- 1Department of Molecular Science and Technology, Ajou University, Suwon, South Korea
- 2S&K Therapeutics, Suwon, South Korea
Toll-like receptors (TLRs) are the pattern recognition receptors, which are activated by foreign and host molecules in order to initiate the immune response. They play a crucial role in the regulation of innate immunity, and several studies have shown their importance in bacterial, viral, and fungal infections, autoimmune diseases, and cancers. The consensus view from an immunological perspective is that TLR agonists can serve either as a possible therapeutic agent or as a vaccine adjuvant toward cancers or infectious diseases and that TLR inhibitors may be a promising approach to the treatment of autoimmune diseases, some cancers, bacterial, and viral infections. These notions are based on the fact that TLR agonists stimulate the secretion of proinflammatory cytokines and in general, the development of proinflammatory responses. Some of the TLR-based inhibitory agents have shown to be efficacious in preclinical models and have now entered clinical trials. Therefore, TLRs seem to hold the potential to serve as a perfect target in the era of immunotherapies. We offer a perspective on TLR-based therapeutics that sheds light on their usefulness and on combination therapies. We also highlight various therapeutics that are in the discovery phase or in clinical trials.
Introduction
Infectious diseases and immune disorders have presented a substantial impediment to the growth, stability, and progress of all civilizations for centuries. Efforts to prevent or eradicate such diseases are arguably the most significant benefit that society has reaped from the contemporary era of biomedical sciences. For combatting various disorders and infections, it is crucial to gain a deeper understanding of how immune responses are triggered and modulated. In this regard, Toll-like receptors (TLRs) have emerged as a major topic of biomedical studies, because this family of proteins acts as one of the early determinants of immune activation (Fitzgerald and Kagan, 2020).
Toll-like receptors are type I transmembrane proteins containing conserved motifs known as the leucine-rich repeat and Toll/interleukin-1 receptor (TIR) domain (Nie et al., 2018). TLRs fall under the category of pattern-recognition receptors (PRRs) of the host defense system. PRRs recognize specific molecular patterns in microbes known as microbe-associated molecular patterns (MAMPs), pathogen-associated molecular patterns (PAMPs) as well as molecules released by a damaged cell of the host, which are known as damage-associated molecular patterns (DAMPs) (Vijay, 2018). Thus, PRRs participate in the development of an immune response against pathogens and help with the self-healing process of the cell. The other types of PRRs include Nod-like receptors, C-type lectin receptors, and RIG-like receptors (Takeuchi and Akira, 2010). TLRs were first identified in Drosophila embryos. Further studies have revealed that these receptors are involved in the development of antifungal responses in adult flies. Later, it was discovered that TLRs are also present in mammals. This finding has led to an understanding of a crucial part of the mechanism behind innate-immune-response development (Takeda and Akira, 2015).
Toll-like receptor recognition of microbial invaders activates a downstream signaling cascade that produces secreted cytokines and chemokines, which then activates both the innate and adaptive immune responses to clean infections (Shi et al., 2016). TLRs are present both on immune cells, including monocytes, macrophages, dendritic cells, neutrophils, B cells, T cells, mast cells, natural killer cells, and on non-immune cells, including fibroblasts, epithelial cells, astrocytes, keratinocytes, and platelets (Ospelt and Gay, 2010; Cognasse et al., 2015; Aletaha et al., 2017). TLRs are also presented by various tumor cells and their activation can promote or reduce the tumor growth. High level of TLRs has been found to be associated in disease aggressiveness, poor treatment outcome and development of therapeutic resistance in the cancer cells. Similarly in case of viral diseases, both TLR agonists and antagonists are used for the development of anti-viral state in the body (Muccioli and Benencia, 2014). Bacterial and autoimmune diseases are being treated with the help of TLR antagonists (Knapp, 2010; Hosseini et al., 2015). We discuss the role of TLRs in infectious diseases, cancers, and autoimmune diseases and therapeutic regimens which involve immune system modulation. Moreover, this review highlights the challenges in the discovery of TLR-based immunotherapies.
Toll-Like Receptor Family: Phylogeny and Structure
Structurally, TLRs are remarkably conserved between humans and mice. To date, 13 TLRs have been identified. Humans have 11, whereas murine species have 13 TLRs (Yang et al., 2017). Humans do not have TLRs 11, 12, and 13, while some other species possess these TLRs. TLR10 is not expressed and TLR8 is not functional in mice (Huang et al., 2008). TLRs are homodimeric or heterodimeric transmembrane proteins that are expressed on the cell surface (TLRs 1, 2, 5, and 6), endosomal surface (TLRs 3, 7, 8, and 9), or both (TLR4) (Shekarian et al., 2017).
On the basis of phylogenetic analysis, human TLRs can be categorized into three subfamilies. The first subfamily consists of TLRs 1, 2, 6, and 10. The second subfamily is composed of TLRs 4 and 5, whereas the third one consists of TLRs 3, 7, 8, and 9. TLR subfamilies are classified based on the cellular localization. Subfamilies 1 and 2 are localized on the cell membrane. However, the subfamily 3 is localized in endoplasmic reticulum, which is trafficked to endosome upon activation (Lai et al., 2017).
Domain organization is similar among all TLRs because each TLR consists of a type 1 transmembrane protein. The ectodomain is composed of 16–28 leucine-rich repeats, while the conserved cytosolic signaling domain is known as the TIR domain, which activates TLR-specific adaptor proteins (Braunstein et al., 2018). Ectodomain leucine-rich repeats mediate the recognition of PAMPs (Kawai and Akira, 2011). The ectodomain is sandwiched between the N- and C-terminal domains. Both domains are joined by a single transmembrane helix (Matsushima et al., 2007). The TIR domain is present in the C-terminal region of mammalian TLRs. It is an important molecule in downstream signaling of the immune cascade. It recruits signaling adaptor proteins that are responsible for further downstream signal transduction necessary for an appropriate innate immune response (Ve et al., 2017).
The Toll-Like Receptor Signaling Pathway
Upon stimulation, TLRs act via different signaling mechanisms, which induce the synthesis of various cytokines (Fitzgerald and Kagan, 2020). TLR signaling is initiated by the interaction between a TLR and ligand, leading to receptor dimerization. TIR domain–containing adaptor proteins (TIRAPs) are recruited by the TIR domain. There are five TLR adaptor proteins: MyD88 (myeloid differentiation primary-response gene 88), MAL (MyD88 adaptor-like protein), TRIF (TIR domain-containing adaptor protein inducing interferon-β (IFN-β), and TRAM (TRIF-related adaptor molecule) (O’Neill and Bowie, 2007).
Toll-Like receptors function in either MyD88-dependent or MyD88-independent pathways. Upon TLR activation, MyD88 and TRIF form myddosomes and triffosomes, respectively and initiate further signaling by recruiting kinases and downstream signaling molecules (Gay, 2019). In case of myddosome formation, IL-1R-associated kinases 1/4 (IRAK1/4) associates with TNF receptor–associated factor 6 (TRAF6), TRAF6 promotes polyubiquitination of both TRAF6 itself and the TAK1 protein kinase complex. TAK1 then activates two different pathways that lead to activation of the IKK complex-NF-κB pathway and -MAPK pathway. The IKK complex is composed of the catalytic subunits IKKα, IKKβ, and IKKγ (Israël, 2010). TAK1 activates IKKβ. The IKK complex phosphorylates the NF-κB inhibitory protein IκBα, which undergoes proteasome degradation, allowing NF-κB to translocate into the nucleus to induce proinflammatory gene expression. TAK1 activation prompt activation of mitogen-activated protein kinases (MAPK) which leads to recruitment of cyclic AMP-responsive element-binding protein (CREB), and activator protein 1 (AP-1) family transcription factors or stabilization of mRNA to regulate inflammatory responses (Kawasaki and Kawai, 2014; Achek et al., 2016). TLR3, which is triggered by double-stranded RNA, recruits the adaptor protein TRIF, which activates TRAF3 and IRF3. These two events induce type 1 and type 3 interferon secretion. Interferons are very important for efficient generation of antiviral responses. In TLR7, TLR8, and TLR9 signaling cascades, MyD88 activation leads to expression of genes of proinflammatory cytokines and type I and type III IFN through TRAF6 and interferon regulatory transcription factor (IRF) and NF-κB activation (Takeuchi and Akira, 2007; Lester and Li, 2014). TLRs also direct the adaptive immune response toward the T helper 1 phenotype by causing dendritic cells and macrophages to produce IL-12. In this way, a TLR(s) not only initiates an immune response against a pathogen, such as a bacterium, virus, or fungus, but also serves as a bridge between innate and adaptive immune systems (Huang et al., 2008).
Toll-like receptor 4, however, is unique in terms of MyD88 signaling. TLR4 utilizes MyD88-dependent and TRIF-dependent pathways for signal transduction upon engagement by PAMP as presented in Figure 1 (O’Neill et al., 2013). In resting state, TLR4 is present on the plasma membrane and Golgi apparatus. Myeloid Differentiation factor 2 (MD-2), a secreted glycoprotein also known as LY96, is crucial for the translocation of TLR4 from Golgi apparatus to plasma membrane. Upon activation by LPS, TLR4 activates NF-κB via MAL and MyD88 complex formation. Then TLR4 translocate to endosome in a clathrin and dynamin dependent manner and binds to TRAM and TRIF, which results in the activation of IRF3 pathway. Thus, TLR4 activation is induced by LPS through TRIF and MyD88 pathways. However, transport to endosome limits MyD88 activity and enhances TRIF activity (McGettrick and O’Neill, 2010; Cheng et al., 2015). When LPS interacts with TLR4, different cofactors including MD2, LPS binding protein (LBP), and CD14 are involved in the activation of TLR4. CD14 recognizes the complex of TLR4 and LBP, CD14 binds to LBP and is involved in delivering the LPS-LBP complex to the TLR4-MD2 complex, localized on the plasma membrane (Yang et al., 2016).
