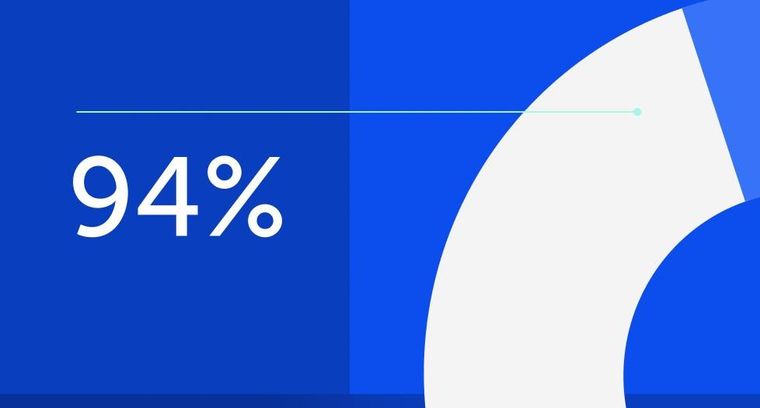
94% of researchers rate our articles as excellent or good
Learn more about the work of our research integrity team to safeguard the quality of each article we publish.
Find out more
MINI REVIEW article
Front. Cell Dev. Biol., 15 October 2021
Sec. Signaling
Volume 9 - 2021 | https://doi.org/10.3389/fcell.2021.757305
This article is part of the Research TopicThe Subcellular Architecture of Mitochondria in Driving Cellular ProcessesView all 8 articles
Across different cell types and within single cells, mitochondria are heterogeneous in form and function. In skeletal muscle cells, morphologically and functionally distinct subpopulations of mitochondria have been identified, but the mechanisms by which the subcellular specialization of mitochondria contributes to energy homeostasis in working muscles remains unclear. Here, we discuss the current data regarding mitochondrial heterogeneity in skeletal muscle cells and highlight potential new lines of inquiry that have emerged due to advancements in cellular imaging technologies.
Skeletal muscle cells function to convert biological fuels to mechanical force and are therefore critical to many physiological functions, including movement and metabolism. During sustained muscle contractions, the process of cellular energy transduction is largely mediated by the energy-converting function of the mitochondria and the force-generating capacity of the myofibrils. Specifically, mitochondria convert biological fuels to the high energy molecule adenosine triphosphate (ATP) through oxidative phosphorylation, and ATP is used by the myofibrillar ATPase to generate force during contraction. Although the vast majority of muscle cell volume is dedicated to the myofibrillar matrix (Willingham et al., 2020), mammalian skeletal muscles can be 2–10% mitochondria by volume (Bleck et al., 2018), and muscle cells with higher mitochondrial content have greater energy-converting capacity (Holloszy, 1967; Schwerzmann et al., 1989; Ørtenblad et al., 2018). Like most cell types, not all mitochondria within the muscle cell are the same, and mitochondrial form and function can vary widely within a single muscle cell (Johnson et al., 2007; Lewis et al., 2016; Valm et al., 2017; Benador et al., 2018). For the past half-century, experimental studies have consistently demonstrated that muscle cells contain structurally and functionally distinct subpopulations of mitochondria based on their proximity to the myofibrils, nuclei, capillaries, and cell membrane (Palmer et al., 1977, 1985; Cogswell et al., 1993; Takahashi and Hood, 1996; Rothstein et al., 2005; Glancy et al., 2014, 2015, 2018; Figure 1). However, the defining characteristics (e.g., molecular markers) of mitochondrial subpopulations in skeletal muscle cells remain elusive, and the mechanisms by which the subcellular specialization of mitochondria contributes to energy homeostasis in working muscles remains unclear. Here, we review current data regarding mitochondrial heterogeneity in skeletal muscle cells and discuss potential implications for the subcellular specialization of mitochondrial form and function. Particularly, we focus on how the development of subcellular imaging technologies expands our capacity to simultaneously evaluate muscle structure and function and highlight potential new lines of inquiry regarding the subcellular specialization of mitochondria.
Figure 1. Specialization of Mitochondrial Morphology in Skeletal Muscle. (A) Cross-section diagram of muscle cell showing the morphologically distinct peripherally-located mitochondria (PM) and intermyofibrillar mitochondria (IFM) subpopulations. Inserts provide examples of 3D morphology of PM and IFM. Mitochondria (green) and Myofibrils (gray). (B) Longitudinal view of muscle cell showing the subcellular location of PM and IFM relative to the sarcolemma (blue), capillary (red), Myonuclei (cyan), and myofibrils (gray).
Within the interior region of the muscle cell, the delicate interaction between the mitochondria and myofibrils supports precise coordination among the metabolic and mechanical arms of the muscle cellular energy distribution system. The intrafibrillar mitochondria (IFMs) form complex, elongated shapes (Hoppeler et al., 1973a; Picard et al., 2013a,b, 2015; Glancy et al., 2015; Bleck et al., 2018) with branches that extend between the crevices of the myofibrillar matrix and wrap around the I-band of the sarcomere (Hoppeler et al., 1973a; Picard et al., 2013a,b, 2015; Glancy et al., 2015; Bleck et al., 2018; Vincent et al., 2019; Figure 1). In human muscles, mitochondria entrenched within the intermyofibrillar spaces are nearly double the aspect ratio compared to mitochondria located in the periphery, resulting in morphologies with a high surface area to volume ratio (Picard et al., 2013b; Vincent et al., 2019). This high surface area to volume ratio facilitates the rapid diffusion of high-energy ATP molecules from the mitochondria to the myofibrillar ATPase within this region of the cell (Figure 2). During muscle contraction, the metabolic function of the mitochondria and contractile function of myofibrils are also mediated by Ca2+ signaling from the sarcoplasmic reticulum (SR) (Figure 2), so IFMs also utilize their available surface area to form functional contact-sites (i.e., membranes within 30 nm) with the SR (Eisner et al., 2013; Tubbs et al., 2018; Figure 1). Studies using high-resolution 3D electron microscopy to evaluate connectivity in mouse muscles across several striated muscle types have shown that nearly all IFM (>97%) contact the SR. Moreover, this same work also found that ∼20% of the IFM within oxidative muscle cells directly contact lipid droplets while no lipid droplets were found in glycolytic muscle cells (Bleck et al., 2018). Although these data indicate that mitochondria-lipid interactions may contribute to unique mitochondrial function across different cell types, information regarding differences in mitochondria-organelle interactivity between mitochondrial subgroups remains limited. Studies evaluating the spatial distribution of lipids in human muscle cells have shown that lipid droplets preferentially localize to the interfibrillar region of the cell (Nielsen et al., 2010), but it is still unclear how the subcellular distribution of mitochondria-lipid droplet interactions might contribute to the regional specialization of mitochondrial function.
