptosis (5%), cytoskeletal signaling (5%), GPCR and G-protein (5%) among other (20%) pathways. The library represents a variety of classes of compounds, including a number of autophagy inducing and autophagy inhibiting compounds which at present had not previously been applied to HC damage. Test compounds were initially dissolved in DMSO and diluted in culture media with the total amount of DMSO adjusted to a final concentration of 0.1%. Each experimental oC micro-explant was pretreated for 24 h with one of the library compounds at concentration of 0.1, 1, or 10 μM, performed in triplicate wells. On the following day media containing 200 μM gentamicin as well as the test autophagy compound with the appropriate concentration was added, and the micro-explants were cultured for 72 h. Untreated (negative) controls were maintained in media alone and positive controls were treated with 200 μM GM alone (Figure 3). Compound controls were treated with the highest concentration of the compound (10 μM) alone. Media for all control groups contained 0.1% DMSO, to match the experimental groups. Compounds were screened in duplicated 96-well plates with 5–7 autophagy compounds per plate, plus controls. Green fluorescent protein-positive HCs were imaged by fluorescence microcopy on each day of treatment, and survival curves were generated for each compound and condition. HC counts, including both inner and outer HCs, were evaluated in ImageJ with the Macro “ITCN” or “Cell Counter,” and normalized as percentages to the number of HCs present on D1, prior to the start of GM treatment. Any micro-explants that did not attach and flatten in the well by D1 were excluded, because HC counts could not be accurately quantified at that time. There were sufficient wells on each plate that three micro-explants per condition could almost always be accommodated even with some unattached samples. Autophagy compounds that showed a significant protective effect, as compared to the control, in the initial screen were repeated for a second round at the three different concentrations. A compound was scored as “protective” if there was an overall significance for six wells of any concentration. A dose-dependent protection was attributed if there was a statistical significance at all three concentrations (18 wells).
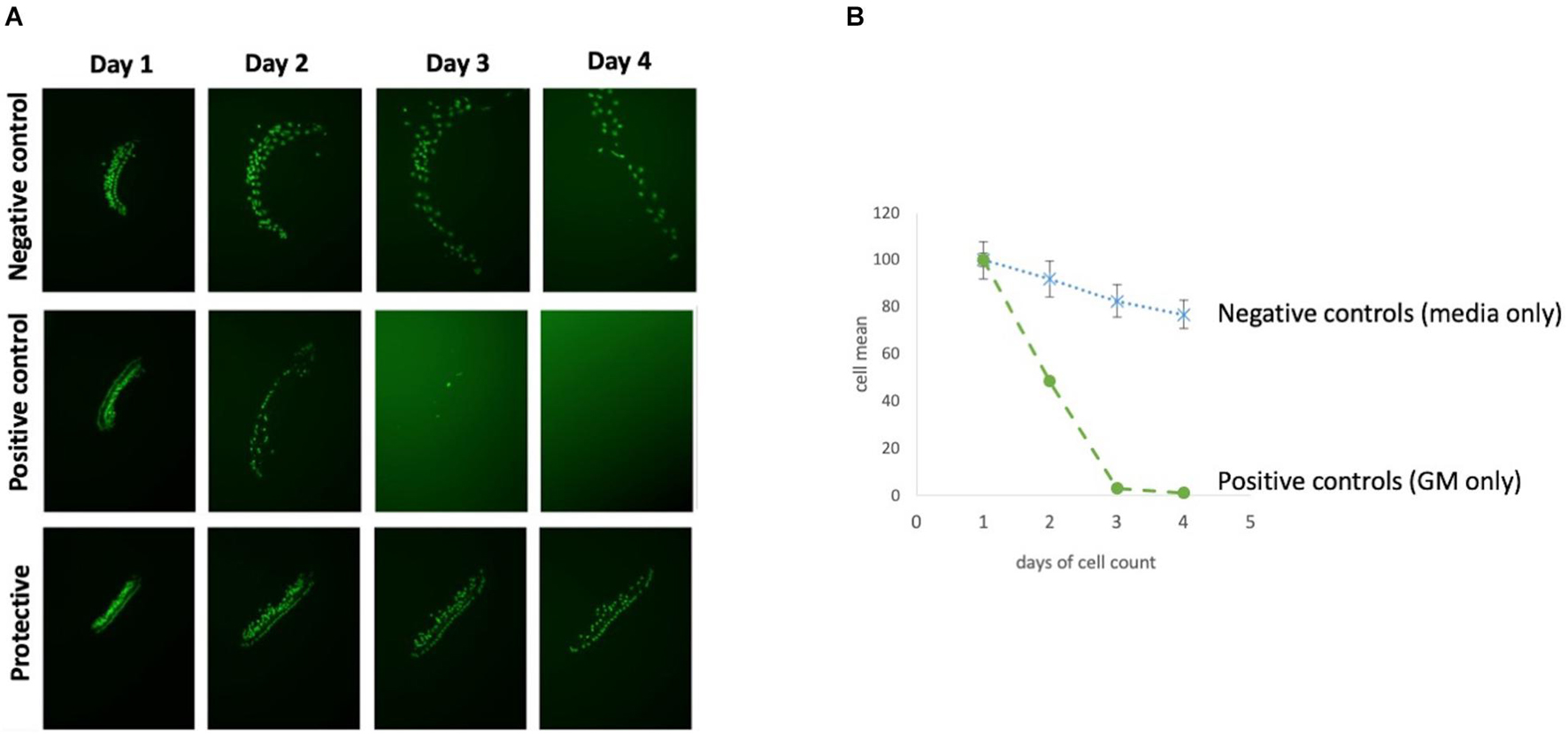
Figure 3. Overview of HC decrease over 72 h. (A) Micro-explant pictures showing the fluorescent HCs over the experimental time course and (B) Illustration survival curves of positive and negative control of an autophagy compound.
Statistical analysis was performed using GraphPad Prism6, StatView5, using the Kruskal–Wallis non-parametric ANOVA to detect treatment effects. Individual condition comparisons were performed using the Mann–Whitney U test, with correction for multiple comparisons. For purposes of the figures, standard deviations were calculated from the non-normalized HC counts. Validation Redox compound “hits” were identified in the initial round of screening as deviating significantly from the controls. Following this initial screen, repeat plates were prepared in an identical manner for all hits, for a total N of six micro-explants. Statistical analysis was then repeated. Compounds that demonstrated a significant effect when the following round was included were considered to be confirmed.
Results
Control Micro-Explants and Gentamicin Cytotoxicity
Each 96 well plate had its own negative and positive control. HC counts from negative and positive control micro-explants were converted to percent survival relative to Day 1 (D1, just prior to GM exposure), and averaged across all plates. Imaging of GFP-positive HCs in control wells typically showed HC survival similar to that illustrated in Figure 3. Untreated (negative control) micro-explants maintained only in culture media showed near-complete HC survival from D1-D3 ranging from 94% on D2 to 91% on D3. On Day 4, HC survival was reduced to a range of 80–68%. In contrast, HCs treated with 200 μM GM (positive control) showed significant losses by D2 with an average HC survival of 52%, D3 HC survival was 13%, while D4 showed continued losses to 8% survival. As expected, Kruskal–Wallis and Mann–Whitney analysis showed a significant difference between negative and positive controls from D2–D4. No differences were observed between explants from the basal or middle regions of the cochlea, consistent with the high dosage of GM. Nevertheless, there were plates in which some variation occurred in the positive and/or negative controls. Therefore the results for each compound were compared statistically to positive controls from the same plate, generated with the same batches of media and GM. Additionally, each compound that was statistically different from the control was repeated for validation.