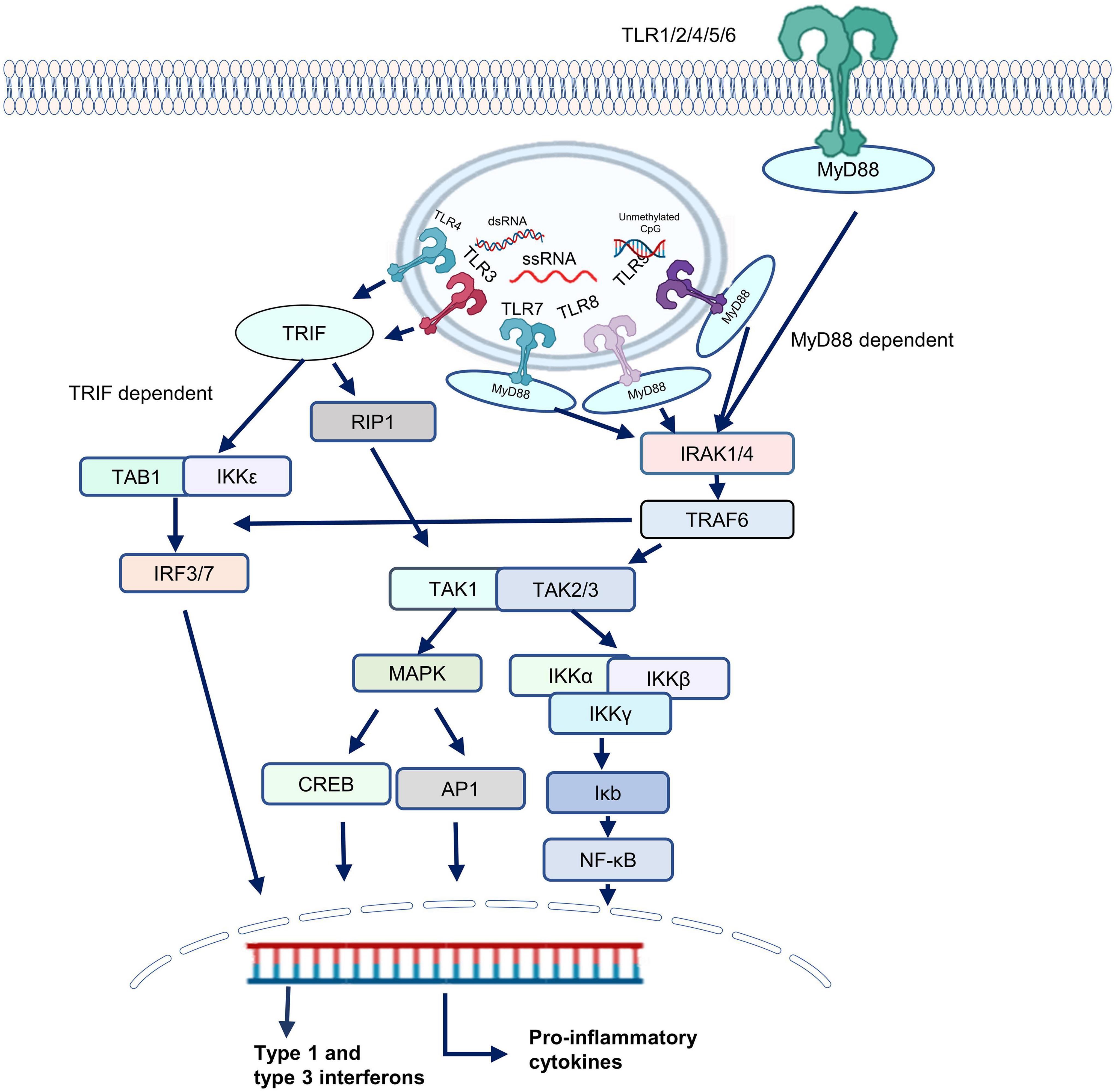
Figure 1. The Toll-like receptor (TLR) signaling pathway. TLRs are categorized into two groups depending upon whether they utilize a MyD88-dependent pathway or a TRIF-dependent pathway. TLRs 1,2, and 5–9 transmit signals through a MyD88-dependent pathway, whereas TLR3 signaling is mediated by a MyD88-independent pathway. By contrast, TLR4 can signal through MyD88-dependent and MyD88-independent pathways. In case of MyD88-dependent signaling, IL-1R-associated kinases 1/4 (IRAK1/4) recruit TNF receptor–associated factor 6 (TRAF6). TRAF6 can also stimulate interferon signaling by recruitment of IRFs. TRAF6 activates TAK1 which activates two distinct pathways, one of which leads to the activation of the IKK complex-NF-κB pathway and the other to the activation of the mitogen activated protein kinases (MAPKs). The catalytic subunits IKKα, IKKβ, and IKKγ make up the IKK complex. IKKβ is also activated by TAK1. The IKK complex phosphorylates the NF-κB inhibitory protein IκBα, which is then degraded by the proteasome, allowing NF-κB to enter the nucleus. TAK1 activation triggers the activation of MAPK family members leads to the activation of transcription factors such cyclic AMP-responsive element-binding protein (CREB), and activator protein 1 (AP-1). CREB, AP-1 and NF-κB then stimulate proinflammatory mediators and interferons. However, in case of TRIF-dependent signaling, IRF3 and IRF7 are recruited which lead to secretion of type 1 and type 3 interferon.
Toll-Like Receptors in Bacterial and Fungal Diseases
Toll-Like Receptors Activation by Bacteria
Bacterial infections have always been a havoc for humanity and the effect has been amplified due to the multi-drug resistant bacterial strains. Infections caused by methicillin resistant Staphylococcus aureus, Pseudomonas aeruginosa, Acinetobacter species, and other pan resistant bacteria are still substantial burden to healthcare setups (Christou, 2011). It is speculated that the cell wall components of bacteria are recognized by the cell surface TLRs whereas the genomic components are recognized by the endosomal TLRs (Wagner, 2004). Activation of TLRs through bacterial ligands lead to development of antimicrobial response by activating production of pro-inflammatory cytokines as well as chemokines (Casilag et al., 2020). Among the TLRs found in mammals, TLR2, TLR4, TLR5, and TLR9 are reported to be stimulated by bacterial ligands. There is a panel of bacterial ligands which activate TLR2. It has been reported that Diacyl lipopeptides, (MALP-2/FSL-1) lipomannan, lipoarabinomannan, and lipoprotein from Mycoplasma, heat-labile enterotoxins from Escherichia coli and Vibrio cholerae, lipoteichoic acid from gram-positive bacteria, peptidoglycan from Staphylococcus, triacyl lipopeptides from bacteria, and porins from Neisseria, Salmonella, and Shigella serve as TLR2 microbial ligands (de Oliviera Nascimento et al., 2012).TLR4 recognizes LPS which is a component of gram-negative bacteria and leads to production of inflammatory cytokines, such as TNF-α, IL6, chemokines monocyte chemoattractant protein-1 (MCP-1), and C-X-C motif chemokine 10 (CXCL10) (Park and Lee, 2013; Yu et al., 2021). It has been reported that CD14 and MD-2 mediates the interaction between LPS and TLR4 as no direct interaction between LPS and TLR4 has been reported (Kawai and Akira, 2009; Płóciennikowska et al., 2015).
Utilization of Bacterial Components as Vaccine Adjuvants and Therapeutic Regimens
The fact that TLRs are activated by bacterial cellular components points out toward the possibility of utilizing these ligands as vaccine adjuvants. Owing to potential to stimulate the appropriate immune response, various bacterial proteins have been proposed to be used as adjuvants against bacterial diseases (Kumar et al., 2019). Casilag et al. (2021) reported that for Streptococcus pneumoniae infection, outcome can be imporved by using the antibiotic in combination with TLR4 agonist. When amoxicillin was administered with monophosphoryl lipid A, it was observed that the antibiotic worked synergistically with the TLR agonist (Casilag et al., 2021). As bacterial DNA contains unmethylated CpG motifs, it activates TLR9 when the bacteria is internalized in the endosomal compartment (Gäbele et al., 2008). It has been reported that TLR9 served as a crucial receptor involved in the induction of type 1 interferons when infected with Staphylococcus aureus (Parker and Prince, 2012).
Multiple TLR4 antagonists have been proposed as therapeutic regimen against septic shock (Nijland et al., 2014). Eritoran is a synthetic lipid A molecule which inhibits the interaction between LPS and TLR4-MD2 (Opal et al., 2013; Kuzmich et al., 2017). It was tested in phase III trials, where it failed to show any improvement in the experimental group as compared to the control group (NCT00334828). Oversights in research design, patient population variances, improved patient care practices, and mixed bacterial infections are among the possible causes for Eritoran’s failure (Anwar et al., 2019). TAK-242, a well-known inhibitor of TLR4, could neither show any reduction in the cytokine levels nor any improvement in the patients when tested in clinical trials phase III (Gabarin et al., 2021). Strategy of inhibition of pro-inflammatory cytokines like IL6, TNF-α, and IL-1β has also failed against sepsis in clinical trials (Barratt-Due et al., 2017).
Nucleic acids released from the bacteria, also known MAMPs, are involved in the TLR activation as well. As bacterial DNA is capable of activating TLR9, its inhibitors hold potential to serve as a drug in various diseases (Savva and Roger, 2013). Chloroquine has been reported to downregulate TLR9 via NF-K B pathway (Zhang et al., 2015). Another compound curcumin, which is polyphenol in nature, has been reported to improve the septic acute kidney injury in Sprague-Dawley (SD) rats by downregulation of TLR9 and consequently, its downstream regulators including MyD88, IRF5, and IRF7 and hence inflammatory factors and organ injury markers (Li et al., 2021).
Fungal Diseases and Toll-Like Receptors
Out of estimated 5 million fungal species present on earth, less than 100 are reported to be pathogenic which include Aspergillus fumigatus, Cryptococcus neoformans, and more recently Cryptococcus gatii, Histoplasma capsulatum, Coccidiosis posadasii, Pneumocystis jirovecii, and the commensal P. jiroveci or the Candida spp. Yet the disease burden caused by the fungal infections is substantial (Lionakis and Levitz, 2018). Currently, a very few antifungals have been discovered (Almeida et al., 2019). Fungi and their cellular components, particularly cell wall components, are recognized by various TLRs including TLR2, TLR6, TLR4, and TLR9 (Netea et al., 2007; Romani, 2011). Various strategies are utilized by fungi in order to evade the recognition by immune system, which includes sensing by TLRs. They can undergo cell wall remodeling and can form large cellular structures which can hamper phagocytosis, ultimately inhibiting the further immune response. It has been reported that DNA from Cryptococcus neoformans can downregulate TLR9 activation (Bourgeois and Kuchler, 2012). Van Der Graaf et al. (2005) reported that Candida blastoconidia lead to secretion of IFN-γ whereas Candida hyphae lead to production of proinflammatory cytokines, pointing toward a possible mechanism of immune evasion due through phenotypic switching.
Toll-Like Receptors and Viral Infections
Toll-Like Receptor Activation by Viral Proteins
Viruses present unique signatures to the immune system through nucleic acids. It has been reported that viruses activate the immune system, thereby starting IFN production to combat the viral infection. Nevertheless, after the discovery of TLRs, viral interactions with the innate immune system have been revised. It was found that TLRs recognize a virus, thus activating the immune system through an IFN-α or -β-dependent or -independent mechanism (Thompson and Iwasaki, 2008).
TLRs 3, 7, and 8 recognize viral RNA components, in contrast to other TLRs, which are located in the plasma membrane. TLRs 3, 7, and 8 are located in intracellular compartments and are triggered in late endosomal-lysosomal stages (Takeuchi and Akira, 2007). In the viral life cycle, enveloped viruses enter the cells by interacting with the receptors or attachment factors, which helps them to be internalized into the cells via a variety of endocytic processes. Examples of endocytic processes include clathrin-mediated endocytosis, micropinocytosis, clathrin- and caveolin-independent endocytosis. Some of the non-enveloped viruses enter the cells through clathrin- and caveolin-independent endocytosis (White and Whittaker, 2016). TLR3 recognizes double-stranded RNA, which is the genome in many virus families or an intermediate in the replication cycle of DNA viruses. TLR3 was also reported to be activated by virus-infected apoptotic cells (Thompson and Iwasaki, 2008).
Toll-like receptor 3 is located on the membrane of the endoplasmic reticulum in normal host cells. A double-stranded RNA molecule of at least 90 bp is recognized by endosomal TLR3 (Leonard et al., 2008). When a ligand stimulates the endosomal membrane, TLR3 dimerizes and is trafficked to the endosomal membrane, where it interacts with the antigen in a pH-dependent manner. The interaction between endoplasmic-reticulum protein UNC-93B and TLR3 is critical for the activation of the latter. Tyrosine phosphorylation on TLR3 is crucial for the recruitment of TIRAP and TRIF. In contrast, TLR7 signaling is MyD88-dependent and TRIF-independent and does not require the phosphorylation of tyrosine (Perales-Linares and Navas-Martin, 2013).
Toll-like receptor 7 and Toll-like receptor 8 have been found to recognize GU-rich and AU-rich single-stranded RNA molecules. This process generates a MyD88-dependent immune response against various viruses, such as vesicular stomatitis virus, influenza virus, Japanese encephalitis virus, and other flaviviruses (Nazmi et al., 2014).
It has been reported that 70–80% of mammalian DNA is methylated in CpG motifs. On the other hand, TLR9 recognizes unmethylated CpG motifs in DNA (Li and Zhang, 2014). In this way, TLR9 differentiates between host DNA and viral or bacterial genomic DNA. TLR9 recognizes various viruses, including murine cytomegalovirus, herpes simplex virus 1 (HSV1), HSV2, adenoviruses, poxviruses, Kaposi’s sarcoma herpesvirus, and varicella zoster virus. Active replication of viruses is not required for TLR9 triggering. UV-inactivated virions are also capable of engaging TLR9 (Lester and Li, 2014).