Figure 2. Specialization of Mitochondrial Function across Subcellular Regions. (A) Membrane potential generated in PM can be distributed throughout the IFM network through mitochondrial connectivity. (B) IFM directly supports myofibrillar contraction by generating ATP. Coordination between myofibrillar contraction and IFM energy conversion are mediated by Ca2+ release from the sarcoplasmic reticulum (SR). Mitochondria (green), SR (magenta), t-tubule (blue), and myofibril (gray). (C) Mitochondrial membrane potential distributed throughout the mitochondrial network of living skeletal muscle cell.
Mitochondria also directly interact with other mitochondria, and studies have shown that IFM connect together to form networks that function to distribute energy throughout the entire cell (Bakeeva et al., 1978; Luo et al., 2013; Picard et al., 2013a,b, 2015; Eisner et al., 2014; Glancy et al., 2015, 2018; Bleck et al., 2018; Vincent et al., 2019; Figure 2). Mitochondria-mitochondria contact sites appear as electron-dense junctions (Glancy et al., 2015; Picard et al., 2015), and high-resolution electron microscopy images of these contact sites have shown that portions of the adjacent outer membranes of these connected mitochondria are in direct contact (<1 nm separation) (Bakeeva et al., 1978; Picard et al., 2013a,b, 2015; Glancy et al., 2015, 2018; Bleck et al., 2018; Vincent et al., 2019) and often exhibit trans-mitochondrial alignment of cristae (Picard et al., 2015). Thus, structural capabilities exist within muscle cells for effective energy distribution, challenging the long-held theory that facilitated diffusion is the primary means of distributing intracellular energy within muscles (Wittenberg, 1970; Bessman and Geiger, 1981). Indeed, the phosphocreatine (PCr) shuttle system may also function to facilitate the subcellular distribution of high energy phosphates within muscle cells (Bessman and Geiger, 1981), though mice lacking creatine and creatine kinase do not exhibit robust changes in muscle function (van Deursen et al., 1993; Lygate et al., 2013). Alternatively, live cell imaging studies have demonstrated that electrically coupled mitochondria can transmit membrane potential greater than 10 μm across the intermyofibrillar space (Bleck et al., 2018), providing an additional mechanism for the rapid distribution of potential energy throughout the subcellular environment. Moreover, when comparing the 3D configuration of mitochondrial networks in different striated muscle cell types, mitochondrial connectivity and overall network configuration are cell-type specific, such that glycolytic, oxidative, and cardiac muscles have perpendicular, grid-like, and parallel orientations, respectively (Bleck et al., 2018; Willingham et al., 2019). These findings suggest that the degree of connectivity and spatial distribution within the IFM network are optimized to meet specific regional demands within different muscle types.
Early imaging studies in muscle cell biology used 2D electron microscopy (Romanul, 1964, 1965; Bubenzer, 1966; Gauthier and Padykula, 1966) to reveal a second, morphologically-distinct, subgroup of peripherally-located mitochondria (PM). In the peripheral regions of the muscle cell, pools of globular mitochondria were found clustered together in the intracellular space between the sarcolemma and myofibrils (Romanul, 1964, 1965; Bubenzer, 1966; Gauthier and Padykula, 1966; Hoppeler et al., 1973a; Figure 1). Not long after these initial observations, studies by Bubenzer (1966), and later Bakeeva et al. (1978), demonstrated that PM extend into the intermyofibrillar space and form direct connections with the IFM. These authors proposed that mitochondria connect across all subcellular regions to work together as an “effective mechanism of energy transport” (Bakeeva et al., 1978). Under this model, the morphologically distinct PM subgroups are functionally specialized to support the intermyofibrillar regions of the mitochondrial networks (Figure 2). When comparing the morphology of individual mitochondria within the IFM and PM subgroups, PM are larger and less branched than IFM (Bakeeva et al., 1978; Picard et al., 2013a,b, 2015; Glancy et al., 2015, 2018; Bleck et al., 2018; Vincent et al., 2019), dedicating more of their volume to cristae and matrix, providing greater structural capacity for energy conversion. Studies in creatine kinase (CK) knockout mice have reported that PM subgroups increase in response to inhibiting the PCr shuttle system suggesting that increasing PM volume may compensate for the loss of the diffusion-mediated energy distribution (Novotova et al., 2006) though these data may simply reflect a fiber type shift toward a more oxidative phenotype. High-resolution imaging of human and mouse muscles have also shown that PM form frequent connections with other mitochondria, but few studies have directly quantified mitochondrial-organelle interactions of PM in skeletal muscle cells (Picard et al., 2013a,b; Bleck et al., 2018). Thus, it is currently unclear how differences in organelle interactivity may contribute to structural mechanisms of mitochondrial specialization.