Library Results
The effects of the 154 compounds could be divided into three groups, autophagy compounds which exhibited:
I. No effect.
II. Protective effect.
III. Toxic effect.
Group I: No Effect
The majority of the 154 autophagy- inducing and/or -inhibiting compounds had no significant effect on HCs after GM treatment. An example is illustrated in Figure 4. When the highest concentration (10 μM) of autophagy compound was added to the micro-explant HC numbers were similar to that seen for negative control micro-explants cultures in media alone. Adding three different concentrations of autophagy compound plus 200 μM GM produced HC survival rates comparable to those observed with GM alone.
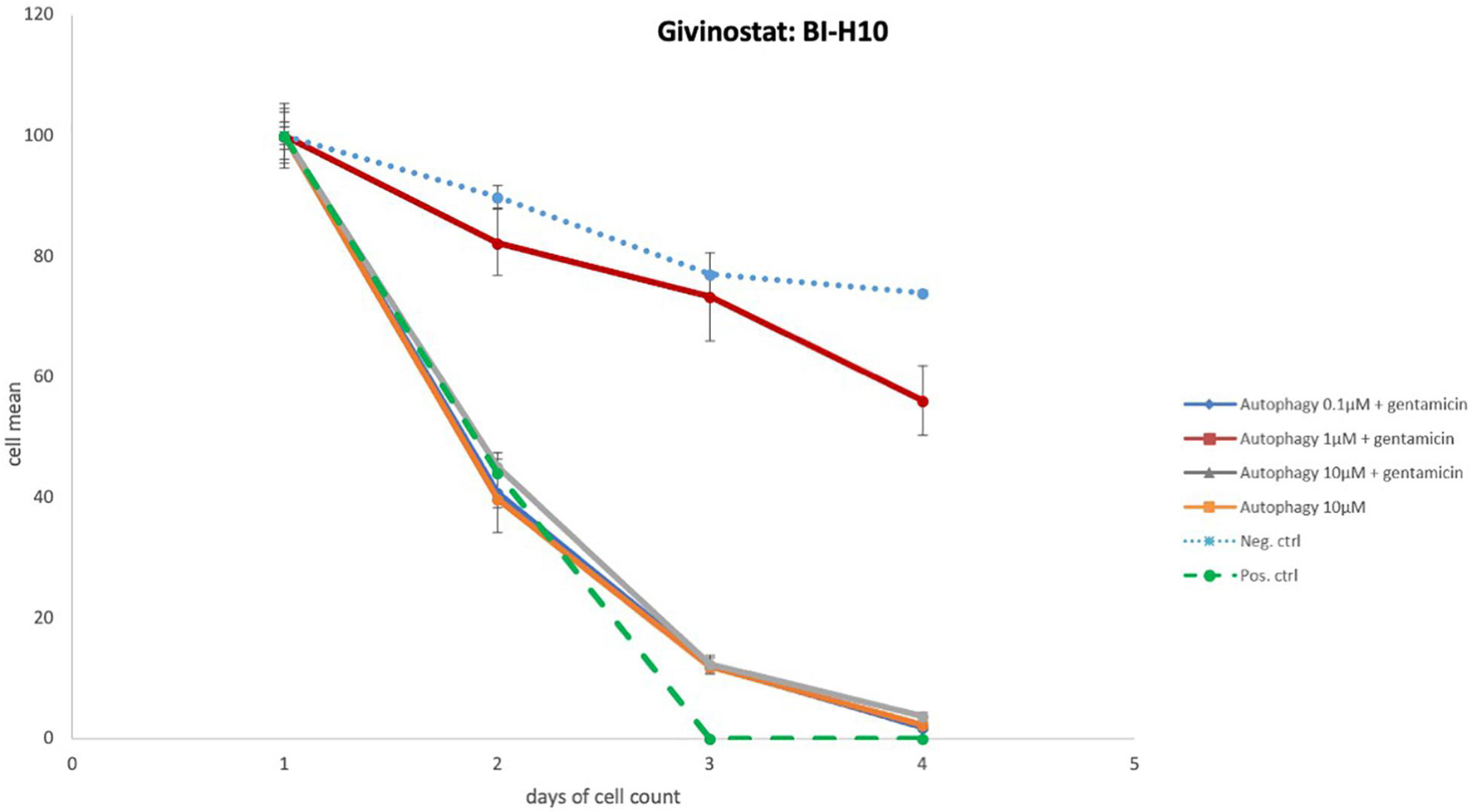
Figure 4. Autophagy compound without protective effects. Illustration of Survival curves of representative compound that had no effect on GM-treated oC explants. Treatment with the highest concentration (10 μM) of autophagy compound resulted in HC numbers similar to that seen for negative control micro-explants cultures in media alone. Treatment with compound plus GM was not significantly different from gentamicin alone.
Group II: Protective
Eighteen autophagy compounds showed statistically significant protection of HCs in micro-explants treated with both the compound and gentamicin (Table 1). Within the group of protective compounds the observed protective effect could be divided into strong, medium and slight protection (Table 2). Strong protective compounds exhibited significant HC survival on all 3 days, varying in concentration (Figure 5A). Medium protective compounds showed improved HC survival on 2 days with one or two concentrations (Figure 5B), while slightly/mildly protective compounds reduced HC loss on D1 and only for 10 μM concentration (Figure 5C). There were two test substances that exhibited a bimodal trait i.e., significant protection at low concentrations, but enhancing GM damage at the highest concentration. Bortezomib and Brefeldin A exhibited significant protective traits at 0.1 μM, Brefeldin A also with 1 μM on Day 2, they both demonstrated significantly enhanced early HC loss due to gentamicin at 10 μM (Figure 6). We observed this “push-pull” effect with tyrosine kinase inhibitors in previous studies (Ryals et al., 2017).