Toll-Like Receptor and SARS-CoV-2
In a recent study, it has been depicted in a ferret model that the injection of TLR2/6 agonist significantly reduced the level of viral RNA in nose and throat (Proud et al., 2021). Similarly, another study reported that SARS-CoV-2 envelop protein was able to upregulate the production of proinflammatory cytokines through TLR2-dependent signaling (Zheng et al., 2021). In silico studies, phylogenetic analysis and preliminary studies on the interaction between SARS-CoV-2 and human cells suggest that endosomal TLRs are also a possible route of entry for SARS-CoV-2 (Bezemer and Garssen, 2021; Gadanec et al., 2021; Salvi et al., 2021). M5049, a small-molecule inhibitor developed by Merck, is an antagonist of TLR7 and TLR8. Currently, it is undergoing clinical trials (NCT04448756). Pul-042 is an agonist of TLR2-TLR6 and TLR9. This agonist is used as a prophylactic drug against COVID-19 and is currently in clinical trials against reducing the infection rate and progression of COVID19 (NCT04313023). Imiquimod, which is preferentially TLR7 agonist also activates TLR8 weakly, is a similar kind of drug that can be employed for prophylaxis in such patients (Scho and Scho, 2008; Avcilar and Eken, 2020). Ruxolitinib is a small-molecule inhibitor of Janus kinase (JAK) is known to downregulate TNF-α, has been tested in clinical trials phase III (NCT04362137). Emapalumab and anakinra are monoclonal antibodies that antagonize IFN-γ and IL-1β, respectively, and are currently in clinical trials (NCT0432402). Tocilizumab and sarilumab are IL-6 inhibitors and belong to a class of monoclonal antibodies. Their effects on COVID-19 patients are being studied too (NCT04479358)(NCT04386239) (Choudhury and Mukherjee, 2020). Anakinra and Tocilizumab are also being used in combination against COVID-19 (NCT04412291).
It has been proved in an in silico study that the S1 subunit of the spike protein of SARS-CoV-2 strongly binds to TLR4 (Choudhury and Mukherjee, 2020). Some researchers proposed a model suggesting that the binding between SARS-CoV-2 and TLR4 enhances the expression of ACE-2 on the cell surface, thus facilitating virus entry (Aboudounya and Heads, 2021; Pantazi et al., 2021). Although there is not much evidence, TLR4 is also considered a possible TLR involved in the entry of SARS-CoV-2 into the cell. Multiple TLR4 agonists and antagonists are currently in clinical trials against SARS-CoV-2. Some of the TLR4 antagonists that are in clinical trials include resveratrol, curcumin, quercetin, and berberine (Kaushik et al., 2021; Figure 2).
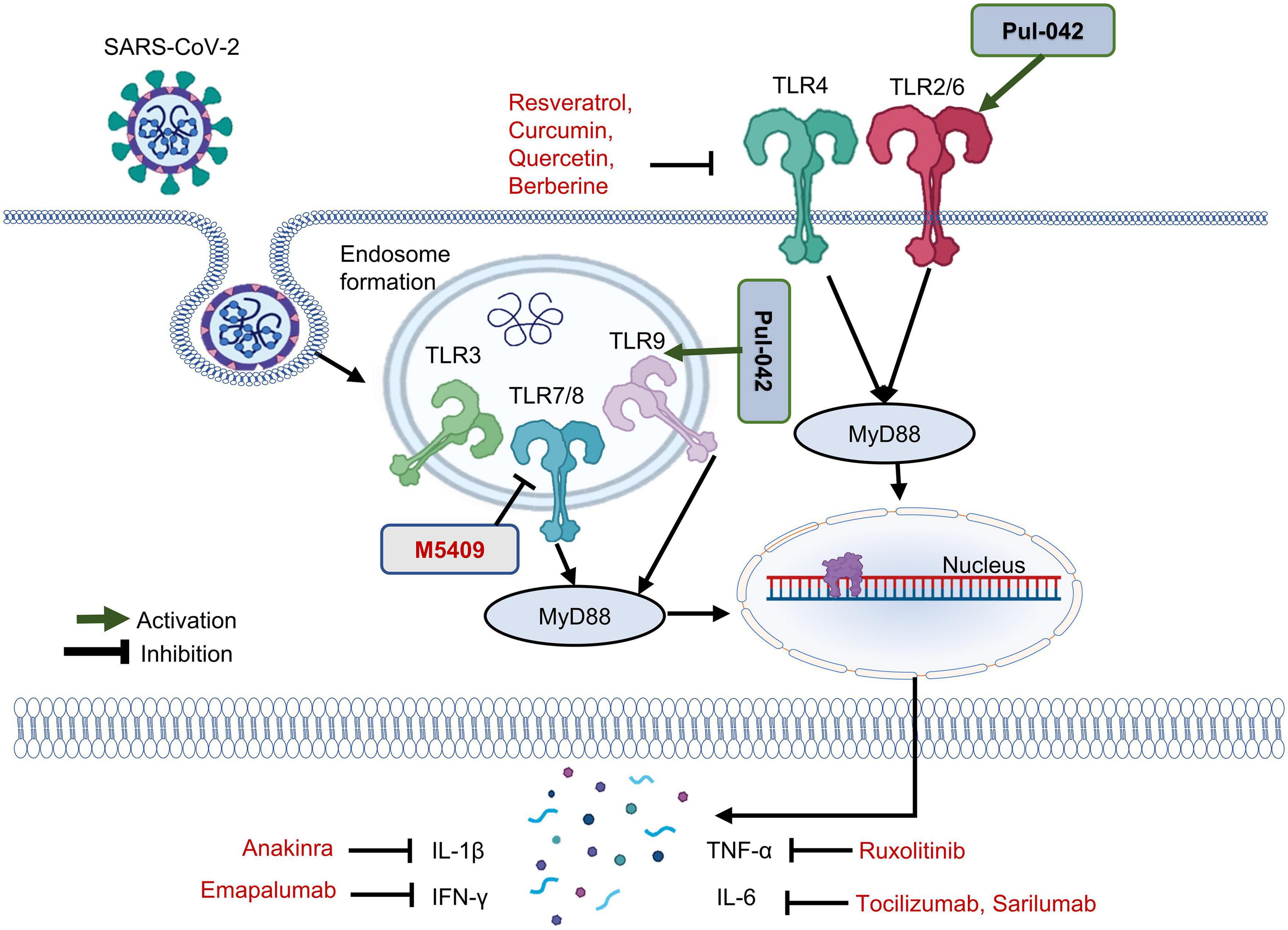
Figure 2. Toll-like receptor (TLR) triggering SARS-CoV-2 infection. SARS-CoV-2, in addition to its main receptor ACE-2, can enter the cell through interaction with TLRs. Currently, TLR4 antagonists including resveratrol, curcumin, quercetin, and berberine are in clinical trials. Pul-042 is a TLR2/6/9 agonist, which stimulates T-helper cells mediated cytokine and interferon secretion. To control a cytokine storm, anakinra, emapalumab, and ruxolitinib are being tested, which are monoclonal antibodies against IL-1β, IFN-γ, and TNF-α. Against IL-6, tocilizumab and sarilumab can be used. M5049, a TLR7/8 antagonist, is currently being tested in clinical trials against COVID.
Viral Manipulation of Host Immune System
Virus recognition by the host immune system is not limited to endosomal components such as endosomal TLRs, STING receptors, and RLRs. Additionally, the viral components produced by the cell are recognized by TLRs located on the plasma membrane. Some investigators reported that microRNAs produced by human cytomegalovirus downregulate TLR2 expression and thus modulate the innate immune response (Landais et al., 2015). TLR2 can recognize human-cytomegalovirus glycoproteins. A large number of viral proteins are known to turn on TLR2, TLR4, and TLR6 (Boehme et al., 2006; Kawai and Akira, 2011). HCMV encoded microRNA miR-UL112-3p has been reported to downregulate the TLR2 expression in a similar fashion to TLR2 targeting siRNA. It was also reported that upon TLR2 stimulation, miR-UL112-3p downregulated the expression of multiple cytokines (Landais et al., 2015).
Vaccinia virus protein A46R contains a TIR domain that interacts with MyD88, TRIF, and TRIF-related adaptor molecules and downregulates TRIF-mediated IFN secretion (Stack et al., 2005). A46R is thought to be an immunomodulatory protein that targets MAL and MyD88 (Azar et al., 2020). Another vaccinia virus protein, A52R, inhibits multiple TLRs by targeting TRAF6 and IRAK2 (Harte et al., 2003). The discovery of these two proteins indicates that it is possible to use viral proteins as TLR ligands (Patel et al., 2014).
Toll-Like Receptor-Based Immunotherapeutics to Combat Viral Infections
Toll-like receptors are employed as therapeutic targets as well as vaccine adjuvants in viral infections. FDA-approved antiviral drugs include many TLR agonists. Examples include monophosphoryl lipid A, which is a TLR4 agonist and is utilized as an adjuvant in vaccines against human papilloma virus and hepatitis B virus. Imiquimod (brand name AldaraTM) which is mainly TLR7 agonist, has been approved for human-papilloma-virus–induced genital and perianal warts. Flagellin (a TLR5 agonist) and Class B CpG (a TLR9 agonist) serve as adjuvants in influenza vaccines. Poly I:C, a TLR3 agonist, is used as an antiviral agent against influenza virus strains (Hedayat et al., 2011). Aside from FDA-approved drugs, many TLR ligands have been experimentally tested for the development of antiviral drugs, and some of them are in clinical trials (Patel et al., 2014).
Immune Sensors for Viruses Other Than Toll-Like Receptors
Intracellular viral RNA is also recognized by another class of PRRs known as retinoic acid-inducible gene-I (RIG-I)-like receptors (RLRs) (Cao et al., 2019). RLRs are dsRNA binding proteins, that recognize RNA which is distinct from the host RNA based upon the molecular characteristics (Zhao and Karijolich, 2019). The three members of RLRs are RIG-1, MDA5 and MAVS. Both RIG-1 and MDA5 are localized in the cytosol. Both of them contain N-terminal tandem caspase-recruitment domains (CARDs) and helicase domains. When dsRNA binds to RIG-1 or MDA5, oligomerization of the RNA sensors occurs which leads to activation of MAVS. As a result, IRF3/7 along with NF-κB gets activated and Interferon production is induced (Uehata and Takeuchi, 2020).
RIG-1 is activated by RNA molecules with 5′ cap, di- or triphosphate groups attached, no ribose and 2′-O-methylation and having a duplex structure. These characteristics save the immune system from getting into trouble of self-recognition as most of these characteristics are present in viral genomes but absent in the host RNAs (Rehwinkel and Gack, 2020).
Activation of MDA5 is not understood completely yet. RNA and DNA viruses have been reported to activate MDA5. It has been proposed that when double stranded RNA viruses infect cells, it leads to accumulation of RNA inside the cells. The accumulation of viral RNA is responsible for the activation of MDA5 (Rehwinkel and Gack, 2020).
Stimulator of interferon genes (STING), also known as transmembrane protein 173 (TMEM173), is a 379 amino acid protein in humans and 378 amino acid protein in mouse cells. It is usually expressed the immune cells and is localized in the endoplasmic reticulum. STING pathway is activated by cyclic dinucleotides (CDNs), which are produced by bacteria after they infect the cells. CDNs are also synthesized by cellular synthase also known as cyclic GMP–AMP synthase (cGAS) (Ahn and Barber, 2019). cGAS is the DNA sensor which recognizes the viral, bacterial and host DNA. CDNs induce the STING which undergo confirmational changes upon activation. The N-terminal domain of STING is responsible for the homodimerization and cellular localization, whereas the C-terminal recruits the other signaling molecules. Once STING is activated, it is transported to the endoplasmic reticulum, then Golgi apparatus and finally to the endosome, through intracellular trafficking or autophagy. It ultimately leads to secretion of type 1 interferon, type III interferon and other proinflammatory genes (Li et al., 2020).