The functional specificity of PM may also be related to their subcellular location. Historically, PM have been classified as subsarcolemmal mitochondria (SSM) (Muller, 1976; Palmer et al., 1977, 1985; Elander et al., 1985; Cogswell et al., 1993; Manneschi and Federico, 1995; Takahashi and Hood, 1996), but much of the subsarcolemmal space within a muscle cell is densely packed with myofibrils rather than mitochondria (Figure 1). More recent studies (Rothstein et al., 2005; Glancy et al., 2014, 2015, 2018) have demonstrated that peripherally-located subgroups are essentially “paravascular” mitochondria (PVM) or “paranuclear” mitochondria (PNM) that specifically localize to the intracellular space surrounding embedded capillaries and nuclei (Rothstein et al., 2005; Glancy et al., 2014, 2015; Figure 1). These high-resolution 3D imaging studies revealed that capillaries favorably embed into oxidative muscle cells and that 50% of capillaries located near oxidative muscle cells had a large proportion of their circumference (≥50%) embedded into the sarcolemma of muscle cell (Glancy et al., 2014). Thus, PVM subgroups were found predominantly within the more metabolically active muscle cells (oxidative fiber types) and may indeed provide additional metabolic support to the high energy demands generated within the intermyofibrillar regions of these cells (Figure 2). Moreover, clusters of PNM (Rothstein et al., 2005; Glancy et al., 2014) were also found in the intracellular space flanking nuclei, suggesting that PM subgroups may indiscriminately fill in any myofibrillar void caused by the presence of embedded capillaries and nuclei (Glancy et al., 2014). Therefore, the available research suggests that mitochondria subpopulations that have been historically associated with the sarcolemma are actually localized to other cellular structures that displace myofibrils at the periphery of the cell. However, limited studies exist that explicitly delineate the morphological or biochemical differences in mitochondria associated with either capillaries or nuclei, and future work is needed to establish the functional implications of peripheral mitochondrial distribution in muscle cells.
While the primary function of the IFM is somewhat intuitive when considering their intricate spatial relationships with the energy-hungry myofibrils, it is unclear why evolution would favor the separate subgroups of morphologically distinct mitochondria found in clusters near the cell boundary. As noted above, greater mitochondrial volume observed near the cell boundary supports greater energy conversion than the smaller mitochondria residing within the intermyofibrillar spaces, and therefore, some investigators have postulated that PM function to directly support the IFM by generating and distributing energy. As early as the 1960s, Romanul and colleagues found mitochondrial oxidative enzyme activity to be higher in mitochondria located adjacent to capillaries compared to mitochondria within the interior regions of the muscle cell (Romanul, 1964, 1965). Undoubtedly, oxygen is a primary substrate for mitochondrial respiration, and it may be beneficial for mitochondria to crowd the intracellular space near the capillary oxygen supply. However, previous experiments have been limited in their ability to evaluate oxygen kinetics and/or mitochondrial function with subcellular resolution, and therefore, the relationship between PVM function and their proximity to the capillary remains unclear.
To explore the subcellular specialization of mitochondrial function within skeletal muscle cells, investigators have established techniques that aim to specifically isolate intermyofibrillar mitochondria from the total mitochondria pool within muscles. These techniques typically incorporate a tiered purification method that separates mitochondria according to their degree of interactivity with contractile proteins. Mitochondria isolated from homogenized muscle tissues before protease treatment are considered to have less interaction with contractile proteins and are therefore classified as SSM, whereas mitochondria isolated after digestion are classified as IFM. Although this approach has been implemented in many studies and models (Palmer et al., 1977; Krieger et al., 1980; Cogswell et al., 1993), experiments directly comparing the protein composition and function between subgroups of isolated mitochondria have produced conflicting results (Krieger et al., 1980; Elander et al., 1985; Cogswell et al., 1993; Manneschi and Federico, 1995; Takahashi and Hood, 1996; Jimenez et al., 2002; Adhihetty et al., 2005; Mollica et al., 2006). In several early experiments using protease to separately isolate the IFM and SSM subgroups in rodent skeletal muscle, it was determined that IFM have higher mitochondrial respiration enzyme activities and greater capacity to metabolize oxygen compared to SSM (Krieger et al., 1980; Cogswell et al., 1993; Adhihetty et al., 2005). Furthermore, subsequent work found that IFM import fuels, such as malate, at a 3–4-fold greater rate than SSM (Takahashi and Hood, 1996). While these initial observations indicate that mitochondrial metabolic capacity may be impacted by subcellular localization, experiments using isolated IFM and SSM from human muscle biopsies found oxygen consumption rates similar between the groups (Elander et al., 1985; Fischer et al., 1985). Indeed, some aspects of mitochondrial specialization may be influenced by species-specific changes in cellular ultrastructure and physiological demand. For example although PM and IFM subpopulations are consistently observed in humans, mice, and shrews, mitochondria within the shrew oxidative muscle fibers are primarily aligned along the transverse axis of the cell (Chung et al., 2021) whereas oxidative mouse and human muscles display more grid-like mitochondrial networks (Bleck et al., 2018; Caffrey et al., 2019). However, inconsistencies surrounding the function of mitochondrial subpopulations may be more closely related to experimental procedures. Specifically, the procedures used to isolate mitochondria disrupt critical functional aspects of mitochondrial structure, such as mitochondria-mitochondria contact sites. Furthermore, work from Hoppel and others has revealed key limitations to mitochondrial subpopulation isolation techniques (Kras et al., 2016; Lai et al., 2019) and demonstrated that previous preparations of SSM may have been contaminated with membranous proteins that would have resulted in lower measures of mitochondrial energy conversion. Using a revised protocol, the same group demonstrated that the rates of energy conversion and enzymatic activities are consistent between the IFM and SSM subpopulations, except for SSM having a 10% lower oxygen consumption rate when stimulated with saturating ADP concentrations. This reduction in oxygen consumption capacity in the SSM was also associated with lower cytochrome C content, but it is unclear if the difference in the cytochrome profile between these two mitochondria subpopulations is related to the metabolic or apoptotic functions. Indeed, cytochrome C is associated with mitochondrial apoptotic pathways, and earlier studies have suggested that IFM may be more sensitive to apoptotic stimuli while PM may be more inclined to produce ROS or proton leak (Adhihetty et al., 2005).