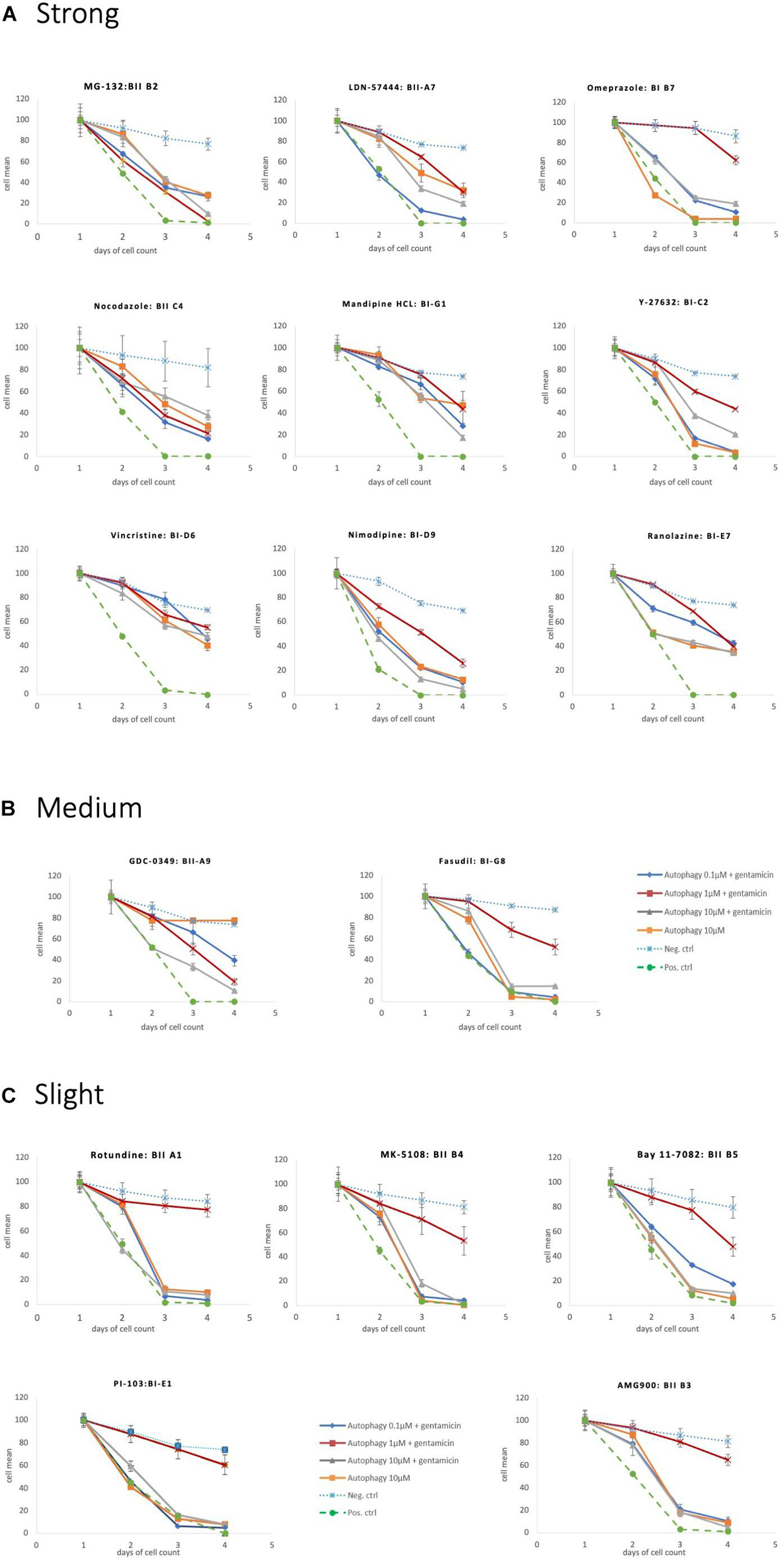
Figure 5. Protective autophagy compounds Survival plots showing the autophagy compounds classified as (A) Strong, (B) medium and (C) slight depending on the HCs survival curves.
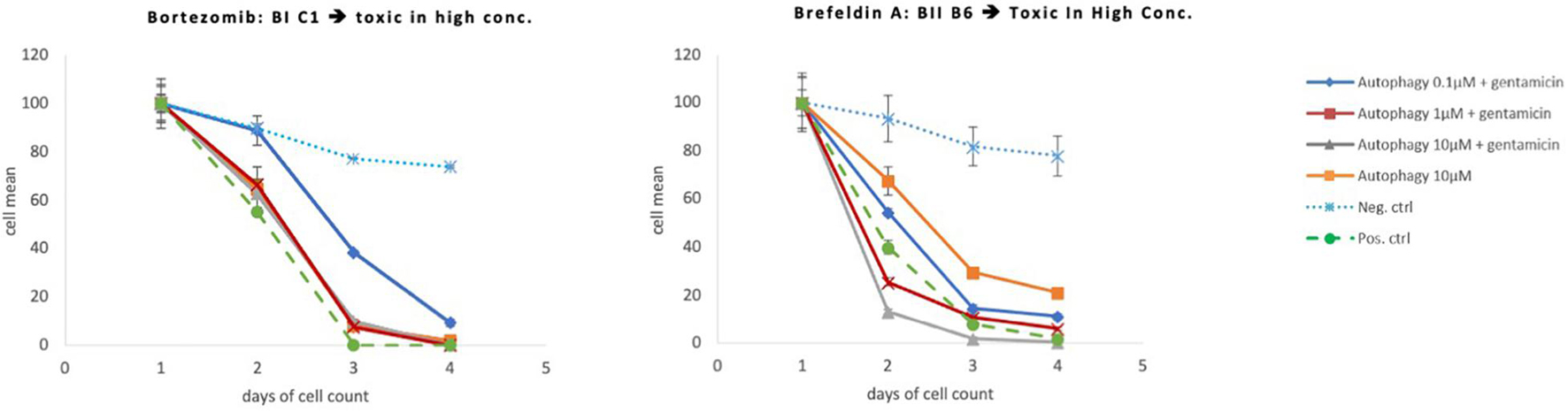
Figure 6. Protective and toxic autophagy compounds. These compounds had a strong protective effects at low doses but were toxic at the highest concentration dose tested (10 μM).
Group III: Toxic
One autophagy compound BGT 226 showed HC decrease when treated with 10 μM of the compound alone (even in the absence of gentamicin). This was significant at 48 and 72 h (Figure 7).