Toll-Like Receptors and Cancers
Deidier was the first person (in the 18th century) to observe a positive correlation between a cure of cancer and infection (Cao et al., 2019). In the 19th century, William Coley upon detailed analysis of the history of the patients, found 47 cases from the hospital record which depicted the advantageous effects of fevers on malignancy. From his observation, he also inferred that a mixture of bacterial toxins from gram-positive and gram-negative bacteria had strong antitumor effects when injected in a person suffering from malignant cancer (Urban-Wojciuk et al., 2019). The first patient in which he observed this effect was suffering from an inoperable tumor in neck (Carlson et al., 2020). A mixture of killed Streptococcus pyogenes and Serratia marcescens was termed as Coley’s toxins, and it was later discovered by Shear et al. (1943) that the ingredient of Coley’s toxins responsible for the antitumor effect is lipopolysaccharide (LPS) (Hiraku et al., 2014). As LPS is the classic agonist for TLR4, so it is clear that in case of Coley’s toxin the activation of TLR4 through LPS lead to clearance of tumor efficiently (Rakoff-Nahoum and Medzhitov, 2009). This phenomenon of successful treatment of tumor through activation of TLR4 points to a promising mechanism of anticancer therapeutic strategies.
Immune Modulation by Tumor Cells
Tumors are thought to be recognized by the host immune system, but aggressive cancers progress via modulation of this system. For cancer immunotherapy to be successful, it is important to overcome the immunosuppressive nature of cancer cells. A recent advancement of cancer immunotherapy is a phenomenon known as “in situ vaccination.” In the tumor microenvironment, this approach creates an immunostimulatory microenvironment similar to that of a tumor that has already been treated (Mao et al., 2020). Hammerich et al. (2019) reported that in situ vaccination can successfully treat indolent non-Hodgkin’s lymphoma. This kind of vaccine contains a TLR3 agonist along with a tyrosine kinase 3 ligand and is combined with radiotherapy. As a result, an anti-tumor CD8+ T-cell response is generated. Even in non-responsive patients, a population of PD1+CD8+ T cells is formed. In this context, untreated distant tumors become sensitive to PD1 treatment; the addition of PD1 treatment increases the therapeutic efficacy from 40% to 80% (Hammerich et al., 2019).
Apoptotic cells trigger the inflammatory pathway via MyD88-mediated TLR activation. This process is known as a “sterile inflammatory response.” Cells undergoing apoptosis release molecules that serve as ligands for TLRs. In cells that are capable of switching on the MyD88-mediated TLR pathway, these molecules from dying cells cause NF-κB activation (Albensi, 2019). NF-κB in turn regulates the transcription of more than 100 genes involved in inflammation, including IL6, TNFA, and IL1B (Pradere et al., 2014). The activation of NF-κB leads to the production of proinflammatory cytokines, ultimately resulting in the inhibition of apoptosis and a progrowth microenvironment. Apoptosis inhibition has been proposed to be the reason for the chemoresistance of cancer. In this regard, TLRs serve as a double-edged sword that can turn against the body by initiating pathways that ultimately enhance cancer progression and induce chemoresistance (Chen et al., 2008).
Controversial Role of Toll-Like Receptors in Cancer
In addition to immune cells, various types of tumor cells also express TLRs. It was demonstrated that the inhibition or promotion of tumor progression is regulated by different TLRs in tumor cells (Huang et al., 2018). The same research group reported that TLR9 is overexpressed in human tumor cells. However, in mice TLR4 is overexpressed in tumor cells (Huang et al., 2008). The role of TLRs in cancer has been considered controversial. Some investigators nominated TLR agonists as the best frenemy of cancer immunotherapy (Kaczanowska et al., 2013).
Nevertheless, TLRs are a double-edged sword in cancer progression. TLR4/MyD88 pathway has been found to be upregulated in breast cancer cell line with high invasion rate as compared to the breast cancer cell line with lower invasion rate. It was also suggested that TLR4/MyD88 levels could serves as a useful prognostic biomarker for breast cancer patients (Wu et al., 2018). Szajnik et al. (2009) suggested that in ovarian cancer, LPS mediated upregulated TLR4/MyD88 might assist cancer cells in progression and development of chemoresistance ultimately aiding them in immune evasion. However, TLR4 agonists have been tested for the development of antitumor response against bladder cancer (Moghadam and Nowroozi, 2019).
There are multiple combinatorial immunotherapies of cancer that involve TLR agonists and antagonists. In 2013, the Science magazine named cancer immunotherapy a “Breakthrough of the year.” Currently, there are a number of TLR agonists that are FDA-approved drugs and are being used for cancer immunotherapy. The predominantly TLR7 ligand imiquimod (also weakly activates TLR8) and a TLR9 ligand (a CpG oligo) have shown promising results in cancer treatment (Murad and Clay, 2009; Huang et al., 2018). The BCG vaccine involves TLR1-TLR2, TLR2-TLR6, TLR4, and TLR9 agonists and is reported to be effective against urothelial tumors (Kaczanowska et al., 2013; Larue et al., 2013). It is currently a standard of care for localized bladder cancer. AS15 is a TLR4 agonist and is employed as a cancer vaccine adjuvant (Braunstein et al., 2018). Several TLR agonists which are currently in clinical trials as cancer immunotherapies are mentioned in the Table 1.
Toll-Like Receptors and Autoimmune Diseases
The immune system, to differentiate self-antigens from foreign antigens, develops tolerance to self-antigens. On the other hand, if the immune system loses the ability to distinguish self- and non-self-antigens, an autoimmune disease develops. More than 80 types of autoimmune diseases have been documented so far (Smatti et al., 2019). TLR signal transduction is necessary for inducing the secretion of proinflammatory cytokines and hence for initiating an adaptive immune response. TLRs serve as a link between innate and adaptive immune responses. Therefore, dysregulation of TLRs is believed to be involved in the pathogenesis of autoimmunity (Hosseini et al., 2015). TLRs 7 and 9 have been found to be associated with systemic lupus erythematosus in humans and in mouse models. Activated TLRs lead to the upregulation of proinflammatory cytokines and interferon response from innate immune cells (Liu et al., 2017; El-Zayat et al., 2019).
Autoreactive B cells and overactive T cells are considered the main drivers of autoimmunity. Autoantigens interact with dendritic cells, which stimulate B cells, thereby resulting in the upregulation of autoantibodies and proinflammatory-cytokine production. In patients with systemic lupus erythematosus, TLR7 and TLR9 play an important part in the expansion of autoreactive B cells (Toubi and Vadasz, 2019).
Role of Toll-Like Receptors in Psoriasis
Endosomal TLRs (TLRs 3, 7, 8, and 9) have been directly linked to the pathogenesis of multiple autoimmune diseases (Theofilopoulos et al., 2017). Psoriasis is a chronic autoimmune dermatosis with a prevalence of 2–3% in the world population. It is a systemic disease that can cause obesity, non-alcoholic fatty liver disease, cardiovascular disease, atherosclerosis, diabetes, hypertension, and inflammatory bowel disease (Korman, 2020). TLRs are also present on non-immune cells. Normally, keratinocytes express TLRs 1, 2, and 5. When cultured in vitro, they express TLRs 2–6 and 9. Activation of TLRs 2–4 results in the secretion of large amounts of proinflammatory cytokines, including TNF-α, IL-2, and a type I IFN (Rahmani and Rezaei, 2016). Heat shock proteins are reported to take part in the triggering of TLRs, thus increasing a proinflammatory-cytokine release, leading to inflammation in psoriasis (Sweeney et al., 2011).
Therapeutic Interventions Targeting Toll-Like Receptors
There are several antibodies, including ixekizumab, adalimumab, and ustekinumab, that are currently used as drugs against psoriasis; they block the cytokines implicated in the development of psoriasis (Kuhn and Luger, 2010; Leonardi et al., 2011, 2019; Ren and Dao, 2013; Burness and McKeage, 2015; Zweegers et al., 2017). Several small-molecule inhibitors, including CPG-52364, IMO-8400, and IMO-3100, that target the activation of endosomal TLRs, are under clinical investigation against psoriasis. Some other small-molecule compounds have been tested in animal models as anti-inflammatory agents and found to effectively target the TLR signaling pathway too. Nonetheless, they have not been tested against psoriasis yet (Lai et al., 2017).
Rheumatoid Arthritis
Rheumatoid arthritis is a chronic autoimmune arthropathy characterized by progressive articular destruction and a loss of function (Firestein and McInnes, 2017). The clinically symptomatic phase is preceded by pre-RA, which may be a few months to years long, and features increased levels of autoantibodies and cytokines in the blood. Clinical onset of the disease involves synovitis and the comorbidities that may affect metabolism, bones, the vascular system, and psychological state (McInnes and Schett, 2017).
Synovium is responsible for the maintenance of cartilage nutrition and joint function in healthy individuals. The synovial lining, composed of fibroblast-like synoviocytes and macrophage-like synoviocytes, expands after RA onset. Upregulation of TLRs 2, 3, 4, and 7 in fibroblast-like synoviocytes drives the expansion of the intimal lining. Overexpression of these TLRs causes upregulation of IL-6 and MMP3 (Arleevskaya et al., 2019). TLR3 and TLR4 are overexpressed in human synovial fibroblasts (Goh and Midwood, 2012). Upregulated TLR4 also contributes to development of neuropathic pain in RA (Pierer et al., 2011). It has been reported that upon inhibition of TLR4, severity of the disease was significantly reduced along with the cytokine level in the mice model (Abdollahi-Roodsaz et al., 2007). TLR4 seems to be an emerging target for controlling persistent pain in RA (Bruno et al., 2018).
Therapeutic Interventions
Sacre et al. (2008) demonstrated that TNF-α is induced upon stimulation of TLRs 3 and 8 in an RA model based on synovial cultures, thus pointing to their importance in RA therapeutic options. Cytokine levels in RA-synovial membranes are reduced upon inhibition of MyD88 and TIRAP, which are adaptor proteins for TLR2 and TLR4. It can be concluded that TLR2 and TLR4 are important in RA pathogenesis (Goh and Midwood, 2012). It was shown that TLRs 3 and 4 are overexpressed in synovial tissues at the early stages of RA, thereby causing chronic inflammation and dysfunction of joints (Ospelt et al., 2008). TAK-242, a specific inhibitor of TLR4-mediated signaling, has good potential for controlling the progression of RA, as illustrated in Figure 3. Samarpita et al. (2020) performed in vitro experiment using human rheumatoid fibroblast-like synoviocyte (FLS) line MH7A or primary human FLS and in an adjuvant-induced arthritis (AIA) rat model. TAK-242 was found to be effective in downregulating IL-6, IL-8, MMP-1, and VEGF level in LPS stimulated cells. It also hindered the translocation of NF-κB in nucleus. In rat model, TAK-242 at 5mg.kg alleviated the symptoms of RA and normalized the serum level of IL-6 and VEGF (Ono et al., 2020; Samarpita et al., 2020).