Despite these methodological concerns surrounding isolated preparations of mitochondrial subgroups, experiments in isolated mitochondria have produced interesting results when evaluating the apoptotic and ROS-generating capacity of mitochondria. For example, Hood and collaborators demonstrated that IFM isolated from the quadriceps muscles of mice are more sensitive to the apoptotic stimulus hydrogen peroxide compared to SSM (Adhihetty et al., 2005). Although IFM released 10-fold the amount of apoptosis-inducing factor upon exposure to hydrogen peroxide, the same work also demonstrated that SSM produced nearly a threefold greater rate of ROS production compared to the IFM (Adhihetty et al., 2005). Moreover, studies looking at proton leak rate and protein expression have found that IFM may express less uncoupling proteins (UCP3) (Jimenez et al., 2002) and be more efficient (less proton leak) than SSM (Iossa et al., 2001; Mollica et al., 2006). These findings suggest that mitochondrial subpopulations differ remarkably in their capacity to handle oxidative stress and may provide insight regarding the distribution of signaling responsibilities within the mitochondrial reticulum. Specifically, the increased capacity of SSM to generate ROS while also being less prone to ROS-induced apoptosis may indicate that the mitochondria residing within these subgroups are uniquely designed to produce ROS as part of a more integrated cell signaling mechanism rather than isolated local apoptotic pathways. Undoubtedly, mitochondria in PM subgroups are in close proximity to embedded capillaries and peripherally-located myonuclei, and therefore, the reported differences in ROS production and thermodynamic driving forces between mitochondrial subgroups may be associated with proximity to a cellular oxygen supply and/or mitochondria-nuclear signaling (Dominy and Puigserver, 2013; Soledad et al., 2019).
More recent imaging studies have brought some clarity to the diversification of mitochondrial function within living cells by using 3D and 4D imaging strategies to directly evaluate mitochondrial respiratory enzymes, mitochondrial redox, and even the rate of mitochondrial energy conversion within an intact network (Schroeder et al., 2010; Glancy et al., 2015; Willingham et al., 2019). Certainly, spatially resolved measurements within intact cells provide far more specificity regarding the subcellular distribution of mitochondrial heterogeneity compared to studies in isolated mitochondria. For example, imaging studies measuring the spatial distribution of mitochondrial enzymes have found that the membrane potential-generating element (complex IV) and the complex responsible for ATP production (complex V) preferentially localize to mitochondria within the peripheral and intermyofibrillar spaces of the muscle cell, respectively. Specifically, the peripheral localization of Complex IV suggests that PM subgroups may support the IFM by generating proton-motive force and distributing it through the IFM network where it is used to synthesize ATP (Figure 2). Moreover, we recently established a metabolic imaging approach to quantitatively assess mitochondrial function and measure redox kinetics with subcellular resolution (Willingham et al., 2019). The application of this technique in living tissues has provided the first spatially-resolved measure of the rate of in vivo mitochondrial energy conversion. Although this work found the energy conversion rate to be consistent across PVM and IFM subpopulations, we found the PVM to be more oxidized than the IFM (Schroeder et al., 2010; Willingham et al., 2019). In keeping with data from isolated mitochondria, these imaging studies demonstrate the functional specialization and differential distribution of thermodynamics across subfractions of the intact mitochondrial reticulum in skeletal muscle cells, but further work is warranted to determine whether subpopulations of muscle mitochondria play distinct roles in other key functions such as Ca2+ signaling, apoptosis, fuel importation, and biosynthesis.
Subpopulations of mitochondria may also be differentially influenced by exercise training and pathologies. For example, over 40 years ago, a series of imaging studies provided evidence that SSM are more responsive to changes in energetic demand than IFM (Hoppeler et al., 1973a; Muller, 1976) by showing that the relative exercise-induced increases in mitochondrial volume were more significant in the SSM compared to the IFM. In human volunteers, Hoppeler et al. (1973b) found PM to be 3.2-fold higher in exercise-trained muscles compared to untrained while IFM was only increased by 30% with training. These findings have been supported by subsequent experiments in isolated mitochondria that demonstrated SSM experience greater increases metabolic capacity with exercise training compared to IFM. For example, Koves et al. (2005) demonstrated that 10 weeks of exercise training increases fatty acid oxidation ∼100% in SSM but only 50% in IFM, indicating that subpopulations of mitochondria differentially adapt to sustain energy homeostasis in the face of metabolic challenge. Although few studies have compared the function of mitochondrial subgroups in models of skeletal muscle pathology, some experiments in isolated mitochondria have also found that SSM may be more susceptible to dysfunction in the presence of metabolic pathology or inactivation (Kras et al., 2018, 2019). While it is difficult to interpret these findings considering the limitations of isolation techniques, these results are consistent with imaging studies demonstrating that PM subgroups are more affected by pathologies associated with obesity and insulin resistance (Ritov et al., 2005; Nielsen et al., 2010; Chomentowski et al., 2011; Lai et al., 2017). Furthermore, obesity and diabetes are also associated with increased accumulation of lipids within the subsarcolemmal space (Nielsen et al., 2010), but it is unclear how changes in muscle lipid content may influence mitochondria-lipid interactivity, and function, of peripherally-located mitochondria. Changes in muscle metabolic capacity may also be regulated by changes in PM and IFM mitochondrial network structures. While the mechanisms that regulate mitochondrial network configuration in skeletal muscle remain unclear, the overall dynamics of mitochondrial morphology are regulated in part by the balance of fusion/fission events and the cytoskeletal framework (Glancy et al., 2020; Glancy and Balaban, 2021), and pathologies and changes in metabolic demand that influence these key regulators may alter muscle metabolism through changes in mitochondrial network ultrastructure (Shah et al., 2019; Liu et al., 2020). Future studies are needed to establish the link between the subcellular specialization of mitochondria and the functional plasticity of skeletal muscle cells and determine how pathology influences the mitochondria-organelle interactome.