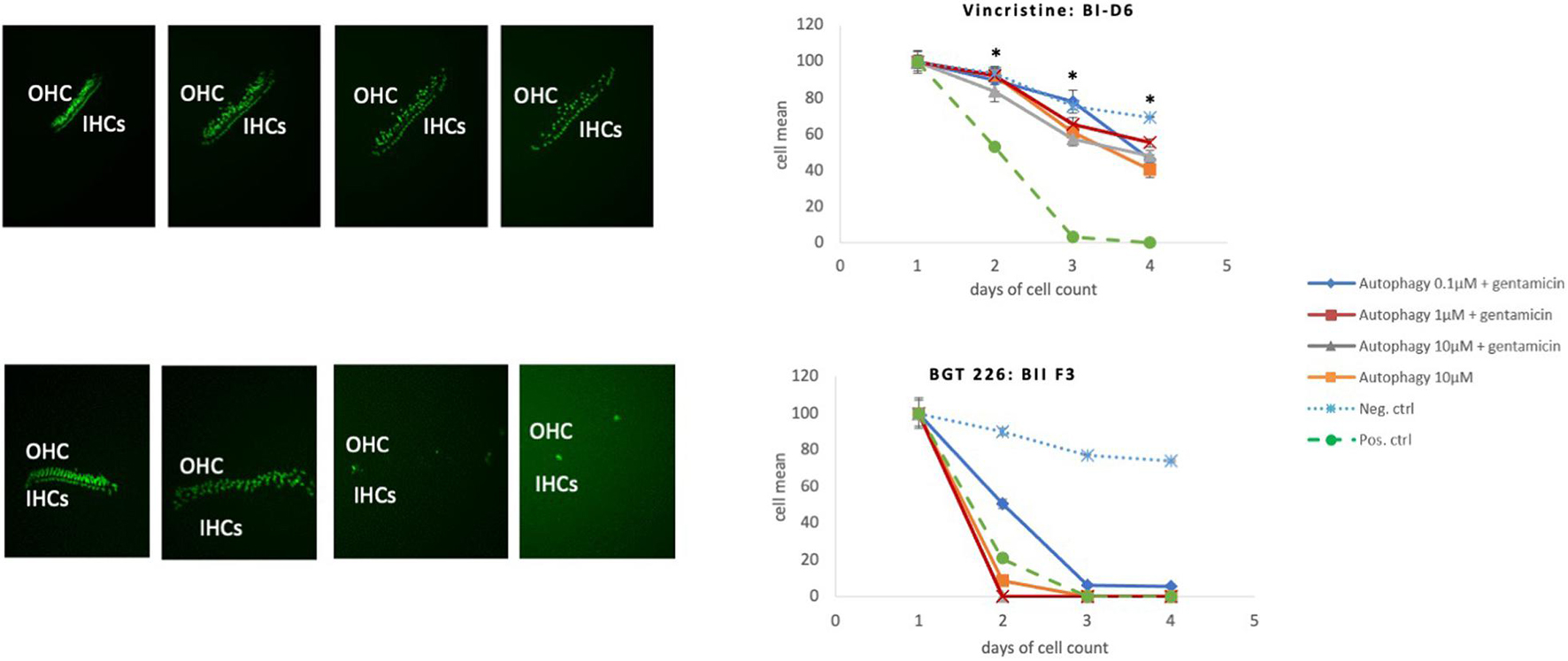
Figure 7. Toxic autophagy compounds. Comparison of an autophagy compounds with a protective effect (Vincristine) with an autophagy compound with a toxic effect (BGT 226). Protective Effect: Vincristine has a significant protective effect in all three dosages *(p < 0.05). Toxic effect of BGT 226 (AMG-IIF3), Inhibitor of the PI3K/AKT/mTOR pathway, exhibiting HC loss even in the absence of GM.
Discussion
Summary
Using an assay based on micro-explants of the neonatal mouse organ of Corti, a variety of autophagy compounds were screened for their ability to alter aminoglycoside damage to mammalian cochlear HCs in vitro. All statistically significant “hits” were confirmed by re-screening. Of a total of 154 compounds tested, 18 exhibited protective effects against GM toxicity, two of these compounds: Bortezomib, a protein homeostasis inhibitor and Brefeldin A, a cell cycle regulator were protective at low dosage but showed enhanced GM toxicity at high dosage. One compound, BGT 226 an Inhibitor of the PI3K/AKT/mTOR pathway was toxic to HCs in the absence of GM.
HC Protective Autophagy Compounds
Among the 154 compounds tested, 18 proved to be protective to HCs. To our knowledge, none of the autophagy compounds identified in the screen have been previously identified as influencing HC damage in vitro or in vivo. While all exhibited statistically significant effects, the range of protection varied from slight to strong. The protective autophagy compounds had a broad spectrum of activities ranging from protein homeostasis inhibitors, cell cycle and receptor kinase inhibitors to calcium channel and calcium uptake blockers. However, unlike our prior screens of kinase inhibitors (Ryals et al., 2017) and antioxidants (Noack et al., 2017), many of the compounds in the autophagy library are not highly specific to autophagy. The protective autophagy compounds found in this screen can be divided into the following categories, listed in order of the number of protective library compounds per category.
Inhibitors of Protein Homeostasis
Proteasomes are protein complexes that degrade unneeded, misfolded or damaged proteins by proteolysis. Misfolded proteins trafficked from the Golgi to the ER are retained in the ER and retro-translocated to the cytosol in vesicles (Omari et al., 2018). Along with damaged proteins, they are tagged for proteasome proteolysis by ubiquitins (Taxis et al., 2002). Autophagy is thought to co-operate with the ubiquitin-proteasome system in responding to cell injury (Ji and Kwon, 2017). The proteasome is known to protect cells from degeneration by removing damaged proteins (Jones and Tepe, 2019), but an overactive proteasome can enhance tissue damage (Meller, 2009; Chen et al., 2010). Six compounds out of the 18 protective compounds were proteasome inhibitors.
a. LDN-57444: Reversible, competitive proteasome inhibitor for Uch-L1.
b. MG-132: Cell permeable proteasome and calpain inhibitor, in low dosage protective, in high dosage toxic.
c. Bortezomib: Proteasome inhibitor.
d. Omeprazole: Proton pump inhibitor. Proton pump inhibition reduces the expression of proteasome subunits, leading to proteasome inhibition (Cao et al., 2020).
e. PYR-41: Ubiquitin Proteasome inhibitor. Reduces ubiquitination, required for proteasome degradation of proteins, but also inhibits NFkB depending on its half-maximal inhibitory concentrations (IC50) which has been identified between 10–25 μM in cells (Yang et al., 2007).
f. Brefeldin A. An inhibitor of macro-autophagy, reduces the release of proteins from the ER.
The library had twelve inhibitory compounds targeting the proteasome pathway and of which six were identified protective in our screen. These results strongly suggest that the proteasome overactivity contributes to HC damage. Cheng et al. (2016) identified that Atoh1 (Atonal BHLH Transcription Factor 1) was regulated by the ubiquitin-proteasome system and hence ubiquitin proteasome pathway appears necessary for hair cell fate determination and survival. Also, we recently reported that multiple proteins of the ubiquitin-proteasome system are up-regulated in the cochlea following noise damage (Jongkamonwiwat et al., 2020), suggesting the possibility of a similar involvement in noise-induced HC damage associated with protein abundance and protein misfolding.
Microtubule Interfering Agents
In autophagy, mature autophagosomes are transported to lysosomes along microtubules with force generated by the actin cytoskeleton. Microtubules are also involved in the transport of other cellular constituents, cell structure and cell migration. The library had twelve compounds associated with autophagy, microtubules and actin of which four inhibitors of were protective.
a. Nocodazole: Reversible inhibitor of Abl and microtubule polymerization:
b. Vincristine: binds tubulin protein and counteracts the formation of microtubules.
c. Y- 27632: Inhibitor of ROCK-1, which promotes contractile force generation in the actin cytoskeleton and movement along microtubules.
d. Fasudil: ROCK inhibitor.