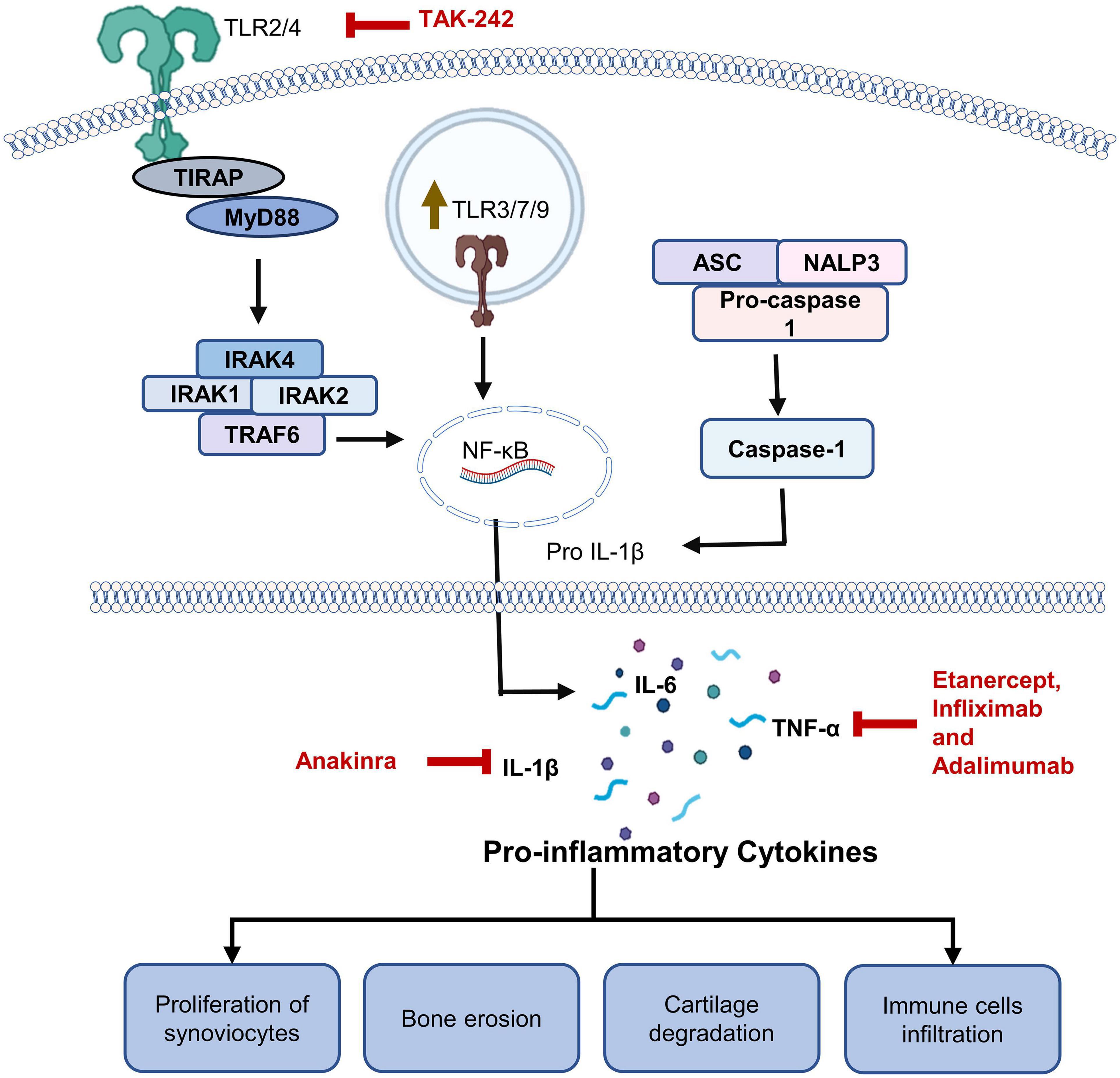
Figure 3. Roles of TLRs in rheumatoid arthritis (RA). Role of TLR2/4 has been extensively studied in RA. Engagement of a TLR by a DAMP promotes release of certain cytokines through MyD88 dependent pathway. Level of TNF-α, IL-6, and IL-1β have been found to be upregulated in RA patients. Upregulated cytokines lead to infiltration of immune cells, proliferation of synoviocytes ultimately leading to bone damage and cartilage degradation. Multiple monoclonal antibodies against TNF-α including etanercept, infliximab and adalimumab are commonly used for RA management. Patients who do not respond to anti-TNF-α antibodies are treated with anti–IL-1β antibodies. TAK-242, which is a TLR4 inhibitor, is also being tested against RA.
NI-0101 is a humanized monoclonal antibody against TLR4, which was tested in clinical trial phase II against rheumatoid arthritis (NCT03241108). No significant response in the treated patients could be observed (Monnet et al., 2020). Some available modalities against RA include etanercept, infliximab, and adalimumab targeting TNF-α and are known to reduce inflammation (Zamora-Atenza et al., 2014). Anakinra is a monoclonal antibody that inhibits IL-1β and has corresponding adverse effects. It is administered subcutaneously to patients who do not respond to a TNF-α inhibitor (Baskar et al., 2016; Abbasi et al., 2019).
Toll-Like Receptortlrs in Pain and Neurodegeneration
Role of TLRs has been studied in neurological pain and TLR2,3,4,5,7,8,9 are supposed to be involved in the development of neurological pain (Thakur et al., 2017; Barrat, 2018). It has been reported that presence of TLRs on the central nervous system cells plays a key role in the maintenance and sustenance of neurological pain (Shah and Choi, 2017). Microglia predominantly express TLR2 and TLR4 and in certain circumstances, astrocytes can also express TLR2 and TLR4. Jurga et al. (2016) suggested that by blocking TLR2/4, pain can be relieved in rat neuropathic pain model. He et al. (2020) explained the involvement of TLR7 in the neuropathic pain in dorsal root ganglia. He suggested that as a result of neuronal injury, activation of TLR7 leads to upregulation of NF-κB, which contributed to the development of neurological pain. This effect can be blocked by inhibiting TLR7 (He et al., 2020).
Neurodegeneration refers to the gradual damage and death of neurons, and it normally occurs as a result of neurodegenerative diseases which include Parkinson’s disease, diabetic neuropathy, Alzheimer’s disease (Simó et al., 2018). There are various pathological conditions which can serve as a contributing factor to neurodegeneration, some of them also point out toward involvement of TLRs. One hypothesis, known as endotoxin hypothesis suggests that endotoxins are the cause or contributing factor for neurodegeneration (Brown, 2019). Zhang et al. (2018) reported that injection of Porphyromonas gingivalis LPS in C57BL/6 mice lead to activation of astrocytes and microglia in the cerebral cortex and hippocampus. It leads to upregulation of proinflammatory cytokines and activation of TLR4/NF-κB pathway. The effects were significantly reduced by using TAK-242, which is an inhibitor of TLR4 (Zhang et al., 2018).
Discussion
Toll-like receptors are a class of PRRs that play a crucial role in the development of an innate immune response. Upon their engagement by a PAMP or DAMP, TLR dimerization results in the activation of transcription factors driving the secretion of proinflammatory cytokines. Endosomal TLRs are strongly involved in the recognition of viral genomic material and thus help to mount an antiviral response. Additionally, it is possible that cell surface TLRs are also turned on by components of viruses other than the genome, namely, by proteins generated for the virus by the cell. As for SARS-CoV-2 infection, TLRs are still a subject of debate. Nonetheless, various TLR agonists and inhibitors are currently being tested in clinical trials against COVID-19. Other viral infections, including those caused by vesicular stomatitis virus, Japanese encephalitis virus, and many flaviviruses, are also being treated with TLR ligands.
Furthermore, TLRs are an important but tricky target when it comes to cancer treatment. They serve as a double-edged sword in cancers. As a result of MyD88 engagement by TLRs, cancer cells undergo apoptosis. As a consequence of the interaction of the molecules released from cells, cytokines are produced, and apoptosis is inhibited. The suppression of apoptosis creates a favorable environment for tumor growth. That is why TLRs have been regarded as the best frenemy in cancer immunotherapy. As for autoimmune diseases, TLRs are involved in successful treatments of various diseases, such as psoriasis and RA. In the era of multi-drug resistant and pan-resistant bacterial infections, TLR-based drugs seem to be a ray of light. As far as fungal diseases are concerned, data suggests that drugs targeting TLRs sound promising. Therefore, it has become crystal clear that TLRs can serve as a promising target for the development of immunotherapeutics against many types of diseases.
Most of the diseases which are contributing a lot toward the global burden of diseases can be treated successfully with the successful discovery of TLR-based therapeutics. TLR-based therapeutics discovery seems to be tough, but it is exciting, and it will open new avenues in terms of drug discovery and development and prevention for infectious diseases, as well as cancer, allergies, asthma, and autoimmune diseases, either directly or through the development of better vaccines.
Author Contributions
MF and MB defined the scope of the manuscript and wrote it. MF, MB, and SC revised the manuscript. MK and SC did the project administration and funding acquisition. All authors read and approved the final manuscript.
Funding
This work was supported by the National Research Foundation of Korea [grant numbers NRF-2020R1F1A1071517, 2019M3D1A1078940, and 2019R1A6A1A11051471].
Conflict of Interest
MB and SC was employed by company S&K Therapeutics, South Korea.
The remaining authors declare that the research was conducted in the absence of any commercial or financial relationships that could be construed as a potential conflict of interest.
Publisher’s Note
All claims expressed in this article are solely those of the authors and do not necessarily represent those of their affiliated organizations, or those of the publisher, the editors and the reviewers. Any product that may be evaluated in this article, or claim that may be made by its manufacturer, is not guaranteed or endorsed by the publisher.