Recent advancements in nanoscale imaging technologies have greatly expanded our capacity to investigate subcellular structure, and applications of contemporary techniques have shed new light on mitochondrial biology. Work from Lippincott-Schwartz (Valm et al., 2017), Nunnari (Lewis et al., 2016), and others have used high-resolution live-cell imaging to demonstrate that cellular functions are modulated by a complex network of functional mitochondrial-organelle interactions. In brief, mitochondria form contact sites with the ER, lipid-droplets, vesicles, and the sarcolemma that facilitates a broad range of cellular functions, including metabolism, biosynthesis, apoptosis, and fusion/fission. Although most of the new information regarding functional relationships among subcellular structures has been derived from cell culture models, some studies have found mitochondria-organelle interactions to be associated with functional specialization of mitochondria in the cells of salivary (Porat-Shliom et al., 2019) glands, pancreatic tissues (Johnson et al., 2003), and cardiomyocytes (Lu et al., 2019). While this work suggests that mitochondrial-organelle interactions play key roles in physiological function, further work is needed to characterize the subcellular distribution of mitochondria-organelle interactions in striated muscle cells and determine if differences in organelle interactivity contribute to the specialization of function between the IFM and PM subgroups. Additionally, the in vivo functions of many mitochondria-organelle contact sites have not been determined in striated muscle cells, and it is unclear whether or not the molecular architecture of mitochondria-organelle contact sites within skeletal muscle cells is consistent with those observed in cell cultures and other tissues. Future experiments may address these questions by using high-resolution and live-cell imaging strategies to map organelle connectivity across large subcellular volumes and comparing the functional capabilities between subpopulations of mitochondria under different experimental conditions. As discussed, recent advancements in high-resolution 3D imaging and automated image analysis have greatly expanded our capacity to measure the subcellular distribution of mitochondrial morphology and mitochondria-organelle interactivity, but contemporary nanoscale imaging strategies, such as EM, are still limited in their ability to provide molecular and functional information. Therefore, answering the many questions that remain surrounding the subcellular specialization of mitochondrial form and function in skeletal muscle cells will require innovative imaging strategies that evaluate mitochondrial function, composition, and structure at the subcellular level with molecular specificity.
All authors listed have made a substantial, direct and intellectual contribution to the work, and approved it for publication.
This work was funded by the Division of Intramural Research of the National Heart Lung, and Blood Institute and the Intramural Research Program of the National Institute of Arthritis and Musculoskeletal and Skin Diseases (ZIAHL006221-02).
The authors declare that the research was conducted in the absence of any commercial or financial relationships that could be construed as a potential conflict of interest.
All claims expressed in this article are solely those of the authors and do not necessarily represent those of their affiliated organizations, or those of the publisher, the editors and the reviewers. Any product that may be evaluated in this article, or claim that may be made by its manufacturer, is not guaranteed or endorsed by the publisher.
Adhihetty, P. J., Ljubicic, V., Menzies, K. J., and Hood, D. A. (2005). Differential susceptibility of subsarcolemmal and intermyofibrillar mitochondria to apoptotic stimuli. Am. J. Physiol. Cell Physiol. 289, C994–C1001. doi: 10.1152/ajpcell.00031.2005
Bakeeva, L. E., Chentsov Yu, S., and Skulachev, V. P. (1978). Mitochondrial framework (reticulum mitochondriale) in rat diaphragm muscle. Biochim. Biophys. Acta 501, 349–369.
Benador, I. Y., Veliova, M., Mahdaviani, K., Petcherski, A., Wikstrom, J. D., Assali, E. A., et al. (2018). Mitochondria bound to lipid droplets have unique bioenergetics, composition, and dynamics that support lipid droplet expansion. Cell Metab. 27, 869–885.e6. doi: 10.1016/j.cmet.2018.03.003 869-885 e866,
Bessman, S. P., and Geiger, P. J. (1981). Transport of energy in muscle: the phosphorylcreatine shuttle. Science 211, 448–452. doi: 10.1126/science.6450446
Bleck, C. K. E., Kim, Y., Willingham, T. B., and Glancy, B. (2018). Subcellular connectomic analyses of energy networks in striated muscle. Nat. Commun. 9:5111.
Bubenzer, H. J. (1966). [The thin and the thick muscular fibers of the rat diaphragm]. Z. Zellforsch Mikrosk Anat. 69, 520–550.
Caffrey, B. J., Maltsev, A. V., Gonzalez-Freire, M., Hartnell, L. M., Ferrucci, L., and Subramaniam, S. (2019). Semi-automated 3D segmentation of human skeletal muscle using focused ion beam-scanning electron microscopic images. J. Struct. Biol. 207, 1–11.
Chomentowski, P., Coen, P. M., Radikova, Z., Goodpaster, B. H., and Toledo, F. G. (2011). Skeletal muscle mitochondria in insulin resistance: differences in intermyofibrillar versus subsarcolemmal subpopulations and relationship to metabolic flexibility. J. Clin. Endocrinol. Metab. 96, 494–503. doi: 10.1210/jc.2010-0822
Chung, D. J., Madison, G. P., Aponte, A. M., Singh, K., Li, Y., Pirooznia, M., et al. (2021). Metabolic design in a model of extreme mammalian metabolism, the North American least shrew (Cryptotis parva). bioRxiv[Preprint] doi: 10.1101/2021.05.28.446190
Cogswell, A. M., Stevens, R. J., and Hood, D. A. (1993). Properties of skeletal muscle mitochondria isolated from subsarcolemmal and intermyofibrillar regions. Am. J. Physiol. 264, C383–C389.