Inhibitors of Calcium Regulation
Calcium regulates autophagy at several different points in the process (Bootman et al., 2018). Increases in intracellular calcium, from calcium channels, calcium uptake or release from mitochondria, can induce autophagy. Conversely, calcium release leading to entry into mitochondria increases ATP production, which inhibits mitochondria (Kondratskyi et al., 2013). However, calcium also plays multiple additional roles in cells. Loss of calcium homeostasis is a major factor in cell injury and death through multiple mechanisms include apoptosis and necrosis (Dong et al., 2006). Intracellular calcium is tightly regulated in part to prevent the accumulation of excessive levels. HCs in particular contain high levels of calcium buffering proteins and express multiple calcium channels and transporters. Calcium subserves multiple normal functions of HCs (Heller et al., 2002). A number of studies have highlighted the role of calcium in HC damage, including release of calcium from the endoplasmic reticulum and mitochondria. It has been suggested that differences in HC vulnerability to damage along the length of the cochlea are related to variation in the ability to handle calcium load (Fettiplace and Nam, 2019). It has been suggested that calcium channel blockers should be investigated as therapeutic agents for acquired hearing loss (Naples, 2017). In this screen, the library had fourteen compounds that influence intracellular calcium levels and four were found to be protective.
a. Manidipine: Calcium channel blocker (L and T gated channels).
b. Nimodipine: Calcium channel blocker (L gated channels).
c. Ranolazine: Calcium uptake inhibitor.
d. Rotundine: Rotundine is a natural plant alkaloid with complex effects. It has been reported to block L-type calcium channels, but also dopamine receptors, and to activates potassium channels. It has been shown to be neuroprotective e.g., in alpha synuclein accumulating diseases such as Parkinson (Ghanem et al., 2021).
The expected results of these inhibitors would be to reduce intracellular calcium. While this strongly supports a critical role for calcium in HC damage, it cannot be attributed with any certainty to the regulation of autophagy.
Inhibitors of the PI3K/AKT/mTOR Pathway
The PI3K/AKT/mTOR is an evolutionally conserved cascade that integrates signals from multiple pathways, including nutrients (e.g., amino acids and glucose), growth factors (e.g., EGF, PDGF, IGF-1), hormones (e.g., leptin), and stresses (e.g., starvation, hypoxia, and DNA damage) to regulate a wide variety of eukaryotic cellular functions, such as autophagy, translation, transcription, protein turnover, metabolism, energy balance, and stress response, as well as cell proliferation, growth, differentiation and survival. mTOR can also be activated independently of PI3K and AKT. The library contains 25 compounds related to PI3K/mTOR and three inhibitors were protective.
a. PI-103: PI3K/mTOR inhibitor.
b. BGT 226: PI3K/mTOR inhibitor.
c. GDC-0349: Selective ATP-competitive inhibitor of mTOR.
Because of the diverse rolls of the PI3K/AKT/mTOR pathway, the mechanism by which these inhibitors protect HCs is not clear. mTOR reduces autophagy by negatively regulating several key molecules (Kim and Guan, 2015), so the promotion of autophagy is one possibility. However, in our prior screen of kinase inhibitors (Ryals et al., 2017), one EGFR inhibitor, two PDGF receptor and two AKT inhibitors were found to be protective. This result is consistent with a damaging role for the PI3K/AKT/mTOR pathway, which is activated by growth factors. However, one of the autophagy compounds, BGT 226, showed a “push pull” relationship with a toxic effect in the highest concentration leading to HC death. Deletion of either AKT1 or AKT2 has been shown to enhance HC death due to aminoglycosides (Brand et al., 2015). It is possible that the three PI3K/mTOR inhibitors preferentially target PI3K/mTOR mediation of stress responses. Alternatively, they may target one of the several mTOR responses that are independent of AKT.
Inhibitors of the Cell Cycle
Aurora kinase A is critical to the process of mitotic spindle formation and separation, and thus of cell division. Inhibition of Aurora kinase A blocks cell proliferation and can induce autophagy (Zou et al., 2012). In our screening assay two inhibitors of Aurora kinase inhibitors showed a protective effect:
a. AMG900: Selective pan-Aurora kinase inhibitor.
b. MK-5108: Selective Aurora kinase A inhibitor.
Overall the library had 20 compounds related to the Aurora kinase pathway and only two compounds were protective. This is consistent with the finding that maintaining the fully differentiated state is critical for HC survival and that forced cell cycle re-entry in differentiated, post-mitotic cells in response to stress can lead to mitotic catastrophe and cell death (Weber et al., 2008; Sharma et al., 2017). This provides strong evidence that one aspect of gentamicin-induced HC damage may be induction of the cell cycle.
Inhibitor of Inflammation and Immunity
NFkB Inhibitor: BAY 11-7082. The role of immune responses and inflammation in HC damage is increasingly recognized (Frye et al., 2019). Damage to HCs results in the release of inflammatory mediators such as the inflammatory cytokine TNFα (Tan et al., 2016). Damaged cells can also release cellular constituents that serve as damage-associated molecular patterns (DAMPs). Damps in turn can activate innate immune receptors such as the Toll-like receptors (TLRs), leading to the production of inflammatory cytokines such as IL1β and TNFα, as well chemokines that attract immune cells including macrophages. Many inflammatory responses are mediated by the transcription factor NFkB, which can also stimulate autophagy. An inhibitor of this factor was protective in the screen. This result supports a role for inflammation in ototoxic HC damage.
Protein Trafficking
An inhibitor of protein trafficking was protective, Brefeldin A. Brefeldin A is an inhibitor of protein trafficking between the endoplasmic reticulum and the Golgi apparatus (Chardin and McCormick, 1999). These results suggest that the movement of intracellular elements, possibly including autophagosomes, contribute to ototoxic HC damage.
The majority of the compounds that proved to be protective to HCs against gentamicin damage would be expected to reduce autophagy. While several of these compounds have complex effects, the fact that multiple autophagy inhibitors reduced HC loss argues for an autophagy role in promoting HC damage due to GM. Levano and Bodmer found that mutation of the Stat1 gene protected HCs from ototoxic damage in part by modulating autophagy genes so as to reduce autophagy (Levano and Bodmer, 2015).
Autophagy is often considered to be protective of cells under stress, but it has been shown that excessive activation of autophagy can lead to autophagic cell death, or autosis (Liu and Levine, 2015). The high dosage of GM used in this study may have induced excessive autophagy. Apoptosis, necrosis and necroptosis have been shown to participate in HC death (Ruhl et al., 2019). However, autosis has not previously been linked to HC damage. Autosis is characterized by nuclear shrinkage with focal concavities and ballooning of the perinuclear space. These characteristics are visible in some published electron micrographs of aminoglycoside-damaged HCs (Lang and Liu, 1997).
While most autophagy inhibiting compounds were protective, we also observed protection with compounds that would be expected to enhance autophagy. Again, while these compounds have complex effects, the results support the concept that autophagy can be protective against aminoglycoside damage. It seems likely that autophagy plays a complex role in ototoxic HC damage, such that manipulation of different aspects of the process can have differing effects. The interactions of identified compounds with the autophagy machinery warrants further mechanistic studies because these candidate leads may employ one or more of several mechanisms. Therefore, differences in their effectiveness may vary with differences in the cellular processes involved and aminoglycoside impact. Given the complexity, it would not be expected that all compounds in a category would have equivalent effects on cell damage and survival.