References
Abbasi, M., Mousavi, M. J., Jamalzehi, S., Alimohammadi, R., Bezvan, M. H., Mohammadi, H., et al. (2019). Strategies toward rheumatoid arthritis therapy; the old and the new. J. Cell. Physiol. 234, 10018–10031. doi: 10.1002/jcp.27860
Abdollahi-Roodsaz, S., Joosten, L. A. B., Roelofs, M. F., Radstake, T. R. D. J., Matera, G., Popa, C., et al. (2007). Inhibition of toll-like receptor 4 breaks the inflammatory loop in autoimmune destructive arthritis. Arthritis Rheum. 56, 2957–2967. doi: 10.1002/ART.22848
Aboudounya, M. M., and Heads, R. J. (2021). COVID-19 and toll-like receptor 4 (TLR4): SARS-CoV-2 may bind and activate TLR4 to increase ACE2 expression, facilitating entry and causing hyperinflammation. Mediators Inflamm. 2021:8874339. doi: 10.1155/2021/8874339
Achek, A., Yesudhas, D., and Choi, S. (2016). Toll-like receptors: promising therapeutic targets for inflammatory diseases. Arch. Pharm. Res. 39, 1032–1049. doi: 10.1007/s12272-016-0806-9
Ahn, J., and Barber, G. N. (2019). STING signaling and host defense against microbial infection. Exp. Mol. Med. 51, 1–10. doi: 10.1038/s12276-019-0333-0
Albensi, B. C. (2019). What is nuclear factor Kappa B (NF-κB) doing in and to the mitochondrion? Front. Cell Dev. Biol. 7:154. doi: 10.3389/FCELL.2019.00154
Aletaha, S., Haddad, L., Roozbehkia, M., Bigdeli, R., Asgary, V., Mahmoudi, M., et al. (2017). M2000 (β-D-mannuronic acid) as a novel antagonist for blocking the TLR2 and TLR4 downstream signalling pathway. Scand. J. Immunol. 85, 122–129. doi: 10.1111/sji.12519
Almeida, F., Rodrigues, M. L., and Coelho, C. (2019). The still underestimated problem of fungal diseases worldwide. Front. Microbiol. 10:214. doi: 10.3389/FMICB.2019.00214
Anwar, M. A., Shah, M., Kim, J., and Choi, S. (2019). Recent clinical trends in toll-like receptor targeting therapeutics. Med. Res. Rev. 39, 1053–1090. doi: 10.1002/MED.21553
Arleevskaya, M. I., Larionova, R. V., Brooks, W. H., Bettacchioli, E., and Renaudineau, Y. (2019). Toll-like receptors, infections, and rheumatoid arthritis. Clin. Rev. Allergy Immunol 58, 172–181. doi: 10.1007/S12016-019-08742-Z
Avcilar, H., and Eken, A. (2020). Could imiquimod (Aldara 5% cream) or other TLR7 agonists be used in the treatment of COVID-19? Med. Hypotheses 144:110202. doi: 10.1016/J.MEHY.2020.110202
Azar, D. F., Haas, M., Fedosyuk, S., Rahaman, M. H., Hedger, A., Kobe, B., et al. (2020). Vaccinia virus immunomodulator A46: destructive interactions with MAL and MyD88 Shown by negative-stain electron microscopy. Structure 28, 1271–1287.e5. doi: 10.1016/j.str.2020.09.007
Barratt-Due, A., Pischke, S. E., Nilsson, P. H., Espevik, T., and Mollnes, T. E. (2017). Dual inhibition of complement and Toll-like receptors as a novel approach to treat inflammatory diseases—C3 or C5 emerge together with CD14 as promising targets. J. Leukoc. Biol. 101, 193–204. doi: 10.1189/JLB.3VMR0316-132R
Baskar, S., Klein, A. L., and Zeft, A. (2016). The use of IL-1 receptor antagonist (Anakinra) in idiopathic recurrent pericarditis: a narrative review. Cardiol. Res. Pract. 2016:7840724. doi: 10.1155/2016/7840724
Bezemer, G. F. G., and Garssen, J. (2021). TLR9 and COVID-19: a multidisciplinary theory of a multifaceted therapeutic target. Front. Pharmacol. 11:601685. doi: 10.3389/FPHAR.2020.601685
Boehme, K. W., Guerrero, M., and Compton, T. (2006). Human cytomegalovirus envelope glycoproteins B and H are necessary for TLR2 Activation in permissive cells. J. Immunol. 177, 7094–7102. doi: 10.4049/jimmunol.177.10.7094
Bourgeois, C., and Kuchler, K. (2012). Fungal pathogens—a sweet and sour treat for toll-like receptors. Front. Cell. Infect. Microbiol. 2:142. doi: 10.3389/FCIMB.2012.00142
Braunstein, M. J., Kucharczyk, J., and Adams, S. (2018). Targeting Toll-like receptors for cancer therapy. Target. Oncol. 13, 583–598. doi: 10.1007/s11523-018-0589-7
Brown, G. C. (2019). The endotoxin hypothesis of neurodegeneration. J. Neuroinflammation 161, 1–10. doi: 10.1186/S12974-019-1564-7
Bruno, K., Woller, S. A., Miller, Y. I., Yaksh, T. L., Wallace, M., Beaton, G., et al. (2018). Targeting Toll-like receptor-4 (TLR4) – emerging therapeutic target for persistent pain states. Pain 159:1908. doi: 10.1097/J.PAIN.0000000000001306
Burness, C. B., and McKeage, K. (2015). Adalimumab: a review in chronic plaque psoriasis. Drugs 75, 2119–2130. doi: 10.1007/s40265-015-0503-x
Cao, P., Luo, W.-W., Li, C., Tong, Z., Zheng, Z.-Q., Zhou, L., et al. (2019). The heterogeneous nuclear ribonucleoprotein hnRNPM inhibits RNA virus-triggered innate immunity by antagonizing RNA sensing of RIG-I-like receptors. PLoS Pathog. 15:e1007983. doi: 10.1371/JOURNAL.PPAT.1007983
Carlson, R. D., Flickinger, J. C. Jr., and Snook, A. E. (2020). Talkin’ toxins: from coley’s to modern cancer immunotherapy. Toxins (Basel) 12:241. doi: 10.3390/TOXINS12040241
Casilag, F., Franck, S., Matarazzo, L., Figeac, M., Michelet, R., Kloft, C., et al. (2020). Boosting toll-like receptor 4 signaling enhances the therapeutic outcome of antibiotic therapy in pneumococcal pneumonia. bioRxiv [Preprint] doi: 10.1101/2020.02.18.955500
Casilag, F., Matarazzo, L., Franck, S., Figeac, M., Michelet, R., Kloft, C., et al. (2021). The biosynthetic monophosphoryl lipid a enhances the therapeutic outcome of antibiotic therapy in pneumococcal pneumonia. ACS Infect. Dis. 7, 2164–2175. doi: 10.1021/ACSINFECDIS.1C00176
Chen, R., Alvero, A. B., Silasi, D. A., Steffensen, K. D., and Mor, G. (2008). Cancers take their toll - the function and regulation of toll-like receptors in cancer cells. Oncogene 27, 225–233. doi: 10.1038/sj.onc.1210907
Cheng, Z., Taylor, B., Ourthiague, D. R., and Hoffmann, A. (2015). Distinct single-cell signaling characteristics are conferred by the MyD88 and TRIF pathways during TLR4 activation. Sci. Signal. 8:ra69. doi: 10.1126/SCISIGNAL.AAA5208
Choudhury, A., and Mukherjee, S. (2020). In silico studies on the comparative characterization of the interactions of SARS-CoV-2 spike glycoprotein with ACE-2 receptor homologs and human TLRs. J. Med. Virol. 92, 2105–2113. doi: 10.1002/JMV.25987
Christou, L. (2011). The global burden of bacterial and viral zoonotic infections. Clin. Microbiol. Infect. 17, 326–330. doi: 10.1111/J.1469-0691.2010.03441.X
Cognasse, F., Nguyen, K. A., Damien, P., McNicol, A., Pozzetto, B., Hamzeh-Cognasse, H., et al. (2015). The inflammatory role of platelets via their TLRs and siglec receptors. Front. Immunol. 6:83. doi: 10.3389/fimmu.2015.00083
de Oliviera Nascimento, L., Massari, P., and Wetzler, L. M. (2012). The role of TLR2 in infection and immunity. Front. Immunol. 3:79. doi: 10.3389/FIMMU.2012.00079
El-Zayat, S. R., Sibaii, H., and Mannaa, F. A. (2019). Toll-like receptors activation, signaling, and targeting: an overview. Bull. Natl. Res. Cent. 43:187. doi: 10.1186/S42269-019-0227-2
Firestein, G. S., and McInnes, I. B. (2017). Immunopathogenesis of rheumatoid arthritis. Immunity 46, 183–196. doi: 10.1016/j.immuni.2017.02.006
Fitzgerald, K. A., and Kagan, J. C. (2020). Toll-like Receptors and the control of immunity. Cell 180, 1044–1066. doi: 10.1016/J.CELL.2020.02.041
Gabarin, R. S., Li, M., Zimmel, P. A., Marshall, J. C., Li, Y., and Zhang, H. (2021). Intracellular and extracellular lipopolysaccharide signaling in sepsis: avenues for novel therapeutic strategies. J. Innate Immun. 18, 1–10. doi: 10.1159/000515740
Gäbele, E., Mühlbauer, M., Dorn, C., Weiss, T. S., Froh, M., Schnabl, B., et al. (2008). Role of TLR9 in hepatic stellate cells and experimental liver fibrosis. Biochem. Biophys. Res. Commun. 376, 271–276. doi: 10.1016/J.BBRC.2008.08.096
Gadanec, L. K., McSweeney, K. R., Qaradakhi, T., Ali, B., Zulli, A., and Apostolopoulos, V. (2021). Can SARS-CoV-2 virus use multiple receptors to enter host cells? Int. J. Mol. Sci. 22, 1–35. doi: 10.3390/ijms22030992
Gay, N. J. (2019). Role of self-organising myddosome oligomers in inflammatory signalling by toll-like receptors. BMC Biol. 17:15. doi: 10.1186/S12915-019-0637-5
Goh, F. G., and Midwood, K. S. (2012). Intrinsic danger: activation of toll-like receptors in rheumatoid arthritis. Rheumatology 51, 7–23. doi: 10.1093/rheumatology/ker257
Hammerich, L., Marron, T. U., Upadhyay, R., Svensson-Arvelund, J., Dhainaut, M., Hussein, S., et al. (2019). Systemic clinical tumor regressions and potentiation of PD1 blockade with in situ vaccination. Nat. Med. 255, 814–824. doi: 10.1038/s41591-019-0410-x
Harte, M. T., Haga, I. R., Maloney, G., Gray, P., Reading, P. C., Bartlett, N. W., et al. (2003). The poxvirus protein A52R targets toll-like receptor signaling complexes to suppress host defense. J. Exp. Med. 197, 343–351. doi: 10.1084/jem.20021652
He, L., Han, G., Wu, S., Du, S., Zhang, Y., Liu, W., et al. (2020). Toll-like receptor 7 contributes to neuropathic pain by activating NF-κB in primary sensory neurons. Brain. Behav. Immun. 87, 840–851. doi: 10.1016/J.BBI.2020.03.019
Hedayat, M., Netea, M. G., and Rezaei, N. (2011). Targeting of toll-like receptors: a decade of progress in combating infectious diseases. Lancet Infect. Dis. 11, 702–712. doi: 10.1016/S1473-3099(11)70099-8
Hiraku, Y., Kawanishi, S., and Ohshima, H. (2014). Cancer and Inflammation Mechanisms: Chemical, Biological, and Clinical Aspects. Hoboken, NJ: John Wiley & Sons, Inc. doi: 10.1002/9781118826621
Hosseini, A. M., Majidi, J., Baradaran, B., and Yousefi, M. (2015). Toll-like receptors in the pathogenesis of autoimmune diseases. Adv. Pharm. Bull. 5, 605–614. doi: 10.15171/apb.2015.082
Huang, B., Zhao, J., Unkeless, J. C., Feng, Z. H., and Xiong, H. (2008). TLR signaling by tumor and immune cells: a double-edged sword. Oncogene 27, 218–224. doi: 10.1038/sj.onc.1210904
Huang, L., Xu, H., and Peng, G. (2018). TLR-mediated metabolic reprogramming in the tumor microenvironment: potential novel strategies for cancer immunotherapy. Cell. Mol. Immunol. 15, 428–437. doi: 10.1038/cmi.2018.4
Israël, A. (2010). The IKK complex, a central regulator of NF-κB activation. Cold Spring Harb. Perspect. Biol. 2:a000158. doi: 10.1101/CSHPERSPECT.A000158