Dominy, J. E., and Puigserver, P. (2013). Mitochondrial biogenesis through activation of nuclear signaling proteins. Cold Spring Harb. Perspect. Biol. 5:a015008. doi: 10.1101/cshperspect.a015008
Eisner, V., Csordas, G., and Hajnoczky, G. (2013). Interactions between sarco-endoplasmic reticulum and mitochondria in cardiac and skeletal muscle – pivotal roles in Ca(2)(+) and reactive oxygen species signaling. J. Cell Sci. 126, 2965–2978. doi: 10.1242/jcs.093609
Eisner, V., Lenaers, G., and Hajnoczky, G. (2014). Mitochondrial fusion is frequent in skeletal muscle and supports excitation-contraction coupling. J. Cell Biol. 205, 179–195. doi: 10.1083/jcb.201312066
Elander, A., Sjostrom, M., Lundgren, F., Schersten, T., and Bylund-Fellenius, A. C. (1985). Biochemical and morphometric properties of mitochondrial populations in human muscle fibres. Clin. Sci. (Lond.). 69, 153–164. doi: 10.1042/cs0690153
Fischer, J. C., Ruitenbeek, W., Stadhouders, A. M., Trijbels, J. M., Sengers, R. C., Janssen, A. J., et al. (1985). Investigation of mitochondrial metabolism in small human skeletal muscle biopsy specimens. Improvement of preparation procedure. Clin. Chim. Acta 145, 89–99. doi: 10.1016/0009-8981(85)90022-1
Gauthier, G. F., and Padykula, H. A. (1966). Cytological studies of fiber types in skeletal muscle. A comparative study of the mammalian diaphragm. J. Cell Biol. 28, 333–354. doi: 10.1083/jcb.28.2.333
Glancy, B., and Balaban, R. S. (2021). Energy metabolism design of the striated muscle cell. Physiol. Rev. 101, 1561–1607. doi: 10.1152/physrev.00040.2020
Glancy, B., Hartnell, L. M., Combs, C. A., Femnou, A., Sun, J., Murphy, E., et al. (2018). Power grid protection of the muscle mitochondrial reticulum. Cell Rep. 23:2832. doi: 10.1016/j.celrep.2018.05.055
Glancy, B., Hartnell, L. M., Malide, D., Yu, Z. X., Combs, C. A., Connelly, P. S., et al. (2015). Mitochondrial reticulum for cellular energy distribution in muscle. Nature 523, 617–620. doi: 10.1038/nature14614
Glancy, B., Hsu, L. Y., Dao, L., Bakalar, M., French, S., Chess, D. J., et al. (2014). In vivo microscopy reveals extensive embedding of capillaries within the sarcolemma of skeletal muscle fibers. Microcirculation 21, 131–147. doi: 10.1111/micc.12098
Glancy, B., Kim, Y., Katti, P., and Willingham, T. B. (2020). The functional impact of mitochondrial structure across subcellular scales. Front. Physiol. 11:541040. doi: 10.3389/fphys.2020.541040
Holloszy, J. O. (1967). Biochemical adaptations in muscle. Effects of exercise on mitochondrial oxygen uptake and respiratory enzyme activity in skeletal muscle. J. Biol. Chem. 242, 2278–2282.
Hoppeler, H., Luthi, P., Claassen, H., Weibel, E. R., and Howald, H. (1973b). [Ultrastructure of normal human skeletal muscle; a morphometric analysis in controls and men trained in long-distance running]. Hoppe–Seyler’s Zeitschrift Physiol. Chem. 354, 229–230.
Hoppeler, H., Luthi, P., Claassen, H., Weibel, E. R., and Howald, H. (1973a). The ultrastructure of the normal human skeletal muscle. A morphometric analysis on untrained men, women and well–trained orienteers. Pflugers Arch. 344, 217–232.
Iossa, S., Lionetti, L., Mollica, M. P., Crescenzo, R., Botta, M., Samec, S., et al. (2001). Differences in proton leak kinetics, but not in UCP3 protein content, in subsarcolemmal and intermyofibrillar skeletal muscle mitochondria from fed and fasted rats. FEBS Lett. 505, 53–56. doi: 10.1016/s0014-5793(01)02772-7
Jimenez, M., Yvon, C., Lehr, L., Léger, B., Keller, P., Russell, A., et al. (2002). Expression of uncoupling protein-3 in subsarcolemmal and intermyofibrillar mitochondria of various mouse muscle types and its modulation by fasting. Eur. J. Biochem. 269, 2878–2884.
Johnson, D. T., Harris, R. A., French, S., Blair, P. V., You, J., Bemis, K. G., et al. (2007). Tissue heterogeneity of the mammalian mitochondrial proteome. Am. J. Physiol. Cell Physiol. 292, C689–C697. doi: 10.1152/ajpcell.00108.2006
Johnson, P. R., Dolman, N. J., Pope, M., Vaillant, C., Petersen, O. H., Tepikin, A. V., et al. (2003). Non-uniform distribution of mitochondria in pancreatic acinar cells. Cell Tissue Res. 313, 37–45. doi: 10.1007/s00441-003-0741-1
Koves, T. R., Noland, R. C., Bates, A. L., Henes, S. T., Muoio, D. M., and Cortright, R. N. (2005). Subsarcolemmal and intermyofibrillar mitochondria play distinct roles in regulating skeletal muscle fatty acid metabolism. Am. J. Physiol. Cell Physiol. 288, C1074–C1082. doi: 10.1152/ajpcell.00391.2004
Kras, K. A., Hoffman, N., Roust, L. R., Benjamin, T. R., De Filippis, E. A., and Katsanos, C. S. (2019). Adenosine triphosphate production of muscle mitochondria after acute exercise in lean and obese humans. Med. Sci. Sports Exerc. 51, 445–453. doi: 10.1249/MSS.0000000000001812
Kras, K. A., Langlais, P. R., Hoffman, N., Roust, L. R., Benjamin, T. R., De Filippis, E. A., et al. (2018). Obesity modifies the stoichiometry of mitochondrial proteins in a way that is distinct to the subcellular localization of the mitochondria in skeletal muscle. Metabolism 89, 18–26. doi: 10.1016/j.metabol.2018.09.006
Kras, K. A., Willis, W. T., Barker, N., Czyzyk, T., Langlais, P. R., and Katsanos, C. S. (2016). Subsarcolemmal mitochondria isolated with the proteolytic enzyme nagarse exhibit greater protein specific activities and functional coupling. Biochem. Biophys. Rep. 6, 101–107. doi: 10.1016/j.bbrep.2016.03.006
Krieger, D. A., Tate, C. A., McMillin-Wood, J., and Booth, F. W. (1980). Populations of rat skeletal muscle mitochondria after exercise and immobilization. J. Appl. Physiol. Respir. Environ. Exerc. Physiol. 48, 23–28. doi: 10.1152/jappl.1980.48.1.23
Lai, N., Kummitha, C., and Hoppel, C. (2017). Defects in skeletal muscle subsarcolemmal mitochondria in a non-obese model of type 2 diabetes mellitus. PLoS One 12:e0183978. doi: 10.1371/journal.pone.0183978
Lai, N., Kummitha, C., Rosca, M. G., Fujioka, H., Tandler, B., and Hoppel, C. L. (2019). Isolation of mitochondrial subpopulations from skeletal muscle: optimizing recovery and preserving integrity. Acta Physiol. 225, e13182.