Mammalian Organ of Corti Micro-Explant Assay
There are multiple screening assays available today. The assay presented here, like many preclinical assays, has both advantages and disadvantages. A major advantage is that organ of Corti micro-explant screening employs mammalian cochlear HCs. Cochlear HCs in mammals include inner and outer HCs, as in humans, while the HCs of other classes of non-mammalian animals are quite different from human HCs. This is important, since outer HCs are more sensitive to damage than other HC types. They are also highly specialized, with structural and functional features that are very distinct. The damage process in these highly specialized cells may not be adequately modeled by that in other HC types, or by those in immortalized cell lines derived from the organ of Corti. These cell lines are de-differentiated, and express genes of both early developing HCs and supporting cells.
In general, a larger number of compounds can be tested in an in vitro oC assay than can be achieved using in vivo models, which are the only alternatives that allows testing of mammalian cochlear HCs. For this assay, the use of oC micro-explants enables an even larger number of compounds evaluations. Several micro-explants can be generated from each murine organ of Corti. Moreover, because the HCs of pou4f3/GFP mice are selectively fluorescent, they do not need to be stained and can be quantified throughout the period of culture in a single micro-explant. Thus multiple groups of explants are not necessary to determine the kinetics of compound effects. Because of these features, screening of a few hundred compounds is readily achieved. The ototoxin chosen for the assay, GM, is commonly prescribed for life-threatening infections and is thus relevant to human therapy.
Disadvantages include the fact that the assay is based on neonatal HCs, which have known differences from adult HCs (Hur et al., 2018). Mature adult HC do not survive in culture and many studies have demonstrated that cell cycle reactivation in mammalian cochlear HCs results in cell death in vitro and in vivo (Laine et al., 2007; Sulg et al., 2010). Moreover, like in other screening techniques, the micro-explant screening assay identified candidates must be confirmed by post-screen characterization of hits and testing in in vivo. Finally, when compared to immortalized cell line or zebrafish lateral line screens, the assay is only medium-throughput. Very large compound libraries cannot be screened, and the number of compound and ototoxin dosages tested must be limited.
Conclusion
The preponderance of the results from our screen of an autophagy library suggest that autophagy contributes to ototoxic HC damage in a complex manner. However, the compounds tested have many potential alternative effects. For this reason we cannot conclude with confidence that changes in autophagy were the primary reason for HC protection. Additional studies will need to be performed to further validate the results of our screen and to determine the relationship between autophagy and HC damage in vivo. However, the data strongly implicate the proteasome and proteolysis as contributors to HC damage.
Data Availability Statement
The original contributions presented in the study are included in the article/Supplementary Material, further inquiries can be directed to the corresponding author/s.
Ethics Statement
The animal study was reviewed and approved by VA IACUC.
Author Contributions
CD, TW, EC, and KP performed the laboratory experiments and screening assay. CD, AK, and AL analyzed the data. CD, SD, and AR conceived the study and designed the experiments. CD wrote the initial manuscript and generated figures. All authors reviewed and approved the manuscript.
Funding
The research for this study was supported by the German Research Foundation (Deutsche Forschungsgemeinschaft DFG) and by grant BX001205 from the Veterans Health Administration.
Conflict of Interest
AR is a co-founder, shareholder, and consultant of Otonomy Inc., which develops slow-release drug compounds for the treatment of middle and inner ear diseases. The UCSD Committee on Conflict of Interest has approved this relationship. Otonomy Inc., played no part in the research reported here.
The remaining authors declare that the research was conducted in the absence of any commercial or financial relationships that could be construed as a potential conflict of interest.
Publisher’s Note
All claims expressed in this article are solely those of the authors and do not necessarily represent those of their affiliated organizations, or those of the publisher, the editors and the reviewers. Any product that may be evaluated in this article, or claim that may be made by its manufacturer, is not guaranteed or endorsed by the publisher.
Supplementary Material
The Supplementary Material for this article can be found online at: https://www.frontiersin.org/articles/10.3389/fcell.2021.762751/full#supplementary-material
References
Abou-Zeid, A. A., Eissa, A. I., and Salem, H. M. (1978). Mode of action of gentamicin antibiotics produced by Micromonospora purpurea. Zentralbl. Bakteriol. Naturwiss. 133, 362–368. doi: 10.1016/S0323-6056(78)80054-8
Aburto, M. R., Sanchez-Calderon, H., Hurle, J. M., Varela-Nieto, I., and Magarinos, M. (2012). Early otic development depends on autophagy for apoptotic cell clearance and neural differentiation. Cell Death Dis. 3:e394. doi: 10.1038/cddis.2012.132
Al-Malky, G., Dawson, S. J., Sirimanna, T., Bagkeris, E., and Suri, R. (2015). High-frequency audiometry reveals high prevalence of aminoglycoside ototoxicity in children with cystic fibrosis. J. Cyst. Fibros. 14, 248–254. doi: 10.1016/j.jcf.2014.07.009
Arroyo, D. S., Gaviglio, E. A., Peralta Ramos, J. M., Bussi, C., Rodriguez-Galan, M. C., and Iribarren, P. (2014). Autophagy in inflammation, infection, neurodegeneration and cancer. Int. Immunopharmacol. 18, 55–65. doi: 10.1016/j.intimp.2013.11.001