Jurga, A. M., Rojewska, E., Piotrowska, A., Makuch, W., Pilat, D., Przewlocka, B., et al. (2016). Blockade of toll-like receptors (TLR2, TLR4) attenuates pain and potentiates buprenorphine analgesia in a rat neuropathic pain model. Neural Plast. 2016:5238730. doi: 10.1155/2016/5238730
Kaczanowska, S., Joseph, A. M., and Davila, E. (2013). TLR agonists: our best frenemy in cancer immunotherapy. J. Leukoc. Biol. 93, 847–863. doi: 10.1189/JLB.1012501
Kaushik, D., Bhandari, R., and Kuhad, A. (2021). TLR4 as a therapeutic target for respiratory and neurological complications of SARS-CoV-2. Expert Opin. Ther. Targets 25, 491–508. doi: 10.1080/14728222.2021.1918103
Kawai, T., and Akira, S. (2009). The roles of TLRs, RLRs and NLRs in pathogen recognition. Int. Immunol. 21, 317–337. doi: 10.1093/INTIMM/DXP017
Kawai, T., and Akira, S. (2011). Toll-like receptors and their crosstalk with other innate receptors in infection and immunity. Immunity 34, 637–650. doi: 10.1016/j.immuni.2011.05.006
Kawasaki, T., and Kawai, T. (2014). Toll-like receptor signaling pathways. Front. Immunol. 5:461. doi: 10.3389/FIMMU.2014.00461
Knapp, S. (2010). Update on the role of toll-like receptors during bacterial infections and sepsis. Wien. Med. Wochenschr. 160, 107–111. doi: 10.1007/S10354-010-0765-6
Korman, N. J. (2020). Management of psoriasis as a systemic disease: what is the evidence? Br. J. Dermatol. 182, 840–848. doi: 10.1111/bjd.18245
Kuhn, A., and Luger, T. A. (2010). Psoriasis: is ustekinumab superior to etanercept for psoriasis? Nat. Rev. Rheumatol. 6, 500–501. doi: 10.1038/nrrheum.2010.134
Kumar, S., Sunagar, R., and Gosselin, E. (2019). Bacterial protein toll-like-receptor agonists: a novel perspective on vaccine adjuvants. Front. Immunol. 10:1144. doi: 10.3389/FIMMU.2019.01144
Kuzmich, N. N., Sivak, K. V., Chubarev, V. N., Porozov, Y. B., Savateeva-Lyubimova, T. N., and Peri, F. (2017). TLR4 signaling pathway modulators as potential therapeutics in inflammation and sepsis. Vaccines 5:34. doi: 10.3390/VACCINES5040034
Lai, C. Y., Su, Y. W., Lin, K. I., Hsu, L. C., and Chuang, T. H. (2017). Natural modulators of endosomal toll-like receptor-mediated psoriatic skin inflammation. J. Immunol. Res. 2017:7807313. doi: 10.1155/2017/7807313
Landais, I., Pelton, C., Streblow, D., DeFilippis, V., McWeeney, S., and Nelson, J. A. (2015). Human cytomegalovirus miR-UL112-3p Targets TLR2 and modulates the TLR2/IRAK1/NFκB signaling pathway. PLoS Pathog. 11:e1004881. doi: 10.1371/JOURNAL.PPAT.1004881
Larue, H., Ayari, C., Bergeron, A., and Fradet, Y. (2013). Toll-like receptors in urothelial cells – targets for cancer immunotherapy. Nat. Rev. Urol. 10, 537–545. doi: 10.1038/nrurol.2013.153
Leonard, J. N., Ghirlando, R., Askins, J., Bell, J. K., Margulies, D. H., Davies, D. R., et al. (2008). The TLR3 Signaling Complex Forms by Cooperative Receptor Dimerization. Available online at: www.pnas.org/cgi/content/full/ (accessed March 4, 2021)
Leonardi, C., Papp, K., Strober, B., Reich, K., Asahina, A., Gu, Y., et al. (2011). The long-term safety of adalimumab treatment in moderate to severe psoriasis: a comprehensive analysis of all adalimumab exposure in all clinical trials. Am. J. Clin. Dermatol. 12, 321–337. doi: 10.2165/11587890-000000000-00000
Leonardi, C., Papp, K., Strober, B., Thaçi, D., Warren, R. B., Tyring, S., et al. (2019). Comprehensive long-term safety of adalimumab from 18 clinical trials in adult patients with moderate-to-severe plaque psoriasis. Br. J. Dermatol. 180, 76–85. doi: 10.1111/bjd.17084
Lester, S. N., and Li, K. (2014). Toll-like receptors in antiviral innate immunity. J. Mol. Biol. 426, 1246–1264. doi: 10.1016/j.jmb.2013.11.024
Li, E., and Zhang, Y. (2014). DNA methylation in mammals. Cold Spring Harb. Perspect. Biol. 6, 19133–19134. doi: 10.1101/cshperspect.a019133
Li, H., Sun, H., Xu, Y., Xing, G., and Wang, X. (2021). Curcumin plays a protective role against septic acute kidney injury by regulating the TLR9 signaling pathway. Transl. Androl. Urol. 10:2103. doi: 10.21037/TAU-21-385
Li, Z., Cai, S., Sun, Y., Li, L., Ding, S., and Wang, X. (2020). When STING meets viruses: sensing, trafficking and response. Front. Immunol. 11:2064. doi: 10.3389/FIMMU.2020.02064
Lionakis, M. S., and Levitz, S. M. (2018). Host control of fungal infections: lessons from basic studies and human cohorts. Annu. Rev. Immunol. 36, 157–191. doi: 10.1146/annurev-immunol-042617-053318
Liu, F., Li, X., Yue, H., Ji, J., You, M., Ding, L., et al. (2017). TLR-induced SMPD3 defects enhance inflammatory response of B cell and macrophage in the pathogenesis of SLE. Scand. J. Immunol. 86, 377–388. doi: 10.1111/sji.12611
Mao, C., Gorbet, M.-J., Singh, A., Ranjan, A., and Fiering, S. (2020). In situ vaccination with nanoparticles for cancer immunotherapy: understanding the immunology. Int. J. Hyperthermia 37:4. doi: 10.1080/02656736.2020.1810333
Matsushima, N., Tanaka, T., Enkhbayar, P., Mikami, T., Taga, M., Yamada, K., et al. (2007). Comparative sequence analysis of leucine-rich repeats (LRRs) within vertebrate toll-like receptors. BMC Genomics 8:124. doi: 10.1186/1471-2164-8-124
McGettrick, A. F., and O’Neill, L. A. (2010). Localisation and trafficking of Toll-like receptors: an important mode of regulation. Curr. Opin. Immunol. 22, 20–27. doi: 10.1016/J.COI.2009.12.002
McInnes, I. B., and Schett, G. (2017). Pathogenetic insights from the treatment of rheumatoid arthritis. Lancet 389, 2328–2337. doi: 10.1016/S0140-6736(17)31472-1
Moghadam, S. O., and Nowroozi, M. R. (2019). Toll-like receptors: the role in bladder cancer development, progression and immunotherapy. Scand. J. Immunol. 90:e12818. doi: 10.1111/SJI.12818
Monnet, E., Choy, E. H., McInnes, I., Kobakhidze, T., de Graaf, K., Jacqmin, P., et al. (2020). Efficacy and safety of NI-0101, an anti-toll-like receptor 4 monoclonal antibody, in patients with rheumatoid arthritis after inadequate response to methotrexate: a phase II study. Ann. Rheum. Dis. 79, 316–323. doi: 10.1136/ANNRHEUMDIS-2019-216487
Muccioli, M., and Benencia, F. (2014). Toll-like receptors in ovarian cancer as targets for immunotherapies. Front. Immunol. 5:341. doi: 10.3389/FIMMU.2014.00341
Murad, Y. M., and Clay, T. M. (2009). CpG oligodeoxynucleotides as TLR9 agonists: therapeutic applications in cancer. BioDrugs 23, 361–375. doi: 10.2165/11316930-000000000-00000
Nazmi, A., Mukherjee, S., Kundu, K., Dutta, K., Mahadevan, A., Shankar, S. K., et al. (2014). TLR7 is a key regulator of innate immunity against Japanese encephalitis virus infection. Neurobiol. Dis. 69, 235–247. doi: 10.1016/j.nbd.2014.05.036
Netea, M. G., Van derMeer, J. W. M., and Kullberg, B. J. (2007). “Recognition of fungal pathogens by Toll-like receptors,” in Immunology of Fungal Infections, eds G. D. Brown and M. G. Netea (Dordrecht: Springer), 259–272. doi: 10.1007/1-4020-5492-0_11
Nie, L., Cai, S.-Y., Shao, J.-Z., and Chen, J. (2018). Toll-like receptors, associated biological roles, and signaling networks in non-mammals. Front. Immunol. 9:1523. doi: 10.3389/FIMMU.2018.01523
Nijland, R., Hofland, T., and van Strijp, J. A. (2014). Recognition of LPS by TLR4: potential for anti-inflammatory therapies. Mar. Drugs 12, 4260–4273. doi: 10.3390/MD12074260
O’Neill, L. A. J., and Bowie, A. G. (2007). The family of five: TIR-domain-containing adaptors in toll-like receptor signalling. Nat. Rev. Immunol. 7, 353–364. doi: 10.1038/nri2079
O’Neill, L. A. J., Golenbock, D., and Bowie, A. G. (2013). The history of toll-like receptors-redefining innate immunity. Nat. Rev. Immunol. 13, 453–460. doi: 10.1038/nri3446
Ono, Y., Maejima, Y., Saito, M., Sakamoto, K., Horita, S., Shimomura, K., et al. (2020). TAK-242, a specific inhibitor of Toll-like receptor 4 signalling, prevents endotoxemia-induced skeletal muscle wasting in mice. Sci. Rep. 10, 1–13. doi: 10.1038/s41598-020-57714-3
Opal, S. M., Laterre, P.-F., Francois, B., LaRosa, S. P., Angus, D. C., Mira, J.-P., et al. (2013). Effect of eritoran, an antagonist of MD2-TLR4, on mortality in patients with severe sepsis: the ACCESS randomized trial. JAMA 309, 1154–1162. doi: 10.1001/JAMA.2013.2194
Ospelt, C., Brentano, F., Rengel, Y., Stanczyk, J., Kolling, C., Tak, P. P., et al. (2008). Overexpression of toll-like receptors 3 and 4 in synovial tissue from patients with early rheumatoid arthritis: toll-like receptor expression in early and longstanding arthritis. Arthritis Rheum. 58, 3684–3692. doi: 10.1002/art.24140
Ospelt, C., and Gay, S. (2010). TLRs and chronic inflammation. Int. J. Biochem. Cell Biol. 42, 495–505. doi: 10.1016/j.biocel.2009.10.010
Pantazi, I., Al-Qahtani, A. A., Alhamlan, F. S., Alothaid, H., Matou-Nasri, S., Sourvinos, G., et al. (2021). SARS-CoV-2/ACE2 interaction suppresses IRAK-M expression and promotes pro-inflammatory cytokine production in macrophages. Front. Immunol. 12:683800. doi: 10.3389/FIMMU.2021.683800
Park, B. S., and Lee, J.-O. (2013). Recognition of lipopolysaccharide pattern by TLR4 complexes. Exp. Mol. Med. 45:e66. doi: 10.1038/EMM.2013.97
Parker, D., and Prince, A. (2012). Staphylococcus aureus induces type I IFN signaling in dendritic cells via TLR9. J. Immunol. 189, 4040–4046. doi: 10.4049/jimmunol.1201055
Patel, M. C., Shirey, K. A., Pletneva, L. M., Boukhvalova, M. S., Garzino-Demo, A., Vogel, S. N., et al. (2014). Novel drugs targeting toll-like receptors for antiviral therapy. Future Virol. 9, 811–829. doi: 10.2217/fvl.14.70
Perales-Linares, R., and Navas-Martin, S. (2013). Toll-like receptor 3 in viral pathogenesis: friend or foe? Immunology 140, 153–167. doi: 10.1111/imm.12143
Pierer, M., Wagner, U., Rossol, M., and Ibrahim, S. (2011). Toll-like receptor 4 is involved in inflammatory and joint destructive pathways in collagen-induced arthritis in DBA1J mice. PLoS One 6:e23539. doi: 10.1371/JOURNAL.PONE.0023539
Płóciennikowska, A., Hromada-Judycka, A., Borzęcka, K., and Kwiatkowska, K. (2015). Co-operation of TLR4 and raft proteins in LPS-induced pro-inflammatory signaling. Cell. Mol. Life Sci. 72, 557–581. doi: 10.1007/S00018-014-1762-5
Pradere, J. P., Dapito, D. H., and Schwabe, R. F. (2014). The Yin and Yang of toll-like receptors in cancer. Oncogene 33, 3485–3495. doi: 10.1038/onc.2013.302