Lewis, S. C., Uchiyama, L. F., and Nunnari, J. (2016). ER-mitochondria contacts couple mtDNA synthesis with mitochondrial division in human cells. Science 353:aaf5549. doi: 10.1126/science.aaf5549
Liu, Y. J., McIntyre, R. L., Janssens, G. E., and Houtkooper, R. H. (2020). Mitochondrial fission and fusion: a dynamic role in aging and potential target for age-related disease. Mech. Ageing Dev. 186:111212. doi: 10.1016/j.mad.2020.111212
Lu, X., Thai, P. N., Lu, S., Pu, J., and Bers, D. M. (2019). Intrafibrillar and perinuclear mitochondrial heterogeneity in adult cardiac myocytes. J. Mol. Cell Cardiol. 136, 72–84. doi: 10.1016/j.yjmcc.2019.08.013
Luo, G., Yi, J., Ma, C., Xiao, Y., Yi, F., Yu, T., et al. (2013). Defective mitochondrial dynamics is an early event in skeletal muscle of an amyotrophic lateral sclerosis mouse model. PLoS One 8:e82112. doi: 10.1371/journal.pone.0082112
Lygate, C. A., Aksentijevic, D., Dawson, D., ten Hove, M., Phillips, D., de Bono, J. P., et al. (2013). Living without creatine: unchanged exercise capacity and response to chronic myocardial infarction in creatine-deficient mice. Circ. Res. 112, 945–955. doi: 10.1161/CIRCRESAHA.112.300725
Manneschi, L., and Federico, A. (1995). Polarographic analyses of subsarcolemmal and intermyofibrillar mitochondria from rat skeletal and cardiac muscle. J. Neurol. Sci. 128, 151–156. doi: 10.1016/0022-510x(94)00227-f
Mollica, M. P., Lionetti, L., Crescenzo, R., D’Andrea, E., Ferraro, M., Liverini, G., et al. (2006). Heterogeneous bioenergetic behaviour of subsarcolemmal and intermyofibrillar mitochondria in fed and fasted rats. Cell. Mol. Life Sci. 63, 358–366. doi: 10.1007/s00018-005-5443-2
Muller, W. (1976). Subsarcolemmal mitochondria and capillarization of soleus muscle fibers in young rats subjected to an endurance training. A morphometric study of semithin sections. Cell Tissue Res. 174, 367–389. doi: 10.1007/BF00220682
Nielsen, J., Mogensen, M., Vind, B. F., Sahlin, K., Højlund, K., Schrøder, H. D., et al. (2010). Increased subsarcolemmal lipids in type 2 diabetes: effect of training on localization of lipids, mitochondria, and glycogen in sedentary human skeletal muscle. Am. J. Physiol. Endocrinol. Metab. 298, E706–E713. doi: 10.1152/ajpendo.00692.2009
Novotova, M., Pavlovicova, M., Veksler, V. I., Ventura-Clapier, R., and Zahradnik, I. (2006). Ultrastructural remodeling of fast skeletal muscle fibers induced by invalidation of creatine kinase. Am. J. Physiol. Cell Physiol. 291, C1279–C1285. doi: 10.1152/ajpcell.00114.2006
Ørtenblad, N., Nielsen, J., Boushel, R., Söderlund, K., Saltin, B., and Holmberg, H. C. (2018). The muscle fiber profiles, mitochondrial content, and enzyme activities of the exceptionally well-trained arm and leg muscles of elite cross-country skiers. Front. Physiol. 9:1031. doi: 10.3389/fphys.2018.01031
Palmer, J. W., Tandler, B., and Hoppel, C. L. (1977). Biochemical properties of subsarcolemmal and interfibrillar mitochondria isolated from rat cardiac muscle. J. Biol. Chem. 252, 8731–8739.
Palmer, J. W., Tandler, B., and Hoppel, C. L. (1985). Biochemical differences between subsarcolemmal and interfibrillar mitochondria from rat cardiac muscle: effects of procedural manipulations. Arch. Biochem. Biophys. 236, 691–702. doi: 10.1016/0003-9861(85)90675-7
Picard, M., Gentil, B. J., McManus, M. J., White, K., St, Louis K, Gartside, S. E., et al. (2013a). Acute exercise remodels mitochondrial membrane interactions in mouse skeletal muscle. J. Appl. Physiol. 115, 1562–1571. doi: 10.1152/japplphysiol.00819.2013
Picard, M., McManus, M. J., Csordas, G., Varnai, P., Dorn, G. W. II, Williams, D., et al. (2015). Trans-mitochondrial coordination of cristae at regulated membrane junctions. Nat. Commun. 6:6259. doi: 10.1038/ncomms7259
Picard, M., White, K., and Turnbull, D. M. (2013b). Mitochondrial morphology, topology, and membrane interactions in skeletal muscle: a quantitative three-dimensional electron microscopy study. J. Appl. Physiol. (1985) 114, 161–171. doi: 10.1152/japplphysiol.01096.2012
Porat-Shliom, N., Harding, O. J., Malec, L., Narayan, K., and Weigert, R. (2019). Mitochondrial Populations exhibit differential dynamic responses to increased energy demand during exocytosis in vivo. iScience 11, 440–449. doi: 10.1016/j.isci.2018.12.036
Ritov, V. B., Menshikova, E. V., He, J., Ferrell, R. E., Goodpaster, B. H., and Kelley, D. E. (2005). Deficiency of subsarcolemmal mitochondria in obesity and type 2 diabetes. Diabetes 54, 8–14.