Benkafadar, N., François, F., Affortit, C., Casas, F., Ceccato, J. C., Menardo, J., et al. (2019). ROS-Induced activation of DNA damage responses drives senescence-like state in postmitotic cochlear cells: implication for hearing preservation. Mol. Neurobiol. 56, 5950–5969. doi: 10.1007/s12035-019-1493-6
Bootman, M. D., Chehab, T., Bultynck, G., Parys, J. B., and Rietdorf, K. (2018). The regulation of autophagy by calcium signals: do we have a consensus? Cell Calcium 70, 32–46. doi: 10.1016/j.ceca.2017.08.005
Brand, Y., Levano, S., Radojevic, V., Naldi, A. M., Setz, C., Ryan, A. F., et al. (2015). All Akt isoforms (Akt1, Akt2, Akt3) are involved in normal hearing, but only Akt2 and Akt3 are involved in auditory hair cell survival in the mammalian inner ear. PLoS One 10:e0121599. doi: 10.1371/journal.pone.0121599
Cao, Y., Zhu, H., He, R., Kong, L., Shao, J., Zhuang, R., et al. (2020). Proteasome, a promising therapeutic target for multiple diseases beyond cancer. Drug Des. Devel. Ther. 14, 4327–4342. doi: 10.2147/DDDT.S265793
Chardin, P., and McCormick, F. (1999). Brefeldin A: the advantage of being uncompetitive. Cell 97, 153–155. doi: 10.1016/S0092-8674(00)80724-2
Chen, Y., Azad, M. B., and Gibson, S. B. (2010). Methods for detecting autophagy and determining autophagy-induced cell death. Can. J. Physiol. Pharmacol. 88, 285–295. doi: 10.1139/Y10-010
Cheng, Y. F., Tong, M., and Edge, A. S. (2016). Destabilization of Atoh1 by E3 ubiquitin ligase Huwe1 and casein kinase 1 is essential for normal sensory hair cell development. J. Biol. Chem. 291, 21096–21109. doi: 10.1074/jbc.M116.722124
Dong, Z., Saikumar, P., Weinberg, J. M., and Venkatachalam, M. A. (2006). Calcium in cell injury and death. Annu. Rev. Pathol. 1, 405–434. doi: 10.1146/annurev.pathol.1.110304.100218
Esclatine, A., Chaumorcel, M., and Codogno, P. (2009). Macroautophagy signaling and regulation. Curr. Top. Microbiol. Immunol. 335, 33–70. doi: 10.1007/978-3-642-00302-8_2
Fettiplace, R., and Nam, J. H. (2019). Tonotopy in calcium homeostasis and vulnerability of cochlear hair cells. Hear. Res. 376, 11–21. doi: 10.1016/j.heares.2018.11.002
Frye, M. D., Ryan, A. F., and Kurabi, A. (2019). Inflammation associated with noise-induced hearing loss. J. Acoust. Soc. Am. 146, 4020. doi: 10.1121/1.5132545
Ghanem, S. S., Fayed, H. S., Zhu, Q., Lu, J. H., Vaikath, N. N., Ponraj, J., et al. (2021). Natural alkaloid compounds as inhibitors for alpha-synuclein seeded fibril formation and toxicity. Molecules 26:3736. doi: 10.3390/molecules26123736
Hamilton, J. W. (2001). Gentamicin in pharmacogenetic approach to treatment of cystic fibrosis. Lancet 358, 2014–2016. doi: 10.1016/S0140-6736(01)07138-0
He, L., Zhang, J., Zhao, J., Ma, N., Kim, S. W., Qiao, S., et al. (2018). Autophagy: the last defense against cellular nutritional stress. Adv. Nutr. 9, 493–504. doi: 10.1093/advances/nmy011
Heller, S., Bell, A. M., Denis, C. S., Choe, Y., and Hudspeth, A. J. (2002). Parvalbumin 3 is an abundant Ca2+ buffer in hair cells. J. Assoc. Res. Otolaryngol. 3, 488–498. doi: 10.1007/s10162-002-2050-x
Hur, D. G., Kurabi, A., and Ryan, A. F. (2018). Screening antioxidants for the protection of cochlear sensory cells. Neural Regen. Res. 13, 62–64. doi: 10.4103/1673-5374.224371
Ji, C. H., and Kwon, Y. T. (2017). Crosstalk and interplay between the ubiquitin-proteasome system and autophagy. Mol. Cells 40, 441–449.
Jones, C. L., and Tepe, J. J. (2019). Proteasome activation to combat proteotoxicity. Molecules 24:2841. doi: 10.3390/molecules24152841
Jongkamonwiwat, N., Ramirez, M. A., Edassery, S., Wong, A. C. Y., Yu, J., Abbott, T., et al. (2020). Noise exposures causing hearing loss generate proteotoxic stress and activate the proteostasis network. Cell Rep. 33:108431. doi: 10.1016/j.celrep.2020.108431
Kim, Y. C., and Guan, K. L. (2015). mTOR: a pharmacologic target for autophagy regulation. J. Clin. Invest. 125, 25–32. doi: 10.1172/JCI73939
Kim, Y. J., Tian, C., Kim, J., Shin, B., Choo, O. S., Kim, Y. S., et al. (2017). Autophagic flux, a possible mechanism for delayed gentamicin-induced ototoxicity. Sci. Rep. 7:41356. doi: 10.1038/srep41356
Kondratskyi, A., Yassine, M., Kondratska, K., Skryma, R., Slomianny, C., and Prevarskaya, N. (2013). Calcium-permeable ion channels in control of autophagy and cancer. Front. Physiol. 4:272. doi: 10.3389/fphys.2013.00272
Laine, H., Doetzlhofer, A., Mantela, J., Ylikoski, J., Laiho, M., Roussel, M. F., et al. (2007). p19(Ink4d) and p21(Cip1) collaborate to maintain the postmitotic state of auditory hair cells, their codeletion leading to DNA damage and p53-mediated apoptosis. J. Neurosci. 27, 1434–1444. doi: 10.1523/JNEUROSCI.4956-06.2007
Lang, H., and Liu, C. (1997). Apoptosis and hair cell degeneration in the vestibular sensory epithelia of the guinea pig following a gentamicin insult. Hear. Res. 111, 177–184. doi: 10.1016/S0378-5955(97)00098-1
Lee, J., Giordano, S., and Zhang, J. (2012). Autophagy, mitochondria and oxidative stress: cross-talk and redox signalling. Biochem. J. 441, 523–540. doi: 10.1042/BJ20111451
Levano, S., and Bodmer, D. (2015). Loss of STAT1 protects hair cells from ototoxicity through modulation of STAT3, c-Jun, Akt, and autophagy factors. Cell Death Dis. 6:e2019. doi: 10.1038/cddis.2015.362
Levine, B., and Kroemer, G. (2008). Autophagy in the pathogenesis of disease. Cell 132, 27–42. doi: 10.1016/j.cell.2007.12.018
Liu, Y., and Levine, B. (2015). Autosis and autophagic cell death: the dark side of autophagy. Cell Death Differ. 22, 367–376. doi: 10.1038/cdd.2014.143
Magarinos, M., Pulido, S., Aburto, M. R., de Iriarte Rodriguez, R., and Varela-Nieto, I. (2017). Autophagy in the vertebrate inner ear. Front. Cell Dev. Biol. 5:56. doi: 10.3389/fcell.2017.00056
Masuda, M., Dulon, D., Pak, K., Mullen, L. M., Li, Y., Erkman, L., et al. (2011). “Regulation of POU4F3 gene expression in hair cells by 5’ DNA in mice. Neuroscience 197, 48–64. doi: 10.1016/j.neuroscience.2011.09.033
Meller, R. (2009). The role of the ubiquitin proteasome system in ischemia and ischemic tolerance. Neuroscientist 15, 243–260. doi: 10.1177/1073858408327809
Mizushima, N. (2011). Autophagy in protein and organelle turnover. Cold Spring Harb. Symp. Quant. Biol. 76, 397–402. doi: 10.1101/sqb.2011.76.011023