Proud, P. C., Tsitoura, D., Watson, R. J., Chua, B. Y., Aram, M. J., Bewley, K. R., et al. (2021). Prophylactic intranasal administration of a TLR2/6 agonist reduces upper respiratory tract viral shedding in a SARS-CoV-2 challenge ferret model. EBioMedicine 63:103153. doi: 10.1016/J.EBIOM.2020.103153
Rahmani, F., and Rezaei, N. (2016). Therapeutic targeting of toll-like receptors: a review of toll-like receptors and their signaling pathways in psoriasis. Expert Rev. Clin. Immunol. 12, 1289–1298. doi: 10.1080/1744666X.2016.1204232
Rakoff-Nahoum, S., and Medzhitov, R. (2009). Toll-like receptors and cancer. Nat. Rev. Cancer 9, 57–63. doi: 10.1038/nrc2541
Rehwinkel, J., and Gack, M. U. (2020). RIG-I-like receptors: their regulation and roles in RNA sensing. Nat. Rev. Immunol. 201, 537–551. doi: 10.1038/s41577-020-0288-3
Ren, V., and Dao, H. (2013). Potential role of ixekizumab in the treatment of moderate-to-severe plaque psoriasis. Clin. Cosmet. Investig. Dermatol. 6, 75–80. doi: 10.2147/CCID.S42424
Romani, L. (2011). Immunity to fungal infections. Nat. Rev. Immunol. 11, 275–288. doi: 10.1038/nri2939
Sacre, S. M., Lo, A., Gregory, B., Simmonds, R. E., Williams, L., Feldmann, M., et al. (2008). Inhibitors of TLR8 reduce TNF production from human rheumatoid synovial membrane cultures. J. Immunol. 181, 8002–8009. doi: 10.4049/jimmunol.181.11.8002
Salvi, V., Nguyen, H. O., Sozio, F., Schioppa, T., Laffranchi, M., Scapini, P., et al. (2021). SARS-CoV-2-associated ssRNAs activate inflammation and immunity via TLR7/8. bioRxiv [Preprint] doi: 10.1101/2021.04.15.439839
Samarpita, S., Kim, J. Y., Rasool, M. K., and Kim, K. S. (2020). Investigation of toll-like receptor (TLR) 4 inhibitor TAK-242 as a new potential anti-rheumatoid arthritis drug. Arthritis Res. Ther. 22, 1–10. doi: 10.1186/s13075-020-2097-2
Savva, A., and Roger, T. (2013). Targeting toll-like receptors: promising therapeutic strategies for the management of sepsis-associated pathology and infectious diseases. Front. Immunol. 4:387. doi: 10.3389/FIMMU.2013.00387
Scho, M., and Scho, M. (2008). TLR7 and TLR8 as targets in cancer therapy. Oncogene 27, 190–199. doi: 10.1038/sj.onc.1210913
Shah, M., and Choi, S. (2017). Toll-like receptor-dependent negative effects of opioids: a battle between analgesia and hyperalgesia. Front. Immunol. 8:642. doi: 10.3389/FIMMU.2017.00642
Shear, M. J., Turner, F. C., Perrault, A., and Shovelton, T. (1943). Chemical treatment of tumors. V. isolation of the hemorrhage-producing fraction from Serratia marcescens (Bacillus prodigiosus) culture filtrate. JNCI J. Natl. Cancer Inst. 4, 81–97. doi: 10.1093/JNCI/4.1.81
Shekarian, T., Valsesia-Wittmann, S., Brody, J., Michallet, M. C., Depil, S., Caux, C., et al. (2017). Pattern recognition receptors: immune targets to enhance cancer immunotherapy. Ann. Oncol. 28, 1756–1766. doi: 10.1093/annonc/mdx179
Shi, M., Chen, X., Ye, K., Yao, Y., and Li, Y. (2016). Application potential of toll-like receptors in cancer immunotherapy: systematic review. Medicine (Baltimore) 95:e3951. doi: 10.1097/MD.0000000000003951
Simó, R., Stitt, A. W., and Gardner, T. W. (2018). Neurodegeneration in diabetic retinopathy: does it really matter? Diabetol 61, 1902–1912. doi: 10.1007/S00125-018-4692-1
Smatti, M. K., Cyprian, F. S., Nasrallah, G. K., Al Thani, A. A., Almishal, R. O., and Yassine, H. M. (2019). Viruses and autoimmunity: a review on the potential interaction and molecular mechanisms. Viruses 11:762. doi: 10.3390/v11080762
Stack, J., Haga, I. R., Schröder, M., Bartlett, N. W., Maloney, G., Reading, P. C., et al. (2005). Vaccinia virus protein A46R targets multiple toll-like-interleukin-1 receptor adaptors and contributes to virulence. J. Exp. Med. 201, 1007–1018. doi: 10.1084/jem.20041442
Sweeney, C. M., Tobin, A. M., and Kirby, B. (2011). Innate immunity in the pathogenesis of psoriasis. Arch. Dermatol. Res. 303, 691–705. doi: 10.1007/s00403-011-1169-1
Szajnik, M., Szczepanski, M. J., Czystowska, M., Elishaev, E., Mandapathil, M., Nowak-Markwitz, E., et al. (2009). TLR4 signaling induced by lipopolysaccharide or paclitaxel regulates tumor survival and chemoresistance in ovarian cancer. Oncogene 28, 4353–4363. doi: 10.1038/onc.2009.289
Takeda, K., and Akira, S. (2015). Toll-like receptors. Curr. Protoc. Immunol. 109, 14.12.1–14.12.10. doi: 10.1002/0471142735.im1412s109
Takeuchi, O., and Akira, S. (2007). Recognition of viruses by innate immunity. Immunol. Rev. 220, 214–224. doi: 10.1111/j.1600-065X.2007.00562.x
Takeuchi, O., and Akira, S. (2010). Pattern recognition receptors and inflammation. Cell 140, 805–820. doi: 10.1016/j.cell.2010.01.022
Thakur, K. K., Saini, J., Mahajan, K., Singh, D., Jayswal, D. P., Mishra, S., et al. (2017). Therapeutic implications of toll-like receptors in peripheral neuropathic pain. Pharmacol. Res. 115, 224–232. doi: 10.1016/J.PHRS.2016.11.019
Theofilopoulos, A. N., Kono, D. H., and Baccala, R. (2017). The multiple pathways to autoimmunity. Nat. Immunol. 18, 716–724. doi: 10.1038/ni.3731
Thompson, J. M., and Iwasaki, A. (2008). Toll-like receptors regulation of viral infection and disease. Adv. Drug Deliv. Rev. 60, 786–794. doi: 10.1016/j.addr.2007.11.003
Toubi, E., and Vadasz, Z. (2019). Innate immune-responses and their role in driving autoimmunity. Autoimmun. Rev. 18, 306–311. doi: 10.1016/j.autrev.2018.10.005
Uehata, T., and Takeuchi, O. (2020). RNA recognition and immunity—innate immune sensing and its posttranscriptional regulation mechanisms. Cells 9:1701. doi: 10.3390/CELLS9071701
Urban-Wojciuk, Z., Khan, M. M., Oyler, B. L., Fåhraeus, R., Marek-Trzonkowska, N., Nita-Lazar, A., et al. (2019). The role of TLRs in anti-cancer immunity and tumor rejection. Front. Immunol. 10:2388. doi: 10.3389/FIMMU.2019.02388
Van Der Graaf, C. A. A., Netea, M. G., Verschueren, I., Van Der Meer, J. W. M., and Kullberg, B. J. (2005). Differential cytokine production and toll-like receptor signaling pathways by Candida albicans blastoconidia and hyphae. Infect. Immun. 73, 7458–7464. doi: 10.1128/IAI.73.11.7458-7464.2005
Ve, T., Vajjhala, P. R., Hedger, A., Croll, T., Dimaio, F., Horsefield, S., et al. (2017). Structural basis of TIR-domain-assembly formation in MAL- and MyD88-dependent TLR4 signaling. Nat. Struct. Mol. Biol. 24, 743–751. doi: 10.1038/nsmb.3444
Vijay, K. (2018). Toll-like receptors in immunity and inflammatory diseases: past, present, and future. Int. Immunopharmacol. 59, 391–412. doi: 10.1016/J.INTIMP.2018.03.002
Wagner, H. (2004). The immunobiology of the TLR9 subfamily. Trends Immunol. 25, 381–386. doi: 10.1016/J.IT.2004.04.011
White, J. M., and Whittaker, G. R. (2016). Fusion of enveloped viruses in endosomes. Traffic 17:593. doi: 10.1111/TRA.12389
Wu, K., Zhang, H., Fu, Y., Zhu, Y., Kong, L., Chen, L., et al. (2018). TLR4/MyD88 signaling determines the metastatic potential of breast cancer cells. Mol. Med. Rep. 18:3411. doi: 10.3892/MMR.2018.9326
Yang, X., Cheng, Y., and Li, C. (2017). The role of TLRs in cervical cancer with HPV infection: a review. Signal Transduct. Target. Ther. 2, 1–10. doi: 10.1038/sigtrans.2017.55
Yang, Y., Lv, J., Jiang, S., Ma, Z., Wang, D., Hu, W., et al. (2016). The emerging role of toll-like receptor 4 in myocardial inflammation. Cell Death Dis. 7:e2234. doi: 10.1038/CDDIS.2016.140
Yu, X., Chen, R., Wang, F., Liu, W., Zhang, W., Gong, M., et al. (2021). Pattern recognition receptor-initiated innate immune responses in mouse prostatic epithelial cells. Biol. Reprod. 105, 113–127. doi: 10.1093/BIOLRE/IOAB076
Zamora-Atenza, C., Diaz-Torne, C., Geli, C., Diaz-Lopez, C., Ortiz, M. A., Moya, P., et al. (2014). Adalimumab regulates intracellular TNFα production in patients with rheumatoid arthritis. Arthritis Res. Ther. 16:R153. doi: 10.1186/AR4615
Zhang, J., Yu, C., Zhang, X., Chen, H., Dong, J., Lu, W., et al. (2018). Porphyromonas gingivalis lipopolysaccharide induces cognitive dysfunction, mediated by neuronal inflammation via activation of the TLR4 signaling pathway in C57BL/6 mice. J. Neuroinflammation 15, 1–14. doi: 10.1186/S12974-017-1052-X
Zhang, Y., Li, Y., Li, Y., Li, R., Ma, Y., Wang, H., et al. (2015). Chloroquine inhibits MGC803 gastric cancer cell migration via the toll-like receptor 9/nuclear factor kappa B signaling pathway. Mol. Med. Rep. 11, 1366–1371. doi: 10.3892/MMR.2014.2839
Zhao, Y., and Karijolich, J. (2019). Know thyself: RIG-I-like receptor sensing of DNA virus infection. J. Virol. 93:e01085-19. doi: 10.1128/JVI.01085-19
Zheng, M., Karki, R., Williams, E. P., Yang, D., Fitzpatrick, E., Vogel, P., et al. (2021). TLR2 senses the SARS-CoV-2 envelope protein to produce inflammatory cytokines. Nat. Immunol. 227, 829–838. doi: 10.1038/s41590-021-00937-x
Zweegers, J., Groenewoud, J. M. M., van den Reek, J. M. P. A., Otero, M. E., van de Kerkhof, P. C. M., Driessen, R. J. B., et al. (2017). Comparison of the 1- and 5-year effectiveness of adalimumab, etanercept and ustekinumab in patients with psoriasis in daily clinical practice: results from the prospective BioCAPTURE registry. Br. J. Dermatol. 176, 1001–1009. doi: 10.1111/bjd.15023
Keywords: Toll-like receptor, TLR-based immunotherapies, cancer, SARS-CoV-2, infection, autoimmune disorder
Citation: Farooq M, Batool M, Kim MS and Choi S (2021) Toll-Like Receptors as a Therapeutic Target in the Era of Immunotherapies. Front. Cell Dev. Biol. 9:756315. doi: 10.3389/fcell.2021.756315
Received: 10 August 2021; Accepted: 13 September 2021;
Published: 04 October 2021.
Edited by:
Michele Sommariva, University of Milan, ItalyReviewed by:
Amir Ghaemi, Pasteur Institute of Iran, IranAllison M. Owen, Vanderbilt University Medical Center, United States
Tomoko Hayashi, University of California, San Diego, United States
Copyright © 2021 Farooq, Batool, Kim and Choi. This is an open-access article distributed under the terms of the Creative Commons Attribution License (CC BY). The use, distribution or reproduction in other forums is permitted, provided the original author(s) and the copyright owner(s) are credited and that the original publication in this journal is cited, in accordance with accepted academic practice. No use, distribution or reproduction is permitted which does not comply with these terms.
*Correspondence: Sangdun Choi, sangdunchoi@ajou.ac.kr