Romanul, F. C. (1964). Distribution of capillaries in relation to oxidative metabolism of skeletal muscle fibres. Nature 201, 307–308. doi: 10.1038/201307a0
Romanul, F. C. (1965). Capillary supply and metabolism of muscle fibers. Arch. Neurol. 12, 497–509. doi: 10.1001/archneur.1965.00460290053007
Rothstein, E. C., Carroll, S., Combs, C. A., Jobsis, P. D., and Balaban, R. S. (2005). Skeletal muscle NAD(P)H two-photon fluorescence microscopy in vivo: topology and optical inner filters. Biophys. J. 88, 2165–2176.
Schroeder, J. L., Luger-Hamer, M., Pursley, R., Pohida, T., Chefd’hotel, C., Kellman, P., et al. (2010). Short communication: subcellular motion compensation for minimally invasive microscopy, in vivo: evidence for oxygen gradients in resting muscle. Circ. Res. 106, 1129–1133. doi: 10.1161/CIRCRESAHA.109.211946
Schwerzmann, K., Hoppeler, H., Kayar, S. R., and Weibel, E. R. (1989). Oxidative capacity of muscle and mitochondria: correlation of physiological, biochemical, and morphometric characteristics. Proc. Natl. Acad. Sci. U. S. A. 86, 1583–1587. doi: 10.1073/pnas.86.5.1583
Shah, S. I, Paine, J. G., Perez, C., and Ullah, G. (2019). Mitochondrial fragmentation and network architecture in degenerative diseases. PLoS One 14:e0223014. doi: 10.1371/journal.pone.0223014
Soledad, R. B., Charles, S., and Samarjit, D. (2019). The secret messages between mitochondria and nucleus in muscle cell biology. Arch. Biochem. Biophys. 666, 52–62. doi: 10.1016/j.abb.2019.03.019
Takahashi, M., and Hood, D. A. (1996). Protein import into subsarcolemmal and intermyofibrillar skeletal muscle mitochondria. Differential import regulation in distinct subcellular regions. J. Biol. Chem. 271, 27285–27291.
Tubbs, E., Chanon, S., Robert, M., Bendridi, N., Bidaux, G., Chauvin, M. A., et al. (2018). Disruption of mitochondria-associated endoplasmic reticulum membrane (MAM) integrity contributes to muscle insulin resistance in mice and humans. Diabetes 67, 636–650. doi: 10.2337/db17-0316
Valm, A. M., Cohen, S., Legant, W. R., Melunis, J., Hershberg, U., Wait, E., et al. (2017). Applying systems-level spectral imaging and analysis to reveal the organelle interactome. Nature 546, 162–167. doi: 10.1038/nature22369
van Deursen, J., Heerschap, A., Oerlemans, F., Ruitenbeek, W., Jap, P., ter Laak, H., et al. (1993). Skeletal muscles of mice deficient in muscle creatine kinase lack burst activity. Cell 74, 621–631. doi: 10.1016/0092-8674(93)90510-w
Vincent, A. E., White, K., Davey, T., Philips, J., Ogden, R. T., Lawless, C., et al. (2019). Quantitative 3D mapping of the human skeletal muscle mitochondrial network. Cell Rep. 27:321. doi: 10.1016/j.celrep.2019.03.051
Willingham, T. B., Kim, Y., Lindberg, E., Bleck, C. K. E., and Glancy, B. (2020). The unified myofibrillar matrix for force generation in muscle. Nat. Commun. 11:3722. doi: 10.1038/s41467-020-17579-6
Willingham, T. B., Zhang, Y., Andreoni, A., Knutson, J. R., Lee, D. Y., and Glancy, B. (2019). MitoRACE: evaluating mitochondrial function in vivo and in single cells with subcellular resolution using multiphoton NADH autofluorescence. J. Physiol. 597, 5411–5428. doi: 10.1113/JP278611
Keywords: paravascular mitochondria, subsarcolemmal mitochondria, intermyofibrillar mitochondria, organelle interactions, paranuclear mitochondria, mitochondrial respiration, bioenergetics, mitochondrial connectivity
Citation: Willingham TB, Ajayi PT and Glancy B (2021) Subcellular Specialization of Mitochondrial Form and Function in Skeletal Muscle Cells. Front. Cell Dev. Biol. 9:757305. doi: 10.3389/fcell.2021.757305
Received: 12 August 2021; Accepted: 27 September 2021;
Published: 15 October 2021.
Edited by:
Jyoti K. Jaiswal, Children’s National Hospital, United StatesReviewed by:
Jingsong Zhou, University of Texas at Arlington, United StatesCopyright © 2021 Willingham, Ajayi and Glancy. This is an open-access article distributed under the terms of the Creative Commons Attribution License (CC BY). The use, distribution or reproduction in other forums is permitted, provided the original author(s) and the copyright owner(s) are credited and that the original publication in this journal is cited, in accordance with accepted academic practice. No use, distribution or reproduction is permitted which does not comply with these terms.
*Correspondence: T. Bradley Willingham, YnJhZHd3QGljbG91ZC5jb20=
Disclaimer: All claims expressed in this article are solely those of the authors and do not necessarily represent those of their affiliated organizations, or those of the publisher, the editors and the reviewers. Any product that may be evaluated in this article or claim that may be made by its manufacturer is not guaranteed or endorsed by the publisher.
Research integrity at Frontiers
Learn more about the work of our research integrity team to safeguard the quality of each article we publish.