Mizushima, N., and Komatsu, M. (2011). Autophagy: renovation of cells and tissues. Cell 147, 728–741. doi: 10.1016/j.cell.2011.10.026
Mizushima, N., Levine, B., Cuervo, A. M., and Klionsky, D. J. (2008). Autophagy fights disease through cellular self-digestion. Nature 451, 1069–1075. doi: 10.1038/nature06639
Naples, J. G. (2017). Calcium-channel blockers as therapeutic agents for acquired sensorineural hearing loss. Med. Hypotheses 104, 121–125. doi: 10.1016/j.mehy.2017.05.036
Noack, V., Pak, K., Jalota, R., Kurabi, A., and Ryan, A. F. (2017). An antioxidant screen identifies candidates for protection of cochlear hair cells from gentamicin toxicity. Front. Cell. Neurosci. 11:242. doi: 10.3389/fncel.2017.00242
Obregon, A. M., Escalante, L., Gonzalez, R., Rodriguez, R., and Sanchez, S. (1994). Physiological studies on gentamicin: phosphate repression of antibiotic formation. J. Antibiot. (Tokyo) 47, 1442–1446. doi: 10.7164/antibiotics.47.1442
Oh, J. M., Choi, E. K., Carp, R. I., and Kim, Y. S. (2012). Oxidative stress impairs autophagic flux in prion protein-deficient hippocampal cells. Autophagy 8, 1448–1461. doi: 10.4161/auto.21164
Ohsumi, Y. (2001). Molecular dissection of autophagy: two ubiquitin-like systems. Nat. Rev. Mol. Cell Biol. 2, 211–216. doi: 10.1038/35056522
Omari, S., Makareeva, E., Roberts-Pilgrim, A., Mirigian, L., Jarnik, M., Ott, C., et al. (2018). Noncanonical autophagy at ER exit sites regulates procollagen turnover. Proc. Natl. Acad. Sci. U.S.A. 115, E10099–E10108. doi: 10.1073/pnas.1814552115
Rabinowitz, J. D., and White, E. (2010). Autophagy and metabolism. Science 330, 1344–1348. doi: 10.1126/science.1193497
Ruhl, D., Du, T. T., Wagner, E. L., Choi, J. H., Li, S., Reed, R., et al. (2019). Necroptosis and apoptosis contribute to cisplatin and aminoglycoside ototoxicity. J. Neurosci. 39, 2951–2964. doi: 10.1523/JNEUROSCI.1384-18.2019
Ryals, M., Pak, K., Jalota, R., Kurabi, A., and Ryan, A. F. (2017). A kinase inhibitor library screen identifies novel enzymes involved in ototoxic damage to the murine organ of Corti. PLoS One 12:e0186001. doi: 10.1371/journal.pone.0186001
Ryter, S. W., Koo, J. K., and Choi, A. M. (2014). Molecular regulation of autophagy and its implications for metabolic diseases. Curr. Opin. Clin. Nutr. Metab. Care 17, 329–337. doi: 10.1097/MCO.0000000000000068
Sagwa, E. L., Ruswa, N., Mavhunga, F., Rennie, T., Leufkens, H. G., and Mantel-Teeuwisse, A. K. (2015). Comparing amikacin and kanamycin-induced hearing loss in multidrug-resistant tuberculosis treatment under programmatic conditions in a Namibian retrospective cohort. BMC Pharmacol. Toxicol. 16:36. doi: 10.1186/s40360-015-0036-7
Sapizhak, I., Timen, H., Semenova, V., and Staino, L. (2017). The neuronal stem cells effect on inner ear structure in experimental gentamicin ototoxicity. Ukr. Neurosurg. J. 4, 55–59. doi: 10.25305/unj.115236
Sharma, P., Haycocks, J. R. J., Middlemiss, A. D., Kettles, R. A., Sellars, L. E., Ricci, V., et al. (2017). The multiple antibiotic resistance operon of enteric bacteria controls DNA repair and outer membrane integrity. Nat. Commun. 8:1444. doi: 10.1038/s41467-017-01405-7
Sulg, M., Kirjavainen, A., Pajusola, K., Bueler, H., Ylikoski, J., Laiho, M., et al. (2010). Differential sensitivity of the inner ear sensory cell populations to forced cell cycle re-entry and p53 induction. J. Neurochem. 112, 1513–1526. doi: 10.1111/j.1471-4159.2009.06563.x
Tan, W. J., Thorne, P. R., and Vlajkovic, S. M. (2016). Characterisation of cochlear inflammation in mice following acute and chronic noise exposure. Histochem. Cell Biol. 146, 219–230. doi: 10.1007/s00418-016-1436-5
Taxis, C., Vogel, F., and Wolf, D. H. (2002). ER-golgi traffic is a prerequisite for efficient ER degradation. Mol. Biol. Cell 13, 1806–1818. doi: 10.1091/mbc.01-08-0399
Taylor, M. P., and Kirkegaard, K. (2008). Potential subversion of autophagosomal pathway by picornaviruses. Autophagy 4, 286–289. doi: 10.4161/auto.5377
Weber, T., Corbett, M. K., Chow, L. M., Valentine, M. B., Baker, S. J., and Zuo, J. (2008). Rapid cell-cycle reentry and cell death after acute inactivation of the retinoblastoma gene product in postnatal cochlear hair cells. Proc. Natl. Acad. Sci. U.S.A. 105, 781–785. doi: 10.1073/pnas.0708061105
Xing, Y., Ji, Q., Li, X., Ming, J., Zhang, N., Zha, D., et al. (2017). Asiaticoside protects cochlear hair cells from high glucose-induced oxidative stress via suppressing AGEs/RAGE/NF-kappaB pathway. Biomed. Pharmacother. 86, 531–536. doi: 10.1016/j.biopha.2016.12.025
Yang, Y., Kitagaki, J., Dai, R. M., Tsai, Y. C., Lorick, K. L., Ludwig, R. L., et al. (2007). Inhibitors of ubiquitin-activating enzyme (E1), a new class of potential cancer therapeutics. Cancer Res. 67, 9472–9481. doi: 10.1158/0008-5472.CAN-07-0568
Keywords: autophagy, hair cell, aminoglycoside–ototoxicity, in vitro screen, inner ear
Citation: Draf C, Wyrick T, Chavez E, Pak K, Kurabi A, Leichtle A, Dazert S and Ryan AF (2021) A Screen of Autophagy Compounds Implicates the Proteasome in Mammalian Aminoglycoside-Induced Hair Cell Damage. Front. Cell Dev. Biol. 9:762751. doi: 10.3389/fcell.2021.762751
Received: 22 August 2021; Accepted: 05 October 2021;
Published: 26 October 2021.
Edited by:
Isabel Varela-Nieto, Consejo Superior de Investigaciones Científicas (CSIC), SpainReviewed by:
Laura Astolfi, University of Padua, ItalyNesrine Benkafadar, Stanford University, United States
Federico Kalinec, University of California, Los Angeles, United States
Copyright © 2021 Draf, Wyrick, Chavez, Pak, Kurabi, Leichtle, Dazert and Ryan. This is an open-access article distributed under the terms of the Creative Commons Attribution License (CC BY). The use, distribution or reproduction in other forums is permitted, provided the original author(s) and the copyright owner(s) are credited and that the original publication in this journal is cited, in accordance with accepted academic practice. No use, distribution or reproduction is permitted which does not comply with these terms.
*Correspondence: Clara Draf, Y2xhcmFkcmFmQGdtYWlsLmNvbQ==